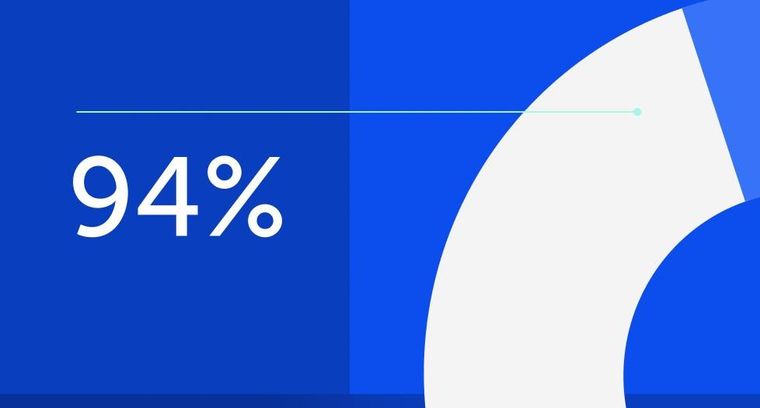
94% of researchers rate our articles as excellent or good
Learn more about the work of our research integrity team to safeguard the quality of each article we publish.
Find out more
MINI REVIEW article
Front. Cell. Infect. Microbiol., 10 April 2025
Sec. Clinical Microbiology
Volume 15 - 2025 | https://doi.org/10.3389/fcimb.2025.1447149
Acute rheumatic fever (ARF) is an autoimmune disease caused by group A streptococcal infection. Recurrent episodes of ARF can lead to rheumatic heart disease (RHD), which is the leading cause of cardiovascular mortality in children worldwide, especially in low- and middle-income countries. Investigations into the etiology of ARF and RHD constitute a crucial milestone in the advancement of both preventive measures and therapeutic interventions. The purpose of this mini review is to delineate the etiology and pathophysiological mechanisms underlying ARF and RHD. Selective searches were conducted in PubMed to retrieve literature published between 1968 and 2024, employing key terms such as “acute rheumatic fever”, “rheumatic heart disease”, “group A Streptococcus”, “streptococcal pharyngitis”, “pathogenesis”, and “pathophysiology”. The pathogenesis of infections caused by group A streptococci, and their effects on ARF and RHD, have been thoroughly examined. A central hypothesis is that autoimmune responses are triggered by molecular mimicry, but alternate pathogenic mechanisms are continuously being explored. There is an urgent need for high-quality research that can inform efforts aimed at decreasing the occurrence of ARF and halting the advancement of RHD, which requires researchers to understand its causes and to develop appropriate preventive and therapeutic programs.
Acute rheumatic fever (ARF) is an immune disorder triggered by repeated infections with group A Streptococcus (GAS) (Yu et al., 2023, Yu et al., 2024). Recurrent ARF can lead to rheumatic heart disease (RHD) (Arvind and Ramakrishnan, 2020; Oliver et al., 2021; Karthikeyan and Guilherme, 2018; Middleton et al., 2022; Armitage et al., 2024; Rivera-Hernandez et al., 2019). Human GAS infection is a multifaceted process involving both host and bacterial factors (Brouwer et al., 2023). From an epidemiological perspective, the global epidemiological profile of GAS is variable. The emergence of new GAS clones is often associated with the acquisition of new pathogenicity or resistance determinants (Brouwer et al., 2023). From an immunological perspective, repeated infections with GAS reduce the tolerance of the host immune system and trigger an autoimmune response.
ARF is a delayed, non-purulent sequela of a GAS pharyngeal infection (Chakravarty et al., 2014; Pilapitiya et al., 2021). After experiencing an initial bout of pharyngitis, an incubation period of one to five weeks precedes the emergence of initial signs or symptoms associated with ARF. Notably, while pharyngitis is a known trigger for both ARF and RHD, other superficial ailments, including scarlet fever, impetigo, and obstructive sleep apnea hypopnea syndrome, also contribute to the occurrence of these conditions (Chakravarty et al., 2014; Liang et al., 2023; Carapetis et al., 2005; Chiou et al., 2004; Barth et al., 2022; Viciani et al., 2016). A diagnosis of ARF is determined by applying clinical criteria (Jones criteria 2015) while excluding other potential differential diagnoses (Carapetis et al., 2016; Gewitz et al., 2015). Clinical criteria are divided into primary and secondary manifestations. Primary manifestations include carditis, arthritis, chorea, subcutaneous nodules, and erythema marginatum. Secondary symptoms include fever, arthralgia, elevated sedimentation rate, increased C-reactive protein, and increased PR interval (Chakravarty et al., 2014; Carapetis et al., 2016). For a confirmed diagnosis of ARF, a patient must exhibit two major symptoms or one major symptom with at least two minor symptoms (Chakravarty et al., 2014). A history of GAS infection must also be demonstrated, which can usually be accomplished by streptococcal serology. Elevated antistreptolysin O titer, elevated anti-DNase B titer, or a positive culture for GAS in the pharynx is considered supportive evidence of GAS infection (Arvind and Ramakrishnan, 2020). It should be noted that damage to the heart valves is a chronic and progressive condition that may ultimately result in heart failure (Kayima and Kaddumukasa, 2024). These symptoms may not become apparent until months after the onset of the causative streptococcal infection, when other symptoms may be absent and streptococcal serologic tests normal.
ARF may be an autoimmune response to GAS pharyngeal infection, especially in genetically susceptible populations, and evidence supports that a molecular mimetic mechanism likely mediates this response. Approximately 0.30–3.00% of patients with GAS pharyngitis may develop ARF, the probability of which is influenced by the host’s genetic susceptibility, recurrent infections, and the virulence level of the infecting strain (Karthikeyan and Guilherme, 2018; Brouwer et al., 2023; Liang et al., 2023; Carapetis et al., 2016; de Crombrugghe et al., 2020; Passos et al., 2021). Host genetic susceptibility is mainly caused by HLA class II alleles DR and DQ, B-cell antigen D8/17, and genetic polymorphisms for tumor necrosis factor–α (TNF-α), interleukin, or transforming growth factor-β1 (TGF-β1), as well as significantly fewer germinal center (GC) -T follicular helper (Tfh) cells (Arvind and Ramakrishnan, 2020; Chakravarty et al., 2014; Guilherme and Kalil, 2010; Bryant et al., 2009; Dan et al., 2019; Crotty, 2019). Included among these, HLA class II alleles associated with RF/RHD susceptibility vary by region (Guilherme et al., 2018). The mechanism of repeated infection is termed “immune priming,” in which repeated infection with GAS renders the immune system intolerant and results in an autoimmune response (Dan et al., 2019). In this process, virulence factors involved in disease progression include those that are surface-bound as well as secreted (Brouwer et al., 2023) (Table 1). These virulence factors act through the following mechanisms: promotion of colonization, evasion of host immune response, induction of inflammation and virulence enhancement. As a result, they play a pivotal role in the pathology of ARF. In a word, these factors play a very important role in the progression of autoimmune reactions, especially in aggressive diseases. Understanding the pathophysiological mechanisms of ARF provides a basis for future research and treatment. One example of its application is the classification of GAS strains responsible for rheumatic fever, which is based on the encoding sequence of the 5’ end of the M protein gene (emm) (Armitage et al., 2024; Brouwer et al., 2023), an important virulence factor known to trigger autoimmune responses (Lacey et al., 2024; Yu et al., 2021). A total of 261 different emm-types have been identified (Brouwer et al., 2023; Walker et al., 2014; Smeesters et al., 2023). Genotypes associated with ARF include emm1, 3, 5, 6, 14, 18, 19, 24, 27, and 29 (de Crombrugghe et al., 2020; Pornrattanarungsi et al., 2022), and genotypes associated with RHD include emm1, 5, 18, 16, and 3 (Pornrattanarungsi et al., 2022). In addition to emm genotypes, other factors that may influence susceptibility include genetic factors, hypersensitivity reactions, circulating immune complexes, toxic effects of streptococcal preparations, streptococcal adherence, and socioeconomic and nutritional factors (Brouwer et al., 2023; Kaplan, 1979).
Molecular mimicry refers to the sharing of antibodies or T-cell epitopes between the host and a microbe. In GAS, the cross-reactive antigen is a molecular structure that resembles host molecules and initiates an autoimmune response against host tissues (such as the heart) during both infection and immunity (Cunningham, 2019). This causes autoimmune GAS sequelae such as ARF and RHD (Jiee et al., 2024). The widely accepted hypothesis is that the alpha helical coil structure of M-protein in GAS triggers an immune response that may cross-react with other coiled proteins in the body such as cardiac myosin, laminin, and myosin.
Molecular mimicry was originally defined as the presence of identical amino acid sequences between host and bacterial antigens (Cunningham, 2019; Fujinami and Oldstone, 1985; Fujinami et al., 1983; Schwimmbeck and Oldstone, 1989). Subsequent studies using human monoclonal antibodies have since identified other types of molecular mimicry. One type involves the recognition of similar structures by antibodies. For instance, the M proteins of streptococci bear a striking resemblance to the alpha-helical coiled-coil structures of host proteins such as myosins, keratins, poikilins, and laminins. The common regions of these molecules share 40% or less identity, and the cross-reactive sites are not completely identical (Cunningham, 2019; Cunningham et al., 1997). Studies of monoclonal antibodies derived from RHD have identified cross-reactive antibodies that target GAS, group A glycans, N-acetyl-β-D-glucosamine(GlcNAc), heart valve endothelium, laminin, and key epitopes of the stratified basement membranes (Chakravarty et al., 2014; Faé et al., 2006; Galvin et al., 2000; Cunningham et al., 1989; Dale and Beachey, 1985). Furthermore, reactivity of streptococcal M proteins and myocardial myosin was observed between T cells isolated from peripheral blood and rheumatic heart valves. Some studies have indicated that the periodicity of the seven amino acid residues common to group A streptococcal M proteins is identical to that of proteins such as myosin, desmin, poikilodin and keratin (Cunningham, 2019; Manjula et al., 1985; Phillips et al., 1981; Dale and Beachey, 1986).
Another type of molecular mimicry may contribute to immunological cross-reactivity between diverse molecules, such as DNA and proteins or carbohydrates and peptides (Cunningham et al., 1989; Putterman and Diamond, 1998). Antibodies induced by group A carbohydrate epitope GlcNAc react with human cardiac proteins, including myosin and laminin, and are capable of causing humoral immune damage (Cunningham, 2019, Cunningham, 2012). It is important to note that elevated antibody responses against group A carbohydrates are associated with unfavorable clinical outcomes in patients with heart valve disease (Dudding and Ayoub, 1968). Goldstein et al. demonstrated that glycoproteins present in heart valves possess N-acetylglucosamine, leading to the hypothesis that GAS may be responsible for triggering immune cross-reactivity with heart valves (Goldstein et al., 1967). Moreover, recent studies have demonstrated that immunoglobulin G2 (IgG2) subclass autoantibodies, particularly those directed against the GlcNAc epitope, are instrumental in the deposition of RHD heart valve tissue. This class of antibodies is particularly active in response to GAS infections, and they appear to play an important role in the early stages of the disease. They may therefore serve as biomarkers to predict the risk of autoimmune responses following GAS infections (Kirvan et al., 2022). This type of immunological cross-reactivity is based on the existence of an epitope that is shared by two distinct proteins. This epitope can be comprised of an identical or homologous amino acid sequence, or it can arise from two chemically diverse structures.
Although the molecular modeling theory is widely accepted as a means of elucidating the pathogenesis of ARF/RHD, mechanisms other than molecular mimicry have been proposed to explain the pathogenesis (Table 2). For example, Dr. Lukomski posits that streptococcal collagen-like (Scl) proteins contributes to streptococcal colonization and pathogenesis in humans and animals (Lukomski et al., 2017; Lukomski and McNitt, 2020). It has been postulated that the response to these antibodies against Scl proteins and human collagen is not related to molecular mimicry, although they are present in ARF patients. Karthikeyan and Guilherme put forth the hypothesis that collagen antibodies are produced by epitope diffusion following initial valve injury (Karthikeyan and Guilherme, 2018). Pilapitiya and his colleagues proposed that there is no molecular mimicry between the Scl proteins of streptococcal bacteria and human collagen (Pilapitiya et al., 2021). One hypothesis posits that a sustained disparity between Th17 cells and regulatory T cells (Tregs) may disrupt immune tolerance, thereby elevating the susceptibility of the host to disease following streptococcal infection (Wang et al., 2010). The mouse nasopharyngeal-associated lymphoid tissue (NALT) colonization model is regarded as an optimal model for investigating Streptococcus pyogenes colonization in the airway (Park et al., 2003, Park et al., 2004). In this process, NALT plays a key role in antigen uptake by promoting the production of Th17 cells and their secretion of antibodies, which in turn activate the mucosal immune response (Chen et al, 2016). TGF-β1 is regarded as a pivotal signaling molecule that facilitates the differentiation of Tregs and Th17 cells. GAS infection induces the production of TGF-β1, thereby promoting the predominant TGF-β1-dependent differentiation of Th17 cells (Wang et al., 2010). Furthermore, evidence indicates that Prothymosin Alpha plays a pivotal role in the pathological immune response of CD8+ T-cells regulated by estradiol receptor alpha (ERα), as well as in the process of recognition of type I collagen, in the context of rheumatic heart disease (Passos et al., 2022).
A GAS infection initiates a host inflammatory response and immune system activation, which in most cases facilitates the clearance of the infection. However, in some susceptible individuals, an aberrant immune system response occurs, leading to the development of autoimmune diseases such as ARF and RHD. The latest research indicates that immune cells and inflammatory cytokines have a dual function in the pathological process of disease, acting both as promoters and regulators (Nagarajan et al., 2023). Additionally, Because GAS is a human pathogen, animal models of ARF and RHD often fail to replicate essential characteristics of the pathophysiology of these two diseases in humans, thereby limiting their application (Brouwer et al., 2023; Rafeek et al., 2021). Osowicki et al. established a dependable control human infection model of Streptococcus pyogenes pharyngitis (Osowicki et al., 2021). Individuals suffering from pharyngitis exhibited elevated levels of salivary pro-inflammatory cytokines, namely IL-1Ra, Interleukin-6 (IL-6), IFNγ, and IP-10. These cytokines were associated with an elevation in the quantity of monocytes and dendritic cells present in the blood, a reduction in the number of conventional CD4+ T cells and B cells, and an elevation in the expression of γδ T-cell activation markers. Additionally, mucosa-associated invariant T (MAIT) cells merit attention due to their capacity to recognize MR1 antigens produced during microbial riboflavin biosynthesis, thereby eliciting protective innate immune responses against microorganisms that generate such metabolites. Emgård et al. Identified MAIT cells contribute to the initial cytokine response to GAS. Studies have also shown high activation of human MAIT cells in patients with streptococcal toxic shock syndrome, and that removal of MAIT cells can reduce the production of cytokines such as IFNγ, IL-1β, IL-2, and TNF (Emgård et al., 2019; Guilherme et al., 2004). The involvement of MAIT cells in the pathogenesis of pharyngitis, invasive GAS disease, and ARF is well-established (Brouwer et al., 2023; Guilherme et al., 2004). Furthermore, infection with GAS, which also impacts the patient’s immune system, is responsible for ARF. Kim et al. systematically identified the immunological mechanisms responsible for the pathogenesis of both diseases, specifically with regard to the interleukin-1β-granulocyte-macrophage colony-stimulating factor (IL-1β-GM-CSF) axis (Kim et al., 2018). Individuals with ARF show continuous production of interleukin-1β in peripheral blood mononuclear cells. This can lead to dysregulated cytokine signaling, specifically the IL-1β-GM-CSF axis, and may be a risk factor for the development of both diseases. Dysregulated cytokine signaling should be considered when evaluating the pathophysiology of ARF, and may provide an explanation for the specialized migration of TH1 cells to the mitral valve of the heart; it may also provide an avenue for the advancement of immunomodulatory drugs aimed at treating patients at high risk for ARF (Kim et al., 2018; Lukens et al., 2012). The main source of GM-CSF is CD4+ T cells, which are also involved in the pathogenesis of myocarditis by promoting the development and survival of Th cells, as well as their role in autoimmunity and inflammation (Noster et al., 2014). Moreover, it is plausible that NLRP3 Inflammasome may contribute to the pathogenesis of RHD via the induction of pro-inflammatory cytokines, such as IL-1β and IL-18, which in turn stimulate CD4+ T lymphocytes and the subsequent release of associated inflammatory cytokines (Rani and Toor, 2023; Kong et al., 2022). In addition, Faé et al. discoveried CXCL9/Mig can enhance T-cell recruitment in valvular tissue lesions, which is linked to the progression of ARF toward RHD (Brouwer et al., 2023; Faé et al., 2013). Recent studies have revealed the important role of C-C chemokine receptor type 2-positive (CCR2+) macrophages in autoimmune diseases and tissue fibrosis, and suggest that they are critical for valve remodeling in RHD (Zhu et al., 2021; Bai et al., 2024). M1 macrophages and the very late antigen 4 (VLA4)/vascular cell adhesion molecule-1 (VCAM-1) pathway may be involved in the inflammatory infiltration and valvular fibrosis in RHD (Xian et al., 2024). The upregulation of VCAM-1 on the surface of endothelial cells represents a key initiating event in the pathogenesis of valve injury, oedema and T-cell infiltration. This leaves the valve vulnerable to immune-mediated damage (Cunningham, 2012; Guilherme et al., 2004; Roberts et al., 2001). In addition, there is a hypothesis that initial infection with GAS may trigger the activation of inflammatory mediators such as TNF-α and IL-6, resulting in endothelial and mesenchymal cells of the heart valves undergoing epigenetic modifications. This modification involves upregulation of the expression of genes that promote cell necrosis and inflammatory responses, including those involved in the TGF-β1 signaling pathway. In the pathogenesis of RHD, TGF-β1 signaling may cause increased valve fibrosis (Kirvan et al., 2022; Karthikeyan et al., 2020).With recurrent streptococcal infections, the accumulation of IgG2 antibodies may activate the inflammatory response in the valve endothelium and promote the attack of inflammatory T-cell subsets such as Th1 and Th17 on the valve endothelium (Kirvan et al., 2022). In addition, the correlation between ARF and GAS infection may be attributed to TGF-β1 overreactivity during mucosal infections, which may enhance Th17 cell activity and impede the proliferation of regulatory T cells (Dileepan et al., 2016). Furthermore, there is growing evidence that the microbiome and the immune system may also affect the development of RHD. Dysbiosis may lead to a breakdown of immune tolerance in the host, followed by systemic immune dysregulation, ultimately favoring a pro-inflammatory response to RHD (Nagarajan et al., 2023). These novel findings provide insights that will aid in gaining a comprehensive understanding of the pathogenesis of ARF and RHD.
The development of vaccines against GAS infections to prevent the disease processes of RF and RHD remains an unresolved challenge in the field of medicine. Since the 20th century, research into GAS vaccines has encompassed M protein–based subunit vaccines, other peptide vaccines, and non-M protein vaccines (Table 3). Despite the advent of peptide subunit vaccines representing the latest trend in vaccine research, there is currently no commercially available GAS vaccine (Fan et al., 2024; Ambari et al., 2024; Castro and Dorfmueller, 2021). It is therefore imperative that a global effort be made to develop a widely applicable, effective, and safe GAS vaccine to prevent GAS infection.
ARF and RHD are known to be triggered by recurrent GAS infections. Molecular mimicry is now recognized as an important mechanism in the pathogenesis of ARF. Studies have revealed the presence of anti-collagen antibodies in RHD, as well as anti-cardiac myosin antibodies. Nonetheless, there is still a dearth of a pathogenetic mechanism for fully elucidating ARF, and a comprehensive investigation into the pathogenesis of ARF and RHF is imperative to enhance the prevention and management of these diseases.
SZ: Data curation, Formal Analysis, Methodology, Writing – original draft. DG: Data curation, Formal Analysis, Writing – original draft. DY: Conceptualization, Funding acquisition, Resources, Writing – review & editing.
The author(s) declare that financial support was received for the research and/or publication of this article. This work was supported by National Natural Science Foundation of China (82304198).
The authors declare that the research was conducted in the absence of any commercial or financial relationships that could be construed as a potential conflict of interest.
All claims expressed in this article are solely those of the authors and do not necessarily represent those of their affiliated organizations, or those of the publisher, the editors and the reviewers. Any product that may be evaluated in this article, or claim that may be made by its manufacturer, is not guaranteed or endorsed by the publisher.
Agniswamy, J., Lei, B., Musser, J. M., Sun, P. D. (2004). Insight of host immune evasion mediated by two variants of group a Streptococcus Mac protein. J. Biol. Chem. 279, 52789–52796. doi: 10.1074/jbc.M410698200
Ambari, A. M., Qhabibi, F. R., Desandri, D. R., Dwiputra, B., Baravia, P. A., Makes, I. K., et al. (2024). Unveiling the group A streptococcus vaccine-based L-rhamnose from backbone of group A carbohydrate: current insight against acute rheumatic fever to reduce the global burden of rheumatic heart disease. F1000Res. 13, 132. doi: 10.12688/f1000research.144903.3
Armitage, E. P., de Crombrugghe, G., Keeley, A. J., Senghore, E., Camara, F. E., Jammeh, M., et al. (2024). Streptococcus pyogenes carriage and infection within households in The Gambia: a longitudinal cohort study. Lancet Microbe. 5, 679–688. doi: 10.1016/S2666-5247(24)00046-6
Arvind, B., Ramakrishnan, S. (2020). Rheumatic fever and rheumatic heart disease in children. Indian J. Pediatr. 87, 305–311. doi: 10.1007/s12098-019-03128-7
Bai, L., Li, Y., Xue, Y., Lu, Z., Meng, Z., Lu, C., et al. (2024). Inhibition of macrophage recruitment to heart valves mediated by the C-C chemokine receptor type 2 attenuates valvular inflammation induced by group A streptococcus in lewis rats. Front. Biosci. (Landmark Ed). 29, 303. doi: 10.31083/j.fbl2908303
Barth, D. D., Mullane, M. J., Sampson, C., Chou, C., Pickering, J., Nicol, M. P., et al. (2022). Missing Piece Study protocol: prospective surveillance to determine the epidemiology of group A streptococcal pharyngitis and impetigo in remote Western Australia. BMJ Open 12, e057296. doi: 10.1136/bmjopen-2021-057296
Brouwer, S., Rivera-Hernandez, T., Curren, B. F., Harbison-Price, N., De Oliveira, D. M. P., Jespersen, M. G., et al. (2023). Pathogenesis, epidemiology and control of Group A Streptococcus infection. Nat. Rev. Microbiol. 21, 431–447. doi: 10.1038/s41579-023-00865-7
Bryant, P. A., Robins-Browne, R., Carapetis, J. R., Curtis, N. (2009). Some of the people, some of the time: susceptibility to acute rheumatic fever. Circulation. 119, 742–753. doi: 10.1161/circulationaha.108.792135
Carapetis, J. R., Beaton, A., Cunningham, M. W., Guilherme, L., Karthikeyan, G., Mayosi, B. M., et al. (2016). Acute rheumatic fever and rheumatic heart disease. Nat. Rev. Dis. Primers. 2, 15084. doi: 10.1038/nrdp.2015.84
Carapetis, J. R., Steer, A. C., Mulholland, E. K., Weber, M. (2005). The global burden of group A streptococcal diseases. Lancet Infect. Dis. 5, 685–694. doi: 10.1016/s1473-3099(05)70267-x
Castro, S. A., Dorfmueller, H. C. (2021). A brief review on Group A Streptococcus pathogenesis and vaccine development. R Soc. Open Sci. 8, 201991. doi: 10.1098/rsos.201991
Chakravarty, S. D., Zabriskie, J. B., Gibofsky, A. (2014). Acute rheumatic fever and streptococci: the quintessential pathogenic trigger of autoimmunity. Clin. Rheumatol. 33, 893–901. doi: 10.1007/s10067-014-2698-8
Chen, X., Li, N., Bi, S., Wang, X., Wang, B. (2016). Co-activation of th17 and antibody responses provides efficient protection against mucosal infection by group A streptococcus. PloS One 11, e0168861. doi: 10.1371/journal.pone.0168861
Chiou, C. S., Liao, T. L., Wang, T. H., Chang, H. L., Liao, J. C., Li, C. C. (2004). Epidemiology and molecular characterization of Streptococcus pyogenes recovered from scarlet fever patients in central Taiwan from 1996 to 1999. J. Clin. Microbiol. 42, 3998–4006. doi: 10.1128/jcm.42.9.3998-4006.2004
Crotty, S. (2019). T follicular helper cell biology: A decade of discovery and diseases. Immunity. 50, 1132–1148. doi: 10.1016/j.immuni.2019.04.011
Cunningham, M. W. (2012). Streptococcus and rheumatic fever. Curr. Opin. Rheumatol. 24, 408–416. doi: 10.1097/BOR.0b013e32835461d3
Cunningham, M. W. (2019). Molecular mimicry, autoimmunity, and infection: the cross-reactive antigens of group A streptococci and their sequelae. Microbiol. Spectr. 7, GPP3–0045-2018. doi: 10.1128/microbiolspec.GPP3-0045-2018
Cunningham, M. W., Antone, S. M., Smart, M., Liu, R., Kosanke, S. (1997). Molecular analysis of human cardiac myosin-cross-reactive B- and T-cell epitopes of the group A streptococcal M5 protein. Infect. Immun. 65, 3913–3923. doi: 10.1128/iai.65.9.3913-3923.1997
Cunningham, M. W., McCormack, J. M., Fenderson, P. G., Ho, M. K., Beachey, E. H., Dale, J. B. (1989). Human and murine antibodies cross-reactive with streptococcal M protein and myosin recognize the sequence GLN-LYS-SER-LYS-GLN in M protein. J. Immunol. 143, 2677–2683.
Cywes, C., Wessels, M. R. (2001). Group A Streptococcus tissue invasion by CD44-mediated cell signalling. Nature. 414, 648–652. doi: 10.1038/414648a
Dale, J. B., Beachey, E. H. (1985). Epitopes of streptococcal M proteins shared with cardiac myosin. J. Exp. Med. 162, 583–591. doi: 10.1084/jem.162.2.583
Dale, J. B., Beachey, E. H. (1986). Sequence of myosin-crossreactive epitopes of streptococcal M protein. J. Exp. Med. 164, 1785–1790. doi: 10.1084/jem.164.5.1785
Dan, J. M., Havenar-Daughton, C., Kendric, K., Al-Kolla, R., Kaushik, K., Rosales, S. L., et al. (2019). Recurrent group A Streptococcus tonsillitis is an immunosusceptibility disease involving antibody deficiency and aberrant T(FH) cells. Sci. Transl. Med. 11, eaau3776. doi: 10.1126/scitranslmed.aau3776
de Crombrugghe, G., Baroux, N., Botteaux, A., Moreland, N. J., Williamson, D. A., Steer, A. C., et al. (2020). The limitations of the rheumatogenic concept for group A streptococcus: systematic review and genetic analysis. Clin. Infect. Dis. 70, 1453–1460. doi: 10.1093/cid/ciz425
Dileepan, T., Smith, E. D., Knowland, D., Hsu, M., Platt, M., Bittner-Eddy, P., et al. (2016). Group A Streptococcus intranasal infection promotes CNS infiltration by streptococcal-specific Th17 cells. J. Clin. Invest. 126, 303–317. doi: 10.1172/jci80792
Dudding, B. A., Ayoub, E. M. (1968). Persistence of streptococcal group A antibody in patients with rheumatic valvular disease. J. Exp. Med. 128, 1081–1098. doi: 10.1084/jem.128.5.1081
Emgård, J., Bergsten, H., McCormick, J. K., Barrantes, I., Skrede, S., Sandberg, J. K., et al. (2019). MAIT cells are major contributors to the cytokine response in group A streptococcal toxic shock syndrome. Proc. Natl. Acad. Sci. U S A. 116, 25923–25931. doi: 10.1073/pnas.1910883116
Faé, K. C., da Silva, D. D., Oshiro, S. E., Tanaka, A. C., Pomerantzeff, P. M., Douay, C., et al. (2006). Mimicry in recognition of cardiac myosin peptides by heart-intralesional T cell clones from rheumatic heart disease. J. Immunol. 176, 5662–5670. doi: 10.4049/jimmunol.176.9.5662
Faé, K. C., Palacios, S. A., Nogueira, L. G., Oshiro, S. E., Demarchi, L. M., Bilate, A. M., et al. (2013). CXCL9/Mig mediates T cells recruitment to valvular tissue lesions of chronic rheumatic heart disease patients. Inflammation. 36, 800–811. doi: 10.1007/s10753-013-9606-2
Fan, J., Toth, I., Stephenson, R. J. (2024). Recent Scientific Advancements towards a Vaccine against Group A Streptococcus. Vaccines (Basel). 12, 272. doi: 10.3390/vaccines12030272
Fujinami, R. S., Oldstone, M. B. (1985). Amino acid homology between the encephalitogenic site of myelin basic protein and virus: mechanism for autoimmunity. Science. 230, 1043–1045. doi: 10.1126/science.2414848
Fujinami, R. S., Oldstone, M. B., Wroblewska, Z., Frankel, M. E., Koprowski, H. (1983). ). Molecular mimicry in virus infection: crossreaction of measles virus phosphoprotein or of herpes simplex virus protein with human intermediate filaments. Proc. Natl. Acad. Sci. U S A. 80, 2346–2350. doi: 10.1073/pnas.80.8.2346
Galvin, J. E., Hemric, M. E., Ward, K., Cunningham, M. W. (2000). Cytotoxic mAb from rheumatic carditis recognizes heart valves and laminin. J. Clin. Invest. 106, 217–224. doi: 10.1172/jci7132
Gewitz, M. H., Baltimore, R. S., Tani, L. Y., Sable, C. A., Shulman, S. T., Carapetis, J., et al. (2015). Revision of the Jones Criteria for the diagnosis of acute rheumatic fever in the era of Doppler echocardiography: a scientific statement from the American Heart Association. Circulation. 131, 1806–1818. doi: 10.1161/cir.0000000000000205
Goldstein, I., Halpern, B., Robert, L. (1967). Immunological Relationship between Streptococcus A Polysaccharide and the Structural Glycoproteins of Heart Valve. nature 213, 44–47. doi: 10.1038/213044a0
Guilherme, L., Cury, P., Demarchi, L. M., Coelho, V., Abel, L., Lopez, A. P., et al. (2004). Rheumatic heart disease: proinflammatory cytokines play a role in the progression and maintenance of valvular lesions. Am. J. Pathol. 165, 1583–1591. doi: 10.1016/s0002-9440(10)63415-3
Guilherme, L., de Barros, S. F., Kohler, K. F., Santos, S. R., Ferreira, F. M., Silva, W. R., et al. (2018). Rheumatic heart disease: pathogenesis and vaccine. Curr. Protein Pept. Sci. 19, 900–908. doi: 10.2174/1389203718666170725115855
Guilherme, L., Kalil, J. (2010). Rheumatic fever and rheumatic heart disease: cellular mechanisms leading autoimmune reactivity and disease. J. Clin. Immunol. 30, 17–23. doi: 10.1007/s10875-009-9332-6
Ji, Y., Schnitzler, N., DeMaster, E., Cleary, P. (1998). Impact of M49, Mrp, Enn, and C5a peptidase proteins on colonization of the mouse oral mucosa by Streptococcus pyogenes. Infect. Immun. 66, 5399–5405. doi: 10.1128/iai.66.11.5399-5405.1998
Jiee, S. F., Joo, L. K., Eng, P. N., Simon Sumeh, A., Jantim, A., Shanmuganathan, S., et al. (2024). At the heart of the community: implementation of echocardiographic screening for rheumatic heart disease in primary care facilities of northern borneo island. J. Prim Care Community Health 15, 21501319241233178. doi: 10.1177/21501319241233178
Kaplan, M. H. (1979). Rheumatic fever, rheumatic heart disease, and the streptococcal connection: the role of streptococcal antigens cross-reactive with heart tissue. Rev. Infect. Diseases. 1, 988–996. doi: 10.1093/clinids/1.6.988
Karthikeyan, G., Fung, E., Foo, R. S. (2020). Alternative hypothesis to explain disease progression in rheumatic heart disease. Circulation. 142, 2091–2094. doi: 10.1161/circulationaha.120.050955
Karthikeyan, G., Guilherme, L. (2018). Acute rheumatic fever. Lancet. 392, 161–174. doi: 10.1016/s0140-6736(18)30999-1
Kayima, J., Kaddumukasa, M. (2024). An urgent need for early diagnosis and treatment of acute rheumatic fever. . Lancet Glob Health 12, e362–e363. doi: 10.1016/S2214-109X(24)00037-8
Kim, M. L., Martin, W. J., Minigo, G., Keeble, J. L., Garnham, A. L., Pacini, G., et al. (2018). Dysregulated IL-1β-GM-CSF axis in acute rheumatic fever that is limited by hydroxychloroquine. Circulation. 138, 2648–2661. doi: 10.1161/circulationaha.118.033891
Kirvan, C. A., Canini, H., Swedo, S. E., Hill, H., Veasy, G., Jankelow, D., et al. (2022). IgG2 rules: N-acetyl-β-D-glucosamine-specific IgG2 and Th17/Th1 cooperation may promote the pathogenesis of acute rheumatic heart disease and be a biomarker of the autoimmune sequelae of Streptococcus pyogenes. Front. Cardiovasc. Med. 9, 919700. doi: 10.3389/fcvm.2022.919700
Kong, R., Sun, L., Li, H., Wang, D. (2022). The role of NLRP3 inflammasome in the pathogenesis of rheumatic disease. Autoimmunity. 55, 1–7. doi: 10.1080/08916934.2021.1995860
Lacey, J. A., Bennett, J., James, T. B., Hines, B. S., Chen, T., Lee, D., et al. (2024). A worldwide population of Streptococcus pyogenes strains circulating among school-aged children in Auckland, New Zealand: a genomic epidemiology analysis. Lancet Reg. Health West Pac. 42, 100964. doi: 10.1016/j.lanwpc.2023.100964
Liang, Y., Yu, D., Lu, Q., Zheng, Y., Yang, Y. (2023). The rise and fall of acute rheumatic fever and rheumatic heart disease: a mini review. Front. Cardiovasc. Med. 10, 1183606. doi: 10.3389/fcvm.2023.1183606
Loh, J. M. S., Rivera-Hernandez, T., McGregor, R., Khemlani, A. H. J., Tay, M. L., Cork, A. J., et al. (2021). A multivalent T-antigen-based vaccine for group A Streptococcus. Sci. Rep. 11, 4353. doi: 10.1038/s41598-021-83673-4
Lukens, J. R., Barr, M. J., Chaplin, D. D., Chi, H., Kanneganti, T. D. (2012). Inflammasome-derived IL-1β regulates the production of GM-CSF by CD4(+) T cells and γδ T cells. J. Immunol. 188, 3107–3115. doi: 10.4049/jimmunol.1103308
Lukomski, S., Bachert, B. A., Squeglia, F., Berisio, R. (2017). Collagen-like proteins of pathogenic streptococci. Mol. Microbiol. 103, 919–930. doi: 10.1111/mmi.13604
Lukomski, S., McNitt, D. H. (2020). Expression and purification of collagen-like proteins of group A streptococcus. Methods Mol. Biol. 2136, 163–179. doi: 10.1007/978-1-0716-0467-0_12
Manjula, B. N., Trus, B. L., Fischetti, V. A. (1985). Presence of two distinct regions in the coiled-coil structure of the streptococcal Pep M5 protein: relationship to mammalian coiled-coil proteins and implications to its biological properties. Proc. Natl. Acad. Sci. U S A. 82, 1064–1068. doi: 10.1073/pnas.82.4.1064
McMillan, D. J., Drèze, P. A., Vu, T., Bessen, D. E., Guglielmini, J., Steer, A. C., et al. (2013). Updated model of group A Streptococcus M proteins based on a comprehensive worldwide study. Clin. Microbiol. Infect. 19, E222–E229. doi: 10.1111/1469-0691.12134
Middleton, F. M., McGregor, R., Webb, R. H., Wilson, N. J., Moreland, N. J. (2022). Cytokine imbalance in acute rheumatic fever and rheumatic heart disease: Mechanisms and therapeutic implications. Autoimmun Rev. 21, 103209. doi: 10.1016/j.autrev.2022.103209
Mills, J. S., Jayashi Flores, C. M., Reynolds, S., Wun, C., Calcutt, A., Baker, S. B., et al. (2020). M-protein based vaccine induces immunogenicity and protection from Streptococcus pyogenes when delivered on a high-density microarray patch (HD-MAP). NPJ Vaccines 5, 74. doi: 10.1038/s41541-020-00222-2
Naegeli, A., Bratanis, E., Karlsson, C., Shannon, O., Kalluru, R., Linder, A., et al. (2019). Streptococcus pyogenes evades adaptive immunity through specific IgG glycan hydrolysis. J. Exp. Med. 216, 1615–1629. doi: 10.1084/jem.20190293
Nagarajan, G., Govindan, R., Poomarimuthu, M., Andiappan, R., Elango, S., Maruthamuthu, S., et al. (2023). The microbiome and rheumatic heart disease: current knowledge and future perspectives. Acta Cardiol. 78, 525–533. doi: 10.1080/00015385.2023.2207933
Nitzsche, R., Köhler, J., Kreikemeyer, B., Oehmcke-Hecht, S. (2016). Streptococcus pyogenes escapes killing from extracellular histones through plasminogen binding and activation by streptokinase. J. Innate Immun. 8, 589–600. doi: 10.1159/000448039
Noster, R., Riedel, R., Mashreghi, M. F., Radbruch, H., Harms, L., Haftmann, C., et al. (2014). IL-17 and GM-CSF expression are antagonistically regulated by human T helper cells. Sci. Transl. Med. 6, 241ra280. doi: 10.1126/scitranslmed.3008706
Oliver, J., Bennett, J., Thomas, S., Zhang, J., Pierse, N., Moreland, N. J., et al. (2021). Preceding group A streptococcus skin and throat infections are individually associated with acute rheumatic fever: evidence from New Zealand. BMJ Glob Health 6, e007038. doi: 10.1136/bmjgh-2021-007038
Osowicki, J., Azzopardi, K. I., Fabri, L., Frost, H. R., Rivera-Hernandez, T., Neeland, M. R., et al. (2021). A controlled human infection model of Streptococcus pyogenes pharyngitis (CHIVAS-M75): an observational, dose-finding study. Lancet Microbe 2, e291–e299. doi: 10.1016/s2666-5247(20)30240-8
Park, H. S., Costalonga, M., Reinhardt, R. L., Dombek, P. E., Jenkins, M. K., Cleary, P. P. (2004). Primary induction of CD4 T cell responses in nasal associated lymphoid tissue during group A streptococcal infection. Eur. J. Immunol. 34, 2843–2853. doi: 10.1002/eji.200425242
Park, H. S., Francis, K. P., Yu, J., Cleary, P. P. (2003). Membranous cells in nasal-associated lymphoid tissue: a portal of entry for the respiratory mucosal pathogen group A streptococcus. J. Immunol. 171, 2532–2537. doi: 10.4049/jimmunol.171.5.2532
Passos, L. S. A., Jha, P. K., Becker-Greene, D., Blaser, M. C., Romero, D., Lupieri, A., et al. (2022). Prothymosin alpha: A novel contributor to estradiol receptor alpha-mediated CD8(+) T-cell pathogenic responses and recognition of type 1 collagen in rheumatic heart valve disease. Circulation. 145, 531–548. doi: 10.1161/circulationaha.121.057301
Passos, L. S. A., Nunes, M. C. P., Aikawa, E. (2021). Rheumatic heart valve disease pathophysiology and underlying mechanisms. Front. Cardiovasc. Med. 7, 612716. doi: 10.3389/fcvm.2020.612716
Phillips, G. N., Jr., Flicker, P. F., Cohen, C., Manjula, B. N., Fischetti, V. A. (1981). Streptococcal M protein: alpha-helical coiled-coil structure and arrangement on the cell surface. Proc. Natl. Acad. Sci. U S A. 78, 4689–4693. doi: 10.1073/pnas.78.8.4689
Pilapitiya, D. H., Harris, P. W. R., Hanson-Manful, P., McGregor, R., Kowalczyk, R., Raynes, J. M., et al. (2021). Antibody responses to collagen peptides and streptococcal collagen-like 1 proteins in acute rheumatic fever patients. Pathog. Dis. 79, ftab033. doi: 10.1093/femspd/ftab033
Pornrattanarungsi, S., Eursiriwan, S., Amornchaicharoensuk, Y., Chavanisakun, C., Sirimongkolchaiyakul, O. (2022). Concomitant rapidly progressive glomerulonephritis and acute rheumatic fever after streptococcus infection: a case report. Paediatrics Int. Child Health 42, 100–104. doi: 10.1080/20469047.2022.2046966
Putterman, C., Diamond, B. (1998). Immunization with a peptide surrogate for double-stranded DNA (dsDNA) induces autoantibody production and renal immunoglobulin deposition. J. Exp. Med. 188, 29–38. doi: 10.1084/jem.188.1.29
Rafeek, R. A. M., Sikder, S., Hamlin, A. S., Andronicos, N. M., McMillan, D. J., Sriprakash, K. S., et al. (2021). Requirements for a robust animal model to investigate the disease mechanism of autoimmune complications associated with ARF/RHD. Front. Cardiovasc. Med. 8, 675339. doi: 10.3389/fcvm.2021.675339
Rani, A., Toor, D. (2023). Plausible role of NLRP3 inflammasome and associated cytokines in pathogenesis of rheumatic heart disease. Crit. Rev. Immunol. 43, 1–14. doi: 10.1615/CritRevImmunol.2023049463
Remmington, A., Turner, C. E. (2018). The DNases of pathogenic Lancefield streptococci. Microbiol. (Reading). 164, 242–250. doi: 10.1099/mic.0.000612
Richter, J., Monteleone, M. M., Cork, A. J., Barnett, T. C., Nizet, V., Brouwer, S., et al. (2021). Streptolysins are the primary inflammasome activators in macrophages during Streptococcus pyogenes infection. Immunol. Cell Biol. 99, 1040–1052. doi: 10.1111/imcb.12499
Rivera-Hernandez, T., Carnathan, D. G., Jones, S., Cork, A. J., Davies, M. R., Moyle, P. M., et al. (2019). An experimental group A streptococcus vaccine that reduces pharyngitis and tonsillitis in a nonhuman primate model. mBio. 10, e00693-19. doi: 10.1128/mBio.00693-19
Roberts, S., Kosanke, S., Terrence Dunn, S., Jankelow, D., Duran, C. M., Cunningham, M. W. (2001). Pathogenic mechanisms in rheumatic carditis: focus on valvular endothelium. J. Infect. Dis. 183, 507–511. doi: 10.1086/318076
Schwimmbeck, P. L., Oldstone, M. B. (1989). Klebsiella pneumoniae and HLA B27-associated diseases of Reiter’s syndrome and ankylosing spondylitis. Curr. Top. Microbiol. Immunol. 145, 45–56. doi: 10.1007/978-3-642-74594-2_4
Smeesters, P. R., de Crombrugghe, G., Tsoi, S. K., Leclercq, C., Baker, C., Osowicki, J., et al. (2023). Global Streptococcus pyogenes strain diversity, disease associations, and implications for vaccine development: a systematic review. Lancet Microbe 5, e181–e193. doi: 10.1016/s2666-5247(23)00318-x
Starr, C. R., Engleberg, N. C. (2006). Role of hyaluronidase in subcutaneous spread and growth of group A streptococcus. Infect. Immun. 74, 40–48. doi: 10.1128/iai.74.1.40-48.2006
Sumitomo, T., Nakata, M., Higashino, M., Terao, Y., Kawabata, S. (2013). Group A streptococcal cysteine protease cleaves epithelial junctions and contributes to bacterial translocation. J. Biol. Chem. 288, 13317–13324. doi: 10.1074/jbc.M113.459875
Velarde, J. J., O’Seaghdha, M., Baddal, B., Bastiat-Sempe, B., Wessels, M. R. (2017). Binding of NAD(+)-glycohydrolase to streptolysin O stabilizes both toxins and promotes virulence of group A streptococcus. mBio 8, e01382-17. doi: 10.1128/mBio.01382-17
Viciani, E., Montagnani, F., Tavarini, S., Tordini, G., Maccari, S., Morandi, M., et al. (2016). Paediatric obstructive sleep apnoea syndrome (OSAS) is associated with tonsil colonisation by Streptococcus pyogenes. Sci. Rep. 6, 20609. doi: 10.1038/srep20609
Vincents, B., von Pawel-Rammingen, U., Björck, L., Abrahamson, M. (2004). Enzymatic characterization of the streptococcal endopeptidase, IdeS, reveals that it is a cysteine protease with strict specificity for IgG cleavage due to exosite binding. Biochemistry. 43, 15540–15549. doi: 10.1021/bi048284d
Walker, M. J., Barnett, T. C., McArthur, J. D., Cole, J. N., Gillen, C. M., Henningham, A., et al. (2014). Disease manifestations and pathogenic mechanisms of Group A Streptococcus. Clin. Microbiol. Rev. 27, 264–301. doi: 10.1128/cmr.00101-13
Wang, B., Dileepan, T., Briscoe, S., Hyland, K. A., Kang, J., Khoruts, A., et al. (2010). Induction of TGF-beta1 and TGF-beta1-dependent predominant Th17 differentiation by group A streptococcal infection. Proc. Natl. Acad. Sci. U S A. 107, 5937–5942. doi: 10.1073/pnas.0904831107
Wang, J., Ma, C., Li, M., Gao, X., Wu, H., Dong, W., et al. (2023). Streptococcus pyogenes: pathogenesis and the current status of vaccines. Vaccines (Basel). 11, 1510. doi: 10.3390/vaccines11091510
Wessels, M. R. (2019). Capsular polysaccharide of group A streptococcus. Microbiol. Spectr. 7, GPP3-0050-2018. doi: 10.1128/microbiolspec.GPP3-0050-2018
Wierzbicki, I. H., Campeau, A., Dehaini, D., Holay, M., Wei, X., Greene, T., et al. (2019). Group A streptococcal S protein utilizes red blood cells as immune camouflage and is a critical determinant for immune evasion. Cell Rep. 29, 2979–2989.e2915. doi: 10.1016/j.celrep.2019.11.001
Xian, S., Li, Y., Bai, L., Tang, S., Meng, Z., Wen, H., et al. (2024). Potential involvement of M1 macrophage and VLA4/VCAM-1 pathway in the valvular damage due to rheumatic heart disease. Front. Biosci. (Landmark Ed). 29, 219. doi: 10.31083/j.fbl2906219
Yu, D., Liang, Y., Lu, Q., Meng, Q., Wang, W., Huang, L., et al. (2021). Molecular characteristics of Streptococcus pyogenes isolated from Chinese children with different diseases. Front. Microbiol. 12, 722225. doi: 10.3389/fmicb.2021.722225
Yu, D., Liang, Y., Lu, Q., Zheng, Y., Gao, G. F., Yang, Y. (2023). Thoughts on group A streptococci. Lancet Microbe. 4, e484–e485. doi: 10.1016/s2666-5247(23)00138-6
Yu, D., Zheng, Y., Gao, G. F., Yang, Y. (2024). Additional thoughts on group A streptococci. Lancet Microbe. 5, 100876. doi: 10.1016/S2666-5247(24)00099-5
Keywords: rheumatic fever, rheumatic heart disease, group A Streptococcus (GAS), pathogenesis, vaccine
Citation: Zhuang S, Guo D and Yu D (2025) A mini review of the pathogenesis of acute rheumatic fever and rheumatic heart disease. Front. Cell. Infect. Microbiol. 15:1447149. doi: 10.3389/fcimb.2025.1447149
Received: 11 June 2024; Accepted: 24 March 2025;
Published: 10 April 2025.
Edited by:
Christos Koutsianas, National and Kapodistrian University of Athens, GreeceReviewed by:
Madeleine W. Cunningham, University of Oklahoma Health Sciences Center, United StatesCopyright © 2025 Zhuang, Guo and Yu. This is an open-access article distributed under the terms of the Creative Commons Attribution License (CC BY). The use, distribution or reproduction in other forums is permitted, provided the original author(s) and the copyright owner(s) are credited and that the original publication in this journal is cited, in accordance with accepted academic practice. No use, distribution or reproduction is permitted which does not comply with these terms.
*Correspondence: Dingle Yu, eXVkaW5nbGU4MTFAMTYzLmNvbQ==
Disclaimer: All claims expressed in this article are solely those of the authors and do not necessarily represent those of their affiliated organizations, or those of the publisher, the editors and the reviewers. Any product that may be evaluated in this article or claim that may be made by its manufacturer is not guaranteed or endorsed by the publisher.
Research integrity at Frontiers
Learn more about the work of our research integrity team to safeguard the quality of each article we publish.