- Institute of Physiology, University Medical Center of the Johannes Gutenberg University Mainz, Mainz, Germany
Animal and human studies revealed that patterned neuronal activity is an inherent feature of developing nervous systems. This review summarizes our current knowledge about the mechanisms generating early electrical activity patterns and their impact on structural and functional development of the cerebral cortex. All neocortical areas display distinct spontaneous and sensory-driven neuronal activity patterns already at early phases of development. At embryonic stages, intermittent spontaneous activity is synchronized within small neuronal networks, becoming more complex with further development. This transition is accompanied by a gradual shift from electrical to chemical synaptic transmission, with a particular role of non-synaptic tonic currents before the onset of phasic synaptic activity. In this review article we first describe functional impacts of classical neurotransmitters (GABA, glutamate) and modulatory systems (e.g., acetylcholine, ACh) on early neuronal activities in the neocortex with special emphasis on electrical synapses, nonsynaptic and synaptic currents. Early neuronal activity influences probably all developmental processes and is crucial for the proper formation of neuronal circuits. In the second part of our review, we illustrate how specific activity patterns might interfere with distinct neurodevelopmental processes like proliferation, migration, axonal and dendritic sprouting, synapse formation and neurotransmitter specification. Finally, we present evidence that transient alterations in neuronal activity during restricted perinatal periods can lead to persistent changes in functional connectivity and therefore might underlie the manifestation of neurological and neuropsychiatric diseases.
Introduction
Animal and human studies have demonstrated that patterned neuronal activity is an inherent feature of developing neuronal networks. Spontaneous activity patterns demonstrate specific properties during distinct developmental stages, in accordance with the establishment of neuronal connectivity (for review Egorov and Draguhn, 2013; Yang et al., 2016). While at embryonic stages intermittent spontaneous activity is synchronized within small neuronal networks, activity patterns become more complex during further development of the cerebral cortex, depending on the maturational state of network connectivity. Experimental evidence, clinical findings and theoretical considerations demonstrated the importance of neuronal activity for the structural and functional maturation of the neocortex (Thivierge, 2009; Ben-Ari and Spitzer, 2010; Kirkby et al., 2013; Rahkonen et al., 2013). This review aims to summarize our current knowledge about the mechanisms by which specific electrical activity patterns can influence or control structural and functional development. For this purpose, we first describe the development of synaptic transmission and its role in the generation of early neuronal activity patterns in the neocortex. Based on this information, we subsequently summarize how neuronal activity interferes with distinct neurodevelopmental events. Finally, we present evidence that transient alterations in neuronal activity during restricted perinatal periods can lead to persistent changes in functional connectivity and might thereby underlie a variety of neurological and neuropsychiatric disorders.
Sequence of Neocortical Developmental Events
Genesis of the Neocortex
Since the developing cerebral cortex shows prominent molecular, anatomical and physiological changes during embryonic and early postnatal stages, we first briefly describe neocortical development and the transient structures occurring during this period (Figure 1). In the mammalian neocortical anlage, the earliest generations of postmitotic cells are produced in the dorsal neuroepithelium. These cells migrate towards the outer surface of the pallium and form the primordial plexiform layer (PPL) or preplate (Bystron et al., 2008), which also contains a dense network of monoaminergic and GABAergic fibers (Levitt and Moore, 1979; Dammerman et al., 2000). Subsequently born glutamatergic (mostly pyramidal) neurons are generated in the ventricular (VZ) and subventricular zone (SVZ), germinal layers adjacent to the ventricular surface. Through radial migration these cells form the cortical plate (CP; in rodents at embryonic day [E] 14–17), which divides the preplate into a superficial marginal zone (MZ) and a deeper subplate (SP; for review Frotscher, 1998; Marín-Padilla, 1998). Later born neurons invade the CP and terminate at the upper border of the CP, establishing the inside-first outside-last pattern in neocortical development. Cajal-Retzius cells, a specific population of neurons located in the MZ, are essential for the establishment of this inside-first outside-last pattern (for review Frotscher, 1998; Kirischuk et al., 2014; Kilb and Frotscher, 2016). Early born neurons in the SP are at the same time elementary for the development of adequate thalamocortical connections and the formation of functional cortical columns (for review Luhmann, 2003; Luhmann et al., 2009; Kanold and Luhmann, 2010). Genetic programs control many early developmental events, but neurotransmitters, released constitutively or via activity-dependent processes, are also involved in these events at surprisingly early stages of corticogenesis (for review Owens and Kriegstein, 2002; Luján et al., 2005). Therefore, we will next summarize the maturation of the main neurotransmitter systems during early development of the cortex.
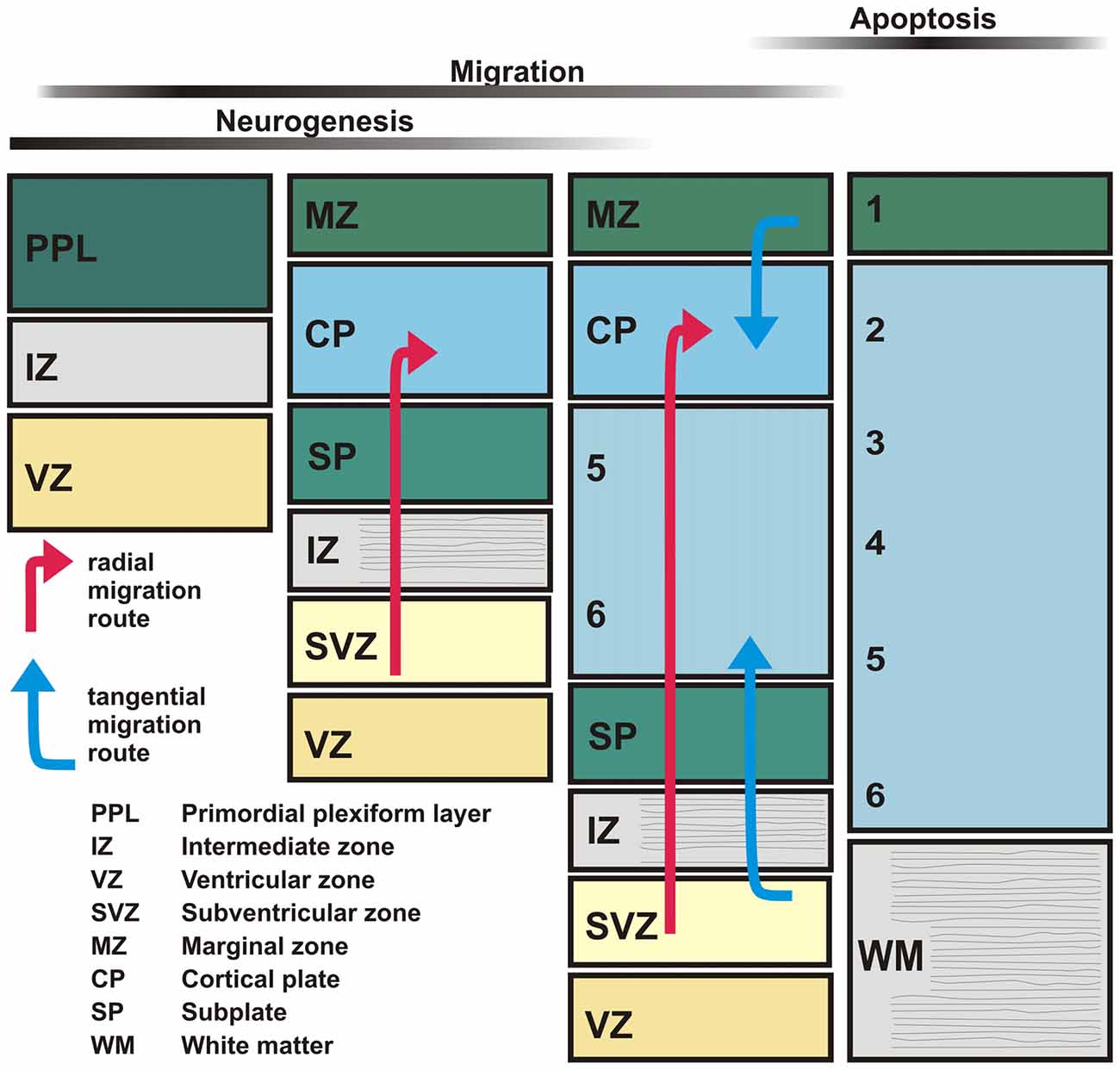
Figure 1. Schematic diagram illustrating the major stages of neocortical development. The earliest-generated neocortical neurons, including Cajal-Retzius and subplate (SP) neurons, form the primordial plexiform layer (PPL). Later-generated neurons generated in the ventricular (VZ) and subventricular zones (SVZ) split the PPL into the marginal zone (MZ) and the SP layer thereby forming the cortical plate (CP). Subsequently newly-generated glutamatergic neurons migrate radially (red arrows) towards the MZ and settle in the CP, thus establishing the inside first-outside last pattern of the developing neocortex. GABAergic interneurons are generated in the ganglionic eminences and reach the neocortex by tangential migration (blue arrows).
Maturation of Neurotransmitter Systems
GABA
GABA is the major inhibitory neurotransmitter in the adult brain and influences neuronal activity and development at prenatal and postnatal stages (for review Ben-Ari et al., 2007; Kilb, 2011; Luhmann et al., 2014). In rodents, the GABA concentration in the cortical anlage at E15 and birth amounts to about 20% and 60% of adult levels, respectively. High levels of GABA in the cerebral anlage during prenatal development can be explained by the fact that the GABA transaminase, an enzyme catalyzing the degradation of GABA, is only weakly expressed around birth (Van Eden et al., 1989). GABAergic cells have been found in the plexiform primordium already at E14. At E16 GABAergic cells are found in all fetal zones of the cerebral anlage but most of them are located in the MZ and the SP, while at E21 GABAergic cells are also present in the CP.
The expression of vesicular GABA transporters is first observed around birth (Minelli et al., 2003), but evidence for GABAergic synaptic transmission has been reported already in embryonic neocortical neurons (Owens et al., 1999; Kilb et al., 2004). However, other pathways, like volume-sensitive chloride channels (Kimelberg et al., 1990), or reversely operating GABA transporters (Richerson and Wu, 2003) can mediate GABA release, leading to non-synaptic tonic GABAergic activation (for review Kilb et al., 2013). Expression of the neuronal GABA transporter isoform GAT-1, as well the typically glial located isoform GAT-3 has been found in the perinatal rodent brain (Minelli et al., 2003), but during development the expression of these transporters is not restricted to the respective cell type. As GAT reversal potential is suggested to be close to the membrane resting potential (Wu et al., 2006), GAT-mediated GABA release can be induced by neuronal activities either directly from neurons (Wu et al., 2007; but see Savtchenko et al., 2015) or indirectly (by K+ elevation or glutamate uptake) from astrocytes (Unichenko et al., 2012).
The expression of GABAA receptors at very early developmental stages was already demonstrated 25 years ago, when GABAA receptor subunits α3, α4, α5, β2/3 and γ2 were shown to be expressed in the neocortical anlage of E17 rat fetuses (Laurie et al., 1992; for review Luján et al., 2005). Developmental changes in GABA receptor subunits were later confirmed by more recent molecular approaches (e.g., Yu et al., 2006), however these data were limited to pre- and early perinatal stages. Functional GABAA receptors have been described on neuronal precursor cells in the neocortical proliferative zone at E16 (LoTurco et al., 1995). At E18 GABAA receptors, identified by benzodiazepine-binding, are mainly located in the MZ and the SP, while around birth GABA receptors are expressed throughout the CP with an inside-out density gradient (Schlumpf et al., 1983; for review Galanopoulou, 2008). In contrast, functional GABAC receptors have been observed in the neocortex only on putative migrating neurons in the intermediate zone (Denter et al., 2010). As ionotropic GABAA/C receptors are directly linked to a chloride conductance and the intracellular chloride concentration is shifted to higher values during development (Yamada et al., 2004), these receptors mediate a depolarizing, and sometimes excitatory action during prenatal and early postnatal development (Ben-Ari et al., 1989; Leinekugel et al., 1997; for review Ben-Ari et al., 2012). Recent experiments demonstrated depolarizing, but inhibitory effects of GABAA receptors in the developing neocortex under in vivo conditions under anesthesia (Kirmse et al., 2015) and that photostimulation of GABAergic neurons in vivo attenuates neuronal activity in the neocortex and hippocampus of immature mice (Valeeva et al., 2016). However, further experimental studies are required in different neuronal populations, under non-anesthetized conditions and with physiological monosynaptic GABAergic activation to understand the role of GABA action in developing cortex (for discussion of this issue see Ben-Ari, 2015). In addition to the ionotropic GABAA/C receptors, metabotropic GABAB receptors have been found in the neocortex at E14 and are expressed in Cajal-Retzius as well as in SP neurons (López-Bendito et al., 2002).
Glutamate
Glutamate, the major excitatory neurotransmitter in the adult mammalian brain mediates important trophic roles in development including proliferation, migration and neuronal survival (for review Lipton and Kater, 1989; Owens and Kriegstein, 2002). The expression of glutaminase, the main enzyme required for glutamate synthesis, shows a rapid increase at E17 soon after the glutamatergic projection neurons enter the CP in rodents (Larsson et al., 1985, compare to http://developingmouse.brain-map.org/gene/show/14436). Vesicular glutamate transporters, which are essential elements for synaptic glutamate release, are expressed in the neocortical anlage of rodents already at E14 (Schuurmans et al., 2004). Glutamate can also be released via nonsynaptic mechanisms. Although the excitatory amino acid transporter (EAAT) 1 and EAAT3 are expressed in the developing neocortex at late embryonic and early postnatal stages (Sutherland et al., 1996; Furuta et al., 1997), these glutamate transporters cannot be reversed under physiological conditions and thus most probably do not contribute to nonsynaptic glutamate release (Levy et al., 1998). Possible candidates mediating nonsynaptic glutamate release upon neuronal activity are gap junction hemichannels (Ye et al., 2003) or ATP receptors (Duan et al., 2003), but their contribution to glutamate release in the perinatal developing cortex has to be elucidated.
The expression of both major subtypes of ionotropic glutamate receptors, AMPA/kainate and NMDA receptors, has been identified by in situ hybridization in the prenatal neocortex. In mice, the NMDA receptor subunits NR1 and NR2B are expressed in the neocortical anlage already at E17, while NR2A expression appears around the end of the first postnatal week (Monyer et al., 1994; for review Luján et al., 2005), a developmental expression pattern that has recently been confirmed in human fetal tissue (Bagasrawala et al., 2017). The AMPA/kainate receptor subunits GluR1, GluR2 and GluR4 are also expressed in the neocortical anlage already at E14 (Monyer et al., 1991; for review Luján et al., 2005). Cells in the VZ have been shown to respond to application of glutamate already at E15–16. As NMDA fails to induce any detectable response at this age, neuron precursor cells in the neocortical proliferative zone most probably only express functional AMPA receptors until E16 (LoTurco et al., 1995). However, the observation that between E16 and E18 NMDA agonists and antagonists modulate neuronal migration (Hirai et al., 1999; Kihara et al., 2002) implies that at least migrating neocortical neurons express functional NMDA receptors during this developmental period.
Glycine
Glycine is an inhibitory neurotransmitter located predominantly in the spinal cord, but it is also important for neocortical development. In situ hybridization and immunohistochemical experiments revealed that α2 and β subunits of glycine receptors are present in the superficial layers of the developing cerebral cortex at embryonic stages (Becker et al., 1993; Avila et al., 2013). In accordance with this data, in embryonic and early postnatal neocortical neurons electrophysiological experiments revealed functional glycine receptors, which are most probably α2/β heterodimeric receptors (Flint et al., 1998; Okabe et al., 2004). Since glycine receptors open ligand-gated Cl− channels, activation of glycine receptors leads to a depolarization of cell membrane and can mediate both, excitatory or inhibitory actions in vitro (Flint et al., 1998; Kilb et al., 2002; Sava et al., 2014). However, as no evidence for synaptic glycine release has been observed in the immature neocortex, it has been proposed that taurine is the main endogenous ligand of this receptor (for review Kilb and Fukuda, 2017, but see Avila et al., 2013). In addition, activity-dependent reversal of the glycine transporter GlyT1, which is expressed at low levels in the immature cortex (Jursky and Nelson, 1996), can mediate a nonsynaptic glycine release pathway (Supplisson and Roux, 2002).
Other Neurotransmitter Systems
Information on the development of other neurotransmitter systems is less clear than for the classical neurotransmitters mentioned above. Cholinergic neurons and axons have been observed in the neocortical anlage at E16 (Schambra et al., 1989) and the acetylcholine (ACh) degrading enzyme ACh esterase can be detected at E15 in the VZ, CP and MZ of the neocortical anlage (Dori et al., 2005). The cholinergic agonist carbachol fails to induce any detectable response in cells in the VZ at E16 (LoTurco et al., 1995), but expression of functional nicotinic and muscarinic ACh receptors has been demonstrated in SP neurons of early postnatal rodents (Hanganu and Luhmann, 2004; Hanganu et al., 2009). Noradrenergic fibers have been also documented in the immature brain. At E18 they arrive in the neocortex and spread in the MZ, CP and SP (Levitt and Moore, 1979). Accordingly, α2 adrenoceptors have also been found in the VZ, CP and in migrating neurons of the embryonic brain (Winzer-Serhan and Leslie, 1999).
Appearance of Activity Patterns during Cortical Development
One intriguing observation in developing neuronal networks, both in vivo and in vitro, is the appearance of spontaneous, mostly correlated neuronal activity (for review Spitzer et al., 2004; Khazipov et al., 2013; Luhmann et al., 2016; Luhmann and Khazipov, 2017). These spontaneous activity patterns reveal specific features during distinct developmental stages, in accordance with the establishment of neuronal connectivity (Egorov and Draguhn, 2013). The temporal sequence and properties of spontaneous neuronal activity in early postnatal animal models recapitulates the appearance of neural activity in human preterm babies (Tolonen et al., 2007). It is assumed that early postnatal stages in rodents are comparable to late fetal phases of human development (Clancy et al., 2007).
The earliest neocortical activity has been observed in the proliferative epithelium of the VZ and in the CP (Owens et al., 2000; Corlew et al., 2004). This early spontaneous activity is represented by Ca2+ transients, which differ in their properties and mechanisms between both regions. Ca2+ transients in the VZ are independent of electrical activity, rely on Ca2+ release from intracellular stores and depend on connexin hemichannels and purinoceptors (Owens et al., 2000; Weissman et al., 2004). They are not correlated between cells, with exception of occasionally synchronous pairs of cells, and are mostly probably generated in proliferating radial glial cells (Weissman et al., 2004). These uncorrelated Ca2+ transients in the proliferative VZ may at least partially resemble the Ca2+ signals that have been observed during mitosis in several cell types (Berridge, 1995; Santella, 1998). In contrast, Ca2+ transients in the CP are synchronized between neurons, are suppressed after inhibition of neuronal activity with the Na+ channel blocker tetrodotoxin (TTX), and rely on voltage-gated Ca2+ channels (Corlew et al., 2004). These findings indicate that electrical activity with subsequent Ca2+ influx is essential for the generation of CP Ca2+ transients.
Large-scale electrical events emerge in the developing neocortex of rodents around birth (Garaschuk et al., 2000; Allène et al., 2008). These TTX-sensitive spontaneous events are termed cortical early network oscillations (cENOs), usually start in the posterior cerebral cortex, occur approximately every 2 min and propagate with ~2 mm/s over the whole cortex towards the anterior pole (Figure 2A). Recent experiments suggest that cENOs are triggered by a population of autonomously active layer 3 neurons (Namiki et al., 2013). Around postnatal day (P) 4–5, cENOs are gradually replaced by cortical giant depolarizing potentials (cGDPs). These cGDPs occur at higher rates (~8 min−1) and show substantially faster kinetics (Figure 2B; Allène et al., 2008). In the visual cortex the majority of spontaneous events is classified as slow activity transients (SATs), long lasting (~10 s) events characterized by a summation of rapid oscillatory bursts (15–30 Hz), occurring after P10 and before eye opening (Colonnese and Khazipov, 2010). SATs disappear after enucleation (Colonnese and Khazipov, 2010), indicating that retinal waves drive this spontaneous activity pattern. Retinal waves are spatially highly correlated spontaneous activity patterns that depend on cholinergic and in later phases also on glutamatergic neurotransmission (Zhou and Zhao, 2000).
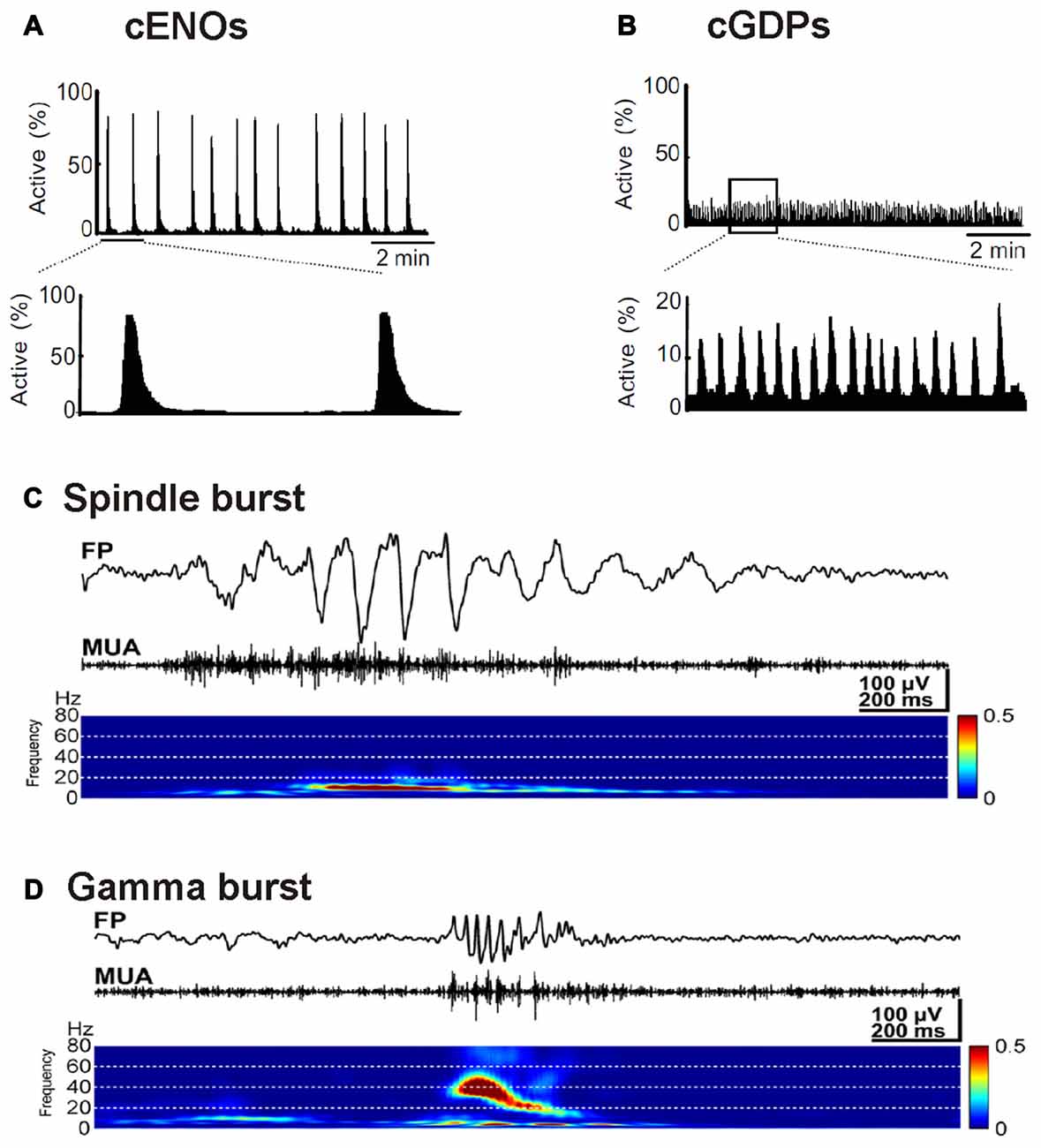
Figure 2. Examples of typical spontaneous activity patterns. (A) Spontaneous cortical early network oscillations (cENOs) in P3 rat neocortical neurons visualized by Ca2+ imaging. (B) In P6 rat neocortical slices, cortical giant depolarizing potentials (cGDPs) replace the cENOs. Note the higher frequency and the lower number of participating neurons in cGDPs (A,B modified from Allène et al., 2008). (C) Field potential recording of spindle bursts occurring spontaneously in the somatosensory cortex of a P1 rat in vivo. Below the FP trace the multi unit activity (MUA) identifies neuronal spikes and the wavelet analysis revealed that the spindle oscillations display a maximal frequency around 10 Hz. (D) Recording of a spontaneous gamma bursts in the somatosensory cortex of a P3 rat in vivo (C,D modified from Yang et al., 2009).
In addition to these patterns that synchronize large neocortical areas, a variety of activity transients that mediate local synchronization has been described (for review Khazipov et al., 2013; Luhmann and Khazipov, 2017). In visual and somatosensory areas of the early postnatal (P0–3) neocortex, local and short network oscillations in a frequency range of 10–20 Hz have been recorded (Khazipov et al., 2004; Hanganu et al., 2006; Minlebaev et al., 2007; Yang et al., 2009, 2013). These short events (0.5–3 s) occur spontaneously every ~10 s and have been termed spindle bursts due to their typical appearance in electrophysiological recordings (Figure 2C; for review Yang et al., 2016). In vitro (Dupont et al., 2006; Hanganu et al., 2009) as well as in vivo data (Yang et al., 2009; Tolner et al., 2012) suggest that SP neurons are involved in the generation or amplification of spindle bursts (for review Luhmann et al., 2009; Kanold and Luhmann, 2010). A substantial part of the spindle oscillations reflects activity in the immature sensory periphery (for review Yang et al., 2016). In neonatal rat primary somatosensory cortex, spatially confined spindle bursts are triggered by spontaneous muscle twitches (Khazipov et al., 2004), which have been observed during intrauterine development in several mammalian species including humans (Khazipov et al., 2004; Tiriac et al., 2014). In somatosensory cortex of premature human babies delta brushes, which have been proposed to be the EEG correlates of spindle bursts, are induced by slight mechanical stimulation, indicating that already at prenatal stages sensory inputs affect cortical activity (Milh et al., 2007). A similar peripheral origin for activity has also been observed for the visual cortex, where spindle bursts and SATs depend on retinal waves (Hanganu et al., 2006; Colonnese and Khazipov, 2010). In the auditory system spontaneous network bursts are recorded in the auditory cortex (Kotak et al., 2007), while also in the periphery spontaneous Ca2+ action potentials occur in fetal inner hair cells of the cochlea (Marcotti et al., 2003). This early spontaneous cochlear activity is synchronized via ATP-dependent signaling and triggers theta-like bursting activity in auditory nerve fibers (Tritsch et al., 2007), which initiate spontaneous burst activity in the auditory brainstem before hearing onset (Tritsch et al., 2010). Taken together, these observations indicate that a substantial proportion of spontaneous, spindle burst activity observed in the developing sensory neocortex is caused by events in the sensory periphery or intermediate relay stations (for review Luhmann et al., 2016).
Around P3, spindle bursts are complemented by spontaneous gamma oscillations with a higher frequency of 30–40 Hz (Figure 2D). They typically have a duration of 100–300 ms and are restricted to local functional columns (Yang et al., 2009; Minlebaev et al., 2011; Khazipov et al., 2013). Interestingly, they are only marginally affected by inhibitory synaptic transmission, indicating that they are functionally distinct from gamma oscillation observed in mature neocortex (Khazipov et al., 2013). At least in the somatosensory system of newborn rodents, gamma oscillations can be recorded in the thalamus and synchronize a single thalamic barreloid with the corresponding neocortical barrel (Minlebaev et al., 2011; Yang et al., 2013), indicating that thalamocortical interactions contribute to the generation of gamma oscillations already in the immature neocortex. Recent experiments demonstrated that neurons in supragranular layers of the developing neocortex are particular capable to generate high frequency oscillatory events (Bitzenhofer et al., 2017).
However, inhibition of peripheral sensors and/or peripheral nerves abolishes only a fraction of spindle bursts and gamma oscillations in the somatosensory cortex of early postnatal mice (Yang et al., 2013; An et al., 2014), suggesting that a substantial proportion of spontaneous activity is generated in subcortical nuclei or within the developing neocortex itself. Such central spontaneous activity transients can directly interfere with activity in adjacent cortical areas independent of thalamic connections (Zagha et al., 2013).
Role of Neurotransmitter Systems in Spontaneous Activity
In particular during early developmental stages spontaneous events seem to be influenced by electrical synapses (Blankenship and Feller, 2010). In vitro correlates of spindle bursts at early postnatal phases are independent of GABA and glutamate, but require gap junctional coupling (Dupont et al., 2006), suggesting that electrical synapses are essential for the generation of oscillatory activity during these stages. Interestingly, this activity undergoes a fast developmental switch at ~P4 when this activity depends mainly on AMPA receptors (Dupont et al., 2006). This observation is consistent with the observation that at comparable developmental stages glutamatergic inputs and local GABAergic interneurons mediate spindle burst firing also in vivo (Bitzenhofer et al., 2015).
The cENOs depend on both AMPA and NMDA receptors, demonstrating the glutamatergic nature of these events (Garaschuk et al., 2000; Allène et al., 2008). In contrast, cGDPs are only partially affected by AMPA/NMDA receptor antagonists, but depend on GABAA receptor-mediated transmission (Allène et al., 2008). The peripherally generated portion of spontaneous spindle and gamma bursts in the cortex depends on thalamocortical synaptic connections, either directly or via SP neurons, and thus on glutamatergic synapses characteristic of these connections (Zhao et al., 2009).
Activation of muscarinic ACh receptors induces in vitro correlates of spindle-like spontaneous activity (Dupont et al., 2006; Hanganu et al., 2009). In line with this, electrical stimulation of the cholinergic basal forebrain nuclei induces spindle busts and inhibition of ACh degradation by the ACh esterase inhibitor physostigmine enhances the occurrence of spontaneous spindle bursts in vivo (Hanganu et al., 2007; Janiesch et al., 2011). In contrast, inhibition of muscarinic ACh receptors reduces the occurrence of spindle busts in the visual cortex (Hanganu et al., 2007). These results imply that the cholinergic system may be relevant for the generation of spontaneous activity in neocortical circuits. Another neurotransmitter system that is important for the generation of spontaneous activity is the purinergic system. In the VZ (Weissman et al., 2004) and the olfactory system (Tritsch et al., 2007) a critical role of purinoceptors and/or ATP as relevant neuromodulator has been shown, indicating that this neurotransmitter system is highly relevant for the generation of ontogenetically early activity.
Impact of Neuronal Activity on Neurodevelopmental Events
There is increasing experimental evidence that the above-mentioned early patterns of spontaneous neuronal activity are not an epiphenomenon reflecting the gradual maturation of membrane excitability, neuronal connectivity or synaptic functions, but rather that these spontaneous activity patterns play an important role in various neurodevelopmental processes. Even in clinical setting it has been demonstrated that the properties of spontaneous activity recorded with EEG in preterm infants provide prognostic value of brain activity for further development (Iyer et al., 2015).
Neurogenesis
A series of influential studies demonstrated that neurogenesis is directly governed by neurotransmitters. In the VZ glutamate, acting on AMPA/kainate receptors, and GABA, acting on GABAA receptors, stimulates neurogenesis, while inhibition of AMPA/kainate or GABAA receptors promotes neurogenesis (LoTurco et al., 1995). In the SVZ, which is another proliferative part of the developmental neocortex, the effect of glutamate and GABA on neurogenesis is opposite (Haydar et al., 2000). These results indicate that both neurotransmitter systems are directly involved in the regulation of neurogenesis. In addition, glycine receptors and muscarinic ACh receptors modulate the rate of neurogenesis in the neocortical anlage (Ma et al., 2000; Avila et al., 2014). While these previous observations only indicate an influence of interstitial neurotransmitter on neurogenesis, without identifying the source of these neurotransmitters and the mechanisms of their action, more recent results indicate that neuronal activity is directly involved in the regulation of neurogenesis. Ca2+ transients occur during mitosis in several cell types and probably directly influence proliferation (Berridge, 1995; Santella, 1998), suggesting that neuronal activity and/or non-synaptic GABA/glutamate release mediate their effect on neurogenesis by interference with these Ca2+ transients. In the VZ of mice, the frequency and spatial properties of Ca2+ waves are directly correlated to the amount of neurogenesis (Weissman et al., 2004), suggesting that such activity transients may modulate the rate of neurogenesis. A more causal evidence comes from experiments in which the suppression of Ca2+ waves by antagonists of purinoceptors reduces proliferation in the VZ (Weissman et al., 2004), suggesting that spontaneous Ca2+ waves can be directly involved in the regulation of neurogenesis. In the visual cortex, pharmacological inhibition of retinal waves increases neurogenesis (Bonetti and Surace, 2010), demonstrating for the first time that also peripherally generated activity is correlated to neurogenesis. An additional hint that neuronal activity might enhance neurogenesis comes from clinical studies in preterm babies, where increased activity in EEG recordings during early developmental stages is correlated with faster growth of brain structures during subsequent months until term age (Benders et al., 2015). However, in this study it could not be excluded that: (i) neuronal activity affects further developmental processes influencing the size of brain structures (e.g., by acting on apoptosis); or (ii) that underlying pathophysiological mechanism have independent effects on both immature activity and further brain development, thereby creating spurious relations. Further experiments are required to evaluate to which extent the effect of the diverse neurotransmitters on neurogenesis is modulated by an interference with spontaneous Ca2+ transients in the VZ.
Determination of Neuronal Identity
Neuronal activity directly influences the expression pattern of various factors and thereby influences the phenotype of neurons (Flavell and Greenberg, 2008). Spontaneous oscillatory activity modulates e.g., the expression of transcription factors (Hanson and Landmesser, 2004), which can subsequently change the phenotype of neurons. A series of studies provided evidence that the neurotransmitter phenotype of neurons is rather plastic and can be modified by electrical activity (for review Spitzer, 2017). In spinal neurons from Xenopus, the frequency of spontaneous Ca2+ transients is positively correlated with the number of GABAergic neurons, while inhibition of Ca2+ transients promotes the generation of excitatory neurons (Gu and Spitzer, 1995; Borodinsky et al., 2004). However, to our knowledge, a homeostatic change in neurotransmitter phenotype has not been demonstrated in the developing neocortex (for review Spitzer, 2017), despite the fact that major properties required for “neurotransmitter plasticity” are present in the mammalian CNS (Demarque and Spitzer, 2012). Other experiments demonstrated that even the expression of basic morphogenic factors like sonic hedgehog (Belgacem and Borodinsky, 2015) is directly regulated by electrical activity. Further studies regarding this interesting issue are required to uncover, whether spontaneous electrical activity is an important determinant of global neurodevelopmental processes, e.g., for area specification (for review Yamamoto and López-Bendito, 2012).
Neuronal Migration
Neuronal migration critically depends on patterned activity, as has been demonstrated in the seminal experiments by Komuro and Rakic (1996), who observed in vitro that repetitive Ca2+ transients in neurons are directly linked to forward migration. Impairment of these repetitive Ca2+ transients by Ca2+ channel agonists (Komuro and Rakic, 1996), by inhibition of neuronal activity (Heck et al., 2007) or by the expression of hyperpolarizing K+ channels (De Marco García et al., 2011) attenuates neuronal migration. In addition, a variety of studies demonstrated that various neurotransmitters influence neuronal migration (for review Luhmann et al., 2015).
In a series of experiments Toby Behar and Jeffery Walker uncovered the essential role of GABA for neuronal migration. In summary, these in vitro experiments with dissociated cell and organotypic cultures demonstrated that GABA acts as chemokine/chemoattractant and that inhibition of GABAA receptors enhances the number of postmitotic neurons reaching the CP, while the unspecific GABAA/C antagonist picrotoxin induces a transient reduction in postmitotic neurons in the CP (Behar et al., 1996, 2000). These results led to the hypothesis that GABAA receptors provide a “stop signal” for migrating neurons, while GABAC receptors are required as a pro-migratory stimulus. Indeed, it could later be verified that a specific inhibition of GABAC receptors impedes radial neuronal migration (Denter et al., 2010). In line with these in vitro results, focal inhibition of GABAA receptors in vivo, using small elvax implants, induces ectopic neurons in superficial neocortical layers that resemble a hypermigratory phenotype (Heck et al., 2007). Intriguingly, a similar phenotype has been also observed after in vivo application of elvax implants containing the GABAA receptor agonist muscimol. This observation is due to the fact that continuous presence of muscimol leads to a massive desensitization of GABAA receptors and/or impairs the repetitive Ca2+ transients required for adequate migration (Heck et al., 2007).
In vitro experiments demonstrated that activation of glycine receptors also impairs radial migration, while inhibition of glycine receptors is without effect, indicating that the glycinergic system has the potential to influence migration, but is not directly involved in the regulation of radial migration in the early postnatal neocortex (Nimmervoll et al., 2011). Genetic deletion of the glycine receptor subunit α2, which is a major α subunit expressed in perinatal cerebral cortex (Malosio et al., 1991), reduces the number of interneurons migrating from the SVZ into the cortex at E15 (Avila et al., 2013). However, it has been demonstrated that activation of glycine receptors enhances GABAergic network activity in the immature neocortex (Sava et al., 2014). Thus it is still unresolved whether activation of glycine receptors is directly linked to neuronal migration or whether glycinergic effects on network activity are the crucial parameter influencing these migratory events.
The glutamatergic system is also directly involved in the modulation of neuronal migration. Both, in vitro (Behar et al., 1999) and in vivo (Reiprich et al., 2005) experiments demonstrated that inhibition of NMDA receptors disturbs neuronal migration. Experiments performed in organotypic slice cultures of the cerebral cortex revealed that both activation (Kihara et al., 2002) and inhibition (Hirai et al., 1999) of NMDA receptors between E16 and E18 slow down cell migration, indicating that moderate activation of NMDA receptors by endogenous glutamate may be required for adequate responses. However, NMDA receptor-mediated effects on cell migration are not changed in slices obtained from munc18-1 knock-out animals, in which synaptic neurotransmitter release has been deleted (Manent et al., 2005), challenging the physiological role of neurotransmitters as regulators of migration. As electrical synapses and non-vesicular release processes play an important role during neuronal development, and since a variety of neurodevelopmental events are directly affected by neurotransmitters or lack of neuronal activity, it can be assumed that non-synaptically mediated activity is sufficient for early neurodevelopmental events (Manent et al., 2005).
Other neurotransmitter systems are also affecting neuronal migration. Activation of D1 dopamine receptors stimulates, while D2 receptor activation impedes tangential migration of GABAergic interneurons (Crandall et al., 2007). The observation that the suppression of a radial glial cell specific splice variant of the ACh esterase attenuates radial migration (Dori et al., 2005) indicates that the cholinergic system also contributes to the regulation of neuronal migration.
Overall, these results clearly demonstrate the essential role of various neurotransmitters as regulators of neuronal migration, but how much of the mostly pharmacologically induced effects are mediated by direct interactions with migrating neurons, and to which extent secondary influences on neuronal activity contribute to these effects remain to be investigated in more detail. Recent studies revealed a direct influence of neuronal activity on neuronal migration. Pharmacological inhibition of retinal waves disturbs the adequate layer formation in the visual cortex (Bonetti and Surace, 2010), suggesting that peripheral activity patterns directly control the structural maturation of the neocortex.
Neuronal Apoptosis
Neuronal apoptosis, i.e., the programmed cell death of neurons, is a fundamental process in the developing brain, which determines the final size and organization of mature neuronal assemblies (for review Kuan et al., 2000; Blanquie et al., 2017a). A number of experiments demonstrated that apoptosis is modified by electrical activity. Silencing of cortical networks with TTX increases the number of apoptotic neurons in vitro (Ruijter et al., 1991; Heck et al., 2008; Schonfeld-Dado and Segal, 2009; Golbs et al., 2011), as well as in vivo (Murase et al., 2011). On the other hand, the number of apoptotic neurons in vitro decreases when spontaneous activity is enhanced by elevated extracellular K+ concentrations (Ghosh et al., 1994), by inhibition of GABAA receptors (Léveillé et al., 2010), or by low NMDA doses (Soriano et al., 2006). In line with the last observation, inhibition of NMDA receptors triggers apoptosis in vivo (Ikonomidou et al., 1999). A direct link between electrical activity and neuronal survival has been demonstrated by simultaneous recordings of electrical activity and monitoring cell death in dissociated neuronal cultures. In neocortical cultures, high-frequency neuronal activity attenuates caspase-3 dependent apoptosis (Figure 3; Heck et al., 2008). The dependence of apoptotic rates on electrical activity could be quantified in experiments with hippocampal neurons, which revealed that inactive neurons are ten times more likely to undergo apoptosis than neurons contributing to spontaneous, synchronous network activity (Murase et al., 2011). Since it has been reported that both, immature activity patterns (Yang et al., 2009; Minlebaev et al., 2011) and the distribution of apoptosis (Ikonomidou et al., 1999; Stankovski et al., 2007) show an uneven distribution across regions and layers of the developing cortex, the amount and pattern of spontaneous neuronal activity might directly influence the structural development of the neocortex by regulating apoptosis rates. This hypothesis has recently been addressed by a study showing that spontaneous and periphery-driven activity patterns refine the final number of cortical neurons in a region-dependent manner and thus can directly influence the structural and functional development of the neocortex (Blanquie et al., 2017c).
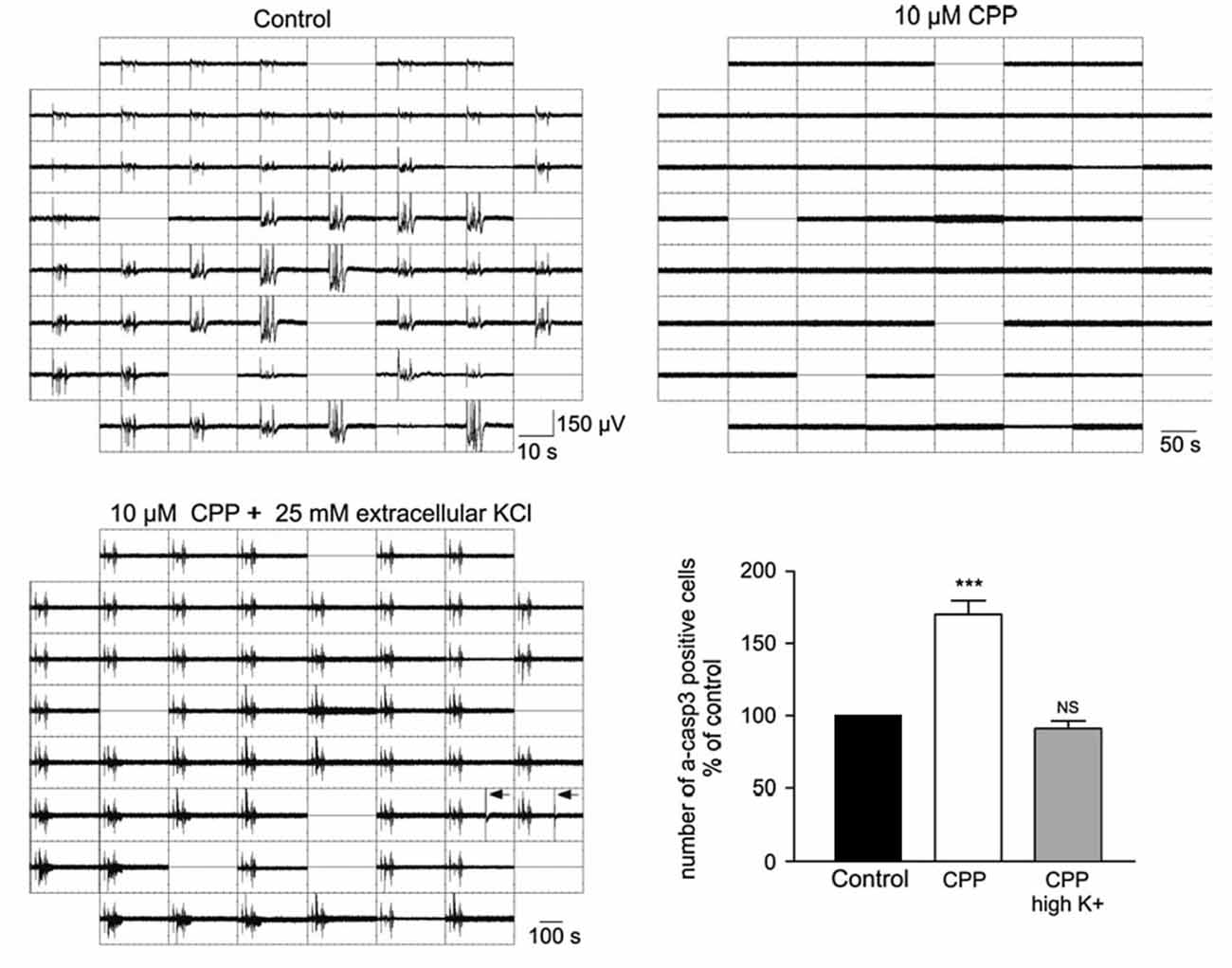
Figure 3. Influence of neuronal activity on apoptosis. Electrophysiological recordings and determination of apoptotic cells by activated Caspase3 immunohistochemistry in dissociated cortical cultures reveal that the NMDA receptor antagonist CPP blocks neuronal activity and enhanced apoptosis (***p < 0.001, Kruskal-Wallis test). This effect of CPP on apoptosis can be rescued in the presence of high K+ concentration, which restores the electrical activity (modified from Heck et al., 2008).
For neocortical dissociated cultures it has been shown that the activity-dependent release of brain-derived neurotrophic factor (BDNF) and activation of phosphatidylinositol 3-kinase are key downstream elements in the activity-dependent apoptosis pathway, which promotes survival via the AKT pathway (Wagner-Golbs and Luhmann, 2012). However, depending on the pattern of electrical activity or the site of calcium entry other pathways are probably also involved in the activity-dependent regulation of neuronal apoptosis (for review Hardingham and Bading, 2010). Suppression of neuronal activity with ethanol or antiepileptic drugs has also been shown to induce apoptosis during early development in vivo (Ikonomidou et al., 2000; Bittigau et al., 2002; Ikonomidou and Turski, 2010; Lebedeva et al., 2017), providing further support that a modification of physiological activity patterns during early development affects neuronal apoptosis. Finally, impairment of presynaptic neurotransmitter secretion in Munc18-1 deleted animals results in a drastic apoptotic response in subcortical areas around the developmental stage when initial synaptic responses should occur (Verhage et al., 2000). Unfortunately, no indication for neocortical apoptosis could be observed in these studies, as these animals die perinatally before the wave of neocortical apoptosis occurs. In this respect it should be considered, that the effect of neuronal activity and/or neurotransmitter on neuronal apoptosis is specific for particular neuronal populations. Recently it has been demonstrated that the transient Cajal-Retzius neurons could be rescued from apoptosis by inhibition of depolarizing GABAergic responses, while the rate of apoptosis in the remaining cortical neurons was unaltered under this condition (Blanquie et al., 2017b). Interestingly, the noradrenergic system also has opposite effects on the rate of apoptosis in Cajal-Retzius cells (Naqui et al., 1999) as compared to other neocortical neurons (Popovik and Haynes, 2000).
The observation that neuronal activity regulates the rate of apoptosis raises the intriguing question whether only the level of neuronal activity or the specific pattern of activity may mediate the pro-survival effect. Direct evidence that the pattern of activity regulates neuronal apoptosis is provided from experiments using organotypic slice cultures (Heck et al., 2008). Inhibition of gap junctions desynchronizes the typical spontaneous network activity, but increases the overall frequency of action potentials. From the observation that under this condition the rate of apoptosis is increased (Heck et al., 2008), it was concluded that synchronicity of discharges is a more important factor for the suppression of neuronal apoptosis than the number of discharges. Subsequent experiments revealed that a moderate increase in extracellular K+ concentration, which slightly enhances the frequency of correlated bursts, or the induction of highly synchronized activity after inhibition of GABAA receptors also reduces the number of apoptotic neurons (Golbs et al., 2011). However, Golbs et al. (2011) also revealed that a further increase in the K+ concentration causes a desynchonization of neuronal activity with the appearance of high frequency tonic firing, which still provide a strong antiapoptotic signal, suggesting that other factors beyond the appearance of synchronous bursts also contribute to activity-dependent neuronal survival. Therefore, further experiments elucidating the “neuronal code” that provides antiapoptotic effects are required to fully understand the relation between neuronal activity and survival.
Establishment of Mature Neuronal Connectivity
It is often assumed, that the major organization of neuronal connectivity is genetically encoded and controlled by the spatio-temporal pattern of transcription factors as well as by intercellular communication via cell-cell interactions or constitutive secretion of growth factors (Chao et al., 2009). The most stringent evidence for activity-independent formation of connectivity comes from SNAP-25 deficient mice, in which vesicular neurotransmitter release and thus network activity is completely abolished, but the prenatal formation of thalamocortical connections is maintained (Molnár et al., 2002).
A variety of studies demonstrated that spontaneous activity influences growth and differentiation of neuronal dendrites and axonal projections and is essential for the formation of mature functional neuronal circuits (for review Chen and Ghosh, 2005; Zheng and Poo, 2007; Yamamoto and López-Bendito, 2012). Many of these studies have been performed on developing visual cortex, in which the organization of ocular dominance columns serves as a model for the establishment of adequate connectivity (Ackman and Crair, 2014). Already the early studies of Hubel and Wiesel demonstrated that correlated visual activity during critical periods represents a prerequisite for adequate connectivity (Hubel and Wiesel, 1970). Inhibition of early spontaneous retinal activity, which provides correlated inputs from the sensory periphery before eye opening, causes a persistent disorganization of ocular dominance columns and a pronounced increase in receptive field size of neurons in primary visual cortex (Huberman et al., 2006). Another causal evidence for the role of spontaneous activity is provided by in vivo experiments, which used optogenetic manipulation of retinal activity to demonstrate that patterned retinal activity, which is asynchronous and has a delay of >100 ms between both eyes, is required for the development of eye segregation (Zhang et al., 2012). Interestingly, spontaneous retinal activity directly influences the expression of several components of the ephrin system, which is essential for the development of adequate neuronal connections in the visual system (Nicol et al., 2007). This result provides a mechanism by which electrical activity can influence the outgrowth of axons. In addition this suggests that molecular cues controlling neocortical connectivity, which are typically considered as activity-independent factors in early development, may at least partially depend on neuronal activity.
In the immature somatosensory cortex a stringent role of spontaneous activity in the formation of functional neuronal circuits has been found. Using a comparable experimental approach as Hubel and Wiesel, trimming of mystacial whiskers during a critical period impaired the formation of “whisker barrels” in layer 4 (Woolsey and Wann, 1976), which are the neocortical representations of single whiskers in the primary somatosensory cortex. In the barrel cortex of early postnatal rats spontaneous activity also appears before the onset of active whisking (Yang et al., 2009) and a considerable portion of this correlated spontaneous activity is independent of activity in the sensory periphery (Yang et al., 2013). Intriguingly, in early postnatal rat the spatial domains of the spontaneous oscillations resemble the characteristic columnar organization of neocortical whisker representations several days before the anatomical correlate of these whisker representations become obvious (Yang et al., 2013; Luhmann, 2017). Attenuation of spontaneous spindle bursts activity by ablation of the SP using p75-immunotoxin severely impairs the columnar organization of the rodent barrel cortex (Figure 4; Tolner et al., 2012), providing causal evidence that spontaneous and locally synchronized neuronal activity is essential for the functional maturation of neocortical architecture in the barrel cortex.
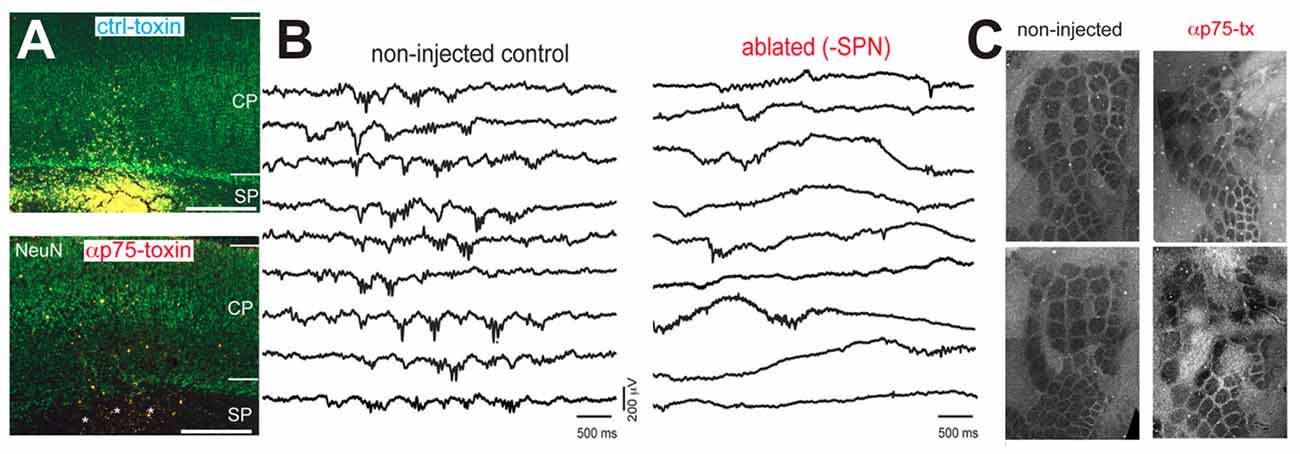
Figure 4. Impairment of neuronal activity affects structural formation of the neocortex. (A) The injection of the neurotoxin αp75 selectively ablates SP neurons. (B) Ablation of SP neurons suppresses the occurrence of spontaneous spindle busts in the somatosensory cortex. (C) Cytochrome-oxidase stained tangential slices of P10 mice indicate that the barrel formation is severely impaired after ablation of SP neurons (modified from Tolner et al., 2012).
In the auditory cortex, peripheral activity also shapes the maturation of neocortical representation (Chen and Yuan, 2015). Repetitive presentation of pure tones during the critical period after onset of hearing massively shifts the tonotopic map, while application of white noise disrupts the formation of tonotopy (de Villers-Sidani et al., 2007; Zhou et al., 2008). In the developing auditory system, it has been also shown that correlated, patterned spontaneous activity before the onset of hearing is required for the formation of the mature, functional connectivity at least in brainstem nuclei (Clause et al., 2014).
In summary, these results suggest that spontaneous neuronal activity present before the appearance of adequate sensory stimulation is required for the normal development of the cortical architecture in primary sensory areas. Neuronal activity plays also an important role in the subsequent functional maturation of initially formed synaptic networks. First, spontaneous synchronous network activity is required to activate silent synapses by incorporating AMPA receptors into the postsynaptic membrane (Durand and Konnerth, 1996; Voigt et al., 2005), thereby shaping the functional connectivity within the existing structural network. Second, neuronal activity plays an essential role in the pruning of redundant neuronal connections (e.g., Hata and Stryker, 1994; for review Wong and Ghosh, 2002).
Axon Myelination
The myelination of axons, required for fast spike propagation, is also directly controlled by neuronal activity. Both, sensory evoked responses as well as spontaneous neuronal activity, regulate axon myelination (Demerens et al., 1996; Barrera et al., 2013). Neuronal activity causes activation of adenosine receptors on oligodendrocyte precursor cells, which subsequently enhances the proliferation and differentiation of these cells (Stevens et al., 2002). In addition, the activity-dependent release of glutamate from active axons directly induces myelin formation in differentiated oligodendrocytes (Wake et al., 2015), which is mediated via activation of NMDA receptors and a subsequent Fyn-kinase dependent release of translational repression (White and Krämer-Albers, 2014). However, to our knowledge it has not been investigated whether single action potentials or patterned activity is required for proper activation of oligodendrocytes and myelination.
Non-neuronal Components of the Central Nervous System
Non-neuronal components of the central nervous system are also affected by electrical activity. As mentioned above, neuronal activity initiates the differentiation of oligodendrocytes via the axonal release of adenosine (Stevens et al., 2002). Immature astrocytes under in vitro conditions also demonstrate massive alterations in gene expression profile if the activity levels on co-cultured neurons are altered (Hasel et al., 2017). Furthermore, it has been recently shown that peripheral-driven neuronal activity also regulates vessel development and patterning in the developing rodent brain (Whiteus et al., 2013; Lacoste et al., 2014). In summary, these observations strongly indicate that neuronal activity can shape the structural development of the neocortex also by influencing non-neuronal components.
Pathophysiological Implications of Disturbed Perinatal Neuronal Activity
The influence of neuronal activity patterns on various developmental events is also supported by clinical studies. We concentrate our review: (i) on studies demonstrating that conditions leading to disturb neuronal activity cause neurodevelopmental diseases; and (ii) on diseases in which putative alterations in immature neuronal activity have been associated with their clinical manifestation.
Substances That Interfere with Early Neuronal Activity
One intriguing observation supporting the role of neuronal activity in the structural and functional development of the neocortex is that substances interfering with early neuronal activity often cause disturbances in neocortical development. Recently, a pilot study performed in preterm babies (26–33 postconceptional weeks) revealed that several drugs (e.g., fentanyl, phenobarbital, theophylline) that are routinely used in neonatal intensive care units alter the properties of spontaneous brain activity (Malk et al., 2014), suggesting that the impact of spontaneous activity patterns in early human development should also be considered to evaluate possible adverse effect of such substances.
The majority of anesthetics suppress neuronal activity by acting either as NMDA antagonists, GABA mimetics or gap junction blockers. Several animal studies demonstrated that such anesthetics can induce severe neurodegeneration in the immature brain. Exposure to isoflurane at a clinical relevant concentration (1.5 vol. % for 6 h) leads to a widespread neurodegeneration in the cortex of immature rats (Jevtovic-Todorovic et al., 2003). Both, the NMDA antagonist ketamine (20–50 mg/kg) and the GABAergic agonist midazolam (9 mg/kg) induced a neuroapoptotic response after 5 h treatment in neonatal mice, which was enhanced upon combination of both substances (Young et al., 2005). Recently it could be shown that the combined application of 40 mg/kg ketamine and 9 mg/kg midazolam indeed reduced spontaneous activity levels by ca. 90%, while sensory evoked neuronal activity was less effected, suggesting that the attenuation of spontaneous activity may be more relevant for the neuroapoptotic responses occurring under this conditions (Lebedeva et al., 2016). While N2O (50%–75% for 6 h) does not induce obvious neurodegeneration in rats (Jevtovic-Todorovic et al., 2003), the combination of N2O (70 vol. %) and isoflurane (0.75 vol. % for 6 h) results in a significant increase in the number of apoptotic neurons in rats (Zhou et al., 2011). Finally, 5 h application of isoflurane (1–1.5 vol. %) also induces apoptosis in fetal Rhesus macaque brains in vivo (Creeley et al., 2014). In cultures from human neural stem cells (hNSCs) it was shown that ketamine (100 μM) induced neuronal apoptosis, but only after a prolonged application of 24 h (Bai et al., 2013), while isoflurane (5%) application for 4 h does not affect neuronal survival in these human neuronal cultures (Sohn et al., 2017). Both results may suggest that human neurons are less prone to anesthetic-induced neuronal apoptosis. In accordance with these in vitro data, a recent multicenter study failed to observe a systematic adverse outcome in 2 year old infants that underwent a general servoflurane (median 54 min) anesthesia in the first postnatal weeks (Davidson et al., 2016). This observation is in line with the results of other clinical studies that reported no systematic cognitive outcomes of anesthesia during early childhood, which however typically include anesthesia in slightly older children (<3–4 years; for review O’Leary and Warner, 2017). In addition, it should be emphasized that at least the adverse effects of ketamine may not directly be related to reduced neuronal activity, since ketamine directly interacts with the mitochondrial potential (Ito et al., 2015).
Several anticonvulsants that interact with GABAA and glutamate receptors and therefore suppress neuronal activity are also associated with an exacerbated apoptotic response in early postnatal rodents (Bittigau et al., 2002; Ikonomidou and Turski, 2010). The anticonvulsant drugs vigabatrin (200 mg/kg/day), valproate (100 mg/kg/day) and lamotrigine (20 mg/kg/day) can cause severe cortical malformations upon fetal exposure (Manent et al., 2007, 2008) and fetal valproate exposure (600 mg/kg at E12.5) disturbs the formation of tonotopic maps (Dubiel and Kulesza, 2015), suggesting that these substances can in animal experiments impair neuronal migration and functional differentiation. Also in cultures of hNSCs chronic application of the anticonvulsant substances phentobarbital (10 μM), valproate (10 μM), lamotrigine (1 μM) or carmazepine (1 μM) reduces cell viability (Cao et al., 2015). In humans, fetal exposure to these drugs is known to trigger long-term structural, behavioral and cognitive defects including modification of the brain mass, learning and memory impairments, anxiety, social interaction and sensorimotor integration disabilities (for review Turski and Ikonomidou, 2012). Since anticonvulsants attenuated spontaneous brain activity in preterm babies (Malk et al., 2014), we suggest that, in addition to other mechanisms (for review Schachtner, 2017), reduced neuronal activity should be considered as putative mechanism for the teratogenic effects of anticonvulsants.
One major clinical implication emerging from these advances in the understanding of the impact of neuronal activity on the structural and functional development of the neocortex is that the use of pharmacological therapies that interfere with neuronal activity during early developmental stages (i.e., during pregnancy or during early postnatal phases) must be carefully evaluated.
Fetal Alcohol Syndrome
The clinically most relevant case for pharmacologically induced neurodevelopmental disorder is still the fetal alcohol spectrum disorder (FASD), caused by ethanol consumption during pregnancy (for review Olney, 2014). Excessive ethanol consumption during a specific developmental stage which coincides with synaptogenesis in the cortex (prenatally in humans and early postnatally in rodents) induces apoptotic neurodegeneration that leads to gross structural brain pathology and a variety of long-term behavioral, cognitive or neuropsychiatric disorders (Olney et al., 2002). Although originally ethanol has been suggested to affect predominantly neurons, ethanol-induced apoptosis of glial cells has been also reported (for review Guizzetti et al., 2014). Long term exposure to ethanol (20 mM, 1 week) increases apoptosis in undifferentiated hNSCs and suppresses astrocyte differentiation, while neuronal differentiation is unaffected (Nash et al., 2012). Thus a loss of astrocytes may partially underlie ethanol neurotoxicity. These results can be explained by ethanol effects on gene expression in hNSCs (Khalid et al., 2014). The devastating effects of ethanol on developing brains has been attributed to a variety of mechanism from genetic and epigenetic alteration to metabolic and nutritional disturbances (for review Muralidharan et al., 2013), however, direct effects of ethanol on neuronal activity may contribute to the FASD (for review Lotfullina and Khazipov, 2017).
Ethanol inhibits NMDA receptor-mediated signals and enhances GABAA receptor-mediated responses (Lovinger et al., 1989; Ikonomidou et al., 2000; Glykys et al., 2007), therefore it should suppress neuronal network activity in the brain. However, in vitro results obtained in acute hippocampal slices of P3–7 rats demonstrated that ethanol significantly increases the frequency of giant depolarizing potentials (GDPs; Galindo et al., 2005), supporting the hypothesis that GABAA receptors mediate excitatory actions (Ben-Ari et al., 2012). However, in vivo GABAA receptors provide a dominant inhibitory action already in early postnatal rodent cortex (Kirmse et al., 2015). In line with this, recent in vivo recordings in neonatal rats demonstrated that at P4–7 alcohol almost completely suppresses gamma oscillations and spindle bursts as well as sensory-evoked responses, whereas its action at P14–17 was much weaker (Lebedeva et al., 2017). In parallel to these electrophysiological observations it has been documented in the same study that the strongest apoptotic effect of ethanol occurs between P4 and P7, while already at P14–17 ethanol fails to increase apoptotic cell death (Lebedeva et al., 2017), suggesting that in vivo an alcohol-induced reduction of neuronal activity appears to contribute to its neurodegenerative effect (for review Lotfullina and Khazipov, 2017). In addition, it has been shown that ethanol also directly impairs the frequency of Ca2+ transients in migrating mouse neurons and the rate of neuronal migration (Kumada et al., 2006). This attenuation of neuronal migration may also contribute to the deleterious effects of ethanol consumption during pregnancy.
Neonatal Encephalopathy
The most common cause of neonatal encephalopathy is perinatal asphyxia, a relevant complication occurring in about 2% of human births. Consequences are several brain dysfunctions like cerebral palsy, sensory and cognitive impairments, epilepsy or autism spectrum disorders (ASD; Vannucci, 2000). Other causes, like sepsis, meningitis or metabolic disturbances have been also discussed as possible causes underlying neonatal encephalopathy. While it is obvious that hypoxic-ischemic periods and/or severe infections impair the development of the cortex by many mechanisms, e.g., by excitotoxicity or apoptosis (Vannucci, 2000), recent experiments suggest that direct and persistent effects of neonatal encephalopathy on neuronal activity may be also considered as potential factors leading to neonatal encephalopathy. A variety of studies demonstrated that hypoxic and/or ischemic conditions in the immature brain induce acute and also long-lasting changes in spontaneous activity in humans (Roberts et al., 2014) and in rodents, both in vitro and in vivo (Brockmann et al., 2013; Ranasinghe et al., 2015; Mordel et al., 2016).
In vitro experiments revealed that immature rodent cortical neurons are more resistant against hypoxic-ischemic conditions (Luhmann and Kral, 1997), whereas SP neurons might be more vulnerable to hypoxia (McQuillen and Ferriero, 2005; but see Albrecht et al., 2005; Okusa et al., 2014). Due to their important role for spontaneous activity and cortical rewiring (Dupont et al., 2006; Tolner et al., 2012), loss of SP neurons can particularly contribute to the long lasting impairment of spontaneous and neuronal activity. In addition, experimentally induced inflammation induces in rodents a rapid modification in the properties of spontaneous activity in vitro and in vivo (Nimmervoll et al., 2013), thus indicating that conditions like bacterial or viral infections may also have an impact on cortical development by modifying neuronal activity. While it is obvious that both hypoxic-ischemic insults as well as inflammation directly impair neuronal survival by various mechanisms (Millar et al., 2017), we suggest that persistent effects of such insults on spontaneous neuronal activity patterns may be an additional factor contributing to acute and chronic neuronal dysfunction.
Epilepsy
Epilepsy is one of the most common neurological disorders. An imbalance between excitation and inhibition, e.g., by disturbances in neuronal excitability or neurotransmitter systems and aberrant connectivity, underlies the generation of seizures (Goldberg and Coulter, 2013). Seizure incidence is higher in the neonatal period as compared to mature stages (Chapman et al., 2012) and seizures occurring in the neonatal period increase the risk of manifesting epilepsy (Pisani et al., 2015). Here we do not cover the mechanisms underlying the enhanced seizure incidence in the immature brain and refer to reviews by Sanchez and Jensen (2001) and Ben-Ari (2006).
The massively enhanced neuronal activity during a seizure causes a functional remodeling of neuronal circuits, which may increase the risk to develop further seizures, a concept termed “seizures beget seizures”. While this concept is still under discussion in the adult brain, experimental data demonstrate that neonatal seizures indeed increase the risk to manifest epilepsy (for review Ben-Ari and Holmes, 2006).
The most striking evidence comes from in vitro experiments with in-toto hippocampal preparations, which demonstrated that seizure-like activity in one hemisphere induces functional alterations in the contralateral hemisphere thereby inducing an epileptogenic mirror focus in rats (Khalilov et al., 2003). The induction of repetitive seizures during the first or second postnatal week enhances mossy fiber sprouting and the number granule cells in the rat hippocampus (Holmes et al., 1998) and persistently reduces spike adaptation, thus enhancing spike frequency (Villeneuve et al., 2000). Spontaneous febrile seizures in the immature rodent brain (at P10) have been shown to induce persistent changes in HCN channels (Chen et al., 2001). While repetitive seizures in rats induced during the first postnatal day reduce the amplitude of GABAergic postsynaptic currents (Isaeva et al., 2006), spontaneous febrile seizures enhance functional GABAergic projections in the hippocampus (Zhang et al., 2004a) and increase the expression of the GABAA receptor α1 subunit (Zhang et al., 2004b). The developmental shift in the expression of chloride transporters, responsible for the shift from depolarizing to hyperpolarizing GABAergic action (Ben-Ari et al., 2012), is not affected by repetitive neonatal seizures (Isaeva et al., 2006). In accordance with these hippocampal results, repetitive seizures during the first 10 postnatal days also enhance the seizure susceptibility in the rat neocortex, which is accompanied by a small reduction in GABAergic postsynaptic events and a small increase in glutamatergic synaptic transmission (Isaeva et al., 2010). In summary, these observations suggest that altered neuronal activity during early developmental stages, e.g., caused by mutations in relevant genes or pre-/perinatal insults, may contribute to the establishment of certain forms of epilepsy, beside other mechanisms (Rakhade and Jensen, 2009).
One intriguing consequence of these observations is that it might be possible to prevent epileptogenesis after neonatal seizures or other early peri-/postnatal insults. A study in a genetic mouse model for neonatal epilepsies demonstrated that epileptiform-like activity and behavioral deficits in adult animals can be prevented by continuous application of the loop diuretics bumetanide, which reduces the depolarizing GABAergic responses and attenuates pathophysiological electrical activity (Ben-Ari et al., 2012) during the first two postnatal weeks (Marguet et al., 2015). However, clinical studies are less promising (Pressler et al., 2015).
Schizophrenia
Schizophrenia is a relatively frequent and complex brain disorder. The risk of manifesting schizophrenia is inheritable in more than 50% of clinical cases (Schwab and Wildenauer, 2013), with environmental factors being an additional determinant. Schizophrenia is often considered as a neurodevelopmental disease, in which the combination of a genetic predisposition with insults during fetal, perinatal or early postnatal stages is required to manifest clinical symptoms (Lewis and Levitt, 2002). Such deleterious insults could be bacterial/viral infection and/or perinatal asphyxia during perinatal stages (Hagberg and Mallard, 2005). Experiments mimicking viral (Hartung et al., 2016) or bacterial (Nimmervoll et al., 2013) infections revealed changes in spontaneous activity patterns and hypoxia-ischemia also alters spontaneous activity in the immature rodent and human brain (Roberts et al., 2014; Ranasinghe et al., 2015; Mordel et al., 2016). Thus disturbances of neuronal activity under these conditions should be considered as an additional possible factor in the etiology of schizophrenia, beside other proposed mechanisms (Lisman et al., 2008). Interestingly, recent results indicate that the expression of the schizophrenia associated gene TCF4, a transcription factor implicated in neuronal development and plasticity, is tightly regulated by neuronal activity and that a point-mutation of this gene found in schizophrenic patients enhances its activity-dependent up-regulation (Sepp et al., 2017).
Recent experiments using mice that express a genetic background found in schizophrenic patients in combination with an intra-uterine insult, in this case a mimeticum for a viral infection, demonstrate that properties of spontaneous activity in the mouse prefrontal cortex changes already at early postnatal stages (Hartung et al., 2016). In line with these animal studies, cell cultures derived from induced pluripotent stem cells of schizophrenic patients exhibit a significant decrease in neuronal connectivity, decreased glutamate receptor expression and altered gene expression (Brennand et al., 2011; Roussos et al., 2016), suggesting that disturbed neuronal activity is an inherent feature of schizophrenia associated mutations. However, whether the altered activity during development only reflects disturbed neuronal connectivity caused by conditions that are associated with schizophrenia or contribute causally to the establishment of the disturbed functional connectivity in schizophrenic brains remains an open question.
Autism Spectrum Disorders
ASD describe a heterogeneous class of disorders associated with social disability, communication impairment, repetitive behaviors and restricted interests (for review in Ecker et al., 2013). The etiology of ASD is complex and enigmatic, but a genetic background as well as environmental insults during early development are discussed (Geschwind and Levitt, 2007). The manifestation of ASD has been linked to prenatal insults such as maternal infections, maternal hormone status or influence of toxins and vitamins, as well as early postnatal insults, like maladaptive caregiver–infant interactions (Mandy and Lai, 2016). Is has been proposed that the complex trajectory of events leading from initial dysfunction to the manifestation of ASD initially involves molecular/cellular alterations causing functional changes (which in-turn alter molecular/cellular properties), suggesting that inappropriate neuronal activity may play an essential role during this process (Ecker et al., 2013).
It has been recently demonstrated that the frequency of GDPs is reduced in the immature hippocampus of BTBR-mice, which represents an animal model of idiopathic autism (Cellot et al., 2016). The same group demonstrated that expression of an ASD-related mutation of the postsynaptic adhesion molecule neuroligin-3 leads to an enhanced frequency of GDPs in the immature hippocampus (Pizzarelli and Cherubini, 2013). Knockout of neuroligin-4, another ASD related member of the neuroligin family, enhances the occurrence and duration of spontaneous spindle bursts in mouse cortex (Unichenko et al., 2017). In summary, these studies illustrate that an adequate amount of spontaneous activity may be the prerequisite for proper neurodevelopment, and both enhanced and reduced activity levels may lead to adverse outcomes.
One unequivocally identified cause for ASD is prenatal exposure of the anticonvulsant valproate (for review Roullet et al., 2013), which again indicates that altered neuronal activity should be considered as a possible factor in the etiology of ASD. Polymorphisms in genes encoding α2-subunit of glycine receptors have also been found in patients affected by ASD (Piton et al., 2011). Since glycine receptors do not considerably contribute to inhibition in the juvenile/mature cortex, this observation suggests that alterations in the glycinergic control of immature neuronal activity (Sava et al., 2014) and/or neuronal migration (Nimmervoll et al., 2011) may contribute to the manifestation of ASD in patients with this mutation. Finally, it is reported that brains from ASD patients often exhibit an excess number of neurons (Courchesne et al., 2011) and that in mice overproduction of cortical neurons causes autism-like disorders (Fang et al., 2014), indicating that enhanced neurogenesis (see “Neurogenesis” section) and/or impaired neuronal apoptosis (see “Neuronal Migration” section) also contribute to the etiology of ASD. In summary, these experimental and clinical findings provide some evidences that disturbances in spontaneous neuronal activity during early developmental stages might be an element that contributes to the etiology of ASD. However, more stringent electrophysiological experiments during early and late postnatal stages are required to reveal whether neuronal activity is a possible decisive factor, which link the diverse genomic and environmental causes to the specific neurophathological symptoms of ASD.
Summary and Conclusion
In this review we provide a body of evidence indicating that spontaneous neuronal activity during early developmental stages is critically involved in a variety of neurodevelopmental processes. Altered neuronal activity seems to be involved in the etiology of neurological and neuropsychiatric diseases. Since the impact of genetic models on spontaneous neuronal activity has only occasionally been monitored, it is possible that several genetic models of neurodevelopmental diseases underestimate the contribution of electrical activity to the etiology of these disorders. In addition, we like to emphasize that our knowledge is rather limited, whether the specific spontaneous activity patterns occurring in a defined spatiotemporal sequence mediate distinct developmental processes. Further experiments, using a combination of electrophysiological, optogenetic and molecular techniques, will be required to address this intriguing question. However, even our limited knowledge on the impact of neuronal activity on early brain development already initiated new therapeutic options for the treatment of neurological and neuropsychiatric diseases and instructed that neurodevelopmental consequences of early pharmacological interventions must be considered.
Author Contributions
SK, AS, OB, J-WY, HJL and WK wrote and corrected the manuscript.
Funding
This review is not based on experiments funded by specified grants, but the authors like to thank the Deutsche Forschungsgemeinschaft (DFG) for their funding of several projects related to the role and consequences of early neuronal activity to HJL, SK and WK.
Conflict of Interest Statement
The authors declare that the research was conducted in the absence of any commercial or financial relationships that could be construed as a potential conflict of interest.
Acknowledgments
We thank all colleagues, who contributed to our understanding of cortical development. We apologize that we were not able to include all relevant publications on this topic due to space limitations. We particularly thank our coworkers and the funding agencies, especially the Deutsche Forschungsgemeinschaft, for their continuous support.
References
Ackman, J. B., and Crair, M. C. (2014). Role of emergent neural activity in visual map development. Curr. Opin. Neurobiol. 24, 166–175. doi: 10.1016/j.conb.2013.11.011
Albrecht, J., Hanganu, I. L., Heck, N., and Luhmann, H. J. (2005). Oxygen and glucose deprivation induces major dysfunction in the somatosensory cortex of the newborn rat. Eur. J. Neurosci. 22, 2295–2305. doi: 10.1111/j.1460-9568.2005.04398.x
Allène, C., Cattani, A., Ackman, J. B., Bonifazi, P., Aniksztejn, L., Ben-Ari, Y., et al. (2008). Sequential generation of two distinct synapse-driven network patterns in developing neocortex. J. Neurosci. 28, 12851–12863. doi: 10.1523/JNEUROSCI.3733-08.2008
An, S., Kilb, W., and Luhmann, H. J. (2014). Sensory-evoked and spontaneous γ and spindle bursts in neonatal rat motor cortex. J. Neurosci. 34, 10870–10883. doi: 10.1523/jneurosci.4539-13.2014
Avila, A., Nguyen, L., and Rigo, J. M. (2013). Glycine receptors and brain development. Front. Cell. Neurosci. 7:184. doi: 10.3389/fncel.2013.00184
Avila, A., Vidal, P. M., Tielens, S., Morelli, G., Laguesse, S., Harvey, R. J., et al. (2014). Glycine receptors control the generation of projection neurons in the developing cerebral cortex. Cell Death Differ. 21, 1696–1708. doi: 10.1038/cdd.2014.75
Bagasrawala, I., Memi, F., Radonjić, N. V., and Zecevic, N. (2017). N-Methyl D-Aspartate receptor expression patterns in the human fetal cerebral cortex. Cereb. Cortex 27, 5041–5053. doi: 10.1093/cercor/bhw289
Bai, X., Yan, Y., Canfield, S., Muravyeva, M. Y., Kikuzchi, C., Zaja, I., et al. (2013). Ketamine enhances human neural stem cell proliferation and induces neuronal apoptosis via reactive oxygen species-mediated mitochondrial pathway. Anesth. Analg. 116, 869–880. doi: 10.1213/ANE.0b013e3182860fc9
Barrera, K., Chu, P., Abramowitz, J., Steger, R., Ramos, R. L., and Brumberg, J. C. (2013). Organization of myelin in the mouse somatosensory barrel cortex and the effects of sensory deprivation. Dev. Neurobiol. 73, 297–314. doi: 10.1002/dneu.22060
Becker, C. M., Betz, H., and Schroder, H. (1993). Expression of inhibitory glycine receptors in postnatal rat cerebral cortex. Brain Res. 606, 220–226. doi: 10.1016/0006-8993(93)90988-y
Behar, T. N., Li, Y. X., Tran, H. T., Ma, W., Dunlap, V., Scott, C., et al. (1996). GABA stimulates chemotaxis and chemokinesis of embryonic cortical neurons via calcium-dependent mechanisms. J. Neurosci. 16, 1808–1818.
Behar, T. N., Schaffner, A. E., Scott, C. A., Greene, C. L., and Barker, J. L. (2000). GABA receptor antagonists modulate postmitotic cell migration in slice cultures of embryonic rat cortex. Cereb. Cortex 10, 899–909. doi: 10.1093/cercor/10.9.899
Behar, T. N., Scott, C. A., Greene, C. L., Wen, X. L., Smith, S. V., Maric, D., et al. (1999). Glutamate acting at NMDA receptors stimulates embryonic cortical neuronal migration. J. Neurosci. 19, 4449–4461.
Belgacem, Y. H., and Borodinsky, L. N. (2015). Inversion of sonic hedgehog action on its canonical pathway by electrical activity. Proc. Natl. Acad. Sci. U S A 112, 4140–4145. doi: 10.1073/pnas.1419690112
Ben-Ari, Y. (2006). Seizures beget seizures: the quest for GABA as a key player. Crit. Rev. Neurobiol. 18, 135–144. doi: 10.1615/critrevneurobiol.v18.i1-2.140
Ben-Ari, Y. (2015). Commentary: GABA depolarizes immature neurons and inhibits network activity in the neonatal neocortex in vivo. Front. Cell. Neurosci. 9:478. doi: 10.3389/fncel.2015.00478
Ben-Ari, Y., Cherubini, E., Corradetti, R., and Gaiarsa, J.-L. (1989). Giant synaptic potentials in immature rat CA3 hippocampal neurones. J. Physiol. 416, 303–325. doi: 10.1113/jphysiol.1989.sp017762
Ben-Ari, Y., Gaiarsa, J. L., Tyzio, R., and Khazipov, R. (2007). GABA: a pioneer transmitter that excites immature neurons and generates primitive oscillations. Physiol. Rev. 87, 1215–1284. doi: 10.1152/physrev.00017.2006
Ben-Ari, Y., and Holmes, G. L. (2006). Effects of seizures on developmental processes in the immature brain. Lancet Neurol. 5, 1055–1063. doi: 10.1016/s1474-4422(06)70626-3
Ben-Ari, Y., and Spitzer, N. C. (2010). Phenotypic checkpoints regulate neuronal development. Trends Neurosci. 33, 485–492. doi: 10.1016/j.tins.2010.08.005
Ben-Ari, Y., Woodin, M. A., Sernagor, E., Cancedda, L., Vinay, L., Rivera, C., et al. (2012). Refuting the challenges of the developmental shift of polarity of GABA actions: GABA more exciting than ever! Front. Cell. Neurosci. 6:35. doi: 10.3389/fncel.2012.00035
Benders, M. J., Palmu, K., Menache, C., Borradori-Tolsa, C., Lazeyras, F., Sizonenko, S., et al. (2015). Early brain activity relates to subsequent brain growth in premature infants. Cereb. Cortex 25, 3014–3024. doi: 10.1093/cercor/bhu097
Berridge, M. J. (1995). Calcium signalling and cell proliferation. Bioessays 17, 491–500. doi: 10.1002/bies.950170605
Bittigau, P., Sifringer, M., Genz, K., Reith, E., Pospischil, D., Govindarajalu, S., et al. (2002). Antiepileptic drugs and apoptotic neurodegeneration in the developing brain. Proc. Natl. Acad. Sci. U S A 99, 15089–15094. doi: 10.1073/pnas.222550499
Bitzenhofer, S. H., Ahlbeck, J., Wolff, A., Wiegert, J. S., Gee, C. E., Oertner, T. G., et al. (2017). Layer-Specific optogenetic activation of pyramidal neurons causes β–γ entrainment of neonatal networks. Nat. Commun. 8:14563. doi: 10.1038/ncomms14563
Bitzenhofer, S. H., Sieben, K., Siebert, K. D., Spehr, M., and Hanganu-Opatz, I. L. (2015). Oscillatory activity in developing prefrontal networks results from theta-γ-modulated synaptic inputs. Cell Rep. 11, 486–497. doi: 10.1016/j.celrep.2015.03.031
Blankenship, A. G., and Feller, M. B. (2010). Mechanisms underlying spontaneous patterned activity in developing neural circuits. Nat. Rev. Neurosci. 11, 18–29. doi: 10.1038/nrn2759
Blanquie, O., Kilb, W., Sinning, A., and Luhmann, H. J. (2017a). Homeostatic interplay between electrical activity and neuronal apoptosis in the developing neocortex. Neuroscience 358, 190–200. doi: 10.1016/j.neuroscience.2017.06.030
Blanquie, O., Liebmann, L., Hübner, C. A., Luhmann, H. J., and Sinning, A. (2017b). NKCC1-mediated GABAergic signaling promotes postnatal cell death in neocortical cajal-retzius cells. Cereb. Cortex 27, 1644–1659. doi: 10.1093/cercor/bhw004
Blanquie, O., Yang, J-W., Kilb, W., Sharopov, S., Sinning, A., and Luhmann, H. J. (2017c). Electrical activity controls area-specific expression of neuronal apoptosis in the mouse developing cerebral cortex. Elife 6:e27696. doi: 10.7554/eLife.27696
Bonetti, C., and Surace, E. M. (2010). Mouse embryonic retina delivers information controlling cortical neurogenesis. PLoS One 5:e15211. doi: 10.1371/journal.pone.0015211
Borodinsky, L. N., Root, C. M., Cronin, J. A., Sann, S. B., Gu, X., and Spitzer, N. C. (2004). Activity-dependent homeostatic specification of transmitter expression in embryonic neurons. Nature 429, 523–530. doi: 10.1038/nature02518
Brennand, K. J., Simone, A., Jou, J., Gelboin-Burkhart, C., Tran, N., Sangar, S., et al. (2011). Modelling schizophrenia using human induced pluripotent stem cells. Nature 473, 221–225. doi: 10.1038/nature09915
Brockmann, M. D., Kukovic, M., Schönfeld, M., Sedlacik, J., and Hanganu-Opatz, I. L. (2013). Hypoxia-ischemia disrupts directed interactions within neonatal prefrontal-hippocampal networks. PLoS One 8:e83074. doi: 10.1371/journal.pone.0083074
Bystron, I., Blakemore, C., and Rakic, P. (2008). Development of the human cerebral cortex: boulder committee revisited. Nat. Rev. Neurosci. 9, 110–122. doi: 10.1038/nrn2252
Cao, C. W., Livesey, J. C., and Halliwell, R. F. (2015). An evaluation of a human stem cell line to identify risk of developmental neurotoxicity with antiepileptic drugs. Toxicol. In Vitro 29, 592–599. doi: 10.1016/j.tiv.2015.01.010
Cellot, G., Maggi, L., Di Castro, M. A., Catalano, M., Migliore, R., Migliore, M., et al. (2016). Premature changes in neuronal excitability account for hippocampal network impairment and autistic-like behavior in neonatal BTBR T+Tf/J mice. Sci. Rep. 7:31696. doi: 10.1038/srep31696
Chao, D. L., Ma, L., and Shen, K. (2009). Transient cell-cell interactions in neural circuit formation. Nat. Rev. Neurosci. 10, 262–271. doi: 10.1038/nrn2594
Chapman, K. E., Raol, Y. H., and Brooks-Kayal, A. (2012). Neonatal seizures: controversies and challenges in translating new therapies from the lab to the isolette. Eur. J. Neurosci. 35, 1857–1865. doi: 10.1111/j.1460-9568.2012.08140.x
Chen, K., Aradi, I., Thon, N., Eghbal-Ahmadi, M., Baram, T. Z., and Soltesz, I. (2001). Persistently modified h-channels after complex febrile seizures convert the seizure-induced enhancement of inhibition to hyperexcitability. Nat. Med. 7, 331–337. doi: 10.1038/85480
Chen, Y., and Ghosh, A. (2005). Regulation of dendritic development by neuronal activity. J. Neurobiol. 64, 4–10. doi: 10.1002/neu.20150
Chen, Z. J., and Yuan, W. (2015). Central plasticity and dysfunction elicited by aural deprivation in the critical period. Front. Neural Circuits 9:26. doi: 10.3389/fncir.2015.00026
Clancy, B., Finlay, B. L., Darlington, R. B., and Anand, K. J. (2007). Extrapolating brain development from experimental species to humans. Neurotoxicology 28, 931–937. doi: 10.1016/j.neuro.2007.01.014
Clause, A., Kim, G., Sonntag, M., Weisz, C. J. C., Vetter, D. E., Rubsamen, R., et al. (2014). The precise temporal pattern of prehearing spontaneous activity is necessary for tonotopic map refinement. Neuron 82, 822–835. doi: 10.1016/j.neuron.2014.04.001
Colonnese, M. T., and Khazipov, R. (2010). “Slow activity transients” in infant rat visual cortex: a spreading synchronous oscillation patterned by retinal waves. J. Neurosci. 30, 4325–4337. doi: 10.1523/JNEUROSCI.4995-09.2010
Corlew, R., Bosma, M. M., and Moody, W. J. (2004). Spontaneous, synchronous electrical activity in neonatal mouse cortical neurones. J. Physiol. 560, 377–390. doi: 10.1113/jphysiol.2004.071621
Courchesne, E., Mouton, P. R., Calhoun, M. E., Semendeferi, K., Ahrens-Barbeau, C., Hallet, M. J., et al. (2011). Neuron number and size in prefrontal cortex of children with autism. JAMA 306, 2001–2010. doi: 10.1001/jama.2011.1638
Crandall, J. E., McCarthy, D. M., Araki, K. Y., Sims, J. R., Ren, J. Q., and Bhide, P. G. (2007). Dopamine receptor activation modulates GABA neuron migration from the basal forebrain to the cerebral cortex. J. Neurosci. 27, 3813–3822. doi: 10.1523/jneurosci.5124-06.2007
Creeley, C. E., Dikranian, K. T., Dissen, G. A., Back, S. A., Olney, J. W., and Brambrink, A. M. (2014). Isoflurane-induced apoptosis of neurons and oligodendrocytes in the fetal rhesus macaque brain. Anesthesiology 120, 626–638. doi: 10.1097/ALN.0000000000000037
Dammerman, R. S., Flint, A. C., Noctor, S., and Kriegstein, A. R. (2000). An excitatory GABAergic plexus in developing neocortical layer 1. J. Neurophysiol. 84, 428–434.
Davidson, A. J., Disma, N., de Graaff, J. C., Withington, D. E., Dorris, L., Bell, G., et al. (2016). Neurodevelopmental outcome at 2 years of age after general anaesthesia and awake-regional anaesthesia in infancy (GAS): an international multicentre, randomised controlled trial. Lancet 387, 239–250. doi: 10.1016/S0140-6736(15)00608-X
De Marco García, N. V., Karayannis, T., and Fishell, G. (2011). Neuronal activity is required for the development of specific cortical interneuron subtypes. Nature 472, 351–356. doi: 10.1038/nature09865
Demarque, M., and Spitzer, N. C. (2012). Neurotransmitter phenotype plasticity: an unexpected mechanism in the toolbox of network activity homeostasis. Devel. Neurobio. 72, 22–32. doi: 10.1002/dneu.20909
Demerens, C., Stankoff, B., Logak, M., Anglade, P., Allinquant, B., Couraud, F., et al. (1996). Induction of myelination in the central nervous system by electrical activity. Proc. Natl. Acad. Sci. U S A 93, 9887–9892. doi: 10.1073/pnas.93.18.9887
Denter, D. G., Heck, N., Riedemann, T., White, R., Kilb, W., and Luhmann, H. J. (2010). GABAC receptors are functionally expressed in the intermediate zone and regulate radial migration in the embryonic mouse neocortex. Neuroscience 167, 124–134. doi: 10.1016/j.neuroscience.2010.01.049
de Villers-Sidani, E., Chang, E. F., Bao, S. W., and Merzenich, M. M. (2007). Critical period window for spectral tuning defined in the primary auditory cortex (A1) in the rat. J. Neurosci. 27, 180–189. doi: 10.1523/JNEUROSCI.3227-06.2007
Dori, A., Cohen, J., Silverman, W. F., Pollack, Y., and Soreq, H. (2005). Functional manipulations of acetylcholinesterase splice variants highlight alternative splicing contributions to murine neocortical development. Cereb. Cortex 15, 419–430. doi: 10.1093/cercor/bhh145
Duan, S., Anderson, C. M., Keung, E. C., Chen, Y., Chen, Y., and Swanson, R. A. (2003). P2X7 receptor-mediated release of excitatory amino acids from astrocytes. J. Neurosci. 23, 1320–1328.
Dubiel, A., and Kulesza, R. J. (2015). Prenatal valproic acid exposure disrupts tonotopic c-Fos expression in the rat brainstem. Neuroscience 311, 349–361. doi: 10.1016/j.neuroscience.2015.10.043
Dupont, E., Hanganu, I. L., Kilb, W., Hirsch, S., and Luhmann, H. J. (2006). Rapid developmental switch in the mechanisms driving early cortical columnar networks. Nature 439, 79–83. doi: 10.1038/nature04264
Durand, G. M., and Konnerth, A. (1996). Long-term potentiation as a mechanism of functional synapse induction in the developing hippocampus. J. Physiol. Paris 90, 313–315. doi: 10.1016/s0928-4257(97)87905-3
Ecker, C., Spooren, W., and Murphy, D. G. M. (2013). Translational approaches to the biology of autism: false dawn or a new era? Mol. Psychiatry 18, 435–442. doi: 10.1038/mp.2012.102
Egorov, A. V., and Draguhn, A. (2013). Development of coherent neuronal activity patterns in mammalian cortical networks: common principles and local hetereogeneity. Mech. Dev. 130, 412–423. doi: 10.1016/j.mod.2012.09.006
Fang, W. Q., Chen, W. W., Jiang, L., Liu, K., Yung, W. H., Fu, A. K., et al. (2014). Overproduction of upper-layer neurons in the neocortex leads to autism-like features in mice. Cell Rep. 9, 1635–1643. doi: 10.1016/j.celrep.2014.11.003
Flavell, S. W., and Greenberg, M. E. (2008). Signaling mechanisms linking neuronal activity to gene expression and plasticity of the nervous system. Annu. Rev. Neurosci. 31, 563–590. doi: 10.1146/annurev.neuro.31.060407.125631
Flint, A. C., Liu, X., and Kriegstein, A. R. (1998). Nonsynaptic glycine receptor activation during early neocortical development. Neuron 20, 43–53. doi: 10.1016/s0896-6273(00)80433-x
Frotscher, M. (1998). Cajal-Retzius cells, Reelin, and the formation of layers. Curr. Opin. Neurobiol. 8, 570–575. doi: 10.1016/s0959-4388(98)80082-2
Furuta, A., Rothstein, J. D., and Martin, L. J. (1997). Glutamate transporter protein subtypes are expressed differentially during rat CNS development. J. Neurosci. 17, 8363–8375.
Galanopoulou, A. S. (2008). GABAA receptors in normal development and seizures: friends or foes? Curr. Neuropharmacol. 6, 1–20. doi: 10.2174/157015908783769653
Galindo, R., Zamudio, P. A., and Valenzuela, C. F. (2005). Alcohol is a potent stimulant of immature neuronal networks: implications for fetal alcohol spectrum disorder. J. Neurochem. 94, 1500–1511. doi: 10.1111/j.1471-4159.2005.03294.x
Garaschuk, O., Linn, J., Eilers, J., and Konnerth, A. (2000). Large-scale oscillatory calcium waves in the immature cortex. Nat. Neurosci. 3, 452–459. doi: 10.1038/74823
Geschwind, D. H., and Levitt, P. (2007). Autism spectrum disorders: developmental disconnection syndromes. Curr. Opin. Neurobiol. 17, 103–111. doi: 10.1016/j.conb.2007.01.009
Ghosh, A., Carnahan, J., and Greenberg, M. E. (1994). Requirement for BDNF in activity-dependent survival of cortical neurons. Science 263, 1618–1623. doi: 10.1126/science.7907431
Glykys, J., Peng, Z., Chandra, D., Homanics, G. E., Houser, C. R., and Mody, I. (2007). A new naturally occurring GABAA receptor subunit partnership with high sensitivity to ethanol. Nat. Neurosci. 10, 40–48. doi: 10.1038/nn1813
Golbs, A., Nimmervoll, B., Sun, J. J., Sava, I. E., and Luhmann, H. J. (2011). Control of programmed cell death by distinct electrical activity patterns. Cereb. Cortex 21, 1192–1202. doi: 10.1093/cercor/bhq200
Goldberg, E. M., and Coulter, D. A. (2013). Mechanisms of epileptogenesis: a convergence on neural circuit dysfunction. Nat. Rev. Neurosci. 14, 337–349. doi: 10.1038/nrn3482
Gu, X., and Spitzer, N. C. (1995). Distinct aspects of neuronal differentiation encoded by frequency of spontaneous Ca2+ transients. Nature 375, 784–787. doi: 10.1038/375784a0
Guizzetti, M., Zhang, X., Goeke, C., and Gavin, D. P. (2014). Glia and neurodevelopment: focus on fetal alcohol spectrum disorders. Front. Pediatr. 2:123. doi: 10.3389/fped.2014.00123
Hagberg, H., and Mallard, C. (2005). Effect of inflammation on central nervous system development and vulnerability. Curr. Opin. Neurol. 18, 117–123. doi: 10.1097/01.wco.0000162851.44897.8f
Hanganu, I. L., Ben-Ari, Y., and Khazipov, R. (2006). Retinal waves trigger spindle bursts in the neonatal rat visual cortex. J. Neurosci. 26, 6728–6736. doi: 10.1523/JNEUROSCI.0752-06.2006
Hanganu, I. L., and Luhmann, H. J. (2004). Functional nicotinic acetylcholine receptors on subplate neurons in neonatal rat somatosensory cortex. J. Neurophysiol. 92, 189–198. doi: 10.1152/jn.00010.2004
Hanganu, I. L., Okabe, A., Lessmann, V., and Luhmann, H. J. (2009). Cellular mechanisms of subplate-driven and cholinergic input-dependent network activity in the neonatal rat somatosensory cortex. Cereb. Cortex 19, 89–105. doi: 10.1093/cercor/bhn061
Hanganu, I. L., Staiger, J. F., Ben-Ari, Y., and Khazipov, R. (2007). Cholinergic modulation of spindle bursts in the neonatal rat visual cortex in vivo. J. Neurosci. 27, 5694–5705. doi: 10.1523/JNEUROSCI.5233-06.2007
Hanson, M. G., and Landmesser, L. T. (2004). Normal patterns of spontaneous activity are required for correct motor axon guidance and the expression of specific guidance molecules. Neuron 43, 687–701. doi: 10.1016/j.neuron.2004.08.018
Hardingham, G. E., and Bading, H. (2010). Synaptic versus extrasynaptic NMDA receptor signalling: implications for neurodegenerative disorders. Nat. Rev. Neurosci. 11, 682–696. doi: 10.1038/nrn2911
Hartung, H., Cichon, N., De Feo, V., Riemann, S., Schildt, S., Lindemann, C., et al. (2016). From shortage to surge: a developmental switch in hippocampal-prefrontal coupling in a gene-environment model of neuropsychiatric disorders. Cereb. Cortex 26, 4265–4281. doi: 10.1093/cercor/bhw274
Hasel, P., Dando, O., Jiwaji, Z., Baster, P., Todd, A. C., Heron, S., et al. (2017). Neurons and neuronal activity control gene expression in astrocytes to regulate their development and metabolism. Nat. Commun. 8:15132. doi: 10.1038/ncomms15132
Hata, Y., and Stryker, M. P. (1994). Control of thalamocortical afferent rearrangement by postsynaptic activity in developing visual cortex. Science 265, 1732–1735. doi: 10.1126/science.8085163
Haydar, T. F., Wang, F., Schwartz, M. L., and Rakic, P. (2000). Differential modulation of proliferation in the neocortical ventricular and subventricular zones. J. Neurosci. 20, 5764–5774.
Heck, N., Golbs, A., Riedemann, T., Sun, J. J., Lessmann, V., and Luhmann, H. J. (2008). Activity-dependent regulation of neuronal apoptosis in neonatal mouse cerebral cortex. Cereb. Cortex 18, 1335–1349. doi: 10.1093/cercor/bhm165
Heck, N., Kilb, W., Reiprich, P., Kubota, H., Furukawa, T., Fukuda, A., et al. (2007). GABA-A receptors regulate neocortical neuronal migration in vitro and in vivo. Cereb. Cortex 17, 138–148. doi: 10.1093/cercor/bhj135
Hirai, K., Yoshioka, H., Kihara, M., Hasegawa, K., Sakamoto, T., Sawada, T., et al. (1999). Inhibiting neuronal migration by blocking NMDA receptors in the embryonic rat cerebral cortex: a tissue culture study. Dev. Brain Res. 114, 63–67. doi: 10.1016/s0165-3806(99)00019-x
Holmes, G. L., Gairsa, J. L., Chevassus, L., and Ben-Ari, Y. (1998). Consequences of neonatal seizures in the rat: morphological and behavioral effects. Ann. Neurol. 44, 845–857. doi: 10.1002/ana.410440602
Hubel, D. H., and Wiesel, T. N. (1970). The period of susceptibility to the physiological effects of unilateral eye closure in kittens. J. Physiol. 206, 419–436. doi: 10.1113/jphysiol.1970.sp009022
Huberman, A. D., Speer, C. M., and Chapman, B. (2006). Spontaneous retinal activity mediates development of ocular dominance columns and binocular receptive fields in v1. Neuron 52, 247–254. doi: 10.1016/j.neuron.2006.07.028
Ikonomidou, C., Bittigau, P., Ishimaru, M. J., Wozniak, D. F., Koch, C., Genz, K., et al. (2000). Ethanol-induced apoptotic neurodegeneration and fetal alcohol syndrome. Science 287, 1056–1060. doi: 10.1126/science.287.5455.1056
Ikonomidou, C., Bosch, F., Miksa, M., Bittigau, P., Vöckler, J., Dikranian, K., et al. (1999). Blockade of NMDA receptors and apoptotic neurodegeneration in the developing brain. Science 283, 70–74. doi: 10.1126/science.283.5398.70
Ikonomidou, C., and Turski, L. (2010). Antiepileptic drugs and brain development. Epilepsy Res. 88, 11–22. doi: 10.1016/j.eplepsyres.2009.09.019
Isaeva, E., Isaev, D., Khazipov, R., and Holmes, G. L. (2006). Selective impairment of GABAergic synaptic transmission in the flurothyl model of neonatal seizures. Eur. J. Neurosci. 23, 1559–1566. doi: 10.1111/j.1460-9568.2006.04693.x
Isaeva, E., Isaev, D., Savrasova, A., Khazipov, R., and Holmes, G. L. (2010). Recurrent neonatal seizures result in long-term increases in neuronal network excitability in the rat neocortex. Eur. J. Neurosci. 31, 1446–1455. doi: 10.1111/j.1460-9568.2010.07179.x
Ito, H., Uchida, T., and Makita, K. (2015). Ketamine causes mitochondrial dysfunction in human induced pluripotent stem cell-derived neuron. PLoS One 10:e0128445. doi: 10.1371/journal.pone.0128445
Iyer, K. K., Roberts, J. A., Hellstrom-Westas, L., Wikström, S., Hansen-Pupp, I., Ley, D., et al. (2015). Cortical burst dynamics predict clinical outcome early in extremely preterm infants. Brain 138, 2206–2218. doi: 10.1093/brain/awv129
Janiesch, P. C., Krüger, H. S., Pöschel, B., and Hanganu-Opatz, I. L. (2011). Cholinergic control in developing prefrontal-hippocampal networks. J. Neurosci. 31, 17955–17970. doi: 10.1523/JNEUROSCI.2644-11.2011
Jevtovic-Todorovic, V., Hartman, R. E., Izumi, Y., Benshoff, N. D., Dikranian, K., Zorumski, C. F., et al. (2003). Early exposure to common anesthetic agents causes widespread neurodegeneration in the developing rat brain and persistent learning deficits. J. Neurosci. 23, 876–882.
Jursky, F., and Nelson, N. (1996). Developmental expression of the glycine transporters GLYT1 and GLYT2 in mouse brain. J. Neurochem. 67, 336–344. doi: 10.1046/j.1471-4159.1996.67010336.x
Kanold, P. O., and Luhmann, H. J. (2010). The subplate and early cortical circuits. Annu. Rev. Neurosci. 33, 23–48. doi: 10.1146/annurev-neuro-060909-153244
Khalid, O., Kim, J. J., Kim, H. S., Hoang, M., Tu, T. G., Elie, O., et al. (2014). Gene expression signatures affected by alcohol-induced DNA methylomic deregulation in human embryonic stem cells. Stem Cell Res. 12, 791–806. doi: 10.1016/j.scr.2014.03.009
Khalilov, I., Holmes, G. L., and Ben-Ari, Y. (2003). In vitro formation of a secondary epileptogenic mirror focus by interhippocampal propagation of seizures. Nat. Neurosci. 6, 1079–1085. doi: 10.1038/nn1125
Khazipov, R., Minlebaev, M., and Valeeva, G. (2013). Early γ oscillations. Neuroscience 250, 240–252. doi: 10.1016/j.neuroscience.2013.07.019
Khazipov, R., Sirota, A., Leinekugel, X., Holmes, G. L., Ben-Ari, Y., and Buzsáki, G. (2004). Early motor activity drives spindle bursts in the developing somatosensory cortex. Nature 432, 758–761. doi: 10.1038/nature03132
Kihara, M., Yoshioka, H., Hirai, K., Hasegawa, K., Kizaki, Z., and Sawada, T. (2002). Stimulation of N-methyl-D-aspartate (NMDA) receptors inhibits neuronal migration in embryonic cerebral cortex: a tissue culture study. Dev. Brain Res. 138, 195–198. doi: 10.1016/s0165-3806(02)00490-x
Kilb, W. (2011). Development of the GABAergic system from birth to adolescence. Neuroscientist 18, 613–630. doi: 10.1177/1073858411422114
Kilb, W., and Frotscher, M. (2016). Cajal-retzius cells: organizers of cortical development. e-Neuroforum 7, 82–88. doi: 10.1007/s13295-016-0031-5
Kilb, W., and Fukuda, A. (2017). Taurine as an essential neuromodulator during perinatal cortical development. Front. Cell. Neurosci. 11:328. doi: 10.3389/fncel.2017.00328
Kilb, W., Ikeda, M., Uchida, K., Okabe, A., Fukuda, A., and Luhmann, H. J. (2002). Depolarizing glycine responses in Cajal-Retzius cells of neonatal rat cerebral cortex. Neuroscience 112, 299–307. doi: 10.1016/s0306-4522(02)00071-4
Kilb, W., Hartmann, D., Saftig, P., and Luhmann, H. J. (2004). Altered morphological and electrophysiological properties of Cajal-Retzius cells in cerebral cortex of embryonic Presenilin-1 knockout mice. Eur. J. Neurosci. 20, 2749–2756. doi: 10.1111/j.1460-9568.2004.03732.x
Kilb, W., Kirischuk, S., and Luhmann, H. J. (2013). Role of tonic GABAergic currents during pre- and early postnatal rodent development. Front. Neural Circuits 7:139. doi: 10.3389/fncir.2013.00139
Kimelberg, H. K., Goderie, S. K., Higman, S., Pang, S., and Waniewski, R. A. (1990). Swelling-induced release of glutamate, aspartate and taurine from astrocyte cultures. J. Neurosci. 10, 1583–1591.
Kirischuk, S., Luhmann, H. J., and Kilb, W. (2014). Cajal-retzius cells: update on structural and functional properties of these mystic neurons that bridged the 20th century. Neuroscience 275, 33–46. doi: 10.1016/j.neuroscience.2014.06.009
Kirkby, L. A., Sack, G. S., Firl, A., and Feller, M. B. (2013). A role for correlated spontaneous activity in the assembly of neural circuits. Neuron 80, 1129–1144. doi: 10.1016/j.neuron.2013.10.030
Kirmse, K., Kummer, M., Kovalchuk, Y., Witte, O. W., Garaschuk, O., and Holthoff, K. (2015). GABA depolarizes immature neurons and inhibits network activity in the neonatal neocortex in vivo. Nat. Commun. 6:7750. doi: 10.1038/ncomms8750
Komuro, H., and Rakic, P. (1996). Intracellular Ca2+ fluctuations modulate the rate of neuronal migration. Neuron 17, 275–285. doi: 10.1016/s0896-6273(00)80159-2
Kotak, V. C., Sadahiro, M., and Fall, C. P. (2007). Developmental expression of endogenous oscillations and waves in the auditory cortex involves calcium, gap junctions, and GABA. Neuroscience 146, 1629–1639. doi: 10.1016/j.neuroscience.2007.03.039
Kuan, C. Y., Roth, K. A., Flavell, R. A., and Rakic, P. (2000). Mechanisms of programmed cell death in the developing brain. Trends Neurosci. 23, 291–297. doi: 10.1016/s0166-2236(00)01581-2
Kumada, T., Lakshmana, M. K., and Komuro, H. (2006). Reversal of neuronal migration in a mouse model of fetal alcohol syndrome by controlling second-messenger signalings. J. Neurosci. 26, 742–756. doi: 10.1523/JNEUROSCI.4478-05.2006
Lacoste, B., Comin, C. H., Ben Zvi, A., Kaeser, P. S., Xu, X. Y., Costa, L. D., et al. (2014). Sensory-related neural activity regulates the structure of vascular networks in the cerebral cortex. Neuron 83, 1117–1130. doi: 10.1016/j.neuron.2014.07.034
Larsson, O. M., Drejer, J., Kvamme, E., Svenneby, G., Hertz, L., and Schousboe, A. (1985). Ontogenetic development of glutamate and GABA metabolizing enzymes in cultured cerebral cortex interneurons and in cerebral cortex in vivo. Int. J. Dev. Neurosci. 3, 177–185. doi: 10.1016/0736-5748(85)90008-5
Laurie, D. J., Wisden, W., and Seeburg, P. H. (1992). The distribution of thirteen GABAA receptor subunit mRNAs in the rat brain. III. Embryonic and postnatal development. J. Neurosci. 12, 4151–4172.
Lebedeva, J., Zakharov, A., Ogievetsky, E., Minlebaeva, A., Kurbanov, R., Gerasimova, E., et al. (2017). Inhibition of cortical activity and apoptosis caused by ethanol in neonatal rats in vivo. Cereb. Cortex 27, 1068–1082. doi: 10.1093/cercor/bhv293
Lebedeva, Y. A., Zakharova, A. V., Sitdikova, G. F., Zefirov, A. L., and Khazipov, R. N. (2016). Ketamine-midazolam anesthesia induces total inhibition of cortical activity in the brain of newborn rats. Bull. Exp. Biol. Med. 161, 15–19. doi: 10.1007/s10517-016-3334-1
Leinekugel, X., Medina, I., Khalilov, I., Ben-Ari, Y., and Khazipov, R. (1997). Ca2+ oscillations mediated by the synergistic excitatory actions of GABAA and NMDA receptors in the neonatal hippocampus. Neuron 18, 243–255. doi: 10.1016/s0896-6273(00)80265-2
Léveillé, F., Papadia, S., Fricker, M., Bell, K. F., Soriano, F. X., Martel, M. A., et al. (2010). Suppression of the intrinsic apoptosis pathway by synaptic activity. J. Neurosci. 30, 2623–2635. doi: 10.1523/JNEUROSCI.5115-09.2010
Levitt, P., and Moore, R. Y. (1979). Development of the noradrenergic innervation of neocortex. Brain Res. 162, 243–259. doi: 10.1016/0006-8993(79)90287-7
Levy, L. M., Warr, O., and Attwell, D. (1998). Stoichiometry of the glial glutamate transporter GLT-1 expressed inducibly in a Chinese hamster ovary cell line selected for low endogenous Na+-dependent glutamate uptake. J. Neurosci. 18, 9620–9628.
Lewis, D. A., and Levitt, P. (2002). Schizophrenia as a disorder of neurodevelopment. Annu. Rev. Neurosci. 25, 409–432. doi: 10.1146/annurev.neuro.25.112701.142754
Lipton, S. A., and Kater, S. B. (1989). Neurotransmitter regulation of neuronal outgrowth, plasticity and survival. Trends Neurosci. 12, 265–270. doi: 10.1016/0166-2236(89)90026-x
Lisman, J. E., Coyle, J. T., Green, R. W., Javitt, D. C., Benes, F. M., Heckers, S., et al. (2008). Circuit-based framework for understanding neurotransmitter and risk gene interactions in schizophrenia. Trends Neurosci. 31, 234–242. doi: 10.1016/j.tins.2008.02.005
López-Bendito, G., Shigemoto, R., Kulik, A., Paulsen, O., Fairén, A., and Luján, R. (2002). Expression and distribution of metabotropic GABA receptor subtypes GABA-BR1 and GABA-BR2 during rat neocortical development. Eur. J. Neurosci. 15, 1766–1778. doi: 10.1046/j.1460-9568.2002.02032.x
Lotfullina, N., and Khazipov, R. (2017). Ethanol and the developing brain: inhibition of neuronal activity and neuroapoptosis. Neuroscientist doi: 10.1177/1073858417712667 [Epub ahead of print].
LoTurco, J. J., Owens, D. F., Heath, M. J., Davis, M. B., and Kriegstein, A. R. (1995). GABA and glutamate depolarize cortical progenitor cells and inhibit DNA synthesis. Neuron 15, 1287–1298. doi: 10.1016/0896-6273(95)90008-x
Lovinger, D. M., White, G., and Weight, F. F. (1989). Ethanol inhibits NMDA-activated ion current in hippocampal neurons. Science 243, 1721–1724. doi: 10.1126/science.2467382
Luhmann, H. J. (2003). “Cajal-retzius and subplate cells,” in Comprehensive Developmental Neuroscience: Cellular Migration and Formation of Neuronal Connections, 2nd Edn. ed. J. L. R. Rubenstein (Amsterdam, NL: Elsevier), 843–856.
Luhmann, H. J. (2017). Review of imaging network activities in developing rodent cerebral cortex in vivo. Neurophotonics 4:031202. doi: 10.1117/1.NPh.4.3.031202
Luhmann, H. J., Fukuda, A., and Kilb, W. (2015). Control of cortical neuronal migration by glutamate and GABA. Front. Cell. Neurosci. 9:4. doi: 10.3389/fncel.2015.00004
Luhmann, H. J., and Khazipov, R. (2017). Neuronal activity patterns in the developing barrel cortex. Neuroscience doi: 10.1016/j.neuroscience.2017.05.025 [Epub ahead of print].
Luhmann, H. J., Kilb, W., and Hanganu-Opatz, I. L. (2009). Subplate cells: amplifiers of neuronal activity in the developing cerebral cortex. Front. Neuroanat. 3:19. doi: 10.3389/neuro.05.019.2009
Luhmann, H. J., Kirischuk, S., Sinning, A., and Kilb, W. (2014). Early GABAergic circuitry in the cerebral cortex. Curr. Opin. Neurobiol. 26, 72–78. doi: 10.1016/j.conb.2013.12.014
Luhmann, H. J., and Kral, T. (1997). Hypoxia-induced dysfunction in developing rat neocortex. J. Neurophysiol. 78, 1212–1221.
Luhmann, H. J., Sinning, A., Yang, J. W., Reyes-Puerta, V., Stüttgen, M. C., Kirischuk, S., et al. (2016). Spontaneous neuronal activity in developing neocortical networks: from single cells to large-scale interactions. Front. Neural Circuits 10:40. doi: 10.3389/fncir.2016.00040
Luján, R., Shigemoto, R., and López-Bendito, G. (2005). Glutamate and GABA receptor signalling in the developing brain. Neuroscience 130, 567–580. doi: 10.1016/j.neuroscience.2004.09.042
Ma, W., Maric, D., Li, B. S., Hu, Q., Andreadis, J. D., Grant, G. M., et al. (2000). Acetylcholine stimulates cortical precursor cell proliferation in vitro via muscarinic receptor activation and MAP kinase phosphorylation. Eur. J. Neurosci. 12, 1227–1240. doi: 10.1046/j.1460-9568.2000.00010.x
Malk, K., Metsaranta, M., and Vanhatalo, S. (2014). Drug effects on endogenous brain activity in preterm babies. Brain Dev. 36, 116–123. doi: 10.1016/j.braindev.2013.01.009
Malosio, M. L., Marquèze-Pouey, B., Kuhse, J., and Betz, H. (1991). Widespread expression of glycine receptor subunit mRNAs in the adult and developing rat brain. EMBO J. 10, 2401–2409.
Mandy, W., and Lai, M. C. (2016). Annual research review: the role of the environment in the developmental psychopathology of autism spectrum condition. J. Child Psychol. Psychiatry 57, 271–292. doi: 10.1111/jcpp.12501
Manent, J. B., Demarque, M., Jorquera, I., Pellegrino, C., Ben-Ari, Y., Aniksztejn, L., et al. (2005). A noncanonical release of GABA and glutamate modulates neuronal migration. J. Neurosci. 25, 4755–4765. doi: 10.1523/jneurosci.0553-05.2005
Manent, J. B., Jorquera, I., Franco, V., Ben-Ari, Y., Perucca, E., and Represa, A. (2008). Antiepileptic drugs and brain maturation: fetal exposure to lamotrigine generates cortical malformations in rats. Epilepsy Res. 78, 131–139. doi: 10.1016/j.eplepsyres.2007.10.014
Manent, J. B., Jorquera, I., Mazzucchelli, I., Depaulis, A., Perucca, E., Ben-Ari, Y., et al. (2007). Fetal exposure to GABA-acting antiepileptic drugs generates hippocampal and cortical dysplasias. Epilepsia 48, 684–693. doi: 10.1111/j.1528-1167.2007.01056.x
Marcotti, W., Johnson, S. L., Holley, M. C., and Kros, C. J. (2003). Developmental changes in the expression of potassium currents of embryonic, neonatal and mature mouse inner hair cells. J. Physiol. 548, 383–400. doi: 10.1111/j.1469-7793.2003.00383.x
Marguet, S. L., Le-Schulte, V. T. Q., Merseburg, A., Neu, A., Eichler, R., Jakovcevski, I., et al. (2015). Treatment during a vulnerable developmental period rescues a genetic epilepsy. Nat. Med. 21, 1436–1446. doi: 10.1038/nm.3987
Marín-Padilla, M. (1998). Cajal-Retzius cells and the development of the neocortex. Trends Neurosci. 21, 64–71. doi: 10.1016/s0166-2236(97)01164-8
McQuillen, P. S., and Ferriero, D. M. (2005). Perinatal subplate neuron injury: implications for cortical development and plasticity. Brain Pathol. 15, 250–260. doi: 10.1111/j.1750-3639.2005.tb00528.x
Milh, M., Kaminska, A., Huon, C., Lapillonne, A., Ben-Ari, Y., and Khazipov, R. (2007). Rapid cortical oscillations and early motor activity in premature human neonate. Cereb. Cortex 17, 1582–1594. doi: 10.1093/cercor/bhl069
Millar, L. J., Shi, L., Hoerder-Suabedissen, A., and Molnár, Z. (2017). Neonatal hypoxia ischaemia: mechanisms, models and therapeutic challenges. Front. Cell. Neurosci. 8:78. doi: 10.3389/fncel.2017.00078
Minelli, A., Barbaresi, P., and Conti, F. (2003). Postnatal development of high-affinity plasma membrane GABA transporters GAT-2 and GAT-3 in the rat cerebral cortex. Dev. Brain Res. 142, 7–18. doi: 10.1016/s0165-3806(03)00007-5
Minlebaev, M., Ben-Ari, Y., and Khazipov, R. (2007). Network mechanisms of spindle-burst oscillations in the neonatal rat barrel cortex in vivo. J. Neurophysiol. 97, 692–700. doi: 10.1152/jn.00759.2006
Minlebaev, M., Colonnese, M., Tsintsadze, T., Sirota, A., and Khazipov, R. (2011). Early γ oscillations synchronize developing thalamus and cortex. Science 334, 226–229. doi: 10.1126/science.1210574
Molnár, Z., López-Bendito, G., Small, J., Partridge, L. D., Blakemore, C., and Wilson, M. C. (2002). Normal development of embryonic thalamocortical connectivity in the absence of evoked synaptic activity. J. Neurosci. 22, 10313–10323.
Monyer, H., Burnashev, N., Laurie, D. J., Sakmann, B., and Seeburg, P. H. (1994). Developmental and regional expression in the rat brain and functional properties of four NMDA receptors. Neuron 12, 529–540. doi: 10.1016/0896-6273(94)90210-0
Monyer, H., Seeburg, P. H., and Wisden, W. (1991). Glutamate-operated channels: developmentally early and mature forms arise by alternative splicing. Neuron 6, 799–810. doi: 10.1016/0896-6273(91)90176-z
Mordel, J., Sheikh, A., Tsohataridis, S., Kanold, P. O., Zehendner, C. M., and Luhmann, H. J. (2016). Mild systemic inflammation and moderate hypoxia transiently alter neuronal excitability in mouse somatosensory cortex. Neurobiol. Dis. 88, 29–43. doi: 10.1016/j.nbd.2015.12.019
Muralidharan, P., Sarmah, S., Zhou, F. C., and Marrs, J. A. (2013). Fetal alcohol spectrum disorder (FASD) associated neural defects: complex mechanisms and potential therapeutic targets. Brain Sci. 3, 964–991. doi: 10.3390/brainsci3020964
Murase, S., Owens, D. F., and McKay, R. D. (2011). In the newborn hippocampus, neurotrophin-dependent survival requires spontaneous activity and integrin signaling. J. Neurosci. 31, 7791–7800. doi: 10.1523/JNEUROSCI.0202-11.2011
Namiki, S., Norimoto, H., Kobayashi, C., Nakatani, K., Matsuki, N., and Ikegaya, Y. (2013). Layer III neurons control synchronized waves in the immature cerebral cortex. J. Neurosci. 33, 987–1001. doi: 10.1523/JNEUROSCI.2522-12.2013
Naqui, S. Z. H., Harris, B. S., Thomaidou, D., and Parnavelas, J. G. (1999). The noradrenergic system influences the fate of cajal-retzius cells in the developing cerebral cortex. Dev. Brain Res. 113, 75–82. doi: 10.1016/s0165-3806(99)00003-6
Nash, R., Krishnamoorthy, M., Jenkins, A., and Csete, M. (2012). Human embryonic stem cell model of ethanol-mediated developmental toxicity. Exp. Neurol. 234, 127–135. doi: 10.1016/j.expneurol.2011.12.022
Nicol, X., Voyatzis, S., Muzerelle, A., Narboux-Nême, N., Südhof, T. C., Miles, R., et al. (2007). cAMP oscillations and retinal activity are permissive for ephrin signaling during the establishment of the retinotopic map. Nat. Neurosci. 10, 340–347. doi: 10.1038/nn1842
Nimmervoll, B., Denter, D. G., Sava, I., Kilb, W., and Luhmann, H. J. (2011). Glycine receptors influence radial migration in the embryonic mouse neocortex. Neuroreport 22, 509–513. doi: 10.1097/WNR.0b013e328348aafe
Nimmervoll, B., White, R., Yang, J. W., An, S., Henn, C., Sun, J. J., et al. (2013). LPS-induced microglial secretion of TNF-α increases activity-dependent neuronal apoptosis in neonatal cerebral cortex. Cereb. Cortex 23, 1742–1755. doi: 10.1093/cercor/bhs156
O’Leary, J. D., and Warner, D. O. (2017). What do recent human studies tell us about the association between anaesthesia in young children and neurodevelopmental outcome? Br. J. Anaesth. 119, 458–464. doi: 10.1093/bja/aex141
Okabe, A., Kilb, W., Shimizu-Okabe, C., Hanganu, I. L., Fukuda, A., and Luhmann, H. J. (2004). Homogenous glycine receptor expression in cortical plate neurons and cajal-retzius cells of neonatal rat cerebral cortex. Neuroscience 123, 715–724. doi: 10.1016/j.neuroscience.2003.10.014
Okusa, C., Oeschger, F., Ginet, V., Wang, W. Z., Hoerder-Suabedissen, A., Matsuyama, T., et al. (2014). Subplate in a rat model of preterm hypoxia-ischemia. Ann. Clin. Transl. Neurol. 1, 679–691. doi: 10.1002/acn3.97
Olney, J. W. (2014). Focus on apoptosis to decipher how alcohol and many other drugs disrupt brain development. Front. Pediatr. 2:81. doi: 10.3389/fped.2014.00081
Olney, J. W., Wozniak, D. F., Farber, N. B., Jevtovic-Todorovic, V., Bittigau, P., and Ikonomidou, C. (2002). The enigma of fetal alcohol neurotoxicity. Ann. Med. 34, 109–119. doi: 10.1080/07853890252953509
Owens, D. F., Flint, A. C., Dammerman, R. S., and Kriegstein, A. R. (2000). Calcium dynamics of neocortical ventricular zone cells. Dev. Neurosci. 22, 25–33. doi: 10.1159/000017424
Owens, D. F., and Kriegstein, A. R. (2002). Developmental neurotransmitters? Neuron 36, 989–991. doi: 10.1016/S0896-6273(02)01136-4
Owens, D. F., Liu, X. L., and Kriegstein, A. R. (1999). Changing properties of GABAA receptor-mediated signaling during early neocortical development. J. Neurophysiol. 82, 570–583.
Pisani, F., Facini, C., Pavlidis, E., Spagnoli, C., and Boylan, G. (2015). Epilepsy after neonatal seizures: literature review. Eur. J. Paediatr. Neurol. 19, 6–14. doi: 10.1016/j.ejpn.2014.10.001
Piton, A., Gauthier, J., Hamdan, F. F., Lafrenière, R. G., Yang, Y., Henrion, E., et al. (2011). Systematic resequencing of X-chromosome synaptic genes in autism spectrum disorder and schizophrenia. Mol. Psychiatry 16, 867–880. doi: 10.1038/mp.2010.54
Pizzarelli, R., and Cherubini, E. (2013). Developmental regulation of GABAergic signalling in the hippocampus of neuroligin 3 R451C knock-in mice: an animal model of autism. Front. Cell. Neurosci. 7:85. doi: 10.3389/fncel.2013.00085
Popovik, E., and Haynes, L. W. (2000). Survival and mitogenesis of neuroepithelial cells are influenced by noradrenergic but not cholinergic innervation in cultured embryonic rat neopallium. Brain Res. 853, 227–235. doi: 10.1016/s0006-8993(99)02242-8
Pressler, R. M., Boylan, G. B., Marlow, N., Blennow, M., Chiron, C., Cross, J. H., et al. (2015). Bumetanide for the treatment of seizures in newborn babies with hypoxic ischaemic encephalopathy (NEMO): an open-label, dose finding, and feasibility phase 1/2 trial. Lancet Neurol. 14, 469–477. doi: 10.1016/s1474-4422(14)70303-5
Rahkonen, P., Nevalainen, P., Lauronen, L., Pihko, E., Lano, A., Vanhatalo, S., et al. (2013). Cortical somatosensory processing measured by magnetoencephalography predicts neurodevelopment in extremely low-gestational-age infants. Pediatr. Res. 73, 763–771. doi: 10.1038/pr.2013.46
Rakhade, S. N., and Jensen, F. E. (2009). Epileptogenesis in the immature brain: emerging mechanisms. Nat. Rev. Neurol. 5, 380–391. doi: 10.1038/nrneurol.2009.80
Ranasinghe, S., Or, G., Wang, E. Y., Ievins, A., McLean, M. A., Niell, C. M., et al. (2015). Reduced cortical activity impairs development and plasticity after neonatal hypoxia ischemia. J. Neurosci. 35, 11946–11959. doi: 10.1523/JNEUROSCI.2682-14.2015
Reiprich, P., Kilb, W., and Luhmann, H. J. (2005). Neonatal NMDA receptor blockade disturbs neuronal migration in rat somatosensory cortex in vivo. Cereb. Cortex 15, 349–358. doi: 10.1093/cercor/bhh137
Richerson, G. B., and Wu, Y. (2003). Dynamic equilibrium of neurotransmitter transporters: not just for reuptake anymore. J. Neurophysiol. 90, 1363–1374. doi: 10.1152/jn.00317.2003
Roberts, J. A., Iyer, K. K., Finnigan, S., Vanhatalo, S., and Breakspear, M. (2014). Scale-free bursting in human cortex following hypoxia at birth. J. Neurosci. 34, 6557–6572. doi: 10.1523/JNEUROSCI.4701-13.2014
Roullet, F. I., Lai, J. K. Y., and Foster, J. A. (2013). in utero exposure to valproic acid and autism—a current review of clinical and animal studies. Neurotoxicol. Teratol. 36, 47–56. doi: 10.1016/j.ntt.2013.01.004
Roussos, P., Guennewig, B., Kaszorowski, D. C., Barry, G., and Brennard, K. J. (2016). Activity-dependent changes in gene expression in schizophrenia human-induced pluripotent stem cell neurons. JAMA Psychiatry 73, 1180–1188. doi: 10.1001/jamapsychiatry.2016.2575
Ruijter, J. M., Baker, R. E., De Jong, B. M., and Romijn, H. J. (1991). Chronic blockade of bioelectric activity in neonatal rat cortex grown in vitro: morphological effects. Int. J. Dev. Neurosci. 9, 331–338. doi: 10.1016/0736-5748(91)90054-p
Sanchez, R. M., and Jensen, F. E. (2001). Maturational aspects of epilepsy mechanisms and consequences for the immature brain. Epilepsia 42, 577–585. doi: 10.1046/j.1528-1157.2001.12000.x
Santella, L. (1998). The role of calcium in the cell cycle: facts and hypotheses. Biochem. Biophys. Res. Commun. 244, 317–324. doi: 10.1006/bbrc.1998.8086
Sava, B. A., Chen, R. Q., Sun, H. Y., Luhmann, H. J., and Kilb, W. (2014). Taurine activates GABAergic networks in the neocortex of immature mice. Front. Cell. Neurosci. 8:26. doi: 10.3389/fncel.2014.00026
Savtchenko, L., Megalogeni, M., Rusakov, D. A., Walker, M. C., and Pavlov, I. (2015). Synaptic GABA release prevents GABA transporter type-1 reversal during excessive network activity. Nat. Commun. 6:6597. doi: 10.1038/ncomms7597
Schachtner, S. C. (2017). “Antiseizure drugs: mechanism of action, pharmacology, and adverse effects,” in UpToDate, ed. P. Garcia (Waltham, MA: UpToDate Inc.). Available online at: http://www.uptodate.com. [Accessed on October 21, 2017].
Schambra, U. B., Sulik, K. K., Petrusz, P., and Lauder, J. M. (1989). Ontogeny of cholinergic neurons in the mouse forebrain. J. Comp. Neurol. 288, 101–122. doi: 10.1002/cne.902880109
Schlumpf, M., Richards, J. G., Lichtensteiger, W., and Möhler, H. (1983). An autoradiographic study of the prenatal development of benzodiazepine-binding sites in rat brain. J. Neurosci. 3, 1478–1487.
Schonfeld-Dado, E., and Segal, M. (2009). Activity-dependent survival of neurons in culture: a model of slow neurodegeneration. J. Neural. Transm. 116, 1363–1369. doi: 10.1007/s00702-009-0256-3
Schuurmans, C., Armant, O., Nieto, M., Stenman, J. M., Britz, O., Klenin, N., et al. (2004). Sequential phases of cortical specification involve Neurogenin-dependent and -independent pathways. EMBO J. 23, 2892–2902. doi: 10.1038/sj.emboj.7600278
Schwab, S. G., and Wildenauer, D. B. (2013). Genetics of psychiatric disorders in the GWAS era: an update on schizophrenia. Eur. Arch. Psychiatry Clin. Neurosci. 263, S147–S154. doi: 10.1007/s00406-013-0450-z
Sepp, M., Vihma, H., Nurm, K., Urb, M., Page, S. C., Roots, K., et al. (2017). The intellectual disability and schizophrenia associated transcription factor TCF4 is regulated by neuronal activity and protein kinase A. J. Neurosci. 37, 10516–10527. doi: 10.1523/JNEUROSCI.1151-17.2017
Sohn, H. M., Kim, H. Y., Park, S., Han, S. H., and Kim, J. H. (2017). Isoflurane decreases proliferation and differentiation, but none of the effects persist in human embryonic stem cell-derived neural progenitor cells. J. Anesth. 31, 36–43. doi: 10.1007/s00540-016-2277-z
Soriano, F. X., Papadia, S., Hofmann, F., Hardingham, N. R., Bading, H., and Hardingham, G. E. (2006). Preconditioning doses of NMDA promote neuroprotection by enhancing neuronal excitability. J. Neurosci. 26, 4509–4518. doi: 10.1523/JNEUROSCI.0455-06.2006
Spitzer, N. C. (2017). Neurotransmitter switching in the developing and adult brain. Annu. Rev. Neurosci. 40, 1–19. doi: 10.1146/annurev-neuro-072116-031204
Spitzer, N. C., Root, C. M., and Borodinsky, L. N. (2004). Orchestrating neuronal differentiation: patterns of Ca2+ spikes specify transmitter choice. Trends Neurosci. 27, 415–421. doi: 10.1016/j.tins.2004.05.003
Stankovski, L., Alvarez, C., Ouimet, T., Vitalis, T., El-Hachimi, K. H., Price, D., et al. (2007). Developmental cell death is enhanced in the cerebral cortex of mice lacking the brain vesicular monoamine transporter. J. Neurosci. 27, 1315–1324. doi: 10.1523/JNEUROSCI.4395-06.2007
Stevens, B., Porta, S., Haak, L. L., Gallo, V., and Fields, R. D. (2002). Adenosine: a neuron-glial transmitter promoting myelination in the CNS in response to action potentials. Neuron 36, 855–868. doi: 10.1016/S0896-6273(02)01067-X
Supplisson, S., and Roux, M. J. (2002). Why glycine transporters have different stoichiometries. FEBS Lett. 529, 93–101. doi: 10.1016/s0014-5793(02)03251-9
Sutherland, M. L., Delaney, T. A., and Noebels, J. L. (1996). Glutamate transporter mRNA expression in proliferative zones of the developing and adult murine CNS. J. Neurosci. 16, 2191–2207.
Thivierge, J. P. (2009). How does non-random spontaneous activity contribute to brain development? Neural Netw. 22, 901–912. doi: 10.1016/j.neunet.2009.01.001
Tiriac, A., Del Rio-Bermudez, C., and Blumberg, M. S. (2014). Self-generated movements with “unexpected” sensory consequences. Curr. Biol. 24, 2136–2141. doi: 10.1016/j.cub.2014.07.053
Tolner, E. A., Sheikh, A., Yukin, A. Y., Kaila, K., and Kanold, P. O. (2012). Subplate neurons promote spindle bursts and thalamocortical patterning in the neonatal rat somatosensory cortex. J. Neurosci. 32, 692–702. doi: 10.1523/JNEUROSCI.1538-11.2012
Tolonen, M., Palva, J. M., Andersson, S., and Vanhatalo, S. (2007). Development of the spontaneous activity transients and ongoing cortical activity in human preterm babies. Neuroscience 145, 997–1006. doi: 10.1016/j.neuroscience.2006.12.070
Tritsch, N. X., Rodríguez-Contreras, A., Crins, T. T., Wang, H. C., Borst, J. G., and Bergles, D. E. (2010). Calcium action potentials in hair cells pattern auditory neuron activity before hearing onset. Nat. Neurosci. 13, 1050–1052. doi: 10.1038/nn.2604
Tritsch, N. X., Yi, E., Gale, J. E., Glowatzki, E., and Bergles, D. E. (2007). The origin of spontaneous activity in the developing auditory system. Nature 450, 50–55. doi: 10.1038/nature06233
Turski, C. A., and Ikonomidou, C. (2012). Neuropathological sequelae of developmental exposure to antiepileptic and anesthetic drugs. Front. Neurol. 3:120. doi: 10.3389/fneur.2012.00120
Unichenko, P., Myakhar, O., and Kirischuk, S. (2012). Intracellular Na+ concentration influences short-term plasticity of glutamate transporter-mediated currents in neocortical astrocytes. Glia 60, 605–614. doi: 10.1002/glia.22294
Unichenko, P., Yang, J. W., Kirischuk, S., Kolbaev, S., Kilb, W., Hammar, M., et al. (2017). Autism related neuroligin-4 knockout impairs intracortical processing but not sensory inputs in mouse barrel cortex. Cereb. Cortex doi: 10.1093/cercor/bhx165 [Epub ahead of print].
Valeeva, G., Tressard, T., Mukhtarov, M., Baude, A., and Khazipov, R. (2016). An optogenetic approach for investigation of excitatory and inhibitory network GABA actions in mice expressing channelrhodopsin-2 in GABAergic neurons. J. Neurosci. 36, 5961–5973. doi: 10.1523/JNEUROSCI.3482-15.2016
Van Eden, C. G., Mrzljak, L., Voorn, P., and Uylings, H. B. (1989). Prenatal development of GABA-ergic neurons in the neocortex of the rat. J. Comp. Neurol. 289, 213–227. doi: 10.1002/cne.902890204
Vannucci, R. C. (2000). Hypoxic-ischemic encephalopathy. Am. J. Perinatol. 17, 113–120. doi: 10.1055/s-2000-9293
Verhage, M., Maia, A. S., Plomp, J. J., Brussaard, A. B., Heeroma, J. H., Vermeer, H., et al. (2000). Synaptic assembly of the brain in the absence of neurotransmitter secretion. Science 287, 864–869. doi: 10.1126/science.287.5454.864
Villeneuve, N., Ben-Ari, Y., Holmes, G. L., and Gaiarsa, J. L. (2000). Neonatal seizures induced persistent changes in intrinsic properties of CA1 rat hippocampal cells. Ann. Neurol. 47, 729–738. doi: 10.1002/1531-8249(200006)47:6<729::aid-ana5>3.0.co;2-c
Voigt, T., Opitz, T., and de Lima, A. D. (2005). Activation of early silent synapses by spontaneous synchronous network activity limits the range of neocortical connections. J. Neurosci. 25, 4605–4615. doi: 10.1523/JNEUROSCI.3803-04.2005
Wagner-Golbs, A., and Luhmann, H. J. (2012). Activity-dependent survival of developing neocortical neurons depends on PI3K signalling. J. Neurochem. 120, 495–501. doi: 10.1111/j.1471-4159.2011.07591.x
Wake, H., Ortiz, F. C., Woo, D. H., Lee, P. R., Angulo, M. C., and Fields, R. D. (2015). Nonsynaptic junctions on myelinating glia promote preferential myelination of electrically active axons. Nat. Commun. 6:7844. doi: 10.1038/ncomms8844
Weissman, T. A., Riquelme, P. A., Ivic, L., Flint, A. C., and Kriegstein, A. R. (2004). Calcium waves propagate through radial glial cells and modulate proliferation in the developing neocortex. Neuron 43, 647–661. doi: 10.1016/j.neuron.2004.08.015
White, R., and Krämer-Albers, E. M. (2014). Axon-glia interaction and membrane traffic in myelin formation. Front. Cell. Neurosci. 7:284. doi: 10.3389/fncel.2013.00284
Whiteus, C., Freitas, C., and Grutzendler, J. (2013). Perturbed neural activity disrupts cerebral angiogenesis during a postnatal critical period. Nature 505, 407–411. doi: 10.1038/nature12821
Winzer-Serhan, U. H., and Leslie, F. M. (1999). Expression of α2A adrenoceptors during rat neocortical development. J. Neurobiol. 38, 259–269. doi: 10.1002/(sici)1097-4695(19990205)38:2<259::aid-neu8>3.0.co;2-u
Wong, R. O., and Ghosh, A. (2002). Activity-dependent regulation of dendritic growth and patterning. Nat. Rev. Neurosci. 3, 803–812. doi: 10.1038/nrn941
Woolsey, T. A., and Wann, J. R. (1976). Areal changes in mouse cortical barrels following vibrissal damage at different postnatal ages. J. Comp. Neurol. 170, 53–66. doi: 10.1002/cne.901700105
Wu, Y., Wang, W., Díez-Sampedro, A., and Richerson, G. B. (2007). Nonvesicular inhibitory neurotransmission via reversal of the GABA transporter GAT-1. Neuron 56, 851–865. doi: 10.1016/j.neuron.2007.10.021
Wu, Y., Wang, W., and Richerson, G. B. (2006). The transmembrane sodium gradient influences ambient GABA concentration by altering the equilibrium of GABA transporters. J. Neurophysiol. 96, 2425–2436. doi: 10.1152/jn.00545.2006
Yamada, J., Okabe, A., Toyoda, H., Kilb, W., Luhmann, H. J., and Fukuda, A. (2004). Cl- uptake promoting depolarizing GABA actions in immature rat neocortical neurones is mediated by NKCC1. J. Physiol. 557, 829–841. doi: 10.1113/jphysiol.2004.062471
Yamamoto, N., and López-Bendito, G. (2012). Shaping brain connections through spontaneous neural activity. Eur. J. Neurosci. 35, 1595–1604. doi: 10.1111/j.1460-9568.2012.08101.x
Yang, J. W., An, S., Sun, J. J., Reyes-Puerta, V., Kindler, J., Berger, T., et al. (2013). Thalamic network oscillations synchronize ontogenetic columns in the newborn rat barrel cortex. Cereb. Cortex 23, 1299–1316. doi: 10.1093/cercor/bhs103
Yang, J. W., Hanganu-Opatz, I. L., Sun, J. J., and Luhmann, H. J. (2009). Three patterns of oscillatory activity differentially synchronize developing neocortical networks in vivo. J. Neurosci. 29, 9011–9025. doi: 10.1523/JNEUROSCI.5646-08.2009
Yang, J. W., Reyes-Puerta, V., Kilb, W., and Luhmann, H. J. (2016). Spindle bursts in neonatal rat cerebral cortex. Neural Plast. 2016:3467832. doi: 10.1155/2016/3467832
Ye, Z. C., Wyeth, M. S., Baltan-Tekkok, S., and Ransom, B. R. (2003). Functional hemichannels in astrocytes: a novel mechanism of glutamate release. J. Neurosci. 23, 3588–3596.
Young, C., Jevtovic-Todorovic, V., Qin, Y. Q., Tenkova, T., Wang, H., Labruyere, J., et al. (2005). Potential of ketamine and midazolam, individually or in combination, to induce apoptotic neurodegeneration in the infant mouse brain. Br. J. Pharmacol. 146, 189–197. doi: 10.1038/sj.bjp.0706301
Yu, Z. Y., Wang, W., Fritschy, J. M., Witte, O. W., and Redecker, C. (2006). Changes in neocortical and hippocampal GABAA receptor subunit distribution during brain maturation and aging. Brain Res. 1099, 73–81. doi: 10.1016/j.brainres.2006.04.118
Zagha, E., Casale, A. E., Sachdev, R. N., McGinley, M. J., and McCormick, D. A. (2013). Motor cortex feedback influences sensory processing by modulating network state. Neuron 79, 567–578. doi: 10.1016/j.neuron.2013.06.008
Zhang, J. Y., Ackman, J. B., Xu, H. P., and Crair, M. C. (2012). Visual map development depends on the temporal pattern of binocular activity in mice. Nat. Neurosci. 15, 298–307. doi: 10.1038/nn.3007
Zhang, G., Raol, Y. H., Hsu, F. C., and Brooks-Kayal, A. R. (2004a). Long-term alterations in glutamate receptor and transporter expression following early-life seizures are associated with increased seizure susceptibility. J. Neurochem. 88, 91–101. doi: 10.1046/j.1471-4159.2003.02124.x
Zhang, G., Raol, Y. H., Hsu, F. C., Coulter, D. A., and Brooks-Kayal, A. R. (2004b). Effects of status epilepticus on hippocampal GABAA receptors are age-dependent. Neuroscience 125, 299–303. doi: 10.1016/j.neuroscience.2004.01.040
Zheng, J. Q., and Poo, M.-M. (2007). Calcium signaling in neuronal motility. Ann. Rev. Cell Dev. Biol. 23, 375–404. doi: 10.1146/annurev.cellbio.23.090506.123221
Zhao, C., Kao, J. P., and Kanold, P. O. (2009). Functional excitatory microcircuits in neonatal cortex connect thalamus and layer 4. J. Neurosci. 29, 15479–15488. doi: 10.1523/JNEUROSCI.4471-09.2009
Zhou, X., Nagarajan, N., Mossop, B. J., and Merzenich, M. M. (2008). Influences of un-modulated acoustic inputs on functional maturation and critical-period plasticity of the primary auditory cortex. Neuroscience 154, 390–396. doi: 10.1016/j.neuroscience.2008.01.026
Zhou, Z. W., Shu, Y., Li, M., Guo, X., Pac-Soo, C., Maze, M., et al. (2011). The glutaminergic, GABAergic, dopaminergic but not cholinergic neurons are susceptible to anaesthesia-induced cell death in the rat developing brain. Neuroscience 174, 64–70. doi: 10.1016/j.neuroscience.2010.10.009
Keywords: development, cerebral cortex, subplate, spontaneous activity, somatosensory cortex, rodent, human, review
Citation: Kirischuk S, Sinning A, Blanquie O, Yang J-W, Luhmann HJ and Kilb W (2017) Modulation of Neocortical Development by Early Neuronal Activity: Physiology and Pathophysiology. Front. Cell. Neurosci. 11:379. doi: 10.3389/fncel.2017.00379
Received: 24 August 2017; Accepted: 13 November 2017;
Published: 29 November 2017.
Edited by:
Enrico Cherubini, Scuola Internazionale Superiori di Studi Avanzati (SISSA), ItalyReviewed by:
Xavier Leinekugel, INSERM, FranceMichael Telias, University of California, Berkeley, United States
Copyright © 2017 Kirischuk, Sinning, Blanquie, Yang, Luhmann and Kilb. This is an open-access article distributed under the terms of the Creative Commons Attribution License (CC BY). The use, distribution or reproduction in other forums is permitted, provided the original author(s) or licensor are credited and that the original publication in this journal is cited, in accordance with accepted academic practice. No use, distribution or reproduction is permitted which does not comply with these terms.
*Correspondence: Heiko J. Luhmann, bHVobWFubkB1bmktbWFpbnouZGU=
†Present address: Oriane Blanquie, German Center for Neurodegenerative Diseases (DZNE), Bonn, Germany