- Laboratory of Pathophysiology, Graduate School of Pharmaceutical Sciences, Kyushu University, 3-1-1 Maidashi, Higashi-ku, Fukuoka, Japan
It is widely accepted that there is a close relationship between the endocrine system and the central nervous system (CNS). Among hormones closely related to the nervous system, thyroid hormones (THs) are critical for the development and function of the CNS; not only for neuronal cells but also for glial development and differentiation. Any impairment of TH supply to the developing CNS causes severe and irreversible changes in the overall architecture and function of the human brain, leading to various neurological dysfunctions. In the adult brain, impairment of THs, such as hypothyroidism and hyperthyroidism, can cause psychiatric disorders such as schizophrenia, bipolar disorder, anxiety and depression. Although impact of hypothyroidism on synaptic transmission and plasticity is known, its effect on glial cells and related cellular mechanisms remain enigmatic. This mini-review article summarizes how THs are transported into the brain, metabolized in astrocytes and affect microglia and oligodendrocytes, demonstrating an example of glioendocrine system. Neuroglial effects may help to understand physiological and/or pathophysiological functions of THs in the CNS and how hypo- and hyper-thyroidism may cause mental disorders.
Introduction
Thyroid hormones (THs; Rothsschild et al., 2006) are critical for the development and function of the central nervous system (CNS; Zoeller and Rovet, 2004; Stenzel and Huttner, 2013). THs regulate development and differentiation of neurons and neuroglia (Gomes et al., 1999; Billon et al., 2001; Lima et al., 2001; Jones et al., 2003; Baxi et al., 2014; Dezonne et al., 2015). There are 2 major types of THs in the CNS represented by L-tri-iodothyronine (T3) and L-thyroxine (T4). The T4 is the major TH secreted by the follicular cells of thyroid gland; whereas T3, the most powerful TH, is mainly produced locally within the brain tissue by 5’-deiodination of T4. The T3 is an active form of the thyroid hormone (TH) essential for the development and function of the CNS.
Hyperthyroidism and hypothyroidism result from overactivation or suppression of thyroid grand leading to either excessive or insufficient production of THs. The prevalence of subclinical hyperthyroidism ranges from 1–15%, and of subclinical hypothyroidism from 3–16% in individuals aged 60 years and older was reported, which also suggested that there are differences in age, gender, and dietary iodine intake in the populations studied (Biondi and Cooper, 2008).
Any impairment of THs supply to the developing CNS causes severe and irreversible changes to the overall architecture and function of human brain, leading to various neurological dysfunctions (Di Liegro, 2008; Henrichs et al., 2010; Duntas and Maillis, 2013). Although in many respects the hypothyroid brain appears morphologically normal, clinical observations reported that hypothyroidism may be associated with both neurological and behavioral abnormalities as well as with functional impairments including mental retardation, ataxia and spasticity (Thompson and Potter, 2000). Psychiatric symptoms of hypothyroidism can include psychosis, mood instability, mania, hypersomnia, apathy, anergia, impaired memory mimicking dementia (Osterweil et al., 1992; Goh et al., 2014), psychomotor slowing, and attentional problems (Awad, 2000). The incidence of hypothyroidism increases with age, and adult-onset hypothyroidism is one of the most common causes of cognitive impairment (Mallett et al., 1995; Dugbartey, 1998).
On the other hand, hyperthyroidism may induce emotional lability, impatience and irritability, distractible overactivity, exaggerated sensitivity to noise, problems with sleep and the appetite (Awad, 2000) or depression and anxiety (Demet et al., 2002). Even at subclinical level, hyperthyroidism in the elderly is suggested to remarkably increase the risk of cognitive decline, dementia and Alzheimer’s disease (AD; Kalmijn et al., 2000; van Osch et al., 2004; Wijsman et al., 2013).
Multiple studies have reported that both hypo- and hyperthyroidism may potentially increase the risk of cognitive impairment and neurodegeneration. It has been also reported that both hyper- and hypothyroidism can affect the immune system (Klecha et al., 2008; De Vito et al., 2012). Hyperthyroidism decreases the proinflammatory activities of monocytes and macrophages. On the other hand, during hypothyroidism enhancement of phagocytosis and increased levels of ROS may occur so that the expression of proinflammatory molecules such as macrophage inflammatory protein-1α and interleukin-1β increases (De Vito et al., 2011). In contrast, hypothyroidism is reported to produce opposite effects on the immune function, such as decrease in immune response, antibody production, cell migration, and lymphocyte proliferation markers (Klecha et al., 2000, 2006), antioxidant enzymes and their activity (De Vito et al., 2011). The role of microglia, an immune cell population in the CNS, in this relationship between thyroid dysfunctions and neuropsychological disorders remains to be elucidated. In addition, knowledge of how other glial cells are involved in neuropsychological disorders, especially in the TH-sensitive regions of the brain (Fonseca et al., 2013), needs to be considered.
Transportation of THs to The Brain and Metabolism in Astrocytes
Circulating T4 is transported across the blood-brain barrier via specific transporters such as organic anion-transporting polypeptides (OATPs) containing OATP14/SLCO1C1 (OATP1c1) (Sugiyama et al., 2003; Tohyama et al., 2004) and OATP1a2 (Gao et al., 2000; Lee et al., 2005; Hagenbuch, 2007), L-type amino acid transporters (LAT1 and LAT2), mainly LAT1 (Taylor and Ritchie, 2007), and monocarboxylate transporters 8 (MCT8) (SLC16A2) (for both T3 and T4) (Roberts et al., 2008). T4 also enters into astroytes through OATP1c1 (Dezonne et al., 2015), where it is de-iodinated by type 2-deiodinase (D2) to produce T3 (Guadaño-Ferraz et al., 1997; Fliers et al., 2006; Di Liegro, 2008). Subsequently T3 is released by LAT (Francon et al., 1989; Blondeau et al., 1993), presumably LAT2, and taken by other cells via distinct transporters; For example neurons express MCT8, while microglia express OATP4a1, LAT2 and MCT10 (Braun et al., 2011; Figure 1).
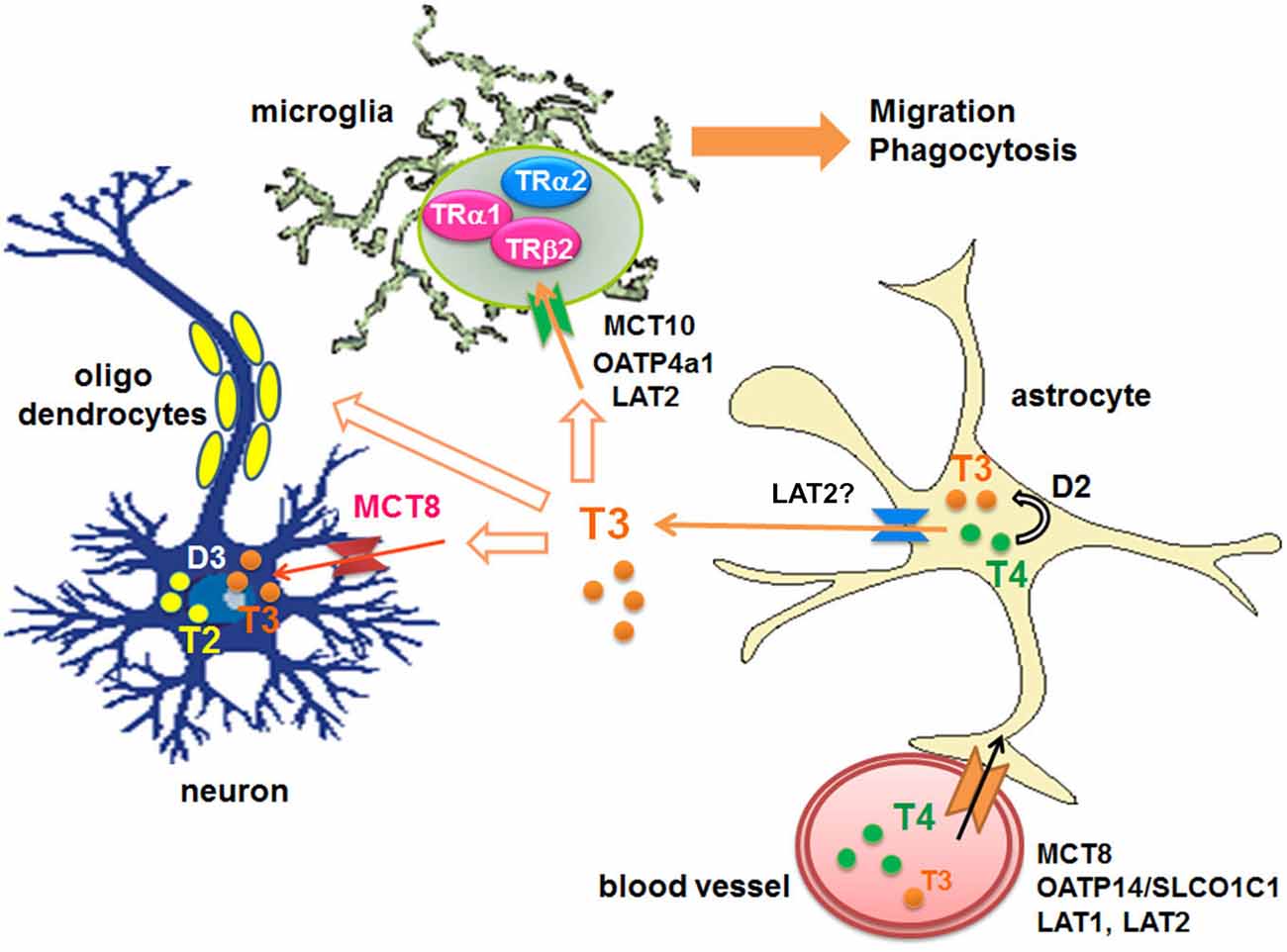
Figure 1. Transport of THs to the brains and their metabolism. D: iodothyronine deiodinases, LAT: L-type amino acid transporter, MCT: monocarboxylate transporter, OATP: organic anion-transporting polypeptide, T2: diiodothyronines, T3: triiodothyronine, T4: thyroxine, TR: thyroid hormone receptor.
Thyroid Hormone Receptors
The majority of TH effects are mediated through TH receptors (TRs), which belong to the members of the nuclear receptor superfamily and which function as T3-inducible transcription factors that are expressed in a tissue-specific and developmentally regulated manner (Cheng et al., 2010). In mammals, there are several TR isoforms: TRα1, TRα2, TRβ1, TRβ2a and TRβ3 (Koenig et al., 1989; Macchia et al., 2001). Among TRs, TRα1 is predominantly and widely expressed in the developing brain. The genomic actions of THs are exerted by the binding of T3 to nuclear TRs, which can either repress or activate gene expression.
Expression of TRα1 and TRβ1 have been identified in primary cultured rat microglia (Lima et al., 2001). Mutation of TRα1 in humans is associated with abnormal levels of TH but normal levels of thyrotropin as well as with growth retardation, and mildly delayed motor and cognitive development (van Mullem et al., 2012). A child with classic features of hypothyroidism with a de novo heterozygous nonsense mutation in a gene encoding TRα was also identified (Bochukova et al., 2012).
In addition to genomic effects of TRs, nongenomic signaling of THs through a plasma membrane–localized receptor has been recently described (Kalyanaraman et al., 2014; Mori et al., 2015). Potential mechanism for integrating regulation of development and metabolism by thyroid hormone and receptor tyrosine kinases through association of TRβ with PI3K was also suggested (Martin et al., 2014). These nongenomic effects of T3 may be important for glial function, which will be discussed later. In addition, it was suggested that the heterogeneity of TR expression throughout brain regions and between different cell types might lead to diverse effects on neuronal morphogenesis. The complexity may also result from not only the direct action of the hormone on neurons but also from indirect actions triggered by astrocytes (Dezonne et al., 2015) or other glial cell type.
Effects of T3 on Microglia
Microglia, the resident macrophages of the CNS are generally considered the primary immune cells of the brain (Kim and de Vellis, 2005). In healthy CNS, ramified microglia are widely distributed to detect any environmental changes by their motile processes (Streit et al., 1988; Kettenmann et al., 2011). Pathological insults of multiple etiologies trigger microglial activation, represented by multi-stage and complex remodeling involving rapid migration towards the lesion site and phagocytosis of damaged cells (Becher et al., 2000; Hanisch and Kettenmann, 2007; Tanaka et al., 2009). It is generally recognized that the microglial phenotype may change depending on the microenvironment, which can be modified by various factors associated with specific types and stages of pathology (Perry et al., 1993; Scheffel et al., 2012; Solito and Sastre, 2012).
Microglial activation contribute to various pathologies (including, for example, Alzheimers disease) (El Khoury and Luster, 2008; Solito and Sastre, 2012). Recently, activated microglia have been indicated to cause also psychiatric disorders. Positron emission tomography imaging and postmortem studies have revealed microglial activation and abnormalities in schizophrenia, depression and autism (Kato et al., 2013; Mizoguchi et al., 2014; Monji et al., 2014).
T3 is important for microglial development (Lima et al., 2001), and could directly or indirectly stimulate morphological maturation of amoeboid microglial cells and limit their degeneration (Mallat et al., 2002). Recently, it has been demonstrated that T3 stimulates microglial migration and phagocytosis in vitro and in vivo (Mori et al., 2015). Microglial migration is mediated through T3 uptake by TH transporters and binding to the TRs. Then TH signaling in microglia involved several signaling pathways including Gi/o-protein, PI3K, and MAPK/ERK, as reported in ATP-induced microglial migration (Honda et al., 2001). T3-induced nitric oxide signaling (Kalyanaraman et al., 2014) is also present in microglia (Mori et al., 2015). In addition, Na+/K+-ATPase, Na+/Ca2+ exchanger operating in the reverse mode, and GABA receptors contribute to T3-induced microglial migration (Figure 2; Mori et al., 2015), although the precise mechanism is still unknown. Since dysfunction of T3 in the aged brain significantly affected microglial morphology (Mori, 2014), microglial dysfunction may be closely related to psychological impairment in hypo- or hyper-thyroidism in elderly patients which will be investigated in the future.
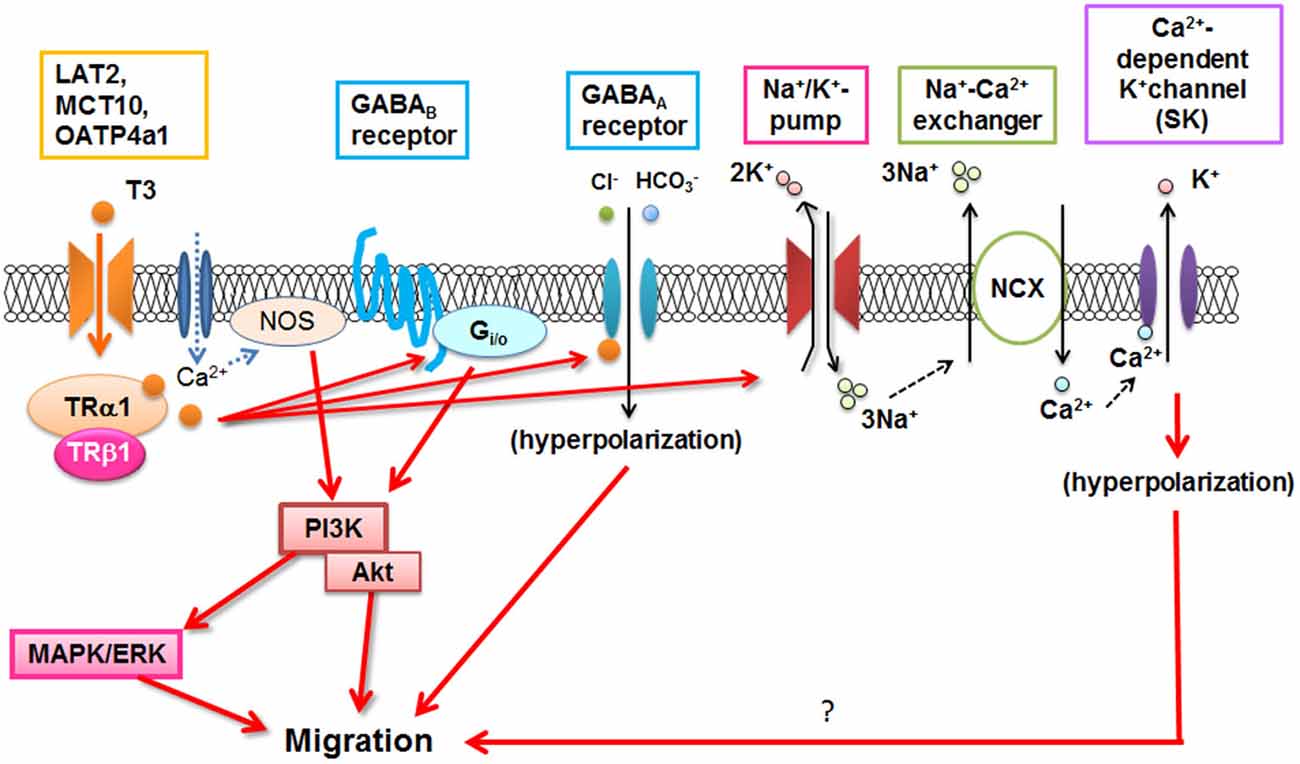
Figure 2. T3-induced signal transduction in microglia. NOS: Nitric oxide synthase, NCX: Na+/Ca2+ exchanger. (Modified from Mori et al., 2015).
Astrocytes and Oligodendrocytes Differentiation by T3
In developing CNS T3 exerts numerous effects regulating axonal myelination and dendritic growth (Walravens and Chase, 1969; Legrand, 1982; Porterfield and Hendrich, 1993; Bernal and Nunez, 1995; Vose et al., 2013) and astrocyte and oligodendrocyte differentiation (Martinez-Galan et al., 1997, 2004; Jones et al., 2003; Schoonover et al., 2004; Manzano et al., 2007; Dezonne et al., 2009; Baxi et al., 2014). Effects of TH on astrocytes have been recently reviewed (Dezonne et al., 2015). Also, differentiation of human cultured CD34+ stem cells into oligodendrocyte precursors under THs action was also reported (Venkatesh et al., 2014). Expression alterations of genes using hypothyroidism model rats showed that immature astrocytes immunoreactive for vimentin and glial fibrillary acidic protein (GFAP) were increased, while oligodendrocyte lineage transcription factor 2 were decreased in the corpus callosum (Shiraki et al., 2014). Effects and molecular mechanisms of T3 action on astrocytes and oligodendrocytes in matured or aged brain remain to be investigated.
Dysfunction of Glial Cells and Psychiatric Symptoms
As mentioned above, THs are not only important for neuronal development (Rami et al., 1986; Gould and Butcher, 1989) but it also support development of microglia (Lima et al., 2001), astrocytes (Gould et al., 1990; Manzano et al., 2007) including radial glial cells (Martinez-Galan et al., 2004), and oligodendrocytes (Walravens and Chase, 1969; Jones et al., 2003). Hypothyroid animals and TR mutant mice exhibit severe deficits in glial development (Morte et al., 2004). Therefore, indirect action of THs that occurs through astrocytes at different stages of brain development may contribute to neuronal progenitor proliferation, neuronal migration and differentiation, axonal growth and synapse function (Lima et al., 1998; Gomes et al., 1999; Martinez and Gomes, 2002; Martinez et al., 2011; Dezonne et al., 2013). Therapeutic use of THs in psychiatric disorders, mainly in depression, came to the light thus contributing to better understanding the action of THs in the brain (Weissel, 1999). Perhaps the major role of thyroxine therapy on depression might be due to hypothalamus-pituitary-thyroid axis activity and serotonin function in depressive episodes (Gomes et al., 2001). Neuroglial cells, as well as neurons contribute to psychiatric symptoms. For example, activated microglia and astrocytes in immunologically induced fatigue (Ifuku et al., 2014), microglial oxidative reactions in schizophrenia (Kato et al., 2011; Monji et al., 2013), and alteration of astrocytes or oligodendrocyte function in bipolar disorder (Dong and Zhen, 2015) have been reported. On the other hand, decreased glial density in association with glial hypotrophy in bipolar disorder or major depression (Rajkowska et al., 2001; Bowley et al., 2002) was also reported. Considering these reports, it is likely that indirect actions of THs through glial cells are important for neuronal activity and their impairment may at least in part, induce psychiatric symptoms.
These psychiatric symptoms can be seen in both hyperthyroidism and hypothyroidism. When it comes to the metabolism and balance of TH levels, it must be noted that propylthiouracyl (PTU) is a thiouracil-derived drug that inhibits thyroid peroxidase and type 1 deiodinase (D1), which is used to treat hyperthyroidism by decreasing TH level by suppression of T4 and T3 production. However, unlike D1 which is expressed mainly in the liver, kidney and testis, the major deiodinase D2 in the brain is known to be insensitive to PTU. Both mRNA concentration and activity of D2 are increased in hypothyroidism (van Doorn et al., 1982, 1983) and decreased in hyperthyroidism (Leonard et al., 1981; van Doorn et al., 1984; Croteau et al., 1996; Burmeister et al., 1997). D2 was also reported to be up-regulated in reactive astrocytes following traumatic brain injury (Zou et al., 1998). Thus, D2 is believed to serve a protective role to preserve the concentration of intracerebral T3 during states of thyroid hormone deficiency. This may explain, in part, why both hypo- and hyperthyroidism cause similar neurological symptoms.
Conclusion
T3 is important not only for neuronal development but also for differentiation of astrocytes and oligodendrocytes, and for microglial development. In addition, T3 is an important signaling factor that affects microglial functions via complex mechanisms. Therefore, dysfunction of THs may impair glial function and thus disturb of the brain, which may cause mental disorders.
Conflict of Interest Statement
The author declares that the research was conducted in the absence of any commercial or financial relationships that could be construed as a potential conflict of interest.
Acknowledgments
I thank Prof. David A. Brown (UCL, UK) and Prof. Alexej Verkhratsky (University of Manchester, UK) for critical reading of the manuscript.
References
Awad, A. G. (2000). The thyroid and the mind and emotions/thyroid dysfunction and mental disorders. Thyrobulletin 7, 3.e1–3.e2.
Baxi, E. G., Schott, J. T., Fairchild, A. N., Kirby, L. A., Karani, R., Uapinyoying, P., et al. (2014). A selective thyroid hormone beta receptor agonist enhances human and rodent oligodendrocyte differentiation. Glia 62, 1513–1529. doi: 10.1002/glia.22697
Becher, B., Prat, A., and Antel, J. P. (2000). Brain-immune connection: immuno-regulatory properties of CNS-resident cells. Glia 29, 293–304. doi: 10.1002/(sici)1098-1136(20000215)29:4<293::aid-glia1>3.3.co;2-1
Bernal, J., and Nunez, J. (1995). Thyroid hormones and brain development. Eur. J. Endocrinol. 133, 390–398. doi: 10.1530/eje.0.1330390
Billon, N., Tokumoto, Y., Forrest, D., and Raff, M. (2001). Role of thyroid hormone receptors in timing oligodendrocyte differentiation. Dev. Biol. 235, 110–120. doi: 10.1006/dbio.2001.0293
Biondi, B., and Cooper, D. S. (2008). The clinical significance of subclinical thyroid dysfunction. Endocr. Rev. 29, 76–131. doi: 10.1210/er.2006-0043
Blondeau, J. P., Beslin, A., Chantoux, F., and Francon, J. (1993). Triiodothyronine is a high-affinity inhibitor of amino acid transport system L1 in cultured astrocytes. J. Neurochem. 60, 1407–1413. doi: 10.1111/j.1471-4159.1993.tb03302.x
Bochukova, E., Schoenmakers, N., Agostini, M., Schoenmakers, E., Rajanayagam, O., Keogh, J. M., et al. (2012). A mutation in the thyroid hormone receptor alpha gene. N. Engl. J. Med. 366, 243–249. doi: 10.1056/NEJMoa1110296
Bowley, M. P., Drevets, W. C., Ongür, D., and Price, J. L. (2002). Low glial numbers in the amygdala in major depressive disorder. Biol. Psychiatry 52, 404–412. doi: 10.1016/s0006-3223(02)01404-x
Braun, D., Kinne, A., Bräuer, A. U., Sapin, R., Klein, M. O., Köhrle, J., et al. (2011). Developmental and cell type-specific expression of thyroid hormone transporters in the mouse brain and in primary brain cells. Glia 59, 463–471. doi: 10.1002/glia.21116
Burmeister, L. A., Pachucki, J., and St Germain, D. L. (1997). Thyroid hormones inhibit type 2 iodothyronine deiodinase in the rat cerebral cortex by both pre- and posttranslational mechanisms. Endocrinology 138, 5231–5237. doi: 10.1210/en.138.12.5231
Cheng, S. Y., Leonard, J. L., and Davis, P. J. (2010). Molecular aspects of thyroid hormone actions. Endocr. Rev. 31, 139–170. doi: 10.1210/er.2009-0007
Croteau, W., Davey, J. C., Galton, V. A., and St Germain, D. L. (1996). Cloning of the mammalian type II iodothyronine deiodinase. A selenoprotein differentially expressed and regulated in human and rat brain and other tissues. J. Clin. Invest. 98, 405–417. doi: 10.1172/jci118806
Demet, M. M., Ozmen, B., Deveci, A., Boyvada, S., Adigüzel, H., and Aydemir, O. (2002). Depression and anxiety in hyperthyroidism. Arch. Med. Res. 33, 552–556. doi: 10.1016/s0188-4409(02)00410-1
De Vito, P., Balducci, V., Leone, S., Percario, Z., Mangino, G., Davis, P. J., et al. (2012). Nongenomic effects of thyroid hormones on the immune system cells: new targets, old players. Steroids 77, 988–995. doi: 10.1016/j.steroids.2012.02.018
De Vito, P., Incerpi, S., Pedersen, J. Z., Luly, P., Davis, F. B., and Davis, P. J. (2011). Thyroid hormones as modulators of immune activities at the cellular level. Thyroid 21, 879–890. doi: 10.1089/thy.2010.0429
Dezonne, R. S., Lima, F. R., Trentin, A. G., and Gomes, F. C. (2015). Thyroid hormone and astroglia: endocrine control of the neural environment. J. Neuroendocrinol. doi: 10.1111/jne.12283 [Epub ahead of print].
Dezonne, R. S., Stipursky, J., Araujo, A. P., Nones, J., Pavão, M. S., Porcionatto, M., et al. (2013). Thyroid hormone treated astrocytes induce maturation of cerebral cortical neurons through modulation of proteoglycan levels. Front. Cell. Neurosci. 7:125. doi: 10.3389/fncel.2013.00125
Dezonne, R. S., Stipursky, J., and Gomes, F. C. (2009). Effect of thyroid hormone depletion on cultured murine cerebral cortex astrocytes. Neurosci. Lett. 467, 58–62. doi: 10.1016/j.neulet.2009.10.001
Di Liegro, I. (2008). Thyroid hormones and the central nervous system of mammals (Review). Mol. Med. Rep. 1, 279–295. doi: 10.3892/mmr.1.3.279
Dong, X. H., and Zhen, X. C. (2015). Glial pathology in bipolar disorder: potential therapeutic implications. CNS Neurosci. Ther. 21, 393–397. doi: 10.1111/cns.12390
Dugbartey, A. T. (1998). Neurocognitive aspects of hypothyroidism. Arch. Intern. Med. 158, 1413–1418. doi: 10.1001/archinte.158.13.1413
Duntas, L. H., and Maillis, A. (2013). Hypothyroidism and depression: salient aspects of pathogenesis and management. Minerva Endocrinol. 38, 365–377. doi: 10.1155/2013/312378
El Khoury, J., and Luster, A. D. (2008). Mechanisms of microglia accumulation in Alzheimer’s disease: therapeutic implications. Trends Pharmacol. Sci. 29, 626–632. doi: 10.1016/j.tips.2008.08.004
Fliers, E., Unmehopa, U. A., and Alkemade, A. (2006). Functional neuroanatomy of thyroid hormone feedback in the human hypothalamus and pituitary gland. Mol. Cell. Endocrinol. 251, 1–8. doi: 10.1016/j.mce.2006.03.042
Fonseca, T. L., Correa-Medina, M., Campos, M. P. O., Wittmann, G., Werneck-de-Castro, J. P., Arrojo e Drigo, R., et al. (2013). Coordination of hypothalamic and pituitary T3 production regulates TSH expression. J. Clin. Invest. 123, 1492–1500. doi: 10.1172/JCI61231
Francon, J., Chantoux, F., and Blondeau, J. P. (1989). Carrier-mediated transport of thyroid hormones into rat glial cells in primary culture. J. Neurochem. 53, 1456–1463. doi: 10.1111/j.1471-4159.1989.tb08538.x
Gao, B., Hagenbuch, B., Kullak-Ublick, G. A., Benke, D., Aguzzi, A., and Meier, P. J. (2000). Organic anion-transporting polypeptides mediate transport of opioid peptides across blood-brain barrier. J. Pharmacol. Exp. Ther. 294, 73–79.
Goh, K. K., Chiu, Y. H., and Shen, W. W. (2014). Hashimoto’s encephalopathy mimicking presenile dementia. Gen. Hosp. Psychiatry 36, 360.e9–360.e11. doi: 10.1016/j.genhosppsych.2014.01.006
Gomes, F. C., Lima, F. R., Trentin, A. G., and Moura Neto, V. (2001). Thyroid hormone role in nervous system morphogenesis. Prog. Brain Res. 132, 41–50. doi: 10.1016/s0079-6123(01)32064-2
Gomes, F. C., Maia, C. G., de Menezes, J. R., and Neto, V. M. (1999). Cerebellar astrocytes treated by thyroid hormone modulate neuronal proliferation. Glia 25, 247–255. doi: 10.1002/(sici)1098-1136(19990201)25:3<247::aid-glia5>3.3.co;2-u
Gould, E., and Butcher, L. L. (1989). Developing cholinergic basal forebrain neurons are sensitive to thyroid hormone. J. Neurosci. 9, 3347–3358.
Gould, E., Frankfurt, M., Westlind-Danielsson, A., and McEwen, B. S. (1990). Developing forebrain astrocytes are sensitive to thyroid hormone. Glia 3, 283–292. doi: 10.1002/glia.440030408
Guadaño-Ferraz, A., Obregón, M. J., StGermain, D. L., and Bernal, J. (1997). The type 2 iodothyronine deiodinase is expressed primarily in glial cells in the neonatal rat brain. Proc. Natl. Acad. Sci. U S A 94, 10391–10396. doi: 10.1073/pnas.94.19.10391
Hagenbuch, B. (2007). Cellular entry of thyroid hormones by organic anion transporting polypeptides. Best Pract. Res. Clin. Endocrinol. Metab. 21, 209–221. doi: 10.1016/j.beem.2007.03.004
Hanisch, U. K., and Kettenmann, H. (2007). Microglia: active sensor and versatile effector cells in the normal and pathologic brain. Nat. Neurosci. 10, 1387–1394. doi: 10.1038/nn1997
Henrichs, J., Bongers-Schokking, J. J., Schenk, J. J., Ghassabian, A., Schmidt, H. G., Visser, T. J., et al. (2010). Maternal thyroid function during early pregnancy and cognitive functioning in early childhood: the generation R study. J. Clin. Endocrinol. Metab. 95, 4227–4234. doi: 10.1210/jc.2010-0415
Honda, S., Sasaki, Y., Ohsawa, K., Imai, Y., Nakamura, Y., Inoue, K., et al. (2001). Extracellular ATP or ADP induce chemotaxis of cultured microglia through Gi/o-coupled P2Y receptors. J. Neurosci. 21, 1975–1982.
Ifuku, M., Hossain, S. M., Noda, M., and Katafuchi, T. (2014). Induction of interleukin-1beta by activated microglia is a prerequisite for immunologically induced fatigue. Eur. J. Neurosci. 40, 3253–3263. doi: 10.1111/ejn.12668
Jones, S. A., Jolson, D. M., Cuta, K. K., Mariash, C. N., and Anderson, G. W. (2003). Triiodothyronine is a survival factor for developing oligodendrocytes. Mol. Cell. Endocrinol. 199, 49–60. doi: 10.1016/s0303-7207(02)00296-4
Kalmijn, S., Mehta, K. M., Pols, H. A., Hofman, A., Drexhage, H. A., and Breteler, M. M. (2000). Subclinical hyperthyroidism and the risk of dementia. The Rotterdam study. Clin. Endocrinol. (Oxf) 53, 733–737. doi: 10.1046/j.1365-2265.2000.01146.x
Kalyanaraman, H., Schwappacher, R., Joshua, J., Zhuang, S., Scott, B. T., Klos, M., et al. (2014). Nongenomic thyroid hormone signaling occurs through a plasma membrane-localized receptor. Sci. Signal. 7:ra48. doi: 10.1126/scisignal.2004911
Kato, T. A., Monji, A., Yasukawa, K., Mizoguchi, Y., Horikawa, H., Seki, Y., et al. (2011). Aripiprazole inhibits superoxide generation from phorbol-myristate-acetate (PMA)-stimulated microglia in vitro: implication for antioxidative psychotropic actions via microglia. Schizophr. Res. 129, 172–182. doi: 10.1016/j.schres.2011.03.019
Kato, T. A., Yamauchi, Y., Horikawa, H., Monji, A., Mizoguchi, Y., Seki, Y., et al. (2013). Neurotransmitters, psychotropic drugs and microglia: clinical implications for psychiatry. Curr. Med. Chem. 20, 331–344. doi: 10.2174/0929867311320030003
Kettenmann, H., Hanisch, U. K., Noda, M., and Verkhratsky, A. (2011). Physiology of microglia. Physiol. Rev. 91, 461–553. doi: 10.1152/physrev.00011.2010
Kim, S. U., and de Vellis, J. (2005). Microglia in health and disease. J. Neurosci. Res. 81, 302–313. doi: 10.1002/jnr.20562
Klecha, A. J., Barreiro Arcos, M. L., Frick, L., Genaro, A. M., and Cremaschi, G. (2008). Immune-endocrine interactions in autoimmune thyroid diseases. Neuroimmunomodulation 15, 68–75. doi: 10.1159/000135626
Klecha, A. J., Genaro, A. M., Gorelik, G., Barreiro Arcos, M. L., Silberman, D. M., Schuman, M., et al. (2006). Integrative study of hypothalamus-pituitary-thyroid-immune system interaction: thyroid hormone-mediated modulation of lymphocyte activity through the protein kinase C signaling pathway. J. Endocrinol. 189, 45–55. doi: 10.1677/joe.1.06137
Klecha, A. J., Genaro, A. M., Lysionek, A. E., Caro, R. A., Coluccia, A. G., and Cremaschi, G. A. (2000). Experimental evidence pointing to the bidirectional interaction between the immune system and the thyroid axis. Int. J. Immunopharmacol. 22, 491–500. doi: 10.1016/s0192-0561(00)00012-6
Koenig, R. J., Lazar, M. A., Hodin, R. A., Brent, G. A., Larsen, P. R., Chin, W. W., et al. (1989). Inhibition of thyroid-hormone action by a non-hormone binding c-erbA protein generated by alternative mRNA Splicing. Nature 337, 659–661. doi: 10.1038/337659a0
Lee, W., Glaeser, H., Smith, L. H., Roberts, R. L., Moeckel, G. W., Gervasini, G., et al. (2005). Polymorphisms in human organic anion-transporting polypeptide 1A2 (OATP1A2): implications for altered drug disposition and central nervous system drug entry. J. Biol. Chem. 280, 9610–9617. doi: 10.1074/jbc.m411092200
Legrand, J. (1982). [Thyroid hormones and maturation of the nervous system]. J. Physiol. (Paris) 78, 603–652.
Leonard, J. L., Kaplan, M. M., Visser, T. J., Silva, J. E., and Larsen, P. R. (1981). Cerebral cortex responds rapidly to thyroid hormones. Science 214, 571–573. doi: 10.1126/science.7291997
Lima, F. R., Gervais, A., Colin, C., Izembart, M., Neto, V. M., and Mallat, M. (2001). Regulation of microglial development: a novel role for thyroid hormone. J. Neurosci. 21, 2028–2038.
Lima, F. R., Goncalves, N., Gomes, F. C., de Freitas, M. S., and Moura Neto, V. (1998). Thyroid hormone action on astroglial cells from distinct brain regions during development. Int. J. Dev. Neurosci. 16, 19–27. doi: 10.1016/s0736-5748(98)00002-1
Macchia, P. E., Takeuchi, Y., Kawai, T., Cua, K., Gauthier, K., Chassande, O., et al. (2001). Increased sensitivity to thyroid hormone in mice with complete deficiency of thyroid hormone receptor alpha. Proc. Natl. Acad. Sci. U S A 98, 349–354. doi: 10.1073/pnas.011306998
Mallat, M., Lima, F. R., Gervais, A., Colin, C., and Moura Neto, V. (2002). New insights into the role of thyroid hormone in the CNS: the microglial track. Mol. Psychiatry 7, 7–8. doi: 10.1038/sj/mp/4000988
Mallett, P., Andrew, M., Hunter, C., Smith, J., Richards, C., Othman, S., et al. (1995). Cognitive function, thyroid status and postpartum depression. Acta Psychiatr. Scand. 91, 243–246. doi: 10.1111/j.1600-0447.1995.tb09776.x
Manzano, J., Bernal, J., and Morte, B. (2007). Influence of thyroid hormones on maturation of rat cerebellar astrocytes. Int. J. Dev. Neurosci. 25, 171–179. doi: 10.1016/j.ijdevneu.2007.01.003
Martin, N. P., Marron Fernandez de Velasco, E., Mizuno, F., Scappini, E. L., Gloss, B., Erxleben, C., et al. (2014). A rapid cytoplasmic mechanism for PI3 kinase regulation by the nuclear thyroid hormone receptor, TRbeta and genetic evidence for its role in the maturation of mouse hippocampal synapses in vivo. Endocrinology 155, 3713–3724. doi: 10.1210/en.2013-2058
Martinez, R., Eller, C., Viana, N. B., and Gomes, F. C. (2011). Thyroid hormone induces cerebellar neuronal migration and Bergmann glia differentiation through epidermal growth factor/mitogen-activated protein kinase pathway. Eur. J. Neurosci. 33, 26–35. doi: 10.1111/j.1460-9568.2010.07490.x
Martinez, R., and Gomes, F. C. (2002). Neuritogenesis induced by thyroid hormone-treated astrocytes is mediated by epidermal growth factor/mitogen-activated protein kinase-phosphatidylinositol 3-kinase pathways and involves modulation of extracellular matrix proteins. J. Biol. Chem. 277, 49311–49318. doi: 10.1074/jbc.m209284200
Martinez-Galan, J. R., Escobar del Rey, F., Morreale de Escobar, G., Santacana, M., and Ruiz-Marcos, A. (2004). Hypothyroidism alters the development of radial glial cells in the term fetal and postnatal neocortex of the rat. Brain Res. Dev. Brain Res. 153, 109–114. doi: 10.1016/j.devbrainres.2004.08.002
Martinez-Galan, J. R., Pedraza, P., Santacana, M., Escobar del Ray, F., Morreale de Escobar, G., and Ruiz-Marcos, A. (1997). Early effects of iodine deficiency on radial glial cells of the hippocampus of the rat fetus. A model of neurological cretinism. J. Clin. Invest. 99, 2701–2709. doi: 10.1172/jci119459
Mizoguchi, Y., Kato, T. A., Horikawa, H., and Monji, A. (2014). Microglial intracellular Ca(2+) signaling as a target of antipsychotic actions for the treatment of schizophrenia. Front. Cell. Neurosci. 8:370. doi: 10.3389/fncel.2014.00370
Monji, A., Kato, T. A., Mizoguchi, Y., Horikawa, H., Seki, Y., Kasai, M., et al. (2013). Neuroinflammation in schizophrenia especially focused on the role of microglia. Prog. Neuropsychopharmacol. Biol. Psychiatry 42, 115–121. doi: 10.1016/j.pnpbp.2011.12.002
Monji, A., Mizoguchi, Y., and Kato, T. A. (2014). Microglial abnormalities in the pathophysiology of schizophrenia. J. Neurol. Disord. Stroke 2, 1059.e1–1059.e11.
Mori, Y. (2014). Association between thyroid hormones and brain function via regulation of microglial migration and phagocytosis. PhD Thesis (Kyushu University, Graduate School of Pharmaceutical Sciences), 1–60.
Mori, Y., Tomonaga, D., Kalashnikova, A., Furuya, F., Akimoto, N., Ifuku, M., et al. (2015). Effects of 3, 3’, 5-triiodothyronine on microglial functions. Glia 63, 906–920. doi: 10.1002/glia.22792
Morte, B., Manzano, J., Scanlan, T. S., Vennstrom, B., and Bernal, J. (2004). Aberrant maturation of astrocytes in thyroid hormone receptor alpha 1 knockout mice reveals an interplay between thyroid hormone receptor isoforms. Endocrinology 145, 1386–1391. doi: 10.1210/en.2003-1123
Osterweil, D., Syndulko, K., Cohen, S. N., Pettler-Jennings, P. D., Hershman, J. M., Cummings, J. L., et al. (1992). Cognitive function in non-demented older adults with hypothyroidism. J. Am. Geriatr. Soc. 40, 325–335. doi: 10.1111/j.1532-5415.1992.tb02130.x
Perry, V. H., Matyszak, M. K., and Fearn, S. (1993). Altered antigen expression of microglia in the aged rodent CNS. Glia 7, 60–67. doi: 10.1002/glia.440070111
Porterfield, S. P., and Hendrich, C. E. (1993). The role of thyroid hormones in prenatal and neonatal neurological development—current perspectives. Endocr. Rev. 14, 94–106. doi: 10.1210/er.14.1.94
Rajkowska, G., Halaris, A., and Selemon, L. D. (2001). Reductions in neuronal and glial density characterize the dorsolateral prefrontal cortex in bipolar disorder. Biol. Psychiatry 49, 741–752. doi: 10.1016/s0006-3223(01)01080-0
Rami, A., Patel, A. J., and Rabié, A. (1986). Thyroid hormone and development of the rat hippocampus: morphological alterations in granule and pyramidal cells. Neuroscience 19, 1217–1226. doi: 10.1016/0306-4522(86)90135-1
Roberts, L. M., Woodford, K., Zhou, M., Black, D. S., Haggerty, J. E., Tate, E. H., et al. (2008). Expression of the thyroid hormone transporters monocarboxylate transporter-8 (SLC16A2) and organic ion transporter-14 (SLCO1C1) at the blood-brain barrier. Endocrinology 149, 6251–6261. doi: 10.1210/en.2008-0378
Rothsschild, B. L., Shim, A. H., Ammer, A. G., Kelley, L. C., Irby, K. B., Head, J. A., et al. (2006). Cortactin overexpression regulates actin-related protein 2/3 complex activity, motility and invasion in carcinomas with chromosome 11q13 amplification. Cancer Res. 66, 8017–8025. doi: 10.1158/0008-5472.can-05-4490
Scheffel, J., Regen, T., Van Rossum, D., Seifert, S., Ribes, S., Nau, R., et al. (2012). Toll-like receptor activation reveals developmental reorganization and unmasks responder subsets of microglia. Glia 60, 1930–1943. doi: 10.1002/glia.22409
Schoonover, C. M., Seibel, M. M., Jolson, D. M., Stack, M. J., Rahman, R. J., Jones, S. A., et al. (2004). Thyroid hormone regulates oligodendrocyte accumulation in developing rat brain white matter tracts. Endocrinology 145, 5013–5020. doi: 10.1210/en.2004-0065
Shiraki, A., Saito, F., Akane, H., Takeyoshi, M., Imatanaka, N., Itahashi, M., et al. (2014). Expression alterations of genes on both neuronal and glial development in rats after developmental exposure to 6-propyl-2-thiouracil. Toxic. Lett. 228, 225–234. doi: 10.1016/j.toxlet.2014.04.018
Solito, E., and Sastre, M. (2012). Microglia function in Alzheimer’s disease. Front. Pharmacol. 3:14. doi: 10.3389/fphar.2012.00014
Stenzel, D., and Huttner, W. B. (2013). Role of maternal thyroid hormones in the developing neocortex and during human evolution. Front. Neuroanat. 7:19. doi: 10.3389/fnana.2013.00019
Streit, W. J., Graeber, M. B., and Kreutzberg, G. W. (1988). Functional plasticity of microglia: a review. Glia 1, 301–307. doi: 10.1002/glia.440010502
Sugiyama, D., Kusuhara, H., Taniguchi, H., Ishikawa, S., Nozaki, Y., Aburatani, H., et al. (2003). Functional characterization of rat brain-specific organic anion transporter (Oatp14) at the blood-brain barrier: high affinity transporter for thyroxine. J. Biol. Chem. 278, 43489–43495. doi: 10.1074/jbc.m306933200
Tanaka, T., Ueno, M., and Yamashita, T. (2009). Engulfment of axon debris by microglia requires p38 MAPK activity. J. Biol. Chem. 284, 21626–21636. doi: 10.1074/jbc.m109.005603
Taylor, P. M., and Ritchie, J. W. (2007). Tissue uptake of thyroid hormone by amino acid transporters. Best Pract. Res. Clin. Endocrinol. Metab. 21, 237–251. doi: 10.1016/j.beem.2007.03.002
Thompson, C. C., and Potter, G. B. (2000). Thyroid hormone action in neural development. Cereb. Cortex 10, 939–945. doi: 10.1093/cercor/10.10.939
Tohyama, K., Kusuhara, H., and Sugiyama, Y. (2004). Involvement of multispecific organic anion transporter, Oatp14 (Slc21a14), in the transport of thyroxine across the blood-brain barrier. Endocrinology 145, 4384–4391. doi: 10.1210/en.2004-0058
van Doorn, J., Roelfsema, F., and van der Heide, D. (1982). Contribution fron local conversion of thyroxine to 3,5,3′-triiodothyronine to intracellular 3,5,3′-triiodothyronine in several organs in hypothyroid rats at isotope equilibrium. Acta Endocrinol. (Copenh) 101, 386–396. doi: 10.1530/acta.0.1010386
van Doorn, J., van der Heide, D., and Roelfsema, F. (1983). Sources and quantity of 3,5,3′-triiodothyronine in several tissues of the rat. J. Clin. Invest. 72, 1778–1792. doi: 10.1172/jci111138
van Doorn, J., van der Heide, D., and Roelfsema, F. (1984). The contribution of local thyroxine monodeiodination to intracellular 3,5, 3′-triiodothyronine in several tissues of hyperthyroid rats at isotopic equilibrium. Endocrinology 115, 174–182. doi: 10.1210/endo-115-1-174
van Mullem, A., van Heerebeek, R., Chrysis, D., Visser, E., Medici, M., Andrikoula, M., et al. (2012). Clinical phenotype and mutant TR alpha 1. N. Engl. J. Med. 366, 1451–1453. doi: 10.1056/NEJMc1113940
van Osch, L. A., Hogervorst, E., Combrinck, M., and Smith, A. D. (2004). Low thyroid-stimulating hormone as an independent risk factor for Alzheimer disease. Neurology 62, 1967–1971. doi: 10.1212/01.wnl.0000128134.84230.9f
Venkatesh, K., Srikanth, L., Vengamma, B., Chandrasekhar, C., Prasad, B. C., and Sarma, P. V. (2014). In vitro transdifferentiation of human cultured CD34+ stem cells into oligodendrocyte precursors using thyroid hormones. Neurosci. Lett. 588, 36–41. doi: 10.1016/j.neulet.2014.12.050
Vose, L. R., Vinukonda, G., Jo, S., Miry, O., Diamond, D., Korumilli, R., et al. (2013). Treatment with thyroxine restores myelination and clinical recovery after intraventricular hemorrhage. J. Neurosci. 33, 17232–17246. doi: 10.1523/jneurosci.2713-13.2013
Walravens, P., and Chase, H. P. (1969). Influence of thyroid on formation of myelin lipids. J. Neurochem. 16, 1477–1484. doi: 10.1111/j.1471-4159.1969.tb09900.x
Weissel, M. (1999). [Administration of thyroid hormones in therapy of psychiatric illnesses]. Acta Med. Austriaca 26, 129–131.
Wijsman, L. W., de Craen, A. J., Trompet, S., Gussekloo, J., Stott, D. J., Rodondi, N., et al. (2013). Subclinical thyroid dysfunction and cognitive decline in old age. PLoS One 8:e59199. doi: 10.1371/journal.pone.0059199
Zoeller, R. T., and Rovet, J. (2004). Timing of thyroid hormone action in the developing brain: clinical observations and experimental findings. J. Neuroendocrinol. 16, 809–818. doi: 10.1111/j.1365-2826.2004.01243.x
Keywords: thyroid hormones, triiodothyronine, microglia, migration, phagocytosis
Citation: Noda M (2015) Possible role of glial cells in the relationship between thyroid dysfunction and mental disorders. Front. Cell. Neurosci. 9:194. doi: 10.3389/fncel.2015.00194
Received: 08 January 2015; Accepted: 04 May 2015;
Published online: 03 June 2015.
Edited by:
Johann Steiner, University of Magdeburg, GermanyReviewed by:
Andrew MacLean, Tulane University School of Medicine, USAAkira Monji, Saga University, Japan
Copyright © 2015 Noda. This is an open-access article distributed under the terms of the Creative Commons Attribution License (CC BY). The use, distribution and reproduction in other forums is permitted, provided the original author(s) or licensor are credited and that the original publication in this journal is cited, in accordance with accepted academic practice. No use, distribution or reproduction is permitted which does not comply with these terms.
*Correspondence: Mami Noda, Laboratory of Pathophysiology, Graduate School of Pharmaceutical Sciences, Kyushu University, 3-1-1 Maidashi, Higashi-ku, Fukuoka 812-8582, Japan,bm9kYUBwaGFyLmt5dXNodS11LmFjLmpw