- 1Department of Histology and Embryology, Chongqing Key Laboratory of Neurobiology, Third Military Medical University, Chongqing, China
- 2College of Pharmacy and Medicine, Joint Laboratory of Biological Psychiatry Between Shantou University Medical College and the College of Medicine, University of Manitoba, Winnipeg, MB, Canada
Schizophrenia (SZ) is a chronic and severe mental illness for which currently there is no cure. At present, the exact molecular mechanism involved in the underlying pathogenesis of SZ is unknown. The disease is thought to be caused by a combination of genetic, biological, psychological, and environmental factors. Recent studies have shown that epigenetic regulation is involved in SZ pathology. Specifically, DNA methylation, one of the earliest found epigenetic modifications, has been extensively linked to modulation of neuronal function, leading to psychiatric disorders such as SZ. However, increasing evidence indicates that glial cells, especially dysfunctional oligodendrocytes undergo DNA methylation changes that contribute to the pathogenesis of SZ. This review primarily focuses on DNA methylation involved in glial dysfunctions in SZ. Clarifying this mechanism may lead to the development of new therapeutic interventional strategies for the treatment of SZ and other illnesses by correcting abnormal methylation in glial cells.
Introduction
Schizophrenia (SZ) is a chronic and severe mental illness that affects approximately 1% of the population (Roussos and Haroutunian, 2014). This mental illness is characterized by clinical deficits featured by socially inappropriate behaviors such as paranoia, hallucinations, and inappropriate emotional responses. Although the pathogenesis of SZ is unknown, it has been commonly accepted to be caused by a combination of genetic, biological, psychological, and environmental factors (reviewed in Akbarian, 2014; Shorter and Miller, 2015).
In the past decade, several hypotheses concerning the pathogenesis of SZ have been raised up and tested. Among them, dysfunction of neurotransmitters, such as dopamine, gamma-aminobutyric acid (GABA), glutamate, as well as 5–hydroxytryptamine (5-HT) have been implicated as primary etiologies of SZ. In general, most studies have focused on the dysfunction of neurons in different regions of gray matter that include the ventral tegmental portion, the limbic system and the prefrontal cortex (Plitman et al., 2014; Taylor and Tso, 2015).
However, recent evidence has demonstrated the involvement of glial changes in the underlying pathogenesis of SZ. For example, in SZ, ultra-structural signs of oligodendrocyte deficiency have been implicated in myelinated fiber damage in gray matter (Barley et al., 2009; Williams et al., 2013b; Hercher et al., 2014). Moreover, increasing evidence from neural imaging, genetic analysis, post-mortem morphological, molecular biological and pharmacological studies revealed a wide range of white matter abnormalities such as dysfunctional oligodendrocytes with associated myelin deficits in the patients with SZ (Hof et al., 2002; Flynn et al., 2003; Voineskos et al., 2013; reviewed in Nave and Ehrenreich, 2014). Evidence has also shown that the abnormal activation of astrocytes or microglia may also be implicated in SZ (Katsel et al., 2011a; Frick et al., 2013). As such, glial cell dysfunction seems to be a primary deficit in SZ, which can alter the synaptic function and/or circuitry and cause neuronal deficits (Stewart and Davis, 2004; Segal et al., 2007; Barley et al., 2009; Williams et al., 2013a). Therefore, glial cells may function as key players that drive the underlying pathology of SZ (recently reviewed in Bernstein et al., 2015; Wang et al., 2015). The advanced understanding of the mechanisms that regulate glial function may provide a new insight into the development of therapeutic intervention strategies for SZ or related mental illnesses.
Most glial cells (i.e., astrocytes and oligodendrocytes) are differentiated from the neural stem cells (NSCs). After specification, glial progenitor cells/oligodendrocytes precursor cells (OPCs) can further differentiate into oligodendroglial lineage cells or astrocytes, and the processes are controlled by specific transcriptional programs (de Castro et al., 2013). Recently, studies have implicated specific epigenetic changes to SZ pathology (reviewed in Akbarian, 2014; Shorter and Miller, 2015). Epigenetic does not alter gene sequences but can directly influence gene expression (reviewed in Chen and Riggs, 2011; Smith and Meissner, 2013). For example, DNA methylation, one of the earliest types of epigenetic modifications, has been extensively linked to SZ (reviewed in Grayson and Guidotti, 2013). It has been shown that DNA methylation was correlated with oligodendrocyte dysfunction and/or myelin deficits in the brain of patients with SZ (Iwamoto et al., 2005, 2006; Wockner et al., 2014). Moreover, antipsychotic drugs have been shown to be able to alter the pathological changes associated with DNA methylation or demethylation and are thereby thought to be beneficial in the treatment of SZ (reviewed in Guidotti and Grayson, 2014). In this comprehensive review, we primarily focus on the role of DNA methylation in glial cells’ generation and aberrant methylation involved in glial dysfunction leading to SZ and other related illnesses.
Glial Abnormalities in SZ
Glial cells mainly include astrocytes, oligodendrocytes, microglia, ependymal cells and retinal Müller cells in the central nervous system (CNS). In addition, the term glial cells also extend to Schwann cells and satellite cells in the peripheral nervous system (PNS). Besides basic functions like nutritional support or neuronal protection, glial cells have other integral roles including governing myelination, synapse formation and immunological reactions (Namihira and Nakashima, 2013). Increased evidence shows that glial cells, especially oligodendrocyte dysfunction with corresponding myelin deficits occur in the white matter or gray matter of SZ (reviewed in Bernstein et al., 2015; Miyata et al., 2015). Additional abnormalities have also been noted in astrocytes and microglia (Katsel et al., 2011a; Chew et al., 2013; Frick et al., 2013).
Oligodendroglial cells are generated from neural progenitor cells (NPCs) in the gliogenic phase of gestation. After specification, OPCs continued to differentiate into immature oligodendrocytes and finally the mature oligodendrocytes (de Castro et al., 2013). There are different oligodendrocyte markers identifying different stages of oligodendrocytes, including platelet derived growth factor receptor alpha (PDGFRα) for OPCs, O4 for immature oligodendrocytes, myelin associated glycoprotein (MAG), proteolipid protein (PLP), and myelin basic protein (MBP) for the mature oligodendrocytes (de Castro et al., 2013). Abnormal expression of these markers along with some oligodendrocyte related transcription factors have been found in SZ.
For example, PLP1 was shown to be down-regulated in SZ. It is believed that genetic polymorphisms of PLP1 in males are likely to cause an increased susceptibility to SZ (Qin et al., 2005). Similarly, a genetic association of the 2,3-cyclicnucleotide-phosphodiesterase (CNP) and MAG genes were also found in SZ (Wan et al., 2005; Yang et al., 2005). The CNP risk polymorphism was associated with down-regulation of gene expression in SZ (Peirce et al., 2006). Other studies have shown that although the expression of MAG, CNP and oligodendrocyte transcriptional factor 2 (Olig2) in the gray or white matter did not differ between SZ patients and normal controls, myelin-associated oligodendrocytic basic protein (MOBP) mRNA levels were increased in the white matter of patients with SZ (Usui et al., 2006). The myelin oligodendrocyte glycoprotein (MOG) gene is considered to be a key biological target for SZ due to its association with white matter abnormalities and its importance in mediating the complement cascade (Zai et al., 2005). Some oligodendrocyte related transcriptional factors, such as Olig2, a basic helix-loop-helix (bHLH) oligodendrocyte transcription factor, together with Olig1, are required for normal oligodendrocyte development and functioning. Genetic association analysis showed that variations in oligodendrocyte transcription factor Olig2 together with Olig1 result in abnormal oligodendrocyte development and functioning, thereby enhancing susceptibility to SZ (Georgieva et al., 2006; Prata et al., 2013). Another target factor called sex-determining region Y-box contains gene 10 (Sox10), has also been shown to be responsible for the terminal differentiation of oligodendrocytes by combining with Olig1/2 (Li et al., 2007; Küspert et al., 2011). The abnormal expression of Sox10 has also been found to be associated with SZ (Iwamoto et al., 2005).
Moreover, Disrupted-in-schizophrenia 1 (DISC1) has also been cited as a strong candidate target gene for SZ. Rationale involving its implication in SZ revolves around the ability of DISC1 to negatively regulate the differentiation of oligodendrocytes (Katsel et al., 2011b; Boyd et al., 2015). The neuregulin 1 (NRG1)/ErbB signaling pathway is one of the important pathways responsible for the regulation of myelination (Tao et al., 2009). Henceforth, it was also considered as a key target for intervention to assist the treatment for SZ (Alaerts et al., 2009). Moreover, recent evidence suggests a key role of astrocytes in SZ due to their ability to interact with neurons, and function in the maintenance of glutamate homeostasis and recycling. The expression of astrocytic genes such as S100 beta (S100β), diodinase type II, aquaporin-4, glutaminase, excitatory amino-acid transporter 2 (EAAT2) and thrombospondin are significantly reduced in the deep layers of the anterior cingulate gyrus in patients with SZ (Katsel et al., 2011a). In addition, post-mortem and imaging studies have also suggested a critical role for microglial activation in the underlying pathogenesis of SZ patients (Frick et al., 2013). As such, these recent findings suggest that glial dysfunction may be involved in the underlying pathogenesis of SZ. However, the exact molecular mechanisms underlying the pathological process are currently unknown.
DNA Methylation
DNA methylation is one of the earliest found epigenetic modifications thought to drive the pathology of various psychiatric diseases such as SZ (Grayson and Guidotti, 2013; Numata et al., 2014). It is mediated by DNA methytransferases (Dnmts), which can transfer a methyl group (CH3) from S-adenosyl-L-methionine (SAM) to the fifth carbon position of cytosine. The methylated cytosine at fifth carbon position is named 5-methylcytosine (5mC). During the methylation process, Dnmts transfer the methyl group from SAM to cytosine residues, generating S-adeno-sylhomocysteine (SAH; reviewed in Smith and Meissner, 2013). In general, the methyl-binding domain proteins (MBDs) recruit histone deacetylase (HDACs) and co-repressors (co-rep) by Dnmts to form transcription repressor complexes. By forming the complexes, they prevent transcription factors from binding with their specific DNA sequences, causing silence or inhibition of gene expression (reviewed in Smith and Meissner, 2013; Figure 1). There are six members belonging to the MBD family, including MBD1–4, MeCP2 (methyl-CpG-binding protein 2) and Kaiso. At present, MeCP2 have two biologically active isoforms called MeCP2E1 and MeCP2E2 (Olson et al., 2014). Dnmts isoforms include Dnmt1, which maintains the methylation on the genes, and Dnmt3a and Dnmt3b for de novo establishment of DNA methylation (reviewed in Houston et al., 2013; Smith and Meissner, 2013). Cytosine methylation of promoter regions usually represses gene transcription (Figure 1). Conversely, demethylation is the process which removes a methyl group (CH3) from 5mC, finally a hydrogen atom is added to the site, resulting in a net loss of one carbon and two hydrogen atoms. The ten-eleven translocases (TETs) family of methylcytosine dioxygenases including TET1–3, collaborate with DNA damage 45-beta (Gadd45β), Dnmts and HDACs, to catalyze oxidation of 5mC to 5-hydroxymethylcytosine (5hmC), thus promoting DNA demethylation (reviewed in Chen and Riggs, 2011).
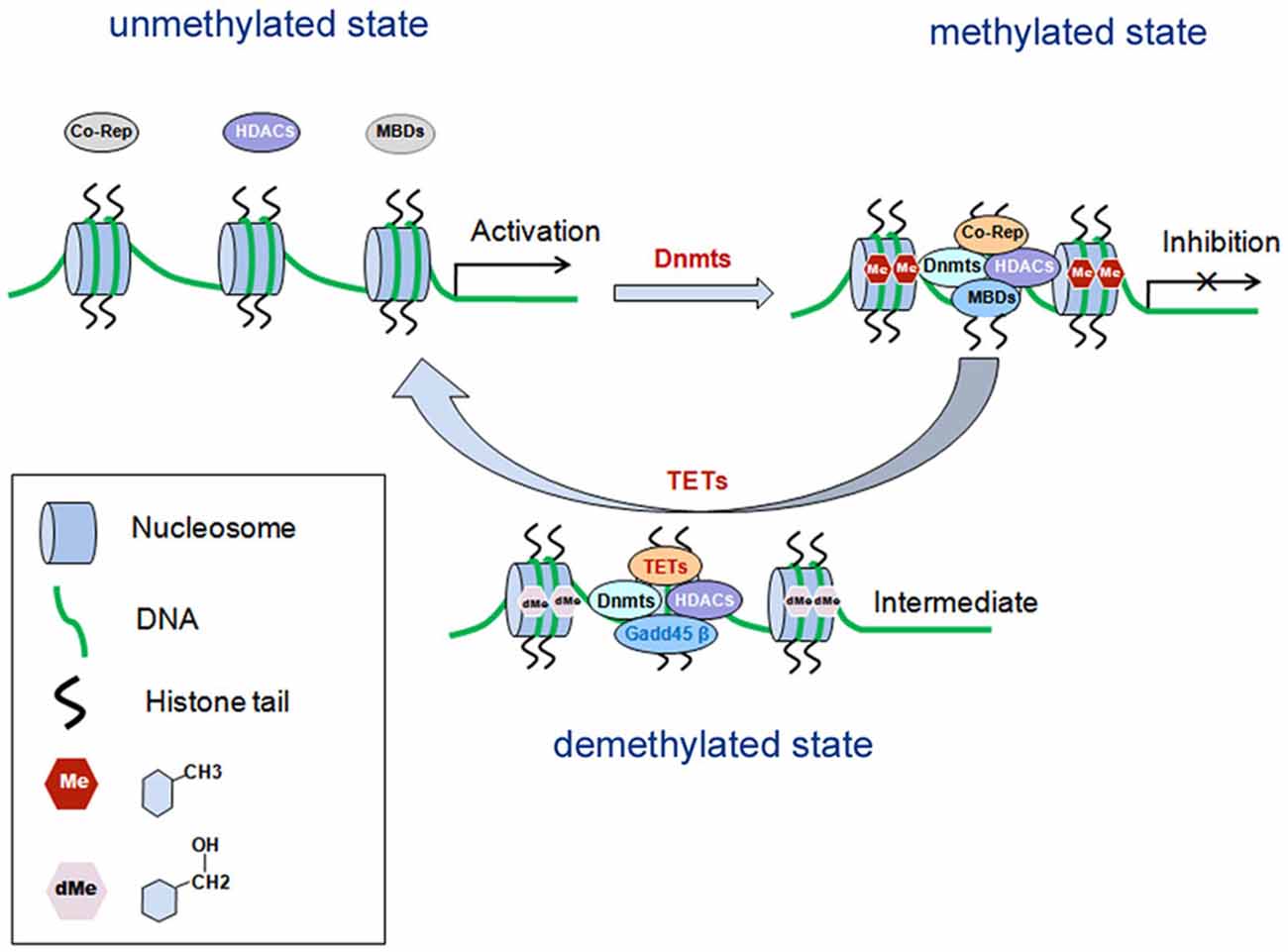
Figure 1. Schematic diagram: DNA methylation in gene transcriptional regulation. DNA methylation is mediated by Dnmts, which is recruited by MBDs and form transcription repressor complexes together with co-repressors (co-rep) and HDACs, and induces transcriptional inhibition. Demethylation is mediated by TETs, which can catalyze oxidation of 5mC to 5-hydroxymethylcytosine (5hmC), and finally leads to disassembly of the repressor complexes and gene transcriptional activation. MBDs, methyl-binding domain proteins; Dnmts, DNA methyltransferases; HDACs, histone deacetylase; Co-Rep, co-repressors; TETs, the ten-eleven translocases; Gadd45β, growth arrest and DNA damage 45-beta.
In post-mortem brains of patients with SZ, DNA methylation has been assayed for a number of genes mainly expressed in neurons, namely, Reelin, catechyl-O-methyltransferase (COMT), OPRM (opioid receptor, mu), the serotonin-2A receptor gene (HTR2A), brain derived neurotrophic factor (BDNF) and arachidonate 5-lipoxygenase (ALOX5; reviewed in Grayson and Guidotti, 2013). Research has reported an approximately twofold increase of SAM level in SZ (Guidotti et al., 2007). In addition, other researchers have reported that SAM levels regulate the DNA methylome of Schwann cells, which are myelination glial cells in the PNS (Varela-Rey et al., 2014). Furthermore, it was also found that there was a higher expression of Dnmt1 and Dnmt3a in SZ than patients without SZ (Guidotti et al., 2007; Zhubi et al., 2009). Besides neurons, Dnmt1, Dnmt3a and Dnmt3b are also expressed in glial cells (Feng et al., 2005). Dnmt3a-deficient NSCs tend to differentiate into astrocytes and oligodendrocytes as a result of demethylation of glial genes (Wu et al., 2012b). This data suggests a potential role of DNA methylation in glial cells which may partially explain the etiology of SZ. Moreover, evidence has shown that abnormal glial cells, such as astrocyte or oligodendrocyte dysfunction conjunction with myelin deficits occur in white matter. Interestingly, these changes are thought to be the result of their DNA methylated status changes in SZ (Iwamoto et al., 2005, 2006; Wockner et al., 2014).
DNA Methylation in Oligodendrocytes
Studies of Dnmt3a knockout NSCs indicates that Dnmt3a may be involved in regulating fate determination of the oligodendroglial lineage (Table 1). In Dnmt3a-deficient NSCs, the methylation levels of oligodendroglial differentiation related genes such as PDGFRα, Olig1, Sox10, MBP, Id2, Id4, Nkx2.2 and Nkx6.2 are decreased, which results in up-regulation of these genes and enhanced generation of oligodendroglial cells (Wu et al., 2010, 2012b). In addition, other researchers have shown that in MeCP2 null mice the loss of MeCP2 in the oligodendrocyte lineage cells specifically resulted in more active behaviors with corresponding severe hind limb clasping phenotypes. Moreover, these MeCP2 null mice displayed reduced expression of some myelin-related proteins such as CNPase and MBP (Vora et al., 2010; Wu et al., 2012a; Nguyen et al., 2013). On the other hand, Id2/4 was shown to be demethylated during oligodendroglial differentiation, which is mediated by protein arginine N-methyltransferase 5 (PRMT5; Huang et al., 2011).
In vitro studies involving OPC cultures have identified two Hpa2 sites located at −1836 and −39 of the MAG gene that are progressively demethylated during differentiation (Grubinska et al., 1994), thereby altering the normal myelination process. Recently, it was found that TET1-3 family members can regulate the differentiation of OPCs. Specifically, TET2 is thought to be critical for the expression of some important myelin genes, such as MBP (Zhao et al., 2014; Table 1). Furthermore, the level of 5hmC exhibited dynamic changes, indicating that the combination of DNA methylation along with demethylation may play an important role in the regulation of myelin gene expression and OPCs’ differentiation (Zhao et al., 2014).
In SZ, Sox10 hyper-methylation was found to be correlated with its reduced expression and oligodendrocyte dysfunction. However, the CpG island of Olig2 and the methylated state of MOBP, which encodes the structural protein in mature oligodendrocytes, is rarely methylated in normal brains (Iwamoto et al., 2005, 2006; Wockner et al., 2014). Recent investigations on the corpus callosum following the administration of cocaine showed a change in DNA methylation at the promoter region of the Sox10 gene. However, the methylated state of the myelin proteins such as MBP or PLP1 was not affected (Nielsen et al., 2012).
Methylation has also been shown to be involved in myelin protein integrity. For example, MBP arginine methylation level decreased following brain development, which is critical for MBP synthesis. However, in rats exposed to arsenic, MBP arginine methylation level was increased as compared to normal controls (Chanderkar et al., 1986; Zarazua et al., 2010). Moreover, methylation in the promoter of the peptidylarginine deiminase 2 (PAD2; a key enzyme which converts arginines of MBP into citrullines) was decreased to one-third of normal control in white matter of patients with multiple sclerosis (MS; Mastronardi et al., 2007). Recently, transcriptional regulation of BDNF by MeCP2 was believed as a novel mechanism for re-myelination and/or myelin repair involved in the treatment of MS (KhorshidAhmad et al., 2015).
DNA Methylation in Astrocyte
Astrocytes are the most abundant glial cells which are known to play crucial roles in brain development (Namihira and Nakashima, 2013). Astrocytes are generated from NPCs at early-gestational to mid-gestational stages. They are located in the ventricular and subventricular zone in late gestation. During astrocytogenesis, cytokine-induced activation of the janus kinase (JAK)-signal transducer and activator of transcription (Stat) pathway are necessary. Activated Stat3 binds the promoter of Gfap gene to active gene expression, thus inducing NPCs to differentiate into astrocytes (Namihira and Nakashima, 2013). On embryonic day 11.5 (E11.5), the Stat3 binding site at the promoter region of Gfap gene is highly methylated, causing its expression silenced. However, on E14.5, the promoter of Gfap gene is demethylated thereby promoting its expression (Takizawa et al., 2001; Hatada et al., 2008). In addition to the promoter, the exon 1 of Gfap gene can also be modified by methylation. MeCP2E1, an isoform of MeCP2, binds to exon 1 of Gfap gene to suppress Gfap gene expression (Tsujimura et al., 2009). Moreover, under its ectopic expression, MeCP2 can prevent Gfap transcription and astrocytic differentiation from NSCs, even in the presence of astrocytic induced cytokine (Urayama et al., 2013).
As exposed to bromo-deoxyduridene (BrdU) or azacytidine, Gfap is demethylated and this induces astrocytic differentiation from NSCs (Schneider and d’Adda di Fagagna, 2012). Several other targets have been linked to the demethylation of the Gfap promoter. Specifically, Notch signaling, the hypoxia-inducible factor 1a (HIF1α), Old astrocyte specifically induced substance (OASIS), the murine homologs of chicken ovalbumin upstream promoter transcription factors I and II (COUP-TFI/II) are typical target molecules that contribute to the demethylation of the Gfap promoter (Naka et al., 2008; Mutoh et al., 2012; Saito et al., 2012). Interestingly, the CpG sites in Gfap gene promoter are heavily methylated in microglia, while demethylated in astrocytes (Barresi et al., 1999). Without methylation, histone modifications by H3K9me3 or H3K27me3 are not sufficient for activation of Stat3-binding site in the Gfap promoter region in NSCs. These facts indicate that DNA methylation plays a critical role in deciding the timing of astrocytogenesis (Urayama et al., 2013). In addition, the gene of S100β is also hypo-methylated during astrocytogenesis on E11.5. It has also been shown that following chemical exposure of ethanol, the protein levels of Dnmt3a and activity of Dnmts become increased, causing demethylation of the tissue plasminogen activator (tPA) promoter and resulting in increased expression in of these proteins in astrocytes (Zhang et al., 2014).
Recent research has also shown that hypo-methylation in astrocytes alters the expression of EAAT2, a family member of glutamate transporters (Zschocke et al., 2007). Interestingly, researchers have also reported that the down-regulation of MeCP2 did not affect cell morphology, growth, and cytotoxic reaction, but reduced expression of EAAT1/2 in astrocytes (Okabe et al., 2012). However, MeCP2 deficiency in astrocytes has been shown to cause significant abnormalities in BDNF regulation, cytokine production, and neuronal dendritic induction via non-cell-autonomous mechanism on gap junction (Maezawa et al., 2009). In SZ, the expression of EAAT2 was significantly reduced in astrocytes, suggesting that abnormal methylation status of EAAT1/2 in astrocytes may be involved in its pathogenesis (Katsel et al., 2011a).
DNA Methylation in Glial Cells and Other Mental Disorders
Rett syndrome (RTT) is a childhood white matter disorder caused by mutations in MeCP2. It is an X-linked neuro-developmental disorder featured by autism and severe mental retardation and neurological dysfunction in females. Although RTT is generally attributed to be a primary neuronal dysfunction, it has recently been shown that astrocytes, oligodendrocytes and microglia also contribute to RTT pathophysiology (Maezawa et al., 2009; Derecki et al., 2012; Nguyen et al., 2013). MeCP2 deficiency results in oligodendrocytes and astrocytes abnormities as mentioned above. MeCP2-null microglia was shown to produce a fivefold higher level of glutamate. As such, the blockage of microglial glutamate released by gap junction and glutamate receptor antagonists attenuated the neurotoxicity associated with MeCP2-null microglia (Maezawa and Jin, 2010).
Genome-wide methylation map has identified significant changes in methylation of astrocytic markers such as Gfap, aldehyde dehydrogenase 1 family, member L1 (ALDH1L1), SOX9, glutamate-ammonia ligase (GLUL), the sodium/phosphate cotransporters NaPi-IIc (SCL34A3), gap junction alpha 1 (GJA1) and gap junction beta 6 (GJB6), brain-enriched guanylate kinase-associated protein (BEGAIN) and glutamate receptor ionotropic kainate 2 (GRIK2). These markers can serve as functional candidates involved in the clinical screening of patients for susceptibility to SZ (Nagy et al., 2015). In addition, the expression pattern of these markers has been suggested to correlate with changes in depression that is often suffered by patients with SZ. For these reasons, the significant differences in the methylation patterns specific to astrocytic dysfunction are now thought to be associated with depressive psychopathology (Nagy et al., 2015).
Kir4.1 is a glial-specific potassium (K+) channel which is essential for the development of the CNS. As such, decreased Kir4.1 expression is associated with Dnmt1-mediated DNA hyper-methylation in medical conditions such as ischemic injury, epilepsy, and Alzheimer’s disease (Nwaobi et al., 2014). In astrocytes, hypermethylation of monocarboxylate transporter 4 (MCT4) gene results in a significantly lower expression of MCT4, which subsequently leads to neuronal hyper-excitability and epileptogenesis in temporal lobe epilepsy patients (Liu et al., 2014).
Clinical Implications and Future Directions
Based on the comprehensive review of the current literature, we have identified the importance of abnormal DNA methylation and demethylation in psychiatric disorders such as SZ. Specifically, research has identified aberrant methylation, growth arrest and DNA damage 45-beta (Gadd45β), as part of the DNA-demethylation pathway component, whose expression was increased by nearly threefold in SZ (Matrisciano et al., 2011). DNA methylation combineds with histone deacetylation regulate gene expression and represent two key focal points for epigenetic therapeutic interventional strategies for SZ. As such, evidence shows that adjunctive therapy for SZ may be more effective by combining demethylation with histone deacetylation (Dong et al., 2008, 2010). Because aberrant DNA methylation and histone deacetylation patterns are potentially irreversible, it has been suggested that therapy based on inhibiting DNA demethylation and histone deacetylation may serve as novel intervention strategies for early stage SZ. For example, when SZ-like mice being treated with antipsychotic drug clozapine and HDAC inhibitor VPA, Gadd45β expression can be activated and bind specific promoter regions of Reelin and GAD67 to cause hypomethylation, and thereby be beneficial for SZ treatment (Dong et al., 2008, 2010; Matrisciano et al., 2011).
Since glial abnormalities are thought to be key players in SZ pathology, new strategies that target the glial cells may be beneficial in the treatment of SZ (Takahashi and Sakurai, 2013; Guidotti and Grayson, 2014; Bernstein et al., 2015). Furthermore, since DNA methylation has an important role in regulating expression of glial genes DNA methylation (Table 1), studies involving more glial candidate genes should be conducted to elucidate their effects in the underlying pathogenesis of SZ. Henceforth, the advanced understanding of the specific mechanisms involving DNA methylation in regulating glial genesis and their pathological roles in SZ provide new insights into interventional treatment strategies for SZ and other related illnesses.
Conflict of Interest Statement
The authors declare that the research was conducted in the absence of any commercial or financial relationships that could be construed as a potential conflict of interest.
Acknowledgment
This work is supported by the National Natural Science Foundation of China (NSCF 81471297, 31171046).
Abbreviations
Basic helix-loop-helix, bHLH; Brain derived neurotrophic factor, BDNF; Central nervous system, CNS; 2,3-cyclicnucleotide-phosphodiesterase, CNP; Cytosine-guanine dinucleotide, CpG; Disrupted-in-schizophrenia 1, DISC1; DNA methytransferases, Dnmts; The excitatory amino-acid transporter, EAAT; Embryonic day 11.5, E11.5 d; Growth arrest and DNA damage 45-beta, Gadd45β; Glial fibrillary acidic protein, Gfap; Histone deacetylases, HDACs; 5-hydroxymethylcytosine, 5hmC; Inhibitor of DNA binding 2 and 4, Id2/4; Myelin associated glycoprotein, MAG; Methyl-CpG-binding protein 2, MeCP2; Methyl—binding domain protein, MBD; 5-Methylcytosine, 5mC; Monocarboxylate transporter 4, MCT4; Myelin oligodendrocyte glycoprotein, MOG; Myelin-associated oligodendrocytic basic protein, MOBP; Multiple sclerosis, MS; Neuroepithelial cells, NPCs; Neuregulin 1, NRG1; Neural stem cells, NSCs; Oligodendrocytes precursor cells, OPCs; Oligodendrocyte transcriptional factor 2, Olig2; Oligodendrocytes, OLs; Platelet derived growth factor receptor alpha, PDGFRα; Proteolipid protein, PLP; Peripheral nervous system, PNS; Protein arginine N-methyltransferase 5, PRMT5; S-adenosyl homocysteine, SAH; S-adenosyl-L-methionine, SAM; S100 beta, S100β; Sex-determining region Y-box containing gene 10, Sox10; Signal transducer and activator of transcription 3, Stat3; Schizophrenia, SZ; The ten-eleven translocases, TETs; The tissue plasminogen activator, tPA.
References
Alaerts, M., Ceulemans, S., Forero, D., Moens, L. N., De Zutter, S., Heyrman, L., et al. (2009). Support for NRG1 as a susceptibility factor for schizophrenia in a northern Swedish isolated population. Arch. Gen. Psychiatry 66, 828–837. doi: 10.1001/archgenpsychiatry.2009.82
Alelú-Paz, R., González-Corpas, A., Ashour, N., Escanilla, A., Monje, A., Guerrero Marquez, C., et al. (2015). DNA methylation pattern of gene promoters of major neurotransmitter systems in older patients with schizophrenia with severe and mild cognitive impairment. Int. J. Geriatr. Psychiatry 30, 558–565. doi: 10.1002/gps.4182
Barley, K., Dracheva, S., and Byne, W. (2009). Subcortical oligodendrocyte- and astrocyte-associated gene expression in subjects with schizophrenia, major depression and bipolar disorder. Schizophr. Res. 112, 54–64. doi: 10.1016/j.schres.2009.04.019
Barresi, V., Condorelli, D. F., and Giuffrida Stella, A. M. (1999). GFAP gene methylation in different neural cell types from rat brain. Int. J. Dev. Neurosci. 17, 821–828. doi: 10.1016/s0736-5748(99)00059-3
Bernstein, H. G., Steiner, J., Guest, P. C., Dobrowolny, H., and Bogerts, B. (2015). Glial cells as key players in schizophrenia pathology: recent insights and concepts of therapy. Schizophr. Res. 161, 4–18. doi: 10.1016/j.schres.2014.03.035
Boyd, P. J., Cunliffe, V. T., Roy, S., and Wood, J. D. (2015). Sonic hedgehog functions upstream of disrupted-in-schizophrenia 1 (disc1): implications for mental illness. Biol. Open 4, 1336–1343. doi: 10.1242/bio.012005
Chanderkar, L. P., Paik, W. K., and Kim, S. (1986). Studies on myelin-basic-protein methylation during mouse brain development. Biochem. J. 240, 471–479. doi: 10.1042/bj2400471
Chen, Z. X., and Riggs, A. D. (2011). DNA methylation and demethylation in mammals. J. Biol. Chem. 286, 18347–18353. doi: 10.1074/jbc.R110.205286
Chew, L. J., Fusar-Poli, P., and Schmitz, T. (2013). Oligodendroglial alterations and the role of microglia in white matter injury: relevance to schizophrenia. Dev. Neurosci. 35, 102–129. doi: 10.1159/000346157
de Castro, F., Bribián, A., and Ortega, M. C. (2013). Regulation of oligodendrocyte precursor migration during development, in adulthood and in pathology. Cell. Mol. Life Sci. 70, 4355–4368. doi: 10.1007/s00018-013-1365-6
Derecki, N. C., Cronk, J. C., Lu, Z., Xu, E., Abbott, S. B., Guyenet, P. G., et al. (2012). Wild-type microglia arrest pathology in a mouse model of Rett syndrome. Nature 484, 105–109. doi: 10.1038/nature10907
Dong, E., Chen, Y., Gavin, D. P., Grayson, D. R., and Guidotti, A. (2010). Valproate induces DNA demethylation in nuclear extracts from adult mouse brain. Epigenetics 5, 730–735. doi: 10.4161/epi.5.8.13053
Dong, E., Nelson, M., Grayson, D. R., Costa, E., and Guidotti, A. (2008). Clozapine and sulpiride but not haloperidol or olanzapine activate brain DNA demethylation. Proc. Natl. Acad. Sci. U S A 105, 13614–13619. doi: 10.1073/pnas.0805493105
Feng, J., Chang, H., Li, E., and Fan, G. (2005). Dynamic expression of de novo DNA methyltransferases Dnmt3a and Dnmt3b in the central nervous system. J. Neurosci. Res. 79, 734–746. doi: 10.1002/jnr.20404
Flynn, S. W., Lang, D. J., Mackay, A. L., Goghari, V., Vavasour, I. M., Whittall, K. P., et al. (2003). Abnormalities of myelination in schizophrenia detected in vivo with MRI and post-mortem with analysis of oligodendrocyte proteins. Mol. Psychiatry 8, 811–820. doi: 10.1038/sj.mp.4001337
Frick, L. R., Williams, K., and Pittenger, C. (2013). Microglial dysregulation in psychiatric disease. Clin. Dev. Immunol. 2013:608654. doi: 10.1155/2013/608654
Georgieva, L., Moskvina, V., Peirce, T., Norton, N., Bray, N. J., Jones, L., et al. (2006). Convergent evidence that oligodendrocyte lineage transcription factor 2 (OLIG2) and interacting genes influence susceptibility to schizophrenia. Proc. Natl. Acad. Sci. U S A 103, 12469–12474. doi: 10.1073/pnas.0603029103
Grayson, D. R., and Guidotti, A. (2013). The dynamics of DNA methylation in schizophrenia and related psychiatric disorders. Neuropsychopharmacology 38, 138–166. doi: 10.1038/npp.2012.125
Grubinska, B., Laszkiewicz, I., Royland, J., Wiggins, R. C., and Konat, G. W. (1994). Differentiation-specific demethylation of myelin associated glycoprotein gene in cultured oligodendrocytes. J. Neurosci. Res. 39, 233–242. doi: 10.1002/jnr.490390302
Guidotti, A., and Grayson, D. R. (2014). DNA methylation and demethylation as targets for antipsychotic therapy. Dialogues Clin. Neurosci. 16, 419–429.
Guidotti, A., Ruzicka, W., Grayson, D. R., Veldic, M., Pinna, G., Davis, J. M., et al. (2007). S-adenosyl methionine and DNA methyltransferase-1 mRNA overexpression in psychosis. Neuroreport 18, 57–60. doi: 10.1097/wnr.0b013e32800fefd7
Hatada, I., Namihira, M., Morita, S., Kimura, M., Horii, T., and Nakashima, K. (2008). Astrocyte-specific genes are generally demethylated in neural precursor cells prior to astrocytic differentiation. PLoS One 3:e3189. doi: 10.1371/journal.pone.0003189
Hercher, C., Chopra, V., and Beasley, C. L. (2014). Evidence for morphological alterations in prefrontal white matter glia in schizophrenia and bipolar disorder. J. Psychiatry Neurosci. 39, 376–385. doi: 10.1503/jpn.130277
Hof, P. R., Haroutunian, V., Copland, C., Davis, K. L., and Buxbaum, J. D. (2002). Molecular and cellular evidence for an oligodendrocyte abnormality in schizophrenia. Neurochem. Res. 27, 1193–1200. doi: 10.1023/A:1020981510759
Houston, I., Peter, C. J., Mitchell, A., Straubhaar, J., Rogaev, E., and Akbarian, S. (2013). Epigenetics in the human brain. Neuropsychopharmacology 38, 183–197. doi: 10.1038/npp.2012.78
Huang, J., Vogel, G., Yu, Z., Almazan, G., and Richard, S. (2011). Type II arginine methyltransferase PRMT5 regulates gene expression of inhibitors of differentiation/DNA binding Id2 and Id4 during glial cell differentiation. J. Biol. Chem. 286, 44424–44432. doi: 10.1074/jbc.m111.277046
Iwamoto, K., Bundo, M., Yamada, K., Takao, H., Iwayama, Y., Yoshikawa, T., et al. (2006). A family-based and case-control association study of SOX10 in schizophrenia. Am. J. Med. Genet. B Neuropsychiatr. Genet. 141B, 477–481. doi: 10.1002/ajmg.b.30304
Iwamoto, K., Bundo, M., Yamada, K., Takao, H., Iwayama-Shigeno, Y., Yoshikawa, T., et al. (2005). DNA methylation status of SOX10 correlates with its downregulation and oligodendrocyte dysfunction in schizophrenia. J. Neurosci. 25, 5376–5381. doi: 10.1523/jneurosci.0766-05.2005
Katsel, P., Byne, W., Roussos, P., Tan, W., Siever, L., and Haroutunian, V. (2011a). Astrocyte and glutamate markers in the superficial, deep and white matter layers of the anterior cingulate gyrus in schizophrenia. Neuropsychopharmacology 36, 1171–1177. doi: 10.1038/npp.2010.252
Katsel, P., Tan, W., Abazyan, B., Davis, K. L., Ross, C., Pletnikov, M. V., et al. (2011b). Expression of mutant human DISC1 in mice supports abnormalities in differentiation of oligodendrocytes. Schizophr. Res. 130, 238–249. doi: 10.1016/j.schres.2011.04.021
KhorshidAhmad, T., Acosta, C., Cortes, C., Lakowski, T. M., Gangadaran, S., and Namaka, M. (2015). Transcriptional regulation of brain-derived neurotrophic factor (BDNF) by methyl CpG binding protein 2 (MeCP2): a novel mechanism for re-myelination and/or myelin repair involved in the treatment of multiple sclerosis (MS). Mol. Neurobiol. doi: 10.1007/s12035-014-9074-1 [Epub ahead of print].
Küspert, M., Hammer, A., Bösl, M. R., and Wegner, M. (2011). Olig2 regulates Sox10 expression in oligodendrocyte precursors through an evolutionary conserved distal enhancer. Nucleic Acids Res. 39, 1280–1293. doi: 10.1093/nar/gkq951
Li, H., Lu, Y., Smith, H. K., and Richardson, W. D. (2007). Olig1 and Sox10 interact synergistically to drive myelin basic protein transcription in oligodendrocytes. J. Neurosci. 27, 14375–14382. doi: 10.1523/jneurosci.4456-07.2007
Liu, B., Niu, L., Shen, M. Z., Gao, L., Wang, C., Li, J., et al. (2014). Decreased astroglial monocarboxylate transporter 4 expression in temporal lobe epilepsy. Mol. Neurobiol. 50, 327–338. doi: 10.1007/s12035-013-8619-z
Maezawa, I., and Jin, L. W. (2010). Rett syndrome microglia damage dendrites and synapses by the elevated release of glutamate. J. Neurosci. 30, 5346–5356. doi: 10.1523/JNEUROSCI.5966-09.2010
Maezawa, I., Swanberg, S., Harvey, D., LaSalle, J. M., and Jin, L. W. (2009). Rett syndrome astrocytes are abnormal and spread MeCP2 deficiency through gap junctions. J. Neurosci. 29, 5051–5061. doi: 10.1523/JNEUROSCI.0324-09.2009
Mastronardi, F. G., Noor, A., Wood, D. D., Paton, T., and Moscarello, M. A. (2007). Peptidyl argininedeiminase 2 CpG island in multiple sclerosis white matter is hypomethylated. J. Neurosci. Res. 85, 2006–2016. doi: 10.1002/jnr.21329
Matrisciano, F., Dong, E., Gavin, D. P., Nicoletti, F., and Guidotti, A. (2011). Activation of group II metabotropic glutamate receptors promotes DNA demethylation in the mouse brain. Mol. Pharmacol. 80, 174–182. doi: 10.1124/mol.110.070896
Miyata, S., Hattori, T., Shimizu, S., Ito, A., and Tohyama, M. (2015). Disturbance of oligodendrocyte function plays a key role in the pathogenesis of schizophrenia and major depressive disorder. Biomed. Res. Int. 2015:492367. doi: 10.1155/2015/492367
Mutoh, T., Sanosaka, T., Ito, K., and Nakashima, K. (2012). Oxygen levels epigenetically regulate fate switching of neural precursor cells via hypoxia-inducible factor 1alpha-notch signal interaction in the developing brain. Stem Cells 30, 561–569. doi: 10.1002/stem.1019
Nagy, C., Suderman, M., Yang, J., Szyf, M., Mechawar, N., Ernst, C., et al. (2015). Astrocytic abnormalities and global DNA methylation patterns in depression and suicide. Mol. Psychiatry 20, 320–328. doi: 10.1038/mp.2014.21
Naka, H., Nakamura, S., Shimazaki, T., and Okano, H. (2008). Requirement for COUP-TFI and II in the temporal specification of neural stem cells in CNS development. Nat. Neurosci. 11, 1014–1023. doi: 10.1038/nn.2168
Namihira, M., and Nakashima, K. (2013). Mechanisms of astrocytogenesis in the mammalian brain. Curr. Opin. Neurobiol. 23, 921–927. doi: 10.1016/j.conb.2013.06.002
Nave, K. A., and Ehrenreich, H. (2014). Myelination and oligodendrocyte functions in psychiatric diseases. JAMA Psychiatry 71, 582–584. doi: 10.1001/jamapsychiatry.2014.189
Nguyen, M. V., Felice, C. A., Du, F., Covey, M. V., Robinson, J. K., Mandel, G., et al. (2013). Oligodendrocyte lineage cells contribute unique features to Rett syndrome neuropathology. J. Neurosci. 33, 18764–18774. doi: 10.1523/JNEUROSCI.2657-13.2013
Nielsen, D. A., Huang, W., Hamon, S. C., Maili, L., Witkin, B. M., Fox, R. G., et al. (2012). Forced abstinence from cocaine self-administration is associated with DNA methylation changes in myelin genes in the corpus callosum: a preliminary study. Front. Psychiatry 3:60. doi: 10.3389/fpsyt.2012.00060
Numata, S., Ye, T., Herman, M., and Lipska, B. K. (2014). DNA methylation changes in the postmortem dorsolateral prefrontal cortex of patients with schizophrenia. Front. Genet. 5:280. doi: 10.3389/fgene.2014.00280
Nwaobi, S. E., Lin, E., Peramsetty, S. R., and Olsen, M. L. (2014). DNA methylation functions as a critical regulator of Kir4.1 expression during CNS development. Glia 62, 411–427. doi: 10.1002/glia.22613
Okabe, Y., Takahashi, T., Mitsumasu, C., Kosai, K., Tanaka, E., and Matsuishi, T. (2012). Alterations of gene expression and glutamate clearance in astrocytes derived from an MeCP2-null mouse model of Rett syndrome. PLoS One 7:e35354. doi: 10.1371/journal.pone.0035354
Olson, C. O., Zachariah, R. M., Ezeonwuka, C. D., Liyanage, V. R., and Rastegar, M. (2014). Brain region-specific expression of MeCP2 isoforms correlates with DNA methylation within Mecp2 regulatory elements. PLoS One 9:e90645. doi: 10.1371/journal.pone.0090645
Peirce, T. R., Bray, N. J., Williams, N. M., Norton, N., Moskvina, V., Preece, A., et al. (2006). Convergent evidence for 2′,3′-cyclic nucleotide 3′-phosphodiesterase as a possible susceptibility gene for schizophrenia. Arch. Gen. Psychiatry 63, 18–24. doi: 10.1001/archpsyc.63.1.18
Plitman, E., Nakajima, S., de la Fuente-Sandoval, C., Gerretsen, P., Chakravarty, M. M., Kobylianskii, J., et al. (2014). Glutamate-mediated excitotoxicity in schizophrenia: a review. Eur. Neuropsychopharmacol. 24, 1591–1605. doi: 10.1016/j.euroneuro.2014.07.015
Prata, D. P., Kanaan, R. A., Barker, G. J., Shergill, S., Woolley, J., Georgieva, L., et al. (2013). Risk variant of oligodendrocyte lineage transcription factor 2 is associated with reduced white matter integrity. Hum. Brain Mapp. 34, 2025–2031. doi: 10.1002/hbm.22045
Qin, W., Gao, J., Xing, Q., Yang, J., Qian, X., Li, X., et al. (2005). A family-based association study of PLP1 and schizophrenia. Neurosci. Lett. 375, 207–210. doi: 10.1016/j.neulet.2004.11.013
Roussos, P., and Haroutunian, V. (2014). Schizophrenia: susceptibility genes and oligodendroglial and myelin related abnormalities. Front. Cell. Neurosci. 8:5. doi: 10.3389/fncel.2014.00005
Saito, A., Kanemoto, S., Kawasaki, N., Asada, R., Iwamoto, H., Oki, M., et al. (2012). Unfolded protein response, activated by OASIS family transcription factors, promotes astrocyte differentiation. Nat. Commun. 3:967. doi: 10.1038/ncomms1971
Schneider, L., and d’Adda di Fagagna, F. (2012). Neural stem cells exposed to BrdU lose their global DNA methylation and undergo astrocytic differentiation. Nucleic Acids Res. 40, 5332–5342. doi: 10.1093/nar/gks207
Segal, D., Koschnick, J. R., Slegers, L. H., and Hof, P. R. (2007). Oligodendrocyte pathophysiology: a new view of schizophrenia. Int. J. Neuropsychopharmacol. 10, 503–511. doi: 10.1017/s146114570600722x
Shorter, K. R., and Miller, B. H. (2015). Epigenetic mechanisms in schizophrenia. Prog. Biophys. Mol. Biol. 118, 1–7. doi: 10.1016/j.pbiomolbio.2015.04.008
Smith, Z. D., and Meissner, A. (2013). DNA methylation: roles in mammalian development. Nat. Rev. Genet. 14, 204–220. doi: 10.1038/nrg3354
Stewart, D. G., and Davis, K. L. (2004). Possible contributions of myelin and oligodendrocyte dysfunction to schizophrenia. Int. Rev. Neurobiol. 59, 381–424. doi: 10.1016/s0074-7742(04)59015-3
Takahashi, N., and Sakurai, T. (2013). Roles of glial cells in schizophrenia: possible targets for therapeutic approaches. Neurobiol. Dis. 53, 49–60. doi: 10.1016/j.nbd.2012.11.001
Takizawa, T., Nakashima, K., Namihira, M., Ochiai, W., Uemura, A., Yanagisawa, M., et al. (2001). DNA methylation is a critical cell-intrinsic determinant of astrocyte differentiation in the fetal brain. Dev. Cell 1, 749–758. doi: 10.1016/s1534-5807(01)00101-0
Tang, F., Qu, M., Wang, L., Ruan, Y., Lu, T., Zhang, H., et al. (2007). Case-control association study of the 2′,3′-cyclic nucleotide 3′-phosphodiesterase (CNP) gene and schizophrenia in the Han Chinese population. Neurosci. Lett. 416, 113–116. doi: 10.1016/j.neulet.2007.01.054
Tao, Y., Dai, P., Liu, Y., Marchetto, S., Xiong, W. C., Borg, J. P., et al. (2009). Erbin regulates NRG1 signaling and myelination. Proc. Natl. Acad. Sci. U S A 106, 9477–9482. doi: 10.1073/pnas.0901844106
Taylor, S. F., and Tso, I. F. (2015). GABA abnormalities in schizophrenia: a methodological review of in vivo studies. Schizophr. Res. 167, 84–90. doi: 10.1016/j.schres.2014.10.011
Tsujimura, K., Abematsu, M., Kohyama, J., Namihira, M., and Nakashima, K. (2009). Neuronal differentiation of neural precursor cells is promoted by the methyl-CpG-binding protein MeCP2. Exp. Neurol. 219, 104–111. doi: 10.1016/j.expneurol.2009.05.001
Urayama, S., Semi, K., Sanosaka, T., Hori, Y., Namihira, M., Kohyama, J., et al. (2013). Chromatin accessibility at a STAT3 target site is altered prior to astrocyte differentiation. Cell Struct. Funct. 38, 55–66. doi: 10.1247/csf.12034
Usui, H., Takahashi, N., Saito, S., Ishihara, R., Aoyama, N., Ikeda, M., et al. (2006). The 2′,3′-cyclic nucleotide 3′-phosphodiesterase and oligodendrocyte lineage transcription factor 2 genes do not appear to be associated with schizophrenia in the Japanese population. Schizophr. Res. 88, 245–250. doi: 10.1016/j.schres.2006.07.019
Varela-Rey, M., Iruarrizaga-Lejarreta, M., Lozano, J. J., Aransay, A. M., Fernandez, A. F., Lavin, J. L., et al. (2014). S-adenosylmethionine levels regulate the schwann cell DNA methylome. Neuron 81, 1024–1039. doi: 10.1016/j.neuron.2014.01.037
Voineskos, A. N., Felsky, D., Kovacevic, N., Tiwari, A. K., Zai, C., Chakravarty, M. M., et al. (2013). Oligodendrocyte genes, white matter tract integrity and cognition in schizophrenia. Cereb. Cortex 23, 2044–2057. doi: 10.1093/cercor/bhs188
Vora, P., Mina, R., Namaka, M., and Frost, E. E. (2010). A novel transcriptional regulator of myelin gene expression: implications for neurodevelopmental disorders. Neuroreport 21, 917–921. doi: 10.1097/WNR.0b013e32833da500
Wan, C., Yang, Y., Feng, G., Gu, N., Liu, H., Zhu, S., et al. (2005). Polymorphisms of myelin-associated glycoprotein gene are associated with schizophrenia in the Chinese Han population. Neurosci. Lett. 388, 126–131. doi: 10.1016/j.neulet.2005.06.051
Wang, C., Aleksic, B., and Ozaki, N. (2015). Glia-related genes and their contribution to schizophrenia. Psychiatry Clin. Neurosci. 69, 448–461. doi: 10.1111/pcn.12290
Williams, M. R., Hampton, T., Pearce, R. K., Hirsch, S. R., Ansorge, O., Thom, M., et al. (2013a). Astrocyte decrease in the subgenual cingulate and callosal genu in schizophrenia. Eur. Arch. Psychiatry Clin. Neurosci. 263, 41–52. doi: 10.1007/s00406-012-0328-5
Williams, M. R., Harb, H., Pearce, R. K., Hirsch, S. R., and Maier, M. (2013b). Oligodendrocyte density is changed in the basolateral amygdala in schizophrenia but not depression. Schizophr. Res. 147, 402–403. doi: 10.1016/j.schres.2013.04.013
Wockner, L. F., Noble, E. P., Lawford, B. R., Young, R. M., Morris, C. P., Whitehall, V. L., et al. (2014). Genome-wide DNA methylation analysis of human brain tissue from schizophrenia patients. Transl. Psychiatry 4:e339. doi: 10.1038/tp.2013.111
Wu, H., Coskun, V., Tao, J., Xie, W., Ge, W., Yoshikawa, K., et al. (2010). Dnmt3a-dependent nonpromoter DNA methylation facilitates transcription of neurogenic genes. Science 329, 444–448. doi: 10.1126/science.1190485
Wu, W., Gu, W., Xu, X., Shang, S., and Zhao, Z. (2012a). Downregulation of CNPase in a MeCP2 deficient mouse model of Rett syndrome. Neurol. Res. 34, 107–113. doi: 10.1179/016164111X13214359296301
Wu, Z., Huang, K., Yu, J., Le, T., Namihira, M., Liu, Y., et al. (2012b). Dnmt3a regulates both proliferation and differentiation of mouse neural stem cells. J. Neurosci. Res. 90, 1883–1891. doi: 10.1002/jnr.23077
Yang, Y. F., Qin, W., Shugart, Y. Y., He, G., Liu, X. M., Zhou, J., et al. (2005). Possible association of the MAG locus with schizophrenia in a Chinese Han cohort of family trios. Schizophr. Res. 75, 11–19. doi: 10.1016/j.schres.2004.11.013
Zai, G., King, N., Wigg, K., Couto, J., Wong, G. W., Honer, W. G., et al. (2005). Genetic study of the myelin oligodendrocyte glycoprotein (MOG) gene in schizophrenia. Genes Brain Behav. 4, 2–9. doi: 10.1111/j.1601-183x.2004.00089.x
Zarazua, S., Rios, R., Delgado, J. M., Santoyo, M. E., Ortiz-Perez, D., and Jimenez-Capdeville, M. E. (2010). Decreased arginine methylation and myelin alterations in arsenic exposed rats. Neurotoxicology 31, 94–100. doi: 10.1016/j.neuro.2009.10.014
Zhang, X., Kusumo, H., Sakharkar, A. J., Pandey, S. C., and Guizzetti, M. (2014). Regulation of DNA methylation by ethanol induces tissue plasminogen activator expression in astrocytes. J. Neurochem. 128, 344–349. doi: 10.1111/jnc.12465
Zhao, X., Dai, J., Ma, Y., Mi, Y., Cui, D., Ju, G., et al. (2014). Dynamics of ten-eleven translocation hydroxylase family proteins and 5-hydroxymethylcytosine in oligodendrocyte differentiation. Glia 62, 914–926. doi: 10.1002/glia.22649
Zhubi, A., Veldic, M., Puri, N. V., Kadriu, B., Caruncho, H., Loza, I., et al. (2009). An upregulation of DNA-methyltransferase 1 and 3a expressed in telencephalic GABAergic neurons of schizophrenia patients is also detected in peripheral blood lymphocytes. Schizophr. Res. 111, 115–122. doi: 10.1016/j.schres.2009.03.020
Keywords: DNA methylation, glial genesis, oligodendrocyte, astrocyte, schizophrenia, mental illness
Citation: Chen X-S, Huang N, Michael N and Xiao L (2015) Advancements in the Underlying Pathogenesis of Schizophrenia: Implications of DNA Methylation in Glial Cells. Front. Cell. Neurosci. 9:451. doi: 10.3389/fncel.2015.00451
Received: 30 August 2015; Accepted: 02 November 2015;
Published: 02 December 2015.
Edited by:
Johann Steiner, University of Magdeburg, GermanyReviewed by:
Constanze I. Seidenbecher, Leibniz Institute for Neurobiology, GermanyNatalya A. Uranova, Mental Health Research Center, Russian Federation
Copyright © 2015 Chen, Huang, Michael and Xiao. This is an open-access article distributed under the terms of the Creative Commons Attribution License (CC BY). The use, distribution and reproduction in other forums is permitted, provided the original author(s) or licensor are credited and that the original publication in this journal is cited, in accordance with accepted academic practice. No use, distribution or reproduction is permitted which does not comply with these terms.
*Correspondence: Lan Xiao, eGlhb2xhbjM1QGhvdG1haWwuY29t