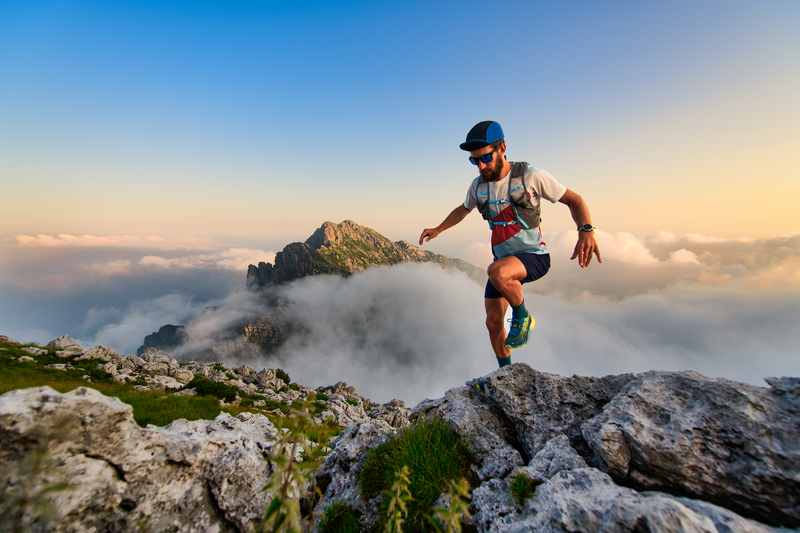
95% of researchers rate our articles as excellent or good
Learn more about the work of our research integrity team to safeguard the quality of each article we publish.
Find out more
ORIGINAL RESEARCH article
Front. Cell. Neurosci. , 06 May 2015
Sec. Cellular Neuropathology
Volume 9 - 2015 | https://doi.org/10.3389/fncel.2015.00172
This article is part of the Research Topic CNS recovery after structural and / or physiological / psychological damage View all 11 articles
The central nervous system (CNS) monitors modifications in metabolic parameters or hormone levels and elicits adaptive responses such as food intake regulation. Particularly, within the hypothalamus, leptin modulates the activity of pro-opiomelanocortin (POMC) neurons which are critical regulators of energy balance. Consistent with a pivotal role of the melanocortin system in the control of energy homeostasis, disruption of the POMC gene causes hyperphagia and obesity. MicroRNAs (miRNAs) are short noncoding RNA molecules that post-transcriptionally repress the expression of genes by binding to 3′-untranslated regions (3′UTR) of the target mRNAs. However, little is known regarding the role of miRNAs that target POMC 3′UTR in the central control energy homeostasis. Particularly, their interaction with the leptin signaling pathway remain unclear. First, we used common prediction programs to search for potential miRNAs target sites on 3′UTR of POMC mRNA. This screening identified a set of conserved miRNAs seed sequences for mir-383, mir-384-3p, and mir-488. We observed that mir-383, mir-384-3p, and mir-488 are up-regulated in the hypothalamus of leptin deficient ob/ob mice. In accordance with these observations, we also showed that mir-383, mir-384-3p, and mir-488 were increased in db/db mice that exhibit a non-functional leptin receptor. The intraperitoneal injection of leptin down-regulated the expression of these miRNAs of interest in the hypothalamus of ob/ob mice showing the involvement of leptin in the expression of mir-383, mir-384-3p, and mir-488. Finally, the evaluation of responsivity to intracerebroventricular administration of leptin exhibited that a chronic treatment with leptin decreased mir-488 expression in hypothalamus of C57BL/6 mice. In summary, these results suggest that leptin modulates the expression of miRNAs that target POMC mRNA in hypothalamus.
The control of energy homeostasis is finely tuned by endocrine and neural mechanisms that cooperate to maintain the balance between caloric intake and energy expenditure. In this respect, the central nervous system (CNS) continuously monitors modifications in metabolic parameters (blood glucose) and/or hormones (insulin or leptin) and elicits adaptive responses such as food intake regulation and autonomic nervous system modulation (Cowley et al., 2001; Ibrahim et al., 2003; Plum et al., 2006; Mounien et al., 2010). It is now clearly established that specific neuronal networks of the hypothalamus play a pivotal role in energy homeostasis regulation (Morton et al., 2006). For instance, within the arcuate nucleus of the hypothalamus, pro-opiomelanocortin (POMC) neurons are critical regulators of energy balance and glucose homeostasis (Porte et al., 2002; Mounien et al., 2005, 2009, 2010; Parton et al., 2007; Hill et al., 2010). In accordance with this aspect, it has been shown that the disruption of the POMC and melanocortin receptor 4 (MC4R) genes in mice models causes obesity (Huszar et al., 1997; Yaswen et al., 1999), while MC3R gene-deficient mice have normal food consumption but accumulate fat (Chen et al., 2000). In humans, obesity can result from genetic deficiencies which produce a lack in the leptin receptor, POMC, or MC3/4R (Lee, 2009).
One important goal of current research is to identify the molecular mechanisms involved in the control of the expression of genes that are important to maintain energy homeostasis. It has long been acknowledged that the leptin acts as a key regulator of hypothalamic genes expression via different signaling cascades (Morton et al., 2006). For instance, when the leptin binds to the extracellular domain of its receptor (LepR), it recruits and activates the Janus kinase (JAK). JAK binds to and phosphorylates LepR at the same time. This mechanism activates signal transducer and activator of transcription 3 (STAT3). Once phosphorylated, STAT3 binds to POMC promoters, stimulating POMC expression (Morton et al., 2006). Interestingly, mice with genetic inactivation of STAT3 gain body weight (Gao et al., 2004). Altogether, the above collected data strongly suggest that the stability of energy homeostasis during environmental variation requires metabolic adjustements that are achieved through a fine regulation of genes's expression.
Because microRNAs (miRNAs) have been depicted to be another layer of gene regulation, it is not surprising that they are also involved in leptin-regulated gene expression. The miRNAs are endogenous, single-stranded, small, ~22-nucleotides noncoding RNAs, and are generally regarded as negative regulators of gene expression because they inhibe translation and/or promot mRNA degradation by base pairing to complementary sequences within the 3′untranslated region (3′UTR) of protein-coding mRNA transcripts (Bagga et al., 2005). Several studies identified miRNAs that are differentially expressed in the liver, pancreas, and adipose tissue of leptin-deficient (ob/ob) or leptin receptor-deficient (db/db) mice compared to the control animals (Lovis et al., 2008; Li et al., 2009; Nakanishi et al., 2009; Xie et al., 2009). Among these miRNAs, it has been shown that the pancreatic expression levels of mir-375 are aberrant in ob/ob mice, indicating that they contribute to insulin resistance in this model (Poy et al., 2009). Additional studies provide evidence for the involvement of mir-335 in lipid metabolism of the liver and the adipose tissue of ob/ob and db/db mice (Nakanishi et al., 2009). In the context of CNS, it has been shown that mir-200a, mir-200b, and mir-429 are up-regulated in the hypothalamus of ob/ob and db/db mice (Crépin et al., 2014).
Recently, it has been shown that conditional deletion of the RNAse III ribonuclease Dicer (involved in miRNAs maturation) from POMC-expressing cells results in obesity and diabetes which is associated with a neurodegenerescence of POMC neurons in the hypothalamus (Schneeberger et al., 2012; Greenman et al., 2013). These observations strongly suggest that miRNAs are important regulators of POMC neuron activity. In this context, the characterization of the miRNAs that target directly POMC mRNA and their interaction with the leptin signaling pathway remain unclear.
In the present study, we focused our attention on the specific miRNAs targeting POMC 3′UTR. Based on bioinformatic predictions of their involvement in POMC-signaling pathway and their conservation among vertebrates, the expression of mir-383, mir-384-3p, and mir-488 were investigated in models of obesity characterized by a decrease of POMC mRNA expression and leptin insufficiency (ob/ob) or leptin insensitivity (db/db) (Mizuno et al., 1998). Then, we further analyzed the role of leptin on the expression level of these miRNAs using different models of leptin-treated mice.
Experiments were carried out on different types of mice: C57BL/6, ob/ob, and db/db mice were purchased from Charles River (France). Fluorescence in situ hybridization (FISH) experiments were performed using male POMC-Tau-Topaz GFP transgenic mice developed by Pinto et al. (2004). To assess GFP expression in POMC-Tau-Topaz GFP mice, we carried out PCR on tail genomic DNA. GFP transgene was detected using the forward primer 5′-GCCACAAGTTCAGCGTGTCC-3′ and the reverse primer 5′-GCTTCTCGTTGGGGTCTTTGC-3′, with the following PCR conditions: 5 min at 95°C, 36 cycles at 95°C for 30 s, 64°C for 30 s, and 72°C for 40 s, followed by a final step at 72°C for 7 min. The amplicon size was 573 bp.
All animals were individually housed in a pathogen-free facility at controlled temperature on a 12/12 h light/dark cycle (lights from 0700 to 1900 h) with standard pellet diet (AO4) and water available ad libitum. All experiments were conducted in conformity with the rules set by the EC Council Directive (2010/63/UE) and the French “Direction Départementale de la Protection des Populations des Bouches-du-Rhône” (License no. 13.435 and no. 13.430). Protocols used are in agreement with the rules set by the Comité d'Ethique de Marseille, our local Committee for Animal Care and Research. Every precaution was taken to minimize animal stress and the number of animals used.
To search for miRNAs that might regulate mouse POMC expression, we used the following public prediction algorithms and database: Targetscan (http://www.targetscan.org/) and miRanda (http://www.microrna.org/microrna/home.do). Using these different algorithms, we selected the miRNAs that are conserved among the vertebrates (Targetscan) and that have a good mirSVR scores (miRanda).
For the intraperitoneal injection (i.p.), ob/ob, and C57BL/6 mice were injected between 1100 and 1200 h with recombinant murine leptin (Peprotech, France) (n = 5, 5 mg/kg) or saline. Mice were sacrified 4 h after i.p. injection.
Intracerebroventricular (i.c.v) cannula placement and injections were performed as described previously (Girardet et al., 2011). Animals were anesthetized by an i.p. injection of ketamine (100 mg/kg; Imalgen 1000, Merial, France) and xylazine (6 mg/kg; Rompun, Bayer, France), and placed in a digital stereotaxic apparatus (Model 502600, WPI) coupled to the neurostar software (Neurostar GmbH, Germany). A 26-gauge stainless steel cannula was implanted into the lateral ventricle at the following coordinates: 0.3 mm posterior to bregma, 1.1 mm lateral to the midline, and 2.6 mm ventral to the skull surface. The cannula was secured to the skull with dental cement and sealed with removable obturators. The animals were sutured, placed in individual cages and allowed to recover for 7 days. During this recovery period, animals were injected with physiological saline every day for habituation. One week post-surgery, mice were administered either 10 μl (2 μl/min) of physiological saline or leptin (0.5 μg/μl) solution at the beginning of the dark phase. The correct cannula positioning was checked for each animal at the end of the experiment by cresyl violet staining of brain sections (Supplementary Figure 1).
The exogenous leptin was detected in the hypothalamus of ob/ob and C57BL/6 mice after i.p. and i.c.v injection by western blotting (Supplementary Figure 2 and Supplementary Material).
Mice were killed by decapitation and the different brain regions were collected and frozen in liquid nitrogen and stored at −80°C until protein or RNA extraction. Total RNA was extracted with TRI Reagent (Sigma-Aldrich, France). For RNA quantification, cDNA was synthetized by 2 μg total RNA with M-MLV Reverse Transcriptase (Promega Corporation, WI, USA). For miRNAs quantification, cDNA was synthetized by 1 μg total RNA by the qScript microRNA Quantification System (Quanta Biosciences, MD, USA). For real-time PCR, we used LightCycler 480 (Roche, Germany). We used Pomc forward primer 5′-TGAACATCTTTGTCCCCAGAGA-3′ and reverse 5′-TGCAGAGGCAAACAAGATTGG-3′; β Actin forward primer 5′-GATCTGGCACCACACCTTCTACA-3′ and reverse 5′- TGGCGTGAGGGAGAGCATAG-3′; Gapdh forward primer 5′- TTCTCAAGCTCATTTCCTGGTATG-3′ and reverse primer 5′- GGATAGGGCCTCTCTTGCTCA-3′. PCR was initiated by one cycle of 95°C for 10 min, followed by 40 cycles of 10 s at 95°C, 30 s at 60°C, and 2 s at 72°C, followed by a holding at 40°C. For miRNAs, U6 and Sno202 were used as normalizers for miRNA quantification. For U6 we used forward primer 5′-ATTGGAACGATACAGAGAAGATT-3′ and reverse primer 5′-GGAACGCTTCACGAATTTG-3′; Sno202 forward primer 5′-CTTTTGAACCCTTTTCCATCTG-3′ mir-383 forward primer 5′-CAGATCAGAAGGTGACTGTG-3′; mir-384-3p forward primer 5′- TGTAAACAATTCCTAGGCAATGA-3′; mir-471-3p forward primer 5′- TGAAAGGTGCCATACTATGTAT-3′, mir-488 forward primer 5′- CCCAGATAATAGCACTCTCAA-3′; for the reverse primers we used the Universal Primer (Quanta Biosciences). We performed quantitative PCR according to the manufacturer's instructions (Quanta Biosciences).
For the detection of miRNAs in POMC neurons, POMC-Tau-Topaz GFP transgenic mice were perfused intracardially with heparin 10% in PBS-Diethylpyrocarbonate (DEPC)-treated (0.1% PBS, 0.1 M, pH 7.4) followed by 4% PFA in PBS-DEPC maintained at 4°C under ketamine/xylazine anesthesia (100 and 15 mg/kg, respectively). The brains were removed and postfixed for 2 h in 4% PFA/DEPC, cryoprotected for 48 h in 30% sucrose in PBS/DEPC at 4°C and frozen in O.C.T. (Tissue-Tek; Sakura Finetek, USA). Subsequently the brains were sliced at 12 μm thickness from −1.34 to −2.70 mm relative to bregma and transferred serially on poly-L-lysine and gelatin-coated Super Frost slides (Fisher, PA, USA). Slides were stored at −20°C until FISH. The miRNA FISH was based on Thompson et al. (2007) protocol. Slides were removed from storage at −20°C and air dried at 37°C for 30 min then placed in 4 % PFA in PBS-DEPC for 20 min and washed in PBS-DEPC 2 times for 10 min. Sections were treated with 10 mg/ml proteinase K for 6 min at room temperature and washed in PBS-DEPC for 10 min. Fixation with 4% PFA in PBS-DEPC was performed for 15 min and rinse in DEPC-treated water. Slides were treated with acetic anhydride (Triethanolamine, 0.1 M, pH 8.0; Acetic anhydride 1:400) 2 times for 5 min and washed with PBS-DEPC for 10 min. Sections were incubated in pre-hybridization buffer (50 % Formamide; 5× SSC; 0.3 mg/ml RNA Yeast; 100 μg/ml heparin; 1× Denhardt's solution (2% bovine serum albumin, 2% polyvinylpyrrolidone; 2% Ficoll 400); 0.1% Tween 20; 0.1% CHAPS; 5 mM EDTA; 0.3 nmole/ml DNA Random Primer 12-mer) for 2.5 h. Hybridization was performed overnight at 37°C in the same buffer with 3′end 6 Fluorescein amidite (6FAM)-labeled DNA oligonucleotide probes at 5 μg/ml. We used the antisense probes 5′-AGCCACAGTCACCTTCTGATCTTT-3′-6FAM for mir-383; 5′-TTACATTGCCTAGGAATTGTTTACATA-3′-6FAM for mir-384-3p; 5′-AAAACTCTCACGATAATAGACCCTT-3′-6FAM for mir-488. Scrambled probes were used for negative control for each experiment. The scramble sequences were 5′-TTCCGACAACTGCACTCTATGTTC-3′6FAM for scramble (sc) mir-383, 5′-CATGAATATTCCGTGGTTAATCATTTA-3′6FAM for scmir-384-3p and 5′-AGATTCTCAACCTGCTTTACAAAGC-3′6FAM for scmir-488. Slides were washed with 2× SSC for 15 min at 37°C. High-stringency tetramethyl ammonium chloride (TMAC, Acros Organics, NJ, USA) washes were performed 2 times for 5 min at 54°C and 1 time for 10 min at 54°C then rinsed in PBS-DEPC with 0.1% Triton X-100 (PBT) for 10 min. Slides were incubated in PBT with 20% horse serum for 1 h for blocking. Rabbit polyclonal antibody anti-fluorescein (1:500, Gen Tex, TX, USA) and mouse monoclonal anti-GFP (1:500, Abcam, MA, USA) antibodies in PBT with 20% horse serum were added to sections and incubated for overnight at 4°C. Slides were washed three times in PBT for 10 min. Alexa 488 fluor-conjugated goat anti-mouse and Alexa 594 fluor-conjugated donkey anti-rabbit (1:500, Life Technologies, France) with PBS 3% horse serum and 0.3% Triton X-100 were added sequentially to slides for 1.5 h at room temperature and sections were washed three times with PBS.
Sections were finally coverslipped with mounting medium for fluorescence microscope preparation. Sections were observed using a Zeiss LSM 700 confocal microscope (Zeiss, France) associated to ZEN 2012 software and a DXM 1200 Camera (Nikon, France) coupled to ACT-1 software. For quantitative analysis, cells were counted manually using the Image J analysis system (National Institutes of Health, USA). For sections stained for both eGFP and miRNA probes, the double labeled cells were examined at multiple focal levels and at appropriate magnifications to ensure that single cells were indeed immunoreactive for both eGFP and miRNA probes. Because POMC neurons constitute a heterogeneous population in relation to their sensitivity to regulatory factors (Mounien et al., 2006a,b; Williams et al., 2010), the average number of cells counted bilaterally in 8 sections at the anterior and posterior levels of the arcuate nucleus in each animal (n = 4) was used for statistical comparisons.
All data are expressed as mean ± SEM. Statistical analysis was performed by an unpaired 2-tailed Mann-Whitney test. P < 0.05 was considered significant.
We used common prediction programs (http://www.targetscan.org and http://www.microrna.org) to search for potential miRNAs target sites on the 3′UTR of Pomc mRNA. In one hand, we identified three conserved miRNAS (mir-383, mir-384-3p, and mir-488) with targetscan.org. In the other hand, the conserved miRNAS with a good mirSVR scores identified with microrna.org are mir-384-3p, mir-371-3p, and mir-488 (Figure 1A). The distributions of these miRNAs of interest in the mouse CNS were investigated by means of quantitative PCR.
Figure 1. Characterization of microRNAs identified as potential regulators of POMC mRNA expression. (A) Predicted conserved binding sites for miRNAs were bioinformatically identified in the sequence of the POMC mRNA 3' UTR. (B) The four miRNAs with the highest conservation degree were quantified by qRT-PCR for their presence in the hypothalamus (Hpt), brainstem (BS), cerebellum (Cb), hippocampus (Hpc), cortex (Cx), and olfactory bulb (OB) of wild-type mice. Relative expression levels of mir-383, mir-384-3p, mir-471-3p, and mir-488 are expressed in fold change of the normalized level obtained in the hypothalamus. Values represent the mean ± SEM (n = 7). *P < 0.05, **P < 0.01 vs. hypothalamus.
The expression profiles of mir-383, mir-384-3p, and mir-488 miRNAs presented many similarities, but also major differences. Thus, in the CNS, the hypothalamus, brainstem, and cortex were the three regions that contained the highest densities of mir-383 miRNAs (Figure 1B). In other CNS structures such as the cerebellum, hippocampus, and olfactory bulb, much lower concentrations of mir-383 miRNA were recorded (Figure 1B). The highest amounts of mir-384-3p miRNAs were found in the brainstem, cerebellum, and olfactory bulb (Figure 1B). Lower levels of mir-384-3p miRNAs were detected in the hypothalamus, hippocampus, and cortex (Figure 1B). The mir-488 miRNAs were widely expressed throughout the CNS with the same intensity (Figure 1B). It should be noted that mir-471-3p miRNA expression was undetectable whatever the brain region studied (Figure 1B). Considering these expression patterns, we focused our work on mir-383, mir-384-3p as mir-488 which are expressed in the hypothalamus.
In POMC-Tau-Topaz GFP mice model, double-staining of brain sections with the fluorescein-labeled mir-383 probe and the antibody against GFP showed that a large proportion of neurons in the arcuate nucleus contained simultaneously GFP and mir-383 miRNAs (Figure 2A). Several GFP neurons did not contain mir-383 miRNAs and, reciprocally, several mir-383 miRNAs-expressing neurons did not contain GFP protein (Figure 2A). Similarly, double staining of brain sections with the GFP antibody and mir-384-3p or mir-488 probes showed that a large proportion of GFP neurons expressed mir-384-3p or mir-488 miRNAs (Figures 2B,C). Quantitative analysis of double-labeled neurons showed that, in the whole arcuate nucleus, 80.7 ± 3.4% of the GFP-positive neurons expressed mir-383 miRNAs, 39.8 ± 2.5% of the GFP-positive cells expressed mir-384-3p miRNAs and 64.9 ± 3.2% of the GFP- immunoreactive cells contained mir-488 miRNAs (Figures 2D–F). The proportion of GFP neurons that exhibited mir-383, mir-384-3p, or mir-488 miRNAs were not different in the anterior and posterior subdivisions of the arcuate nucleus (Figures 2D,F). The control scrambled probes did not produce any labeling (Supplementary Figure 3).
Figure 2. Expression of mir-383, mir-384-3p, and mir-488 in POMC neurons of the arcuate nucleus. (A–C) In situ hybridization and immunofluorescence microscopy detection of miRNAs expression in GFP positive neurons at the arcuate nucleus level of POMC-Tau-Topaz GFP mice model. (D–F) Quantitative analysis of GFP-positive neurons that were labeled with miRNA oligonucleotide probes at anterior (arc. ant.) and posterior (arc. post.) level of the arcuate nucleus. Scale bar = 50 μm. White arrowheads point to POMC neurons expressing miR-383, miR-384-3p or miR-488 miRNA.
To evaluate whether leptin signaling in hypothalamus is essential for the expression of mir-383, mir-384-3p, or mir-488 miRNAs, we analyzed the expression of these miRNAs among groups of leptin-deficient (ob/ob) mice and C57BL/6 controls. The levels of mir-383, mir-384-3p, and mir-488 miRNAs in the hypothalamus of 16-week-old ob/ob and C57BL/6 mice were determined by qRT-PCR. Absence of leptin resulted in a significant increase in mir-383, mir-384-3p, and mir-488 miRNAs (+400%, +101%; P < 0.05 and +605%; P < 0.001, respectively) (Figure 3A).
Figure 3. Expression of miRNAs in the hypothalamus of ob/ob and db/db mice. (A) Hypothalamic mir-383, mir-384-3p, and mir-488 expression in ob/ob and control mice at 16 weeks of age. (B) Hypothalamic miRNAs expression in db/db and control mice at 16 weeks of age. Values represent the mean ± SEM (n = 5 − 10). *P < 0.05, ***P < 0.001.
Because the lack of circulating leptin was associated with a significant increase in the miRNAs of interest, we measured the expression of mir-383, mir-384-3p, and mir-488 miRNAs in the hypothalamus of db/db mice, a model that exhibits a non-functional leptin receptor leading to impaired leptin signaling. As shown in Figure 3B, the mir-383, mir-384-3p, and mir-488 miRNAs levels were over-expressed in 16-week-old db/db mice compared to C57BL/6 animals (+25, +32 and +110%, respectively; P < 0.001).
The above data suggest that leptin may be involved in the regulation of the expression of mir-383, mir-384-3p, and mir-488 miRNAs in the hypothalamus. To directly test this hypothesis, ob/ob mice were i.p. injected with leptin at dose of 5 mg/kg and the hypothalamic expression of mir-383, mir-384-3p, and mir-488 miRNAs was evaluated by qRT-PCR 4 h later. We observed an up-regulation of mir-383, mir-384-3p, and mir-488 miRNAs in 12-week-old ob/ob animals treated with vehicle compared to C57BL/6 control mice (+42, +32%; P < 0.05 and +137%; P < 0.01, respectively) (Figure 4). As expected, leptin treatment significantly reduced hypothalamic expression of mir-383, mir-384-3p, and mir-488 miRNAs in 12-week-old ob/ob animals treated with leptin compared to vehicle-treated ob/ob mice (−25, −21%; P < 0.05 and −58%; P < 0.01, respectively) (Figure 4).
Figure 4. Expression of miRNAs in the hypothalamus of peripheral leptin-treated ob/ob mice and saline-treated ob/ob and wild-type animals. Hypothalamic miRNA expression in peripheral saline-treated animals and leptin-treated ob/ob mice at 12 weeks of age. Values represent the mean ± SEM (n = 5). *P < 0.05, **P < 0.01.
Because leptin exhibits a large range of effect at peripheral level (Margetic et al., 2002), we next investigated the effect of a daily i.c.v leptin administration (5 μg/mice) during 4 days on hypothalamic expression of mir-383, mir-384-3p, and mir-488 miRNAs. The sub-chronic i.c.v leptin administration significantly reduced food consumption and body weight gain in C57BL/6 mice when compared with the control animals (Figures 5A,B). The i.c.v administration of leptin significantly increased POMC mRNA level in the hypothalamus of leptin-treated group compared with the vehicle-treated animals (+62%; P < 0.05) (Figure 5C). Concurrently, the effects of leptin on miRNAs levels were evaluated. Chronic i.c.v administration of leptin had no effect on mir-383 and mir-384-3p miRNAs levels (Figure 5D). In contrast, leptin significantly decreased mir-488 miRNA contents in the hypothalamus after treatment (−60%; P < 0.01) (Figure 5D).
Figure 5. Effect of leptin central administration on miRNA levels in the hypothalamus. (A) Daily food intake in saline-injected and leptin-treated mice at baseline (day 1 before injection) and with daily i.c.v injections of leptin (2.5 μg; days 2–5). (B) Effect of leptin treatment on body weight. (C) Hypothalamic POMC mRNA expression in i.c.v leptin-treated mice and saline-treated animals. (D) Hypothalamic mir-383, mir-384-3p, and mir-488 expression in i.c.v leptin-treated mice and saline-treated animals. Arrows indicate the injections. Values represent the mean ± SEM (n = 7). *P < 0.05, **P < 0.01, ***P < 0.001 vs. saline-treated animals.
The relevance of miRNAs in the function of melanocortin pathways has been recently highlighted by the deletion of Dicer in POMC-expressing cells which led to a postnatal ablation of POMC neurons resulting in obesity (Schneeberger et al., 2012; Greenman et al., 2013). In this models, the absence of Dicer in POMC-positive neurons led to hyperphagia (Schneeberger et al., 2012) and a decrease of energy expenditure without any increase in food intake (Greenman et al., 2013). Taken together, the data suggests that miRNAs are essential for the integrity of POMC neuron activity.
Firstly, we studied the pattern of expression of miRNAs, i.e., mir-383, mir-384-3p, and mir-488, in the CNS. It is already established that these miRNAs are highly expressed in the brain (Chiang et al., 2010). In agreement with this data, we have found by using qRT-PCR that the highest concentrations of mir-383 occurred in the hypothalamus, brainstem, and cortex. The highest amounts of mir-384-3p miRNAs were found in the brainstem, cerebellum, and olfactory bulb while mir-488 miRNAs were widely expressed throughout the structures studied. In many brain regions, mir-383, mir-384-3p, and mir-488 miRNAs distribution patterns did not match each other suggesting that the three miRNAs also exert specific roles. For the first time, we found that the miRNAs encoding the mir-383, mir-384-3p, and mir-488 miRNAs are abundant in the arcuate nucleus. This indicates that a large proportion of the different cell types present in the nucleus are equipped with these miRNAs of interest. The double-staining of POMC-Tau-Topaz GFP mice brain sections with the fluorescein-labeled probes and an antibody against GFP revealed that a high proportion of POMC neurons express mir-383, mir-384-3p, and mir-488, suggesting that these miRNAs target the expression of protein-coding genes that are distributed in this neuronal population. Although mir-383, mir-384-3p, and mir-488 miRNAs were evenly distributed along the rostro-caudal axis of the arcuate nucleus, the proportion of double labeled GFP/mir-383, GFP/mir-384-3p, and GFP/mir-488 neurons did not reflect each other in terms of the proportion of their distribution. These observations are consistent with previous reports demonstrating that POMC neurons constitute an heterogeneous population in relation to their sensitivity to regulatory factors (Mounien et al., 2006a,b; Williams et al., 2010).
In the present context, of special interest is the fact that the POMC neurons are essential for the integration of peripheral signals such as hormones (leptin and insulin) and/or nutrients (glucose) (Morton et al., 2006). For instance, it has been shown recently that leptin directly acts through POMC neurons to stimulate energy expenditure (Berglund et al., 2012). One important goal of current research is to clarify the molecular mechanism involved in the integration of these multiple peripheral metabolic signals such as leptin. One path to reach this goal is to identify the intracellular mediators that allow these POMC neurons to respond to energy status modifications. Interestingly, we observed that the miRNAs targeting POMC mRNAs are up-regulated in ob/ob and db/db mice models. This data provides the first evidence that the expressions of mir-383, mir-384-3p, and mir-488 are associated with an impaired leptin signaling pathway. However, the miRNAs that target POMC 3′UTR may modulate the melanocortin system at other levels notably by regulating the expression and/or activity of the prohormone convertases PC1 and PC2 which are required for the processing of POMC and the formation of α-melanocyte-stimulating hormone (Smith and Funder, 1988). In accordance with this hypothesis, we observed in the databases that mir-488 can also target PC1 3′UTR. In accordance with this hypothesis, PC1 gene expression is different in the arcuate nucleus of leptin-treated obese ob/ob mice (Nilaweera et al., 2003). It is also conceivable that miRNAs may control the biosynthesis of agouti-related protein; the endogenous antagonist of melanocortin receptors MC3R and MC4R (Ollmann et al., 1997).
Several miRNAs have been reported to be differentially expressed in the hypothalamus of ob/ob and db/db mice, but their precise role in the regulation of the hypothalamus function remains to be determined. Recently, it has been shown that mir-200a, mir-200b, and mir-429 are up-regulated in the hypothalamus of ob/ob and db/db mice (Crépin et al., 2014). The same group showed that daily injection of a pegylated leptin antagonist predisposed rats to obesity, promoted leptin resistance and modified the hypothalamic miRNA expression profile (Benoit et al., 2013). In our study, we identified the presence of new miRNAs that are expressed differently in the hypothalamus of ob/ob and db/db animals compared to control mice. Additional studies will be necessary to understand the regulation of these different miRNAs in the hypothalamus during the progressive onset of the obese phenotype and to determine their complex role in the regulation of feeding behavior.
We observed that the fold change modification of the selected miRNAs in the ob/ob mice increased in an age dependent way. These results suggest that age positively regulates miRNAs expression in ob/ob mice. In accordance with this hypothesis, it has been also shown that mir-184 is differentially expressed in the pancreatic islets of ob/ob mice from age 4–16 weeks (Tattikota et al., 2014). The present study shows that leptin peripheral treatment rescues the expression of mir-383, mir-384-3p, and mir-488 in ob/ob mice. Thus, together with the altered expression of these miRNAs in ob/ob and db/db mice, these results support the idea that leptin plays an important role in the expression of the miRNAs that target POMC mRNA in the hypothalamus. However, leptin exhibits a wide range of effects at peripheral level (Margetic et al., 2002) and its effect on miRNAs expression in the hypothalamus could thus be indirect. In order to test the effect at central level, we then performed chronic i.c.v infusion of leptin. Surprisingly, i.c.v administration of leptin in mice only decreased the expression of mir-488 in the hypothalamus suggesting that the expression of mir-488 is under the control of leptin. The absence of effect by central infusion of leptin on the hypothalamic expression of mir-383 and mir-384-3p can be explained by the peripheral effect of leptin. For instance, it has been shown that leptin increases insulin secretion which in turn modulates the POMC neurons activity and may modulate miRNA expression (Ceddia et al., 1999; Hill et al., 2010).
Interestingly, bioinformatics prediction analysis indicates that the miRNAs targeting POMC can also target genes involved in the leptin signaling pathways such as STAT3 as well as the leptin receptor. For instance, mir-488 could modulate the expression of STAT3. These observations suggest that, mir-383, mir-384-3p, and mir-488 in the arcuate nucleus are involved in a complex network controlling the sensitivity of POMC neurons to peripheral signals. Additional in vitro experiments could clarify this last point. Recently, numerous studies identified several miRNAs as important for the hypothalamic regulation of energy homeostasis. Herzer et al. identified mir-7a as a hypothalamic-enriched miRNA with a high expression in Neuropeptide Y/Agouti-related orexigenic peptide neurons (Herzer et al., 2012). Interestingly, two extreme conditions of nutritional stress, i.e., caloric restriction and high fat diet-induced obesity, modified the hypothalamic pattern of expression of a set of miRNAs including let7a, mir-9*, mir-30e, mir-132, mir-145, mir-200a, and mir-218 (Sangiao-Alvarellos et al., 2014). Taken altogether, these different studies point to the importance of miRNAs as regulators and sensors of central energy homeostasis.
In conclusion, we observed that the impairment of leptin synthesis or signaling, induced a defect in the hypothalamic expression of a subset of miRNAs that target POMC 3′UTR. It was observed that mir-383, mir-384-3p, and mir-488 were up-regulated in ob/ob and db/db mice and mir-488 was down-regulated in leptin-treated mice. This indicates that the miRNAs that target POMC important mediators of leptin action in the hypothalamus. New miRNAs modulated by leptin could open important therapeutic perspectives for controlling metabolic disorders.
LM planned the project. AD, MD, CA, CP, MD, VT, and CT performed experiments. LM wrote the manuscript. JT, BB, and JT helped with data evaluation, interpretation, and manuscript preparation.
The authors declare that the research was conducted in the absence of any commercial or financial relationships that could be construed as a potential conflict of interest.
This research was supported by funding obtained from Aix-Marseille University, the “Région Provence-Alpes-Côte d'Azur,” the “Conseil Général des Bouches-du-Rhône” (PACA, CG13) and Benjamin Delessert foundation. We also thank the Aix-Marseille University Microscopy Center CP2M for the access to confocal Microscopy and the AVB platform (Analyse et Valorisation de la Biodiversité, iSm2, UMR 7313, Marseille). AD is the recipient of a doctoral fellowship from the Ministry of Education. The authors are grateful to O. Knowles and S. Mounien for critical reading of the manuscript. We thank D. Chabbert for expert help with intracerebroventricular injection and Dr. M. Maresca for the gift of antibodies.
The Supplementary Material for this article can be found online at: http://journal.frontiersin.org/article/10.3389/fncel.2015.00172/abstract
Bagga, S., Bracht, J., Hunter, S., Massirer, K., Holtz, J., Eachus, R., et al. (2005). Regulation by let-7 and lin-4 miRNAs results in target mRNA degradation. Cell 122, 553–563. doi: 10.1016/j.cell.2005.07.031
PubMed Abstract | Full Text | CrossRef Full Text | Google Scholar
Benoit, C., Ould-Hamouda, H., Crepin, D., Gertler, A., Amar, L., and Taouis, M. (2013). Early leptin blockade predisposes fat-fed rats to overweight and modifies hypothalamic microRNAs. J. Endocrinol. 218, 35–47. doi: 10.1530/JOE-12-0561
PubMed Abstract | Full Text | CrossRef Full Text | Google Scholar
Berglund, E. D., Vianna, C. R., Donato, J. Jr., Kim, M. H., Chuang, J.-C., Lee, C. E., et al. (2012). Direct leptin action on POMC neurons regulates glucose homeostasis and hepatic insulin sensitivity in mice. J. Clin. Invest. 122, 1000–1009. doi: 10.1172/JCI59816
PubMed Abstract | Full Text | CrossRef Full Text | Google Scholar
Ceddia, R. B., William, W. N. Jr., Carpinelli, A. R., and Curi, R. (1999). Modulation of insulin secretion by leptin. Gen. Pharmacol. 32, 233–237. doi: 10.1016/S0306-3623(98)00185-2
PubMed Abstract | Full Text | CrossRef Full Text | Google Scholar
Chen, A. S., Marsh, D. J., Trumbauer, M. E., Frazier, E. G., Guan, X. M., Yu, H., et al. (2000). Inactivation of the mouse melanocortin-3 receptor results in increased fat mass and reduced lean body mass. Nat. Genet. 26, 97–102. doi: 10.1038/79254
PubMed Abstract | Full Text | CrossRef Full Text | Google Scholar
Chiang, H. R., Schoenfeld, L. W., Ruby, J. G., Auyeung, V. C., Spies, N., Baek, D., et al. (2010). Mammalian microRNAs: experimental evaluation of novel and previously annotated genes. Genes Dev. 24, 992–1009. doi: 10.1101/gad.1884710
PubMed Abstract | Full Text | CrossRef Full Text | Google Scholar
Cowley, M. A., Smart, J. L., Rubinstein, M., Cerdán, M. G., Diano, S., Horvath, T. L., et al. (2001). Leptin activates anorexigenic POMC neurons through a neural network in the arcuate nucleus. Nature 411, 480–484. doi: 10.1038/35078085
PubMed Abstract | Full Text | CrossRef Full Text | Google Scholar
Crépin, D., Benomar, Y., Riffault, L., Amine, H., Gertler, A., and Taouis, M. (2014). The over-expression of miR-200a in the hypothalamus of ob/ob mice is linked to leptin and insulin signaling impairment. Mol. Cell. Endocrinol. 384, 1–11. doi: 10.1016/j.mce.2013.12.016
PubMed Abstract | Full Text | CrossRef Full Text | Google Scholar
Gao, Q., Wolfgang, M. J., Neschen, S., Morino, K., Horvath, T. L., Shulman, G. I., et al. (2004). Disruption of neural signal transducer and activator of transcription 3 causes obesity, diabetes, infertility, and thermal dysregulation. Proc. Natl. Acad. Sci. U.S.A. 101, 4661–4666. doi: 10.1073/pnas.0303992101
PubMed Abstract | Full Text | CrossRef Full Text | Google Scholar
Girardet, C., Bonnet, M. S., Jdir, R., Sadoud, M., Thirion, S., Tardivel, C., et al. (2011). Central inflammation and sickness-like behavior induced by the food contaminant deoxynivalenol: a PGE2-independent mechanism. Toxicol. Sci. 124, 179–191. doi: 10.1093/toxsci/kfr219
PubMed Abstract | Full Text | CrossRef Full Text | Google Scholar
Greenman, Y., Kuperman, Y., Drori, Y., Asa, S. L., Navon, I., Forkosh, O., et al. (2013). Postnatal ablation of POMC neurons induces an obese phenotype characterized by decreased food intake and enhanced anxiety-like behavior. Mol. Endocrinol. (Baltimore Md) 27, 1091–1102. doi: 10.1210/me.2012-1344
PubMed Abstract | Full Text | CrossRef Full Text | Google Scholar
Herzer, S., Silahtaroglu, A., and Meister, B. (2012). Locked nucleic acid-based in situ hybridisation reveals miR-7a as a hypothalamus-enriched microRNA with a distinct expression pattern. J. Neuroendocrinol. 24, 1492–1504. doi: 10.1111/j.1365-2826.2012.02358.x
PubMed Abstract | Full Text | CrossRef Full Text | Google Scholar
Hill, J. W., Elias, C. F., Fukuda, M., Williams, K. W., Berglund, E. D., Holland, W. L., et al. (2010). Direct insulin and leptin action on pro-opiomelanocortin neurons is required for normal glucose homeostasis and fertility. Cell Metab. 11, 286–297. doi: 10.1016/j.cmet.2010.03.002
PubMed Abstract | Full Text | CrossRef Full Text | Google Scholar
Huszar, D., Lynch, C. A., Fairchild-Huntress, V., Dunmore, J. H., Fang, Q., Berkemeier, L. R., et al. (1997). Targeted disruption of the melanocortin-4 receptor results in obesity in mice. Cell 88, 131–141. doi: 10.1016/S0092-8674(00)81865-6
PubMed Abstract | Full Text | CrossRef Full Text | Google Scholar
Ibrahim, N., Bosch, M. A., Smart, J. L., Qiu, J., Rubinstein, M., Rønnekleiv, O. K., et al. (2003). Hypothalamic proopiomelanocortin neurons are glucose responsive and express K(ATP) channels. Endocrinology 144, 1331–1340. doi: 10.1210/en.2002-221033
PubMed Abstract | Full Text | CrossRef Full Text | Google Scholar
Lee, Y. S. (2009). The role of leptin-melanocortin system and human weight regulation: lessons from experiments of nature. Ann. Acad. Med. Singapore 38, 34–11.
Li, S., Chen, X., Zhang, H., Liang, X., Xiang, Y., Yu, C., et al. (2009). Differential expression of microRNAs in mouse liver under aberrant energy metabolic status. J. Lipid Res. 50, 1756–1765. doi: 10.1194/jlr.M800509-JLR200
PubMed Abstract | Full Text | CrossRef Full Text | Google Scholar
Lovis, P., Roggli, E., Laybutt, D. R., Gattesco, S., Yang, J.-Y., Widmann, C., et al. (2008). Alterations in microRNA expression contribute to fatty acid-induced pancreatic beta-cell dysfunction. Diabetes 57, 2728–2736. doi: 10.2337/db07-1252
PubMed Abstract | Full Text | CrossRef Full Text | Google Scholar
Margetic, S., Gazzola, C., Pegg, G. G., and Hill, R. A. (2002). Leptin: a review of its peripheral actions and interactions. Int. J. Obes. Relat. Metab. Disord. 26, 1407–1433. doi: 10.1038/sj.ijo.0802142
PubMed Abstract | Full Text | CrossRef Full Text | Google Scholar
Mizuno, T. M., Kleopoulos, S. P., Bergen, H. T., Roberts, J. L., Priest, C. A., and Mobbs, C. V. (1998). Hypothalamic Pro-opiomelanocortin mRNA is reduced by fasting in ob/ob and db/db mice, but is stimulated by Leptin. Diabetes 47, 294–297. doi: 10.2337/diab.47.2.294
PubMed Abstract | Full Text | CrossRef Full Text | Google Scholar
Morton, G. J., Cummings, D. E., Baskin, D. G., Barsh, G. S., and Schwartz, M. W. (2006). Central nervous system control of food intake and body weight. Nature 443, 289–295. doi: 10.1038/nature05026
PubMed Abstract | Full Text | CrossRef Full Text | Google Scholar
Mounien, L., Bizet, P., Boutelet, I., Gourcerol, G., Basille, M., Gonzalez, B., et al. (2006a). Expression of PACAP receptor mRNAs by neuropeptide Y neurons in the rat arcuate nucleus. Ann. N.Y. Acad. Sci. 1070, 457–461. doi: 10.1196/annals.1317.061
PubMed Abstract | Full Text | CrossRef Full Text | Google Scholar
Mounien, L., Bizet, P., Boutelet, I., Gourcerol, G., Fournier, A., Vaudry, H., et al. (2006b). Pituitary adenylate cyclase-activating polypeptide directly modulates the activity of proopiomelanocortin neurons in the rat arcuate nucleus. Neuroscience 143, 155–163. doi: 10.1016/j.neuroscience.2006.07.022
PubMed Abstract | Full Text | CrossRef Full Text | Google Scholar
Mounien, L., Bizet, P., Boutelet, I., Vaudry, H., and Jégou, S. (2005). Expression of melanocortin MC3 and MC4 receptor mRNAs by neuropeptide Y neurons in the rat arcuate nucleus. Neuroendocrinology 82, 164–170. doi: 10.1159/000091737
PubMed Abstract | Full Text | CrossRef Full Text | Google Scholar
Mounien, L., Do Rego, J.-C., Bizet, P., Boutelet, I., Gourcerol, G., Fournier, A., et al. (2009). Pituitary adenylate cyclase-activating polypeptide inhibits food intake in mice through activation of the hypothalamic melanocortin system. Neuropsychopharmacology 34, 424–435. doi: 10.1038/npp.2008.73
PubMed Abstract | Full Text | CrossRef Full Text | Google Scholar
Mounien, L., Marty, N., Tarussio, D., Metref, S., Genoux, D., Preitner, F., et al. (2010). Glut2-dependent glucose-sensing controls thermoregulation by enhancing the leptin sensitivity of NPY and POMC neurons. FASEB J. 24, 1747–1758. doi: 10.1096/fj.09-144923
PubMed Abstract | Full Text | CrossRef Full Text | Google Scholar
Nakanishi, N., Nakagawa, Y., Tokushige, N., Aoki, N., Matsuzaka, T., Ishii, K., et al. (2009). The up-regulation of microRNA-335 is associated with lipid metabolism in liver and white adipose tissue of genetically obese mice. Biochem. Biophys. Res. Commun. 385, 492–496. doi: 10.1016/j.bbrc.2009.05.058
PubMed Abstract | Full Text | CrossRef Full Text | Google Scholar
Nilaweera, K. N., Barrett, P., Mercer, J. G., and Morgan, P. J. (2003). Precursor-protein convertase 1 gene expression in the mouse hypothalamus: differential regulation by ob gene mutation, energy deficit and administration of leptin, and coexpression with prepro-orexin. Neuroscience 119, 713–720. doi: 10.1016/S0306-4522(02)00869-2
PubMed Abstract | Full Text | CrossRef Full Text | Google Scholar
Ollmann, M. M., Wilson, B. D., Yang, Y. K., Kerns, J. A., Chen, Y., Gantz, I., et al. (1997). Antagonism of central melanocortin receptors in vitro and in vivo by agouti-related protein. Science 278, 135–138. doi: 10.1126/science.278.5335.135
PubMed Abstract | Full Text | CrossRef Full Text | Google Scholar
Parton, L. E., Ye, C. P., Coppari, R., Enriori, P. J., Choi, B., Zhang, C.-Y., et al. (2007). Glucose sensing by POMC neurons regulates glucose homeostasis and is impaired in obesity. Nature 449, 228–232. doi: 10.1038/nature06098
PubMed Abstract | Full Text | CrossRef Full Text | Google Scholar
Pinto, S., Roseberry, A. G., Liu, H., Diano, S., Shanabrough, M., Cai, X., et al. (2004). Rapid rewiring of arcuate nucleus feeding circuits by Leptin. Science 304, 110–115. doi: 10.1126/science.1089459
PubMed Abstract | Full Text | CrossRef Full Text | Google Scholar
Plum, L., Belgardt, B. F., and Brüning, J. C. (2006). Central insulin action in energy and glucose homeostasis. J. Clin. Invest. 116, 1761–1766. doi: 10.1172/JCI29063
PubMed Abstract | Full Text | CrossRef Full Text | Google Scholar
Porte, D. Jr., Baskin, D. G., and Schwartz, M. W. (2002). Leptin and insulin action in the central nervous system. Nutr. Rev. 60, S20–S29. discussion: S68–S84, 85–87. doi: 10.1301/002966402320634797
PubMed Abstract | Full Text | CrossRef Full Text | Google Scholar
Poy, M. N., Hausser, J., Trajkovski, M., Braun, M., Collins, S., Rorsman, P., et al. (2009). miR-375 maintains normal pancreatic alpha- and beta-cell mass. Proc. Natl. Acad. Sci. U.S.A. 106, 5813–5818. doi: 10.1073/pnas.0810550106
PubMed Abstract | Full Text | CrossRef Full Text | Google Scholar
Sangiao-Alvarellos, S., Pena-Bello, L., Manfredi-Lozano, M., Tena-Sempere, M., and Cordido, F. (2014). Perturbation of hypothalamic microRNA expression patterns in male rats after metabolic distress: impact of obesity and conditions of negative energy balance. Endocrinology 155, 1838–1850. doi: 10.1210/en.2013-1770
PubMed Abstract | Full Text | CrossRef Full Text | Google Scholar
Schneeberger, M., Altirriba, J., García, A., Esteban, Y., Castaño, C., García-Lavandeira, M., et al. (2012). Deletion of miRNA processing enzyme Dicer in POMC-expressing cells leads to pituitary dysfunction, neurodegeneration and development of obesity. Mol. Metab. 2, 74–85. doi: 10.1016/j.molmet.2012.10.001
PubMed Abstract | Full Text | CrossRef Full Text | Google Scholar
Smith, A. I., and Funder, J. W. (1988). Proopiomelanocortin processing in the pituitary, central nervous system, and peripheral tissues. Endocr. Rev. 9, 159–179. doi: 10.1210/edrv-9-1-159
PubMed Abstract | Full Text | CrossRef Full Text | Google Scholar
Tattikota, S. G., Rathjen, T., McAnulty, S. J., Wessels, H.-H., Akerman, I., van de Bunt, M., et al. (2014). Argonaute2 mediates compensatory expansion of the pancreatic β cell. Cell Metab. 19, 122–134. doi: 10.1016/j.cmet.2013.11.015
PubMed Abstract | Full Text | CrossRef Full Text | Google Scholar
Thompson, R. C., Deo, M., and Turner, D. L. (2007). Analysis of microRNA expression by in situ hybridization with RNA oligonucleotide probes. Methods (San Diego Calif.) 43, 153–161. doi: 10.1016/j.ymeth.2007.04.008
PubMed Abstract | Full Text | CrossRef Full Text | Google Scholar
Williams, K. W., Margatho, L. O., Lee, C. E., Choi, M., Lee, S., Scott, M. M., et al. (2010). Segregation of acute leptin and insulin effects in distinct populations of arcuate proopiomelanocortin neurons. J. Neurosci. 30, 2472–2479. doi: 10.1523/JNEUROSCI.3118-09.2010
PubMed Abstract | Full Text | CrossRef Full Text | Google Scholar
Xie, H., Lim, B., and Lodish, H. F. (2009). MicroRNAs induced during adipogenesis that accelerate fat cell development are downregulated in obesity. Diabetes 58, 1050–1057. doi: 10.2337/db08-1299
PubMed Abstract | Full Text | CrossRef Full Text | Google Scholar
Yaswen, L., Diehl, N., Brennan, M. B., and Hochgeschwender, U. (1999). Obesity in the mouse model of pro-opiomelanocortin deficiency responds to peripheral melanocortin. Nat. Med. 5, 1066–1070. doi: 10.1038/12506
PubMed Abstract | Full Text | CrossRef Full Text | Google Scholar
Keywords: microRNA, melanocortin, hypothalamus, leptin, food intake
Citation: Derghal A, Djelloul M, Airault C, Pierre C, Dallaporta M, Troadec J-D, Tillement V, Tardivel C, Bariohay B, Trouslard J and Mounien L (2015) Leptin is required for hypothalamic regulation of miRNAs targeting POMC 3′UTR. Front. Cell. Neurosci. 9:172. doi: 10.3389/fncel.2015.00172
Received: 14 November 2014; Accepted: 18 April 2015;
Published: 06 May 2015.
Edited by:
Marie Z. Moftah, Alexandria University, EgyptReviewed by:
Alexander K. Murashov, East Carolina University, USACopyright © 2015 Derghal, Djelloul, Airault, Pierre, Dallaporta, Troadec, Tillement, Tardivel, Bariohay, Trouslard and Mounien. This is an open-access article distributed under the terms of the Creative Commons Attribution License (CC BY). The use, distribution or reproduction in other forums is permitted, provided the original author(s) or licensor are credited and that the original publication in this journal is cited, in accordance with accepted academic practice. No use, distribution or reproduction is permitted which does not comply with these terms.
*Correspondence: Lourdes Mounien, Unité de Recherche Physiologie et Physio-pathologie du Système Nerveux Somato-moteur et Neurovégétatif, Faculté des Sciences, Université d'Aix-Marseille, Campus St. Jérôme, BP 351-352, 13397 Marseille, France,bG91cmRlcy5tb3VuaWVuQHVuaXYtYW11LmZy
Disclaimer: All claims expressed in this article are solely those of the authors and do not necessarily represent those of their affiliated organizations, or those of the publisher, the editors and the reviewers. Any product that may be evaluated in this article or claim that may be made by its manufacturer is not guaranteed or endorsed by the publisher.
Research integrity at Frontiers
Learn more about the work of our research integrity team to safeguard the quality of each article we publish.