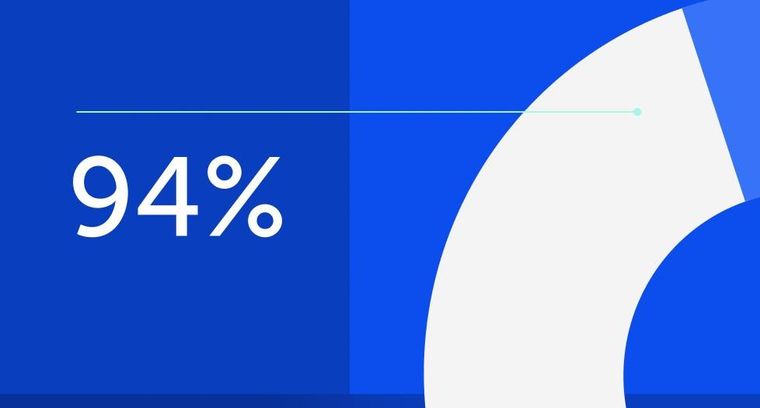
94% of researchers rate our articles as excellent or good
Learn more about the work of our research integrity team to safeguard the quality of each article we publish.
Find out more
MINI REVIEW article
Front. Cell. Neurosci., 14 March 2018
Sec. Cellular Neurophysiology
Volume 12 - 2018 | https://doi.org/10.3389/fncel.2018.00066
This article is part of the Research TopicSynaptic vesicle cycle: cellular and molecular mechanismsView all 15 articles
The intact synaptic structure is critical for information processing in neural circuits. During synaptic transmission, rapid vesicle exocytosis increases the size of never terminals and endocytosis counteracts the increase. Accumulating evidence suggests that SV exocytosis and endocytosis are tightly connected in time and space during SV recycling, and this process is essential for synaptic function and structural stability. Research in the past has illustrated the molecular details of synaptic vesicle (SV) exocytosis and endocytosis; however, the mechanisms that timely connect these two fundamental events are poorly understood at central synapses. Here we discuss recent progress in SV recycling and summarize several emerging mechanisms by which synapses can “sense” the occurrence of exocytosis and timely initiate compensatory endocytosis. They include Ca2+ sensing, SV proteins sensing, and local membrane stress sensing. In addition, the spatial organization of endocytic zones adjacent to active zones provides a structural basis for efficient coupling between SV exocytosis and endocytosis. Through linking different endocytosis pathways with SV fusion, these mechanisms ensure necessary plasticity and robustness of nerve terminals to meet diverse physiological needs.
Synaptic transmission is fundamental to brain function. Nerve terminals release neurotransmitter at a different speed, depending on the types of synapses and stimulation. The rate of synaptic vesicle (SV) exocytosis directly controls neurotransmission strength. It can vary a few orders of magnitude at a single terminal (from <1 Hz up to ~1,000 Hz) (Lou et al., 2005) and thus provide a large dynamic range of synaptic transmission (Schneggenburger and Rosenmund, 2015). Depending on release probability and readily releasable vesicle pool (RRP) size, a presynaptic terminal can rapidly release numerous SVs during a brief train of action potentials (APs) (Neher, 2015).
Given the smaller size of AZs, each SV fusion can significantly expand the plasma membrane (PM) of AZs and thus impact its ultrastructure and function (Figure 1). Remarkably, synapses are capable to counteract this structural change by endocytosis in a timely fashion (Ceccarelli et al., 1972; Heuser and Reese, 1973). The temporal coupling between exocytosis and endocytosis ensure the structural stability and functional integrity of chemical synapses during active SV recycling.
Figure 1. The transient expansion of the presynaptic surface area. (A) Ultrastructure of the presynaptic nerve terminals in a mouse cerebellum (chemical fixation). Note two AZs with high electronic density (between the arrowheads). (B) The surface area of a single SV after it fuses and merges with the PM at an AZ. The scheme size is shown in scale, the AZ area is 0.4 μm2 (Holderith et al., 2012) and SV diameter is 45 nm (Lou et al., 2008). (C) Transient changes of the surface area are recorded at the calyx of Held during a 20 ms depolarization pulse (arrow) (Lou et al., 2008). Note the rapid increase and subsequent recovery of Cm, suggesting SV fusion and compensatory endocytosis.
Decades of research have generated a wealth of knowledge on the molecular mechanisms of SV exocytosis (Jahn and Fasshauer, 2012; Südhof, 2013; Herman and Rosenmund, 2015) and endocytosis (Saheki and De Camilli, 2012; Wu L. G. et al., 2014; Soykan et al., 2016). However, exactly how synapses timely coordinate these two fundamental events remains largely ambiguous. SV exocytosis, which occurs within a millisecond, is much faster than any type of SV endocytosis reported so far. During rapid neurotransmission, repeated SV release places an important task for synapses to avoid SV traffic jam. The presence of synaptic active zones (AZs), a highly-specialized structure that regulates SV trafficking (recruitment, docking, fusion and coupling to endocytosis), makes the exo-endocytosis coupling of SVs more complex than dense core vesicles (DCVs) in other types secretory cells (Liang et al., 2017; Neher, 2018). This paper focuses primarily on SV exocytosis-endocytosis coupling at the presynaptic nerve terminals.
SV exocytosis–endocytosis coupling exists at a variety of nerve terminals. The direct evidence in living synapses comes from two types of experiments. One is capacitance recordings, which monitor cell surface area (Neher and Marty, 1982). The presynaptic membrane capacitance (Cm) shows a depolarization-triggered Cm increase and a subsequent recovery to baseline (Sun and Wu, 2001; Lou et al., 2008), indicating SV fusion with the PM and an equal amount of membrane retrieval afterward (Figure 1C). The PM expansion is transient in response to a short pulse but lasts longer during continuous stimulation, indicating the net balance of exocytosis and endocytosis. Similar Cm changes have been reported at other types of nerve terminals, such as ribbon synapses in the retina (von Gersdorff and Matthews, 1994; Neves and Lagnado, 1999) and hair cells (Moser and Beutner, 2000), mossy fiber boutons in the hippocampus (Hallermann et al., 2003) and cerebellum (Delvendahl et al., 2016). The second evidence is from interference reflection microscopy at living ribbon synapses. Direct imaging demonstrates rapid cell surface expansion and subsequent recovery that matches with the Cm responses (Llobet et al., 2003).
Optical imaging with pH-sensors also supports the tight exocytosis-endocytosis coupling. At individual synapse levels, APs trigger a rise-and-fall of fluorescence from pHluorin-tagged SV proteins (Sankaranarayanan and Ryan, 2000). At the single SV level, the timely coupling is also demonstrated by VAMP2-pHluorin (Gandhi and Stevens, 2003), quantal dots (Zhang et al., 2009), synaptophysin-pHluorin (Granseth et al., 2006; Zhu et al., 2009), and vGlut-pHluorin (Balaji and Ryan, 2007; Leitz and Kavalali, 2014). Similarly, cypHer-tagged probes produce a mirror response (fall-and-rise) to pHluorin sensors because of its opposite pH sensitivity (Hua et al., 2013). These data suggest a tight balance between SV exocytosis and endocytosis at central synaptic terminals.
In addition, electron microscopy (EM) studies support the ultrastructure changes of motor nerve terminals in frog neuromuscular junctions (NMJs) (Ceccarelli et al., 1972; Heuser and Reese, 1973). The quick-freezing EM examines the nerve terminals at different intervals post an AP and demonstrates the brief surface expansion and subsequent recovery (Miller and Heuser, 1984), with an AZ ultrastructure recovery within ~90 s. A recent flash-freezing EM study in hippocampus cultures reported a much fast coupling (within ~100 ms) between SV exocytosis and endocytosis. On average, ~0.7 SV exocytosis is coupled with ~0.6 SV-equivalent membrane retrieval per synapse (Watanabe et al., 2013b). Cortical synapses are able to maintain their size both in cultures (Hayashi et al., 2008) and intact brain circuitry (Lou et al., 2008) even in the absence of dynamin-1, a protein involved in vesicle fission. Under strong stimulation, bulk endocytosis is upregulated about 2-fold in dynamin KO synapses to counteract the nerve terminal expansion (Hayashi et al., 2008; Wu Y. et al., 2014).
The timely coupling of exocytosis and endocytosis has a profound impact on synaptic structure and function. First, it preserves the overall size of nerve terminals. Second, it recycles SV components that are required for new SV production (Dittman and Ryan, 2009). Long-term neurotransmission relies on endocytosis and SV recycling, even though RRP and reserve pool SVs can support short-term neural activity (Rizzoli and Betz, 2005; Neher, 2015). In addition, only a very small portion of total SVs participate actively in vesicle recycling during sustained transmission (Denker et al., 2011), adding another layer of urgency for rapid SV regeneration.
Third, the timely retrieval of SV components is important for release-site clearance (Neher, 2010). The limited release-sites must be re-used during sustained neurotransmission. Each SV fusion leads to two basic impacts: (1) vesicle components (e.g., SV proteins Takamori et al., 2006, used SNAREs) occupy release sites and need to be cleared away rapidly (Neher, 2010). The efficient clearance would make release-site re-competent for a new round of vesicle fusion, and this process may become rate-limiting during fast neurotransmission (Neher, 2010; Hua et al., 2013; Mahapatra et al., 2016). 2) ultrastructure change of AZs. Given the average size of SVs (~45 nm in diameter) (Lou et al., 2008) and AZs (~0.04 μm2, ranging from 0.02 ~ 0.2 μm2) (Han et al., 2011; Holderith et al., 2012) (Figure 1), each SV fusion adds an additional membrane area of ~6,362 nm2 (A = 4 π r2), ~16% of the original AZ area (Figure 1B). This expansion can alter AZ nanostructure and release-site organization (t-SNARE, complexin, Munc-13, Ca2+ channels, etc). The quick-freezing EM study in NMJs (Heuser and Reese, 1981) has captured such effects. These two impacts may become prominent during repeated SV fusion since most SVs fuse at AZs (Figure 1A) rather than randomly. Thus, tight SV exocytosis-endocytosis coupling helps to maintain AZ ultrastructure integrity.
Four types of endocytosis are proposed at synapses: “kiss and run” (K&R), clathrin-mediated endocytosis (CME), bulk endocytosis, and ultrafast endocytosis (for details, see Saheki and De Camilli, 2012; Alabi and Tsien, 2013; Wu L. G. et al., 2014; Soykan et al., 2016; Watanabe and Boucrot, 2017). None of them alone can account for all the experimental observations at synapses in literature. Each endocytosis route likely couples differently with exocytosis events, depending on neural activity as well as synapse types.
First, endocytosis couples with exocytosis at the same site via K&R (or Kiss-and-stay). An SV fuses with the PM and pinches off at the same location without collapsing (Alabi and Tsien, 2013). This mechanism is first proposed by Ceccarelli and colleague based on EM studies (Ceccarelli et al., 1972), and it remains highly debatable since then. K&R at synapses completes within ~0.5 s (Gandhi and Stevens, 2003; Zhang et al., 2009) or ~0.3 s (He et al., 2006). This coupling mode is thought to promote rapid SV recycling because of its fast speed than CME.
Second, SVs fuse at AZs and are retrieved by CME at endocytic zones (EZs) (Dittman and Ryan, 2009; Saheki and De Camilli, 2012). CME is proposed by Heuser and Reese (Heuser and Reese, 1973, 1981) and subsequently investigated extensively (Dittman and Ryan, 2009; Saheki and De Camilli, 2012). It operates at a time constant (τ) of ~15 −20 s according to pHluorin assays (Granseth et al., 2006; Balaji and Ryan, 2007), consistent with the Cm assays at the calyx of Held (τ = ~10–25 s) (Wu et al., 2005; Yamashita et al., 2005; Lou et al., 2008). CME accelerates at physiological temperature (PT, 37°C) (Renden and von Gersdorff, 2007; Wu et al., 2016) as compared to room temperature (RT), despite the estimated endocytosis rate varies largely among different research groups [e.g., τ = ~3 s (Leitz and Kavalali, 2011), 6 s (Balaji and Ryan, 2007; Armbruster et al., 2013), and 20 s (Soykan et al., 2017)]. Multiple lines of evidence support that CME is a dominant endocytosis pathway at nerve terminals (Heuser and Reese, 1973; Granseth et al., 2006; Dittman and Ryan, 2009; Saheki and De Camilli, 2012). For example, typical SV endocytosis is severely impaired after clathrin-knockdown (Granseth et al., 2006; Nicholson-Fish et al., 2015) and AP-2 α-μ2 double mutants in C. elegans (Gu et al., 2013). CME is also critical in squid giant synapses (10–15°C) (Augustine et al., 2006), whose physiological temperature is low; perturbations of clathrin assembly (or uncoating) showed a loss of SVs and expansion of presynaptic PM area (Morgan et al., 1999, 2000). However, this notion is challenged by recent studies (Sato et al., 2009; Kononenko et al., 2014; Watanabe et al., 2014; Delvendahl et al., 2016; Soykan et al., 2017). This is a critical question especially for mammals in which synapses operate routinely at 37°C. Therefore, more work is required to address SV CME under physiological condition.
Third, SV fusion couples with bulk endocytosis. Bulk endocytosis is observed frequently under EM, where it displays as large membrane vacuoles with variable sizes (~80–300 nm in diameter) (Miller and Heuser, 1984; Hayashi et al., 2008; Wu Y. et al., 2014). Further EM tomography demonstrates that those vacuole structures are unconnected with the presynaptic PM (Hayashi et al., 2008). Bulk endocytosis requires VAMP-4 (Nicholson-Fish et al., 2015) and F-actin (Shupliakov et al., 2002; Holt et al., 2003), and it occurs mainly during high-frequency APs as shown in neuronal cultures (Clayton et al., 2008; Wu Y. et al., 2014). Bulk endocytosis has a higher capacity to retrieve the PM (Shupliakov et al., 2002; Wu and Wu, 2007; Lou et al., 2008) but possesses a poorer cargo-selectivity and quality control than CME (Miller and Heuser, 1984; Nicholson-Fish et al., 2015). In addition, some large PM cisterns are also observed 15 min after stimulation in frog NMJs (Heuser and Reese, 1973), indicating a delayed or different form of bulk endocytosis. It is unclear whether this type of bulk endocytosis is relevant to the step-like Cm decrease recorded at the calyx of Held (Wu and Wu, 2007; Lou et al., 2008). Bulk endocytosis likely serves as an emergency endocytosis route for synapses to counteract their surface expansion during high neural activity.
Finally, SV fusion couples with ultrafast endocytosis. Ultrafast endocytosis is reported by Watanabe et al using high-pressure freezing EM in combination with optogenetic stimulation (Watanabe et al., 2013a,b). Synapses expressing channelrhodopsin are stimulated with a blue light to trigger neurotransmitter release. Ultrafast endocytosis peaks at 50–100 ms of stimulation and generates uncoated vesicles with a uniform size (~82 nm in diameter, ~2-fold larger than SVs) (Watanabe et al., 2013b, 2014). Ultrafast endocytosis operates selectively at 34 ~ 37°C (but fails at 20°C) in mammalian synapses (Watanabe et al., 2014) although it also appears at RT in motor nerve terminals of C. elegans (Watanabe et al., 2013a) (whose cultivation temperature spans 15 ~ 25°C). Ultrafast endocytosis requires SV fusion and F-actin. It is also sensitive to dynasore, a dynamin inhibitor, but its effect on F-actin and other targets (Park et al., 2013; Mahapatra et al., 2016) should be carefully considered. This new form of SV exocytosis-endocytosis coupling adds a new layer of complexity to SV recycling.
Interestingly, in the time-resolved quick-freezing EM study (with a single AP stimulation at RT) (Heuser and Reese, 1981), Heuser and Reese have reported both CME and “a second form of membrane retrieval.” The latter operates through “a random bite” of a large piece of plasma membrane without clathrin-coat, similar to bulk endocytosis. Moreover, it occurs “in the first a few milliseconds after stimulation” (Heuser and Reese, 1981), similar to (if not faster than) the ultrafast endocytosis under flash-freezing EM at PT. It is unclear whether they are the same form of endocytosis.
While the details of CME have been addressed, the mechanism for other forms of endocytosis remains poorly understood. Future work on molecular characterization may help to better define these different forms of endocytosis. Ultrafast endocytosis and bulk endocytosis exhibit different properties (in speed, retrieval size, temperature sensitivity, and stimulation strength to trigger them), but they also have some common features (e.g., high capacity, clathrin-independence, F-actin dependence, and endosome sorting).
Despite the significant progress on SV exocytosis as well as endocytosis, very little is known about their temporal and spatial coupling. What triggers SV endocytosis at the right time and place remains a longstanding question. Recent work suggests that several factors are involved in SV exocytosis-endocytosis coupling, in which a synapse “senses” the SV exocytosis event and initiates endocytosis. Here, we discuss the potential means of coupling at nerve terminals.
Intracellular Ca2+ classically regulates both exocytosis and endocytosis (Neher and Sakaba, 2008) and is therefore a suitable candidate for the coupling (Figure 2A). Intracellular Ca2+ micro- or nano-domains (Neher, 1998) are generated during an AP due to the uneven distribution of Ca2+ channels at AZs (Althof et al., 2015; Nakamura et al., 2015). While the local Ca2+ domains tightly regulate SV fusion (Eggermann et al., 2011; Schneggenburger et al., 2012), they are also required in SV endocytosis as demonstrated at the mature calyx of Held (Yamashita et al., 2010). Ca2+ uncaging experiments suggest that a ~15 μM Ca2+ increase is needed to trigger rapid endocytosis in inner hair cells, despite endocytosis remaining constant (τ = 16 s) below that Ca2+ level (Beutner et al., 2001). A minimal of ~10 μM Ca2+ is necessary to initiate endocytosis at the calyx of Held (Hosoi et al., 2009), consistent with the result (~11 μM) from lamprey reticulospinal synapses (Gad et al., 1998). These Ca2+ values are as high as that for triggering SV fusion (Bollmann et al., 2000; Schneggenburger and Neher, 2000; Lou et al., 2005; Neher and Sakaba, 2008; Kochubey et al., 2011), implying that endocytosis occurs much closer to Ca2+ channels than previously thought. Accordingly, AP-2 is shown to interact with Ca2+ channels at the synprint region (Watanabe et al., 2010). Therefore, intracellular Ca2+ is likely a trigger for endocytosis.
Figure 2. Four hypothetical mechanisms by which synapses sense exocytosis and initiate endocytosis in time and space. (A) Ca2+ is a trigger, and synaptotagmin and CaM are sensors in the SV exocytosis-endocytosis coupling. (B) SV fusion is a trigger, and SV proteins (both transmembrane and associated proteins) are sensors to initiate CME by interacting with AP-2. (C) SV fusion is a trigger to generate the local membrane stress. (D) The annular spatial arrangement of EZs surrounding AZs. The large scaffolds are critical for SV exocytosis-endocytosis coupling by facilitating SV proteins transportation and recapture.
Following Ca2+ elevation, synaptotagmin acts as a Ca2+ sensor for endocytosis, similar to its function in exocytosis (Geppert et al., 1994; Chapman, 2008). Genetic perturbations of synaptotagmin severely impair SV endocytosis (Poskanzer et al., 2003; Llinás et al., 2004; Nicholson-Tomishima and Ryan, 2004; Yao et al., 2012). The Ca2+ sensing property of synaptotagmin is critical for this effect (Poskanzer et al., 2006; Yao et al., 2011). It is reported that different Ca2+ binding affinity of Ca2+ sensors (e.g., synaptotagmin-1 and−7) allows differential endocytic regulation under weak and strong stimulations (Li et al., 2017). Similarly, Ca2+ sensor otoferlin is found to regulate endocytosis in hair cells (Duncker et al., 2013).
In addition to synaptotagmin and otoferlin, calmodulin (CaM) is another Ca2+ sensor for endocytosis (Figure 2A). Intracellular Ca2+ elevation activates CaM-calcineurin to dephosphorylate dephosphins, a group of endocytosis proteins that are constitutively phosphorylated at rest (Cousin and Robinson, 2001). Interruptions of CaM (Artalejo et al., 1996; Wu et al., 2009; Yao and Sakaba, 2012) and calcineurin (Marks and McMahon, 1998; Sun et al., 2010; Yamashita et al., 2010; Wu X. S. et al., 2014) inhibit endocytosis, although results vary with stimulation conditions (Yao and Sakaba, 2012) and age (Yamashita et al., 2010). Dynamin-1 (Serines at 774 and Ser 778) dephosphorylation and phosphorylation mutations abolish the biphasic regulation of Ca2+ on SV endocytosis (Armbruster et al., 2013). This effect depends on dynamin interactions with syndapin-1 rather than endophilin or synaptophysin (Anggono et al., 2006). After the neural activity, intracellular Ca2+ recovery and CaM-calcineurin activity decreases; meanwhile cyclin-dependent kinase 5 (Cdk5) rephosphorylates dephosphins to terminate endocytosis (Tan et al., 2003). This CaM-calcineurin/Cdk5 balance offers another way of Ca2+-sensing in SV exocytosis-endocytosis coupling. In addition, it is reported that CaM interacts with N-BAR proteins Rvs167 (in yeast), amphiphysin and endophilin-A (Myers et al., 2016), suggesting a calcineurin-independent regulation of CaM on endocytosis.
Two relevant questions are noteworthy. First, Ca2+ elevation alone appears insufficient in triggering endocytosis, as demonstrated by the experiment in munc13-1 and−2 double KO synapses (Watanabe et al., 2013b). Accordingly, perturbations of Ca2+ downstream molecules (e.g., synaptotagmin, CaM, and calcineurin) do not abolish endocytosis in many studies. High sucrose stimulation also triggers efficient endocytosis (Yao et al., 2011). These data suggest that Ca2+ needs to work with other factors. Second, Ca2+ has a more complex role in endocytosis than in exocytosis (Leitz and Kavalali, 2016). For example, local Ca2+ domains accelerate endocytosis but a global dialysis of Ca2+ inhibits it (Wu and Wu, 2014); similar counteracting effects also occurs at the same synapses under different conditions (Armbruster et al., 2013): accelerating endocytosis under moderate stimulation and slowing it down under strong stimulation.
There are a large number of proteins on individual SVs. After SV fusion, some of SV proteins that newly added to the PM are suitable candidates for SV exocytosis-endocytosis coupling, which allow synapses to “sense” SV fusion events and initiate endocytosis in a timely fashion (Figure 2B).
This molecule has a high copy number on each SV (15 copies/SV) (Takamori et al., 2006). After its delivery to the PM, synaptotagmin serves as a nucleating factor to recruit AP-2 (Haucke and De Camilli, 1999), a key component of CME. it can directly interact with clathrin-associated sorting protein stonin-2 (Jung et al., 2007), adaptor protein AP-2 (Willox and Royle, 2012) and SV2A/B (Kaempf et al., 2015). Consequently, synaptotagmin shows dual roles in SV endocytosis: sensing Ca2+ and nucleating CME components.
VAMP2 (also called Synaptobrevin-2) is a core protein of SNARE complex and has the highest copy number (~70 copies/SV) on SVs (Takamori et al., 2006). After VAMP-2 interruptions, endocytosis is impaired as shown in experiments using VAMP-2 KO (Deák et al., 2004), knockdown (Zhang et al., 2013) and its blocking peptide or cleavage toxin (tetanus toxin) (Hosoi et al., 2009; Xu et al., 2013). VAMP-4 is reported to regulate bulk endocytosis selectively (Nicholson-Fish et al., 2015). Interestingly, other components of SNARE machinery have also been reported to regulate endocytosis, including syntaxin1a (Xu et al., 2013), SNAP-25 (Xu et al., 2013; Zhang et al., 2013), and complexin (Li et al., 2017). Syntaxin1a and SNAP-25 probably play a permissive role rather than acting as a trigger in SV endocytosis, since they are already present abundantly on the PM before SV fusion.
Synaptophysin-1 has the second highest copy number on SVs (32 copies/SV) and resides exclusively on SVs. Both properties make it a suited endocytosis sensor. Synaptophysin-1 KO blocks VAMP-2 endocytosis (Gordon et al., 2011) in a stimulation- and frequency-dependent manner (e.g., 200 AP at 20 Hz) (Kwon and Chapman, 2011; Rajappa et al., 2016). Endophilin plays a role in CME and SV uncoating (Milosevic et al., 2011); it's activity-dependent sub-synaptic translocation (from SV clusters to EZs) in C-elegant DA neurons (Bai et al., 2010) indicates its potential role in facilitating efficient coupling. Moreover, endophilin can bind dynamin, synaptojanin (Milosevic et al., 2011), and vGlut-1 (Voglmaier et al., 2006), and thus accelerates CME. Knockdown of vGlut-1 also slows down endocytosis of SV2 and synaptophysin, suggesting a new role of vGlut-1 in regulating cargo sorting and endocytosis during CME (Pan et al., 2015).
This is an SV-associated protein with Ca2+-permeable channel property (Yao et al., 2009). Once inserting in the PM after SV fusion, Flower is an ideal factor to generate local Ca2+ elevation for endocytosis. The flower was identified by a forward genetic screening in Drosophila, in which Flower mutants exhibited impaired endocytosis and basal Ca2+ (Yao et al., 2009). It is able to form Ca2+ permeable channels in vitro and to increase intracellular Ca2+ concentration when expressed in salivary gland cells. This phenomenon is similar to the case of P-type Ca2+ channels in sea urchin eggs (Smith et al., 2000), in which the channels on secretory granules insert in the PM after exocytosis and determine the endocytosis location. However, the channel activity of Flower is undetectable at the calyx of Held synapses (Xue et al., 2012); its Ca2+ permeability appears to be critical selectively for bulk endocytosis (Yao et al., 2017).
SV fusion and endocytosis occur at adjacent PM domains: AZs and EZs, respectively. The AZ is a tiny presynaptic area with high electron-density (Gundelfinger and Fejtova, 2012). AZs contain a set of large, multiple-domain proteins (called the cytomatrix of AZs, CAZs), including CAST/ELKS/Bruchpilot protein (Brp), Liprin-α, Rab3-interacting molecules (RIMs), RIM-binding proteins (RIM-BPs), Bassoon, Piccolo/Aczonin, and UNC-13/Munc-13 (Gundelfinger and Fejtova, 2012; Südhof, 2012; Ackermann et al., 2015). CAZs control SV dynamics and release probability by regulating AZ ultrastructure. Genetic perturbations of key genes encoding CAZ proteins impair neurotransmission (Südhof, 2012; Ackermann et al., 2015). For example, Brp mutation in fly NMJ causes AZ (T-bar) disassembly, Ca2+ channel cluster loss, and exocytosis defect (Fouquet et al., 2009). ELSK and RIM double KO synapses in mice display similar phenotypes: AZ disassembly, lack of docked SVs, decreased transmitter release, and degradation of other CAZ proteins (Wang et al., 2016). In addition to functioning in SV fusion, CAZ proteins also coordinate exocytosis-endocytosis coupling (Haucke et al., 2011) by promoting SV protein sorting, transportation from AZs to EZs, and recruitment of endocytosis proteins. Available work focuses primarily on transmitter release—future work is required to explore their roles in SV exocytosis-endocytosis coupling.
EZs have abundant endocytosis proteins such as AP-2, clathrin, dynamin, DAP160, and intersection (Wahl et al., 2013; Gimber et al., 2015). The nanoscale organization of EZs at nerve terminals remains largely unclear. It seems excluded from but adjacent to AZs. An annulus EZ surrounding an AZ is reported at drosophila NMJs (Roos and Kelly, 1999); this spatial arrangement between EZs and AZs can facilitate re-capture of SV proteins once they diffuse away from AZs, providing a structural base for the efficient exocytosis-endocytosis coupling (Figure 2D). Based on free diffusion of VAMP2 at a diffusion coefficient (D) of 0.2 μm2/s at the presynaptic PM (Ramadurai et al., 2009; Gimber et al., 2015; Joensuu et al., 2016), it takes ~2.5 s to diffuse ~1 μm [t = x2/(2 * D)]. In addition to annulus EZs, other forms of EZ organization is also possible in different types of never terminals, including random distribution in the terminals or in patches at peri-AZs (similar to the EZ around PSDs; Lu et al., 2007). EZs appear relatively stable during stimulation and offer a platform where some endocytosis proteins with multiple domains can stabilize other endocytosis proteins and recruit SV proteins. For example, intersectin/DAP160, which has five SH3 domains, binds many proteins including dynamin, synaptojanin, stonin-2, N-WASP, Eps15 homology (EH) domains, and SNAP-25 (Roos and Kelly, 1998; Evergren et al., 2007). Loss of Dap16/Intersectin impairs the FM1-43 loading in fly NMJs and destabilizes dynamin, synaptojanin, and endophilin (Koh et al., 2004; Marie et al., 2004), suggesting its role in stabilizing endocytosis machinery at EZs (Pechstein et al., 2010). However, intersectin-1 KO synapses (Yu et al., 2008) exhibit little endocytosis defect (Sakaba et al., 2013), possibly due to its redundancy in mammals.
Filamentous actin (F-actin) is highly enriched in EZs. An annulus of F-actin is shown to surround the AZ in motor terminals of lamprey (Shupliakov et al., 2002; Bloom et al., 2003; Morgan et al., 2004) and NMJs (Richards et al., 2004), implying its important role in endocytosis. Disruption of F-actin inhibits multiple forms of endocytosis at nerve terminals (Shupliakov et al., 2002; Watanabe et al., 2013b; Wu et al., 2016) (but see Sankaranarayanan et al., 2003). Meanwhile, F-actin also enhances SV replenishment, priming, and fusion at synapses (Sakaba and Neher, 2003; Cingolani and Goda, 2008; Lee et al., 2012, 2013). The dual-role of F-actin in both exocytosis and endocytosis suggests its potential role in coupling these two processes. The underlying details are unclear. F-actin likely enhances SV protein diffusion between AZs to EZs, traps SV proteins in EZs and slows their escaping, and promotes SV scission.
Dynamin is a key component that regulates different endocytosis at nerve terminals. Among three dynamin genes in mammals, Dynamin-1 is the major isoform in neurons (Ferguson et al., 2007). Dynamin-1 KO impairs CME but increases bulk endocytosis ~2-fold (Hayashi et al., 2008; Wu Y. et al., 2014), suggesting its different roles in CME and bulk endocytosis. Dynamin-1 KO calyces alter the short-term plasticity via different mechanisms (Mahapatra et al., 2016; Mahapatra and Lou, 2017). The reduction of synaptic depression selectively at high frequency (>100 Hz) APs (Mahapatra et al., 2016) agrees with the change of endocytosis from CME to the enhanced bulk endocytosis in the absence of dynamin-1 (Mahapatra et al., 2016). Dynamin-1 and-3 double KO exaggerates the phenotypes of single dynamin-1 KO (Raimondi et al., 2011; Fan et al., 2016). In the native brain circuitry, dynamin-mediated endocytosis is required for synapse development and maturation (Fan et al., 2016). Dynamin inhibitors are useful tools in endocytosis studies, but the data interpretation may be more complex than it seems because of its off-target effects (Park et al., 2013; Mahapatra et al., 2016). This sometimes can lead to different conclusions. For example, dynasore blocks ultrafast endocytosis, but it also affects actin (Park et al., 2013), a factor is critical for ultrafast endocytosis (Watanabe et al., 2013b). Moreover, it seems challenging for dynamin molecules to recruit, polymerize and disassemble at fission necks in ultrafast endocytosis, because these processes are known to be slow, ~24 s in CME as measured by direct TIRF imaging in non-excitatory cells (Merrifield et al., 2002; Doyon et al., 2011; Taylor et al., 2011). That is several orders of magnitude slower than 100 ms. The presence of preassembled clathrin coatsd (Wienisch and Klingauf, 2006) can accelerate endocytosis, but clathrin is not required in ultrafast endocytosis at PT (Watanabe et al., 2014) (which agrees with the slow assembly dynamics of clathrin coats; Cocucci et al., 2012). Another possibility is that the dynamics and process of dynamin assembly at fission sites are somewhat different between non-neuronal cells and synapses, such as stronger membrane bending property of dyanmin-1 at synapses than dynamin-2 at non excitatory cells (Liu et al., 2011) and a higher number of dynamin molecules pre-localized at EZs. Therefore, future work is required to address how synapses may use dynamin differently as the fission machinery to regulate different modes of SV exocytosis-endocytosis coupling.
There exists dramatic membrane stress at presynaptic terminals during exocytosis and endocytosis. The local stress can arise from SV fusion itself. SVs are the smallest bi-layer membranous structures that nature can make, with an outer/inner diameter ratio of up to ~4:3 or higher. SV fusion adds more membrane on the inner leaflet than the outer leaflet of the PM, which can produce an asymmetric membrane stress at the local PM. This local stress may delay SV flattening after fusion, promote PM lateral diffusion, and/or enhance PM invagination at EZs (only ~100 nm away from AZs). Furthermore, insertion of SV proteins alters the rigidity of local PM, a factor playing important role in endocytosis (Hassinger et al., 2017).
The local membrane stress likely facilitates the coupling between exocytosis and endocytosis (Figure 2C). The mechanical spread of membrane stress can be fast at AZs and is suitable to trigger those fast forms of endocytosis (e.g., ultrafast endocytosis, K&R, and bulk endocytosis). For example, while it is challenging to utilize traditional molecule signaling as in CME for ultrafast endocytosis, the local membrane stress generated by SV fusion may transfer instantly from AZs to EZs. The downside of this mechanism is that SV components removing from AZs may not be as fast as the force transfer and thus limit the benefit of ultrafast endocytosis during high-frequency neurotransmission.
The characterization of local membrane mechanics is not well-established during SV exocytosis-endocytosis, and it is likely affected by several factors including membrane tension, membrane stiffness, and local force (Hassinger et al., 2017). As a key force generator in cells, F-actin is shown to be required in multiform of endocytosis including ultrafast endocytosis (Watanabe et al., 2013b) and bulk endocytosis (Shupliakov et al., 2002; Holt et al., 2003). It likely generates local vertical force (against the PM) during membrane bending and fission or radial force (parallel the PM plane) at the fission neck (Walani et al., 2015; Hassinger et al., 2017). Endophilin may enhance the local membrane stress (Simunovic et al., 2017) via its curvature-sensing and curvature-generating properties (McMahon and Gallop, 2005). It has been shown to regulate clathrin-independent endocytosis in non-excitatory cells (Boucrot et al., 2015; Renard et al., 2015).
SV exocytosis-endocytosis coupling has a profound role in synaptic transmission, a process that is essential for neural circuit function and brain performance. Several factors emerge as sensors for endocytosis events, and we are only at an initial stage toward the mechanistic understanding of SV exocytosis-endocytosis coupling at synapses; even how SVs are born under physiological conditions remains a conundrum. The major challenge arises mainly from the complex biophysical features of exocytosis-endocytosis coupling at presynaptic terminals, such as the transient reaction, small structure, and poor accessibility. Despite these hurdles, the field is steadily moving forward with the application of new cutting-edge approaches spanning super-resolution fluorescence microscopy, single SV fusion detection, acute optogenetic manipulations (Ji et al., 2017), flash-freezing EM, and in-situ cryo-EM tomography. With advances in novel techniques and an increasing need to understand synaptic mechanisms, there has never been a better time to engage in investigating this fundamental process of brain function.
The author confirms being the sole contributor of this work and approved it for publication.
NIH R01DK093953, NIH R21NS101584, and research funding from the Medical College of Wisconsin.
The author declares that the research was conducted in the absence of any commercial or financial relationships that could be construed as a potential conflict of interest.
This work is supported by NIH grants and the funding from the Medical College of Wisconsin. I thank Dr. John Heuser (Washington University) for critical reading the manuscript.
Ackermann, F., Waites, C. L., and Garner, C. C. (2015). Presynaptic active zones in invertebrates and vertebrates. EMBO Rep. 16, 923–938. doi: 10.15252/embr.201540434
Alabi, A. A., and Tsien, R. W. (2013). Perspectives on kiss-and-run: role in exocytosis, endocytosis, and neurotransmission. Annu. Rev. Physiol. 75, 393–422. doi: 10.1146/annurev-physiol-020911-153305
Althof, D., Baehrens, D., Watanabe, M., Suzuki, N., Fakler, B., and Kulik, Á. (2015). Inhibitory and excitatory axon terminals share a common nano-architecture of their Cav2.1 (P/Q-type) Ca(2+) channels. Front. Cell. Neurosci. 9:315. doi: 10.3389/fncel.2015.00315
Anggono, V., Smillie, K. J., Graham, M. E., Valova, V. A., Cousin, M. A., and Robinson, P. J. (2006). Syndapin I is the phosphorylation-regulated dynamin I partner in synaptic vesicle endocytosis. Nat. Neurosci. 9, 752–760. doi: 10.1038/nn1695
Armbruster, M., Messa, M., Ferguson, S. M., De Camilli, P., and Ryan, T. A. (2013). Dynamin phosphorylation controls optimization of endocytosis for brief action potential bursts. Elife 2:e00845. doi: 10.7554/eLife.00845
Artalejo, C. R., Elhamdani, A., and Palfrey, H. C. (1996). Calmodulin is the divalent cation receptor for rapid endocytosis, but not exocytosis, in adrenal chromaffin cells. Neuron 16, 195–205. doi: 10.1016/S0896-6273(00)80036-7
Augustine, G. J., Morgan, J. R., Villalba-Galea, C. A., Jin, S., Prasad, K., and Lafer, E. M. (2006). Clathrin and synaptic vesicle endocytosis: studies at the squid giant synapse. Biochem. Soc. Trans. 34, 68–72. doi: 10.1042/BST0340068
Bai, J., Hu, Z., Dittman, J. S., Pym, E. C., and Kaplan, J. M. (2010). Endophilin functions as a membrane-bending molecule and is delivered to endocytic zones by exocytosis. Cell 143, 430–441. doi: 10.1016/j.cell.2010.09.024
Balaji, J., and Ryan, T. A. (2007). Single-vesicle imaging reveals that synaptic vesicle exocytosis and endocytosis are coupled by a single stochastic mode. Proc. Natl. Acad. Sci. U.S.A. 104, 20576–20581. doi: 10.1073/pnas.0707574105
Beutner, D., Voets, T., Neher, E., and Moser, T. (2001). Calcium dependence of exocytosis and endocytosis at the cochlear inner hair cell afferent synapse. Neuron 29, 681–690. doi: 10.1016/S0896-6273(01)00243-4
Bloom, O., Evergren, E., Tomilin, N., Kjaerulff, O., Löw, P., Brodin, L., et al. (2003). Colocalization of synapsin and actin during synaptic vesicle recycling. J. Cell Biol. 161, 737–747. doi: 10.1083/jcb.200212140
Bollmann, J. H., Sakmann, B., and Borst, J. G. (2000). Calcium sensitivity of glutamate release in a calyx-type terminal. Science 289, 953–957. doi: 10.1126/science.289.5481.953
Boucrot, E., Ferreira, A. P., Almeida-Souza, L., Debard, S., Vallis, Y., Howard, G., et al. (2015). Endophilin marks and controls a clathrin-independent endocytic pathway. Nature 517, 460–465. doi: 10.1038/nature14067
Ceccarelli, B., Hurlbut, W. P., and Mauro, A. (1972). Depletion of vesicles from frog neuromuscular junctions by prolonged tetanic stimulation. J. Cell Biol. 54, 30–38. doi: 10.1083/jcb.54.1.30
Chapman, E. R. (2008). How does synaptotagmin trigger neurotransmitter release? Annu. Rev. Biochem. 77, 615–641. doi: 10.1146/annurev.biochem.77.062005.101135
Cingolani, L. A., and Goda, Y. (2008). Actin in action: the interplay between the actin cytoskeleton and synaptic efficacy. Nat. Rev. Neurosci. 9, 344–356. doi: 10.1038/nrn2373
Clayton, E. L., Evans, G. J., and Cousin, M. A. (2008). Bulk synaptic vesicle endocytosis is rapidly triggered during strong stimulation. J. Neurosci. 28, 6627–6632. doi: 10.1523/JNEUROSCI.1445-08.2008
Cocucci, E., Aguet, F., Boulant, S., and Kirchhausen, T. (2012). The first five seconds in the life of a clathrin-coated pit. Cell 150, 495–507. doi: 10.1016/j.cell.2012.05.047
Cousin, M. A., and Robinson, P. J. (2001). The dephosphins: dephosphorylation by calcineurin triggers synaptic vesicle endocytosis. Trends Neurosci. 24, 659–665. doi: 10.1016/S0166-2236(00)01930-5
Deák, F., Schoch, S., Liu, X., Südhof, T. C., and Kavalali, E. T. (2004). Synaptobrevin is essential for fast synaptic-vesicle endocytosis. Nat. Cell Biol. 6, 1102–1108. doi: 10.1038/ncb1185
Delvendahl, I., Vyleta, N. P., von Gersdorff, H., and Hallermann, S. (2016). Fast, temperature-sensitive and clathrin-independent endocytosis at central synapses. Neuron 90, 492–498. doi: 10.1016/j.neuron.2016.03.013
Denker, A., Bethani, I., Kröhnert, K., Körber, C., Horstmann, H., Wilhelm, B. G., et al. (2011). A small pool of vesicles maintains synaptic activity in vivo. Proc. Natl. Acad. Sci. U.S.A. 108, 17177–17182. doi: 10.1073/pnas.1112688108
Dittman, J., and Ryan, T. A. (2009). Molecular circuitry of endocytosis at nerve terminals. Annu. Rev. Cell Dev. Biol. 25, 133–160. doi: 10.1146/annurev.cellbio.042308.113302
Doyon, J. B., Zeitler, B., Cheng, J., Cheng, A. T., Cherone, J. M., Santiago, Y., et al. (2011). Rapid and efficient clathrin-mediated endocytosis revealed in genome-edited mammalian cells. Nat. Cell Biol. 13, 331–337. doi: 10.1038/ncb2175
Duncker, S. V., Franz, C., Kuhn, S., Schulte, U., Campanelli, D., Brandt, N., et al. (2013). Otoferlin couples to clathrin-mediated endocytosis in mature cochlear inner hair cells. J. Neurosci. 33, 9508–9519. doi: 10.1523/JNEUROSCI.5689-12.2013
Eggermann, E., Bucurenciu, I., Goswami, S. P., and Jonas, P. (2011). Nanodomain coupling between Ca(2)(+) channels and sensors of exocytosis at fast mammalian synapses. Nat. Rev. Neurosci. 13, 7–21. doi: 10.1038/nrn3125
Evergren, E., Gad, H., Walther, K., Sundborger, A., Tomilin, N., and Shupliakov, O. (2007). Intersectin is a negative regulator of dynamin recruitment to the synaptic endocytic zone in the central synapse. J. Neurosci. 27, 379–390. doi: 10.1523/JNEUROSCI.4683-06.2007
Fan, F., Funk, L., and Lou, X. (2016). Dynamin 1- and 3-Mediated endocytosis is essential for the development of a large central synapse in vivo. J. Neurosci. 36, 6097–6115. doi: 10.1523/JNEUROSCI.3804-15.2016
Ferguson, S. M., Brasnjo, G., Hayashi, M., Wölfel, M., Collesi, C., Giovedi, S., et al. (2007). A selective activity-dependent requirement for dynamin 1 in synaptic vesicle endocytosis. Science 316, 570–574. doi: 10.1126/science.1140621
Fouquet, W., Owald, D., Wichmann, C., Mertel, S., Depner, H., Dyba, M., et al. (2009). Maturation of active zone assembly by Drosophila Bruchpilot. J. Cell Biol. 186, 129–145. doi: 10.1083/jcb.200812150
Gad, H., Löw, P., Zotova, E., Brodin, L., and Shupliakov, O. (1998). Dissociation between Ca2+-triggered synaptic vesicle exocytosis and clathrin-mediated endocytosis at a central synapse. Neuron 21, 607–616. doi: 10.1016/S0896-6273(00)80570-X
Gandhi, S. P., and Stevens, C. F. (2003). Three modes of synaptic vesicular recycling revealed by single-vesicle imaging. Nature 423, 607–613. doi: 10.1038/nature01677
Geppert, M., Goda, Y., Hammer, R. E., Li, C., Rosahl, T. W., Stevens, C. F., et al. (1994). Synaptotagmin I: a major Ca2+ sensor for transmitter release at a central synapse. Cell 79, 717–727. doi: 10.1016/0092-8674(94)90556-8
Gimber, N., Tadeus, G., Maritzen, T., Schmoranzer, J., and Haucke, V. (2015). Diffusional spread and confinement of newly exocytosed synaptic vesicle proteins. Nat. Commun. 6:8392. doi: 10.1038/ncomms9392
Gordon, S. L., Leube, R. E., and Cousin, M. A. (2011). Synaptophysin is required for synaptobrevin retrieval during synaptic vesicle endocytosis. J. Neurosci. 31, 14032–14036. doi: 10.1523/JNEUROSCI.3162-11.2011
Granseth, B., Odermatt, B., Royle, S. J., and Lagnado, L. (2006). Clathrin-mediated endocytosis is the dominant mechanism of vesicle retrieval at hippocampal synapses. Neuron 51, 773–786. doi: 10.1016/j.neuron.2006.08.029
Gu, M., Liu, Q., Watanabe, S., Sun, L., Hollopeter, G., Grant, B. D., et al. (2013). AP2 hemicomplexes contribute independently to synaptic vesicle endocytosis. Elife 2:e00190. doi: 10.7554/eLife.00190
Gundelfinger, E. D., and Fejtova, A. (2012). Molecular organization and plasticity of the cytomatrix at the active zone. Curr. Opin. Neurobiol. 22, 423–430. doi: 10.1016/j.conb.2011.10.005
Hallermann, S., Pawlu, C., Jonas, P., and Heckmann, M. (2003). A large pool of releasable vesicles in a cortical glutamatergic synapse. Proc. Natl. Acad. Sci. U.S.A. 100, 8975–8980. doi: 10.1073/pnas.1432836100
Han, Y., Kaeser, P. S., Südhof, T. C., and Schneggenburger, R. (2011). RIM determines Ca(2)+ channel density and vesicle docking at the presynaptic active zone. Neuron 69, 304–316. doi: 10.1016/j.neuron.2010.12.014
Hassinger, J. E., Oster, G., Drubin, D. G., and Rangamani, P. (2017). Design principles for robust vesiculation in clathrin-mediated endocytosis. Proc. Natl. Acad. Sci. U.S.A. 114, E1118–E1127. doi: 10.1073/pnas.1617705114
Haucke, V., and De Camilli, P. (1999). AP-2 recruitment to synaptotagmin stimulated by tyrosine-based endocytic motifs. Science 285, 1268–1271. doi: 10.1126/science.285.5431.1268
Haucke, V., Neher, E., and Sigrist, S. J. (2011). Protein scaffolds in the coupling of synaptic exocytosis and endocytosis. Nat. Rev. Neurosci. 12, 127–138. doi: 10.1038/nrn2948
Hayashi, M., Raimondi, A., O'Toole, E., Paradise, S., Collesi, C., Cremona, O., et al. (2008). Cell- and stimulus-dependent heterogeneity of synaptic vesicle endocytic recycling mechanisms revealed by studies of dynamin 1-null neurons. Proc. Natl. Acad. Sci. U.S.A. 105, 2175–2180. doi: 10.1073/pnas.0712171105
He, L., Wu, X. S., Mohan, R., and Wu, L. G. (2006). Two modes of fusion pore opening revealed by cell-attached recordings at a synapse. Nature 444, 102–105. doi: 10.1038/nature05250
Herman, M. A., and Rosenmund, C. (2015). On the brink: a new synaptic vesicle release model at the calyx of held. Neuron 85, 6–8. doi: 10.1016/j.neuron.2014.12.038
Heuser, J. E., and Reese, T. S. (1981). Structural changes after transmitter release at the frog neuromuscular junction. J. Cell Biol. 88, 564–580. doi: 10.1083/jcb.88.3.564
Heuser, J. E., and Reese, T. S. (1973). Evidence for recycling of synaptic vesicle membrane during transmitter release at the frog neuromuscular junction. J. Cell Biol. 57, 315–344. doi: 10.1083/jcb.57.2.315
Holderith, N., Lorincz, A., Katona, G., Rózsa, B., Kulik, A., Watanabe, M., et al. (2012). Release probability of hippocampal glutamatergic terminals scales with the size of the active zone. Nat. Neurosci. 15, 988–997. doi: 10.1038/nn.3137
Holt, M., Cooke, A., Wu, M. M., and Lagnado, L. (2003). Bulk membrane retrieval in the synaptic terminal of retinal bipolar cells. J. Neurosci. 23, 1329–1339.
Hosoi, N., Holt, M., and Sakaba, T. (2009). Calcium dependence of exo- and endocytotic coupling at a glutamatergic synapse. Neuron 63, 216–229. doi: 10.1016/j.neuron.2009.06.010
Hua, Y., Woehler, A., Kahms, M., Haucke, V., Neher, E., and Klingauf, J. (2013). Blocking endocytosis enhances short-term synaptic depression under conditions of normal availability of vesicles. Neuron 80, 343–349. doi: 10.1016/j.neuron.2013.08.010
Jahn, R., and Fasshauer, D. (2012). Molecular machines governing exocytosis of synaptic vesicles. Nature 490, 201–207. doi: 10.1038/nature11320
Ji, C., Fan, F., and Lou, X. (2017). Vesicle docking is a key target of local PI(4,5)P2 metabolism in the secretory pathway of INS-1 Cells. Cell Rep. 20, 1409–1421. doi: 10.1016/j.celrep.2017.07.041
Joensuu, M., Padmanabhan, P., Durisic, N., Bademosi, A. T., Cooper-Williams, E., Morrow, I. C., et al. (2016). Subdiffractional tracking of internalized molecules reveals heterogeneous motion states of synaptic vesicles. J. Cell Biol. 215, 277–292. doi: 10.1083/jcb.201604001
Jung, N., Wienisch, M., Gu, M., Rand, J. B., Müller, S. L., Krause, G., et al. (2007). Molecular basis of synaptic vesicle cargo recognition by the endocytic sorting adaptor stonin 2. J. Cell Biol. 179, 1497–1510. doi: 10.1083/jcb.200708107
Kaempf, N., Kochlamazashvili, G., Puchkov, D., Maritzen, T., Bajjalieh, S. M., Kononenko, N. L., et al. (2015). Overlapping functions of stonin 2 and SV2 in sorting of the calcium sensor synaptotagmin 1 to synaptic vesicles. Proc. Natl. Acad. Sci. USA. 112, 7297–7302. doi: 10.1073/pnas.1501627112
Kochubey, O., Lou, X., and Schneggenburger, R. (2011). Regulation of transmitter release by Ca(2+) and synaptotagmin: insights from a large CNS synapse. Trends Neurosci. 34, 237–246. doi: 10.1016/j.tins.2011.02.006
Koh, T. W., Verstreken, P., and Bellen, H. J. (2004). Dap160/intersectin acts as a stabilizing scaffold required for synaptic development and vesicle endocytosis. Neuron 43, 193–205. doi: 10.1016/j.neuron.2004.06.029
Kononenko, N. L., Puchkov, D., Classen, G. A., Walter, A. M., Pechstein, A., Sawade, L., et al. (2014). Clathrin/AP-2 mediate synaptic vesicle reformation from endosome-like vacuoles but are not essential for membrane retrieval at central synapses. Neuron 82, 981–988. doi: 10.1016/j.neuron.2014.05.007
Kwon, S. E., and Chapman, E. R. (2011). Synaptophysin regulates the kinetics of synaptic vesicle endocytosis in central neurons. Neuron 70, 847–854. doi: 10.1016/j.neuron.2011.04.001
Lee, J. S., Ho, W. K., and Lee, S. H. (2012). Actin-dependent rapid recruitment of reluctant synaptic vesicles into a fast-releasing vesicle pool. Proc. Natl. Acad. Sci. U.S.A. 109, E765–E774. doi: 10.1073/pnas.1114072109
Lee, J. S., Ho, W. K., Neher, E., and Lee, S. H. (2013). Superpriming of synaptic vesicles after their recruitment to the readily releasable pool. Proc. Natl. Acad. Sci. U.S.A. 110, 15079–15084. doi: 10.1073/pnas.1314427110
Leitz, J., and Kavalali, E. T. (2011). Ca(2)(+) influx slows single synaptic vesicle endocytosis. J. Neurosci. 31, 16318–16326. doi: 10.1523/JNEUROSCI.3358-11.2011
Leitz, J., and Kavalali, E. T. (2014). Fast retrieval and autonomous regulation of single spontaneously recycling synaptic vesicles. Elife 3:e03658. doi: 10.7554/eLife.03658
Leitz, J., and Kavalali, E. T. (2016). Ca2+ Dependence of synaptic vesicle endocytosis. Neuroscientist 22, 464–476. doi: 10.1177/1073858415588265
Li, Y. C., Chanaday, N. L., Xu, W., and Kavalali, E. T. (2017). Synaptotagmin-1- and Synaptotagmin-7-Dependent fusion mechanisms target synaptic vesicles to kinetically distinct endocytic pathways. Neuron 93, 616.e613–631.e613. doi: 10.1016/j.neuron.2016.12.010
Liang, K., Wei, L., and Chen, L. (2017). Exocytosis, endocytosis, and their coupling in excitable cells. Front. Mol. Neurosci. 10:109. doi: 10.3389/fnmol.2017.00109
Liu, Y. W., Neumann, S., Ramachandran, R., Ferguson, S. M., Pucadyil, T. J., and Schmid, S. L. (2011). Differential curvature sensing and generating activities of dynamin isoforms provide opportunities for tissue-specific regulation. Proc. Natl. Acad. Sci. U.S.A. 108, E234–E242. doi: 10.1073/pnas.1102710108
Llinás, R. R., Sugimori, M., Moran, K. A., Moreira, J. E., and Fukuda, M. (2004). Vesicular reuptake inhibition by a synaptotagmin I C2B domain antibody at the squid giant synapse. Proc. Natl. Acad. Sci. U.S.A. 101, 17855–17860. doi: 10.1073/pnas.0408200101
Llobet, A., Beaumont, V., and Lagnado, L. (2003). Real-time measurement of exocytosis and endocytosis using interference of light. Neuron 40, 1075–1086. doi: 10.1016/S0896-6273(03)00765-7
Lou, X., Paradise, S., Ferguson, S. M., and De Camilli, P. (2008). Selective saturation of slow endocytosis at a giant glutamatergic central synapse lacking dynamin 1. Proc. Natl. Acad. Sci. U.S.A. 105, 17555–17560. doi: 10.1073/pnas.0809621105
Lou, X., Scheuss, V., and Schneggenburger, R. (2005). Allosteric modulation of the presynaptic Ca2+ sensor for vesicle fusion. Nature 435, 497–501. doi: 10.1038/nature03568
Lu, J., Helton, T. D., Blanpied, T. A., Rácz, B., Newpher, T. M., Weinberg, R. J., et al. (2007). Postsynaptic positioning of endocytic zones and AMPA receptor cycling by physical coupling of dynamin-3 to Homer. Neuron 55, 874–889. doi: 10.1016/j.neuron.2007.06.041
Mahapatra, S., Fan, F., and Lou, X. (2016). Tissue-specific dynamin-1 deletion at the calyx of Held decreases short-term depression through a mechanism distinct from vesicle resupply. Proc. Natl. Acad. Sci. U.S.A. 113, E3150–E3158. doi: 10.1073/pnas.1520937113
Mahapatra, S., and Lou, X. (2017). Dynamin-1 deletion enhances post-tetanic potentiation and quantal size after tetanic stimulation at the calyx of Held. J. Physiol. 595, 193–206. doi: 10.1113/JP271937
Marie, B., Sweeney, S. T., Poskanzer, K. E., Roos, J., Kelly, R. B., and Davis, G. W. (2004). Dap160/intersectin scaffolds the periactive zone to achieve high-fidelity endocytosis and normal synaptic growth. Neuron 43, 207–219. doi: 10.1016/j.neuron.2004.07.001
Marks, B., and McMahon, H. T. (1998). Calcium triggers calcineurin-dependent synaptic vesicle recycling in mammalian nerve terminals. Curr. Biol. 8, 740–749. doi: 10.1016/S0960-9822(98)70297-0
McMahon, H. T., and Gallop, J. L. (2005). Membrane curvature and mechanisms of dynamic cell membrane remodelling. Nature 438, 590–596. doi: 10.1038/nature04396
Merrifield, C. J., Feldman, M. E., Wan, L., and Almers, W. (2002). Imaging actin and dynamin recruitment during invagination of single clathrin-coated pits. Nat. Cell Biol. 4, 691–698. doi: 10.1038/ncb837
Miller, T. M., and Heuser, J. E. (1984). Endocytosis of synaptic vesicle membrane at the frog neuromuscular junction. J. Cell Biol. 98, 685–698. doi: 10.1083/jcb.98.2.685
Milosevic, I., Giovedi, S., Lou, X., Raimondi, A., Collesi, C., Shen, H., et al. (2011). Recruitment of endophilin to clathrin-coated pit necks is required for efficient vesicle uncoating after fission. Neuron 72, 587–601. doi: 10.1016/j.neuron.2011.08.029
Morgan, J. R., Di Paolo, G., Werner, H., Shchedrina, V. A., Pypaert, M., Pieribone, V. A., et al. (2004). A role for talin in presynaptic function. J. Cell Biol. 167, 43–50. doi: 10.1083/jcb.200406020
Morgan, J. R., Prasad, K., Hao, W., Augustine, G. J., and Lafer, E. M. (2000). A conserved clathrin assembly motif essential for synaptic vesicle endocytosis. J. Neurosci. 20, 8667–8676.
Morgan, J. R., Zhao, X., Womack, M., Prasad, K., Augustine, G. J., and Lafer, E. M. (1999). A role for the clathrin assembly domain of AP180 in synaptic vesicle endocytosis. J. Neurosci. 19, 10201–10212.
Moser, T., and Beutner, D. (2000). Kinetics of exocytosis and endocytosis at the cochlear inner hair cell afferent synapse of the mouse. Proc. Natl. Acad. Sci. U.S.A. 97, 883–888. doi: 10.1073/pnas.97.2.883
Myers, M. D., Ryazantsev, S., Hicke, L., and Payne, G. S. (2016). Calmodulin promotes N-BAR domain-mediated membrane constriction and endocytosis. Dev. Cell 37, 162–173. doi: 10.1016/j.devcel.2016.03.012
Nakamura, Y., Harada, H., Kamasawa, N., Matsui, K., Rothman, J. S., Shigemoto, R., et al. (2015). Nanoscale distribution of presynaptic Ca(2+) channels and its impact on vesicular release during development. Neuron 85, 145–158. doi: 10.1016/j.neuron.2014.11.019
Neher, E. (1998). Vesicle pools and Ca2+ microdomains: new tools for understanding their roles in neurotransmitter release. Neuron 20, 389–399. doi: 10.1016/S0896-6273(00)80983-6
Neher, E. (2010). What is Rate-Limiting during sustained synaptic activity: vesicle supply or the availability of release sites. Front. Synaptic Neurosci. 2:144. doi: 10.3389/fnsyn.2010.00144
Neher, E. (2015). Merits and limitations of vesicle pool models in view of heterogeneous populations of synaptic vesicles. Neuron 87, 1131–1142. doi: 10.1016/j.neuron.2015.08.038
Neher, E. (2018). Neurosecretion: what can we learn from chromaffin cells. Pflugers Arch. 470, 7–11. doi: 10.1007/s00424-017-2051-6
Neher, E., and Marty, A. (1982). Discrete changes of cell membrane capacitance observed under conditions of enhanced secretion in bovine adrenal chromaffin cells. Proc. Natl. Acad. Sci. U.S.A. 79, 6712–6716. doi: 10.1073/pnas.79.21.6712
Neher, E., and Sakaba, T. (2008). Multiple roles of calcium ions in the regulation of neurotransmitter release. Neuron 59, 861–872. doi: 10.1016/j.neuron.2008.08.019
Neves, G., and Lagnado, L. (1999). The kinetics of exocytosis and endocytosis in the synaptic terminal of goldfish retinal bipolar cells. J. Physiol. 515(Pt 1), 181–202. doi: 10.1111/j.1469-7793.1999.181ad.x
Nicholson-Fish, J. C., Kokotos, A. C., Gillingwater, T. H., Smillie, K. J., and Cousin, M. A. (2015). VAMP4 Is an Essential Cargo Molecule for Activity-Dependent bulk endocytosis. Neuron 88, 973–984. doi: 10.1016/j.neuron.2015.10.043
Nicholson-Tomishima, K., and Ryan, T. A. (2004). Kinetic efficiency of endocytosis at mammalian CNS synapses requires synaptotagmin I. Proc. Natl. Acad. Sci. U.S.A. 101, 16648–16652. doi: 10.1073/pnas.0406968101
Pan, P. Y., Marrs, J., and Ryan, T. A. (2015). Vesicular glutamate transporter 1 orchestrates recruitment of other synaptic vesicle cargo proteins during synaptic vesicle recycling. J. Biol. Chem. 290, 22593–22601. doi: 10.1074/jbc.M115.651711
Park, R. J., Shen, H., Liu, L., Liu, X., Ferguson, S. M., and De Camilli, P. (2013). Dynamin triple knockout cells reveal off target effects of commonly used dynamin inhibitors. J. Cell Sci. 126, 5305–5312. doi: 10.1242/jcs.138578
Pechstein, A., Shupliakov, O., and Haucke, V. (2010). Intersectin 1: a versatile actor in the synaptic vesicle cycle. Biochem. Soc. Trans. 38, 181–186. doi: 10.1042/BST0380181
Poskanzer, K. E., Fetter, R. D., and Davis, G. W. (2006). Discrete residues in the c(2)b domain of synaptotagmin I independently specify endocytic rate and synaptic vesicle size. Neuron 50, 49–62. doi: 10.1016/j.neuron.2006.02.021
Poskanzer, K. E., Marek, K. W., Sweeney, S. T., and Davis, G. W. (2003). Synaptotagmin I is necessary for compensatory synaptic vesicle endocytosis in vivo. Nature 426, 559–563. doi: 10.1038/nature02184
Raimondi, A., Ferguson, S. M., Lou, X., Armbruster, M., Paradise, S., Giovedi, S., et al. (2011). Overlapping role of dynamin isoforms in synaptic vesicle endocytosis. Neuron 70, 1100–1114. doi: 10.1016/j.neuron.2011.04.031
Rajappa, R., Gauthier-Kemper, A., Böning, D., Hüve, J., and Klingauf, J. (2016). Synaptophysin 1 clears synaptobrevin 2 from the presynaptic active zone to prevent short-term depression. Cell Rep. 14, 1369–1381. doi: 10.1016/j.celrep.2016.01.031
Ramadurai, S., Holt, A., Krasnikov, V., van den Bogaart, G., Killian, J. A., and Poolman, B. (2009). Lateral diffusion of membrane proteins. J. Am. Chem. Soc. 131, 12650–12656. doi: 10.1021/ja902853g
Renard, H. F., Simunovic, M., Lemière, J., Boucrot, E., Garcia-Castillo, M. D., Arumugam, S., et al. (2015). Endophilin-A2 functions in membrane scission in clathrin-independent endocytosis. Nature 517, 493–496. doi: 10.1038/nature14064
Renden, R., and von Gersdorff, H. (2007). Synaptic vesicle endocytosis at a CNS nerve terminal: faster kinetics at physiological temperatures and increased endocytotic capacity during maturation. J. Neurophysiol. 98, 3349–3359. doi: 10.1152/jn.00898.2007
Richards, D. A., Rizzoli, S. O., and Betz, W. J. (2004). Effects of wortmannin and latrunculin A on slow endocytosis at the frog neuromuscular junction. J. Physiol. 557, 77–91. doi: 10.1113/jphysiol.2004.062158
Rizzoli, S. O., and Betz, W. J. (2005). Synaptic vesicle pools. Nat. Rev. Neurosci. 6, 57–69. doi: 10.1038/nrn1583
Roos, J., and Kelly, R. B. (1998). Dap160, a neural-specific Eps15 homology and multiple SH3 domain-containing protein that interacts with Drosophila dynamin. J. Biol. Chem. 273, 19108–19119. doi: 10.1074/jbc.273.30.19108
Roos, J., and Kelly, R. B. (1999). The endocytic machinery in nerve terminals surrounds sites of exocytosis. Curr. Biol. 9, 1411–1414. doi: 10.1016/S0960-9822(00)80087-1
Saheki, Y., and De Camilli, P. (2012). Synaptic vesicle endocytosis. Cold Spring Harb. Perspect. Biol. 4:a005645. doi: 10.1101/cshperspect.a005645
Sakaba, T., Kononenko, N. L., Bacetic, J., Pechstein, A., Schmoranzer, J., Yao, L., et al. (2013). Fast neurotransmitter release regulated by the endocytic scaffold intersectin. Proc. Natl. Acad. Sci. U.S.A. 110, 8266–8271. doi: 10.1073/pnas.1219234110
Sakaba, T., and Neher, E. (2003). Involvement of actin polymerization in vesicle recruitment at the calyx of Held synapse. J. Neurosci. 23, 837–846.
Sankaranarayanan, S., Atluri, P. P., and Ryan, T. A. (2003). Actin has a molecular scaffolding, not propulsive, role in presynaptic function. Nat. Neurosci. 6, 127–135. doi: 10.1038/nn1002
Sankaranarayanan, S., and Ryan, T. A. (2000). Real-time measurements of vesicle-SNARE recycling in synapses of the central nervous system. Nat. Cell Biol. 2, 197–204. doi: 10.1038/35008615
Sato, K., Ernstrom, G. G., Watanabe, S., Weimer, R. M., Chen, C. H., Sato, M., et al. (2009). Differential requirements for clathrin in receptor-mediated endocytosis and maintenance of synaptic vesicle pools. Proc. Natl. Acad. Sci. U.S.A. 106, 1139–1144. doi: 10.1073/pnas.0809541106
Schneggenburger, R., Han, Y., and Kochubey, O. (2012). Ca(2+) channels and transmitter release at the active zone. Cell Calcium 52, 199–207. doi: 10.1016/j.ceca.2012.04.011
Schneggenburger, R., and Neher, E. (2000). Intracellular calcium dependence of transmitter release rates at a fast central synapse. Nature 406, 889–893. doi: 10.1038/35022702
Schneggenburger, R., and Rosenmund, C. (2015). Molecular mechanisms governing Ca(2+) regulation of evoked and spontaneous release. Nat. Neurosci. 18, 935–941. doi: 10.1038/nn.4044
Shupliakov, O., Bloom, O., Gustafsson, J. S., Kjaerulff, O., Low, P., Tomilin, N., et al. (2002). Impaired recycling of synaptic vesicles after acute perturbation of the presynaptic actin cytoskeleton. Proc. Natl. Acad. Sci. U.S.A. 99, 14476–14481. doi: 10.1073/pnas.212381799
Simunovic, M., Manneville, J. B., Renard, H. F., Evergren, E., Raghunathan, K., Bhatia, D., et al. (2017). Friction mediates scission of tubular membranes scaffolded by BAR proteins. Cell 170, 172.e111–184.e111. doi: 10.1016/j.cell.2017.05.047
Smith, R. M., Baibakov, B., Ikebuchi, Y., White, B. H., Lambert, N. A., Kaczmarek, L. K., et al. (2000). Exocytotic insertion of calcium channels constrains compensatory endocytosis to sites of exocytosis. J. Cell Biol. 148, 755–767. doi: 10.1083/jcb.148.4.755
Soykan, T., Kaempf, N., Sakaba, T., Vollweiter, D., Goerdeler, F., Puchkov, D., et al. (2017). Synaptic vesicle endocytosis occurs on multiple timescales and is mediated by formin-dependent actin assembly. Neuron 93, 854–866.e854. doi: 10.1016/j.neuron.2017.02.011
Soykan, T., Maritzen, T., and Haucke, V. (2016). Modes and mechanisms of synaptic vesicle recycling. Curr. Opin. Neurobiol. 39, 17–23. doi: 10.1016/j.conb.2016.03.005
Südhof, T. C. (2012). The presynaptic active zone. Neuron 75, 11–25. doi: 10.1016/j.neuron.2012.06.012
Südhof, T. C. (2013). Neurotransmitter release: the last millisecond in the life of a synaptic vesicle. Neuron 80, 675–690. doi: 10.1016/j.neuron.2013.10.022
Sun, J. Y., and Wu, L. G. (2001). Fast kinetics of exocytosis revealed by simultaneous measurements of presynaptic capacitance and postsynaptic currents at a central synapse. Neuron 30, 171–182. doi: 10.1016/S0896-6273(01)00271-9
Sun, T., Wu, X. S., Xu, J., McNeil, B. D., Pang, Z. P., Yang, W., et al. (2010). The role of calcium/calmodulin-activated calcineurin in rapid and slow endocytosis at central synapses. J. Neurosci. 30, 11838–11847. doi: 10.1523/JNEUROSCI.1481-10.2010
Takamori, S., Holt, M., Stenius, K., Lemke, E. A., Grønborg, M., Riedel, D., et al. (2006). Molecular anatomy of a trafficking organelle. Cell 127, 831–846. doi: 10.1016/j.cell.2006.10.030
Tan, T. C., Valova, V. A., Malladi, C. S., Graham, M. E., Berven, L. A., Jupp, O. J., et al. (2003). Cdk5 is essential for synaptic vesicle endocytosis. Nat. Cell Biol. 5, 701–710. doi: 10.1038/ncb1020
Taylor, M. J., Perrais, D., and Merrifield, C. J. (2011). A high precision survey of the molecular dynamics of mammalian clathrin-mediated endocytosis. PLoS Biol. 9:e1000604. doi: 10.1371/journal.pbio.1000604
Voglmaier, S. M., Kam, K., Yang, H., Fortin, D. L., Hua, Z., Nicoll, R. A., et al. (2006). Distinct endocytic pathways control the rate and extent of synaptic vesicle protein recycling. Neuron 51, 71–84. doi: 10.1016/j.neuron.2006.05.027
von Gersdorff, H., and Matthews, G. (1994). Dynamics of synaptic vesicle fusion and membrane retrieval in synaptic terminals. Nature 367, 735–739. doi: 10.1038/367735a0
Wahl, S., Katiyar, R., and Schmitz, F. (2013). A local, periactive zone endocytic machinery at photoreceptor synapses in close vicinity to synaptic ribbons. J. Neurosci. 33, 10278–10300. doi: 10.1523/JNEUROSCI.5048-12.2013
Walani, N., Torres, J., and Agrawal, A. (2015). Endocytic proteins drive vesicle growth via instability in high membrane tension environment. Proc. Natl. Acad. Sci. U.S.A. 112, E1423–E1432. doi: 10.1073/pnas.1418491112
Wang, S. S. H., Held, R. G., Wong, M. Y., Liu, C., Karakhanyan, A., and Kaeser, P. S. (2016). Fusion competent synaptic vesicles persist upon active zone disruption and loss of vesicle docking. Neuron 91, 777–791. doi: 10.1016/j.neuron.2016.07.005
Watanabe, H., Yamashita, T., Saitoh, N., Kiyonaka, S., Iwamatsu, A., Campbell, K. P., et al. (2010). Involvement of Ca2+ channel synprint site in synaptic vesicle endocytosis. J. Neurosci. 30, 655–660. doi: 10.1523/JNEUROSCI.3214-09.2010
Watanabe, S., Liu, Q., Davis, M. W., Hollopeter, G., Thomas, N., Jorgensen, N. B., et al. (2013a). Ultrafast endocytosis at Caenorhabditis elegans neuromuscular junctions. Elife 2:e00723. doi: 10.7554/eLife.00723
Watanabe, S., and Boucrot, E. (2017). Fast and ultrafast endocytosis. Curr. Opin. Cell Biol. 47, 64–71. doi: 10.1016/j.ceb.2017.02.013
Watanabe, S., Rost, B. R., Camacho-Pérez, M., Davis, M. W., Söhl-Kielczynski, B., Rosenmund, C., et al. (2013b). Ultrafast endocytosis at mouse hippocampal synapses. Nature 504, 242–247. doi: 10.1038/nature12809
Watanabe, S., Trimbuch, T., Camacho-Pérez, M., Rost, B. R., Brokowski, B., Söhl-Kielczynski, B., et al. (2014). Clathrin regenerates synaptic vesicles from endosomes. Nature 515, 228–233. doi: 10.1038/nature13846
Wienisch, M., and Klingauf, J. (2006). Vesicular proteins exocytosed and subsequently retrieved by compensatory endocytosis are nonidentical. Nat. Neurosci. 9, 1019–1027. doi: 10.1038/nn1739
Willox, A. K., and Royle, S. J. (2012). Stonin 2 is a major adaptor protein for clathrin-mediated synaptic vesicle retrieval. Curr. Biol. 22, 1435–1439. doi: 10.1016/j.cub.2012.05.048
Wu, L. G., Hamid, E., Shin, W., and Chiang, H. C. (2014). Exocytosis and endocytosis: modes, functions, and coupling mechanisms. Annu. Rev. Physiol. 76, 301–331. doi: 10.1146/annurev-physiol-021113-170305
Wu, W., and Wu, L. G. (2007). Rapid bulk endocytosis and its kinetics of fission pore closure at a central synapse. Proc. Natl. Acad. Sci. U.S.A. 104, 10234–10239. doi: 10.1073/pnas.0611512104
Wu, W., Xu, J., Wu, X. S., and Wu, L. G. (2005). Activity-dependent acceleration of endocytosis at a central synapse. J. Neurosci. 25, 11676–11683. doi: 10.1523/JNEUROSCI.2972-05.2005
Wu, X. S., Lee, S. H., Sheng, J., Zhang, Z., Zhao, W. D., Wang, D., et al. (2016). Actin is crucial for all kinetically distinguishable forms of endocytosis at synapses. Neuron 92, 1020–1035. doi: 10.1016/j.neuron.2016.10.014
Wu, X. S., McNeil, B. D., Xu, J., Fan, J., Xue, L., Melicoff, E., et al. (2009). Ca(2+) and calmodulin initiate all forms of endocytosis during depolarization at a nerve terminal. Nat. Neurosci. 12, 1003–1010. doi: 10.1038/nn.2355
Wu, X. S., and Wu, L. G. (2014). The yin and yang of calcium effects on synaptic vesicle endocytosis. J. Neurosci. 34, 2652–2659. doi: 10.1523/JNEUROSCI.3582-13.2014
Wu, X. S., Zhang, Z., Zhao, W. D., Wang, D., Luo, F., and Wu, L. G. (2014). Calcineurin is universally involved in vesicle endocytosis at neuronal and nonneuronal secretory cells. Cell Rep. 7, 982–988. doi: 10.1016/j.celrep.2014.04.020
Wu, Y., O'Toole, E. T., Girard, M., Ritter, B., Messa, M., Liu, X., et al. (2014). A dynamin 1-, dynamin 3- and clathrin-independent pathway of synaptic vesicle recycling mediated by bulk endocytosis. Elife 3:e01621. doi: 10.7554/eLife.01621
Xu, J., Luo, F., Zhang, Z., Xue, L., Wu, X. S., Chiang, H. C., et al. (2013). SNARE proteins synaptobrevin, SNAP-25, and syntaxin are involved in rapid and slow endocytosis at synapses. Cell Rep. 3, 1414–1421. doi: 10.1016/j.celrep.2013.03.010
Xue, L., Zhang, Z., McNeil, B. D., Luo, F., Wu, X.-S., Sheng, J., et al. (2012). Voltage-dependent calcium channels at the plasma membrane, but not vesicular channels, couple exocytosis to endocytosis. Cell Rep. 1, 632–638. doi: 10.1016/j.celrep.2012.04.011
Yamashita, T., Eguchi, K., Saitoh, N., von Gersdorff, H., and Takahashi, T. (2010). Developmental shift to a mechanism of synaptic vesicle endocytosis requiring nanodomain Ca2+. Nat. Neurosci. 13, 838–844. doi: 10.1038/nn.2576
Yamashita, T., Hige, T., and Takahashi, T. (2005). Vesicle endocytosis requires dynamin-dependent GTP hydrolysis at a fast CNS synapse. Science 307, 124–127. doi: 10.1126/science.1103631
Yao, C. K., Lin, Y. Q., Ly, C. V., Ohyama, T., Haueter, C. M., Moiseenkova-Bell, V. Y., et al. (2009). A synaptic vesicle-associated Ca2+ channel promotes endocytosis and couples exocytosis to endocytosis. Cell 138, 947–960. doi: 10.1016/j.cell.2009.06.033
Yao, C. K., Liu, Y. T., Lee, I. C., Wang, Y. T., and Wu, P. Y. (2017). A Ca2+ channel differentially regulates Clathrin-mediated and activity-dependent bulk endocytosis. PLoS Biol. 15:e2000931. doi: 10.1371/journal.pbio.2000931
Yao, J., Kwon, S. E., Gaffaney, J. D., Dunning, F. M., and Chapman, E. R. (2011). Uncoupling the roles of synaptotagmin I during endo- and exocytosis of synaptic vesicles. Nat. Neurosci. 15, 243–249. doi: 10.1038/nn.3013
Yao, L. H., Rao, Y., Varga, K., Wang, C. Y., Xiao, P., Lindau, M., et al. (2012). Synaptotagmin 1 is necessary for the Ca2+ dependence of clathrin-mediated endocytosis. J. Neurosci. 32, 3778–3785. doi: 10.1523/JNEUROSCI.3540-11.2012
Yao, L., and Sakaba, T. (2012). Activity-dependent modulation of endocytosis by calmodulin at a large central synapse. Proc. Natl. Acad. Sci. U.S.A. 109, 291–296. doi: 10.1073/pnas.1100608109
Yu, Y., Chu, P. Y., Bowser, D. N., Keating, D. J., Dubach, D., Harper, I., et al. (2008). Mice deficient for the chromosome 21 ortholog Itsn1 exhibit vesicle-trafficking abnormalities. Hum. Mol. Genet. 17, 3281–3290. doi: 10.1093/hmg/ddn224
Zhang, Q., Li, Y., and Tsien, R. W. (2009). The dynamic control of kiss-and-run and vesicular reuse probed with single nanoparticles. Science 323, 1448–1453. doi: 10.1126/science.1167373
Zhang, Z., Wang, D., Sun, T., Xu, J., Chiang, H. C., Shin, W., et al. (2013). The SNARE proteins SNAP25 and synaptobrevin are involved in endocytosis at hippocampal synapses. J. Neurosci. 33, 9169–9175. doi: 10.1523/JNEUROSCI.0301-13.2013
Keywords: exocytosis-endocytosis coupling, active zone, endocytic zone, sensors, dynamin, membrane tension
Citation: Lou X (2018) Sensing Exocytosis and Triggering Endocytosis at Synapses: Synaptic Vesicle Exocytosis–Endocytosis Coupling. Front. Cell. Neurosci. 12:66. doi: 10.3389/fncel.2018.00066
Received: 30 November 2017; Accepted: 26 February 2018;
Published: 14 March 2018.
Edited by:
Shigeki Watanabe, Johns Hopkins University, United StatesReviewed by:
Jennifer R. Morgan, Marine Biological Laboratory (MBL), United StatesCopyright © 2018 Lou. This is an open-access article distributed under the terms of the Creative Commons Attribution License (CC BY). The use, distribution or reproduction in other forums is permitted, provided the original author(s) and the copyright owner are credited and that the original publication in this journal is cited, in accordance with accepted academic practice. No use, distribution or reproduction is permitted which does not comply with these terms.
*Correspondence: Xuelin Lou, eHVsb3VAbWN3LmVkdQ==
Disclaimer: All claims expressed in this article are solely those of the authors and do not necessarily represent those of their affiliated organizations, or those of the publisher, the editors and the reviewers. Any product that may be evaluated in this article or claim that may be made by its manufacturer is not guaranteed or endorsed by the publisher.
Research integrity at Frontiers
Learn more about the work of our research integrity team to safeguard the quality of each article we publish.