- 1Department of Biomedical Sciences, College of Osteopathic Medicine of the Pacific, Western University of Health Sciences, Pomona, CA, United States
- 2Department of Biomedical and Pharmaceutical Sciences, Chapman University School of Pharmacy, Irvine, CA, United States
- 3Department of Pharmaceutics, Faculty of Pharmacy, The Islamia University of Bahawalpur, Bahawalpur, Pakistan
- 4Department of Biomedical Sciences, College of Osteopathic Medicine of the Pacific-Northwest, Western University of Health Sciences, Lebanon, OR, United States
Background: Mycobacterium avium (M. avium) is a nontuberculous mycobacterium (NTM) that can cause pulmonary and extrapulmonary infections mostly in immunocompromised individuals, such as those with HIV and diabetes. Traditionally, rifampicin (RIF) and azithromycin (AZ) have been used for a 12-month duration as first-line antibiotics against M. avium. Due to the increased multidrug resistance, novel ways, such as enhancement of macrophages response, are needed to provide adequate immune response required to clear M. avium infection.
Methods and findings: In this study, we aim to study the effects of using THP-1 cells, which are monocyte-like cells, to induce a macrophage response and control M. avium infection when used in combination with traditional treatments such as RIF and AZ in free and liposomal forms. Traditional treatments’ effects are studied when used alone and in combination therapy with cyclic peptide [R4W4] (liposomal encapsulated and liposomal combination). Colony-forming units (CFU) counts were assessed for all samples 3 hours, 4 days, and 8 days post-treatment. A significant reduction in the intracellular viability of M. avium was observed when THP-1 cells were treated with liposomal combination [R4W4]+RIF and liposomal combination [R4W4]+AZ compared to when treated with liposomal RIF or liposomal AZ alone, respectively.
Conclusion: Our findings show that liposomal combination [R4W4] is a promising adjuvant therapy to increase M. avium susceptibility to known antibiotics.
1 Introduction
The global prevalence of nontuberculous mycobacteria (NTM) infection is steadily rising (Adjemian et al., 2012; Wang et al., 2023; Wang et al., 2023). A retrospective review by Wang et al. found that in Southwest China, prevalence of NTM increased significantly each year between 2017-2022 (Wang et al., 2023). The most predominant species identified was Mycobacterium avium, followed by Mycobacterium abscessus and Mycobacterium intracellulare (Wang et al., 2023). Mycobacterium avium complex (MAC) is the most common group of NTM and M. avium subsp. avium is the most clinically significant species within MAC (Busatto et al., 2019). M. avium are acid-fast slow-growing mycobacteria that inhabit a wide range of environmental sources including soil, animal reservoirs, and animal byproducts such as milk and food products (Busatto et al., 2019). As an opportunistic human pathogen, M. avium disproportionately impacts immunocompromised individuals like those with HIV or diabetes, as well as individuals with pre-existing lung disease like cystic fibrosis, chronic obstructive pulmonary disease (COPD), or prior Mycobacterium tuberculosis infection and cavitary lesions (Diel et al., 2018b; Cano Rodríguez et al., 2023; Wang et al., 2023).
M. avium may be transmitted to humans through contact, inhalation, and ingestion (Busatto et al., 2019). Infection control is mediated through a combination of innate and adaptive immunity in the host. M. avium primarily targets mononuclear phagocytes (Li et al., 2010). Like M. tuberculosis, M. avium is consumed by macrophages, utilizing a range of pathogenic tools to survive within the phagosome-lysosome (Li et al., 2010). M. avium can inhibit phagosome-lysosome fusion and inhibit key enzymes that function to generate damaging reactive oxygen species (ROS) (Kaczmarkowska et al., 2022). In immunocompromised individuals, such as those with HIV, macrophages fail to receive their activating signal from natural killer (NK) cells and T-lymphocytes to contain M. avium growth (Kaczmarkowska et al., 2022).
M. avium produces three primary diseases in humans: pulmonary MAC (MAC-PD), disseminated MAC (D-MAC), and MAC lymphadenitis (MAC-L) (Kaczmarkowska et al., 2022). M. avium specifically results in approximately 80% of pulmonary NTM diseases (Busatto et al., 2019; To et al., 2020). A systematic review found that patients with MAC-PD have a five-year all-cause mortality rate of 27% (95% CI 21.3-37.8%) (Diel et al., 2018a; Fujishima et al., 2023). The guideline-directed therapy in place by the American Thoracic Society (ATS), European Respiratory Society (ERS), European Society of Clinical Microbiology and Infectious Diseases (ESCMID), and Infectious Disease Society of America (IDSA) suggests a once-daily regimen of azithromycin (AZ), rifampicin (RIF) or rifabutin, and ethambutol for fibrocavitary or severe nodular MAC-PD, thrice-weekly for non-severe nodular MAC-PD, and the addition of an aminoglycoside, amikacin, injection for severe fibrocavitary cases (Ito et al., 2022). This regimen is intended to be continued for more than 12 months after sputum identification. The current guideline has a history of low success rate (61.4%) plagued by adverse effects, duration of treatment, and macrolide resistance (Diel et al., 2018b). Long-term amikacin therapy has a success rate of 75% and is associated with nephrotoxicity in 6.3% of patients and ototoxicity in 25% of patients (Lee et al., 2023). A recent study found that the addition of rifampicin to the macrolide regimen did not add to the antimycobacterial effect, nor did it reduce the emergence of macrolide resistance, further demonstrating the need for novel antimycobacterial therapies (Schildkraut et al., 2023). Clofazimine, a phenazine molecule, has demonstrated a synergistic effect against M. avium when added to amikacin, however, it possesses frequent side effects and there is currently no current recommendation for standardized dosage or duration of treatment (Haworth et al., 2017; McGuffin et al., 2017; Daley et al., 2020; Lee et al., 2023).
One such approach for treating multidrug-resistant bacteria involves the use of antimicrobial peptides (AMPs). These are naturally occurring peptides, consisting of 8 or more amino acids, are an essential part of the innate immune system across eukaryotes, including humans, animals, and plants. AMPs have broad-spectrum antimicrobial activity against bacteria, fungi, viruses, and parasites. They typically disrupt the cell membranes of pathogens, leading to rapid cell death, which lowers the risk of resistance development - a common issue with traditional antibiotics. Additionally, AMPs are generally less harmful to the host, as they selectively target microbial cells while sparing human cells. Due to their unique mode of action and natural origin, AMPs are considered safer than traditional antibiotics (Rathinakumar et al., 2009; Lei et al., 2019). They have employed targeted delivery to the site of infection to reduce adverse effects. However, they face limitations such as poor oral absorption and rapid metabolism, resulting in a short duration of effectiveness, necessitating multiple dosing through the injectable route of administration (Joo, 2012). Liposomal formulations have been found effective in overcoming several of these issues, offering enhanced drug delivery and reduced toxicity (Ferreira et al., 2021). Liposomes are spherical vesicles composed of phospholipids that enclose an inner aqueous layer (Maqbool et al., 2019). The inclusion of cholesterol in their composition increases stability and protects them from degradation (Chibowski and Szcześ, 2016). Their non-immunogenic properties have garnered significant scientific attention (Mehta et al., 2020), leading to their use in treating infections and in clinical applications, such as the FDA-approved liposomal formulations for Amphotericin B (Ambisome®, Abelcet®, and Amphotec®) and Amikacin (Arikayce® Kit) (Bulbake et al., 2017; He et al., 2022). [R4W4] is an amphiphilic cyclic peptide containing four arginine and four tryptophan amino acids, demonstrating broad-spectrum antimicrobial activity (Findlay et al., 2010). [R4W4] can form ionic bonds with the negative charge on the bacterial membrane and perturb it through hydrophobic interactions with membrane lipids (Joo, 2012). The efficacy of [R4W4] has been proven against various human pathogens, including methicillin-resistant Staphylococcus aureus, Escherichia coli, Klebsiella pneumonia, Pseudomonas aeruginosa, Mycobacterium tuberculosis, and M. avium in recent studies (Oh et al., 2014; Hernandez et al., 2020; Sajid et al., 2022; Kelley et al., 2023). More specifically, our laboratory has recently demonstrated that [R4W4] reduces M. avium survival when added alongside RIF or AZ more significantly than either antibiotic alone (Kelley et al., 2023). In this study, we aim to evaluate the optimal delivery method for [R4W4]. We developed liposomes using dioleoyl phosphatidylcholine (DOPC), cholesterol, and 1,2-distearoyl-sn-glycero-3-phosphoethanolamine-N-[carbonyl-amino(polyethylene glycol)-2000] (DSPE-PEG2000) for effective delivery of [R4W4], RIF, and AZ. Our objective was to determine whether [R4W4] is more effective when delivered as a liposomal encapsulated formulation, a liposomal combination formulation with RIF or AZ, or whether the therapy is more effective in its liposomal form compared to the free formulation.
2 Materials and methods
2.1 Liposomal preparation and characterization
Liposomes were prepared using the thin film hydration method as reported elsewhere, with some modifications (Zhang, 2017). Lipids and 1,2-distearoyl-sn-glycero-3-phosphoethanolamine-N-[carbonyl-amino(polyethylene glycol)-2000] were purchased from Avanti Polar Lipids, Alabama, USA. Cholesterol and other chemicals for liposomal preparation were purchased from Miliporesigma (USA). Briefly, lipids and the drug were dissolved at different compositions (Table 1) in 15 mL of chloroform in a round-bottom flask, and the organic solvent was evaporated using a rotary evaporator under 500 mbar pressure, 90 RPM, and at 45 °C, which is above the phase transition temperature of the lipids. A fine thin film formed in the round-bottom flask after 5 to 6 hours, which was then dried overnight. Phosphate-buffered saline (PBS) was added to the flask until the lipid film was fully dispersed in the aqueous medium. After the hydration process, the liposomal formulation was sonicated for 30 minutes. The formulation was then passed through an extruder five times to achieve uniformity. Finally, the liposomal vesicles were stored at 4 °C. The size, zeta potential, and polydispersity index (PDI) of the liposomes were performed using dynamic light scattering (DLS) with a Zetasizer Nano ZS (Malvern Instrument Ltd., UK). The liposomal dispersion was added to a glass cuvette and placed in the instrument (temperature 25°C, light scattering angle 90°). A capillary cuvette was used to measure the zeta potential. All measurements were performed in triplicate (Laouini et al., 2012).
2.2 Bacterial processing
All experiments utilized a laboratory strain of M. avium obtained from KWIKSTIK™, which was derived from ATCC 25291™. The M. avium culture was cultivated in 7H9 media from Hi Media (Santa Maria, CA, USA). The bacterial culture flasks and 7H9 media supplemented with albumin dextrose complex (ADC) were maintained in a 37°C incubator with 5% CO2. Before harvesting, the absorbance of the M. avium cell culture was measured at an optical density of 600 nm. M. avium was then harvested and processed in a static environment (without shaking) until it reached a logarithmic phase of growth. To disintegrate bacterial clumps and create a single-cell suspension, harvested M. avium was centrifuged and rinsed with 1X phosphate-buffered saline (PBS). The washed M. avium was then vortexed with 3 mm sterile glass beads for 3-minute intervals to break up bacterial clumps. The vortexed solution was filtered through a 5 µm filter to remove any remaining bacterial aggregates. The processed M. avium was serially diluted, plated on 7H11 agar, and incubated at 37°C to determine the bacterial count. Aliquots of the processed stock were placed in individual tubes, which were stored in a -80°C freezer until needed. All procedures were carried out under aseptic conditions within a Class II biochemical safety cabinet.
2.3 THP cell differentiation, infection, and antibiotic treatment
THP-1 cells from ATCC were cultured in RPMI-1640 medium obtained from Millipore Sigma-Aldrich and maintained in a 37°C incubator with 5% CO2. The cells were harvested for subsequent experiments. Before initiating the experiments, THP-1 cells were enumerated using a hemocytometer and trypan blue stain. A poly-L-lysine solution was applied to each well of a 96-well tissue culture plate for 1 h. The harvested THP-1 cells were treated with a 10 ng/mL solution of phorbol 12-myristate 13-acetate (PMA). The PMA-treated THP-1 cells (2x10^5 per well) were then added to each well in the 96-well tissue culture plate. The plate was placed in a 37°C incubator with 5% CO2 overnight to facilitate the differentiation of cells into macrophages before Day 0. After overnight incubation, each well was examined under a microscope to confirm the formation of a differentiated monolayer of cells. The supernatant was subsequently removed from each well. RPMI with 10% FBS,
infected with M. avium, was added to each well at a 1:1 concentration of M. avium to THP-1 cells (2x10^5 M. avium: 2x10^5 THP-1 cells). Following this addition, the 96-well plate was placed in a 37°C incubator with 5% CO2 for 1 h. After the incubation period, the supernatant was discarded, and unphagocytosed bacteria were removed by washing with a 1X PBS solution three times. Once unphagocytosed bacteria were removed, fresh RPMI with 10% FBS was added to each well. Various treatments were then administered to their corresponding wells, including liposomal [R4W4], liposomal AZ, liposomal RIF, liposomal encapsulated RIF+[R4W4], liposomal encapsulated AZ+[R4W4], free [R4W4], free RIF, free AZ, free AZ and [R4W4] together, and free RIF and [R4W4] together, each at different concentrations as specified in Table 2. Free peptides and antibiotics were dissolved in nanopure water and sterilized through a 0.22 µm filter prior to treatment. Water was used as a vehicle control for untreated cells.
Each treatment category was duplicated within the 96-well plate. After adding the treatments to their respective wells, the plate was incubated at 37°C with 5% CO2 for 3 hours. On Day 0, a specific number of wells were terminated for analysis. Sections of the 96-well plate were terminated on Day 0, Day 4, and Day 8, while treatments were administered on Day 0, Day 3, and Day 6. To terminate each well, the supernatant was discarded, and ice-cold nano-pure water was added. Slight friction was applied to release the cells, and the entire contents were removed. The samples were spread onto MiddleBrook 7H11 Agar Medium and placed in a 37°C incubator without CO2 for 11 days. After incubation, colonies of M. avium were counted.
2.4 Statistical analysis
Data was analyzed using GraphPad Prism software version 9.5.1. Treatment categories were analyzed using [ANOVA]. A p-value < 0.05 was considered statistically significant.
3 Results
3.1 Liposomal formulation and characterization
The liposomal formulations were characterized, and the results are presented in Table 3. The physicochemical properties of the formulations revealed mean particle sizes ranging from 126 to 232 nm. The blank formulation exhibited the smallest size at 126 nm, while the [R4W4]-loaded formulation showed an increased size of 156 nm. The addition of antibiotics further increased the particle size, with RIF-loaded liposomes measuring 181 nm and AZ-loaded liposomes measuring 210 nm. When both the [R4W4] and RIF were incorporated, the size reached 220 nm. Similarly, [R4W4] and AZ showed a size of 232 nm, which is the largest among all the prepared formulations. The polydispersity index (PDI) values for all formulations ranged from 0.125 to 0.255, indicating stable formulations. Additionally, the zeta potential of the formulations ranged from -3 to -7 mV, with these negative values suggesting reduced toxicity toward biological membranes. The cyclic peptide [R4W4] used in the formulations was synthesized using Fmoc/tBu solid-phase synthesis and characterized as previously reported (Riahifard et al., 2018). In this studies, Liposomal Combination (LC) refers to the use of two or more distinct liposomal formulations administered together to evaluate their comparative efficacy. In contrast, a Liposomal Encapsulated (LE) formulation refers to a single liposomal formulation in which either the cyclic peptide, a drug (AZ or RIF), or both are encapsulated within the liposomal structure. This study incorporates both approaches to assess their effectiveness in combating M. avium infections.
3.2 Survival of M. avium inside THP-1 macrophages treated with free form [R4W4]
In this study, we examined the antimicrobial effects of [R4W4] alone against M. avium to demonstrate its baseline antimicrobial activity on M. avium survivability. We evaluated M. avium survivability with untreated, serving as our control, and increasing concentrations of [R4W4] at 3 h and 4 d post-treatment. At 3 h post-treatment, [R4W4] demonstrated no significant reduction in M. avium colonies at 2 µg/mL and 4 µg/mL compared to control. However, [R4W4] at 8 µg/mL exhibited a significant reduction in M. avium colonies 3 h post-treatment (Figure 1A; Table 4). By day 4 post-treatment, [R4W4] showed a significant reduction in M. avium colonies at all 3 concentrations (Figure 1B; Table 4).
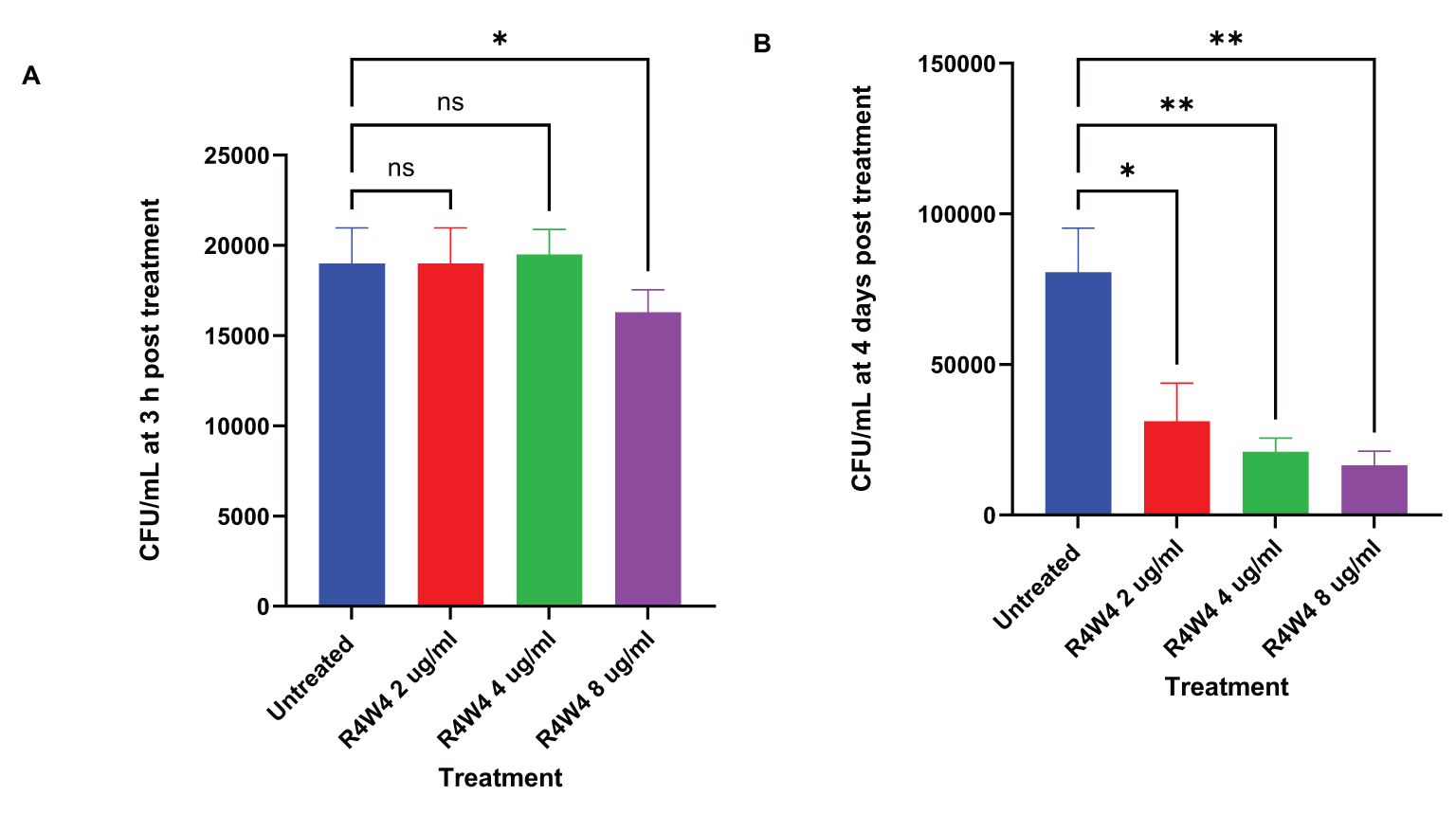
Figure 1. CFU counts of M. avium culture inside THP-1 cells treated with free form [R4W4]. (A) CFU/mL of M. avium treated with 2, 4, 8 µg/mL free [R4W4] at 3 h post-treatment. (B) CFU/mL of M. avium treated with 2, 4, 8 µg/mL free [R4W4] at 4 days post-treatment. M. avium was treated, then incubated at 37°C, and then terminated at 3 h and 4 days post-treatment. GraphPad Prism Software version 9.5.1 was utilized for analysis. Statistical analysis was performed using ANOVA. p-values are indicated at the top of each graph, and <0.05 (*) and <0.01 (**) were considered significant. Nonsignificant p-values are indicated as ns.
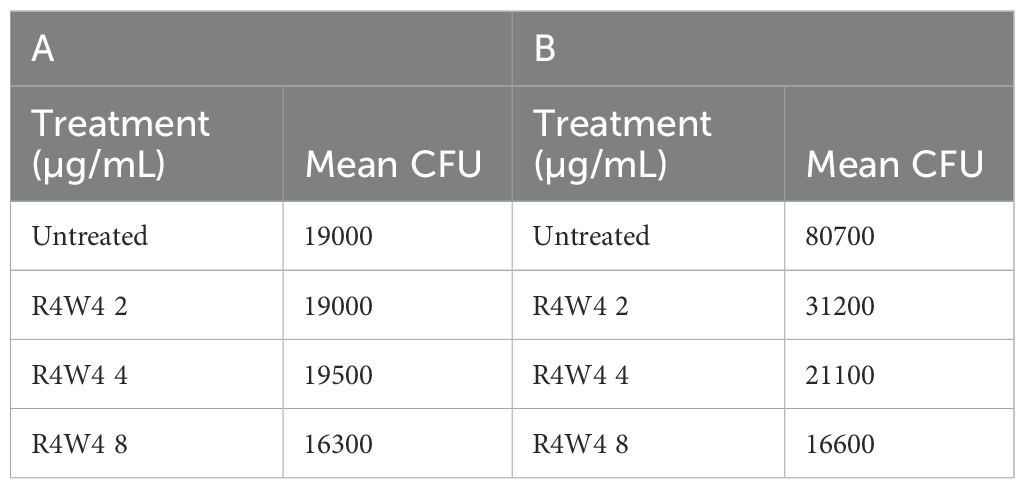
Table 4. Mean CFU Counts of M. avium treated with 2, 4, 8 µg/mL free [R4W4] at 3 h post-treatment (A) and at 4 days post-treatment (B).
3.3 Survival of M. avium inside THP-1 macrophages treated with free forms of rifampicin (RIF) and combination [R4W4]+RIF
We assessed the antimicrobial activity of RIF with and without adjunctive [R4W4] to determine if the addition of [R4W4] led to a significant reduction in M. avium survivability when compared to RIF alone. We again employed our control as untreated and evaluated increasing concentrations of RIF, as well as increasing concentrations of combination [R4W4]+RIF in their free forms at 3 h, 4 d, and 8 d post-treatment. At both 3 h and 4 days post-treatment, all treatments exhibited a significant reduction in M. avium colonies when compared to the untreated sample. Furthermore, each treatment demonstrated a more pronounced reduction in M. avium colonies at 4 days post-treatment than at 3 h post-treatment. Combination [R4W4]+RIF exhibited a significant reduction in M. avium colonies at RIF 4 µg/mL + [R4W4] 2 µg/mL when compared to RIF 4 µg/mL alone at both 3 h and 4 days post-treatment. However, other combination treatment concentrations did not display a significant reduction in M. avium colonies when compared to their respective treatment of free RIF at 3 h and 4 days post-treatment (Figures 2A, B; Table 5).
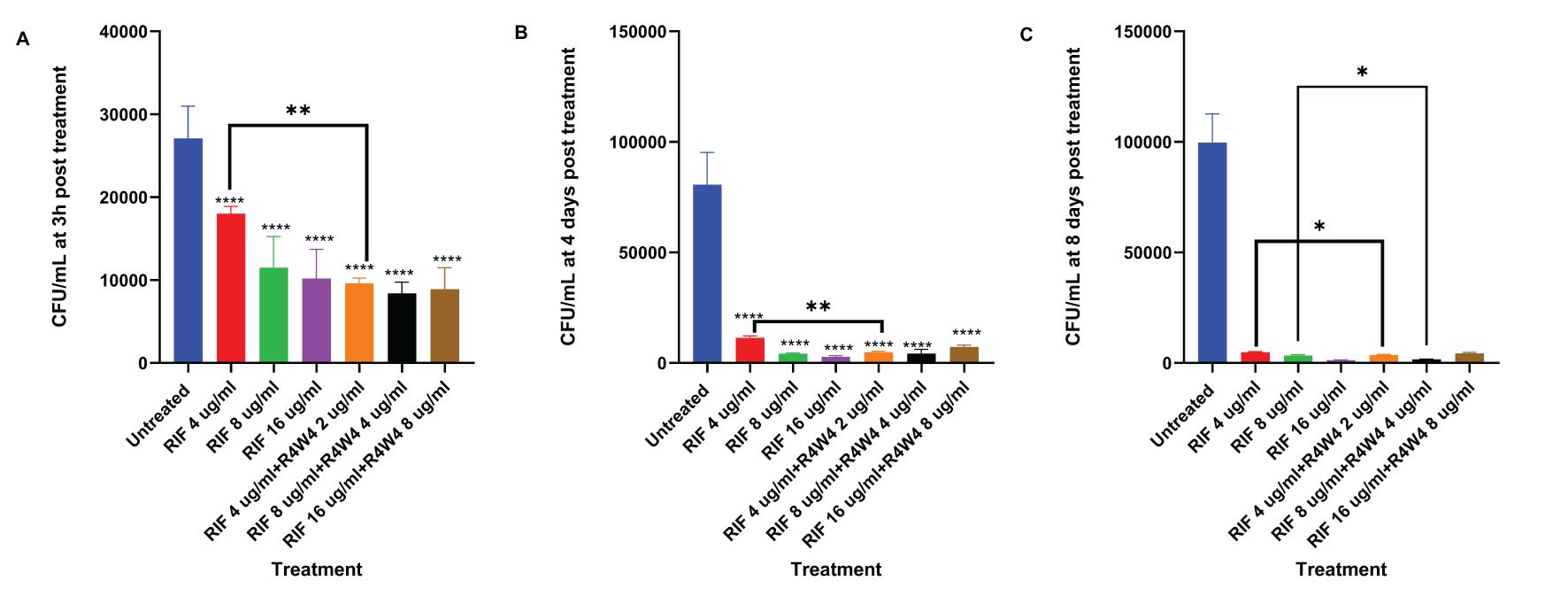
Figure 2. CFU counts M. avium culture inside THP-1 cells treated with free forms of RIF and combination [R4W4]+RIF. (A) CFU/mL of M. avium treated with 4, 8, 16 µg/mL free RIF and with 4, 8, 16 µg/mL free RIF with 2, 4, 8 µg/mL [R4W4], respectively, at 3 h post-treatment. (B) CFU/mL of M. avium treated with 4, 8, 16 µg/mL free RIF and with 4, 8, 16 µg/mL free RIF with 2, 4, 8 µg/mL [R4W4], respectively, at 4 days post-treatment. (C) CFU/mL of M. avium treated with 4, 8, 16 µg/mL free RIF and with 4, 8, 16 µg/mL free RIF with 2, 4, 8 µg/mL [R4W4], respectively, at 8 days post-treatment. GraphPad Prism Software version 9.5.1 was utilized for analysis. Statistical analysis was performed using ANOVA. p-values are indicated at the top of each graph, and <0.05 (*) and <0.01 (**) were considered significant. ****=P value <0.0001.
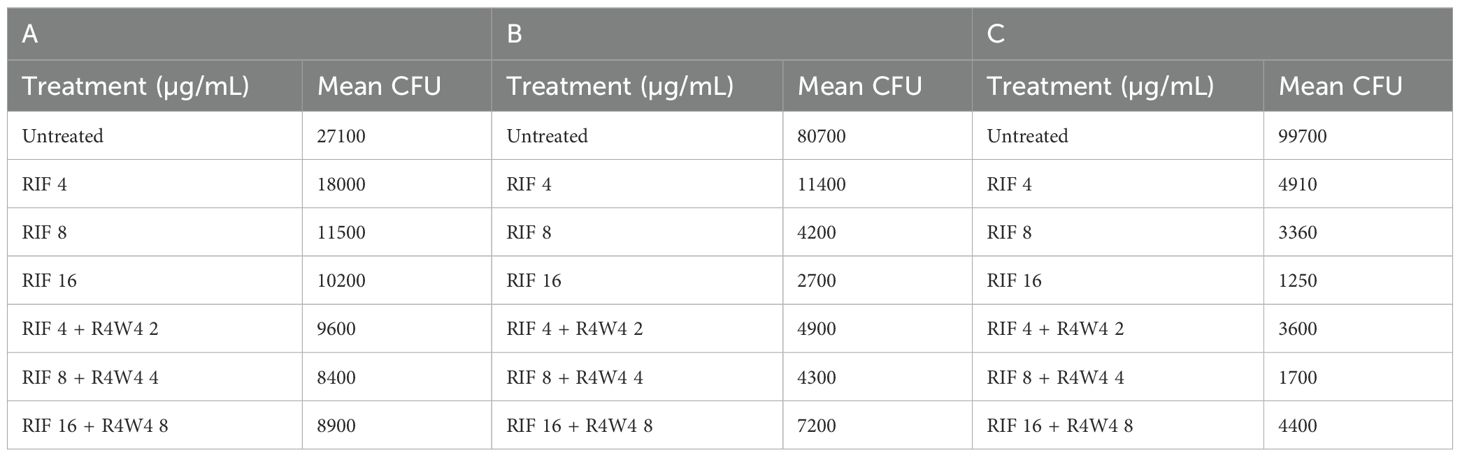
Table 5. Mean CFU Counts of M. avium treated with 4, 8, 16 µg/mL free RIF and with 4, 8, 16 µg/mL free RIF with 2, 4, 8 µg/mL [R4W4], respectively, at 3 h post-treatment (A), 4 days post-treatment (B), and 8 days post-treatment (C).
By 8 days post-treatment, all treatments showed a significant reduction in M. avium colonies compared to the untreated sample. Combination [R4W4]+RIF continued to exhibit a significant reduction of M. avium colonies at RIF 4 µg/mL + [R4W4] 2 µg/mL compared to RIF 4 µg/mL alone. Similarly, combination [R4W4]+RIF demonstrated a significant reduction of M. avium colonies at RIF 8µg/mL + [R4W4] 4 µg/mL compared to RIF 8 µg/mL alone at 8 days post-treatment. However, RIF 16µg/mL + [R4W4] 8 µg/mL showed no statistical significance when compared to RIF 8 µg/mL alone at 8 days post-treatment (Figure 2C; Table 5).
3.4 Survival of M. avium inside THP-1 macrophages treated with liposomal forms of RIF, liposomal combination [R4W4]+RIF (LC), and liposomal encapsulated [R4W4]+RIF (LE)
In order to assess the optimal combination of RIF and [R4W4], we proceeded to compare the liposomal combination with the liposomal encapsulated formulation of RIF and [R4W4]. Utilizing the same concentration titration and time intervals, we separated the [R4W4]+RIF into liposomal combination and liposomal encapsulated formulations. At 3 h post-treatment, only liposomal RIF at concentrations of 4 µg/mL and 8 µg/mL exhibited a statistically significant reduction in M. avium colonies compared to the untreated sample. None of the combination treatments demonstrated a statistically significant reduction in M. avium colonies at 3h when compared to their corresponding concentration of RIF alone (Figure 3A; Table 6). However, liposomal combination [R4W4] 4 µg/mL + RIF 8 µg/mL (LC) showed a significant increase in M. avium colonies compared to RIF 8 µg/mL (LC) alone.
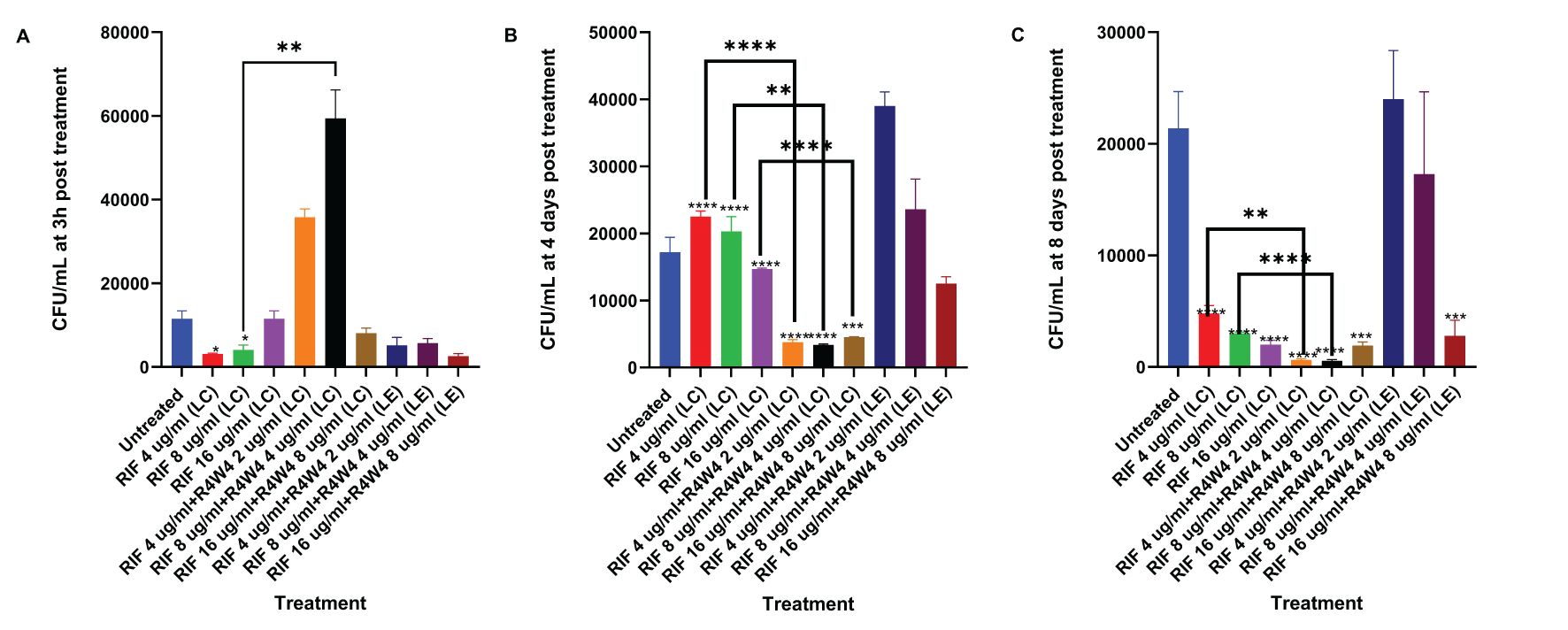
Figure 3. CFU counts of M. avium culture inside THP-1 cells treated with liposomal forms of RIF, liposomal combination [R4W4]+RIF (LC), and liposomal encapsulated [R4W4]+RIF (LE). (A) CFU/mL of M. avium treated with 4, 8, 16 µg/mL liposomal RIF and with 4, 8, 16 µg/mL liposomal RIF combination (LC) and encapsulated (LE) with 2, 4, 8 µg/mL [R4W4], respectively, at 3 h post-treatment. (B) CFU/mL of M. avium treated with 4, 8, 16 µg/mL liposomal RIF and with 4, 8, 16 µg/mL liposomal RIF combination (LC) and encapsulated (LE) with 2, 4, 8 µg/mL [R4W4], respectively, at 4 days post-treatment. (A–C) CFU/mL of M. avium treated with 4, 8, 16 µg/mL liposomal RIF and with 4, 8, 16 µg/mL liposomal RIF combination (LC) and encapsulated (LE) with 2, 4, 8 µg/mL [R4W4], respectively, at 8 days post-treatment. GraphPad Prism Software version 9.5.1 was utilized for analysis. Statistical analysis was performed using ANOVA. p-values are indicated at the top of each graph, and <0.05 (*), <0.01 (**), and <0.0001 (****) were considered significant.
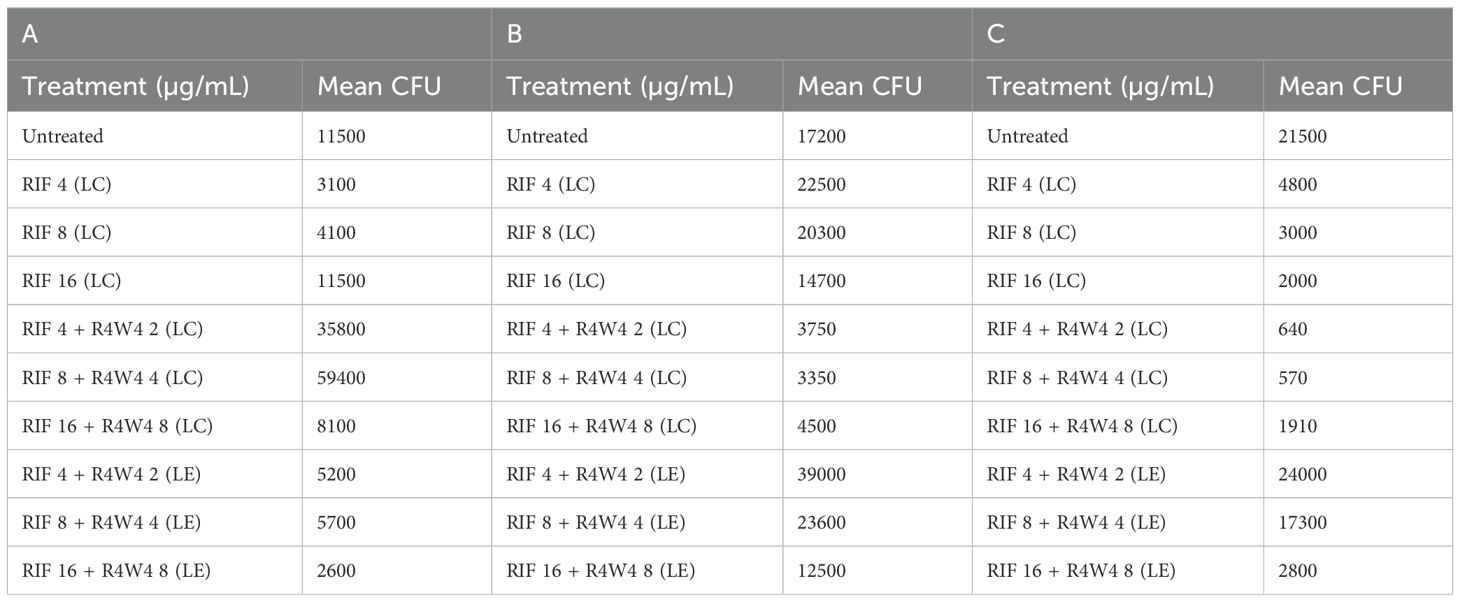
Table 6. Mean CFU Counts of M. avium treated with 4, 8, 16 µg/mL liposomal RIF and with 4, 8, 16 µg/mL liposomal RIF combination (LC) and encapsulated (LE) with 2, 4, 8 µg/mL [R4W4], respectively, at 3 h post-treatment (A), 4 days post-treatment (B), and 8 days post-treatment (C).
By 4 days post-treatment, all liposomal combination treatments of [R4W4]+RIF (LC) displayed a statistically significant reduction in M. avium colonies compared to their respective concentrations of liposomal RIF alone. However, liposomal encapsulated [R4W4]+RIF (LE) did not show any statistically significant reduction in M. avium colonies compared to their respective concentrations of RIF alone (Figure 3B; Table 6).
At 8 days post-treatment, liposomal combination treatments of [R4W4]+RIF (LC) at RIF 4 µg/mL + [R4W4] 2 µg/mL and at RIF 8 µg/mL + [R4W4] 4 µg/mL (LC) showed a statistically significant reduction in M. avium colonies compared to liposomal RIF 4 µg/mL and 8 µg/mL alone, respectively. However, liposomal combination treatments of [R4W4]+RIF (LC) at concentration of RIF 16 µg/mL + [R4W4] 8 µg/mL (LC) did not show a statistically significant reduction in M. avium colonies compared to liposomal RIF 8 µg/mL alone. All liposomal encapsulated [R4W4]+RIF (LE) formulations did not show any statistically significant reduction in M. avium colonies compared to their respective concentrations of RIF alone (Figure 3C; Table 6). Next we turned our attention to another first-line therapy for comparison, Azithromycin.
3.5 Survival of M. avium inside THP-1 macrophages treated with free forms of azithromycin (AZ) and combination [R4W4]+AZ
We began by evaluating the antimicrobial efficacy of AZ against M. avium survivability with and without [R4W4]. We evaluated M. avium survivability with untreated, serving as our control, and increasing concentrations of AZ and [R4W4]+AZ in their free forms at 3 h, 4 d, and 8 d post-treatment. At 3 h, 4 days, and 8 days post-treatment, there was no statistically significant decrease in M. avium cell counts observed for any of the treatment concentrations of AZ+[R4W4] compared to AZ alone at their respective concentrations (Figures 4A–C; Table 7). We then investigated whether AZ and [R4W4] demonstrated a significant difference in efficacy when in liposomal combination or liposomal encapsulated formulations.
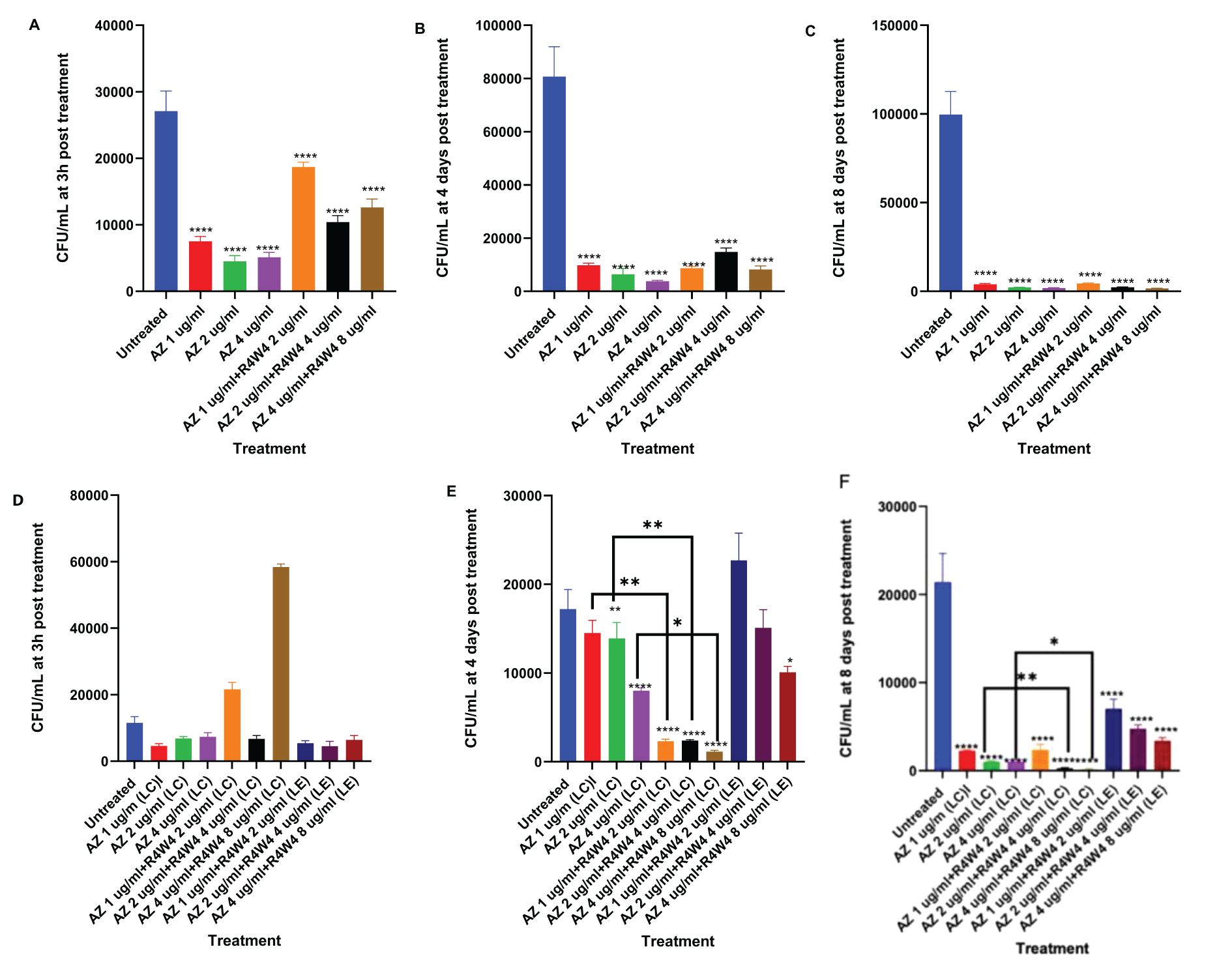
Figure 4. (A–C) CFU counts M. avium culture inside THP-1 cells treated with free forms of AZ and unconjugated [R4W4]+AZ. (A) CFU/mL of M. avium treated with 1, 2, 4 µg/mL free AZ and with 1, 2, 4 µg/mL free AZ with 2, 4, 8 µg/mL [R4W4] (unconjugated), respectively, at 3 h post-treatment. (B) CFU/mL of M. avium treated with 1, 2, 4 µg/mL free AZ and with 1, 2, 4 µg/mL free AZ with 2, 4, 8 µg/mL [R4W4] (unconjugated), respectively, at 4 days post-treatment. (C) CFU/mL of M. avium treated with 1, 2, 4 µg/mL free AZ and with 1, 2, 4 µg/mL free AZ with 2, 4, 8 µg/mL [R4W4] (unconjugated), respectively, at 8 days post-treatment. M. avium was treated, then incubated at 37°C and then terminated at 3 h, 4 days, and 8 days post-treatment. (D–F) CFU counts of M. avium culture inside THP-1 cells treated with liposomal forms of AZ, liposomal combination [R4W4]+AZ (LC), and liposomal encapsulated [R4W4]+AZ (LE). (D) CFU/mL of M. avium treated with 1, 2, 4 µg/mL liposomal AZ and with 1, 2, 4 µg/mL liposomal AZ combination (LC) and encapsulated (LE) with 2, 4, 8 µg/mL [R4W4], respectively, at 3 h post-treatment. (E) CFU/mL of M. avium treated with 1, 2, 4 µg/mL liposomal AZ and with 1, 2, 4 µg/mL liposomal AZ combination (LC) and encapsulated (LE) with 2, 4, 8 µg/mL [R4W4], respectively, at 4 days post-treatment. (F) CFU/mL of M. avium treated with 1, 2, 4 µg/mL liposomal AZ and with 1, 2, 4 µg/mL liposomal AZ combination (LC) and encapsulated (LE) with 2, 4, 8 µg/mL [R4W4], respectively, at 8 days post-treatment. GraphPad Prism Software version 9.5.1 was utilized for analysis. Statistical analysis was performed using ANOVA. p-values are indicated at the top of each graph, and <0.05 (*), <0.01 (**), and <0.0001 (****) were considered significant.
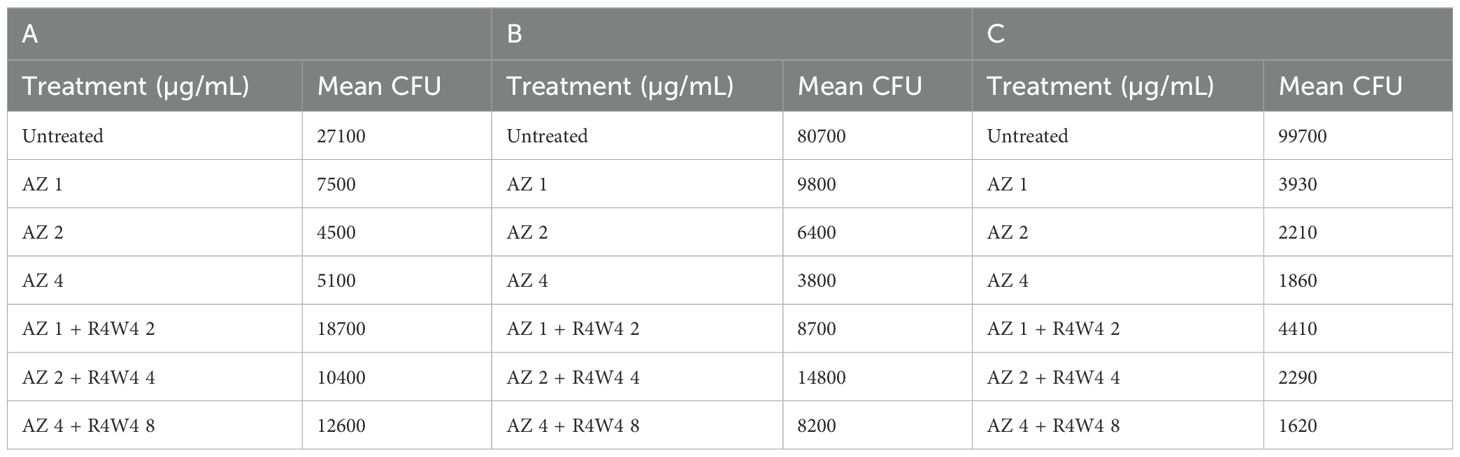
Table 7. Mean CFU Counts of M. avium treated with 1, 2, 4 µg/mL free AZ and with 1, 2, 4 µg/mL free AZ with 2, 4, 8 µg/mL [R4W4] (unconjugated), respectively, at 3 h post-treatment (A), 4 days post-treatment (B), and 8 days post-treatment (C).
3.6 Survival of M. avium inside THP-1 macrophages treated with liposomal forms of azithromycin (AZ), liposomal combination [R4W4]+AZ (LC), and liposomal encapsulated [R4W4]+AZ (LE)
To determine the optimal delivery for AZ and [R4W4], we again tested the liposomal combination and liposomal encapsulated formulations to determine if there was a significant difference in M. avium survivability. Utilizing the same concentration titration and time intervals, we separated AZ and [R4W4]+AZ into liposomal combination and liposomal encapsulated formulations. At 3 h post-treatment, none of the AZ+[R4W4] combination treatments, both in encapsulated (LE) and combination (LC) formulations, demonstrated a statistically significant decrease in M. avium colonies compared to AZ alone at their respective concentrations (Figure 4D; Table 8).
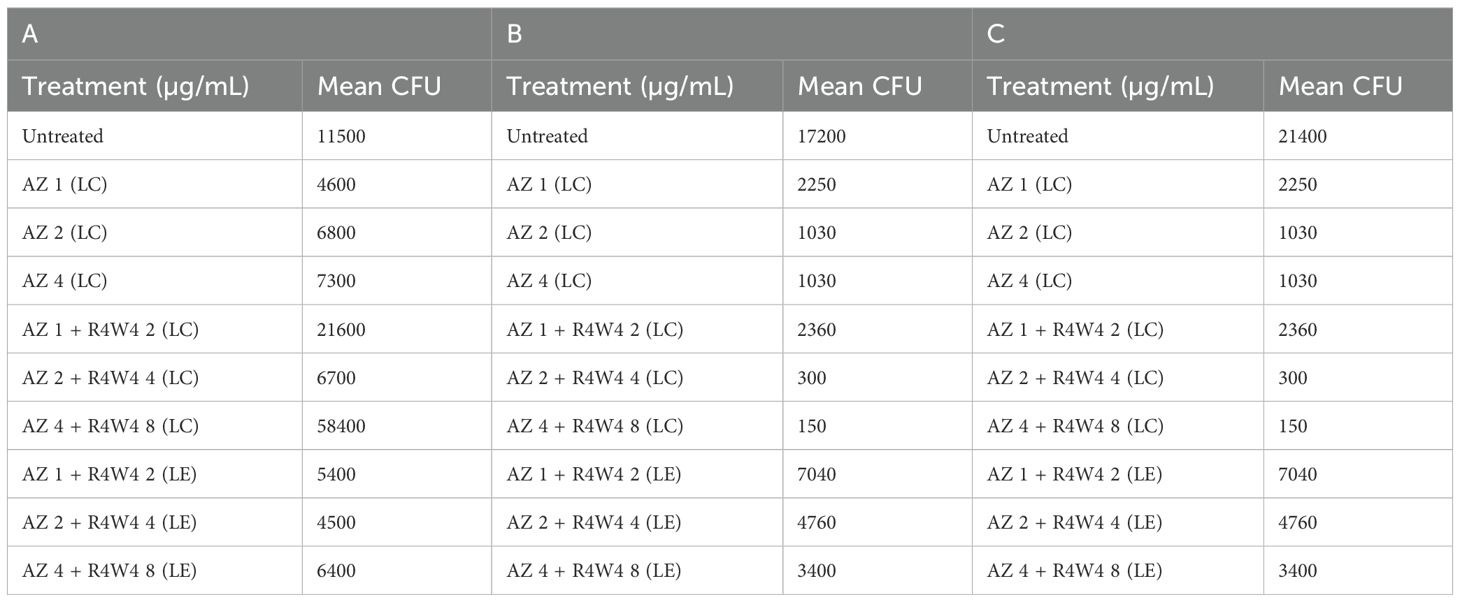
Table 8. Mean CFU Counts of M. avium treated with 1, 2, 4 µg/mL liposomal AZ and with 1, 2, 4 µg/mL liposomal AZ combination (LC) and encapsulated (LE) with 2, 4, 8 µg/mL [R4W4], respectively, at 3 h post-treatment (A), 4 days post-treatment (B), and 8 days post-treatment (C).
By 4 days post-treatment, all liposomal combination treatments of [R4W4]+AZ (LC) exhibited a statistically significant reduction in M. avium colonies compared to liposomal AZ alone at their respective concentrations. However, all liposomal encapsulated treatments of [R4W4]+AZ (LE) did not display a statistically significant reduction in M. avium colonies compared to liposomal AZ alone at their respective concentrations (Figure 4E; Table 8).
At 8 days post-treatment, liposomal combination treatments of [R4W4]+AZ (LC) at AZ 1 µg/mL + [R4W4] 2 µg/mL (LC) did not show a significant reduction in M. avium colonies compared to liposomal AZ 1 µg/mL alone. Liposomal combination treatments of [R4W4]+AZ (LC) at AZ 2 µg/mL + [R4W4] 4 µg/mL (LC) and at AZ 4 µg/mL + [R4W4] 8 µg/mL (LC) demonstrated a statistically significant reduction in M. avium colonies compared to liposomal AZ 2 µg/mL and AZ 4 µg/mL alone, respectively. However, none of the liposomal encapsulated treatments of [R4W4]+AZ (LE) showed a statistically significant decrease in M. avium colonies compared to liposomal AZ alone at their respective concentrations (Figure 4F; Table 8).
4 Discussion
While guideline-directed therapy has led to a standardized treatment intended to contain infection, the increased use of first-line antibiotics yields drug-resistant strains of bacteria (Diel et al., 2018b; Ito et al., 2022). For example, RIF resistance in M. tuberculosis results from the mutated rifampicin resistance determining region (RRDR) of the rpoB gene (Rukasha et al., 2016; Muthaiah et al., 2017). The rpoB gene encodes the M. tuberculosis RNA polymerase (RNAP) beta subunit which is the target of RIF (Campbell et al., 2001; Muthaiah et al., 2017). AZ resistance arises most often from a point mutation at position 2058 or 2059 in the 23S ribosomal ribonucleic acid (rRNA) gene, which results in AZ being unable to bind ribosomes (Saxena et al., 2021; Nguyen and Daley, 2023). Recent research has explored the use of cyclic peptides as treatment options for multidrug-resistant bacterial infections. Several cyclic peptides have been studied against mycobacteria, including Ecumicin, Cyclomarin A, and Lassomycin (Gao et al., 2015; Jin et al., 2016; Maurer et al., 2019; Zhu et al., 2019). Our laboratory has previously demonstrated that [R4W4] is efficacious against M. tuberculosis when used in conjunction with first-line antibiotics isoniazid (INH) and pyrazinamide (PZA). We have also demonstrated that [R4W4] is efficacious against M. avium when used in conjunction with first-line antibiotics RIF and AZ (Hernandez et al., 2020; Kelley et al., 2023). There has yet to be research evaluating the optimal delivery method for adjunctive therapy of [R4W4].
This study aimed to determine the efficacy of [R4W4] in controlling the M. avium burden in THP-1 cells when used in combination with or encapsulated to first-line antibiotic therapy and when delivered in free form or a liposomal formulation. We used biocompatible lipids in the liposome composition, which presents a promising approach for drug delivery (Dymek and Sikora, 2022), particularly in pulmonary applications as reported by others (Elhissi, 2017). Liposomal formulations with DOPCE, Cholesterol, and DSPE-mPEG-2000 are particularly suited for drugs that are hydrophobic, prone to degradation, require controlled release, or have poor pharmacokinetic profiles. The inclusion of PEGylation helps in prolonging circulation time and reducing immunogenicity, making these formulations highly effective for targeted drug delivery and improving therapeutic outcomes (Dymek and Sikora, 2022). The small diameter of the prepared liposomes, especially those under 300 nm, offers a significant advantage in pulmonary drug delivery, as these smaller sizes have been shown to enhance drug deposition in the lungs (Chono et al., 2009; Mehta et al., 2020). The polydispersity index (PDI) values of all formulations were below 0.3, indicating the homogeneity of the prepared liposomes with minimal aggregation (Danaei et al., 2018). The zeta potential of the liposomes serves as an important indicator of formulation stability, with a negative charge contributing to reduced toxicity for biological membranes (Martins et al., 2013). These characteristics further support the potential of the prepared liposomal formulation for safe and effective pulmonary therapies. [R4W4] exerts its antibacterial effect due to its amphipathic nature and interaction with negatively charged phospholipids to perturb bacterial membranes (Oh et al., 2014). We first demonstrated the antibacterial effect of [R4W4] by treating THP-1 with free [R4W4] and observing CFU at 3 h and 4 days post-infection. These results are consistent with previous findings (Kelley et al., 2023).
In this study, we utilized THP-1-derived macrophages as an in vitro model to evaluate the intracellular efficacy of our liposomal cyclic peptide formulation in combination with first-line therapy against M. avium. While primary human alveolar macrophages would more closely mimic the in vivo lung environment, THP-1 cells are widely used in mycobacterial research due to their consistency, ease of culture, and ability to differentiate into macrophage-like cells that exhibit key functional properties, such as phagocytosis and intracellular bacterial survival. One limitation of using THP-1 cells is that they may not fully replicate the heterogeneity and immune responses of primary alveolar macrophages. However, the reproducibility and controlled conditions provided by THP-1 cells allow for reliable comparative analyses of treatment efficacy. Future studies utilizing primary human alveolar macrophages or in vivo models will be essential to further validate our findings and confirm the translational potential of this adjunct therapy for drug-resistant M. avium.
Our study revealed a significant reduction in the intracellular viability of M. avium when THP-1 cells were treated with liposomal formulations containing combination RIF+[R4W4] and AZ+[R4W4] when compared to liposomes containing either RIF or AZ alone, respectively. We found that in their free forms, there was no significant difference in M. avium survival when comparing AZ+[R4W4] versus AZ alone. For free-form RIF and RIF+[R4W4], there was a significant difference in M. avium survival when comparing 4 µg/mL RIF and 4 µg/mL RIF + 2 µg/mL [R4W4] at 3 hours, 4 days, and 8 days. There was also a significant difference between 8 µg/mL RIF and 8 µg/mL RIF + 4 µg/mL [R4W4] in only 8 days. These results are consistent with our previous findings demonstrating that cyclic peptide [R4W4] efficacy did not change at 8 µg/mL (Kelley et al., 2023).
When delivered in liposomal formulation, we observed a significant increase in synergy between [R4W4] and AZ or RIF as well as a significant difference in potency between liposomal encapsulated and liposomal combination forms. In Figures 3A, 4D, the treatments with liposomal combinations of RIF or AZ and [R4W4] showed a 5-10 fold increase in CFU after 3 hours compared to pretreatment levels. Both experiments were conducted simultaneously, and this unexpected increase in CFU may be attributed to experimental errors, such as human error, or possibly to the excess lipids in the liposomal formulations. The presence of these lipids might have provided a growth substrate for the bacteria, particularly in cases where there was limited drug or peptide release from the liposomes. However, it is important to note that as the treatment progressed to 4 and 8 days, there was a marked reduction in CFU counts for these formulations compared to pretreatment levels. Thus, we believe it is valuable to include the original CFU data in the figures, even though it underscores an unusual observation in the early time points.
All concentrations of liposomal combination RIF+[R4W4] and AZ+[R4W4] demonstrated significant efficacy at 4 days when compared to either antibiotic alone. At 8 days, a significant reduction in M. avium survival was observed in liposomal combination 4 µg/mL RIF + 2 µg/mL [R4W4] and 8 µg/mL RIF + 4 µg/mL [R4W4] versus RIF alone, as well as liposomal combination 2 µg/mL AZ + 4 µg/mL [R4W4] and 4 µg/mL AZ + 8 µg/mL [R4W4] versus AZ alone. All liposomal encapsulated forms yielded no significant reduction in M. avium survival. The findings in our study demonstrate that potency of RIF and AZ against M. avium can be enhanced by delivering the antibiotics in a liposomal combination with [R4W4]. In contrast, the encapsulated liposomal formulation may exhibit altered release kinetics, likely due to the strong entrapment of antibiotics or [R4W4] within the liposome core, stemming from differences in their physicochemical properties. This could result in less effective synergistic interactions, leading to reduced therapeutic efficacy compared to the combination of separate liposomal formulations. The variability in the combinational effects of [R4W4] with RIF or AZ likely arises from several factors, including experimental conditions, the specific formulations used, and the pharmacokinetics of the drugs. Positive combinational effects were observed under conditions where the concentrations of RIF or AZ and the cyclic peptide, along with the release rates and uptake by macrophages, were optimal, resulting in enhanced bacterial killing. Conversely, antagonistic effects were observed in other conditions, which may be attributed to suboptimal drug release from the liposomal formulations or potential interference between the drugs and the peptide. For example, the liposomal formulations might have altered the bioavailability of the drugs, impacting their interaction with M. avium. Additionally, variations in macrophage uptake or the bacteria’s ability to utilize the liposomal lipids for growth could have further contributed to this inconsistency. Further studies are needed to optimize and validate the release of antibiotics and [R4W4] from the encapsulated liposomal preparation.
The findings of this study are presented with limitations. The strain of M. avium (Mycobacterium avium subsp. avium) employed in this study was obtained from the liver of a hen infected with M. avium, raising the possibility of constraints on the relevance of the findings to M. avium strains prevalent in humans (Sattar et al., 2021). Hence, we suggest conducting additional cyclic peptide [R4W4] studies specifically on M. avium subsp. hominissuis, the strain commonly identified in humans. This would help validate the effectiveness of cyclic peptide [R4W4] as a treatment approach for M. avium complex disease in human subjects (Shin and Shin, 2021).
Exploring novel approaches to test macrophage response opens avenues to monitor the effectiveness of emerging treatments in eliciting an appropriate macrophage response. In a study examining macrophage–pathogen interactions in zebrafish models, diverse methods were employed to assess macrophage functions. These methods included measuring reactive oxygen species (ROS) and reactive nitrogen species (RNS) response levels, calcium effluxes, apoptosis, and ATP usage (Torraca et al., 2014). Utilizing these techniques enables the evaluation of the efficacy of cyclic peptides in restraining M. avium infection by gauging the activation of macrophages.
Our findings suggest that the liposomal cyclic peptide formulation, when used in combination with RIF and AZ, has the potential to enhance treatment efficacy against M. avium, particularly in the context of drug-resistant infections. One of the major challenges in treating mycobacterial infections is the intracellular persistence of bacteria within macrophages, which limits drug penetration and reduces therapeutic efficacy. The use of liposomal encapsulation in our formulation improves drug delivery by enhancing cellular uptake, prolonging drug release, and maintaining therapeutic concentrations at the site of infection. Additionally, cyclic peptides have demonstrated antimicrobial activity through membrane disruption and potential inhibition of efflux pumps, mechanisms that could enhance the intracellular activity of rifampin and isoniazid. By increasing bacterial membrane permeability and reducing active drug efflux, the cyclic peptide component of our formulation may potentiate the effects of conventional antibiotics, thereby improving bacterial clearance. Furthermore, adjunctive combination therapy offers a strategy to combat antimicrobial resistance by targeting multiple bacterial pathways simultaneously. This reduces the selective pressure on individual drugs and minimizes the risk of resistance development. Given the increasing prevalence of drug-resistant M. avium and other nontuberculous mycobacterial infections, our approach represents a promising avenue for improving treatment outcomes.
In this study, we utilized M. avium of hen origin as a model to evaluate the efficacy of our liposomal cyclic peptide formulation in combination with first-line therapy. While there may be strain-specific differences between avian and human M. avium isolates, previous research has demonstrated that avian-derived M. avium strains share key genetic, phenotypic, and pathogenic characteristics with clinical human isolates. Notably, both avian and human strains exhibit similar mechanisms of intracellular survival, drug susceptibility patterns, and biofilm formation, making avian M. avium a suitable model for studying potential therapeutic interventions. However, we acknowledge that direct generalization to human infections requires further validation. Future studies utilizing clinical human M. avium isolates will be essential to confirm the translational relevance of our findings and further assess the efficacy of our liposomal formulation in a broader range of M. avium complex (MAC) infections. Despite this limitation, our results provide valuable insights into the potential application of liposomal cyclic peptides in combination therapy for drug-resistant M. avium infections.
Moving forward, the next steps for these findings involve assessing the effectiveness of cyclic peptide [R4W4] and combination treatments in the context of an active pulmonary Mycobacterium avium complex (MAC) infection using a murine model. Traditionally, C57BL/6, Balb/c, nude, and beige mice have been employed in M. avium infection studies (Verma et al., 2019). However, the C3HeB/Fej mouse strain has recently demonstrated necrotic foci during granuloma formation, resembling observations in humans and not typically seen in other mouse models. This makes the C3HeB/Fej mice a promising model for evaluating the efficacy of cyclic [R4W4] combination treatment during an active pulmonary MAC infection (Henao-Tamayo et al., 2015; Verma et al., 2019). [R4W4] could potentially be used both systemically and locally, depending on the delivery method. While systemic delivery would require further optimization to ensure safety and efficacy, localized delivery—such as targeting the lungs in MAC infections—could be a viable approach, particularly with liposomal formulations. To date, none of the preparations used in this study have been tested in animal models. Future work will focus on evaluating these formulations in vivo to determine their therapeutic potential and pharmacokinetics. Additionally, we aim to study the efficacy of our combination therapy when used on human-specific M. avium subspecies, such as M. avium subsp. hominissuis and M. avium subsp. paratuberculosis (Verma et al., 2019). Even though cytotoxicity doses have been documented in vitro, it is imperative to conduct randomized placebo-controlled clinical trials (RCTs) in healthy human subjects to comprehensively evaluate the safety and tolerability of the compound. Following the establishment of safety parameters, RCTs involving patients with active pulmonary Mycobacterium avium complex (MAC) infection are essential to substantiate cyclic [R4W4] as an adjunctive treatment for human MAC infection. This progression would pave the way for implementing these findings in the treatment of immunocompromised individuals with M. avium infection. However, careful consideration of safety, dosage volumes, and potential alterations in administration methods would be crucial in this transition.
Data availability statement
The original contributions presented in the study are included in the article/supplementary material. Further inquiries can be directed to the corresponding authors.
Ethics statement
Ethical approval was not required for the studies on humans in accordance with the local legislation and institutional requirements because only commercially available established cell lines were used.
Author contributions
MK: Data curation, Writing – original draft, Writing – review & editing. KS: Data curation, Methodology, Writing – original draft, Writing – review & editing. AB: Data curation, Writing – original draft, Writing – review & editing. IG: Writing – original draft, Writing – review & editing. AA: Data curation, Writing – review & editing. NR: Data curation, Writing – original draft, Writing – review & editing. RT: Conceptualization, Data curation, Investigation, Writing – original draft, Writing – review & editing. VV: Conceptualization, Data curation, Funding acquisition, Investigation, Methodology, Writing – original draft, Writing – review & editing.
Funding
The author(s) declare that financial support was received for the research and/or publication of this article. This study was supported in part by the Potts Memorial Foundation, Hudson, NY, USA and NIH-NHLBI (2R15HL143545-02).
Acknowledgments
We appreciate the feedback from Drs. Maria Lambros and Miou Zhou.
Conflict of interest
The authors declare that the research was conducted in the absence of any commercial or financial relationships that could be construed as a potential conflict of interest.
Generative AI statement
The author(s) declare that no Generative AI was used in the creation of this manuscript.
Publisher’s note
All claims expressed in this article are solely those of the authors and do not necessarily represent those of their affiliated organizations, or those of the publisher, the editors and the reviewers. Any product that may be evaluated in this article, or claim that may be made by its manufacturer, is not guaranteed or endorsed by the publisher.
References
Adjemian, J., Olivier, K. N., Seitz, A. E., Holland, S. M., Prevots, D. R. (2012). Prevalence of nontuberculous mycobacterial lung disease in U.S. Medicare beneficiaries. Am. J. Respir. Crit. Care Med. 185, 881–886. doi: 10.1164/rccm.201111-2016OC
Bulbake, U., Doppalapudi, S., Kommineni, N., Khan, W. (2017). Liposomal formulations in clinical use: an updated review. Pharmaceutics. 9. doi: 10.3390/pharmaceutics9020012
Busatto, C., Vianna, J. S., da Silva, L. V. J., Ramis, I. B., da Silva, P. E. A. (2019). Mycobacterium avium: an overview. Tuberculosis (Edinb). 114, 127–134. doi: 10.1016/j.tube.2018.12.004
Campbell, E. A., Korzheva, N., Mustaev, A., Murakami, K., Nair, S., Goldfarb, A., et al. (2001). Structural mechanism for rifampicin inhibition of bacterial RNA polymerase. Cell. 104, 901–912. doi: 10.1016/S0092-8674(01)00286-0
Cano Rodríguez, C., Castañer González, E., Andreu Magarolas, M., Gallardo Cistare, X., González López, A., Cuevas Lobato, Ó., et al. (2023). Lung infection with nontuberculous mycobacteria. Radiología (English Edition). 65, 392–401. doi: 10.1016/j.rxeng.2023.09.001
Chibowski, E., Szcześ, A. (2016). Zeta potential and surface charge of DPPC and DOPC liposomes in the presence of PLC enzyme. Adsorption 22, 755–765. doi: 10.1007/s10450-016-9767-z
Chono, S., Fukuchi, R., Seki, T., Morimoto, K. (2009). Aerosolized liposomes with dipalmitoyl phosphatidylcholine enhance pulmonary insulin delivery. J. Control Release. 137, 104–109. doi: 10.1016/j.jconrel.2009.03.019
Daley, C. L., Iaccarino, J. M., Lange, C., Cambau, E., Wallace, R. J., Jr., Andrejak, C., et al. (2020). Treatment of nontuberculous mycobacterial pulmonary disease: an official ATS/ERS/ESCMID/IDSA clinical practice guideline. Clin. Infect. Dis. 71, e1–e36. doi: 10.1183/13993003.00535-2020
Danaei, M., Dehghankhold, M., Ataei, S., Hasanzadeh Davarani, F., Javanmard, R., Dokhani, A., et al. (2018). Impact of particle size and polydispersity index on the clinical applications of lipidic nanocarrier systems. Pharmaceutics 10. doi: 10.3390/pharmaceutics10020057
Diel, R., Lipman, M., Hoefsloot, W. (2018a). High mortality in patients with Mycobacterium avium complex lung disease: a systematic review. BMC Infect. diseases. 18, 1–10. doi: 10.1186/s12879-018-3113-x
Diel, R., Nienhaus, A., Ringshausen, F. C., Richter, E., Welte, T., Rabe, K. F., et al. (2018b). Microbiologic outcome of interventions against mycobacterium avium complex pulmonary disease: A systematic review. Chest. 153, 888–921. doi: 10.1016/j.chest.2018.01.024
Dymek, M., Sikora, E. (2022). Liposomes as biocompatible and smart delivery systems - the current state. Adv. Colloid Interface Sci. 309, 102757. doi: 10.1016/j.cis.2022.102757
Elhissi, A. (2017). Liposomes for pulmonary drug delivery: the role of formulation and inhalation device design. Curr. Pharm. Des. 23, 362–372. doi: 10.2174/1381612823666161116114732
Ferreira, M., Ogren, M., Dias, J. N. R., Silva, M., Gil, S., Tavares, L., et al. (2021). Liposomes as antibiotic delivery systems: A promising nanotechnological strategy against antimicrobial resistance. Molecules 26. doi: 10.3390/molecules26072047
Findlay, B., Zhanel, G. G., Schweizer, F. (2010). Cationic amphiphiles, a new generation of antimicrobials inspired by the natural antimicrobial peptide scaffold. Antimicrob. Agents Chemother. 54, 4049–4058. doi: 10.1128/AAC.00530-10
Fujishima, N., Komiya, K., Yamasue, M., Hiramatsu, K., Kadota, J.-I. (2023). A Systematic Review of Factors Associated with Mortality among Patients with Mycobacterium avium Complex Lung Disease. Pathogens. 12, 1331. doi: 10.3390/pathogens12111331
Gao, W., Kim, J.-Y., Anderson, J. R., Akopian, T., Hong, S., Jin, Y.-Y., et al. (2015). The cyclic peptide ecumicin targeting ClpC1 is active against Mycobacterium tuberculosis in vivo. Antimicrobial Agents chemotherapy 59, 880–889. doi: 10.1128/AAC.04054-14
Haworth, C. S., Banks, J., Capstick, T., Fisher, A. J., Gorsuch, T., Laurenson, I. F., et al. (2017). British Thoracic Society guidelines for the management of non-tuberculous mycobacterial pulmonary disease (NTM-PD). Thorax 72, ii1–ii64. doi: 10.1136/thoraxjnl-2017-210929
He, Y., Zhang, W., Xiao, Q., Fan, L., Huang, D., Chen, W., et al. (2022). Liposomes and liposome-like nanoparticles: From anti-fungal infection to the COVID-19 pandemic treatment. Asian J. Pharm. Sci. 17, 817–837. doi: 10.1016/j.ajps.2022.11.002
Henao-Tamayo, M., Obregón-Henao, A., Creissen, E., Shanley, C., Orme, I., Ordway, D. J. (2015). Differential Mycobacterium bovis BCG vaccine-derived efficacy in C3Heb/FeJ and C3H/HeOuJ mice exposed to a clinical strain of Mycobacterium tuberculosis. Clin. Vaccine Immunol. 22, 91–98. doi: 10.1128/CVI.00466-14
Hernandez, J., Ashley, D., Cao, R., Abrahem, R., Nguyen, T., To, K., et al. (2020). Cyclic peptide [R4W4] in improving the ability of first-line antibiotics to inhibit Mycobacterium tuberculosis inside in vitro human granulomas. Front. Immunol. 11, 1677. doi: 10.3389/fimmu.2020.01677
Ito, M., Koga, Y., Hachisu, Y., Murata, K., Sunaga, N., Maeno, T., et al. (2022). Treatment strategies with alternative treatment options for patients with Mycobacterium avium complex pulmonary disease. Respir. Investig. 60, 613–624. doi: 10.1016/j.resinv.2022.05.006
Jin, Y.-Y., Kim, J.-Y., Yang, S. H., Lee, H., Suh, J.-W. (2016). Improvement of the productivity of ecumicin, a novel anti-tuberculosis agent, from new Nonomuraea sp. MJM5123. J. Antibiotics. 69, 362–367. doi: 10.1038/ja.2015.122
Joo, S. H. (2012). Cyclic peptides as therapeutic agents and biochemical tools. Biomol Ther. (Seoul). 20, 19–26. doi: 10.4062/biomolther.2012.20.1.019
Kaczmarkowska, A., Didkowska, A., Kwiecień, E., Stefańska, I., Rzewuska, M., Anusz, K. (2022). The Mycobacterium avium complex - an underestimated threat to humans and animals. Ann. Agric. Environ. Med. 29, 22–27. doi: 10.26444/aaem/136398
Kelley, M., Sasaninia, K., Abnousian, A., Badaoui, A., Owens, J., Beever, A., et al. (2023). Additive Effects of Cyclic Peptide [R4W4] When Added Alongside Azithromycin and Rifampicin against Mycobacterium avium Infection. Pathogens. 12, 1057. doi: 10.3390/pathogens12081057
Laouini, A. J.-M. C., Limayem-Blouza, I., Sfar, S., Charcosset, C., Fessi, H. (2012). Preparation, characterization and applications of liposomes: state of the art. J. colloid Sci. Biotechnol. 1, 147–168. doi: 10.1166/jcsb.2012.1020
Lee, I., Hwang, E. J., Kim, J.-Y., Yim, J.-J., Kwak, N. (2023). Treatment outcomes of clofazimine-containing regimens in severe mycobacterium avium complex pulmonary disease. Open Forum Infect. Diseases. 11. doi: 10.1093/ofid/ofad682
Lei, J., Sun, L., Huang, S., Zhu, C., Li, P., He, J., et al. (2019). The antimicrobial peptides and their potential clinical applications. Am. J. Transl. Res. 11, 3919–3931.
Li, Y. J., Danelishvili, L., Wagner, D., Petrofsky, M., Bermudez, L. E. (2010). Identification of virulence determinants of Mycobacterium avium that impact on the ability to resist host killing mechanisms. J. Med. Microbiol. 59, 8–16. doi: 10.1099/jmm.0.012864-0
Maqbool, F., Moyle, P. M., Thurecht, K. J., Falconer, J. R. (2019). Dispersibility of phospholipids and their optimization for the efficient production of liposomes using supercritical fluid technology. Int. J. Pharmaceutics. 563, 174–183. doi: 10.1016/j.ijpharm.2019.03.053
Martins, S. M., Sarmento, B., Nunes, C., Lucio, M., Reis, S., Ferreira, D. C. (2013). Brain targeting effect of camptothecin-loaded solid lipid nanoparticles in rat after intravenous administration. Eur. J. Pharm. Biopharm. 85, 488–502. doi: 10.1016/j.ejpb.2013.08.011
Maurer, M., Linder, D., Franke, K. B., Jäger, J., Taylor, G., Gloge, F., et al. (2019). Toxic activation of an AAA+ protease by the antibacterial drug cyclomarin A. Cell Chem. Biol. 26, 1169–79.e4. doi: 10.1016/j.chembiol.2019.05.008
McGuffin, S. A., Pottinger, P. S., Harnisch, J. P. (2017). Clofazimine in nontuberculous mycobacterial infections: A growing niche. Open Forum Infect. Dis. 4, ofx147. doi: 10.1093/ofid/ofx147
Mehta, P. P., Ghoshal, D., Pawar, A. P., Kadam, S. S., Dhapte-Pawar, V. S. (2020). Recent advances in inhalable liposomes for treatment of pulmonary diseases: Concept to clinical stance. J. Drug delivery Sci. technology. 56, 101509. doi: 10.1016/j.jddst.2020.101509
Muthaiah, M., Shivekar, S. S., Kapalamurthy, V. R. C., Alagappan, C., Sakkaravarthy, A., Brammachary, U. (2017). Prevalence of mutations in genes associated with rifampicin and isoniazid resistance in Mycobacterium tuberculosis clinical isolates. J. Clin. Tuberculosis Other Mycobacterial Diseases. 8, 19–25. doi: 10.1016/j.jctube.2017.06.001
Nguyen, M.-V. H., Daley, C. L. (2023). Treatment of mycobacterium avium complex pulmonary disease: when should I treat and what therapy should I start? Clinics Chest Med. 44, 771–783. doi: 10.1016/j.ccm.2023.06.009
Oh, D., Sun, J., Nasrolahi Shirazi, A., LaPlante, K. L., Rowley, D. C., Parang, K. (2014). Antibacterial activities of amphiphilic cyclic cell-penetrating peptides against multidrug-resistant pathogens. Mol. pharmaceutics. 11, 3528–3536. doi: 10.1021/mp5003027
Rathinakumar, R., Walkenhorst, W. F., Wimley, W. C. (2009). Broad-spectrum antimicrobial peptides by rational combinatorial design and high-throughput screening: the importance of interfacial activity. J. Am. Chem. Soc 131, 7609–7617. doi: 10.1021/ja8093247
Riahifard, N., Mozaffari, S., Aldakhil, T., Nunez, F., Alshammari, Q., Alshammari, S., et al. (2018). Design, synthesis, and evaluation of amphiphilic cyclic and linear peptides composed of hydrophobic and positively-charged amino acids as antibacterial agents. Molecules 23. doi: 10.3390/molecules23102722
Rukasha, I., Said, H. M., Omar, S. V., Koornhof, H., Dreyer, A. W., Musekiwa, A., et al. (2016). Correlation of rpoB mutations with minimal inhibitory concentration of rifampin and rifabutin in Mycobacterium tuberculosis in an HIV/AIDS endemic setting, South Africa. Front. Microbiol. 7, 1947. doi: 10.3389/fmicb.2016.01947
Sajid, M., Lohan, S., Kato, S., Tiwari, R. (2022). Combination of amphiphilic cyclic peptide [R4W4] and levofloxacin against multidrug-resistant bacteria. Antibiotics 11, 416. doi: 10.3390/antibiotics11030416
Sattar, A., Zakaria, Z., Abu, J., Aziz, S. A., Rojas-Ponce, G. (2021). Isolation of Mycobacterium avium and other nontuberculous mycobacteria in chickens and captive birds in peninsular Malaysia. BMC Vet. Res. 17, 13. doi: 10.1186/s12917-020-02695-8
Saxena, S., Spaink, H. P., Forn-Cuní, G. (2021). Drug resistance in nontuberculous mycobacteria: mechanisms and models. Biol. (Basel). 10. doi: 10.3390/biology10020096
Schildkraut, J. A., Raaijmakers, J., Aarnoutse, R., Hoefsloot, W., Wertheim, H. F. L., van Ingen, J. (2023). The role of rifampicin within the treatment of Mycobacterium avium pulmonary disease. Antimicrobial Agents Chemotherapy. 67, e00874–e00823. doi: 10.1128/aac.00874-23
Shin, M. K., Shin, S. J. (2021). Genetic involvement of mycobacterium avium complex in the regulation and manipulation of innate immune functions of host cells. Int. J. Mol. Sci. 22. doi: 10.3390/ijms22063011
To, K., Cao, R., Yegiazaryan, A., Owens, J., Venketaraman, V. (2020). General Overview of Nontuberculous Mycobacteria Opportunistic Pathogens: Mycobacterium avium and Mycobacterium abscessus. J. Clin. Med. 9. doi: 10.3390/jcm9082541
Torraca, V., Masud, S., Spaink, H. P., Meijer, A. H. (2014). Macrophage-pathogen interactions in infectious diseases: new therapeutic insights from the zebrafish host model. Dis. Model. Mech. 7, 785–797. doi: 10.1242/dmm.015594
Verma, D., Stapleton, M., Gadwa, J., Vongtongsalee, K., Schenkel, A. R., Chan, E. D., et al. (2019). Mycobacterium avium infection in a C3HeB/feJ mouse model. Front. Microbiol. 10, 693. doi: 10.3389/fmicb.2019.00693
Wang, D. M., Liu, H., Zheng, Y. L., Xu, Y. H., Liao, Y. (2023). Epidemiology of nontuberculous mycobacteria in tuberculosis suspects, Southwest of China, 2017-2022. Front. Cell Infect. Microbiol. 13, 1282902. doi: 10.3389/fcimb.2023.1282902
Wang, P.-H., Shu, C.-C., Chen, C.-Y., Wei, Y.-F., Cheng, S.-L. (2023). The role of treatment regimen and duration in treating patients with Mycobacterium avium complex lung disease: A real-world experience and case–control study. J. Microbiology Immunol. Infection, 13. doi: 10.3389/fcimb.2023.1282902
Zhang, H. (2017). Thin-film hydration followed by extrusion method for liposome preparation. Methods Mol. Biol. 1522, 17–22. doi: 10.1007/978-1-4939-6591-5_2
Zhu, S., Su, Y., Shams, S., Feng, Y., Tong, Y., Zheng, G. (2019). Lassomycin and lariatin lasso peptides as suitable antibiotics for combating mycobacterial infections: current state of biosynthesis and perspectives for production. Appl. Microbiol. Biotechnol. 103, 3931–3940. doi: 10.1007/s00253-019-09771-6
Keywords: Mycobacterium avium, antimicrobial, cyclic peptide, antibiotics, macrophages
Citation: Kelley M, Sasaninia K, Badaoui A, Glassman I, Abnousian A, Rai N, Tiwari RK and Venketaraman V (2025) The effects of cyclic peptide [R4W4] in combination with first-line therapy on the survival of Mycobacterium avium. Front. Cell. Infect. Microbiol. 15:1547376. doi: 10.3389/fcimb.2025.1547376
Received: 13 January 2025; Accepted: 10 March 2025;
Published: 16 April 2025.
Edited by:
Sanhita Roy, LV Prasad Eye Institute, IndiaReviewed by:
Tiago Beites, Universidade do Porto, PortugalMadhur Sachan, Brigham and Women’s Hospital and Harvard Medical School, United States
Copyright © 2025 Kelley, Sasaninia, Badaoui, Glassman, Abnousian, Rai, Tiwari and Venketaraman. This is an open-access article distributed under the terms of the Creative Commons Attribution License (CC BY). The use, distribution or reproduction in other forums is permitted, provided the original author(s) and the copyright owner(s) are credited and that the original publication in this journal is cited, in accordance with accepted academic practice. No use, distribution or reproduction is permitted which does not comply with these terms.
*Correspondence: Vishwanath Venketaraman, dnZlbmtldGFyYW1hbkB3ZXN0ZXJudS5lZHU=; Rakesh K. Tiwari, cnRpd2FyaUB3ZXN0ZXJudS5lZHU=