- 1Malaria Parasite Biology Group, Max Planck Institute for Infection Biology (MPIIB), Berlin, Germany
- 2Membrane Protein Structural Biology Group, Center for Structural Systems Biology (CSSB), Hamburg, Germany
- 3Institute of Transfusion Medicine, University Medical Center Hamburg-Eppendorf (UKE), Hamburg, Germany
Host iron deficiency is protective against severe malaria as the human malaria parasite Plasmodium falciparum depends on bioavailable iron from its host to proliferate. The essential pathways of iron acquisition, storage, export, and detoxification in the parasite differ from those in humans, as orthologs of the mammalian transferrin receptor, ferritin, or ferroportin, and a functional heme oxygenase are absent in P. falciparum. Thus, the proteins involved in these processes may be excellent targets for therapeutic development, yet remain largely unknown. Here, we show that parasites cultured in erythrocytes from an iron-deficient donor displayed significantly reduced growth rates compared to those grown in red blood cells from healthy controls. Sequencing of parasite RNA revealed diminished expression of genes involved in overall metabolism, hemoglobin digestion, and metabolite transport under low-iron versus control conditions. Supplementation with hepcidin, a specific ferroportin inhibitor, resulted in increased labile iron levels in erythrocytes, enhanced parasite replication, and transcriptional upregulation of genes responsible for merozoite motility and host cell invasion. Through endogenous GFP tagging of differentially expressed putative transporter genes followed by confocal live-cell imaging, proliferation assays with knockout and knockdown lines, and protein structure predictions, we identified six proteins that are likely required for ferrous iron transport in P. falciparum. Of these, we localized PfVIT and PfZIPCO to cytoplasmic vesicles, PfMRS3 to the mitochondrion, and the novel putative iron transporter PfE140 to the plasma membrane for the first time in P. falciparum. PfNRAMP/PfDMT1 and PfCRT were previously reported to efflux Fe2+ from the digestive vacuole. Our data support a new model for parasite iron homeostasis, in which PfE140 is involved in iron uptake across the plasma membrane, PfMRS3 ensures non-redundant Fe2+ supply to the mitochondrion as the main site of iron utilization, PfVIT transports excess iron into cytoplasmic vesicles, and PfZIPCO exports Fe2+ from these organelles in case of iron scarcity. These results provide new insights into the parasite’s response to differential iron availability in its environment and into the mechanisms of iron transport in P. falciparum as promising candidate targets for future antimalarial drugs.
1 Introduction
Iron is an essential micronutrient for all living organisms and has been associated with virulence of many pathogens. Iron abundance increases the replication of human immunodeficiency virus (HIV) (Drakesmith and Prentice, 2008) and Mycobacterium tuberculosis (Zhang et al., 2020), and promotes biofilm formation in Pseudomonas aeruginosa (Kang and Kirienko, 2018). A “fight for iron” has been described between bacteria and the human host in the gastrointestinal tract (Sousa Gerós et al., 2020), where the metal skews the composition of the gut microbiome by facilitating the growth of enteropathogenic Escherichia coli and Salmonella (Paganini and Zimmermann, 2017). Similarly, cancer cells require more iron compared to healthy cells (Brown et al., 2020) and higher ferritin levels in individuals diagnosed with COVID-19 were associated with increased disease severity and lethality (Taneri et al., 2020).
Host iron deficiency is known to be protective against severe malaria (Nyakeriga et al., 2004; Gwamaka et al., 2012; Clark et al., 2014; Brabin et al., 2020) and iron chelators have cytocidal effects on the human malaria parasite Plasmodium falciparum (Thipubon et al., 2015). This obligate intracellular parasite depends on bioavailable iron for its proliferation and relies entirely on the host to meet its nutrient requirements (Scholl et al., 2005). Furthermore, P. falciparum senses environmental fluctuations (Brancucci et al., 2017; Mancio-Silva et al., 2017; Chou et al., 2018) and modulates its virulence in response (Mancio-Silva et al., 2017). While iron is crucial for DNA replication and repair, mitochondrial electron transport, and redox regulation, it becomes toxic when in excess, as it is a source of damaging reactive oxygen species (Sigala and Goldberg, 2014). Importantly for therapeutic development, the mechanisms of iron acquisition, storage, detoxification, and export in the parasite are different from those in humans, as orthologs of the mammalian transferrin receptor, ferritin, or ferroportin, and a functional heme oxygenase are absent in Plasmodium (Mach and Sutak, 2020).
While human blood plasma contains between 10 and 30 µM total iron and an erythrocyte carries approximately 20 mM Fe (Egan et al., 2002), only 3 µM labile iron is present in the cytosol of uninfected red blood cells, and 1.6 µM in P. falciparum-infected ones (Loyevsky et al., 1999). An estimated total iron concentration of 500 mM (Becker et al., 2004) is reached within the parasite’s digestive vacuole (DV), where iron-containing hemoglobin (Hb) is digested and the released heme is detoxified by biocrystallization into hemozoin (Wunderlich et al., 2012). However, P. falciparum cannot access this iron source and is thought to acquire bioavailable Fe2+ from the host cell (Kloehn et al., 2020). Over-elevated ferrous iron levels likely compromise the integrity of the DV membrane and cytosolic iron also needs to be regulated to prevent oxidative stress (Mach and Sutak, 2020). Iron detoxification in the parasite can be achieved by translocating the metal ion into dynamic intracellular Fe2+ stores, which may include acidocalcisomes – cytoplasmic vesicles that contain high concentrations of phosphate, calcium, iron, and zinc (Huang et al., 2014). In contrast to Trypanosoma brucei (Huang et al., 2014), no transport proteins have yet been experimentally shown to localize to the acidocalcisome membrane in P. falciparum (Magowan et al., 1997; Ruiz et al., 2004). Like the DV, these organelles are thought to be acidified by the plant-like V-ATPase and their low internal pH may fuel secondary active transport processes (Wunderlich et al., 2012; de Oliveira et al., 2021).
P. falciparum encodes approximately 200 transmembrane or membrane-associated transport proteins (channels, pores, carriers, and pumps), many of which are essential for parasite growth and lack human homologs (Wunderlich, 2022). For instance, the vacuolar iron transporter PfVIT (PF3D7_1223700), an ortholog of Arabidopsis thaliana VIT1 (expect value (E) = 5 x 10-29, 30.5% identity, 87% coverage, as determined by position-specific iterated BLAST (Altschul et al., 1997)), is a Fe2+/H+ exchanger that plays a role in iron detoxification (Slavic et al., 2016; Labarbuta et al., 2017; Sharma et al., 2021). While its orthologs were localized to the endoplasmic reticulum in Plasmodium berghei (Slavic et al., 2016) and to the vacuolar compartment in Toxoplasma gondii (Aghabi et al., 2023), the subcellular localization in P. falciparum had not been investigated experimentally prior to this study. Similarly, the Zrt-, Irt-like protein domain-containing protein (ZIPCO) was suggested to import Fe2+ and Zn2+ into the cytosol and localized to the parasite plasma membrane (PPM) in P. berghei sporozoites in indirect immunofluorescence assays (Sahu et al., 2014), but PfZIPCO (ZIP domain-containing protein, PF3D7_1022300) had not been studied yet. The chloroquine resistance transporter PfCRT (PF3D7_0709000) and the natural resistance-associated macrophage protein PfNRAMP (PF3D7_0523800, also called PfDMT1 for divalent metal transporter 1, although this abbreviation is already in use for the drug/metabolite transporter 1) have been detected at the digestive vacuolar (DV) membrane (Waller et al., 2003; Wichers et al., 2022). Both proteins were proposed to export Fe2+ into the cytosol in symport with protons on the basis of transport assays using Xenopus oocytes (Bakouh et al., 2017) and proliferation assays with a conditional knockdown line under different iron conditions (Loveridge and Sigala, 2024), respectively.
In Saccharomyces cerevisiae, a model organism for eukaryotic iron homeostasis, the mitochondrial carrier protein MRS3 (mitochondrial RNA-splicing protein 3) was shown to ensure Fe2+ supply to the mitochondrion (Mühlenhoff et al., 2003; Froschauer et al., 2009; Brazzolotto et al., 2014) and its ortholog TgMIT (mitochondrial iron transporter) was detected at the same organelle in T. gondii (Aghabi et al., 2023). The mitochondrion of P. falciparum is also the focal point for cellular iron metabolism and contains iron-dependent proteins implicated in the biosynthesis of heme and iron-sulfur clusters, redox reactions, and electron transport (Mach and Sutak, 2020). Because of sequence similarity (35.1% identity with the yeast ortholog, E = 3 x 10-14, 26% coverage), it was proposed that PfMRS3 (also known as mitoferrin (PfMFRN), PF3D7_0905200) mediates Fe2+ import into the mitochondrion in P. falciparum (Mather et al., 2007). However, no experimental evidence was collected and it is known that not only the localization but also the structure and function of homologous proteins can vary in related apicomplexan parasites (Mather et al., 2007; Sloan et al., 2021).
Despite the importance of iron for P. falciparum virulence, the fundamental understanding of the molecular mechanisms of iron sensing, acquisition, utilization, and regulation in the parasite remains limited. The goal of this exploratory study was to dissect how the parasite responds to differences in iron availability in its environment and to identify putative iron transporters as potential new antimalarial drug targets. We investigated growth and gene expression of the laboratory P. falciparum strain 3D7 under control (iron-replete), high-iron and low-iron conditions, and in the presence of the iron-regulatory peptide hormone hepcidin. In the human body, hepcidin is produced to reduce the concentration of serum iron when it rises above a certain threshold. The hormone binds specifically to ferroportin on the surface of many cell types including erythrocytes (Zhang et al., 2018) and can sterically inhibit the transporter’s iron export activity, thereby increasing intracellular and decreasing serum iron levels (Aschemeyer et al., 2018; Billesbølle et al., 2020). Here, whole-transcriptome sequencing was used to identify putative iron transport proteins on the basis of differential gene expression patterns between high vs. low-iron conditions. We then characterized these proteins by analyzing their subcellular localization in live parasites, their predicted 3D structures, and the growth rates of the respective knockout or knockdown parasite lines under various iron conditions.
2 Materials and methods
2.1 P. falciparum culture and proliferation assays
The P. falciparum strain 3D7 was cultured according to modified standard procedures (Trager and Jensen, 1976) at 5% hematocrit using human 0 Rh+ erythrocytes from the University Medical Center Hamburg-Eppendorf (UKE), Germany, at 1% O2, 5% CO2 and 94% N2. RPMI 1640 medium was supplemented with 0.5% (w/v) AlbuMAX II, 20 µg/mL gentamicin and 100 µM hypoxanthine (Thermo Fisher Scientific). Mature schizonts were obtained by treating schizonts at 40 hours post invasion (hpi) with 1 mM compound 2 (4-[7-[(dimethylamino)methyl]-2-(4-fluorphenyl)imidazo[1,2-α]pyridine-3-yl]pyrimidin-2-amine, LifeArc) for 8 h. To count the number of merozoites per mature schizont, Giemsa-stained blood smears were analyzed by light microscopy. Only single-infected cells with one digestive vacuole were taken into account.
To assess parasite proliferation over six days, a previously described assay on the basis of flow cytometry was employed (Malleret et al., 2011). Parasites were synchronized to a 3-h age window by isolating late schizonts from a 60% Percoll (GE Healthcare) gradient and culturing these for 3 h with fresh erythrocytes (Rivadeneira et al., 1983), followed by controlled elimination of advanced parasite stages using 5% (w/v) D-sorbitol (Carl Roth) for 10 min at 37°C (Lambros and Vanderberg, 1979). The growth assay was started at 0.1% parasitemia using the resulting ring-stage parasites at 0 – 3 hpi. The parasitemia was determined at the trophozoite stage every two days by flow cytometry and culture media with the respective supplements were exchanged daily.
2.2 Flow cytometry
To determine parasitemia, 20 µL of resuspended parasite culture was added to 80 μL culture medium and stained with 5 μg/mL SYBR Green I (Thermo Fisher Scientific) and 4.5 μg/mL dihydroethidium (DHE, Sigma-Aldrich) in the dark for 20 min at room temperature. Stained cells were washed with PBS three times and analyzed with an ACEA NovoCyte flow cytometer and NovoExpress Software (version 1.6.1, Agilent). Forward and side scatter gating was used to identify erythrocytes and SYBR Green I fluorescence intensity to determine the number of parasitized cells per 100,000 events recorded for each replicate. For Phen Green SK measurements, uninfected erythrocytes were washed with PBS and incubated with 10 µM Phen Green SK in PBS at 37°C for 60 min. DHE at 4.5 μg/mL was added during the last 20 min of incubation. After three washes with PBS, the cells were analyzed as described above.
2.3 Sample collection for RNA extraction and RNA sequencing
Parasites were synchronized to a three-hour window after invasion of erythrocytes from the respective donor as described above. Samples for RNA-sequencing were prepared in triplicate for each condition and time point, i.e., three separate parasite cultures each were grown in parallel for a total of at least two weeks. During the second IDC, two 10-mL dishes each were harvested for parasites at the ring stage (6 – 9 hpi) and one 10-mL dish each for trophozoites (26 – 29 hpi). Samples were collected by centrifuging the culture for 5 min at 800 g and 37°C and dissolving the erythrocyte pellet using 5 mL TRIzol (ThermoFisher Scientific) prewarmed to 37°C, followed by immediate transfer to -80°C for storage. The parasitemia was 0.3% at the start of the experiments with high, control and low-iron donor blood and 2 – 3% at the time of harvest. Parasite cultures treated with 0.7 µM hepcidin (Bachem) had a starting parasitemia of 0.6% and untreated cultures 1% to reach a parasitemia of 4 – 5% during the second cycle. For each experiment, the parasitemia was kept consistent at the point of harvest as high parasite densities can affect transcription (Chou et al., 2018).
For RNA extraction, the samples frozen in TRIzol were thawed, mixed thoroughly with 0.1 volume cold chloroform, and incubated at room temperature for 3 min. Following centrifugation at 20,000 g and 4°C for 30 min, the supernatants were transferred to fresh vials and combined with 70% ethanol of equal volume. RNA was purified using the RNeasy MinElute Kit (Qiagen) by on-column DNase I digest for 30 min and elution with 14 µL water. The GLOBINclear Human Kit (ThermoFisher Scientific) was then employed to deplete human globin mRNA in all samples. The Qubit RNA HS Assay Kit and Qubit 3.0 fluorometer (ThermoFisher Scientific) were used for RNA quantification. Upon arrival at the EMBL Genomics Core Facility (GeneCore Heidelberg, Germany), the RNA quality of each sample was evaluated using the RNA 6000 Nano kit and Bioanalyzer 2100 (Agilent). The median RNA integrity number (RIN) of all samples was 7.30 (IQR: 6.85 – 8.15, Supplementary Figure S1). Individually barcoded strand-specific libraries for mRNA sequencing were prepared from total RNA samples of high quality (approximately 150 ng per sample) using the NEBNext® RNA Ultra II Directional RNA Library Prep Kit (New England Biolabs) for 12 PCR cycles on the liquid handler Biomek i7 (Beckman Coulter) at GeneCore. Libraries that passed quality control were pooled in equimolar amounts, and a 2 pM solution of this pool was sequenced unidirectionally on a NextSeq® 500 System (Illumina) at GeneCore, resulting in about 500 million reads of 85 bases each.
2.4 RNA-sequencing read mapping and data analysis
Following successful initial quality control of the RNA-sequencing reads with FastQC version 0.11.8 (Andrews, 2010), sequencing adapters were trimmed using Cutadapt version 2.10 (Martin, 2011). A genome index was generated using the FASTA sequence file of the P. falciparum 3D7 genome release 46 (PlasmoDB-46_Pfalciparum3D7_Genome.fasta) and the GFF3 annotation file (PlasmoDB-46_Pfalciparum3D7.gff), both obtained from PlasmoDB (Aurrecoechea et al., 2009), with STAR version 2.7.5c (Dobin et al., 2013). The same R package was used to align reads to the genome with a maximum of three allowed mismatches (–outFilterMismatchNmax 3). To consolidate the results obtained with FastQC and STAR alignments, a single report file was created using MultiQC version 1.9 (Ewels et al., 2016).
The mapped reads were then summarized in Sequence Alignment/Map (SAM) format using featureCounts (Liao et al., 2014) from the R package Rsubread version 2.2.1 (Liao et al., 2013). For counting mapped reads per gene using featureCounts, fragments with a minimum length of 50 bases were considered (minFragLength = 50). Therefore, gene IDs and lengths of transcripts were extracted from PlasmoDB-46_Pfalciparum3D7_AnnotatedTranscripts.fasta with SAMtools faidx version 1.10.2 (Li et al., 2009). The R package edgeR 3.30.3 (Robinson et al., 2010) was used to compute RPKM values (reads per kilobase per million mapped reads) and for differential gene expression analysis. Gene annotations were retrieved from PlasmoDB (Aurrecoechea et al., 2009) and PhenoPlasm (Sanderson and Rayner, 2017). The results of these analyses were visualized with volcano plots using the R package Enhanced Volcano version 1.15.0 (Blighe et al., 2022). The raw and processed data (FASTA files, RPKM values and results of the differential gene expression analysis) can be accessed at https://www.ebi.ac.uk/biostudies/studies/E-MTAB-13411.
The highly polymorphic var, stevor, and rifin gene families were excluded from downstream analyses because of their great sequence diversity between parasites of the same strain during mitotic growth (Bozdech et al., 2003; Kidgell et al., 2006). Genes that were significantly regulated (defined as P < 0.05 according to the exact test for the negative binomial distribution with Benjamini-Hochberg correction (Benjamini and Hochberg, 1995) and an absolute value of log2 FC ≥ 0.2) were subjected to functional enrichment analysis with g:Profiler (https://biit.cs.ut.ee/gprofiler/gost (Raudvere et al., 2019), accessed on August 17, 2022). The resulting GO, KEGG and REAC terms were summarized using REVIGO (http://revigo.irb.hr/) with the similarity value set to 0.5 (Supek et al., 2011) and visualized as in Thomson-Luque et al (Thomson-Luque et al., 2021) using the scientific color map “roma” (Crameri et al., 2020). To estimate parasite age, an algorithm developed by Avi Feller and Jacob Lemieux (Lemieux et al., 2009) was adapted to use expression data from Broadbent et al (Broadbent et al., 2015) with the time points 6, 14, 20, 24, 28, 32, 36, 40, 44, and 48 hpi as reference. The code and data used were deposited to Zenodo with the record ID 7996302 (https://zenodo.org/record/7996302).
2.5 Cloning of DNA constructs
For generating the GFP reporter lines, a homologous region of approximately 800 base pairs (bp) at the 3’ end of the respective gene was amplified without the stop codon from 3D7 gDNA using Phusion high fidelity DNA polymerase (New England Biolabs). A homology region of about 400 bp at the 5’ end of the respective gene was used for targeted gene disruption. The fragments were then inserted into pSLI-GFP (Birnbaum et al., 2017) using NotI and AvrII restriction sites. For glmS constructs, pSLI-GFP-glmS (Burda et al., 2020) was used as a vector instead. All oligonucleotides and plasmids used in this study are listed in Supplementary Table S3.
2.6 Transfection of P. falciparum
As described previously (Moon et al., 2013), parasites at the late schizont stage were purified using 60% Percoll (Rivadeneira et al., 1983) and electroporated with 50 μg DNA of the respective plasmid in a 0.2-cm gap cuvette (Bio-Rad Laboratories) using Amaxa Nucleofector 2b (Lonza). Either 4 nM WR99210 (Jacobus Pharmaceuticals) or 2 μg/mL blasticidin S (Life Technologies) was used for selecting transfectants. For the selection of parasites that were genomically modified using the SLI system (Birnbaum et al., 2017), 400 μg/mL G418 (ThermoFisher Scientific) was added to the culture medium once the parasitemia reached 5%. After the selection of modified parasites, genomic DNA was isolated with the QIAamp DNA Mini Kit (Qiagen) and diagnostic tests for correct integration into the genome were performed as specified earlier (Birnbaum et al., 2017).
2.7 Confocal live-cell microscopy
Erythrocytes infected with parasites at different stages at 3 – 6% parasitemia were incubated in culture medium with 20 nM MitoTracker Red, 200 nM ER Tracker Red or 100 nM LysoTracker Deep Red (Invitrogen, if applicable) at 37°C for 20 min. Then, 200 nM Hoechst-33342 (Invitrogen) was added for 10 min prior to washing the cells with Ringer’s solution (122.5 mM NaCl, 5.4 mM KCl, 1.2 mM CaCl2, 0.8 mM MgCl2, 11 mM D-glucose, 25 mM HEPES, 1 mM NaH2PO4, pH 7.4) prewarmed to 37°C and seeding on a chambered No. 1.5 polymer cover slip (Ibidi). After 5 min, unbound erythrocytes were removed by washing with Ringer’s solution and the sample was placed into an incubation chamber that maintained the microscope work area including the objective at 37°C. Images and videos were acquired using an SP8 confocal microscope system with a 63x oil-corrected lens (C-Apochromat, numerical aperture = 1.4) and Lightning deconvolution software (Leica), and processed using ImageJ version 2.9.0/1.53t (Schindelin et al., 2012). If fluorescence intensities were to be quantified, no averaging or deconvolution software was applied.
2.8 Conditional knockdown mediated by glmS ribozyme
For glmS-based knockdown induction (Prommana et al., 2013), highly synchronous parasites at the early ring stage were cultured with or without supplementation with 2.5 mM glucosamine (GlcN, Sigma-Aldrich). The knockdown was quantified by confocal live-cell microscopy using schizonts 36 h post GlcN treatment initiation. Images of parasites of similar size were acquired with the same settings and background-corrected fluorescence intensities (integrated density) as well as the size of the region of interest were determined using ImageJ version 2.9.0/1.53t (Schindelin et al., 2012), and the data visualized using Graph Pad Prism version 9.4.1.
2.9 Protein structure prediction
Structure predictions for monomeric proteins were obtained from AlphaFold Protein Structure Database version 3 (Jumper et al., 2021; Varadi et al., 2022) and homodimeric proteins were predicted using AlphaFold2-multimer version 2.2.2, database version 2.2.0 (Evans et al., 2022) deployed at the EMBL Hamburg computer cluster. Molecular visualization was performed with UCSF ChimeraX version 1.3 (Goddard et al., 2018). UCSF Chimera MatchMaker and Match → Align tools with default settings were used for structural comparison of the predicted structures of P. falciparum proteins with putative orthologs and sequence alignments were generated using the Match → Align tool (Meng et al., 2006). The DeepFRI server (https://beta.deepfri.flatironinstitute.org) was used to identify possible functional residues with the DeepFRI graph convolutional network (Gligorijević et al., 2021).
3 Results
3.1 Elevated erythrocyte labile iron levels promote P. falciparum proliferation in vitro
To investigate whether labile iron levels in the erythrocyte correlate with parasite replication rates, we established different iron conditions in vitro. The first approach was to culture P. falciparum 3D7 parasites in 0 Rh+ erythrocytes from voluntary blood donations by Caucasians aged 18 to 21 at the University Medical Center Hamburg-Eppendorf in Germany. Therefore, samples from a person with an elevated ferritin level (greater than 200 µg/L (Marfil-Rivera, 2015), in this case 231 µg/L ferritin, 18.2 g/dL Hb, 51.5% hematocrit), an iron-deficient individual (serum ferritin < 12 ng/ml (Knovich et al., 2009), here: 3 µg/L ferritin, 11.4 g/dL Hb, 36.3% hematocrit) and a healthy donor (21 µg/L ferritin, 15.0 g/dL Hb, 42.3% hematocrit) were used. Secondly, infection of red blood cells from other healthy individuals with or without the addition of 0.7 µM hepcidin to the culture medium was compared. This concentration was chosen as it had the strongest effect on parasite proliferation in preliminary experiments, and is expected to increase intracellular Fe2+ as it is twice as high as the hepcidin level needed to reduce 55Fe export from preloaded mature erythrocytes by 30% within one hour of incubation (Zhang et al., 2018).
Relative labile iron levels in uninfected erythrocytes were estimated by determining the mean fluorescence intensity (MFI) of the iron-sensitive dye Phen Green SK in 100,000 cells per replicate using flow cytometry. As binding of ferrous iron to the metal-binding moiety causes fluorescence quenching of the fluorophore, a reduction in fluorescence intensity indicates higher labile iron levels (Petrat et al., 1999). In erythrocytes from the iron-deficient donor, the Phen Green SK MFI was 43% higher relative to control, confirming reduced labile iron levels (Figure 1A). The parasite replication rate after one intraerythrocytic developmental cycle (IDC) decreased by 16% (Figure 1B), the DNA content of late schizonts by 19% (Figure 1C) and the number of merozoites counted per late schizont by 14% (Figure 1D). In contrast, labile iron levels of erythrocytes from the donor with higher iron status were only slightly increased (without statistical support, two-tailed unpaired t tests with Welch’s correction for unequal variances and adjusted with the Holm-Šídák method for multiple comparisons, P = 0.25) relative to blood with normal iron level (healthy control) – as were the parasite proliferation rate, the DNA content and the merozoite number of mature schizonts (Figure 1). To further increase intracellular labile iron levels, we incubated parasites with 0.7 µM hepcidin during one IDC, resulting in 11% reduced Phen Green SK MFI compared to control (Figure 1A). Under these conditions, the parasite growth rate increased by 57% (Figure 1B), the DNA content per schizont by 16% (Figure 1C), and the number of merozoites produced per schizont by 15% (Figure 1D).
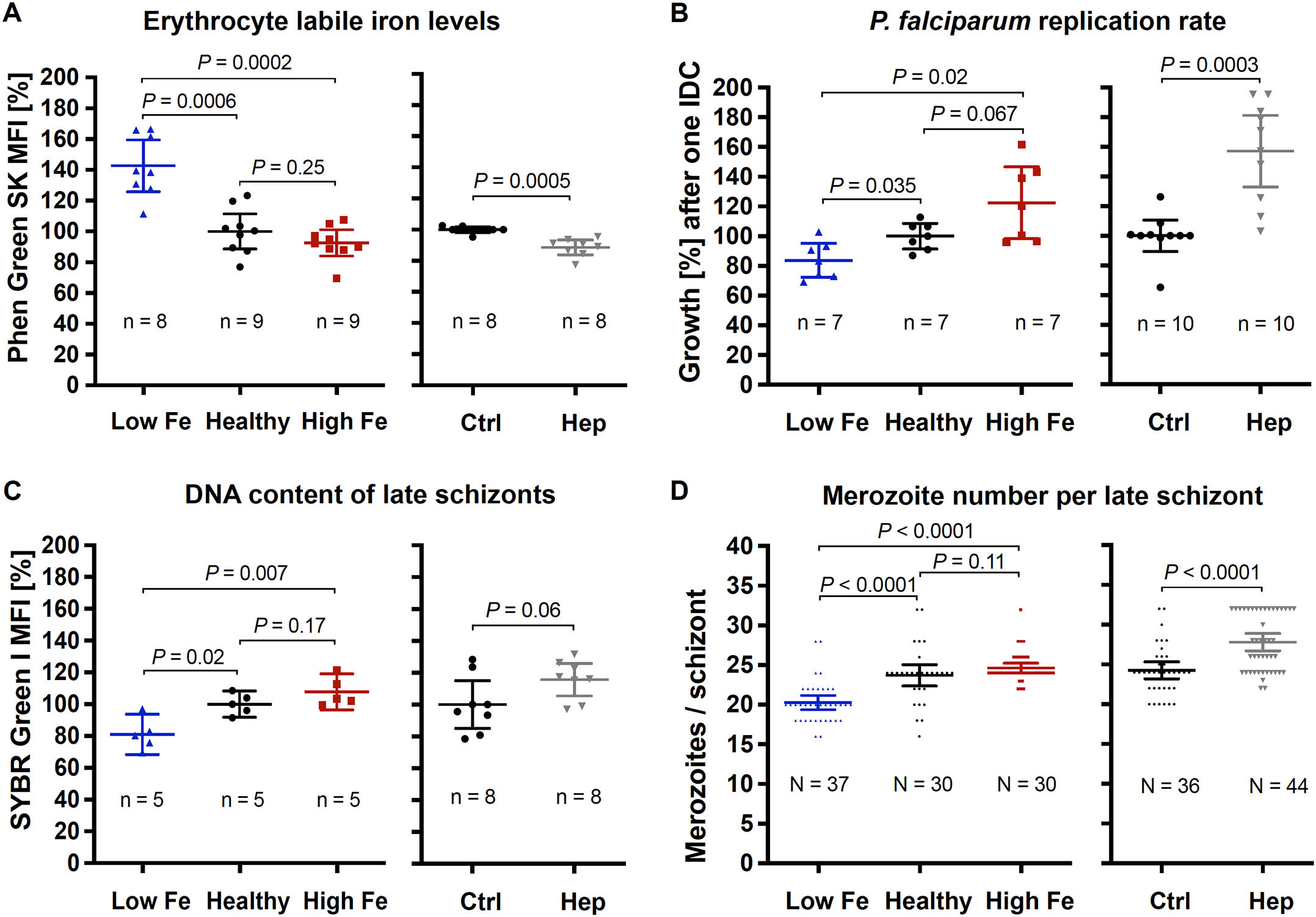
Figure 1. Effects of the iron status of the blood donor and of hepcidin on (A) erythrocyte labile iron levels, (B) P. falciparum 3D7 growth rates, (C) DNA content per mature schizont, and (D) the number of merozoites per mature schizont. The relative labile iron level and DNA content per cell were assessed in the presence or absence of 0.7 µM hepcidin (Hep) by measuring the mean fluorescence intensity (MFI) of Phen Green SK or SYBR Green I compared to control (Ctrl, untreated, normal hemoglobin level) using flow cytometry. Therefore, 100,000 cells were analyzed per replicate. Parasite growth rates refer to the fold change in parasitemia after one intraerythrocytic developmental cycle in vitro relative to control as determined by flow cytometry with SYBR Green I (Malleret et al., 2011). Mature schizonts were obtained by treating schizonts at 40 hpi with 1 mM compound 2 (4-[7-[(dimethylamino)methyl]-2-(4-fluorphenyl)imidazo[1,2-α]pyridine-3-yl]pyrimidin-2-amine) for 8 h. To count the number of merozoites, Giemsa-stained blood smears were analyzed microscopically and only single-infected cells with one digestive vacuole were considered. Means and 95% confidence intervals (indicated by error bars) are shown. Statistical significance was calculated with two-tailed unpaired t tests with Welch’s correction for unequal variances and adjusted with the Holm-Šídák method for multiple comparisons except for merozoite numbers, which were compared using Mann-Whitney test. N represents the number of parasites and n the number of independent experiments.
Taken together, these data show that parasites grown in erythrocytes from an iron-deficient donor displayed significantly reduced growth rates compared to healthy control. Our in vitro results also demonstrate that hepcidin treatment of control erythrocytes elevated intracellular Fe2+ concentrations and promoted parasite proliferation.
3.2 RNA-sequencing reveals differential expression of iron transporter genes
To identify iron-regulated mechanisms and putative iron transporters in P. falciparum, we carried out whole-transcriptome profiling using bulk RNA-sequencing (Figure 2). P. falciparum 3D7 parasites were cultured either using erythrocytes from a donor with higher, control (healthy) or low iron status (experiment 1); or with red blood cells from another healthy donor in the presence or absence of 0.7 µM hepcidin (experiment 2). Samples from three biological replicates per condition were harvested at the ring and trophozoite stage (6 – 9 hpi and 26 – 29 hpi) during the second IDC under the conditions specified.
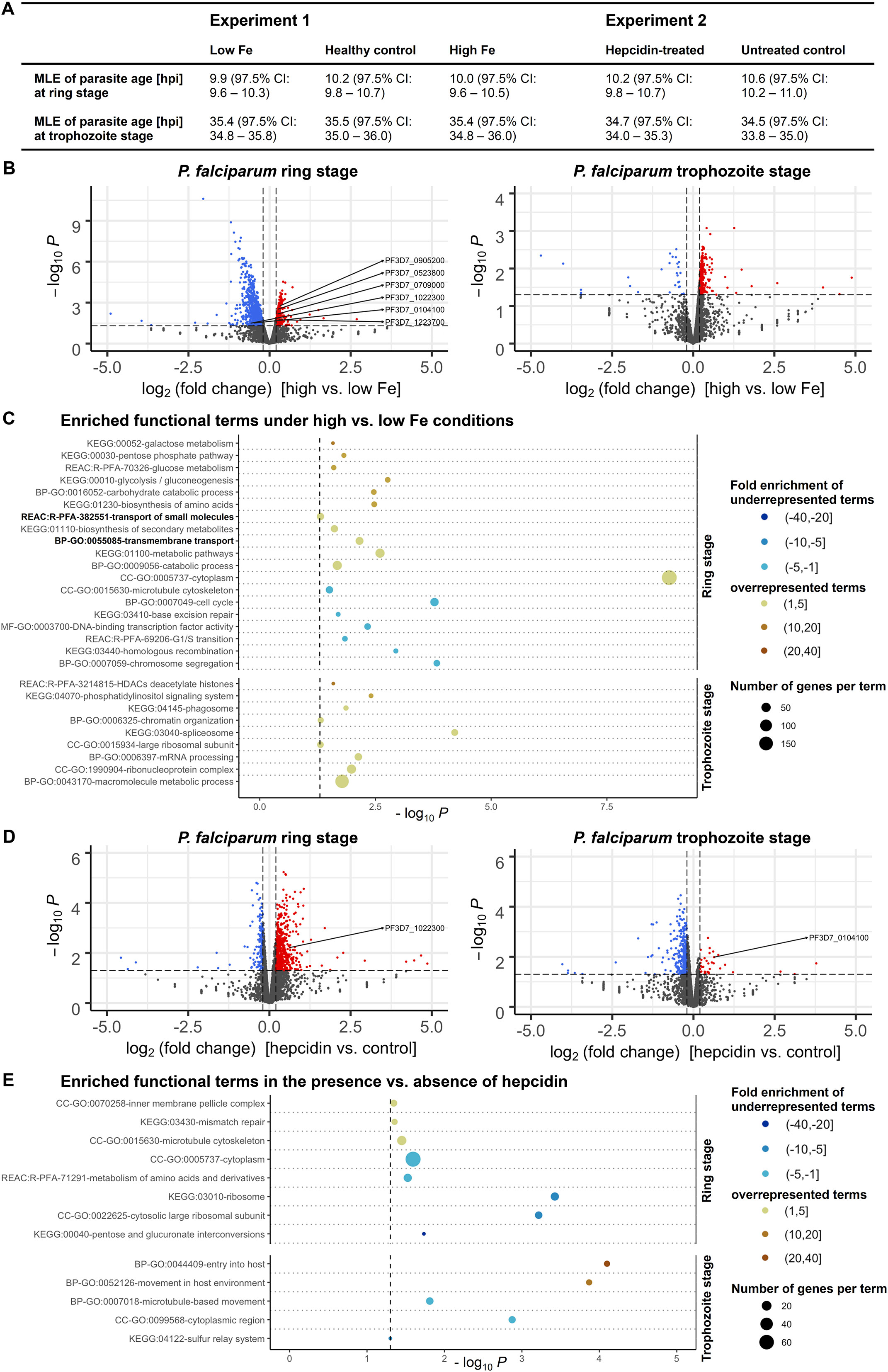
Figure 2. Differential expression of P. falciparum 3D7 genes under various iron conditions. Parasites were cultured with erythrocytes from an individual with high, medium (healthy) or low iron status (experiment 1) or with red blood cells from another healthy donor in the presence or absence of 0.7 µM hepcidin (experiment 2). Samples were harvested at the ring and trophozoite stage (6 – 9 and 26 – 29 hours post invasion, hpi) with three biological replicates per time point and condition. The maximum likelihood estimation (MLE) of the average developmental age of the parasites for each condition and time point (A) was calculated using an algorithm developed by Avi Feller and Jacob Lemieux (Lemieux et al., 2009). CI, confidence interval. The volcano plots (B, D) show transcriptional changes of all parasite genes. Red dots indicate significantly (P < 0.05, exact test for negative binomial distribution) upregulated genes (log2 (fold change) ≥ 0.2), blue dots stand for significantly downregulated genes (log2 (fold change) ≤ -0.2), while grey dots represent genes that did not significantly differ in transcription under the conditions described (P ≥ 0.05 and/or -0.2 < log2 (fold change) < 0.2). Differentially expressed genes encoding putative iron transporters (see Table 1) are labeled. Panels (C, E) show the enrichment of Gene Ontology (GO), Kyoto Encyclopedia of Genes and Genomes (KEGG) and Reactome (REAC) terms among significantly regulated genes excluding var, stevor and rifin gene families at the two time points. The functional terms were summarized using REVIGO (Supek et al., 2011) to remove redundancies, represented by circles and plotted according to the significance of their enrichment (-log10 (adjusted P), hypergeometric test). The size of the circle is proportional to the number of differentially regulated genes in the dataset that are associated with the respective term, while the color stands for the fold enrichment. The gray dashed line indicates the threshold of the adjusted P value (-log10 0.05 = 1.3).
To exclude the possibility that differences in mRNA abundance were a consequence of divergent progression through the IDC under different nutritional conditions, we assessed the average developmental age of the parasites in each sample on the basis of a statistical maximum likelihood estimation (MLE) method of transcriptional patterns according to Lemieux et al. (Lemieux et al., 2009). The general transcriptional patterns of parasites were highly similar at individual time points and consistent across different experimental treatments, corresponding to those of a 3D7 reference strain (Broadbent et al., 2015) at approximately 10 hpi and 35 hpi (Figure 2A). This indicates that differences in mRNA abundance of parasites were not caused by divergent progression through the IDC but by direct effects of the experimental treatments. As the 3D7 strain we used for the experiments had a reduced total IDC length of 44 h instead of 48 h, possibly because of gene deletions that may have occurred during long-term culturing (Wichers et al., 2019; Stewart et al., 2020), it progresses through the cycle faster than the 3D7 reference strain (Broadbent et al., 2015). This may explain why the calculated MLEs of parasite age were higher than the actual values of 6 – 9 hpi and 26 – 29 hpi (Figure 2A).
Using a threshold of 1.5 for the fold change (FC) in gene expression (log2 FC of 0.585 or -0.585) yielded twelve significantly upregulated and 175 downregulated genes in ring-stage parasites under high vs. low-iron conditions (P < 0.05, exact test for the negative binomial distribution with Benjamini-Hochberg correction (Benjamini and Hochberg, 1995)). As differences in transporter gene transcription are typically small (Küpper and Kochian, 2010; Abrahamian et al., 2016), we examined the 351 upregulated and 770 downregulated genes with a significant expression change and a minimum absolute value of the log2 FC of 0.2 for this comparison (Figure 2B). The full RNA-sequencing datasets are available in the BioStudies repository (Sarkans et al., 2018) under accession number E-MTAB-13411 (https://www.ebi.ac.uk/biostudies/studies/E-MTAB-13411) and differential gene expression test results for individual genes are shown in Supplementary Tables S1 and S2. The highly polymorphic var, stevor, and rifin gene families were excluded from downstream analyses because of their significant sequence diversity between parasites of the same strain during mitotic growth (Bozdech et al., 2003; Kidgell et al., 2006). Functional Gene Ontology (GO), Kyoto Encyclopedia of Genes and Genomes (KEGG) and Reactome (REAC) term enrichment analyses of differentially expressed genes (DEGs) were performed using the g:Profiler web server (Raudvere et al., 2019).
Under high vs. low-iron conditions at the ring stage (6 – 9 hpi), the GO term for biological process GO:0055085 “transmembrane transport” was 2.8-fold enriched (P = 0.007, hypergeometric test) among significantly upregulated parasite genes (Figure 2C). Using the recently updated P. falciparum transporter list (Wunderlich, 2022), all genes with differential expression levels at the ring stage were then screened for transport proteins and all of the five genes previously proposed as iron transporters in Plasmodium (VIT, ZIPCO, NRAMP/DMT1, CRT, MRS3/MFRN (Mach and Sutak, 2020; Sloan et al., 2021)) were found differentially expressed (Table 1). The putative transporter PfE140 (Wunderlich, 2022), which is predicted to be essential (Zhang et al., 2018), may also be involved in iron transport, as it was differentially expressed under low-iron vs. control conditions at the ring stage (Table 1) as well as in the presence vs. absence of hepcidin in trophozoites (Figure 2D). Other significantly enriched functional terms at the ring stage under high-iron conditions were GO:0009056 “catabolic process”, GO:0020020 “food vacuole”, KEGG:01100 “metabolic pathways”, and GO:0005737 “cytoplasm” (Figure 2C). Among downregulated genes under high vs. low-iron conditions at the ring stage, KEGG:03440 “homologous recombination”, KEGG:03410 “base excision repair”, GO:0007049 “cell cycle”, and GO:0015630 “microtubule cytoskeleton” were enriched (Figure 2C). At the more metabolically active trophozoite stage (26 – 29 hpi), processes related to mRNA splicing and protein production were overrepresented in upregulated genes, as indicated by the 2.9-fold enrichment (P = 0.00006) of the KEGG:03040 pathway “spliceosome” and the 2.5-fold enrichment (P < 0.05) of the GO:0015934 term “large ribosomal subunit” (Figure 2C).
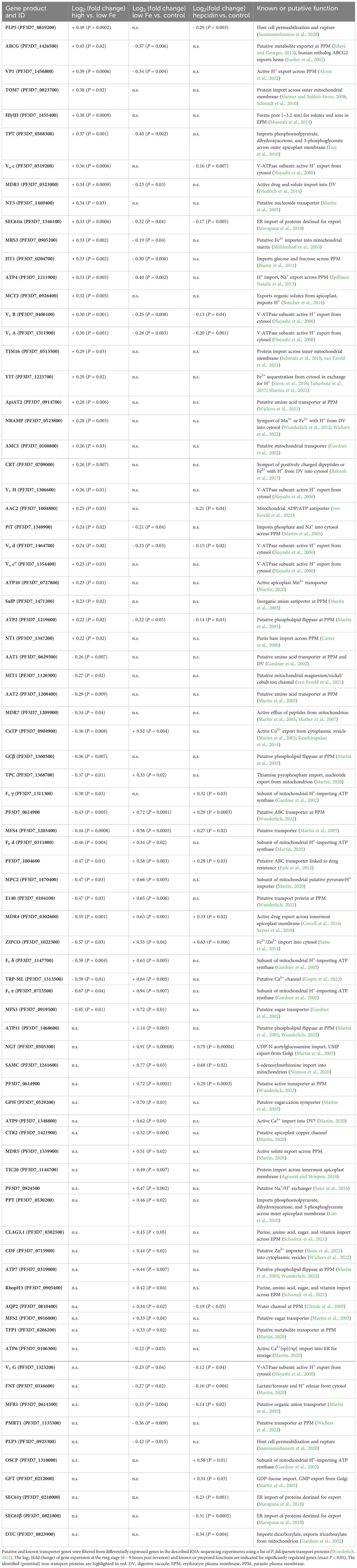
Table 1. P. falciparum transport proteins with differential gene expression under various iron conditions.
In contrast, hepcidin treatment resulted in reduced metabolism compared to control conditions, as KEGG:00040 “pentose and glucuronate interconversions”, REAC:R-PFA-71291 “metabolism of amino acids and derivatives”, GO:0005737 “cytoplasm”, and GO:0015934 “large ribosomal subunit” were significantly enriched in downregulated genes during the parasite ring stage at 6 – 9 hpi (Figure 2E). Among significantly upregulated genes in the presence vs. absence of hepcidin, the terms GO:0070258 “inner membrane pellicle complex” (P < 0.05), KEGG:03430 “mismatch repair” (P = 0.04), and GO:0015630 “microtubule cytoskeleton” (P = 0.04) were enriched at the ring stage. GO:0044409 “entry into host” (P = 0.00008) and GO:0052126 “movement in host environment” (P = 0.0001) were overrepresented at the trophozoite stage (Figure 2E), possibly linked to the observed increase in parasite proliferation (Figure 1B).
Our RNA-sequencing data also revealed the differential expression of genes involved in epigenetic, transcriptional, translational, and post-translational regulation. Under high vs. low-iron conditions, histone deacetylation and chromatin organization processes as well as GO:1990904 “ribonucleoprotein complex” were significantly enriched in upregulated genes at the trophozoite stage, and GO:000370 “DNA binding transcription factor activity” in downregulated genes at the ring stage (Figure 2C). Furthermore, the known iron-regulatory protein PfIRP or aconitate hydratase (Loyevsky et al., 2001; Hodges et al., 2005) was upregulated during the ring stage under high vs. low-iron conditions (log2 FC = 0.49, P = 0.00003) and downregulated in the presence of hepcidin (log2 FC = -0.27, P = 0.01) as compared to control. Many protein kinases involved in post-translational modifications and endocytosis were also upregulated at 26 – 29 hpi at high vs. low iron levels, as indicated by the enriched terms GO:0043170 “macromolecule metabolic process” and KEGG:04070 “phosphatidylinositol signaling system” (Figure 2C).
3.3 Localization of PfMRS3, PfVIT, PfZIPCO, and PfE140 in P. falciparum
On the basis of transcriptomic profiles and the P. falciparum transporter list (Wunderlich, 2022), six proteins with a potential role in iron transport were identified (Tables 1, 2). The subcellular localization of the four proteins that had not yet been localized in P. falciparum (PfMRS3, PfVIT, PfZIPCO, and PfE140) was then examined by endogenous tagging with GFP and confocal imaging of live parasites under physiological control conditions. At least two cell lines were generated per transporter candidate with consistent results and representative example images are shown in Figure 3. Diagnostic PCRs confirmed the fusion of gfp to the respective gene of interest and the absence of parental DNA at the original locus (Supplementary Figure S2). Only the PfMRS3 reporter cell line still contained wild-type DNA of the parental parasites even after prolonged WR99210/G418 selection and limiting dilution cloning (Supplementary Figure S2), indicating the importance of this mitochondrial transporter for asexual parasite growth during the blood stage.
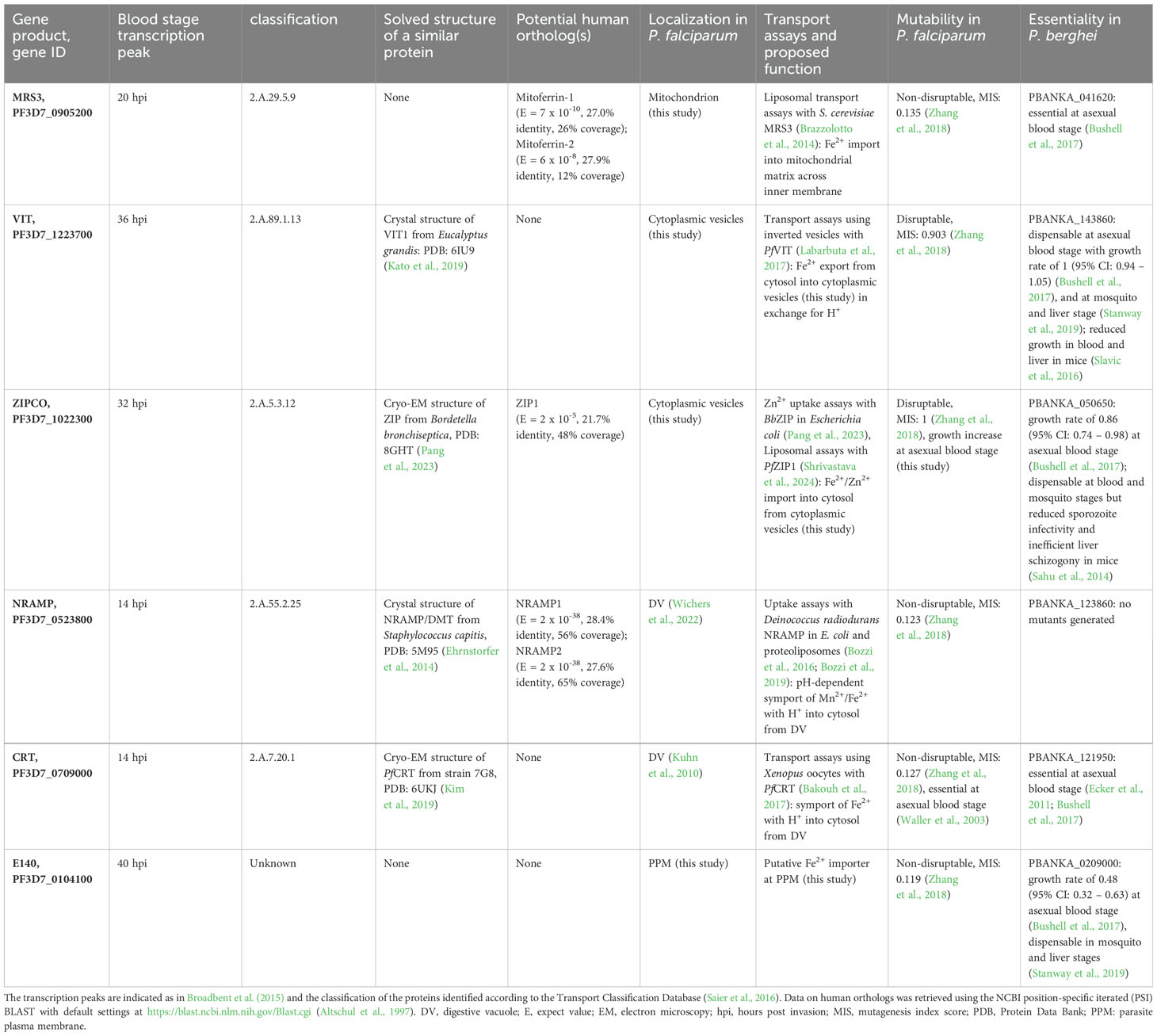
Table 2. Proteins identified by RNA-sequencing that may be involved in iron transport in P. falciparum.
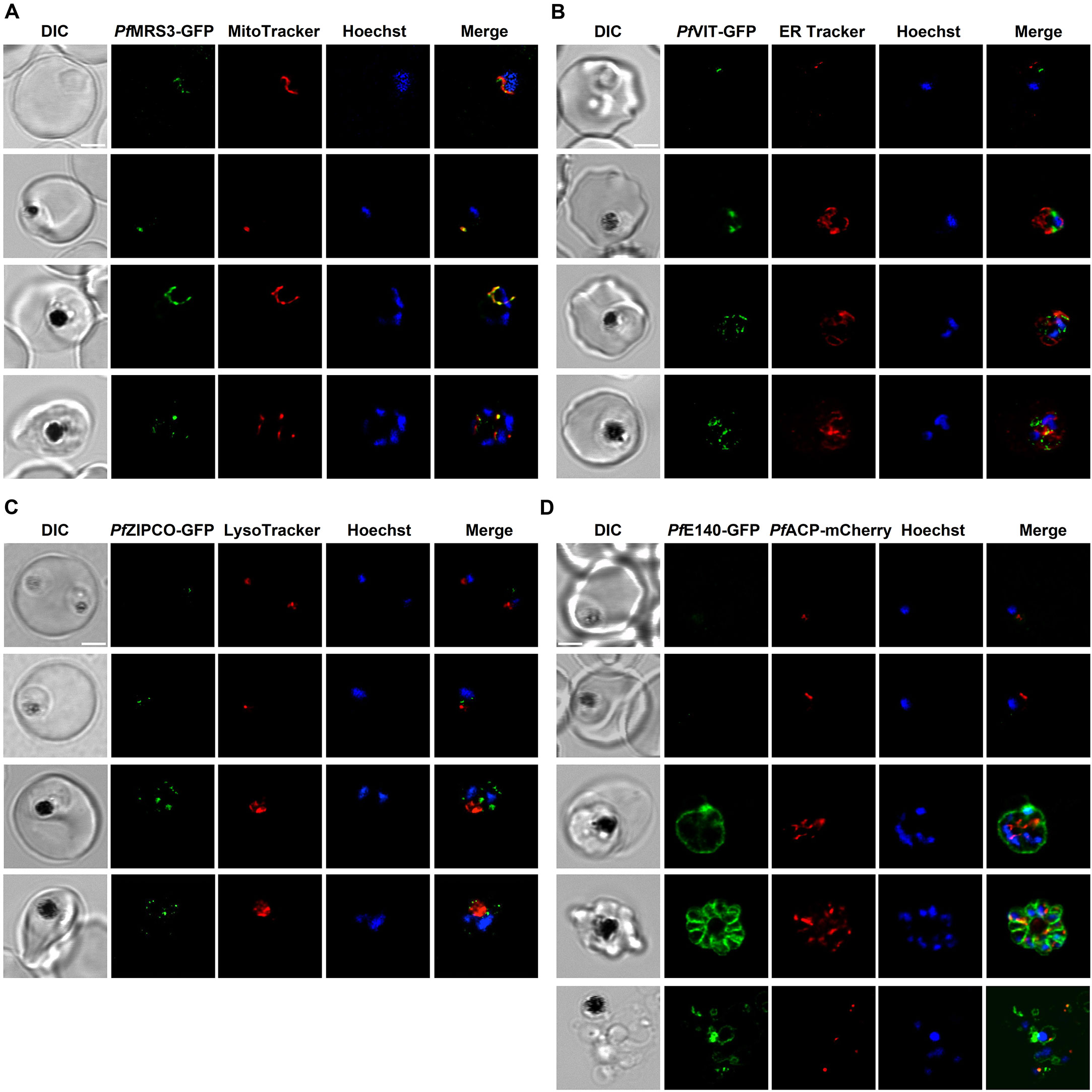
Figure 3. Subcellular localization of known and putative iron transport proteins. Representative erythrocytes infected with P. falciparum 3D7 parasites endogenously expressing GFP-tagged PfMRS3 (A), PfVIT (B), PfZIPCO (C) or PfE140 (D) were additionally stained with the fluorescent dyes Hoechst-33342, MitoTracker Red, ER Tracker Red and/or LysoTracker Deep Red. Co-transfection with a construct that encodes the 60 N-terminal amino acids of acyl carrier protein (PfACP) tagged with mCherry (Birnbaum et al., 2020) resulted in red fluorescence of the apicoplast. Live-cell images were taken under physiological conditions at 37°C using an SP8 confocal laser-scanning microscope (Leica). DIC, differential interference contrast. Scale bar, 2 µm.
The GFP-tagged mitochondrial carrier protein PfMRS3 exclusively localized to the mitochondrion, as determined by colocalization with MitoTracker Red (Figure 3A, Supplementary Video S1). PfVIT-GFP displayed a punctate fluorescence pattern within the cytoplasm (Figure 3B; Supplementary Video S2), which did not colocalize with ER Tracker Red in live cells (Figure 3B; Supplementary Video S2). Expression of PfZIPCO-GFP resulted in highly similar fluorescent cytoplasmic dots (Figure 3C; Supplementary Video S3). To test whether these could be acidocalcisomes, we employed LysoTracker Deep Red, commonly used to visualize small acidic organelles in T. brucei (Huang et al., 2014). However, the fluorescent dye only stained the DV in P. falciparum (Figure 3C; Supplementary Video S3) and no acidocalcisome-specific marker is currently available for this parasite. For both PfVIT-GFP and PfZIPCO-GFP, the number of cytoplasmic foci increased as the parasites matured from the ring to the late schizont stage (Figures 3B, C).
GFP-tagged PfE140 (PF3D7_0104100), also known as conserved Plasmodium membrane protein or CPMP (Lerch et al., 2017), localized to the parasite plasma membrane, as evidenced by the ring-like fluorescence pattern around newly formed merozoites (Figure 3D; Supplementary Video S4). The fluorescence intensity was very low at the ring and early trophozoite stage compared to schizonts. Because of amino acid sequence similarity (E = 9 x 10-5, 22.5% identity, 66% coverage) to the essential apicoplast transporter PfDER1-2 (Altschul et al., 1997; Spork et al., 2009), we also investigated the potential colocalization with the apicoplast marker PfACP (acyl carrier protein), which could not be detected (Figure 3D; Supplementary Video S4).
3.4 Role of identified proteins for asexual malaria parasite growth
To study the function of the putative transport proteins identified, we used targeted gene disruption (TGD) by selection-linked integration (SLI) to generate the corresponding knockout parasite lines for the putative iron transporters that are non-essential during P. falciparum blood stage: PfVIT and PfZIPCO (Figure 4A; Supplementary Figure S2). The cloning strategy requires a homology region of at least 400 bp, and GFP was cloned in frame with the truncated version of the respective transporter (the N-terminal 143 amino acids (aa) of 274-aa PfVIT or 117 of the 325 aa of PfZIPCO). The subcellular localization of the resulting GFP fusion protein was also assessed to confirm protein expression and elucidate the position of targeting signals. PfVIT (1–143)-GFP localized to cytoplasmic structures and PfZIPCO(1-117)-GFP to the DV and cytoplasmic vesicles (Figure 4A). Hence, both truncated proteins contained (a) sequence(s) for targeting to the observed vesicles. As additional DV staining is often non-specific, another part of the protein located within the C-terminal 208 aa of PfZIPCO may be required for exclusively vesicular localization.
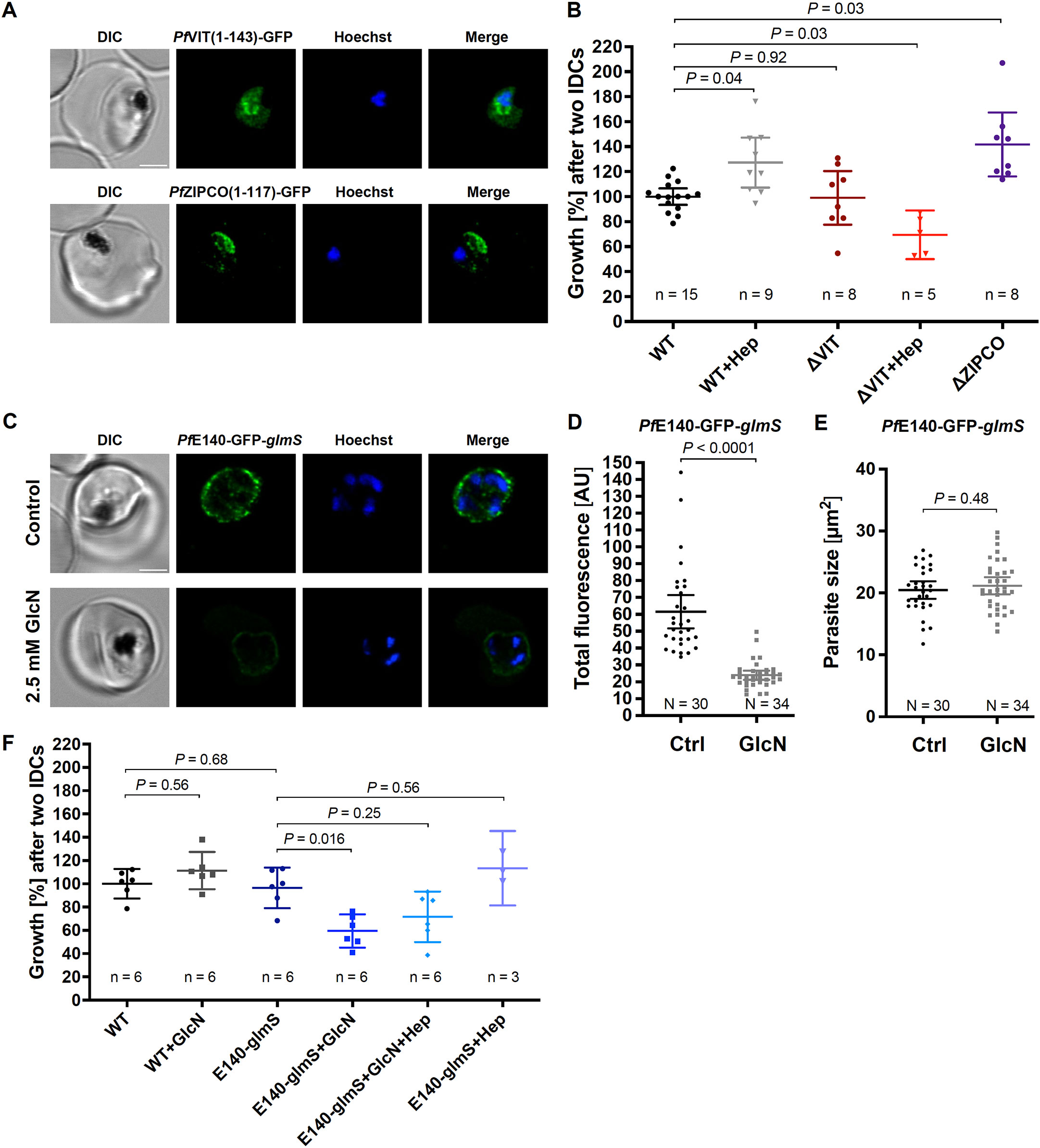
Figure 4. PfVIT and PfE140 are important for P. falciparum growth and may be involved in intracellular iron homeostasis. (A) Representative erythrocytes infected with P. falciparum 3D7 parasites that endogenously express a truncated version of PfVIT or PfZIPCO tagged with GFP (green). (B) Growth rates of knockout parasite lines generated. (C) Representative live 3D7 parasites endogenously expressing PfE140-GFP whose green fluorescence was reduced by glmS-mediated knockdown induced by treatment with 2.5 mM glucosamine for 36 h (GlcN) compared to untreated control (Ctrl). (D) Total parasite fluorescence intensities were quantified as background-corrected integrated densities using ImageJ version 2.9.0/1.53t (Schindelin et al., 2012) and compared using Mann-Whitney test. Images to which no averaging or deconvolution software was applied were used for quantification. (E) The size of the parasites was measured as the area of the region of interest and compared using equal variance unpaired t test. (F) Conditional knockdown of PfE140 induced by treatment with 2.5 mM GlcN results in a growth defect during asexual blood stage development. Live parasites were stained with Hoechst-33342 (blue) and imaged under physiological conditions at 37°C using an SP8 confocal laser-scanning microscope (Leica). DIC, differential interference contrast. Scale bar, 2 µm. Error bars represent 95% confidence intervals of the mean, N the number of parasites analyzed, n the number of independent experiments and Hep treatment with 0.7 µM hepcidin. Growth rates refer to the fold change in parasitemia after two intraerythrocytic developmental cycles in vitro relative to untreated wild-type 3D7 parasites (WT) as determined by flow cytometry with SYBR Green I (Malleret et al., 2011). Statistical significance of growth differences was calculated with two-tailed unpaired t tests with Welch’s correction for unequal variances and adjusted with the Holm-Šídák method for multiple comparisons.
Proliferation assays were then performed to determine the importance of the respective transporter for parasite growth. While the PfVIT knockout had no effect on parasite growth under standard conditions, addition of hepcidin reduced the growth rate of the ΔVIT line by 30% (Figure 4B). Of note, hepcidin generally had a smaller effect after two cycles of incubation (Figure 4B) than after one cycle compared to the first IDC (Figure 1B). Unexpectedly, knocking out PfZIPCO led to a growth rate increase by 42% after two IDCs relative to wild-type 3D7 parasites, and thus a rescue by hepcidin treatment was not tested for this knockout line (Figure 4B).
PfE140 is predicted to be essential (Zhang et al., 2018) and the only putative iron transporter identified that localized to the PPM (Figure 3), thus potentially important for iron uptake in P. falciparum. For an inducible knockdown, a glmS ribozyme sequence (Prommana et al., 2013) was introduced upstream of the 3’ untranslated region in the pSLI plasmid, allowing for conditional mRNA degradation by adding 2.5 mM glucosamine (GlcN) to the culture medium (Figure 4C; Supplementary Figure S2). The knockdown led to a 61% decrease in total parasite fluorescence intensity after 36 hours of GlcN treatment (Figure 4D) without affecting parasite size compared to untreated control (Figure 4E). Addition of GlcN also caused a 38% growth rate reduction of the PfE140-GFP-glmS line, which was rescued by hepcidin treatment to a proliferation level that was not significant different from that under standard culture conditions (P = 0.25, Figure 4F). The generation of a PfMRS3-knockdown line was not successful after four independent attempts of G418 selection for integrants after transient transfection, supporting the essentiality of the gene for asexual parasite growth (Zhang et al., 2018).
3.5 Functional implications of predicted transport protein structures
We next took advantage of the recent progress in protein structure prediction and generated models of the putative iron transport proteins identified (Table 2) using AlphaFold2 (Jumper et al., 2021; Varadi et al., 2022) and AlphaFold2-multimer (Evans et al., 2022). The transmembrane regions of the proteins typically exhibited the highest confidence score, while some other protein portions appeared unstructured (Figure 5A). Regions that are likely located within a membrane were validated by inspecting the molecular lipophilicity potential of the protein surfaces (Figure 5B). A clear hydrophobic belt was observed for all proteins and their orientation in the membrane was determined on the basis of orthologous proteins. As transport cavities with negatively charged residues are a hallmark of heavy metal ion transporters, we analyzed the distribution of charge on the surface of the proteins and looked for negatively charged regions to assess the capacity to bind cations like Fe2+ (Figure 5C). To gain further insights into the functions of the proteins identified, we also compared the predicted structures with those of well-characterized homologs from S. cerevisiae, Eucalyptus grandis, Bordetella bronchiseptica and Staphylococcus capitis (Table 2; Supplementary Figures S3, S4).
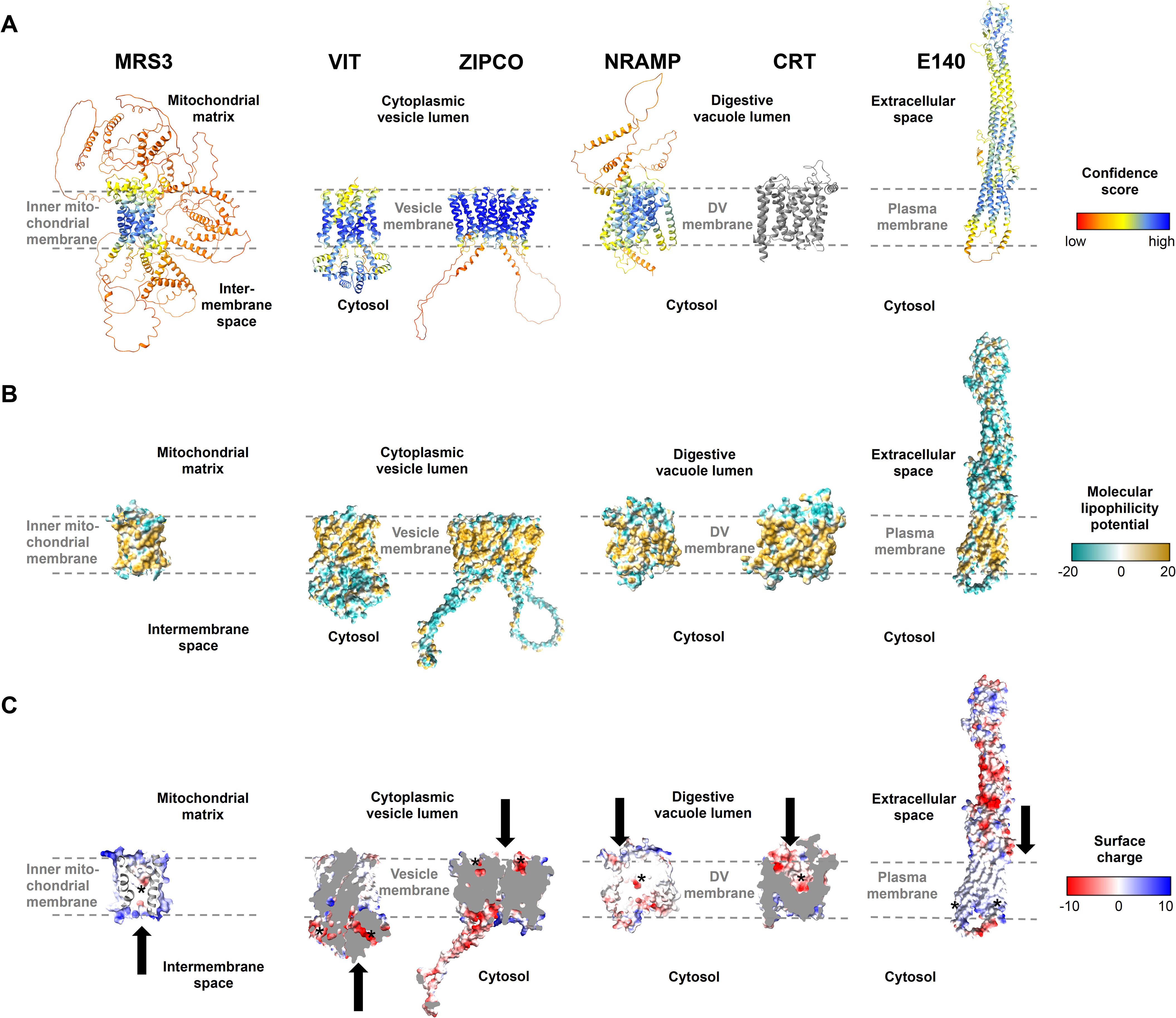
Figure 5. Structures of known and putative P. falciparum iron transporters as viewed from the membrane plane. (A) Predicted protein structures with per-residue pLDDT (predicted local distance difference test) confidence scores on a scale from 0 to 100, where blue represents high and red low confidence, respectively. The experimentally determined structure of PfCRT is shown in gray. (B) Molecular lipophilicity potential of the protein surfaces as implemented in UCSF ChimeraX; tan is hydrophobic and cyan hydrophilic. Dashed lines above and below the tan regions of all proteins indicate the respective membrane and disordered loops were removed for clarity. (C) Surface charge of the proteins with positively charged areas colored blue and negatively charged ones red. Putative cation-binding site are indicated with an asterisk and transport directions by arrows. PfE140 likely forms a dimer but is shown as a monomer, as no predicted dimer structure could be obtained using AlphaFold2-multimer. The putative cation-binding sites for this protein are based on DeepFRI gradCAM scores for the functional term GO:0015075 “monoatomic ion transmembrane transporter activity” (Supplementary Figure S5).
The outer surface of PfMRS3 (transport classification (TC): 2.A.29, mitochondrial carrier family) is positively charged (Figure 5C) and there is a clear negatively charged patch in the putative binding pocket facing the mitochondrial intermembrane space. We compared the predicted PfMRS3 structure with that of S. cerevisiae MRS3, which is known to import ferrous iron into the mitochondrial matrix across the inner membrane (Mühlenhoff et al., 2003; Froschauer et al., 2009; Brazzolotto et al., 2014). The predicted structures of PfMRS3 and S. cerevisiae MRS3 were superimposed with an average root mean square deviation of Cα atoms (Cα RMSD) of the 205 matched residues of 2.3 Å (Supplementary Figures S3A, S4A). The conserved histidine residues His48 and His105 that were required for Fe2+ transport by S. cerevisiae MRS3 in reconstituted liposomes (Brazzolotto et al., 2014) are also present in PfMRS3 and are in a similar molecular context in both structures (Supplementary Figures S3A, S4A). This suggests that MRS3 may elicit similar molecular functions in S. cerevisiae and P. falciparum.
PfVIT is highly similar to VIT1 from E. grandis (E = 7 x 10-27, 30.3% identity, 84% coverage), for which an experimental structure is available (Protein Data Bank (PDB) identifier: 6IU4). The plant protein crystallized as a homodimer (Kato et al., 2019), and the same oligomeric state was suggested for the vacuolar iron transporter family (TC: 2.A.89) protein in P. falciparum (Sharma et al., 2021). A PfVIT monomer also has five transmembrane domains and comprises a negatively charged region facing the cytosol that may enable cation transport (Figure 5C). In agreement with this, one Fe2+ ion and two Zn2+ ions were bound by a strongly charged region on the cytosolic side of the E. grandis VIT1 monomer (Kato et al., 2019) and a highly similar putative binding pocket is present in the parasite protein (Supplementary Figure S4B). In the structural alignment, 219 of the 227 residues of the experimental EgVIT123-249 structure are within 5 Å of the predicted structure of PfVIT with an average Cα RMSD of 1.9 Å and the key residues in the metal-binding domain (Glu102, Glu105, Glu113, Glu116, using EgVIT123-249 numbering) are placed in a similar molecular context in the predicted structure of PfVIT (Supplementary Figures S3B, S4B). The residues in the transmembrane domain that are in the vicinity of the Co2+ ion in the EgVIT123-249 structure (Met80 and Asp43) are also conserved (Supplementary Figure S3B), which is in line with a similar function of PfVIT and EgVIT1.
PfZIPCO contains seven transmembrane domains and was modeled as a homodimer (Figure 5A), as it is part of the zinc (Zn2+)-iron (Fe2+) permease (ZIP) family (TC: 2.A.5), whose members usually function as homo- or heterodimers (Saier et al., 2016). The negatively charged patch in each binding pocket facing the vesicle lumen (Figure 5C) may be involved in cation transport to the cytosolic side. In an overlay of the PfZIPCO model with the cryo-EM structure (PDB: 8GHT) of a ZIP transporter from B. bronchiseptica in the presence of either Zn2+ or Cd2+ ions (Pang et al., 2023), the average Cα RMSD of 140 sequence-aligned residues was 2.0 Å (Supplementary Figures S3C, S4C). Several key residues of the metal binding site M1 of BbZIP (Met99, His177, Glu181, Glu211) were also found in PfZIPCO, whereas others (Asn178, Gln207, Asp208, Glu240) were different (Supplementary Figure S3C), possibly resulting in divergent substrate specificity.
PfNRAMP (TC: 2.A.55, metal ion (Mn2+-iron) transporter family) is a homolog of the human endosomal Fe2+ transporter 2/DMT1 (Martin et al., 2005) and contains twelve transmembrane domains (Figure 5A). Like PfCRT (TC 2.A.7, drug/metabolite exporter family), for which a recent cryo-EM structure (PDB: 6UKJ) is available (Kim et al., 2019), the predicted structure possesses a negatively charged region within its binding pocket facing the digestive vacuolar lumen (Figure 5C). This is consistent with binding of cations such as Fe2+. The PfNRAMP model was superimposed on the solved crystal structure of S. capitis NRAMP/DMT (PDB: 5M95, E = 1 x 10-32, 26.3% identity, 60% coverage), which was shown to bind Mn2+, Fe2+, Co2+, Ni2+, Cd2+ and Pb2+ (Ehrnstorfer et al., 2014). In the overlay, the average Cα RMSD of the 349 matched residues was 1.6 Å and the negatively charged cavity of PfNRAMP was in close proximity to the Mn2+ ion bound to S. capitis NRAMP (Supplementary Figure S3D, Supplementary Figure S4D). Two of the four key residues required for ion coordination in the binding pocket of the bacterial protein (Asn52 and Asp49) are present in PfNRAMP, whereas the two other residues (Met226 and Ala223) are changed to serine. The functional implications of the latter are unclear; Ehrnstorfer et al (Ehrnstorfer et al., 2014) showed that Met226Ala mutant of S.capitis NRAMP exhibited reduced transport activity and binding, however, transport could not be completely abolished by these mutations. While potential effects of these differences on substrate specificity and/or transport activity remain to be elucidated, PfNRAMP is likely to perform cation transport from the DV into the cytosol.
PfE140 is predicted to be anchored in the parasite plasma membrane by a bundle of five transmembrane domains (Meerstein-Kessel et al., 2021). In the AlphaFold prediction, it forms a coiled coil with a hydrophilic region that displays negatively charged patches exposed to the extracellular side (Figures 5A, B). No human orthologs could be identified for this highly conserved Plasmodium protein (Altschul et al., 1997). As there is no obvious channel or cavity in the transmembrane region of the PfE140 monomer (Figures 5B, C), the helical bundles may form a dimer to enable ion transport. However, we were not able to obtain a PfE140 dimer model with AlphaFold2-multimer because of its sequence length. To predict functional residues on the basis of the amino acid sequence and the AlphaFold2 structure of PfE140, we used DeepFRI graph convolutional network (Gligorijević et al., 2021), which has significant denoising capability and can reliably assign GO terms to residues in the protein. In particular, the terms GO:0022857 “transmembrane transporter activity” (DeepFRI gradCAM score 0.94), GO:0015075 “monoatomic ion transmembrane transporter activity” (score 0.78), and GO:0046873 “metal ion transmembrane transporter activity” (score 0.67) were assigned to a putative transmembrane region of PfE140 with high confidence (Supplementary Figure S5). We thus speculate that the protein is a transporter of metal ions.
4 Discussion
Here, we studied the role of iron in growth and transcription of P. falciparum by using blood from individuals of different iron status and by adding hepcidin as an iron-regulatory hormone and ferroportin inhibitor. Overall, our data demonstrate the importance of Fe2+ in parasite replication and development and highlight areas for further study. We showed that in vitro growth rates of P. falciparum 3D7 and the number of merozoites formed per schizont were reduced within erythrocytes that contain lower concentrations of labile iron, while culturing in blood from an individual with higher iron status did not lead to a significant increase in labile iron levels within erythrocytes or in parasite growth relative to control (Figure 1). Consistent with this, reduced propagation of P. falciparum 3D7, Dd2, and FCR3-FMG was reported when erythrocyte samples from iron-deficient individuals used for parasite culture (Clark et al., 2014; Goheen et al., 2017). This effect was eliminated after these donors were iron-supplemented, whereas supplementation of healthy (iron-replete) donors did not significantly promote parasite growth (Clark et al., 2014). The strong increase in parasite replication in the presence of hepcidin relative to control conditions (Figure 1B) may have been a result of enhanced invasion efficiency in addition to the increased number of merozoites formed (Figure 1D). Earlier studies also found that higher hepcidin levels in blood samples were associated with elevated P. falciparum growth rates in vitro (Goheen et al., 2017) and severe malaria in vivo (Muriuki et al., 2021), however, the effect of experimental hepcidin addition on parasite growth had not been assessed previously.
To identify putative iron transporters and iron-regulated processes, we carried out RNA-sequencing analyses of P. falciparum during the ring and trophozoite stages cultured under the different iron conditions described above. A higher number of biological processes and pathways were significantly enriched among DEGs when erythrocytes from donors with different iron status were used for parasite culture (Figure 2C) compared to red blood cells from the same healthy donor in the presence vs. absence of hepcidin (total of 28 vs. 13 functional terms, Figure 2E). This may reflect greater differences in the culture conditions; for instance, blood from the donor with high serum ferritin and Hb levels may have also contained more glucose or copper (Newhouse et al., 1993; Kim et al., 2011), potentially explaining the more diverse physiological response of the parasite. Including erythrocyte samples from more individuals in the growth experiments and RNA-sequencing analysis would have provided further insights, however, the provision of sufficient blood from iron-deficient donors is limited by ethical constraints.
The availability of additional nutrients likely resulted in increased endocytosis and digestion of host cell contents in the DV of the parasite, leading to enhanced metabolism, mRNA splicing, and protein production. Interestingly, the terms KEGG:01100 “metabolic pathways” and GO:0005737 “cytoplasm” were also found to be enriched in upregulated parasite genes in children with high vs. low parasitemia (Milner et al., 2012; Thomson-Luque et al., 2021). RNA binding and mRNA splicing processes were previously reported to be overrepresented in upregulated genes in severe malaria linked to high parasite density (Milner et al., 2012; Lee et al., 2018; Thomson-Luque et al., 2021). Hence, an increase in overall parasite fitness under high vs. low-iron conditions may explain the increase in parasite multiplication (Figure 1B) and could be associated with higher parasitemia and disease severity. Consistent with the observed upregulation of transmembrane transporters at 6 – 9 hpi under high vs. low-iron conditions, Mancio-Silva et al. found that the functional term “ion transporter activity” was enriched in P. berghei genes that were downregulated under caloric restriction at 6 and 10 hpi (Mancio-Silva et al., 2017). Thus, transmembrane transporter genes may need to be transcribed at the beginning of the IDC to ensure that the appropriate level of transport proteins is available for nutrient acquisition and metabolite efflux during the subsequent metabolically active trophozoite and schizont stages.
Hepcidin plays a central role in mammalian iron homeostasis and reduces serum iron concentrations (Muckenthaler et al., 2017). It is also known that hepcidin levels are elevated in P. falciparum-infected individuals, especially those with high parasitemia (Cercamondi et al., 2010; Muriuki et al., 2021), and that malaria causes iron deficiency (Muriuki et al., 2021). The transcription profile of parasites treated with 0.7 µM hepcidin showed similarities to those cultured in erythrocytes from the iron-deficient donor compared to standard conditions in terms of downregulated catabolic and translation processes as well as transport protein regulation (Figure 2; Table 1). This may be related to the fact that an aberrant hepcidin increase causes systemic iron deficiency as a result of restricted iron availability (Ginzburg, 2019). The upregulation of genes involved in merozoite motility (PfMTIP, PfGAP45, and various inner membrane complex proteins) and host cell entry (such as PfAMA1, PfMSP3, PfMSP7, and PfEBA181) when hepcidin was present (Figure 2) may suggest an improved ability of the released merozoites to invade erythrocytes. Thus, the addition of the peptide hormone to the culture media could be a signal for the parasite to reduce metabolic processes and to increase its invasion efficiency.
In addition to roles in parasite proliferation and development, different levels of labile iron may induce regulatory processes at various levels. Under high-iron conditions, the observed upregulation of histone deacetylation (Figure 2C) may lead to the condensation and thus deactivation of certain chromatin regions (Duraisingh and Skillman, 2018). The binding sites and target genes of the differentially expressed transcription factors and of PfIRP remain to be identified in P. falciparum. Moreover, protein phosphorylation may play a role in iron-dependent regulatory mechanisms. As a serine/threonine kinase (KIN) serves as a nutrient sensor in P. berghei, driving a fast response that leads to increased parasite multiplication and virulence (Mancio-Silva et al., 2017), a similar kinase may sense iron and lead to increased replication in P. falciparum.
On the basis of our RNA-sequencing results (Figure 2, Table 1) and the P. falciparum transporter list (Wunderlich, 2022), we identified six proteins that are likely involved in iron transport in the parasite (Table 2; Figure 6) and analyzed their subcellular localization (Figure 3), their importance for growth (Figure 4), and their predicted structures (Figure 5). PfMRS3 transcription was upregulated at the ring stage under high vs. low-iron conditions (log2 FC = 0.33, P = 0.002, Figure 2B), and fluorescence of the GFP-tagged protein was exclusively detected at the mitochondrion (Figure 3A). As a disruption of the gene was reported to fail (Zhang et al., 2018), and parental DNA of the original gene locus was still present in the GFP reporter line (Supplementary Figure S2), PfMRS3 is likely essential for asexual growth like PBANKA_041620 (E = 1 x 10-69, 71.4% identity, 25% coverage) in P. berghei (Bushell et al., 2017). A knockdown of the gene could not be evaluated because we were not able to select stable integrants with G418 after transient transfection of the glmS construct upon four independent attempts. Thus, the parasite may not tolerate an insertion of the glmS sequence into its genome, as this can already lead to a slight gene knockdown even without glucosamine addition to the culture media. The orthologous mitochondrial iron transporter (TgMIT, TGME49_277090, E = 7 x 10-19, 26.0% identity, 28% coverage) also localized to the mitochondrion in T. gondii and was upregulated at the protein level upon iron overload in consequence of a TgVIT knock out in the related apicomplexan parasite (Aghabi et al., 2023). Our structural analyses (Figure 5; Supplementary Figures S3A, S4A) further support that PfMRS3 imports ferrous iron into the mitochondrion, the main iron user of the cell, thereby reducing the cytosolic Fe2+ concentration (Figure 6) as a means of detoxification, which has been reported for yeast (Li et al., 2010). The protein’s substrate specificity as well as its iron- binding and transport activities remain to be confirmed experimentally.
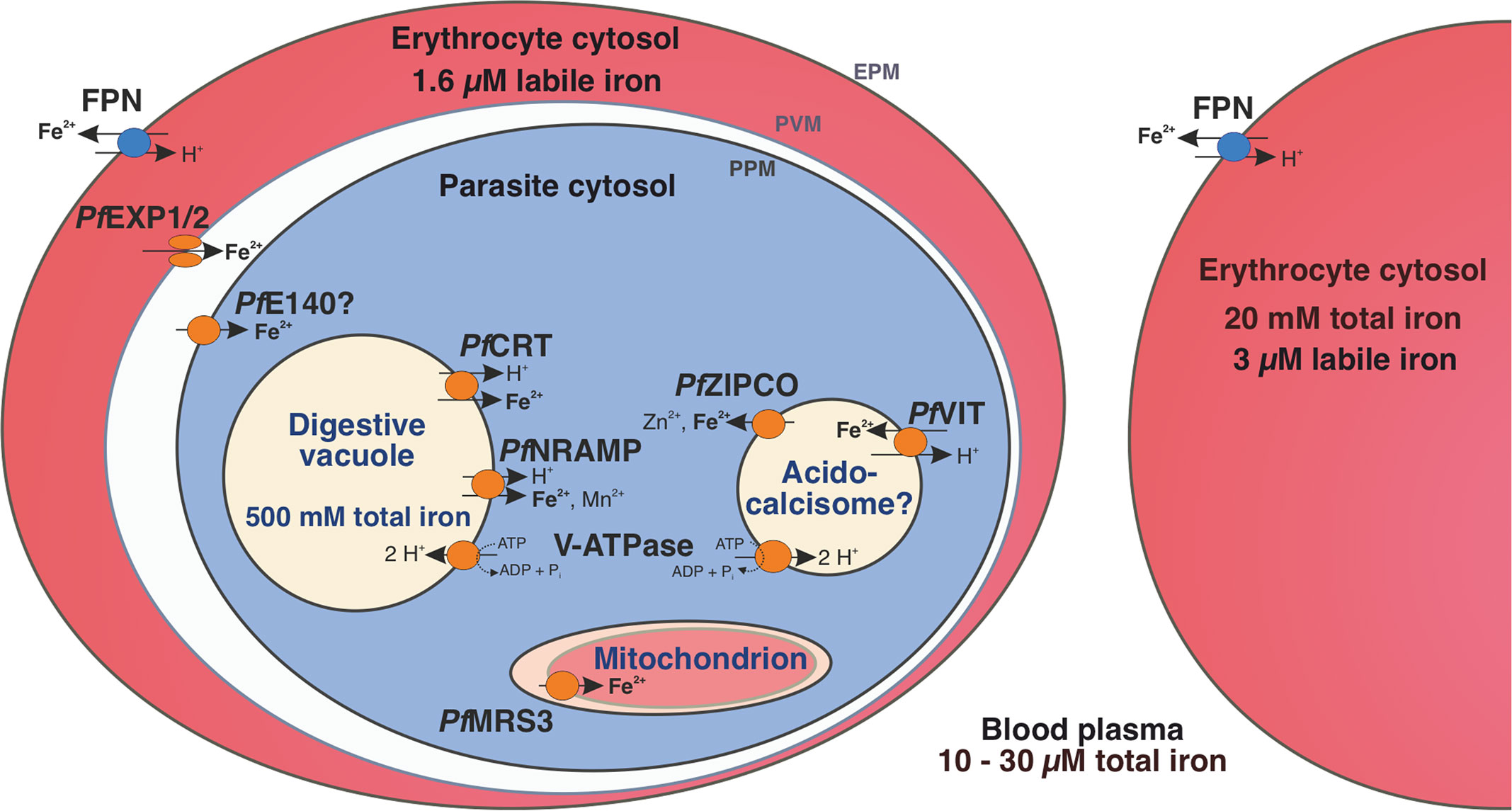
Figure 6. Iron homeostasis in a P. falciparum-infected erythrocyte. The human blood plasma contains between 10 and 30 µM total iron and the erythrocyte cytosol approximately 20 mM (Egan et al., 2002). However, the labile iron pool is only 3 µM in an uninfected erythrocyte and 1.6 µM in a P. falciparum-infected one (Loyevsky et al., 1999). Human ferroportin (FPN) at the host cell surface (erythrocyte plasma membrane, EPM) exports ferrous iron from the erythrocyte (Ward and Kaplan, 2012) and the nutrient pore formed by PfEXP1 and PfEXP2 allows the passage of ions through the parasitophorous vacuole membrane (PVM) (Mesén-Ramírez et al., 2021). PfE140 at the parasite plasma membrane (PPM) may mediate iron uptake into the parasite cytosol and the mitochondrial carrier protein PfMRS3 likely translocates Fe2+ into the mitochondrion, a site of de novo heme biosynthesis (this study). We propose that the vacuolar iron transporter (PfVIT) is involved in iron detoxification by transporting excess Fe2+ from the cytosol into cytoplasmic vesicles that may be acidocalcisomes, whereas PfZIPCO releases Fe2+ from these organelles under low-iron conditions. The digestive vacuole (DV) contains a high amount of total iron as it is the site of hemoglobin degradation and hemozoin formation (Becker et al., 2004). The chloroquine resistance transporter (PfCRT) and the natural resistance-associated macrophage protein (PfNRAMP, also called PfDMT1 for divalent metal transporter 1) were suggested to mediate proton-coupled export of Fe2+ from the DV into the parasite cytosol (Martin et al., 2005; Bakouh et al., 2017). Both acidocalcisomes and the DV are likely acidified by the plant-like H+-pump V-ATPase, which can fuel secondary active transport processes (Wunderlich et al., 2012; de Oliveira et al., 2021). Parasite-encoded proteins are shown in orange and human-encoded transporters in blue.
Complementation assays in S. cerevisiae indicated a role for PfVIT in iron detoxification (Slavic et al., 2016; Sharma et al., 2021) and we observed that the expression of the gene was upregulated under high vs. low-iron conditions in P. falciparum (log2 FC = 0.29, P = 0.02, Figure 2B). The fluorescence pattern of PfVIT-GFP in live cells (Figure 3B) was consistent with cytoplasmic vesicles that may be acidocalcisomes, as described for T. brucei VIT1 (Huang et al., 2014). An increase in the number of fluorescent punctate structures during parasite development (Figure 3B) was also observed for VIT in T. gondii (Aghabi et al., 2023). PfVIT shares 47.0% identity with TgVIT (E = 8 x 10-84, 95% coverage) and 36.9% identity with TbVIT1 (E = 9 x 10-39, 98% coverage). In contrast, P. berghei VIT (PBANKA_143860, E = 3 x 10-160, 79.3% identity, 98% coverage) was shown to localize to the ER in indirect immunofluorescence assays (Slavic et al., 2016). This may be explained by differences between species or variation in methodology such as fixation, permeabilization, and immunolabeling techniques as opposed to live-cell imaging (Schembri et al., 2007; Schnell et al., 2012; Mathew et al., 2021).
Transport assays using inverted vesicles that were prepared using recombinant PfVIT expressed in E. coli demonstrated that the protein is a Fe2+/H+ antiporter (Labarbuta et al., 2017). The translocation of Fe2+ in exchange for H+ is likely fueled by the pH gradient across the membrane of the acidic vesicles and the high similarity of the putative Fe2+-binding pocket at the cytosolic side of the predicted PfVIT structure with that of experimentally characterized EgVIT1 (Figure 5; Supplementary Figure S4B) provide further evidence for our hypothesis. While not essential during asexual blood stages (Zhang et al., 2018), a knockout of VIT resulted in reduced liver stage development in P. berghei (Slavic et al., 2016) and increased sensitivity to high iron levels in both P. berghei (Slavic et al., 2016) and T. gondii (Aghabi et al., 2023). Similarly, growth of the ΔVIT P. falciparum line was not affected under standard conditions, whereas the addition of hepcidin – which increases intracellular labile iron levels (Figure 1A) – compromised parasite proliferation in our study (Figure 4B). Thus, we hypothesize that the transporter sequesters Fe2+ into cytoplasmic vesicles, which is important for iron detoxification under high-iron conditions. While ΔVIT P. falciparum is more sensitive to elevated intracellular Fe2+ concentrations (Figure 4B) as a consequence of impaired removal of excess iron from the cytosol, PfMRS3 may compensate for a loss of PfVIT under standard conditions by transporting ferrous iron from the cytosol into the mitochondrion (Figure 6).
In contrast to the PPM staining of P. berghei sporozoites in immunofluorescence assays, PfZIPCO-GFP expression resulted in a punctate fluorescence pattern in the cytoplasm of live blood-stage P. falciparum (Figure 3C), similar to that of PfVIT-GFP (Figure 3B). Whereas the PfZIPCO knockout caused a growth increase under standard conditions (Figure 4B), ΔZIPCO P. berghei parasites displayed normal blood-stage development but impaired sporozoite infectivity as well as reduced replication at the liver stage in mice (Sahu et al., 2014). Interestingly, the ortholog TGME49_225530 is also dispensable in T. gondii tachyzoites with a phenotype score of −2.94 (values below -1.5 are considered non-essential (Sidik et al., 2016)). Hence, Fe2+ efflux from cytoplasmic vesicles (potentially acidocalcisomes) into the cytosol via PfZIPCO may be dispensable in P. falciparum under iron-replete conditions during the blood stage because of the redundancy with iron import mechanisms into the parasite, and the production of the protein may result in a fitness cost. In contrast, liver-stage parasites in low-iron environments may rely on the transporter’s activity when the demand for iron is high during schizogony. As the transcription of PfZIPCO was upregulated at low vs. control iron levels (log2 FC = 0.55, P = 0.04, Table 1) and in response to hepcidin treatment (log2 FC = 0.63, P = 0.006, Figure 2D; Table 1), the transport protein may release Fe2+ and Zn2+ ions from intracellular stores, in this case cytoplasmic vesicles (Figure 6), in case of scarcity, thereby increasing cytosolic ion levels like other ZIP transporters (Sloan et al., 2021). While our analyses of the predicted structure and its alignment with BbZIP indicate that PfZIPCO likely has the capacity to bind and transport cations like Fe2+ or Zn2+ (Figure 5; Supplementary Figures S3C, S4C), its substrate specificity can only be conclusively established by characterizing the purified protein. Liposomal assays with the putative zinc transporter PfZIP1 (PF3D7_0609100, 24.5% identity with PfZIPCO, E = 1 x 10-19, 78% coverage), which localized to the plasma membrane in schizonts, demonstrated that this ZIP transporter preferentially binds Zn2+ over Fe2+ (Shrivastava et al., 2024). Interestingly, this preference was abolished if the histidine-rich loop at the C-terminus of PfZIP1, which is not present in PfZIPCO, was truncated. As mRNA levels of PfZIP1 were enhanced at low cytosolic Zn2+ levels (Shrivastava et al., 2024) but not differentially regulated under various iron conditions (Supplementary Tables S1, S2), it may play a role in zinc rather than iron homeostasis under physiological conditions.
As the highest intracellular iron concentration in P. falciparum is reached within the DV (Becker et al., 2004; Rohrbach et al., 2005), the free form of the metal may need to be exported from this compartment under high-iron conditions to prevent damage to the DV membrane (Figure 6). This function may be fulfilled by PfCRT (Bakouh et al., 2017) and/or PfNRAMP (Martin et al., 2005), which were both upregulated under high vs. low-iron conditions in our RNA-sequencing analysis (log2 FC = 0.26, P = 0.007 and log2 FC = 0.28, P = 0.003, respectively, Figure 2B, Table 1) and are essential in asexual parasites (Waller et al., 2003; Zhang et al., 2018; Wichers et al., 2022). The predicted structure of PfNRAMP (Figure 5) reflects the state that is open towards the cytosol as in the crystal structure of NRAMP from Deinococcus radiodurans (Bozzi et al., 2016). While a negatively charged cavity inside the protein is clearly visible in the PfNRAMP model, the proposed outward-facing permeation pathway for metal ions is likely occluded in this conformation (Figure 5C). It is conceivable that Fe2+ ions permeate through this pathway from the DV lumen and bind to the charged cavity like the Mn2+ ion to S. capitis NRAMP/DMT (Supplementary Figure S4D). PfNRAMP might function similarly to its ortholog in D. radiodurans, which was shown to mediate pH-dependent transport of Fe2+ and Mn2+ in symport with H+ using uptake assays in E. coli, HEK293T cells, and proteoliposomes (Bozzi et al., 2016; Bozzi et al., 2019).
Expression of the surface protein PfE140 was upregulated when iron levels were low compared to standard conditions (log2 FC = 0.65, P = 0.0006, Table 1) and the GFP fusion protein localized to the PPM only, as evidenced by the fluorescent edges of free merozoites (Figure 3D). This observation is consistent with the fact that the extracellular portions of this protein are highly polymorphic because of their exposure to the immune system at the sporozoite stage (Meerstein-Kessel et al., 2021). Interestingly, vaccines targeting PyE140 in Plasmodium yoelii were reported to induce up to 100% sterile protection mediated by antibodies in mice (Smith et al., 2020). The reduced parasite replication rate upon its conditional knockdown demonstrates the importance of PfE140 for parasite growth and the rescue of the PfE140 knockdown by hepcidin treatment support a role of this putative transporter in iron uptake (Figure 4F). Its predicted essential nature (Zhang et al., 2018), in addition to the absence of orthologs in humans, make it an excellent drug target candidate. While our P. falciparum gene expression data (Figures 2B, D) point towards a role of PfE140 in iron homeostasis, its precise function is still unclear and it remains to be clarified whether the large coiled-coil domain exposed to the extracellular space (Figure 5) can mediate dimerization upon substrate binding. Given our experimental results and the functional annotations (Figure 5C; Supplementary Figure S5), we hypothesize that PfE140 is a plasma membrane transporter for inorganic cations such as metal ions.
In conclusion, this is the first study to investigate P. falciparum transcriptomics under different iron conditions and to determine the subcellular localization of the known and putative iron transport proteins PfMRS3, PfVIT, PfZIPCO and PfE140 as well as the growth effects of a PfVIT or PfZIPCO knockout and an inducible PfE140 knockdown. Our results reveal how the human malaria parasite reacts to alterations in host iron status and provide new insights into the mechanisms of iron transport in P. falciparum in addition to offering avenues for the development of novel therapeutic strategies against malaria. We propose a model for the regulation of iron homeostasis in the P. falciparum-infected erythrocyte with a series of six organelle-specific iron transport proteins in the parasite (Figure 6): One route of iron uptake into the parasite is through the release of Fe2+ upon hemoglobin digestion in the DV and the efflux of the ion into the cytosol mediated by PfNRAMP (Loveridge and Sigala, 2024) and/or PfCRT (Bakouh et al., 2017). Ferrous iron likely also enters the parasite cytosol across the PPM via PfE140 and this pathway may be particularly important during schizogony, when the putative transporter gene is abundantly transcribed and new merozoites without a DV are formed (Broadbent et al., 2015). Once inside the cytosol, iron concentrations need to be tightly regulated to avoid toxicity, which could be achieved by Fe2+ import into the mitochondrion as the main site of iron utilization via PfMRS3 and through transport into (PfVIT) and out of (PfZIPCO) cytoplasmic vesicles functioning as labile iron pools. To confirm the hypotheses of our exploratory study, transport assays with purified proteins like those performed with recombinant PfVIT (Labarbuta et al., 2017) are required for the formal demonstration of substrate specificities and activities of the other transporters in addition to further functional characterization of the proteins during the mosquito, liver and asexual blood stages of the parasite. As no ortholog of the essential PfE140 and only a distant homolog of the non-redundant mitochondrial iron importer PfMRS3 (Mather et al., 2007) are present in humans, these provide candidate targets for urgently needed new antimalarial drugs. Furthermore, dissecting how P. falciparum senses changes in micronutrient availability in its environment and how it modulates its virulence accordingly is an area of considerable interest for future investigation, as iron is an essential regulatory signal for virulence factors in many pathogens.
Data availability statement
The full RNA-sequencing datasets generated and analyzed in this study are available in the BioStudies repository (89) under accession number E-MTAB-13411: https://www.ebi.ac.uk/biostudies/studies/E-MTAB-13411. The code and data used for parasite age estimation can be accessed at Zenodo with the record ID 7996302: https://zenodo.org/record/7996302.
Ethics statement
Ethical approval was not required for the studies involving humans because the erythrocytes used in this study were residuals from voluntary blood donations at the University Medical Center Hamburg-Eppendorf. All donors provided written informed consent that their blood donation could be used for research purposes. The studies were conducted in accordance with the local legislation and institutional requirements. The human samples used in this study were acquired from a by-product of routine care or industry. Written informed consent to participate in this study was not required from the participants or the participants’ legal guardians/next of kin in accordance with the national legislation and the institutional requirements.
Author contributions
JW: Conceptualization, Data curation, Formal Analysis, Investigation, Methodology, Project administration, Software, Validation, Visualization, Writing – original draft, Writing – review & editing. VK: Formal Analysis, Software, Visualization, Writing – original draft, Writing – review & editing, Data curation, Investigation, Methodology, Validation. LV-N: Software, Visualization, Writing – review & editing, Formal Analysis. CN: Investigation, Writing – original draft. MG: Resources, Project administration, Writing – original draft. SPe: Project administration, Resources, Writing – original draft. SPo: Funding acquisition, Resources, Supervision, Writing – review & editing. JS: Conceptualization, Formal Analysis, Funding acquisition, Methodology, Project administration, Software, Visualization, Writing – original draft, Writing – review & editing, Data curation, Validation.
Funding
The author(s) declare that financial support was received for the research, authorship, and/or publication of this article. This work was supported by a Boehringer Ingelheim Foundation Exploration Grant, the Partnership for Innovation, Education and Research (PIER) of Hamburg University and DESY (project PIF-2018-87) and the European Molecular Biology Laboratory (EMBL). JW was additionally funded by the European Research Council (ERC) under the European Union’s Horizon 2020 Research and Innovation Program (grant agreement 759534) and VK by a research fellowship from the EMBL Interdisciplinary Postdoc (EIPOD) Program under Marie Curie Cofund Actions MSCA-COFUND-FP (grant agreement 847543). The funders had no role in study design, data collection and analysis, decision to publish, or preparation of the manuscript.
Acknowledgments
The authors thank the Genomics Core Facility at EMBL Heidelberg, especially Vladimir Benes, for the RNA-sequencing service, and EMBL Hamburg for the provision of research and technical support as well as access to research infrastructures. Grzegorz Chojnowski and the group of Jan Kosinski at EMBL Hamburg enabled the AlphaFold2 workflow at the EMBL Hamburg computer cluster. The Advanced Light and Fluorescence Microscopy Facility at CSSB Hamburg, in particular Roland Thünauer, supported microscopy experiments and the Bernhard Nocht Institute for Tropical Medicine (BNITM) provided lab space. We gratefully acknowledge Tobias Spielmann for pSLI-GFP and pARL-PfACP (1–60)-mCherry, Paul Burda for pSLI-GFP-glmS, Jacobus Pharmaceuticals for WR99210, Anna Bachmann and Mayka Sánchez for helpful advice, Eileen Devaney, Katharina Jungnickel, and Samuel Pažický for critical reading of the manuscript, and Heidrun von Thien, Yannick Höppner, and Gabriela Guédez for technical assistance. A preprint of this article is available on bioRxiv at https://doi.org/10.1101/2024.04.18.590068 (Wunderlich et al., 2024).
Conflict of interest
The authors declare that the research was conducted in the absence of any commercial or financial relationships that could be construed as a potential conflict of interest.
Publisher’s note
All claims expressed in this article are solely those of the authors and do not necessarily represent those of their affiliated organizations, or those of the publisher, the editors and the reviewers. Any product that may be evaluated in this article, or claim that may be made by its manufacturer, is not guaranteed or endorsed by the publisher.
Supplementary material
The Supplementary Material for this article can be found online at: https://www.frontiersin.org/articles/10.3389/fcimb.2024.1480076/full#supplementary-material
References
Abrahamian, M., Ah-Fong, A. M., Davis, C., Andreeva, K., Judelson, H. S. (2016). Gene expression and silencing studies in Phytophthora infestans reveal infection-specific nutrient transporters and a role for the nitrate reductase pathway in plant pathogenesis. PloS Pathog. 12, e1006097. doi: 10.1371/journal.ppat.1006097
Aghabi, D., Sloan, M., Gill, G., Hartmann, E., Antipova, O., Dou, Z., et al. (2023). The vacuolar iron transporter mediates iron detoxification in Toxoplasma gondii. Nat. Commun. 14, 3659. doi: 10.1038/s41467-023-39436-y
Agrawal, S., Striepen, B. (2010). More membranes, more proteins: complex protein import mechanisms into secondary plastids. Protist. 161, 672–687. doi: 10.1016/j.protis.2010.09.002
Ahiya, A. I., Bhatnagar, S., Morrisey, J. M., Beck, J. R., Vaidya, A. B. (2022). Dramatic consequences of reducing erythrocyte membrane cholesterol on Plasmodium falciparum. Microbiol. Spectr. 10, e0015822. doi: 10.1128/spectrum.00158-22
Altschul, S. F., Madden, T. L., Schäffer, A. A., Zhang, J., Zhang, Z., Miller, W., et al. (1997). Gapped BLAST and PSI-BLAST: a new generation of protein database search programs. Nucleic Acids Res. 25, 3389–3402. doi: 10.1093/nar/25.17.3389
Andrews, S. (2010). FastQC: a quality control tool for high throughput sequence data (Cambridge, UK: Babraham Bioinformatics, Babraham Institute).
Aschemeyer, S., Qiao, B., Stefanova, D., Valore, E. V., Sek, A. C., Ruwe, T. A., et al. (2018). Structure-function analysis of ferroportin defines the binding site and an alternative mechanism of action of hepcidin. Blood. 131, 899–910. doi: 10.1182/blood-2017-05-786590
Aurrecoechea, C., Brestelli, J., Brunk, B. P., Dommer, J., Fischer, S., Gajria, B., et al. (2009). PlasmoDB: a functional genomic database for malaria parasites. Nucleic Acids Res. 37, D539–DD43. doi: 10.1093/nar/gkn814
Bakouh, N., Bellanca, S., Nyboer, B., Moliner Cubel, S., Karim, Z., Sanchez, C. P., et al. (2017). Iron is a substrate of the Plasmodium falciparum chloroquine resistance transporter PfCRT in Xenopus oocytes. J. Biol. Chem. 292, 16109–16121. doi: 10.1074/jbc.M117.805200
Becker, K., Tilley, L., Vennerstrom, J. L., Roberts, D., Rogerson, S., Ginsburg, H. (2004). Oxidative stress in malaria parasite-infected erythrocytes: host–parasite interactions. Int. J. Parasitol. 34, 163–189. doi: 10.1016/j.ijpara.2003.09.011
Benjamini, Y., Hochberg, Y. (1995). Controlling the false discovery rate: a practical and powerful approach to multiple Testing. J. R. Stat. Soc. Ser. B. 57, 289–300. doi: 10.1111/j.2517-6161.1995.tb02031.x
Billesbølle, C. B., Azumaya, C. M., Kretsch, R. C., Powers, A. S., Gonen, S., Schneider, S., et al. (2020). Structure of hepcidin-bound ferroportin reveals iron homeostatic mechanisms. Nature. 586, 807–811. doi: 10.1038/s41586-020-2668-z
Birnbaum, J., Flemming, S., Reichard, N., Soares, A. B., Mesén-Ramírez, P., Jonscher, E., et al. (2017). A genetic system to study Plasmodium falciparum protein function. Nat. Methods 14, 450–456. doi: 10.1038/nmeth.4223
Birnbaum, J., Scharf, S., Schmidt, S., Jonscher, E., Hoeijmakers, W. A. M., Flemming, S., et al. (2020). A Kelch13-defined endocytosis pathway mediates artemisinin resistance in malaria parasites. Science. 367, 51–59. doi: 10.1126/science.aax4735
Blighe, K., Rana, S., Lewis, M. (2022). EnhancedVolcano: Publication-ready volcano plots with enhanced colouring and labeling. R Package version. 1, 15. doi: 10.18129/B9.bioc.EnhancedVolcano
Blume, M., Hliscs, M., Rodriguez-Contreras, D., Sanchez, M., Landfear, S., Lucius, R., et al. (2011). A constitutive pan-hexose permease for the Plasmodium life cycle and transgenic models for screening of antimalarial sugar analogs. FASEB J. 25, 1218–1229. doi: 10.1096/fj.10-173278
Boucher, M. J., Ghosh, S., Zhang, L., Lal, A., Jang, S. W., Ju, A., et al. (2018). Integrative proteomics and bioinformatic prediction enable a high-confidence apicoplast proteome in malaria parasites. PloS Biol. 16, e2005895. doi: 10.1371/journal.pbio.2005895
Bozdech, Z., Llinás, M., Pulliam, B. L., Wong, E. D., Zhu, J., DeRisi, J. L. (2003). The transcriptome of the intraerythrocytic developmental cycle of Plasmodium falciparum. PloS Biol. 1, E5. doi: 10.1371/journal.pbio.0000005
Bozzi, A. T., Bane, L. B., Weihofen, W. A., Singharoy, A., Guillen, E. R., Ploegh, H. L., et al. (2016). Crystal structure and conformational change mechanism of a bacterial Nramp-family divalent metal transporter. Structure. 24, 2102–2114. doi: 10.1016/j.str.2016.09.017
Bozzi, A. T., Bane, L. B., Zimanyi, C. M., Gaudet, R. (2019). Unique structural features in an Nramp metal transporter impart substrate-specific proton cotransport and a kinetic bias to favor import. J. Gen. Physiol. 151, 1413–1429. doi: 10.1085/jgp.201912428
Brabin, L., Roberts, S. A., Tinto, H., Gies, S., Diallo, S., Brabin, B. (2020). Iron status of Burkinabé adolescent girls predicts malaria risk in the following rainy season. Nutrients. 12 (5), 1446. doi: 10.3390/nu12051446
Brancucci, N. M. B., Gerdt, J. P., Wang, C., De Niz, M., Philip, N., Adapa, S. R., et al. (2017). Lysophosphatidylcholine regulates sexual stage differentiation in the human malaria parasite Plasmodium falciparum. Cell 171, 1532–44.e15. doi: 10.1016/j.cell.2017.10.020
Brazzolotto, X., Pierrel, F., Pelosi, L. (2014). Three conserved histidine residues contribute to mitochondrial iron transport through mitoferrins. Biochem. J. 460, 79–89. doi: 10.1042/BJ20140107
Broadbent, K. M., Broadbent, J. C., Ribacke, U., Wirth, D., Rinn, J. L., Sabeti, P. C. (2015). Strand-specific RNA sequencing in Plasmodium falciparum malaria identifies developmentally regulated long non-coding RNA and circular RNA. BMC Genomics 16, 454. doi: 10.1186/s12864-015-1603-4
Brown, R. A. M., Richardson, K. L., Kabir, T. D., Trinder, D., Ganss, R., Leedman, P. J. (2020). Altered iron metabolism and impact in cancer biology, metastasis, and immunology. Front. Oncol. 10, 476. doi: 10.3389/fonc.2020.00476
Burda, P. C., Crosskey, T., Lauk, K., Zurborg, A., Söhnchen, C., Liffner, B., et al. (2020). Structure-based identification and functional characterization of a lipocalin in the malaria parasite Plasmodium falciparum. Cell Rep. 31, 107817. doi: 10.1016/j.celrep.2020.107817
Bushell, E., Gomes, A. R., Sanderson, T., Anar, B., Girling, G., Herd, C., et al. (2017). Functional profiling of a Plasmodium genome reveals an abundance of essential genes. Cell. 170, 260–72.e8. doi: 10.1016/j.cell.2017.06.030
Carter, N. S., Ben Mamoun, C., Liu, W., Silva, E. O., Landfear, S. M., Goldberg, D. E., et al. (2000). Isolation and functional characterization of the PfNT1 nucleoside transporter gene from Plasmodium falciparum. J. Biol. Chem. 275, 10683–10691. doi: 10.1074/jbc.275.14.10683
Cercamondi, C. I., Egli, I. M., Ahouandjinou, E., Dossa, R., Zeder, C., Salami, L., et al. (2010). Afebrile Plasmodium falciparum parasitemia decreases absorption of fortification iron but does not affect systemic iron utilization: a double stable-isotope study in young Beninese women. Am. J. Clin. Nutr. 92, 1385–1392. doi: 10.3945/ajcn.2010.30051
Chitale, M., Hawkins, T., Park, C., Kihara, D. (2009). ESG: extended similarity group method for automated protein function prediction. Bioinformatics. 25, 1739–1745. doi: 10.1093/bioinformatics/btp309
Chou, E. S., Abidi, S. Z., Teye, M., Leliwa-Sytek, A., Rask, T. S., Cobbold, S. A., et al. (2018). A high parasite density environment induces transcriptional changes and cell death in Plasmodium falciparum blood stages. FEBS J. 285, 848–870. doi: 10.1111/febs.2018.285.issue-5
Clark, M. A., Goheen, M. M., Fulford, A., Prentice, A. M., Elnagheeb, M. A., Patel, J., et al. (2014). Host iron status and iron supplementation mediate susceptibility to erythrocytic stage Plasmodium falciparum. Nat. Commun. 5, 4446. doi: 10.1038/ncomms5446
Cowell, A. N., Istvan, E. S., Lukens, A. K., Gomez-Lorenzo, M. G., Vanaerschot, M., Sakata-Kato, T., et al. (2018). Mapping the malaria parasite druggable genome by using in vitro evolution and chemogenomics. Science. 359, 191–199. doi: 10.1126/science.aan4472
Crameri, F., Shephard, G. E., Heron, P. J. (2020). The misuse of color in science communication. Nat. Commun. 11, 5444. doi: 10.1038/s41467-020-19160-7
de Oliveira, L. S., Alborghetti, M. R., Carneiro, R. G., Bastos, I. M. D., Amino, R., Grellier, P., et al. (2021). Calcium in the backstage of malaria parasite biology. Front. Cell Infect. Microbiol. 11, 708834. doi: 10.3389/fcimb.2021.708834
Dobin, A., Davis, C. A., Schlesinger, F., Drenkow, J., Zaleski, C., Jha, S., et al. (2013). STAR: ultrafast universal RNA-seq aligner. Bioinformatics. 29, 15–21. doi: 10.1093/bioinformatics/bts635
Drakesmith, H., Prentice, A. (2008). Viral infection and iron metabolism. Nat. Rev. Microbiol. 6, 541–552. doi: 10.1038/nrmicro1930
Duraisingh, M. T., Skillman, K. M. (2018). Epigenetic variation and regulation in malaria parasites. Annu. Rev. Microbiol. 72, 355–375. doi: 10.1146/annurev-micro-090817-062722
Ecker, A., Lakshmanan, V., Sinnis, P., Coppens, I., Fidock, D. A. (2011). Evidence that mutant PfCRT facilitates the transmission to mosquitoes of chloroquine-treated Plasmodium gametocytes. J. Infect. Dis. 203, 228–236. doi: 10.1093/infdis/jiq036
Edayé, S., Georges, E. (2015). Characterization of native PfABCG protein in Plasmodium falciparum. Biochem. Pharmacol. 97, 137–146. doi: 10.1016/j.bcp.2015.06.035
Egan, T. J., Combrinck, J. M., Egan, J., Hearne, G. R., Marques, H. M., Ntenteni, S., et al. (2002). Fate of haem iron in the malaria parasite Plasmodium falciparum. Biochem. J. 365, 343–347. doi: 10.1042/bj20020793
Ehrnstorfer, I. A., Geertsma, E. R., Pardon, E., Steyaert, J., Dutzler, R. (2014). Crystal structure of a SLC11 (NRAMP) transporter reveals the basis for transition-metal ion transport. Nat. Struct. Mol. Biol. 21, 990–996. doi: 10.1038/nsmb.2904
Evans, R., O’Neill, M., Pritzel, A., Antropova, N., Senior, A., Green, T., et al. (2022). Protein complex prediction with AlphaFold-Multimer. bioRxiv. doi: 10.1101/2021.10.04.463034
Ewels, P., Magnusson, M., Lundin, S., Käller, M. (2016). MultiQC: summarize analysis results for multiple tools and samples in a single report. Bioinformatics. 32, 3047–3048. doi: 10.1093/bioinformatics/btw354
Friedrich, O., Reiling, S. J., Wunderlich, J., Rohrbach, P. (2014). Assessment of Plasmodium falciparum PfMDR1 transport rates using Fluo-4. J. Cell Mol. Med. 18, 1851–1862. doi: 10.1111/jcmm.2014.18.issue-9
Froschauer, E. M., Schweyen, R. J., Wiesenberger, G. (2009). The yeast mitochondrial carrier proteins Mrs3p/Mrs4p mediate iron transport across the inner mitochondrial membrane. Biochim. Biophys. Acta 1788, 1044–1050. doi: 10.1016/j.bbamem.2009.03.004
Gardner, M. J., Hall, N., Fung, E., White, O., Berriman, M., Hyman, R. W., et al. (2002). Genome sequence of the human malaria parasite Plasmodium falciparum. Nature 419, 498–511. doi: 10.1038/nature01097
Ginzburg, Y. Z. (2019). Hepcidin-ferroportin axis in health and disease. Vitam Horm. 110, 17–45. doi: 10.1016/bs.vh.2019.01.002
Gligorijević, V., Renfrew, P. D., Kosciolek, T., Leman, J. K., Berenberg, D., Vatanen, T., et al. (2021). Structure-based protein function prediction using graph convolutional networks. Nat. Commun. 12, 3168. doi: 10.1038/s41467-021-23303-9
Goddard, T. D., Huang, C. C., Meng, E. C., Pettersen, E. F., Couch, G. S., Morris, J. H., et al. (2018). UCSF ChimeraX: Meeting modern challenges in visualization and analysis. Protein Sci. 27, 14–25. doi: 10.1002/pro.v27.1
Goheen, M. M., Bah, A., Wegmüller, R., Verhoef, H., Darboe, B., Danso, E., et al. (2017). Host iron status and erythropoietic response to iron supplementation determines susceptibility to the RBC stage of falciparum malaria during pregnancy. Sci. Rep. 7, 17674. doi: 10.1038/s41598-017-16896-z
Gupta, Y., Sharma, N., Singh, S., Romero, J. G., Rajendran, V., Mogire, R. M., et al. (2022). The multistage antimalarial compound calxinin perturbates P. falciparum Ca2+ homeostasis by targeting a unique ion channel. Pharmaceutics. 14, 1371. doi: 10.3390/pharmaceutics14071371
Gwamaka, M., Kurtis, J. D., Sorensen, B. E., Holte, S., Morrison, R., Mutabingwa, T. K., et al. (2012). Iron deficiency protects against severe Plasmodium falciparum malaria and death in young children. Clin. Infect. Dis. 54, 1137–1144. doi: 10.1093/cid/cis010
Hayashi, M., Yamada, H., Mitamura, T., Horii, T., Yamamoto, A., Moriyama, Y. (2000). Vacuolar H+-ATPase localized in plasma membranes of malaria parasite cells, Plasmodium falciparum, is involved in regional acidification of parasitized erythrocytes. J. Biol. Chem. 275, 34353–34358. doi: 10.1074/jbc.M003323200
Hodges, M., Yikilmaz, E., Patterson, G., Kasvosve, I., Rouault, T. A., Gordeuk, V. R., et al. (2005). An iron regulatory-like protein expressed in Plasmodium falciparum displays aconitase activity. Mol. Biochem. Parasitol. 143, 29–38. doi: 10.1016/j.molbiopara.2005.05.004
Huang, G., Ulrich, P. N., Storey, M., Johnson, D., Tischer, J., Tovar, J. A., et al. (2014). Proteomic analysis of the acidocalcisome, an organelle conserved from bacteria to human cells. PloS Pathog. 10, e1004555. doi: 10.1371/journal.ppat.1004555
Jonker, J. W., Buitelaar, M., Wagenaar, E., van der Valk, M. A., Scheffer, G. L., Scheper, R. J., et al. (2002). The breast cancer resistance protein protects against a major chlorophyll-derived dietary phototoxin and protoporphyria. Proc. Natl. Acad. Sci. U S A. 99, 15649–15654. doi: 10.1073/pnas.202607599
Jumper, J., Evans, R., Pritzel, A., Green, T., Figurnov, M., Ronneberger, O., et al. (2021). Highly accurate protein structure prediction with AlphaFold. Nature. 596, 583–589. doi: 10.1038/s41586-021-03819-2
Kang, D., Kirienko, N. V. (2018). Interdependence between iron acquisition and biofilm formation in Pseudomonas aeruginosa. J. Microbiol. 56, 449–457. doi: 10.1007/s12275-018-8114-3
Kato, T., Kumazaki, K., Wada, M., Taniguchi, R., Nakane, T., Yamashita, K., et al. (2019). Crystal structure of plant vacuolar iron transporter VIT1. Nat. Plants. 5, 308–315. doi: 10.1038/s41477-019-0367-2
Kenthirapalan, S., Waters, A. P., Matuschewski, K., Kooij, T. W. (2014). Copper-transporting ATPase is important for malaria parasite fertility. Mol. Microbiol. 91, 315–325. doi: 10.1111/mmi.2014.91.issue-2
Kidgell, C., Volkman, S. K., Daily, J., Borevitz, J. O., Plouffe, D., Zhou, Y., et al. (2006). A systematic map of genetic variation in Plasmodium falciparum. PloS Pathog. 2, e57. doi: 10.1371/journal.ppat.0020057
Kim, C. H., Kim, H. K., Bae, S. J., Park, J. Y., Lee, K. U. (2011). Association of elevated serum ferritin concentration with insulin resistance and impaired glucose metabolism in Korean men and women. Metabolism. 60, 414–420. doi: 10.1016/j.metabol.2010.03.007
Kim, J., Tan, Y. Z., Wicht, K. J., Erramilli, S. K., Dhingra, S. K., Okombo, J., et al. (2019). Structure and drug resistance of the Plasmodium falciparum transporter PfCRT. Nature. 576, 315–320. doi: 10.1038/s41586-019-1795-x
Kloehn, J., Harding, C. R., Soldati-Favre, D. (2021). Supply and demand - heme synthesis, salvage and utilization by Apicomplexa. FEBS J. 288 (2), 382–404. doi: 10.1111/febs.15445
Knovich, M. A., Storey, J. A., Coffman, L. G., Torti, S. V., Torti, F. M. (2009). Ferritin for the clinician. Blood Rev. 23, 95–104. doi: 10.1016/j.blre.2008.08.001
Kuhn, Y., Sanchez, C. P., Ayoub, D., Saridaki, T., Van Dorsselaer, A., Lanzer, M. (2010). Trafficking of the phosphoprotein PfCRT to the digestive vacuolar membrane in Plasmodium falciparum. Traffic 11, 236–249. doi: 10.1111/j.1600-0854.2009.01018.x
Küpper, H., Kochian, L. V. (2010). Transcriptional regulation of metal transport genes and mineral nutrition during acclimatization to cadmium and zinc in the Cd/Zn hyperaccumulator, Thlaspi caerulescens (Ganges population). New Phytol. 185, 114–129. doi: 10.1111/j.1469-8137.2009.03051.x
Labarbuta, P., Duckett, K., Botting, C. H., Chahrour, O., Malone, J., Dalton, J. P., et al. (2017). Recombinant vacuolar iron transporter family homologue PfVIT from human malaria-causing Plasmodium falciparum is a Fe2+/H+exchanger. Sci. Rep. 7, 42850. doi: 10.1038/srep42850
Lambros, C., Vanderberg, J. P. (1979). Synchronization of Plasmodium falciparum erythrocytic stages in culture. J. Parasitol. 65, 418–420. doi: 10.2307/3280287
Lee, H. J., Georgiadou, A., Walther, M., Nwakanma, D., Stewart, L. B., Levin, M., et al. (2018). Integrated pathogen load and dual transcriptome analysis of systemic host-pathogen interactions in severe malaria. Sci. Transl. Med. 10 (447), eaar3619. doi: 10.1126/scitranslmed.aar3619
Lemieux, J. E., Gomez-Escobar, N., Feller, A., Carret, C., Amambua-Ngwa, A., Pinches, R., et al. (2009). Statistical estimation of cell-cycle progression and lineage commitment in Plasmodium falciparum reveals a homogeneous pattern of transcription in ex vivo culture. Proc. Natl. Acad. Sci. U S A. 106, 7559–7564. doi: 10.1073/pnas.0811829106
Lerch, A., Koepfli, C., Hofmann, N. E., Messerli, C., Wilcox, S., Kattenberg, J. H., et al. (2017). Development of amplicon deep sequencing markers and data analysis pipeline for genotyping multi-clonal malaria infections. BMC Genomics 18, 864. doi: 10.1186/s12864-017-4260-y
Li, H., Handsaker, B., Wysoker, A., Fennell, T., Ruan, J., Homer, N., et al. (2009). The sequence alignment/map format and SAMtools. Bioinformatics. 25, 2078–2079. doi: 10.1093/bioinformatics/btp352
Li, L., Murdock, G., Bagley, D., Jia, X., Ward, D. M., Kaplan, J. (2010). Genetic dissection of a mitochondria-vacuole signaling pathway in yeast reveals a link between chronic oxidative stress and vacuolar iron transport. J. Biol. Chem. 285, 10232–10242. doi: 10.1074/jbc.M109.096859
Liao, Y., Smyth, G. K., Shi, W. (2013). The Subread aligner: fast, accurate and scalable read mapping by seed-and-vote. Nucleic Acids Res. 41, e108. doi: 10.1093/nar/gkt214
Liao, Y., Smyth, G. K., Shi, W. (2014). featureCounts: an efficient general purpose program for assigning sequence reads to genomic features. Bioinformatics. 30, 923–930. doi: 10.1093/bioinformatics/btt656
Lim, L., Linka, M., Mullin, K. A., Weber, A. P., McFadden, G. I. (2010). The carbon and energy sources of the non-photosynthetic plastid in the malaria parasite. FEBS Lett. 584, 549–554. doi: 10.1016/j.febslet.2009.11.097
Loveridge, K. M., Sigala, P. A. (2024). Unraveling mechanisms of iron acquisition in malaria parasites. bioRxiv. doi: 10.1101/2024.05.10.587216
Loyevsky, M., John, C., Dickens, B., Hu, V., Miller, J. H., Gordeuk, V. R. (1999). Chelation of iron within the erythrocytic Plasmodium falciparum parasite by iron chelators. Mol. Biochem. Parasitol. 101, 43–59. doi: 10.1016/S0166-6851(99)00053-5
Loyevsky, M., LaVaute, T., Allerson, C. R., Stearman, R., Kassim, O. O., Cooperman, S., et al. (2001). An IRP-like protein from Plasmodium falciparum binds to a mammalian iron-responsive element. Blood. 98, 2555–2562. doi: 10.1182/blood.V98.8.2555
Mach, J., Sutak, R. (2020). Iron in parasitic protists – from uptake to storage and where we can interfere. Metallomics. 12, 1335–1347. doi: 10.1039/d0mt00125b
Magowan, C., Brown, J. T., Liang, J., Heck, J., Coppel, R. L., Mohandas, N., et al. (1997). Intracellular structures of normal and aberrant Plasmodium falciparum malaria parasites imaged by soft x-ray microscopy. Proc. Natl. Acad. Sci. U S A. 94, 6222–6227. doi: 10.1073/pnas.94.12.6222
Malleret, B., Claser, C., Ong, A. S., Suwanarusk, R., Sriprawat, K., Howland, S. W., et al. (2011). A rapid and robust tri-color flow cytometry assay for monitoring malaria parasite development. Sci. Rep. 1, 118. doi: 10.1038/srep00118
Mancio-Silva, L., Slavic, K., Grilo Ruivo, M. T., Grosso, A. R., Modrzynska, K. K., Vera, I. M., et al. (2017). Nutrient sensing modulates malaria parasite virulence. Nature. 547, 213–216. doi: 10.1038/nature23009
Marapana, D. S., Dagley, L. F., Sandow, J. J., Nebl, T., Triglia, T., Pasternak, M., et al. (2018). Plasmepsin V cleaves malaria effector proteins in a distinct endoplasmic reticulum translocation interactome for export to the erythrocyte. Nat. Microbiol. 3, 1010–1022. doi: 10.1038/s41564-018-0219-2
Marfil-Rivera, L. J. (2015). Iron overload. Medicina Universitaria. 17, 240–242. doi: 10.1016/j.rmu.2015.08.001
Martin, M. (2011). Cutadapt removes adapter sequences from high-throughput sequencing reads. EMBnet J. 17, 10–12. doi: 10.14806/ej.17.1.200
Martin, R. E. (2020). The transportome of the malaria parasite. Biol. Rev. Camb Philos. Soc 95, 305–332. doi: 10.1111/brv.12565
Martin, R. E., Henry, R. I., Abbey, J. L., Clements, J. D., Kirk, K. (2005). The 'permeome' of the malaria parasite: an overview of the membrane transport proteins of Plasmodium falciparum. Genome Biol. 6, R26. doi: 10.1186/gb-2005-6-3-r26
Mather, M. W., Henry, K. W., Vaidya, A. B. (2007). Mitochondrial drug targets in apicomplexan parasites. Curr. Drug Targets. 8, 49–60. doi: 10.2174/138945007779315632
Mathew, R., Wunderlich, J., Thivierge, K., Cwiklinski, K., Dumont, C., Tilley, L., et al. (2021). Biochemical and cellular characterization of the Plasmodium falciparum M1 alanyl aminopeptidase (PfM1AAP) and M17 leucyl aminopeptidase (PfM17LAP). Sci. Rep. 11, 2854. doi: 10.1038/s41598-021-82499-4
Meerstein-Kessel, L., Venhuizen, J., Garza, D., Proellochs, N. I., Vos, E. J., Obiero, J. M., et al. (2021). Novel insights from the Plasmodium falciparum sporozoite-specific proteome by probabilistic integration of 26 studies. PloS Comput. Biol. 17, e1008067. doi: 10.1371/journal.pcbi.1008067
Meng, E. C., Pettersen, E. F., Couch, G. S., Huang, C. C., Ferrin, T. E. (2006). Tools for integrated sequence-structure analysis with UCSF Chimera. BMC Bioinf. 7, 339. doi: 10.1186/1471-2105-7-339
Mesén-Ramírez, P., Bergmann, B., Elhabiri, M., Zhu, L., von Thien, H., Castro-Peña, C., et al. (2021). The parasitophorous vacuole nutrient channel is critical for drug access in malaria parasites and modulates the artemisinin resistance fitness cost. Cell Host Microbe. 29 (12), 1774–1787.e9. doi: 10.1016/j.chom.2021.11.002
Milner, D. A., Jr., Pochet, N., Krupka, M., Williams, C., Seydel, K., Taylor, T. E., et al. (2012). Transcriptional profiling of Plasmodium falciparum parasites from patients with severe malaria identifies distinct low vs. high parasitemic clusters. PloS One 7, e40739. doi: 10.1371/journal.pone.0040739
Moon, R. W., Hall, J., Rangkuti, F., Ho, Y. S., Almond, N., Mitchell, G. H., et al. (2013). Adaptation of the genetically tractable malaria pathogen Plasmodium knowlesi to continuous culture in human erythrocytes. Proc. Natl. Acad. Sci. U S A. 110, 531–536. doi: 10.1073/pnas.1216457110
Moonah, S., Sanders, N. G., Persichetti, J. K., Sullivan, D. J., Jr. (2014). Erythrocyte lysis and Xenopus laevis oocyte rupture by recombinant Plasmodium falciparum hemolysin III. Eukaryot Cell. 13, 1337–1345. doi: 10.1128/EC.00088-14
Muckenthaler, M. U., Rivella, S., Hentze, M. W., Galy, B. (2017). A red carpet for iron metabolism. Cell. 168, 344–361. doi: 10.1016/j.cell.2016.12.034
Mühlenhoff, U., Stadler, J. A., Richhardt, N., Seubert, A., Eickhorst, T., Schweyen, R. J., et al. (2003). A specific role of the yeast mitochondrial carriers Mrs3/4p in mitochondrial iron acquisition under iron-limiting conditions. J. Biol. Chem. 278, 40612–40620. doi: 10.1074/jbc.M307847200
Muriuki, J. M., Mentzer, A. J., Mitchell, R., Webb, E. L., Etyang, A. O., Kyobutungi, C., et al. (2021). Malaria is a cause of iron deficiency in African children. Nat. Med. 27, 653–658. doi: 10.1038/s41591-021-01238-4
Newhouse, I. J., Clement, D. B., Lai, C. (1993). Effects of iron supplementation and discontinuation on serum copper, zinc, calcium, and magnesium levels in women. Med. Sci. Sports Exerc. 25, 562–571. doi: 10.1249/00005768-199305000-00006
Nozawa, A., Ito, D., Ibrahim, M., Santos, H. J., Tsuboi, T., Tozawa, Y. (2020). Characterization of mitochondrial carrier proteins of malaria parasite Plasmodium falciparum based on in vitro translation and reconstitution. Parasitol. Int. 79, 102160. doi: 10.1016/j.parint.2020.102160
Nyakeriga, A. M., Troye-Blomberg, M., Dorfman, J. R., Alexander, N. D., Bäck, R., Kortok, M., et al. (2004). Iron deficiency and malaria among children living on the coast of Kenya. J. Infect. Dis. 190, 439–447. doi: 10.1086/jid.2004.190.issue-3
Paganini, D., Zimmermann, M. B. (2017). The effects of iron fortification and supplementation on the gut microbiome and diarrhea in infants and children: a review. Am. J. Clin. Nutr. 106, 1688s–1693s. doi: 10.3945/ajcn.117.156067
Pang, C., Chai, J., Zhu, P., Shanklin, J., Liu, Q. (2023). Structural mechanism of intracellular autoregulation of zinc uptake in ZIP transporters. Nat. Commun. 14, 3404. doi: 10.1038/s41467-023-39010-6
Park, D. J., Lukens, A. K., Neafsey, D. E., Schaffner, S. F., Chang, H. H., Valim, C., et al. (2012). Sequence-based association and selection scans identify drug resistance loci in the Plasmodium falciparum malaria parasite. Proc. Natl. Acad. Sci. U S A. 109, 13052–13067. doi: 10.1073/pnas.1210585109
Petrat, F., Rauen, U., de Groot, H. (1999). Determination of the chelatable iron pool of isolated rat hepatocytes by digital fluorescence microscopy using the fluorescent probe, phen green SK. Hepatology. 29, 1171–1179. doi: 10.1002/hep.510290435
Prommana, P., Uthaipibull, C., Wongsombat, C., Kamchonwongpaisan, S., Yuthavong, Y., Knuepfer, E., et al. (2013). Inducible knockdown of Plasmodium gene expression using the glmS ribozyme. PloS One 8, e73783. doi: 10.1371/journal.pone.0073783
Raudvere, U., Kolberg, L., Kuzmin, I., Arak, T., Adler, P., Peterson, H., et al. (2019). g:Profiler: a web server for functional enrichment analysis and conversions of gene lists (2019 update). Nucleic Acids Res. 47, W191–W1W8. doi: 10.1093/nar/gkz369
Rivadeneira, E. M., Wasserman, M., Espinal, C. T. (1983). Separation and concentration of schizonts of Plasmodium falciparum by Percoll gradients. J. Protozool. 30, 367–370. doi: 10.1111/j.1550-7408.1983.tb02932.x
Robinson, M. D., McCarthy, D. J., Smyth, G. K. (2010). edgeR: a Bioconductor package for differential expression analysis of digital gene expression data. Bioinformatics. 26, 139–140. doi: 10.1093/bioinformatics/btp616
Rohrbach, P., Friedrich, O., Hentschel, J., Plattner, H., Fink, R. H., Lanzer, M. (2005). Quantitative calcium measurements in subcellular compartments of Plasmodium falciparum-infected erythrocytes. J. Biol. Chem. 280, 27960–27969. doi: 10.1074/jbc.M500777200
Ruiz, F. A., Luo, S., Moreno, S. N., Docampo, R. (2004). Polyphosphate content and fine structure of acidocalcisomes of Plasmodium falciparum. Microsc Microanal 10, 563–567. doi: 10.1017/S1431927604040875
Sahu, T., Boisson, B., Lacroix, C., Bischoff, E., Richier, Q., Formaglio, P., et al. (2014). ZIPCO, a putative metal ion transporter, is crucial for Plasmodium liver-stage development. EMBO Mol. Med. 6, 1387–1397. doi: 10.15252/emmm.201403868
Saier, M. H., Jr., Reddy, V. S., Tsu, B. V., Ahmed, M. S., Li, C., Moreno-Hagelsieb, G. (2016). The Transporter Classification Database (TCDB): recent advances. Nucleic Acids Res. 44, D372–D3D9. doi: 10.1093/nar/gkv1103
Sanderson, T., Rayner, J. C. (2017). PhenoPlasm: a database of disruption phenotypes for malaria parasite genes. Wellcome Open Res. 2, 45. doi: 10.12688/wellcomeopenres
Sarkans, U., Gostev, M., Athar, A., Behrangi, E., Melnichuk, O., Ali, A., et al. (2018). The BioStudies database - one stop shop for all data supporting a life sciences study. Nucleic Acids Res. 46, D1266–D1D70. doi: 10.1093/nar/gkx965
Sassmannshausen, J., Pradel, G., Bennink, S. (2020). Perforin-like proteins of apicomplexan parasites. Front. Cell Infect. Microbiol. 10, 578883. doi: 10.3389/fcimb.2020.578883
Sayers, C. P., Mollard, V., Buchanan, H. D., McFadden, G. I., Goodman, C. D. (2018). A genetic screen in rodent malaria parasites identifies five new apicoplast putative membrane transporters, one of which is essential in human malaria parasites. Cell Microbiol. 20, e12789. doi: 10.1111/cmi.12789
Schembri, L., Dalibart, R., Tomasello, F., Legembre, P., Ichas, F., De Giorgi, F. (2007). The HA tag is cleaved and loses immunoreactivity during apoptosis. Nat. Methods 4, 107–108. doi: 10.1038/nmeth0207-107
Schindelin, J., Arganda-Carreras, I., Frise, E., Kaynig, V., Longair, M., Pietzsch, T., et al. (2012). Fiji: an open-source platform for biological-image analysis. Nat. Methods 9, 676–682. doi: 10.1038/nmeth.2019
Schmidt, O., Pfanner, N., Meisinger, C. (2010). Mitochondrial protein import: from proteomics to functional mechanisms. Nat. Rev. Mol. Cell Biol. 11, 655–667. doi: 10.1038/nrm2959
Schnell, U., Dijk, F., Sjollema, K. A., Giepmans, B. N. (2012). Immunolabeling artifacts and the need for live-cell imaging. Nat. Methods 9, 152–158. doi: 10.1038/nmeth.1855
Scholl, P. F., Tripathi, A. K., Sullivan, D. J. (2005). Bioavailable iron and heme metabolism in Plasmodium falciparum. Curr. Top. Microbiol. Immunol. 295, 293–324. doi: 10.1007/3-540-29088-5_12
Schureck, M. A., Darling, J. E., Merk, A., Shao, J., Daggupati, G., Srinivasan, P., et al. (2021). Malaria parasites use a soluble RhopH complex for erythrocyte invasion and an integral form for nutrient uptake. eLife. 10, e65282. doi: 10.7554/eLife.65282
Sharma, P., Tóth, V., Hyland, E. M., Law, C. J. (2021). Characterization of the substrate binding site of an iron detoxifying membrane transporter from Plasmodium falciparum. Malar J. 20, 295. doi: 10.1186/s12936-021-03827-7
Sheiner, L., Soldati-Favre, D. (2008). Protein trafficking inside Toxoplasma gondii. Traffic 9, 636–646. doi: 10.1111/j.1600-0854.2008.00713.x
Shrivastava, D., Jha, A., Kabrambam, R., Vishwakarma, J., Mitra, K., Ramachandran, R., et al. (2024). Plasmodium falciparum ZIP1 is a zinc-selective transporter with stage-dependent targeting to the apicoplast and plasma membrane in erythrocytic parasites. ACS Infect. Dis. 10, 155–169. doi: 10.1021/acsinfecdis.3c00426
Sidik, S. M., Huet, D., Ganesan, S. M., Huynh, M. H., Wang, T., Nasamu, A. S., et al. (2016). A genome-wide CRISPR screen in Toxoplasma identifies essential apicomplexan genes. Cell. 166, 1423–35.e12. doi: 10.1016/j.cell.2016.08.019
Sigala, P. A., Goldberg, D. E. (2014). The peculiarities and paradoxes of Plasmodium heme metabolism. Annu. Rev. Microbiol. 68, 259–278. doi: 10.1146/annurev-micro-091313-103537
Slavic, K., Krishna, S., Lahree, A., Bouyer, G., Hanson, K. K., Vera, I., et al. (2016). A vacuolar iron-transporter homologue acts as a detoxifier in Plasmodium. Nat. Commun. 7, 10403. doi: 10.1038/ncomms10403
Sloan, M. A., Aghabi, D., Harding, C. R. (2021). Orchestrating a heist: uptake and storage of metals by apicomplexan parasites. Microbiol. (Reading). 167, 001114. doi: 10.1099/mic.0.001114
Smith, E. C., Limbach, K. J., Rangel, N., Oda, K., Bolton, J. S., Du, M., et al. (2020). Novel malaria antigen Plasmodium yoelii E140 induces antibody-mediated sterile protection in mice against malaria challenge. PloS One 15, e0232234. doi: 10.1371/journal.pone.0232234
Sousa Gerós, A., Simmons, A., Drakesmith, H., Aulicino, A., Frost, J. N. (2020). The battle for iron in enteric infections. Immunology. 161, 186–199. doi: 10.1111/imm.v161.3
Spillman Natalie, J., Allen Richard, J. W., McNamara Case, W., Yeung Bryan, K. S., Winzeler Elizabeth, A., Diagana Thierry, T., et al. (2013). Na+ regulation in the malaria parasite Plasmodium falciparum involves the cation ATPase PfATP4 and is a target of the spiroindolone antimalarials. Cell Host Microbe 13, 227–237. doi: 10.1016/j.chom.2012.12.006
Spork, S., Hiss, J. A., Mandel, K., Sommer, M., Kooij, T. W., Chu, T., et al. (2009). An unusual ERAD-like complex is targeted to the apicoplast of Plasmodium falciparum. Eukaryot Cell 8, 1134–1145. doi: 10.1128/EC.00083-09
Stanway, R. R., Bushell, E., Chiappino-Pepe, A., Roques, M., Sanderson, T., Franke-Fayard, B., et al. (2019). Genome-scale identification of essential metabolic processes for targeting the Plasmodium liver stage. Cell. 179, 1112–28.e26. doi: 10.1016/j.cell.2019.10.030
Stewart, L. B., Diaz-Ingelmo, O., Claessens, A., Abugri, J., Pearson, R. D., Goncalves, S., et al. (2020). Intrinsic multiplication rate variation and plasticity of human blood stage malaria parasites. Commun. Biol. 3, 624. doi: 10.1038/s42003-020-01349-7
Supek, F., Bošnjak, M., Škunca, N., Šmuc, T. (2011). REVIGO summarizes and visualizes long lists of gene ontology terms. PloS One 6, e21800. doi: 10.1371/journal.pone.0021800
Taneri, P. E., Gómez-Ochoa, S. A., Llanaj, E., Raguindin, P. F., Rojas, L. Z., Roa-Díaz, Z. M., et al. (2020). Anemia and iron metabolism in COVID-19: a systematic review and meta-analysis. Eur. J. Epidemiol. 35, 763–773. doi: 10.1007/s10654-020-00678-5
Thipubon, P., Uthaipibull, C., Kamchonwongpaisan, S., Tipsuwan, W., Srichairatanakool, S. (2015). Inhibitory effect of novel iron chelator, 1-(N-acetyl-6-aminohexyl)-3-hydroxy-2-methylpyridin-4-one (CM1) and green tea extract on growth of Plasmodium falciparum. Malaria J. 14, 382. doi: 10.1186/s12936-015-0910-1
Thomson-Luque, R., Votborg-Novél, L., Ndovie, W., Andrade, C. M., Niangaly, M., Attipa, C., et al. (2021). Plasmodium falciparum transcription in different clinical presentations of malaria associates with circulation time of infected erythrocytes. Nat. Commun. 12, 4711. doi: 10.1038/s41467-021-25062-z
Trager, W., Jensen, J. B. (1976). Human malaria parasites in continuous culture. Science. 193, 673–675. doi: 10.1126/science.781840
van Esveld, S. L., Meerstein-Kessel, L., Boshoven, C., Baaij, J. F., Barylyuk, K., Coolen, J. P. M., et al. (2021). A prioritized and validated resource of mitochondrial proteins in Plasmodium identifies unique biology. mSphere. 6, e0061421. doi: 10.1128/mSphere.00614-21
Varadi, M., Anyango, S., Deshpande, M., Nair, S., Natassia, C., Yordanova, G., et al. (2022). AlphaFold Protein Structure Database: massively expanding the structural coverage of protein-sequence space with high-accuracy models. Nucleic Acids Res. 50, D439–DD44. doi: 10.1093/nar/gkab1061
Waller, K. L., Muhle, R. A., Ursos, L. M., Horrocks, P., Verdier-Pinard, D., Sidhu, A. B., et al. (2003). Chloroquine resistance modulated in vitro by expression levels of the Plasmodium falciparum chloroquine resistance transporter. J. Biol. Chem. 278, 33593–33601. doi: 10.1074/jbc.M302215200
Ward, D. M., Kaplan, J. (2012). Ferroportin-mediated iron transport: Expression and regulation. Biochim. Biophys. Acta (BBA) - Mol. Cell Res. 1823, 1426–1433. doi: 10.1016/j.bbamcr.2012.03.004
Wichers, J. S., Mesén-Ramírez, P., Fuchs, G., Yu-Strzelczyk, J., Stäcker, J., von Thien, H., et al. (2022). PMRT1, a Plasmodium-specific parasite plasma membrane transporter, is essential for asexual and sexual blood stage development. mBio. 13, e00623–e00622. doi: 10.1128/mbio.00623-22
Wichers, J. S., Scholz, J. A. M., Strauss, J., Witt, S., Lill, A., Ehnold, L. I., et al. (2019). Dissecting the gene expression, localization, membrane topology, and function of the Plasmodium falciparum STEVOR protein family. mBio 10 (4), e01500-19. doi: 10.1128/mBio.01500-19
Wichers, J. S., van Gelder, C., Fuchs, G., Ruge, J. M., Pietsch, E., Ferreira, J. L., et al. (2021). Characterization of Apicomplexan Amino Acid Transporters (ApiATs) in the malaria parasite Plasmodium falciparum. mSphere 6, e00743–e00721. doi: 10.1128/mSphere.00743-21
Wunderlich, J. (2022). Updated list of transport proteins in Plasmodium falciparum. Front. Cell Infect. Microbiol. 12, 926541. doi: 10.3389/fcimb.2022.926541
Wunderlich, J., Kotov, V., Votborg-Novél, L., Ntalla, C., Geffken, M., Peine, S., et al. (2024). Iron transport pathways in the human malaria parasite Plasmodium falciparum revealed by RNA-sequencing. bioRxiv. doi: 10.1101/2024.04.18.590068
Wunderlich, J., Rohrbach, P., Dalton, J. P. (2012). The malaria digestive vacuole. Front. Biosci. (Schol Ed). 4, 1424–1448. doi: 10.2741/s344
Zhang, L., Hendrickson, R. C., Meikle, V., Lefkowitz, E. J., Ioerger, T. R., Niederweis, M. (2020). Comprehensive analysis of iron utilization by Mycobacterium tuberculosis. PloS Pathog. 16, e1008337. doi: 10.1371/journal.ppat.1008337
Zhang, M., Wang, C., Otto, T. D., Oberstaller, J., Liao, X., Adapa, S. R., et al. (2018). Uncovering the essential genes of the human malaria parasite Plasmodium falciparum by saturation mutagenesis. Science 360, eaap7847. doi: 10.1126/science.aap7847
Keywords: Plasmodium falciparum, malaria, drug target, iron deficiency, transporters, nutrient uptake, gene expression, AlphaFold
Citation: Wunderlich J, Kotov V, Votborg-Novél L, Ntalla C, Geffken M, Peine S, Portugal S and Strauss J (2024) Iron transport pathways in the human malaria parasite Plasmodium falciparum revealed by RNA-sequencing. Front. Cell. Infect. Microbiol. 14:1480076. doi: 10.3389/fcimb.2024.1480076
Received: 13 August 2024; Accepted: 14 October 2024;
Published: 07 November 2024.
Edited by:
Sheila Nardelli, Oswaldo Cruz Foundation, BrazilReviewed by:
Melisa Saye, Instituto de Investigaciones Medicas (IDIM, UBA-CONICET), ArgentinaEstefanía Calvo Alvarez, University of Milan, Italy
Copyright © 2024 Wunderlich, Kotov, Votborg-Novél, Ntalla, Geffken, Peine, Portugal and Strauss. This is an open-access article distributed under the terms of the Creative Commons Attribution License (CC BY). The use, distribution or reproduction in other forums is permitted, provided the original author(s) and the copyright owner(s) are credited and that the original publication in this journal is cited, in accordance with accepted academic practice. No use, distribution or reproduction is permitted which does not comply with these terms.
*Correspondence: Juliane Wunderlich, anVsaWFuZS53dW5kZXJsaWNoQG1haWwubWNnaWxsLmNh
†Present address: Juliane Wunderlich, Robert Koch Institute, Department of Infectious Disease Epidemiology, Berlin, Germany
Jan Strauss, German Maritime Center, Hamburg, Germany