- Department of Neuroimmunology, Research Institute of Environmental Medicine, Nagoya University, Nagoya, Japan
Microglia are macrophage-like resident immune cells that contribute to the maintenance of homeostasis in the central nervous system (CNS). Abnormal activation of microglia can cause damage in the CNS, and accumulation of activated microglia is a characteristic pathological observation in neurologic conditions such as trauma, stroke, inflammation, epilepsy, and neurodegenerative diseases. Activated microglia secrete high levels of glutamate, which damages CNS cells and has been implicated as a major cause of neurodegeneration in these conditions. Glutamate-receptor blockers and microglia inhibitors (e.g., minocycline) have been examined as therapeutic candidates for several neurodegenerative diseases; however, these compounds exerted little therapeutic benefit because they either perturbed physiological glutamate signals or suppressed the actions of protective microglia. The ideal therapeutic approach would hamper the deleterious roles of activated microglia without diminishing their protective effects. We recently found that abnormally activated microglia secrete glutamate via gap-junction hemichannels on the cell surface. Moreover, administration of gap-junction inhibitors significantly suppressed excessive microglial glutamate release and improved disease symptoms in animal models of neurologic conditions such as stroke, multiple sclerosis, amyotrophic lateral sclerosis, and Alzheimer's disease. Recent evidence also suggests that neuronal and glial communication via gap junctions amplifies neuroinflammation and neurodegeneration. Elucidation of the precise pathologic roles of gap junctions and hemichannels may lead to a novel therapeutic strategies that can slow and halt the progression of neurodegenerative diseases.
Introduction
Microglia are macrophage-like immune cells that reside in the central nervous system (CNS), where they play multiple roles: presenting antigen to initiate immunological reactions, directing attack against non-self antigens, debris clearance, support of neuronal circuit development (Kreutzberg, 1996; Kempermann and Neumann, 2003; Block et al., 2007; Takeuchi, 2010; Boche et al., 2013), and so on. Microglia contribute to maintenance of CNS homeostasis, but abnormal activation of these cells often causes damage to surrounding cells and tissues. Microgliosis, the accumulation of activated microglia, is a characteristic pathological feature in many neurologic conditions such as trauma, stroke, inflammation, epilepsy, and neurodegenerative diseases (Cagnin et al., 2001; Eikelenboom et al., 2002; McGeer and McGeer, 2002; Nelson et al., 2002; Orr et al., 2002; Bruijn et al., 2004; Pavese et al., 2006). Activated microglia release massive amounts of glutamate, at much higher levels than astrocytes and neurons (mM vs. μM), and destroy neural cells; these processes have been implicated as a major cause of neuronal damage in neurologic diseases (Piani et al., 1992; Barger and Basile, 2001; Schwartz et al., 2003; Ye et al., 2003; Kipnis et al., 2004; Takeuchi et al., 2005, 2008b; Herman and Jahr, 2007; Liang et al., 2008; Yawata et al., 2008). Therefore, blockade of glutamate signaling and inhibition of microglial activation have been explored as therapeutic candidates for several neurodegenerative diseases. However, glutamate receptor blockers also perturb physiological glutamate signals and cause severe adverse side effects (Parsons et al., 2007). Tetracycline and two of its derivatives (doxycycline and minocycline) have been used as inhibitors of microglial activation, but these compounds exerted little therapeutic benefit, because activated microglia also exert neuroprotective effects such as production of neurotrophic factors and sequestration of neurotoxic substances (Zietlow et al., 1999; Kempermann and Neumann, 2003; Kipnis et al., 2004; Koenigsknecht and Landreth, 2004; Schwab and Schluesener, 2004). Thus, the optimal therapeutic strategy would inhibit the deleterious effects of activated microglia without diminishing their protective roles (Takeuchi, 2010). We recently found that neurotoxic activated microglia secrete glutamate through gap-junction hemichannels. Recent evidence also suggests that neuronal and glial communication by gap junctions amplifies neuroinflammation and neurodegeneration. Therefore, elucidation of the pathologic roles of gap junctions and hemichannels may provide us with new therapeutic strategies against many neurologic diseases.
Microglia as the “Enemy Within”
Microglia, which originate from bone marrow–derived myeloid cells, account for approximately 10% of cells in the human CNS (Del Rio-Hortega, 1932). Microglia are predominantly observed in gray matter, especially in the olfactory bulb, hippocampus, basal ganglia, and substantia nigra (Lawson et al., 1990). Under healthy physiological conditions, microglia persist in a quiescent state with ramified morphology (resting microglia) and survey the environment of the CNS (Davalos et al., 2005; Nimmerjahn et al., 2005). Under pathological conditions, microglia dramatically change their morphology and adopt an amoeboid appearance in the activated state. Activated microglia express surface molecules such as Fc receptor, CD11b, CD11c, CD14, major histocompatibility complex (MHC) molecules, Toll-like receptors (TLRs), scavenger receptors, and cytokine/chemokine receptors, and they can act as both antigen-presenting cells and immunological effector cells (Suzumura et al., 1987; Rock et al., 2004). In addition to innate immunity, activated microglia also play other beneficial roles, such as neuroprotection mediated by release of neurotrophic factors (Zietlow et al., 1999; Bessis et al., 2007; Liang et al., 2010), maintenance of CNS homeostasis by clearance of cellular debris and toxic substances (Upender and Naegele, 1999; Marin-Teva et al., 2004; Iribarren et al., 2005; Simard et al., 2006; Richard et al., 2008), and guidance of stem-cell migration in neuronal repair and neurogenesis (Aarum et al., 2003; Ziv et al., 2006a,b).
TLRs and scavenger receptors may contribute to diminishing neurotoxicity by sequestering neurotoxic substances such as amyloid β (El Khoury et al., 1996; Coraci et al., 2002; Bamberger et al., 2003; Liu et al., 2005); however, signals downstream of these receptors also enhance microglial neurotoxic effects by producing neurotoxic factors such as cytokines/chemokines, nucleic acids, excitatory amino acids, reactive oxygen species (ROS), and proteases (Kempermann and Neumann, 2003; Takeuchi et al., 2005, 2006; Kawanokuchi et al., 2006; Block et al., 2007). In fact, expression levels of TLRs and scavenger receptors are upregulated in a variety of neurologic diseases (Akiyama and McGeer, 1990; Grewal et al., 1997; El Khoury et al., 1998; Bsibsi et al., 2002; Cho et al., 2005; Carpentier et al., 2008). Therefore, whether activated microglia exert a neurotoxic or a neuroprotective effect may depend on their environment, the spatiotemporal distribution of the microglia themselves, and the type and magnitude of stimuli (Jimenez et al., 2008; Wu et al., 2008; Nakanishi and Wu, 2009; Sawada, 2009). A recently proposed hypothesis suggests, by analogy to macrophage activation, that activated microglia comprise two subpopulations, the neurotoxic (M1) and neuroprotective (M2) species (Mantovani et al., 2002; Henkel et al., 2009; Boche et al., 2013); however, this hypothesis is still open to debate. At least in pathological conditions, deleterious microglial activation is probably involved in the progression of various neurological disorders (Yrjanheikki et al., 1998; Wu et al., 2002; Zhu et al., 2002; Stirling et al., 2004; Boillee et al., 2006; Seabrook et al., 2006). Thus, elucidating the precise mechanism of microglial neurotoxicity is a necessary step toward development of effective therapeutic strategies against neurologic diseases.
Glutamate as a Major Neurotoxic Factor from Microglia
Glutamate is the most potently neurotoxic factor released from activated microglia. Excessive glutamate induces severe neuronal damage via excitotoxicity (Piani et al., 1992; Barger and Basile, 2001; Takeuchi et al., 2005, 2006). A common misconception is that inflammatory cytokines produced by activated microglia directly induce neuronal damage. In fact, these cytokines have little direct neurotoxic effect (Takeuchi et al., 2006; Takeuchi, 2010). Although tumor necrosis factor-α (TNF-α) and interferon-γ (IFN-γ) are considered to be the most deleterious inflammatory cytokines produced by activated microglia, these cytokines have only weak direct neurotoxic effects because they also enhance neuroprotective cascades involving mitogen-activated protein kinase (MAPK) and expression of nuclear factor κB (NF-κB) (Ghezzi and Mennini, 2001; Kamata et al., 2005). In general, inflammatory cytokines induce neurotoxicity indirectly by stimulating microglia in an autocrine/paracrine manner. These stimuli induce microglia to release high levels of glutamate, resulting in neuronal damage via excitotoxicity. Moreover, a recent paper showed that activated microglial glutamate suppresses astrocytic glutamate transporters, which play a pivotal role in maintenance of the physiological extracellular glutamate level (Takaki et al., 2012); this suppression probably worsens excitotoxic neuronal damage. Although microglia also express glutamate transporters, they seem much less effective at maintaining extracellular glutamate homeostasis than astrocytic glutamate transporters (Liang et al., 2008).
One of the earliest pathologic features of excitotoxicity is formation of neuritic beading, i.e., focal bead-like swelling in dendrites and axons (Takeuchi et al., 2005; Mizuno et al., 2008). Neuritic beading is a common neuropathological hallmark of many neurologic conditions such as ischemia, epilepsy, mechanical pressure, brain tumor, aging, neuroinflammatory diseases, and neurodegenerative diseases such as multiple sclerosis (MS), Alzheimer's disease (AD), Parkinson's disease (PD), and amyotrophic lateral sclerosis (ALS) (Delisle and Carpenter, 1984; Hori and Carpenter, 1994; Takahashi et al., 1997; Trapp et al., 1998; Dickson et al., 1999; Mattila et al., 1999; Swann et al., 2000; Goel et al., 2003; Pavlidis et al., 2003; Saito et al., 2003; Dutta and Trapp, 2007). Recent studies elucidated the detailed role of microglial glutamate in formation of neuritic beading and subsequent neuronal death. Glutamate produced by activated microglia activates neuronal N-methyl-D-aspartate (NMDA) receptor signaling, which promotes Ca2+ influx and activates Ca2+/calmodulin-dependent protein kinase (CaMK). CaMK activates neuronal nitric oxide synthase (nNOS) and increases the intracellular concentration of nitric oxide (NO). NO in turn inhibits mitochondrial respiratory chain complex IV, resulting in a rapid reduction in intracellular ATP levels. Ultimately, the loss of intracellular energy pools suppresses dendritic and axonal transport, leading to bead-like accumulation of cytoskeletal and motor proteins along neurites and the formation of neuritic beading. Thus, a low-energy state results in neuronal dysfunction. Persistence of this neuronal dysfunction eventually causes neuronal death [i.e., excitotoxic neuronal death or non-cell-autonomous neuronal death (Lobsiger and Cleveland, 2007)].
Recent studies have revealed the precise mechanism of glutamate production by activated microglia (Takeuchi et al., 2005, 2006) (Figure 1). Two pathways are involved in cellular glutamate synthesis (Newsholme and Newsholme, 1989; Newsholme and Calder, 1997; Yudkoff, 1997; Nissim, 1999). One of these pathways is mediated by glutamate dehydrogenase, which converts α-ketoglutarate to glutamate. Most cells use this pathway to maintain cellular homeostasis of glutamate levels. The other pathway is mediated by glutaminase, which produces glutamate from extracellular glutamine brought into the cell via glutamine transporters. Resting microglia maintain their physiological glutamate level via the glutamate dehydrogenase pathway, as in other cell types, and secrete very little glutamate into the extracellular space (Figure 1). By contrast, activated microglia produce excessive amounts of glutamate as a result of upregulation of glutaminase, but not glutamate dehydrogenase. Subsequently, activated microglia release massive amounts of glutamate via gap-junction hemichannels. Inflammatory cytokines such as TNF-α and IFN-γ enhance not only glutaminase expression level but also cell-surface localization of hemichannels in microglia (Eugenin et al., 2001; Takeuchi et al., 2006). These two phenomena may act synergistically to release excess glutamate, leading to excitotoxic neuronal damage (Figure 1). Moreover, the extracellular glutamine level is critical for microglial glutamate production (Takeuchi et al., 2006). In the CNS, glutamine from astrocytes is essential for glutamate production in neurons (Tsacopoulos and Magistretti, 1996), suggesting that it also plays an important role in microglial glutamate production.
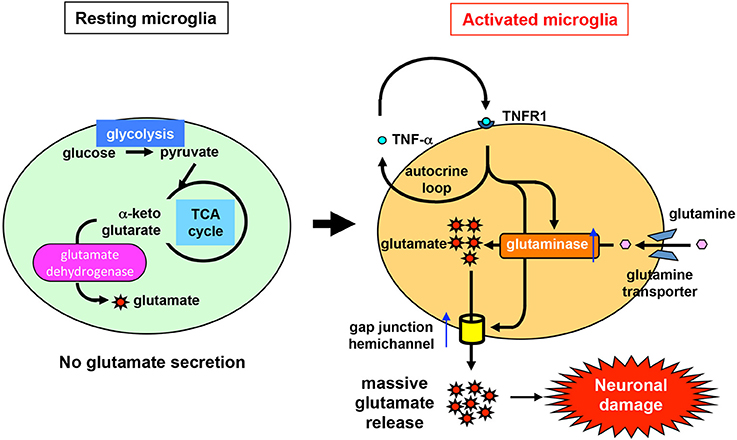
Figure 1. Mechanism of glutamate production and release by activated microglia. Like other types of cells, resting microglia use glutamate dehydrogenase to synthesize glutamate from intracellular α-ketoglutarate in order to maintain a physiologically normal level of glutamate. Under resting conditions, microglia release very little glutamate into the extracellular space. By contrast, under pathological conditions, glutaminase and gap-junction hemichannels are upregulated in activated microglia (e.g., in response to stimulation by TNF-α). Glutaminase synthesizes excess glutamate from extracellular glutamine, which is brought into the cell via glutamine transporters. Subsequently, high levels of glutamate are secreted through gap-junction hemichannels, resulting in eventual neuronal damage.
Gap Junctions in CNS Cells
Gap junctions contribute to formation of intercellular channels that directly connect the cytoplasmic compartments of neighboring cells (Yeager and Harris, 2007). These channels pass various small molecules (~1000 Da) and ions, although the charges and shapes of these molecules may affect the rate of transfer through gap junctions (Goldberg et al., 2004). Each gap junction is composed of a pair of hemichannels docked in a head-to-head configuration. Hemichannels are organized as hexagonal cylinders with central pores, and each hemichannel consists of a hexameric cluster of protein subunits called connexins (in vertebrates) or innexins (in invertebrates). Connexins are encoded by a conserved family of genes with at least 21 members in mammals. There are 21 connexin genes in the human genome and 20 connexin genes in the mouse genome; 19 of these proteins have orthologs in both humans and mice (Willecke et al., 2002; Laird, 2006). The connexin isoforms structurally interact in multiple ways. Homomeric hemichannels consist of a single connexin isoform, whereas heteromeric hemichannels contain two or more different connexin isoforms. Likewise, a homotypic gap junction channel is composed of two identical hemichannels, whereas a heterotypic gap junction channel contains two different hemichannels. Thus, the compositions of gap junctions can be classified into four types: homomeric and homotypic; heteromeric and homotypic; homomeric and heterotypic; and heteromeric and heterotypic (Figure 2). This heterogeneity of connexin configurations confers complexity to the gap junction/hemichannel system.
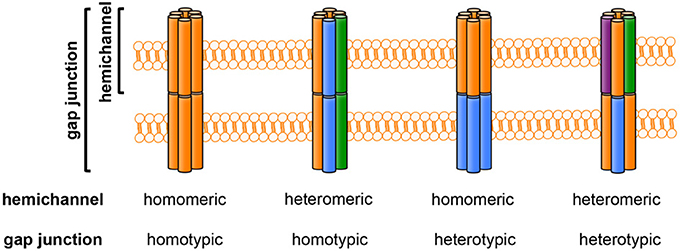
Figure 2. The composition of gap junctions and hemichannels. Each colored column (orange, blue, green, or purple) represents a different connexin isoform. Hemichannels may be homomeric (composed of one connexin isoform) or heteromeric (composed of more than one connexin isoform). Gap-junction channels may be homotypic (formed by identical hemichannels) or heterotypic (formed by different hemichannels).
Gap junctions allow direct intracellular propagation of second messengers (e.g., Ca2+, IP3, cAMP, and cGMP), metabolites (e.g., glutamate, glucose, and glutathione), and nucleotides (e.g., ATP, ADP, and RNA) between adjacent cells (Goldberg et al., 1999, 2002; Harris, 2001, 2007; Saez et al., 2003; Valiunas et al., 2005; Laird, 2006). Moreover, recent studies revealed that uncoupled “free” hemichannels facilitate two-way transfer of molecules between the cytosol and extracellular milieu (De Vuyst et al., 2007; Retamal et al., 2007; Laird, 2010). Intracellular communication via gap junctions and hemichannels is regulated by such mechanisms as channel gating via chemicals, pH, and voltage, as well as by changes in connexin transcription, translation, post-translational phosphorylation and ubiquitination, membrane insertion, and hemichannel internalization and degradation (Laird, 2006; Leithe and Rivedal, 2007; Solan and Lampe, 2009). The time courses of these changes range from milliseconds to hours and are influenced by the environmental conditions in cells and tissues.
Whereas vertebrate cells use connexins to form gap junctions and hemichannels, invertebrate cells use innexins, which lack sequence homology to connexins. A search of the human genome identified three innexin-related genes (Barbe et al., 2006). Because of the occurrence of homologous genes in both vertebrates and invertebrates, the corresponding proteins were termed pannexins: pannexin1 (Panx1), pannexin2 (Panx2), and pannexin3 (Panx3). Pannexins have the same transmembrane topology as connexins, with four transmembrane domains and cytoplasmic amino-terminal and carboxyl-terminal domains. Recent evidence indicates that pannexins also form uncoupled hemichannels in mammalian cells; however, it is not clear whether they can form functional gap junctions (Dahl and Locovei, 2006).
Tissues have characteristic connexin expression profiles, and neural cells in the CNS express multiple connexins (Dermietzel et al., 1989, 2000; Bittman and Loturco, 1999; Chang et al., 1999; Nagy and Rash, 2000; Eugenin et al., 2001; Rash et al., 2001; Teubner et al., 2001; Altevogt et al., 2002; Parenti et al., 2002; Rouach et al., 2002; Odermatt et al., 2003; Takeuchi et al., 2006). All neurons express Cx36 and Cx45, whereas other neural connexins are expressed with more specific spatiotemporal profiles (Sohl et al., 2005). Electrical coupling between neurons has been implicated in neuronal synchronization in the CNS (Christie et al., 1989; Bouskila and Dudek, 1993; Wong et al., 1995). Neuronal gap junctions composed of Cx36 and Cx45 are thought to be homomeric and homotypic (Al-Ubaidi et al., 2000; Teubner et al., 2001), and these junctions are important for formation of electrical synapses (Deans et al., 2001; Hormuzdi et al., 2001). Rodent knockout models have shown that other connexins can compensate for the functions of Cx36 and Cx45, despite differences in conformation or permeability (Frank et al., 2010; Zlomuzica et al., 2010). Furthermore, there is accumulating evidence that gap-junction coupling plays a pivotal role in neuronal differentiation. Mice lacking Cx43 exhibit neonatal death and abnormal migration in the neural crest and neocortex (Lo et al., 1999; Xu et al., 2001; Fushiki et al., 2003). Blockade of gap junctions also hampers retinoic acid–induced neuronal differentiation of NT2 and P19 cells (Bani-Yaghoub et al., 1999a,b). Moreover, Cx36-containing gap junctions are important in neuronal remodeling and short-term spatial memory in some mature organisms (Allen et al., 2011; Hartfield et al., 2011). In contrast to neuron–neuron coupling, for which the evidence is convincing, the existence of functional neuron–glia coupling in the CNS is still a matter of debate (Nadarajah et al., 1996; Alvarez-Maubecin et al., 2000; Rash et al., 2001, 2007).
Astrocytes, the main CNS cells coupled via gap junctions, primarily express Cx43 and Cx30 (Dermietzel et al., 1991; Nagy and Rash, 2000). Consistent with this, Cx43/Cx30 double-knockout mice exhibit minimal gap-junction communication between astrocytes (Wallraff et al., 2006; Rouach et al., 2008), suggesting that functional astrocytic gap junctions are composed predominantly of these two connexins. Cx43-deficient astrocytes exhibit reduced gap-junction coupling, although they express other connexin subtypes including Cx30, Cx26, Cx40, Cx45, and Cx46 (Naus et al., 1997; Scemes et al., 1998; Dermietzel et al., 2000). Although Cx30 has been detected exclusively in astrocytes, Cx30 knockout mice develop only mild abnormalities, including hearing loss due to cochlear degeneration (Teubner et al., 2003). Thus, other astrocytic connexin subtypes do not seem to compensate for a lack of Cx43. Astrocytic gap junctions facilitate the formation of functional syncytium that buffers extracellular glutamate elevation, pH, and K+ concentrations associated with firing neurons, and also propagates intracellular Ca2+ waves that modulate neuronal activities (Walz and Hertz, 1983; Jefferys, 1995; Charles, 1998; Anderson and Swanson, 2000; Ransom et al., 2003). Moreover, astrocytic gap-junction communication facilitates trafficking of glucose and its metabolites, thereby mediating interactions between cerebral vascular endothelium and neurons (Giaume et al., 1997; Goldberg et al., 1999; Tabernero et al., 2006). Thus, astrocytic gap junctions play pivotal roles in modulating neuronal activities and maintaining CNS homeostasis. Astrocyte–astrocyte coupling can be achieved by any of the allowed combinations of homomeric or heteromeric hemichannels in homotypic or heterotypic configurations. Cx30 and Cx26 form both heteromeric and heterotypic channels (Nagy et al., 2003; Altevogt and Paul, 2004), whereas Cx43 forms homomeric and homotypic channels (Orthmann-Murphy et al., 2007). A previous report demonstrated that gap-junction coupling in astrocytes results in two distinct subpopulations of cells. Astrocytes expressing glutamate transporters are extensively coupled to each other, whereas strocytes expressing glutamate receptors are not coupled to other astrocytes (Wallraff et al., 2004), suggesting that these cells play a role in buffering extracellular glutamate (Anderson and Swanson, 2000).
Oligodendrocytes primarily express Cx29, Cx32, and Cx47 (Dermietzel et al., 1989; Altevogt et al., 2002; Odermatt et al., 2003). Oligodendrocytic gap junctions facilitate the trafficking of ions and nutrients from somas to myelin layers (Paul, 1995). Mice lacking Cx32 exhibit reduced myelin volume, enhanced excitability in the CNS, and progressive peripheral neuropathies (Anzini et al., 1997; Sutor et al., 2000). Cx32/Cx47 double-knockout mice develop abnormal movements and seizures associated with vacuolated myelin and axonal degeneration in the CNS, whereas Cx47-deficient mice exhibit only minimal CNS abnormalities (Menichella et al., 2003). Cx32 and Cx47 in oligodendrocytes are essential for spatial buffering of K+ in response to neuronal activity; failure of this function leads to myelin swelling and subsequent axonal degeneration (Menichella et al., 2006). Oligodendrocyte–oligodendrocyte coupling is mediated by gap junctions in homotypic configurations with homomeric or heteromeric hemichannels containing Cx32 or Cx47 (Orthmann-Murphy et al., 2007). Furthermore, oligodendrocytes also couple with astrocytes. Astrocyte–oligodendrocyte coupling may include heterotypic configurations of Cx43–Cx47, Cx30–Cx32, or Cx26–Cx32 (Nagy et al., 2003; Altevogt and Paul, 2004; Orthmann-Murphy et al., 2007). In addition to astrocyte–astrocyte coupling, astrocyte–oligodendrocyte coupling is important in the glial syncytium to facilitate the propagation of Ca2+ waves and the buffering of extracellular K+ and neurotransmitters such as glutamate (Walz and Hertz, 1983; Jefferys, 1995; Charles, 1998; Anderson and Swanson, 2000; Ransom et al., 2003).
Microglia express Cx32, Cx36, and Cx43 (Eugenin et al., 2001; Parenti et al., 2002; Garg et al., 2005; Takeuchi et al., 2006; Kielian, 2008; Talaveron et al., 2014), but form few functional gap junctions under resting conditions. The expression of connexins rises in activated microglia, although it remains unclear whether upregulated expression of connexins leads to enhanced formation of functional gap junctions with microglia and other CNS cells (Eugenin et al., 2001; Garg et al., 2005; Kielian, 2008; Takeuchi, 2010; Wasseff and Scherer, 2014). Recent evidence demonstrates that uncoupled microglial hemichannels play important roles in bidirectional trafficking of small molecules between the cytoplasm and extracellular space (Takeuchi et al., 2011; Eugenin et al., 2012).
The evidence described above might give the false impression that CNS cells express only a narrow range of combinations of homomeric hemichannels and gap junctions. However, the precise configuration of these hemichannels [i.e., homomeric and homotypic; heteromeric and homotypic; homomeric and heterotypic; and heteromeric and heterotypic (Figure 2)] has yet to be elucidated. In addition, our recent reverse transcription–PCR analysis using mouse primary cultures indicated that gap junctions/hemichannels in neurons and glial cells may consist of a wider range of combinations of connexins than expected (Table 1) (Takeuchi et al., 2014): neurons predominantly express Cx43, Cx50, Cx31, Cx30.3, Cx29, and Cx36; microglia predominantly express Cx46, Cx37, Cx40, Cx33, Cx57, Cx32, Cx31, Cx30.3, Cx47, Cx36, Cx30.2, Cx39, and Cx23; astrocytes predominantly express Cx43, Cx37, Cx57, Cx26, Cx31, Cx30.3, Cx45, Cx30.2, Cx39, and Cx23; and oligodendrocytes predominantly express Cx46, Cx37, Cx40, Cx33, Cx57, Cx32, Cx26, Cx31, Cx30.3, Cx31.1, Cx30, Cx29, Cx36, Cx30.2, Cx39, and Cx23. These findings imply that connexin expression profiles in the CNS are dynamic, both in healthy and pathological states.
Gap Junctions Composed of Connexins as a Novel Therapeutic Target for Neurologic Diseases
As mentioned above, glial gap junctions play an important role in maintenance of homeostasis in the CNS under the physiological conditions. These structures, however, also contribute to the initiation and propagation of pathologic conditions (Orellana et al., 2009). Stroke and trauma provide examples that illustrate this mechanism. Ischemia or contusion leads to a rapid decrease in intracellular oxygen levels and subsequent reduction in ATP synthesis, resulting in eventual cell death (Kalogeris et al., 2012). Injured cells contain toxic ions and molecules at high concentrations (e.g., Ca2+, K+, ROS, and NO). These toxic molecules are propagated from injured cells to healthier cells through gap junctions. Ischemic conditions also induce uncoupled hemichannels to open, leading to paracrine transfer of toxic molecules (Thompson et al., 2006; De Vuyst et al., 2007). These waves of death signals activate astrocytes and microglia, inducing the release of toxic molecules including glutamate, ROS, NO, and pro-inflammatory cytokines and chemokines. This vicious amplification spiral of signaling could worsen neuroinflammation by recruiting leukocytes and increasing the lesion area (Orellana et al., 2009) (Figure 3). Moreover, gap junction and hemichannel blockers have exerted therapeutic effects in experimental models of stroke and spinal cord injury (Rawanduzy et al., 1997; Frantseva et al., 2002; De Pina-Benabou et al., 2005; Takeuchi et al., 2008a; Tamura et al., 2011; Huang et al., 2012; Umebayashi et al., 2014).
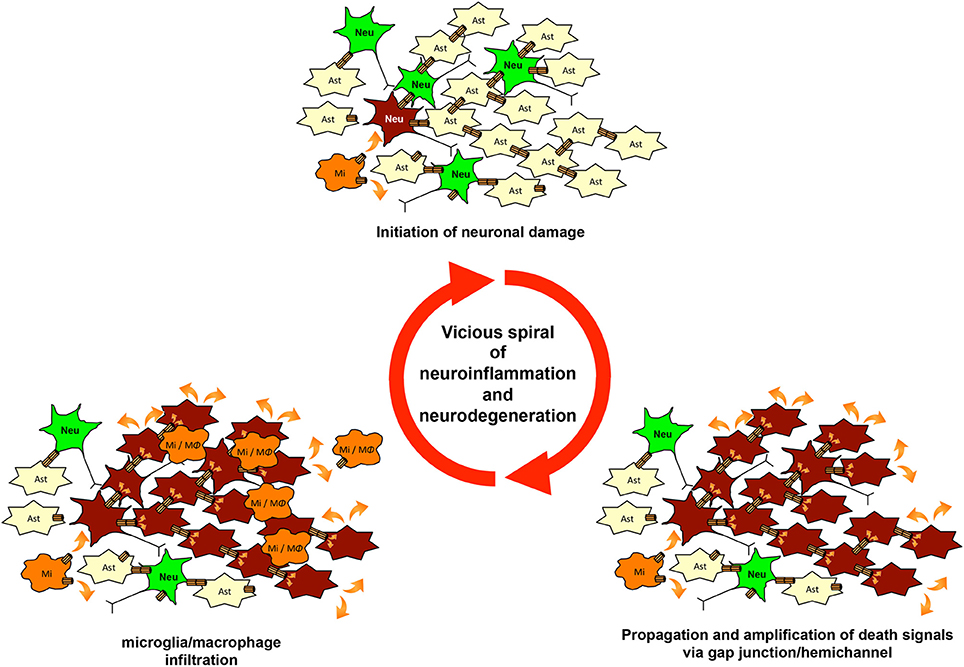
Figure 3. The vicious spiral of neuroinflammation and neurodegeneration mediated by gap junctions and hemichannels. Microglial glutamate release via hemichannels initiates neuronal damage. Then, waves of death signals are propagated and amplified via glial and neuronal gap-junction communication. Chemoattractants from damaged cells induce infiltration by microglia and macrophages. These infiltrating cells initiate further neuronal damage and enlarge the lesion of neuroinflammation and neurodegeneration. This vicious spiral of neuroinflammation and neurodegeneration may be involved in the progression of various neurologic diseases.
Abnormal expression of glial connexins has been observed in the inflamed lesions in multiple sclerosis (MS) and an animal model of this disease, experimental autoimmune encephalomyelitis (EAE). In particular, downregulation of oligodendrocytic Cx32 and Cx47 and astrocytic Cx43 have been observed in the active lesions of MS patients and EAE mice (Brand-Schieber et al., 2005; Eugenin et al., 2012; Markoullis et al., 2012). Expression levels of Cx47 and Cx32 were upregulated during remyelination, but downregulated in the relapsing phase, and Cx32 deletion resulted in exacerbates symptoms in EAE, specifically increased demyelination and axonal loss (Markoullis et al., 2012). Whereas mice lacking astrocytic expression of Cx43/Cx30 exhibited white-matter vacuolation and hypomyelination, the severity of EAE in these animals was similar to that in wild-type mice (Lutz et al., 2012). Therefore, oligodendrocytic expression levels of Cx32 and Cx47 appear to be associated with the degree of damage and remyelination, whereas astrocytic expression levels of Cx43 do not. However, recent studies showed that a loss of Cx43 in astrocytes precedes demyelination in the MS-related disorders neuromyelitis optica and Balo's disease (Matsushita et al., 2011; Masaki et al., 2012), suggesting that the temporal expressional pattern of astrocytic Cx43 plays a significant role in the disease process.
Accumulating evidence has also implicated neuroinflammation, including gliosis by activated astrocytes and microglia, in the pathogenesis of such neurodegenerative diseases as HIV encephalopathy, AD, PD, and ALS (Glass et al., 2010; Valcour et al., 2011). Microglial activation followed by astrocytic activation is the earliest pathologic feature in the pre-symptomatic phases of these diseases. Our recent studies have shown that activated microglia release excess glutamate through Cx32 hemichannels, resulting in excitotoxic neuronal death (Takeuchi et al., 2006, 2008a; Yawata et al., 2008). Furthermore, microglia-derived glutamate and pro-inflammatory cytokines induce dysfunction of gap junctions and hemichannels in astrocytes (Kielian, 2008), thereby potentially disrupting CNS homeostasis. On the other hand, reactive astrocytes neighboring amyloid β (Aβ) plaques in the brains of AD patients expressed elevated levels of Cx43 and Cx30 (Koulakoff et al., 2012). Aβ peptide induces the release of glutamate and ATP via uncoupled hemichannels in microglia and astrocytes, leading to neuronal death (Orellana et al., 2011). Corroborating this observation, blockade of gap junctions/hemichannels improved memory impairment in a mouse model of AD (Takeuchi et al., 2011). Recent studies also revealed that astrocytic gap junctions/hemichannels are involved in the disease progression of HIV encephalopathy (Eugenin and Berman, 2013; Orellana et al., 2014). PD animal models (MTPT-treated mice and rotenone-treated rats) exhibited upregulation of astrocytic Cx43 expression in affected areas (Rufer et al., 1996; Kawasaki et al., 2009), and a gap junction/hemichannel blocker ameliorated the disease symptoms of a PD mouse model (Suzuki et al., 2014). A recent report revealed that α-synuclein directly binds Cx32, and that overexpression of α-synuclein suppresses the activity of Cx32 in the SH-SY5Y dopaminergic neuroblastoma cell line (Sung et al., 2007). Other studies have shown that microglia and astrocytes are determinants of disease progression in ALS (the non-autonomous neuronal death hypothesis) (Boillee et al., 2006; Yamanaka et al., 2008). Activation of microglia and astrocytes is associated with elevated expression of gap junctions and hemichannels (Cui et al., 2014). In fact, treatment with a gap junction/hemichannel blocker ameliorated disease progression in a mouse model of ALS (Takeuchi et al., 2011). Juvenile neuronal ceroid lipofuscinosis (JNCL) also shows the activation of microglia and astrocytes preceding neuronal loss (Pontikis et al., 2005; Xiong and Kielian, 2013), and treatment with a gap junction/hemichannel blocker attenuated the disease symptoms of a JNCL mouse model (Burkovetskaya et al., 2014). Few reports, however, have focused on the expression profiles and functions of connexins in these diseases. Further studies are needed to elucidate the precise role of glial connexins in the pathogenesis of these diseases.
Conclusions
A growing body of evidence has demonstrated the pathologic roles of gap junctions and hemichannels in various neurologic diseases. For example, dysfunction and dysregulation of gap junctions and hemichannels in glial cells contribute to neuroinflammation in the CNS, which results in neuronal damage (a situation in which glial cells are “bad neighbors” of neurons) (Block et al., 2007). Despite recent progress in elucidating the pathological roles of gap junctions and hemichannels, many challenges remain, due in part to technical limitations. For instance, few high-quality antibodies against each connexin are available for immunostaining and immunoblotting. Moreover, reagents that are commonly used to block connexin channels are not specific for those channels. In fact, connexin channel blockers such as glycyrrhetinic acid, its derivative carbenoxolone, niflumic acid, and octanol also block pannexin channels. Although the most specific gap junction and hemichannel blockers currently available are mimetic peptides with sequences very similar to that of the extracellular loop of connexins, recent studies showed that mimetic peptides specific for Cx32 (32gap 24 and 32gap 27), Cx43 (43gap 27), or Panx1 (10panx1) non-specifically block both connexins and pannexins (Wang et al., 2007). Although aptamers and siRNA may be used as blockers for specific connexins (Knieps et al., 2007; Xu et al., 2014), they still have a problem of the blood–brain barrier penetration. The heterogeneity of gap-junction and hemichannel configurations (Figure 2) and the ability of various connexins to compensate for the loss of other isoforms (e.g., in connexin-knockout studies) also complicate analysis of this system. Although EGFP-tagged connexins have facilitated live-cell imaging, tagging and/or overexpression of connexins in cultured cells often produce abnormally large gap-junction plaques (Lopez et al., 2001; Gaietta et al., 2002; Hunter et al., 2003). Moreover, tagging the amino-termini of connexins results in non-functional channels, whereas tagging the carboxyl-termini alters the properties of the channels (Bukauskas et al., 2000; Contreras et al., 2003). Therefore, future investigations should attempt to elucidate the spatiotemporal expression profiles of connexin isoforms under pathological conditions in the CNS; this work will require development of specific blockers and tracers for each connexin isoform, hemichannel, and gap junction. Understanding the precise pathologic roles of gap junctions and hemichannels may lead to new therapeutic strategies against multiple chronic neurodegenerative diseases.
Conflict of Interest Statement
The authors declare that the research was conducted in the absence of any commercial or financial relationships that could be construed as a potential conflict of interest.
Acknowledgments
This work was supported by the Program for Promotion of Fundamental Studies in Health Sciences of the National Institute of Biomedical Innovation (NIBIO); grants from the Ministry of Health, Labour and Welfare of Japan; a grant-in-aid for Scientific Research on Innovative Areas; and a grant-in-aid for the Global Center of Excellence Program from the Ministry of Education, Culture, Sports, Science and Technology of Japan.
References
Aarum, J., Sandberg, K., Haeberlein, S. L., and Persson, M. A. (2003). Migration and differentiation of neural precursor cells can be directed by microglia. Proc. Natl. Acad. Sci. U.S.A. 100, 15983–15988. doi: 10.1073/pnas.2237050100
Akiyama, H., and McGeer, P. L. (1990). Brain microglia constitutively express beta-2 integrins. J. Neuroimmunol. 30, 81–93. doi: 10.1016/0165-5728(90)90055-R
Allen, K., Fuchs, E. C., Jaschonek, H., Bannerman, D. M., and Monyer, H. (2011). Gap junctions between interneurons are required for normal spatial coding in the hippocampus and short-term spatial memory. J. Neurosci. 31, 6542–6552. doi: 10.1523/JNEUROSCI.6512-10.2011
Al-Ubaidi, M. R., White, T. W., Ripps, H., Poras, I., Avner, P., Gomes, D., et al. (2000). Functional properties, developmental regulation, and chromosomal localization of murine connexin36, a gap-junctional protein expressed preferentially in retina and brain. J. Neurosci. Res. 59, 813–826. doi: 10.1002/(SICI)1097-4547(20000315)59:6<813::AID-JNR14>3.0.CO;2-#
Altevogt, B. M., Kleopa, K. A., Postma, F. R., Scherer, S. S., and Paul, D. L. (2002). Connexin29 is uniquely distributed within myelinating glial cells of the central and peripheral nervous systems. J. Neurosci. 22, 6458–6470.
Altevogt, B. M., and Paul, D. L. (2004). Four classes of intercellular channels between glial cells in the CNS. J. Neurosci. 24, 4313–4323. doi: 10.1523/JNEUROSCI.3303-03.2004
Alvarez-Maubecin, V., Garcia-Hernandez, F., Williams, J. T., and Van Bockstaele, E. J. (2000). Functional coupling between neurons and glia. J. Neurosci. 20, 4091–4098.
Anderson, C. M., and Swanson, R. A. (2000). Astrocyte glutamate transport: review of properties, regulation, and physiological functions. Glia 32, 1–14. doi: 10.1002/1098-1136(200010)32:1<1::AID-GLIA10>3.0.CO;2-W
Anzini, P., Neuberg, D. H., Schachner, M., Nelles, E., Willecke, K., Zielasek, J., et al. (1997). Structural abnormalities and deficient maintenance of peripheral nerve myelin in mice lacking the gap junction protein connexin 32. J. Neurosci. 17, 4545–4551.
Bamberger, M. E., Harris, M. E., McDonald, D. R., Husemann, J., and Landreth, G. E. (2003). A cell surface receptor complex for fibrillar beta-amyloid mediates microglial activation. J. Neurosci. 23, 2665–2674.
Bani-Yaghoub, M., Bechberger, J. F., Underhill, T. M., and Naus, C. C. (1999a). The effects of gap junction blockage on neuronal differentiation of human NTera2/clone D1 cells. Exp. Neurol. 156, 16–32. doi: 10.1006/exnr.1998.6950
Bani-Yaghoub, M., Underhill, T. M., and Naus, C. C. (1999b). Gap junction blockage interferes with neuronal and astroglial differentiation of mouse P19 embryonal carcinoma cells. Dev. Genet. 24, 69–81. doi: 10.1002/(SICI)1520-6408(1999)24:1/2<69::AID-DVG8>3.0.CO;2-M
Barbe, M. T., Monyer, H., and Bruzzone, R. (2006). Cell-cell communication beyond connexins: the pannexin channels. Physiology (Bethesda) 21, 103–114. doi: 10.1152/physiol.00048.2005
Barger, S. W., and Basile, A. S. (2001). Activation of microglia by secreted amyloid precursor protein evokes release of glutamate by cystine exchange and attenuates synaptic function. J. Neurochem. 76, 846–854. doi: 10.1046/j.1471-4159.2001.00075.x
Bessis, A., Bechade, C., Bernard, D., and Roumier, A. (2007). Microglial control of neuronal death and synaptic properties. Glia 55, 233–238. doi: 10.1002/glia.20459
Bittman, K. S., and Loturco, J. J. (1999). Differential regulation of connexin 26 and 43 in murine neocortical precursors. Cereb. Cortex 9, 188–195. doi: 10.1093/cercor/9.2.188
Block, M. L., Zecca, L., and Hong, J. S. (2007). Microglia-mediated neurotoxicity: uncovering the molecular mechanisms. Nat. Rev. Neurosci. 8, 57–69. doi: 10.1038/nrn2038
Boche, D., Perry, V. H., and Nicoll, J. A. (2013). Review: activation patterns of microglia and their identification in the human brain. Neuropathol. Appl. Neurobiol. 39, 3–18. doi: 10.1111/nan.12011
Boillee, S., Yamanaka, K., Lobsiger, C. S., Copeland, N. G., Jenkins, N. A., Kassiotis, G., et al. (2006). Onset and progression in inherited ALS determined by motor neurons and microglia. Science 312, 1389–1392. doi: 10.1126/science.1123511
Bouskila, Y., and Dudek, F. E. (1993). Neuronal synchronization without calcium-dependent synaptic transmission in the hypothalamus. Proc. Natl. Acad. Sci. U.S.A. 90, 3207–3210. doi: 10.1073/pnas.90.8.3207
Brand-Schieber, E., Werner, P., Iacobas, D. A., Iacobas, S., Beelitz, M., Lowery, S. L., et al. (2005). Connexin43, the major gap junction protein of astrocytes, is down-regulated in inflamed white matter in an animal model of multiple sclerosis. J. Neurosci. Res. 80, 798–808. doi: 10.1002/jnr.20474
Bruijn, L. I., Miller, T. M., and Cleveland, D. W. (2004). Unraveling the mechanisms involved in motor neuron degeneration in ALS. Annu. Rev. Neurosci. 27, 723–749. doi: 10.1146/annurev.neuro.27.070203.144244
Bsibsi, M., Ravid, R., Gveric, D., and Van Noort, J. M. (2002). Broad expression of Toll-like receptors in the human central nervous system. J. Neuropathol. Exp. Neurol. 61, 1013–1021.
Bukauskas, F. F., Jordan, K., Bukauskiene, A., Bennett, M. V., Lampe, P. D., Laird, D. W., et al. (2000). Clustering of connexin 43-enhanced green fluorescent protein gap junction channels and functional coupling in living cells. Proc. Natl. Acad. Sci. U.S.A. 97, 2556–2561. doi: 10.1073/pnas.050588497
Burkovetskaya, M., Karpuk, N., Xiong, J., Bosch, M., Boska, M. D., Takeuchi, H., et al. (2014). Evidence for Aberrant Astrocyte Hemichannel Activity in Juvenile Neuronal Ceroid Lipofuscinosis (JNCL). PLoS ONE 9:e95023. doi: 10.1371/journal.pone.0095023
Cagnin, A., Brooks, D. J., Kennedy, A. M., Gunn, R. N., Myers, R., Turkheimer, F. E., et al. (2001). In-vivo measurement of activated microglia in dementia. Lancet 358, 461–467. doi: 10.1016/S0140-6736(01)05625-2
Carpentier, P. A., Duncan, D. S., and Miller, S. D. (2008). Glial toll-like receptor signaling in central nervous system infection and autoimmunity. Brain Behav. Immun. 22, 140–147. doi: 10.1016/j.bbi.2007.08.011
Chang, Q., Gonzalez, M., Pinter, M. J., and Balice-Gordon, R. J. (1999). Gap junctional coupling and patterns of connexin expression among neonatal rat lumbar spinal motor neurons. J. Neurosci. 19, 10813–10828.
Charles, A. (1998). Intercellular calcium waves in glia. Glia 24, 39–49. doi: 10.1002/(SICI)1098-1136(199809)24:1<39::AID-GLIA5>3.0.CO;2-W
Cho, S., Park, E. M., Febbraio, M., Anrather, J., Park, L., Racchumi, G., et al. (2005). The class B scavenger receptor CD36 mediates free radical production and tissue injury in cerebral ischemia. J. Neurosci. 25, 2504–2512. doi: 10.1523/JNEUROSCI.0035-05.2005
Christie, M. J., Williams, J. T., and North, R. A. (1989). Electrical coupling synchronizes subthreshold activity in locus coeruleus neurons in vitro from neonatal rats. J. Neurosci. 9, 3584–3589.
Contreras, J. E., Saez, J. C., Bukauskas, F. F., and Bennett, M. V. (2003). Gating and regulation of connexin 43 (Cx43) hemichannels. Proc. Natl. Acad. Sci. U.S.A. 100, 11388–11393. doi: 10.1073/pnas.1434298100
Coraci, I. S., Husemann, J., Berman, J. W., Hulette, C., Dufour, J. H., Campanella, G. K., et al. (2002). CD36, a class B scavenger receptor, is expressed on microglia in Alzheimer's disease brains and can mediate production of reactive oxygen species in response to beta-amyloid fibrils. Am. J. Pathol. 160, 101–112. doi: 10.1016/S0002-9440(10)64354-4
Cui, Y., Masaki, K., Yamasaki, R., Imamura, S., Suzuki, S. O., Hayashi, S., et al. (2014). Extensive dysregulations of oligodendrocytic and astrocytic connexins are associated with disease progression in an amyotrophic lateral sclerosis mouse model. J. Neuroinflammation 11:42. doi: 10.1186/1742-2094-11-42
Dahl, G., and Locovei, S. (2006). Pannexin: to gap or not to gap, is that a question? IUBMB Life 58, 409–419. doi: 10.1080/15216540600794526
Davalos, D., Grutzendler, J., Yang, G., Kim, J. V., Zuo, Y., Jung, S., et al. (2005). ATP mediates rapid microglial response to local brain injury in vivo. Nat. Neurosci. 8, 752–758. doi: 10.1038/nn1472
Deans, M. R., Gibson, J. R., Sellitto, C., Connors, B. W., and Paul, D. L. (2001). Synchronous activity of inhibitory networks in neocortex requires electrical synapses containing connexin36. Neuron 31, 477–485. doi: 10.1016/S0896-6273(01)00373-7
Delisle, M. B., and Carpenter, S. (1984). Neurofibrillary axonal swellings and amyotrophic lateral sclerosis. J. Neurol. Sci. 63, 241–250. doi: 10.1016/0022-510X(84)90199-0
Del Rio-Hortega, P. (1932). Cytology and Cellular Pathology of the Nervous System. New York, NY: Penfield Wed.
De Pina-Benabou, M. H., Szostak, V., Kyrozis, A., Rempe, D., Uziel, D., Urban-Maldonado, M., et al. (2005). Blockade of gap junctions in vivo provides neuroprotection after perinatal global ischemia. Stroke 36, 2232–2237. doi: 10.1161/01.STR.0000182239.75969.d8
Dermietzel, R., Gao, Y., Scemes, E., Vieira, D., Urban, M., Kremer, M., et al. (2000). Connexin43 null mice reveal that astrocytes express multiple connexins. Brain Res. Brain Res. Rev. 32, 45–56. doi: 10.1016/S0165-0173(99)00067-3
Dermietzel, R., Hertberg, E. L., Kessler, J. A., and Spray, D. C. (1991). Gap junctions between cultured astrocytes: immunocytochemical, molecular, and electrophysiological analysis. J. Neurosci. 11, 1421–1432.
Dermietzel, R., Traub, O., Hwang, T. K., Beyer, E., Bennett, M. V., Spray, D. C., et al. (1989). Differential expression of three gap junction proteins in developing and mature brain tissues. Proc. Natl. Acad. Sci. U.S.A. 86, 10148–10152. doi: 10.1073/pnas.86.24.10148
De Vuyst, E., Decrock, E., De Bock, M., Yamasaki, H., Naus, C. C., Evans, W. H., et al. (2007). Connexin hemichannels and gap junction channels are differentially influenced by lipopolysaccharide and basic fibroblast growth factor. Mol. Biol. Cell 18, 34–46. doi: 10.1091/mbc.E06-03-0182
Dickson, T. C., King, C. E., McCormack, G. H., and Vickers, J. C. (1999). Neurochemical diversity of dystrophic neurites in the early and late stages of Alzheimer's disease. Exp. Neurol. 156, 100–110. doi: 10.1006/exnr.1998.7010
Dutta, R., and Trapp, B. D. (2007). Pathogenesis of axonal and neuronal damage in multiple sclerosis. Neurology 68, S22–S31; discussion S43–S54.
Eikelenboom, P., Bate, C., Van Gool, W. A., Hoozemans, J. J., Rozemuller, J. M., Veerhuis, R., et al. (2002). Neuroinflammation in Alzheimer's disease and prion disease. Glia 40, 232–239. doi: 10.1002/glia.10146
El Khoury, J., Hickman, S. E., Thomas, C. A., Cao, L., Silverstein, S. C., and Loike, J. D. (1996). Scavenger receptor-mediated adhesion of microglia to beta-amyloid fibrils. Nature 382, 716–719. doi: 10.1038/382716a0
El Khoury, J., Hickman, S. E., Thomas, C. A., Loike, J. D., and Silverstein, S. C. (1998). Microglia, scavenger receptors, and the pathogenesis of Alzheimer's disease. Neurobiol. Aging 19, S81–S84. doi: 10.1016/S0197-4580(98)00036-0
Eugenin, E. A., Basilio, D., Saez, J. C., Orellana, J. A., Raine, C. S., Bukauskas, F., et al. (2012). The role of gap junction channels during physiologic and pathologic conditions of the human central nervous system. J. Neuroimmune Pharmacol. 7, 499–518. doi: 10.1007/s11481-012-9352-5
Eugenin, E. A., and Berman, J. W. (2013). Cytochrome c dysregulation induced by HIV infection of astrocytes results in bystander apoptosis of uninfected astrocytes by an IP3 and calcium-dependent mechanism. J. Neurochem. 127, 644–651. doi: 10.1111/jnc.12443
Eugenin, E. A., Eckardt, D., Theis, M., Willecke, K., Bennett, M. V., and Saez, J. C. (2001). Microglia at brain stab wounds express connexin 43 and in vitro form functional gap junctions after treatment with interferon-gamma and tumor necrosis factor-alpha. Proc. Natl. Acad. Sci. U.S.A. 98, 4190–4195. doi: 10.1073/pnas.051634298
Frank, M., Eiberger, B., Janssen-Bienhold, U., De Sevilla Muller, L. P., Tjarks, A., Kim, J. S., et al. (2010). Neuronal connexin-36 can functionally replace connexin-45 in mouse retina but not in the developing heart. J. Cell Sci. 123, 3605–3615. doi: 10.1242/jcs.068668
Frantseva, M. V., Kokarovtseva, L., and Perez Velazquez, J. L. (2002). Ischemia-induced brain damage depends on specific gap-junctional coupling. J. Cereb. Blood Flow Metab. 22, 453–462. doi: 10.1097/00004647-200204000-00009
Fushiki, S., Perez Velazquez, J. L., Zhang, L., Bechberger, J. F., Carlen, P. L., and Naus, C. C. (2003). Changes in neuronal migration in neocortex of connexin43 null mutant mice. J. Neuropathol. Exp. Neurol. 62, 304–314.
Gaietta, G., Deerinck, T. J., Adams, S. R., Bouwer, J., Tour, O., Laird, D. W., et al. (2002). Multicolor and electron microscopic imaging of connexin trafficking. Science 296, 503–507. doi: 10.1126/science.1068793
Garg, S., Md Syed, M., and Kielian, T. (2005). Staphylococcus aureus-derived peptidoglycan induces Cx43 expression and functional gap junction intercellular communication in microglia. J. Neurochem. 95, 475–483. doi: 10.1111/j.1471-4159.2005.03384.x
Ghezzi, P., and Mennini, T. (2001). Tumor necrosis factor and motoneuronal degeneration: an open problem. Neuroimmunomodulation 9, 178–182. doi: 10.1159/000049024
Giaume, C., Tabernero, A., and Medina, J. M. (1997). Metabolic trafficking through astrocytic gap junctions. Glia 21, 114–123. doi: 10.1002/(SICI)1098-1136(199709)21:1<114::AID-GLIA13>3.0.CO;2-V
Glass, C. K., Saijo, K., Winner, B., Marchetto, M. C., and Gage, F. H. (2010). Mechanisms underlying inflammation in neurodegeneration. Cell 140, 918–934. doi: 10.1016/j.cell.2010.02.016
Goel, S., Wharton, S. B., Brett, L. P., and Whittle, I. R. (2003). Morphological changes and stress responses in neurons in cerebral cortex infiltrated by diffuse astrocytoma. Neuropathology 23, 262–270. doi: 10.1046/j.1440-1789.2003.00510.x
Goldberg, G. S., Lampe, P. D., and Nicholson, B. J. (1999). Selective transfer of endogenous metabolites through gap junctions composed of different connexins. Nat. Cell Biol. 1, 457–459. doi: 10.1038/15693
Goldberg, G. S., Moreno, A. P., and Lampe, P. D. (2002). Gap junctions between cells expressing connexin 43 or 32 show inverse permselectivity to adenosine and ATP. J. Biol. Chem. 277, 36725–36730. doi: 10.1074/jbc.M109797200
Goldberg, G. S., Valiunas, V., and Brink, P. R. (2004). Selective permeability of gap junction channels. Biochim. Biophys. Acta 1662, 96–101. doi: 10.1016/j.bbamem.2003.11.022
Grewal, R. P., Yoshida, T., Finch, C. E., and Morgan, T. E. (1997). Scavenger receptor mRNAs in rat brain microglia are induced by kainic acid lesioning and by cytokines. Neuroreport 8, 1077–1081. doi: 10.1097/00001756-199703240-00003
Harris, A. L. (2001). Emerging issues of connexin channels: biophysics fills the gap. Q. Rev. Biophys. 34, 325–472. doi: 10.1017/S0033583501003705
Harris, A. L. (2007). Connexin channel permeability to cytoplasmic molecules. Prog. Biophys. Mol. Biol. 94, 120–143. doi: 10.1016/j.pbiomolbio.2007.03.011
Hartfield, E. M., Rinaldi, F., Glover, C. P., Wong, L. F., Caldwell, M. A., and Uney, J. B. (2011). Connexin 36 expression regulates neuronal differentiation from neural progenitor cells. PLoS ONE 6:e14746. doi: 10.1371/journal.pone.0014746
Henkel, J. S., Beers, D. R., Zhao, W., and Appel, S. H. (2009). Microglia in ALS: the good, the bad, and the resting. J. Neuroimmune Pharmacol. 4, 389–398. doi: 10.1007/s11481-009-9171-5
Herman, M. A., and Jahr, C. E. (2007). Extracellular glutamate concentration in hippocampal slice. J. Neurosci. 27, 9736–9741. doi: 10.1523/JNEUROSCI.3009-07.2007
Hori, N., and Carpenter, D. O. (1994). Functional and morphological changes induced by transient in vivo ischemia. Exp. Neurol. 129, 279–289. doi: 10.1006/exnr.1994.1170
Hormuzdi, S. G., Pais, I., Lebeau, F. E., Towers, S. K., Rozov, A., Buhl, E. H., et al. (2001). Impaired electrical signaling disrupts gamma frequency oscillations in connexin 36-deficient mice. Neuron 31, 487–495. doi: 10.1016/S0896-6273(01)00387-7
Huang, C., Han, X., Li, X., Lam, E., Peng, W., Lou, N., et al. (2012). Critical role of connexin 43 in secondary expansion of traumatic spinal cord injury. J. Neurosci. 32, 3333–3338. doi: 10.1523/JNEUROSCI.1216-11.2012
Hunter, A. W., Jourdan, J., and Gourdie, R. G. (2003). Fusion of GFP to the carboxyl terminus of connexin43 increases gap junction size in HeLa cells. Cell Commun. Adhes. 10, 211–214. doi: 10.1080/714040429
Iribarren, P., Chen, K., Hu, J., Gong, W., Cho, E. H., Lockett, S., et al. (2005). CpG-containing oligodeoxynucleotide promotes microglial cell uptake of amyloid beta 1-42 peptide by up-regulating the expression of the G-protein- coupled receptor mFPR2. FASEB J. 19, 2032–2034.
Jefferys, J. G. (1995). Nonsynaptic modulation of neuronal activity in the brain: electric currents and extracellular ions. Physiol. Rev. 75, 689–723.
Jimenez, S., Baglietto-Vargas, D., Caballero, C., Moreno-Gonzalez, I., Torres, M., Sanchez-Varo, R., et al. (2008). Inflammatory response in the hippocampus of PS1M146L/APP751SL mouse model of Alzheimer's disease: age-dependent switch in the microglial phenotype from alternative to classic. J. Neurosci. 28, 11650–11661. doi: 10.1523/JNEUROSCI.3024-08.2008
Kalogeris, T., Baines, C. P., Krenz, M., and Korthuis, R. J. (2012). Cell biology of ischemia/reperfusion injury. Int. Rev. Cell Mol. Biol. 298, 229–317. doi: 10.1016/B978-0-12-394309-5.00006-7
Kamata, H., Honda, S., Maeda, S., Chang, L., Hirata, H., and Karin, M. (2005). Reactive oxygen species promote TNFalpha-induced death and sustained JNK activation by inhibiting MAP kinase phosphatases. Cell 120, 649–661. doi: 10.1016/j.cell.2004.12.041
Kawanokuchi, J., Mizuno, T., Takeuchi, H., Kato, H., Wang, J., Mitsuma, N., et al. (2006). Production of interferon-gamma by microglia. Mult. Scler. 12, 558–564. doi: 10.1177/1352458506070763
Kawasaki, A., Hayashi, T., Nakachi, K., Trosko, J. E., Sugihara, K., Kotake, Y., et al. (2009). Modulation of connexin 43 in rotenone-induced model of Parkinson's disease. Neuroscience 160, 61–68. doi: 10.1016/j.neuroscience.2009.01.080
Kempermann, G., and Neumann, H. (2003). Neuroscience. Microglia: the enemy within? Science 302, 1689–1690. doi: 10.1126/science.1092864
Kielian, T. (2008). Glial connexins and gap junctions in CNS inflammation and disease. J. Neurochem. 106, 1000–1016. doi: 10.1111/j.1471-4159.2008.05405.x
Kipnis, J., Avidan, H., Caspi, R. R., and Schwartz, M. (2004). Dual effect of CD4+CD25+ regulatory T cells in neurodegeneration: a dialogue with microglia. Proc. Natl. Acad. Sci. U.S.A. 101(Suppl. 2), 14663–14669. doi: 10.1073/pnas.0404842101
Knieps, M., Herrmann, S., Lehmann, C., Loer, B., Hoch, M., and Famulok, M. (2007). Anti-innexin 2 aptamers specifically inhibit the heterologous interaction of the innexin 2 and innexin 3 carboxyl-termini in vitro. Biol. Chem. 388, 561–568. doi: 10.1515/BC.2007.074
Koenigsknecht, J., and Landreth, G. (2004). Microglial phagocytosis of fibrillar beta-amyloid through a beta1 integrin-dependent mechanism. J. Neurosci. 24, 9838–9846. doi: 10.1523/JNEUROSCI.2557-04.2004
Koulakoff, A., Mei, X., Orellana, J. A., Saez, J. C., and Giaume, C. (2012). Glial connexin expression and function in the context of Alzheimer's disease. Biochim. Biophys. Acta 1818, 2048–2057. doi: 10.1016/j.bbamem.2011.10.001
Kreutzberg, G. W. (1996). Microglia: a sensor for pathological events in the CNS. Trends Neurosci. 19, 312–318. doi: 10.1016/0166-2236(96)10049-7
Laird, D. W. (2006). Life cycle of connexins in health and disease. Biochem. J. 394, 527–543. doi: 10.1042/BJ20051922
Laird, D. W. (2010). The gap junction proteome and its relationship to disease. Trends Cell Biol. 20, 92–101. doi: 10.1016/j.tcb.2009.11.001
Lawson, L. J., Perry, V. H., Dri, P., and Gordon, S. (1990). Heterogeneity in the distribution and morphology of microglia in the normal adult mouse brain. Neuroscience 39, 151–170. doi: 10.1016/0306-4522(90)90229-W
Leithe, E., and Rivedal, E. (2007). Ubiquitination of gap junction proteins. J. Membr. Biol. 217, 43–51. doi: 10.1007/s00232-007-9050-z
Liang, J., Takeuchi, H., Doi, Y., Kawanokuchi, J., Sonobe, Y., Jin, S., et al. (2008). Excitatory amino acid transporter expression by astrocytes is neuroprotective against microglial excitotoxicity. Brain Res. 1210, 11–19. doi: 10.1016/j.brainres.2008.03.012
Liang, J., Takeuchi, H., Jin, S., Noda, M., Li, H., Doi, Y., et al. (2010). Glutamate induces neurotrophic factor production from microglia via protein kinase C pathway. Brain Res. 1322, 8–23. doi: 10.1016/j.brainres.2010.01.083
Liu, Y., Walter, S., Stagi, M., Cherny, D., Letiembre, M., Schulz-Schaeffer, W., et al. (2005). LPS receptor (CD14): a receptor for phagocytosis of Alzheimer's amyloid peptide. Brain 128, 1778–1789. doi: 10.1093/brain/awh531
Lo, C. W., Waldo, K. L., and Kirby, M. L. (1999). Gap junction communication and the modulation of cardiac neural crest cells. Trends Cardiovasc. Med. 9, 63–69. doi: 10.1016/S1050-1738(99)00015-8
Lobsiger, C. S., and Cleveland, D. W. (2007). Glial cells as intrinsic components of non-cell-autonomous neurodegenerative disease. Nat. Neurosci. 10, 1355–1360. doi: 10.1038/nn1988
Lopez, P., Balicki, D., Buehler, L. K., Falk, M. M., and Chen, S. C. (2001). Distribution and dynamics of gap junction channels revealed in living cells. Cell Commun. Adhes. 8, 237–242. doi: 10.3109/15419060109080730
Lutz, S. E., Raine, C. S., and Brosnan, C. F. (2012). Loss of astrocyte connexins 43 and 30 does not significantly alter susceptibility or severity of acute experimental autoimmune encephalomyelitis in mice. J. Neuroimmunol. 245, 8–14. doi: 10.1016/j.jneuroim.2012.01.007
Mantovani, A., Sozzani, S., Locati, M., Allavena, P., and Sica, A. (2002). Macrophage polarization: tumor-associated macrophages as a paradigm for polarized M2 mononuclear phagocytes. Trends Immunol. 23, 549–555. doi: 10.1016/S1471-4906(02)02302-5
Marin-Teva, J. L., Dusart, I., Colin, C., Gervais, A., Van Rooijen, N., and Mallat, M. (2004). Microglia promote the death of developing Purkinje cells. Neuron 41, 535–547. doi: 10.1016/S0896-6273(04)00069-8
Markoullis, K., Sargiannidou, I., Gardner, C., Hadjisavvas, A., Reynolds, R., and Kleopa, K. A. (2012). Disruption of oligodendrocyte gap junctions in experimental autoimmune encephalomyelitis. Glia 60, 1053–1066. doi: 10.1002/glia.22334
Masaki, K., Suzuki, S. O., Matsushita, T., Yonekawa, T., Matsuoka, T., Isobe, N., et al. (2012). Extensive loss of connexins in Balo's disease: evidence for an auto-antibody-independent astrocytopathy via impaired astrocyte-oligodendrocyte/myelin interaction. Acta Neuropathol. 123, 887–900. doi: 10.1007/s00401-012-0972-x
Matsushita, T., Masaki, K., Suzuki, S., Matsuoka, T., Yonekawa, T., Wu, X. M., et al. (2011). Astrocytopathy in neuromyelitis optica, multiple sclerosis and Balo's disease. Rinsho Shinkeigaku 51, 898–900. doi: 10.5692/clinicalneurol.51.898
Mattila, P. M., Rinne, J. O., Helenius, H., and Roytta, M. (1999). Neuritic degeneration in the hippocampus and amygdala in Parkinson's disease in relation to Alzheimer pathology. Acta Neuropathol. 98, 157–164. doi: 10.1007/s004010051064
McGeer, P. L., and McGeer, E. G. (2002). Inflammatory processes in amyotrophic lateral sclerosis. Muscle Nerve 26, 459–470. doi: 10.1002/mus.10191
Menichella, D. M., Goodenough, D. A., Sirkowski, E., Scherer, S. S., and Paul, D. L. (2003). Connexins are critical for normal myelination in the CNS. J. Neurosci. 23, 5963–5973.
Menichella, D. M., Majdan, M., Awatramani, R., Goodenough, D. A., Sirkowski, E., Scherer, S. S., et al. (2006). Genetic and physiological evidence that oligodendrocyte gap junctions contribute to spatial buffering of potassium released during neuronal activity. J. Neurosci. 26, 10984–10991. doi: 10.1523/JNEUROSCI.0304-06.2006
Mizuno, T., Zhang, G., Takeuchi, H., Kawanokuchi, J., Wang, J., Sonobe, Y., et al. (2008). Interferon-gamma directly induces neurotoxicity through a neuron specific, calcium-permeable complex of IFN-gamma receptor and AMPA GluR1 receptor. FASEB J. 22, 1797–1806. doi: 10.1096/fj.07-099499
Nadarajah, B., Thomaidou, D., Evans, W. H., and Parnavelas, J. G. (1996). Gap junctions in the adult cerebral cortex: regional differences in their distribution and cellular expression of connexins. J. Comp. Neurol. 376, 326–342. doi: 10.1002/(SICI)1096-9861(19961209)376:2<326::AID-CNE13>3.0.CO;2-J
Nagy, J. I., Ionescu, A. V., Lynn, B. D., and Rash, J. E. (2003). Coupling of astrocyte connexins Cx26, Cx30, Cx43 to oligodendrocyte Cx29, Cx32, Cx47: implications from normal and connexin32 knockout mice. Glia 44, 205–218. doi: 10.1002/glia.10278
Nagy, J. I., and Rash, J. E. (2000). Connexins and gap junctions of astrocytes and oligodendrocytes in the CNS. Brain Res. Brain Res. Rev. 32, 29–44. doi: 10.1016/S0165-0173(99)00066-1
Nakanishi, H., and Wu, Z. (2009). Microglia-aging: roles of microglial lysosome- and mitochondria-derived reactive oxygen species in brain aging. Behav. Brain Res. 201, 1–7. doi: 10.1016/j.bbr.2009.02.001
Naus, C. C., Bechberger, J. F., Zhang, Y., Venance, L., Yamasaki, H., Juneja, S. C., et al. (1997). Altered gap junctional communication, intercellular signaling, and growth in cultured astrocytes deficient in connexin43. J. Neurosci. Res. 49, 528–540. doi: 10.1002/(SICI)1097-4547(19970901)49:5<528::AID-JNR3>3.0.CO;2-D
Nelson, P. T., Soma, L. A., and Lavi, E. (2002). Microglia in diseases of the central nervous system. Ann. Med. 34, 491–500. doi: 10.1080/078538902321117698
Newsholme, E. A., and Calder, P. C. (1997). The proposed role of glutamine in some cells of the immune system and speculative consequences for the whole animal. Nutrition 13, 728–730. doi: 10.1016/S0899-9007(97)83034-1
Newsholme, P., and Newsholme, E. A. (1989). Rates of utilization of glucose, glutamine and oleate and formation of end-products by mouse peritoneal macrophages in culture. Biochem. J. 261, 211–218.
Nimmerjahn, A., Kirchhoff, F., and Helmchen, F. (2005). Resting microglial cells are highly dynamic surveillants of brain parenchyma in vivo. Science 308, 1314–1318. doi: 10.1126/science.1110647
Nissim, I. (1999). Newer aspects of glutamine/glutamate metabolism: the role of acute pH changes. Am. J. Physiol. 277, F493–F497.
Odermatt, B., Wellershaus, K., Wallraff, A., Seifert, G., Degen, J., Euwens, C., et al. (2003). Connexin 47 (Cx47)-deficient mice with enhanced green fluorescent protein reporter gene reveal predominant oligodendrocytic expression of Cx47 and display vacuolized myelin in the CNS. J. Neurosci. 23, 4549–4559.
Orellana, J. A., Saez, J. C., Bennett, M. V., Berman, J. W., Morgello, S., and Eugenin, E. A. (2014). HIV increases the release of dickkopf-1 protein from human astrocytes by a Cx43 hemichannel-dependent mechanism. J. Neurochem. 128, 752–763. doi: 10.1111/jnc.12492
Orellana, J. A., Saez, P. J., Shoji, K. F., Schalper, K. A., Palacios-Prado, N., Velarde, V., et al. (2009). Modulation of brain hemichannels and gap junction channels by pro-inflammatory agents and their possible role in neurodegeneration. Antioxid. Redox Signal. 11, 369–399. doi: 10.1089/ars.2008.2130
Orellana, J. A., Shoji, K. F., Abudara, V., Ezan, P., Amigou, E., Saez, P. J., et al. (2011). Amyloid beta-induced death in neurons involves glial and neuronal hemichannels. J. Neurosci. 31, 4962–4977. doi: 10.1523/JNEUROSCI.6417-10.2011
Orr, C. F., Rowe, D. B., and Halliday, G. M. (2002). An inflammatory review of Parkinson's disease. Prog. Neurobiol. 68, 325–340. doi: 10.1016/S0301-0082(02)00127-2
Orthmann-Murphy, J. L., Freidin, M., Fischer, E., Scherer, S. S., and Abrams, C. K. (2007). Two distinct heterotypic channels mediate gap junction coupling between astrocyte and oligodendrocyte connexins. J. Neurosci. 27, 13949–13957. doi: 10.1523/JNEUROSCI.3395-07.2007
Parenti, R., Campisi, A., Vanella, A., and Cicirata, F. (2002). Immunocytochemical and RT-PCR analysis of connexin36 in cultures of mammalian glial cells. Arch. Ital. Biol. 140, 101–108.
Parsons, C. G., Stoffler, A., and Danysz, W. (2007). Memantine: a NMDA receptor antagonist that improves memory by restoration of homeostasis in the glutamatergic system–too little activation is bad, too much is even worse. Neuropharmacology 53, 699–723. doi: 10.1016/j.neuropharm.2007.07.013
Paul, D. L. (1995). New functions for gap junctions. Curr. Opin. Cell Biol. 7, 665–672. doi: 10.1016/0955-0674(95)80108-1
Pavese, N., Gerhard, A., Tai, Y. F., Ho, A. K., Turkheimer, F., Barker, R. A., et al. (2006). Microglial activation correlates with severity in Huntington disease: a clinical and PET study. Neurology 66, 1638–1643. doi: 10.1212/01.wnl.0000222734.56412.17
Pavlidis, M., Stupp, T., Naskar, R., Cengiz, C., and Thanos, S. (2003). Retinal ganglion cells resistant to advanced glaucoma: a postmortem study of human retinas with the carbocyanine dye DiI. Invest. Ophthalmol. Vis. Sci. 44, 5196–5205. doi: 10.1167/iovs.03-0614
Piani, D., Spranger, M., Frei, K., Schaffner, A., and Fontana, A. (1992). Macrophage-induced cytotoxicity of N-methyl-D-aspartate receptor positive neurons involves excitatory amino acids rather than reactive oxygen intermediates and cytokines. Eur. J. Immunol. 22, 2429–2436. doi: 10.1002/eji.1830220936
Pontikis, C. C., Cotman, S. L., Macdonald, M. E., and Cooper, J. D. (2005). Thalamocortical neuron loss and localized astrocytosis in the Cln3Deltaex7/8 knock-in mouse model of Batten disease. Neurobiol. Dis. 20, 823–836. doi: 10.1016/j.nbd.2005.05.018
Ransom, B., Behar, T., and Nedergaard, M. (2003). New roles for astrocytes (stars at last). Trends Neurosci. 26, 520–522. doi: 10.1016/j.tins.2003.08.006
Rash, J. E., Olson, C. O., Davidson, K. G., Yasumura, T., Kamasawa, N., and Nagy, J. I. (2007). Identification of connexin36 in gap junctions between neurons in rodent locus coeruleus. Neuroscience 147, 938–956. doi: 10.1016/j.neuroscience.2007.04.061
Rash, J. E., Yasumura, T., Dudek, F. E., and Nagy, J. I. (2001). Cell-specific expression of connexins and evidence of restricted gap junctional coupling between glial cells and between neurons. J. Neurosci. 21, 1983–2000.
Rawanduzy, A., Hansen, A., Hansen, T. W., and Nedergaard, M. (1997). Effective reduction of infarct volume by gap junction blockade in a rodent model of stroke. J. Neurosurg. 87, 916–920. doi: 10.3171/jns.1997.87.6.0916
Retamal, M. A., Schalper, K. A., Shoji, K. F., Bennett, M. V., and Saez, J. C. (2007). Opening of connexin 43 hemichannels is increased by lowering intracellular redox potential. Proc. Natl. Acad. Sci. U.S.A. 104, 8322–8327. doi: 10.1073/pnas.0702456104
Richard, K. L., Filali, M., Prefontaine, P., and Rivest, S. (2008). Toll-like receptor 2 acts as a natural innate immune receptor to clear amyloid beta 1–42 and delay the cognitive decline in a mouse model of Alzheimer's disease. J. Neurosci. 28, 5784–5793. doi: 10.1523/JNEUROSCI.1146-08.2008
Rock, R. B., Gekker, G., Hu, S., Sheng, W. S., Cheeran, M., Lokensgard, J. R., et al. (2004). Role of microglia in central nervous system infections. Clin. Microbiol. Rev. 17, 942–964. doi: 10.1128/CMR.17.4.942-964.2004
Rouach, N., Avignone, E., Meme, W., Koulakoff, A., Venance, L., Blomstrand, F., et al. (2002). Gap junctions and connexin expression in the normal and pathological central nervous system. Biol. Cell 94, 457–475. doi: 10.1016/S0248-4900(02)00016-3
Rouach, N., Koulakoff, A., Abudara, V., Willecke, K., and Giaume, C. (2008). Astroglial metabolic networks sustain hippocampal synaptic transmission. Science 322, 1551–1555. doi: 10.1126/science.1164022
Rufer, M., Wirth, S. B., Hofer, A., Dermietzel, R., Pastor, A., Kettenmann, H., et al. (1996). Regulation of connexin-43, GFAP, and FGF-2 is not accompanied by changes in astroglial coupling in MPTP-lesioned, FGF-2-treated parkinsonian mice. J. Neurosci. Res. 46, 606–617. doi: 10.1002/(SICI)1097-4547(19961201)46:5<606::AID-JNR9>3.0.CO;2-N
Saez, J. C., Berthoud, V. M., Branes, M. C., Martinez, A. D., and Beyer, E. C. (2003). Plasma membrane channels formed by connexins: their regulation and functions. Physiol. Rev. 83, 1359–1400.
Saito, Y., Kawashima, A., Ruberu, N. N., Fujiwara, H., Koyama, S., Sawabe, M., et al. (2003). Accumulation of phosphorylated alpha-synuclein in aging human brain. J. Neuropathol. Exp. Neurol. 62, 644–654.
Sawada, M. (2009). Neuroprotective and toxic changes in microglia in neurodegenerative disease. Parkinsonism. Relat. Disord. 15(Suppl. 1), S39–S41. doi: 10.1016/S1353-8020(09)70011-2
Scemes, E., Dermietzel, R., and Spray, D. C. (1998). Calcium waves between astrocytes from Cx43 knockout mice. Glia 24, 65–73. doi: 10.1002/(SICI)1098-1136(199809)24:1<65::AID-GLIA7>3.0.CO;2-#
Schwab, J. M., and Schluesener, H. J. (2004). Microglia rules: insights into microglial-neuronal signaling. Cell Death Differ. 11, 1245–1246. doi: 10.1038/sj.cdd.4401487
Schwartz, M., Shaked, I., Fisher, J., Mizrahi, T., and Schori, H. (2003). Protective autoimmunity against the enemy within: fighting glutamate toxicity. Trends Neurosci. 26, 297–302. doi: 10.1016/S0166-2236(03)00126-7
Seabrook, T. J., Jiang, L., Maier, M., and Lemere, C. A. (2006). Minocycline affects microglia activation, Abeta deposition, and behavior in APP-tg mice. Glia 53, 776–782. doi: 10.1002/glia.20338
Simard, A. R., Soulet, D., Gowing, G., Julien, J. P., and Rivest, S. (2006). Bone marrow-derived microglia play a critical role in restricting senile plaque formation in Alzheimer's disease. Neuron 49, 489–502. doi: 10.1016/j.neuron.2006.01.022
Sohl, G., Maxeiner, S., and Willecke, K. (2005). Expression and functions of neuronal gap junctions. Nat. Rev. Neurosci. 6, 191–200. doi: 10.1038/nrn1627
Solan, J. L., and Lampe, P. D. (2009). Connexin43 phosphorylation: structural changes and biological effects. Biochem. J. 419, 261–272. doi: 10.1042/BJ20082319
Stirling, D. P., Khodarahmi, K., Liu, J., McPhail, L. T., McBride, C. B., Steeves, J. D., et al. (2004). Minocycline treatment reduces delayed oligodendrocyte death, attenuates axonal dieback, and improves functional outcome after spinal cord injury. J. Neurosci. 24, 2182–2190. doi: 10.1523/JNEUROSCI.5275-03.2004
Sung, J. Y., Lee, H. J., Jeong, E. I., Oh, Y., Park, J., Kang, K. S., et al. (2007). Alpha-synuclein overexpression reduces gap junctional intercellular communication in dopaminergic neuroblastoma cells. Neurosci. Lett. 416, 289–293. doi: 10.1016/j.neulet.2007.02.025
Sutor, B., Schmolke, C., Teubner, B., Schirmer, C., and Willecke, K. (2000). Myelination defects and neuronal hyperexcitability in the neocortex of connexin 32-deficient mice. Cereb. Cortex 10, 684–697. doi: 10.1093/cercor/10.7.684
Suzuki, H., Ono, K., and Sawada, M. (2014). Protective effect of INI-0602, a gap junction inhibitor, on dopaminergic neurodegeneration of mice with unilateral 6-hydroxydopamine injection. J. Neural Transm. doi: 10.1007/s00702-014-1209-z. [Epub ahead of print].
Suzumura, A., Mezitis, S. G., Gonatas, N. K., and Silberberg, D. H. (1987). MHC antigen expression on bulk isolated macrophage-microglia from newborn mouse brain: induction of Ia antigen expression by gamma-interferon. J. Neuroimmunol. 15, 263–278. doi: 10.1016/0165-5728(87)90121-4
Swann, J. W., Al-Noori, S., Jiang, M., and Lee, C. L. (2000). Spine loss and other dendritic abnormalities in epilepsy. Hippocampus 10, 617–625. doi: 10.1002/1098-1063(2000)10:5<617::AID-HIPO13>3.0.CO;2-R
Tabernero, A., Medina, J. M., and Giaume, C. (2006). Glucose metabolism and proliferation in glia: role of astrocytic gap junctions. J. Neurochem. 99, 1049–1061. doi: 10.1111/j.1471-4159.2006.04088.x
Takahashi, T., Yagishita, S., Amano, N., Yamaoka, K., and Kamei, T. (1997). Amyotrophic lateral sclerosis with numerous axonal spheroids in the corticospinal tract and massive degeneration of the cortex. Acta Neuropathol. 94, 294–299. doi: 10.1007/s004010050707
Takaki, J., Fujimori, K., Miura, M., Suzuki, T., Sekino, Y., and Sato, K. (2012). L-glutamate released from activated microglia downregulates astrocytic L-glutamate transporter expression in neuroinflammation: the ‘collusion’ hypothesis for increased extracellular L-glutamate concentration in neuroinflammation. J. Neuroinflammation 9:275. doi: 10.1186/1742-2094-9-275
Takeuchi, H. (2010). Neurotoxicity by microglia: mechanisms and potential therapeutic strategy. Clin. Exp. Neuroimmunol. 1, 12–21. doi: 10.1111/j.1759-1961.2009.00001.x
Takeuchi, H., Jin, S., Suzuki, H., Doi, Y., Liang, J., Kawanokuchi, J., et al. (2008a). Blockade of microglial glutamate release protects against ischemic brain injury. Exp. Neurol. 214, 144–146. doi: 10.1016/j.expneurol.2008.08.001
Takeuchi, H., Jin, S., Suzuki, H., Doi, Y., Liang, J., Kawanokuchi, J., et al. (2008b). Blockade of microglial glutamate release protects against ischemic brain injury. Exp. Neurol. 214, 144–146. doi: 10.1016/j.expneurol.2008.08.001
Takeuchi, H., Jin, S., Wang, J., Zhang, G., Kawanokuchi, J., Kuno, R., et al. (2006). Tumor necrosis factor-alpha induces neurotoxicity via glutamate release from hemichannels of activated microglia in an autocrine manner. J. Biol. Chem. 281, 21362–21368. doi: 10.1074/jbc.M600504200
Takeuchi, H., Kawanokuchi, J., Mizuno, T., and Suzumura, A. (2014). Expression profile of connexins in the central nervous system. Clini. Exp. Neuroimmunol. doi: 10.1111/cen3.12106. [Epub ahead of print].
Takeuchi, H., Mizoguchi, H., Doi, Y., Jin, S., Noda, M., Liang, J., et al. (2011). Blockade of gap junction hemichannel suppresses disease progression in mouse models of amyotrophic lateral sclerosis and Alzheimer's disease. PLoS ONE 6:e21108. doi: 10.1371/journal.pone.0021108
Takeuchi, H., Mizuno, T., Zhang, G., Wang, J., Kawanokuchi, J., Kuno, R., et al. (2005). Neuritic beading induced by activated microglia is an early feature of neuronal dysfunction toward neuronal death by inhibition of mitochondrial respiration and axonal transport. J. Biol. Chem. 280, 10444–10454. doi: 10.1074/jbc.M413863200
Talaveron, R., Matarredona, E. R., De La Cruz, R. R., Macias, D., Galvez, V., and Pastor, A. M. (2014). Implanted neural progenitor cells regulate glial reaction to brain injury and establish gap junctions with host glial cells. Glia 62, 623–638. doi: 10.1002/glia.22630
Tamura, K., Alessandri, B., Heimann, A., and Kempski, O. (2011). The effect of a gap-junction blocker, carbenoxolone, on ischemic brain injury and cortical spreading depression. Neuroscience 194, 262–271. doi: 10.1016/j.neuroscience.2011.07.043
Teubner, B., Michel, V., Pesch, J., Lautermann, J., Cohen-Salmon, M., Sohl, G., et al. (2003). Connexin30 (Gjb6)-deficiency causes severe hearing impairment and lack of endocochlear potential. Hum. Mol. Genet. 12, 13–21. doi: 10.1093/hmg/ddg001
Teubner, B., Odermatt, B., Guldenagel, M., Sohl, G., Degen, J., Bukauskas, F., et al. (2001). Functional expression of the new gap junction gene connexin47 transcribed in mouse brain and spinal cord neurons. J. Neurosci. 21, 1117–1126.
Thompson, R. J., Zhou, N., and Macvicar, B. A. (2006). Ischemia opens neuronal gap junction hemichannels. Science 312, 924–927. doi: 10.1126/science.1126241
Trapp, B. D., Peterson, J., Ransohoff, R. M., Rudick, R., Mork, S., and Bo, L. (1998). Axonal transection in the lesions of multiple sclerosis. N. Engl. J. Med. 338, 278–285. doi: 10.1056/NEJM199801293380502
Tsacopoulos, M., and Magistretti, P. J. (1996). Metabolic coupling between glia and neurons. J. Neurosci. 16, 877–885.
Umebayashi, D., Natsume, A., Takeuchi, H., Hara, M., Nishimura, Y., Fukuyama, R., et al. (2014). Blockade of gap junction hemichannel protects secondary spinal cord injury from activated microglia-mediated glutamate exito-neurotoxicity. J. Neurotrauma. doi: 10.1089/neu.2013.3223. [Epub ahead of print].
Upender, M. B., and Naegele, J. R. (1999). Activation of microglia during developmentally regulated cell death in the cerebral cortex. Dev. Neurosci. 21, 491–505. doi: 10.1159/000017416
Valcour, V., Sithinamsuwan, P., Letendre, S., and Ances, B. (2011). Pathogenesis of HIV in the central nervous system. Curr. HIV/AIDS Rep. 8, 54–61. doi: 10.1007/s11904-010-0070-4
Valiunas, V., Polosina, Y. Y., Miller, H., Potapova, I. A., Valiuniene, L., Doronin, S., et al. (2005). Connexin-specific cell-to-cell transfer of short interfering RNA by gap junctions. J. Physiol. 568, 459–468. doi: 10.1113/jphysiol.2005.090985
Wallraff, A., Kohling, R., Heinemann, U., Theis, M., Willecke, K., and Steinhauser, C. (2006). The impact of astrocytic gap junctional coupling on potassium buffering in the hippocampus. J. Neurosci. 26, 5438–5447. doi: 10.1523/JNEUROSCI.0037-06.2006
Wallraff, A., Odermatt, B., Willecke, K., and Steinhauser, C. (2004). Distinct types of astroglial cells in the hippocampus differ in gap junction coupling. Glia 48, 36–43. doi: 10.1002/glia.20040
Walz, W., and Hertz, L. (1983). Functional interactions between neurons and astrocytes. II. Potassium homeostasis at the cellular level. Prog. Neurobiol. 20, 133–183. doi: 10.1016/0301-0082(83)90013-8
Wang, J., Ma, M., Locovei, S., Keane, R. W., and Dahl, G. (2007). Modulation of membrane channel currents by gap junction protein mimetic peptides: size matters. Am. J. Physiol. Cell Physiol. 293, C1112–C1119. doi: 10.1152/ajpcell.00097.2007
Wasseff, S. K., and Scherer, S. S. (2014). Activated microglia do not form functional gap junctions in vivo. J. Neuroimmunol. 269, 90–93. doi: 10.1016/j.jneuroim.2014.02.005
Willecke, K., Eiberger, J., Degen, J., Eckardt, D., Romualdi, A., Guldenagel, M., et al. (2002). Structural and functional diversity of connexin genes in the mouse and human genome. Biol. Chem. 383, 725–737. doi: 10.1515/BC.2002.076
Wong, R. O., Chernjavsky, A., Smith, S. J., and Shatz, C. J. (1995). Early functional neural networks in the developing retina. Nature 374, 716–718. doi: 10.1038/374716a0
Wu, D. C., Jackson-Lewis, V., Vila, M., Tieu, K., Teismann, P., Vadseth, C., et al. (2002). Blockade of microglial activation is neuroprotective in the 1-methyl-4-phenyl-1,2,3,6-tetrahydropyridine mouse model of Parkinson disease. J. Neurosci. 22, 1763–1771.
Wu, Z., Tokuda, Y., Zhang, X. W., and Nakanishi, H. (2008). Age-dependent responses of glial cells and leptomeninges during systemic inflammation. Neurobiol. Dis. 32, 543–551. doi: 10.1016/j.nbd.2008.09.002
Xiong, J., and Kielian, T. (2013). Microglia in juvenile neuronal ceroid lipofuscinosis are primed toward a pro-inflammatory phenotype. J. Neurochem. 127, 245–258. doi: 10.1111/jnc.12385
Xu, Q., Cheong, Y. K., He, S. Q., Tiwari, V., Liu, J., Wang, Y., et al. (2014). Suppression of spinal connexin 43 expression attenuates mechanical hypersensitivity in rats after an L5 spinal nerve injury. Neurosci. Lett. 566, 194–199. doi: 10.1016/j.neulet.2014.03.004
Xu, X., Li, W. E., Huang, G. Y., Meyer, R., Chen, T., Luo, Y., et al. (2001). Modulation of mouse neural crest cell motility by N-cadherin and connexin 43 gap junctions. J. Cell Biol. 154, 217–230. doi: 10.1083/jcb.200105047
Yamanaka, K., Chun, S. J., Boillee, S., Fujimori-Tonou, N., Yamashita, H., Gutmann, D. H., et al. (2008). Astrocytes as determinants of disease progression in inherited amyotrophic lateral sclerosis. Nat. Neurosci. 11, 251–253. doi: 10.1038/nn2047
Yawata, I., Takeuchi, H., Doi, Y., Liang, J., Mizuno, T., and Suzumura, A. (2008). Macrophage-induced neurotoxicity is mediated by glutamate and attenuated by glutaminase inhibitors and gap junction inhibitors. Life Sci. 82, 1111–1116. doi: 10.1016/j.lfs.2008.03.010
Ye, Z. C., Wyeth, M. S., Baltan-Tekkok, S., and Ransom, B. R. (2003). Functional hemichannels in astrocytes: a novel mechanism of glutamate release. J. Neurosci. 23, 3588–3596.
Yeager, M., and Harris, A. L. (2007). Gap junction channel structure in the early 21st century: facts and fantasies. Curr. Opin. Cell Biol. 19, 521–528. doi: 10.1016/j.ceb.2007.09.001
Yrjanheikki, J., Keinanen, R., Pellikka, M., Hokfelt, T., and Koistinaho, J. (1998). Tetracyclines inhibit microglial activation and are neuroprotective in global brain ischemia. Proc. Natl. Acad. Sci. U.S.A. 95, 15769–15774. doi: 10.1073/pnas.95.26.15769
Yudkoff, M. (1997). Brain metabolism of branched-chain amino acids. Glia 21, 92–98. doi: 10.1002/(SICI)1098-1136(199709)21:1<92::AID-GLIA10>3.0.CO;2-W
Zhu, S., Stavrovskaya, I. G., Drozda, M., Kim, B. Y., Ona, V., Li, M., et al. (2002). Minocycline inhibits cytochrome c release and delays progression of amyotrophic lateral sclerosis in mice. Nature 417, 74–78. doi: 10.1038/417074a
Zietlow, R., Dunnett, S. B., and Fawcett, J. W. (1999). The effect of microglia on embryonic dopaminergic neuronal survival in vitro: diffusible signals from neurons and glia change microglia from neurotoxic to neuroprotective. Eur. J. Neurosci. 11, 1657–1667. doi: 10.1046/j.1460-9568.1999.00583.x
Ziv, Y., Avidan, H., Pluchino, S., Martino, G., and Schwartz, M. (2006a). Synergy between immune cells and adult neural stem/progenitor cells promotes functional recovery from spinal cord injury. Proc. Natl. Acad. Sci. U.S.A. 103, 13174–13179. doi: 10.1073/pnas.0603747103
Ziv, Y., Ron, N., Butovsky, O., Landa, G., Sudai, E., Greenberg, N., et al. (2006b). Immune cells contribute to the maintenance of neurogenesis and spatial learning abilities in adulthood. Nat. Neurosci. 9, 268–275. doi: 10.1038/nn1629
Zlomuzica, A., Reichinnek, S., Maxeiner, S., Both, M., May, E., Worsdorfer, P., et al. (2010). Deletion of connexin45 in mouse neurons disrupts one-trial object recognition and alters kainate-induced gamma-oscillations in the hippocampus. Physiol. Behav. 101, 245–253. doi: 10.1016/j.physbeh.2010.05.007
Keywords: glutamate, microglia, neuroinflammation, neurodegeneration, gap junction, hemichannel, connexin
Citation: Takeuchi H and Suzumura A (2014) Gap junctions and hemichannels composed of connexins: potential therapeutic targets for neurodegenerative diseases. Front. Cell. Neurosci. 8:189. doi: 10.3389/fncel.2014.00189
Received: 31 March 2014; Paper pending published: 07 April 2014;
Accepted: 19 June 2014; Published online: 02 September 2014.
Edited by:
Juan Andrés Orellana, Pontificia Universidad Católica de Chile, ChileReviewed by:
Juan C. Saez, Universidad Catolica de Chile, ChileGeorg Zoidl, York University, Canada
Eliseo A. Eugenin, Public Health Research Institute, USA
Copyright © 2014 Takeuchi and Suzumura. This is an open-access article distributed under the terms of the Creative Commons Attribution License (CC BY). The use, distribution or reproduction in other forums is permitted, provided the original author(s) or licensor are credited and that the original publication in this journal is cited, in accordance with accepted academic practice. No use, distribution or reproduction is permitted which does not comply with these terms.
*Correspondence: Hideyuki Takeuchi, Department of Neuroimmunology, Research Institute of Environmental Medicine, Nagoya University, Furo-cho, Chikusa-ku, Nagoya 464-8601, Japan e-mail:aHRha2VAcmllbS5uYWdveWEtdS5hYy5qcA==