- Department of Basic Neuroscience, Geneva Medical Center, University of Geneva, Geneva, Switzerland
Inhibitory transmission through the neurotransmitter γ-aminobutyric acid (GABA) shapes network activity in the mammalian cerebral cortex by filtering synaptic incoming information and dictating the activity of principal cells. The incredibly diverse population of cortical neurons that use GABA as neurotransmitter shows an equally diverse range of mechanisms that regulate changes in the strength of GABAergic synaptic transmission and allow them to dynamically follow and command the activity of neuronal ensembles. Similarly to glutamatergic synaptic transmission, activity-dependent functional changes in inhibitory neurotransmission are accompanied by alterations in GABAergic synapse structure that range from morphological reorganization of postsynaptic density to de novo formation and elimination of inhibitory contacts. Here we review several aspects of structural plasticity of inhibitory synapses, including its induction by different forms of neuronal activity, behavioral and sensory experience and the molecular mechanisms and signaling pathways involved. We discuss the functional consequences of GABAergic synapse structural plasticity for information processing and memory formation in view of the heterogenous nature of the structural plasticity phenomena affecting inhibitory synapses impinging on somatic and dendritic compartments of cortical and hippocampal neurons.
Introduction: GABAergic System as a Substrate for Brain Plasticity
During the last decades neuroscientists and physiologists worldwide have made an herculean effort to elucidate the mechanisms of activity-driven changes in synaptic strength and understand its physiological significance as the brain substrate for learning and memory (Malenka and Bear, 2004; Mayford et al., 2012). Recent observations have added an additional level of complexity to activity-dependent synaptic plasticity of excitatory synaptic transmission by showing that adaptive changes in glutamatergic synapse strength are accompanied by a dynamic regulation of excitatory synapse structure (Yuste and Bonhoeffer, 2001; Matsuzaki et al., 2004). Activity-driven structural changes include actin-dependent enlargement of the postsynaptic density (Matus, 2000; Honkura et al., 2008) and formation and elimination of excitatory synapses (Holtmaat and Svoboda, 2009). In vivo studies have shown that dendritic spines, the morphological correlate of glutamatergic synapses in excitatory neurons, are formed and eliminated in response to synaptic activity patterns induced by learning behavior (Xu et al., 2009) and that formation of durable memories is directly correlated with the stability and formation of new excitatory synapses (Yang et al., 2009). In addition to functional plasticity, the structural rearrangement of glutamatergic synapses is critically involved in the brain processes leading to learning and memory formation (Caroni et al., 2012).
The plasticity of inhibitory neurotransmission has received relatively less attention than its excitatory counterpart despite its potential to deeply alter the function of cortical networks. A recent attempt to unravel different forms of plasticity in inhibitory γ-aminobutiric acid (GABA) releasing neurons has proven to be unexpectedly successful and cover many different aspects of the physiological properties of inhibitory cell’s, including glutamatergic inputs, dendritic and axonal structure, passive properties and GABAergic synapses onto target cells (Kullmann et al., 2012). As glutamatergic contacts, inhibitory synapses have the remarkable property of being able to alter the efficiency of synaptic transmission according to the patterns of activity that flow through them. Research carried out during the last two decades has made clear that inhibitory synapses undergo short- and long-term forms of plasticity and numerous examples of activity dependent changes in synaptic strength of GABAergic synapses have been described in different brain areas, including hippocampus and cortex (Gaiarsa et al., 2002; Castillo et al., 2011; Méndez and Bacci, 2011). The complex and varied collection of pre- and postsynaptic mechanisms that underlie the induction and expression of GABAergic synapse plasticity mirrors the heterogeneous nature of different inhibitory neuron subtypes that form cortical GABAergic synapses (Méndez and Bacci, 2011).
It is now clear that, similar to excitatory synapses, the molecular composition and structure of GABAergic synapses show a high degree of dynamism (Kittler and Moss, 2003; Lévi et al., 2008; Chen et al., 2012; van Versendaal et al., 2012). The changing nature of inhibitory synapse structure raises the possibility that functional alterations in inhibitory neurotransmission may occur through structural rearrangements. Indeed, synaptic activity driven functional changes of inhibitory neurotransmission are accompanied by modifications in the structure of GABAergic synapses with two major consequences: alteration of synaptic size and morphology and formation and elimination of inhibitory contacts (Figure 1). Here we review several aspects of the structural plasticity of mammalian cortical and hippocampal GABAergic synapses. What is the nature of the morphological changes affecting hippocampal and cortical inhibitory synapses? What is the role of synaptic activity and experience in the induction of structural alterations of inhibitory synapses and what molecular mechanisms underlie this form of plasticity? In view of the staggering diversity of different inhibitory neuron subtypes that configure cortical networks, what are the potential physiological consequences of the structural remodeling of GABAergic synapses? We attempt to answer these questions with the aim of providing a framework to understand the characteristics, mechanisms and functions of inhibitory synapse structural remodeling.
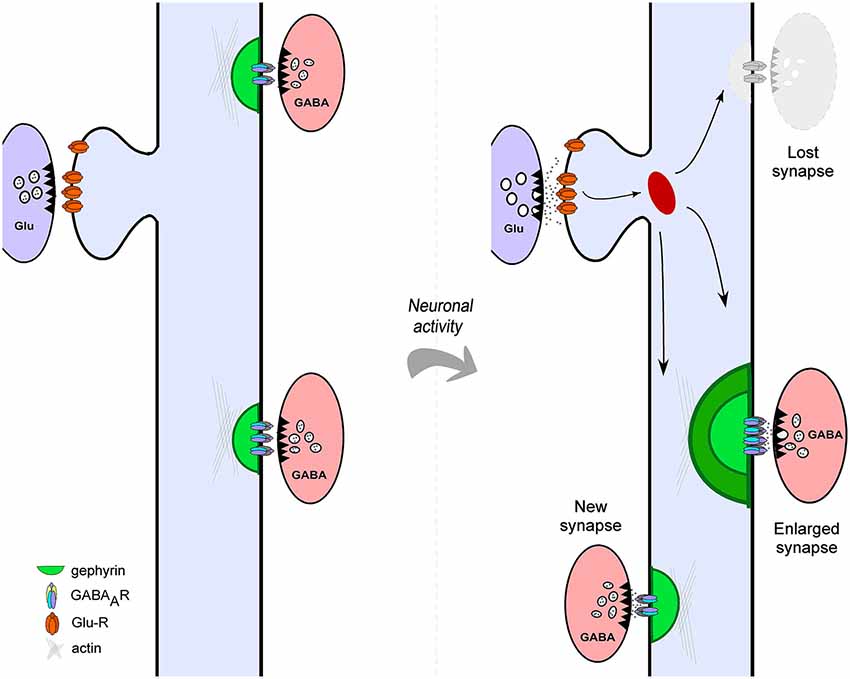
Figure 1. Structural plasticity of cortical GABAergic Synapses. Neuronal activity drives different forms of structural plasticity of gephyrin-containing inhibitory synapses. Neuronal activity may alter the size of pre-existing inhibitory contacts (left panel) or trigger complete elimination of GABAergic synapses (shaded gray, right panel). In addition, new gephyrin clusters are formed at different dendritic locations in response to altered levels of network activity (right panel).
GABAergic Synapses and Functional Plasticity
Inhibitory synapses, also known as symmetrical synapses (or type II) by their ultrastructural features (Figure 2A; Gray, 1959; Colonnier, 1968) are arranged around the scaffold protein gephyrin, the main molecular organizer of inhibitory synapses (Sassoè-Pognetto et al., 2011; Tyagarajan and Fritschy, 2014). Gephyrin forms submembranous hexagonal macromolecular complexes (Xiang et al., 2001; Fritschy et al., 2008) that orchestrate multiple protein-protein interactions with GABAA Receptors (GABAA R), the cytoskeleton and various cell adhesion and signal transduction proteins (Figure 2B).
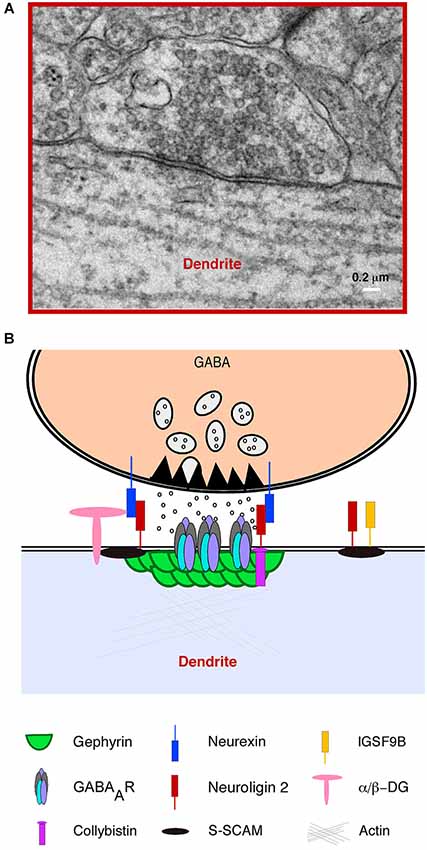
Figure 2. Structural features and molecular composition of an hippocampal GABAergic Synapse. (A) Electron microscopy (EM) image of an inhibitory (symmetrical synapse) between a GABAergic presynaptic terminal and a proximal apical dendrite of a CA1 hippocampal pyramidal neuron. The image shows typical ultrastructural features of inhibitory synapses: a distinguishable synaptic cleft, pleomorphic GABA containing vesicles and a thin post synaptic density (PSD) facing an active zone of similar width. (B) A simplified cartoon of a typical GABAergic synapse illustrating the presynaptic terminal with vesicles containing GABA, the active zone, presynaptic neurexins and the inhibitory postsynaptic density showing a vast number of postsynaptic proteins including GABAA receptors and the scaffold protein gephyrin.
Inhibitory synapses are non-uniformly distributed along the different subcellular compartments of pyramidal cells. In the hippocampus, the highest density of GABAergic inputs is found in the soma and proximal dendrites compared to intermediate and distal dendrites (Megías et al., 2001). GABAergic synapses are formed by a highly heterogeneous group of cells (Markram et al., 2004; Ascoli et al., 2008) that mostly correspond to the definition of interneuron (locally projecting neuron with axonal arborization, dendritic and somatic compartments in the same anatomic structure). Interestingly, some interneurons (INs) form synapses exclusively on the dendrites of other neurons (Maccaferri, 2005), while other target the somatic compartment (Freund and Katona, 2007). This highly stereotyped axonal targeting of GABAergic synapses to specific cellular compartments allows different INs subtypes to selectively affect the different computational processes that actively integrate synaptic inputs in the soma and dendrites (Miles et al., 1996; Pouille and Scanziani, 2001, 2004). Adult INs have a critical role in maintaining physiological activity levels, stabilizing neuronal networks and preventing runaway excitation through different forms of GABA mediated inhibition. In addition, INs are able to form synaptic contacts with a large number of neighboring neurons and provide synchronous inhibition to functionally significant portions of the network (McBain and Fisahn, 2001). In this way, INs coordinate the spiking activity of large number of cells and are critically involved in the genesis of the wide variety of rhythmic network activities that are the basis of cognition and behavior (Klausberger and Somogyi, 2008; Buzsáki and Wang, 2012).
Functional Plasticity of Cortical GABAergic Synapses
The heterogeneous population of cortical INs shows an equally diverse range of mechanisms of activity driven changes in synaptic strength of GABAergic neurotransmission. Retrograde signaling has been shown to play a prominent role in the modulation of GABAergic synaptic plasticity. Activity-dependent synthesis and release of different signaling molecules by post-synaptic excitatory neurons induce short and long-term forms of plasticity in cortical inhibitory synapses. Endocannabinoids are synthesized by postsynaptic neurons in response to increased Ca2+ concentrations, action potential trains and metabotropic glutamate, dopamine, and acetylcholine receptor activation (Kano et al., 2009). Endocannabinoids travel retrogradely and activate CB1 receptor, a G-protein coupled receptor located mainly on presynaptic terminals, resulting in short or long term decreases in GABA release (Piomelli, 2003; Chevaleyre et al., 2006). Another form of desinhibition by retrograde signaling is mediated by postsynaptic somatodendritic glutamate release and activation of presynaptic metabotropic glutamate receptors (Zilberter, 2000). In addition, several examples of spike-timing dependent plasticity of GABAergic synapses have been described in the cortex and hippocampus. This form of plasticity requires near coincident pre- and postsynaptic spiking. The precise rules that dictate the sign of plasticity, potentiation or depression of GABAergic synapses seem to differ between hippocampus (Woodin et al., 2003), entorhinal cortex (Haas et al., 2006) and neocortex (Holmgren and Zilberter, 2001). High frequency stimulation of synaptic inputs has also the potential to produce long term depression (LTD) and potentiation of GABAergic neurotransmission (Komatsu, 1996; Komatsu and Yoshimura, 2000; Patenaude et al., 2003) by pre- (Chevaleyre and Castillo, 2003) and postsynaptic mechanisms (Lu et al., 2000). Astrocyte-dependent Ca2+ signaling (Kang et al., 1998) has been shown to modulate the strength of GABA synaptic signaling in the hippocampus. In the neocortex layer 4 excitatory neurons coupling of postsynaptic subthreshold depolarizations with presynaptic action potentials of presynaptic INs triggers a form of long term potentiation (LTP) of GABAergic synaptic transmission that is modulated by sensory activity (Maffei et al., 2006).
All these forms of induction and expression of functional plasticity at inhibitory GABAergic synapses occur through a large variety of mechanisms. Changes in GABAergic synaptic strength are mediated by mechanisms that range from post-transcriptional modifications of GABA receptors such as phosphorylation (Vithlani and Moss, 2009), ubiquitination (Saliba et al., 2007), trafficking (Vithlani et al., 2011) and lateral diffusion (Lévi et al., 2008; Bannai et al., 2009), to alterations in presynaptic GABA release and variations in chloride (the main GABAA receptor permeable ion) driving force (Woodin et al., 2003) and give rise to short and long terms changes in inhibitory synapse efficacy. Other forms of plasticity of inhibitory transmission depend on GABA and glutamate postsynaptic signaling through metabotropic and ionotropic receptors (Lu et al., 2000; Wang and Maffei, 2014). Although during the last decades our knowledge of the modes and mechanisms of activity dependent changes in GABAergic synaptic strength has grown enormously (Kullmann et al., 2012), we have a limited knowledge of the structural remodeling that accompanies most of these functional changes. Structural remodeling of GABAergic synapses may represent an essential mechanism for activity dependent regulation of GABAergic function.
Structural Plasticity of GABAergic Synapses
Examples of activity mediated structural plasticity of GABAergic synapses have been observed during the development of the mammalian brain. Brain patterning occurs through genetic programs that guide the generation and migration of INs and the innervation patterns, geometry and target specificity of GABAergic axonal projections (Hébert and Fishell, 2008; Bartolini et al., 2013). In mice, maturation of GABAergic connectivity occurs both embryonically and during the first postnatal weeks, leaving open the possibility of an experience dependent modulation of inhibitory synapse formation. Indeed, during early stages of brain formation, experience and activity dependent processes overlap with genetically encoded mechanisms of development and regulate several aspects of GABAergic synaptogenesis, including axonal branching, formation of GABAergic synaptic contacts and synaptic strength (Huang et al., 1999; Doischer et al., 2008; Huang, 2009). It has been shown that reduced synaptic activity induced by sensory deprivation in young, but not adult animals, produces specific reductions in the number of perisomatic inhibitory synapses on cortical excitatory cells, unmasking a regulatory role of neuronal activity in determining the density of inhibitory cell contacts in this specific cellular compartment (Jiao et al., 2006). Other studies have provided similar results using pharmacological and genetic manipulation of activity in developing hippocampal cultures (Marty et al., 2000; Hartman et al., 2006). Interestingly, these studies point to a homeostatic role of inhibitory synapse structural plasticity in compensating alterations in global activity levels of developing cortical and hippocampal networks. GABA content in INs is a critical mediator of GABAergic innervation in the developing visual cortex. Knocking down Glutamic Acid Decarboxilase 67 (GAD67), the main GABA synthesizing enzyme in cortical INs, resulted in serious deficits in axonal branching and decreased formation and size of perisomatic synapses on cortical pyramidal neurons (Chattopadhyaya et al., 2004, 2007). The regulation of GAD67 expression and function by synaptic activity suggests that GABA itself could be a mediator of activity dependent structural remodeling of GABAergic synapses.
Activity Dependent Ultrastructural Changes in GABAergic Synapses
Subtle activity induced changes in adult GABA synapse morphology have been studied using electron microscopy (EM), which allows the unequivocal identification of symmetrical GABAergic synapses and the analysis of synaptic ultrastructure at very high resolution. This technique has shown that patterns of activity that produce functional and structural changes in excitatory synapses also induce structural remodeling of inhibitory synapses. Both in vitro (Lushnikova et al., 2011) and in vivo (Nusser et al., 1998), the rise in synaptic activity levels increased inhibitory synaptic junctional area and complexity and proportion of somatic cell surface covered with inhibitory postsynaptic densitiy (PSD). In other cases, different aspects of inhibitory synapse plasticity occur in a coordinated manner. Bourne and Harris (2011) used LTP inducing protocols and EM three dimensional reconstructions of CA1 pyramidal neurons dendritic segments in acute hippocampal slices to show that plasticity inducing protocols produce a decrease in dendritic inhibitory PSD density that is counterbalanced by an extension of the individual PSD areas. These experiments demonstrate that the adult inhibitory PSD is endowed with mechanisms that allow dynamic changes in the structure in response to alterations in the levels of network activity.
Chronic sensory deprivation by whisker trimming induces a net decrease in the number of symmetric GABAergic synapses in the dendrites of layer 4 neurons of barrel cortex, the main target neurons for the thalamocortical axons relaying sensory information from the whiskers (Micheva and Beaulieu, 1995). Artificially increasing single whisker activity by passive stimulation leads to a rise in dendritic inhibitory synapse density in the correspondent barrel but not in the neighboring ones (Knott et al., 2002). Interestingly, sensory deprivation (through whisker trimming) and stimulation (by artificial chronic movement of the whiskers) result in opposite effects on the number of dendritic inhibitory synapses by preferentially affecting inhibitory synapses contacting dendritic spines and, to a much lower extent, those formed in the shaft of dendrites of principal cells (Micheva and Beaulieu, 1995; Knott et al., 2002). Inhibitory synapses of the barrel cortex are also remodeled in response to learning. A conditioning paradigm involving a group of whiskers induces an increase in the number of symmetric synapses and GABA content of inhibitory axons impinging on dendrites of layer 4 pyramids of the barrel corresponding to the trained whiskers (Jasinska et al., 2010). As in the cases described above, this de novo, learning induced GABAergic synaptogenesis affected inhibitory contacts on dendritic spines but not those on the dendritic shafts of layer 4 excitatory neurons. It is clear that a direct positive relation exists between synaptic activity and GABA synapse formation in the adult somatosensory cortex and that spine GABA synapses, represent a highly structurally dynamic pool of synapses.
Inhibitory Axon Plasticity
A major difficulty in the study of inhibitory synapse dynamics is the lack of morphological markers at the optical level. However, different approaches have been used to track cortical GABAergic structural plasticity. Transgenic mouse lines with genetically labeled subpopulation of INs allow the visualization of inhibitory axon dynamics in organotypic hippocampal slice cultures and in the cortex in vivo. A pioneer study gained insight in the mechanisms of formation of new inhibitory synapses on CA1 pyramidal cell dendrites in vitro using high resolution fluorescence confocal imaging of the sites of contact of inhibitory axons and postsynaptic structures (Wierenga et al., 2008). Although the vast majority of putative contact sites were stable, the authors were able to detect formation of new inhibitory contacts between GABAergic axons and dendrites of excitatory neurons. Interestingly, new stable contacts were formed at location were pre- and postsynaptic structures were in close apposition (Wierenga et al., 2008). Close observation of the presynaptic component alone has shown that the majority of putative presynaptic structures is stable and shows abundant expression of inhibitory pre and post synaptic proteins (Schuemann et al., 2013). Short-lived boutons show however, lower levels of GABA synapse markers (Schuemann et al., 2013), suggesting a protracted maturation of new GABAergic contacts. Interestingly, the level of network activity directly controlls inhibitory axon plasticity and produces subtle but significant changes in bouton turnover and morphology (Schuemann et al., 2013). Tracking GABAergic axons dynamics in vivo has been possible by implanting cranial widows in mice expressing a fluorescent protein in a subpopulation of inhibitory neurons and visualizing inhibitory presynaptic structures using confocal microscopy in superficial cortical layers (Keck et al., 2011). A certain degree of inhibitory axons structural remodeling has been observed even in conditions of normal sensory activity in the somatosensory cortex: while the length of axonal projections from inhibitory cells in the barrel cortex remains constant, boutons are added and eliminated at a rate of 10% per week (Marik et al., 2010). However, under conditions of altered sensory input by whisker removal, axons from inhibitory neurons in the deprived barrels suffer intense structural modifications, retracting terminals in the vicinity of their cell body and extending collaterals beyond its normal projection range towards non-deprived barrels two days after sensory deprivation. In addition, whisker trimming produces at the same time a general decrease in bouton density (Marik et al., 2010). Similar manipulation of sensory inputs in the visual cortex, synaptic input deprivation by permanent lesion of the retina, induces the disappearance of a fraction of GABAergic boutons in few hours (Keck et al., 2011), suggesting that the rapid loss of functional inhibitory synapses may represent a general adaptive mechanism to decreased levels of synaptic activity that is conserved in different functional areas of the cortex. Axons of GABAergic neurons are dynamic structures, able to alter structural properties in response to altered levels of synaptic activity and sensory experience. By growing and retracting axons, INs are able to increase or decrease the number and change the identity of their postsynaptic targets. The appearance and elimination of boutons suggest the involvement of a mechanism that coordinates changes in pre- and postsynaptic structures during structural plasticity of GABAergic synapses.
The Postsynaptic Side Plasticity
Although tracking presynaptic structures identifies changes in putative inhibitory synaptic contacts the fluorescent tagging of the scaffolding protein gephyrin allows dynamic visualization of the postsynaptic component of GABAergic synapses (Figure 3). During developmental inhibitory synaptogenesis, gephyrin accumulates progressively at sites of new synapse formation following a similar pattern as presynaptic components such as the vesicular GABA transporter VGAT, (Dobie and Craig, 2011). Developing inhibitory postsynapses show a high degree of structural plasticity including translational movements along dendrites in a coordinated manner with presynaptic axons and trafficking of synaptic vesicles from pre-existing boutons to new ones (Dobie and Craig, 2011). However, synaptic activity drives the maturation of inhibitory neurotransmission and results in stable and functionally stronger GABAergic synapses compared with those observed during the early phases of inhibitory synaptogenesis (Dobie and Craig, 2011; Vlachos et al., 2013).
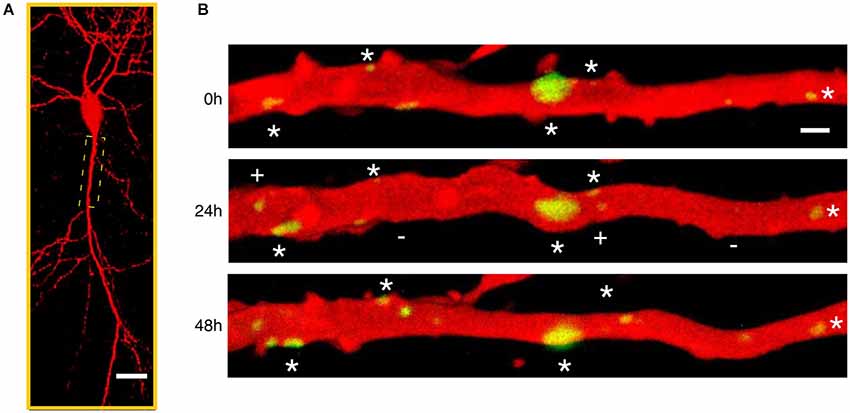
Figure 3. Time-lapse imaging of gephyrin containing inhibitory synapses. (A) Low magnification confocal microscope view of a CA1 pyramidal neuron in a mature hippocampal organotypic culture imaged after biolistic transfection with Red Fluorescent Protein to reveal neuronal structure and Green Fluorescent Protein tagged gephyrin to visualize the postsynaptic component of inhibitory synapses. (B) Repetitive laser scanning confocal imaging of an apical proximal dendrite (boxed region in A) taken every 24 h during 3 consecutive days. A large fraction of gephyrin-containing inhibitory synapses (green) are stable throughout the imaging period (stars) despite the high level of structural variability. In addition, gephyrin clusters appear (plus (+) sign) and disappear (minus (−) sign) at different dendritic locations suggesting continuous formation and elimination of inhibitory synapses.
The use of postsynaptic tagging of inhibitory synapses has the advantage of resolving the subcellular compartments where GABAergic axons impinge on postsynaptic neurons. Using this approach, two recent studies (Chen et al., 2012; van Versendaal et al., 2012) have revealed important features of structural plasticity of GABAergic synapses in visual cortical circuits in vivo. Under normal conditions, a continuos turnover affects a low proportion of GABAergic synapses in dendrites of layer 2/3 pyramidal neurons of the visual cortex. New GABA synapses replace lost inhibitory contacts and tend to be persistent in the majority of the cases (Chen et al., 2012), which contrasts with the low stability of new excitatory connections observed in young and adult animals (Holtmaat et al., 2005). GABAergic synapses on apical dendrites of layer 2/3 pyramidal neurons are preferentially removed in response to decreased sensory inputs by visual deprivation. Interestingly, not all GABAergic synapses on dendrites of pyramidal cells seem to be equally plastic. The most dynamic population of GABA synapses are those contacting dendritic spines (specially abundant in distal parts of layer 2/3 cortical pyramidal neurons) compared with inhibitory synapses made on the dendritic shafts (Chen et al., 2012; van Versendaal et al., 2012). Inhibitory synapses are thus dynamic structures formed and eliminated in cortical neurons in vivo in response to physiological changes in network activity levels. Under these same conditions, no alteration in density or turnover of excitatory connections is observed in the same cells (Hofer et al., 2009), suggesting that structural plasticity of GABA synapses and subsequent rewiring of inhibitory microcircuits is a fundamental mechanism for brain adaptation to sensory experience in layer 2/3 pyramidal cells.
Molecular Mechanisms of Activity-Dependent Structural Plasticity of GABAergic Synapses
The experiments discussed above clearly show that network activity is a major driving force for structural remodeling of inhibitory synapses. Central to the molecular machinery that links synaptic activity with such plastic changes is the Neuronal Per Arnt Sim domain protein 4 (NPAS4), a brain-specific basic helix-loop-helix transcription factor, whose expression is tightly regulated by synaptic activity and postsynaptic calcium influx (Flavell and Greenberg, 2008). Neuronal Per Arnt Sim domain protein 4 regulates the formation of somatic and dendritic inhibitory synapses during development with little effect on excitatory contacts (Lin et al., 2008). In addition, experience-dependent inhibitory synapse formation critically depends on NPAS4-mediated gene expression and results in differential modulation of somatic and dendritic inhibition (Bloodgood et al., 2013). Interestingly, NPAS4 is a critical mediator of activity dependent expression of the neurotrophin Brain Derived Neurotrophic Factor (BDNF) that regulates developmental and activity dependent formation and elimination of inhibitory synapses (Marty et al., 2000; Berghuis et al., 2004; Jovanovic et al., 2004; Kohara et al., 2007). Structural plasticity of GABAergic synapses is at least in part controled by a postsynaptic mechanism that links neuronal activity with alteration in nuclear gene expression.
In a pioneering attempt to elucidate the molecular mechanisms of inhibitory synaptic plasticity, Nusser and colleagues (Nusser et al., 1998) used a combination of quantal analysis of evoked inhibitory postsynaptic currents with quantitative immunogold labeling of synaptic GABAA Rs in hippocampal granule cells. They found that an increase in amplitude of synaptic currents corresponded to a proportional increase in the number of GABAARs at inhibitory synapses on somata and axon initial segments of hippocampal granule cells. The increased density of GABAA Rs was accompanied by an enlargement of synaptic area and presynaptic boutons (Nusser et al., 1998). Although our knowledge of the mechanisms of activity-dependent structural plasticity of inhibitory synapses is still limited, this study suggests that, like excitatory synapses, inhibitory synaptic plasticity might not only depend on changes in the biophysical properties of the channels, but might also involve a structural reorganization of its inhibitory postsynaptic density, by altering the number of channels and the necessary structural components. Recent research shows that gephyrin forms dynamic domains within the inhibitory PSD that change size, form and localization in minutes (Specht et al., 2013). In the the hexagonal bidimensional lattice formed by gephyrin at the postsynaptic membrane, every single gephyrin interacts with GABA receptors in a one to one ratio approximately (Specht et al., 2013). Increasing synaptic gephyrin would then create new slots for allocating inhibitory receptors. Interestingly, functional and structural plasticity of excitatory synapses share a complete set of molecular machinery that allows coordinated changes in synaptic strength and architecture (Lüscher et al., 2000). Increasing our knowledge of the molecular mechanisms that regulate clustering of gephyrin and trafficking of GABAA R at inhibitory synapses will surely increase our understanding of molecular mechanisms that regulate structural plasticity of GABAergic synapses.
Activity Dependent Regulation of Gephyrin Function
One of the most intensively studied molecule involved in the inhibitory synapse function is gephyrin, the major scaffold protein of the inhibitory synapse. Crystallographic techniques show that gephyrin oligomerizes via G-domain trimerization and E-domain dimerization forming an hexagonal submembrane lattice (Xiang et al., 2001). Gephyrin undergoes several post-translational modifications that affect its clustering and the interactions with GABAARs and a variety of regulatory proteins, signaling complexes and cytoskeleton (Tyagarajan and Fritschy, 2014). Dejanovic et al. (2014) have recently identified gephyrin palmitoylation as a mechanism of gephyrin synaptic targeting. As for PSD95 in excitatory synapses, gephyrin palmitoylation is essential for the interaction with cell membrane and therefore facilitates its postsynaptic clustering at GABAergic synapses. Gephyrin palmitoylation is dependent of synaptic activity since the application of bicuculline that blocks GABAA Rs and increases network activity reduces gephyrin palmitoylation. In contrast, GABA application increases gephyrin palmitoylation (Dejanovic et al., 2014). Additionally, synaptic activity may alter gephyrin clustering via modulation of nitric oxide (NO) synthesis by neuronal NO synthase (Dejanovic and Schwarz, 2014).
Phosphorylation is a critical regulator of gephyrin function (Moss et al., 1995; Zacchi et al., 2014). Some of the signaling cascades that modulate gephyrin phosphorylation and GABAergic structural plasticity could represent a cross-road between excitatory and inhibitory synapse function, thus providing a mechanism of extreme importance to maintain the homeostasis of synaptic networks. Clear examples for such common molecular players are Glycogen Synthase Kinase 3 (GSK3β) and extracellular signal-regulated protein kinase/mitogen-activated protein kinase (ERK). At excitatory synapses, GSK3β phosphorylates PSD95 and modulates excitatory synaptic plasticity in an N-Methyl-D-Aspartate (NMDA) receptor-dependent manner by inducing α-Amino-3-hydroxy- 5-Methyl-4-isoxazolepropionic acid (AMPA) receptor internalization and thus LTD (Nelson et al., 2013) while ERKs boost excitatory synaptic and structural plasticity at different levels (Zhu et al., 2002; Patterson et al., 2010). At inhibitory synapses, gephyrin phosphorylation by both GSK3β and ERK1 at at Ser 270 and Ser 268 respectively modulates the structure and function of GABAergic synapses by altering the number and size of gephyrin clusters (Tyagarajan et al., 2011b, 2013). Glycogen Synthase Kinase 3-dependent phosphorylation decreases gephyrin cluster size, whereas phosphorylation by ERK decreases size and density of postsynaptic gephyrin clusters and is critical for the Ca2+-dependent cysteine protease (calpain-1) degradation of gephyrin. Phosphorylation of gephyrin by GSK3β and ERK1 is accompanied by parallel decrease in GABAergic mIPSCs. ERK and GSK3β activity are tightly regulated by neuronal activity making gephyrin phosphorylation a key mechanism for the coordination of structural remodeling of GABAergic synapses and network activity levels.
Ca2+/Calmodulin Dependent Protein Kinase II Regulates GABAergic Synapse Function and Structure
Trafficking and stability of GABAA Rs can be modulated by direct phosphorylation of the channel subunits (Vithlani et al., 2011). Several kinases target GABAA R such as Protein Kinase A (Brandon et al., 2003; Jovanovic et al., 2004), Protein Kinase C (Brandon et al., 2002) and Ca2+/calmodulin-dependent protein kinase II (CaMKII; McDonald and Moss, 1997). CaMKII directly phosphorylates α1, β2, β3, and γ2 subunits (McDonald and Moss, 1997; Churn et al., 2002; Houston et al., 2009; Petrini et al., 2014). Activation of the NMDA subtype of glutamate receptors has been linked to potentiation of GABAA R-mediated currents in different brain regions through activation of CaMKII (Marsden et al., 2007; Petrini et al., 2014). In addition, CaMKII translocates from dendritic spines to inhibitory synapses upon weak chemical stimulation by NMDA and promotes the insertion of GABAA Rs to inhibitory synapses and enhancement of inhibitory transmission (Marsden et al., 2010). Are these functional changes accompanied by structural remodeling? A recent published work by Petrini et al. (2014) found that the chemical inhibitory long term potentiation (iLTP) triggered by NMDA application (up to 30 min) requires CaMKII-dependent phosphorylation of GABAA R subunit β3 at serine 383 that in turn promotes the synaptic recruitment of gephyrin from extrasynaptic sites. The increase of gephyrin at synapses is not explained by de novo synthesis, but rather by a regulation of the mechanisms that control the redistribution of gephyrin. In turn, using single-particle tracking of quantum dots labeled GABAARs, they found that gephyrin recruitment at the synapses stabilizes GABAA Rs. The recruitment of gephyrin by an activity dependent phosphorylation of GABAA R subunit represents a way in which functional changes are coordinated with structural changes.
Cell Adhesion Molecules and Activity Dependent Regulation of GABAergic Synapse
Gephyrin clustering at GABAergic synapses requires its interaction with a neuron specific Guanine Nucleotide Exchange Factor (GEF) Collybistin (CB; Kins et al., 2000; Harvey et al., 2004; Papadopoulos et al., 2007). CB is expressed specifically in neurons and activates cell division control protein 42 homologue (Cdc42; Xiang et al., 2006) and the small Rho-like GTPase TC10 (Mayer et al., 2013) that in turn regulate cluster formation and the aggregation GABAA Rs (Poulopoulos et al., 2009; Tyagarajan et al., 2011a). Inhibitory synapse formation, maturation, maintenance and function are also regulated by synaptic cell adhesion molecules (CAMs; Yamagata et al., 2003; Gerrow and El-Husseini, 2006). At inhibitory synapses, one of the most prominent CAMs belongs to the Neuroligin family (Craig and Kang, 2007; Südhof, 2008). Neuroligin 2 (NL2) is specifically localized at inhibitory synapses (Varoqueaux et al., 2004) where it modulates their formation, maturation and function (Graf et al., 2004; Chih et al., 2005; Chubykin et al., 2007). NL2 deficient mice lack postsynaptic specialization at perisomatic inhibitory synapses (Poulopoulos et al., 2009) and show decreased inhibitory synaptic transmission (Chubykin et al., 2007). Through a conserved cytoplasmatic domain, NL2 binds gephyrin (Poulopoulos et al., 2009) while an extracelullar motif mediates NL2 trans-synaptic interaction with presynaptic Neurexins (NRXs) boosting inhibitory presynaptic axonal differentiation during development (Chih et al., 2005). The interaction with the scaffold protein gephyrin brings NL2 close to GABA receptors and other gephyrin binding proteins (Fritschy et al., 2012). NRXs are CAMs that have two isoforms, α-NRXs and β-NRXs (Südhof, 2008). α-NRXs are expressed primarily at GABAergic synapses, whereas β-NRXs are localized at both excitatory and inhibitory synapses (Chih et al., 2005) where they form a dense transynaptic assembly (Tanaka et al., 2012). Interestingly, β-NRX1 has high turn over rate in presynaptic membranes and is stabilized by neuronal activity and GABA release (Fu and Huang, 2010). In addition, NRXs directly interact with GABAA Rs, thus modulating GABAergic transmission in a NL independent fashion (Zhang et al., 2010).
GABA dependent stabilization of presynaptic NRXs may represent a mechanism for activity dependent GABAergic synapse remodeling. NRXs stabilized by local GABA release (Fu and Huang, 2010) drive the clustering of NL2 postsynaptically. Neuroligin 2 interaction with gephyrin brings CB close to NL2, where CB/NL2 interaction releases SH3 domain and activates CB (Poulopoulos et al., 2009). Interaction with GABAAR α2 subunit may also activate CB (Tretter et al., 2008). Activated CB leads gephyrin and GABAA Rs to the postsynaptic membrane and further stabilizes them at the synapses. Neuroligin 2 initiates clustering of other molecules essential for synapse function (Lévi et al., 2002; Woo et al., 2013; Pribiag et al., 2014) strengthening in this way synaptic adhesion. Finally, NL2 intracellular interactions with γ2 subunit of GABAAR stabilize both molecules at inhibitory synapses (Dong et al., 2007). Thus, GABA unchained downstream signaling may play a role in coordinating the formation and remodeling of the pre- and postsynapses (Fritschy et al., 2008; Tretter et al., 2012).
Functional Role and Specificity
The study of structural plasticity of glutamatergic synapses has focused on the relationship between morphological parameters of dendritic spines and physiological properties of its excitatory synapse (Nimchinsky et al., 2002; Bourne and Harris, 2008; Kasai et al., 2010). Recent studies have shown that although inhibitory post-synapses do not have such morphological fingerprint, fluorescently tagged gephyrin can be used to visualize inhibitory synapses and track dynamic changes (Dobie and Craig, 2011; Chen et al., 2012; van Versendaal et al., 2012). Indeed, virtually all gephyrin clusters detected at the optical level using fluorescent microscopy had a correlate with a GABAergic synapse detected at the ultrastructural level using EM (Chen et al., 2012; van Versendaal et al., 2012). Although the functional correlation between optically measurable parameters such as size and intensity of gephyrin clusters and inhibitory synapse function is currently unknown, EM studies have shown that increased synaptic strength in inhibitory synapses produces a coordinated insertion of GABAARs and enlargement of the PSD (Nusser et al., 1998). Whether such structural rearrangement can be visualized at the optical level using fluorescently-tagged gephyrin or other inhibitory synapse fluorescent markers requires further research that will provide invaluable information about structure-function relationship in inhibitory synapses.
During brain development, when structural dynamism of GABAergic synapses is high, the functional consequences of inhibitory synaptic rearrangements are determined by the depolarizing effects that activation of GABAARs has on postsynaptic target cells (Cherubini et al., 1991). In adulthood, however, coordination of the amount of excitatory and inhibitory inputs becomes an essential function of structural plasticity of GABAergic synapses. Both perisomatic (Nusser et al., 1998; Lushnikova et al., 2011) and dendritic inhibitory synapses (Knott et al., 2006; Jasinska et al., 2010; Chen et al., 2012; van Versendaal et al., 2012) show high levels of structural plasticity, suggesting that excitation/inhibition balance could be controled independently in different subcellular compartments. Indeed, recent reports show that active excitatory synaptic inputs are not randomly distributed along dendritic arbors in principal cells neurons (Kleindienst et al., 2011; Makino and Malinow, 2011; Takahashi et al., 2012). The ability of inhibitory synapses to target specific subcellular compartments could allow local control of such clustered inputs. In addition, an homeostatic role is a reasonable interpretation for studies showing simultaneous rearrangement of perisomatic inhibitory and excitatory synapses in response to patterns of activity known to induce potentiation of glutamatergic synaptic transmission (Nusser et al., 1998; Bourne and Harris, 2011; Lushnikova et al., 2011). In the barrel cortex, increases and decreases in whisker-induced neuronal activity are directly related to the formation or elimination of GABAergic spine synapses respectively (Micheva and Beaulieu, 1995; Knott et al., 2002). This form of GABAergic structural plasticity may participate in the homeostatic adjustment of circuit activity levels after long-term changes in somatosensory evoked synaptic activity. It is increasingly clear that patterns of synaptic activity that induce structural rearrangements of inhibitory circuits also induce plasticity (functional and/or structural) of excitatory synapses. Functional plasticity of GABAergic synapses has been shown to modulate excitatory neurotransmission and neuronal output (Saraga et al., 2008; Wang and Maffei, 2014). This could reflect network’s requirement for a coordinated plasticity of inhibitory and excitatory inputs to maintain homeostasis, keep excitation/inhibition balance and prevent abnormal levels of activity.
One of the main components of LTP in the hippocampus is the increase in the efficacy of coupling between excitatory postsynaptic depolarization and spiking activity (Linden, 1999; Lu et al., 2000). Since perisomatic inhibition plays an essential role in determining the time window for spike generation (Pouille and Scanziani, 2001), increased inhibition through structural rearrangements of perisomatic synapses after plasticity inducing network activity may help in ensuring precise temporal synaptic integration. Addition or removal of GABAergic spine synapses in close proximity glutamatergic synapses in dendritic spines of cortical neurons allow the control of excitatory inputs through shunting inhibition (Maccaferri, 2005) and modulation of calcium signaling by near coincident activation of the GABAergic and excitatory inputs impinging on a particular spine (Chiu et al., 2013; Hayama et al., 2013) and it has the potential to affect processes of local, branch or dendritic segment specific computation (Pérez-Garci et al., 2006). In addition, dendrite inhibition can be very effective in damping neuronal activity by long-range shunting of excitatory inputs close to the soma. This means that plasticity of a few strategically located synapses could have important consequences for determining neuronal output (Gidon and Segev, 2012). In addition to its homeostatic role, inhibitory synapse structural plasticity may refine the synaptic basis of computational operations performed by hippocampal and cortical networks.
Experience shapes the formation and function of neuronal circuits during critical periods in early life (Berardi et al., 2000; Hensch, 2004). It seems increasingly clear that during these sensitive periods, special plasticity mechanisms that have a much smaller prevalence during adulthood are potentiated. In the visual cortex, where critical period has been extensively studied, the transition in and out of this critical period has been demonstrated to be under a strict control of the GABAergic system with a prominent role of perisomatic inhibition (Hensch, 2005). Changes in the rules of functional GABAergic synapse plasticity in cortical synapses that take place after the developmental switch in GABA synaptic signal polarity may play a prominent role in the transition into these periods of enhanced plasticity (Lefort et al., 2013). In addition, developmental and activity driven structural plasticity of GABAergic synapses may have important consequences for critical periods. In line with this, several studies have demonstrated that cortical sensory areas undergo an early disinhibition upon sensory deprivation (Micheva and Beaulieu, 1995; Keck et al., 2011; Chen et al., 2012; van Versendaal et al., 2012). It is likely that GABAergic synapse elimination is fundamental in allowing subsequent plastic changes in the cortex that may affect glutamatergic transmission. It is tempting to speculate that the interaction between GABAergic and glutamatergic synapses could involve a bidirectional crosstalk that include signals from the excitatory synapse that regulate GABAergic synapse strength and persistence (Marsden et al., 2010). Perineuronal nets (PNNs) are well organized structures formed by extracellular matrix molecules condensed around cell body and proximal dendrites of some types of neurons (Kwok et al., 2011). PNNs are formed by aggregation of heavily glycosilated proteins (proteoglycans) and, in the cortex, are preferentially associated with GABAergic cells (Morris and Henderson, 2000). Interestingly, PNNs are emerging as key structural regulators of INs plasticity (Wang and Fawcett, 2012). PNNs expression restricts neuronal plasticity by stabilizing synaptic connections and inhibiting activity dependent changes in neurotransmission (Berardi et al., 2004). Interestingly, disruption of PNNs con reactivate plasticity in some brain areas (Gogolla et al., 2009) and allows the reactivation of critical periods in the adult brain (Pizzorusso et al., 2002).
Changes in GABAergic transmission are essential for certain forms of memory and learning (Cui et al., 2008) but the information about the involvement of GABAergic synapse structural plasticity in network mechanisms of memory is still scarce. Some experiments have shown that learning-related behavior can drive long-lasting inhibitory synapse formation and elimination (Jasinska et al., 2010; Bloodgood et al., 2013) that may be responsible for durable changes in network connectivity underlying learning and memory. Behaviorally induced structural plasticity does not involve the general population of inhibitory synapses but differentially affects synapses impinging onto dendrites, spines or perisomatic compartment (Bloodgood et al., 2013). As a consequence of this differential regulation, experience may change the spread of inhibitory input among the different compartments and may affect information processing (Miles et al., 1996). Interestingly, selective deletion of Npas4 gene that codes for an activity regulated transcription factor responsible for differential remodeling of hippocampal dendritic and somatic GABA synapses in response to spatial exploration (Bloodgood et al., 2013), blocks hippocampal-dependent contextual learning (Ramamoorthi et al., 2011). Although Npas4 has been shown to control numerous genetic pathways that regulate both excitatory and inhibitory synapse function (Spiegel et al., 2014), its role in contextual memory formation could be at least in part mediated by an activity dependent remodeling of GABAergic synapses.
Conclusions and Future Directions
In this review we have emphasized the role of synaptic activity in the remodeling of GABAergic synapse structure and discussed the possible roles of structural plasticity in sensory processing and memory formation. Although there is substantial experimental evidence of activity driven structural plasticity of GABAergic synapses, we have only partial knowledge of the implications of this type of plasticity for the function of inhibitory synapses and circuits and the molecular mechanisms that regulate different aspects of GABAergic synapse remodeling. In particular, several questions remain open for future investigation: (i) what are the structural determinants of GABAergic synapse function? (ii) what is the driving force for remodeling of GABA synapses, GABAergic or glutamatergic neurotransmission (or both)? (iii) how is the persistence of GABAergic synapses controled? (iv) how is structural plasticity of GABAergic and Glutamatergic synapses coordinated? (v) why spine inhibitory synapses are more dynamic than shaft inhibitory synapses? Addressing all these questions will surely advance our knowledge of the brain mechanisms of plasticity and define the precise roles of inhibitory synapse remodeling in the neuronal adaptation to experience, and in particular, for learning and memory.
Conflict of Interest Statement
The authors declare that the research was conducted in the absence of any commercial or financial relationships that could be construed as a potential conflict of interest.
Acknowledgments
The authors would like to thank Dominique Muller for intellectual and financial support and Irina Nikonenko for providing the EM image and critically reading the manuscript. Work in Dominique Muller’s lab is financed by SNF grant Sinergia and grant 310030B-144080.
References
Ascoli, G. A., Alonso-Nanclares, L., Anderson, S. A., Barrionuevo, G., Benavides-Piccione, R., Burkhalter, A., et al. (2008). Petilla terminology: nomenclature of features of GABAergic interneurons of the cerebral cortex. Nat. Rev. Neurosci. 9, 557–568. doi: 10.1038/nrn2402
Bannai, H., Lévi, S., Schweizer, C., Inoue, T., Launey, T., Racine, V., et al. (2009). Activity-dependent tuning of inhibitory neurotransmission based on GABAAR diffusion dynamics. Neuron 62, 670–682. doi: 10.1016/j.neuron.2009.04.023
Pubmed Abstract | Pubmed Full Text | CrossRef Full Text | Google Scholar
Bartolini, G., Ciceri, G., and Marín, O. (2013). Integration of GABAergic interneurons into cortical cell assemblies: lessons from embryos and adults. Neuron 79, 849–864. doi: 10.1016/j.neuron.2013.08.014
Pubmed Abstract | Pubmed Full Text | CrossRef Full Text | Google Scholar
Berardi, N., Pizzorusso, T., and Maffei, L. (2000). Critical periods during sensory development. Curr. Opin. Neurobiol. 10, 138–145. doi: 10.1016/s0959-4388(99)00047-1
Pubmed Abstract | Pubmed Full Text | CrossRef Full Text | Google Scholar
Berardi, N., Pizzorusso, T., and Maffei, L. (2004). Extracellular matrix and visual cortical plasticity: freeing the synapse. Neuron 44, 905–908. doi: 10.1016/j.neuron.2004.12.008
Pubmed Abstract | Pubmed Full Text | CrossRef Full Text | Google Scholar
Berghuis, P., Dobszay, M. B., Sousa, K. M., Schulte, G., Mager, P. P., Härtig, W., et al. (2004). Brain-derived neurotrophic factor controls functional differentiation and microcircuit formation of selectively isolated fast-spiking GABAergic interneurons. Eur. J. Neurosci. 20, 1290–1306. doi: 10.1111/j.1460-9568.2004.03561.x
Pubmed Abstract | Pubmed Full Text | CrossRef Full Text | Google Scholar
Bloodgood, B. L., Sharma, N., Browne, H. A., Trepman, A. Z., and Greenberg, M. E. (2013). The activity-dependent transcription factor NPAS4 regulates domain-specific inhibition. Nature 503, 121–125. doi: 10.1038/nature12743
Pubmed Abstract | Pubmed Full Text | CrossRef Full Text | Google Scholar
Bourne, J. N., and Harris, K. M. (2008). Balancing structure and function at hippocampal dendritic spines. Annu. Rev. Neurosci. 31, 47–67. doi: 10.1146/annurev.neuro.31.060407.125646
Pubmed Abstract | Pubmed Full Text | CrossRef Full Text | Google Scholar
Bourne, J. N., and Harris, K. M. (2011). Coordination of size and number of excitatory and inhibitory synapses results in a balanced structural plasticity along mature hippocampal CA1 dendrites during LTP. Hippocampus 21, 354–373. doi: 10.1002/hipo.20768
Pubmed Abstract | Pubmed Full Text | CrossRef Full Text | Google Scholar
Brandon, N. J., Jovanovic, J. N., Colledge, M., Kittler, J. T., Brandon, J. M., Scott, J. D., et al. (2003). A-kinase anchoring protein 79/150 facilitates the phosphorylation of GABA(A) receptors by cAMP-dependent protein kinase via selective interaction with receptor beta subunits. Mol. Cell. Neurosci. 22, 87–97. doi: 10.1016/s1044-7431(02)00017-9
Pubmed Abstract | Pubmed Full Text | CrossRef Full Text | Google Scholar
Brandon, N. J., Jovanovic, J. N., Smart, T. G., and Moss, S. J. (2002). Receptor for activated C kinase-1 facilitates protein kinase C-dependent phosphorylation and functional modulation of GABA(A) receptors with the activation of G-protein-coupled receptors. J. Neurosci. 22, 6353–6361.
Buzsáki, G., and Wang, X. J. (2012). Mechanisms of gamma oscillations. Annu. Rev. Neurosci. 35, 203–225. doi: 10.1146/annurev-neuro-062111-150444
Pubmed Abstract | Pubmed Full Text | CrossRef Full Text | Google Scholar
Caroni, P., Donato, F., and Muller, D. (2012). Structural plasticity upon learning: regulation and functions. Nat. Rev. Neurosci. 13, 478–490. doi: 10.1038/nrn3258
Pubmed Abstract | Pubmed Full Text | CrossRef Full Text | Google Scholar
Castillo, P. E., Chiu, C. Q., and Carroll, R. C. (2011). Long-term plasticity at inhibitory synapses. Curr. Opin. Neurobiol. 21, 328–338. doi: 10.1016/j.conb.2011.01.006
Pubmed Abstract | Pubmed Full Text | CrossRef Full Text | Google Scholar
Chattopadhyaya, B., Di Cristo, G., Higashiyama, H., Knott, G. W., Kuhlman, S. J., Welker, E., et al. (2004). Experience and activity-dependent maturation of perisomatic GABAergic innervation in primary visual cortex during a postnatal critical period. J. Neurosci. 24, 9598–9611. doi: 10.1523/jneurosci.1851-04.2004
Pubmed Abstract | Pubmed Full Text | CrossRef Full Text | Google Scholar
Chattopadhyaya, B., Di Cristo, G., Wu, C. Z., Knott, G., Kuhlman, S., Fu, Y., et al. (2007). GAD67-mediated GABA synthesis and signaling regulate inhibitory synaptic innervation in the visual cortex. Neuron 54, 889–903. doi: 10.1016/j.neuron.2007.05.015
Pubmed Abstract | Pubmed Full Text | CrossRef Full Text | Google Scholar
Chen, J. L., Villa, K. L., Cha, J. W., So, P. T., Kubota, Y., and Nedivi, E. (2012). Clustered dynamics of inhibitory synapses and dendritic spines in the adult neocortex. Neuron 74, 361–373. doi: 10.1016/j.neuron.2012.02.030
Pubmed Abstract | Pubmed Full Text | CrossRef Full Text | Google Scholar
Cherubini, E., Gaiarsa, J. L., and Ben-Ari, Y. (1991). GABA: an excitatory transmitter in early postnatal life. Trends Neurosci. 14, 515–519. doi: 10.1016/0166-2236(91)90003-d
Pubmed Abstract | Pubmed Full Text | CrossRef Full Text | Google Scholar
Chevaleyre, V., and Castillo, P. E. (2003). Heterosynaptic LTD of hippocampal GABAergic synapses: a novel role of endocannabinoids in regulating excitability. Neuron 38, 461–472. doi: 10.1016/s0896-6273(03)00235-6
Pubmed Abstract | Pubmed Full Text | CrossRef Full Text | Google Scholar
Chevaleyre, V., Takahashi, K. A., and Castillo, P. E. (2006). Endocannabinoid-mediated synaptic plasticity in the CNS. Annu. Rev. Neurosci. 29, 37–76. doi: 10.1146/annurev.neuro.29.051605.112834
Pubmed Abstract | Pubmed Full Text | CrossRef Full Text | Google Scholar
Chih, B., Engelman, H., and Scheiffele, P. (2005). Control of excitatory and inhibitory synapse formation by neuroligins. Science 307, 1324–1328. doi: 10.1126/science.1107470
Pubmed Abstract | Pubmed Full Text | CrossRef Full Text | Google Scholar
Chiu, C. Q., Lur, G., Morse, T. M., Carnevale, N. T., Ellis-Davies, G. C., and Higley, M. J. (2013). Compartmentalization of GABAergic inhibition by dendritic spines. Science 340, 759–762. doi: 10.1126/science.1234274
Pubmed Abstract | Pubmed Full Text | CrossRef Full Text | Google Scholar
Chubykin, A. A., Atasoy, D., Etherton, M. R., Brose, N., Kavalali, E. T., Gibson, J. R., et al. (2007). Activity-dependent validation of excitatory versus inhibitory synapses by neuroligin-1 versus neuroligin-2. Neuron 54, 919–931. doi: 10.1016/j.neuron.2007.05.029
Pubmed Abstract | Pubmed Full Text | CrossRef Full Text | Google Scholar
Churn, S. B., Rana, A., Lee, K., Parsons, J. T., De Blas, A., and Delorenzo, R. J. (2002). Calcium/calmodulin-dependent kinase II phosphorylation of the GABAA receptor alpha1 subunit modulates benzodiazepine binding. J. Neurochem. 82, 1065–1076. doi: 10.1046/j.1471-4159.2002.01032.x
Pubmed Abstract | Pubmed Full Text | CrossRef Full Text | Google Scholar
Colonnier, M. (1968). Synaptic patterns on different cell types in the different laminae of the cat visual cortex. An electron microscope study. Brain Res. 9, 268–287. doi: 10.1016/0006-8993(68)90234-5
Pubmed Abstract | Pubmed Full Text | CrossRef Full Text | Google Scholar
Craig, A. M., and Kang, Y. (2007). Neurexin-neuroligin signaling in synapse development. Curr. Opin. Neurobiol. 17, 43–52. doi: 10.1016/j.conb.2007.01.011
Pubmed Abstract | Pubmed Full Text | CrossRef Full Text | Google Scholar
Cui, Y., Costa, R. M., Murphy, G. G., Elgersma, Y., Zhu, Y., Gutmann, D. H., et al. (2008). Neurofibromin regulation of ERK signaling modulates GABA release and learning. Cell 135, 549–560. doi: 10.1016/j.cell.2008.09.060
Pubmed Abstract | Pubmed Full Text | CrossRef Full Text | Google Scholar
Dejanovic, B., and Schwarz, G. (2014). Neuronal nitric oxide synthase-dependent S-nitrosylation of gephyrin regulates gephyrin clustering at GABAergic synapses. J. Neurosci. 34, 7763–7768. doi: 10.1523/JNEUROSCI.0531-14.2014
Pubmed Abstract | Pubmed Full Text | CrossRef Full Text | Google Scholar
Dejanovic, B., Semtner, M., Ebert, S., Lamkemeyer, T., Neuser, F., Lüscher, B., et al. (2014). Palmitoylation of gephyrin controls receptor clustering and plasticity of GABAergic synapses. PLoS Biol. 12:e1001908. doi: 10.1371/journal.pbio.1001908
Pubmed Abstract | Pubmed Full Text | CrossRef Full Text | Google Scholar
Dobie, F. A., and Craig, A. M. (2011). Inhibitory synapse dynamics: coordinated presynaptic and postsynaptic mobility and the major contribution of recycled vesicles to new synapse formation. J. Neurosci. 31, 10481–10493. doi: 10.1523/JNEUROSCI.6023-10.2011
Pubmed Abstract | Pubmed Full Text | CrossRef Full Text | Google Scholar
Doischer, D., Hosp, J. A., Yanagawa, Y., Obata, K., Jonas, P., Vida, I., et al. (2008). Postnatal differentiation of basket cells from slow to fast signaling devices. J. Neurosci. 28, 12956–12968. doi: 10.1523/JNEUROSCI.2890-08.2008
Pubmed Abstract | Pubmed Full Text | CrossRef Full Text | Google Scholar
Dong, N., Qi, J., and Chen, G. (2007). Molecular reconstitution of functional GABAergic synapses with expression of neuroligin-2 and GABAA receptors. Mol. Cell. Neurosci. 35, 14–23. doi: 10.1016/j.mcn.2007.01.013
Pubmed Abstract | Pubmed Full Text | CrossRef Full Text | Google Scholar
Flavell, S. W., and Greenberg, M. E. (2008). Signaling mechanisms linking neuronal activity to gene expression and plasticity of the nervous system. Annu. Rev. Neurosci. 31, 563–590. doi: 10.1146/annurev.neuro.31.060407.125631
Pubmed Abstract | Pubmed Full Text | CrossRef Full Text | Google Scholar
Freund, T. F., and Katona, I. (2007). Perisomatic inhibition. Neuron 56, 33–42. doi: 10.1016/j.neuron.2007.09.012
Pubmed Abstract | Pubmed Full Text | CrossRef Full Text | Google Scholar
Fritschy, J. M., Harvey, R. J., and Schwarz, G. (2008). Gephyrin: where do we stand, where do we go? Trends Neurosci. 31, 257–264. doi: 10.1016/j.tins.2008.02.006
Pubmed Abstract | Pubmed Full Text | CrossRef Full Text | Google Scholar
Fritschy, J. M., Panzanelli, P., and Tyagarajan, S. K. (2012). Molecular and functional heterogeneity of GABAergic synapses. Cell. Mol. Life Sci. 69, 2485–2499. doi: 10.1007/s00018-012-0926-4
Pubmed Abstract | Pubmed Full Text | CrossRef Full Text | Google Scholar
Fu, Y., and Huang, Z. J. (2010). Differential dynamics and activity-dependent regulation of alpha- and beta-neurexins at developing GABAergic synapses. Proc. Natl. Acad. Sci. U S A 107, 22699–22704. doi: 10.1073/pnas.1011233108
Pubmed Abstract | Pubmed Full Text | CrossRef Full Text | Google Scholar
Gaiarsa, J. L., Caillard, O., and Ben-Ari, Y. (2002). Long-term plasticity at GABAergic and glycinergic synapses: mechanisms and functional significance. Trends Neurosci. 25, 564–570. doi: 10.1016/s0166-2236(02)02269-5
Pubmed Abstract | Pubmed Full Text | CrossRef Full Text | Google Scholar
Gerrow, K., and El-Husseini, A. (2006). Cell adhesion molecules at the synapse. Front. Biosci. 11, 2400–2419. doi: 10.2741/1978
Gidon, A., and Segev, I. (2012). Principles governing the operation of synaptic inhibition in dendrites. Neuron 75, 330–341. doi: 10.1016/j.neuron.2012.05.015
Pubmed Abstract | Pubmed Full Text | CrossRef Full Text | Google Scholar
Gogolla, N., Caroni, P., Lüthi, A., and Herry, C. (2009). Perineuronal nets protect fear memories from erasure. Science 325, 1258–1261. doi: 10.1126/science.1174146
Pubmed Abstract | Pubmed Full Text | CrossRef Full Text | Google Scholar
Graf, E. R., Zhang, X., Jin, S. X., Linhoff, M. W., and Craig, A. M. (2004). Neurexins induce differentiation of GABA and glutamate postsynaptic specializations via neuroligins. Cell 119, 1013–1026. doi: 10.1016/j.cell.2004.11.035
Pubmed Abstract | Pubmed Full Text | CrossRef Full Text | Google Scholar
Gray, E. G. (1959). Axo-somatic and axo-dendritic synapses of the cerebral cortex: an electron microscope study. J. Anat. 93, 420–433.
Haas, J. S., Nowotny, T., and Abarbanel, H. D. (2006). Spike-timing-dependent plasticity of inhibitory synapses in the entorhinal cortex. J. Neurophysiol. 96, 3305–3313. doi: 10.1152/jn.00551.2006
Pubmed Abstract | Pubmed Full Text | CrossRef Full Text | Google Scholar
Hartman, K. N., Pal, S. K., Burrone, J., and Murthy, V. N. (2006). Activity-dependent regulation of inhibitory synaptic transmission in hippocampal neurons. Nat. Neurosci. 9, 642–649. doi: 10.1038/nn1677
Pubmed Abstract | Pubmed Full Text | CrossRef Full Text | Google Scholar
Harvey, K., Duguid, I. C., Alldred, M. J., Beatty, S. E., Ward, H., Keep, N. H., et al. (2004). The GDP-GTP exchange factor collybistin: an essential determinant of neuronal gephyrin clustering. J. Neurosci. 24, 5816–5826. doi: 10.1523/jneurosci.1184-04.2004
Pubmed Abstract | Pubmed Full Text | CrossRef Full Text | Google Scholar
Hayama, T., Noguchi, J., Watanabe, S., Takahashi, N., Hayashi-Takagi, A., Ellis-Davies, G. C., et al. (2013). GABA promotes the competitive selection of dendritic spines by controlling local Ca2+ signaling. Nat. Neurosci. 16, 1409–1416. doi: 10.1038/nn.3496
Pubmed Abstract | Pubmed Full Text | CrossRef Full Text | Google Scholar
Hébert, J. M., and Fishell, G. (2008). The genetics of early telencephalon patterning: some assembly required. Nat. Rev. Neurosci. 9, 678–685. doi: 10.1038/nrn2463
Pubmed Abstract | Pubmed Full Text | CrossRef Full Text | Google Scholar
Hensch, T. K. (2004). Critical period regulation. Annu. Rev. Neurosci. 27, 549–579. doi: 10.1146/annurev.neuro.27.070203.144327
Pubmed Abstract | Pubmed Full Text | CrossRef Full Text | Google Scholar
Hensch, T. K. (2005). Critical period plasticity in local cortical circuits. Nat. Rev. Neurosci. 6, 877–888. doi: 10.1038/nrn1787
Pubmed Abstract | Pubmed Full Text | CrossRef Full Text | Google Scholar
Hofer, S. B., Mrsic-Flogel, T. D., Bonhoeffer, T., and Hübener, M. (2009). Experience leaves a lasting structural trace in cortical circuits. Nature 457, 313–317. doi: 10.1038/nature07487
Pubmed Abstract | Pubmed Full Text | CrossRef Full Text | Google Scholar
Holmgren, C. D., and Zilberter, Y. (2001). Coincident spiking activity induces long-term changes in inhibition of neocortical pyramidal cells. J. Neurosci. 21, 8270–8277.
Holtmaat, A., and Svoboda, K. (2009). Experience-dependent structural synaptic plasticity in the mammalian brain. Nat. Rev. Neurosci. 10, 647–658. doi: 10.1038/nrn2699
Pubmed Abstract | Pubmed Full Text | CrossRef Full Text | Google Scholar
Holtmaat, A. J., Trachtenberg, J. T., Wilbrecht, L., Shepherd, G. M., Zhang, X., Knott, G. W., et al. (2005). Transient and persistent dendritic spines in the neocortex in vivo. Neuron 45, 279–291. doi: 10.1016/j.neuron.2005.01.003
Pubmed Abstract | Pubmed Full Text | CrossRef Full Text | Google Scholar
Honkura, N., Matsuzaki, M., Noguchi, J., Ellis-Davies, G. C., and Kasai, H. (2008). The subspine organization of actin fibers regulates the structure and plasticity of dendritic spines. Neuron 57, 719–729. doi: 10.1016/j.neuron.2008.01.013
Pubmed Abstract | Pubmed Full Text | CrossRef Full Text | Google Scholar
Houston, C. M., He, Q., and Smart, T. G. (2009). CaMKII phosphorylation of the GABA(A) receptor: receptor subtype- and synapse-specific modulation. J. Physiol. 587, 2115–2125. doi: 10.1113/jphysiol.2009.171603
Pubmed Abstract | Pubmed Full Text | CrossRef Full Text | Google Scholar
Huang, Z. J. (2009). Activity-dependent development of inhibitory synapses and innervation pattern: role of GABA signalling and beyond. J. Physiol. 587, 1881–1888. doi: 10.1113/jphysiol.2008.168211
Pubmed Abstract | Pubmed Full Text | CrossRef Full Text | Google Scholar
Huang, Z. J., Kirkwood, A., Pizzorusso, T., Porciatti, V., Morales, B., Bear, M. F., et al. (1999). BDNF regulates the maturation of inhibition and the critical period of plasticity in mouse visual cortex. Cell 98, 739–755. doi: 10.1016/s0092-8674(00)81509-3
Pubmed Abstract | Pubmed Full Text | CrossRef Full Text | Google Scholar
Jasinska, M., Siucinska, E., Cybulska-Klosowicz, A., Pyza, E., Furness, D. N., Kossut, M., et al. (2010). Rapid, learning-induced inhibitory synaptogenesis in murine barrel field. J. Neurosci. 30, 1176–1184. doi: 10.1523/JNEUROSCI.2970-09.2010
Pubmed Abstract | Pubmed Full Text | CrossRef Full Text | Google Scholar
Jiao, Y., Zhang, C., Yanagawa, Y., and Sun, Q. Q. (2006). Major effects of sensory experiences on the neocortical inhibitory circuits. J. Neurosci. 26, 8691–8701. doi: 10.1523/jneurosci.2478-06.2006
Pubmed Abstract | Pubmed Full Text | CrossRef Full Text | Google Scholar
Jovanovic, J. N., Thomas, P., Kittler, J. T., Smart, T. G., and Moss, S. J. (2004). Brain-derived neurotrophic factor modulates fast synaptic inhibition by regulating GABA(A) receptor phosphorylation, activity and cell-surface stability. J. Neurosci. 24, 522–530. doi: 10.1523/jneurosci.3606-03.2004
Pubmed Abstract | Pubmed Full Text | CrossRef Full Text | Google Scholar
Kang, J., Jiang, L., Goldman, S. A., and Nedergaard, M. (1998). Astrocyte-mediated potentiation of inhibitory synaptic transmission. Nat. Neurosci. 1, 683–692. doi: 10.1038/3684
Pubmed Abstract | Pubmed Full Text | CrossRef Full Text | Google Scholar
Kano, M., Ohno-Shosaku, T., Hashimotodani, Y., Uchigashima, M., and Watanabe, M. (2009). Endocannabinoid-mediated control of synaptic transmission. Physiol. Rev. 89, 309–380. doi: 10.1152/physrev.00019.2008
Pubmed Abstract | Pubmed Full Text | CrossRef Full Text | Google Scholar
Kasai, H., Fukuda, M., Watanabe, S., Hayashi-Takagi, A., and Noguchi, J. (2010). Structural dynamics of dendritic spines in memory and cognition. Trends Neurosci. 33, 121–129. doi: 10.1016/j.tins.2010.01.001
Pubmed Abstract | Pubmed Full Text | CrossRef Full Text | Google Scholar
Keck, T., Scheuss, V., Jacobsen, R. I., Wierenga, C. J., Eysel, U. T., Bonhoeffer, T., et al. (2011). Loss of sensory input causes rapid structural changes of inhibitory neurons in adult mouse visual cortex. Neuron 71, 869–882. doi: 10.1016/j.neuron.2011.06.034
Pubmed Abstract | Pubmed Full Text | CrossRef Full Text | Google Scholar
Kins, S., Betz, H., and Kirsch, J. (2000). Collybistin, a newly identified brain-specific GEF, induces submembrane clustering of gephyrin. Nat. Neurosci. 3, 22–29. doi: 10.1038/71096
Pubmed Abstract | Pubmed Full Text | CrossRef Full Text | Google Scholar
Kittler, J. T., and Moss, S. J. (2003). Modulation of GABAA receptor activity by phosphorylation and receptor trafficking: implications for the efficacy of synaptic inhibition. Curr. Opin. Neurobiol. 13, 341–347. doi: 10.1016/s0959-4388(03)00064-3
Pubmed Abstract | Pubmed Full Text | CrossRef Full Text | Google Scholar
Klausberger, T., and Somogyi, P. (2008). Neuronal diversity and temporal dynamics: the unity of hippocampal circuit operations. Science 321, 53–57. doi: 10.1126/science.1149381
Pubmed Abstract | Pubmed Full Text | CrossRef Full Text | Google Scholar
Kleindienst, T., Winnubst, J., Roth-Alpermann, C., Bonhoeffer, T., and Lohmann, C. (2011). Activity-dependent clustering of functional synaptic inputs on developing hippocampal dendrites. Neuron 72, 1012–1024. doi: 10.1016/j.neuron.2011.10.015
Pubmed Abstract | Pubmed Full Text | CrossRef Full Text | Google Scholar
Knott, G. W., Holtmaat, A., Wilbrecht, L., Welker, E., and Svoboda, K. (2006). Spine growth precedes synapse formation in the adult neocortex in vivo. Nat. Neurosci. 9, 1117–1124. doi: 10.1038/nn1747
Pubmed Abstract | Pubmed Full Text | CrossRef Full Text | Google Scholar
Knott, G. W., Quairiaux, C., Genoud, C., and Welker, E. (2002). Formation of dendritic spines with GABAergic synapses induced by whisker stimulation in adult mice. Neuron 34, 265–273. doi: 10.1016/s0896-6273(02)00663-3
Pubmed Abstract | Pubmed Full Text | CrossRef Full Text | Google Scholar
Kohara, K., Yasuda, H., Huang, Y., Adachi, N., Sohya, K., and Tsumoto, T. (2007). A local reduction in cortical GABAergic synapses after a loss of endogenous brain-derived neurotrophic factor, as revealed by single-cell gene knock-out method. J. Neurosci. 27, 7234–7244. doi: 10.1523/jneurosci.1943-07.2007
Pubmed Abstract | Pubmed Full Text | CrossRef Full Text | Google Scholar
Komatsu, Y. (1996). GABAB receptors, monoamine receptors and postsynaptic inositol trisphosphate-induced Ca2+ release are involved in the induction of long-term potentiation at visual cortical inhibitory synapses. J. Neurosci. 16, 6342–6352.
Komatsu, Y., and Yoshimura, Y. (2000). Activity-dependent maintenance of long-term potentiation at visual cortical inhibitory synapses. J. Neurosci. 20, 7539–7546.
Kullmann, D. M., Moreau, A. W., Bakiri, Y., and Nicholson, E. (2012). Plasticity of inhibition. Neuron 75, 951–962. doi: 10.1016/j.neuron.2012.07.030
Pubmed Abstract | Pubmed Full Text | CrossRef Full Text | Google Scholar
Kwok, J. C., Dick, G., Wang, D., and Fawcett, J. W. (2011). Extracellular matrix and perineuronal nets in CNS repair. Dev. Neurobiol. 71, 1073–1089. doi: 10.1002/dneu.20974
Pubmed Abstract | Pubmed Full Text | CrossRef Full Text | Google Scholar
Lefort, S., Gray, A. C., and Turrigiano, G. G. (2013). Long-term inhibitory plasticity in visual cortical layer 4 switches sign at the opening of the critical period. Proc. Natl. Acad. Sci. U S A 110, E4540–E4547. doi: 10.1073/pnas.1319571110
Pubmed Abstract | Pubmed Full Text | CrossRef Full Text | Google Scholar
Lévi, S., Grady, R. M., Henry, M. D., Campbell, K. P., Sanes, J. R., and Craig, A. M. (2002). Dystroglycan is selectively associated with inhibitory GABAergic synapses but is dispensable for their differentiation. J. Neurosci. 22, 4274–4285.
Lévi, S., Schweizer, C., Bannai, H., Pascual, O., Charrier, C., and Triller, A. (2008). Homeostatic regulation of synaptic GlyR numbers driven by lateral diffusion. Neuron 59, 261–273. doi: 10.1016/j.neuron.2008.05.030
Pubmed Abstract | Pubmed Full Text | CrossRef Full Text | Google Scholar
Lin, Y., Bloodgood, B. L., Hauser, J. L., Lapan, A. D., Koon, A. C., Kim, T. K., et al. (2008). Activity-dependent regulation of inhibitory synapse development by Npas4. Nature 455, 1198–1204. doi: 10.1038/nature07319
Pubmed Abstract | Pubmed Full Text | CrossRef Full Text | Google Scholar
Linden, D. J. (1999). The return of the spike: postsynaptic action potentials and the induction of LTP and LTD. Neuron 22, 661–666. doi: 10.1016/S0896-6273(00)80726-6
Pubmed Abstract | Pubmed Full Text | CrossRef Full Text | Google Scholar
Lu, Y. M., Mansuy, I. M., Kandel, E. R., and Roder, J. (2000). Calcineurin-mediated LTD of GABAergic inhibition underlies the increased excitability of CA1 neurons associated with LTP. Neuron 26, 197–205. doi: 10.1016/s0896-6273(00)81150-2
Pubmed Abstract | Pubmed Full Text | CrossRef Full Text | Google Scholar
Lüscher, C., Nicoll, R. A., Malenka, R. C., and Muller, D. (2000). Synaptic plasticity and dynamic modulation of the postsynaptic membrane. Nat. Neurosci. 3, 545–550. doi: 10.1038/75714
Pubmed Abstract | Pubmed Full Text | CrossRef Full Text | Google Scholar
Lushnikova, I., Skibo, G., Muller, D., and Nikonenko, I. (2011). Excitatory synaptic activity is associated with a rapid structural plasticity of inhibitory synapses on hippocampal CA1 pyramidal cells. Neuropharmacology 60, 757–764. doi: 10.1016/j.neuropharm.2010.12.014
Pubmed Abstract | Pubmed Full Text | CrossRef Full Text | Google Scholar
Maccaferri, G. (2005). Stratum oriens horizontal interneurone diversity and hippocampal network dynamics. J. Physiol. 562, 73–80. doi: 10.1113/jphysiol.2004.077081
Pubmed Abstract | Pubmed Full Text | CrossRef Full Text | Google Scholar
Maffei, A., Nataraj, K., Nelson, S. B., and Turrigiano, G. G. (2006). Potentiation of cortical inhibition by visual deprivation. Nature 443, 81–84. doi: 10.1038/nature05079
Pubmed Abstract | Pubmed Full Text | CrossRef Full Text | Google Scholar
Makino, H., and Malinow, R. (2011). Compartmentalized versus global synaptic plasticity on dendrites controlled by experience. Neuron 72, 1001–1011. doi: 10.1016/j.neuron.2011.09.036
Pubmed Abstract | Pubmed Full Text | CrossRef Full Text | Google Scholar
Malenka, R. C., and Bear, M. F. (2004). LTP and LTD: an embarrassment of riches. Neuron 44, 5–21. doi: 10.1016/j.neuron.2004.09.012
Pubmed Abstract | Pubmed Full Text | CrossRef Full Text | Google Scholar
Marik, S. A., Yamahachi, H., McManus, J. N., Szabo, G., and Gilbert, C. D. (2010). Axonal dynamics of excitatory and inhibitory neurons in somatosensory cortex. PLoS Biol. 8:e1000395. doi: 10.1371/journal.pbio.1000395
Pubmed Abstract | Pubmed Full Text | CrossRef Full Text | Google Scholar
Markram, H., Toledo-Rodriguez, M., Wang, Y., Gupta, A., Silberberg, G., and Wu, C. (2004). Interneurons of the neocortical inhibitory system. Nat. Rev. Neurosci. 5, 793–807. doi: 10.1038/nrn1519
Pubmed Abstract | Pubmed Full Text | CrossRef Full Text | Google Scholar
Marsden, K. C., Beattie, J. B., Friedenthal, J., and Carroll, R. C. (2007). NMDA receptor activation potentiates inhibitory transmission through GABA receptor-associated protein-dependent exocytosis of GABA(A) receptors. J. Neurosci. 27, 14326–14337. doi: 10.1523/jneurosci.4433-07.2007
Pubmed Abstract | Pubmed Full Text | CrossRef Full Text | Google Scholar
Marsden, K. C., Shemesh, A., Bayer, K. U., and Carroll, R. C. (2010). Selective translocation of Ca2+/calmodulin protein kinase IIalpha (CaMKIIalpha) to inhibitory synapses. Proc. Natl. Acad. Sci. U S A 107, 20559–20564. doi: 10.1073/pnas.1010346107
Pubmed Abstract | Pubmed Full Text | CrossRef Full Text | Google Scholar
Marty, S., Wehrlé, R., and Sotelo, C. (2000). Neuronal activity and brain-derived neurotrophic factor regulate the density of inhibitory synapses in organotypic slice cultures of postnatal hippocampus. J. Neurosci. 20, 8087–8095.
Matsuzaki, M., Honkura, N., Ellis-Davies, G. C., and Kasai, H. (2004). Structural basis of long-term potentiation in single dendritic spines. Nature 429, 761–766. doi: 10.1038/nature02617
Pubmed Abstract | Pubmed Full Text | CrossRef Full Text | Google Scholar
Matus, A. (2000). Actin-based plasticity in dendritic spines. Science 290, 754–758. doi: 10.1126/science.290.5492.754
Pubmed Abstract | Pubmed Full Text | CrossRef Full Text | Google Scholar
Mayer, S., Kumar, R., Jaiswal, M., Soykan, T., Ahmadian, M. R., Brose, N., et al. (2013). Collybistin activation by GTP-TC10 enhances postsynaptic gephyrin clustering and hippocampal GABAergic neurotransmission. Proc. Natl. Acad. Sci. U S A 110, 20795–20800. doi: 10.1073/pnas.1309078110
Pubmed Abstract | Pubmed Full Text | CrossRef Full Text | Google Scholar
Mayford, M., Siegelbaum, S. A., and Kandel, E. R. (2012). Synapses and memory storage. Cold Spring Harb. Perspect. Biol. 4:a005751. doi: 10.1101/cshperspect.a005751
Pubmed Abstract | Pubmed Full Text | CrossRef Full Text | Google Scholar
McBain, C. J., and Fisahn, A. (2001). Interneurons unbound. Nat. Rev. Neurosci. 2, 11–23. doi: 10.1038/35049047
Pubmed Abstract | Pubmed Full Text | CrossRef Full Text | Google Scholar
McDonald, B. J., and Moss, S. J. (1997). Conserved phosphorylation of the intracellular domains of GABA(A) receptor beta2 and beta3 subunits by cAMP-dependent protein kinase, cGMP-dependent protein kinase protein kinase C and Ca2+/calmodulin type II-dependent protein kinase. Neuropharmacology 36, 1377–1385. doi: 10.1016/s0028-3908(97)00111-1
Pubmed Abstract | Pubmed Full Text | CrossRef Full Text | Google Scholar
Megías, M., Emri, Z., Freund, T. F., and Gulyás, A. I. (2001). Total number and distribution of inhibitory and excitatory synapses on hippocampal CA1 pyramidal cells. Neuroscience 102, 527–540. doi: 10.1016/s0306-4522(00)00496-6
Pubmed Abstract | Pubmed Full Text | CrossRef Full Text | Google Scholar
Méndez, P., and Bacci, A. (2011). Assortment of GABAergic plasticity in the cortical interneuron melting pot. Neural Plast. 2011:976856. doi: 10.1155/2011/976856
Pubmed Abstract | Pubmed Full Text | CrossRef Full Text | Google Scholar
Micheva, K. D., and Beaulieu, C. (1995). An anatomical substrate for experience-dependent plasticity of the rat barrel field cortex. Proc. Natl. Acad. Sci. U S A 92, 11834–11838. doi: 10.1073/pnas.92.25.11834
Pubmed Abstract | Pubmed Full Text | CrossRef Full Text | Google Scholar
Miles, R., Tóth, K., Gulyás, A. I., Hájos, N., and Freund, T. F. (1996). Differences between somatic and dendritic inhibition in the hippocampus. Neuron 16, 815–823. doi: 10.1016/s0896-6273(00)80101-4
Pubmed Abstract | Pubmed Full Text | CrossRef Full Text | Google Scholar
Morris, N. P., and Henderson, Z. (2000). Perineuronal nets ensheath fast spiking, parvalbumin-immunoreactive neurons in the medial septum/diagonal band complex. Eur. J. Neurosci. 12, 828–838. doi: 10.1046/j.1460-9568.2000.00970.x
Pubmed Abstract | Pubmed Full Text | CrossRef Full Text | Google Scholar
Moss, S. J., Gorrie, G. H., Amato, A., and Smart, T. G. (1995). Modulation of GABAA receptors by tyrosine phosphorylation. Nature 377, 344–348. doi: 10.1038/377344a0
Pubmed Abstract | Pubmed Full Text | CrossRef Full Text | Google Scholar
Nelson, C. D., Kim, M. J., Hsin, H., Chen, Y., and Sheng, M. (2013). Phosphorylation of threonine-19 of PSD-95 by GSK-3beta is required for PSD-95 mobilization and long-term depression. J. Neurosci. 33, 12122–12135. doi: 10.1523/jneurosci.0131-13.2013
Pubmed Abstract | Pubmed Full Text | CrossRef Full Text | Google Scholar
Nimchinsky, E. A., Sabatini, B. L., and Svoboda, K. (2002). Structure and function of dendritic spines. Annu. Rev. Physiol. 64, 313–353. doi: 10.1146/annurev.physiol.64.081501.160008
Pubmed Abstract | Pubmed Full Text | CrossRef Full Text | Google Scholar
Nusser, Z., Hájos, N., Somogyi, P., and Mody, I. (1998). Increased number of synaptic GABA(A) receptors underlies potentiation at hippocampal inhibitory synapses. Nature 395, 172–177. doi: 10.1038/25999
Pubmed Abstract | Pubmed Full Text | CrossRef Full Text | Google Scholar
Papadopoulos, T., Korte, M., Eulenburg, V., Kubota, H., Retiounskaia, M., Harvey, R. J., et al. (2007). Impaired GABAergic transmission and altered hippocampal synaptic plasticity in collybistin-deficient mice. EMBO J. 26, 3888–3899. doi: 10.1038/sj.emboj.7601819
Pubmed Abstract | Pubmed Full Text | CrossRef Full Text | Google Scholar
Patenaude, C., Chapman, C. A., Bertrand, S., Congar, P., and Lacaille, J. C. (2003). GABAB receptor- and metabotropic glutamate receptor-dependent cooperative long-term potentiation of rat hippocampal GABAA synaptic transmission. J. Physiol. 553, 155–167. doi: 10.1113/jphysiol.2003.049015
Pubmed Abstract | Pubmed Full Text | CrossRef Full Text | Google Scholar
Patterson, M. A., Szatmari, E. M., and Yasuda, R. (2010). AMPA receptors are exocytosed in stimulated spines and adjacent dendrites in a Ras-ERK-dependent manner during long-term potentiation. Proc. Natl. Acad. Sci. U S A 107, 15951–15956. doi: 10.1073/pnas.0913875107
Pubmed Abstract | Pubmed Full Text | CrossRef Full Text | Google Scholar
Pérez-Garci, E., Gassmann, M., Bettler, B., and Larkum, M. E. (2006). The GABAB1b isoform mediates long-lasting inhibition of dendritic Ca2+ spikes in layer 5 somatosensory pyramidal neurons. Neuron 50, 603–616. doi: 10.1016/j.neuron.2006.04.019
Pubmed Abstract | Pubmed Full Text | CrossRef Full Text | Google Scholar
Petrini, E. M., Ravasenga, T., Hausrat, T. J., Iurilli, G., Olcese, U., Racine, V., et al. (2014). Synaptic recruitment of gephyrin regulates surface GABAA receptor dynamics for the expression of inhibitory LTP. Nat. Commun. 5:3921. doi: 10.1038/ncomms4921
Pubmed Abstract | Pubmed Full Text | CrossRef Full Text | Google Scholar
Piomelli, D. (2003). The molecular logic of endocannabinoid signalling. Nat. Rev. Neurosci. 4, 873–884. doi: 10.1038/nrn1247
Pubmed Abstract | Pubmed Full Text | CrossRef Full Text | Google Scholar
Pizzorusso, T., Medini, P., Berardi, N., Chierzi, S., Fawcett, J. W., and Maffei, L. (2002). Reactivation of ocular dominance plasticity in the adult visual cortex. Science 298, 1248–1251. doi: 10.1126/science.1072699
Pubmed Abstract | Pubmed Full Text | CrossRef Full Text | Google Scholar
Pouille, F., and Scanziani, M. (2001). Enforcement of temporal fidelity in pyramidal cells by somatic feed-forward inhibition. Science 293, 1159–1163. doi: 10.1126/science.1060342
Pubmed Abstract | Pubmed Full Text | CrossRef Full Text | Google Scholar
Pouille, F., and Scanziani, M. (2004). Routing of spike series by dynamic circuits in the hippocampus. Nature 429, 717–723. doi: 10.1038/nature02615
Pubmed Abstract | Pubmed Full Text | CrossRef Full Text | Google Scholar
Poulopoulos, A., Aramuni, G., Meyer, G., Soykan, T., Hoon, M., Papadopoulos, T., et al. (2009). Neuroligin 2 drives postsynaptic assembly at perisomatic inhibitory synapses through gephyrin and collybistin. Neuron 63, 628–642. doi: 10.1016/j.neuron.2009.08.023
Pubmed Abstract | Pubmed Full Text | CrossRef Full Text | Google Scholar
Pribiag, H., Peng, H., Shah, W. A., Stellwagen, D., and Carbonetto, S. (2014). Dystroglycan mediates homeostatic synaptic plasticity at GABAergic synapses. Proc. Natl. Acad. Sci. U S A 111, 6810–6815. doi: 10.1073/pnas.1321774111
Pubmed Abstract | Pubmed Full Text | CrossRef Full Text | Google Scholar
Ramamoorthi, K., Fropf, R., Belfort, G. M., Fitzmaurice, H. L., McKinney, R. M., Neve, R. L., et al. (2011). Npas4 regulates a transcriptional program in CA3 required for contextual memory formation. Science 334, 1669–1675. doi: 10.1126/science.1208049
Pubmed Abstract | Pubmed Full Text | CrossRef Full Text | Google Scholar
Saliba, R. S., Michels, G., Jacob, T. C., Pangalos, M. N., and Moss, S. J. (2007). Activity-dependent ubiquitination of GABA(A) receptors regulates their accumulation at synaptic sites. J. Neurosci. 27, 13341–13351. doi: 10.1523/jneurosci.3277-07.2007
Pubmed Abstract | Pubmed Full Text | CrossRef Full Text | Google Scholar
Saraga, F., Balena, T., Wolansky, T., Dickson, C. T., and Woodin, M. A. (2008). Inhibitory synaptic plasticity regulates pyramidal neuron spiking in the rodent hippocampus. Neuroscience 155, 64–75. doi: 10.1016/j.neuroscience.2008.05.009
Pubmed Abstract | Pubmed Full Text | CrossRef Full Text | Google Scholar
Sassoè-Pognetto, M., Frola, E., Pregno, G., Briatore, F., and Patrizi, A. (2011). Understanding the molecular diversity of GABAergic synapses. Front. Cell. Neurosci. 5:4. doi: 10.3389/fncel.2011.00004
Pubmed Abstract | Pubmed Full Text | CrossRef Full Text | Google Scholar
Schuemann, A., Klawiter, A., Bonhoeffer, T., and Wierenga, C. J. (2013). Structural plasticity of GABAergic axons is regulated by network activity and GABAA receptor activation. Front. Neural Circuits 7:113. doi: 10.3389/fncir.2013.00113
Pubmed Abstract | Pubmed Full Text | CrossRef Full Text | Google Scholar
Specht, C. G., Izeddin, I., Rodriguez, P. C., El Beheiry, M., Rostaing, P., Darzacq, X., et al. (2013). Quantitative nanoscopy of inhibitory synapses: counting gephyrin molecules and receptor binding sites. Neuron 79, 308–321. doi: 10.1016/j.neuron.2013.05.013
Pubmed Abstract | Pubmed Full Text | CrossRef Full Text | Google Scholar
Spiegel, I., Mardinly, A. R., Gabel, H. W., Bazinet, J. E., Couch, C. H., Tzeng, C. P., et al. (2014). Npas4 regulates excitatory-inhibitory balance within neural circuits through cell-type-specific gene programs. Cell 157, 1216–1229. doi: 10.1016/j.cell.2014.03.058
Pubmed Abstract | Pubmed Full Text | CrossRef Full Text | Google Scholar
Südhof, T. C. (2008). Neuroligins and neurexins link synaptic function to cognitive disease. Nature 455, 903–911. doi: 10.1038/nature07456
Pubmed Abstract | Pubmed Full Text | CrossRef Full Text | Google Scholar
Takahashi, N., Kitamura, K., Matsuo, N., Mayford, M., Kano, M., Matsuki, N., et al. (2012). Locally synchronized synaptic inputs. Science 335, 353–356. doi: 10.1126/science.1210362
Pubmed Abstract | Pubmed Full Text | CrossRef Full Text | Google Scholar
Tanaka, H., Miyazaki, N., Matoba, K., Nogi, T., Iwasaki, K., and Takagi, J. (2012). Higher-order architecture of cell adhesion mediated by polymorphic synaptic adhesion molecules neurexin and neuroligin. Cell Rep. 2, 101–110. doi: 10.1016/j.celrep.2012.06.009
Pubmed Abstract | Pubmed Full Text | CrossRef Full Text | Google Scholar
Tretter, V., Jacob, T. C., Mukherjee, J., Fritschy, J. M., Pangalos, M. N., and Moss, S. J. (2008). The clustering of GABA(A) receptor subtypes at inhibitory synapses is facilitated via the direct binding of receptor alpha 2 subunits to gephyrin. J. Neurosci. 28, 1356–1365. doi: 10.1523/jneurosci.5050-07.2008
Pubmed Abstract | Pubmed Full Text | CrossRef Full Text | Google Scholar
Tretter, V., Mukherjee, J., Maric, H. M., Schindelin, H., Sieghart, W., and Moss, S. J. (2012). Gephyrin, the enigmatic organizer at GABAergic synapses. Front. Cell. Neurosci. 6:23. doi: 10.3389/fncel.2012.00023
Pubmed Abstract | Pubmed Full Text | CrossRef Full Text | Google Scholar
Tyagarajan, S. K., and Fritschy, J. M. (2014). Gephyrin: a master regulator of neuronal function? Nat. Rev. Neurosci. 15, 141–156. doi: 10.1038/nrn3670
Pubmed Abstract | Pubmed Full Text | CrossRef Full Text | Google Scholar
Tyagarajan, S. K., Ghosh, H., Harvey, K., and Fritschy, J. M. (2011a). Collybistin splice variants differentially interact with gephyrin and Cdc42 to regulate gephyrin clustering at GABAergic synapses. J. Cell Sci. 124, 2786–2796. doi: 10.1242/jcs.086199
Pubmed Abstract | Pubmed Full Text | CrossRef Full Text | Google Scholar
Tyagarajan, S. K., Ghosh, H., Yévenes, G. E., Imanishi, S. Y., Zeilhofer, H. U., Gerrits, B., et al. (2013). Extracellular signal-regulated kinase and glycogen synthase kinase 3beta regulate gephyrin postsynaptic aggregation and GABAergic synaptic function in a calpain-dependent mechanism. J. Biol. Chem. 288, 9634–9647. doi: 10.1074/jbc.m112.442616
Pubmed Abstract | Pubmed Full Text | CrossRef Full Text | Google Scholar
Tyagarajan, S. K., Ghosh, H., Yévenes, G. E., Nikonenko, I., Ebeling, C., Schwerdel, C., et al. (2011b). Regulation of GABAergic synapse formation and plasticity by GSK3beta-dependent phosphorylation of gephyrin. Proc. Natl. Acad. Sci. U S A 108, 379–384. doi: 10.1073/pnas.1011824108
Pubmed Abstract | Pubmed Full Text | CrossRef Full Text | Google Scholar
van Versendaal, D., Rajendran, R., Saiepour, M. H., Klooster, J., Smit-Rigter, L., Sommeijer, J. P., et al. (2012). Elimination of inhibitory synapses is a major component of adult ocular dominance plasticity. Neuron 74, 374–383. doi: 10.1016/j.neuron.2012.03.015
Pubmed Abstract | Pubmed Full Text | CrossRef Full Text | Google Scholar
Varoqueaux, F., Jamain, S., and Brose, N. (2004). Neuroligin 2 is exclusively localized to inhibitory synapses. Eur. J. Cell Biol. 83, 449–456. doi: 10.1078/0171-9335-00410
Pubmed Abstract | Pubmed Full Text | CrossRef Full Text | Google Scholar
Vithlani, M., and Moss, S. J. (2009). The role of GABAAR phosphorylation in the construction of inhibitory synapses and the efficacy of neuronal inhibition. Biochem. Soc. Trans. 37, 1355–1358. doi: 10.1042/bst0371355
Pubmed Abstract | Pubmed Full Text | CrossRef Full Text | Google Scholar
Vithlani, M., Terunuma, M., and Moss, S. J. (2011). The dynamic modulation of GABA(A) receptor trafficking and its role in regulating the plasticity of inhibitory synapses. Physiol. Rev. 91, 1009–1022. doi: 10.1152/physrev.00015.2010
Pubmed Abstract | Pubmed Full Text | CrossRef Full Text | Google Scholar
Vlachos, A., Reddy-Alla, S., Papadopoulos, T., Deller, T., and Betz, H. (2013). Homeostatic regulation of gephyrin scaffolds and synaptic strength at mature hippocampal GABAergic postsynapses. Cereb. Cortex 23, 2700–2711. doi: 10.1093/cercor/bhs260
Pubmed Abstract | Pubmed Full Text | CrossRef Full Text | Google Scholar
Wang, D., and Fawcett, J. (2012). The perineuronal net and the control of CNS plasticity. Cell Tissue Res. 349, 147–160. doi: 10.1007/s00441-012-1375-y
Pubmed Abstract | Pubmed Full Text | CrossRef Full Text | Google Scholar
Wang, L., and Maffei, A. (2014). Inhibitory plasticity dictates the sign of plasticity at excitatory synapses. J. Neurosci. 34, 1083–1093. doi: 10.1523/jneurosci.4711-13.2014
Pubmed Abstract | Pubmed Full Text | CrossRef Full Text | Google Scholar
Wierenga, C. J., Becker, N., and Bonhoeffer, T. (2008). GABAergic synapses are formed without the involvement of dendritic protrusions. Nat. Neurosci. 11, 1044–1052. doi: 10.1038/nn.2180
Pubmed Abstract | Pubmed Full Text | CrossRef Full Text | Google Scholar
Woo, J., Kwon, S. K., Nam, J., Choi, S., Takahashi, H., Krueger, D., et al. (2013). The adhesion protein IgSF9b is coupled to neuroligin 2 via S-SCAM to promote inhibitory synapse development. J. Cell Biol. 201, 929–944. doi: 10.1083/jcb.201209132
Pubmed Abstract | Pubmed Full Text | CrossRef Full Text | Google Scholar
Woodin, M. A., Ganguly, K., and Poo, M. M. (2003). Coincident pre- and postsynaptic activity modifies GABAergic synapses by postsynaptic changes in Cl- transporter activity. Neuron 39, 807–820. doi: 10.1016/s0896-6273(03)00507-5
Pubmed Abstract | Pubmed Full Text | CrossRef Full Text | Google Scholar
Xiang, S., Kim, E. Y., Connelly, J. J., Nassar, N., Kirsch, J., Winking, J., et al. (2006). The crystal structure of Cdc42 in complex with collybistin II, a gephyrin-interacting guanine nucleotide exchange factor. J. Mol. Biol. 359, 35–46. doi: 10.1016/j.jmb.2006.03.019
Pubmed Abstract | Pubmed Full Text | CrossRef Full Text | Google Scholar
Xiang, S., Nichols, J., Rajagopalan, K. V., and Schindelin, H. (2001). The crystal structure of Escherichia coli MoeA and its relationship to the multifunctional protein gephyrin. Structure 9, 299–310. doi: 10.1016/s0969-2126(01)00588-3
Pubmed Abstract | Pubmed Full Text | CrossRef Full Text | Google Scholar
Xu, T., Yu, X., Perlik, A. J., Tobin, W. F., Zweig, J. A., Tennant, K., et al. (2009). Rapid formation and selective stabilization of synapses for enduring motor memories. Nature 462, 915–919. doi: 10.1038/nature08389
Pubmed Abstract | Pubmed Full Text | CrossRef Full Text | Google Scholar
Yamagata, M., Sanes, J. R., and Weiner, J. A. (2003). Synaptic adhesion molecules. Curr. Opin. Cell Biol. 15, 621–632. doi: 10.1016/S0955-0674(03)00107-8
Pubmed Abstract | Pubmed Full Text | CrossRef Full Text | Google Scholar
Yang, G., Pan, F., and Gan, W. B. (2009). Stably maintained dendritic spines are associated with lifelong memories. Nature 462, 920–924. doi: 10.1038/nature08577
Pubmed Abstract | Pubmed Full Text | CrossRef Full Text | Google Scholar
Yuste, R., and Bonhoeffer, T. (2001). Morphological changes in dendritic spines associated with long-term synaptic plasticity. Annu. Rev. Neurosci. 24, 1071–1089. doi: 10.1146/annurev.neuro.24.1.1071
Pubmed Abstract | Pubmed Full Text | CrossRef Full Text | Google Scholar
Zacchi, P., Antonelli, R., and Cherubini, E. (2014). Gephyrin phosphorylation in the functional organization and plasticity of GABAergic synapses. Front. Cell. Neurosci. 8:103. doi: 10.3389/fncel.2014.00103
Pubmed Abstract | Pubmed Full Text | CrossRef Full Text | Google Scholar
Zhang, C., Atasoy, D., Araç, D., Yang, X., Fucillo, M. V., Robison, A. J., et al. (2010). Neurexins physically and functionally interact with GABA(A) receptors. Neuron 66, 403–416. doi: 10.1016/j.neuron.2010.04.008
Pubmed Abstract | Pubmed Full Text | CrossRef Full Text | Google Scholar
Zhu, J. J., Qin, Y., Zhao, M., Van Aelst, L., and Malinow, R. (2002). Ras and Rap control AMPA receptor trafficking during synaptic plasticity. Cell 110, 443–455. doi: 10.1016/s0092-8674(02)00897-8
Pubmed Abstract | Pubmed Full Text | CrossRef Full Text | Google Scholar
Zilberter, Y. (2000). Dendritic release of glutamate suppresses synaptic inhibition of pyramidal neurons in rat neocortex. J. Physiol. 528, 489–496. doi: 10.1111/j.1469-7793.2000.00489.x
Pubmed Abstract | Pubmed Full Text | CrossRef Full Text | Google Scholar
Keywords: GABAergic synapses, structural plasticity, activity-dependent plasticity, interneuron, memory, cerebral cortex, hippocampus
Citation: Flores CE and Méndez P (2014) Shaping inhibition: activity dependent structural plasticity of GABAergic synapses. Front. Cell. Neurosci. 8:327. doi: 10.3389/fncel.2014.00327
Received: 21 August 2014; Accepted: 28 September 2014;
Published online: 27 October 2014.
Edited by:
Andrea Barberis, Fondazione Istituto Italiano di Tecnologia, ItalyCopyright © 2014 Flores and Méndez. This is an open-access article distributed under the terms of the Creative Commons Attribution License (CC BY). The use, distribution and reproduction in other forums is permitted, provided the original author(s) or licensor are credited and that the original publication in this journal is cited, in accordance with accepted academic practice. No use, distribution or reproduction is permitted which does not comply with these terms.
*Correspondence: Carmen E. Flores and Pablo Méndez, Department of Basic Neuroscience, Geneva Medical Center, University of Geneva, 1 Rue Michel Servet, 1211, Geneva 4, Switzerland e-mail:Y2FybWVuLmZsb3Jlc0B1bmlnZS5jaA==;
cGFibG8ubWVuZGV6QHVuaWdlLmNo