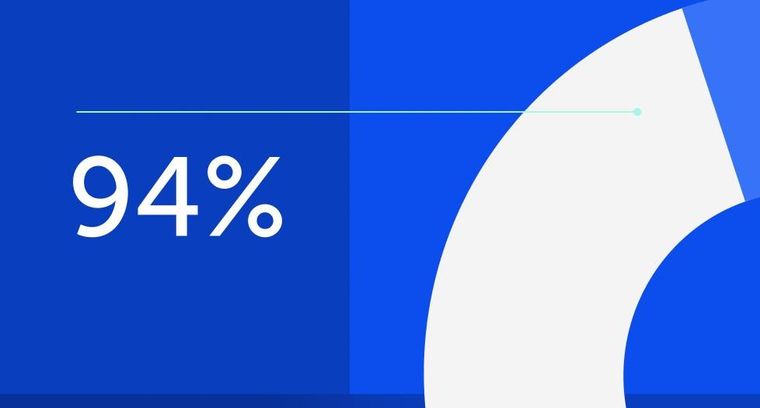
94% of researchers rate our articles as excellent or good
Learn more about the work of our research integrity team to safeguard the quality of each article we publish.
Find out more
REVIEW article
Front. Cell. Infect. Microbiol., 04 July 2024
Sec. Intestinal Microbiome
Volume 14 - 2024 | https://doi.org/10.3389/fcimb.2024.1389925
This article is part of the Research TopicNatural Products and Intestinal HealthView all 5 articles
Gut barrier is not only part of the digestive organ but also an important immunological organ for the hosts. The disruption of gut barrier can lead to various diseases such as obesity and colitis. In recent years, traditional Chinese medicine (TCM) has gained much attention for its rich clinical experiences enriched in thousands of years. After orally taken, TCM can interplay with gut microbiota. On one hand, TCM can modulate the composition and function of gut microbiota. On the other hand, gut microbiota can transform TCM compounds. The gut microbiota metabolites produced during the actions of these interplays exert noticeable pharmacological effects on the host especially gut barrier. Recently, a large number of studies have investigated the repairing and fortifying effects of TCM on gut barriers from the perspective of gut microbiota and its metabolites. However, no review has summarized the mechanism behand this beneficiary effects of TCM. In this review, we first briefly introduce the unique structure and specific function of gut barrier. Then, we summarize the interactions and relationship amidst gut microbiota, gut microbiota metabolites and TCM. Further, we summarize the regulative effects and mechanisms of TCM on gut barrier including physical barrier, chemical barrier, immunological barrier, and microbial barrier. At last, we discuss the effects of TCM on diseases that are associated gut barrier destruction such as ulcerative colitis and type 2 diabetes. Our review can provide insights into TCM, gut barrier and gut microbiota.
Gut barriers are essential defenses in the intestinal tract that provide robust protection against harmful microorganisms, both internal and external. These barriers include physical, chemical, immunological, and microbial components, each with its own distinct structure and function. Dysfunction of the gut barrier has been implicated in various diseases such as irritable bowel syndrome, celiac disease, enteric infections, nonalcoholic fatty liver disease (NAFLD), type 2 diabetes (T2D), insulin resistance (IR), and inflammatory bowel disease (Crohn’s disease and ulcerative colitis) (Turner, 2009; König et al., 2016). Because of the importance of gut barrier in the development of diseases, gut barrier has played increasingly important role in understanding the development of diseases and developing drugs for disease treatment.
Gut microbiota, the largest micro-ecological system in the human body, plays a crucial role in maintaining the normal function of the host (Ma et al., 2019). The gut microbiota is composed of over 1500 species, including bacteria, viruses and other microorganisms, with certain phyla such as Firmicutes, Bacteroides, Actinobacteria, Proteobacteria, and Verrucomicrobia being particularly important (Fava et al., 2019). The composition of the gut microbiota is closely associated with the function of gut barriers and the development of various diseases. A healthy gut microbiota suppresses the growth and translocation of pathogens, strengthens the gut mucosal barrier, and reduces the absorption of toxins (Paolella et al., 2014). It also supports the immune system by enhancing the secretion of antimicrobial factors (Mills et al., 2019). On the contrary, gut microbiota dysbiosis can lead to the development of diseases such as hypertension, gastrointestinal disease, cardiovascular disease, metabolic disease, anxiety, depression and cancer (Afzaal et al., 2022). Consequently, the study of gut microbiota has become an important area of research for understanding disease development and treatment strategies.
In addition to gut microbiota, its metabolites such as short-chain fatty acids (SCFAs), bile acids (BAs), and tryptophan (Trp), also play a crucial role in influencing gut barriers. These metabolites are involved in maintaining the normal function of hosts and the development of diseases. For example, SCFAs are viewed as crucial energy sources and anti-pathogen factors for intestinal epithelial cells (Afzaal et al., 2022). Other than that, SCFAs can upregulate the expressions of zonula occludens 1 (ZO-1), occludin and claudin, which are beneficial for reinforcement of gut physical barrier (Ghosh et al., 2021). Besides, BAs can regulate gut microbial barrier via inducing the transcription of antimicrobial agents such as inducible nitric oxide synthase and interleukin (IL)-18 (Inagaki et al., 2006). Moreover, Trp and its metabolites have been found to be beneficial for strengthening intestinal barrier function by upregulating the expression of the gene concerning mucosal barrier and mucin production (Bansal et al., 2010). Hence, given the importance of gut microbiota metabolites, numerous studies have directed attention to both gut microbiota and its metabolites simultaneously.
In recent years, several evidence has revealed that TCM can directly regulate the structure of gut microbiota and the production of its metabolites, then exerting therapeutic influences (Lyu et al., 2017; Xia et al., 2022). For example, hydroxysafflor yellow A has been demonstrated to alleviate obesity through modulation of the gut microbiota and itsmetabolites. Specifically, supplementation with hydroxysafflor yellow A was found to increase the abundances of Akkermansia, Butyricimonas, Alloprevotella, and Romboutsia, while decreasing the levels of Lachnospiraceae. Concurrently, hydroxysafflor yellow A promoted the production of acetate, propionate, and butyrate (Liu et al., 2018a). TCM-gut microbiota interactions also play a role in repairing and strengthening the gut barrier. Qiwei Baizhu Decoction can increase butyrate levels, aiding in the repair of the gut chemical barrier by enhancing mucin 2, a core component of gut chemical barrier (Etienne-Mesmin et al., 2019). Similarly, the extract of Paeoniae Radix Alba promoted Akkermansia levels in DSS-induced colitis mice, benefiting the gut chemical barrier in colitis (Yan et al., 2022). Thus, studying the gut microbiota and its metabolites is crucial for understanding how TCM impacts the gut barrier and produces therapeutic effects.
Currently, there is a lack of review explaining how the gut barrier can be regulated through the interfaces amidst gut microbiota, its metabolites and TCM. In this review, we discuss the composition and function of gut barrier, and then the interactions amidst gut microbiota, its metabolites and TCM. Then, we unravel how TCM exert protective and therapeutic influences on gut barrier via modulating gut microbiota and its metabolites. We also discuss the role of TCM in some common diseases with destroyed gut barrier via regulating gut microbiota and its metabolites. Our review can provide insights into TCM, gut barrier and gut microbiota, enabling the development of novel strategy to treat diseases by modulating gut barrier.
The gut barrier is a sophisticated system providing two important functions for the survival of the individual. Firstly, it permits nutrient absorption and secondly, it protects the body from the invasion of deleterious microorganisms. Generally, gut barriers comprise four aspects, i.e., physical barrier, chemical barrier, immunological barrier, and microbial barrier (Figure 1).
Figure 1 The composition of gut barrier. AJ, adherens junction; AMP, antimicrobial peptide; DC, dendritic cell; IEC, intestinal epithelial cell; IgA, immunoglobulin A; M1, macrophage 1; M2 macrophage 2; PP, Peyer’s patch; TFF, trefoil factor; TJ, tight junction; ZO, zonula occludens.
The gut physical barrier is composed of intestinal epithelial cells (IECs) and the tight junctions between them. It is composed of a single layer of epithelial cells that are interspersed with specialized differentiated cells, including six distinct cell lineages. Enterocytes, comprising 80–90% of all differentiated cells, are highly efficient in absorbing nutrients and water. Microfold cells (M cells) play a crucial role in sampling the contents of the gut and transporting luminal antigens to the underlying immune cells within the Peyer’s patches (PPs), thus regulating immune responses. Goblet cells are responsible for secreting mucus, which serves to lubricate and protect the intestinal surface against bacteria. Paneth cells produce antimicrobial compounds and provide niche factors for the growth and maintenance of intestinal stem cells. Chemosensory tuft cells mediate immune responses, while enteroendocrine cells produce hormones involved in regulating appetite and insulin release (Beumer and Clevers, 2021). Paneth cells and microfold cells are exclusively present in the small intestine, whereas enterocytes, goblet cells, enteroendocrine cells, and tuft cells are found in both small intestine and colon. These cells form a continuous and polarized layer, effectively acting as the boundary between luminal content and lamina propria (Ghosh et al., 2021).
Gut physical barrier can prevent the luminal content (endotoxin, viruses, food antigens, and toxins) and the invading pathogens from intruding into the underlying tissues and producing adverse effects on the host. However, the barrier formed by IECs can be selectively permeable to substances beneficial to the host, such as sugars, water, amino acids, electrolyte, SCFAs, and other gut microbial metabolites. The robust defensive function of IECs and their maintenance of cellular integrity rely on their architectural advantage: tight junction (TJ), adherence junction, gap junction and desmosomes. Besides, these structures make contributions to directing intracellular transportation and functional substances secretion (antimicrobial peptide (AMP), mucin protein), and more significantly, mediating organelle movements (Natividad and Verdu, 2013).
TJ can effectively modify the ability of IECs to regulate the degree of adhesion between cells, the paracellular and transcellular permeability, as well as gut barrier health (Chiba et al., 2008). TJ structure is composed of transmembrane proteins. They are claudin, occludin, tricellulin and junctional adhesion molecules (JAMs) and intracellular plaque proteins such as zonula occluudens (ZO) and cingulin (Suzuki, 2020). Claudins, a diverse protein family consisting of at least 27 members, exhibit various functions in cellular barriers. Some claudins (claudin-3,4,7 and 14) (Van Itallie and Anderson, 2006) act as barriers for macromolecules and ions, while others, like claudin-2, possess properties that form pores facilitating the passage of ions and water (Lu et al., 2013). Besides, claudin-1 can help to modulate epithelial homeostasis by the regulation of notch-signaling and more importantly it can enhance epithelial proliferation in a notch-dependent manner (Pope et al., 2014).
Occludin, associated with cytosolic plaque proteins, plays a role in providing a scaffold for TJ. The scaffold molecules involved in TJ formation include ZO-1 and cingulin. ZO-1, in particular, is essential for coordinating TJ assembly and cell polarization by connecting transmembrane TJ proteins (such as claudin and JAMs) with components of the cytoskeleton (Ikenouchi et al., 2007). In addition to the role in TJ formation, ZO-1 is associated with epithelial proliferation, repair, and survival. For example, epithelial cells lacking ZO-1 exhibited significant impairments in their ability to efficiently initiate proliferation during wound healing. This impairment is attributed to defects in WNT signaling and the orientation of the mitotic spindle (Kuo et al., 2022).
The mucus layer in the intestinal tract acts as a protective barrier, attaching to the surface of intestinal epithelial cells and safeguarding them against mechanical, chemical, and biological attacks. This mucus layer functions as a chemical barrier and is composed of multiple proteins (mucin 2, chloride channel accessory-1, Fc fragment of IgG binding protein, and zymogen granule protein 16), lipids, ions, and water (constituting approximately 95%) (Suriano et al., 2022). The core of the mucus layer is mucus protein, also called mucin, which forms a tight and protective coating over the intestinal tissue, providing lubrication and safeguarding the cells (Paone and Cani, 2020). The composition and thickness of the mucus layer vary throughout the intestinal tract, giving rise to different physiological functions. In the small intestine, the mucus layer is a single layer characterized by the presence of antimicrobial peptides immunoglobulin A (IgA) and endogenous enzyme inhibitors. It is described as a loosely organized but efficient barrier for epithelial cells (Suriano et al., 2022). In contrast, the mucus layer in the large intestine consists of two distinct layers: the inner and the outer layer (Johansson et al., 2011). The inner mucus layer undergoes continuous replenishment through the production of mucin 2. It is firmly anchored to goblet cells and maintains its attachment to the epithelium, ensuring a persistent barrier. In mice, the inner mucus layer has a pore size 0.5 μm, making it impermeable to bacteria. However, endogenous proteases can convert the inner mucus into the outer mucus layer (Birchenough et al., 2015; Hansson, 2019). The outer mucus layer has a loose and net-like structure with larger pores, allowing for the permeability of bacteria or beads up to 0.5 μm (Paone and Cani, 2020). The mucus layer in the large intestine plays a dual role: it prevents direct contact between luminal bacteria and epithelial cells, while also providing a habitat for commensal bacteria through its favorable ecological niche (Suriano et al., 2022).
The primary component of mucus is the mucin 2, a glycoprotein consisting of approximately 5,200 amino acids and containing around 80% glycans, predominantly O-glycans. These highly glycosylated mucins possess multiple O-glycan epitopes that serve as attachment points for commensal bacteria. Through the action of glycan enzymes, commensal bacteria can cleave and utilize the O-glycans present in mucin as an energy source while preserving the overall structure of mucin 2 (Luis and Hansson, 2023). The biosynthesis, glycosylation, storage, and secretion of mucins are carried out by goblet cells. After secretion, the packed mucins require exposure to increased pH and reduced calcium concentration in order to properly expand. This process involves the participation of bicarbonate ions, which are provided by the cystic fibrosis transmembrane conductance regulator channel. The expansion of the packed mucins results in the formation of a net-like structure that can expand in volume by 100–1000 times, effectively binding water and creating a protective barrier (Paone and Cani, 2020). Mucin 2 is found in both the small and large intestine, where it forms the framework of the mucus layer. Besides, mucin 2 is involved in the clearance of outside pathogens. After being infected with Citrobacter rodentium, a murine pathogen associated with diarrheagenic A/E E. coli, mice lacking mucin 2 (Muc2−/−) showed more severe symptoms compared to wild-type mice. They experienced colon ulceration, overt pathogen and commensal translocation into colonic mucosa and had a high mortality rate of up to 90% (Bergstrom et al., 2010).
The mucus barrier’s ability to operate depends on the specific relationship between the gut microbiota and the mucus layer. In Germ-free rats, it has been observed that the absence of bacteria leads to a thinner or even absent mucus layer in the colon (Szentkuti et al., 1990). Additionally, compared to mice raised conventionally, Germ-free mice were found to have a more permeable inner colonic mucus layer with a lower relative abundance of mucin 2 (Johansson and Hansson, 2012). This highlights the role of bacteria in ensuring the integrity of the mucus barrier. Furthermore, the mucus layer serves as an alternative nutrient source for the gut microbiota, supporting their colonization and persistence in the gut (Schroeder, 2019), which emphasizes the importance of the mucus layer in providing nourishment to the microbiota. In summary, maintaining the integrity of the mucus barrier and promoting the development and survival of the microbiota depend on the interaction between the gut microbiota and the mucus layer. The mucus layer’s health and this interaction are also influenced by the diet. Consuming more fiber encourages the production of gut microbiota metabolites, such as SCFAs (Suriano et al., 2022). Particularly, butyrate can improve the function of the mucus barrier by regulating mucin 2 production and secretion (Etienne-Mesmin et al., 2019). However, diets low in fiber, such as Western diets and high-fat diets, can disrupt the composition of the gut microbiota, reducing its diversity. This disruption weakens the mucus barrier and disturbs immune balance (Statovci et al., 2017; Birchenough et al., 2019). In the absence of dietary fiber, the gut microbiota adapts by relying on host-glycans from mucins as an energy source (Sonnenburg and Sonnenburg, 2014; Earle et al., 2015). Unfortunately, this alteration negatively impacts the integrity of the mucus barrier, may causing it to break down. As a result, the gut becomes more vulnerable to gastrointestinal pathogen infections.
Gut immunological barrier is a complicated mucosal immune system, primarily comprising three lymphoid structures: Peyer’s patches, the lamina propria and the intestinal epithelia cells (Richards et al., 2016). Immune cells, including Paneth cells, intestinal B cells, T cells, and enterocytes, possess the remarkable ability to secrete bactericidal substances (Lehrer, 2004; Tezuka and Ohteki, 2019). These substances, such as sIgA, lysozyme, α-defensin, β-defensin, and C-type lectins (such as Reg3-γ and Reg3-β) (Mukherjee et al., 2009), serve as the first layer of gut immune barrier, contributing significantly to the overall defense mechanisms in the gut. sIgA, a widely distributed antibody, serves dual functions in response to pathogens: pathogen elimination and induction of tolerance. It can eliminate pathogens through immune exclusion, providing nonspecific immunity. Additionally, sIgA possesses neutralizing properties, directly impacting bacterial viability or altering pathogenicity (Li et al., 2020b). For instance, the toxins such as Clostridium difficile toxin A and enteropathogenic E. coli intimin can both be neutralized by galactose residues of sIgA (Perrier et al., 2006). Furthermore, sIgA can regulate T cells by binding to specific receptors, suggesting its potential role in immunoregulation (Diana et al., 2013). α-defensin is the most abundant antimicrobial peptide in the human intestine which can directly kill Gram-negative and Gram-positive bacteria (Bevins and Salzman, 2011). Besides, human α defensin 5 (HD5) can neutralize bacterial endotoxin and exert an important influence on regulating host antimicrobial activities (Bevins and Salzman, 2011). Furthermore, the damaged expression of α-defensin has been revealed to be correlated with disruption of the intestinal homoeostasis, such as increased intestinal permeability, mucosal damage, inflammation and endotoxin, and HD5 treatment can prevent these injuries through suppressing the proinflammatory cytokines such as IL-1β, tumor necrosis factor (TNF)-α, IL-6 and chemokines such as CCL5 and MCP1 (Shukla et al., 2018). The similar function has also been exhibited by C-type lectins and Lysozyme, which have been broadly reviewed (Ganz et al., 2003; Bevins and Salzman, 2011; Vaishnava et al., 2011).
Functioning as the secondary layer of the gut immune barrier, intestinal epithelial cells can directly partake in immune monitoring of the gut. Beyond offering direct defense against opportunistic pathogens, these epithelial cells are also involved in transmitting signals to the mucosal immune system by producing cytokines and chemokines (Kuhn et al., 2014). Located in epithelial cell layer, both innate lymphoid cells and intraepithelial lymphocytes (IELs) can produce cytokines that protect the host. For example, upon activation, IELs will express cytokines like IFN-γ and keratinocyte growth factor, which act to defend the epithelial cells against injury (Shi et al., 2017). Dendritic cells (DCs) possess the ability to collect mucosal antigens by extending their dendrites and transporting them across the barrier to the mucosal-associated lymphoid tissue. Additionally, DCs can directly eliminate pathogens such as Salmonella and E. coli by opening the tight junctions and penetrating into the lumen to phagocytose them (Rescigno et al., 2001). In the gut immunological barrier, other immune cells such as regulatory T cells (Tregs) and T helper (Th) cells are also important. These cells help control inflammation and immunological tolerance in addition to aiding in elimination of pathogens (Shi et al., 2017).
The lamina propria is located in the lower layer of intestinal epithelial cells, and it is the third component of gut immune barrier, fulfilling a crucial role in the initial priming and differentiation of adaptive immune cells. PPs are a key constituent of the lamina propria, with their luminal surface covered by a specialized follicle-associated epithelium (FAE) that includes M cells (Owen and Jones, 1974). In mice, these M cells transport luminal antigens, such as those from bacteria and viruses, into the PPs to support optimal IgA responses (Mörbe et al., 2021). PPs contain mature B cells, and the B cell follicles are covered by the subepithelial dome, which is located under the follicle-associated FAE and contains DCs. B cells and T cells get activated when DCs presenting antigen, which starts an immune response (Li et al., 2020b). The activated B cells in the PPs continuously promote the production of plasma cells, which secrete IgA to protect the intestinal tract (Bemark et al., 2012). Concurrently, T cells can rapidly mount an immune response to stimuli from the intestinal tract, producing IL-17 and IL-22 to modulate inflammation (Muñoz et al., 2009).
The microenvironment created by the gut microbiota and its products is a crucial factor that influences the immunity of the intestinal region. Early in life, the process of beneficial microbes colonizing and growing in mucosal tissues is important. It can actively boost and train the host’s immune system in addition to decreasing the incidence of gastrointestinal infections (Weström et al., 2020). For instance, certain bacterial proteins in the mother’s gut during pregnancy, like metabolites of Escherichia coli HA107, can trigger antibody production in the mother’s bloodstream. These antibodies can cross the placenta and impact the immune system of the newborn, promoting the production of fetal group 3 innate lymphoid cells (ILC3) and F4/80+ CD11c+ mononuclear cells, which are crucial for early postpartum innate immunity (Gomez de Agüero et al., 2016). Another example is the segmented filamentous bacterium, a commensal bacterium that has been shown to induce and stimulate various types of intestinal lymphoid tissues, leading to the production of sIgA (Lécuyer et al., 2014). Importantly, the immune system also plays a role in maintaining the balance of the gut microbiota. For example, mice lacking matrix metalloproteinase 7 (MMP7) have reduced levels of α-defensin, while mice with the human HD5 gene have increased levels. This imbalance affects the composition of bacteria in their intestines, with MMP7-deficient mice having fewer Bacteroidetes and more Firmicutes, and HD5 mice showing the opposite pattern. However, the total amount of bacteria remains unchanged in both types of mice, underscoring the vital role of α-defensin in maintaining microbiota balance (Salzman et al., 2010).
The narrow gut microbial barrier solely consists of bacteria, whilst the generalized one is principally composed of bacteria, viruses, helminths, bacteriophages, fungi, and archaea (Garcia-Bonete et al., 2023). The main focus of this discussion revolves around bacteria within the gut microbiota. They have been extensively studied due to their significant representation (>90%) among the microorganisms in the gut, and their ease of culture and isolation adds to their research accessibility (Garcia-Bonete et al., 2023). Firstly, the adhesion, chimerism, and binding of microorganisms to intestinal mucosa comprise the specific structure of the gut microbial barrier, which can effectively defend against extraneous pathogens through competing for the mucosal colonization sites and nutrient intake (Hynönen and Palva, 2013). Secondly, gut microorganisms show an evident colonization distribution. Viewed as the most important beneficial bacteria in the gut (Turroni et al., 2009), Bifidobacterium and Lactobacillus exhibit long-term gut colonization potential (Xiao et al., 2020). They make up the intestinal membranous microbial community, which can be stirred with mucus, then producing protective effect. Besides, the gut microbial barrier is capable of inhibiting intestinal pathogenic bacteria from overgrowing and translocating through promoting epithelial cell proliferation and differentiation, and activating intestinal mucosal immunity (Purchiaroni et al., 2013), by which severe inflammation responses and oxidative stress can be greatly relieved. Moreover, the bacterial surface layer protein or S layer protein are important to the ability to adhere, aggregate, and regulate T cell immune response and antigen variation, which also impact gut immune function. Lactobacillus surface S protein layer can adhere to the host epithelial cell, and competitively suppress the colonization and invasion of pathogenic microorganisms (Hynönen and Palva, 2013).
It has been unraveled that host mucosal binding protein can be found in hairs of Lactobacillus rhamnosus GG, which may be connected to competitive colonization of mucosa sites (Kankainen et al., 2009). Bifidobacterium bifidum has been proved to greatly suppress the attachment and intrusion of salmonella and E.coli O157:H7 by regulating relative virulence factors (Bayoumi and Griffiths, 2012). Additionally, probiotics can produce organic acid lowering intestinal pH and Eh, assisting to suppress the growth of pathogens and excluding harmful substances (Vollaard and Clasener, 1994). When in intestinal homeostasis or active intestinal inflammation, hypoxia and hypoxia-inducing factors dominate the regulation of normal intestinal metabolism and barrier function (Glover et al., 2016). The consumption of oxygen by probiotics in the gut contributes to maintaining an oxygen-free environment and affecting the growth of bacteria, then regulating the ecological balance of gut microbiota (Glover et al., 2016). Furthermore, studies have shown that Lacotobacillus acidophilus was able to adhere to colorectal cancer-associated cells HT-29 and Caco-2, promoting the expression of ZO-1 and phosphorylation of claudin and occludin, enhancing the assembly of TJ then consolidating gut physical barrier (Montalto et al., 2004; Ashida et al., 2011). Moreover, the protective function of the gut chemical barrier can be indirectly achieved because gut microbiota composition can affect the gut mucosal barrier function, which has been demonstrated by a study (Jakobsson et al., 2015) where two genetically identical mouse colonies, respectively raised into two different rooms with the same facilities, showed discrepancies in gut mucus properties and gut microbiota strains. Interestingly, this caused the ensuing fact that their ilka defensive function of mucus layers differed a lot. Besides, the probiotic community also plays a crucial role in regulating intestinal immunity. the function of sole specific bacteria has been reported such as Bifidobacterium longum BL-10, which can exhibit great immune protective functionality by modulating inflammatory cytokines and TLR4/NF-κB signaling pathways, through which the gut microbiota disorders and immunological injuries caused by lipopolysaccharide (LPS) in mice can be greatly restored (Dong et al., 2022). Moreover, probiotics can exert a modulatory influence on intestinal macrophages and DCs, leading to the altered secretion of anti-inflammatory cytokines, such as IL-10, IL-12, and TGF-β. Furthermore, probiotics have the capacity to regulate the balance between Th17/Tregs and Th1/Th2 cell subsets, as well as promote the levels of immunoglobulins in the intestinal fluid. Collectively, these probiotic-mediated mechanisms serve to boost the overall intestinal immune response (Purchiaroni et al., 2013).
In recent years, the interactions between gut microbiota and TCM has been extensively studied to explore the mechanisms of how diseases can be ameliorated by TCM compounds. In the whole process of transmission in gastrointestinal tract, some TCM molecules will be absorbed in the small intestine, which can act on the immune system by promoting the production and secretion of antimicrobial enzymes and antimicrobial substance to avoid colonization of extraneous and indigenous pathogens (Bevins and Salzman, 2011). Other TCM compounds that are hard to absorb will reach the colon and interact with gut microbiota, the processes of which mainly include: (1) TCM can be metabolized into other type of metabolites with better bioavailability and stronger therapeutic effects on the host, (2) TCM can directly and noticeably alter the composition of gut microbiota community, (3) TCM can modulate the gut microbiota metabolites (Figure 2).
Figure 2 Interactions between gut microbiota and TCM. BAs, bile acids; SCFAs, short chain fatty acids; TCM, Traditional Chinese Medicine; Trp, tryptophan.
After orally taken-in, TCM will not be completely absorbed especially those with low oral bioavailability such as polyphenols, flavonoid, polysaccharide, and saponins (Yue et al., 2017). Thus, they can encounter gut bacteria and interact with each other once arriving at the colon, and these undigested compounds can be transformed into smaller molecules by microbial fermentation. These reactions include hydrolysis, oxidation, decarboxylation, reduction, isomerization, demethylation, deamination, and others (Wilson and Nicholson, 2017). For example, most complex polysaccharides can be translated into SCFAs under the action of carbohydrate enzymes secreted by gut microbiota (El Kaoutari et al., 2013). Another example is polyphenols, which are characterized by low bioavailability. The phenols are converted into molecules with low molecular weight and higher bioavailability, thus they can be absorbed more easily by the host (Aura, 2008). Intriguingly, these metabolites can in turn change the composition of microbiota in a beneficial way (Filosa et al., 2018). To accomplish the reactions above, a battery of enzymes with excellent ability to metabolically activate or inactivate drugs are necessary, such as β-glucuronidases, β-glucosidase, dioxygenase, CoA ligase (Currò, 2018). What is worth noting is that the reactions between TCM compounds and gut microbiota may not be achieved in one step but in succession. To take flavonoid glycosides as an example, they are transformed by two steps: de-glycosylation and de-methylation, and then the ensuing skeleton fission (Selma et al., 2009). Some of the gut microbiota-transformed TCM compounds can undergo enterohepatic circulation, in which these compounds will be irreversibly combined with glucuronic acid by hepatic enzymes such as UDP-glucuronosyltransferase, and then be released into duodenum. Once reaching the intestine, β-glucuronidase from microbiota will disassemble these conjugates, which means the prototype TCM compounds could be produced again and then be utilized by the host repeatedly (Feng et al., 2019). Baicalin can be a standard representative of the compounds discussed above for its performance in enterohepatic circulation (Xing et al., 2005).
TCM has four types of effects on gut microbiota: promotion, inhibition, elimination, and new colonization. Promotion, inhibition, and elimination directly impact gut microbiota abundance, while indirect effects can include all four types (Feng et al., 2019). Through these mechanisms, TCM selectively helps to preserve the balance of gut microbiota composition in the face of pathogen invasion, thereby preventing the establishment of exogenous or endogenous pathogens in both healthy and diseased states. There are numerous TCM with the function of promotion and inhibition (Tables 1, 2). For example, the abundance of the genera Enterorhabdus, Odoribacter, Ruminococcaceae_UCG_014, Ruminococcaceae_UCG_010, Enterococcus and Ruminiclostridium_5 showed a noticeable increase after treatment of glycyrrhiza polysaccharide (GCP) in mice intestine, and this change probably revealed the evidence supporting the anti-tumor function of GCP (Zhang et al., 2018a). Cinnamon essential oil can inhibit Escherichia coli and Staphylococcus by directly interacting with the targeted bacteria (Zhang et al., 2016). Besides, researches examining the elimination of gut microbiota have revealed that groups receiving treatment with TCM showed the presence of specific operational taxonomic units (OTUs) that were absent in the model group. Conversely, the model or normal group contained OTUs that were not detected in the TCM-treated group (Xu et al., 2013; Yang et al., 2017).
Table 1 Therapeutic efficacy of TCM complex extract on gut barrier, gut microbiota, and gut microbiota metabolites.
TCM indirectly affects the gut microbiota mainly in two ways. Firstly, it influences the growth environment of the bacteria, impacting their composition. Secondly, TCM can modify the transit time in the gastrointestinal tract. Gastrointestinal pH plays a critical role in modulating the bacteria density and its metabolite such as SCFAs. The population of the butyrate-producing Roseburia group presents a declining trend when pH is altered from 5.5 to 6.5, and paralleled concentration of butyrate presents a similar tide (Walker et al., 2005). Besides, the biological activity of enzymes can be impacted as well. TCM can also shape the bacteria-living environment from another aspect by modulating the immune responses in the gastrointestinal tract. For example, Fei-Xi-Tiao-Zhi-Fang can increase the sIgA in the model rats’ intestine, which may be the result of modulation of different microbial genera, as the abundance of g_Lactobacillus was observed to negatively change compared to sIgA (Liu et al., 2018b). Increased slgA provided a barrier against noxious substances, potentially guaranteeing other beneficial microbiota density and activity. In addition to the indirect reactions mentioned above, TCM can also enhance gut barrier function and increase nutrients for beneficial microbiota (Kamada et al., 2013; Lu et al., 2018). Besides, gastrointestinal transit time can affect gut microbiota metabolism. Transit time determines how long TCM compounds interact with gut microbiota, influencing their composition. Shorter transit time reduces metabolite production. A study has shown that TCM decoctions can modify transit time by influencing the interstitial cells of Cajal (Hwang et al., 2015).
When orally administered into the gastrointestinal tract, TCM can affect the metabolism of the gut microbiota and change the type and content of its metabolites (SCFA, BA, Trp, etc.). The reactions producing gut microbiota metabolites are as follows: (1) TCM can be the direct source of gut microbiota metabolite by interacting with gut microbiota, (2) TCM regulates the gut microbiota composition and therefore influence the production of metabolite, (3) TCM alters the activity and abundance of the enzymes essential for gut metabolite production, (4) an aggregation of these effects.
Known as the most exhaustively studied microbiota metabolite, SCFAs are a group of fatty acids that typically contain no more than six carbon atoms. SCFAs include formate, acetate, propionate, butyrate, valerate, and their corresponding isomeric forms. SCFAs mainly originate from the fermentation of non-digestible carbohydrates through a sequence of enzymatic reactions mediated by gut microbiota in the colon. The majority of SCFAs undergo enzyme-associated decomposition reactions to be metabolized by certain gut microorganisms such as the phylum Bacteroidetes and Firmicutes, which can encode genes to degrade polysaccharides (Xu et al., 2013). As the most comprehensively studied SCFA, butyrate can be obtained after the reaction that butyryl-CoA is converted into butyrate by phosphotransbutyrylase and butyrate kinase. What is worth noticing is that by the butyryl-CoA: acetate CoA-transferase route, gut microbiota can also convert butyryl-CoA into butyrate (Duncan et al., 2002; Louis et al., 2004). In terms of the production of other SCFAs, acetate arises from pyruvate via acetyl-CoA as well as Wood-Ljungdahl pathway, and propionate can derive from the reduction of lactate in acylate pathway. And an array of gut microbiota partakes in the reactions mentioned above (Scott et al., 2006; Rey et al., 2010; Louis et al., 2004).
Via acting as signaling molecules, SCFAs are equipped with quantities of functions such as modulation of gut integrity, gut hormone production, oxidative stress, appetite, and immune function (Feng et al., 2022). SCFAs can also serve as staple and preferred energy resources for the gut microbiota and host. SCFAs can either act as agonists for G protein-coupled receptors (GPRs) or exert suppressive influence on nuclear 1 histone deacetylase (HDAC) (HDAC1 and HDAC3) to affect the homeostasis. Through interfaces with GPR41 (free fatty acid receptor 3 or FFAR3) and GPR43 (free fatty acid receptor 2 or FFAR2) (Stoddart et al., 2008) as ligands, SCFAs are eligible of promoting the secretion of insulin by regulating the release of intestinal peptide hormones glucagon-like peptide-1 (GLP-1) and peptide YY (PYY) in pancreatic β cells, and hence can bring a restoration to aberrant insulin sensitivity and gluconeogenesis (Christiansen et al., 2018; Larraufie et al., 2018; Priyadarshini et al., 2018). When activating nod-like receptor family pyrin domain containing 3 (NLRP3), SCFAs can decline the amount of pro-inflammatory makers while they can enhance the proliferation and function of pancreatic β cells by suppressing the activity of HDAC (Zhang et al., 2021; Zhang et al., 2022b). Moreover, a positive influence on immunology responses can also be produced through inhibition of HDAC by the SCFAs (Koh et al., 2016; Zhang et al., 2021). A few SCFAs, most of which are acetate and propionate, can directly affect the adipose tissue, brain, and liver, contributing to a sequence of beneficial effects on the host (Koh et al., 2016).
Both the increase and decrease of SCFAs abundance can be triggered by TCM. Polysaccharides can directly act as the abundant source of SCFAs. These macromolecular substances escaping from digestion and absorption will be turned into SCFAs by the fermentation of gut microbiota (Zhang et al., 2022b). TCM can regulate the levels of SCFAs by affecting gut microbiota and enzymes. For example, Mulberry leaf has been reported to reverse the decrease of SCFAs induced by cyclophosphamide, and it can establish a cozy intestinal microenvironment for butyrate-producing bacteria which has been damaged by inflammatory cytokines before (Chen et al., 2021a). Additionally, the abundance of SCFAs exhibited a declining trend with the utilization of Pueraria lobata in antimicrobiota-associated diarrhea mice, and this treatment also altered the richness of the gut microbiota associated with SCFAs production (Chen et al., 2020). Furthermore, Shenling Baizhu powder can increase the abundance of Bifidobacterium and Anaerostipes, which can produce promotive metabolic impact on metabolism of SCFAs (Zhang et al., 2018b). Apart from modulating the levels of gut microbiota, TCM can also alter the metabolism of SCFAs by regulating the expression and activity of SCFA biosynthesis-associated enzymes. It has been demonstrated that berberine can significantly augment the formation of acetate, propionate and butyrate in rats, where prominent enzymes including Butyryl-CoA:acetate-CoA transferase (BUT), acetate kinase, and methylmalonyl-CoA decarboxylase were observed to increase (Wang et al., 2017a). And when the expressions of BUT and crotonyl-CoA and butyryl-CoA (viewed as butyrate synthesis-associated key substances) were promoted by berberine, blood lipid and glucose levels were consequently decreased (Wang et al., 2017b).
Deriving from cholesterol in the hepatocytes, BAs are a series of metabolites with a steroid structure and can be classified into two categories according to their resource (Fiorucci and Distrutti, 2015): primary BAs and secondary BAs. BAs play important roles in attenuating hyperlipidemia (Kong et al., 2021) and ameliorating systematic inflammation (Wei et al., 2018). Moreover, BAs are involved in correcting abnormal insulin sensitivity and glucose metabolism, while also serving as signaling molecules that facilitate inter-tissue communication between the liver and other organs (Perino et al., 2021). Cholesterol can be converted into primary BAs in two ways: the classic pathway (responsible for most production of primary BA) and the alternative pathway, which contains sophisticated successive reactions (Cheng et al., 2022a). Primary BAs can be transformed into bile salts by bile acid cholyl-CoA synthetase and bile acid-CoA: amino acid Nacyltransterase before entering intestine from gallbladder (Martinot et al., 2017). Bile salts will undergo deconjugation by bile salts hydrolase and then, free BAs can be released, which is the premise of secondary BAs formation. And many gut microbiota such as Lactobacillus, Bifidobacteria, and Bacteroides fragilis have been proved to produce bile salts hydrolases (Cheng et al., 2022a). The majority of free BAs can be reabsorbed and then transported back to liver, which is known as enterohepatic circulation. The rest of free BAs can be transformed into secondary BAs. The formation of secondary BAs involves a sequence of enzymatic reactions such as deconjugation, dehydroxylation and reconjugation, which are mediated by gut microbiota (Ridlon et al., 2014). In addition, gut microbial enzymes such as 7α-dehydroxylase and bacterial stereospecific hydroxysteroid dehydrogenases have been recognized as important factors for the formation of secondary BAs (Cheng et al., 2022a).
BAs can regulate lipids, amino acids, glucose metabolism by activating quantities of BA receptors including the cell surface receptor such as Takeda G-protein receptor (TGR5) and the intracellular receptors such as vitamins D3 receptor (VDR), farnesoid X receptor (FXR), pregnane X receptor and constitutive androstane receptor (Fiorucci and Distrutti, 2015; Xie et al., 2020). Disturbance of BAs receptors activity will trigger mass of host diseases including obesity, T2D, NAFLD, nonalcoholic steatohepatitis (NASH) and colon cancer (Jia et al., 2021).
TCM compounds can regulate BA metabolism via affecting enzymes and receptors involved in BA metabolism. These modulations are partly linked to the control of gut microbiota. For instance, the administration of Sophora alopecuroides has been shown to ameliorate colitis in DSS-induced mice, with concurrent changes in gut microbiota composition. Furthermore, this ameliorative effect on colitis was associated with an increase in BA levels (Jia et al., 2020). In another case of NAFLD mice, the extract of Penthorum chinense Pursh. was found to reduce the abundance of bacteria producing bile acid salt hydrolase (Clostridium_IV, Clostridium_XIVb, and Lactobacillus), resulting in decreased BA hydrolysis. This led to the accumulation of tauro-conjugated bile acids, which then decreased the levels of intestinal FXR and FGF15. Consequently, BA synthase activity was activated, promoting the conversion of cholesterol to bile acids and ultimately reducing cholesterol levels (Li et al., 2022d). Besides, in a study examining the therapeutic effects of Cryptotanshinone on mice with radiation-induced lung fibrosis, the levels of specific BAs, such as DCA and LCA, which activate FXR, were positively correlated with the relative abundance of Lachnospiraceae and Akkermansia. The decrease in Lachnospiraceae bacteria may reduce BA metabolism in cancer cachexia, while Akkermansia can enhance the production of coenzyme A and increase the synthesis of conjugated primary BAs. Hence, Cryptotanshinone may regulate BA metabolism by increasing the abundance of Lachnospiraceae and Akkermansia (Li et al., 2023). Other than that, Pu-er tea can increase BAs synthesis-associated enzymes in high-fat diet mice, including oxysterol 7α-hydroxylase (CYP7B1), mitochondrial sterol 27-hydroxylase (CYP27A1), and cholesterol 7α-hydroxylase (CYP7A1) (Huang et al., 2019).
Trp is an amino acid whose metabolism in the gut mainly covers three types: Trp can either be transformed into kynurenine, kynurenic tryptophan 2,3-dioxygenase and indoleamine 2,3-dioxigenase (kynurenine pathway) or be converted into serotonin and melatonin by Trp hydroxylase 1 (serotonin pathway) (Agus et al., 2018). Besides, Trp can be directly transformed into several substances by gut microbiota, such as indole and indole acid derivatives (indole propionic acid, indole acetic acid) (Agus et al., 2018). These metabolites can act as ligands for Aryl hydrocarbon receptor (AhR), and AhR signaling is crucial for regulating gut immune response and intestinal health (Agus et al., 2018). The metabolism of Trp has been proved to be of great value for the health of the host. As an example, the Trp-derived metabolites indolepropionic acid and indole were found to decrease in the serum of mice with DSS-induced colitis (Alexeev et al., 2018). And other diseases, including NAFLD, Crohn’s disease, obesity, stroke, mucosal candidiasis, chronic kidney disease, autism spectrum disorder, and Alzheimer’s disease, are also proved to be associated with impaired metabolism of Trp (Liu et al., 2022).
Studies demonstrating the potential of TCM to influence the metabolism of Trp abound. Remarkably increased Trp and lysine have been discovered in diabetic rats after the treatment of tea polysaccharide (Li et al., 2020a). Turmeric polysaccharides can recover damaged gut microbiota composition and increase indole-3-acetaldehyde (IALD) and indole-3-acetic acid levels (Yang et al., 2021c). Ganoderma lucidum polysaccharide can decrease the amount of indole lactate in colon while increasing the levels of Roseburia, Oxalobacter, Akkermansia, and decreasing those of Coprococcus, Eubacterium, Mogibacterium, Coprobacillus, and Ruminococcus (Jin et al., 2019). Considering gut bacteria such as Lactobacillus and Akkermansia are capable of promoting Trp metabolism (Gao et al., 2021; Yin et al., 2021), it is quite possible that the therapeutical effects of Ganoderma lucidum polysaccharide was achieved by modulation of microbial Trp metabolism.
TCM usually exerts a comprehensive effect on multiple gut barriers instead of on a sole one. On one hand, TCM can regulate gut microbiota and its metabolites to enhance the gut barriers. On the other hand, gut barrier can be directly regulated by TCM.
The damaged physical barrier is associated with a number of diseases such as inflammatory bowel disease, leaky gut, celiac disease, graft-versus-host-disease, diabetes, multiple sclerosis, and autism (Turner, 2009). Extraneous and endogenous opportunistic pathogens can damage the gut physical barrier, which in turn leads to increased permeability of the gut. LPS has been proved to be a detrimental factor to intestinal homeostasis, for it can give rise to enterocyte autophagy and destruction of gut barrier (Nguyen et al., 2013; Feng et al., 2018). LPS are structural components of the cell wall in gram-negative bacteria, and are derived from the death and lysis of these bacteria (Zhang et al., 2022a). The increased permeability of physical barrier will cause high LPS translocation in the blood stream, a condition known as metabolic endotoxemia (Cani et al., 2007). SCFAs can defend the barrier against the intestinal damage induced by LPS (Jiang et al., 2017). SCFAs can also consolidate the assembly of TJ proteins via activating AMP-activated protein kinase and promoting transcription of claudin-1 and expression of ZO-1 (Feng et al., 2018).
TCM can consolidate the physical barrier via modulating gut microbiota composition and gut microbiota metabolites such as SCFAs (Figure 3). Polygonatum kingianum polysaccharide has been documented to attenuate glucose and lipid metabolic disorder by repairing the gut physical barrier in high-fat diet rats. After its administration, the levels of ZO-1 and occludin were increased and the translocation of LPS was suppressed. And the increased levels of acetate and propionate and regulated Lactobacillus, Psychrobacter, Bacteroides, Allobaculum, Blautia, and Phascolarctobacterium also helped to fortify the gut physical barrier (Yan et al., 2017). Besides, Shugan Xiaozhi Decoction, a formula that can attenuate NAFLD in high-fat diet-induced rats, can also inhibit the translocation of LPS to avoid inflammation damage and increase ZO-1, occludin to fortify the gut barrier. This effect may be correlated with the lifting levels of f_Prevotellaceae and declining those of f_Desulfovibrionaceae and f_Erysi-Pelotrichaceae (Yang et al., 2022a). Mulberry leaf polysaccharide (MLP) can enhance the gene expression of occludin 1, ZO-1 and claudin 5 and relive intestinal villus damages. And the upregulation of the richness of butyrate-producing bacteria and downregulation of the Eubacterium-to-Bacteroidetes ratio can also be achieved by MLP to activate intestinal immunity and strengthen physical barrier. Ultimately, all these restorations boosted the recovery from immunosuppressed symptoms in cyclophosphamide-treated mice (Chen et al., 2021a). Another example is Astragluse polysaccharide, which can modulate the levels of Anaerofustis, Anaeroplasma, Anaerotruncus, Lachnospira, and Ruminococcus, then may potentially affecting the metabolism of butyrate (Li et al., 2022c). Apart from that, Astragluse polysaccharide was also found to fortify the gut physical barrier by increasing villus height and crypt depth to strengthen mucosal immunity in broiler chickens (Yang et al., 2021b). Thus, it is highly possible that the reinforcement of gut barrier was induced by increased SCFAs. But there exist exceptions. For example, in DSS-induced mice, the declining trend in mRNA expression of ZO-1, claudin-1 and occludin was reversed by Puerarin, while the amount of SCFAs showed a contradictory variation (Jeon et al., 2020). Hence, when the host gut physical barrier is enhanced, the variation of the TJ proteins expression and the SCFAs levels are not always consistent. But mounts of facts remind us that there might be a specifically causal linking between the enhancement of TJ proteins and SCFAs. To figure out the detailed mechanism, more studies need to be carried out.
Figure 3 TCM enhance gut barriers via regulating gut microbiota and its metabolites. (A) Via regulating gut microbiota and its metabolites, TCM improved TJ structure (ZO-1, occludin, claudin) and restored damaged intestinal epithelial cells, and thus improved gut physical barrier. The enhanced physical barrier further prevents translocation of pathogens. (B) Via regulating gut microbiota and its metabolites, TCM improved the abundance of goblet cells and increased the expression mucin 2 and TFF, and thus strengthened the gut chemical barrier. (C) Via regulating gut microbiota and its metabolites, TCM acted on immune cells to increase the expression of antimicrobial peptides (e.g., sIgA), enhanced the synthesis and release of anti-inflammatory cytokines and inhibited that of pro-inflammatory cytokines. Besides, TCM helped to activate immune cells (e.g., T-cells) and facilitate the transformation of immune cells (e.g., M1 to M2). The aggregation of these effects enhanced the gut immunological barrier. (D) TCM directly regulated the community structure, the diversity and richness, and the specific abundance of gut microbiota to restore damaged gut microbial barrier. AMP, antimicrobial peptide; IEC, intestinal epithelial cell; IgA, immunoglobulin A; LPS, lipopolysaccharide; M1, macrophage 1; M2, macrophage 2; SCFA, short chain fatty acid; TCM, Traditional Chinese Medicine; TFF, trefoil factor; TJ, tight junction; ZO-1, zonula occludens 1.
Mucin 2 plays an important role in maintaining the function of the gut chemical barrier. Bacteria are found to penetrate down into the crypts and epithelium in mice without mucin 2, and the bacterial invasion has been proved to be capable of triggering inflammation, diarrhea, rectal bleeding, colitis, and risen risk of colon cancer (Van der Sluis et al., 2006; Petersson et al., 2011). SCFAs can lift the levels of mucin 2. Butyrate, one of the most common SCFAs, is closely connected to the protection of intestinal epithelial cells and metabolism in the gut. More vitally, butyrate can enhance the mucin 2 gene expression in a dose-associated way, and this effect is achieved by mediation via AP-1 and acetylation/methylation of histones at the mucin 2 promoter (Burger-van Paassen et al., 2009). Apart from butyrate, acetate, and propionate can also positively alter the levels of mucin 2 (Burger-van Paassen et al., 2009).
Defects of chemical barrier in UC patients are commonly observed. These defects are attributed to two factors: the active inflammatory environment, which reduces the number of goblet cells, and long-term changes in mucin secretion that persist even when inflammation is absent (Singh et al., 2022). TCM has the potential to restore damaged chemical barrier by improving gut microbial metabolites. A specific example is the administration of puerarin in mice with UC induced by DSS, which has been shown to rebuild the damaged barrier and attenuate lesions. Puerarin ingestion improved the production of SCFAs and prevented the overgrowth of mucin-utilizing microbiota. It also enhanced goblet cell differentiation, secretion, and restored the thickness of the mucus layer. These effects likely contributed to the restoration of mucus and goblet cell function, and the changes in SCFAs and bacteria community may be the underlying causes (Wu et al., 2020).
In addition to mucin 2, trefoil factor 3 (TFF3) is another component of the mucus layer secreted by goblet cells that aids in mucosal repair and content renewal. TFF3 promotes viscosity by binding to the von Willebrand factor C domain of mucin 2, thereby enhancing mucus integrity (Tomasetto et al., 2000). Cinnamaldehyde has been proved to directly promote the expression of TFF3 and mucin 2 in early-weaned rats (Qi et al., 2022). The richness of Akkermansia, Bacteroides, Clostridium III, Psychrobacter, and Intestinimonas were increased and those of Ruminococcus and Escherichia/Shigella were declined in the experiment. As a result, the repaired gut chemical barrier effectively inhibited inflammatory response in the intestinal tract. Furthermore, it has been documented that Rhubard extract (RE) was conducive to promoting goblet cells to increase the density of mucin 2 and TFF3, then strengthening the concentration, viscosity, and function of the mucus layer in constipation mice model induced by diphenoxylate (Gao et al., 2021). RE also increased the level of Bacteroides, decreased that of Alistipes, and lifted the concentration of butyric acid, isobutyric acid, and isovaleric acid. Apart from that, it is important to note that there is a potential relationship between mucin 2 and TFF3. Supplementation of mucin 2 has been found to restore decreased mRNA levels of TFF3 in rats with acute necrotizing pancreatitis (Huang et al., 2023). However, the regulation in gut microbiota and its metabolites by TCM may be the key reason for the improvement in mucus. Further research is needed to fully understand the interplay between gut microbiota, its metabolites, mucin 2, TFF3, and their effects on mucus.
As one of the bacteria that benefit from TCM, Akkermansia has gained attention for its well-established role as a significant contributor to mucus maintenance and its anti-inflammatory properties (Shin et al., 2019; Leong et al., 2022). It shows an excellent ability to multiply the abundance of intestinal goblet cells and increase the levels of mucin 2, and can upregulate the mucin gene and the regeneration of mucin, which eventually contribute to normalization and incrassation of the mucus layer (Tropini et al., 2018; Shin et al., 2019). Qiwei Baizhu Decoction, a classic herbal formula, has been widely used in clinical practice to treat diarrhea by repairing the mucus barrier. It has been observed to enhance the expression of mucin 2 in juvenile rats with diarrhea. This positive effect may mainly be attributed to the increased abundance of Akkermansia and the simultaneous increase in the expression of SCFAs stimulated by the decoction (Sun et al., 2021b). While Akkermansia utilizes mucin as an energy source, it is more accurate to describe it as a mucin utilizer rather than a mucin degrader. Unlike pathogens or other mucin-degrading bacteria, Akkermansia utilizes mucins by specifically degrading O-glycans without disrupting the integrity of the inner mucus layer barrier. This allows for a balanced rate of mucus utilization in coordination with mucus synthesis, resulting in a dynamic and stable mucus structure (Suriano et al., 2022). In contrast, pathogens have the ability to break down the polymeric network of mucins, leading to the collapse of the mucus structure (Luis and Hansson, 2023). However, there are still other underlying mechanisms that remain unknown and require further investigation.
SCFAs can partake in immune responses, change the expression of AMP, and consolidate the gut immune barrier in multiple ways. SCFAs can directly interact with GPR43 to upregulate the production of Reg3-γ and β-defensins in IECs, which relies on the activation of the mammalian target of rapamycin via GPR43 (Zhao et al., 2018). Besides, it has also been studied that SCFAs can activate the NLRP3 inflammasome through G-protein coupled receptors signaling, enhancing the defense against intestinal inflammation (Macia et al., 2015). SCFAs can regulate the differentiation of Treg (Arpaia et al., 2013). Additionally, SCFAs can suppress macrophage 1 (M1) and facilitate macrophage 2 (M2) polarization to enhance intestinal and host immune functions (Wang et al., 2020b). M1 is conducive to the recruitment of pro-inflammatory factors such as IL-1β, IL-6, and TNF-α, whereas M2 shows anti-inflammatory and tissue-repairing function (Biswas et al., 2006; Gabrilovich et al., 2012). Apart from gut microbial metabolites (SCFAs), gut microbiota is also indispensable for maintaining the function of the gut immune barrier. It has been found that there exist few AMPs (such as C-type lectin Reg3-γ and Reg3-β) in Germ-free mice compared with normally raised ones (Hooper et al., 2003; Cash et al., 2006; Vaishnava et al., 2011). Certain gut microbiota such as Clostridium spp., Schaedler, and Bacteroides fragilis have been proved to shift immune responses through promoting the development of certain subtypes of lymphocytes (Takiishi et al., 2017).
TCM can modulate the gut microbiota composition and its metabolites to complete the repairment and enhancement of the gut immune barrier (Figure 3). For example, the relative abundance of Bifidobacterium, a bacterium responsible for SCFAs production, showed an increasing trend with the administration of BuFei-JianPi-fang (BJF) in chronic obstructive pulmonary disease model rats (Mao et al., 2022). And the BJF also reinforced the gut immune barrier via counteracting the declined expression of sIgA and SCFAs in the colon mucus layer and downregulating NLRP3, caspase-1, IL-1β, and IL-18 levels, thereby delaying the aggravation of inflammation in the host (Mao et al., 2022).
The interplays between TCM and gut microbiota can also modulate the Paneth cell and intestinal macrophages in the intestine. Poria cocos polysaccharide (PCP) showed the ability to increase the abundance of Paneth cell and SCFA-producing bacteria: Bacteroides intestinihominis, Butyricicoccus pullicaecorum, Lactobacillus johnsoni, Bifidobactrium choerinum, and Eubacterium spp. in ApcMin/+ mice (Yin et al., 2022). PCP also suppressed M1 macrophages and facilitated M2 macrophages to increase the anti-inflammatory factors such as IL-4, IL-10, and IL-13 to enhance the fight against the intestinal side effects of 5-fluorouracil treatment (Yin et al., 2022). Another example is traditional Patchouli Essential oil (PEO) and its derivatives, they can increase the levels of phylum Firmicutes and Akkermansia muciniphila and decrease those of phylum Bacteroidetes in ApcMin/+ mice (Leong et al., 2022). Apart from that, the expression of SCFA receptors (GPR41, GPR43, and GPR109a) and Paneth cells were augmented and M1 macrophages were directly shifted to M2 macrophages by PEO and its derivatives (Leong et al., 2022). As a result of these joint regulations, the gut immune barrier was enhanced, resulting in the improvement of the immunity and metabolism of the host. Another example is polysaccharides from fermented coix (PFC), which can enrich the abundance of Bacteroidetes and decrease those of Tenericutes, Firmicutes Anaeroplasma, Oscillospira, Peptococcaceae and Ruminococcus in BALB/c mice exposed to high relative humidity and thus regulate the levels of SCFAs (acetic acid, propionic acid, butyric acid, isobutyric acid, valeric acid and isovaleric acid) (Wang et al., 2022a). As the gut microbiota was regulated by PFC, so were Th1/Th2 cytokines (Wang et al., 2022a), and these two parts collaborated on restoring gut immune function and circulating nitrogen disorder of the host. Altogether, to strengthen the gut immune barrier, TCM can modulate the secretion of AMP, proinflammatory and anti-inflammatory cells and cytokines. And the occurring of the reactions mentioned above usually coincides with the modulation of gut microbiota and its metabolites.
As the core of the gut microbial barrier, the composition of the gut microbiota community is essential to ensure the efficacy of TCM. It has been documented that deviated gut microbiota cannot offer the amelioration of steatohepatitis and resistance to colonization of extraneous pathogens. For example, Pien Tze Huang (PTH), a TCM with the function of alleviating NASH, failed to provide mice which are fed with antibiotics cocktail with protection against NASH (Zeng et al., 2024).
TCM usually directly modulates the richness of multiple gut microbiota to enhance gut barrier function (Figure 3). For example, a study has revealed that curcumin plays an important role in preventing and ameliorating gut microbial dysbiosis in diabetic rats, whose administration can decrease the abundance of Enterobacterales and Firmicutes and lift those of Bacteroidetes and Bifidobacterium (Huang et al., 2021). Bifidobacterium can form the biofilm on the surface of the intestinal mucosa, and regulate microenvironment and microecological balance of intestine, therefore contributing to the repairment of gut barrier (Schwiertz et al., 2010). Another example is that Sini Decoction can enhance the gut microbial barrier by lifting the abundance of Bacillus coagulans, Lactobacillus, Akkermansia muciniphila and Bifidobacterium in DSS-induced colorectal cancer mice (Wang et al., 2021). The similar result was also repeated by Ge Gen Qin Lian Decoction (GQD) in colorectal cancer patients, which promoted the abundance of Bacteroides, Akkermansia and Prevotella and decreased those of Megamonas and Veillonella (Li et al., 2020c). Via modulating gut microbiota composition, both of these two decoctions postponed the development of colorectal cancer (Li et al., 2020b; Wang et al., 2021).
Furthermore, the gut microbiota modulated by TCM can produce SCFAs, which lower the pH of the intestines, thereby inhibiting the growth of harmful microorganisms. Moreover, these TCM-modulated microbiota outcompete pathogens for oxygen and nutrients, preventing their overgrowth and invasion into underlying tissues, including the bloodstream (Hebbandi Nanjundappa et al., 2017). The function of lifting the abundance of SCFA-producing bacteria has been revealed in the Baicalin treatment in spontaneously hypertensive rats (Wu et al., 2019a). And the levels of acetic acid, propionic acid, butyric acid, isobutyric acid, valeric acid, and isovaleric acid were observed to increase. As a result, the gut microbial barrier was enhanced and the ulcerative intestinal lesions in rats were effectively relived.
TCM inclines to produce a comprehensive and protective function on multiple barriers instead of a sole one. GQD helped to promote other gut barrier function and inhibit the development of intestinal inflammation by altering gut microbiota composition in colorectal cancer patients. Because GQD can lift the expression of ZO-1 and occludin, inhibit the NF-κB inflammatory signaling pathway, reduce the inflammatory factor TNF-α in blood and decrease tumor tissues (Li et al., 2020c). Furthermore, Inulin (INU) and Lycium barbarum polysaccharide (LBP) can lift the abundance of Bifidobacterium and Lactobacillus in T2D model rats (Lu et al., 2021). These two bacteria can promote CD8 T-cells activation and costimulation and strengthen DC defensive function (Ljungh and Wadström, 2006; Sivan et al., 2015). Besides, the enhanced gut microbial barrier and increased SCFAs (acetic acid, propionic acid, butyric acid, and valeric acid) induced by INU and LBP have been shown to be positively correlated with the promotion of ZO-1 and occludin, as well as the upregulation of γδ IELs and TLR2+ γδ IELs. These changes collectively improved both the gut physical and immune barrier, subsequently relieving systemic inflammation in the host. Hence, by the interfaces between TCM and microbiota, both gut microbial barrier and other gut barriers can be consolidated.
An array of diseases, such as celiac disease, enteric infections, Crohn’s disease, UC (Turner, 2009), T2D (Ma et al., 2019) and NASH (Albillos et al., 2020), have been documented to be correlated with the dysbiosis of gut microbiota, and TCM compounds have presented excellent ability to maintain the intestinal homeostasis by regulating gut microbiota and further consolidating the gut barriers (Wu et al., 2018). In Tables 1, 2, we have listed the effects of TCM on gut microbiota, its metabolites, gut barriers, and diseases.
Table 2 Therapeutic efficacy of TCM compounds on gut barrier, gut microbiota, and gut microbiota metabolites.
T2D is a prevalent chronic clinical condition characterized by absolute or relative inadequate insulin synthesis, often accompanied by IR and reduced sensitivity of target organs to insulin. T2D is associated with metabolic disorders involving fat, water, electrolytes, and other imbalances (Canfora et al., 2019; Yoo et al., 2020) The pathogenesis of T2D is closely linked to dysbiosis of the gut microbiota, obesity, genetic factors, and dysfunction of pancreatic islets (Ma et al., 2019). Growing evidence has supported the correlation between T2D occurrence and an imbalanced gut microbiota ecology, as well as damaged gut barrier (Cani et al., 2012). The density of Faecalibacterium, Roseburia, Dialister, Flavonifractor, Alistipes, Haemophilus, and Akkermansia muciniphila has shown a declining trend in the pathogenesis of T2D (Letchumanan et al., 2022). Akkermansia muciniphila plays a protective role in maintaining the gut chemical barrier and promoting glucose tolerance. Its decreased concentration has been associated with increased permeability of the intestinal tract (Letchumanan et al., 2022). Furthermore, T2D patients exhibit lower levels of SCFAs such as acetic acid, propionic acid, and butyric acid (Salamone et al., 2022). The dysbiosis of the gut microbiota community, along with the shortage of SCFAs, compromise the integrity and function of the gut barrier, thereby exacerbating T2D symptoms. In T2D patients, the physical barrier and microbial barrier of the gut are primarily affected (Ma et al., 2019). Notably, intestinal bacteria have been detected in the bloodstream of T2D patients, indicating evident damage to the gut barriers (Sircana et al., 2018). This damage facilitates the colonization of pathogenic microorganisms in the intestinal mucus layer and subsequent translocation of gut microbiota. As a result, endogenous infections and metabolic endotoxemia may occur (Ma et al., 2019).
Increased gut permeability and translocation of intestinal LPS are closely associated with metabolic inflammation in T2D. The compound of Scutellaria baicalensis and Coptis chinensis (SC) has been found to ameliorate these phenomena in T2D by modulating the gut microbiota and reinforcing the physical barrier in diabetic rats (Zhang et al., 2020a). Administration of SC led to a decline in the levels of Proteobacteria, Enterobacteriaceae, Enterococcus, Escherichia-Shigella, and Enterobacter, while promoting the abundance of certain bacteria belonging to Lachnospiraceae and Prevotellacae. Additionally, SC promoted the levels of TJ proteins (ZO-1, occludin, and claudin-1), which play a role in inhibiting intestinal inflammatory responses related to TLR-4/TRIF and TNFR-1/NF-κB signaling pathways, thus preventing LPS translocation (Zhang et al., 2020a). Ultimately, these changes attenuated abnormal glucose metabolism and chronic inflammation caused by LPS leakage in T2D (Cani et al., 2012). Apart from reinforcing gut barriers, SCFAs elevated by SC can exert a beneficial effect on T2D by interacting with GPR43, which promotes the levels of GLP-1 (Zhu and Goodarzi, 2020). GLP-1 can improve insulin release, restore IR, inhibit glucagon secretion and promote satiety (Tolhurst et al., 2012).
In db/db mice (obese and diabetic), Andrographolide has demonstrated its potential to modulate the gut microbiota and improve metabolic health. Specifically, it can elevate the Bacteroidetes/Firmicutes ratio and promote the abundance of Akkermansia muciniphila, a bacterium known for its beneficial effects in delaying the development of diet-induced obesity and insulin resistance (Cani and de Vos, 2017). It also reversed the decreased SCFAs in mice by increasing the levels of acetate, propionate, butyrate, and valeric acid. Moreover, Andrographolide promoted the levels of mucin 2, enhancing the gut chemical barrier, as well as ZO-1 and occludin, enhancing the gut physical barrier. These effects may be associated with the downregulation of pro-inflammatory markers such as IL-6 and TNF-α after Andrographolide administration. Overall, based on these observed changes, Andrographolide has demonstrated its effectiveness in reducing oxidative stress and inflammation, hence alleviating glucose intolerance and insulin resistance (Su et al., 2020).
Ramulus Mori Alkaloids (SZ-A) have demonstrated therapeutic effects on inflammatory damage in T2D KKAy mice (Liu et al., 2021). SZ-A increased the concentration of propionate and acetate while significantly elevating the expression of MCT1 and SMCT1 (SLC5A8), which are crucial for the transcellular transfer of SCFAs into the intestinal epithelium. This resulted in enhanced consumption of SCFAs by intestinal cells (Liu et al., 2021). SZ-A also reduced the abundance of Desulfovibrio, Alistipes, and Aerococcus, while promoting those of Bacteroides, Faecalibaculum, and Allobaculum, thereby assisting in the restoration of the gut microbial barrier. Moreover, SZ-A enhanced the gut immune barrier by reducing the percentage of macrophages (CD11c+/F4/80+) and the cell mass of CD11c-positive monocytes (Liu et al., 2021). These effects worked together to downregulate the expression of inflammatory cytokines (IL-1β, IL-6, CCL4, CCL5) in the intestinal tract, thereby alleviating T2D (Liu et al., 2021).
In conclusion, TCM plays a role in alleviating T2D and its related symptoms by increasing the expression of TJ proteins, reinforcing the function of the gut physical barrier to attenuate LPS leakage. TCM also maintains the normal secretion of GLP-1 through the modulation of the gut microbiota community. Furthermore, TCM ensures an adequate supply of SCFAs and the normal function of SCFA transporters and receptors, which are essential for the normal function of gut barriers. Additionally, TCM regulates the gut immune barrier and reduces the levels of pro-inflammatory cytokines. Most importantly, most of these therapeutic functions are based on the regulation of the gut microbiota.
NAFLD encompasses a spectrum of diseases, ranging from simple steatosis to advanced NASH, and ultimately hepatocellular carcinoma. The pathogenesis of NAFLD is primarily driven by lipid accumulation, which leads to lipotoxicity and mitochondrial dysfunction. These alterations can trigger hepatocyte injury, inflammation, and varying degrees of fibrosis (Pierantonelli and Svegliati-Baroni, 2019). Inflammatory response is a hallmark of NASH pathogenesis, involving the release of various proinflammatory cytokines and hepatocyte damage (Lan et al., 2021). Apart from that, the activation of TLR hepatic inflammatory factors mediated by LPS and oxidative stress are crucial factors in the occurrence and development of NASH (Kiziltas, 2016). Importantly, a compromised gut physical barrier has been observed in NAFLD patients (Dai and Wang, 2015). Furthermore, under conditions of a high-fat diet, NAFLD individuals showed aberrant bacterial growth and altered microbiota composition when compared to healthy controls. Recent research has underscored the growing recognition of the significant role that gut barrier malfunction and the subsequent translocation of harmful bacteria and pathogen-associated molecular patterns (such as LPS) play in the development of hepatic inflammation and the progression of both NAFLD and NASH (Turner, 2009; Albillos et al., 2020; Ghosh et al., 2021).
BAs are an important factor related to the amelioration of NAFLD (Volynets et al., 2010). The levels of both primary and secondary BAs are observed to increase in NAFLD patients (Jiao et al., 2018). BAs not only suppress hepatic gluconeogenesis, facilitate insulin secretion and glycogen synthesis, and relieve inflammation, but also regulate the balance between Th17 and Treg cells to maintain the function of the gut immune barrier and alleviate inflammation (Shapiro et al., 2018). SCFAs help maintain the integrity of the gut physical barrier, preventing the migration of toxic compounds to the liver (Kelly et al., 2015; Wang et al., 2021). Butyrate, in particular, has shown the capability to inhibit inflammation in mice by promoting the differentiation of colonic anti-inflammatory regulatory T cells, thereby delaying the progression of NAFLD (Canfora et al., 2019).
The gut microbiota and its metabolites play a vital role in the development of NASH through their interaction with the liver, a relationship known as the gut-liver axis (Marra and Svegliati-Baroni, 2018; Albillos et al., 2020). TCM exhibits a comprehensive function in maintaining the gut-liver axis and alleviating NASH symptoms. For example, Fufang Zhenzhu Tiaozhi Capsule has been shown to attenuate NASH and progressive liver fibrosis in NAFLD mice by inhibiting hepatic inflammation, stellate cell activation, and collagen deposition. This is achieved by regulating tight junctions, consolidating intestinal barrier function, and restoring the dysregulated gut microbiota (Bacteroidia, Verrucomicrobiae, Clostridia, Epsilonproteobacteria) and its metabolites, especially BAs (Lan et al., 2022). Notably, the pool size, composition as well as chemical and signaling properties of BAs all are modulated by gut microbiota through enzymatic reactions such as deconjugation and epimerization (Ridlon et al., 2014; Parséus et al., 2017).
Another example is PTH, a TCM that has been demonstrated to protect the liver by lowering steatosis and inhibiting inflammatory cells (Zeng et al., 2024). Its administration in mice reversed diet-induced steatosis and liver injury by increasing the abundance of beneficial bacteria such as Lactobacillus acidophilus (L. acidophilus), Lactobacillus plantarum, Lactococcus lactis, Bacillus subtilis and L. acidophilus (Zeng et al., 2024), hence increasing the levels of therapeutic BAs, such as tauroursodeoxycholic acid (TUDCA), taurocholic acid (TCA), taurodeoxycholic acid (TDCA), and glycocholic acid (GCA). Furthermore, PTH treatment lifted the expression of E-cadherin, thereby enhancing the integrity of gut physical barrier (Zeng et al., 2024).
Besides, Zhishi daozhi decoction (ZDD) effectively attenuated uncontrolled inflammatory responses in high-fat diet-induced NAFLD mice by optimizing the gut microbial structure, increasing the levels of Faecalibacterium and Bacteroidetes, and reducing those of Brautia and Colidextribacter (Bi et al., 2023). Additionally, ZDD increased the concentration of acetic acid, butyric acid, and propionic acid, as well as the levels of ZO-1 and occludin, thereby strengthening the gut physical barrier and promoting NAFLD recovery (Bi et al., 2023). And in treatment of NAFLD rats, the opportunistic pathogen Escherichia/Shigella was decreased and the SCFA producer Collinsella was increased by Qushi Huayu Decoction. Its active components can also improve Treg cells activity. These effects contributed to repairing gut immune barrier and then inhibiting the exposure of liver to harmful substances such as LPS and other noxious gut microbiota products (Feng et al., 2017).
In conclusion, damaged gut barriers are commonly observed in NAFLD and NASH hosts. BAs and SCFAs play crucial roles in the therapeutic functions of NAFLD. TCM can restore the dysregulated gut microbiota community and simultaneously restore levels of corresponding therapeutic gut microbiota metabolites in the treatment of NAFLD. Based on the regulation of gut microbiota and its metabolites, multiple gut barriers can be repaired or enhanced to prevent the progression of NAFLD or NASH.
UC is a chronic inflammatory bowel disease prone to relapsing and characterized by clinical symptoms such as diarrhea, weight loss, abdominal pain, and bloody stool. It is also associated with an increased risk of colorectal cancer. The lesions in UC are typically found in the colonic mucosa and submucosa (Ungaro et al., 2017; Dong et al., 2020). While the exact etiology and pathogenesis of UC are not fully understood, studies have shown that it is mainly influenced by factors such as predisposition, dysfunction of the intestinal epithelial barrier, dysbiosis of the gut microbiota, immune response disorders, and environmental factors (Zhang and Li, 2014).
Apart from inflammation-induced injuries in the gastrointestinal tract, patients with UC also suffer from mucosal inflammation extending from the colon to the rectum and gut barrier dysfunction, which is principally caused by depleted colonic goblet cells and increasing permeability of the gut chemical barrier (Johansson et al., 2014).The pathogenesis of UC involves the participation of innate lymphoid cells (Buonocore et al., 2010) and cellular immunity, in which ILC3, T-helper-2 (Th2), and IL-4, IL-1β, IL-13 TNF-α are involved (Ungaro et al., 2017; Miao et al., 2021). Furthermore, a decreased biodiversity of the gut microbiota has been associated with the development of UC. Specifically, UC patients have been found to have decreased levels of Firmicutes and increased those of Gamma-proteobacteria and Enterobacteriaceae (Frank et al., 2007).
TCM has shown promise in the treatment of intestinal tract injuries in UC. For example, Baitouweng decoction (BTW), a commonly used formula in UC therapy, has been found to increase the abundance of beneficial bacteria such as Lactobacillus and Akkermansia, while decreasing the abundance of harmful bacteria such as Escherichia-Shigella in UC mice (Chen et al., 2021b). BTW also increased the production of butyrate, propionate and acetate and enhanced the expression of ZO-1 and occludin (Miao et al., 2021). Additionally, BTW can modulate the Th17/Treg cell balance, thereby preventing inflammatory cytokines like TNF-α and IL-1β from damaging TJ proteins and further compromising intestinal integrity (Miao et al., 2021). These changes helped strengthen both the physical and immune barriers of the gut, leading to improvements in inflammatory symptoms associated with UC.
Another TCM remedy, the aqueous extract of Paeoniae radix alba ((paeonia lactiflora pall) (PRADG)), has also shown to protect the intestinal tract against inflammation. PRADG attenuated the population of Th17 cells and downregulated the IL-23/IL-17 axis, thereby reducing inflammation. Moreover, PRADG promoted the restoration of injured goblet cells, epithelial cells, and colonic crypts in mice with DSS-induced colitis. This restoration enhanced both the physical and chemical barriers of the gut (Yan et al., 2022), preventing detrimental effects caused by local/systematic inflammatory or immunology responses when gut bacterial metabolites translocation occurring (Yan et al., 2022). And after PRADG treatment, the abundance of Bacteroidetes, Verrucomicrobia, Actinobacteria, norank_f_Muribaculaceae, Lactobacillus, and Akkermansia were increased and those of Proteobacteria, Fusobacteria, Bacteroides, Escherichia-Shigella, Romboutsia, and Fusobacterium were decreased.
Additionally, Ginsenoside Rg 1 can restore the colonic injury and colonic inflammation via reversing general gut microbiota structure and abundance disturbed in DSS-induced mice (Cheng et al., 2022b) and promoting the relative abundance of beneficial bacteria such as Lactobacillus Akkermansia norank_f_Muribaculaceae, which can catabolize Trp into its metabolites by activating AhR agonist (Cheng et al., 2022b). These gut microbiota metabolites and AhR agonists, including (3-indolylacrylic acid (ILA), indole propionic acid (IPA), IALD, nicotinamide (Nam)), decrease the levels of pro-inflammatory cytokines, thereby protecting the physical barrier of the gut. Moreover, the interface between AhR and its ligands (ILA, IPA, IALD) can protect this barrier by increasing the production of anti-inflammatory cytokines such as IL-22 (Cheng et al., 2022b).
In conclusion, TCM can treat UC via modulation of physical barrier, chemical barrier, immunological barrier, and the mechanisms are linked to modulation of gut microbiota and its metabolites.
The gut barrier plays a crucial role in maintaining the normal function and balance of the intestinal tract. In this review, we provide an overview of the composition and function of the four main gut barriers. Additionally, we analyze the intricate interactions between the predominant gut microbiota, its metabolites, and TCM compounds. These interactions between TCM compounds and the gut microbiota hold great potential for therapeutic applications. Understanding the mechanisms involved in these interactions will not only enhance our knowledge of TCM pharmacodynamics but also pave the way for the development of novel therapeutics targeting gut microbiota imbalance and impaired gut barrier. Although great progresses have been made in understanding how TCM can improve gut microbiota, there are still considerations that warrant attention in future studies examining the mechanisms by which TCM can enhance gut barrier function and treat associated diseases.
The complexity of TCM compounds and the diversity of gut microbiota present significant challenges in studying the pharmacodynamics of TCM. Factors such as different origins, processing methods, and combinations can affect the therapeutic properties and functions of TCM when administered clinically. Therefore, it is crucial to establish standardized and processed criteria to ensure consistent and effective therapeutic outcomes.
In order to demonstrate the causal relationship between TCM and its medicinal effects, it is important to refine the measurement of TCM effects on the microbiota and assess gut barrier indicators in a more comprehensive manner. Merely testing the composition or ratio of gut microbiota, along with a few simple biochemical and immunological indicators, may not provide sufficient evidence. Additionally, there is a lack of in-depth exploration of the pathways of action, related enzymatic pathways, and therapeutic outcomes interpretated by TCM theory.
To better understand and regulate the gut microbiota response to TCM, detailed analysis of intestinal contents is necessary. This will help identify specific changes and enable personalized medication approaches. Exploring the molecular mechanisms and signal pathways involved in the interaction between TCM and the gut microbiota is also important for achieving desired therapeutic outcomes. Moreover, while there have been studies investigating the metabolism of individual active ingredients (monomers) of TCM by the gut microbiota, research on drug interactions becomes more challenging when multiple components are metabolized simultaneously. Therefore, future studies should focus on analyzing the impact of the gut microbiota on the metabolism of TCM as a whole. This comprehensive approach will enhance our understanding and utilization of TCM in clinical practice.
YZ: Investigation, Methodology, Visualization, Writing – original draft, Writing – review & editing. JL: Writing – review & editing, Funding acquisition, Investigation. DZ: Writing – review & editing, Investigation. HC: Investigation, Writing – review & editing. JW: Investigation, Writing – review & editing. WF: Conceptualization, Funding acquisition, Supervision, Writing – review & editing, Writing – original draft. CP: Conceptualization, Funding acquisition, Supervision, Writing – review & editing.
The author(s) declare financial support was received for the research, authorship, and/or publication of this article. This work was supported by the National Natural Science Foundation of China (No. 82104409, 82304850), Sichuan Science and Technology Program (No. 2023NSFSC1778, 2024NSFJQ0044, 2024NSFSC0054), National Interdisciplinary Innovation Team of Traditional Chinese Medicine (ZYYCXTD-D-202209), the China Postdoctoral Science Foundation (No. 2023MD734103), and Sichuan Provincial Administration of Traditional Chinese Medicine (2022C001).
The authors declare that the research was conducted in the absence of any commercial or financial relationships that could be construed as a potential conflict of interest.
All claims expressed in this article are solely those of the authors and do not necessarily represent those of their affiliated organizations, or those of the publisher, the editors and the reviewers. Any product that may be evaluated in this article, or claim that may be made by its manufacturer, is not guaranteed or endorsed by the publisher.
AhR, Aryl hydrocarbon receptor; AMP, antimicrobial peptide; BA, bile acid; DC, dendritic cell; GLP-1, glucagon-like peptide-1; GPR, G protein-coupled receptor; HD5, human α defensin 5; HDAC, nuclear 1 histone deacetylase; IEC, intestinal epithelial cell; IEL, intraepithelial lymphocyte; IgA, immunoglobulin A; IL, interleukin; IR insulin resistance; LPS, lipopolysaccharide; M cell, microfold cell; M1 macrophage 1; M2, macrophage 2; NAFLD, non-alcoholic fatty liver disease; NLRP3, nod-like receptor family pyrin domain containing 3; PP, Peyer’s patch; PRADG, the aqueous extract of Paeoniae radix alba; PYY, peptide YY; SC, Scutellaria baicalensis and Coptis chinensis; SCFA, short chain fatty acid; sIgA, secreted immunoglobulin A; T2D, type 2 diabetes; TCM, traditional Chinese medicine; TFF3, trefoil factor 3; Th cell, T helper cell; TJ, tight junction; TNF, tumor necrosis factor; Treg, regulatory T cell; Trp, tryptophan; ZO-1, zonula occludens 1.
Afzaal, M., Saeed, F., Shah, Y. A., Hussain, M., Rabail, R., Socol, C. T., et al. (2022). Human gut microbiota in health and disease: Unveiling the relationship. Front. Microbiol. 13. doi: 10.3389/fmicb.2022.999001
Agus, A., Planchais, J., Sokol, H. (2018). Gut microbiota regulation of tryptophan metabolism in health and disease. Cell Host Microbe 23, 716–724. doi: 10.1016/j.chom.2018.05.003
Albillos, A., de Gottardi, A., Rescigno, M. (2020). The gut-liver axis in liver disease: Pathophysiological basis for therapy. J. Hepatol. 72, 558–577. doi: 10.1016/j.jhep.2019.10.003
Alexeev, E. E., Lanis, J. M., Kao, D. J., Campbell, E. L., Kelly, C. J., Battista, K. D., et al. (2018). Microbiota-derived indole metabolites promote human and murine intestinal homeostasis through regulation of interleukin-10 receptor. Am. J. Pathol. 188, 1183–1194. doi: 10.1016/j.ajpath.2018.01.011
Arpaia, N., Campbell, C., Fan, X., Dikiy, S., van der Veeken, J., deRoos, P., et al. (2013). Metabolites produced by commensal bacteria promote peripheral regulatory T-cell generation. Nature 504, 451–455. doi: 10.1038/nature12726
Ashida, N., Yanagihara, S., Shinoda, T., Yamamoto, N. (2011). Characterization of adhesive molecule with affinity to Caco-2 cells in Lactobacillus acidophilus by proteome analysis. J. Biosci. Bioeng. 112, 333–337. doi: 10.1016/j.jbiosc.2011.06.001
Aura, A.-M. (2008). Microbial metabolism of dietary phenolic compounds in the colon. Phytochem. Rev. 7, 407–429. doi: 10.1007/s11101-008-9095-3
Bai, X., Fu, R., Duan, Z., Wang, P., Zhu, C., Fan, D. (2021). Ginsenoside Rk3 alleviates gut microbiota dysbiosis and colonic inflammation in antibiotic-treated mice. Food Res. Int. 146, 110465. doi: 10.1016/j.foodres.2021.110465
Bansal, T., Alaniz, R. C., Wood, T. K., Jayaraman, A. (2010). The bacterial signal indole increases epithelial-cell tight-junction resistance and attenuates indicators of inflammation. Proc. Natl. Acad. Sci. U.S.A. 107, 228–233. doi: 10.1073/pnas.0906112107
Bayoumi, M. A., Griffiths, M. W. (2012). In vitro inhibition of expression of virulence genes responsible for colonization and systemic spread of enteric pathogens using Bifidobacterium bifidum secreted molecules. Int. J. Food Microbiol. 156, 255–263. doi: 10.1016/j.ijfoodmicro.2012.03.034
Bemark, M., Boysen, P., Lycke, N. Y. (2012). Induction of gut IgA production through T cell-dependent and T cell-independent pathways. Ann. N Y Acad. Sci. 1247, 97–116. doi: 10.1111/j.1749-6632.2011.06378.x
Bergstrom, K. S. B., Kissoon-Singh, V., Gibson, D. L., Ma, C., Montero, M., Sham, H. P., et al. (2010). Muc2 protects against lethal infectious colitis by disassociating pathogenic and commensal bacteria from the colonic mucosa. PloS Pathog. 6, e1000902. doi: 10.1371/journal.ppat.1000902
Beumer, J., Clevers, H. (2021). Cell fate specification and differentiation in the adult mammalian intestine. Nat. Rev. Mol. Cell Biol. 22, 39–53. doi: 10.1038/s41580-020-0278-0
Bevins, C. L., Salzman, N. H. (2011). Paneth cells, antimicrobial peptides and maintenance of intestinal homeostasis. Nat. Rev. Microbiol. 9, 356–368. doi: 10.1038/nrmicro2546
Bi, C.-R., Sun, J.-T., Du, J., Chu, L.-Y., Li, Y.-J., Jia, X.-Y., et al. (2023). Effects of Zhishi Daozhi Decoction on the intestinal flora of nonalcoholic fatty liver disease mice induced by a high-fat diet. Front. Cell Infect. Microbiol. 12. doi: 10.3389/fcimb.2022.1005318
Birchenough, G. M. H., Johansson, M. E. V., Gustafsson, J. K., Bergström, J. H., Hansson, G. C. (2015). New developments in goblet cell mucus secretion and function. Mucosal Immunol. 8, 712–719. doi: 10.1038/mi.2015.32
Birchenough, G., Schroeder, B. O., Bäckhed, F., Hansson, G. C. (2019). Dietary destabilisation of the balance between the microbiota and the colonic mucus barrier. Gut Microbes 10, 246–250. doi: 10.1080/19490976.2018.1513765
Biswas, S. K., Gangi, L., Paul, S., Schioppa, T., Saccani, A., Sironi, M., et al. (2006). and unique transcriptional program expressed by tumor-associated macrophages (defective NF-kappaB and enhanced IRF-3/STAT1 activation). Blood 107, 2112–2122. doi: 10.1182/blood-2005-01-0428
Buonocore, S., Ahern, P. P., Uhlig, H. H., Ivanov, I. I., Littman, D. R., Maloy, K. J., et al. (2010). Innate lymphoid cells drive interleukin-23-dependent innate intestinal pathology. Nature 464, 1371–1375. doi: 10.1038/nature08949
Burger-van Paassen, N., Vincent, A., Puiman, P. J., van der Sluis, M., Bouma, J., Boehm, G., et al. (2009). The regulation of intestinal mucin MUC2 expression by short-chain fatty acids: implications for epithelial protection. Biochem. J. 420, 211–219. doi: 10.1042/BJ20082222
Canfora, E. E., Meex, R. C. R., Venema, K. (2019). Gut microbial metabolites in obesity, NAFLD and T2DM. E. Nat. Rev. Endocrinol. 15, 261–273. doi: 10.1038/s41574-019-0156-z
Cani, P. D., Amar, J., Iglesias, M. A., Poggi, M., Knauf, C., Bastelica, D., et al. (2007). Metabolic endotoxemia initiates obesity and insulin resistance. Diabetes 56, 1761–1772. doi: 10.2337/db06-1491
Cani, P. D., de Vos, W. M. (2017). Next-generation beneficial microbes: the case of akkermansia muciniphila. Front. Microbiol. 8. doi: 10.3389/fmicb.2017.01765
Cani, P. D., Osto, M., Geurts, L., Everard, A. (2012). Involvement of gut microbiota in the development of low-grade inflammation and type 2 diabetes associated with obesity. Gut Microbes 3, 279–288. doi: 10.4161/gmic.19625
Cash, H. L., Whitham, C. V., Behrendt, C. L., Hooper, L. V. (2006). Symbiotic bacteria direct expression of an intestinal bactericidal lectin. Science 313, 1126–1130. doi: 10.1126/science.1127119
Chang, C.-J., Lu, C.-C., Lin, C.-S., Martel, J., Ko, Y.-F., Ojcius, D. M., et al. (2018). Antrodia cinnamomea reduces obesity and modulates the gut microbiota in high-fat diet-fed mice. Int. J. Obes. (Lond) 42, 231–243. doi: 10.1038/ijo.2017.149
Chen, X., Cai, B., Wang, J., Sheng, Z., Yang, H., Wang, D., et al. (2021a). Mulberry leaf-derived polysaccharide modulates the immune response and gut microbiota composition in immunosuppressed mice. J. Funct. Foods 83, 104545. doi: 10.1016/j.jff.2021.104545
Chen, R., Liu, B., Wang, X., Chen, K., Zhang, K., Zhang, L., et al. (2020). Effects of polysaccharide from Pueraria lobata on gut microbiota in mice. Int. J. Biol. Macromol. 158, 740–749. doi: 10.1016/j.ijbiomac.2020.04.201
Chen, X., Lv, X., Liu, S. (2021b). Baitouweng decoction alleviates dextran sulfate sodium-induced ulcerative colitis by regulating intestinal microbiota and the IL-6/STAT3 signaling pathway. J. Ethnopharmacol. 265, 113357. doi: 10.1016/j.jep.2020.113357
Cheng, H., Liu, J., Zhang, D., Tan, Y., Feng, W., Peng, C. (2022a). Gut microbiota, bile acids, and nature compounds. Phytother. Res. 36, 3102–3119. doi: 10.1002/ptr.7517
Cheng, H., Liu, J., Zhang, D., Wang, J., Tan, Y., Feng, W., et al. (2022b). Ginsenoside rg1 alleviates acute ulcerative colitis by modulating gut microbiota and microbial tryptophan metabolism. Front. Immunol. 13. doi: 10.3389/fimmu.2022.817600
Chiba, H., Osanai, M., Murata, M., Kojima, T., Sawada, N. (2008). Transmembrane proteins of tight junctions. Biochim. Biophys. Acta (BBA) - Biomembranes 1778, 588–600. doi: 10.1016/j.bbamem.2007.08.017
Christiansen, C. B., Gabe, M. B. N., Svendsen, B., Dragsted, L. O., Rosenkilde, M. M., Holst, J. J. (2018). The impact of short-chain fatty acids on GLP-1 and PYY secretion from the isolated perfused rat colon. J. Am. J. Physiol. Gastrointest Liver Physiol. 315, G53–G65. doi: 10.1152/ajpgi.00346.2017
Cui, H.-X., Zhang, L.-S., Luo, Y., Yuan, K., Huang, Z.-Y., Guo, Y. (2019). A purified anthraquinone-glycoside preparation from rhubarb ameliorates type 2 diabetes mellitus by modulating the gut microbiota and reducing inflammation. Front. Microbiol. 10. doi: 10.3389/fmicb.2019.01423
Currò, D. (2018). The role of gut microbiota in the modulation of drug action: a focus on some clinically significant issues. Expert Rev. Clin. Pharmacol. 11, 171–183. doi: 10.1080/17512433.2018.1414598
Dai, X., Wang, B. (2015). Role of gut barrier function in the pathogenesis of nonalcoholic Fatty liver disease. Gastroenterol. Res. Pract. 2015, 287348. doi: 10.1155/2015/287348
Diana, J., Moura, I. C., Vaugier, C., Gestin, A., Tissandie, E., Beaudoin, L., et al. (2013). Secretory IgA induces tolerogenic dendritic cells through SIGNR1 dampening autoimmunity in mice. J. Immunol. 191, 2335–2343. doi: 10.4049/jimmunol.1300864
Dong, C., Metzger, M., Holsbø, E., Perduca, V., Carbonnel, F. (2020). Systematic review with meta-analysis: mortality in acute severe ulcerative colitis. Aliment Pharmacol. Ther. 51, 8–33. doi: 10.1111/apt.15592
Dong, J., Ping, L., Cao, T., Sun, L., Liu, D., Wang, S., et al. (2022). Immunomodulatory effects of the Bifidobacterium longum BL-10 on lipopolysaccharide-induced intestinal mucosal immune injury. Front. Immunol. 13. doi: 10.3389/fimmu.2022.947755
Duncan, S. H., Barcenilla, A., Stewart, C. S., Pryde, S. E., Flint, H. J. (2002). Acetate utilization and butyryl coenzyme a (CoA):Acetate-coa transferase in butyrate-producing bacteria from the human large intestine. Appl. Environ. Microbiol. 68, 5186–5190. doi: 10.1128/AEM.68.10.5186-5190.2002
Earle, K. A., Billings, G., Sigal, M., Lichtman, J. S., Hansson, G. C., Elias, J. E., et al. (2015). Quantitative imaging of gut microbiota spatial organization. Cell Host Microbe 18, 478–488. doi: 10.1016/j.chom.2015.09.002
El Kaoutari, A., Armougom, F., Gordon, J. I., Raoult, D., Henrissat, B. (2013). The abundance and variety of carbohydrate-active enzymes in the human gut microbiota. Nat. Rev. Microbiol. 11, 497–504. doi: 10.1038/nrmicro3050
Etienne-Mesmin, L., Chassaing, B., Desvaux, M., De Paepe, K., Gresse, R., Sauvaitre, T., et al. (2019). Experimental models to study intestinal microbes-mucus interactions in health and disease. FEMS Microbiol. Rev. 43, 457–489. doi: 10.1093/femsre/fuz013
Fang, S., Wang, T., Li, Y., Xue, H., Zou, J., Cai, J., et al. (2022). Gardenia jasminoides Ellis polysaccharide ameliorates cholestatic liver injury by alleviating gut microbiota dysbiosis and inhibiting the TLR4/NF-κB signaling pathway. Int. J. Biol. Macromol. 205, 23–36. doi: 10.1016/j.ijbiomac.2022.02.056
Fava, F., Rizzetto, L., Tuohy, K. M. (2019). Gut microbiota and health: connecting actors across the metabolic system. Proc. Nutr. Soc. 78, 177–188. doi: 10.1017/S0029665118002719
Feng, W., Ao, H., Peng, C., Yan, D. (2019). Gut microbiota, a new frontier to understand traditional Chinese medicines. Pharmacol. Res. 142, 176–191. doi: 10.1016/j.phrs.2019.02.024
Feng, Q., Liu, W., Baker, S. S., Li, H., Chen, C., Liu, Q., et al. (2017). Multi-targeting therapeutic mechanisms of the Chinese herbal medicine QHD in the treatment of non-alcoholic fatty liver disease. Oncotarget 8, 27820–27838. doi: 10.18632/oncotarget.v8i17
Feng, W., Liu, J., Cheng, H., Zhang, D., Tan, Y., Peng, C. (2022). Dietary compounds in modulation of gut microbiota-derived metabolites. Front. Nutr. 9. doi: 10.3389/fnut.2022.939571
Feng, Y., Wang, Y., Wang, P., Huang, Y., Wang, F. (2018). Short-chain fatty acids manifest stimulative and protective effects on intestinal barrier function through the inhibition of NLRP3 inflammasome and autophagy. Cell Physiol. Biochem. 49, 190–205. doi: 10.1159/000492853
Filosa, S., Di Meo, F., Crispi, S. (2018). Polyphenols-gut microbiota interplay and brain neuromodulation. Neural Regener. Res. 13, 2055–2059. doi: 10.4103/1673-5374.241429
Fiorucci, S., Distrutti, E. (2015). Bile acid-activated receptors, intestinal microbiota, and the treatment of metabolic disorders. Trends Mol. Med. 21, 702–714. doi: 10.1016/j.molmed.2015.09.001
Frank, D. N., St Amand, A. L., Feldman, R. A., Boedeker, E. C., Harpaz, N., Pace, N. R. (2007). Molecular-phylogenetic characterization of microbial community imbalances in human inflammatory bowel diseases. Proc. Natl. Acad. Sci. U.S.A. 104, 13780–13785. doi: 10.1073/pnas.0706625104
Gabrilovich, D. I., Ostrand-Rosenberg, S., Bronte, V. (2012). Coordinated regulation of myeloid cells by tumours. Nat. Rev. Immunol. 12, 253–268. doi: 10.1038/nri3175
Ganz, T., Gabayan, V., Liao, H.-I., Liu, L., Oren, A., Graf, T., et al. (2003). Increased inflammation in lysozyme M-deficient mice in response to Micrococcus luteus and its peptidoglycan. Blood 101, 2388–2392. doi: 10.1182/blood-2002-07-2319
Gao, C.-C., Li, G.-W., Wang, T.-T., Gao, L., Wang, F.-F., Shang, H.-W., et al. (2021). Rhubarb extract relieves constipation by stimulating mucus production in the colon and altering the intestinal flora. BioMed. Pharmacother. 138, 111479. doi: 10.1016/j.biopha.2021.111479
Garcia-Bonete, M. J., Rajan, A., Suriano, F., Layunta, E. (2023). The underrated gut microbiota helminths, bacteriophages, fungi, and archaea. Life (Basel) 13, 1765–1782. doi: 10.3390/life13081765
Ghosh, S., Whitley, C. S., Haribabu, B., Jala (2021). Regulation of intestinal barrier function by microbial metabolites. V. R. Cell. Mol. Gastroenterol. Hepatol. 11, 1463–1482. doi: 10.1016/j.jcmgh.2021.02.007
Glover, L. E., Lee, J. S., Colgan, S. P. (2016). Oxygen metabolism and barrier regulation in the intestinal mucosa. J. Clin. Invest. 126, 3680–3688. doi: 10.1172/JCI84429
Gomez de Agüero, M., Ganal-Vonarburg, S. C., Fuhrer, T., Rupp, S., Uchimura, Y., Li, H., et al. (2016). The maternal microbiota drives early postnatal innate immune development. Science 351, 1296–1302. doi: 10.1126/science.aad2571
Hansson, G. C. (2019). Mucus and mucins in diseases of the intestinal and respiratory tracts. J. Intern. Med. 285, 479–490. doi: 10.1111/joim.12910
He, X., Liu, J., Long, G., Xia, X.-H., Liu, M. (2021). 2,3,5,4’-Tetrahydroxystilbene-2-O-β-D-glucoside, a major bioactive component from Polygoni multiflori Radix (Heshouwu) suppresses DSS induced acute colitis in BALb/c mice by modulating gut microbiota. BioMed. Pharmacother. 137, 111420. doi: 10.1016/j.biopha.2021.111420
Hebbandi Nanjundappa, R., Ronchi, F., Wang, J., Clemente-Casares, X., Yamanouchi, J., Sokke Umeshappa, C., et al. (2017). A gut microbial mimic that hijacks diabetogenic autoreactivity to suppress colitis. Cell 171, 655–667.e17. doi: 10.1016/j.cell.2017.09.022
Hooper, L. V., Stappenbeck, T. S., Hong, C. V., Gordon, J. I. (2003). Angiogenins: a new class of microbicidal proteins involved in innate immunity. Nat. Immunol. 4, 269–273. doi: 10.1038/ni888
Hu, J., Huang, H., Che, Y., Ding, C., Zhang, L., Wang, Y., et al. (2021). Qingchang Huashi Formula attenuates DSS-induced colitis in mice by restoring gut microbiota-metabolism homeostasis and goblet cell function. J. Ethnopharmacol. 266, 113394. doi: 10.1016/j.jep.2020.113394
Huang, J., Guan, B., Lin, L., Wang, Y. (2021). Improvement of intestinal barrier function, gut microbiota, and metabolic endotoxemia in type 2 diabetes rats by curcumin. Bioengineered 12, 11947–11958. doi: 10.1080/21655979.2021.2009322
Huang, S., Pang, D., Li, X., You, L., Zhao, Z., Cheung, P. C.-K., et al. (2019). A sulfated polysaccharide from Gracilaria Lemaneiformis regulates cholesterol and bile acid metabolism in high-fat diet mice. Food Funct. 10, 3224–3236. doi: 10.1039/C9FO00263D
Huang, Z., Wu, H., Fan, J., Mei, Q., Fu, Y., Yin, N., et al. (2023). Colonic mucin-2 attenuates acute necrotizing pancreatitis in rats by modulating intestinal homeostasis. FASEB J. 37, e22994. doi: 10.1096/fj.202201998R
Hwang, M. W., Ahn, T. S., Hong, N. R., Jeong, H.-S., Jung, M. H., Ha, K.-T., et al. (2015). Effects of traditional Chinese herbal medicine San-Huang-Xie-Xin-Tang on gastrointestinal motility in mice. World J. Gastroenterol. 21, 1117–1124. doi: 10.3748/wjg.v21.i4.1117
Hynönen, U., Palva, A. (2013). Lactobacillus surface layer proteins: structure, function and applications. Appl. Microbiol. Biotechnol. 97, 5225–5243. doi: 10.1007/s00253-013-4962-2
Ikenouchi, J., Umeda, K., Tsukita, S., Furuse, M., Tsukita, S. (2007). Requirement of ZO-1 for the formation of belt-like adherens junctions during epithelial cell polarization. J. Cell Biol. 176, 779–786. doi: 10.1083/jcb.200612080
Inagaki, T., Moschetta, A., Lee, Y.-K., Peng, L., Zhao, G., Downes, M., et al. (2006). Regulation of antibacterial defense in the small intestine by the nuclear bile acid receptor. Proc. Natl. Acad. Sci. U.S.A. 103, 3920–3925. doi: 10.1073/pnas.0509592103
Jakobsson, H. E., Rodríguez-Piñeiro, A. M., Schütte, A., Ermund, A., Boysen, P., Bemark, M., et al. (2015). The composition of the gut microbiota shapes the colon mucus barrier. EMBO Rep. 16, 164–177. doi: 10.15252/embr.201439263
Jeon, Y.-D., Lee, J.-H., Lee, Y.-M., Kim, D.-K. (2020). Puerarin inhibits inflammation and oxidative stress in dextran sulfate sodium-induced colitis mice model. BioMed. Pharmacother. 124, 109847. doi: 10.1016/j.biopha.2020.109847
Jia, W., Wei, M., Rajani, C., Zheng, X. (2021). Targeting the alternative bile acid synthetic pathway for metabolic diseases. Protein Cell 12, 411–425. doi: 10.1007/s13238-020-00804-9
Jia, Y.-Q., Yuan, Z.-W., Zhang, X.-S., Dong, J.-Q., Liu, X.-N., Peng, X.-T., et al. (2020). Total alkaloids of Sophora alopecuroides L. ameliorated murine colitis by regulating bile acid metabolism and gut microbiota. J. Ethnopharmacol. 255, 112775. doi: 10.1016/j.jep.2020.112775
Jiang, P., Guo, Y., Dang, R., Yang, M., Liao, D., Li, H., et al. (2017). Salvianolic acid B protects against lipopolysaccharide-induced behavioral deficits and neuroinflammatory response: involvement of autophagy and NLRP3 inflammasome. J. Neuroinflamm. 14, 239. doi: 10.1186/s12974-017-1013-4
Jiao, N., Baker, S. S., Chapa-Rodriguez, A., Liu, W., Nugent, C. A., Tsompana, M., et al. (2018). Suppressed hepatic bile acid signalling despite elevated production of primary and secondary bile acids in NAFLD. Gut 67, 1881–1891. doi: 10.1136/gutjnl-2017-314307
Jin, M., Zhang, H., Wang, J., Shao, D., Yang, H., Huang, Q., et al. (2019). Response of intestinal metabolome to polysaccharides from mycelia of Ganoderma lucidum. Int. J. Biol. Macromol. 122, 723–731. doi: 10.1016/j.ijbiomac.2018.10.224
Johansson, M. E. V., Hansson, G. (2012). Preservation of mucus in histological sections, immunostaining of mucins in fixed tissue, and localization of bacteria with FISH. C. Methods Mol. Biol. 842, 229–235. doi: 10.1007/978–1-61779–513-8_13
Johansson, M. E. V., Ambort, D., Pelaseyed, T., Schütte, A., Gustafsson, J. K., Ermund, A., et al. (2011). Composition and functional role of the mucus layers in the intestine. Cell. Mol. Life Sci. 68, 3635–3641. doi: 10.1007/s00018-011-0822-3
Johansson, M. E. V., Gustafsson, J. K., Holmén-Larsson, J., Jabbar, K. S., Xia, L., Xu, H., et al. (2014). Bacteria penetrate the normally impenetrable inner colon mucus layer in both murine colitis models and patients with ulcerative colitis. Gut 63, 281–291. doi: 10.1136/gutjnl-2012-303207
Kamada, N., Chen, G. Y., Inohara, N., Núñez, G. (2013). Control of pathogens and pathobionts by the gut microbiota. Nat. Immunol. 14, 685–690. doi: 10.1038/ni.2608
Kankainen, M., Paulin, L., Tynkkynen, S., von Ossowski, I., Reunanen, J., Partanen, P., et al. (2009). Comparative genomic analysis of Lactobacillus rhamnosus GG reveals pili containing a human- mucus binding protein. Proc. Natl. Acad. Sci. U.S.A. 106, 17193–17198. doi: 10.1073/pnas.0908876106
Kelly, C. J., Zheng, L., Campbell, E. L., Saeedi, B., Scholz, C. C., Bayless, A. J., et al. (2015). Crosstalk between microbiota-derived short-chain fatty acids and intestinal epithelial HIF augments tissue barrier function. Cell Host Microbe 17, 662–671. doi: 10.1016/j.chom.2015.03.005
Kiziltas, S. (2016). Toll-like receptors in pathophysiology of liver diseases. World J. Hepatol. 8, 1354–1369. doi: 10.4254/wjh.v8.i32.1354
Koh, A., De Vadder, F., Kovatcheva-Datchary, P., Bäckhed, F. (2016). From dietary fiber to host physiology: short-chain fatty acids as key bacterial metabolites. Cell 165, 1332–1345. doi: 10.1016/j.cell.2016.05.041
Kong, Y., Li, Y., Dai, Z.-R., Qin, M., Fan, H.-L., Hao, J.-G., et al. (2021). Glycosaminoglycan from Ostrea rivularis attenuates hyperlipidemia and regulates gut microbiota in high-cholesterol diet-fed zebrafish. Food Sci. Nutr. 9, 5198–5210. doi: 10.1002/fsn3.2492
König, J., Wells, J., Cani, P. D., García-Ródenas, C. L., MacDonald, T., Mercenier, A., et al. (2016). Human intestinal barrier function in health and disease. Clin. Transl. Gastroenterol. 7, e196. doi: 10.1038/ctg.2016.54
Kuhn, K. A., Pedraza, I., Demoruelle, M. K. (2014). Mucosal immune responses to microbiota in the development of autoimmune disease. Rheum Dis. Clin. North Am. 40, 711–725. doi: 10.1016/j.rdc.2014.07.013
Kuo, W.-T., Odenwald, M. A., Turner, J. R., Zuo, L. (2022). Tight junction proteins occludin and ZO-1 as regulators of epithelial proliferation and survival. Ann. N Y Acad. Sci. 1514, 21–33. doi: 10.1111/nyas.14798
Lan, T., Xu, T., Fu, Y., Jiang, S., Liang, X., Yu, Z., et al. (2022). Fufang zhenzhu tiaozhi capsule prevents intestinal inflammation and barrier disruption in mice with non-alcoholic steatohepatitis. Front. Endocrinol. (Lausanne) 13. doi: 10.3389/fendo.2022.864703
Lan, T., Yu, Y., Zhang, J., Li, H., Weng, Q., Jiang, S., et al. (2021). Cordycepin ameliorates nonalcoholic steatohepatitis by activation of the AMP-activated protein kinase signaling pathway. Hepatology 74, 686–703. doi: 10.1002/hep.31749
Larraufie, P., Martin-Gallausiaux, C., Lapaque, N., Dore, J., Gribble, F. M., Reimann, F., et al. (2018). SCFAs strongly stimulate PYY production in human enteroendocrine cells. Sci. Rep. 8, 74. doi: 10.1038/s41598–017-18259–0
Lécuyer, E., Rakotobe, S., Lengliné-Garnier, H., Lebreton, C., Picard, M., Juste, C., et al. (2014). Segmented filamentous bacterium uses secondary and tertiary lymphoid tissues to induce gut IgA and specific T helper 17 cell responses. Immunity 40, 608–620. doi: 10.1016/j.immuni.2014.03.009
Leong, W., Huang, G., Liao, W., Xia, W., Li, X., Su, Z., et al. (2022). Traditional Patchouli essential oil modulates the host’s immune responses and gut microbiota and exhibits potent anti-cancer effects in ApcMin/+ mice. Pharmacol. Res. 176, 106082. doi: 10.1016/j.phrs.2022.106082
Letchumanan, G., Abdullah, N., Marlini, M., Baharom, N., Lawley, B., Omar, M. R., et al. (2022). Gut microbiota composition in prediabetes and newly diagnosed type 2 diabetes: a systematic review of observational studies. Front. Cell. Infect. Microbiol. 12, 943427. doi: 10.3389/fcimb.2022.943427
Li, H., Fang, Q., Nie, Q., Hu, J., Yang, C., Huang, T., et al. (2020a). Hypoglycemic and hypolipidemic mechanism of tea polysaccharides on type 2 diabetic rats via gut microbiota and metabolism alteration. J. Agric. Food Chem. 68, 10015–10028. doi: 10.1021/acs.jafc.0c01968
Li, Y., Jin, L., Chen, T. (2020b). The effects of secretory igA in the mucosal immune system. BioMed. Res. Int. 2020, 2032057. doi: 10.1155/2020/2032057
Li, Y., Li, Z. X., Xie, C. Y., Fan, J., Lv, J., Xu, X. J., et al. (2020c). Gegen Qinlian decoction enhances immunity and protects intestinal barrier function in colorectal cancer patients via gut microbiota. World J. Gastroenterol. 26, 7633–7651. doi: 10.3748/wjg.v26.i48.7633
Li, S., Lin, R., Chen, J., Hussain, R., Zhang, S., Su, Y., et al. (2022c). Integrated gut microbiota and metabolomic analysis reveals immunomodulatory effects of EChinacea extract and Astragalus polysaccharides. Front. Vet. Sci. 9. doi: 10.3389/fvets.2022.971058
Li, Y., Liu, T., Yan, C., Xie, R., Guo, Z., Wang, S., et al. (2018). Diammonium Glycyrrhizinate Protects against Nonalcoholic Fatty Liver Disease in Mice through Modulation of Gut Microbiota and Restoration of Intestinal Barrier. Mol. Pharm. 15, 3860–3870. doi: 10.1021/acs.molpharmaceut.8b00347
Li, Z., Shen, Y., Xin, J., Xu, X., Ding, Q., Chen, W., et al. (2023). Cryptotanshinone alleviates radiation-induced lung fibrosis via modulation of gut microbiota and bile acid metabolism. Phytother. Res. 37, 4557–4571. doi: 10.1002/ptr.7926
Li, C., Yu, S., Li, X., Cao, Y., Li, M., Ji, G., et al. (2022a). Medicinal formula huazhi-rougan attenuates non-alcoholic steatohepatitis through enhancing fecal bile acid excretion in mice. Front. Pharmacol. 13. doi: 10.3389/fphar.2022.833414
Li, M., Yu, L., Zhai, Q., Liu, B., Zhao, J., Chen, W., et al. (2022b). Ganoderma lucidum ethanol extraction promotes dextran sulphate sodium induced colitis recovery and modulation in microbiota. Foods 11, 4023–4036. doi: 10.3390/foods11244023
Li, X., Zhao, W., Xiao, M., Yu, L., Chen, Q., Hu, X., et al. (2022d). Penthorum chinense Pursh. extract attenuates non-alcholic fatty liver disease by regulating gut microbiota and bile acid metabolism in mice. J. Ethnopharmacology 294, 115333. doi: 10.1016/j.jep.2022.115333
Liu, J., Cai, J., Fan, P., Dong, X., Zhang, N., Tai, J., et al. (2023). Salidroside protects mice from high-fat diet-induced obesity by modulating the gut microbiota. Int. Immunopharmacol. 120, 110278. doi: 10.1016/j.intimp.2023.110278
Liu, Q., Liu, S., Cao, H., Ji, W., Li, C., Huan, Y., et al. (2021). Ramulus mori (Sangzhi) alkaloids (SZ-A) ameliorate glucose metabolism accompanied by the modulation of gut microbiota and ileal inflammatory damage in type 2 diabetic KKAy mice. Front. Pharmacol. 12. doi: 10.3389/fphar.2021.642400
Liu, J., Tan, Y., Cheng, H., Zhang, D., Feng, W., Peng, C. (2022). Functions of gut microbiota metabolites, current status and future perspectives. Aging Dis. 13, 1106–1126. doi: 10.14336/AD.2022.0104
Liu, J., Yue, S., Yang, Z., Feng, W., Meng, X., Wang, A., et al. (2018a). Oral hydroxysafflor yellow A reduces obesity in mice by modulating the gut microbiota and serum metabolism. Pharmacol. Res. 134, 40–50. doi: 10.1016/j.phrs.2018.05.012
Liu, T.-H., Zhang, X.-M., Han, N.-P., Liu, Y., Wu, Y.-Y., Li, X.-Y., et al. (2018b). Regulation effect of a chinese herbal formula on flora and mucosal immune secretory immunoglobulin A in rats. Evid Based Complement Alternat Med. 2018, 4821821. doi: 10.1155/2018/4821821
Ljungh, A., Wadström, T. (2006). Lactic acid bacteria as probiotics. Curr. Issues Intest. Microbiol. 7, 73–89.
Louis, P., Duncan, S. H., McCrae, S. I., Millar, J., Jackson, M. S., Flint, H. J. (2004). Restricted distribution of the butyrate kinase pathway among butyrate-producing bacteria from the human colon. J. Bacteriol 186, 2099–2106. doi: 10.1128/JB.186.7.2099-2106.2004
Lu, Z., Ding, L., Lu, Q., Chen, Y.-H. (2013). Claudins in intestines: Distribution and functional significance in health and diseases. Tissue Barriers 1, e24978. doi: 10.4161/tisb.24978
Lu, H., Liu, P., Zhang, X., Bao, T., Wang, T., Guo, L., et al. (2021). Inulin and Lycium barbarum polysaccharides ameliorate diabetes by enhancing gut barrier via modulating gut microbiota and activating gut mucosal TLR2+ intraepithelial γδ T cells in rats. J. Funct. Foods 79, 104407. doi: 10.1016/j.jff.2021.104844
Lu, L., Yan, L., Yuan, J., Ye, Q., Lin, J. (2018). Shuganyin decoction improves the intestinal barrier function in a rat model of irritable bowel syndrome induced by water-avoidance stress. Chin. Med. 13, 6. doi: 10.1186/s13020-017-0161-x
Luis, A. S., Hansson, G. C. (2023). Intestinal mucus and their glycans: A habitat for thriving microbiota. Cell Host Microbe 31, 1087–1100. doi: 10.1016/j.chom.2023.05.026
Luo, S., Wen, R., Wang, Q., Zhao, Z., Nong, F., Fu, Y., et al. (2019). Rhubarb Peony Decoction ameliorates ulcerative colitis in mice by regulating gut microbiota to restoring Th17/Treg balance. J. Ethnopharmacol. 231, 39–49. doi: 10.1016/j.jep.2018.08.033
Lyu, M., Wang, Y., Fan, G., Wang, X., Xu, S., Zhu, Y. (2017). Balancing herbal medicine and functional food for prevention and treatment of cardiometabolic diseases through modulating gut microbiota. Front. Microbiol. 8. doi: 10.3389/fmicb.2017.02146
Ma, Q., Li, Y., Li, P., Wang, M., Wang, J., Tang, Z., et al. (2019). Research progress in the relationship between type 2 diabetes mellitus and intestinal flora. Biomed. Pharmacother. 117, 109138. doi: 10.1016/j.biopha.2019.109138
Macia, L., Tan, J., Vieira, A. T., Leach, K., Stanley, D., Luong, S., et al. (2015). Metabolite-sensing receptors GPR43 and GPR109A facilitate dietary fibre-induced gut homeostasis through regulation of the inflammasome. Nat. Commun. 6, 6734–6748. doi: 10.1038/ncomms7734
Mao, J., Li, Y., Bian, Q., Xuan, Y., Li, J., Wang, Z., et al. (2022). The bufei jianpi formula improves mucosal immune function by remodeling gut microbiota through the SCFAs/GPR43/NLRP3 pathway in chronic obstructive pulmonary disease rats. Int. J. Chron Obstruct Pulmon Dis. 17, 1285–1298. doi: 10.2147/COPD.S359428
Marra, F., Svegliati-Baroni, G. (2018). Lipotoxicity and the gut-liver axis in NASH pathogenesis. J. Hepatol. 68, 280–295. doi: 10.1016/j.jhep.2017.11.014
Martinot, E., Sèdes, L., Baptissart, M., Lobaccaro, J.-M., Caira, F., Beaudoin, C., et al. (2017). Bile acids and their receptors. Mol. Aspects Med. 56, 2–9. doi: 10.1016/j.mam.2017.01.006
Miao, Z., Chen, L., Feng, H., Gu, M., Yan, J., Xu, Y., et al. (2021). Baitouweng decoction ameliorates ulcerative colitis in mice partially attributed to regulating th17/treg balance and restoring intestinal epithelial barrier. Front. Pharmacol. 11. doi: 10.3389/fphar.2020.531117
Mills, S., Stanton, C., Lane, J. A., Smith, G. J., Ross, R. P. (2019). Precision nutrition and the microbiome, part I: current state of the science. Nutrients 11, 923. doi: 10.3390/nu11040923
Mörbe, U. M., Jørgensen, P. B., Fenton, T. M., von Burg, N., Riis, L. B., Spencer, J., et al. (2021). Human gut-associated lymphoid tissues (GALT); diversity, structure, and function. Mucosal Immunol. 14, 793–802. doi: 10.1038/s41385-021-00389-4
Mukherjee, S., Partch, C. L., Lehotzky, R. E., Whitham, C. V., Chu, H., Bevins, C. L., et al. (2009). Regulation of C-type lectin antimicrobial activity by a flexible N-terminal prosegment. J. Biol. Chem. 284, 4881–4888. doi: 10.1074/jbc.M808077200
Muñoz, M., Heimesaat, M. M., Danker, K., Struck, D., Lohmann, U., Plickert, R., et al. (2009). Interleukin (IL)-23 mediates Toxoplasma gondii-induced immunopathology in the gut via matrixmetalloproteinase-2 and IL-22 but independent of IL-17. J. Exp. Med. 206, 3047–3059. doi: 10.1084/jem.20090900
Natividad, J. M. M., Verdu, E. F. (2013). Modulation of intestinal barrier by intestinal microbiota: Pathological and therapeutic implications. Pharmacol. Res. 69, 42–51. doi: 10.1016/j.phrs.2012.10.007
Nguyen, H. T. T., Lapaquette, P., Bringer, M.-A., Darfeuille-Michaud, A. (2013). Autophagy and crohn’s disease. J. Innate Immun. 5, 434–443. doi: 10.1159/000345129
Ning, Y., Gong, Y., Zheng, T., Xie, Y., Yuan, S., Ding, W. (2022). Lingguizhugan decoction targets intestinal microbiota and metabolites to reduce insulin resistance in high-fat diet rats. Diabetes Metab. Syndr. Obes. 15, 2427–2442. doi: 10.2147/DMSO.S370492
Owen, R. L., Jones, A. L. (1974). Epithelial cell specialization within human Peyer’s patches: an ultrastructural study of intestinal lymphoid follicles. Gastroenterology 66, 189–203. doi: 10.1016/S0016-5085(74)80102-2
Paolella, G., Mandato, C., Pierri, L., Poeta, M., Stasi, M. D., Vajro, P. (2014). Gut-liver axis and probiotics: Their role in non-alcoholic fatty liver disease. World J. Gastroenterol. 20, 15518–15531. doi: 10.3748/wjg.v20.i42.15518
Paone, P., Cani, P. D. (2020). Mucus barrier, mucins and gut microbiota: the expected slimy partners? Gut 69, 2232–2243. doi: 10.1136/gutjnl-2020–322260
Parséus, A., Sommer, N., Sommer, F., Caesar, R., Molinaro, A., Ståhlman, M., et al. (2017). Microbiota-induced obesity requires farnesoid X receptor. Gut 66, 429–437. doi: 10.1136/gutjnl-2015-310283
Perino, A., Demagny, H., Velazquez-Villegas, L., Schoonjans, K. (2021). Molecular physiology of bile acid signaling in health, disease, and aging. Physiol. Rev. 101, 683–731. doi: 10.1152/physrev.00049.2019
Perrier, C., Sprenger, N., Corthésy, B. (2006). Glycans on secretory component participate in innate protection against mucosal pathogens. J. Biol. Chem. 281, 14280–14287. doi: 10.1074/jbc.M512958200
Petersson, J., Schreiber, O., Hansson, G. C., Gendler, S. J., Velcich, A., Lundberg, J. O., et al. (2011). Importance and regulation of the colonic mucus barrier in a mouse model of colitis. Am. J. Physiology-Gastrointestinal Liver Physiol. 300, G327–G333. doi: 10.1152/ajpgi.00422.2010
Pierantonelli, I., Svegliati-Baroni, G. (2019). Nonalcoholic fatty liver disease: basic pathogenetic mechanisms in the progression from NAFLD to NASH. Transplantation 103, e1–e13. doi: 10.1097/TP.0000000000002480
Pope, J. L., Bhat, A. A., Sharma, A., Ahmad, R., Krishnan, M., Washington, M. K., et al. (2014). Claudin-1 regulates intestinal epithelial homeostasis through the modulation of Notch-signalling. Gut 63, 622–634. doi: 10.1136/gutjnl-2012-304241
Purchiaroni, F., Tortora, A., Gabrielli, M., Bertucci, F., Gigante, G., Ianiro, G., et al. (2013). The role of intestinal microbiota and the immune system. Eur. Rev. Med. Pharmacol. Sci. 17, 323–333.
Priyadarshini, M., Kotlo, K. U., Dudeja, P. K., Layden, B. T. (2018). “Role of short chain fatty acid receptors in intestinal physiology and pathophysiology”, in Compr. Physiol. Ed. Prakash, Y.S. (Wiley), 1091–1115. doi: 10.1002/cphy.c170050
Qi, L., Mao, H., Lu, X., Shi, T., Wang, J. (2022). Corrigendum: Cinnamaldehyde promotes the intestinal barrier functions and reshapes gut microbiome in early weaned rats. Front. Nutr. 9. doi: 10.3389/fnut.2022.1038451
Rescigno, M., Urbano, M., Valzasina, B., Francolini, M., Rotta, G., Bonasio, R., et al. (2001). Dendritic cells express tight junction proteins and penetrate gut epithelial monolayers to sample bacteria. Nat. Immunol. 2, 361–367. doi: 10.1038/86373
Rey, F. E., Faith, J. J., Bain, J., Muehlbauer, M. J., Stevens, R. D., Newgard, C. B., et al. (2010). Dissecting the in vivo metabolic potential of two human gut acetogens. J. Biol. Chem. 285, 22082–22090. doi: 10.1074/jbc.M110.117713
Richards, J. L., Yap, Y. A., McLeod, K. H., Mackay, C. R., Mariño, E. (2016). Dietary metabolites and the gut microbiota: an alternative approach to control inflammatory and autoimmune diseases. Clin. Transl. Immunol. 5, e82. doi: 10.1038/cti.2016.29
Ridlon, J. M., Kang, D. J., Hylemon, P. B., Bajaj, J. S. (2014). Bile acids and the gut microbiome. Curr. Opin. Gastroenterol. 30, 332–338. doi: 10.1097/MOG.0000000000000057
Salamone, D., Costabile, G., Corrado, A., Della Pepa, G., Vitale, M., Giacco, R., et al. (2022). Circulating short-chain fatty acids in type 2 diabetic patients and overweight/obese individuals. Acta Diabetol. 59, 1653–1656. doi: 10.1007/s00592-022-01934-8
Salzman, N. H., Hung, K., Haribhai, D., Chu, H., Karlsson-Sjöberg, J., Amir, E., et al. (2010). Enteric defensins are essential regulators of intestinal microbial ecology. Nat. Immunol. 11, 76–83. doi: 10.1038/ni.1825
Schroeder, B. O. (2019). Fight them or feed them: how the intestinal mucus layer manages the gut microbiota. Gastroenterol. Rep. 7, 3–12. doi: 10.1093/gastro/goy052
Schwiertz, A., Jacobi, M., Frick, J.-S., Richter, M., Rusch, K., Köhler, H. (2010). Microbiota in pediatric inflammatory bowel disease. J. Pediatr. 157, 240–244.e1. doi: 10.1016/j.jpeds.2010.02.046
Scott, K. P., Martin, J. C., Campbell, G., Mayer, C.-D., Flint, H. J. (2006). Whole-genome transcription profiling reveals genes up-regulated by growth on fucose in the human gut bacterium “Roseburia inulinivorans. J. Bacteriol. 188, 4340–4349. doi: 10.1128/JB.00137-06
Selma, M. V., Espín, J. C., Tomás-Barberán, F. A. (2009). Interaction between phenolics and gut microbiota: role in human health. J. Agric. Food Chem. 57, 6485–6501. doi: 10.1021/jf902107d
Shapiro, H., Kolodziejczyk, A. A., Halstuch, D., Elinav, E. (2018). Bile acids in glucose metabolism in health and disease. J. Exp. Med. 215, 383–396. doi: 10.1084/jem.20171965
Shi, N., Li, N., Duan, X., Niu, H. (2017). Interaction between the gut microbiome and mucosal immune system. Mil Med. Res. 4, 14. doi: 10.1186/s40779-017-0122-9
Shin, J., Noh, J.-R., Chang, D.-H., Kim, Y.-H., Kim, M. H., Lee, E. S., et al. (2019). Elucidation of akkermansia muciniphila probiotic traits driven by mucin depletion. Front. Microbiol. 10. doi: 10.3389/fmicb.2019.01137
Shukla, P. K., Meena, A. S., Rao, V., Rao, R. G., Balazs, L., Rao, R. (2018). Human defensin-5 blocks ethanol and colitis-induced dysbiosis, tight junction disruption and inflammation in mouse intestine. Sci. Rep. 8, 16241. doi: 10.1038/s41598–018-34263–4
Singh, V., Johnson, K., Yin, J., Lee, S., Lin, R., Yu, H., et al. (2022). Chronic inflammation in ulcerative colitis causes long-term changes in goblet cell function. Cell. Mol. Gastroenterol. Hepatol. 13, 219–232. doi: 10.1016/j.jcmgh.2021.08.010
Sircana, A., Framarin, L., Leone, N., Berrutti, M., Castellino, F., Parente, R., et al. (2018). Altered gut microbiota in type 2 diabetes: just a coincidence? Curr. Diab. Rep. 18, 98. doi: 10.1007/s11892-018-1057-6
Sivan, A., Corrales, L., Hubert, N., Williams, J. B., Aquino-Michaels, K., Earley, Z. M., et al. (2015). Commensal Bifidobacterium promotes antitumor immunity and facilitates anti-PD-L1 efficacy. Science 350, 1084–1089. doi: 10.1126/science.aac4255
Sonnenburg, E. D., Sonnenburg, J. L. (2014). Starving our microbial self: the deleterious consequences of a diet deficient in microbiota-accessible carbohydrates. Cell Metab. 20, 779–786. doi: 10.1016/j.cmet.2014.07.003
Statovci, D., Aguilera, M., MacSharry, J., Melgar, S. (2017). The impact of western diet and nutrients on the microbiota and immune response at mucosal interfaces. Front. Immunol. 8. doi: 10.3389/fimmu.2017.00838
Stoddart, L. A., Smith, N. J., Milligan, G. (2008). International Union of Pharmacology. LXXI. Free fatty acid receptors FFA1, -2, and -3: pharmacology and pathophysiological functions. Pharmacol. Rev. 60, 405–417. doi: 10.1124/pr.108.00802
Su, H., Mo, J., Ni, J., Ke, H., Bao, T., Xie, J., et al. (2020). Andrographolide Exerts Antihyperglycemic Effect through Strengthening Intestinal Barrier Function and Increasing Microbial Composition of Akkermansia muciniphila. Oxid. Med. Cell Longev 2020, 6538930. doi: 10.1155/2020/6538930
Sun, Z., Li, J., Wang, W., Liu, Y., Liu, J., Jiang, H., et al. (2021c). Qingchang wenzhong decoction accelerates intestinal mucosal healing through modulation of dysregulated gut microbiome, intestinal barrier and immune responses in mice. Front. Pharmacol. 12. doi: 10.3389/fphar.2021.738152
Sun, S., Yang, Y., Lin, X., Chen, P., Ye, L., Zeng, L., et al. (2021b). Qiweibaizhu decoction treats diarrheal juvenile rats by modulating the gut microbiota, short-chain fatty acids, and the mucus barrier. Evidence-Based Complementary Altern. Medicine : eCAM 2021, 1–15. doi: 10.1155/2021/8873294
Sun, J.-N., Yu, X.-Y., Hou, B., Ai, M., Qi, M.-T., Ma, X.-Y., et al. (2021a). Vaccarin enhances intestinal barrier function in type 2 diabetic mice. Eur. J. Pharmacol. 908, 174375. doi: 10.1016/j.ejphar.2021.174375
Suriano, F., Nyström, E. E. L., Sergi, D., Gustafsson, J. K. (2022). Diet, microbiota, and the mucus layer: The guardians of our health. Front. Immunol. 13. doi: 10.3389/fimmu.2022.953196
Suzuki, T. (2020). Regulation of the intestinal barrier by nutrients: The role of tight junctions. Anim. Sci. J. 91, e13357. doi: 10.1111/asj.13357
Szentkuti, L., Riedesel, H., Enss, M. L., Gaertner, K., Von Engelhardt, W. (1990). Pre-epithelial mucus layer in the colon of conventional and germ-free rats. Histochem J. 22, 491–497. doi: 10.1007/BF01007234
Takiishi, T., Fenero, C. I. M., Câmara, N. O. S. (2017). Intestinal barrier and gut microbiota: Shaping our immune responses throughout life. Tissue Barriers 5, e1373208. doi: 10.1080/21688370.2017.1373208
Tezuka, H., Ohteki, T. (2019). Regulation of igA production by intestinal dendritic cells and related cells. Front. Immunol. 10. doi: 10.3389/fimmu.2019.01891
Tian, B., Geng, Y., Wang, P., Cai, M., Neng, J., Hu, J., et al. (2022). Ferulic acid improves intestinal barrier function through altering gut microbiota composition in high-fat diet-induced mice. Eur. J. Nutr. 61, 3767–3783. doi: 10.1007/s00394-022-02927-7
Tian, B., Zhao, J., Zhang, M., Chen, Z., Ma, Q., Liu, H., et al. (2021). Lycium ruthenicum anthocyanins attenuate high-fat diet-induced colonic barrier dysfunction and inflammation in mice by modulating the gut microbiota. Mol. Nutr. Food Res. 65, e2000745. doi: 10.1002/mnfr.202000745
Tolhurst, G., Heffron, H., Lam, Y. S., Parker, H. E., Habib, A. M., Diakogiannaki, E., et al. (2012). Short-chain fatty acids stimulate glucagon-like peptide-1 secretion via the G-protein-coupled receptor FFAR2. Diabetes 61, 364–371. doi: 10.2337/db11-1019
Tomasetto, C., Masson, R., Linares, J. L., Wendling, C., Lefebvre, O., Chenard, M. P., et al. (2000). pS2/TFF1 interacts directly with the VWFC cysteine-rich domains of mucins. Gastroenterology 118, 70–80. doi: 10.1016/S0016-5085(00)70415-X
Tropini, C., Moss, E. L., Merrill, B. D., Ng, K. M., Higginbottom, S. K., Casavant, E. P., et al. (2018). Transient osmotic perturbation causes long-term alteration to the gut microbiota. Cell 173, 1742–1754.e17. doi: 10.1016/j.cell.2018.05.008
Turner, J. R. (2009). Intestinal mucosal barrier function in health and disease. Nat. Rev. Immunol. 9, 799–809. doi: 10.1038/nri2653
Turroni, F., Marchesi, J. R., Foroni, E., Gueimonde, M., Shanahan, F., Margolles, A., et al. (2009). Microbiomic analysis of the bifidobacterial population in the human distal gut. ISME J. 3, 745–751. doi: 10.1038/ismej.2009.19
Ungaro, R., Mehandru, S., Allen, P. B., Peyrin-Biroulet, L., Colombel, J.-F. (2017). Ulcerative colitis. Lancet 389, 1756–1770. doi: 10.1016/S0140-6736(16)32126-2
Vaishnava, S., Yamamoto, M., Severson, K. M., Ruhn, K. A., Yu, X., Koren, O., et al. (2011). The antibacterial lectin RegIIIgamma promotes the spatial segregation of microbiota and host in the intestine. Science 334, 255–258. doi: 10.1126/science.1209791
Van der Sluis, M., De Koning, B. A. E., De Bruijn, A. C. J. M., Velcich, A., Meijerink, J. P. P., Van Goudoever, J. B., et al. (2006). Muc2-deficient mice spontaneously develop colitis, indicating that MUC2 is critical for colonic protection. Gastroenterology 131, 117–129. doi: 10.1053/j.gastro.2006.04.020
Van Itallie, C. M., Anderson, J. M. (2006). Claudins and epithelial paracellular transport. Annu. Rev. Physiol. 68, 403–429. doi: 10.1146/annurev.physiol.68.040104.131404
Vollaard, E. J., Clasener, H. A. (1994). Colonization resistance. Antimicrob. Agents Chemother. 38, 409–414. doi: 10.1128/AAC.38.3.409
Volynets, V., Spruss, A., Kanuri, G., Wagnerberger, S., Bischoff, S. C., Bergheim, I. (2010). Protective effect of bile acids on the onset of fructose-induced hepatic steatosis in mice. J. Lipid Res. 51, 3414–3424. doi: 10.1194/jlr.M007179
Walker, A. W., Duncan, S. H., McWilliam Leitch, E. C., Child, M. W., Flint, H. J. (2005). pH and peptide supply can radically alter bacterial populations and short-chain fatty acid ratios within microbial communities from the human colon. Appl. Environ. Microbiol. 71, 3692–3700. doi: 10.1128/AEM.71.7.3692-3700.2005
Wang, K., Bao, L., Zhou, N., Zhang, J., Liao, M., Zheng, Z., et al. (2018). Structural modification of natural product ganomycin I leading to discovery of a α-glucosidase and HMG-coA reductase dual inhibitor improving obesity and metabolic dysfunction in vivo. J. Med. Chem. 61, 3609–3625. doi: 10.1021/acs.jmedchem.8b00107
Wang, L.-L., Guo, H.-H., Huang, S., Feng, C.-L., Han, Y.-X., Jiang, J.-D. (2017a). Comprehensive evaluation of SCFA production in the intestinal bacteria regulated by berberine using gas-chromatography combined with polymerase chain reaction. J. Chromatogr B Analyt Technol. BioMed. Life Sci. 1057, 70–80. doi: 10.1016/j.jchromb.2017.05.004
Wang, X., Huang, S., Zhang, M., Su, Y., Pan, Z., Liang, J., et al. (2023). Gegen Qinlian decoction activates AhR/IL-22 to repair intestinal barrier by modulating gut microbiota-related tryptophan metabolism in ulcerative colitis mice. J. Ethnopharmacol. 302, 115919. doi: 10.1016/j.jep.2022.115919
Wang, M.-X., Lin, L., Chen, Y.-D., Zhong, Y.-P., Lin, Y.-X., Li, P., et al. (2020a). Evodiamine has therapeutic efficacy in ulcerative colitis by increasing Lactobacillus acidophilus levels and acetate production. Pharmacol. Res. 159, 104978. doi: 10.1016/j.phrs.2020.104978
Wang, Y., Shou, J.-W., Li, X.-Y., Zhao, Z.-X., Fu, J., He, C.-Y., et al. (2017b). Berberine-induced bioactive metabolites of the gut microbiota improve energy metabolism. Metabolism 70, 72–84. doi: 10.1016/j.metabol.2017.02.003
Wang, H., Yin, H., Zhong, Y., Hu, J., Xia, S., Wang, Z., et al. (2022a). Polysaccharides from fermented coix seed modulates circulating nitrogen and immune function by altering gut microbiota. Curr. Res. Food Sci. 5, 1994–2003. doi: 10.1016/j.crfs.2022.10.007
Wang, H., Zhang, H., Gao, Z., Zhang, Q., Gu, C. (2022b). The mechanism of berberine alleviating metabolic disorder based on gut microbiome. Front. Cell Infect. Microbiol. 12. doi: 10.3389/fcimb.2022.854885
Wang, L., Zhang, P., Li, C., Xu, F., Chen, J. (2022c). A polysaccharide from Rosa roxburghii Tratt fruit attenuates high-fat diet-induced intestinal barrier dysfunction and inflammation in mice by modulating the gut microbiota. Food Funct. 13, 530–547. doi: 10.1039/d1fo03190b
Wang, Y., Zhang, X., Li, J., Zhang, Y., Guo, Y., Chang, Q., et al. (2021). Sini decoction ameliorates colorectal cancer and modulates the composition of gut microbiota in mice. Front. Pharmacol. 12. doi: 10.3389/fphar.2021.609992
Wang, Z., Zhang, X., Zhu, L., Yang, X., He, F., Wang, T., et al. (2020b). Inulin alleviates inflammation of alcoholic liver disease via SCFAs-inducing suppression of M1 and facilitation of M2 macrophages in mice. Int. Immunopharmacol. 78, 106062. doi: 10.1016/j.intimp.2019.106062
Wang, Y., Zhu, X., Liang, Y., Li, X., Wang, Y., Li, J. (2022d). Sishen wan treats ulcerative colitis in rats by regulating gut microbiota and restoring the treg/th17 balance. Evid Based Complement Alternat Med. 2022, 1432816. doi: 10.1155/2022/1432816
Wei, X., Tao, J., Xiao, S., Jiang, S., Shang, E., Zhu, Z., et al. (2018). Xiexin Tang improves the symptom of type 2 diabetic rats by modulation of the gut microbiota. Sci. Rep. 8, 3685–3696. doi: 10.1038/s41598–018-22094–2
Wen, J., Teng, B., Yang, P., Chen, X., Li, C., Jing, Y., et al. (2016). The potential mechanism of Bawei Xileisan in the treatment of dextran sulfate sodium-induced ulcerative colitis in mice. J. Ethnopharmacol. 188, 31–38. doi: 10.1016/j.jep.2016.04.054
Weström, B., Arévalo Sureda, E., Pierzynowska, K., Pierzynowski, S. G., Pérez-Cano, F.-J. (2020). The immature gut barrier and its importance in establishing immunity in newborn mammals. Front. Immunol. 11. doi: 10.3389/fimmu.2020.01153
Wilson, I. D., Nicholson, J. K. (2017). Gut microbiome interactions with drug metabolism, efficacy, and toxicity. Transl. Res. 179, 204–222. doi: 10.1016/j.trsl.2016.08.002
Wu, D., Ding, L., Tang, X., Wang, W., Chen, Y., Zhang, T. (2019a). Baicalin protects against hypertension-associated intestinal barrier impairment in part through enhanced microbial production of short-chain fatty acids. Front. Pharmacol. 10. doi: 10.3389/fphar.2019.01271
Wu, Y., Li, Y., Ruan, Z., Li, J., Zhang, L., Lu, H., et al. (2020). Puerarin rebuilding the mucus layer and regulating mucin-utilizing bacteria to relieve ulcerative colitis. J. Agric. Food Chem. 68, 11402–11411. doi: 10.1021/acs.jafc.0c04119
Wu, T.-R., Lin, C.-S., Chang, C.-J., Lin, T.-L., Martel, J., Ko, Y.-F., et al. (2019b). Gut commensal Parabacteroides goldsteinii plays a predominant role in the anti-obesity effects of polysaccharides isolated from Hirsutella sinensis. Gut 68, 248–262. doi: 10.1136/gutjnl-2017–315458
Wu, T., Liu, T., Xing, L., Ji, G. (2018). Baicalin and puerarin reverse epithelial-mesenchymal transition via the TGF-β1/Smad3 pathway in vitro. Exp. Ther. Med. 16, 1968–1974. doi: 10.3892/etm.2018.6400
Xia, W., Liu, B., Tang, S., Yasir, M., Khan, I. (2022). The science behind TCM and Gut microbiota interaction—their combinatorial approach holds promising therapeutic applications. Front. Cell. Infect. Microbiol. 12. doi: 10.3389/fcimb.2022.875513
Xiao, Y., Zhao, J., Zhang, H., Zhai, Q., Chen, W. (2020). Mining Lactobacillus and Bifidobacterium for organisms with long-term gut colonization potential. Clin. Nutr. 39, 1315–1323. doi: 10.1016/j.clnu.2019.05.014
Xie, M., Zhang, L., Li, L., Fan, M., Hou, L. (2020). MiR-339 attenuates LPS-induced intestinal epithelial cells inflammatory responses and apoptosis by targeting TLR4. Genes Genomics 42, 1097–1105. doi: 10.1007/s13258-020-00977-x
Xing, J., Chen, X., Zhong, D. (2005). Absorption and enterohepatic circulation of baicalin in rats. Life Sci. 78, 140–146. doi: 10.1016/j.lfs.2005.04.072
Xu, H., Wang, S., Jiang, Y., Wu, J., Chen, L., Ding, Y., et al. (2023). Poria cocos Polysaccharide Ameliorated Antibiotic-Associated Diarrhea in Mice via Regulating the Homeostasis of the Gut Microbiota and Intestinal Mucosal Barrier. Int. J. Mol. Sci. 24, 1423–1443. doi: 10.3390/ijms24021423
Xu, S., Wang, Y., Wang, J., Geng, W. (2022). Kombucha reduces hyperglycemia in type 2 diabetes of mice by regulating gut microbiota and its metabolites. Foods 11, 754. doi: 10.3390/foods11050754
Xu, X., Xu, P., Ma, C., Tang, J., Zhang, X. (2013). Gut microbiota, host health, and polysaccharides. Biotechnol. Adv. 31, 318–337. doi: 10.1016/j.biotechadv.2012.12.009
Yan, B.-F., Chen, X., Chen, Y.-F., Liu, S.-J., Xu, C.-X., Chen, L., et al. (2022). Aqueous extract of Paeoniae Radix Alba (Paeonia lactiflora Pall.) ameliorates DSS-induced colitis in mice by tunning the intestinal physical barrier, immune responses, and microbiota. J. Infect. 294, 115365. doi: 10.1016/j.jep.2022.115365
Yan, H., Lu, J., Wang, Y., Gu, W., Yang, X., Yu, J. (2017). Intake of total saponins and polysaccharides from Polygonatum kingianum affects the gut microbiota in diabetic rats. Phytomedicine 26, 45–54. doi: 10.1016/j.phymed.2017.01.007
Yang, Y., Chen, G., Yang, Q., Ye, J., Cai, X., Tsering, P., et al. (2017). Gut microbiota drives the attenuation of dextran sulphate sodium-induced colitis by Huangqin decoction. Oncotarget 8, 48863–48874. doi: 10.18632/oncotarget.v8i30
Yang, C., Du, Y., Ren, D., Yang, X., Zhao, Y. (2021a). Gut microbiota-dependent catabolites of tryptophan play a predominant role in the protective effects of turmeric polysaccharides against DSS-induced ulcerative colitis. Food Funct. 12, 9793–9807. doi: 10.1039/d1fo01468d
Yang, H., Feng, L., Xu, L., Jiang, D., Zhai, F., Tong, G., et al. (2022a). Intervention of shugan xiaozhi decoction on nonalcoholic fatty liver disease via mediating gut-liver axis. BioMed. Res. Int. 2022, 4801695. doi: 10.1155/2022/4801695
Yang, S.-B., Qin, Y.-J., Ma, X., Luan, W.-M., Sun, P., Ju, A.-Q., et al. (2021b). Effects of in ovo injection of astragalus polysaccharide on the intestinal development and mucosal immunity in broiler chickens. Front. Vet. Sci. 8. doi: 10.3389/fvets.2021.738816
Yang, W., Ren, D., Zhao, Y., Liu, L., Yang, X. (2021c). Fuzhuan brick tea polysaccharide improved ulcerative colitis in association with gut microbiota-derived tryptophan metabolism. J. Agric. Food Chem. 69, 8448–8459. doi: 10.1021/acs.jafc.1c02774
Yang, Y., Zhou, Z., Liu, Y., Xu, X., Xu, Y., Zhou, W., et al. (2022b). Non-alcoholic components in huangjiu as potential factors regulating the intestinal barrier and gut microbiota in mouse model of alcoholic liver injury. Foods 11, 1537–1558. doi: 10.3390/foods11111537
Ye, H., Ma, S., Qiu, Z., Huang, S., Deng, G., Li, Y., et al. (2022). Poria cocos polysaccharides rescue pyroptosis-driven gut vascular barrier disruption in order to alleviates non-alcoholic steatohepatitis. J. Ethnopharmacol. 296, 115457. doi: 10.1016/j.jep.2022.115457
Yin, L., Huang, G., Khan, I., Su, L., Xia, W., Law, B. Y. K., et al. (2022). Poria cocos polysaccharides exert prebiotic function to attenuate the adverse effects and improve the therapeutic outcome of 5-FU in ApcMin/+ mice. Chin. Med. 17, 116. doi: 10.1186/s13020-022-00667-8
Yin, J., Song, Y., Hu, Y., Wang, Y., Zhang, B., Wang, J., et al. (2021). Dose-dependent beneficial effects of tryptophan and its derived metabolites on akkermansia in vitro: A preliminary prospective study. Microorganisms 9, 1511–1524. doi: 10.3390/microorganisms9071511
Yoo, J. Y., Groer, M., Dutra, S. V. O., Sarkar, A., McSkimming, D. I. (2020). Gut microbiota and immune system interactions. Microorganisms 8, 1587–1607. doi: 10.3390/microorganisms8101587
Yue, S. J., Xin, L. T., Fan, Y. C., Li, S. J., Tang, Y. P., Duan, J. A., et al. (2017). Herb pair Danggui-Honghua: mechanisms underlying blood stasis syndrome by system pharmacology approach. Sci. Rep. 7, 40318. doi: 10.1038/srep40318
Zeng, X., Zhang, X., Su, H., Gou, H., Lau, H. C.-H., Hu, X., et al. (2024). Pien tze huang protects against non-Alcoholic steatohepatitis by modulating the gut microbiota and metabolites in mice. Engineering 35, 257–269. doi: 10.1016/j.eng.2022.10.010
Zhang, X.-Y., Chen, J., Yi, K., Peng, L., Xie, J., Gou, X., et al. (2020b). Phlorizin ameliorates obesity-associated endotoxemia and insulin resistance in high-fat diet-fed mice by targeting the gut microbiota and intestinal barrier integrity. Gut Microbes 12, 1–18. doi: 10.1080/19490976.2020.1842990
Zhang, W., Cheng, C., Han, Q., Chen, Y., Guo, J., Wu, Q., et al. (2019). Flos Abelmoschus manihot extract attenuates DSS-induced colitis by regulating gut microbiota and Th17/Treg balance. BioMed. Pharmacother. 117, 109162. doi: 10.1016/j.biopha.2019.109162
Zhang, Y.-Z., Li, Y.-Y. (2014). Inflammatory bowel disease: pathogenesis. World J. Gastroenterol. 20, 91–99. doi: 10.3748/wjg.v20.i1.91
Zhang, D., Liu, J., Cheng, H., Wang, H., Tan, Y., Feng, W., et al. (2022b). Interactions between polysaccharides and gut microbiota: A metabolomic and microbial review. Food Res. Int. 160, 111653. doi: 10.1016/j.foodres.2022.111653
Zhang, Y., Liu, X., Wang, Y., Jiang, P., Quek, S. (2016). Antibacterial activity and mechanism of cinnamon essential oil against Escherichia coli and Staphylococcus aureus. Food Control 59, 282–289. doi: 10.1016/j.foodcont.2015.05.032
Zhang, B., Liu, K., Yang, H., Jin, Z., Ding, Q., Zhao, L. (2022a). Gut microbiota: the potential key target of TCM’s therapeutic effect of treating different diseases using the same method—UC and T2DM as examples. Front. Cell. Infect. Microbiol. 12. doi: 10.3389/fcimb.2022.855075
Zhang, Y., Tang, K., Deng, Y., Chen, R., Liang, S., Xie, H., et al. (2018b). Effects of shenling baizhu powder herbal formula on intestinal microbiota in high-fat diet-induced NAFLD rats. BioMed. Pharmacother. 102, 1025–1036. doi: 10.1016/j.biopha.2018.03.158
Zhang, C.-E., Yu, X.-H., Cui, Y.-T., Wang, H.-J., Chen, X., Ma, X.-J., et al. (2023). Shengjiang Xiexin Decoction ameliorates antibiotic-associated diarrhea by altering the gut microbiota and intestinal metabolic homeostasis. Phytomedicine 113, 154737. doi: 10.1016/j.phymed.2023.154737
Zhang, B., Yue, R., Chen, Y., Huang, X., Yang, M., Shui, J., et al. (2020a). The herbal medicine scutellaria-coptis alleviates intestinal mucosal barrier damage in diabetic rats by inhibiting inflammation and modulating the gut microbiota. Evid Based Complement Alternat Med. 2020, 4568629. doi: 10.1155/2020/4568629
Zhang, X., Zhao, S., Song, X., Jia, J., Zhang, Z., Zhou, H., et al. (2018a). Inhibition effect of glycyrrhiza polysaccharide (GCP) on tumor growth through regulation of the gut microbiota composition. J. Pharmacol. Sci. 137, 324–332. doi: 10.1016/j.jphs.2018.03.006
Zhang, S., Zhao, J., Xie, F., He, H., Johnston, L. J., Dai, X., et al. (2021). Dietary fiber-derived short-chain fatty acids: A potential therapeutic target to alleviate obesity-related nonalcoholic fatty liver disease. Obes. Rev. 22, e13316. doi: 10.1111/obr.13316
Zhao, Y., Chen, F., Wu, W., Sun, M., Bilotta, A. J., Yao, S., et al. (2018). GPR43 mediates microbiota metabolite SCFA regulation of antimicrobial peptide expression in intestinal epithelial cells via activation of mTOR and STAT3. Mucosal Immunol. 11, 752–762. doi: 10.1038/mi.2017.118
Keywords: gut barrier, gut microbiota, gut microbiota metabolite, traditional Chinese medicine, intestinal tract integrity
Citation: Zhou Y, Zhang D, Cheng H, Wu J, Liu J, Feng W and Peng C (2024) Repairing gut barrier by traditional Chinese medicine: roles of gut microbiota. Front. Cell. Infect. Microbiol. 14:1389925. doi: 10.3389/fcimb.2024.1389925
Received: 22 February 2024; Accepted: 14 June 2024;
Published: 04 July 2024.
Edited by:
Caiyun Yu, Nanjing Agricultural University, ChinaCopyright © 2024 Zhou, Zhang, Cheng, Wu, Liu, Feng and Peng. This is an open-access article distributed under the terms of the Creative Commons Attribution License (CC BY). The use, distribution or reproduction in other forums is permitted, provided the original author(s) and the copyright owner(s) are credited and that the original publication in this journal is cited, in accordance with accepted academic practice. No use, distribution or reproduction is permitted which does not comply with these terms.
*Correspondence: Juan Liu, bGl1anVhbnBlMjEyQDEyNi5jb20=; Wuwen Feng, amlhb3hpYWtlLTFAZm94bWFpbC5jb20=; Cheng Peng, cGVuZ2NoZW5nY3h5QDEyNi5jb20=
Disclaimer: All claims expressed in this article are solely those of the authors and do not necessarily represent those of their affiliated organizations, or those of the publisher, the editors and the reviewers. Any product that may be evaluated in this article or claim that may be made by its manufacturer is not guaranteed or endorsed by the publisher.
Research integrity at Frontiers
Learn more about the work of our research integrity team to safeguard the quality of each article we publish.