- Department of Neuroscience, Physiology and Pharmacology, University College London, London, UK
The potency of GABA is vitally important for its primary role in activating GABAA receptors and acting as an inhibitory neurotransmitter. Although numerous laboratories have presented information, directly or indirectly, on GABA potency, it is often difficult to compare across such studies given the inevitable variations in the methods used, the cell types studied, whether native or recombinant receptors are examined, and their relevance to native synaptic and extrasynaptic GABAA receptors. In this review, we list the most relevant isoforms of synaptic and extrasynaptic GABAA receptors that are thought to assemble in surface membranes of neurons in the central nervous system. Using consistent methodology in one cell type, the potencies of the endogenous neurotransmitter GABA are compared across a spectrum of GABAA receptors. The highest potency for GABA is measured when activating extrasynaptic-type α6 subunit-containing receptors, whereas synaptic-type α2β3γ2 and α3β3γ2 receptors exhibited the lowest potency, and other GABAA receptor subtypes that are found both in synaptic and extrasynaptic compartments, showed intermediate sensitivities to GABA. The relatively simple potency relationship between GABA and its target receptors is important as it serves as one of the major determinants of GABAA receptor activation, with consequences for the development of inhibition, either by tonic or phasic mechanisms.
Introduction
The neurotransmitter γ-aminobutyric acid (GABA) targets GABAA and GABAB receptors which, in the mature central nervous system (CNS), provide the main basis for inhibitory neurotransmission and the subsequent integration of neuronal excitation (Luscher and Keller, 2004). When considering how effective an inhibitory GABAergic synapse will be in terms of reducing cell excitation, a number of factors are important. At the presynaptic terminal, this includes the GABA concentration and time profile in the synaptic cleft following GABA release. In addition, GABA overspill from inhibitory synapses to perisynaptic GABAA receptors will also be important. Postsynaptically, other factors come into consideration such as the subunit composition of GABAA receptors; the number and density of these receptors and their targeting to precise inhibitory synaptic compartments; and their residence time at synapses before endocytosis or lateral mobility causes them to exit the synaptic environment – these are all of equal significance (Moss and Smart, 2001; Jacob et al., 2008; Luscher et al., 2011). Many of the above factors will also be relevant to the effectiveness of extrasynaptic GABAA receptors in underpinning tonic inhibition.
One further factor that remains paramount to the effectiveness of GABA in activating specific isoforms of synaptic and extrasynaptic GABAA receptors is the potency of GABA. The activation of the receptor by GABA will depend on several factors, including the speed of the initial binding reaction, the potential for a shut but pre-activated receptor state, the final gating reaction that causes the channel to open, and the potential for the receptor to rapidly enter into one or more desensitized states. These factors will all impact on the potency of GABA to varying extents. Despite knowing that GABA can appear more potent on some receptor isoforms compared to others, what is currently lacking is a controlled and consolidated comparison, under identical conditions, of a series of receptor isoforms that are regarded as physiologically relevant to inhibitory synaptic and extrasynaptic GABAA receptors.
GABAA receptors are pentamers formed from a selection of 19 subunits: α(1–6), β(1–3), γ(1–3), δ, ε, θ, π, and ρ(1–3; Sieghart, 1995; Korpi et al., 2002). Although the potential for receptor diversity in neurons is considerable this is naturally contained by two principle factors: differential gene expression, whereby specific neuronal subtypes usually express a subset of GABAA receptor subunit genes (Wisden et al., 1992; Pirker et al., 2000); and the imposition of receptor subunit assembly rules that cause particular subunits to preferentially co-assemble (Taylor et al., 1999, 2000; Klausberger et al., 2000, 2001), e.g., α6 and δ subunits in cerebellar granule neurons (Jones et al., 1997). Despite the potential for receptor heterogeneity, the majority of GABAA receptors will contain two α subunits, two β subunits, and a γ subunit (Farrar et al., 1999). This is particularly relevant for synaptic αβγ GABAA receptors since a number of intracellular proteins have been shown to interact with these subunits regulating GABAA receptor transport to synaptic sites, their anchoring at synapses, turnover and degradation, and possibly assembly (e.g., α1–3 subunits and gephyrin, Tretter et al., 2008, 2011; Mukherjee et al., 2011; α, β subunits and Plic1, Bedford et al., 2001; β subunits and Hap1, Kittler et al., 2004; γ2 subunits and gephyrin and GABARAP, Wang et al., 1999; Kneussel et al., 2000; and GODZ, Keller et al., 2004; see Luscher et al., 2011 for review). In addition, it should be noted that αβγ GABAA receptors also diffuse laterally in the surface membrane (Thomas et al., 2005; Triller and Choquet, 2005; Bogdanov et al., 2006), where they can also be found in significant numbers in extrasynaptic compartments of neurons (Kasugai et al., 2010).
GABAA receptors that are specifically considered to populate the extrasynaptic domain principally contain the δ subunit and often a specific α subunit, such as α4 (e.g., thalamic relay cells or dentate granule cells) or α6 (cerebellar granule cells; Sieghart and Sperk, 2002). However, extrasynaptic GABAA receptors are not restricted to those containing δ subunits as further evidence suggests that α5βγ and other αβγ receptors will be present in this domain (Thomas et al., 2005; Glykys et al., 2008). Moreover, αβ GABAA receptors can also form a constituent part of the extrasynaptic GABAA receptor population (Sieghart and Sperk, 2002; Mortensen and Smart, 2006). It is presently unclear whether homomeric GABAA receptors (e.g., β), apart from those containing ρ subunits, are expressed in significant numbers compared to more frequent αβγ and αβδ isoforms.
Ascertaining the most physiologically relevant GABAA receptors that are expressed in the CNS is not straightforward. Extensive in situ hybridization, immunocytochemical, and immunoprecipitation studies, using complementary DNA or RNA probes and subunit-selective antisera, together with transgenic mice, have been used to deduce the distribution profiles for the majority of individual GABAA receptor subunits (Wisden et al., 1992; Whiting et al., 1995; Pirker et al., 2000; Korpi et al., 2002). From such studies, GABAA receptor subunit compositions have been deduced with the aid of corroborating functional and pharmacological data. As a result, it is now possible, to tentatively list the likeliest native GABAA receptor subtypes that are expressed in the CNS (Sperk et al., 1997; Hutcheon et al., 2004; Olsen and Sieghart, 2008).
For native GABAA receptors in situ, one of the most important factors determining their functional response to released GABA is the potency of the neurotransmitter at specific receptor isoforms. Although over previous decades, some studies have examined the action of GABA in detail on a variety of GABAA receptor isoforms, some of which (e.g., α1β2γ2) are clearly relevant neuronal isoforms (Sigel et al., 1990; Verdoorn et al., 1990), these predate the wealth of immunocytochemical and immunoprecipitation data that is now available. These studies have modified our perception of physiologically relevant neuronal GABAA receptor isoforms. Here, we reappraise the potency of GABA at recombinant GABAA receptor isoforms designed to emulate the most prevalent GABAA receptors that are expressed in neuronal tissues, and also discuss the relative importance of the various subunits.
Methods for Assessing GABA Potency
In providing an assessment of GABA potency, the relevant GABAA receptor isoforms can be conveniently expressed in heterologous expression systems such as Xenopus oocytes or human embryonic kidney cells (HEK293). Normally, HEK293, CHO, Ltk, and other such immortalized cell lines are preferred, not only because they efficiently accommodate protein assembly and cell-surface insertion, but also because of their smaller cell size compared with oocytes, where the speed of drug application can be compromised often leading to an underestimation of ligand potency. By using HEK cells, the GABAA receptors are not exposed to endogenous regulators such as neurosteroids or Zn2+ that may affect GABA potency and pH is closely controlled. It is possible that phosphorylation may alter GABA potency, but under basal conditions, where kinases are not specifically and directly activated, this is unlikely to be a confounding factor. Moreover, phosphorylation often involves a change in GABA current amplitude rather than an alteration to GABA sensitivity (e.g., Krishek et al., 1994).
HEK Cell Culture and Expression of Recombinant GABAA Receptors
HEK293 cells were cultured in Dulbecco’s modified Eagle’s medium (DMEM) supplemented with 10% v/v fetal calf serum (FCS), 2 mM L-glutamine, 100 units/ml penicillin-G, and 100 mg/ml streptomycin, and maintained at 37°C in a humidified 95% air/5% CO2 atmosphere (Krishek et al., 1994; Wooltorton et al., 1997). Cells were transfected with equimolar ratios of cDNAs encoding for α1–6, β1–3, γ2S, δ, ε, and θ GABAA receptor subunits, representing the predominant GABAA receptor subunits expressed in the CNS.
Whole-Cell Voltage-Clamp Electrophysiology
Whole-cell GABA-activated and spontaneous currents were recorded from transfected HEK cells using patch clamp recording with electrodes filled with a solution containing (mM): 120 KCl, 1 MgCl2, 11 EGTA, 30 KOH, 10 HEPES, 1 CaCl2, and 2 K2ATP; pH 7.2 with 1 M NaOH. The HEK cells were constantly superfused with a Krebs solution containing (mM): 140 NaCl, 4.7 KCl, 1.2 MgCl2, 2.52 CaCl2, 11 Glucose, and 5 HEPES; pH 7.4. Membrane currents were recorded from voltage clamped cells at −60 mV, and routinely compensated for series resistance (Rs) of >70%, and filtered at 5 kHz. For assessing GABA potency on physiologically relevant GABAA receptor isoforms we used a U-tube fast drug application system (Mortensen and Smart, 2007). The recording parameters were designed to ensure near identical experimental conditions and thus valid comparative determinations of GABA potency.
Measuring GABA Potency
This requires GABA concentration response relationships to be determined by normalizing GABA currents to the response induced by a maximal, saturating concentration of GABA (Imax) and subsequently curve fitting the data using the Hill equation:

where the GABA potency, EC50, represents the concentration of the agonist ([A]) inducing 50% of the maximal current evoked by a saturating concentration of the agonist and n is the Hill coefficient. The potency of GABA can then be simply deduced from the relative EC50 values for each curve and potency ratios can also be derived from these data.
Given that dose response data are distributed on a logarithmic scale, EC50 values are converted to pEC50 values using: pEC50 = −log(EC50). Unlike EC50s, the pEC50 values are distributed on a linear scale from which mean ± SEM values can be obtained. To facilitate data interpretation, mean pEC50 values can be transformed into EC50 values. The potency histograms shown in this review depict left ordinate axes corresponding to mean pEC50 values ± SEM, and right ordinate logarithmic axes for EC50 values (note that the error bars only relate to pEC50).
Finally, some receptor subunit combinations can exhibit spontaneous channel activity in the absence of GABA. To determine the level of spontaneous activity of, for example, ε subunit-containing receptors, the maximal inhibition of spontaneous channel activity was observed as a decrease in the membrane holding current in the presence of a saturating concentration of the allosteric GABAA receptor blocker, picrotoxin (1 mM; IPTX, Max). This was quantified by dividing IPTX, Max by the total range of GABA channel activity (IPTX, Max + IGABA, Max), according to the following ratio:

where IGABA, Max, is the maximal current activated by a saturating concentration of GABA at the same spontaneously opening receptors (Mortensen et al., 2003).
Results
Comparison of GABA Potency on GABAA Receptor α-Subunits
All six α subunits were sequentially expressed with β3 and γ2 subunits to compare GABA potency by determining the GABA EC50s. GABA concentration response curves have been established by measuring whole-cell currents (Figure 1Aa) for a range of GABA concentrations on α1–6 subunit-containing receptors (Figure 1Ab). It is apparent from the EC50 values for each isoform that the α-subunit-containing receptors form three distinct groups, with GABA exhibiting its lowest potency at α2 and α3 containing receptors (EC50: 13.4 and 12.5 μM, respectively; Figure 1Ac; Table 1), increasing to an intermediate potency for activating α1, α4, and α5-containing receptors (2.1, 2.1, and 1.4 μM, respectively; Figure 1Ac; Table 1), with the highest potency measured for α6 subunit-containing receptors (0.17 μM; Figure 1Ac; Table 1). The difference in potency between α2/3- and α6-containing receptors is ∼80-fold.
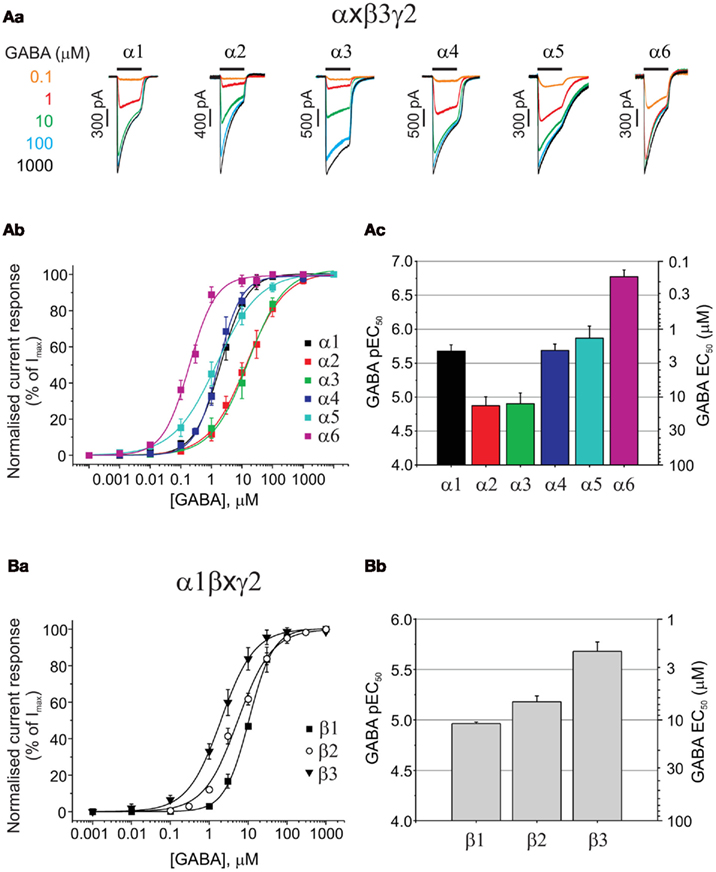
Figure 1. Assessing the impact of α, and β GABAA receptor subunits on GABA potency. (Aa) Superimposed membrane currents activated by GABA concentrations ranging from 0.1 μM to 1 mM for α1–6β3γ2 GABAA receptors expressed in HEK293 cells. Note the slow desensitization for α3β3γ2 and slow deactivation for α5β3γ2. (Ab) GABA concentration response curves for α1–6β3γ2. Points are mean ± SEM (5–7 cells). (Ac) Bar chart of mean GABA pEC50 values ± SEM (left ordinate) and equivalent EC50 values (right ordinate) for α1–6β3γ2 receptors. (Ba) Displays GABA concentration response curves for α1β1–3γ2 receptors (n = 5–34 cells), and (Bb) depicts the pEC50 values (mean ± SEM) and the equivalent EC50 values (mean).
Although in such experiments GABA is usually delivered to single GABAA receptor expressing cells with a latency of 20–30 ms, it is clear from the current profiles that the rate of desensitization was not simply related to GABA potency as both α2 (low GABA potency) and α6 (high potency) receptor isoforms showed relatively fast current desensitization, whilst α3 (low potency) exhibited the slowest desensitization kinetics (Figure 1Aa). By comparing between α subunit isoforms, it is clear that only α5 showed a dramatically slow current deactivation (Figure 1Aa). All these recombinant GABAA receptor isoforms (and others reviewed below) that incorporate the γ2 subunit, display robust expression in HEK293 cells with maximal GABA currents in the range of 2–4 nA (Table 1) without exhibiting any spontaneous activity.
GABA Binds Most Tightly to Synaptic-Type β3 Subunit-Containing Receptors
The importance of the β subunit (1–3) for GABA potency has been examined in receptors co-expressing α1 and γ2 (Figures 1Ba,b). GABA EC50 values for α1β1γ2 (10.9 μM), α1β2γ2 (6.6 μM), and α1β3γ2 (2.1 μM) were significantly different (ANOVA, P = 0.0022), with the β3-containing isoform being the most sensitive to GABA. Membrane current profiles were similar and all isoforms showed robust expression in HEK cells after only 14–18 h (GABA Imax values (pA) for α1β1γ2: 3575 ± 799, α1β2γ2: 2230 ± 193, and α1β3γ2: 3367 ± 662; Table 1). A similar rank order of GABA potency has been observed from comparative expression studies of human GABAA receptor constructs expressed in Xenopus oocytes, although the EC50 values were higher overall by ∼2–3-fold (Hadingham et al., 1993).
GABA Potency at α4 and α6 Subunit-Containing GABAA Receptors
Some of the most abundant extrasynaptic GABAA receptors are formed from α4βδ (Jia et al., 2005; Belelli et al., 2009) and α6βδ subtypes (Farrant and Nusser, 2005). There is also evidence in support of extrasynaptic α4β and α6β receptors in the CNS (Bencsits et al., 1999; Sinkkonen et al., 2004) as well as synaptic and/or extrasynaptic α4βγ and α6βγ GABAA receptors (Quirk et al., 1994; Peng et al., 2004). Whereas α4-containing receptors have a wide distribution throughout the brain, α6 subunits are exclusively expressed in cerebellar granule cells and the cochlear nucleus (Pirker et al., 2000). GABA EC50 values for α4β3 (0.97 μM), α4β3γ2 (2.1 μM), and α4β3δ (1.7 μM) GABAA receptors displayed similar sensitivities to GABA, although GABA is slightly more potent in activating α4β3 compared to α4β3γ2 (P = 0.0172; Figure 2A; Table 1). Similarly, for α6-containing receptors, GABA is again more potent at α6β3 (EC50: 0.076 μM) than either α6β3γ2 (0.17 μM; P = 0.0377) or α6β3δ (0.17 μM; P = 0.0437; Figure 2B; Table 1).
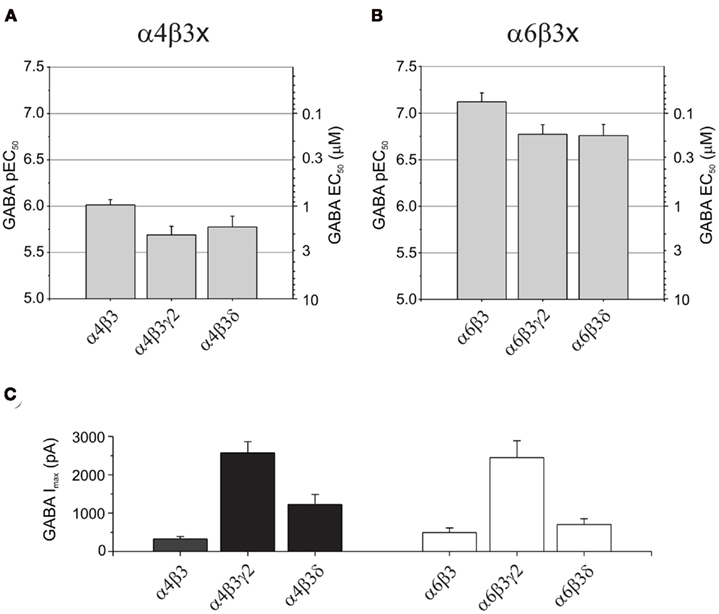
Figure 2. GABA potencies and maximum currents at α4- and α6-containing GABAA receptors. Bar charts showing pEC50 (mean ± SEM) and EC50 (mean) values for: (A) α4β3, α4β3γ2, and α4β3δ, and (B) α6β3, α6β3γ2, and α6β3δ GABAA receptors. Note that α6-containing receptors consistently display a higher potency for GABA than α4-containing receptors. (C) Bar charts of the maximum GABA-induced currents (mean ± SEM) for α4- and α6-containing GABAA receptors expressed in HEK293 cells.
Interestingly, by comparing α4- with α6-containing receptors, GABA consistently exhibited a higher potency at α6-containing receptors (P = 0 < 0.001; Figures 2A,B; Table 1). Furthermore, α4β3γ2 or α6β3γ2 receptors also showed significantly higher expression levels compared appropriately with either α4β3 and α6β3 or α4β3δ and α6β3δ receptors (P < 0.01; Figure 2C; Table 1).
GABA Potency at Extrasynaptic-Type αβ Receptors
In addition to α4 and α6 subunit-containing receptors, there is now evidence supporting the existence of αβ GABAA receptors at extrasynaptic locations in cerebellar granule cells and hippocampal pyramidal cells (Brickley et al., 1999; Mortensen and Smart, 2006). The genes encoding for α1, β2, and γ2 subunits are clustered on human chromosome 5q34 (mouse: 11, rat:10; Simon et al., 2004) and following their co-expression, could be one reason why α1β2γ2 receptors are one of the most abundant GABAA receptor isoforms in CNS neurons (Sieghart, 1995; McKernan and Whiting, 1996; Mehta and Ticku, 1999), and potentially why α1β2 receptors can be assembled and inserted in extrasynaptic membrane compartments in the same neurons and brain regions as α1β2γ2 receptors. However, gene clusters are not absolute predictors of receptor subunit combinations since the α6 subunit gene, GABRA6, also clusters with those for α1, β2, and γ2 (Simon et al., 2004) yet has a much more restricted expression profile.
In noradrenergic locus coeruleus cells and hypothalamic and thalamic nuclei, α3, θ, and ε subunits are expressed (Sinkkonen et al., 2000; Pape et al., 2009) and it is possible that extrasynaptic α3β2/3 receptors form in these brain areas. In addition, there is indeed evidence for α4β3 (Bencsits et al., 1999) and α6β3 isoforms (Sinkkonen et al., 2004) being present extrasynaptically in thalamic and cerebellar neurons respectively, where α4β3δ and α6β3δ are also expressed.
When comparing the potency of GABA in activating these αβ receptors, GABA was least potent at α3β3 (4.5 μM, P < 0.01), exhibited intermediate potency at α1β2 (1.7 μM) and α4β3 (0.97 μM), and displayed the highest potency at α6β3 receptors (0.076 μM, P < 0.001; Figures 3Aa,b; Table 1).
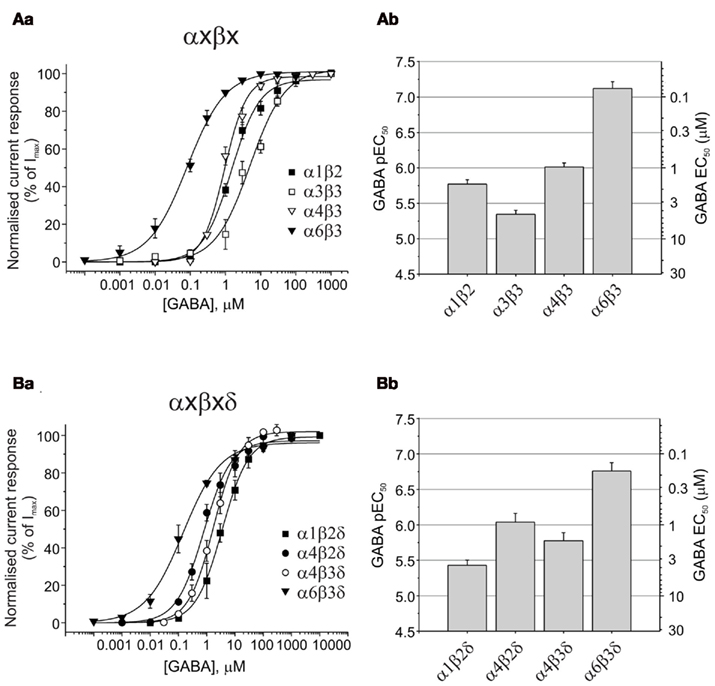
Figure 3. GABA potencies at αβ and αβδ GABAA receptors. (Aa) GABA concentration response curves for α1β2, α3β3, α4β3, and α6β3 (n = 20). (Ab) Bar chart of pEC50 (mean ± SEM) and equivalent EC50 values for αβ receptors. (Ba) GABA concentration response curves for α1β2δ, α4β2δ, α4β3δ, and α6β3δ (n = 20). (Bb) Bar chart of pEC50 (mean ± SEM) and equivalent EC50 values for αβδ GABAA receptors expressed in HEK293 cells.
Curiously, the maximum GABA currents obtained with α4β3 (328 ± 67 pA) and α6β3 (490 ± 125 pA) receptors were significantly smaller than those obtained with α1β2 (1863 ± 333 pA) and in particular with α3β3 (3924 ± 288 pA, P < 0.01; Table 1).
Comparison of GABA Potencies at Extrasynaptic-Type δ-Containing Receptors
The δ-containing receptors are generally considered to be extrasynaptic. By contrast with the limited distribution of α6βδ receptors in cerebellar granule cells (Jechlinger et al., 1998), α4βδ is found in dentate granule cells and thalamic relay cells, and also expressed at lower levels in striatum and the cerebral cortex (Pirker et al., 2000). Current evidence indicates that the α4β2δ isoform predominates in dentate granule cells (Herd et al., 2008), whereas α4β2δ and α4β3δ are found in thalamic relay cells (Pirker et al., 2000). A novel δ-containing GABAA receptor, α1βδ, has also been proposed as a naturally expressed extrasynaptic receptor in hippocampal interneurons (Glykys et al., 2007).
GABA EC50 values revealed a higher potency for GABA at α6β3δ receptors [EC50: 0.17 μM compared to α1β2δ (3.7 μM), α4β2δ (0.91 μM), and α4β3δ (1.7 μM); ANOVA: P < 0.0001; Figures 3Ba,b; Table 1]. GABA potency at α1β2δ was also significantly lower than that at α4β2δ and α4β3δ GABAA receptors (P = 0.0028 and P = 0.0349, respectively). The largest GABA Imax currents were observed for α4β2δ and α4β3δ (1544 ± 263 and 1224 ± 264 pA, respectively), which were significantly higher than those observed for α1β2δ (398 ± 147 pA; P < 0.05); α4β2δ also displayed higher currents than α6β3δ (706 ± 148 pA, P = 0.024; Table 1).
GABA Potencies at α3β3 Subunit-Containing Receptors with γ2, θ, or ε
The potential co-expression of α3, θ, and ε subunits in noradrenergic cells of the locus coeruleus and also hypothalamic nuclei (primarily ventromedial and dorsomedial; Sinkkonen et al., 2000), further suggests the existence of neuronal GABAA receptor isoforms such as α3β3, α3β3γ2, α3β3θ, and α3β3ε. In accord with our previous observations of GABA displaying a higher potency at αβ compared to αβγ receptors, the GABA EC50 for α3β3 (4.5 μM) was significantly higher than that for α3β3γ2 (12.5 μM; P = 0.0306; Figures 4A,B; Table 1).
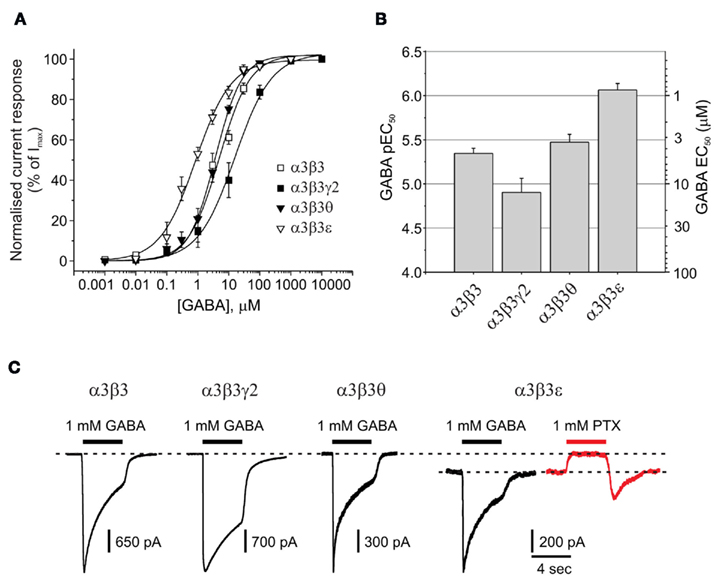
Figure 4. Impact of θ or ε on GABA potency and spontaneous channel opening. (A) GABA concentration response curves for α3β3, α3β3γ2, α3β3θ, and α3β3ε GABAA receptors expressed in HEK293 cells (n = 20). (B) Bar chart of pEC50 (mean ± SEM) and EC50 values for α3β3x receptors. (C) Membrane currents activated by 1 mM GABA for α3β3, α3β3γ2, α3β3θ, and α3β3ε (black traces), including an example of outward current (red trace) generated by picrotoxin (PTX) blocking the spontaneous current of α3β3ε in the absence of GABA.
The mean GABA EC50 for α3β3θ (3.4 μM) was not significantly different from that determined with the α3β3 (4.5 μM) isoform, which could have been due to the θ subunit not being incorporated efficiently into the α3β3θ receptor. However, from the maximal GABA currents, it was clear that Imax was significantly reduced for α3β3θ compared with α3β3, indicative of θ being assembled into the receptor (1680 ± 508 and 3924 ± 288 pA, respectively; P = 0.003; Figure 4C; Table 1). For this group of receptors, GABA had the highest potency at α3β3ε (EC50: 0.86 μM; P < 0.01; Figures 4A,B). Similarly to α3β3θ, the GABA Imax for α3β3ε (811 ± 300 pA) was also reduced compared with that for α3β3 and α3β3γ2 (P < 0.001).
Of all the GABAA receptors that have been reviewed, the only isoform that displayed significant spontaneous channel activity in the absence of GABA was α3β3ε receptors. This was blocked by 1 mM picrotoxin (PTX; Figure 4C). The PTX-sensitive spontaneous current accounted for 24 ± 5% (n = 8) of the maximum total current that could be passed by these receptors.
Discussion
The purpose of this review has been to consider the comparative potency data for GABA in activating 18 of the most likely isoforms of the GABAA receptor to be expressed in the CNS (Olsen and Sieghart, 2008). This catalog of neuronal GABAA receptor isoforms was accrued from in situ hybridization (Laurie et al., 1992; Wisden et al., 1992), immunocytochemical (Fritschy and Mohler, 1995; Pirker et al., 2000; Fritschy and Brunig, 2003), and immunoprecipitation (Khan et al., 1994) data, with supporting evidence from electrophysiological studies (Whiting et al., 1995; McKernan and Whiting, 1996). Of necessity however, the composition of the less common neuronal GABAA receptor isoforms is still subject to speculation given the difficulties of precisely determining native receptor subunit composition.
It is apparent that significant variations in potency can occur when comparing the same ligand against receptors expressed in different cell types. In particular, potencies measured with receptors expressed in Xenopus oocytes have yielded results that differ from similar determinations conducted in mammalian cell types (e.g., HEK293, COS, and NG108-15 cells). Variable determinations of potency can also arise from using different DNA transfection ratios, which might influence receptor subunit composition, and by using different speeds of drug application. This makes exact comparison of EC50 values from different isoforms of the receptor more difficult if they are not measured under exactly the same experimental conditions. In this review, we have ensured that experimental conditions are consistent, thereby enabling exact comparisons between neuronally relevant GABAA receptor isoforms.
Previous extensive and comparative studies of the effects of GABA and other GABAA receptor specific ligands on a range of GABAA receptor isoforms have been conducted on oocytes (Sigel et al., 1990; Ebert et al., 1994), but since this period, our understanding of GABAA receptor assembly and naturally occurring isoforms in the CNS has changed considerably.
Xenopus oocytes offer a robust expression system perfectly suited for screening the pharmacology of multiple receptor isoforms; however, due to their large size, drug application speeds are often slower than expected with mammalian cells, which may be the most likely reason why EC50 values from oocyte studies for a specific receptor isoform are usually notably higher than those determined with smaller immortalized cell lines (e.g., HEK293) more akin to a neuron (Verdoorn et al., 1990). Indeed, the EC50 values, noted in this review obtained from HEK293 cells were consistently lower than those reported from oocyte studies.
It is clear from appraising GABA potency on different GABAA receptor isoforms that the identity of the α-subunit is most important with an almost 80-fold difference in EC50 values from the low potency α2β3γ2 and α3β3γ2 receptors, to the high potency isoform, α6β3γ2. The low potency of α2/α3βγ receptors would be suited to inhibitory synaptic compartments where the GABA concentration transient in the synaptic cleft will rise to >1 mM during vesicular release (Mozrzymas et al., 2003). For extrasynaptic receptors that are likely to be exposed to much lower basal and spillover GABA concentrations of ∼20–500 nM (Mortensen and Smart, 2006; Lee et al., 2010), a higher GABA potency is advantageous. The high potency of α6β3γ2 indicates that this isoform may be mainly located extrasynaptically in cerebellar granule cells and the cochlear nucleus, similar to α6βδ, although α6 subunit-containing receptors are also reported to access inhibitory synapses (Tia et al., 1996; Mellor et al., 2000; Santhakumar et al., 2006).
Interestingly, α1-, α4-, and α5-containing receptors assembled with βγ subunits displayed intermediate potencies for GABA suggesting these receptors could participate equally at inhibitory synapses as well as in extrasynaptic compartments. It has been previously demonstrated that α1βγ receptors are also located in extrasynaptic domains (Thomas et al., 2005). Similarly, it has been shown that α5-containing GABAA receptors play an important part in tonic inhibition in hippocampal pyramidal neurons, and that these can also contribute to synaptic inhibition (Caraiscos et al., 2004; Serwanski et al., 2006). By contrast, α4βδ receptors are regarded as important extrasynaptic receptors in the thalamus and the dentate gyrus, and there is also evidence for an α4βγ isoform, which has been reported to be located both within and outside inhibitory synapses (Peng et al., 2004).
Previous evidence suggests that extrasynaptic αβ receptors are present on hippocampal pyramidal cells and play a part (∼10%) in tonic inhibition (Mortensen and Smart, 2006). The presence of α6β receptors has similarly been observed in Thy1α6 transgenic mice with ectopic α6-expression, outside of the cerebellum (Sinkkonen et al., 2004). Although this is an abnormal expression pattern, it indicates that α6β receptors have the ability to be expressed and may, under normal conditions, be present in cerebellar granule cells where the expression of α6 is high. Similarly, there is evidence for the presence of α4β receptors in the brain (Bencsits et al., 1999) and this suggests the possibility that various αβ isoforms may be present in other brain areas could have been previously underestimated (e.g., α1β2 throughout the CNS, α4β2/3 in thalamus and dentate gyrus, α3β3 in the hypothalamus and locus coeruleus, and α6β2/3 in the cerebellum). The observation that αβ always displays a higher GABA potency than its αβγ counterpart, underlines the potential value of these γ-lacking αβ as extrasynaptic receptors, helping to set the level of tonic inhibitory tone. However, it is expected that their single channel conductances will be smaller compared with αβγ or αβδ counterparts (Moss et al., 1990; Angelotti and MacDonald, 1993; Mortensen and Smart, 2006).
Typically, prevalent forms of extrasynaptic GABAA receptors are those containing the δ-subunit that populate the dentate gyrus (α4β2δ), thalamus (α4β2δ and α4β3δ), and cerebellar granule cells (α6βδ). In addition, α1βδ receptors may also be expressed in the dentate gyrus (Glykys et al., 2007). Comparing the four δ-containing isoforms, GABA potency was highest at α6β3δ compared to α1β2δ, α4β2δ, and α4β3δ. These potency differences may reflect regional differences in ambient GABA concentrations, where the highly GABA sensitive α6β3δ would be ideally suited to an environment where the GABA concentration was lower.
The less abundant GABAA receptor subunits, ε and θ, which have defined expression patterns in the locus coeruleus, hypothalamus, tegmentum, and pontine nuclei, have been proposed to assemble as α3βε and α3βθ isoforms due to the co-localization of α3, ε, and θ on the same chromosome (Pape et al., 2009). These receptors have not previously been subject to a full characterization, but their relatively high potency for GABA (in particular α3β3ε) indicates that they would also be likely contributors to tonic inhibition and thus candidates for being located at extrasynaptic sites.
Of all the 18 GABAA receptor isoforms reviewed in this study, only α3β3ε receptors showed significant spontaneous activity. Spontaneous activity has been reported before for α1β3ε (Neelands et al., 1999; Mortensen et al., 2003) suggesting that the ε subunit is mainly responsible for spontaneity given that the α1 or β3 subunits do not impart this profile onto α1β3γ2 receptors.
In summary we have observed differences in GABA potency ranging up to 175-fold between GABAA receptor isoforms with GABA being most potent at extrasynaptic α6-containing receptors and least potent at synaptic-type α2β3γ2 and α3β3γ2 receptors. This range of GABA potency will clearly impact on the activation of GABAA receptors and influence the roles they play in controlling excitability from either synaptic or extrasynaptic locations.
Conflict of Interest Statement
The authors declare that the research was conducted in the absence of any commercial or financial relationships that could be construed as a potential conflict of interest.
Acknowledgments
This work was supported by the MRC.
References
Angelotti, T. P., and MacDonald, R. L. (1993). Assembly of GABAA receptor subunits: α1 β1 and α1 β1 γ2S subunits produce unique ion channels with dissimilar single-channel properties. J. Neurosci. 13, 1429–1440.
Bedford, F. K., Kittler, J. T., Muller, E., Thomas, P., Uren, J. M., Merlo, D., Wisden, W., Triller, A., Smart, T. G., and Moss, S. J. (2001). GABAA receptor cell surface number and subunit stability are regulated by the ubiquitin-like protein Plic-1. Nat. Neurosci. 4, 908–916.
Belelli, D., Harrison, N. L., Maguire, J., MacDonald, R. L., Walker, M. C., and Cope, D. W. (2009). Extrasynaptic GABAA receptors: form, pharmacology, and function. J. Neurosci. 29, 12757–12763.
Bencsits, E., Ebert, V., Tretter, V., and Sieghart, W. (1999). A significant part of native γ-aminobutyric acid A receptors containing α4 subunits do not contain γ or δ subunits. J. Biol. Chem. 274, 19613–19616.
Bogdanov, Y., Michels, G., Armstrong-Gold, C., Haydon, P. G., Lindstrom, J., Pangalos, M., and Moss, S. J. (2006). Synaptic GABAA receptors are directly recruited from their extrasynaptic counterparts. EMBO J. 25, 4381–4389.
Brickley, S. G., Cull-Candy, S. G., and Farrant, M. (1999). Single-channel properties of synaptic and extrasynaptic GABAA receptors suggest differential targeting of receptor subtypes. J. Neurosci. 19, 2960–2973.
Caraiscos, V. B., Elliott, E. M., You, T., Cheng, V. Y., Belelli, D., Newell, J. G., Jackson, M. F., Lambert, J. J., Rosahl, T. W., Wafford, K. A., MacDonald, J. F., and Orser, B. A. (2004). Tonic inhibition in mouse hippocampal CA1 pyramidal neurons is mediated by a5 subunit-containing g-aminobutyric acid type A receptors. Proc. Natl. Acad. Sci. U.S.A. 101, 3662–3667.
Ebert, B., Wafford, K. A., Whiting, P. J., Krogsgaard-Larsen, P., and Kemp, J. A. (1994). Molecular pharmacology of γ-aminobutyric acid type A receptor agonists and partial agonists in oocytes injected with different α, β, and γ receptor subunit combinations. Mol. Pharmacol. 46, 957–963.
Farrant, M., and Nusser, Z. (2005). Variations on an inhibitory theme: phasic and tonic activation of GABAA receptors. Nat. Rev. Neurosci. 6, 215–229.
Farrar, S. J., Whiting, P. J., Bonnert, T. P., and McKernan, R. M. (1999). Stoichiometry of a ligand-gated ion channel determined by fluorescence energy transfer. J. Biol. Chem. 274, 10100–10104.
Fritschy, J. M., and Brunig, I. (2003). Formation and plasticity of GABAergic synapses: physiological mechanisms and pathophysiological implications. Pharmacol. Ther. 98, 299–323.
Fritschy, J.-M., and Mohler, H. (1995). GABAA receptor heterogeneity in the adult rat brain: differential regional and cellular distribution of seven major subunits. J. Comp. Neurol. 359, 154–194.
Glykys, J., Mann, E. O., and Mody, I. (2008). Which GABAA receptor subunits are necessary for tonic inhibition in the hippocampus? J. Neurosci. 28, 1421–1426.
Glykys, J., Peng, Z., Chandra, D., Homanics, G. E., Houser, C. R., and Mody, I. (2007). A new naturally occurring GABAA receptor subunit partnership with high sensitivity to ethanol. Nat. Neurosci. 10, 40–48.
Hadingham, K. L., Wingrove, P. B., Wafford, K. A., Bain, C., Kemp, J. A., Palmer, K. J., Wilson, A. W., Wilcox, A. S., Sikela, J. M., Ragan, C. I., and Whiting, P. J. (1993). Role of the β subunit in determining the pharmacology of human y-aminobutyric acid type A receptors. Mol. Pharmacol. 44, 1211–1218.
Herd, M. B., Haythornthwaite, A. R., Rosahl, T. W., Wafford, K. A., Homanics, G. E., Lambert, J. J., and Belelli, D. (2008). The expression of GABAA b subunit isoforms in synaptic and extrasynaptic receptor populations of mouse dentate gyrus granule cells. J. Physiol. (Lond) 586, 989–1004.
Hutcheon, B., Fritschy, J. M., and Poulter, M. O. (2004). Organization of GABA receptor α-subunit clustering in the developing rat neocortex and hippocampus. Eur. J. Neurosci. 19, 2475–2487.
Jacob, T. C., Moss, S. J., and Jurd, R. (2008). GABAA receptor trafficking and its role in the dynamic modulation of neuronal inhibition. Nat. Rev. Neurosci. 9, 331–343.
Jechlinger, M., Pelz, R., Tretter, V., Klausberger, T., and Sieghart, W. (1998). Subunit composition and quantitative importance of hetero-oligomeric receptors: GABAA receptors containing α6 subunits. J. Neurosci. 18, 2449–2457.
Jia, F., Pignataro, L., Schofield, C. M., Yue, M., Harrison, N. L., and Goldstein, P. A. (2005). An extrasynaptic GABAA receptor mediates tonic inhibition in thalamic VB neurons. J. Neurophysiol. 94, 4491–4501.
Jones, A., Korpi, E. R., McKernan, R. M., Pelz, R., Nusser, Z., Makela, R., Mellor, J. R., Pollard, S., Bahn, S., Stephenson, F. A., Randall, A. D., Sieghart, W., Somogyi, P., Smith, A. J., and Wisden, W. (1997). Ligand-gated ion channel subunit partnerships: GABAA receptor α6 subunit gene inactivation inhibits delta subunit expression. J. Neurosci. 17, 1350–1362.
Kasugai, Y., Swinny, J. D., Roberts, J. D., Dalezios, Y., Fukazawa, Y., Sieghart, W., Shigemoto, R., and Somogyi, P. (2010). Quantitative localisation of synaptic and extrasynaptic GABAA receptor subunits on hippocampal pyramidal cells by freeze-fracture replica immunolabelling. Eur. J. Neurosci. 32, 1868–1888.
Keller, C. A., Yuan, X., Panzanelli, P., Martin, M. L., Alldred, M., Sassoe-Pognetto, M., and Luscher, B. (2004). The γ2 subunit of GABAA receptors is a substrate for palmitoylation by GODZ. J. Neurosci. 24, 5881–5891.
Khan, Z. U., Gutierrez, A., and De Blas, A. L. (1994). The subunit composition of a GABAA/benzodiazepine receptor from rat cerebellum. J. Neurochem. 63, 371–374.
Kittler, J. T., Thomas, P., Tretter, V., Bogdanov, Y. D., Haucke, V., Smart, T. G., and Moss, S. J. (2004). Huntingtin-associated protein 1 regulates inhibitory synaptic transmission by modulating γ-aminobutyric acid type A receptor membrane trafficking. Proc. Natl. Acad. Sci. U.S.A. 101, 12736–12741.
Klausberger, T., Fuchs, K., Mayer, B., Ehya, N., and Sieghart, W. (2000). GABAA receptor assembly. Identification and structure of γ2 sequences forming the intersubunit contacts with α1 and β3 subunits. J. Biol. Chem. 275, 8921–8928.
Klausberger, T., Sarto, I., Ehya, N., Fuchs, K., Furtmuller, R., Mayer, B., Huck, S., and Sieghart, W. (2001). Alternate use of distinct intersubunit contacts controls GABAA receptor assembly and stoichiometry. J. Neurosci. 21, 9124–9133.
Kneussel, M., Haverkamp, S., Fuhrmann, J. C., Wang, H., Wassle, H., Olsen, R. W., and Betz, H. (2000). The gamma-aminobutyric acid type A receptor (GABAAR)-associated protein GABARAP interacts with gephyrin but is not involved in receptor anchoring at the synapse. Proc. Natl. Acad. Sci. U.S.A. 97, 8594–8599.
Korpi, E. R., Grunder, G., and Luddens, H. (2002). Drug interactions at GABAA receptors. Prog. Neurobiol. 67, 113–159.
Krishek, B. J., Xie, X., Blackstone, C., Huganir, R. L., Moss, S. J., and Smart, T. G. (1994). Regulation of GABAA receptor function by protein kinase C phosphorylation. Neuron 12, 1081–1095.
Laurie, D. J., Wisden, W., and Seeburg, P. H. (1992). The distribution of thirteen GABAA receptor subunit mRNAs in the rat brain. III. Embryonic and postnatal development. J. Neurosci. 12, 4151–4172.
Lee, S., Yoon, B. E., Berglund, K., Oh, S. J., Park, H., Shin, H. S., Augustine, G. J., and Lee, C. J. (2010). Channel-mediated tonic GABA release from glia. Science 330, 790–796.
Luscher, B., Fuchs, T., and Kilpatrick, C. (2011). GABAA receptor trafficking-mediated plasticity of inhibitory synapses. Neuron 70, 385–409.
Luscher, B., and Keller, C. A. (2004). Regulation of GABAA receptor trafficking, channel activity, and functional plasticity of inhibitory synapses. Pharmacol. Ther. 102, 195–221.
McKernan, R. M., and Whiting, P. J. (1996). Which GABAA-receptor subtypes really occur in the brain? Trends Neurosci. 19, 139–143.
Mehta, A. K., and Ticku, M. K. (1999). An update on GABAA receptors. Brain Res. Brain Res. Rev. 29, 196–217.
Mellor, J. R., Wisden, W., and Randall, A. D. (2000). Somato-synaptic variation of GABA(A) receptors in cultured murine cerebellar granule cells: investigation of the role of the alpha6 subunit. Neuropharmacology 39, 1495–1513.
Mortensen, M., and Smart, T. G. (2006). Extrasynaptic αβ subunit GABAA receptors on rat hippocampal pyramidal neurons. J. Physiol. (Lond.) 577, 841–856.
Mortensen, M., and Smart, T. G. (2007). Single-channel recording of ligand-gated ion channels. Nat. Protoc. 2, 2826–2841.
Mortensen, M., Wafford, K. A., Wingrove, P., and Ebert, B. (2003). Pharmacology of GABAA receptors exhibiting different levels of spontaneous activity. Eur. J. Pharmacol. 476, 17–24.
Moss, S. J., and Smart, T. G. (2001). Constructing inhibitory synapses. Nat. Rev. Neurosci. 2, 240–250.
Moss, S. J., Smart, T. G., Porter, N. M., Nayeem, N., Devine, J., Stephenson, F. A., MacDonald, R. L., and Barnard, E. A. (1990). Cloned GABA receptors are maintained in a stable cell line: allosteric and channel properties. Eur. J. Pharmacol. 189, 77–88.
Mozrzymas, J. W., Zarnowska, E. D., Pytel, M., and Mercik, K. (2003). Modulation of GABAA receptors by hydrogen ions reveals synaptic GABA transient and a crucial role of the desensitization process. J. Neurosci. 23, 7981–7992.
Mukherjee, J., Kretschmannova, K., Gouzer, G., Maric, H. M., Ramsden, S., Tretter, V., Harvey, K., Davies, P. A., Triller, A., Schindelin, H., and Moss, S. J. (2011). The residence time of GABAARs at inhibitory synapses is determined by direct binding of the receptor α1 subunit to gephyrin. J. Neurosci. 31, 14677–14687.
Neelands, T. R., Fisher, J. L., Bianchi, M., and MacDonald, R. L. (1999). Spontaneous and γ-aminobutyric acid (GABA)-activated GABAA receptor channels formed by ε subunit-containing isoforms. Mol. Pharmacol. 55, 168–178.
Olsen, R. W., and Sieghart, W. (2008). International Union of Pharmacology. LXX. Subtypes of gamma-aminobutyric acid(A) receptors: classification on the basis of subunit composition, pharmacology, and function. Pharmacol. Rev. 60, 243–260.
Pape, J. R., Bertrand, S. S., Lafon, P., Odessa, M. F., Chaigniau, M., Stiles, J. K., and Garret, M. (2009). Expression of GABAA receptor α3-, θ-, and ε-subunit mRNAs during rat CNS development and immunolocalization of the ε subunit in developing postnatal spinal cord. Neuroscience 160, 85–96.
Peng, Z., Huang, C. S., Stell, B. M., Mody, I., and Houser, C. R. (2004). Altered expression of the d subunit of the GABAA receptor in a mouse model of temporal lobe epilepsy. J. Neurosci. 24, 8629–8639.
Pirker, S., Schwarzer, C., Wieselthaler, A., Sieghart, W., and Sperk, G. (2000). GABAA receptors: immunocytochemical distribution of 13 subunits in the adult rat brain. Neuroscience 101, 815–850.
Quirk, K., Gillard, N. P., Ragan, C. I., Whiting, P. J., and McKernan, R. M. (1994). Model of subunit composition of g-aminobutyric acidA receptor subtypes expressed in rat cerebellum with respect to their a and g/d subunits. J. Biol. Chem. 269, 16020–16028.
Santhakumar, V., Hanchar, H. J., Wallner, M., Olsen, R. W., and Otis, T. S. (2006). Contributions of the GABAA receptor α6 subunit to phasic and tonic inhibition revealed by a naturally occurring polymorphism in the α6 gene. J. Neurosci. 26, 3357–3364.
Serwanski, D. R., Miralles, C. P., Christie, S. B., Mehta, A. K., Li, X., and De Blas, A. L. (2006). Synaptic and nonsynaptic localization of GABAA receptors containing the a5 subunit in the rat brain. J. Comp. Neurol. 499, 458–470.
Sieghart, W. (1995). Structure and pharmacology of γ-aminobutyric acidA receptor subtypes. Pharmacol. Rev. 47, 181–234.
Sieghart, W., and Sperk, G. (2002). Subunit composition, distribution and function of GABAA receptor subtypes. Curr. Top. Med. Chem. 2, 795–816.
Sigel, E., Baur, R., Trube, G., Mohler, H., and Malherbe, P. (1990). The effect of subunit composition of rat brain GABAA receptors on channel function. Neuron 5, 703–711.
Simon, J., Wakimoto, H., Fujita, N., Lalande, M., and Barnard, E. A. (2004). Analysis of the set of GABAA receptor genes in the human genome. J. Biol. Chem. 279, 41422–41435.
Sinkkonen, S. T., Hanna, M. C., Kirkness, E. F., and Korpi, E. R. (2000). GABAA receptor e and q subunits display unusual structural variation between species and are enriched in the rat locus ceruleus. J. Neurosci. 20, 3588–3595.
Sinkkonen, S. T., Vekovischeva, O. Y., Moykkynen, T., Ogris, W., Sieghart, W., Wisden, W., and Korpi, E. R. (2004). Behavioural correlates of an altered balance between synaptic and extrasynaptic GABAAergic inhibition in a mouse model. Eur. J. Neurosci. 20, 2168–2178.
Sperk, G., Schwarzer, C., Tsunashima, K., Fuchs, K., and Sieghart, W. (1997). GABAA receptor subunits in the rat hippocampus I: immunocytochemical distribution of 13 subunits. Neuroscience 80, 987–1000.
Taylor, P. M., Connolly, C. N., Kittler, J. T., Gorrie, G. H., Hosie, A., Smart, T. G., and Moss, S. J. (2000). Identification of residues within GABA(A) receptor alpha subunits that mediate specific assembly with receptor beta subunits. J. Neurosci. 20, 1297–1306.
Taylor, P. M., Thomas, P., Gorrie, G. H., Connolly, C. N., Smart, T. G., and Moss, S. J. (1999). Identification of amino acid residues within GABAA receptor β subunits that mediate both homomeric and heteromeric receptor expression. J. Neurosci. 19, 6360–6371.
Thomas, P., Mortensen, M., Hosie, A. M., and Smart, T. G. (2005). Dynamic mobility of functional GABAA receptors at inhibitory synapses. Nat. Neurosci. 8, 889–897.
Tia, S., Wang, J. F., Kotchabhakdi, N., and Vicini, S. (1996). Developmental changes of inhibitory synaptic currents in cerebellar granule neurons: role of GABAA receptor α6 subunit. J. Neurosci. 16, 3630–3640.
Tretter, V., Jacob, T. C., Mukherjee, J., Fritschy, J. M., Pangalos, M. N., and Moss, S. J. (2008). The clustering of GABAA receptor subtypes at inhibitory synapses is facilitated via the direct binding of receptor α2 subunits to gephyrin. J. Neurosci. 28, 1356–1365.
Tretter, V., Kerschner, B., Milenkovic, I., Ramsden, S. L., Ramerstorfer, J., Saiepour, L., Maric, H. M., Moss, S. J., Schindelin, H., Harvey, R. J., Sieghart, W., and Harvey, K. (2011). Molecular basis of the γ-aminobutyric acid a receptor α3 subunit interaction with the clustering protein gephyrin. J. Biol. Chem. 286, 37702–37711.
Triller, A., and Choquet, D. (2005). Surface trafficking of receptors between synaptic and extrasynaptic membranes: and yet they do move! Trends Neurosci. 28, 133–139.
Verdoorn, T. A., Draguhn, A., Ymer, S., Seeburg, P. H., and Sakmann, B. (1990). Functional properties of recombinant rat GABAA receptors depend upon subunit composition. Neuron 4, 919–928.
Wang, H. B., Bedford, F. K., Brandon, N. J., Moss, S. J., and Olsen, R. W. (1999). GABAA-receptor-associated protein links GABAA receptors and the cytoskeleton. Nature 397, 69–72.
Whiting, P. J., McKernan, R. M., and Wafford, K. A. (1995). Structure and pharmacology of vertebrate GABAA receptor subtypes. Int. Rev. Neurobiol. 38, 95–138.
Wisden, W., Laurie, D. J., Monyer, H., and Seeburg, P. H. (1992). The distribution of 13 GABAA receptor subunit mRNAs in the rat brain. I. Telencephalon, diencephalon, mesencephalon. J. Neurosci. 12, 1040–1062.
Keywords: GABAA receptor, synaptic and extrasynaptic receptors, GABA, neurons
Citation: Mortensen M, Patel B and Smart TG (2012) GABA potency at GABAA receptors found in synaptic and extrasynaptic zones. Front. Cell. Neurosci. 6:1. doi: 10.3389/fncel.2012.00001
Received: 29 November 2011;
Accepted: 03 January 2012;
Published online: 20 January 2012.
Edited by:
Enrico Cherubini, International School for Advanced Studies, ItalyReviewed by:
William Wisden, Imperial College, UKPierrick Poisbeau, CNRS and University of Strasbourg, France
Copyright: © 2012 Mortensen, Patel and Smart. This is an open-access article distributed under the terms of the Creative Commons Attribution Non Commercial License, which permits non-commercial use, distribution, and reproduction in other forums, provided the original authors and source are credited.
*Correspondence: Trevor G. Smart, University College London, Gower Street, London WC1E 6BT, UK. e-mail:dC5zbWFydEB1Y2wuYWMudWs=