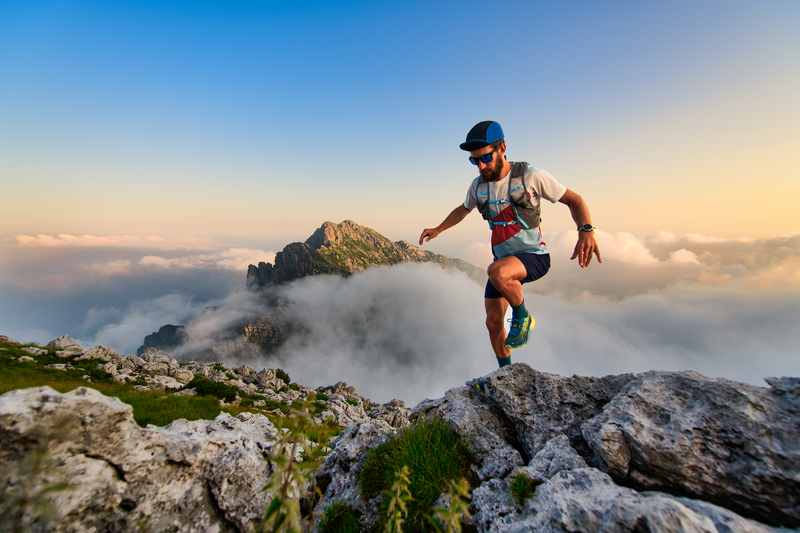
95% of researchers rate our articles as excellent or good
Learn more about the work of our research integrity team to safeguard the quality of each article we publish.
Find out more
REVIEW article
Front. Cell. Neurosci. , 26 January 2015
Sec. Cellular Neurophysiology
Volume 8 - 2014 | https://doi.org/10.3389/fncel.2014.00455
This article is part of the Research Topic Determinants of Synaptic Information Transfer: from Ca2+ Binding Proteins to Ca2+ Signaling Domains View all 13 articles
Here we summarize the evidence from two “giant” presynaptic terminals—the squid giant synapse and the mammalian calyx of Held—supporting the involvement of nanodomain calcium signals in triggering of neurotransmitter release. At the squid synapse, there are three main lines of experimental evidence for nanodomain signaling. First, changing the size of the unitary calcium channel current by altering external calcium concentration causes a non-linear change in transmitter release, while changing the number of open channels by broadening the presynaptic action potential causes a linear change in release. Second, low-affinity calcium indicators, calcium chelators, and uncaging of calcium all suggest that presynaptic calcium concentrations are as high as hundreds of micromolar, which is more compatible with a nanodomain type of calcium signal. Finally, neurotransmitter release is much less affected by the slow calcium chelator, ethylene glycol tetraacetic acid (EGTA), in comparison to the rapid chelator 1,2-bis(o-aminophenoxy)ethane-N,N,N’,N’-tetraacetic acid (BAPTA). Similarly, as the calyx of Held synapse matures, EGTA becomes less effective in attenuating transmitter release while the number of calcium channels required to trigger a single fusion event declines. This suggests a developmental transformation of microdomain to nanodomain coupling between calcium channels and transmitter release. Calcium imaging and uncaging experiments, in combination with simulations of calcium diffusion, indicate the peak calcium concentration seen by presynaptic calcium sensors reaches at least tens of micromolar at the calyx of Held. Taken together, data from these provide a compelling argument that nanodomain calcium signaling gates very rapid transmitter release.
The concept of local “domains” of intracellular calcium concentration developed from mathematical models of the diffusion of Ca ions (Ca2+) away from the mouth of open calcium channels. The first published model, by Chad and Eckert (1984), laid out several key features of this concept. This paper proposed that opening of individual Ca2+ channels could form discrete spatial domains where Ca2+ concentration is very high locally—within nanometers of a single open Ca2+ channel—but drops off steeply with distance away from the channel. In addition, increasingly large depolarizations of the membrane potential should have dual effects: increasing the number of such domains, by increasing the number of open Ca2+ channels, yet reducing the magnitude of the local Ca2+ concentration change within a domain because of the reduced unitary current through an open channel.
Simon and Llinás (1985) independently developed the Ca2+ domain concept and considered the possible role of such domains in triggering of neurotransmitter release from presynaptic terminals. In addition to confirming the deductions of Chad and Eckert (1984) regarding the basic properties of Ca2+ domains, they further concluded that these domains usually do not overlap appreciably for the case of squid presynaptic terminals. When many Ca2+ channels are opened by large, prolonged depolarizations, there can be a small degree of spatial overlap due to summation of Ca2+ from adjacent open channels. The modeling studies of Zucker and Fogelson (1986) reached a similar conclusion. Notably, Simon and Llinás (1985) concluded that an endogenous Ca2+ buffer could eliminate summation of Ca2+ signals associated with neighboring domains, but should have minimal effect on the peak Ca2+ concentration change occurring in the immediate vicinity of an open channel.
At its simplest, diffusion of Ca2+ from an open channel can be described by the following equation (Smith and Augustine, 1988):
where x is distance from the channel, t is time, JCa is the flux of Ca2+ through a single open channel, D is the diffusion coefficient for Ca2+ and erfc is the complementary error function. This equation predicts that (1) while the channel is open, there will be an inverse relationship between Ca2+ concentration and distance from the channel; and (2) Ca2+ diffusion time will increase with the square of distance. To aid comprehension of these relationships, we provide “rule of thumb” Ca2+ diffusion parameters in Table 1. These parameters are to be taken as order-of-magnitude approximations because their precise values will depend on the value chosen for D, as well as the unitary current flowing through the open Ca2+ channel and the properties of cellular Ca2+ buffering. More complete mathematical treatments can be found elsewhere, for example in Smith and Augustine (1988), Naraghi and Neher (1997) and Neher (1998), as well as in the pioneering domain papers described above.
As mentioned above, it is possible that Ca2+ signals from adjacent open Ca2+ channels could overlap under some conditions. The degree of overlap leads to several qualitatively different forms of local Ca2+ signaling that are depicted in Figure 1. If there is no spatial overlap between the local Ca2+ domains, relative to the position of the relevant Ca2+ sensor, then a nanodomain occurs (Figure 1A). This type of signal is called a nanodomain because Ca2+ signaling will occur over dimensions of nanometers (Kasai, 1993; Schweizer et al., 1995; Augustine et al., 2003). If there is some degree of spatial overlap between neighboring domains and the Ca2+ sensor receives contributions from domains summing over a distance of approximately a micrometer, then this is microdomain-style Ca2+ signaling (Figure 1B). Finally, if the sensor receives Ca2+ ions diffusing over distances greater than a micrometer, due to summation of microdomains, then a radial gradient style of Ca2+ signaling is said to occur (Figure 1C). The differences in the spatial range of these different modes of Ca2+ signaling necessarily are associated with differences in the speed of Ca2+ triggering of biological processes, as indicated by the t-term in equation (1) and in the right column of Table 1.
Figure 1. Three types of local Ca2+ signaling. (A) Nanodomains arise from Ca2+ (blue) diffusing away from a single open Ca2+ channel (red) in the presynaptic plasma membrane (orange). The green spherical structure is a synaptic vesicle and a synaptotagmin-like Ca2+ sensor is shown protruding from the surface of the vesicle. (B) Microdomains arise from spatial overlap of local Ca2+ signals contributed by multiple open Ca2+ channels within a micrometer of each other. (C) Terminal-wide radial gradients of Ca2+ arise from long-distance diffusion of Ca2+ from channels at the plasma membrane. Derived from illustrations in Schweizer et al. (1995) and Augustine et al. (2003).
From a physical perspective, there is no question that local domains of Ca2+ signaling occur in the immediate vicinity of an open Ca2+ channel. The question addressed in this review is how relevant such domain signaling is for release of neurotransmitters from presynaptic terminals. We will compare Ca2+ signaling at two presynaptic terminals whose large size makes them particularly amenable to experimental analysis: the squid giant synapse and the mammalian calyx of Held. From results obtained at these synapses, as well as selected results from other synapses, we will develop the argument that nanodomain Ca2+ signaling is prevalent at presynaptic terminals that rapidly release neurotransmitters, though microdomain signaling also has been established for some types of presynaptic terminals. Radial gradients are likely to be important only for presynaptic terminals that slowly release neuropeptides or for slower modulatory presynaptic actions of Ca2+ within fast-acting synapses, for example Ca2+-dependent forms of synaptic plasticity.
Virtually all of the early experimental tests of the role of Ca2+ domains in triggering neurotransmitter release were performed at the squid giant synapse. As a result, work at this synapse established most of the experimental paradigms that have been used to probe local Ca2+ signaling in presynaptic terminals. In this section we will describe some of these key experiments.
The first clues regarding the possible role of presynaptic domain signaling came from analysis of the kinetics of transmitter release. The minimum synaptic delay between a presynaptic Ca2+ “tail” current (following a depolarization to a very positive membrane potential) and the resultant postsynaptic response is very brief, on the order of hundreds of microseconds (Llinás et al., 1981; Augustine et al., 1985). Given the “rule of thumb” relationships for local Ca2+ signaling described above (Table 1), this indicates that Ca2+ must diffuse on the order of 100 nm or less before binding to the Ca2+ sensor for neurotransmitter release. This is consistent with a nanodomain type of presynaptic Ca2+ signal. Further, Simon and Llinás (1985) indicated that the relationship between presynaptic Ca2+ current and postsynaptic response could be explained by a model where domains do not overlap appreciably, i.e., nanodomain signaling.
While these initial observations provided indirect support for the role of nanodomain Ca2+ signals in triggering transmitter release at the squid giant synapse, they were open to alternative interpretations. However, several additional experiments have greatly bolstered acceptance of this notion. This experimental evidence can be grouped into three categories.
Since the classic work of Dodge and Rahamimoff (1967), it has been known that Ca2+ acts “cooperatively”, meaning that there is a high-order relationship between extracellular Ca2+ concentration and the amount of neurotransmitter release evoked by presynaptic action potentials. Cooperative triggering of transmitter release can be used as a tool to probe the spatial organization of presynaptic Ca2+ signaling.
Voltage clamp experiments at the squid giant synapse allowed the first direct measurement of Ca2+ entry into a presynaptic terminal and established that this cooperativity arises from a non-linear relationship between Ca2+ entry and the resultant release of neurotransmitters. Initially Ca2+ entry was varied by depolarizing the presynaptic membrane potential to different levels, thereby varying the number of open Ca2+ channels. While an initial account proposed a linear relationship between Ca2+ entry and transmitter release (Llinás et al., 1981), a follow-up study that optimized spatial control of presynaptic membrane potential indicated a third- or fourth-power relationship (Augustine et al., 1985). Because depolarizing to different membrane potentials changes the number of Ca2+ domains, as well as the Ca2+ concentration within each domain, these results are not easily interpreted in terms of local Ca2+ signals. A follow-up study by Augustine and Charlton (1986) circumvented this problem by depolarizing to a constant presynaptic membrane potential, to open a constant number of Ca2+ channels, while varying Ca2+ entry by changing external Ca2+ concentration. Under such conditions, an approximate fourth-power relationship between Ca2+ entry and transmitter release was observed (Figure 2A). Given that the number of Ca2+ domains will be constant under these conditions (Figure 2A insets), this result reveals that cooperativity occurs within the domains.
Figure 2. Experimental strategies for probing local Ca2+ signaling during neurotransmitter release. (A) At a voltage-clamped squid giant synapse, varying external Ca2+ concentration changes presynaptic Ca2+ influx by keeping number of Ca2+ domains constant, while changing the amount of Ca2+ within each domain (insets). Under these conditions, the relationship between presynaptic Ca2+ current and amount of transmitter release, assessed by amplitude of the postsynaptic current, is a highly non-linear power function. Both presynaptic and postsynaptic responses are normalized to the maximum values measured at the highest external Ca2+ concentration. Data replotted from Augustine and Charlton (1986). (B) Pharmacologically broadening the presynaptic action potential at the squid giant synapse varies Ca2+ influx by opening additional Ca2+ channels, thereby increasing the number of Ca2+ domains (insets). Under these conditions, the relationship between presynaptic Ca2+ current and amount of transmitter release is a linear function. Both presynaptic and postsynaptic responses are normalized to the values measured under control conditions (narrowest presynaptic action potential). Data replotted from Augustine et al. (1991).
A strikingly different result is obtained when Ca2+ entry is varied by altering the duration of the presynaptic action potential. Blockade of potassium channels prolongs the duration of the presynaptic action potential and thereby increases Ca2+ entry into the terminal by increasing the number of open Ca2+ channels (Klein and Kandel, 1980). Under such conditions, a linear relationship between action potential duration and postsynaptic response was observed (Augustine, 1990). Although Ca influx was not measured directly, the known gating properties of the squid presynaptic Ca2+ channels allowed calculation of the presynaptic Ca2+ current and indicated a linear relationship between action potential duration and Ca2+ current magnitude (Augustine, 1990). Thus, there is a linear relationship between Ca2+ current and transmitter release when Ca2+ influx is varied by changing the number of open Ca2+ channels (Figure 2B; Augustine et al., 1991).
Given that cooperativity occurs within a domain (Figure 2A), the fact that increasing the number of domains causes a linear increase in transmitter release (Figure 2B) leads to the conclusion that domains do not overlap during a presynaptic action potential. The logic behind this deduction is shown in Figure 3. In brief, if domains do not overlap then transmitter release will increase linearly according to the number of Ca2+ channels opened (Figures 3A,B). If domains overlap, then release will increase supralinearly due to summation of Ca2+ from neighboring domains, as well as the cooperative action of Ca2+ within a domain (Figure 3C). The results of Figure 2B are consistent with the expectations if domains do not overlap (Figure 3B, right). Thus, domains do not overlap during a presynaptic action potential at the squid giant synapse. Similar lines of experimentation have been used to probe the spatial dimensions of Ca2+ signaling in other presynaptic terminals (e.g., Wu and Saggau, 1994; Mintz et al., 1995).
Figure 3. Evaluating local Ca2+ signaling during broadening of presynaptic action potentials. (A) Diagram of spatial distribution of Ca2+ associated with a single open Ca2+ channel. (B) If open Ca2+ channels are sufficiently far apart, then Ca2+ signals associated with each channel will not overlap, creating a nanodomain arrangement (left). In this case, broader presynaptic action potentials will increase the number of discrete domains, leading to a proportional increase in transmitter release and a linear relationship between Ca2+ influx and release (right). (C) If open Ca2+ channels are close together, then Ca2+ signals associated with each channel will overlap, causing an increase in Ca2+ concentration (red bracket) within the local Ca2+ signal associated with each channel (left). This creates a microdomain Ca2+ signal. In this case, broader presynaptic action potentials will increase the Ca2+ concentration within each domain, leading to a supralinear increase in transmitter release due to the cooperative triggering of transmitter release by Ca2+. This spatial summation will yield a supralinear relationship between Ca2+ influx and release (right) when presynaptic action potentials are broadened. After Augustine et al. (1991).
If the Ca2+ sensor for neurotransmitter release is located within nanometers of an open Ca2+ channel, as postulated by a nanodomain coupling scheme, then the local Ca2+ concentration experienced by this sensor should be in the range of tens to hundreds of micromolar (Table 1). Two types of experimental paradigms have tested this premise at the squid giant synapse.
The first experiment compared the ability of Ca2+ chelators of differing affinities to block transmitter release. For this purpose, analogs of the Ca2+ chelator 1,2-bis(o-aminophenoxy)ethane-N,N,N’,N’-tetraacetic acid (BAPTA) were microinjected into the giant presynaptic terminal. A complex relationship between Ca2+ affinity and chelator efficacy was observed: the most potent inhibitor of transmitter release was dibromoBAPTA, a chelator with intermediate Ca2+ affinity (4.9 µM), while chelators with lower or higher affinities were less potent (Adler et al., 1991). A numerical model could predict such results only by assuming that the presynaptic Ca2+ sensor for transmitter release encounters Ca2+ levels on the order of 100 µM or higher (Augustine et al., 1991). This prediction is consistent with the results of experiments employing photolysis of a caged Ca2+ chelator; even raising presynaptic Ca2+ concentration to 50 µM produced rates of transmitter release lower than those evoked by a presynaptic action potential (Hsu et al., 1996). Together, these results are compatible with the predictions of a nanodomain coupling model.
The second experiment imaged presynaptic Ca2+ concentration during transmitter release. For this purpose, Llinás et al. (1992) used a version of the bioluminescent Ca2+-binding protein, aequorin, that was engineered to have an especially low affinity for Ca2+. This indicator protein, called n-Aequorin-J, only emits light at Ca2+ concentrations in the micromolar range or higher, with the amount of light emission increasing steeply as a function of Ca2+ concentration. Based on the amount of bioluminescence emitted by n-Aequorin-J injected into the squid giant presynaptic terminal, Llinás et al. (1992) concluded that presynaptic Ca2+ concentration reached 200–300 µM during transmitter release. Further, their measurements indicated that the regions of high Ca2+ concentration were quite localized, being a fraction of a square micrometer in area. While there are caveats regarding calibration of this indicator, as well as the spatial resolution of the Ca2+ imaging method, the results are compatible with the nanodomain coupling model.
In sum, estimates provided by two complementary experimental approaches indicate that Ca2+ concentration rises on the order of hundreds of micromolar in the squid giant presynaptic terminal during transmitter release. Such results favor nanodomain coupling of Ca2+ channels to transmitter release at this synapse.
The final experimental paradigm—and the one that has proven most useful for probing local Ca2+ signaling in presynaptic terminals—is based on comparison of Ca2+ chelators with different rates of Ca2+ binding (Adler et al., 1991). BAPTA binds Ca2+ very rapidly, with its on rate being diffusion-limited, while ethylene glycol tetraacetic acid (EGTA) binds Ca2+ more slowly because it is protonated at physiological pH and the bound proton must dissociate before Ca2+ can bind. This greatly slows the binding of Ca2+ to EGTA in comparison to BAPTA, even though the equilibrium affinity of these two chelators is roughly similar (Augustine et al., 1991). The slow binding of Ca2+ allows EGTA to serve as a high-pass temporal (and spatial) filter for Ca2+.
Neurotransmitter release evoked by presynaptic action potentials is completely inhibited when BAPTA is microinjected into the squid giant presynaptic terminal (Figure 4A). In contrast, neurotransmitter release is unaffected when EGTA is injected even at concentrations as high as 80 mM (Figure 4B). Injection of EGTA is capable of buffering slower Ca2+ signaling processes, such as Ca2+ triggering of synaptic augmentation (Swandulla et al., 1991).
Figure 4. Differential effects of EGTA and BAPTA on transmitter release at the squid giant synapse. While presynaptic microinjection of the rapid Ca2+ chelator, BAPTA, blocks transmitter release (A), microinjection of the slower chelator, EGTA, has no effect. (B) Each family of traces shows five superimposed presynaptic action potentials (Pre), as well as the postsynaptic responses (Post) that they evoke, during the course of chelator injection. While BAPTA reduces synaptic transmission to the point that postsynaptic responses are subthreshold, EGTA has no effect on postsynaptic response strength. Traces originally from Adler et al. (1991).
The interpretation of these results is that differences in the Ca2+ binding rates of these two chelators are responsible for their differential effects on neurotransmitter release. Thus, BAPTA can block transmitter release because it binds Ca2+ more rapidly than the Ca2+ sensor for transmitter release, while EGTA fails to block transmitter release because it binds Ca2+ slower than this sensor. Under conditions appropriate for the squid giant synapse, 80 mM EGTA will bind Ca2+ with a time constant of approximately 50 µsec, while the same concentration of BAPTA requires only a fraction of a microsecond (Augustine et al., 1991). Thus, the Ca2+ sensor for transmitter release must bind Ca2+ in more than 1 µsec and in less than 50 µsec. Knowledge of this temporal constraint has been useful in establishing synaptotagmin as the Ca2+ sensor for transmitter release (Hui et al., 2005). In regard to the spatial dimensions of Ca2+ signaling during transmitter release, a time window of tens of microseconds means that Ca2+ diffuses no more than tens of nanometers prior to triggering transmitter release (Table 1). Thus, a process that is blocked by BAPTA but not by EGTA must be mediated by nanodomain Ca2+ signaling and this is the case for transmitter release at the squid giant synapse.
In summary, a variety of different experimental paradigms all point to the conclusion that transmitter release at the squid giant synapse is caused by nanodomain-style coupling of presynaptic Ca2+ channels to the presynaptic Ca2+ sensor, which presumably is synaptotagmin. In the next section we will see how these and other paradigms have been used to probe the spatial dimensions of presynaptic Ca2+ signaling at a different “giant” synapse, the mammalian calyx of Held.
The giant calyx of Held synapse has been one of the most prominent preparations developed over the past two decades for analysis of synaptic transmission in the mammalian brain (Forsythe, 1994; Borst et al., 1995). Unfortunately, there is conflicting evidence about whether microdomain or nanodomain Ca2+ signaling mediates transmitter release at this synapse (Borst and Sakmann, 1996; Fedchyshyn and Wang, 2005). In this section we will survey this evidence and suggest that the conflict can be resolved by concluding that the spatial range of presynaptic Ca2+ signaling changes over the course of maturation of this synapse.
The calyx of Held synapse undergoes tremendous morphological remodeling in the course of its development, with its presynaptic terminal transforming from a spoon- or club-like structure with thin filopodia before the onset of hearing (P11–12) to a highly digitated structure with stalks and swellings containing a total of 500–800 active zones at maturity (>P16; Figure 5). Such structural transformations are thought to be important for supporting release and replenishment of synaptic vesicles (SVs), as well as facilitating clearance of neurotransmitter to alleviate postsynaptic receptor desensitization during high-frequency transmission (Trussell, 1999; von Gersdorff and Borst, 2002). In practice, calyces become less visible in brainstem slices for patch-clamping as myelination progresses during maturation, meaning that most early studies on the calyx of Held synapse focused on immature synapses (e.g., P8–11).
Figure 5. Age-dependent morphological remodeling of the calyx of Held. Images of the 3-dimensional structure of P8 (A) and P17 (B) calyces filled with the fluorescent marker biotinylated dextran amine (courtesy of G. Grande). The diameter of each calyx sphere is approximately 15–20 micrometers.
Using recordings of presynaptic Ca2+ currents (ICa) and excitatory postsynaptic currents (IEPSC) from young (P8–11) calyx of Held synapses, Borst and Sakmann (1996) first demonstrated that the amplitude of excitatory postsynaptic currents (EPSCs) follows the fourth power of presynaptic Ca2+ influx, similar to the squid giant synapse (Figure 2A). However, unlike the squid giant synapse, injection of EGTA at a concentration as low as 1 mM attenuated EPSCs by about 50%, leading to the conclusion that Ca2+ influx from many open channels (specifically >60) triggers fusion of a single synaptic vesicle. Furthermore, N-, P/Q, and R-type Ca2+ channels were found to jointly mediate transmitter release at this synapse, with P/Q-type Ca2+ channels being the most prominent subtype (Forsythe et al., 1998; Iwasaki and Takahashi, 1998; Wu et al., 1998, 1999). By photolysis of caged Ca2+ and computer simulations with Ca2+ buffer models (Bollmann et al., 2000; Schneggenburger and Neher, 2000), it was estimated that the peak Ca2+ concentration at Ca2+ sensors on SV is as low as 10 µM, an order of magnitude lower than that deduced for the squid giant synapse. These results are in striking contrast to what would be expected for nanodomain coupling, arguing in favor of a microdomain mode of Ca2+ signaling at the immature calyx of Held synapse (Meinrenken et al., 2002).
In parallel with morphological remodeling at the developing calyx of Held, presynaptic action potentials undergo a dramatic shortening in their duration (Taschenberger and von Gersdorff, 2000), which would reduce the number of activated Ca2+ channels and thereby attenuate Ca2+ influx. Given that the relationship between ICa and IEPSC is a power function
where n denotes Ca2+ cooperativity (typically a value of 3–5; Augustine et al., 1985), spike narrowing would lead to a significant reduction in IEPSC. In fact, quantal output is increased in older mice (Joshi and Wang, 2002) or maintained in rats (Iwasaki and Takahashi, 2001). This created a paradox: how could the fewer Ca2+ channels recruited by narrower action potentials during development somehow become more effective in triggering transmitter release (Yang and Wang, 2006; Kochubey et al., 2009; Leão and von Gersdorff, 2009)?
Two possible mechanisms have been considered: (1) the spatial coupling between open Ca2+ channels and Ca2+ sensors tightens to yield nanodomain signaling; and/or (2) the Ca2+ sensor (e.g., synaptotagmin) becomes more sensitive to Ca2+ to detect smaller rises in presynaptic Ca2+ concentration. To test the first possibility, Fedchyshyn and Wang (2005) injected EGTA (10 mM) into developing calyx of Held synapses and found that while this slow Ca2+ buffer potently attenuates transmitter release in immature terminals (P8–12 mice), as previously reported (Borst and Sakmann, 1996), EGTA had surprisingly little effect at mature synapses (P16–18). This indicates that the distance between Ca2+ channels and Ca2+ sensors shortens over the course of development. A similar conclusion was reached by using capacitance measurements of exocytosis under different Ca2+ buffering conditions (Leão and von Gersdorff, 2009). By using a depolarizing paradigm that recruits an increasing number of Ca2+ channels without changing the kinetics of ICa or the driving force for Ca2+, Fedchyshyn and Wang (2005) further demonstrated that Ca2+ cooperativity values (n) were significantly higher in immature synapses than in mature synapses. Based on the logic depicted in Figure 3, this suggests that the number of Ca2+ channels required for triggering release of a synaptic vesicle decreases. It has also been shown that while transmitter release at P8–12 synapses requires both N- and P/Q-type Ca2+ channels, only P/Q-type Ca2+ channels are involved at mature synapses. This suggests that nanodomains contain specific type of Ca2+ channels as part of their molecular architecture.
To test the second possibility, Ca2+ sensor sensitivity was measured in the developing calyx of Held synapse via simultaneous Ca2+ imaging and flash photolysis of caged Ca2+ chelators (Wang et al., 2008; Kochubey et al., 2009). Flash photolysis of caged Ca2+ has the advantage of bypassing Ca2+ entry through Ca2+ channels, instead directly triggering release by increasing intracellular Ca2+ concentration ([Ca2+]i) in a spatially homogeneous manner. Surprisingly, Ca2+ sensor sensitivity decreases over development (e.g., KD values rise from ~80 µM for P9–P11 vs. ~120 µM for P16–P19 synapses) while cooperativity (n) remains the same. Taken together, these studies led to the conclusion that the spatial coupling between Ca2+ channels and Ca2+ sensors switches from microdomain to nanodomain signaling.
Such changes can account for the upregulation of transmitter release over the course of development of the calyx of Held synapse. Computer simulations with a linearized buffered Ca2+ diffusion model and a five-site kinetic model of transmitter release (Naraghi and Neher, 1997; Schneggenburger and Neher, 2000) deduced that during development the peak Ca2+ concentration seen by a Ca2+ sensor during an action potential increases from 35 to 56 µM (Figures 6A,B). This is a consequence of tightened spatial coupling between Ca2+ channels and sensors; the modeling results indicate that the microdomain signaling during an action potential in immature synapses results from 12 Ca2+ channels with 50% open probability and 61 nm separation, while the nanodomain coupling at mature synapses results from 9 Ca2+ channels with 35% open probability and 23 nm spacing (Figure 6C; Wang et al., 2008, 2009). This change in the spatial dimensions of presynaptic Ca2+ signaling nearly doubles the release rate and enables the peak of the local [Ca2+]i transient to occur ~410 µs earlier in mature synapses than in immature synapses (Figures 6A,B). Although direct evidence for the topographic organization of Ca2+ channels at the developing calyx remains elusive, these simulation results provide a theoretical framework for envisaging how a developmental transition from microdomain to nanodomain coupling can recapitulate a number of experimental observations, including an explanation for how narrower action potentials become more effective in triggering transmitter release (Taschenberger and von Gersdorff, 2000).
Figure 6. Developmental change in presynaptic Ca2+ signaling at the calyx of Held synapse. (A,B) Developmental tightening of coupling between Ca2+ channels and synaptic vesicles boosts the local [Ca2+]i transient seen by the Ca2+ sensor (red traces) and the rate of transmitter release (blue traces) as action potentials (black traces) narrow (from Wang et al., 2008). (C) Transformation from microdomain to nanodomain coupling of Ca2+ channels to transmitter release. Note that nanodomains are associated with shorter distance between Ca2+ channels and synaptic vesicles (SV) both in the plane of the presynaptic plasma membrane, as well as orthogonal to this membrane. This spatial reorganization of Ca2+ signaling is associated with translocation of septins (green) away from the active zone during development.
The squid giant synapse and calyx of Held synapse are often considered to be specialized synapses because of their extraordinary size, speed and strength of synaptic transmission. Hence nanodomain coupling could be a feature unique to such high-fidelity, excitatory “relay” synapses. However, this clearly is not the case because a number of synapses have now been shown to release neurotransmitters via nanodomain coupling.
In line with experiments suggesting nanodomain coupling of Ca2+ influx to transmitter release at the squid giant synapse and calyx of Held, early studies indicated that Ca2+ channels are tightly coupled to Ca2+ sensors to enable highly efficient transmission at neuromuscular synapses (Yoshikami et al., 1989; Delaney et al., 1991). In some cases, opening of a single Ca2+ channel is sufficient for triggering of fusion events at the active zones of chick ciliary ganglion synapses (Stanley, 1993; Gentile and Stanley, 2005).
Initial studies in mammalian synapses appeared to argue in favor of microdomain coupling (Luebke et al., 1993; Takahashi and Momiyama, 1993; Wheeler et al., 1994; Wu and Saggau, 1994; Mintz et al., 1995). These studies combined Ca2+ imaging in presynaptic terminals with patch-clamp recordings from postsynaptic neurons and treatment with subtype-specific Ca2+ channel blockers. The results collectively showed that not only are multiple Ca2+ channels involved in presynaptic Ca2+ signaling but also that different subtypes of Ca2+ channels can mediate transmitter release at a synapse, leading to the idea that opening of many adjacent Ca2+ channels forms microdomains.
It should be noted that the conditions employed in these different experiments differ in many ways. For example, the fraction of Ca2+ channels activated by an action potential differ, ranging from ~10% at the squid giant synapse to >70% at some mammalian central synapses. In addition, extracellular Ca2+ concentrations ranged from 11 mM for the squid synapse to 1–2 mM for mammalian central synapses and experimental temperatures varied from 15 to 37°C. All of these variables profoundly influence the number of Ca2+ channels opened by an action potential, as well as the driving force on Ca2+ and the kinetics of Ca2+ diffusion within nerve terminals, thereby affecting the coupling of Ca2+ channels to transmitter release.
Under experimental conditions where these variables have been somewhat controlled and are comparable to conditions employed at the developing calyx of Held synapse, emerging evidence suggests that several conventional mammalian central synapses employ nanodomain Ca2+ signaling (Eggermann et al., 2011). For example, nanodomain Ca2+ signaling is found both at hippocampal basket cell-granule cell inhibitory synapses and at cerebellar parallel fiber-Purkinje cell excitatory synapses (Bucurenciu et al., 2008; Eggermann and Jonas, 2011; Schmidt et al., 2013). In addition, transmission at sensory synapses other than the calyx of Held apparently employ nanodomain coupling (Brandt et al., 2005; Jarsky et al., 2010). Together, results from a wide variety of mammalian synapses support the notion that nanodomain coupling is highly conserved and is therefore a general scheme for Ca2+ triggering of rapid neurotransmitter release.
We have highlighted key evidence from two giant synapses, the squid giant synapse and the calyx of Held synapse, that firmly establish nanodomains as the predominant mode of Ca2+ signaling that couples Ca2+ influx through presynaptic Ca2+ channels to vesicular fusion and synaptic transmission. There is no doubt that nanodomains exist at many synapses, but it is interesting to note that this mode of Ca2+ signaling appears to be primarily associated with synapses for fast-spiking neurons, where synaptic transmission is particularly important for preserving temporal precision of information flow (Yang et al., 2014). Nanodomains with tight Ca2+ signaling may be particularly suited to trigger highly synchronized fusion events with minimal synaptic delay, temporal jitter, or asynchronous transmitter release. The narrow action potentials typical of fast-spiking neurons are likely to produce brief Ca2+ signals with rapid rise and collapse of local [Ca2+]. Brief Ca2+ transients will also serve to prevent presynaptic Ca2+ overload and facilitate Ca2+ clearance during prolonged high-frequency transmission. On the other hand, microdomains with loose coupling between Ca2+ channels and Ca2+ sensors may allow a broader dynamic range for tuning quantal output at synapses where activity-dependent forms of synaptic plasticity play a key role in dynamically regulating synaptic efficacy (Vyleta and Jonas, 2014).
Given the dimensions of SV and active zones, it is reasonable to postulate that the nanodomain Ca2+ signaling requires specific molecular specializations to optimize physical interactions between Ca2+ channels and SV (Catterall and Few, 2008; Südhof, 2013). To advance our understanding of local Ca2+ signaling in presynaptic terminals, it will next be important to define the specific molecular specializations underlying both microdomain and nanodomain Ca2+ signaling, and how these two modes of Ca2+ signaling are regulated during development. Emerging evidence indicate that several presynaptic proteins help tether Ca2+ channels to SV; these proteins include RIM (Kiyonaka et al., 2007; Kaeser et al., 2011), Munc 13 (Chen et al., 2013), bassoon (Davydova et al., 2014) and even synaptotagmin (Young and Neher, 2009). Genetic deletion of the cytometrix protein Bruchpilot loosens the coupling of Ca2+ channels and sensors at Drosophila presynaptic terminals (Kittel et al., 2006). In contrast, knockout of septin 5, a filamentous protein that interacts with the SNARE proteins that mediate exocytosis, transforms microdomain coupling typical of immature synapses to nanodomain coupling with functional and morphological phenotypes nearly identical to mature calyces (Figure 6C; Yang et al., 2010). These results suggest that these two modes of local Ca2+ signaling are differentiated by specific molecular substrates and potentially could be inter-convertible to confer distinctive Ca2+ signaling properties to synapses.
The authors declare that the research was conducted in the absence of any commercial or financial relationships that could be construed as a potential conflict of interest.
We thank Karen Chung for her help in preparing this paper. This work was supported by a CRP grant from the National Research Foundation of Singapore and by the World Class Institute (WCI) Program of the National Research Foundation of Korea (NRF) funded by the Ministry of Education, Science and Technology of Korea (MEST) (NRF Grant Number: WCI 2009-003) (to George J. Augustine), and by Operating Grants from the Canadian Institutes of Health Research (MOP-77610, MOP-81159, MOP-14692, VIH-105441) and Canada Research Chair (to Lu-Yang Wang).
Adler, E. M., Augustine, G. J., Duffy, S. N., and Charlton, M. P. (1991). Alien intracellular calcium chelators attenuate neurotransmitter release at the squid giant synapse. J. Neurosci. 11, 1496–1507.
Augustine, G. J. (1990). Regulation of transmitter release at the squid giant synapse by presynaptic delayed rectifier potassium current. J. Physiol. 431, 343–364. doi: 10.1113/jphysiol.1990.sp018333
Pubmed Abstract | Pubmed Full Text | CrossRef Full Text | Google Scholar
Augustine, G. J., Adler, E. M., and Charlton, M. P. (1991). The calcium signal for transmitter secretion from presynaptic nerve terminals. Ann. N Y Acad. Sci. 635, 365–381. doi: 10.1111/j.1749-6632.1991.tb36505.x
Pubmed Abstract | Pubmed Full Text | CrossRef Full Text | Google Scholar
Augustine, G. J., and Charlton, M. P. (1986). Calcium dependence of presynaptic calcium current and post-synaptic response at the squid giant synapse. J. Physiol. 381, 619–640.
Augustine, G. J., Charlton, M. P., and Smith, S. J. (1985). Calcium entry and transmitter release at voltage-clamped nerve terminals of squid. J. Physiol. 367, 163–181.
Augustine, G. J., Santamaria, F., and Tanaka, K. (2003). Local calcium signaling in neurons. Neuron 40, 331–346. doi: 10.1016/s0896-6273(03)00639-1
Pubmed Abstract | Pubmed Full Text | CrossRef Full Text | Google Scholar
Bollmann, J. H., Sakmann, B., and Borst, J. G. (2000). Calcium sensitivity of glutamate release in a calyx-type terminal. Science 289, 953–957. doi: 10.1126/science.289.5481.953
Pubmed Abstract | Pubmed Full Text | CrossRef Full Text | Google Scholar
Borst, J. G., Helmchen, F., and Sakmann, B. (1995). Pre- and postsynaptic whole-cell recordings in the medial nucleus of the trapezoid body of the rat. J. Physiol. 489, 825–840. doi: 10.1113/jphysiol.1995.sp021095
Pubmed Abstract | Pubmed Full Text | CrossRef Full Text | Google Scholar
Borst, J. G., and Sakmann, B. (1996). Calcium influx and transmitter release in a fast CNS synapse. Nature 383, 431–434. doi: 10.1038/383431a0
Pubmed Abstract | Pubmed Full Text | CrossRef Full Text | Google Scholar
Brandt, A., Khimich, D., and Moser, T. (2005). Few CaV1.3 channels regulate the exocytosis of a synaptic vesicle at the hair cell ribbon synapse. J. Neurosci. 25, 11577–11585. doi: 10.1523/jneurosci.3411-05.2005
Pubmed Abstract | Pubmed Full Text | CrossRef Full Text | Google Scholar
Bucurenciu, I., Kulik, A., Schwaller, B., Frotscher, M., and Jonas, P. (2008). Nanodomain coupling between Ca2+ channels and Ca2+ sensors promotes fast and efficient transmitter release at a cortical GABAergic synapse. Neuron 57, 536–545. doi: 10.1016/j.neuron.2007.12.026
Pubmed Abstract | Pubmed Full Text | CrossRef Full Text | Google Scholar
Catterall, W. A., and Few, A. P. (2008). Calcium channel regulation and presynaptic plasticity. Neuron 59, 882–901. doi: 10.1016/j.neuron.2008.09.005
Pubmed Abstract | Pubmed Full Text | CrossRef Full Text | Google Scholar
Chad, J. E., and Eckert, R. (1984). Calcium domains associated with individual channels can account for anomalous voltage relations of Ca-dependent responses. Biophys. J. 45, 993–999. doi: 10.1016/s0006-3495(84)84244-7
Pubmed Abstract | Pubmed Full Text | CrossRef Full Text | Google Scholar
Chen, Z., Cooper, B., Kalla, S., Varoqueaux, F., and Young, S. M. (2013). The Munc13 proteins differentially regulate readily releasable pool dynamics and calcium-dependent recovery at a central synapse. J. Neurosci. 33, 8336–8351. doi: 10.1523/jneurosci.5128-12.2013
Pubmed Abstract | Pubmed Full Text | CrossRef Full Text | Google Scholar
Davydova, D., Marini, C., King, C., Klueva, J., Bischof, F., Romorini, S., et al. (2014). Bassoon specifically controls presynaptic P/Q-type Ca2+ channels via RIM-binding protein. Neuron 82, 181–194. doi: 10.1016/j.neuron.2014.02.012
Pubmed Abstract | Pubmed Full Text | CrossRef Full Text | Google Scholar
Delaney, K., Tank, D. W., and Zucker, R. S. (1991). Presynaptic calcium and serotonin-mediated enhancement of transmitter release at crayfish neuromuscular junction. J. Neurosci. 11, 2631–2643.
Dodge, F. A. Jr., and Rahamimoff, R. (1967). Co-operative action a calcium ions in transmitter release at the neuromuscular junction. J. Physiol. 193, 419–432.
Eggermann, E., Bucurenciu, I., Goswami, S. P., and Jonas, P. (2011). Nanodomain coupling between Ca2+ channels and sensors of exocytosis at fast mammalian synapses. Nat. Rev. Neurosci. 13, 7–21. doi: 10.1038/nrn3125
Pubmed Abstract | Pubmed Full Text | CrossRef Full Text | Google Scholar
Eggermann, E., and Jonas, P. (2011). How the ‘slow’ Ca2+ buffer parvalbumin affects transmitter release in nanodomain-coupling regimes. Nat. Neurosci. 15, 20–22. doi: 10.1038/nn.3002
Pubmed Abstract | Pubmed Full Text | CrossRef Full Text | Google Scholar
Fedchyshyn, M. J., and Wang, L. Y. (2005). Developmental transformation of the release modality at the calyx of Held synapse. J. Neurosci. 25, 4131–4140. doi: 10.1523/jneurosci.0350-05.2005
Pubmed Abstract | Pubmed Full Text | CrossRef Full Text | Google Scholar
Forsythe, I. D. (1994). Direct patch recording from identified presynaptic terminals mediating glutamatergic EPSCs in the rat CNS, in vitro. J. Physiol. 479, 381–387. doi: 10.1113/jphysiol.1994.sp020303
Pubmed Abstract | Pubmed Full Text | CrossRef Full Text | Google Scholar
Forsythe, I. D., Tsujimoto, T., Barnes-Davies, M., Cuttle, M. F., and Takahashi, T. (1998). Inactivation of presynaptic calcium current contributes to synaptic depression at a fast central synapse. Neuron 20, 797–807. doi: 10.1016/s0896-6273(00)81017-x
Pubmed Abstract | Pubmed Full Text | CrossRef Full Text | Google Scholar
Gentile, L., and Stanley, E. F. (2005). A unified model of presynaptic release site gating by calcium channel domains. Eur. J. Neurosci. 21, 278–282. doi: 10.1111/j.1460-9568.2004.03841.x
Pubmed Abstract | Pubmed Full Text | CrossRef Full Text | Google Scholar
Hsu, S. F., Augustine, G. J., and Jackson, M. B. (1996). Adaptation of Ca2+-triggered exocytosis in presynaptic terminals. Neuron 17, 501–512. doi: 10.1016/s0896-6273(00)80182-8
Pubmed Abstract | Pubmed Full Text | CrossRef Full Text | Google Scholar
Hui, E., Bai, J., Wang, P., Sugimori, M., Llinas, R. R., and Chapman, E. R. (2005). Three distinct kinetic groupings of the synaptotagmin family: candidate sensors for rapid and delayed exocytosis. Proc. Natl. Acad. Sci. U S A 102, 5210–5214. doi: 10.1073/pnas.0500941102
Pubmed Abstract | Pubmed Full Text | CrossRef Full Text | Google Scholar
Iwasaki, S., and Takahashi, T. (1998). Developmental changes in calcium channel types mediating synaptic transmission in rat auditory brainstem. J. Physiol. 509, 419–423. doi: 10.1111/j.1469-7793.1998.419bn.x
Pubmed Abstract | Pubmed Full Text | CrossRef Full Text | Google Scholar
Iwasaki, S., and Takahashi, T. (2001). Developmental regulation of transmitter release at the calyx of Held in rat auditory brainstem. J. Physiol. 534, 861–871. doi: 10.1111/j.1469-7793.2001.00861.x
Pubmed Abstract | Pubmed Full Text | CrossRef Full Text | Google Scholar
Jarsky, T., Tian, M., and Singer, J. H. (2010). Nanodomain control of exocytosis is responsible for the signaling capability of a retinal ribbon synapse. J. Neurosci. 30, 11885–11895. doi: 10.1523/jneurosci.1415-10.2010
Pubmed Abstract | Pubmed Full Text | CrossRef Full Text | Google Scholar
Joshi, I., and Wang, L. Y. (2002). Developmental profiles of glutamate receptors and synaptic transmission at a single synapse in the mouse auditory brainstem. J. Physiol. 540, 861–873. doi: 10.1111/j.1469-7793.2002.00861.x
Pubmed Abstract | Pubmed Full Text | CrossRef Full Text | Google Scholar
Kaeser, P. S., Deng, L., Wang, Y., Dulubova, I., Liu, X., Rizo, J., et al. (2011). RIM proteins tether Ca2+ channels to presynaptic active zones via a direct PDZ-domain interaction. Cell 144, 282–295. doi: 10.1016/j.cell.2010.12.029
Pubmed Abstract | Pubmed Full Text | CrossRef Full Text | Google Scholar
Kasai, H. (1993). Cytosolic Ca2+ gradients, Ca2+ binding proteins and synaptic plasticity. Neurosci. Res. 16, 1–7. doi: 10.1159/000154621
Pubmed Abstract | Pubmed Full Text | CrossRef Full Text | Google Scholar
Kittel, R. J., Wichmann, C., Rasse, T. M., Fouquet, W., Schmidt, M., Schmid, A., et al. (2006). Bruchpilot promotes active zone assembly, Ca2+ channel clustering and vesicle release. Science 312, 1051–1054. doi: 10.1126/science.1126308
Pubmed Abstract | Pubmed Full Text | CrossRef Full Text | Google Scholar
Kiyonaka, S., Wakamori, M., Miki, T., Uriu, Y., Nonaka, M., Bito, H., et al. (2007). RIM1 confers sustained activity and neurotransmitter vesicle anchoring to presynaptic Ca2+ channels. Nat. Neurosci. 10, 691–701. doi: 10.1038/nn1904
Pubmed Abstract | Pubmed Full Text | CrossRef Full Text | Google Scholar
Klein, M., and Kandel, E. R. (1980). Mechanism of calcium current modulation underlying presynaptic facilitation and behavioral sensitization in Aplysia. Proc. Natl. Acad. Sci. U S A 77, 6912–6916. doi: 10.1073/pnas.77.11.6912
Pubmed Abstract | Pubmed Full Text | CrossRef Full Text | Google Scholar
Kochubey, O., Han, Y., and Schneggenburger, R. (2009). Developmental regulation of the intracellular Ca2+ sensitivity of vesicle fusion and Ca2+-secretion coupling at the rat calyx of Held. J. Physiol. 587, 3009–3023. doi: 10.1113/jphysiol.2009.172387
Pubmed Abstract | Pubmed Full Text | CrossRef Full Text | Google Scholar
Leão, R. M., and von Gersdorff, H. (2009). Synaptic vesicle pool size, release probability and synaptic depression are sensitive to Ca2+ buffering capacity in the developing rat calyx of Held. Braz. J. Med. Biol. Res. 42, 94–104. doi: 10.1590/s0100-879x2009000100014
Pubmed Abstract | Pubmed Full Text | CrossRef Full Text | Google Scholar
Llinás, R., Steinberg, I. Z., and Walton, K. (1981). Relationship between presynaptic calcium current and postsynaptic potential in squid giant synapse. Biophys. J. 33, 323–351. doi: 10.1016/s0006-3495(81)84899-0
Pubmed Abstract | Pubmed Full Text | CrossRef Full Text | Google Scholar
Llinás, R., Sugimori, M., and Silver, R. B. (1992). Microdomains of high calcium concentration in a presynaptic terminal. Science 256, 677–679. doi: 10.1126/science.1350109
Pubmed Abstract | Pubmed Full Text | CrossRef Full Text | Google Scholar
Luebke, J. I., Dunlap, K., and Turner, T. J. (1993). Multiple calcium channel types control glutamatergic synaptic transmission in the hippocampus. Neuron 11, 895–902. doi: 10.1016/0896-6273(93)90119-c
Pubmed Abstract | Pubmed Full Text | CrossRef Full Text | Google Scholar
Meinrenken, C. J., Borst, J. G., and Sakmann, B. (2002). Calcium secretion coupling at calyx of held governed by nonuniform channel-vesicle topography. J. Neurosci. 22, 1648–1667.
Mintz, I. M., Sabatini, B. L., and Regehr, W. G. (1995). Calcium control of transmitter release at a cerebellar synapse. Neuron 15, 675–688. doi: 10.1016/0896-6273(95)90155-8
Pubmed Abstract | Pubmed Full Text | CrossRef Full Text | Google Scholar
Naraghi, M., and Neher, E. (1997). Linearized buffered Ca2+ diffusion in microdomains and its implications for calculation of [Ca2+] at the mouth of a calcium channel. J. Neurosci. 15, 6961–6973.
Neher, E. (1998). Usefulness and limitations of linear approximations to the understanding of Ca2+ signals. Cell Calcium 24, 345–357. doi: 10.1016/s0143-4160(98)90058-6
Pubmed Abstract | Pubmed Full Text | CrossRef Full Text | Google Scholar
Schmidt, H., Brachtendorf, S., Arendt, O., Hallermann, S., Ishiyama, S., Bornschein, G., et al. (2013). Nanodomain coupling at an excitatory cortical synapse. Curr. Biol. 23, 244–249. doi: 10.1016/j.cub.2012.12.007
Pubmed Abstract | Pubmed Full Text | CrossRef Full Text | Google Scholar
Schneggenburger, R., and Neher, E. (2000). Intracellular calcium dependence of transmitter release rates at a fast central synapse. Nature 406, 889–893. doi: 10.1038/35022702
Pubmed Abstract | Pubmed Full Text | CrossRef Full Text | Google Scholar
Schweizer, F. E., Betz, H., and Augustine, G. J. (1995). From vesicle docking to endocytosis: intermediate reactions of exocytosis. Neuron 14, 689–696. doi: 10.1016/0896-6273(95)90213-9
Pubmed Abstract | Pubmed Full Text | CrossRef Full Text | Google Scholar
Simon, S. M., and Llinás, R. R. (1985). Compartmentalization of the submembrane calcium activity during calcium influx and its significance in transmitter release. Biophys. J. 48, 485–498. doi: 10.1016/s0006-3495(85)83804-2
Pubmed Abstract | Pubmed Full Text | CrossRef Full Text | Google Scholar
Smith, S. J., and Augustine, G. J. (1988). Calcium ions, active zones and synaptic transmitter release. Trends Neurosci. 11, 458–464. doi: 10.1016/0166-2236(88)90199-3
Pubmed Abstract | Pubmed Full Text | CrossRef Full Text | Google Scholar
Stanley, E. F. (1993). Single calcium channels and acetylcholine release at a presynaptic nerve terminal. Neuron 11, 1007–1011. doi: 10.1016/0896-6273(93)90214-c
Pubmed Abstract | Pubmed Full Text | CrossRef Full Text | Google Scholar
Südhof, T. C. (2013). Neurotransmitter release: the last millisecond in the life of a synaptic vesicle. Neuron 80, 675–690. doi: 10.1016/j.neuron.2013.10.022
Pubmed Abstract | Pubmed Full Text | CrossRef Full Text | Google Scholar
Swandulla, D., Hans, M., Zipser, K., and Augustine, G. J. (1991). Role of residual calcium in synaptic depression and posttetanic potentiation: fast and slow calcium signaling in nerve terminals. Neuron 7, 915–926. doi: 10.1016/0896-6273(91)90337-y
Pubmed Abstract | Pubmed Full Text | CrossRef Full Text | Google Scholar
Takahashi, T., and Momiyama, A. (1993). Different types of calcium channels mediate central synaptic transmission. Nature 366, 156–158. doi: 10.1038/366156a0
Pubmed Abstract | Pubmed Full Text | CrossRef Full Text | Google Scholar
Taschenberger, H., and von Gersdorff, H. (2000). Fine-tuning an auditory synapse for speed and fidelity: developmental changes in presynaptic waveform, EPSC kinetics and synaptic plasticity. J. Neurosci. 20, 9162–9173.
Trussell, L. O. (1999). Synaptic mechanisms for coding timing in auditory neurons. Annu. Rev. Physiol. 61, 477–496. doi: 10.1146/annurev.physiol.61.1.477
Pubmed Abstract | Pubmed Full Text | CrossRef Full Text | Google Scholar
von Gersdorff, H., and Borst, J. G. (2002). Short-term plasticity at the calyx of held. Nat. Rev. Neurosci. 3, 53–64. doi: 10.1038/nrn705
Pubmed Abstract | Pubmed Full Text | CrossRef Full Text | Google Scholar
Vyleta, N. P., and Jonas, P. (2014). Loose coupling between Ca2+ channels and release sensors at a plastic hippocampal synapse. Science 343, 665–670. doi: 10.1126/science.1244811
Pubmed Abstract | Pubmed Full Text | CrossRef Full Text | Google Scholar
Wang, L. Y., Fedchyshyn, M. J., and Yang, Y. M. (2009). Action potential evoked transmitter release in central synapses: insights from the developing calyx of Held. Mol. Brain 2:36. doi: 10.1186/1756-6606-2-36
Pubmed Abstract | Pubmed Full Text | CrossRef Full Text | Google Scholar
Wang, L. Y., Neher, E., and Taschenberger, H. (2008). Synaptic vesicles in mature calyx of Held synapses sense higher nanodomain calcium concentrations during action potential-evoked glutamate release. J. Neurosci. 28, 14450–14458. doi: 10.1523/jneurosci.4245-08.2008
Pubmed Abstract | Pubmed Full Text | CrossRef Full Text | Google Scholar
Wheeler, D. B., Randall, A., and Tsien, R. W. (1994). Roles of N-type and Q-type Ca2+ channels in supporting hippocampal synaptic transmission. Science 264, 107–111. doi: 10.1126/science.7832825
Pubmed Abstract | Pubmed Full Text | CrossRef Full Text | Google Scholar
Wu, L. G., Borst, J. G., and Sakmann, B. (1998). R-type Ca2+ currents evoke transmitter release at a rat central synapse. Proc. Natl. Acad. Sci. U S A 95, 4720–4725. doi: 10.1073/pnas.95.8.4720
Pubmed Abstract | Pubmed Full Text | CrossRef Full Text | Google Scholar
Wu, L. G., and Saggau, P. (1994). Pharmacological identification of two types of presynaptic voltage-dependent calcium channels at CA3-CA1 synapses of the hippocampus. J. Neurosci. 14, 5613–5622.
Wu, L. G., Westenbroek, R. E., Borst, J. G., Catterall, W. A., and Sakmann, B. (1999). Calcium channel types with distinct presynaptic localization couple differentially to transmitter release in single calyx-type synapses. J. Neurosci. 19, 726–736.
Yang, Y. M., Fedchyshyn, M. J., Grande, G., Aitoubah, J., Tsang, C. W., Xie, H., et al. (2010). Septins regulate developmental switching from microdomain to nanodomain coupling of Ca2+ influx to neurotransmitter release at a central synapse. Neuron 67, 100–115. doi: 10.1016/j.neuron.2010.06.003
Pubmed Abstract | Pubmed Full Text | CrossRef Full Text | Google Scholar
Yang, Y. M., and Wang, L. Y. (2006). Amplitude and kinetics of action potential-evoked Ca2+ current and its efficacy in triggering transmitter release at the developing calyx of held synapse. J. Neurosci. 26, 5698–5708. doi: 10.1523/jneurosci.4889-05.2006
Pubmed Abstract | Pubmed Full Text | CrossRef Full Text | Google Scholar
Yang, Y. M., Wang, W., Fedchyshyn, M. J., Zhou, Z., Ding, J., and Wang, L. Y. (2014). Enhancing the fidelity of neurotransmission by activity-dependent facilitation of presynaptic potassium currents. Nat. Commun. 5:4564. doi: 10.1038/ncomms5564
Pubmed Abstract | Pubmed Full Text | CrossRef Full Text | Google Scholar
Yoshikami, D., Bagabaldo, Z., and Olivera, B. M. (1989). The inhibitory effects of omega-conotoxins on Ca channels and synapses. Ann. N Y Acad. Sci. 560, 230–248. doi: 10.1111/j.1749-6632.1989.tb24100.x
Pubmed Abstract | Pubmed Full Text | CrossRef Full Text | Google Scholar
Young, S. M., and Neher, E. (2009). Synaptotagmin has an essential function in synaptic vesicle positioning for synchronous release in addition to its role as a calcium sensor. Neuron 63, 482–496. doi: 10.1016/j.neuron.2009.07.028
Pubmed Abstract | Pubmed Full Text | CrossRef Full Text | Google Scholar
Zucker, R. S., and Fogelson, A. L. (1986). Relationship between transmitter release and presynaptic calcium influx when calcium enters through discrete channels. Proc. Natl. Acad. Sci. U S A 83, 3032–3036. doi: 10.1073/pnas.83.9.3032
Pubmed Abstract | Pubmed Full Text | CrossRef Full Text | Google Scholar
Keywords: neurotransmitter release, calcium signaling, calcium channels, presynaptic terminals, synaptic vesicle trafficking
Citation: Wang L-Y and Augustine GJ (2015) Presynaptic nanodomains: a tale of two synapses. Front. Cell. Neurosci. 8:455. doi: 10.3389/fncel.2014.00455
Received: 16 October 2014; Paper pending published: 18 November 2014;
Accepted: 16 December 2014; Published online: 26 January 2015.
Edited by:
Hartmut Schmidt, University of Leipzig, GermanyReviewed by:
Manfred Heckmann, University of Wuerzburg, GermanyCopyright © 2015 Wang and Augustine. This is an open-access article distributed under the terms of the Creative Commons Attribution License (CC BY). The use, distribution and reproduction in other forums is permitted, provided the original author(s) or licensor are credited and that the original publication in this journal is cited, in accordance with accepted academic practice. No use, distribution or reproduction is permitted which does not comply with these terms.
*Correspondence: George J. Augustine, Lee Kong Chian School of Medicine, Nanyang Technological University, 61 Biopolis Drive, Proteos, Singapore 138673, Singapore e-mail:Z2VvcmdlLmF1Z3VzdGluZUBudHUuZWR1LnNn
Disclaimer: All claims expressed in this article are solely those of the authors and do not necessarily represent those of their affiliated organizations, or those of the publisher, the editors and the reviewers. Any product that may be evaluated in this article or claim that may be made by its manufacturer is not guaranteed or endorsed by the publisher.
Research integrity at Frontiers
Learn more about the work of our research integrity team to safeguard the quality of each article we publish.