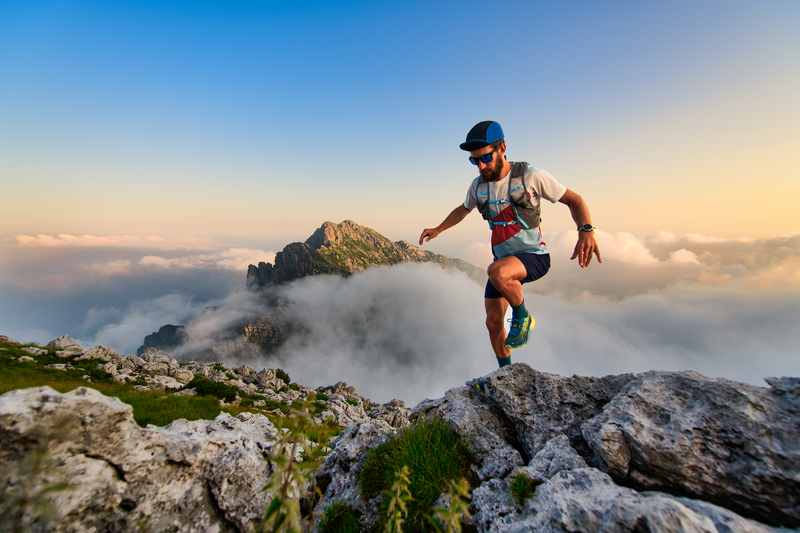
94% of researchers rate our articles as excellent or good
Learn more about the work of our research integrity team to safeguard the quality of each article we publish.
Find out more
MINI REVIEW article
Front. Cell. Neurosci. , 12 February 2019
Sec. Non-Neuronal Cells
Volume 13 - 2019 | https://doi.org/10.3389/fncel.2019.00044
This article is part of the Research Topic Glial and Neural Stem Cells as New Therapeutic Targets for Neurodegenerative Disorders View all 11 articles
To an increasing extent, astrocytes are connected with various neuropathologies. Astrocytes comprise of a heterogeneous population of cells with region- and species-specific properties. The frontal cortex exhibits high levels of plasticity that is required for high cognitive functions and memory making this region especially susceptible to damage. Aberrations in the frontal cortex are involved with several cognitive disorders, including Alzheimer’s disease, Huntington’s disease and frontotemporal dementia. Human induced pluripotent stem cells (iPSCs) provide an alternative for disease modeling and offer possibilities for studies to investigate pathological mechanisms in a cell type-specific manner. Patient-specific iPSC-derived astrocytes have been shown to recapitulate several disease phenotypes. Addressing astrocyte heterogeneity may provide an improved understanding of the mechanisms underlying neurodegenerative diseases.
Astrocytes are implicated as active mediators of synaptic activity, synaptogenesis and neurogenesis and are crucial in maintaining extracellular homeostasis and controlling blood-brain barrier permeability (Zhao et al., 2015; Allen and Lyons, 2018; Marina et al., 2018). Considering their versatile role in regulating brain function, it is no surprise that astrocyte malfunctions have been connected to various neurodegenerative disorders (Phatnani and Maniatis, 2015). Astrocytes are known as a morphologically and functionally diverse population of cells that differ both between distinct brain regions as well as within specific areas (Vasile et al., 2017). This diversity is reflected in their pathological features observed in psychiatric disorders (Rajkowska et al., 2002; Wallingford et al., 2017). Drugs used to treat mood disorders affect astrocytes as well and antidepressant effect on neurons is considered to be partially due to induction of astrocytic release of trophic factors (Marathe et al., 2018). Combined astrocyte and neuron-mediated effects also influence responses of antipsychotics (Khan et al., 2001). However, typical and atypical antipsychotics may display differential effects on the gliotransmitter release and the inflammatory response of astrocytes (Tanahashi et al., 2012; Bobermin et al., 2018). Understanding the function of astrocytes is therefore crucial for disease modeling and for developing treatments. Heterogeneity of the cell population and species-specific differences pose a challenge in the study of astrocytes. A method developed by Takahashi et al. (2007) allows the reprogramming of somatic cells into iPSCs, which can be used to generate patient-specific cells of a desired type (Takahashi et al., 2007). In this review, we describe how iPSC-derived astrocytes have been used to model neurodegenerative disorders involving frontal lobe malfunctions.
The generation of cortical glia is initiated once neurogenesis has been completed. The temporal patterning is based on a positive feedback signal from new-born neurons (Molofsky and Deneen, 2015; Takouda et al., 2017). Astrocyte progenitors migrate radially, obtaining their region specific properties upon maturation and this process continues postnatally (Colombo et al., 1997; Tsai et al., 2012). Several genes enriched in neuronal progenitors are also expressed in astrocytes, suggesting that astrocytes retain some proliferative potential even in the mature brain (Cahoy et al., 2008). However, only a distinct subset of astrocytes show neurogenic potential (Ghashghaei et al., 2007; Bardehle et al., 2013).
Mature astrocytes can be distinguished based on their morphology and functional properties. In the human cortex, astrocytes are morphologically categorized into four subtypes; interlaminar, protoplasmic, varicose projections and fibrous astroglia, located in the layers I and II, III and IV, V and VI and in the white matter, respectively (Vasile et al., 2017). The brain also contains other, both morphologically and functionally, distinct astrocytes such as elongated radial glia-like tanycytes and unipolar BG with several radially ascending processes. Tanycytes specialized in the regulation of neuroendocrine functions are located in the hypothalamus (Prevot et al., 2018) while BG modulate the efficacy of the synaptic transmission of Purkinje cells in the cerebellum (De Zeeuw and Hoogland, 2015).
Some astrocyte subtypes found in the human cortex are not represented in the rodent brain. Furthermore, human cortical astrocytes exceed their mouse counterparts both in complexity and size, and propagate calcium signals several times faster (Oberheim et al., 2009). Although human and mouse astrocytes share similar properties related to their effects on synapse formation, they differ in their function and transcriptional profiles (Zhang et al., 2016). Species-specific functional differences in glial cells are supported by improved learning and memory in chimeric animals following engrafting human glia into mouse brain (Han et al., 2013).
A higher relative number of astrocytes in the human frontal cortex, compared to that of many other species including other primates, is thought to be due to the high metabolomic cost of maintaining a bigger brain size (Bass et al., 1971; Sherwood et al., 2006). One of the key mechanism’s astrocytes apply to provide energy to neurons is via the astrocyte-neuron lactate shuttle. This metabolomic coupling is known to be crucial for memory formation (Alberini et al., 2018). Astrocytes respond to neuronal activity with spatially and temporally regulated Ca2+ fluctuations that shape neuronal activity via the regulation of the gliotransmitter release (Semyanov, 2019). Molecular and functional variations in astrocytes are considered to contribute to differences in distinct neural circuit signaling (Chai et al., 2017; Morel et al., 2017; Xin and Bonci, 2018). Another central role of astrocytes is the regulation of neurotransmitter uptake including glutamate via excitatory amino acid transporters 1 and 2 (EAAT1, EAAT2, respectively) in humans, or glutamate/aspartate transporter (GLAST) and glutamate transporter-1 (GLT-1) in rodents (Roberts et al., 2014; Meunier et al., 2017). Regulation of the extracellular neurotransmitter levels is affected in a number of neuropsychiatric disorders (John et al., 2012).
The frontal cortex is responsible for higher executive functions such as cognition and working memory (Fuster, 2002). The expression of genes involved in processes mediating synaptic plasticity, memory and learning is, respectively, enriched in a human and primate frontal cortex (Sjostedt et al., 2015; Garcia-Cabezas et al., 2017). A high level of flexibility is necessary for learning and memory functions but may also lead to increased structural vulnerability, which may explain why aberrations in the frontal cortex are connected to several neuropathologies (John et al., 2012; Feresten et al., 2013; Torres-Platas et al., 2016).
Astrocytes contribute to the regulation of neuronal activity that is altered in several frontal cortex pathologies (Braun et al., 2009; Cao et al., 2013; Lima et al., 2014; Bull et al., 2015; Ebrahimi et al., 2016; Beamer et al., 2017). A common feature for brain diseases is the activation of astrocytes into an inflammatory, reactive state (Chanaday and Roth, 2016). White matter astrocytes in the frontal cortex appear to be especially vulnerable to ischemic stroke, leading to disrupted gliovascular interactions caused by astrogliosis (Chen et al., 2016). Astrogliosis in the frontal cortex, upon aging, is also linked to mood disorders (Miguel-Hidalgo et al., 2000; Narita et al., 2006). Neurodegenerative disorders have overlapping characteristics suggesting common underlying pathological mechanisms. For instance, CTE caused by repeated head injuries, displays a similar accumulation of neurofibrillary tangles to that in AD but can be differentiated from AD by astrocytic tangles that are considered a hallmark of CTE (Turner et al., 2016; Hsu et al., 2018). Below, examples of neurodegenerative disorders with fronto-temporal pathologies and studies employing iPSC-derived astrocytes are described.
Alzheimer’s disease is a progressive neurodegenerative disease that manifests through cognitive impairment, motor abnormalities and behavioral changes. AD pathology is hallmarked by the accumulation of insoluble amyloid-β (Aβ) plaques, amyloid deposits in the blood vessel walls and aggregation of the microtubule protein tau, within neurons. The abnormalities seen in AD usually occur first in the frontotemporal region then spread progressively to other areas of the neocortex (Masters et al., 2015).
The contribution of astrocytes in AD pathology comprises of both the loss of neuroprotective features as well as the acquirement of pathological properties. Initially, astrocytes uptake and degrade Aβ and have a neuroprotective role. However, disease progression often leads to impaired astrocytic Aβ clearance and induces toxic gain-of-functions that contribute to disease progression (Garwood et al., 2017). Neural plaques adjacent to GFAP expressing astrocytes are known to induce hypertrophy and there is also evidence showing that astrocyte reactivity may precede plaque formation (Teneka et al., 2005; Olabarria et al., 2010; Rodrieguez-Veitez et al., 2015). Morphological aberrances in AD astrocytes that compromise vascular coverage are detrimental to neurovascular regulation, while disrupted potassium (K+) mediated neurovascular coupling, due to downregulation of K+ channels Kir4.1 and BKCa, result in abnormal regional cerebral blood flow (Acosta et al., 2017).
Aβ has been shown to alter the expression of metabotropic glutamate receptor 5 (mGluR5) and nicotinic acetylcholine receptors (nAchRs) in astrocytes, which leads to changes in Ca2+ homeostasis and signaling (Haughey and Mattson, 2003; Xiu et al., 2005; Lim et al., 2013). Excitotoxicity is a common characteristic of AD and astrocytes contribute to excessive glutamate signaling. Insufficient clearance of glutamate is connected to the reduced expression of glutamate transporters and their aberrant trafficking, which has been linked to altered cholesterol synthesis (Masliah et al., 1996; Tian et al., 2010; Merlini et al., 2011; Talantova et al., 2013). Furthermore, the release of glutamate has been shown to be enhanced in AD astrocytes (Talantova et al., 2013).
Reactivity is a common feature of AD astrocytes. Aβ induces the astrocytic release of pro-inflammatory mediators and, in turn, pro-inflammatory signals stimulate astrocytic Aβ production leading to a positive feedback loop between astrocyte Aβ response and production (Gonzáles-Reyes et al., 2017). S100β-positive astrocytes are connected to AD pathology and they are reduced following immunization against Aβ (Neus Bosch et al., 2015). S100β expressed by astrocytes is important for the regulation of neuronal oscillations associated with cognitive flexibility and depressive behavior (Stroth and Svenningsson, 2015; Brockett et al., 2018).
Glucose hypometabolism can precede clinical symptoms of AD (Mosconi et al., 2006). There is evidence that carriers of apolipoprotein E𝜀4 (APOE𝜀4) allele, with an increased risk for AD, have lower levels of glucose metabolism in various brain regions, including the prefrontal cortex, before the manifestation of clinical symptoms (Reiman et al., 2004). Dementia in AD is related to altered lactate processing. Under normal circumstances lactate-producing enzymes are down-regulated with age and an increase in the expression of these enzymes improve memory in wild type mice but leads to memory deficits in AD mice (Harris et al., 2016). Astrocyte defects in AD have been described extensively in a recent review (Acosta et al., 2017).
Huntington’s disease (HD) is characterized by motor dysfunction, cognitive impairment and neuropsychiatric features. HD is an inherited neurological disorder caused by CAG trinucleotide repeat expansion in the gene encoding Htt. The expansion gives rise to a mutated form of Htt (mHtt) with an abnormally long polyglutamine sequence which leads to the formation of mHtt aggregates (Bates et al., 2015). Clearance of aggregates is more efficient from astrocytes than from neurons, rendering astrocytes more resistant to mHtt accumulation (Zhao et al., 2016; Jansen et al., 2017; Zhao T. et al., 2017). Eventual accumulation of mHtt into astrocytes results in altered glutamate homeostasis and, sub-sequentially, neuronal excitotoxicity (Shin et al., 2005; Bradford et al., 2009). In addition to the enhanced release of glutamate, the presence of mHtt in astrocytes decreases the expression of glutamate transporters in an age-dependent manner (Lievens et al., 2001; Estrada-Sanchez et al., 2009; Faideau et al., 2010; Lee et al., 2013). However, excitotoxicity in HD neurons has also been reported without defects in the glutamate clearance (Parsons et al., 2016).
Huntington’s disease astrocytes possess an altered K+ signaling due to the decreased expression of Kir4.1 (Tong et al., 2014; Zhang et al., 2018). Restoration of Kir4.1 function can ameliorate impaired GLT1-mediated homeostasis and, sub-sequentially astrocyte Ca2+ signaling, implying a causative effect of Kir4.1 dysfunction on these mechanisms (Tong et al., 2014; Jiang et al., 2016). Kir4.1 defects precede the appearance of reactive astrocytes, indicating that inflammation is a secondary effect of HD pathology possibly induced by neurotoxicity (Tong et al., 2014).
Both reactive astrocytes and microglia have been implicated in the pathogenesis of HD (Khakh et al., 2017). Microglia promote the reactivity of astrocytes via the secretion of pro-inflammatory factors such as Il-1α, TNFα, and C1q (Liddelow et al., 2017). Reactive astrocytes have an impaired ability for synaptic maintenance and decreased phagocytic capacity (Bradford et al., 2009; Haim et al., 2015). Additionally, they promote degeneration of a subset of neurons and mature oligodendrocytes (Liddelow et al., 2017). Activation of the JAK/STAT3 pathway appears to be a common pathological feature of HD and AD. Astrocyte specific inhibition of this pathway, in animal models, reduces the reactive astrocyte phenotype (Haim et al., 2015). Interestingly, some studies have shown that reactive astrocytes can also have a neuroprotective role in HD (Haim et al., 2015; Liddelow et al., 2017).
Accumulation of mHtt disrupts exosome secretion from astrocytes (Hong et al., 2017). This can be connected to the reduced BDNF release from astrocytes (Hong et al., 2016). BDNF signaling is associated with HD pathogenesis and restoration of BDNF release from astrocytes has been shown to have neuroprotective effects (Giralt et al., 2010; Hong et al., 2016; Reick et al., 2016). However, there is also evidence that at early stages of HD, TrkB signaling is altered due to an indirect effect of p75 neurotrophic receptor (p75NTR) activity, indicating that signaling defects may precede aberrant secretion of BDNF, a ligand of both TrkB and p75NTR (Plotkin et al., 2014).
Frontotemporal dementia (FTD) is an umbrella term for neurodegenerative diseases affecting the frontal or temporal lobes. Behavioral changes and deficits in executive functioning and language characterize FTD (Bang et al., 2015). The role of astrocytes in FTD is not fully understood. However, FTD pathology is known to involve astrogliosis that occurs at an early stage of the disease progression and precedes neuronal loss (Su et al., 2000; Kersaitis et al., 2004). Astrocytic degeneration is marked by the expression of apoptotic markers, such as caspase-3, and morphological changes (Su et al., 2000; Broe et al., 2004). Apoptotic astrocytes in FTD have been correlated with the degree of frontotemporal atrophy and significant astrogliosis has been observed to overlap with areas showing disturbed cerebral perfusion (Martinac et al., 2001; Broe et al., 2004). In theory, astrocyte degeneration could cause disruptions similar to those seen in AD and HD, but the possible role of astrocyte degeneration in FTD pathogenesis remains unclear (Su et al., 2000).
In recent years, a number of astrocyte differentiation methods have been developed and advances on the use of iPSC-derived astrocytes have been reviewed in a recent paper (Zheng et al., 2018). Below, the application of iPSC-derived astrocytes and their use to model frontal cortex defects are discussed. The studies represented are summarized in Table 1.
Defects in both the clearance and production of Aβ, associated with AD, can also be seen in iPSC-derived AD astrocytes and appear to involve aberrant lipid metabolism (Oksanen et al., 2017; Fong et al., 2018; Lin et al., 2018). When studying the effects of APOE genotype Lin et al. (2018) demonstrated that APOE4 astrocytes show differences in the transcriptomic profile compared to isogenic APOE3 cells, as well as a diminished ability in clearing Aβ (Lin et al., 2018). The role of ApoE in the Aβ clearance is still unresolved and some studies claim that ApoE is crucial for the degradation and removal of Aβ, while others have shown that ApoE promotes neurodegeneration (Holtzman et al., 1999; Koistinaho et al., 2004; Liao et al., 2014; Shi et al., 2017). In co-culture studies APOE3 exhibited a greater ability to promote neuronal support and synaptogenesis (Zhao J. et al., 2017). Different properties of APOE isoforms in human astrocytes are in agreement with previous studies in mice (Wang et al., 2005). Jones et al. (2017) studied the function of AD astrocytes generated from iPSCs modeling early-onset FAD with mutation in PSEN and late-onset SAD with the APOE4 genotype. Both FAD and SAD astrocytes showed reduced morphological heterogeneity and aberrant expression of S100β. However, altered distribution of EAAT1 was only seen in SAD astrocytes (Jones et al., 2017). Altered secretion of inflammatory cytokines was found in both FAD and SAD, as well as in astrocytes with the PSEN1 ΔE9 genotype generated by Oksanen et al. (Jones et al., 2017; Oksanen et al., 2017). PSEN1 ΔE9 astrocytes also displayed changes in Ca2+ homeostasis, mitochondrial metabolism, ROS production and lactate secretion, thus covering all classical features of AD pathology (Oksanen et al., 2017).
Inflammatory responses were studied by Hsiao et al. (2015) in iPSC-derived HD astrocytes and an increase in the expression of VEGF-A, with further up-regulation after inflammatory cytokine treatment, was found. This leads to the enhanced proliferation of endothelial cells and the compromised survival of pericytes. As a result, poor pericyte coverage of blood vessels cause vascular reactivity and disrupts the blood-brain-barrier (Hsiao et al., 2015). Additionally, they demonstrated that the TNFα inhibitor XPro1595 successfully suppressed the inflammatory responses both in human astrocytes as well as primary astrocytes propagated from the brain of a transgenic HD mouse model (R6/2) (Hsiao et al., 2014). Juopperi et al. (2012) showed that HD astrocytes display increased cytoplasmic vacuolization (Juopperi et al., 2012). This phenotype is also present in HD lymphoblasts (Nagata et al., 2004; Martinez-Vicente et al., 2010). The findings in iPSC-derived HD astrocytes are consistent with astrogliosis as a key characteristic of HD pathology.
Frontotemporal dementia astrocytes, derived from iPSCs with mutations in genes encoding microtubule-associated protein TAU (MAPT) and TDP-34, demonstrated an increased susceptibility to oxidative stress and compromised survival (Serio et al., 2013; Hallmann et al., 2017). In M337V TDP-34 astrocytes, lowered survival paralleled the accumulation of TDP-43 (Serio et al., 2013). This phenomenon has been implicated in astrocyte dysfunction in CTE (Jayakumar et al., 2017). In N279K MAPT astrocytes, the expression of 4R-TAU isoform was increased as reported in FTD patients (Ghetti et al., 2015; Hallmann et al., 2017). N279K MAPT astrocytes displayed morphological changes and increased GFAP expression, usually linked to reactivity, as well as altered gene expression profiles. In co-culture assays with healthy neurons, N279K MAPT astrocytes increased the vulnerability of neurons to oxidative stress (Hallmann et al., 2017). However, M337V TDP-34 astrocytes did not exert toxic effects on neurons, although astrocytic expression of mutated TDP-43 has been reported to induce neuronal cell death (Tong et al., 2013; Serio et al., 2013) suggesting that other cell types, such as microglia, are required for the neurotoxic effect. Altogether, the results indicate that astrocyte degeneration is a common feature of FTD.
An increasing number of studies have connected astrocyte defects to frontal cortex pathologies. Species-specificity of astrocytes poses a challenge in translating results obtained from animal studies to humans, and patient-derived iPSCs offer an alternative to disease modeling. Studies presented above demonstrate that iPSC-derived astrocytes successfully recapitulate various disease phenotypes. Further challenges still include addressing the heterogeneity within the astrocyte population and developing protocols to generate regionally defined human astrocyte subtypes.
U-KP and MN wrote the manuscript in consultation with MC. MC performed the critical revision of the paper.
The authors declare that the research was conducted in the absence of any commercial or financial relationships that could be construed as a potential conflict of interest.
The authors wish to express their thanks for the financial support of the Academy of Finland, the Arvo and Lea Ylppö Foundation, the Finnish Konkordia Fund, the Finnish Cultural Foundation, and Finnish Brain Foundation.
5-HT, 5-hydroxytryptamine receptor; Aβ, amyloid β; AD, Alzheimer’s disease; APOE, apolipoprotein E; APP, amyloid precursor protein; BDNF, brain-derived neurotrophic factor; BG, Bergmann glia; cAMP, cyclic adenosine monophosphate; CTE, chronic traumatic encephalopathy; C1q, complement component 1q; EAAT, excitatory amino acid transporter; FAD, familial Alzheimer’s disease; FTD, frontotemporal dementia; GFAP, glial fibrillary acidic protein; GLAST, glutamate aspartate transporter; GLT-1, glutamate transporter 1; HD, Huntington’s disease; Htt, huntingtin; Il-1α, interleukin 1α; iPSC, induced pluripotent stem cell; JAK/STAT3, Janus kinase/signal transducers and activators of transcription 3; ROS, reactive oxygen species; SAD, sporadic Alzheimer’s disease; TNFα, tumor necrosis factor α; TDP-34, transactive response DNA-binding protein 34; TrkB, tyrosine-related kinase B; VEGF-A, vascular endothelial growth factor A.
Acosta, C., Anderson, H. D., and Anderson, C. M. (2017). Astrocyte dysfunction in Alzheimer disease. J. Neurosci. Res. 95, 2430–2447. doi: 10.1002/jnr.24075
Alberini, C. M., Cruz, E., Descalzi, G., Bessieres, B., and Gao, V. (2018). Astrocyte glycogen and lactate: new insights into learning and memory mechanisms. Glia 66, 1244–1262. doi: 10.1002/glia.23250
Allen, N. J., and Lyons, D. A. (2018). Glia as architects of central nervous system formation and function. Science 362, 181–185. doi: 10.1126/science.aat0473
Bang, J., Spina, S., and Miller, B. L. (2015). Non-Alzheimer’s dementia 1: frontotemporal dementia. Lancet 386, 1672–1682. doi: 10.1016/S0140-6736(15)00461-4
Bardehle, S., Krüger, M., Buggenthin, F., Schwausch, J., Ninkovic, J., Clevers, H., et al. (2013). Live imaging of astrocyte responses to acute injury reveals selective juxtavascular proliferation. Nat. Neurosci. 16:580. doi: 10.1038/nn.3371
Bass, N. H., Hess, H. H., Pope, A., and Thalheimer, C. (1971). Quantitative cytoarchitectonic distribution of neurons, glia, and DNA in rat cerebral cortex. J. Comp. Neurol. 143, 481–490. doi: 10.1002/cne.901430405
Bates, G. P., Dorsey, R., Gusella, J. F., Hayden, M. R., Kay, C., Leavitt, B. R., et al. (2015). Huntington disease. Nat. Rev. Dis. Primers 1:15005. doi: 10.1038/nrdp.2015.5
Beamer, E., Kovacs, G., and Sperlagh, B. (2017). ATP released from astrocytes modulates action potential threshold and spontaneous excitatory postsynaptic currents in the neonatal rat prefrontal cortex. Brain Res. Bull. 135, 129–142. doi: 10.1016/j.brainresbull.2017.10.006
Bobermin, L. D., da Silva, A., Souza, D. O., and Quincozes-Santos, A. (2018). Differential effects of typical and atypical antipsychotics on astroglial cells in vitro. Int. J. Dev. Neurosci. 69, 1–9. doi: 10.1016/j.ijdevneu.2018.06.001
Bradford, J., Shin, J.-Y., Roberts, M., Wang, C.-E., Li, X.-J., and Li, S. (2009). Expression of mutant huntingtin in mouse brain astrocytes causes age-dependent neurological symptoms. Proc. Natl. Acad. Sci. U.S.A. 106,22480–22485. doi: 10.1073/pnas.0911503106
Braun, K., Antemano, R., Helmeke, C., Buchner, M., and Poeggel, G. (2009). Juvenile separation stress induces rapid region- and layer-specific changes in S100ss- and glial fibrillary acidic protein-immunoreactivity in astrocytes of the rodent medial prefrontal cortex. Neuroscience 160, 629–638. doi: 10.1016/j.neuroscience.2009.02.074
Brockett, A. T., Kane, G. A., Monari, P. K., Briones, B. A., Vigneron, P. A., Barber, G. A., et al. (2018). Evidence supporting a role for astrocytes in the regulation of cognitive flexibility and neuronal oscillations through the Ca2+ binding protein S100beta. PLoS One 13:e0195726. doi: 10.1371/journal.pone.0195726
Broe, M., Kril, J., and Halliday, G. M. (2004). Astrocytic degeneration relates to the severity of disease in frontotemporal dementia. Brain 127(Pt 10), 2214–2220. doi: 10.1093/brain/awh250
Bull, C., Syed, W. A., Minter, S. C., and Bowers, M. S. (2015). Differential response of glial fibrillary acidic protein-positive astrocytes in the rat prefrontal cortex following ethanol self-administration. Alcohol. Clin. Exp. Res. 39, 650–658. doi: 10.1111/acer.12683
Cahoy, J. D., Emery, B., Kaushal, A., Foo, L. C., Zamanian, J. L., Christopherson, K. S., et al. (2008). A transcriptome database for astrocytes, neurons, and oligodendrocytes: a new resource for understanding brain development and function. J. Neurosci. 28, 264–278. doi: 10.1523/JNEUROSCI.4178-07.2008
Cao, X., Li, L. P., Wang, Q., Wu, Q., Hu, H. H., Zhang, M., et al. (2013). Astrocyte-derived ATP modulates depressive-like behaviors. Nat. Med. 19, 773–777. doi: 10.1038/nm.3162
Chai, H., Diaz-Castro, B., Shigetomi, E., Monte, E., Octeau, J. C., Yu, X., et al. (2017). Neural circuit-specialized astrocytes: transcriptomic, proteomic, morphological, and functional evidence. Neuron 95, 531–549.e9. doi: 10.1016/j.neuron.2017.06.029
Chanaday, N. L., and Roth, G. A. (2016). Microglia and astrocyte activation in the frontal cortex of rats with experimental autoimmune encephalomyelitis. Neuroscience 314, 160–169. doi: 10.1016/j.neuroscience.2015.11.060
Chen, A., Akinyemi, R. O., Hase, Y., Firbank, M. J., Ndung’u, M. N., Foster, V., et al. (2016). Frontal white matter hyperintensities, clasmatodendrosis and gliovascular abnormalities in ageing and post-stroke dementia. Brain 139, 242–258. doi: 10.1093/brain/awv328
Colombo, J. A., Lipina, S., Yanez, A., and Puissant, V. (1997). Postnatal development of interlaminar astroglial processes in the cerebral cortex of primates. Int. J. Dev. Neurosci. 15, 823–833. doi: 10.1016/S0736-5748(97)00043-9
De Zeeuw, C. I., and Hoogland, T. M. (2015). Reappraisal of Bergmann glial cells as modulators of cerebellar circuit function. Front. Cell. Neurosci. 9:246. doi: 10.3389/fncel.2015.00246
Ebrahimi, M., Yamamoto, Y., Sharifi, K., Kida, H., Kagawa, Y., Yasumoto, Y., et al. (2016). Astrocyte-expressed FABP7 regulates dendritic morphology and excitatory synaptic function of cortical neurons. Glia 64, 48–62. doi: 10.1002/glia.22902
Estrada-Sanchez, A. M., Montiel, T., Segovia, J., and Massieu, L. (2009). Glutamate toxicity in the striatum of the R6/2 Huntington’s disease transgenic mice is age-dependent and correlates with decreased levels of glutamate transporters. Neurobiol. Dis. 34, 78–86. doi: 10.1016/j.nbd.2008.12.017
Faideau, M., Kim, J., Cormier, K., Gilmore, R., Welch, M., Auregan, G., et al. (2010). In vivo expression of polyglutamine-expanded huntingtin by mouse striatal astrocytes impairs glutamate transport: a correlation with Huntington’s disease subjects. Hum. Mol. Genet. 19, 3053–3067. doi: 10.1093/hmg/ddq212
Feresten, A. H., Barakauskas, V., Ypsilanti, A., Barr, A. M., and Beasley, C. L. (2013). Increased expression of glial fibrillary acidic protein in prefrontal cortex in psychotic illness. Schizophr. Res. 150, 252–257. doi: 10.1016/j.schres.2013.07.024
Fong, L. K., Yang, M. M., Dos Santos Chaves, R., Reyna, S. M., Langness, V. F., Woodruff, G., et al. (2018). Full-length amyloid precursor protein regulates lipoprotein metabolism and amyloid-beta clearance in human astrocytes. J. Biol. Chem. 293, 11341–11357. doi: 10.1074/jbc.RA117.000441
Fuster, J. M. (2002). Frontal lobe and cognitive development. J. Neurocytol. 31, 373–385. doi: 10.1023/A:1024190429920
Garcia-Cabezas, M. A., Joyce, M. K. P., John, Y. J., Zikopoulos, B., and Barbas, H. (2017). Mirror trends of plasticity and stability indicators in primate prefrontal cortex. Eur. J. Neurosci. 46, 2392–2405. doi: 10.1111/ejn.13706
Garwood, C. J., Ratcliffe, L. E., Simpson, J. E., Heath, P. R., Ince, P. G., and Wharton, S. B. (2017). Review: astrocytes in Alzheimer’s disease and other age-associated dementias: a supporting player with a central role. Neuropathol. Appl. Neurobiol. 43, 281–298. doi: 10.1111/nan.12338
Ghashghaei, H. T., Weimer, J. M., Schmid, R. S., Yokota, Y., McCarthy, K. D., Popko, B., et al. (2007). Reinduction of ErbB2 in astrocytes promotes radial glial progenitor identity in adult cerebral cortex. Genes Dev. 21, 3258–3271. doi: 10.1101/gad.1580407
Ghetti, B., Oblak, A. L., Boeve, B. F., Johnson, K. A., Dickerson, B. C., and Goedert, M. (2015). Invited review: frontotemporal dementia caused by microtubule-associated protein tau gene (MAPT) mutations: a chameleon for neuropathology and neuroimaging. Neuropathol. Appl. Neurobiol. 41, 24–46. doi: 10.1111/nan.12213
Giralt, A., Friedman, H. C., Caneda-Ferron, B., Urban, N., Moreno, E., Rubio, N., et al. (2010). BDNF regulation under GFAP promoter provides engineered astrocytes as a new approach for long-term protection in Huntington’s disease. Gene Ther. 17, 1294–1308. doi: 10.1038/gt.2010.71
Gonzáles-Reyes, R. E., Nava-Mesa, M. O., Vargas-Sánchez, K., Ariza-Salamanca, D., and Mora-Muñoz, L. (2017). Involvement of astrocytes in Alzheimer’s disease from a neuroinflammatory and oxidative stress perspective. Front. Mol. Neurosci. 10:427. doi: 10.3389/fnmol.2017.00427
Haim, L. B., Ceyzériat, K., Carrillo-de Sauvage, M. A., Aubry, F., Auregan, G., Guillermier, M., et al. (2015). The JAK/STAT3 pathway is a common inducer of astrocyte reactivity in Alzheimer’s and Huntington’s diseases. J. Neurosci. 35, 2817–2829. doi: 10.1523/JNEUROSCI.3516-14.2015
Hallmann, A. L., Arauzo-Bravo, M. J., Mavrommatis, L., Ehrlich, M., Ropke, A., Brockhaus, J., et al. (2017). Astrocyte pathology in a human neural stem cell model of frontotemporal dementia caused by mutant TAU protein. Sci. Rep. 7:42991. doi: 10.1038/srep42991
Han, X., Chen, M., Wang, F., Windrem, M., Wang, S., Shanz, S., et al. (2013). Forebrain engraftment by human glial progenitor cells enhances synaptic plasticity and learning in adult mice. Cell Stem Cell 12, 342–353. doi: 10.1016/j.stem.2012.12.015
Harris, R. A., Tindale, L., Lone, A., Singh, O., Macauley, S. L., Stanley, M., et al. (2016). Aerobic glycolysis in the frontal cortex correlates with memory performance in wild-type mice but not the APP/PS1 mouse model of cerebral amyloidosis. J. Neurosci. 36, 1871–1878. doi: 10.1523/JNEUROSCI.3131-15.2016
Haughey, N. J., and Mattson, M. P. (2003). Alzheimer’s amyloid beta-peptide enhances ATP/gap junction-mediated calcium-wave propagation in astrocytes. Neuromolecular Med. 3, 173–180. doi: 10.1385/NMM:3:3:173
Holtzman, D. M., Bales, K. R., Wu, S., Bhat, P., Parsadanian, M., Fagan, A. M., et al. (1999). Expression of human apolipoprotein E reduces amyloid-beta deposition in a mouse model of Alzheimer’s disease. J. Clin. Invest. 103, R15–R21. doi: 10.1172/JCI6179
Hong, Y., Zhao, T., Li, X.-J., and Li, S. (2016). Mutant huntingtin impairs BDNF release from astrocytes by disrupting conversion of Rab3a-GTP into Rab3a-GDP. J. Neurosci. 36, 8790–8801. doi: 10.1523/JNEUROSCI.0168-16.2016
Hong, Y., Zhao, T., Li, X. J., and Li, S. (2017). Mutant huntingtin inhibits alphaB-crystallin expression and impairs exosome secretion from astrocytes. J. Neurosci. 37, 9550–9563. doi: 10.1523/JNEUROSCI.1418-17.2017
Hsiao, H.-Y., Chen, Y.-C., Huang, C.-H., Chen, C.-C., Hsu, Y.-H., Chen, H.-M., et al. (2015). Aberrant astrocytes impair vascular reactivity in Huntington disease. Ann. Neurol. 78, 178–192. doi: 10.1002/ana.24428
Hsiao, H. Y., Chiu, F. L., Chen, C. M., Wu, Y. R., Chen, H. M., Chen, Y. C., et al. (2014). Inhibition of soluble tumor necrosis factor is therapeutic in Huntington’s disease. Hum. Mol. Genet. 23, 4328–4344. doi: 10.1093/hmg/ddu151
Hsu, E. T., Gangolli, M., Su, S., Holleran, L., Stein, T. D., Alvarez, V. E., et al. (2018). Astrocytic degeneration in chronic traumatic encephalopathy. Acta Neuropathol. 136, 955–972. doi: 10.1007/s00401-018-1902-3
Jansen, A. H., van Hal, M., Op den Kelder, I. C., Meier, R. T., de Ruiter, A. A., Schut, M. H., et al. (2017). Frequency of nuclear mutant huntingtin inclusion formation in neurons and glia is cell-type-specific. Glia 65, 50–61. doi: 10.1002/glia.23050
Jayakumar, A. R., Tong, X. Y., Shamaladevi, N., Barcelona, S., Gaidosh, G., Agarwal, A., et al. (2017). Defective synthesis and release of astrocytic thrombospondin-1 mediates the neuronal TDP-43 proteinopathy, resulting in defects in neuronal integrity associated with chronic traumatic encephalopathy: in vitro studies. J. Neurochem. 140, 645–661. doi: 10.1111/jnc.13867
Jiang, R., Diaz-Castro, B., Looger, L. L., and Khakh, B. S. (2016). Dysfunctional calcium and glutamate signaling in striatal astrocytes from Huntington’s disease model mice. J. Neurosci. 36, 3453–3470. doi: 10.1523/JNEUROSCI.3693-15.2016
John, C. S., Smith, K. L., Van’t Veer, A., Gompf, H. S., Carlezon, W. A. Jr., et al. (2012). Blockade of astrocytic glutamate uptake in the prefrontal cortex induces anhedonia. Neuropsychopharmacology 37, 2467–2475. doi: 10.1038/npp.2012.105
Jones, V. C., Atkinson-Dell, R., Verkhratsky, A., and Mohamet, L. (2017). Aberrant iPSC-derived human astrocytes in Alzheimer’s disease. Cell Death Dis. 8:e2696. doi: 10.1038/cddis.2017.89
Juopperi, T. A., Kim, W. R., Chiang, C. -H., Yu, H., Margolis, R. L., Ross, C. A., et al. (2012). Astrocytes generated from patient induced pluripotent stem cells recapitulate features of Huntington’s disease patient cells. Mol. Brain 5:17. doi: 10.1186/1756-6606-5-17
Kersaitis, C., Halliday, G. M., and Kril, J. J. (2004). Regional and cellular pathology in frontotemporal dementia: relationship to stage of disease in cases with and without Pick bodies. Acta Neuropathol. 108, 515–523. doi: 10.1007/s00401-004-0917-0
Khakh, B. S., Beaumont, V., Cachope, R., Munoz-Sanjuan, I., Goldman, S. A., and Grantyn, R. (2017). Unravelling and exploiting astrocyte dysfunction in Huntington’s disease. Trends Neurosci. 40, 422–437. doi: 10.1016/j.tins.2017.05.002
Khan, Z. U., Koulen, P., Rubinstein, M., Grandy, D. K., and Goldman-Rakic, P. S. (2001). An astroglia-linked dopamine D2-receptor action in prefrontal cortex. Proc. Natl. Acad. Sci. U.S.A. 98, 1964–1969. doi: 10.1073/pnas.98.4.1964
Koistinaho, M., Lin, S., Wu, X., Esterman, M., Koger, D., Hanson, J., et al. (2004). Apolipoprotein E promotes astrocyte colocalization and degradation of deposited amyloid-β peptides. Nat. Med. 10, 719–726. doi: 10.1038/nm1058
Lee, W., Reyes, R. C., Gottipati, M. K., Lewis, K., Lesort, M., Parpura, V., et al. (2013). Enhanced Ca2+-dependent glutamate release from astrocytes of the BACHD Huntington’s disease mouse model. Neurobiol. Dis. 58, 192–199. doi: 10.1016/j.nbd.2013.06.002
Liao, F., Hori, Y., Hudry, E., Bauer, A. Q., Jiang, H., Mahan, T. E., et al. (2014). Anti-ApoE antibody given after plaque onset decreases Aβ accumulation and improves brain function in a mouse model of Aβ amyloidosis. J. Neurosci. 34, 7281–7292. doi: 10.1523/JNEUROSCI.0646-14.2014
Liddelow, S. A., Guttenplan, K. A., Clarke, L. E., Bennett, F. C., Bohlen, C. J., Schirmer, L., et al. (2017). Neurotoxic reactive astrocytes are induced by activated microglia. Nature 541, 481–487. doi: 10.1038/nature21029
Lievens, J. C., Woodman, B., Mahal, A., Spasic-Boscovic, O., Samuel, D., Kerkerian-Le Goff, L., et al. (2001). Impaired glutamate uptake in the R6 Huntington’s disease transgenic mice. Neurobiol. Dis. 8, 807–821. doi: 10.1006/nbdi.2001.0430
Lim, D., Iyer, A., Ronco, V., Grolla, A. A., Canonico, P. L., Aronica, E., et al. (2013). Amyloid beta deregulates astroglial mGluR5-mediated calcium signaling via calcineurin and Nf-kB. Glia 61, 1134–1145. doi: 10.1002/glia.22502
Lima, A., Sardinha, V. M., Oliveira, A. F., Reis, M., Mota, C., Silva, M. A., et al. (2014). Astrocyte pathology in the prefrontal cortex impairs the cognitive function of rats. Mol. Psychiatry 19, 834–841. doi: 10.1038/mp.2013.182
Lin, Y. T., Seo, J., Gao, F., Feldman, H. M., Wen, H. L., Penney, J., et al. (2018). APOE4 causes widespread molecular and cellular alterations associated with Alzheimer’s disease phenotypes in human iPSC-derived brain cell types. Neuron 98, 1141–1154.e7. doi: 10.1016/j.neuron.2018.05.008
Marathe, S. V., D’Almeida, P. L., Virmani, G., Bathini, P., and Alberi, L. (2018). Effects of monoamines and antidepressants on astrocyte physiology: implications for monoamine hypothesis of depression. J. Exp. Neurosci. 12:1179069518789149. doi: 10.1177/1179069518789149
Marina, N., Turovsky, E., Christie, I. N., Hosford, P. S., Hadjihambi, A., Korsak, A., et al. (2018). Brain metabolic sensing and metabolic signaling at the level of an astrocyte. Glia 66, 1185–1199. doi: 10.1002/glia.23283
Martinac, J. A., Craft, D. K., Su, J. H., Kim, R. C., and Cotman, C. W. (2001). Astrocytes degenerate in frontotemporal dementia: possible relation to hypoperfusion. Neurobiol. Aging 22, 195–207. doi: 10.1016/S0197-4580(00)00231-1
Martinez-Vicente, M., Talloczy, Z., Wong, E., Tang, G., Koga, H., Kaushik, S., et al. (2010). Cargo recognition failure is responsible for inefficient autophagy in Huntington’s disease. Nat. Neurosci. 13, 567–576. doi: 10.1038/nn.2528
Masliah, E., Alford, M., Mallory, M., and Hansen, L. (1996). Deficient glutamate transport is associated with neurodegeneration in Alzheimer’s disease. Ann. Neurol. 40, 759–766. doi: 10.1002/ana.410400512
Masters, C. L., Bateman, R., Blennow, K., Rowe, C. C., Sperling, R. A., and Cummings, J. L. (2015). Alzheimer’s disease. Nat. Rev. Dis. Primers 1:15056. doi: 10.1038/nrdp.2015.56
Merlini, M., Meyer, E. P., Ulmann-Schuler, A., and Nitsch, R. M. (2011). Vascular β-amyloid and early astrocyte alterations impair cerebrovascular function and cerebral metabolism in transgenic arcAβ mice. Acta Neuropathol. 12, 293–311. doi: 10.1007/s00401-011-0834-y
Meunier, C., Wang, N., Yi, C., Dallerac, G., Ezan, P., Koulakoff, A., et al. (2017). Contribution of astroglial Cx43 hemichannels to the modulation of glutamatergic currents by D-serine in the mouse prefrontal cortex. J. Neurosci. 37, 9064–9075. doi: 10.1523/JNEUROSCI.2204-16.2017
Miguel-Hidalgo, J. J., Baucom, C., Dilley, G., Overholser, J. C., Meltzer, H. Y., Stockmeier, C. A., et al. (2000). Glial fibrillary acidic protein immunoreactivity in the prefrontal cortex distinguishes younger from older adults in major depressive disorder. Biol. Psychiatry 48, 861–873. doi: 10.1016/S0006-3223(00)00999-9
Molofsky, A. V., Deneen, B. (2015). Astrocyte development: a guide for the perplexed. Glia 63, 1320–1329. doi: 10.1002/glia.22836
Morel, L., Chiang, M. S. R., Higashimori, H., Shoneye, T., Iyer, L. K., Yelick, J., et al. (2017). Molecular and functional properties of regional astrocytes in the adult brain. J. Neurosci. 37, 8706–8717. doi: 10.1523/JNEUROSCI.3956-16.2017
Mosconi, L., Sorbi, S., de Leon, M. J., Li, Y., Nacmias, B., Myoung, P. S., et al. (2006). Hypometabolism exceeds atrophy in presymptomatic early-onset familial Alzheimer’s disease. J. Nucl. Med. 47, 1778–1786.
Nagata, E., Sawa, A., Ross, C. A., and Snyder, S. H. (2004). Autophagosome-like vacuole formation in Huntington’s disease lymphoblasts. Neuroreport 15, 1325–1328. doi: 10.1097/01.wnr.0000127073.66692.8f
Narita, M., Kuzumaki, N., Narita, M., Kaneko, C., Tamai, E., Khotib, J., et al. (2006). Age-related emotionality is associated with cortical delta-opioid receptor dysfunction-dependent astrogliosis. Neuroscience 137, 1359–1367. doi: 10.1016/j.neuroscience.2005.10.067
Neus Bosch, M., Pugliese, M., Andrade, C., Gimeno-Bayon, J., Mahy, N., and Rodriguez, M. J. (2015). Amyloid-beta immunotherapy reduces amyloid plaques and astroglial reaction in aged domestic dogs. Neurodegener. Dis. 15, 24–37. doi: 10.1159/000368672
Oberheim, N. A., Takano, T., Han, X., He, W., Lin, J. H. C., Wang, F., et al. (2009). Uniquely hominid features of adult human astrocytes. J. Neurosci. 29, 3276–3287. doi: 10.1523/JNEUROSCI.4707-08.2009
Oksanen, M., Petersen, A. J., Naumenko, N., Puttonen, K., Lehtonen,Š., Gubert Olivé, M., et al. (2017). PSEN1 mutant iPSC-derived model reveals severe astrocyte pathology in Alzheimer’s disease. Stem Cell Rep. 9, 1885–1897. doi: 10.1016/j.stemcr.2017.10.016
Olabarria, M., Noristani, H. N., Verkhratsky, A., and Rodríguez, J. J. (2010). Concomitant astroglial atrophy and astrogliosis in a triple transgenic animal model of Alzheimer’s disease. Glia 58, 831–838. doi: 10.1002/glia.20967
Parsons, M. P., Vanni, M. P., Woodard, C. L., Kang, R., Murphy, T. H., and Raymond, L. A. (2016). Real-time imaging of glutamate clearance reveals normal striatal uptake in Huntington disease mouse models. Nat. Commun. 7:11251. doi: 10.1038/ncomms11251
Phatnani, H., and Maniatis, T. (2015). Astrocytes in neurodegenerative disease. Cold Spring Harb. Perspect. Biol. 7:a020628. doi: 10.1101/cshperspect.a020628
Plotkin, J. L., Joshua L., Day, M., Peterson, Jayms D., Xie, Z., Kress, Geraldine J., et al. (2014). Impaired TrkB receptor signaling underlies corticostriatal dysfunction in Huntington’s disease. Neuron 83, 178–188. doi: 10.1016/j.neuron.2014.05.032
Prevot, V., Dehouck, B., Sharif, A., Ciofi, P., Giacobini, P., and Clasadonte, J. (2018). The versatile tanycyte: a hypothalamic integrator of reproduction and energy metabolism. Endocr. Rev. 39, 333–368. doi: 10.1210/er.2017-00235
Rajkowska, G., Miguel-Hidalgo, J. J., Makkos, Z., Meltzer, H., Overholser, J., and Stockmeier, C. (2002). Layer-specific reductions in GFAP-reactive astroglia in the dorsolateral prefrontal cortex in schizophrenia. Schizophr. Res. 57, 127–138. doi: 10.1016/S0920-9964(02)00339-0
Reick, C., Ellrichmann, G., Tsai, T., Lee, D. H., Wiese, S., Gold, R., et al. (2016). Expression of brain-derived neurotrophic factor in astrocytes - Beneficial effects of glatiramer acetate in the R6/2 and YAC128 mouse models of Huntington’s disease. Exp. Neurol. 285, 12–23. doi: 10.1016/j.expneurol.2016.08.012
Reiman, E. M., Chen, K., Alexander, G. E., Caselli, R. J., Bandy, D., Osborne, D., et al. (2004). Functional brain abnormalities in young adults at genetic risk for late-onset Alzheimer’s dementia. Proc. Natl. Acad. Sci. U.S.A. 101, 284–289. doi: 10.1073/pnas.2635903100
Roberts, R. C., Roche, J. K., and McCullumsmith, R. E. (2014). Localization of excitatory amino acid transporters EAAT1 and EAAT2 in human postmortem cortex: a light and electron microscopic study. Neuroscience 277, 522–540. doi: 10.1016/j.neuroscience.2014.07.019
Rodrieguez-Veitez, E., Ni, R., Gulyás, B., Tóth, M., Häggkvist, J., Halldin, C., et al. (2015). Astrocytosis precedes amyloid plaque deposition in Alzheimer APPswe transgenic mouse brain: a correlative positron emission tomography and in vitro imaging study. Eur. J. Nucl. Med. Mol. Imaging 42, 1119–1132. doi: 10.1007/s00259-015-3047-0
Semyanov, A. (2019). Spatiotemporal pattern of calcium activity in astrocytic network. Cell Calcium 78, 15–25. doi: 10.1016/j.ceca.2018.12.007
Serio, A., Bilican, B., Barmada, S. J., Ando, D. M., Zhao, C., Siller, R., et al. (2013). Astrocyte pathology and the absence of non-cell autonomy in an induced pluripotent stem cell model of TDP-43 proteinopathy. Proc. Natl. Acad. Sci. U.S.A. 110, 4697–4702. doi: 10.1073/pnas.1300398110
Sherwood, C. C., Stimpson, C. D., Raghanti, M. A., Wildman, D. E., Uddin, M., Grossman, L. I., et al. (2006). Evolution of increased glia–neuron ratios in the human frontal cortex. Proc. Natl. Acad. Sci. U.S.A. 103, 13606–13611. doi: 10.1073/pnas.0605843103
Shi, Y., Yamada, K., Liddelow, S. A., Smith, S. T., Zhao, L., Luo, W., et al. (2017). ApoE4 markedly exacerbates tau-mediated neurodegeneration in a mouse model of tauopathy. Nature 549, 523–527. doi: 10.1038/nature24016
Shin, J. Y., Fang, Z. H., Yu, Z. X., Wang, C. E., Li, S. H., and Li, X. J. (2005). Expression of mutant huntingtin in glial cells contributes to neuronal excitotoxicity. J. Cell Biol. 171, 1001–1012. doi: 10.1083/jcb.200508072
Sjostedt, E., Fagerberg, L., Hallstrom, B. M., Haggmark, A., Mitsios, N., Nilsson, P., et al. (2015). Defining the human brain proteome using transcriptomics and antibody-based profiling with a focus on the cerebral cortex. PLoS One 10:e0130028. doi: 10.1371/journal.pone.0130028
Stroth, N., and Svenningsson, P. (2015). S100B interacts with the serotonin 5-HT7 receptor to regulate a depressive-like behavior. Eur. Neuropsychopharmacol. 25, 2372–2380. doi: 10.1016/j.euroneuro.2015.10.003
Su, J. H., Nichol, K. E., Sitch, T., Sheu, P., Chubb, C., Miller, B. L., et al. (2000). DNA damage and activated caspase-3 expression in neurons and astrocytes: evidence for apoptosis in frontotemporal dementia. Exp. Neurol. 163, 9–19. doi: 10.1006/exnr.2000.7340
Takahashi, K., Tanabe, K., Ohnuki, M., Narita, M., Ichisaka, T., Tomoda, K., et al. (2007). Induction of pluripotent stem cells from adult human fibroblasts by defined factors. Cell 131, 861–872. doi: 10.1016/j.cell.2007.11.019
Takouda, J., Katada, S., and Nakashima, K. (2017). Emerging mechanisms underlying astrogenesis in the developing mammalian brain. Proc. Jpn. Acad. Ser. B Phys. Biol. Sci. 93, 386–398. doi: 10.2183/pjab.93.024
Talantova, M., Sanz-Blasco, S., Zhang, X., Xia, P., Akhtar, M. W., Okamoto, S.-I., et al. (2013). Aβ induces astrocytic glutamate release, extrasynaptic NMDA receptor activation, and synaptic loss. Proc. Natl. Acad. Sci. U.S.A. 110,E2518–E2527. doi: 10.1073/pnas.1306832110
Tanahashi, S., Yamamura, S., Nakagawa, M., Motomura, E., and Okada, M. (2012). Clozapine, but not haloperidol, enhances glial D-serine and L-glutamate release in rat frontal cortex and primary cultured astrocytes. Br. J. Pharmacol. 165, 1543–1555. doi: 10.1111/j.1476-5381.2011.01638.x
Teneka, M. T., Sastre, M., Dumitrescu-Ozimek, L., Dewachter, I., Walter, J., Klockgether, T., et al. (2005). Focal glial activation coincides with increased BACE1 activation and precedes amyloid plaque deposition in APP[V717I] transgenic mice. J. Neuroinflammation 2:22. doi: 10.1186/1742-2094-2-22
Tian, G., Kong, Q., Lai, L., Ray-Chaudhury, A., and Lin, C.-L. (2010). Increased expression of cholesterol 24S-hydroxylase results in disruption of glial glutamate transporter EAAT2 association with lipid rafts: a potential role in Alzheimer’s disease. J. Neurochem. 113, 978–989. doi: 10.1111/j.1471-4159.2010.06661.x
Tong, J., Huang, C., Bi, F., Wu, Q., Huang, B., Liu, X., et al. (2013). Expression of ALS-linked TDP-43 mutant in astrocytes causes non-cell-autonomous motor neuron death in rats. EMBO J. 32, 1917–1926. doi: 10.1038/emboj.2013.122
Tong, X., Ao, Y., Faas, G. C., Nwaobi, S. E., Xu, J., Haustein, M. D., et al. (2014). Astrocyte Kir4.1 ion channel deficits contribute to neuronal dysfunction in Huntington’s disease model mice. Nat. Neurosci. 17, 694–703. doi: 10.1038/nn.3691
Torres-Platas, S. G., Nagy, C., Wakid, M., Turecki, G., and Mechawar, N. (2016). Glial fibrillary acidic protein is differentially expressed across cortical and subcortical regions in healthy brains and downregulated in the thalamus and caudate nucleus of depressed suicides. Mol. Psychiatry 21, 509–515. doi: 10.1038/mp.2015.65
Tsai, H.-H., Li, H., Fuentealba, L. C., Molofsky, A. V., Taveira-Marques, R., Zhuang, H., et al. (2012). Regional astrocyte allocation regulates CNS synaptogenesis and repair. Science 337, 358–362. doi: 10.1126/science.1222381
Turner, R. C., Lucke-Wold, B. P., Robson, M. J., Lee, J. M., and Bailes, J. E. (2016). Alzheimer’s disease and chronic traumatic encephalopathy: distinct but possibly overlapping disease entities. Brain Inj. 30, 1279–1292. doi: 10.1080/02699052.2016.1193631
Vasile, F., Dossi, E., and Rouach, N. (2017). Human astrocytes: structure and functions in the healthy brain. Brain Struct. Funct. 222, 2017–2029. doi: 10.1007/s00429-017-1383-5
Wallingford, J., Scott, A. L., Rodrigues, K., and Doering, L. C. (2017). Altered developmental expression of the astrocyte-secreted factors hevin and SPARC in the fragile X mouse model. Front. Mol. Neurosci. 10:268. doi: 10.3389/fnmol.2017.00268
Wang, C., Wilson, W. A., Moore, S. D., Mace, B. E., Maeda, N., Schmechel, D. E., et al. (2005). Human apoE4-targeted replacement mice display synaptic deficits in the absence of neuropathology. Neurobiol. Dis. 18, 390–398. doi: 10.1016/j.nbd.2004.10.013
Xin, W., and Bonci, A. (2018). Functional astrocyte heterogeneity and implications for their role in shaping neurotransmission. Front. Cell. Neurosci. 12:141. doi: 10.3389/fncel.2018.00141
Xiu, J., Nordberg, A., Zhang, J. T., and Guan, Z. Z. (2005). Expression of nicotinic receptors on primary cultures of rat astrocytes and up-regulation of the alpha7, alpha4 and beta2 subunits in response to nanomolar concentrations of the beta-amyloid peptide(1-42). Neurochem. Int. 47, 281–290. doi: 10.1016/j.neuint.2005.04.023
Zhang, X., Wan, J. Q., and Tong, X. P. (2018). Potassium channel dysfunction in neurons and astrocytes in Huntington’s disease. CNS Neurosci. Ther. 24, 311–318. doi: 10.1111/cns.12804
Zhang, Y., Sloan, S. A., Clarke, L. E., Caneda, C., Plaza, C. A., Blumenthal, P. D., et al. (2016). Purification and characterization of progenitor and mature human astrocytes reveals transcriptional and functional differences with mouse. Neuron 89, 37–53. doi: 10.1016/j.neuron.2015.11.013
Zhao, J., Davis, M. D., Martens, Y. A., Shinohara, M., Graff-Radford, N. R., Younkin, S. G., et al. (2017). APOE 𝜀4/𝜀4 diminishes neurotrophic function of human iPSC-derived astrocytes. Hum. Mol. Genet. 26, 2690–2700. doi: 10.1093/hmg/ddx155
Zhao, T., Hong, Y., Yin, P., Li, S., and Li, X.-J. (2017). Differential HspBP1 expression accounts for the greater vulnerability of neurons than astrocytes to misfolded proteins. Proc. Natl. Acad. Sci. 114, E7803–E7811. doi: 10.1073/pnas.1710549114
Zhao, T., Hong, Y., Li, S., and Li, X.-J. (2016). Compartment-dependent degradation of mutant huntingtin accounts for its preferential accumulation in neuronal processes. J. Neurosci. 36, 8317–8328. doi: 10.1523/JNEUROSCI.0806-16.2016
Zhao, Z., Nelson, A. R., Betsholtz, C., and Zlokovic, B. V. (2015). Establishment and dysfunction of the blood-brain barrier. Cell 163, 1064–1078. doi: 10.1016/j.cell.2015.10.067
Keywords: astrocyte, frontal cortex, Alzheimer’s disease, Huntington’s disease, frontotemporal dementia, neurodegeneration, induced pluripotent stem cells
Citation: Peteri U-K, Niukkanen M and Castrén ML (2019) Astrocytes in Neuropathologies Affecting the Frontal Cortex. Front. Cell. Neurosci. 13:44. doi: 10.3389/fncel.2019.00044
Received: 31 October 2018; Accepted: 28 January 2019;
Published: 12 February 2019.
Edited by:
Sara Xapelli, Universidade de Lisboa, PortugalReviewed by:
João Filipe Oliveira, University of Minho, PortugalCopyright © 2019 Peteri, Niukkanen and Castrén. This is an open-access article distributed under the terms of the Creative Commons Attribution License (CC BY). The use, distribution or reproduction in other forums is permitted, provided the original author(s) and the copyright owner(s) are credited and that the original publication in this journal is cited, in accordance with accepted academic practice. No use, distribution or reproduction is permitted which does not comply with these terms.
*Correspondence: Maija L. Castrén, TWFpamEuQ2FzdHJlbkBoZWxzaW5raS5maQ==
Disclaimer: All claims expressed in this article are solely those of the authors and do not necessarily represent those of their affiliated organizations, or those of the publisher, the editors and the reviewers. Any product that may be evaluated in this article or claim that may be made by its manufacturer is not guaranteed or endorsed by the publisher.
Research integrity at Frontiers
Learn more about the work of our research integrity team to safeguard the quality of each article we publish.