- Department of Traditional Chinese Medicine, Shanghai Fourth People’s Hospital Affiliated to Tongji University, Shanghai, China
Breast milk is an essential source of infant nutrition. It is also a vital determinant of the structure and function of the infant intestinal microbial community, and it connects the mother and infant intestinal microbiota. Human milk oligosaccharides (HMOs) are a critical component in breast milk. HMOs can reach the baby’s colon entirely from milk and become a fermentable substrate for some intestinal microorganisms. HMOs can enhance intestinal mucosal barrier function and affect the intestinal function of the host through immune function, which has a therapeutic effect on specific infant intestinal diseases, such as necrotizing enterocolitis. In addition, changes in infant intestinal microbiota can reflect the maternal intestinal microbiota. HMOs are a link between the maternal intestinal microbiota and infant intestinal microbiota. HMOs affect the intestinal microbiota of infants and are related to the maternal milk microbiota. Through breastfeeding, maternal microbiota and HMOs jointly affect infant intestinal bacteria. Therefore, HMOs positively influence the establishment and balance of the infant microbial community, which is vital to ensure infant intestinal function. Therefore, HMOs can be used as a supplement and alternative therapy for infant intestinal diseases.
1 Introduction
The disorder of intestinal flora in early life will seriously affect the health of infants and lead to susceptibility to diseases in later life (Canakis et al., 2020; Underwood et al., 2020). The intestine is the organ with the richest source of symbiotic microbiota in the human body. The symbiotic bacteria residing in the colon account for approximately 70% of all bacteria in the human body, and these bacteria include mainly Bacteroides, Firmicutes, Actinomycetes and Proteobacteria (Kohl et al., 2018). Living habits, disease factors and dietary factors regulate and affect the composition of the intestinal microbiota (Pickard et al., 2017). Changes in the intestinal microbiota can cause infection, regulate intestinal permeability and intestinal movement, and thus affect host health. The intestinal microbiota can also affect lipid, glucose, and bone metabolism, which are related to conditions such as atherosclerosis, obesity, diabetes and osteoporosis (Patterson et al., 2016; Chen et al., 2021b). The colonization of infant intestinal microbiota is influenced by breast milk, subsequently impacting the development of food allergies in infants (Cukrowska et al., 2020; Wang et al., 2021). Additionally, intestinal microbiota have the capacity to regulate neuroinflammatory responses and mitigate brain injury following neonatal hypoxia and ischemia (Drobyshevsky et al., 2024). Some studies suggest that it is vital to treat these diseases by adjusting the intestinal microbiota and rebuilding the intestinal microenvironment by improving the microbial community structure (Kim et al., 2019; Zhou et al., 2020; Boggio Marzet et al., 2022).
Breast milk is an essential source of infant nutrition and a vital determinant of the structure and function of the intestinal microbial community (Zmora et al., 2019). Human milk oligosaccharides (HMOs) are a crucial component of breast milk (Thum et al., 2021; Poulsen et al., 2022). HMOs can enrich beneficial microbiota, reduce the abundance of pathogenic bacteria, and affect the health status of newborns (Triantis et al., 2018; Sakanaka et al., 2019b; Quinn et al., 2020). HMOs resist human enzymes in the gastrointestinal tract and can reach the infant colon intact and become a fermentable substrate for some intestinal microorganisms (Salli et al., 2019). For example, HMOs could enrich infant gut bifidobacteria and have an antipathogenic effect against Staphylococcus aureus (Thomson et al., 2018; Yue et al., 2020). Therefore, HMOs positively affect the establishment and balance of the microbial community, which is vital to ensure normal gastrointestinal function (Strandwitz, 2018). At present, infant formula and food for special medical purposes containing 2’-fucosyl lactose (2’-FL), 3-fucosyl lactose (3-FL), 3-sialyl lactose (3’SL), 6-sialyl lactose (6’SL) and lacto-N- tetrasaccharide (LNT) has been approved by the European Union, the EFSA Panel on Nutrition, Novel Foods and Food Allergens (NDA) (Turck et al., 2022; Turck et al., 2023). The US Food and Drug Administration has evaluated the safety and regulatory of HMOs used in infant formula (Morissette et al., 2023). Meanwhile, HMOs is effective in preventing and treating necrotizing enterocolitis (NEC) (Wang et al., 2024). With the exploration of HMOs, some clinical trials of HMOs on irritable bowel syndrome and other diseases are in progress (Iribarren et al., 2020; Iribarren et al., 2021).
There is a correlation between maternal intestinal and milk microbiota. Some studies have suggested that maternal intestinal microbiota can be transported to milk through the intestinal-mammary route. The baby orally consumes the breastmilk, and microbiota reach spread vertically to the intestine (Zhong et al., 2022). In the first four months after birth, the intestinal flora undergoes significant changes due to the influence of breast milk (Vélez-Ixta et al., 2024). Both 2’-FL and LNnT promote the colonization of Bifidobacterium in infants’ intestines (Berger et al., 2020). Fermentation metabolites derived from HMOs serve not only as substrates for bacterial cross-feeding and as mediators of microbial-host interactions but also possess antibacterial properties (Huertas-Díaz et al., 2023). Recent studies have identified a correlation between HMOs and milk microbiota, potentially linked to the extent of HMOs utilization by microorganisms in the milk (Cheema et al., 2022). Furthermore, the secretor/non-secretor status influences HMOs production, affecting the composition of human milk microbiota and the colonization of infant intestinal flora (Soyyılmaz et al., 2021).
Therefore, this review will describe the structure, influencing factors, and utilization pathways of HMOs, exploring the relationship between maternal gut microbiota and HMOs, with particular emphasis on how HMOs establish a stable infant gut microbiome through breastfeeding. Finally, we will elucidate the extensive potential of various HMOs in regulating microbiota-related diseases in infants based on their current clinical applications.
2 Structure of HMOs
HMOs are the third largest solid component of breast milk, following lactose and fat (Thum et al., 2021). The colostrum has a high level of HMOs, and the concentration is 6-15 g/L within one month after delivery. Its concentration decreases with increasing lactation time, and it is 4-6 g/L after 6 months (Thum et al., 2021; Poulsen et al., 2022). HMOs are not digested in the intestines of infants but have essential biological functions. HMOs are considered a bioactive component because of their health benefits to infants (Reverri et al., 2018).
More than 200 HMOs with different structures have been isolated (Peterson and Nagy, 2021). Monosaccharide composition, polymerization rate, charge and acetylation lead to other HMO structures.The oligosaccharides are composed of 3-10 monosaccharides linked by glycosidic bonds to form a straight or branched chain. HMOs are composed of nucleotide sugar molecules linked by glycosidic bonds, and their core structure includes D-glucose (Glc), D-galactose (Gal) and N-acetylglucosamine (GlcNAc), which are further modified by fucose (Fuc) and sialic acid (NeuAc) (Wiciński et al., 2020). HMOs are based on the main chain of lactose (Gal β 1-4LC) as the reducing end, which can be connected by β1-3 or β1-6 and further extended by two disaccharide lactones -N- disaccharide (LNB) or N-acetyllactosamine (Neu5Ac). The backbone structures of complex HMOs generally contain single or repetitive lacto-N-biose (type 1) or lactosamine (type 2) units in either linear or branched chains extending from a lactose core (Hanisch and Kunz, 2021; Yao et al., 2024).
According to the glycosidic bonds of different sugar units, they are mainly divided into fucosylated HMOs, sialylated HMOs, and N- acetylated HMOs (Samuel et al., 2019). Fucosylated HMOs contain 2’-fucosyl lactose (2’-FL), 3-fucosyl lactose (3-FL), lacto-N-fucosyl lactose I (LNFP I), lacto-N-fucosyl lactose II (LNFP II), lacto-N-fucosyl lactose III (LNFP III), etc. Salicylated HMOs include 3-sialyl lactose (3’SL), 6-sialyl lactose (6’SL), disialyllacto-N- tetrasaccharide (DSLNT) and sialyllacto-N- tetrasaccharide (LST), etc. N- acetylated HMOs have lacto-N- tetrasaccharide (LNT), lacto-N-neotetrasaccharide (LNnT), lacto-N- hexaose (LNH) (Figure 1). 2’-FL is the most abundant oligosaccharide in breast milk, reaching 4.1 g/L, and is followed by LNT, LNFP I and 3-FL (Thum et al., 2021). The average concentrations of 2’-FL, 3-FL and LNFP I in breast milk are 0.14-2.74 g/L (Thurl et al., 2017), those of LNFP II and LNFP III are 0.21-0.58 g/L (Thurl et al., 2017), that of LNnT is 0.36-1.12 g/L (Ma et al., 2018), that of 3’-SL is 0.19-0.29 g/L (Gidrewicz and Fenton, 2014), and that of DS-LNT is 0.50-0.77 g/L (Van Niekerk et al., 2014). Thurl et al. and Xu et al. found that the ratio between HMOs changed during lactation (Thurl et al., 2017; Xu et al., 2017).
3 Factors affecting HMOs
3.1 Genetic expression
Genes influence HMO production. The fucosylation of HMOs depends on specific glycosyltransferases in breast cells, including α (Canakis et al., 2020; Underwood et al., 2020)-fucosyltransferase (FUT2) and α1-3/4- fucosyltransferase (FUT3) (Smilowitz et al., 2014; Ewald and Sumner, 2016). The secretion gene (Se) encodes FUT2, and the Lewis blood group gene (Le) encodes the enzyme FUT3 (Soyyılmaz et al., 2021). The difference in gene expression leads to four genetic expression patterns: Se+Le+, Se-Le+, Se+Le- and Se-Le- (Figure 1). Glycosylation of the HMO fucoidan is affected by FUT2 and FUT3. Women lacking FUT2 cannot produce 2’-FL or LNFP I (Kunz et al., 2017), and women without FUT3 cannot produce 3’-FL and LNFP II (Thurl et al., 1997). HMO production is regulated by glycosyltransferases in breast cells and is influenced by monosaccharide substrates. These factors may be related to the mother’s physiology, thus slightly affecting the glycosylation process in the initial stage of lactation (Samuel et al., 2019).
3.2 The length of lactation
HMO composition is related to the length of lactation (Thurl et al., 2017). The total HMO content in breast milk within one month after delivery is higher than that six months after delivery (Thum et al., 2021; Poulsen et al., 2022). A meta-analysis revealed that the proportion of 6’-SL, LNT and LNnT decreased gradually during lactation, and the proportion of 3’-FL increased gradually during lactation (Zhou et al., 2021).
3.3 Maternal diet
The HMO concentration is related to maternal diet. Some studies have shown that different maternal dietary carbohydrates and energy sources have priority in the alterations of HMOs, including fucosylated HMOs (Seferovic et al., 2020). Quin et al. showed that maternal fruit intake was positively correlated with increased HMO absolute abundance (Quin et al., 2020). A cross-sectional study of a cohort of 101 healthy mothers indicated that the diet of secretor mothers had a more significant impact on HMOs than that of nonsecretor mothers. Soluble and insoluble polyphenols and fibers and several insoluble polysaccharides are related to the HMO spectrum of secretors (Selma-Royo et al., 2022).
3.4 Maternal body mass index
The HMO concentration is related to the maternal body mass index. A systematic review showed that the content of 2’-FL is increased in mothers with a high body mass index before pregnancy, which is also related to the baby’s weight. The level of sialylated HMOs is higher after premature delivery (Han et al., 2021). Studies have evaluated the maternal HMO content, infant HMO intake, anthropometric measures and body composition in February and June after delivery. The results show that maternal obesity is related to a low concentration of fucoidan and sialylated HMOs, and a change in HMO concentration is related to infant obesity (Saben et al., 2021). Larsson and others propose that maternal body mass index is negatively correlated with 6-SL and positively correlated with 2’-FL and total HMOs (Larsson et al., 2019). Samuel et al. showed that 3′ SL, DSLNT, and 6′ SL levels were higher in low-weight mothers, but the concentrations of LNnT and LNT were lower (Samuel et al., 2019).
3.5 Delivery mode and number of deliveries
The concentration of HMOs may also be related to the delivery mode and number of deliveries (Azad et al., 2018; Wang et al., 2020). A study included 290 milk samples from healthy mothers from 7 European countries. It was observed that the concentrations of LNT and 6’-SL of mothers who delivered by caesarean section were higher than those of mothers who delivered vaginally. In comparison, the concentrations of 2’-FL and 3’-SL were lower (Samuel et al., 2019). However, studies have shown no correlation between maternal delivery mode and HMOs (Azad et al., 2018). A study has shown that there are more HMOs in primiparous mothers, among which the concentrations of DSLNT and LNnT are significantly higher, and the total HMO concentration negatively correlates with time (Van Niekerk et al., 2014; Samuel et al., 2019). However, Azad et al. noted that parity increased the total HMO concentration (Azad et al., 2018). Additionally, the concentration of HMOs may be affected by whether the infant was preterm or full-term. However, there is no definite conclusion (Samuel et al., 2019; Jackson et al., 2022) (Figure 2).
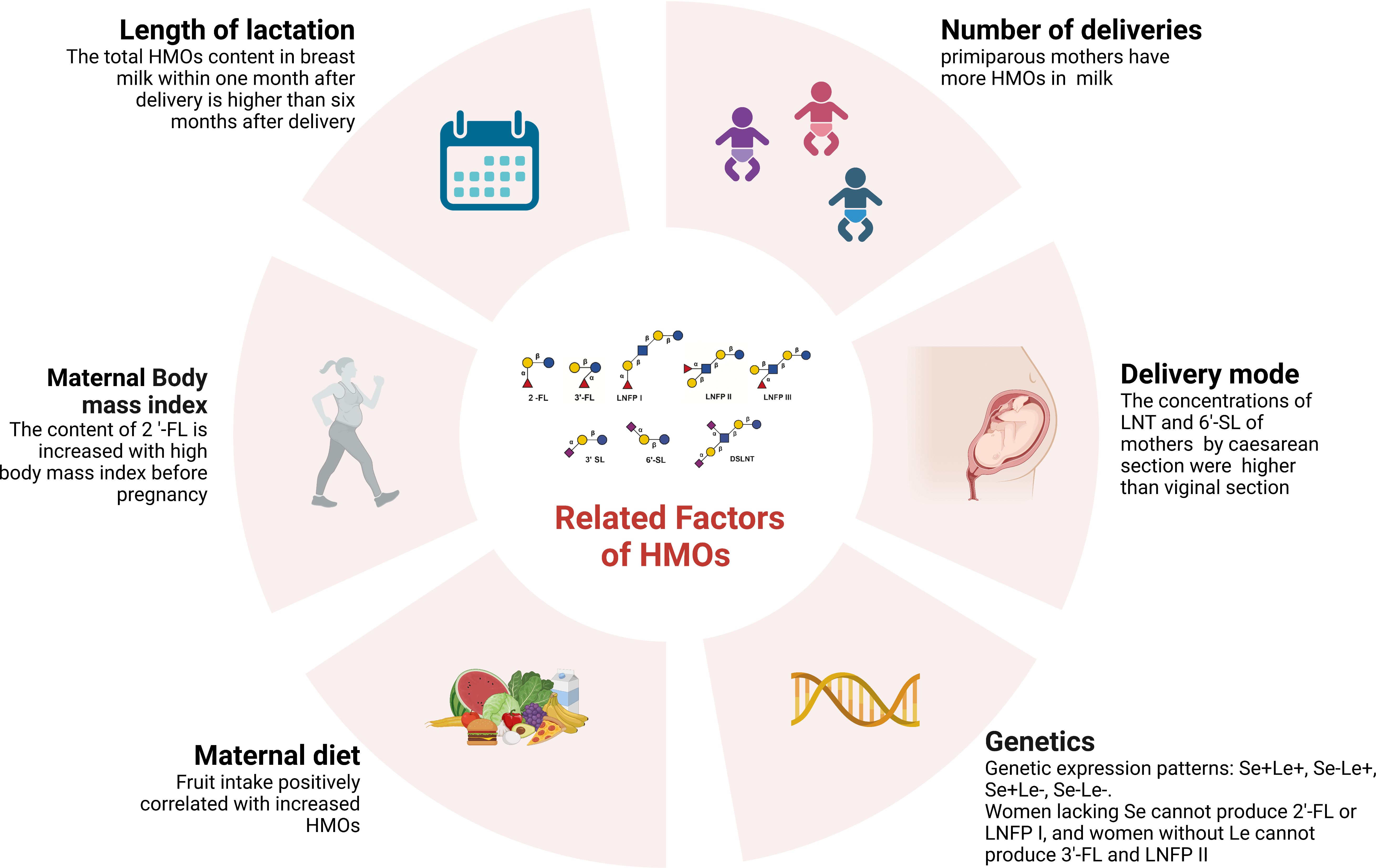
Figure 2. Factors affecting HMOs (Created with BioRender.com).
3.6 Other factors
Furthermore, the difference in HMO concentration may be related to different determination methods (Austin and Bénet, 2018; Huang et al., 2019). The usual determination methods include high-performance liquid chromatography−mass spectrometry (HPLCMS), HPLC time-of-flight mass spectrometry, and high-performance anion-exchange chromatography with pulsed amperometric detection (HPAEC-PAD) (Porfirio et al., 2020; Catenza and Donkor, 2021). HPAEC-PAD needs to separate neutral and acidic oligosaccharides in advance (Ruhaak and Lebrilla, 2012). Micellar electrokinetic chromatography (MEKC) is sensitive to Salicylated HMOs (Bao et al., 2007). The sensitivity of capillary electrophoresis (CE) is also limited (Sarkozy et al., 2021). The reference mass spectrometry library of HMOs developed in the laboratory relies on software and databases (Remoroza et al., 2018). Another complicated factor is the lack of analytical standards for the quantitative evaluation of HMO concentrations (Jackson et al., 2022).
4 Utilization of HMOs by intestinal microbiota
Some intestinal microbiota can utilize and decompose HMOs. The utilization process of HMOs mainly consists of two parts. HMOs are transported from bacteria extracellularly to intracellularly and hydrolyzed by enzymes.
4.1 Bifidobacterium
Most Bifidobacterium participate in the metabolism of the HMOs core structure (Ward et al., 2007). The analysis of many sequenced genomes of Bifidobacterium showed that many of their codes were related to glycoside hydrolases that are needed to metabolize mucin and HMOs (Zúñiga et al., 2018). Bifidobacterium usually needs to remove fucosyl and sialic acid residues when utilizing HMOs. Then, the core of the oligosaccharide is broken down into monosaccharide by different glycosidases (Thongaram et al., 2017). Studies have shown that there are sialidase genes related to the degradation and transport of fucoidan and sialylated HMOs in the genome analysis of Bifidobacterium; these genes include oligosaccharide ABC transporter (O'Callaghan et al., 2015; Matsuki et al., 2016), family 1 solute binding proteins (SBPs) and glycosyl hydrolase (Thomson et al., 2018). Bifidobacterium can synthesize many oligosaccharide hydrolases, such as fucosidase (GH95 and GH29), β-galactosidase and other glycoside hydrolases, and selectively use HMOs (Verkhnyatskaya et al., 2019). Bifidobacterium 614 and 418 can utilize 2’-FL, LNDFHI, LNFP I, LNFPII, LNH, LNT and LNnT (Borewicz et al., 2019). Bifidobacterium longum, infant ATCC 15697 and infant Bifidobacterium M-63 can utilize 2’-FL and 3’-FL (Kong et al., 2021). Bifidobacterium mainly hydrolyses LNT and LNnT through the β-galactosidases Bga42A and Bga2A and releases β-1,3- and β-1,4-linked galactose and LNTII from LNT and LNnT, respectively. LNTII is further catabolized by β-N-acetylglucosaminidase of the GH20 glycosidase family, removing N-acetylglucosaminide GlcNAc and lactose. The latter is hydrolysed into galactose and glucose by β-galactosidase and enters the Leloir pathway, phosphoketolase pathway, amino sugar metabolism pathway, and bifurcation pathway (Yamada et al., 2017). Bifidobacterium dentium strains utilize the galactose moiety and release lacto-N-triose from the LNT and LNnT (Moya-Gonzálvez et al., 2021).
4.2 Lactobacilli
Most Lactobacilli usually have limited ability to utilize HMOs. Nevertheless, Lacticaseibacillus casei and Lacticaseibacillus rhamnosus contain hydrolases, which rely on the phosphotransferase system to phosphorylate oligosaccharides through a phosphate cascade reaction. Then, they are transported into cells and participate in the metabolism of the HMO core structure (Thongaram et al., 2017). Studies have shown that there are three kinds of α-L-fucosidase (AlfA, AlfB and AlfC) belonging to the GH29 family in Lactobacillus, which can release α 1,6-linked fucose residues from some oligosaccharides (Rodríguez-Díaz et al., 2011). Lacticaseibacillus casei transports fucosylation substrates from mannose PTS encoded by the alfEFG gene to cells, and AlfB digests disaccharides in cells into L-fucose and GlcNAc. AlfB and AlfC are active on α-1,3 and α-1,6 bonds in fucosyl-GlcNAc, respectively. AlfC also metabolizes the core fucosylation structure of N-glycosylated protein (Becerra et al., 2020). A sialic acid catabolism nan cluster exists in Lactobacillus L. sakei 23K (Zúñiga et al., 2018). Some strains of Lactobacillus plantarum, Ligilactobacillus salivarius and Lacticaseibacillus paracasei also contain genes for liquid acid metabolism (Hammer et al., 2017). Lactobacillus acidophilus NCFM can degrade LNnT (Kong et al., 2020b) most effectively. Thongaram et al. showed that Lactobacillus acidophilus grew by using extracellular β-galactosidase encoded by the lacL gene to cleave the terminal galactose of LNnT (Thongaram et al., 2017). Lacticaseibacillus casei utilizes LNT II and LNB (Zunga et al., 2021).
4.3 Akkermansia
Akkermansia can grow in human milk and degrade HMOs. Protein group analysis of Lactobacillus mucilaginous showed that the key glycan-degrading enzymes (α-L-fucosidase, β-galactosidase, exoα-sialidase and β-acetylhexosaminidase) could degrade 2’-FL, and LNT could survive in the intestinal tract in early life and settle in the mucous membrane (Kostopoulos et al., 2020). A. muciniphila can grow on human milk and is able to degrade HMOs by using glycan-degrading enzymes. These glycan-degrading enzymes hydrolyze HMOs extracellularly into mono- and disaccharides, and then, the liberated sugars are imported into the cell by transporters (Ottman et al., 2017). A. muciniphila may use 2′-fucosyllactose, 3′-siallylactose, lacto-N-tetraose, and lacto-N-triose II. A. muciniphila is able to survive in the early life environment by consuming oligosaccharides from either breast milk or infant formula (Kostopoulos et al., 2020).
4.4 Strains variants
Different strains of probiotics utilize HMOs variably due to genetic differences. For instance, within the HMO utilization gene cluster of Bifidobacterium longum subsp. infantis, two main variants exist. Some strains possess a complete set of HMOs utilization genes (H5-positive strains), while H5-negative strains lack the ABC transporters required for the core structure of HMOs. H5-positive strains demonstrate significant growth when exposed to LNnT and LNT. Notably, H5-positive strains are more prevalent than H5-negative strains in breastfed infants (Duar et al., 2020).
5 Decomposition and metabolites of HMOs: short-chain fatty acids
The core of oligosaccharides is degraded into monosaccharides and short-chain fatty acids (SCFAs) by different glycosidases. Short-chain fatty acids include acetic, propionic, butyric, and succinic acids (Kong et al., 2021). Acetate and propionate are mainly produced by Bifidobacterium spp. and mucin-degrading bacteria such as Akkermansia muciniphila. Eubacterium hallii and Limosilactobacillus reuteri were able to utilize 1,2-propanediol derived from the fermentation of fucose and rhamnose to produce propionate (Amin et al., 2013; Engels et al., 2016). Butyrate-producing microbiota include bacteria from the families Ruminococcaceae, Erysipelotrichaceae and Lachnospiraceae as well as bacteria such as Anaerobutyricum hallii and Anaerostipes spp. (Parada Venegas et al., 2019). The commensal bacterium Akkermansia muciniphila also produces propionate from this latter pathway (Belzer et al., 2017). Some bacteria, notably in the Lachnospiraceae family, can produce both propionate and butyrate but from different substrates, e.g., Roseburia inulivorans (Martin-Gallausiaux et al., 2021).
SCFAs produced by intestinal microbiota can inhibit pathogen replication (Suez et al., 2019), regulate host intestinal immunity, establish immune homeostasis and control intestinal inflammatory diseases (Guzman-Bautista et al., 2020; Porro et al., 2022). Butyrate is the most active SCFA in inhibiting intestinal inflammatory reactions, which helps to protect the intestinal barrier (Leth et al., 2022). Butyrate has been shown to induce colon regulatory T (Treg) cells, which play a major role in preventing local inflammation and regulating the IFN-g and granzyme B gene table of CD8+ T cells (Bachem et al., 2019). Butyrate can induce the expression of ID2 in CD8+ T cells through IL-12 signaling and directly enhance the cytotoxicity of T cells. Valeric acid and butyric acid promote the expression of effector molecules such as IFNγ and TNF (Lu et al., 2022). Acetate promotes the differentiation of Tfh cells (Lu et al., 2022). Butyrate and acetate promote intestinal IgA production through the G protein-coupled receptors GPR41 and GPR43 (Guo et al., 2019). SCFAs, such as indoleacetic acid, can stimulate aromatic hydrocarbon receptors (AHR) (Gutiérrez-Vázquez and Quintana, 2018), mediate anti-inflammatory effects by producing tissue-protective intraepithelial lymphocytes (IELs), and regulate the polarization of innate lymphocytes (ILC) to control intestinal pathogens (Rothhammer and Quintana, 2019). Propionate has been shown to inhibit allergic inflammation in allergic animal models (Trompette et al., 2014).
SCFAs regulate host immunity, the colonization of intestinal pathogens and the expression of their virulence genes, which directly affects the occurrence of diseases. Acetic acid is a key factor in regulating intestinal physiological function and preventing colonization by pathogenic bacteria. It can reduce the pH value of the intestinal tract, has antibacterial effects and inhibits the growth of pathogenic bacteria. Propionate and butyrate inhibited gene expression in Salmonella invasion (Hung et al., 2013). In addition, SCFAs and other organic acids produced during fermentation can be used as cross-utilization nutrients among intestinal bacteria, which may affect the colonization and adhesion of symbiotic bacteria on host epithelial cells (Bianchi et al., 2018).
SCFAs can affect the disease process by regulating metabolism. SCFAs provide energy for intestinal immune cells, such as B cells, memory T cells and effector T cells, by regulating glycolysis, the TCA cycle and β-oxidation (Lu et al., 2022). Colon cells can absorb SCFAs, and the remaining SCFAs can be transported to all body parts through blood. These SCFAs can be used as substrates for synthesizing sugar or lipids and can also be used as cytokines for regulating metabolism (Porro et al., 2022). Butyrate oxidation provides approximately 70% of the energy requirements of colon cells (Leth et al., 2022). Propionates and acetates are necessary for lipogenesis and gluconeogenesis in the liver (He et al., 2020).
6 HMOs as a bridge between the intestinal microbiota of mothers and infants
HMOs are produced by the mother and are affected by many factors, including maternal intestinal microbiota. HMOs are related to the maternal intestinal microbiota through milk and affect the infant intestinal microbiota. Therefore, we have reason to believe that HMOs are the link between maternal intestinal microbiota and infant intestinal microbiota. Infants can acquire HMOs through maternal breastfeeding. These HMOs affect the proliferation and adhesion of pathogens in infants and directly reduce the occurrence of intestinal diseases. Furthermore, HMOs can strengthen the intestinal mucosal barrier of infants and regulate their immunity, which can alleviate infant intestinal diseases.
6.1 Maternal intestinal microbiota affects human milk microbiota
There is a correlation between maternal intestinal microbiota and milk microbiota. The microbiota in milk may come from maternal breast tissue, maternal intestine, breast skin, or the baby’s mouth. The presence of anaerobic bacteria such as Bifidobacterium in breast milk indicates that the microbiota may be transferred from the mother’s intestine to breast milk (Lyons et al., 2020). There colostrum collected before the first infant lactation contains microbiota, and this indicates that the intestinal-mammary pathway may transport the maternal intestinal microbiota to the lactating mammary gland (Rodríguez, 2014; Damaceno et al., 2017). Using the biomarker strain animal Bifidobacterium subspecies M8 for metagenomics, a small number of bacteria ingested by the mother could be transported to the baby’s intestine through the oral/intestinal-mammary route (Zhong et al., 2022). This pathway is related to physiological and hormonal changes in the third trimester of pregnancy and the permeability of the intestinal epithelium. Intestinal dendritic cells can penetrate the intestinal epithelium by loosening the tight connection between intestinal epithelial cells and absorbing bacteria from the intestinal cavity. Then, the bacteria are transported to mesenteric lymph nodes by macrophages and finally reach the mammary gland (McGuire and McGuire, 2017). Studies have shown that microorganisms in breast tissue can lead to breast inflammation and breast cancer (Kovács et al., 2020; Marwaha et al., 2020). According to the characteristics of the mammary duct, microorganisms may enter the deep parts of the ducts through the opening of the nipple duct (Spina and Cowin, 2021). Moreover, this method can also affect the microbial community in breast milk. Ultrasonic imaging of nursing mothers demonstrated the retrograde flow of milk from the baby’s mouth due to the baby’s sucking motion, indicating that bacteria can transfer from the baby’s mouth to the mother’s breast (Geddes, 2009). Breastfeeding patterns (direct breastfeeding and breastfeeding with a bottle) are significantly related to the composition of breast milk microbiota, which provides evidence for the retrograde mechanism (Moossavi and Azad, 2020). Milk microorganisms may be derived from breast skin, which contains more Staphylococcus and Corynebacterium (Pannaraj et al., 2017; Demmelmair et al., 2020).
6.2 Influence of milk microbiota on infant intestinal microbiota
The microbiota in milk can establish the intestinal microbiota of infants. The changes in infant intestinal microbiota can reflect the maternal intestinal microbiota (Zhang et al., 2022). Studies have shown that most of the intestinal bacteria of babies who are born naturally are breastfed come from the mother’s intestine (Shao et al., 2019). A study conducted a longitudinal analysis of the intestinal microbiota and metabolomics of 70 mothers and infants from the third trimester to their children’s first year. It was found that some mothers’ intestinal microbiota (such as some Bacteroides species) transferred genes to infants’ intestinal microbiota through movable genetic elements to shape the structure and function of the infants’ intestinal microbiota (such as the ability to use HMOs) (Vatanen et al., 2022). Additionally, the results of the microbial analysis show that the composition of microbial groups is related to breastfeeding in babies, indicating that there are bacteria from milk in the baby’s intestines (Banić et al., 2022). Bacterial diversity (Faith species diversity) and composition changes are dose-dependent with the daily breast milk intake ratio (Pannaraj et al., 2017). There is a decrease in Bacteroides in infants who are breastfed during the neonatal period (Shao et al., 2019). The diversity and richness of microorganisms in breast milk are the highest in colostrum and gradually decrease with the passage of breastfeeding time. Among these microorganisms, Staphylococcus, Streptococcus, Acinetobacter, Pseudomonas and Lactobacillus are dominant in colostrum samples (Wan et al., 2020). The regulation of the intestinal microbiota of lactating mothers by diet or probiotics will affect the intestinal microbiota of milk, which may affect the intestinal microbiota of infants (Sindi et al., 2021).
6.3 The correlation between milk microbiota and HMOs
Recent studies have shown a relationship between HMOs and milk microbiota that is related to the degree of HMO utilization by milk microorganisms. The relative abundance of milk bacteria is related to the concentration of fucosylation and sialylation HMOs (Cheema et al., 2022). Moossavi et al. analyzed the milk samples of 393 mothers. They found that HMOs were related to the diversity of the microbial community in milk, and Staphylococcus was negatively correlated with HMOs (r= -0.60 p=0.038) (Moossavi et al., 2019). Rubio et al. found that the lower the HMO concentration was, the higher the level of Staphylococcus in milk (Cabrera-Rubio et al., 2019). However, some studies have tested healthy breast milk samples and found that the relative abundance of Staphylococcus in milk positively correlates with HMO content (Aakko et al., 2017; Williams et al., 2017). The total HMOs in colostrum and transitional milk were positively correlated with the level of Streptococcus (Cabrera-Rubio et al., 2019). LNnT and 2’-FL were negatively correlated with Proteus and Actinomycetes (Gómez-Gallego et al., 2018). In another study, Streptococcus and Staphylococcus were positively correlated with DFL and LNFPIII (Borewicz et al., 2019).
Rubio et al. found that higher levels of Lactobacillus in colostrum and mature milk were related to higher concentrations of 2’-FL. The level of Lactobacillus in mature milk is also associated with a higher concentration of LNFP I. Acetylated HMOs were positively correlated with Lactobacillus, Enterococcus and Streptococcus, while negatively correlated with Bifidobacterium (Cabrera-Rubio et al., 2019). Studies have observed that Bifidobacterium in milk is positively correlated with sialylated HMOs and LNT and negatively correlated with DS-LNT (Aakko et al., 2017; Moossavi et al., 2019). In addition, there is a positive correlation between fucosylated HMOs and Akkermansia (Aakko et al., 2017).
Therefore, maternal intestinal and milk microbiota, as well as milk microbiota and infant intestinal microbiota, are closely related. Moreover, HMOs are widely present in milk and are connected to milk microbiota. Through maternal lactation, the maternal intestinal microbiota establishes contact with HMOs through milk, and through the effects of the milk microbiota and HMOs on the infant’s intestine, a healthy intestinal microenvironment is established to treat infant intestinal diseases (Figure 3).
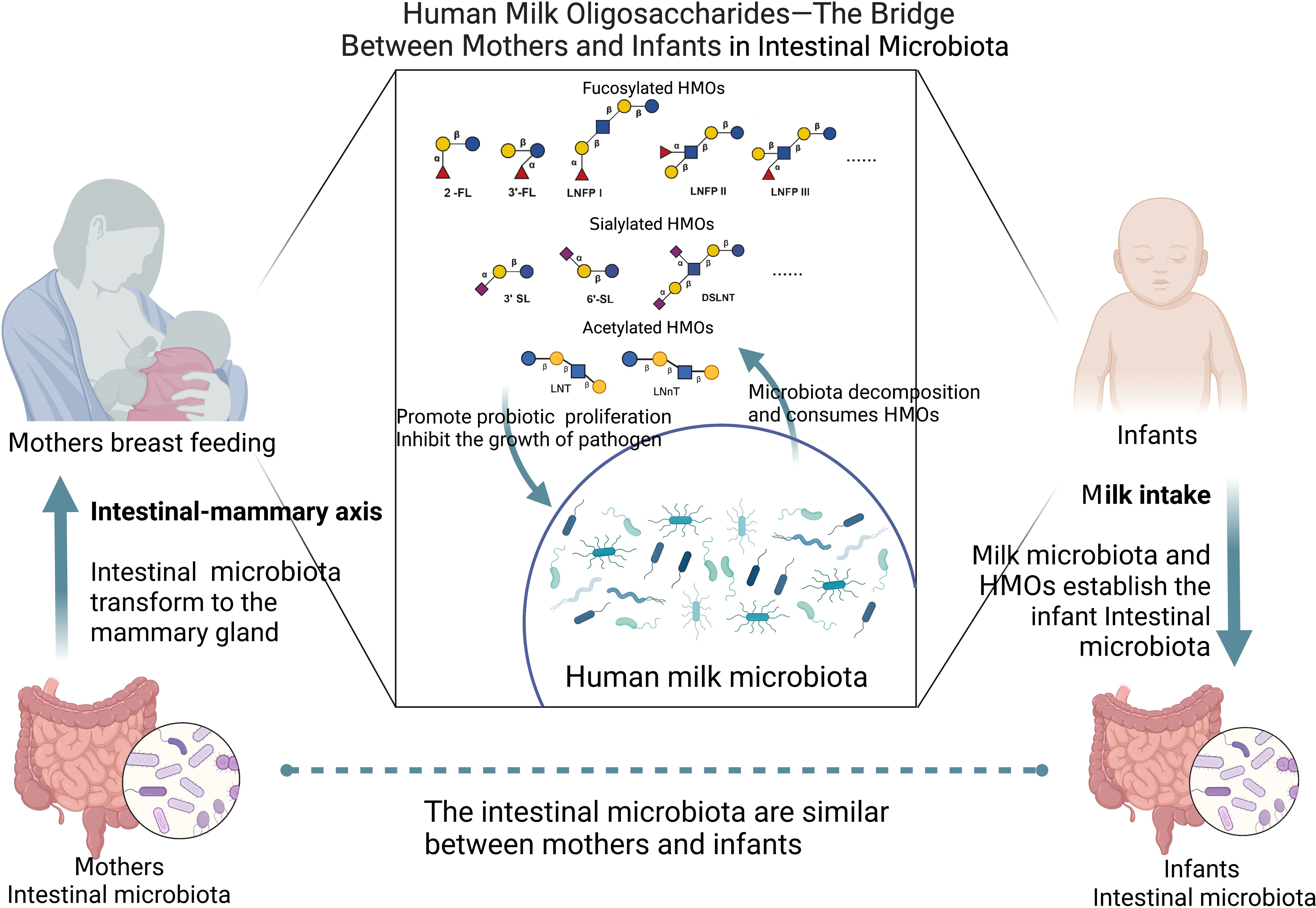
Figure 3. HMOs as a bridge between mothers and infants in intestinal microbiota (Created with BioRender.com).
7 HMOs and infant intestinal microbiota
7.1 HMOs regulate the growth of intestinal microbiota in infants
7.1.1 Promotion of probiotic growth
At present, some in vitro experiments show that HMOs can directly regulate the growth and activity of microbiota. Some studies have used the human intestinal microbial ecosystem simulator (SHIME) and Caco2 cell line to study the effect of HMOs on the adult intestinal microbiota. The results show that 2’-FL and LNnT increase the abundance of Bifidobacterium and the content of SCFAs (Natividad et al., 2022). 2’-FL was added to the infant fecal microbiota for culture in vitro, and the relative abundance of Bifidobacterium was increased as a result (Akkerman et al., 2022; Xu et al., 2022; Dedon et al., 2023). Mass spectrometry analysis of oligosaccharide consumption revealed that Bifidobacterium longum and Bacteroides fragilis could metabolize fucosylated HMOs efficiently in vitro (Marcobal et al., 2010). Single strain culture showed that 2’-FL could promote the growth of Lactobacillus mucilaginous isolated from mouse feces (Gao et al., 2022). Studies have shown that Lacticaseibacillus casei can grow using LNT as a carbon source (Sakanaka et al., 2019a). After 14 hours of in vitro culture, 90.1% of LNT was used in the mixed fecal microbiota of infants, and the richness of Columbian and Bifidobacterium was explicitly increased. After 36 hours, 60.3% of 3’-FL was used, and Bacteroides and Enterococcus were specifically enriched (Kong et al., 2021).
Animal experiments in vivo show that HMOs can regulate the composition and structure of intestinal microbiota and increase the abundance of probiotics (Xiao et al., 2018). HMOs were added to the humanized mouse model of infant feces transplantation. The 16S rRNA sequencing analysis showed that the abundance of Bifidobacterium increased significantly, while that of Eubacteria and Clostridium decreased (Rubio-Del-Campo et al., 2021). The number of Bifidobacterium from the ileum to the colon increased (Mielcarek et al., 2011). HMOs can increase the number of butyrate-producing bacteria, and the ileum mRNA levels of the SCFA receptors Gpr41 (Ffar3), Gpr109 (Hcar2) and Gpr43 (Ffar2) are increased (Jena et al., 2018). 2’-FL can increase the abundance of probiotics, such as Akkermansia, in ageing mice (Wang et al., 2022). In the gut-liver-microbiota axis, 2’-FL upregulates acetic acid, propionic acid, butyric acid and total SCFAs by regulating the intestinal microbiota of colitis mice and is positively correlated with Akkermansia (Yao et al., 2022).
7.1.2 Effects on the growth of pathogens
The mucin MUC2 mucosal barrier, as the first barrier, prevents direct contact between intestinal bacteria and colon epithelial cells (Yao et al., 2021). If pathogenic bacteria penetrate the epithelial layer and enter the stroma, immune sentinel cells (such as macrophages, dendritic cells and innate lymphocytes) trigger inflammation and the adaptive immune response by activating Th1/Th17 cells (Chen et al., 2021a). In many cases, HMOs can directly inhibit the growth of pathogenic bacteria in vitro without relying on host immunity. After HMO treatment, a specific glycosyltransferase gene mutation was identified in the phenotype screen of group B Streptococcus, which may be the reason for the antibacterial ability of HMOs (Lin et al., 2017). Clostridium perfringens translocation was produced in the intestine of mice that ingested HMOs, and decreased in the ileum and the caecum (Mielcarek et al., 2011). 2’-FL can inhibit the growth of group B Streptococcus, such as Streptococcus agalactiae, in vitro and can regulate the biofilm formation of Streptococcus agalactiae (Ackerman et al., 2017). According to the sugar spectrum analysis based on oligosaccharide consumption, Enterococcus, Streptococcus, Veillonella, Eubacteria, Clostridium and Escherichia coli strains grow poorly in HMOs (Marcobal et al., 2010). 2’-FL and LNnT inhibited the level of Clostridium difficile in an intestinal model in vitro (Vigsnaes et al., 2021).
HMOs, such as 2’-FL, affect the intestinal microbiota of newborn mice, thus preventing intestinal diseases such as necrotizing colitis (Good et al., 2016; Gart et al., 2022). HMO administration significantly reduced the colonization of enteropathogenic Escherichia coli in neonatal mice (Manthey et al., 2014). The combination of HMOs and Bifidobacterium longum reduced the Clostridium and G- bacteria count in mice (Musilova et al., 2017). A study established an infection mouse model of E. coli O157 and found that ingestion of 2’-FL can reduce the colonization of E. coli O157 in the intestine of mice by more than 90% (Wang et al., 2020). Intake of 2’-FL reduced the invasion of Campylobacter jejuni by 80% in mice (Yu et al., 2016). Transfection of mother mice with the α 1,2-fucosyltransferase gene of the H-2 antigen can inhibit the colonization of Campylobacter jejuni in young nursing mice (Ruiz-Palacios et al., 2003). In addition, 3’-SL and 6’-SL can reverse the changes in β diversity of colonic mucosa-associated microorganisms caused by emergency stimulation in mice (Tarr et al., 2015). Therefore, the ability of HMOs to regulate the growth of microbiota directly inhibits the number of pathogenic bacteria and reduces the occurrence of intestinal diseases.
However, several in vitro and murine studies indicated that HMOs are directly utilized by either primary degraders or via pathogenic bacteria, including Shigella. S. typhimurium accesses fucose and sialic acid within the lumen of the gut in a microbiota-dependent manner. The increase in sialic acid levels induced by microorganisms in vivo is helpful for the amplification of C. difficile (Ng et al., 2013). The increase in caecal sialidase activity is vital for the growth advantage of Escherichia coli (E. coli) during intestinal inflammation in mice. The increase in sialidase activity mediates the release of sialic acid in intestinal tissue, which promotes the growth of E. coli during inflammation (Huang et al., 2015).
7.2 HMOs change the adhesion of intestinal microbiota in infants
7.2.1 Increasing the adhesion of probiotics
HMOs increase the adhesion of probiotics in the intestinal tract, which benefits intestinal health. Studies have shown that 3’-FL and LNT enhance the adhesion of Lactiplantibacillus plantarum CFS1 1.85-1.90 times (Kong et al., 2020a). LNT can increase the adhesion of Lactiplantibacillus plantarum WCFS1 to intestinal epithelial Caco-2 cells (Kong et al., 2021).
7.2.2 Reducing the adhesion of pathogens
HMOs can combine with pathogens in the intestine, reducing the ability of pathogenic bacteria to colonize the intestine, thus reducing its pathogenic effect on the intestine. Many HMOs are soluble receptor analogues of carbohydrates on the surface of epithelial cells, which act as receptor bait. When pathogens combine with them, they reduce their adhesion to intestinal cells and are excreted. In vitro, competition tests confirmed that combining HMOs and Bifidobacterium longum reduced the Clostridium and G- bacteria count, which could inhibit potential pathogenic bacteria (Musilova et al., 2017). Yue and other studies show that HMOs can reduce the adhesion of Staphylococcus aureus to human colorectal epithelial cells, and the effect is higher than that of oligosaccharides in milk and goat milk (Yue et al., 2020). Manthey et al. showed that HMOs significantly reduced the attachment of enteropathogenic Escherichia coli to cultured epithelial cells (Manthey et al., 2014). 2’-FL can combine with Campylobacter jejuni to reduce infection (Lane et al., 2011). 2’-FL inhibits the planktonic growth and adhesion characteristics of Streptococcus mutans, an oral pathogen associated with dental caries, and reduces the adhesion ability of Streptococcus mutans CI 2366 and DSM 20523 to saliva-coated hydroxyapatite (Salli et al., 2020). Colonization of Campylobacter jejuni to the human intestinal mucosa in vitro was inhibited by fucosylated HMOs (Ruiz-Palacios et al., 2003). Weichert et al. showed that 2’-FL could inhibit the adhesion of Campylobacter jejuni, pathogenic Escherichia coli, Salmonella serotype fyris and Pseudomonas aeruginosa to the intestinal human cell line Caco-2 (decreased by 26%, 18%, 12% and 17%, respectively), and 3-FL could reduce the adhesion of pathogenic Escherichia coli and Pseudomonas aeruginosa (29% and 26%) (Weichert et al., 2013). 2’-FL, 3’-SL and 6’-SL reduced the adhesion and infectivity of human rotavirus (Laucirica et al., 2017). 3’-SL and 6’-SL reduced the adhesion of Clostridium difficile to human colon cells, affected the formation of biofilms of Clostridium difficile, and decreased the expression of the cell wall protein cwp84, which is related to bacterial adhesion (Piotrowski et al., 2022).
7.3 HMOs enhance the intestinal mucosal barrier in infants
7.3.1 Enhancing mucosal barrier function
The intestinal barrier is mainly composed of intestinal mucosal cells and epithelial cells. HMOs increased the transepithelial resistance of Caco-2 cells and decreased the macromolecular permeability of monolayer cells (Wu et al., 2022). HMOs significantly stimulated defensin β-1 (DEFB1) and tight junction protein (ZO-1) in Caco-2 cells (Kong et al., 2020a). Wang et al. showed that HMOs could increase the phosphorylated epidermal growth factor receptor P-EGFR by 58% and decrease P-P38 by 48-89% in Caco-2 cells (Wang et al., 2020). Natividad et al. showed that 2’-FL, LNT, LNnT, 3’-SL and 6’-SL dose-dependently restricted the translocation of fluorescent markers in Caco-2 cells and reduced intestinal permeability (Natividad et al., 2020). Crane et al. showed that HMOs blocked the biochemical action of Escherichia coli in the human colon cancer cell line T84, inhibited cGMP of guanylate cyclase activity stimulated by Escherichia coli in the T84 cell membrane, and inhibited 125I-STa binding (by 17% and 27%, respectively) (Crane et al., 1994). Some studies have used human intestinal organ chips to study the effect of HMOs on intestinal barrier function. The results showed that the expression levels of the claudin-5 and claudin-8 genes of tight junction proteins increased, while the levels of IL-6 decreased (Šuligoj et al., 2020).
In vivo experiments also show that HMOs can enhance the mucosal barrier. When C57BL/C mice with intestinal barrier injury induced by hypoxia ingested HMOs, the number of Ki67-positive cells in the ileum increased by 60-80%, while the number of HIF-1α and caspase-3 in the ileum decreased by 56-71% (Wang et al., 2020). A rat model of necrotizing enterocolitis was treated with HMOs, which directly induced the expression of protein disulfide isomerase (PDI), increased the level of intestinal mucin Muc2, decreased the permeability of the intestine to macromolecular dextran, and reduced bacterial adhesion (Wu et al., 2019). Oral administration of 2’-FL inhibited intestinal permeability in a mouse model of fatty liver disease (Gart et al., 2022). Intake of 2’-FL in mice infected with Escherichia coli O157 increased the expression levels of the intestinal mucin Muc2 and enhanced intestinal barrier function (Wang et al., 2020). 2’-FL can upregulate endothelial nitric oxide synthase (eNOS) and restore intestinal perfusion in young rats (Good et al., 2016). Oral administration of 2’- FL and 3’-FL to C57BL/6J mice with UC increased the expression of locking protein in colon tissue and reversed the intestinal barrier function of Caco-2 cells and the shortening of colon length (Kim et al., 2023).
7.3.2 Increasing the growth and differentiation of intestinal cells
HMOs can stimulate gastrointestinal maturation. HMOs can induce the differentiation of human intestinal cells and small intestinal epithelial crypt cells, inhibit cell growth and directly regulate the growth cycle of intestinal cells (Kuntz et al., 2008). 3’-SL, 6’-SL and 2 ‘-FL reduced the proliferation of the intestinal epithelial HT-29 and Caco-2Bbe cells, promoted the differentiation of HT-29 and Caco-2Bbe cells, reduced the apoptosis and necrosis of Caco-2Bbe cells (Holscher et al., 2017), and increased the alkaline phosphatase activity of Caco-2Bbe cells (Holscher et al., 2014). Salicylated HMOs can prevent Caco-2 cells from damage by LPS, histamine and chymotrypsin by regulating the cell growth cycle and increasing mitochondrial membrane potential (He-Yang et al., 2020).
HMOs can also increase the differentiation of cells, small intestinal cluster cells and Muc2 cells. Bacterial strains cultured in the feces of infants with dysplasia were implanted into sterile mice and fed a diet containing sialylated oligosaccharides. The results showed that the lean body weight of mice was increased depending on the microbiota (Charbonneau et al., 2016), the level of succinic acid in the caecum was increased depending on sialylated oligosaccharides and microbiota, the number of small intestinal cluster cells was increased, and the signaling pathway of cluster cells induced by succinic acid related to the Th2 immune response was activated (Cowardin et al., 2019). Genome-wide analysis showed that HMOs changed 225 unique target genes related to cell proliferation and differentiation, including the stem cell differentiation marker HMGCS2. After HMO intervention in young rats with necrotizing colitis, the differentiation of Muc2 cells in the crypt-villus axis was enhanced, and the expression of HMGCS2 was upregulated (Li et al., 2020). HMOs can improve the intestinal mucosal barrier and increase the differentiation of intestinal cells. Thus, they can protect the intestine from pathogenic bacteria and alleviate the symptoms of intestinal diseases.
7.4 HMOs enhance intestinal immunity in infants
HMOs can reduce intestinal inflammation and regulate intestinal immunity. In vitro, HMOs combined with SCFAs induced the development of tolerant dendritic cells (tDCs) and triggered functional regulatory T cells (Xiao et al., 2018). In the inflammatory model of T84 and H4 intestinal epithelial cells invaded by enterotoxigenic Escherichia coli type 1 (ETEC) in vitro, HMOs reduced the levels of IL-8 that were increased by Escherichia coli infection. They also inhibited the transcription and translation of CD14 (He et al., 2016). In vivo, HMOs produced specific immune responses by regulating IL-10 and IL-6 (Musilova et al., 2017). Consumption of HMOs by infant C57BL/C mice with intestinal barrier injury induced by hypoxia increased the concentration of IL-8 in serum and the ileum, inhibited the expression of toll-like receptor 4 (TLR4) protein, and activated the nuclear factor κB (NF-κB) pathway in the ileum (Wang et al., 2019). Exosomes containing HMOs are ingested by macrophages responsible for establishing intestinal immunity. Mice pretreated with HMOs encapsulated by exosomes are protected from adhesive invasive Escherichia coli infection, and LPS-induced inflammation and intestinal injury are significantly reduced (He et al., 2021).
Fucosylated HMOs, such as 2’-FL, can increase the SCFA content, increase intestinal perfusion, and alleviate the symptoms of intestinal diseases such as necrotizing colitis. 2’-FL attenuates the DC cytokine response in vitro in a manner dependent on the AhR receptor (Akkerman et al., 2022). In vitro studies showed that 2’-FL promoted the secretion of the mucin MUC2 in intestinal goblet cells through NLRP6, significantly decreased the levels of toll-like receptor 4 (TLR4), myeloid differential protein-88 (MyD88) and nuclear factor κB (NF-κB) in inflammatory cells of LS174T, and inhibited inflammation by inhibiting the TLR4/MyD88/NF-κB pathway (Yao et al., 2023). Intake of 2’-FL in mice infected with Escherichia coli O157 can reduce intestinal inflammation and increase the content of SCFAs in feces (Wang et al., 2020). 2’-FL can significantly reduce oxidative stress injury and inflammation in the intestine of ageing mice by regulating sirtuin1 (SIRT1)-related and nuclear factor E2-related factor 2 (Nrf2) and increasing the content of SCFAs in the intestine (Wang et al., 2022). Intake of 2’-FL inhibited the release of 60-70% interleukin IL-8, 80-90% IL-1β and 50% neutrophil chemotactic macrophage inflammatory protein 2 (MIP-2) in C57BL/6 mice infected with Campylobacter jejuni (Yu et al., 2016).
Salicylated HMOs enhance intestinal immunity and reduce intestinal inflammation. Supplementation of sialylated HMOs can reduce the incidence of necrotizing colitis and the pathological injury score in rats, inhibit the accumulation of mast cells in the ileum of necrotizing colitis rats (He-Yang et al., 2020), reduce the accumulation of IL-1β, IL-6 and TNF-α, reduce mTLR4, NLRP3 and caspase-1 levels in the ileum of rats, restore IκB-α levels in the cytoplasm, and reduce the phosphorylation of NF-κ B P65 in the nucleus (Zhang et al., 2021). Intake of 6’-SL can alleviate the symptoms of intestinal diarrhea in an allergic mouse model and inhibit the number of mast cells in the intestine of mice (Castillo-Courtade et al., 2015). Oral administration of 6’-SL to mice and piglets can reduce necrotizing enterocolitis by reducing apoptosis, inflammation, weight loss and histological appearance, reduce the levels of TLR4-mediated nuclear factor κ light chain enhancer of intestinal NF-kB inflammatory signal, and restore the intestinal barrier of mice (Sodhi et al., 2021; Sodhi et al., 2023). Salivary DS-LNT can improve the survival rate of rats with necrotizing colitis and reduce pathological features (Autran et al., 2016).
In addition, acetyl HMOs, such as LNT, specifically activate GPR35 receptors, relieve colon pain and relieve colitis (Foata et al., 2020). In the immature intestinal epithelial cell line FHs 74, LNnT has a high binding capacity to tumor necrosis factor receptor TNFR1, reducing the intestinal epithelial cell inflammation induced by TNF-α (Cheng et al., 2021). Therefore, HMOs, breast milk, and infant intestinal microbiota have complex interactions (Figure 4).
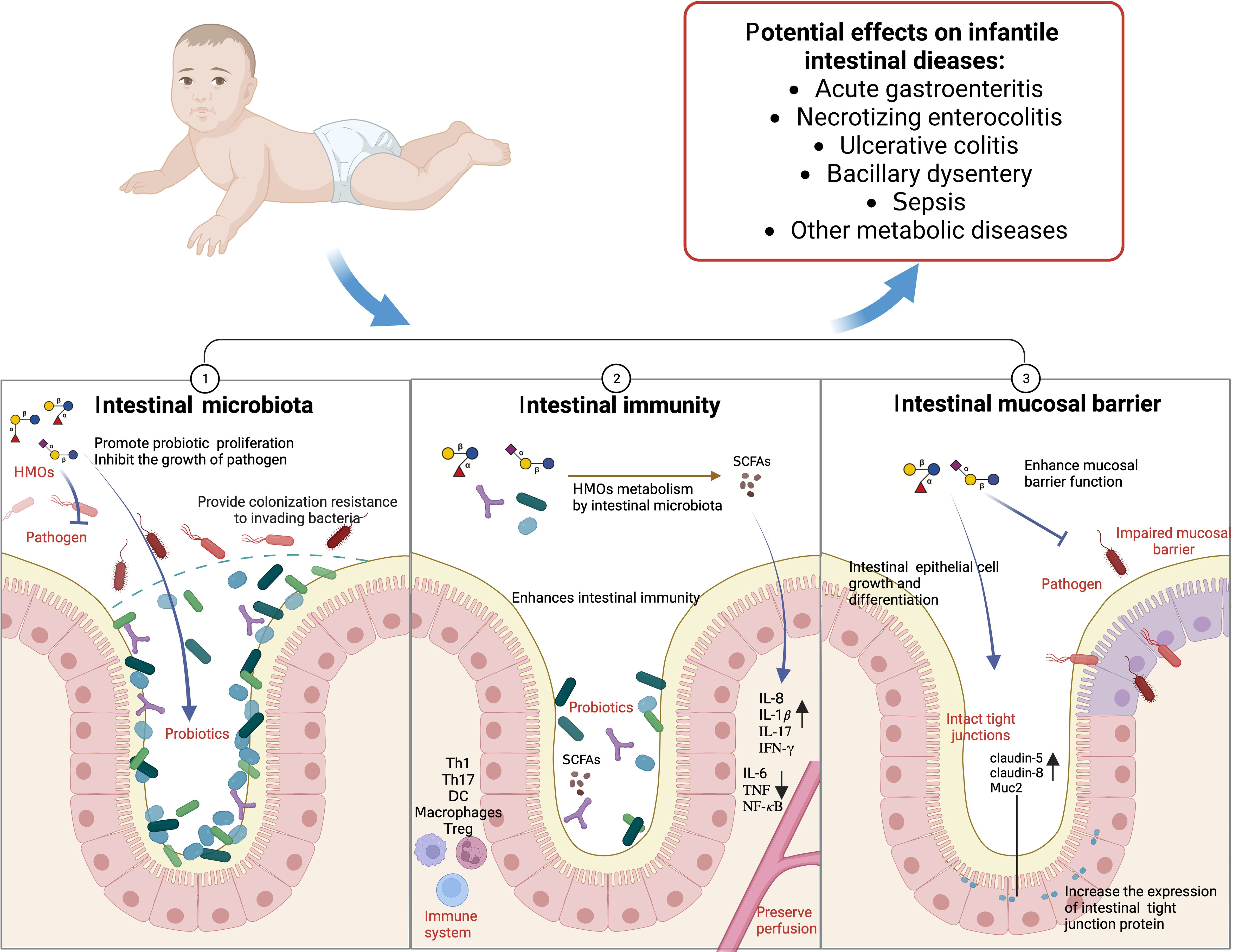
Figure 4. HMOs and infants’ intestinal microbiota (Created with BioRender.com).
7.5 HMOs affect the intestinal activity of infants
HMOs can affect intestinal activity and relieve pain in conditions such as infantile colic or irritable bowel syndrome. In C57BL/6JRj mice with visceral hypersensitivity, the HMO mixture reduced the intestinal distension volume and EMG reaction, promoted most microbial groups and reduced the functional pathways related to visceral hypersensitivity. 2’-FL+DFL and LNT+6’SL decreased the visceral motor response and regulated the serotonin and CGRPα-related pathways (Ferrier et al., 2022).
8 The potential of HMOs to influence the infant intestinal microbiota in the intervention of diseases
8.1 HMOs increase intestinal probiotics in infants
HMOs can increase the abundance of Bifidobacterium in infants’ intestines (Table 1). Bode et al. Bode (2012) and Porro et al (2022) proposed that HMOs could affect intestinal Bifidobacterium, Staphylococcus, Streptococcus, Lactobacillus and Enterococcus in infants, and the proliferation of Bifidobacterium in infants was correlated with a high HMO content in breast milk (Le Doare et al., 2018). The growth of the intestinal Bifidobacterium community is affected by HMOs and is related to the consumption of various HMOs (Borewicz et al., 2019; Nolan et al., 2020; Nogacka et al., 2021). Studies have shown that Bifidobacterium can utilize 2’-FL, and its abundance negatively correlates with the 2’-FL concentration (Thongaram et al., 2017). The analysis of the intestinal microbiota of infants showed that feeding infants HMOs and the animal Bifidobacterium lactis subspecies CNCM I-3446 significantly transformed the subspecies into bifidobacteria (Simeoni et al., 2016). A randomized, double-blind controlled trial also showed that Clostridium in the intestine of infants with 2’-FL was considerably lower than that in infants without 2’-FL, while Bifidobacterium was higher than that in infants without 2’-FL (Alliet et al., 2022). A randomized, double-blind controlled trial of overweight children included 75 overweight children aged 6-12 years who were administered 2’-FL and LNnT or glucose placebo orally for 8 weeks. The relative abundance of Bifidobacterium in the experimental group increased significantly after intervention, but no change was observed in the placebo group (Fonvig et al., 2021). From 6 months to 12 months after feeding HMO-containing milk, the relative abundance of E. coli and E. faecalis in stool samples increased, and the relative level of butyrate increased by 4 times (Nilsen et al., 2020). Consumption of formula containing 2’FL and LNnT increased the levels of acetate and Bifidobacterium in infants’ intestines (Dogra et al., 2021).
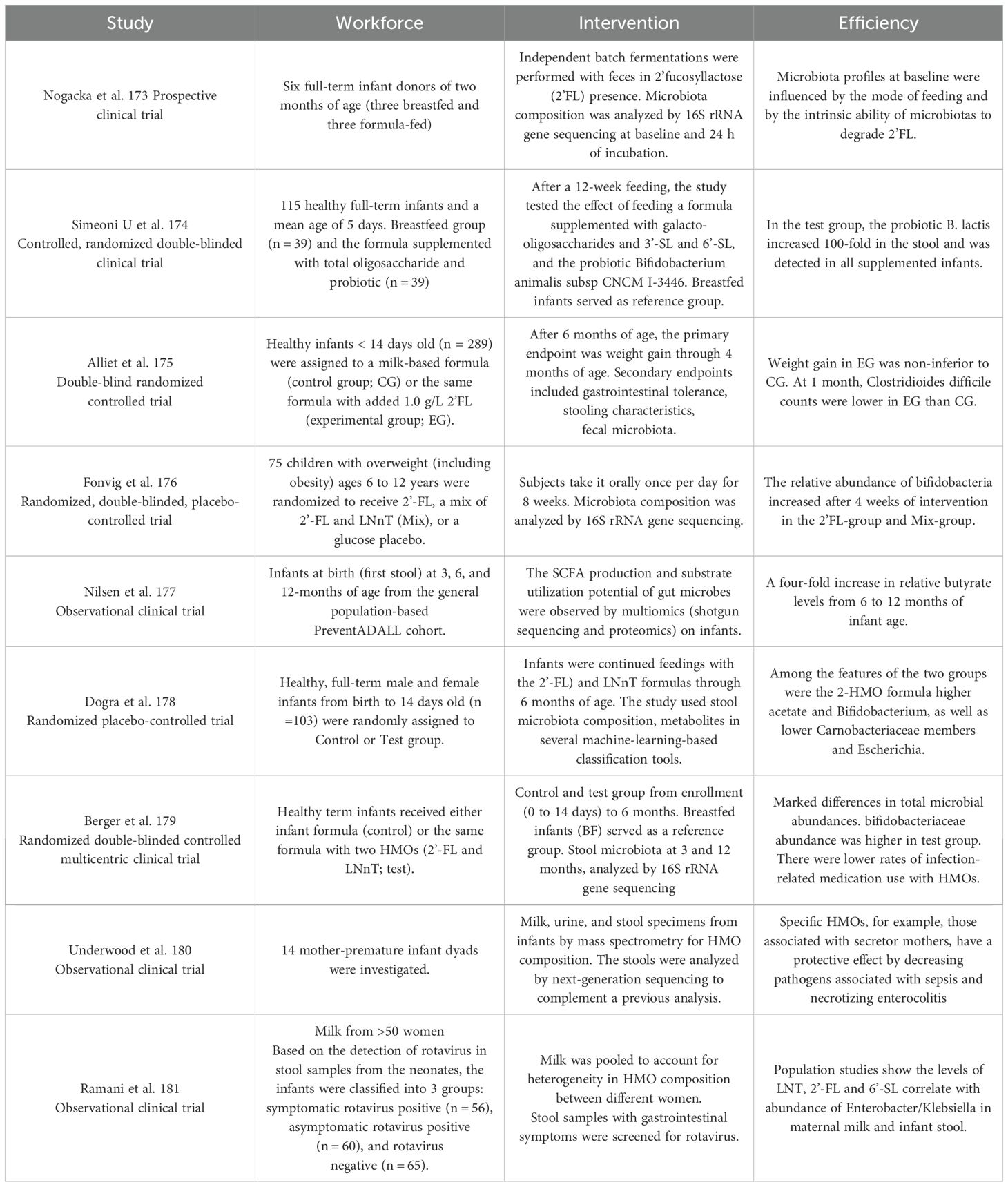
Table 1. Overview of studies used in this review demonstrating the role of HMOs on intestinal in infants (clinical trials).
HMOs and probiotics hold significant potential for treating infant diseases. The combination of Bifidobacterium longum subsp. infantis with HMOs represents a viable strategy to enhance health outcomes for infants and children (Mills et al., 2023; Wong et al., 2024). The inclusion of Lactobacillus rhamnosus has been shown to shorten the duration of rectal bleeding in infants with milk protein allergies (Malekiantaghi et al., 2024). Lactobacillus limousine FPHC2951 has been observed to increase the expression of interleukin-10 (IL-10) mRNA, thereby improving symptoms of colitis (Huang et al., 2024). HMOs may also benefit adolescents with chronic gastrointestinal dysfunction and Autism Spectrum Disorder by modulating the brain-gut axis, potentially improving mood (Aldegheri et al., 2024). Probiotics can produce or metabolize antibacterial substances that inhibit the growth of pathogens or compete with them for nutrients and adhesion to the intestinal epithelium (Kayama et al., 2020; Prete et al., 2020; Huynh et al., 2022). The adhesion of probiotic microorganisms to epithelial cells may trigger a signaling cascade that leads to immune regulation. Additionally, the release of soluble components may directly or indirectly activate immune cells, thus influencing disease progression (Dimidi et al., 2017).
8.2 HMOs reduce the risk of intestinal infection in infants
Pathogens are a significant direct cause of intestinal diseases. The microbiota that causes acute gastroenteritis in infants includes pathogenic Escherichia coli, Campylobacter jejuni, Salmonella, and Clostridium difficile (Denamur et al., 2021).The incidence of Neonatal necrotizing enterocolitis (NEC) and ulcerative colitis (UC) is related to a decrease in the number of Bifidobacterium and Lactobacillus and an increase in the levels of Firmicutes,Bacteroides, Proteus and Staphylococcus (Cui et al., 2021; Moschino et al., 2022). Infantile bacillary dysentery is mainly related to Shigella (Qasim et al., 2022). The characterization of intestinal microbiota has potential as a diagnostic and even preventive tool for predicting NEC and can be used as a biomarker for monitoring intestinal diseases (Niemarkt et al., 2019).
Supplementing probiotics can benefit hosts through interactions with intestinal microbiota and immune function (Méndez et al., 2021; Samara et al., 2022), and thus, it has been proposed as a potential tool to prevent Intestinal Infection (Beghetti et al., 2021). There have been clinical trials to regulate and intervene in the intestinal microbiota through probiotic transplantation, fecal microbiota transplantation and gene manipulation to repair the mucosal barrier and treat UC (Glassner et al., 2020). Escherichia coli Nissle1917 can reduce the intestinal colonization of Salmonella typhi by competing for iron intake and secrete microglobulin to limit the growth of adhesion-invasive Escherichia coli (Sassone-Corsi et al., 2016). The outer membrane vesicle of Bacillus polymorpha inhibited the invasion of Shigella flexneri, the expression of the virulence regulator VirF gene and the level of the virulence protein (Xerri and Payne, 2022). Enterococcus faecalis expressing antibacterial peptides (bacteriocin 21) replaced indigenous enterococcus colonization and eliminated vancomycin-resistant enterococci (Kommineni et al., 2015). In addition, some specific probiotics may benefit Helicobacter pylori gastritis and infantile colic (Vandenplas et al., 2011).
In a randomized, double-blind, controlled multicenter clinical trial, 2’-FL and LNnT were added to infant milk powder and administered for six months. Compared with infants without HMOs, those who did exhibited a significant increase in total microbial abundance. Furthermore, these infants demonstrated a lower risk of infection and reduced antibiotic utilization (Underwood et al., 2015). Mark A et al. sequenced and analyzed infant feces, revealing that HMOs present in secretory mother’s milk decreased the levels of Proteus and Firmicutes in the infants’ intestines, thereby reducing pathogens associated with sepsis and necrotizing enterocolitis (Berger et al., 2020). Additionally, LNT, 2-’FL and 6’-SL are linked to increased levels of Enterobacter and Klebsiella in breast milk and infant feces and they may also inhibit the infectivity of neonatal rotavirus (Ramani et al., 2018).
9 Conclusion and perspectives
Above all, HMOs can regulate the intestinal microbiota of infants, enhance intestinal mucosal barrier function, affect intestinal function and systemic physiological function of the host through immune function. In addition, HMOs are a link between maternal intestinal microbiota and infant intestinal microbiota. HMOs not only affect the intestinal microbiota of infants but are also related to the maternal milk microbiota. HMOs can influence several intestinal diseases of infants through contact with microbiota, and they have great potential be used as supplementary and alternative therapies for intestinal diseases of infants.
However, some problems remain to be solved. First, the effect of HMOs on the whole body needs further exploration. HMOs can affect the disease process by regulating intestinal microbiota. HMOs have the potential to regulate systemic diseases through intestinal microorganisms.
Secondly, while a correlation exists between maternal intestinal microbiota and HMOs, the specific mechanisms governing HMOs composition remain unclear. Therefore, it is important to explore the synthesis pathways of HMOs in the human body and investigate whether maternal intestinal microbiota influence these pathways.
Finally, as a potential therapeutic drug for intestinal diseases, HMOs need more clinical research. HMO-based clinical trials have been performed for infant and adult intestinal diseases. These studies can provide evidence for the clinical application of HMO-based therapy. However, these studies are usually small in sample size and have poor repeatability. Thus, it is necessary to carry out large-scale multicenter studies to improve the effectiveness of HMOs.
10 Literature search methods
Most reviews are based on the research status of HMOs and infant intestinal diseases. The factors influencing HMOs formation and the role of HMOs in the relationship between mother and infant intestinal microbiota are still lacking. We have appraised all forms of published research to ensure that the scientific and clinical implications of the available evidence might be synthesized into useful treatise, which reflects what is known about the intestinal microbiota of mothers and infants and what remains to be elucidated. The literature search was completed up to 20 July 2023 with some updates during the review.
The review was carried out in three stages. First, we described the structure and types of HMOs and explain how organisms use HMOs. Then, we summarized the research on HMOs as a potential treatment for diseases related to the infant intestinal microbiota. Finally, we emphasized the study of HMOs on the relationship between the mother and baby’s intestinal microbiota to illustrate the influence of HMOs on the mother and baby’s microbiota. Preselected keywords, including ‘human milk’, ‘human milk oligosaccharides’, ‘breast’, ‘mammary gland’, ‘microbiota’, ‘infants’, ‘intestinal’, ‘gut’, ‘fucosyllactose’, ‘sialyllactose’, ‘lacto-N-tetraose’, ‘lacto-N-neotetraose’, ‘probiotics’, ‘pathogenic bacteria’, ‘immune’, ‘inflammation’ and ‘gut diseases’, were entered into academic databases and search engines, including PubMed, Medline and Cochrane Library. Although many supporting publications were retrieved, in total, nine human clinical studies (Table 1) were included to help clarify our current understanding of HMOs and diseases. Searches were summarized using a PICO framework where population (P) terms were combined with intervention (I) and, in some cases, comparison (C) terms. Outcomes (O) showed the therapeutic effect of HMOs.
Author contributions
WS: Writing – original draft. LT: Data curation, Writing – review & editing. CQ: Data curation, Writing – review & editing. PPX: Data curation, Writing – review & editing. SSD: Data curation, Writing – review & editing. YNT: Conceptualization, Writing – review & editing.
Funding
The author(s) declare financial support was received for the research, authorship, and/or publication of this article. This work was supported by Project of Integrated Traditional Chinese and Western Medicine in General Hospital of Shanghai Health Commission (ZHYY-ZXY-JHZX-202110); The Taditional Chinese Medicine Department Construction Project of Shanghai Hongkou District Health Committee (HKGYQYXM-2022-05, HKGYQYXM-2022-21); Subject Boosting Program of the Shanghai Fourth People’s Hospital Affiliated to Tongji University (SY-XKZT-2020-2002); Shanghai Hospital of Traditional Chinese Medicine High-level Leading Program of Shanghai Health Commission; The Scientific and Economic Committee Project of Shanghai Hongkou District (HKZYY-2024-03).
Acknowledgments
Throughout the writing of this review, I received a great deal of support and assistance. I would first like to thank the Shanghai Research Institute of Acupuncture and Meridian for their valuable insights in formulating the research questions. I would also like to thank the writing assistance provided by Shanghai Fourth People’s Hospital Affiliated to Tongji University.
Conflict of interest
The authors declare that the research was conducted in the absence of any commercial or financial relationships that could be construed as a potential conflict of interest.
Publisher’s note
All claims expressed in this article are solely those of the authors and do not necessarily represent those of their affiliated organizations, or those of the publisher, the editors and the reviewers. Any product that may be evaluated in this article, or claim that may be made by its manufacturer, is not guaranteed or endorsed by the publisher.
References
Aakko, J., Kumar, H., Rautava, S., Wise, A., Autran, C., Bode, L., et al. (2017). Human milk oligosaccharide categories define the microbiota composition in human colostrum. Benef Microbes 8, 563–567. doi: 10.3920/BM2016.0185
Ackerman, D. L., Doster, R. S., Weitkamp, J. H., Aronoff, D. M., Gaddy, J. A., Townsend, S. D. (2017). Human milk oligosaccharides exhibit antimicrobial and antibiofilm properties against group B streptococcus. ACS Infect. Dis. 3, 595–605. doi: 10.1021/acsinfecdis.7b00064
Akkerman, R., Logtenberg, M. J., Beukema, M., de Haan, B. J., Faas, M. M., Zoetendal, E. G., et al. (2022). Combining galacto-oligosaccharides and 2'-fucosyllactose alters their fermentation kinetics by infant fecal microbiota and influences AhR-receptor dependent cytokine responses in immature dendritic cells. Food Funct. 13, 6510–6521. doi: 10.1039/D2FO00550F
Aldegheri, L., Kharrat, F., Conti, A., Monica, F., Busa, F., Campisciano, G., et al. (2024). Impact of human milk oligosaccharides and probiotics on gut microbiome and mood in autism: A case report. Microorganisms 12 (8), 1625. doi: 10.3390/microorganisms12081625
Alliet, P., Vandenplas, Y., Roggero, P., Jespers, S. N. J., Peeters, S., Stalens, J. P., et al. (2022). Safety and efficacy of a probiotic-containing infant formula supplemented with 2'-fucosyllactose: a double-blind randomized controlled trial. Nutr. J. 21 (1), 11. doi: 10.1186/s12937-022-00764-2
Amin, H. M., Hashem, A. M., Ashour, M. S., Hatti-Kaul, R. (2013). 1,2 Propanediol utilization by Lactobacillus reuteri DSM 20016, role in bioconversion of glycerol to 1,3 propanediol, 3-hydroxypropionaldehyde and 3-hydroxypropionic acid. J. Genet. Eng. Biotechnol. 11, 53–59. doi: 10.1016/j.jgeb.2012.12.002
Austin, S., Bénet, T. (2018). Quantitative determination of non-lactose milk oligosaccharides. Anal. Chim. Acta 1010, 86–96. doi: 10.1016/j.aca.2017.12.036
Autran, C. A., Schoterman, M. H., Jantscher-Krenn, E., Kamerling, J. P., Bode, L. (2016). Sialylated galacto-oligosaccharides and 2'-fucosyllactose reduce necrotising enterocolitis in neonatal rats. Br. J. Nutr. 116, 294–299. doi: 10.1017/S0007114516002038
Azad, M. B., Robertson, B., Atakora, F., Becker, A. B., Subbarao, P., Moraes, T. J., et al. (2018). Human milk oligosaccharide concentrations are associated with multiple fixed and modifiable maternal characteristics, environmental factors, and feeding practices. J. Nutr. 148, 1733–1742. doi: 10.1093/jn/nxy175
Bachem, A., Makhlouf, C., Binger, K. J., de Souza, D. P., Tull, D., Hochheiser, K., et al. (2019). Microbiota-derived short-chain fatty acids promote the memory potential of antigen-activated CD8(+) T cells. Immunity. 51, 285–97.e5. doi: 10.1016/j.immuni.2019.06.002
Banić, M., Butorac, K., Čuljak, N., Leboš Pavunc, A., Novak, J., Bellich, B., et al. (2022). The human milk microbiota produces potential therapeutic biomolecules and shapes the intestinal microbiota of infants. Int. J. Mol. Sci. 23 (22), 14382. doi: 10.3390/ijms232214382
Bao, Y., Zhu, L., Newburg, D. S. (2007). Simultaneous quantification of sialyloligosaccharides from human milk by capillary electrophoresis. Anal. Biochem. 370, 206–214. doi: 10.1016/j.ab.2007.07.004
Becerra, J. E., Rodríguez-Díaz, J., Gozalbo-Rovira, R., Palomino-Schätzlein, M., Zúñiga, M., Monedero, V., et al. (2020). Unique microbial catabolic pathway for the human core N-glycan constituent fucosyl-α-1,6-N-acetylglucosamine-asparagine. mBio 11 (1), e02804-19. doi: 10.1128/mBio.02804-19
Beghetti, I., Panizza, D., Lenzi, J., Gori, D., Martini, S., Corvaglia, L., et al. (2021). Probiotics for preventing necrotizing enterocolitis in preterm infants: A network meta-analysis. Nutrients 13 (1), 192. doi: 10.3390/nu13010192
Belzer, C., Chia, L. W., Aalvink, S., Chamlagain, B., Piironen, V., Knol, J., et al. (2017). Microbial metabolic networks at the mucus layer lead to diet-independent butyrate and vitamin B(12) production by intestinal symbionts. mBio 8 (5), e00770-17. doi: 10.1128/mBio.00770-17
Berger, B., Porta, N., Foata, F., Grathwohl, D., Delley, M., Moine, D., et al. (2020). Linking human milk oligosaccharides, infant fecal community types, and later risk to require antibiotics. mBio 11 (2), e03196-19. doi: 10.1128/mBio.03196-19
Bianchi, F., Larsen, N., de Mello Tieghi, T., Adorno, M. A. T., Kot, W., Saad, S. M. I., et al. (2018). Modulation of gut microbiota from obese individuals by in vitro fermentation of citrus pectin in combination with Bifidobacterium longum BB-46. Appl. Microbiol. Biotechnol. 102, 8827–8840. doi: 10.1007/s00253-018-9234-8
Bode, L. (2012). Human milk oligosaccharides: every baby needs a sugar mama. Glycobiology. 22, 1147–1162. doi: 10.1093/glycob/cws074
Boggio Marzet, C., Burgos, F., Del Compare, M., Gerold, I., Tabacco, O., Vinderola, G. (2022). Approach to probiotics in pediatrics: the role of Lactobacillus rhamnosus GG. Arch. Argent Pediatr. 120, e1–e7. doi: 10.5546/aap.2022.eng.e1
Borewicz, K., Gu, F., Saccenti, E., Arts, I., Penders, J., Thijs, C., et al. (2019). Correlating infant faecal microbiota composition and human milk oligosaccharide consumption by microbiota of one-month old breastfed infants. Mol. Nutr. Food Res. 63 (13), e1801214. doi: 10.1002/mnfr.201801214
Cabrera-Rubio, R., Kunz, C., Rudloff, S., García-Mantrana, I., Crehuá-Gaudiza, E., Martínez-Costa, C., et al. (2019). Association of maternal secretor status and human milk oligosaccharides with milk microbiota: an observational pilot study. J. Pediatr. Gastroenterol. Nutr. 68, 256–263. doi: 10.1097/MPG.0000000000002216
Canakis, A., Haroon, M., Weber, H. C. (2020). Irritable bowel syndrome and gut microbiota. Curr. Opin. Endocrinol. Diabetes Obes. 27, 28–35. doi: 10.1097/MED.0000000000000523
Castillo-Courtade, L., Han, S., Lee, S., Mian, F. M., Buck, R., Forsythe, P. (2015). Attenuation of food allergy symptoms following treatment with human milk oligosaccharides in a mouse model. Allergy. 70, 1091–1102. doi: 10.1111/all.2015.70.issue-9
Catenza, K. F., Donkor, K. K. (2021). Recent approaches for the quantitative analysis of functional oligosaccharides used in the food industry: A review. Food Chem. 355, 129416. doi: 10.1016/j.foodchem.2021.129416
Charbonneau, M. R., O'Donnell, D., Blanton, L. V., Totten, S. M., Davis, J. C., Barratt, M. J., et al. (2016). Sialylated milk oligosaccharides promote microbiota-dependent growth in models of infant undernutrition. Cell. 164, 859–871. doi: 10.1016/j.cell.2016.01.024
Cheema, A. S., Gridneva, Z., Furst, A. J., Roman, A. S., Trevenen, M. L., Turlach, B. A., et al. (2022). Human milk oligosaccharides and bacterial profile modulate infant body composition during exclusive breastfeeding. Int. J. Mol. Sci. 23 (5), 2865. doi: 10.3390/ijms23052865
Chen, Y., Cui, W., Li, X., Yang, H. (2021a). Interaction between commensal bacteria, immune response and the intestinal barrier in inflammatory bowel disease. Front. Immunol. 12, 761981. doi: 10.3389/fimmu.2021.761981
Chen, Y., Zhou, J., Wang, L. (2021b). Role and mechanism of gut microbiota in human disease. Front. Cell Infect. Microbiol. 11, 625913. doi: 10.3389/fcimb.2021.625913
Cheng, L., Kong, C., Wang, W., Groeneveld, A., Nauta, A., Groves, M. R., et al. (2021). The human milk oligosaccharides 3-FL, lacto-N-neotetraose, and LDFT attenuate tumor necrosis factor-α Induced inflammation in fetal intestinal epithelial cells in vitro through shedding or interacting with tumor necrosis factor receptor 1. Mol. Nutr. Food Res. 65, e2000425. doi: 10.1002/mnfr.202000425
Cowardin, C. A., Ahern, P. P., Kung, V. L., Hibberd, M. C., Cheng, J., Guruge, J. L., et al. (2019). Mechanisms by which sialylated milk oligosaccharides impact bone biology in a gnotobiotic mouse model of infant undernutrition. Proc. Natl. Acad. Sci. U S A. 116, 11988–11996. doi: 10.1073/pnas.1821770116
Crane, J. K., Azar, S. S., Stam, A., Newburg, D. S. (1994). Oligosaccharides from human milk block binding and activity of the Escherichia coli heat-stable enterotoxin (STa) in T84 intestinal cells. J. Nutr. 124, 2358–2364. doi: 10.1093/jn/124.12.2358
Cui, L., Guan, X., Ding, W., Luo, Y., Wang, W., Bu, W., et al. (2021). Scutellaria baicalensis Georgi polysaccharide ameliorates DSS-induced ulcerative colitis by improving intestinal barrier function and modulating gut microbiota. Int. J. Biol. Macromol. 166, 1035–1045. doi: 10.1016/j.ijbiomac.2020.10.259
Cukrowska, B., Bierła, J. B., Zakrzewska, M., Klukowski, M., Maciorkowska, E. (2020). The relationship between the infant gut microbiota and allergy. The role of bifidobacterium breve and prebiotic oligosaccharides in the activation of anti-allergic mechanisms in early life. Nutrients. 12 (4), 946. doi: 10.3390/nu12040946
Damaceno, Q. S., Souza, J. P., Nicoli, J. R., Paula, R. L., Assis, G. B., Figueiredo, H. C., et al. (2017). Evaluation of potential probiotics isolated from human milk and colostrum. Probiotics Antimicrob. Proteins. 9, 371–379. doi: 10.1007/s12602-017-9270-1
Dedon, L. R., Hilliard, M. A., Rani, A., Daza-Merchan, Z. T., Story, G., Briere, C. E., et al. (2023). Fucosylated Human Milk Oligosaccharides Drive Structure-Specific Syntrophy between Bifidobacterium infantis and Eubacterium hallii within a Modeled Infant Gut Microbiome. Mol. Nutr. Food Res. 67, e2200851. doi: 10.1002/mnfr.202200851
Demmelmair, H., Jiménez, E., Collado, M. C., Salminen, S., McGuire, M. K. (2020). Maternal and perinatal factors associated with the human milk microbiome. Curr. Dev. Nutr. 4, nzaa027. doi: 10.1093/cdn/nzaa027
Denamur, E., Clermont, O., Bonacorsi, S., Gordon, D. (2021). The population genetics of pathogenic Escherichia coli. Nat. Rev. Microbiol. 19, 37–54. doi: 10.1038/s41579-020-0416-x
Dimidi, E., Christodoulides, S., Scott, S. M., Whelan, K. (2017). Mechanisms of action of probiotics and the gastrointestinal microbiota on gut motility and constipation. Adv. Nutr. 8, 484–494. doi: 10.3945/an.116.014407
Dogra, S. K., Martin, F. P., Donnicola, D., Julita, M., Berger, B., Sprenger, N. (2021). Human milk oligosaccharide-stimulated bifidobacterium species contribute to prevent later respiratory tract infections. Microorganisms. 9 (9), 1939. doi: 10.3390/microorganisms9091939
Drobyshevsky, A., Synowiec, S., Goussakov, I., Fabres, R., Lu, J., Caplan, M. (2024). Intestinal microbiota modulates neuroinflammatory response and brain injury after neonatal hypoxia-ischemia. Gut Microbes 16, 2333808. doi: 10.1080/19490976.2024.2333808
Duar, R. M., Casaburi, G., Mitchell, R. D., Scofield, L. N. C., Ortega Ramirez, C. A., Barile, D., et al. (2020). Comparative Genome Analysis of Bifidobacterium longum subsp. infantis Strains Reveals Variation in Human Milk Oligosaccharide Utilization Genes among Commercial Probiotics. Nutrients 12 (11), 3247. doi: 10.3390/nu12113247
Engels, C., Ruscheweyh, H.-J., Beerenwinkel, N., Lacroix, C., Schwab, C. (2016). The Common Gut Microbe Eubacterium hallii also Contributes to Intestinal Propionate Formation. Front. Microbiol. 7, 713. doi: 10.3389/fmicb.2016.00713
Ewald, D. R., Sumner, S. C. (2016). Blood type biochemistry and human disease. Wiley Interdiscip Rev. Syst. Biol. Med. 8, 517–535. doi: 10.1002/wsbm.1355
Ferrier, L., Eutamène, H., Siegwald, L., Marquard, A. M., Tondereau, V., Chevalier, J., et al. (2022). Human milk oligosaccharides alleviate stress-induced visceral hypersensitivity and associated microbiota dysbiosis. J. Nutr. Biochem. 99, 108865. doi: 10.1016/j.jnutbio.2021.108865
Foata, F., Sprenger, N., Rochat, F., Damak, S. (2020). Activation of the G-protein coupled receptor GPR35 by human milk oligosaccharides through different pathways. Sci. Rep. 10, 16117. doi: 10.1038/s41598-020-73008-0
Fonvig, C. E., Amundsen, I. D., Vigsnæs, L. K., Sørensen, N., Frithioff-Bøjsøe, C., Christiansen, M., et al. (2021). Human milk oligosaccharides modulate fecal microbiota and are safe for use in children with overweight: A randomized controlled trial. J. Pediatr. Gastroenterol. Nutr. 73, 408–414. doi: 10.1097/MPG.0000000000003205
Gao, W., Xiao, M., Gu, Z., Fu, X., Ren, X., Yu, Y., et al. (2022). Genome analysis and 2'-fucosyllactose utilization characteristics of a new Akkermansia muciniphila strain isolated from mice feces. Mol. Genet. Genomics 297, 1515–1528. doi: 10.1007/s00438-022-01937-8
Gart, E., Salic, K., Morrison, M. C., Giera, M., Attema, J., de Ruiter, C., et al. (2022). The human milk oligosaccharide 2'-fucosyllactose alleviates liver steatosis, ER stress and insulin resistance by reducing hepatic diacylglycerols and improved gut permeability in obese ldlr-/-.Leiden Mice. Front. Nutr. 9, 904740. doi: 10.3389/fnut.2022.904740
Geddes, D. T. (2009). The use of ultrasound to identify milk ejection in women - tips and pitfalls. Int. Breastfeed J. 4, 5. doi: 10.1186/1746-4358-4-5
Gidrewicz, D. A., Fenton, T. R. (2014). A systematic review and meta-analysis of the nutrient content of preterm and term breast milk. BMC Pediatr. 14, 216. doi: 10.1186/1471-2431-14-216
Glassner, K. L., Abraham, B. P., Quigley, E. M. M. (2020). The microbiome and inflammatory bowel disease. J. Allergy Clin. Immunol. 145, 16–27. doi: 10.1016/j.jaci.2019.11.003
Gómez-Gallego, C., Morales, J. M., Monleón, D., du Toit, E., Kumar, H., Linderborg, K. M., et al. (2018). Human breast milk NMR metabolomic profile across specific geographical locations and its association with the milk microbiota. Nutrients 10 (10), 1355. doi: 10.3390/nu10101355
Good, M., Sodhi, C. P., Yamaguchi, Y., Jia, H., Lu, P., Fulton, W. B., et al. (2016). The human milk oligosaccharide 2'-fucosyllactose attenuates the severity of experimental necrotising enterocolitis by enhancing mesenteric perfusion in the neonatal intestine. Br. J. Nutr. 116, 1175–1187. doi: 10.1017/S0007114516002944
Guo, C. J., Allen, B. M., Hiam, K. J., Dodd, D., Van Treuren, W., Higginbottom, S., et al. (2019). Depletion of microbiome-derived molecules in the host using Clostridium genetics. Science 366 (6471), eaav1282. doi: 10.1126/science.aav1282
Gutiérrez-Vázquez, C., Quintana, F. J. (2018). Regulation of the immune response by the aryl hydrocarbon receptor. Immunity. 48, 19–33. doi: 10.1016/j.immuni.2017.12.012
Guzman-Bautista, E. R., Suzuki, K., Asami, S., Fagarasan, S. (2020). Bacteria-immune cells dialog and the homeostasis of the systems. Curr. Opin. Immunol. 66, 82–89. doi: 10.1016/j.coi.2020.05.010
Hammer, A. J., Walters, A., Carroll, C., Newell, P. D., Chaston, J. M. (2017). Draft genome sequence of lactobacillus paracasei dmW181, a bacterium isolated from wild drosophila. Genome Announc. 5 (27), e00545-17. doi: 10.1128/genomeA.00545-17
Han, S. M., Derraik, J. G. B., Binia, A., Sprenger, N., Vickers, M. H., Cutfield, W. S. (2021). Maternal and infant factors influencing human milk oligosaccharide composition: beyond maternal genetics. J. Nutr. 151, 1383–1393. doi: 10.1093/jn/nxab028
Hanisch, F. G., Kunz, C. (2021). Novel class of human milk oligosaccharides based on 6'-galactosyllactose containing N-acetylglucosamine branches extended by oligogalactoses. J. Proteome Res. 20, 3865–3874. doi: 10.1021/acs.jproteome.1c00154
He, Y., He, Z., Leone, S., Liu, S. (2021). Milk exosomes transfer oligosaccharides into macrophages to modulate immunity and attenuate adherent-invasive E. coli (AIEC) Infection. Nutrients. 13 (9), 3198. doi: 10.3390/nu13093198
He, Y., Liu, S., Kling, D. E., Leone, S., Lawlor, N. T., Huang, Y., et al. (2016). The human milk oligosaccharide 2'-fucosyllactose modulates CD14 expression in human enterocytes, thereby attenuating LPS-induced inflammation. Gut. 65, 33–46. doi: 10.1136/gutjnl-2014-307544
He, J., Zhang, P., Shen, L., Niu, L., Tan, Y., Chen, L., et al. (2020). Short-chain fatty acids and their association with signalling pathways in inflammation, glucose and lipid metabolism. Int. J. Mol. Sci. 21 (17), 6356. doi: 10.3390/ijms21176356
He-Yang, J., Zhang, W., Liu, J., Xue, P., Zhou, X. (2020). Human breast milk oligosaccharides attenuate necrotizing enterocolitis in rats by suppressing mast cell accumulation, DPPI activity and TLR4 expression in ileum tissue, and regulating mitochondrial damage of Caco-2 cells. Int. Immunopharmacol. 88, 106881. doi: 10.1016/j.intimp.2020.106881
Holscher, H. D., Bode, L., Tappenden, K. A. (2017). Human milk oligosaccharides influence intestinal epithelial cell maturation in vitro. J. Pediatr. Gastroenterol. Nutr. 64, 296–301. doi: 10.1097/MPG.0000000000001274
Holscher, H. D., Davis, S. R., Tappenden, K. A. (2014). Human milk oligosaccharides influence maturation of human intestinal Caco-2Bbe and HT-29 cell lines. J. Nutr. 144, 586–591. doi: 10.3945/jn.113.189704
Huang, Y.-L., Chassard, C., Hausmann, M., von Itzstein, M., Hennet, T. (2015). Sialic acid catabolism drives intestinal inflammation and microbial dysbiosis in mice. Nat. Commun. 6, 8141. doi: 10.1038/ncomms9141
Huang, Z., Liu, B., Xiao, L., Liao, M., Huang, L., Zhao, X., et al. (2024). Effects of breast-fed infants-derived Limosilactobacillus reuteri and Bifidobacterium breve ameliorate DSS-induced colitis in mice. iScience. 27, 110902. doi: 10.1016/j.isci.2024.110902
Huang, X., Zhu, B., Jiang, T., Yang, C., Qiao, W., Hou, J., et al. (2019). Improved simple sample pretreatment method for quantitation of major human milk oligosaccharides using ultrahigh pressure liquid chromatography with fluorescence detection. J. Agric. Food Chem. 67, 12237–12244. doi: 10.1021/acs.jafc.9b03445
Huertas-Díaz, L., Kyhnau, R., Ingribelli, E., Neuzil-Bunesova, V., Li, Q., Sasaki, M., et al. (2023). Breastfeeding and the major fermentation metabolite lactate determine occurrence of Peptostreptococcaceae in infant feces. Gut Microbes 15, 2241209. doi: 10.1080/19490976.2023.2241209
Hung, C. C., Garner, C. D., Slauch, J. M., Dwyer, Z. W., Lawhon, S. D., Frye, J. G., et al. (2013). The intestinal fatty acid propionate inhibits Salmonella invasion through the post-translational control of HilD. Mol. Microbiol. 87, 1045–1060. doi: 10.1111/mmi.2013.87.issue-5
Huynh, U., Qiao, M., King, J., Trinh, B., Valdez, J., Haq, M., et al. (2022). Differential effects of transition metals on growth and metal uptake for two distinct lactobacillus species. Microbiol. Spectr. 10, e0100621. doi: 10.1128/spectrum.01006-21
Iribarren, C., Magnusson, M. K., Vigsnæs, L. K., Aziz, I., Amundsen, I. D., Šuligoj, T., et al. (2021). The effects of human milk oligosaccharides on gut microbiota, metabolite profiles and host mucosal response in patients with irritable bowel syndrome. Nutrients 13 (11), 3836. doi: 10.3390/nu13113836
Iribarren, C., Törnblom, H., Aziz, I., Magnusson, M. K., Sundin, J., Vigsnaes, L. K., et al. (2020). Human milk oligosaccharide supplementation in irritable bowel syndrome patients: A parallel, randomized, double-blind, placebo-controlled study. Neurogastroenterol Motil. 32, e13920. doi: 10.1111/nmo.v32.10
Jackson, P. P. J., Wijeyesekera, A., Rastall, R. A. (2022). Determining the metabolic fate of human milk oligosaccharides: it may just be more complex than you think? Gut Microbiome 3, e9. doi: 10.1017/gmb.2022.8
Jena, P. K., Sheng, L., Nagar, N., Wu, C., Barile, D., Mills, D. A., et al. (2018). The effect of synbiotics Bifidobacterium infantis and milk oligosaccharides on shaping gut microbiota community structure and NASH treatment. Data Brief. 19, 1025–1029. doi: 10.1016/j.dib.2018.05.127
Kayama, H., Okumura, R., Takeda, K. (2020). Interaction between the microbiota, epithelia, and immune cells in the intestine. Annu. Rev. Immunol. 38, 23–48. doi: 10.1146/annurev-immunol-070119-115104
Kim, S. K., Guevarra, R. B., Kim, Y. T., Kwon, J., Kim, H., Cho, J. H., et al. (2019). Role of probiotics in human gut microbiome-associated diseases. J. Microbiol. Biotechnol. 29, 1335–1340. doi: 10.4014/jmb.1906.06064
Kim, Y. J., Kim, H. H., Shin, C. S., Yoon, J. W., Jeon, S. M., Song, Y. H., et al. (2023). 2'-fucosyllactose and 3-fucosyllactose alleviates interleukin-6-induced barrier dysfunction and dextran sodium sulfate-induced colitis by improving intestinal barrier function and modulating the intestinal microbiome. Nutrients 15 (8), 1845. doi: 10.3390/nu15081845
Kohl, K. D., Dearing, M. D., Bordenstein, S. R. (2018). Microbial communities exhibit host species distinguishability and phylosymbiosis along the length of the gastrointestinal tract. Mol. Ecol. 27, 1874–1883. doi: 10.1111/mec.2018.27.issue-8
Kommineni, S., Bretl, D. J., Lam, V., Chakraborty, R., Hayward, M., Simpson, P., et al. (2015). Bacteriocin production augments niche competition by enterococci in the mammalian gastrointestinal tract. Nature. 526, 719–722. doi: 10.1038/nature15524
Kong, C., Akkerman, R., Klostermann, C. E., Beukema, M., Oerlemans, M. M. P., Schols, H. A., et al. (2021). Distinct fermentation of human milk oligosaccharides 3-FL and LNT2 and GOS/inulin by infant gut microbiota and impact on adhesion of Lactobacillus plantarum WCFS1 to gut epithelial cells. Food Funct. 12, 12513–12525. doi: 10.1039/D1FO02563E
Kong, C., Cheng, L., Krenning, G., Fledderus, J., de Haan, B. J., Walvoort, M. T. C., et al. (2020a). Human milk oligosaccharides mediate the crosstalk between intestinal epithelial caco-2 cells and lactobacillus plantarumWCFS1in an in vitro model with intestinal peristaltic shear force. J. Nutr. 150, 2077–2088. doi: 10.1093/jn/nxaa162
Kong, C., Faas, M. M., de Vos, P., Akkerman, R. (2020b). Impact of dietary fibers in infant formulas on gut microbiota and the intestinal immune barrier. Food Funct. 11, 9445–9467. doi: 10.1039/D0FO01700K
Kostopoulos, I., Elzinga, J., Ottman, N., Klievink, J. T., Blijenberg, B., Aalvink, S., et al. (2020). Akkermansia muciniphila uses human milk oligosaccharides to thrive in the early life conditions in vitro. Sci. Rep. 10, 14330. doi: 10.1038/s41598-020-71113-8
Kovács, T., Mikó, E., Ujlaki, G., Sári, Z., Bai, P. (2020). The microbiome as a component of the tumor microenvironment. Adv. Exp. Med. Biol. 1225, 137–153. doi: 10.1007/978-3-030-35727-6_10
Kuntz, S., Rudloff, S., Kunz, C. (2008). Oligosaccharides from human milk influence growth-related characteristics of intestinally transformed and non-transformed intestinal cells. Br. J. Nutr. 99, 462–471. doi: 10.1017/S0007114507824068
Kunz, C., Meyer, C., Collado, M. C., Geiger, L., García-Mantrana, I., Bertua-Ríos, B., et al. (2017). Influence of gestational age, secretor, and lewis blood group status on the oligosaccharide content of human milk. J. Pediatr. Gastroenterol. Nutr. 64, 789–798. doi: 10.1097/MPG.0000000000001402
Lane, J. A., Mehra, R. K., Carrington, S. D., Hickey, R. M. (2011). Development of biosensor-based assays to identify anti-infective oligosaccharides. Anal. Biochem. 410, 200–205. doi: 10.1016/j.ab.2010.11.032
Larsson, M. W., Lind, M. V., Laursen, R. P., Yonemitsu, C., Larnkjær, A., Mølgaard, C., et al. (2019). Human milk oligosaccharide composition is associated with excessive weight gain during exclusive breastfeeding-an explorative study. Front. Pediatr. 7, 297. doi: 10.3389/fped.2019.00297
Laucirica, D. R., Triantis, V., Schoemaker, R., Estes, M. K., Ramani, S. (2017). Milk oligosaccharides inhibit human rotavirus infectivity in MA104 cells. J. Nutr. 147, 1709–1714. doi: 10.3945/jn.116.246090
Le Doare, K., Holder, B., Bassett, A., Pannaraj, P. S. (2018). Mother's milk: A purposeful contribution to the development of the infant microbiota and immunity. Front. Immunol. 9, 361. doi: 10.3389/fimmu.2018.00361
Leth, M. L., Pichler, M. J., Abou Hachem, M. (2022). Butyrate-producing colonic clostridia: picky glycan utilization specialists. Essays Biochem. 67 (3), 415–428. doi: 10.1042/EBC20220125
Li, B., Wu, R. Y., Horne, R. G., Ahmed, A., Lee, D., Robinson, S. C., et al. (2020). Human milk oligosaccharides protect against necrotizing enterocolitis by activating intestinal cell differentiation. Mol. Nutr. Food Res. 64, e2000519. doi: 10.1002/mnfr.202000519
Lin, A. E., Autran, C. A., Szyszka, A., Escajadillo, T., Huang, M., Godula, K., et al. (2017). Human milk oligosaccharides inhibit growth of group B Streptococcus. J. Biol. Chem. 292, 11243–11249. doi: 10.1074/jbc.M117.789974
Lu, Y., Yuan, X., Wang, M., He, Z., Li, H., Wang, J., et al. (2022). Gut microbiota influence immunotherapy responses: mechanisms and therapeutic strategies. J. Hematol. Oncol. 15, 47. doi: 10.1186/s13045-022-01273-9
Lyons, K. E., Ryan, C. A., Dempsey, E. M., Ross, R. P., Stanton, C. (2020). Breast milk, a source of beneficial microbes and associated benefits for infant health. Nutrients. 12 (4), 1039. doi: 10.3390/nu12041039
Ma, L., McJarrow, P., Jan Mohamed, H. J. B., Liu, X., Welman, A., Fong, B. Y. (2018). Lactational changes in the human milk oligosaccharide concentration in Chinese and Malaysian mothers' milk. Int. Dairy J. 87, 1–10. doi: 10.1016/j.idairyj.2018.07.015
Malekiantaghi, A., Ghanaati, F., Shabani-Mirzaee, H., Shariat, M., Mojtahedi, S. Y., Eftekhari, K. (2024). Lactobacillus rhamnosus helps to reduce the duration of bleeding in breastfed infants with allergic proctocolitis. Breastfeed Med. doi: 10.1089/bfm.2024.0185
Manthey, C. F., Autran, C. A., Eckmann, L., Bode, L. (2014). Human milk oligosaccharides protect against enteropathogenic Escherichia coli attachment in vitro and EPEC colonization in suckling mice. J. Pediatr. Gastroenterol. Nutr. 58, 165–168. doi: 10.1097/MPG.0000000000000172
Marcobal, A., Barboza, M., Froehlich, J. W., Block, D. E., German, J. B., Lebrilla, C. B., et al. (2010). Consumption of human milk oligosaccharides by gut-related microbes. J. Agric. Food Chem. 58, 5334–5340. doi: 10.1021/jf9044205
Martin-Gallausiaux, C., Marinelli, L., Blottière, H. M., Larraufie, P., Lapaque, N. (2021). SCFA: mechanisms and functional importance in the gut. Proc. Nutr. Society. 80, 37–49. doi: 10.1017/S0029665120006916
Marwaha, A. K., Morris, J. A., Rigby, R. J. (2020). Hypothesis: Bacterial induced inflammation disrupts the orderly progression of the stem cell hierarchy and has a role in the pathogenesis of breast cancer. Med. Hypotheses. 136, 109530. doi: 10.1016/j.mehy.2019.109530
Matsuki, T., Yahagi, K., Mori, H., Matsumoto, H., Hara, T., Tajima, S., et al. (2016). A key genetic factor for fucosyllactose utilization affects infant gut microbiota development. Nat. Commun. 7, 11939. doi: 10.1038/ncomms11939
McGuire, M. K., McGuire, M. A. (2017). Got bacteria? The astounding, yet not-so-surprising, microbiome of human milk. Curr. Opin. Biotechnol. 44, 63–68. doi: 10.1016/j.copbio.2016.11.013
Méndez, C. S., Bueno, S. M., Kalergis, A. M. (2021). Contribution of gut microbiota to immune tolerance in infants. J. Immunol. Res. 2021, 7823316. doi: 10.1155/2021/7823316
Mielcarek, C., Romond, P. C., Romond, M. B., Bezirtzoglou, E. (2011). Modulation of bacterial translocation in mice mediated through lactose and human milk oligosaccharides. Anaerobe. 17, 361–366. doi: 10.1016/j.anaerobe.2011.09.002
Mills, D. A., German, J. B., Lebrilla, C. B., Underwood, M. A. (2023). Translating neonatal microbiome science into commercial innovation: metabolism of human milk oligosaccharides as a basis for probiotic efficacy in breast-fed infants. Gut Microbes 15, 2192458. doi: 10.1080/19490976.2023.2192458
Moossavi, S., Atakora, F., Miliku, K., Sepehri, S., Robertson, B., Duan, Q. L., et al. (2019). Integrated analysis of human milk microbiota with oligosaccharides and fatty acids in the CHILD cohort. Front. Nutr. 6, 58. doi: 10.3389/fnut.2019.00058
Moossavi, S., Azad, M. B. (2020). Origins of human milk microbiota: new evidence and arising questions. Gut Microbes 12, 1667722. doi: 10.1080/19490976.2019.1667722
Morissette, R., Mihalov, J., Carlson, S. J., Kaneko, K. J. (2023). Trends in ingredients added to infant formula: FDA's experiences in the GRAS notification program. Food Chem. Toxicol. 178, 113876. doi: 10.1016/j.fct.2023.113876
Moschino, L., Verlato, G., Duci, M., Cavicchiolo, M. E., Guiducci, S., Stocchero, M., et al. (2022). The metabolome and the gut microbiota for the prediction of necrotizing enterocolitis and spontaneous intestinal perforation: A systematic review. Nutrients 14 (8), 3859. doi: 10.3390/nu14183859
Moya-Gonzálvez, E. M., Rubio-Del-Campo, A., Rodríguez-Díaz, J., Yebra, M. J. (2021). Infant-gut associated Bifidobacterium dentium strains utilize the galactose moiety and release lacto-N-triose from the human milk oligosaccharides lacto-N-tetraose and lacto-N-neotetraose. Sci. Rep. 11, 23328. doi: 10.1038/s41598-021-02741-x
Musilova, S., Modrackova, N., Hermanova, P., Hudcovic, T., Svejstil, R., Rada, V., et al. (2017). Assessment of the synbiotic properites of human milk oligosaccharides and Bifidobacterium longum subsp. infantis in vitro and in humanised mice. Benef Microbes 8, 281–289. doi: 10.3920/BM2016.0138
Natividad, J. M., Marsaux, B., Rodenas, C. L. G., Rytz, A., Vandevijver, G., Marzorati, M., et al. (2022). Human milk oligosaccharides and lactose differentially affect infant gut microbiota and intestinal barrier in vitro. Nutrients 14 (12), 2546. doi: 10.3390/nu14122546
Natividad, J. M., Rytz, A., Keddani, S., Bergonzelli, G., Garcia-Rodenas, C. L. (2020). Blends of human milk oligosaccharides confer intestinal epithelial barrier protection in vitro. Nutrients. 12 (10), 3047. doi: 10.3390/nu12103047
Ng, K. M., Ferreyra, J. A., Higginbottom, S. K., Lynch, J. B., Kashyap, P. C., Gopinath, S., et al. (2013). Microbiota-liberated host sugars facilitate post-antibiotic expansion of enteric pathogens. Nature. 502, 96–99. doi: 10.1038/nature12503
Niemarkt, H. J., De Meij, T. G., van Ganzewinkel, C. J., de Boer, N. K. H., Andriessen, P., Hütten, M. C., et al. (2019). Necrotizing enterocolitis, gut microbiota, and brain development: role of the brain-gut axis. Neonatology. 115, 423–431. doi: 10.1159/000497420
Nilsen, M., Madelen Saunders, C., Leena Angell, I., Arntzen, M., Lødrup Carlsen, K. C., Carlsen, K. H., et al. (2020). Butyrate levels in the transition from an infant- to an adult-like gut microbiota correlate with bacterial networks associated with eubacterium rectale and ruminococcus gnavus. Genes (Basel) 11 (11), 1245. doi: 10.3390/genes11111245
Nogacka, A. M., Arboleya, S., Nikpoor, N., Auger, J., Salazar, N., Cuesta, I., et al. (2021). Influence of 2'-fucosyllactose on the microbiota composition and metabolic activity of fecal cultures from breastfed and formula-fed infants at two months of age. Microorganisms 9 (7), 1478. doi: 10.3390/microorganisms9071478
Nolan, L., Rimer, J., Good, M. (2020). The role of human milk oligosaccharides and probiotics on the neonatal microbiome and risk of necrotizing enterocolitis: A narrative review. Nutrients. 12 (10), 3052. doi: 10.3390/nu12103052
O'Callaghan, A., Bottacini, F., O'Connell Motherway, M., van Sinderen, D. (2015). Pangenome analysis of Bifidobacterium longum and site-directed mutagenesis through by-pass of restriction-modification systems. BMC Genomics 16, 832. doi: 10.1186/s12864-015-1968-4
Ottman, N., Davids, M., Suarez-Diez, M., Boeren, S., Schaap, P. J., Martins Dos Santos, V. A. P., et al. (2017). Genome-scale model and omics analysis of metabolic capacities of akkermansia muciniphila reveal a preferential mucin-degrading lifestyle. Appl. Environ. Microbiol. 83 (18), e01014-17. doi: 10.1128/AEM.01014-17
Pannaraj, P. S., Li, F., Cerini, C., Bender, J. M., Yang, S., Rollie, A., et al. (2017). Association between breast milk bacterial communities and establishment and development of the infant gut microbiome. JAMA Pediatr. 171, 647–654. doi: 10.1001/jamapediatrics.2017.0378
Parada Venegas, D., de la Fuente, M. K., Landskron, G., González, M. J., Quera, R., Dijkstra, G., et al. (2019). Short chain fatty acids (SCFAs)-mediated gut epithelial and immune regulation and its relevance for inflammatory bowel diseases. Front. Immunol. 10, 277. doi: 10.3389/fimmu.2019.00277
Patterson, E., Ryan, P. M., Cryan, J. F., Dinan, T. G., Ross, R. P., Fitzgerald, G. F., et al. (2016). Gut microbiota, obesity and diabetes. Postgrad Med. J. 92, 286–300. doi: 10.1136/postgradmedj-2015-133285
Peterson, T., Nagy, G. (2021). Toward sequencing the human milk glycome: high-resolution cyclic ion mobility separations of core human milk oligosaccharide building blocks. Analytical Chem. 93, 9397–9407. doi: 10.1021/acs.analchem.1c00942
Pickard, J. M., Zeng, M. Y., Caruso, R., Núñez, G. (2017). Gut microbiota: Role in pathogen colonization, immune responses, and inflammatory disease. Immunol. Rev. 279, 70–89. doi: 10.1111/imr.2017.279.issue-1
Piotrowski, M., Wultańska, D., Pituch, H. (2022). The prebiotic effect of human milk oligosaccharides 3'- and 6'-sialyllactose on adhesion and biofilm formation by Clostridioides difficile - pilot study. Microbes Infect. 24, 104929. doi: 10.1016/j.micinf.2021.104929
Porfirio, S., Archer-Hartmann, S., Moreau, G. B., Ramakrishnan, G., Haque, R., Kirkpatrick, B. D., et al. (2020). New strategies for profiling and characterization of human milk oligosaccharides. Glycobiology. 30, 774–786. doi: 10.1093/glycob/cwaa028
Porro, M., Kundrotaite, E., Mellor, D., Munialo, C. (2022). A narrative review of the functional components of human breast milk and their potential to modulate the gut microbiome, the consideration of maternal and child characteristics, and confounders of breastfeeding, and their impact on risk of obesity later in life. Nutr. Rev. 81 (5), 597–609. doi: 10.1093/nutrit/nuac072
Poulsen, K., Meng, F., Lanfranchi, E., Young, J., Stanton, C., Ryan, C., et al. (2022). Dynamic changes in the human milk metabolome over 25 weeks of lactation. Front. Nutr. 9, 917659. doi: 10.3389/fnut.2022.917659
Prete, R., Long, S. L., Gallardo, A. L., Gahan, C. G., Corsetti, A., Joyce, S. A. (2020). Beneficial bile acid metabolism from Lactobacillus plantarum of food origin. Sci. Rep. 10, 1165. doi: 10.1038/s41598-020-58069-5
Qasim, M., Wrage, M., Nüse, B., Mattner, J. (2022). Shigella outer membrane vesicles as promising targets for vaccination. Int. J. Mol. Sci. 23 (2), 994. doi: 10.3390/ijms23020994
Quin, C., Vicaretti, S. D., Mohtarudin, N. A., Garner, A. M., Vollman, D. M., Gibson, D. L., et al. (2020). Influence of sulfonated and diet-derived human milk oligosaccharides on the infant microbiome and immune markers. J. Biol. Chem. 295, 4035–4048. doi: 10.1074/jbc.RA119.011351
Quinn, E., Joshi, L., Hickey, R. (2020). Symposium review: Dairy-derived oligosaccharides-Their influence on host-microbe interactions in the gastrointestinal tract of infants. J. dairy science. 103, 3816–3827. doi: 10.3168/jds.2019-17645
Ramani, S., Stewart, C. J., Laucirica, D. R., Ajami, N. J., Robertson, B., Autran, C. A., et al. (2018). Human milk oligosaccharides, milk microbiome and infant gut microbiome modulate neonatal rotavirus infection. Nat. Commun. 9, 5010. doi: 10.1038/s41467-018-07476-4
Remoroza, C. A., Mak, T. D., De Leoz, M. L. A., Mirokhin, Y. A., Stein, S. E. (2018). Creating a mass spectral reference library for oligosaccharides in human milk. Anal. Chem. 90, 8977–8988. doi: 10.1021/acs.analchem.8b01176
Reverri, E. J., Devitt, A. A., Kajzer, J. A., Baggs, G. E., Borschel, M. W. (2018). Review of the clinical experiences of feeding infants formula containing the human milk oligosaccharide 2'-fucosyllactose. Nutrients. 10 (10), 1346. doi: 10.3390/nu10101346
Rodríguez, J. M. (2014). The origin of human milk bacteria: is there a bacterial entero-mammary pathway during late pregnancy and lactation? Adv. Nutr. 5, 779–784. doi: 10.3945/an.114.007229
Rodríguez-Díaz, J., Monedero, V., Yebra, M. (2011). Utilization of natural fucosylated oligosaccharides by three novel alpha-L-fucosidases from a probiotic Lactobacillus casei strain. Appl. Environ. Microbiol. 77 (2), 703–705.
Rothhammer, V., Quintana, F. J. (2019). The aryl hydrocarbon receptor: an environmental sensor integrating immune responses in health and disease. Nat. Rev. Immunol. 19, 184–197. doi: 10.1038/s41577-019-0125-8
Rubio-Del-Campo, A., Gozalbo-Rovira, R., Moya-Gonzálvez, E. M., Alberola, J., Rodríguez-Díaz, J., Yebra, M. J. (2021). Infant gut microbiota modulation by human milk disaccharides in humanized microbiome mice. Gut Microbes 13, 1–20. doi: 10.1080/19490976.2021.1914377
Ruhaak, L. R., Lebrilla, C. B. (2012). Advances in analysis of human milk oligosaccharides. Adv. Nutr. 3 (3), 406S–14S. doi: 10.3945/an.112.001883
Ruiz-Palacios, G. M., Cervantes, L. E., Ramos, P., Chavez-Munguia, B., Newburg, D. S. (2003). Campylobacter jejuni binds intestinal H(O) antigen (Fuc alpha 1, 2Gal beta 1, 4GlcNAc), and fucosyloligosaccharides of human milk inhibit its binding and infection. J. Biol. Chem. 278, 14112–14120. doi: 10.1074/jbc.M207744200
Saben, J. L., Sims, C. R., Abraham, A., Bode, L., Andres, A. (2021). Human milk oligosaccharide concentrations and infant intakes are associated with maternal overweight and obesity and predict infant growth. Nutrients. 13 (2), 446. doi: 10.3390/nu13020446
Sakanaka, M., Gotoh, A., Yoshida, K., Odamaki, T., Koguchi, H., Xiao, J., et al. (2019a). BifidobacteriumVaried pathways of infant gut-associated to assimilate human milk oligosaccharides: prevalence of the gene set and its correlation with bifidobacteria-rich microbiota formation. Nutrients 12 (1), 71. doi: 10.3390/nu12010071
Sakanaka, M., Hansen, M. E., Gotoh, A., Katoh, T., Yoshida, K., Odamaki, T., et al. (2019b). Evolutionary adaptation in fucosyllactose uptake systems supports bifidobacteria-infant symbiosis. Sci. Adv. 5, eaaw7696. doi: 10.1126/sciadv.aaw7696
Salli, K., Anglenius, H., Hirvonen, J., Hibberd, A. A., Ahonen, I., Saarinen, M. T., et al. (2019). The effect of 2'-fucosyllactose on simulated infant gut microbiome and metabolites; a pilot study in comparison to GOS and lactose. Sci. Rep. 9, 13232. doi: 10.1038/s41598-019-49497-z
Salli, K., Söderling, E., Hirvonen, J., Gürsoy, U. K., Ouwehand, A. C. (2020). Influence of 2'-fucosyllactose and galacto-oligosaccharides on the growth and adhesion of Streptococcus mutans. Br. J. Nutr. 124, 824–831. doi: 10.1017/S0007114520001956
Samara, J., Moossavi, S., Alshaikh, B., Ortega, V. A., Pettersen, V. K., Ferdous, T., et al. (2022). Supplementation with a probiotic mixture accelerates gut microbiome maturation and reduces intestinal inflammation in extremely preterm infants. Cell Host Microbe 30, 696–711.e5. doi: 10.1016/j.chom.2022.04.005
Samuel, T. M., Binia, A., de Castro, C. A., Thakkar, S. K., Billeaud, C., Agosti, M., et al. (2019). Impact of maternal characteristics on human milk oligosaccharide composition over the first 4 months of lactation in a cohort of healthy European mothers. Sci. Rep. 9, 11767. doi: 10.1038/s41598-019-48337-4
Sarkozy, D., Borza, B., Domokos, A., Varadi, E., Szigeti, M., Meszaros-Matwiejuk, A., et al. (2021). Ultrafast high-resolution analysis of human milk oligosaccharides by multicapillary gel electrophoresis. Food Chem. 341, 128200. doi: 10.1016/j.foodchem.2020.128200
Sassone-Corsi, M., Nuccio, S. P., Liu, H., Hernandez, D., Vu, C. T., Takahashi, A. A., et al. (2016). Microcins mediate competition among Enterobacteriaceae in the inflamed gut. Nature. 540, 280–283. doi: 10.1038/nature20557
Seferovic, M. D., Mohammad, M., Pace, R. M., Engevik, M., Versalovic, J., Bode, L., et al. (2020). Maternal diet alters human milk oligosaccharide composition with implications for the milk metagenome. Sci. Rep. 10, 22092. doi: 10.1038/s41598-020-79022-6
Selma-Royo, M., González, S., Gueimonde, M., Chang, M., Fürst, A., Martínez-Costa, C., et al. (2022). Maternal diet is associated with human milk oligosaccharide profile. Mol. Nutr. Food Res. 66, e2200058. doi: 10.1002/mnfr.202200058
Shao, Y., Forster, S. C., Tsaliki, E., Vervier, K., Strang, A., Simpson, N., et al. (2019). Stunted microbiota and opportunistic pathogen colonization in caesarean-section birth. Nature. 574, 117–121. doi: 10.1038/s41586-019-1560-1
Simeoni, U., Berger, B., Junick, J., Blaut, M., Pecquet, S., Rezzonico, E., et al. (2016). Gut microbiota analysis reveals a marked shift to bifidobacteria by a starter infant formula containing a synbiotic of bovine milk-derived oligosaccharides and Bifidobacterium animalis subsp. lactis CNCM I-3446. Environ. Microbiol. 18, 2185–2195. doi: 10.1111/emi.2016.18.issue-7
Sindi, A. S., Geddes, D. T., Wlodek, M. E., Muhlhausler, B. S., Payne, M. S., Stinson, L. F. (2021). Can we modulate the breastfed infant gut microbiota through maternal diet? FEMS Microbiol. Rev. 45 (5), fuab011. doi: 10.1093/femsre/fuab011
Smilowitz, J. T., Lebrilla, C. B., Mills, D. A., German, J. B., Freeman, S. L. (2014). Breast milk oligosaccharides: structure-function relationships in the neonate. Annu. Rev. Nutr. 34, 143–169. doi: 10.1146/annurev-nutr-071813-105721
Sodhi, C. P., Ahmad, R., Fulton, W. B., Lopez, C., Eke, B., Scheese, D., et al. (2023). Human milk oligosaccharides reduce necrotizing enterocolitis induced-neuroinflammation and cognitive impairment in mice. Am. J. Physiol. Gastrointest Liver Physiol. 325 (1), G23–G41 doi: 10.1152/ajpgi.00233.2022
Sodhi, C. P., Wipf, P., Yamaguchi, Y., Fulton, W. B., Kovler, M., Niño, D. F., et al. (2021). The human milk oligosaccharides 2'-fucosyllactose and 6'-sialyllactose protect against the development of necrotizing enterocolitis by inhibiting toll-like receptor 4 signaling. Pediatr. Res. 89, 91–101. doi: 10.1038/s41390-020-0852-3
Soyyılmaz, B., Mikš, M. H., Röhrig, C. H., Matwiejuk, M., Meszaros-Matwiejuk, A., Vigsnæs, L. K. (2021). The mean of milk: A review of human milk oligosaccharide concentrations throughout lactation. Nutrients. 13 (8), 2737. doi: 10.3390/nu13082737
Spina, E., Cowin, P. (2021). Embryonic mammary gland development. Semin. Cell Dev. Biol. 114, 83–92. doi: 10.1016/j.semcdb.2020.12.012
Strandwitz, P. (2018). Neurotransmitter modulation by the gut microbiota. Brain Res. 1693, 128–133. doi: 10.1016/j.brainres.2018.03.015
Suez, J., Zmora, N., Segal, E., Elinav, E. (2019). The pros, cons, and many unknowns of probiotics. Nat. Med. 25, 716–729. doi: 10.1038/s41591-019-0439-x
Šuligoj, T., Vigsnæs, L. K., Abbeele, P. V. D., Apostolou, A., Karalis, K., Savva, G. M., et al. (2020). Effects of human milk oligosaccharides on the adult gut microbiota and barrier function. Nutrients 12 (9), 2808. doi: 10.3390/nu12092808
Tarr, A. J., Galley, J. D., Fisher, S. E., Chichlowski, M., Berg, B. M., Bailey, M. T. (2015). The prebiotics 3'Sialyllactose and 6'Sialyllactose diminish stressor-induced anxiety-like behavior and colonic microbiota alterations: Evidence for effects on the gut-brain axis. Brain Behav. Immun. 50, 166–177. doi: 10.1016/j.bbi.2015.06.025
Thomson, P., Medina, D. A., Garrido, D. (2018). Human milk oligosaccharides and infant gut bifidobacteria: Molecular strategies for their utilization. Food Microbiol. 75, 37–46. doi: 10.1016/j.fm.2017.09.001
Thongaram, T., Hoeflinger, J. L., Chow, J., Miller, M. J. (2017). Human milk oligosaccharide consumption by probiotic and human-associated bifidobacteria and lactobacilli. J. Dairy Sci. 100, 7825–7833. doi: 10.3168/jds.2017-12753
Thum, C., Wall, C. R., Weiss, G. A., Wang, W., Szeto, I. M., Day, L. (2021). Changes in HMO concentrations throughout lactation: influencing factors, health effects and opportunities. Nutrients. 13 (7), 2272. doi: 10.3390/nu13072272
Thurl, S., Henker, J., Siegel, M., Tovar, K., Sawatzki, G. (1997). Detection of four human milk groups with respect to Lewis blood group dependent oligosaccharides. Glycoconj J. 14, 795–799. doi: 10.1023/A:1018529703106
Thurl, S., Munzert, M., Boehm, G., Matthews, C., Stahl, B. (2017). Systematic review of the concentrations of oligosaccharides in human milk. Nutr. Rev. 75, 920–933. doi: 10.1093/nutrit/nux044
Triantis, V., Bode, L., van Neerven, R. J. J. (2018). Immunological effects of human milk oligosaccharides. Front. Pediatr. 6, 190. doi: 10.3389/fped.2018.00190
Trompette, A., Gollwitzer, E. S., Yadava, K., Sichelstiel, A. K., Sprenger, N., Ngom-Bru, C., et al. (2014). Gut microbiota metabolism of dietary fiber influences allergic airway disease and hematopoiesis. Nat. Med. 20, 159–166. doi: 10.1038/nm.3444
Turck, D., Bohn, T., Castenmiller, J., De Henauw, S., Hirsch-Ernst, K. I., Maciuk, A., et al. (2022). Safety of lacto-N-tetraose (LNT) produced by derivative strains of Escherichia coli BL21 (DE3) as a Novel Food pursuant to Regulation (EU) 2015/2283. Efsa J. 20, e07242. doi: 10.2903/j.efsa.2022.7242
Turck, D., Bohn, T., Castenmiller, J., De Henauw, S., Hirsch-Ernst, K. I., Maciuk, A., et al. (2023). Safety of 2'-fucosyllactose (2'-FL) produced by a derivative strain (Escherichia coli SGR5) of E. coli W (ATCC 9637) as a Novel Food pursuant to Regulation (EU) 2015/2283. Efsa J. 21, e08333. doi: 10.2903/j.efsa.2023.8333
Underwood, M. A., Gaerlan, S., De Leoz, M. L., Dimapasoc, L., Kalanetra, K. M., Lemay, D. G., et al. (2015). Human milk oligosaccharides in premature infants: absorption, excretion, and influence on the intestinal microbiota. Pediatr. Res. 78, 670–677. doi: 10.1038/pr.2015.162
Underwood, M. A., Mukhopadhyay, S., Lakshminrusimha, S., Bevins, C. L. (2020). Neonatal intestinal dysbiosis. J. Perinatol. 40, 1597–1608. doi: 10.1038/s41372-020-00829-2
Vandenplas, Y., Veereman-Wauters, G., De Greef, E., Peeters, S., Casteels, A., Mahler, T., et al. (2011). Probiotics and prebiotics in prevention and treatment of diseases in infants and children. J. Pediatr. (Rio J). 87, 292–300. doi: 10.2223/JPED.2103
Van Niekerk, E., Autran, C. A., Nel, D. G., Kirsten, G. F., Blaauw, R., Bode, L. (2014). Human milk oligosaccharides differ between HIV-infected and HIV-uninfected mothers and are related to necrotizing enterocolitis incidence in their preterm very-low-birth-weight infants. J. Nutr. 144, 1227–1233. doi: 10.3945/jn.113.187799
Vatanen, T., Jabbar, K. S., Ruohtula, T., Honkanen, J., Avila-Pacheco, J., Siljander, H., et al. (2022). Mobile genetic elements from the maternal microbiome shape infant gut microbial assembly and metabolism. Cell. 185, 4921–36 e15. doi: 10.1016/j.cell.2022.11.023
Vélez-Ixta, J. M., Juárez-Castelán, C. J., Ramírez-Sánchez, D., Lázaro-Pérez, N. D. S., Castro-Arellano, J. J., Romero-Maldonado, S., et al. (2024). Post natal microbial and metabolite transmission: the path from mother to infant. Nutrients 16 (3), 1990. doi: 10.3390/nu16131990
Verkhnyatskaya, S., Ferrari, M., de Vos, P., Walvoort, M. T. C. (2019). Shaping the infant microbiome with non-digestible carbohydrates. Front. Microbiol. 10, 343. doi: 10.3389/fmicb.2019.00343
Vigsnaes, L. K., Ghyselinck, J., Van den Abbeele, P., McConnell, B., Moens, F., Marzorati, M., et al. (2021). 2'FL and LNnT Exert Antipathogenic Effects against C. difficile ATCC 9689 In Vitro, Coinciding with Increased Levels of Bifidobacteriaceae and/or Secondary Bile Acids. Pathogens 10 (8), 927. doi: 10.3390/pathogens10080927
Wan, Y., Jiang, J., Lu, M., Tong, W., Zhou, R., Li, J., et al. (2020). Human milk microbiota development during lactation and its relation to maternal geographic location and gestational hypertensive status. Gut Microbes 11, 1438–1449. doi: 10.1080/19490976.2020.1760711
Wang, J., Hu, J. Q., Song, Y. J., Yin, J., Wang, Y. Y., Peng, B., et al. (2022). 2'-fucosyllactose ameliorates oxidative stress damage in d-galactose-induced aging mice by regulating gut microbiota and AMPK/SIRT1/FOXO1 pathway. Foods 11 (2), 151. doi: 10.3390/foods11020151
Wang, X., Li, L., Liu, T., Shi, Y. (2024). More than nutrition: Therapeutic potential and mechanism of human milk oligosaccharides against necrotizing enterocolitis. Life Sci. 339, 122420. doi: 10.1016/j.lfs.2024.122420
Wang, S., Wei, Y., Liu, L., Li, Z. (2021). Association between breastmilk microbiota and food allergy in infants. Front. Cell Infect. Microbiol. 11, 770913. doi: 10.3389/fcimb.2021.770913
Wang, C., Zhang, M., Guo, H., Yan, J., Chen, L., Teng, W., et al. (2020). Human milk oligosaccharides activate epidermal growth factor receptor and protect against hypoxia-induced injuries in the mouse intestinal epithelium and caco2 cells. J. Nutr. 150, 756–762. doi: 10.1093/jn/nxz297
Wang, C., Zhang, M., Guo, H., Yan, J., Liu, F., Chen, J., et al. (2019). Human Milk Oligosaccharides Protect against Necrotizing Enterocolitis by Inhibiting Intestinal Damage via Increasing the Proliferation of Crypt Cells. Mol. Nutr. Food Res. 63, e1900262. doi: 10.1002/mnfr.201900262
Wang, M., Zhao, Z., Zhao, A., Zhang, J., Wu, W., Ren, Z., et al. (2020). Neutral Human Milk Oligosaccharides Are Associated with Multiple Fixed and Modifiable Maternal and Infant Characteristics. Nutrients 12. doi: 10.3390/nu12030826
Wang, Y., Zou, Y., Wang, J., Ma, H., Zhang, B., Wang, S. (2020). The protective effects of 2'-fucosyllactose against E. Coli O157 infection are mediated by the regulation of gut microbiota and the inhibition of pathogen adhesion. Nutrients. 12 (3), 826.
Ward, R. E., Niñonuevo, M., Mills, D. A., Lebrilla, C. B., German, J. B. (2007). In vitro fermentability of human milk oligosaccharides by several strains of bifidobacteria. Mol. Nutr. Food Res. 51, 1398–1405. doi: 10.1002/mnfr.200700150
Weichert, S., Jennewein, S., Hüfner, E., Weiss, C., Borkowski, J., Putze, J., et al. (2013). Bioengineered 2'-fucosyllactose and 3-fucosyllactose inhibit the adhesion of Pseudomonas aeruginosa and enteric pathogens to human intestinal and respiratory cell lines. Nutr. Res. 33, 831–838. doi: 10.1016/j.nutres.2013.07.009
Wiciński, M., Sawicka, E., Gębalski, J., Kubiak, K., Malinowski, B. (2020). Human milk oligosaccharides: health benefits, potential applications in infant formulas, and pharmacology. Nutrients. 12 (1), 266. doi: 10.3390/nu12010266
Williams, J. E., Price, W. J., Shafii, B., Yahvah, K. M., Bode, L., McGuire, M. A., et al. (2017). Relationships among microbial communities, maternal cells, oligosaccharides, and macronutrients in human milk. J. Hum. Lact. 33, 540–551. doi: 10.1177/0890334417709433
Wong, C. B., Huang, H., Ning, Y., Xiao, J. (2024). Probiotics in the New Era of Human Milk Oligosaccharides (HMOs): HMO Utilization and Beneficial Effects of Bifidobacterium longum subsp. infantis M-63 Infant Health Microorganisms. 12 (5), 104. doi: 10.3390/microorganisms12051014
Wu, R. Y., Botts, S. R., Johnson-Henry, K. C., Landberg, E., Abrahamsson, T. R., Sherman, P. M. (2022). Variations in the composition of human milk oligosaccharides correlates with effects on both the intestinal epithelial barrier and host inflammation: A pilot study. Nutrients. 14 (5), 1014. doi: 10.3390/nu14051014
Wu, R. Y., Li, B., Koike, Y., Määttänen, P., Miyake, H., Cadete, M., et al. (2019). Human milk oligosaccharides increase mucin expression in experimental necrotizing enterocolitis. Mol. Nutr. Food Res. 63, e1800658. doi: 10.1002/mnfr.201800658
Xerri, N. L., Payne, S. M. (2022). Bacteroides thetaiotaomicron Outer Membrane Vesicles Modulate Virulence of Shigella flexneri. mBio. 13, e0236022. doi: 10.1128/mbio.02360-22
Xiao, L., Van't Land, B., Engen, P. A., Naqib, A., Green, S. J., Nato, A., et al. (2018). Human milk oligosaccharides protect against the development of autoimmune diabetes in NOD-mice. Sci. Rep. 8, 3829. doi: 10.1038/s41598-018-22052-y
Xu, G., Davis, J. C., Goonatilleke, E., Smilowitz, J. T., German, J. B., Lebrilla, C. B. (2017). Absolute quantitation of human milk oligosaccharides reveals phenotypic variations during lactation. J. Nutr. 147, 117–124. doi: 10.3945/jn.116.238279
Xu, S., Lane, J. A., Chen, J., Zheng, Y., Wang, H., Fu, X., et al. (2022). In vitro infant fecal fermentation characteristics of human milk oligosaccharides were controlled by initial microbiota composition more than chemical structure. Mol. Nutr. Food Res. 66, e2200098. doi: 10.1002/mnfr.202200098
Yamada, C., Gotoh, A., Sakanaka, M., Hattie, M., Stubbs, K. A., Katayama-Ikegami, A., et al. (2017). Molecular Insight into Evolution of Symbiosis between Breast-Fed Infants and a Member of the Human Gut Microbiome Bifidobacterium longum. Cell Chem. Biol. 24, 515–24.e5. doi: 10.1016/j.chembiol.2017.03.012
Yao, D., Dai, W., Dong, M., Dai, C., Wu, S. (2021). MUC2 and related bacterial factors: Therapeutic targets for ulcerative colitis. EBioMedicine. 74, 103751. doi: 10.1016/j.ebiom.2021.103751
Yao, Q., Gao, Y., Fan, L., Wang, J., Zheng, N. (2022). 2'-fucosyllactose remits colitis-induced liver oxygen stress through the gut-liver-metabolites axis. Nutrients. 14 (9), 4186. doi: 10.3390/nu14194186
Yao, Q., Gao, Y., Zheng, N., Delcenserie, V., Wang, J. (2024). Unlocking the mysteries of milk oligosaccharides: Structure, metabolism, and function. Carbohydr Polym. 332, 121911. doi: 10.1016/j.carbpol.2024.121911
Yao, Q., Li, H., Gao, Y., Zheng, N., Delcenserie, V., Wang, J. (2023). The milk active ingredient, 2'-fucosyllactose, inhibits inflammation and promotes MUC2 secretion in LS174T goblet cells in vitro. Foods 12 (1), 186. doi: 10.3390/foods12010186
Yu, Z. T., Nanthakumar, N. N., Newburg, D. S. (2016). The human milk oligosaccharide 2'-fucosyllactose quenches campylobacter jejuni-induced inflammation in human epithelial cells HEp-2 and HT-29 and in mouse intestinal mucosa. J. Nutr. 146, 1980–1990. doi: 10.3945/jn.116.230706
Yue, H., Han, Y., Yin, B., Cheng, C., Liu, L. (2020). Comparison of the antipathogenic effect toward Staphylococcus aureus of N-linked and free oligosaccharides derived from human, bovine, and goat milk. J. Food Sci. 85, 2329–2339. doi: 10.1111/1750-3841.15150
Zhang, W., He-Yang, J., Tu, W., Zhou, X. (2021). Sialylated human milk oligosaccharides prevent intestinal inflammation by inhibiting toll like receptor 4/NLRP3 inflammasome pathway in necrotizing enterocolitis rats. Nutr. Metab. (Lond). 18, 5. doi: 10.1186/s12986-020-00534-z
Zhang, H., Zhang, Z., Liao, Y., Zhang, W., Tang, D. (2022). The complex link and disease between the gut microbiome and the immune system in infants. Front. Cell Infect. Microbiol. 12, 924119. doi: 10.3389/fcimb.2022.924119
Zhong, Z., Tang, H., Shen, T., Ma, X., Zhao, F., Kwok, L. Y., et al. (2022). Bifidobacterium animalis subsp. lactis Probio-M8 undergoes host adaptive evolution by glcU mutation and translocates to the infant's gut via oral-/entero-mammary routes through lactation. Microbiome. 10, 197. doi: 10.1186/s40168-022-01398-6
Zhou, Y., Sun, H., Li, K., Zheng, C., Ju, M., Lyu, Y., et al. (2021). Dynamic Changes in Human Milk Oligosaccharides in Chinese Population: A Systematic Review and Meta-Analysis. Nutrients 13 (9), 2912. doi: 10.3390/nu13092912
Zhou, B., Yuan, Y., Zhang, S., Guo, C., Li, X., Li, G., et al. (2020). Intestinal flora and disease mutually shape the regional immune system in the intestinal tract. Front. Immunol. 11, 575. doi: 10.3389/fimmu.2020.00575
Zmora, N., Suez, J., Elinav, E. (2019). You are what you eat: diet, health and the gut microbiota. Nat. Rev. Gastroenterol. Hepatol. 16, 35–56. doi: 10.1038/s41575-018-0061-2
Zunga, M., Yebra, M. J., Monedero, V. (2021). Complex oligosaccharide utilization pathways in lactobacillus. Curr. Issues Mol. Biol. 40, 49–80. doi: 10.21775/cimb.040.049
Keywords: human milk oligosaccharides, intestinal microbiota, breast milk, infants, nutrition
Citation: Sun W, Tao L, Qian C, Xue PP, Du SS and Tao N (2025) Human milk oligosaccharides: bridging the gap in intestinal microbiota between mothers and infants. Front. Cell. Infect. Microbiol. 14:1386421. doi: 10.3389/fcimb.2024.1386421
Received: 15 February 2024; Accepted: 04 December 2024;
Published: 06 January 2025.
Edited by:
Benoit Chassaing, Institut National de la Santé et de la Recherche Médicale (INSERM), FranceReviewed by:
Francois-Pierre Martin, H&H Group, SwitzerlandCecilia Ximenez, National Autonomous University of Mexico, Mexico
Copyright © 2025 Sun, Tao, Qian, Xue, Du and Tao. This is an open-access article distributed under the terms of the Creative Commons Attribution License (CC BY). The use, distribution or reproduction in other forums is permitted, provided the original author(s) and the copyright owner(s) are credited and that the original publication in this journal is cited, in accordance with accepted academic practice. No use, distribution or reproduction is permitted which does not comply with these terms.
*Correspondence: Ying-na Tao, VGVyYWluYVRhb0AxMjYuY29t