- 1Department of Bioresources, School of Biological Sciences, University of Kashmir, Srinagar, India
- 2Department of Family and Community Medicine, Faculty of Medicine, Al Baha University, Al Bahah, Saudi Arabia
The symbiotic relationship between the human digestive system and its intricate microbiota is a captivating field of study that continues to unfold. Comprising predominantly anaerobic bacteria, this complex microbial ecosystem, teeming with trillions of organisms, plays a crucial role in various physiological processes. Beyond its primary function in breaking down indigestible dietary components, this microbial community significantly influences immune system modulation, central nervous system function, and disease prevention. Despite the strides made in microbiome research, the precise mechanisms underlying how bacterial effector functions impact mammalian and microbiome physiology remain elusive. Unlike the traditional DNA-RNA-protein paradigm, bacteria often communicate through small molecules, underscoring the imperative to identify compounds produced by human-associated bacteria. The gut microbiome emerges as a linchpin in the transformation of natural products, generating metabolites with distinct physiological functions. Unraveling these microbial transformations holds the key to understanding the pharmacological activities and metabolic mechanisms of natural products. Notably, the potential to leverage gut microorganisms for large-scale synthesis of bioactive compounds remains an underexplored frontier with promising implications. This review serves as a synthesis of current knowledge, shedding light on the dynamic interplay between natural products, bacteria, and human health. In doing so, it contributes to our evolving comprehension of microbiome dynamics, opening avenues for innovative applications in medicine and therapeutics. As we delve deeper into this intricate web of interactions, the prospect of harnessing the power of the gut microbiome for transformative medical interventions becomes increasingly tantalizing.
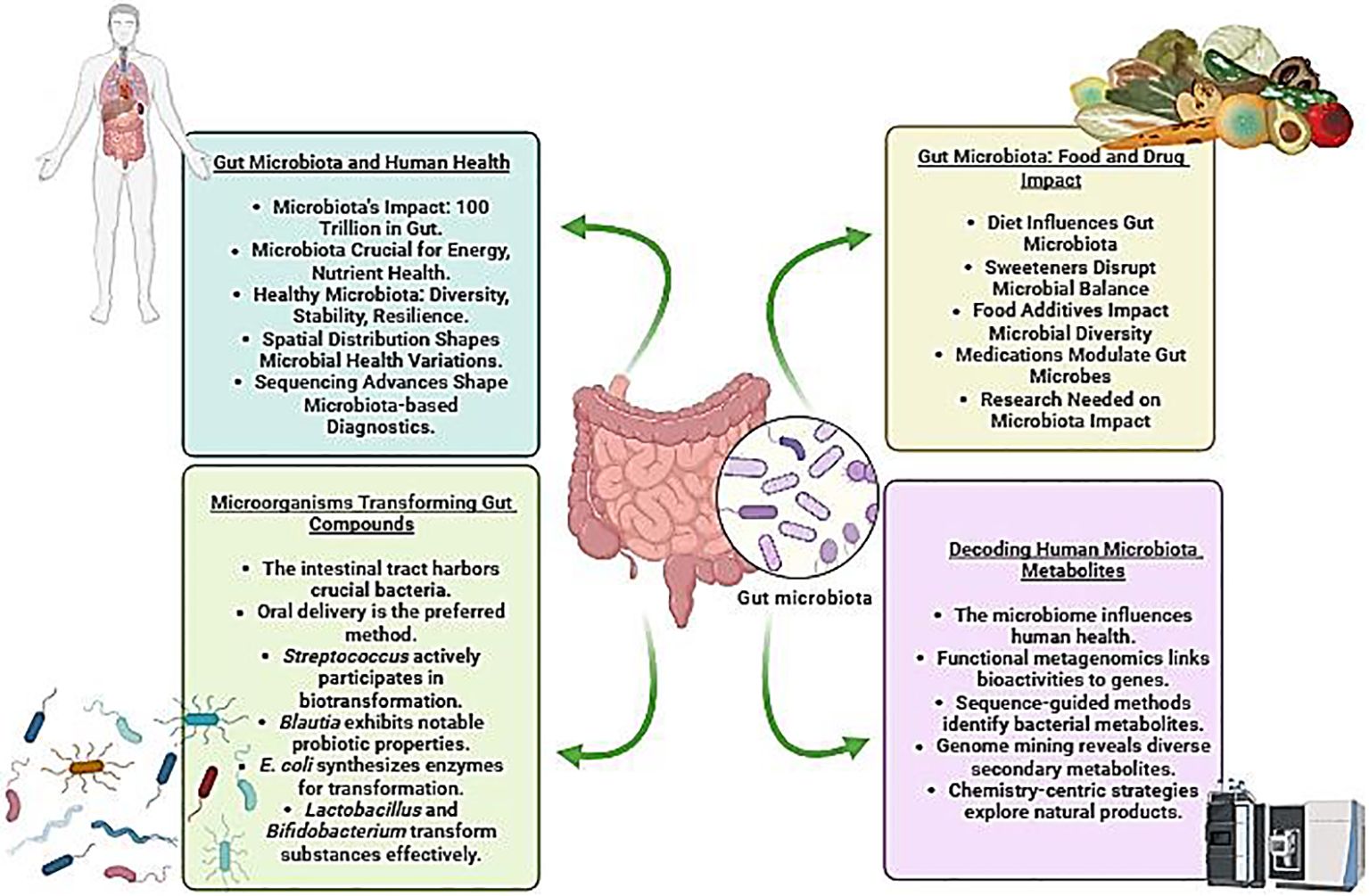
Graphical Abstract Depiction of the intricate interplay between gut microbiota and human health, emphasizing the influence of food and drugs on the gut microbiome, microbial transformation of gut compounds, and the exploration of consequential human microbial metabolites (Created with Biorender).
1 Introduction
The human microbiome encompasses the collective genetic material of microorganisms residing within us, including protozoa, archaea, eukaryotes, viruses, and primarily bacteria, which coexist symbiotically on and inside various regions of the human body. These inhabited sites include the mouth, reproductive organs, airways, skin, and digestive system (Lloyd-Price et al., 2016). Interestingly, the human digestive system harbors a thriving ecosystem of microbes collectively referred to as the microbiota, comprising an astonishing ∼1013-1014entities. This intricate assembly, primarily composed of anaerobic bacteria, encompasses 500–1,000 distinct species with a genetic repertoire estimated to surpass the human genome by a factor of 150 (Kho and Lal, 2018; Marsh et al., 2023; Walker and Hoyles, 2023). Functioning as a metabolic entity finely attuned to human physiology, the microbiota operates as an organ, orchestrating processes that necessitate no evolutionary adaptations on our part. Among its pivotal functions is the processing of dietary components that would otherwise be indigestible like plant polysaccharides (Bäckhed et al., 2004). The human microbiome has emerged as a linchpin in complex physiological processes, influencing a spectrum ranging from immune system modulation to the central nervous system and brain development (Kau et al., 2011; Smith, 2015). Insights derived from mouse models underscore the indispensable part of a healthy bacterial environment in maintaining typical physiological processes, in contrast to imbalances, or dysbiosis, linked to an array of diseases including cancer, diabetes, obesity, and colitis (Figure 1) (Garrett et al., 2007; Ridaura et al., 2013; Smith et al., 2013; Bongers et al., 2014). The burgeoning field of microbiome research is further underscored by a spike in clinical trials and venture capital investments, with over 1,200 clinical trials related to the “gut microbiome” registered in the National Institutes of Health clinical trials database (Gormley, 2016; Marchesi et al., 2016).
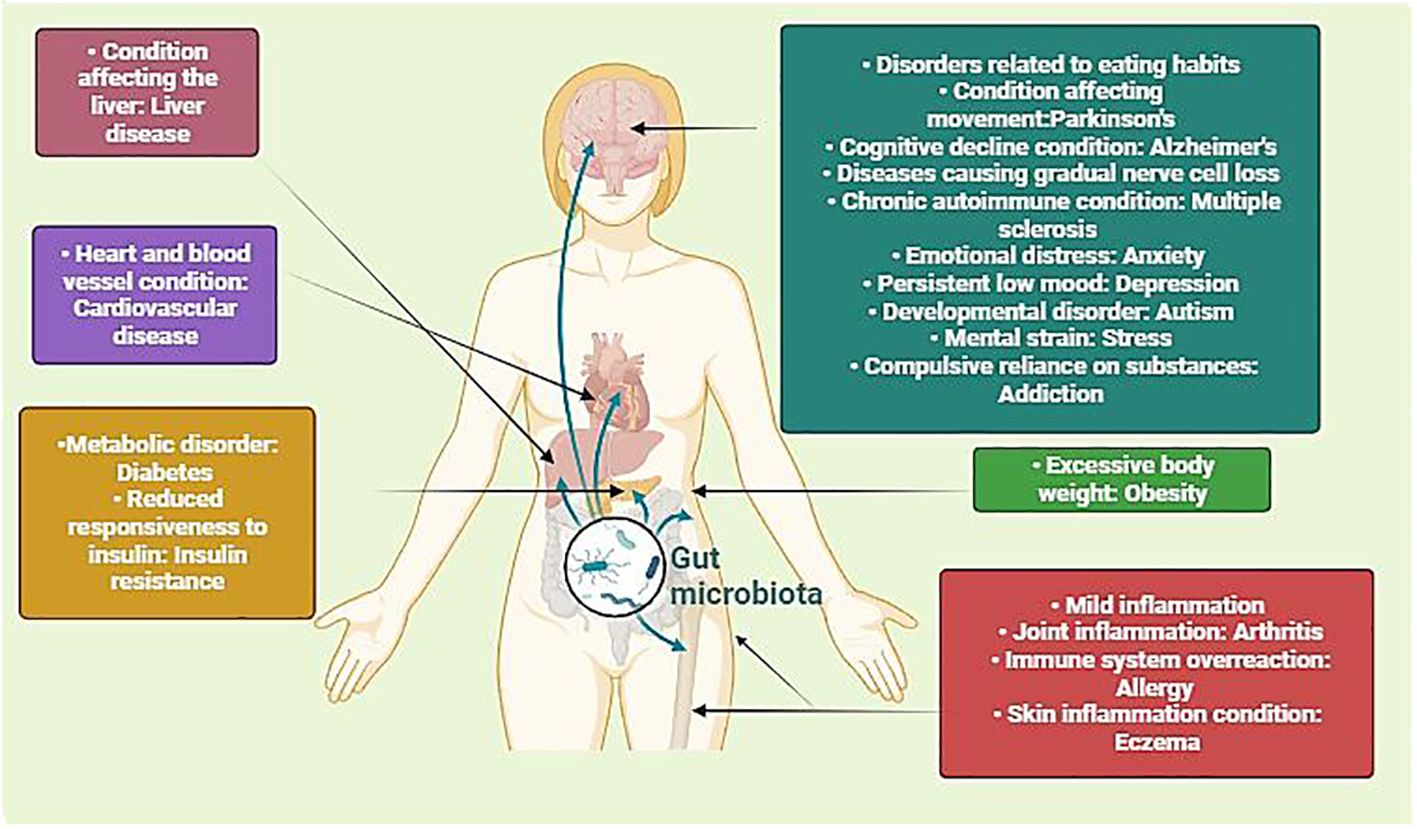
Figure 1 Illustration depicting the intricate interplay between microbiota and human physiology, impacting different health conditions. By portraying how alterations in the gut microbiota can impact diverse physiological processes, including metabolism, immune function, and neurobiology, it underscores the significance of this interplay in both health and disease. Understanding these interactions is crucial for developing targeted interventions to modulate the microbiota and promote optimal health outcomes across diverse populations. (Created with Biorender).
There is still a significant knowledge vacuum regarding the precise mechanisms by which bacterial functions, also known as effector functions, influence mammalian or microbiome physiology, despite growing evidence linking host-associated bacteria to both healthy development and disease in animal models as well as correlational findings in humans. The traditional framework of molecular biology, which follows the linear flow of genetic information from DNA to RNA to protein, may not fully capture the complexity of biological systems. In many cases, biological functions do not solely result in protein synthesis but also involve the generation and utilization of small molecules. This phenomenon is particularly evident in bacteria, where interactions with the environment heavily rely on low-molecular-weight compounds, including small molecules and natural products. While these small molecules are not directly encoded by DNA, they play crucial roles in mediating microbial functions and their interactions with the host organism. Therefore, to comprehensively understand the molecular mechanisms underlying the role of the human microbiome in both health and disease, it is essential to thoroughly identify and characterize the small compounds produced by human-associated bacteria (Milshteyn et al., 2018).
Gut microbes perform a pivotal function in decomposing and transforming natural products, generating a diverse array of metabolites and functional compounds having unique physiological functions that the host organism cannot synthesize on its own (Koppel et al., 2017; Xie et al., 2020). The process of microbial transformation in natural products encompasses various chemical reactions, including demethylation, hydrolysis, methylation, etc, which collectively regulate the form of the natural product substrates (Morgan et al., 2022; Rocchetti et al., 2022). Importantly, the impact of gut microbiota extends beyond mere structural modifications, significantly influencing the chemical landscape, pharmacological activities, and metabolic mechanisms of natural products. Surprisingly, the ability to harness gut microorganisms for the massive manufacture of active metabolites and compound synthesis remains largely unexplored. Delving into the study of these gut microorganisms, their metabolites, and the intricate reactions engaged in the dynamic interplay between gut microbiota and natural products holds immense importance. Unraveling these interactions is essential to both realizing the promise of natural products in several applications and comprehending the pharmacological processes at work (Zhao et al., 2022). This exploration opens avenues for further research into the utilization of gut microbes in the synthesis of bioactive compounds on a larger scale, shedding light on innovative approaches for the production and utilization of these compounds. Consequently, studying the multifaceted relationships between natural products and gut microbiota not only enhances our understanding of pharmacological mechanisms but also paves the way for harnessing the therapeutic potential of these interactions.
Our goal in this review study is to explore and synthesize the present state of information in this dynamic field, shedding light on the interactions between natural products, bacteria, and human health. Recent efforts have illuminated a fascinating facet of microbiome dynamics: the generation of natural products. This burgeoning area of research explores metabolites derived from the microbiome and seeks to unravel their part in human health (Wilson and Nicholson, 2017). In this review, we delve into these recent efforts, describing key approaches used to identify and characterize microbiome-derived natural products. This comprehensive exploration contributes to the evolving understanding of the intricate relationships between the microbiome, natural products, and human health, ultimately paving the way for innovative applications in medicine and therapeutics.
2 Microbiome
Microbial organisms commonly exist in communal arrangements and often form close associations with intricate beings like humans, forming mutualistic, parasitic, pathogenic, and other associations. The ensemble of these microorganisms is referred to as the microbiome or microbiota. While the term “microflora” has been used, it is considered a misnomer as “flora” traditionally represents the plant kingdom (Liang et al., 2018). Initially, the term “microbiome” denoted the group of microbes and the contents of their genomes, while “microbiota” described the microbial community within their hosts. However, the interchangeability of “microbiome” and “microbiota” has become prevalent (Ursell et al., 2012). The human body has a microbiome in every part of it, from the skin to the gut and even in places like the bloodstream that were thought to be sterile in the past (Proal et al., 2014). Numerous reports suggest the presence of over 10,000 microbial species occupying various human body parts (Blaser, 2006; Ley et al., 2006). Although skin and vaginal sites exhibit comparatively minimal diversity of microbes, greater diversity is observed in sites such as the gut (Jiang et al., 2009). There can be a significant relationship between human diseases and the microbiome and vice versa. For instance, long-term lung conditions may change the lung microbiome’s makeup, which may then impact host immunity and defense and worsen the conditions (O’Dwyer et al., 2016). Furthermore, research has revealed that the microbiome present in the blood or lungs is affected by the presence of infection (Jiang et al., 2009; Jostins et al., 2012; Kwan et al., 2013; Leung and Wu, 2015).
2.1 Gut microbiome
The gut microbiome, comprising the genetic material of microorganisms like fungi, protozoa, bacteria, etc, resides in the digestive tracts of humans and other animals (Table 1), including insects (Liang et al., 2018). Having evolved alongside its host for millennia, the human gut microbiome plays a crucial role in various essential activities, including nutrient absorption and digestion (Turnbaugh and Gordon, 2009; Hehemann et al., 2010), detox and body defense (Relman, 2012), host immune system development (Turnbaugh and Gordon, 2009), etc. The mammalian gut hosts a diverse array of microbes, with the majority belonging to the Firmicutes and Bacteroidetes (Ley et al., 2008). This pattern holds correct across different populations, such as Koreans (Nam et al., 2011), Africans (De Filippo et al., 2010; Schnorr et al., 2014), Europeans and Americans (Qin et al., 2010), and Danes though not in the case of Chinese (Wu G. et al., 2014). The diversity of microorganisms can carry distinct consequences for diseases in various populations. For instance, individuals with type 2 diabetes from European and Chinese backgrounds exhibit varying compositions of gut microbiomes, with the Chinese population showing greater species diversity (Qin et al., 2012). Nonetheless, understanding the significant differences among these populations, considering factors like age, environment, and genetics, requires further investigation (Tai et al., 2015).
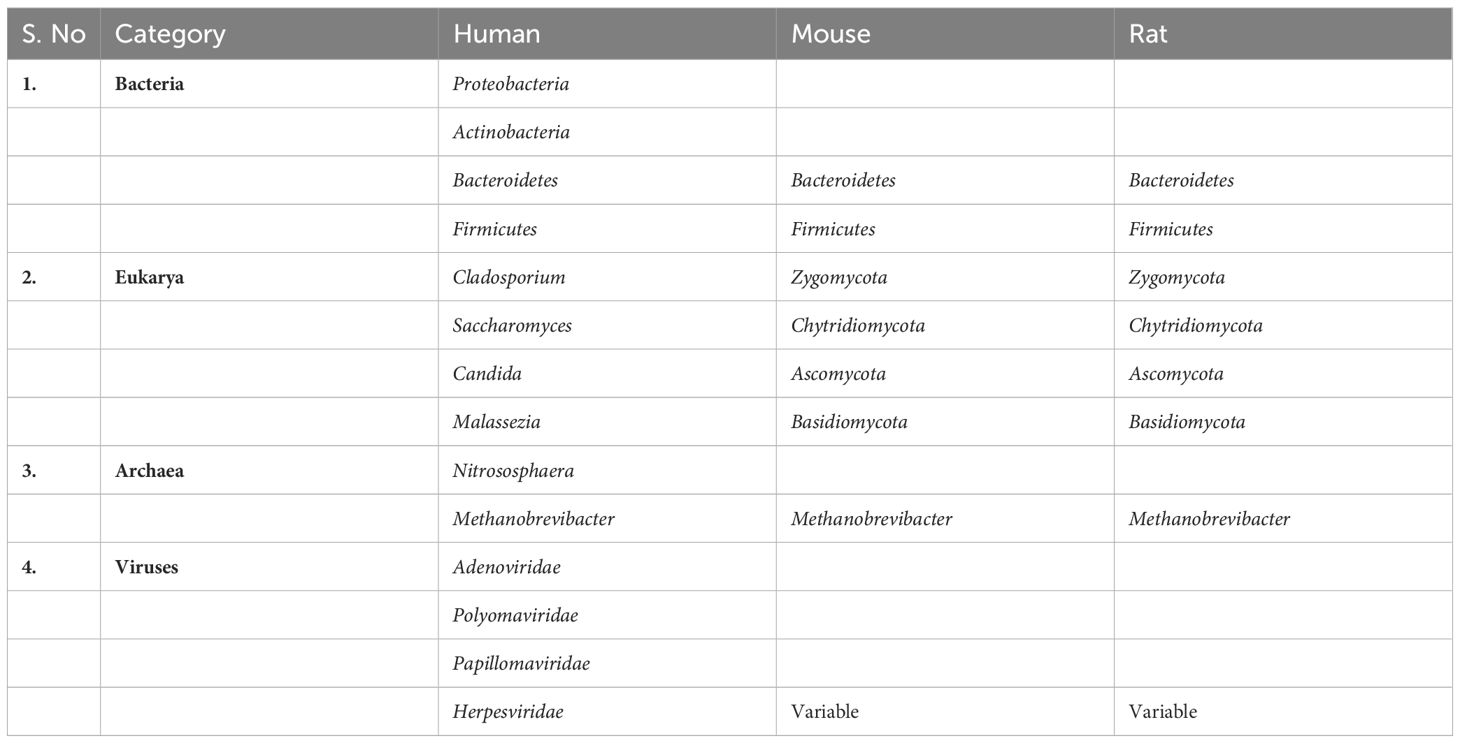
Table 1 Primary categories of gut microbiota in both humans and animal models (Hillman et al., 2017).
The gut microbiome harbors millions of diverse microorganisms, each contributing to its metabolic diversity and adaptability, with some genes potentially acquired from environmental bacteria (Hehemann et al., 2010; Qin et al., 2010). Notably, three primary enterotypes [Enterotypes are defined as clusters or patterns of microbial community composition in the gut microbiome that are driven by various factors such as diet, geography, host genetics, and lifestyle. Rather than discrete and stable classifications, enterotypes are now viewed as dynamic and context-dependent configurations of the gut microbiota. These configurations may shift over time in response to environmental changes, host health status, and other factors, reflecting the complexity and flexibility of the gut microbial ecosystem (Costea et al., 2018)]—Bacteroides, Prevotella, and Ruminococcus—have been identified in the human gut across diverse populations (Arumugam et al., 2011). These enterotypes, observed in Europeans, Japanese, and Americans, demonstrate a consistent presence in mice and chimpanzees (Moeller et al., 2012; Hildebrand et al., 2013; Wang et al., 2014). While the composition is mainly shaped by the host’s evolution, recent findings suggest that diet has a more significant impact on the metabolome than the microbiome. Discrepancies, especially regarding the prevalence of ecotypes like Ruminococcus, warrant further investigation considering factors such as sample size and variations in sampling methods (Arumugam et al., 2011; Wu et al., 2011; Huse et al., 2012; Zupancic et al., 2012; Gorvitovskaia et al., 2016; Liang et al., 2017). The gut microbiota comprises autochthonous microbes (Autochthonous refers to indigenous or native microbial species that are typically well-adapted to a specific environment, such as the gut) residing on the colonic mucosa epithelium and allochthonous microbes (Allochthonous refers to microbial species that are not native to a particular environment but are introduced from external sources) transiently passing through the lumen with digesta (Schnabl and Brenner, 2014). Distinguishing between these “residents” and “passengers” becomes crucial, as their roles are believed to differ significantly. The ratio of autochthonous to non-autochthonous microbes serves as a valuable indicator for assessing the progression of cirrhosis (Bajaj et al., 2014).
Diet and phylogeny are major contributors to the modification of the gut microbial community in various species, including mammals (Ley et al., 2008; Miyake et al., 2015). Genome-scale metabolic modeling reveals that changes in the host diet significantly alter the makeup of key human gut bacteria (eg. B. thetaiotaomicron) (Shoaie et al., 2013). Examples such as the impact of alcohol on intestinal microbiota emphasize the bidirectional relationship between diet and microbial composition, influencing host metabolism and related diseases (Mutlu et al., 2009; Chen et al., 2011; Yan et al., 2011; Mutlu et al., 2012; Queipo-Ortuño et al., 2012). Host genetics also play a crucial role in determining microbiome composition (Garrett et al., 2007; Maslowski et al., 2009; Benson et al., 2010; Brinkman et al., 2011; Lepage et al., 2011; Hashimoto et al., 2012; McKnite et al., 2012; Goodrich et al., 2014; Knights et al., 2014; Benson, 2015; Kubinak et al., 2015; Bonder et al., 2016a; Snijders et al., 2016; Igartua et al., 2017). The identification of shared susceptibility loci between inflammatory bowel disease and specific infectious organisms underscores the significance of exploring the interconnections among susceptibility, microbiome composition, and the development of the disease. This emphasizes the need to develop effective protocols for disease prevention by understanding these relationships (Kane et al., 2011; Henao-Mejia et al., 2012; Jostins et al., 2012; Knights et al., 2014). In the gut, Gram-negative bacteria produce lipopolysaccharide (LPS), a microbial product transported with chylomicrons (Balagopal et al., 2008; Jiang et al., 2009; Hofer et al., 2010; Marchetti et al., 2013). LPS acts as a potent stimulator of innate immunity, with its levels serving as important indicators for survival in peritoneal dialysis patients (Kwan et al., 2013). Similarly, trimethylamine (TMA) and its oxidation product, trimethylamine N-oxide (TMAO), impact patient morbidity, highlighting the far-reaching consequences of localized microbiome activity (Tang et al., 2017). These observations underscore the potential of using plasma levels of LPS and bacterial DNA as markers for both systemic inflammation and prognosis (Alexander and Rietschel, 2001).
3 Gut microbiota and human health
The GI tract of humans houses a vast community of microbes of approximately 100 trillion microorganisms, significantly impacting human health and disease (Figure 2). This intricate association between gut microbiota and fundamental biological mechanisms has been extensively studied, revealing its involvement in immune systems, metabolic processes, and nutrient absorption (Ley et al., 2006). The gut microbiota, comprising bacteria, yeasts, and viruses, plays a crucial role in energy and nutrient extraction through diverse metabolic genes, providing unique enzymes and biochemical pathways. Additionally, it contributes to the manufacture of essential substances like lipids, vitamins, and amino acids (Rowland et al., 2018). In terms of immunity, the human microbiota acts as a defense mechanism by producing antimicrobial substances and influencing intestinal mucosal growth as well as the immune system’s development. When the gut microbiota is in a healthy state, it interacts symbiotically with the host and is stable and resilient. Defining a “healthy” gut microbiota involves considering factors such as high taxonomic diversity, microbial gene richness, and a stable core microbiota (Fan and Pedersen, 2021). Nevertheless, individual variations and dynamic changes influenced by aging, and contextual elements, including the use of medications, are noted.
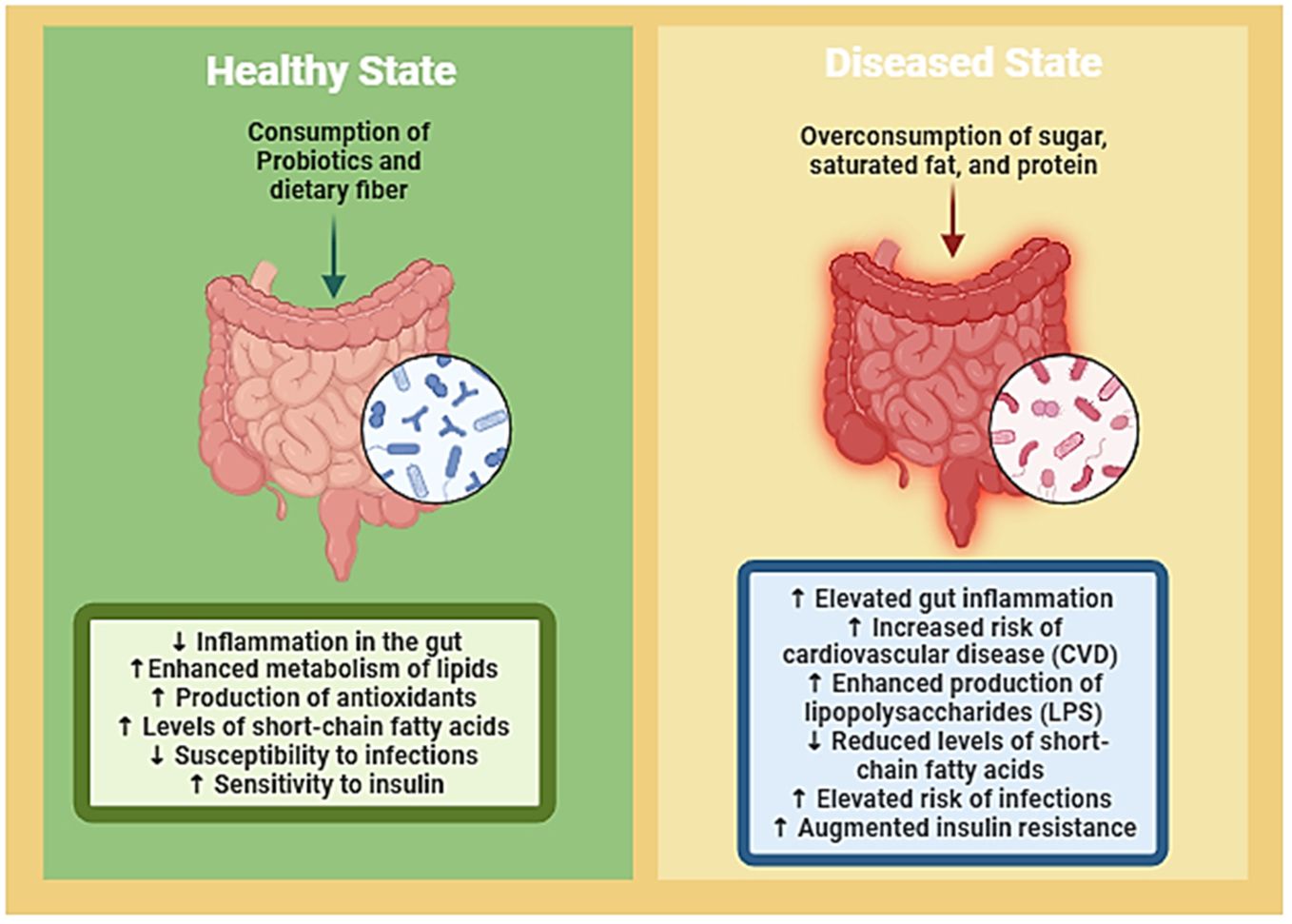
Figure 2 Visual representation delineates the intricate interplay between gut microbiota, nutrition, and the delicate balance between health and disease states. It illustrates how dietary factors can either promote a healthy gut microbiota composition, which contributes to overall well-being, or lead to dysbiosis, predisposing individuals to various diseases. Through highlighting this dynamic relationship, the figure underscores the potential of targeted dietary interventions to restore microbial balance and mitigate disease risk, thereby emphasizing the crucial role of nutrition in maintaining gut health and preventing the onset of illness. (Created with Biorender).
Spatial distribution within the GI tract also contributes to microbial variation, with distinct microbial communities present in the small intestine and colon due to differences in transit time, bile concentration, flow rates, and pH (Flint et al., 2012). Age-related variations indicate an increase in microbiota diversity from childhood to adulthood, followed by a decrease in older age, where shifts in microbial composition, including decreased Bifidobacterium and increased Clostridium and Proteobacteria, are observed (Rinninella et al., 2019). The impact of microbiota on human well-being extends beyond compositional studies. Recent advancements in high-throughput sequencing, microbiota interaction modeling, and simulation techniques have shifted focus toward understanding the causality of microbiota functions. This has significant implications for the advancement of microbiome-based diagnostics and customized medicine (Ley et al., 2006; Bouskra et al., 2008; Amabebe et al., 2020).
Therefore, the intricate interplay between gut microbiota and human health involves dynamic mechanisms influenced by age, spatial distribution within the GI tract, and environmental factors. As research progresses, a deeper understanding of microbiota’s functions is essential to strengthen the advancement of personalized medicine and microbiome-based diagnostics.
3.1 The impact of food and drugs on the composition of the gut microbiota
The quantity of gut bacteria is greatly influenced by particular foods and dietary habits, which in turn affects health. High-intensity sweeteners, widely utilized as sugar substitutes, raise concerns based on animal studies. Despite regulatory agencies deeming them “generally recognized as safe,” sucralose, aspartame, and saccharin disrupt gut microbiota balance and diversity. Rats exposed to sucralose for twelve weeks displayed elevated amounts of total aerobic bacteria, Bacteroides, and Clostridium, along with a significantly higher fecal pH (Abou-Donia et al., 2008). Mice exposed to sucralose for six months exhibited increased expression of pro-inflammatory genes and altered fecal metabolites (Bian et al., 2017). Common food additives that impact gut flora include emulsifiers, which are included in processed meals. Mice given carboxymethylcellulose and polysorbate-80 displayed decreased microbial diversity, decreased Bacteroidales and Verrucomicrobia, and enrichment of inflammation-promoting Proteobacteria (Chassaing et al., 2015). Concerns extend to certain restrictive diets. Studies on vegan diets revealed variations in the gut microbe-produced serum metabolites, but only slight alterations in the bacterial communities (Wu G. et al., 2014). Gluten-free diets, recommended for gluten sensitivity or celiac disease, altered gut microbiota profiles in healthy individuals, potentially affecting useful microbial species (Bonder et al., 2016b). The low FODMAP diet, beneficial for irritable bowel syndrome, led to significant microbiota and metabolome changes (McIntosh et al., 2016; Gibson, 2017; Halmos, 2017; Bennet et al., 2018). Medications also modulate the composition of gut flora. An important research identified drugs such as progesterone, rupatadine, TNF-α inhibitors, and osmotic laxatives, as major modulators (Falony et al., 2016). Proton pump inhibitors and antibiotics, extensively used in humans and livestock, influence gut microbes, potentially contributing to obesity (Jackson et al., 2015; Blaser, 2016).
While clinical evidence is insufficient for clear recommendations, future studies on food additives, drugs, and dietary modifications should consider their effects on the gut microbiota. In medical contexts like cancer treatment and autoimmune disorders (Figure 3), where microbiota changes impact responses, understanding these dynamics becomes crucial (Alexander et al., 2017). Animal studies also highlight the role of specific gut microbes in transforming compounds for protective effects, emphasizing the interconnectedness of diet, gut health, and overall well-being (Spanogiannopoulos et al., 2016).
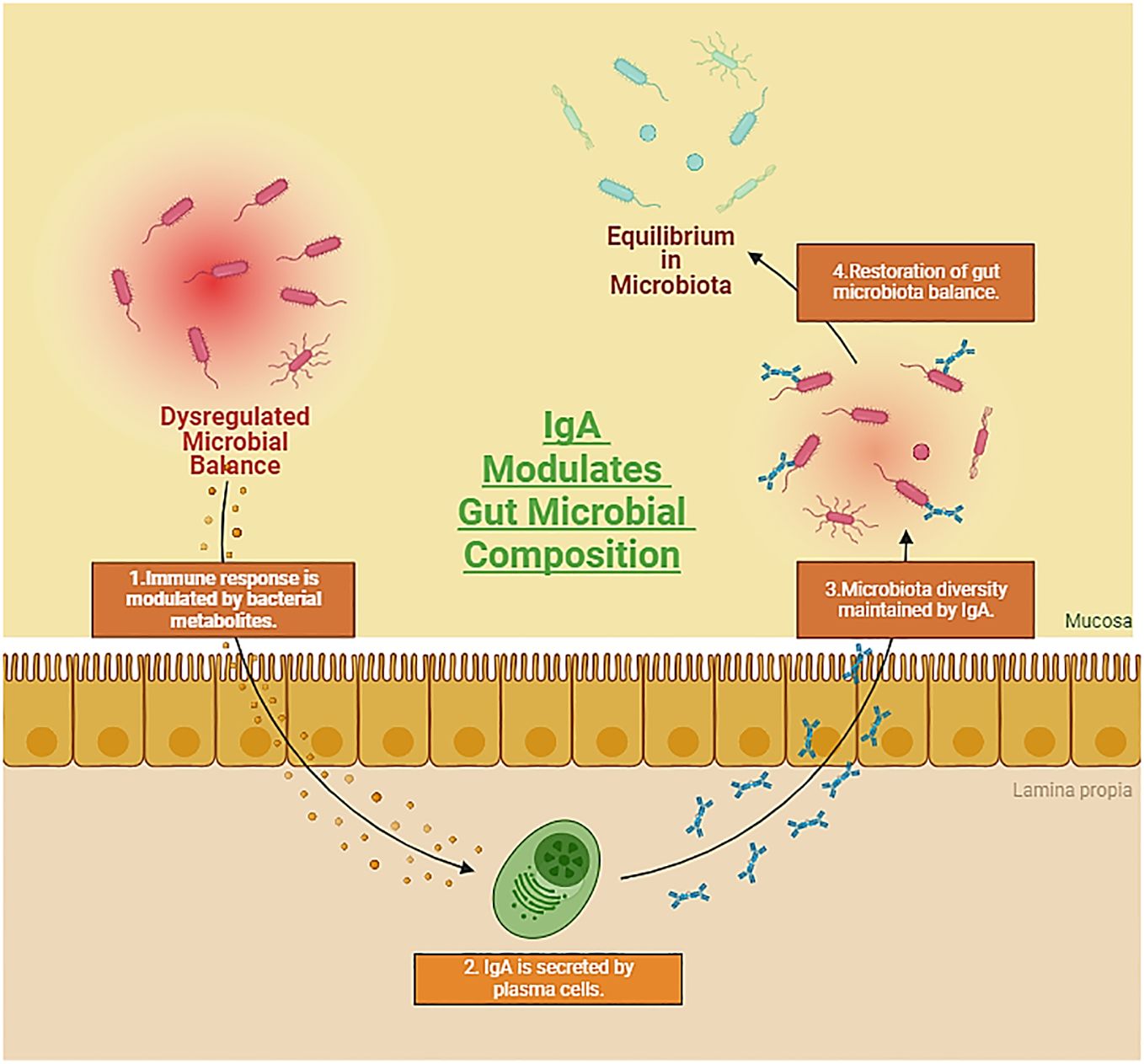
Figure 3 Representation showcasing the symbiotic relationship between the gut microbiota and autoimmune diseases, while emphasizing the crucial role of Immunoglobulin A (IgA) in preserving colonic homeostasis. The dynamic interplay between host immune responses and the gut microbiome, coupled with IgA-mediated regulation, underscores the intricate mechanisms influencing autoimmune processes and maintaining gastrointestinal equilibrium. (Created with Biorender).
4 Crucial gut microorganisms involved in the transformation of natural compounds
Oral administration is the preferred mode of drug delivery, as evidenced by the 84% oral composition of the top 50 best-selling pharmaceuticals in the US and Europe (Vinarov et al., 2021). The impact of gut microbiota on the durability of orally administered natural products has garnered significant interest in recent years. The intestinal tract harbors a plethora of bacteria crucial for normal digestive function, with approximately 98% of gut microorganisms in healthy individuals falling into 4 phyla: Proteobacteria, Actinobacteria, Firmicutes, and Bacteroidetes (Manor et al., 2020; Ye et al., 2021; de Vos et al., 2022). Specific gut microorganisms, like Escherichia coli, Streptococcus, etc. actively take part in the biotransformation of natural products. The resulting metabolites contribute to enhanced intestinal absorption, playing a significant pharmacological role (Zhao et al., 2022).
4.1 Streptococcus
The Streptococcus species, characterized by gram-positive, circular, or ovoid cells organized in chains or pairs, is commonly found in the nasopharynx and human stool (Lannes-Costa et al., 2021). Meta-transcriptomic study reveals that Streptococcus predominantly expresses the phosphotransferase system, suggesting their primary role in utilizing the carbohydrates that are present in the small intestine (Zoetendal et al., 2012). Streptococcus LJ-22 expresses β-glucuronidase activity, converting GL to 18β-glycyrrhetinic acid-3-O-β-D-glucuronic acid (GAMG), known for its anti-allergic properties. This compound has demonstrated anti-allergic potential against LPS-induced RAW264.7 cells (Guo et al., 2018). Furthermore, tannic acid degradation by Streptococcus gallolyticus subsp. Gallolyticus may contribute to colorectal cancer development by neutralizing its toxicity in the case of tumor cells (Oehmcke-Hecht et al., 2020). Streptococcus thermophilus GIM 1.321 demonstrates high β-glucosidase production, facilitating the breakdown of fructus anthocyanins into beneficial compounds like ferulic acid, CAA (caffeic acid), and CHA (chlorogenic acid) (Cheng et al., 2016). Administering CAA and CHA from these processes has shown the potential to lower blood pressure and provide antioxidant effects (Agunloye et al., 2019). Streptococcus strains, acting as commensals, pathogens, or opportunistic pathogens in the gut, require further exploration regarding their impact on the well-being of humans. Comprehending the way Streptococcus metabolizes natural compounds may help regulate the gut microbiota and improve the effectiveness of treatments. In-depth in vivo research is crucial to find out if focusing on bacteria that break down tannic acid could help develop more potent treatments for colorectal cancer. Overall, unraveling the intricacies of Streptococcus metabolism holds promise for advancing our comprehension of the gut microbiota and optimizing treatment interventions (Zhao et al., 2022).
4.2 Blautia
Blautia species, prevalent in the anaerobic environments of mammalian guts and feces, exhibit notable probiotic properties in the biotransformation of natural products. Tremaroli and Bäckhed (2012) (Tremaroli and Bäckhed, 2012) highlight Blautia’s involvement in flavonoid processing, encompassing demethylation, O- and C-deglycosylation, and C-ring cleavage catalyzed by enzymes like O-glycosidase and β-glucosidases (Braune et al., 2016). Notably, Blautia sp. MRG-PMF1 demonstrates hydrolytic prowess, conver ting compounds like 5,7-dimethoxyflavone into chrysin and 5,7,4-trimethoxyflavone into apigenin. This strain also exhibits deglycosylation activity on various isoflavones, flavones, and flavonols, transforming them into their corresponding aglycones (Kim et al., 2014). In anaerobic environments, Blautia sp. MRG-PMF1 catalyzes curcumin to generate demethoxycurcumin, which has anti-inflammatory and anti-cancer characteristics and further metabolizes icariin into desmethylicaritin, which has estrogenic properties (Wu H. et al., 2016; Burapan et al., 2017; Hatamipour et al., 2019). Another strain, Blautia sp. AUH-JLD56, exclusively biotransforms arctiin or arctigenin into demethylated products with enhanced antioxidant potential (Liu et al., 2013). The creation of novel enzymes and bioactive metabolites is greatly encouraged by the increasing scholarly interest in Blautia’s involvement in the biotransformation and metabolism of herbal plants and functional foods (Meng et al., 2020).
4.3 E. coli
Escherichia coli, a facultative anaerobic, non-sporous, gram-negative bacterium, predominantly resides in vertebrate intestines, playing a crucial role in glycosidase production for the transformation of exogenous substances (Foster-Nyarko and Pallen, 2022). Notably, E. coli strain HGU-3 synthesizes β-glucuronidase, facilitating the conversion of baicalin to baicalein, which exhibits superior anti-oxidant and anti-inflammatory potential (Han et al., 2016; Li D. et al., 2019). Additionally, certain E. coli strains, like DH10B, demonstrate high curcumin-converting activity through the expression of NADPH-dependent curcumin/dihydrocurcumin reductase (CurA) (Hassaninasab et al., 2011; Tan et al., 2014). The resulting dihydrocurcumin (DHC) and tetrahydrocurcumin (THC) show promising therapeutic benefits in hepatic steatosis, surpassing the effects of curcumin (Chen et al., 2018; Yu et al., 2018).
Moreover, E. coli strains Nu, MC, and WC-1 exhibit cinnamyl esterase properties, releasing hydroxycinnamic acids with in-vitro and in-vivo anticancer and antioxidant potential (Zhao et al., 2022). Understanding the genetic and biochemical aspects of E. coli opens avenues for synthesizing natural product derivatives with diverse health benefits. This knowledge is pivotal for exploring the potential of E. coli in producing compounds such as DHC (dihydrocaffeic acid) and THC (tetrahydrocurcumin), which effectively regulate triglyceride levels and demonstrate novel therapeutic advantages in hepatic steatosis. Overall, comprehending the capabilities of E. coli contributes to the development of natural product derivatives with diverse health benefits (Chen et al., 2018; Yu et al., 2018; Rodríguez-Daza et al., 2021; Candeliere et al., 2022).
4.4 Eubacterium
The genus Eubacterium, a gram-positive constituent of the human gut microbiota, plays a vital part in metabolizing various substances (Mukherjee et al., 2020). Notably, E. ramulus, a well-studied flavonoid-degrading bacterium in the human intestine, produces enzymes like chalcone isomerase and flavanone-/flavanonol-cleaving reductase (Gall et al., 2014). These enzymes break down flavonoids into dihydrochalcone and its derivatives, known for their anti-inflammatory and antioxidant effects. E. ramulus strain wK1 further degrades flavonol quercetin and flavone luteolin, converting them into specific acids through reduction and ring fission (Zhao et al., 2022).
Another strain, E. cellulosolvens ATCC 43171T, is implicated in deglycosylating flavonoid O- and C-glucosides, exclusively catalyzing the deglycosylation of C-glucosides (Braune and Blaut, 2012; Braune et al., 2016). Additionally, Eubacterium L-8 exhibits the ability to hydrolyze terpenoid glycyrrhizin into 18β-glycyrrhetinic acid (18β-GA), which, in turn, demonstrates anti-inflammatory effects in preventing airway allergic inflammation (Liu et al., 2022). While these metabolic transformations highlight the potential health benefits of Eubacterium spp., it is emphasized that further in vivo studies are imperative to fully comprehend and harness the diverse advantages offered by this genus. This research could unlock opportunities to maximize the positive impact of Eubacterium strains on human health (Zhao et al., 2022).
4.5 Lactobacillus
The genus Lactobacillus, classified within the phylum Firmicutes, plays a crucial role in maintaining microbial balance and safeguarding gastrointestinal mucosal integrity (Dempsey and Corr, 2022). Certain Lactobacillus species are equipped with an array of metabolic enzymes, including α-rhamnosidases, tannase, and gallate decarboxylases, enabling them to transform exogenous substances (Reverón et al., 2017; Li B-C. et al., 2019; Ferreira-Lazarte et al., 2021).
L. rhamnosus NCTC 10302, possessing both β-glucosidase and α-rhamnosidase activities, demonstrates the ability to convert hesperetin-7-O-rutinoside and naringenin-7-O-rutinoside into their respective aglycones and 3-(phenyl) propionic acid through hydrolysis, ring fission, and dehydroxylation (Pereira-Caro et al., 2018). In a similar vein, L. plantarum expresses tannase, facilitating the hydrolysis of gallate and protocatechuate esters, ultimately producing gallic acid (Jiménez et al., 2014). Gallic acid, present in concentrations of 11.5–46 μg/ml, exhibits a protective part against LPS-induced inflammation and oxidative stress by suppressing the MAPK/NF-κB pathway and activating the Akt/AMPK/Nrf2 pathway (Tanaka et al., 2018).
Fang et al. witnessed the production of gallic acid and pyrogallol through the breakdown of gallotannins by gallotannin-metabolizing enzymes in L. plantarum WCFS1, suggesting potential prebiotic-probiotic assocaitions in preventing diet-induced metabolic diseases (Reverón et al., 2015; Fang et al., 2019). Additionally, Lactobacillus sp. Niu-O16 reduces daidzein to dihydrodaidzein with daidzein reductase activity, and dihydrodaidzein, at concentrations of 2.5–5 μM, supresses NF-κB activation and MAPK phosphorylation, therefore enhancing osteoporosis (Wang et al., 2007; Heng et al., 2019; Kim et al., 2019).
L. casei, L. plantarum, and L. acidophilus significantly impact the deglycosylation of piceid to resveratrol, enhancing bioavailability and bioactivity (Basholli-Salihu et al., 2016). Furthermore, feruloyl esterases from L. reuteri, L. helveticus, and L. fermentum hydrolyze chlorogenic acid, releasing caffeic acid (Santos et al., 2018). These results underscore the potential of Lactobacillus in health-improving pharmaceuticals and food products, but more investigation is required to clarify the fundamental transformation mechanisms (Zhao et al., 2022).
4.6 Bifidobacterium
Bifidobacterium, a widely distributed genus within the Actinobacteria phylum, serves as one of the initial colonizers in the human gut microbiota (Satti et al., 2021; He et al., 2023). Key species include B. angulatum, B. breve, B. Catenulatum, B. adolescentis, B. longum etc, collectively constituting less than 10% of the adult human microbiome yet playing a crucial role in host health (Turroni et al., 2008; Turroni et al., 2009; Hidalgo-Cantabrana et al., 2018). Some Bifidobacterium species express feruloyl esterase, enabling the generation of phenolic acids. For example, B. animalis’s feruloyl esterase can hydrolyze chlorogenic acid into caffeic acid (CAA) (Raimondi et al., 2015). CAA (10–30 mg/kg) has demonstrated hepatoprotective effects in mice, preventing acetaminophen-induced acute liver injury by enhancing Nrf2 transcription (Raimondi et al., 2015; Pang et al., 2016). Bifidobacterium’s involvement in the gut facilitates the metabolism of various compounds, including glycosides, saponins, and flavanones (Zhao et al., 2022).
Specific strains of Bifidobacterium, such as B. longum R0175 and B. longum SBT2928, exhibit distinct metabolic activities. B. longum R0175 facilitates the production of 3-(3′-hydroxyphenyl) propionic acid and 3-(phenyl) propionic acid from hesperidin by ring-cleavage and demethylation (Pereira-Caro et al., 2018). B. longum SBT2928 contributes to bile acid metabolism by hydrolyzing main human and animal bile salts, suggesting a potential role in reducing cholesterol levels in vivo (Tanaka et al., 2000). Furthermore, B. breve ATCC 15700 demonstrates the production of β-glucosidase, which cleaves glycosides in ginsenoside Rd., generating deglycosylated ginsenoside compound K (Zhong et al., 2016; Zhang et al., 2019). Such metabolic properties position Bifidobacterium as a viable option for symbiotic establishment, utilizing its natural product synthesis capabilities for potential therapeutic applications (Zhao et al., 2022).
5 The role of biotransformation in uncovering the active substance
The intricate connection between many natural compounds’ poor oral availability and significant pharmacological efficacy is being uncovered by studies on gut microbiota. The challenge lies in the complex structures of glycosides, hindering absorption by intestinal cells and limiting tissue-specific bio-accessibility. These substances undergo microbial enzymatic degradation, transforming into small molecule metabolites that exert diverse impacts on the host (Wardman et al., 2022).
Crucially, the therapeutic effects of natural compounds are significantly influenced by gut microorganisms. This is best illustrated by the conversion of ginsenosides into compound K (CK), which exhibits increased anti-inflammatory, anti-tumor, and lipid-reducing properties (Kim et al., 2013; Kim, 2018). Similarly, curcumin metabolites, influenced by the microbiota, exhibit anti-inflammatory properties through pathways such as PPARγ expression and NF-κB inhibition (Tang et al., 2021; Lu et al., 2022). Urolithin A (UA), a gut microbe-derived compound, showcases neuroprotective and anti-inflammatory properties (Gong et al., 2019; Huang et al., 2022).
Furthermore, the intricate interplay of form, function, composition, etc within the community of gut microbes unveils promising avenues for leveraging natural products. These compounds hold the potential to not only amplify therapeutic efficacy but also mitigate adverse effects, thereby offering a nuanced and refined approach to enhancing health outcomes. Gut microbes can modify the toxicity of certain compounds, such as reducing cardiotoxicity in digoxin metabolism (Kumar et al., 2018). However, harmful substances can also be synthesized by gut microorganisms, emphasizing the need for small molecule inhibitors to regulate specific transformations. Further research is necessary to determine how different doses of natural products affect gut microorganisms and metabolism because excessive consumption can upset the gut microbiota and cause negative effects (Lindell et al., 2022; Zhao et al., 2022).
6 Exploring human microbial metabolites
6.1 Screening to ecological insights
Diverse organisms, encompassing microorganisms, have long been recognized as prolific producers of secondary metabolites, also known as natural products (Law et al., 2020). Since the middle of the 20th century, the identification of these bioactive compounds, ranging from polyketides (PKs) to nonribosomal peptides (NRPs) and their hybrids, has been predominantly achieved through activity-based testing of cultivatable microorganisms, particularly Actinomycetes soil bacteria (Katz and Baltz, 2016). Over time, developments in molecular biology, DNA sequencing, and cultivation techniques have ushered in a new era, enabling the exploration of secondary metabolites from previously unculturable microbes (Lewis et al., 2010; Wilson and Piel, 2013; Sidebottom and Carlson, 2015; Browne et al., 2016). The impact of microbial natural products, as well as their semisynthetic derivatives, on human health, is profound, as they have emerged as vital sources for clinically relevant antibiotics, antifungals, immunosuppressants, anticancer agents, and other pharmaceuticals (Newman and Cragg, 2016). While the primary focus of natural product exploration has historically been medicinal usage, recent research has unveiled the intriguing ecological parts played by secondary metabolites in the organisms that produce them and their broader ecosystems, extending even to the human body (Crawford and Clardy, 2011; Cantley and Clardy, 2015; Wilson et al., 2017).
6.2 Secondary metabolites and health implications
The microbiome, consisting of a myriad of microorganisms that inhabit the human body (Figure 4), plays a crucial role in shaping human health and influencing various diseases. These microorganisms collectively harbor a gene pool that surpasses the human genome by a factor of 150 (Methé et al., 2012; Weinstock, 2012; Kho and Lal, 2018; Marsh et al., 2023; Walker and Hoyles, 2023). An analysis of data from the Human Microbiome Project (HMP), which includes genomic and metagenomic sequencing, has revealed that bacteria associated with humans possess the genetic machinery necessary to produce an extensive range of secondary metabolites (Donia et al., 2014). Despite this, the identities of the majority of such natural products remain undiscovered, and their functions are not fully comprehended. Investigating the ways through which these molecules impact interactions between hosts and microbes, as well as among different microbial species, could unveil factors that shape the impact of the microbiome on its host. This exploration holds the potential to yield targeted therapeutic options and may serve as a wellspring of innovative approaches to address human diseases. Few methods for uncovering natural products, and demonstrating their individual or collaborative use in deciphering the intricate metabolic interactions within the human microbiome have been presented (Wilson et al., 2017; Milshteyn et al., 2018).
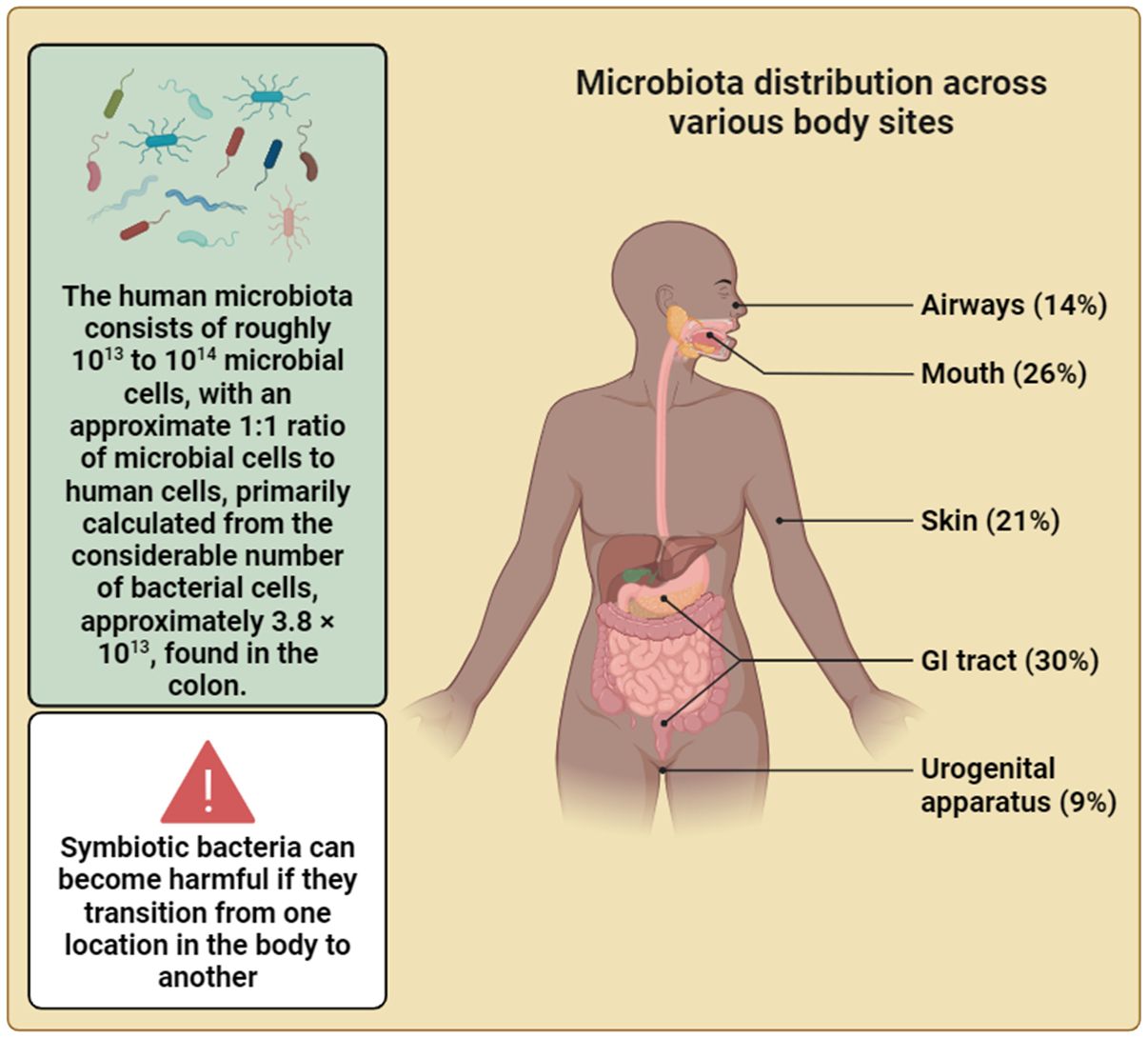
Figure 4 The intricate relationship between humans and their resident microbes is highlighted in this figure. The vast microbial community, comprising bacteria and other microbial organisms, plays a significant part in shaping human health and homeostasis, challenging traditional perceptions of the self as a singular entity. This depiction underscores the profound influence of the microbiota on human physiology and emphasizes the notion that, in the realm of the body’s cellular landscape, we are not alone. Additionally, this depiction underscores the emerging recognition of the gut microbiota as a key orchestrator of systemic health, influencing processes beyond digestion and immunity, including metabolism etc. (Created with Biorender).
6.2.1 Exploring biological functionality in metagenomic libraries through functional metagenomics
Microbial organisms exist widely in nature, thriving in diverse environmental conditions. Many of them cannot be cultured conventionally. Metagenomics allows the exploration of microbial communities directly from environmental samples, regardless of culturability. This method unveils species diversity and offers insights into their functions in natural settings. By cloning and expressing metagenomic DNA in a different host, function-based screenings can uncover novel proteins with industrial applications from previously inaccessible microorganisms. Functional metagenomics holds promise in industries like food and pharmaceuticals, facilitating the discovery of enzymes suitable for various processing conditions, novel bioactives including antimicrobial agents, and addressing concerns like development of antibiotic resistance (Coughlan et al., 2015).
Natural products have long played a pivotal role in the development of therapeutics for a variety of diseases. Traditionally, soil and marine environments have provided a rich reservoir from which diverse chemical scaffolds could be discovered. Recently, the human microbiome has been recognized as a promising niche from which secondary metabolites with therapeutic potential have begun to be isolated. The expansive history of identifying bacterial natural products in other environments is informing the approaches being brought to bear on the study of the human microbiota have also been addressed in many studies. These tools can lead to insights about microbe-microbe and host-microbe interactions and help generate biological hypotheses that may lead to developments of new therapeutic modalities (Milshteyn et al., 2018).
The functional metagenomic strategy seeks to explore bacterial metabolites through the systematic screening of metagenomic libraries for distinct bioactivities. Upon identifying a bioactive clone through functional screening, isolation of the clone and subsequent sequencing can pinpoint the gene or set of genes involved in producing the active metabolite. This direct association between the metabolite and its biosynthetic components is a key advantage of functional metagenomics. In the realm of the human microbiome, where a substantial portion of bacterial species have undergone full sequencing, this approach facilitates the simultaneous linking of bioactive metabolites with their functions inside the host and their producers within the Microbiome (Milshteyn et al., 2018).
Functional metagenomics can be applied in many different ways, but the most successful approaches that involve human microbiome metagenomic libraries have employed testing culture broth filtrates from metagenomic clones that are individually arrayed against assays using human cell reporter assays. In an early endeavor, Lakhdari and colleagues utilized a nuclear factor-kB (NF-kB) activation screen to identify 171 clones from a 2,640-clone human gut microbiome fosmid library, showing modulation of NF-kB reporter activity (Lakhdari et al., 2010). NF-kB, a rapidly inducible transcription factor with wide involvement in diverse cellular responses, is notably implicated in the immuno-inflammatory response in the gut, rendering it a suitable indicator for detecting various microbe-host interactions (Zhang et al., 2017).
In groundbreaking research, Cohen et al. (2015) (Cohen et al., 2015) employed functional metagenomics to identify host-associated bacterial effector genes, leading to the discovery of commendamide, a novel N-acyl amide. Commendamide was found to activate the GPR132/G2A receptor, implicated in immune cell functions. Subsequent research (Cohen et al., 2017) expanded the repertoire of N-acyl amides from the human microbiome, revealing structural similarities to endogenous GPCR ligands. Notably, certain bacterial GPR119 agonists mirrored the effects of endogenous ligands, regulating metabolic hormones and glucose homeostasis in mouse models. This suggests a potential for microbiome-biosynthetic gene therapy as a novel therapeutic modality. These studies highlight how functional metagenomics unveils microbiome-host interactions, identifies therapeutic targets, and explores microbiota-derived small molecules for innovative therapeutic strategies (Milshteyn et al., 2018).
6.2.2 Methods for extracting natural products through sequence-guided exploration of (Meta) genomes
Genome mining represents a computational approach to small-molecule discovery, relying on algorithmic predictions to determine biosynthetic gene clusters (BGCs) within sequence data. Established algorithms, such as antiSMASH, NP.searcher, MultiGeneBlast, etc predominantly utilize conserved sequences from extensively studied BGC groups to pinpoint novel gene clusters within these families (Medema et al., 2013; Reddy et al., 2014; Medema and Fischbach, 2015). Recent developments, exemplified by ClusterFinder, aim to extend this capability to discover previously unknown BGC groups (Cimermancic et al., 2014). The detailed examination of the computational complexities associated with sequence-guided natural product identification has been extensively explored in other sources (Medema and Fischbach, 2015), our focus here centers on the application of sequence-based methods for bacterial metabolite identification within the human microbiome (Milshteyn et al., 2018).
Over the last decade, significant initiatives have been undertaken to sequence and annotate human microbiome bacteria, driven by a keen interest in understanding the microbiota’s impact on human health. This has resulted in a remarkable wealth of sequence data unique to the human microbiome, encompassing over 3,000 full and partial reference genomes, along with numerous (meta)genomic shotgun sequencing datasets from both healthy and sick individuals (Aagaard et al., 2013; Donia et al., 2014). Utilizing this data, researchers are currently employing bioinformatics tools to uncover new secondary metabolite BGCs within the human microbiome. The overarching objective of these bioinformatics-driven discovery endeavors is to elucidate and functionally characterize the metabolites that are encoded by recently identified natural product BGCs (Milshteyn et al., 2018).
Utilizing the ClusterFinder algorithm, researchers identified over fourteen thousand biosynthetic gene clusters (BGCs) in the Human Microbiome Project (HMP) data, revealing the prevalence of various small molecule families, including thiopeptides (Cimermancic et al., 2014). Lactocillin, a novel thiopeptide antibiotic produced by the vaginal isolate Lactobacillus gasseri JV-V03, demonstrated potent activity against pathogenic bacteria (Mullane et al., 2015). Another innovative approach, involving the synthesis of bioinformatically predicted natural product-like structures termed syn-BNPs (Chu et al., 2016), led to the discovery of humimycins with broad-spectrum antibiotic activity. Genome mining also uncovered a family of 47 nonribosomal peptide synthetase (NRPS) BGCs present in over 90% of HMP stool samples, producing pyrazinones and dihydropyrazinones (Guo et al., 2017). Additionally, the exploration of mucin-utilizing bacteria and the identification of the fldAIBC cluster shed light on a specific bacterial metabolic pathway involving indoleacrylic acid, linking it to protective effects in a colitis model and proposing a connection between a healthy mucus layer, anti-inflammatory metabolites, and inflammatory bowel disease (IBD) (Wlodarska et al., 2017). These findings highlight the potential of mining the human microbiome for novel therapeutics and insights into host-microbe interactions (Milshteyn et al., 2018).
6.2.3 Exploring chemistry-centric strategies for uncovering natural products in the human microbiome
Many instances arise where genome-centric approaches are not the primary focus, highlighting the diverse avenues of research that extend beyond the realm of genomics. Essentially, both pathogenic and nonpathogenic bacteria have had their secondary metabolites studied for many years, predominantly through laboratory cultivation and analytical chemistry. The examination of individual bacteria in vitro has yielded a considerable array of compounds, with varied degrees of intricacy and biological significance, and these have been thoroughly reviewed in the latest research (Donia and Fischbach, 2015; Mousa et al., 2017). With the current intense scientific exploration of the human microbiome, there is a growing interest in delving into deeper biological contexts for in vitro-discovered secondary metabolites. This aspect has not always been a forefront consideration in traditional natural product discoveries. Increasingly, robust foundations of chemistry-centric discovery techniques are now employed to formulate intriguing biological hypotheses based on identified natural products. Strong underpinnings of chemistry-focused discovery methods are increasingly being employed to formulate intriguing biological theories rooted in discovered natural compounds (Milshteyn et al., 2018).
Utilizing sophisticated analytical methods, like liquid chromatography-mass spectrometry (LC-MS), has proven instrumental in dissecting bacterial metabolites and unraveling their functional roles. For instance, research in 2009 demonstrated that Lactobacillus plantarum, a beneficial probiotic, might reduce inflammation both in vivo and in vitro by regulating NF-kB (Petrof et al., 2009; Van Baarlen et al., 2009; Bansal et al., 2010). Zvanych et al.’s (2014) (Zvanych et al., 2014) study further applied principal component analysis to LC-MS data, identifying dipeptide molecules with pyroglutamic rings, like pyro-phenylalanine and pyro-tryptophan, which, when introduced into mice, resulted into reduced splenic synthesis of IFN-g. Another notable approach involves the functional evaluation of secreted bacterial metabolites, as illustrated by the discovery of lugdunin, a novel antibiotic from nasal bacteria. Metabolomics platforms, especially those employing HRMS (High-Resolution Mass Spectrometry), have performed an essential part in examining the chemical fingerprint of the human microbiota, offering insights into diverse biological activities and potential biomarkers, as seen in studies comparing germ-free and colonized mice, cardiovascular health, and neurological diseases in mouse models (Table 2) (Wikoff et al., 2009; Hsiao et al., 2013; Brown and Hazen, 2017; Hou et al., 2022). While challenges persist in identifying unknown secondary metabolites, the evolving strategies for HRMS data collection and analysis provide optimism for unearthing therapeutic leads in the future (Peisl et al., 2018).
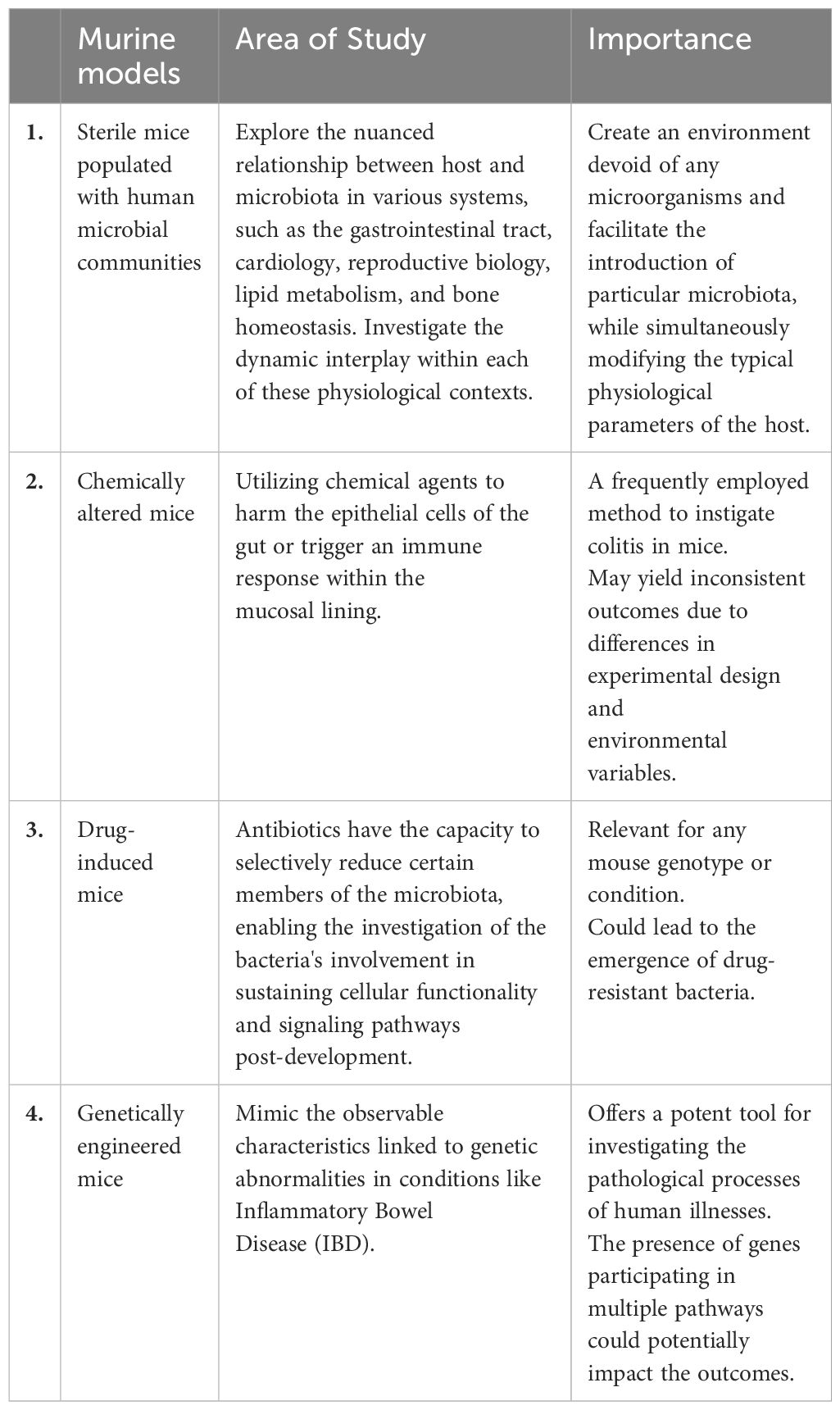
Table 2 Microbiota research: insights from murine models (Hou et al., 2022).
7 Conclusion
The exploration of the human microbiome and its intricate relationship with natural products has unveiled a captivating realm within the field of biology. The human digestive system, teeming with a diverse microbiota, serves as a dynamic ecosystem comprising an astounding number of microorganisms. This microbial assembly, primarily constituted of anaerobic bacteria, orchestrates processes finely tuned to human physiology, functioning as an organ with a genetic repertoire surpassing the human genome. Among its pivotal roles is the breakdown of indigestible dietary components, highlighting its indispensable contribution to our overall well-being.
The impact of the microbiome extends beyond mere digestion, permeating into complex physiological processes, influencing immune system modulation, and perhaps aiding in the growth of the CNS and brain (Table 3) (Ghosh et al., 2021). Mouse models have underscored the vital role of a healthy bacterial environment in maintaining normal physiological processes, while dysbiosis has been associated with an array of diseases. Beyond dietary influences, gut microbiota is significantly affected by various factors (Figure 5) and exhibits rapid responses to system alterations due to the interplay between genetic and environmental factors. The burgeoning field of microbiome research is evidenced due to the increase in clinical studies and investments, emphasizing its potential to revolutionize healthcare. There is increasing evidence linking host-associated bacteria to both healthy development and disease, but there is still a significant knowledge vacuum regarding the precise mechanisms by which bacterial activities impact the physiology of mammals or microbiomes. The traditional molecular biology dogma falls short of elucidating the ultimate outcomes of information transfer within a biological system, particularly when it comes to small molecules and natural products.
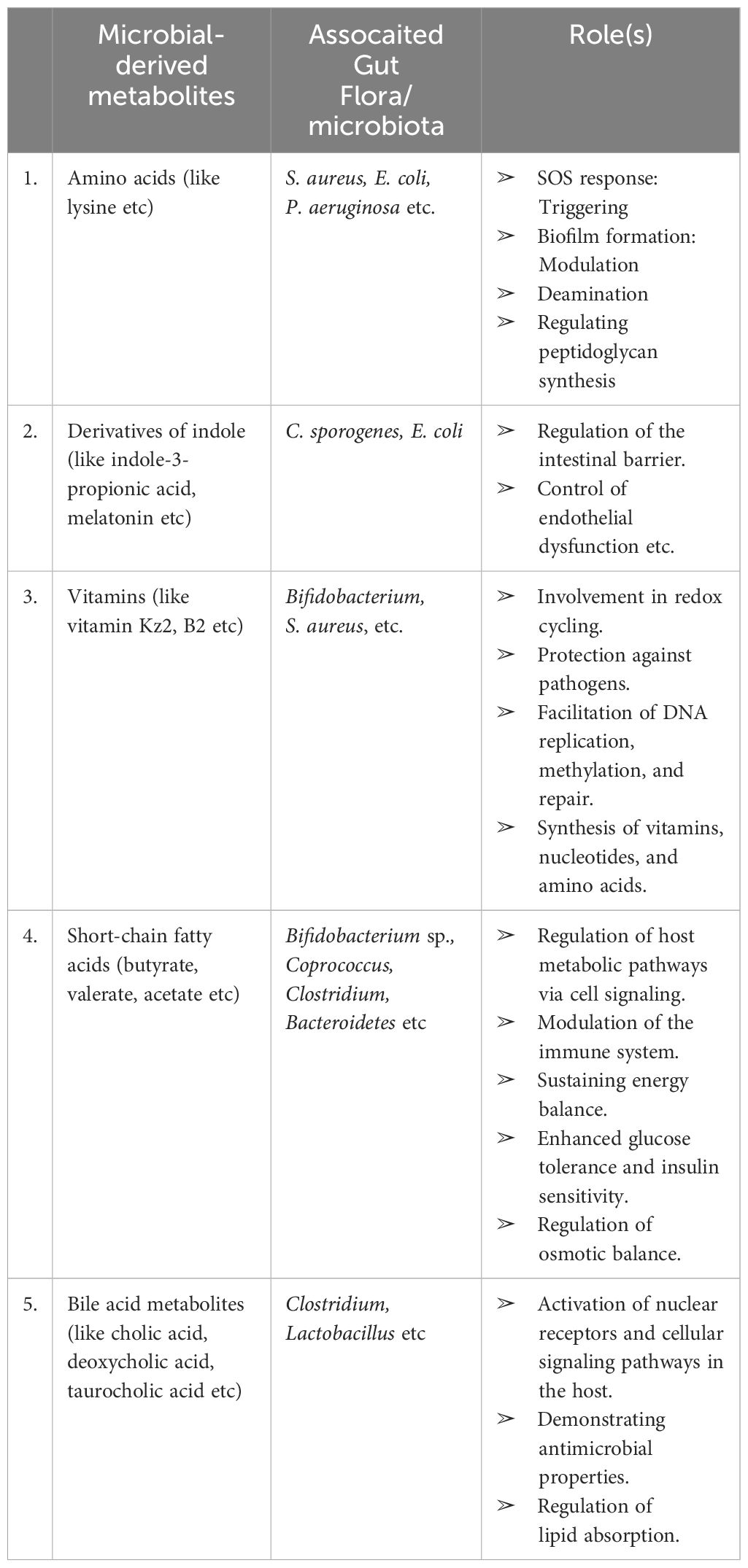
Table 3 Compilation of microbial metabolites, gut microbiota, and their roles (Ghosh et al., 2021).
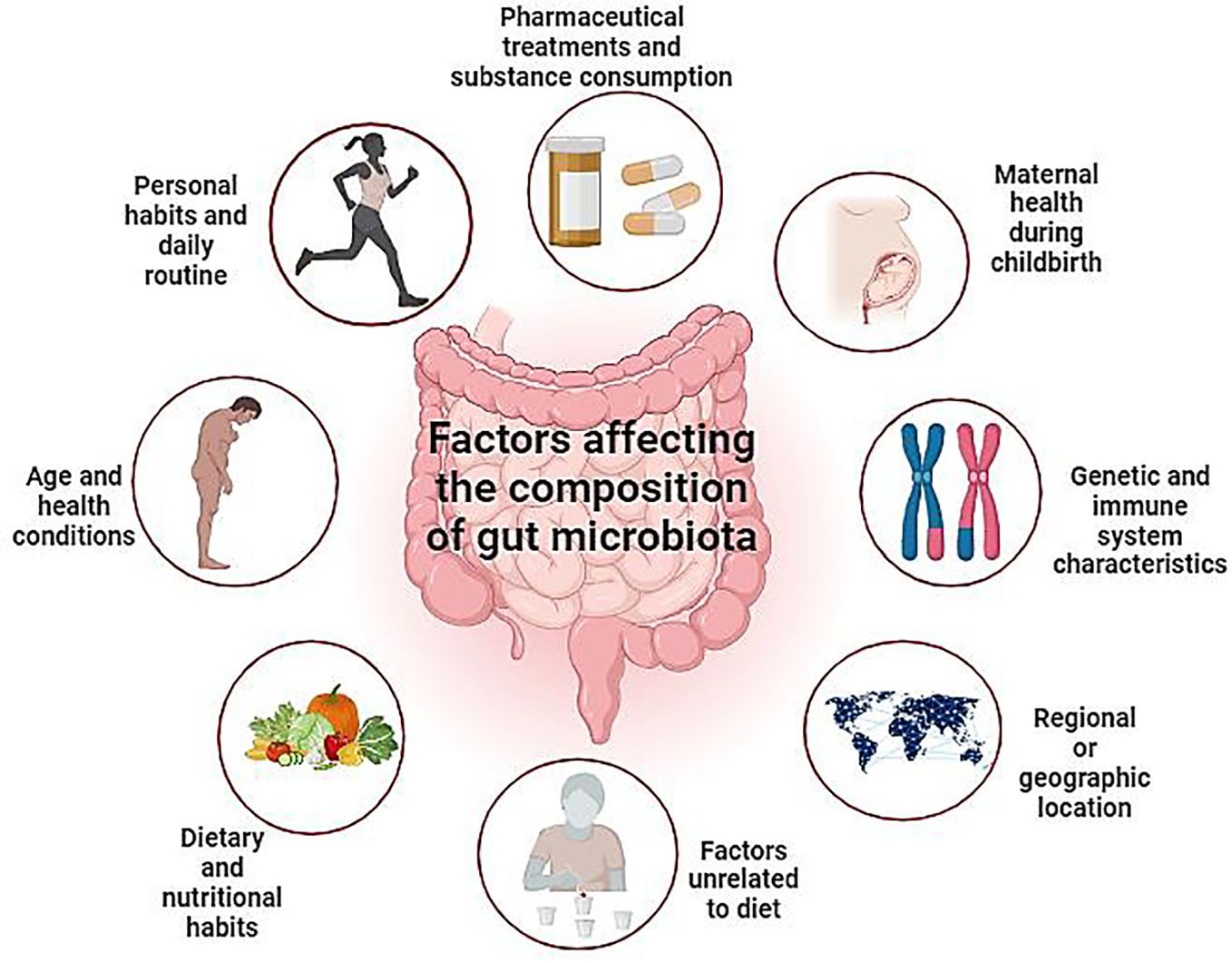
Figure 5 Depicts diverse elements influencing gut microbiota modulation, encompassing lifestyle, age, medication, genetics, geography, and dietary factors. This comprehensive overview highlights the multifaceted nature of gut microbiota dynamics. By highlighting these intricate relationships, this visual representation enhances our understanding of the dynamic interplay between external influences and internal microbial ecosystems, ultimately informing personalized approaches to gut health management.” (Created with Biorender).
Gut microorganisms perform a pivotal function in decomposing and transforming natural products, generating a diverse array of metabolites and functional compounds. The chemical transformations involved significantly regulate the form of natural product substrates. Importantly, the impact of gut microbiota extends beyond structural modifications, influencing the chemical landscape, pharmacological activities, and metabolic mechanisms of natural products. Intriguingly, the perspective of harnessing gut microorganisms for extensive manufacturing of active metabolites and compound synthesis remains largely unexplored. The study of gut microorganisms, their metabolites, and the intricate reactions concerned with the dynamic interplay between natural products and gut microbiota holds immense importance. Unraveling these interactions is not only crucial for understanding pharmacological mechanisms but also for unlocking the untapped potential of natural products in various applications.
8 Future perspectives
Looking ahead, the field stands at the precipice of unprecedented discoveries and applications. Delving into the study of gut microbes and their role in the synthesis of bioactive compounds on a larger scale presents an exciting avenue for future research. The untapped potential of harnessing the therapeutic benefits of these interactions holds promise for innovative approaches to medicine and therapeutics. The multifaceted relationships between natural products and gut microbiota open new doors for drug discovery, as the microbiome becomes a potential source of novel compounds with therapeutic applications. Future research should focus on elucidating the specific mechanisms by which these microbiome-derived naturally occurring compounds exert their effects on the well-being of humans. This involves a deeper understanding of the metabolic pathways involved, the pharmacological activities of these compounds, and their potential applications in treating various diseases.
Moreover, the development of technologies and methodologies for the systematic characterization of small molecules generated by human-associated microorganisms is crucial. Advancements in analytical techniques and high-throughput screening methods will facilitate the identification and isolation of novel microbiome-derived natural products. Collaborations between microbiologists, pharmacologists, and clinicians will be essential in translating these discoveries into practical applications. The integration of microbiome-based therapies into personalized medicine holds the potential to revolutionize healthcare, offering tailored treatments based on an individual’s unique microbiome profile. In a nutshell, the intersection of natural products, bacteria, and human health represents a frontier of scientific exploration with profound implications for medicine and therapeutics. As we navigate this dynamic field, the collaborative efforts of researchers across disciplines will be instrumental in unlocking the full potential of microbiome-derived natural products and ushering in a new era of innovative healthcare solutions.
Author contributions
HQ: Conceptualization, Writing – original draft, Writing – review & editing. AHS: Writing – review & editing. AA: Funding acquisition, Writing – review & editing. MM: Conceptualization, Funding acquisition, Project administration, Supervision, Writing – review & editing.
Funding
The author(s) declare financial support was received for the research, authorship, and/or publication of this article. This work was financially supported by grants to MM by the Science and Engineering Research Board, Department of Science and Technology (SERB-DST) Govt. of India, New Delhi, vide Project Grant No: TAR/001213/2018.
Acknowledgments
HQ acknowledges fellowship from the Indian Council of Medical Research ICMR-SRF (2021–9917). The authors are thankful to the SERB-DST Govt. of India for financial support.
Conflict of interest
The authors declare that the research was conducted in the absence of any commercial or financial relationships that could be construed as a potential conflict of interest.
Publisher’s note
All claims expressed in this article are solely those of the authors and do not necessarily represent those of their affiliated organizations, or those of the publisher, the editors and the reviewers. Any product that may be evaluated in this article, or claim that may be made by its manufacturer, is not guaranteed or endorsed by the publisher.
References
Aagaard, K., Petrosino, J., Keitel, W., Watson, M., Katancik, J., Garcia, N., et al. (2013). The Human Microbiome Project strategy for comprehensive sampling of the human microbiome and why it matters. FASEB J. 27, 1012. doi: 10.1096/fj.12-220806
Abou-Donia, M. B., El-Masry, E. M., Abdel-Rahman, A. A., McLendon, R. E., Schiffman, S. S. (2008). Environmental Health, Splenda alters gut microflora and increases intestinal p-glycoprotein and cytochrome p-450 in male rats. J. Toxicol. Environ. Health A. 71, 1415–1429. doi: 10.1080/15287390802328630
Agunloye, O. M., Oboh, G., Ademiluyi, A. O., Ademosun, A. O., Akindahunsi, A. A., Oyagbemi, A. A., et al. (2019). Cardio-protective and antioxidant properties of caffeic acid and chlorogenic acid: Mechanistic role of angiotensin converting enzyme, cholinesterase and arginase activities in cyclosporine induced hypertensive rats. Biomed. Pharmacother. 109, 450–458. doi: 10.1016/j.biopha.2018.10.044
Alexander, C., Rietschel, E. T. (2001). Invited review: Bacterial lipopolysaccharides and innate immunity. J Endotoxin Res. 7, 167–202. doi: 10.1177/09680519010070030101
Alexander, J. L., Wilson, I. D., Teare, J., Marchesi, J. R., Nicholson, J. K., Kinross, J. M. (2017). Gut microbiota modulation of chemotherapy efficacy and toxicity. Nat. Rev. Gastroenterol. Hepatol. 14, 356–365. doi: 10.1038/nrgastro.2017.20
Amabebe, E., Robert, F. O., Agbalalah, T., Orubu, E. S. (2020). Microbial dysbiosis-induced obesity: role of gut microbiota in homoeostasis of energy metabolism. Br. J. Nutr. 123, 1127–1137. doi: 10.1017/S0007114520000380
Arumugam, M., Raes, J., Pelletier, E., Le Paslier, D., Yamada, T., Mende, D. R., et al. (2011). Enterotypes of the human gut microbiome. Nature 473, 174–180. doi: 10.1038/nature09944
Bäckhed, F., Ding, H., Wang, T., Hooper, L. V., Koh, G. Y., Nagy, A., et al. (2004). The gut microbiota as an environmental factor that regulates fat storage. Proc. Natl. Acad. Sci. 101, 15718–15723. doi: 10.1073/pnas.0407076101
Bajaj, J. S., Heuman, D. M., Hylemon, P. B., Sanyal, A. J., White, M. B., Monteith, P., et al. (2014). Altered profile of human gut microbiome is associated with cirrhosis and its complications. J. Hepatol. 60, 940–947. doi: 10.1016/j.jhep.2013.12.019
Balagopal, A., Philp, F. H., Astemborski, J., Block, T. M., Mehta, A., Long, R., et al. (2008). Human immunodeficiency virus-related microbial translocation and progression of hepatitis C. Gastroenterology 135, 226–233. doi: 10.1053/j.gastro.2008.03.022
Bansal, T., Alaniz, R. C., Wood, T. K., Jayaraman, A. (2010). The bacterial signal indole increases epithelial-cell tight-junction resistance and attenuates indicators of inflammation. Proc. Natl. Acad. Sci. 107, 228–233. doi: 10.1073/pnas.0906112107
Basholli-Salihu, M., Schuster, R., Mulla, D., Praznik, W., Viernstein, H., Mueller, M. J. B., et al. (2016). Bioconversion of piceid to resveratrol by selected probiotic cell extracts. Bioprocess Biosyst. Eng. 39, 1879–1885. doi: 10.1007/s00449-016-1662-1
Bennet, S. M., Böhn, L., Störsrud, S., Liljebo, T., Collin, L., Lindfors, P., et al. (2018). Multivariate modelling of faecal bacterial profiles of patients with IBS predicts responsiveness to a diet low in FODMAPs. Gut 67, 872–881. doi: 10.1136/gutjnl-2016-313128
Benson, A. K. (2015). Host genetic architecture and the landscape of microbiome composition: humans weigh in. Genome Biol. 16, 1–4. doi: 10.1186/s13059-015-0775-1
Benson, A. K., Kelly, S. A., Legge, R., Ma, F., Low, S. J., Kim, J., et al. (2010). Individuality in gut microbiota composition is a complex polygenic trait shaped by multiple environmental and host genetic factors. Proc. Natl. Acad. Sci. 107, 18933–18938. doi: 10.1073/pnas.1007028107
Bian, X., Chi, L., Gao, B., Tu, P., Ru, H., Lu, K. (2017). Gut microbiome response to sucralose and its potential role in inducing liver inflammation in mice. Front, Physiol. 8, 487. doi: 10.3389/fphys.2017.00487
Blaser, M. J. (2006). Who are we? Indigenous microbes and the ecology of human diseases. EMBO reports 7, 956–960. doi: 10.1038/sj.embor.7400812
Blaser, M. J. (2016). Antibiotic use and its consequences for the normal microbiome. Science 352, 544–545. doi: 10.1126/science.aad9358
Bonder, M. J., Kurilshikov, A., Tigchelaar, E. F., Mujagic, Z., Imhann, F., Vila, A. V., et al. (2016a). The effect of host genetics on the gut microbiome. Nat. Genet. 48, 1407–1412. doi: 10.1038/ng.3663
Bonder, M. J., Tigchelaar, E. F., Cai, X., Trynka, G., Cenit, M. C., Hrdlickova, B., et al. (2016b). The influence of a short-term gluten-free diet on the human gut microbiome. Genome Med. 8, 1–11. doi: 10.1186/s13073-016-0295-y
Bongers, G., Pacer, M. E., Geraldino, T. H., Chen, L., He, Z., Hashimoto, D., et al. (2014). Interplay of host microbiota, genetic perturbations, and inflammation promotes local development of intestinal neoplasms in mice. J. Exp. Med. 211, 457–472. doi: 10.1084/jem.20131587
Bouskra, D., Brézillon, C., Bérard, M., Werts, C., Varona, R., Boneca, I. G., et al. (2008). Lymphoid tissue genesis induced by commensals through NOD1 regulates intestinal homeostasis. Nature 456, 507–510. doi: 10.1038/nature07450
Braune, A., Blaut, M. (2012). Intestinal bacterium Eubacterium cellulosolvens deglycosylates flavonoid C-and O-glucosides. Appl. Environ. Microbiol. 78, 8151–8153. doi: 10.1128/AEM.02115-12
Braune, A., Engst, W., Blaut, M. (2016). Identification and functional expression of genes encoding flavonoid O-and C-glycosidases in intestinal bacteria. Environ. Microbiol. 18, 2117–2129. doi: 10.1111/1462-2920.12864
Brinkman, B., Hildebrand, F., Kubica, M., Goosens, D., Del Favero, J., Declercq, W., et al. (2011). Caspase deficiency alters the murine gut microbiome. Cell Death Dis. 2. doi: 10.1038/cddis.2011.101
Brown, J. M., Hazen, S. L. (2017). Targeting of microbe-derived metabolites to improve human health: the next frontier for drug discovery. J. Biol. Chem. 292, 8560–8568. doi: 10.1074/jbc.R116.765388
Browne, H. P., Forster, S. C., Anonye, B. O., Kumar, N., Neville, B. A., Stares, M. D., et al. (2016). Culturing of ‘unculturable’human microbiota reveals novel taxa and extensive sporulation. Nature 533, 543–546. doi: 10.1038/nature17645
Burapan, S., Kim, M., Han, J. (2017). Curcuminoid demethylation as an alternative metabolism by human intestinal microbiota. J. Agric. Food Chem. 65, 3305–3310. doi: 10.1021/acs.jafc.7b00943
Candeliere, F., Raimondi, S., Ranieri, R., Musmeci, E., Zambon, A., Amaretti, A., et al. (2022). β-Glucuronidase pattern predicted from gut metagenomes indicates potentially diversified pharmacomicrobiomics. Front. Microbiol. 13, 826994. doi: 10.3389/fmicb.2022.826994
Cantley, A. M., Clardy, J. (2015). Animals in a bacterial world: opportunities for chemical ecology. Nat. Prod. Rep. 32, 888–892. doi: 10.1039%2Fc4np00141a
Chassaing, B., Koren, O., Goodrich, J. K., Poole, A. C., Srinivasan, S., Ley, R. E., et al. (2015). Dietary emulsifiers impact the mouse gut microbiota promoting colitis and metabolic syndrome. Nature 519, 92–96. doi: 10.1038/nature14232
Chen, J.-W., Kong, Z.-L., Tsai, M.-L., Lo, C.-Y., Ho, C.-T., Lai, C.-S. (2018). Tetrahydrocurcumin ameliorates free fatty acid-induced hepatic steatosis and improves insulin resistance in HepG2 cells. J. Food Drug Anal. 26, 1075–1085. doi: 10.1016/j.jfda.2018.01.005
Chen, Y., Yang, F., Lu, H., Wang, B., Chen, Y., Lei, D., et al. (2011). Characterization of fecal microbial communities in patients with liver cirrhosis. Hepatology 54, 562–572. doi: 10.1002/hep.24423
Cheng, J.-R., Liu, X.-M., Chen, Z.-Y., Zhang, Y.-S., Zhang, Y.-H. (2016). Mulberry anthocyanin biotransformation by intestinal probiotics. Food Chem. 213, 721–727. doi: 10.1016/j.foodchem.2016.07.032
Chu, J., Vila-Farres, X., Inoyama, D., Ternei, M., Cohen, L. J., Gordon, E. A., et al. (2016). Discovery of MRSA active antibiotics using primary sequence from the human microbiome. Nat. Chem. Biol. 12, 1004–1006. doi: 10.1038/nchembio.2207
Cimermancic, P., Medema, M. H., Claesen, J., Kurita, K., Brown, L. C. W., Mavrommatis, K., et al. (2014). Insights into secondary metabolism from a global analysis of prokaryotic biosynthetic gene clusters. Cell 158, 412–421. doi: 10.1016/j.cell.2014.06.034
Cohen, L. J., Esterhazy, D., Kim, S.-H., Lemetre, C., Aguilar, R. R., Gordon, E. A., et al. (2017). Commensal bacteria make GPCR ligands that mimic human signalling molecules. Nature 549, 48–53. doi: 10.1038/nature23874
Cohen, L. J., Kang, H.-S., Chu, J., Huang, Y.-H., Gordon, E. A., Reddy, B. V. B., et al. (2015). Functional metagenomic discovery of bacterial effectors in the human microbiome and isolation of commendamide, a GPCR G2A/132 agonist. Proc. Natl. Acad. Sci. 112, E4825–E4834. doi: 10.1073/pnas.1508737112
Costea, P. I., Hildebrand, F., Arumugam, M., Bäckhed, F., Blaser, M. J., Bushman, F. D., et al. (2018). Enterotypes in the landscape of gut microbial community composition. Nat. Microbiol. 3, 8–16. doi: 10.1038/s41564-017-0072-8
Coughlan, L. M., Cotter, P. D., Hill, C., Alvarez-Ordóñez, A. (2015). Biotechnological applications of functional metagenomics in the food and pharmaceutical industries. Front. Microbiol. 6, 149339. doi: 10.3389/fmicb.2015.00672
Crawford, J. M., Clardy, J. (2011). Bacterial symbionts and natural products. Chem. Commun. 47, 7559–7566. doi: 10.1039/c1cc11574j
De Filippo, C., Cavalieri, D., Di Paola, M., Ramazzotti, M., Poullet, J. B., Massart, S., et al. (2010). Impact of diet in shaping gut microbiota revealed by a comparative study in children from Europe and rural Africa. Proc. Natl. Acad. Sci. 107, 14691–14696. doi: 10.1073/pnas.1005963107
Dempsey, E., Corr, S. C. (2022). Lactobacillus spp. for gastrointestinal health: Current and future perspectives. Front. Immunol. 13, 840245. doi: 10.3389/fimmu.2022.840245
de Vos, W. M., Tilg, H., Van Hul, M., Cani, P. D. (2022). Gut microbiome and health: mechanistic insights. Gut 71, 1020–1032. doi: 10.1136/gutjnl-2021-326789
Donia, M. S., Cimermancic, P., Schulze, C. J., Brown, L. C. W., Martin, J., Mitreva, M., et al. (2014). A systematic analysis of biosynthetic gene clusters in the human microbiome reveals a common family of antibiotics. Cell 158, 1402–1414. doi: 10.1016/j.cell.2014.08.032
Donia, M. S., Fischbach, M. A. (2015). Small molecules from the human microbiota. Science 349, 1254766. doi: 10.1126/science.1254766
Falony, G., Joossens, M., Vieira-Silva, S., Wang, J., Darzi, Y., Faust, K., et al. (2016). Population-level analysis of gut microbiome variation. Science 352, 560–564. doi: 10.1126/science.aad3503
Fan, Y., Pedersen, O. (2021). Gut microbiota in human metabolic health and disease. Nat. Rev. Microbiol. 19, 55–71. doi: 10.1038/s41579-020-0433-9
Fang, C., Kim, H., Yanagisawa, L., Bennett, W., Sirven, M. A., Alaniz, R. C., et al. (2019). Gallotannins and Lactobacillus plantarum WCFS1 mitigate high-fat diet-induced inflammation and induce biomarkers for thermogenesis in adipose tissue in gnotobiotic mice. Mol. Nutr. Food Res. 63, 1800937. doi: 10.1002/mnfr.201800937
Ferreira-Lazarte, A., Plaza-Vinuesa, L., de Las Rivas, B., Villamiel, M., Muñoz, R., Moreno, F. J. (2021). Production of α-rhamnosidases from Lactobacillus plantarum WCFS1 and their role in deglycosylation of dietary flavonoids naringin and rutin. Int. J. Biol. Macromol. 193, 1093–1102. doi: 10.1016/j.ijbiomac.2021.11.053
Flint, H. J., Scott, K. P., Louis, P., Duncan, S. H. (2012). The role of the gut microbiota in nutrition and health. Nat. Rev. Gastroenterol. Hepatol. 9, 577–589. doi: 10.1038/nrgastro.2012.156
Foster-Nyarko, E., Pallen, M. J. (2022). The microbial ecology of Escherichia coli in the vertebrate gut. FEMS Microbiol. Rev. 46, fuac008. doi: 10.1093/femsre/fuac008
Gall, M., Thomsen, M., Peters, C., Pavlidis, I. V., Jonczyk, P., Grünert, P. P., et al. (2014). Enzymatic conversion of flavonoids using bacterial chalcone isomerase and enoate reductase. Angew. Chem. Int. Ed. 53, 1439–1442. doi: 10.1002/anie.201306952
Garrett, W. S., Lord, G. M., Punit, S., Lugo-Villarino, G., Mazmanian, S. K., Ito, S., et al. (2007). Communicable ulcerative colitis induced by T-bet deficiency in the innate immune system. Cell 131, 33–45. doi: 10.1016/j.cell.2007.08.017
Ghosh, S., Whitley, C. S., Haribabu, B., Jala, V. R. (2021). Regulation of intestinal barrier function by microbial metabolites. Cell. Mol. Gastroenterol. Hepatol. 11, 1463–1482. doi: 10.1016/j.jcmgh.2021.02.007
Gibson, P. R. (2017). The evidence base for efficacy of the low FODMAP diet in irritable bowel syndrome: is it ready for prime time as a first-line therapy? J. Gastroenterol. Hepatol. 32, 32–35. doi: 10.1111/jgh.13693
Gong, Z., Huang, J., Xu, B., Ou, Z., Zhang, L., Lin, X., et al. (2019). Urolithin A attenuates memory impairment and neuroinflammation in APP/PS1 mice. J. Neuroinflammation 16, 1–13. doi: 10.1186/s12974-019-1450-3
Goodrich, J. K., Waters, J. L., Poole, A. C., Sutter, J. L., Koren, O., Blekhman, R., et al. (2014). Human genetics shape the gut microbiome. Cell. 159, 789–799. doi: 10.1016/j.cell.2014.09.053
Gorvitovskaia, A., Holmes, S. P., Huse, S. M. (2016). Interpreting Prevotella and Bacteroides as biomarkers of diet and lifestyle. Microbiome 4, 1–12. doi: 10.1186/s40168-016-0160-7
Guo, C.-J., Chang, F.-Y., Wyche, T. P., Backus, K. M., Acker, T. M., Funabashi, M., et al. (2017). Discovery of reactive microbiota-derived metabolites that inhibit host proteases. Cell 168, 517–526. e18. doi: 10.1016/j.cell.2016.12.021
Guo, L., Katiyo, W., Lu, L., Zhang, X., Wang, M., Yan, J., et al. (2018). Glycyrrhetic acid 3-O-mono-β-d-glucuronide (GAMG): an innovative high-potency sweetener with improved biological activities. Compr. Rev. Food Sci. Food Saf. 17, 905–919. doi: 10.1111/1541-4337.12353
Halmos, E. P. (2017). When the low FODMAP diet does not work. J. Gastroenterol. Hepatol. 32, 69–72. doi: 10.1111/jgh.13701
Han, D. H., Lee, Y., Ahn, J.-H. (2016). Biological synthesis of baicalein derivatives using Escherichia coli. J. Microbiol. Biotechnol. 26, 1918–1923. doi: 10.4014/jmb.1605.05050
Hashimoto, T., Perlot, T., Rehman, A., Trichereau, J., Ishiguro, H., Paolino, M., et al. (2012). ACE2 links amino acid malnutrition to microbial ecology and intestinal inflammation. Nature 487, 477–481. doi: 10.1038/nature11228
Hassaninasab, A., Hashimoto, Y., Tomita-Yokotani, K., Kobayashi, M. (2011). Discovery of the curcumin metabolic pathway involving a unique enzyme in an intestinal microorganism. Proc. Natl. Acad. Sci. 108, 6615–6620. doi: 10.1073/pnas.1016217108
Hatamipour, M., Ramezani, M., Tabassi, S. A. S., Johnston, T. P., Sahebkar, A. (2019). Demethoxycurcumin: A naturally occurring curcumin analogue for treating non-cancerous diseases. J. Cell. Physiol. 234, 19320–19330. doi: 10.1002/jcp.28626
He, B.-L., Xiong, Y., Hu, T.-G., Zong, M.-H., Wu, H. (2023). Bifidobacterium spp. as functional foods: A review of current status, challenges, and strategies. Crit. Rev. Food Sci. Nutr. 63, 8048–8065. doi: 10.1080/10408398.2022.2054934
Hehemann, J.-H., Correc, G., Barbeyron, T., Helbert, W., Czjzek, M., Michel, G. (2010). Transfer of carbohydrate-active enzymes from marine bacteria to Japanese gut microbiota. Nature 464, 908–912. doi: 10.1038/nature08937
Henao-Mejia, J., Elinav, E., Jin, C., Hao, L., Mehal, W. Z., Strowig, T., et al. (2012). Inflammasome-mediated dysbiosis regulates progression of NAFLD and obesity. Nature 482, 179–185. doi: 10.1038/nature10809
Heng, Y., Kim, M. J., Yang, H. J., Kang, S., Park, S. (2019). Lactobacillus intestinalis efficiently produces equol from daidzein and chungkookjang, short-term fermented soybeans. Arch. Microbiol. 201, 1009–1017. doi: 10.1007/s00203-019-01665-5
Hidalgo-Cantabrana, C., Delgado, S., Ruiz, L., Ruas-Madiedo, P., Sánchez, B., Margolles, A. (2018). “Bifidobacteria and their health-promoting effects,” in Bugs as Drugs: Therapeutic Microbes for the Prevention and Treatment of Disease, 73–98. doi: 10.1128/9781555819705.ch3
Hildebrand, F., Nguyen, T. L. A., Brinkman, B., Yunta, R. G., Cauwe, B., Vandenabeele, P., et al. (2013). Inflammation-associated enterotypes, host genotype, cage and inter-individual effects drive gut microbiota variation in common laboratory mice. Genome Biol. 14, 1–15. doi: 10.1186/gb-2013-14-1-r4
Hillman, E. T., Lu, H., Yao, T., Nakatsu, C. H. (2017). Microbial ecology along the gastrointestinal tract. Microbes Environ. 32, 300–313. doi: 10.1264/jsme2.ME17017
Hofer, U., Schlaepfer, E., Baenziger, S., Nischang, M., Regenass, S., Schwendener, R., et al. (2010). Inadequate clearance of translocated bacterial products in HIV-infected humanized mice. PLoS Pathog. 6. doi: 10.1371/journal.ppat.1000867
Hou, K., Wu, Z.-X., Chen, X.-Y., Wang, J.-Q., Zhang, D., Xiao, C., et al. (2022). Microbiota in health and diseases. Signal Transduct. Target. Ther. 7, 135. doi: 10.1038/s41392-022-00974-4
Hsiao, E. Y., McBride, S. W., Hsien, S., Sharon, G., Hyde, E. R., McCue, T., et al. (2013). Microbiota modulate behavioral and physiological abnormalities associated with neurodevelopmental disorders. Cell 155, 1451–1463. doi: 10.1016/j.cell.2013.11.024
Huang, W.-C., Liou, C.-J., Shen, S.-C., Hu, S., Chao, J. C., Hsiao, C.-Y., et al. (2022). Urolithin A inactivation of TLR3/TRIF signaling to block the NF-κB/STAT1 axis reduces inflammation and enhances antioxidant defense in poly (I: C)-induced RAW264. 7 cells. Int. J. Mol. Sci. 23, 4697. doi: 10.3390/ijms23094697
Huse, S. M., Ye, Y., Zhou, Y., Fodor, A. A. (2012). A core human microbiome as viewed through 16S rRNA sequence clusters. PloS one 7. doi: 10.1371/journal.pone.0034242
Igartua, C., Davenport, E. R., Gilad, Y., Nicolae, D. L., Pinto, J., Ober, C. (2017). Host genetic variation in mucosal immunity pathways influences the upper airway microbiome. Microbiome 5, 1–17. doi: 10.1186/s40168-016-0227-5
Jackson, M. A., Goodrich, J. K., Maxan, M.-E., Freedberg, D. E., Abrams, J. A., Poole, A. C., et al. (2016). Proton pump inhibitors alter the composition of the gut microbiota. Gut 65, 749–756. doi: 10.1136/gutjnl-2015-310861
Jiang, W., Lederman, M. M., Hunt, P., Sieg, S. F., Haley, K., Rodriguez, B., et al. (2009). Plasma levels of bacterial DNA correlate with immune activation and the magnitude of immune restoration in persons with antiretroviral-treated HIV infection. J. Infect. Dis. 199, 1177–1185. doi: 10.1086/597476
Jiménez, N., Esteban-Torres, M., Mancheño, J. M., de Las Rivas, B., Muñoz, R. (2014). Tannin degradation by a novel tannase enzyme present in some Lactobacillus plantarum strains. Appl. Environ. Microbiol. 80, 2991–2997. doi: 10.1128/AEM.00324-14
Jostins, L., Ripke, S., Weersma, R. K., Duerr, R. H., McGovern, D. P., Hui, K. Y., et al. (2012). Host–microbe interactions have shaped the genetic architecture of inflammatory bowel disease. Nature 491, 119–124. doi: 10.1038/nature11582
Kane, M., Case, L. K., Kopaskie, K., Kozlova, A., MacDearmid, C., Chervonsky, A. V., et al. (2011). Successful transmission of a retrovirus depends on the commensal microbiota. Science 334, 245–249. doi: 10.1126/science.1210718
Katz, L., Baltz, R. H. (2016). Natural product discovery: past, present, and future. J. Ind. Microbiol. Biotechnol. 43, 155–176. doi: 10.1007/s10295-015-1723-5
Kau, A. L., Ahern, P. P., Griffin, N. W., Goodman, A. L., Gordon, J. I. (2011). Human nutrition, the gut microbiome and the immune system. Nature 474, 327–336. doi: 10.1038/nature10213
Kho, Z. Y., Lal, S. K. (2018). The human gut microbiome–a potential controller of wellness and disease. Front. Microbiol. 9, 356589. doi: 10.3389/fmicb.2018.01835
Kim, D.-H. (2018). Gut microbiota-mediated pharmacokinetics of ginseng saponins. J. Ginseng Res. 42, 255–263. doi: 10.1016/j.jgr.2017.04.011
Kim, K.-A., Jung, I.-H., Park, S.-H., Ahn, Y.-T., Huh, C.-S., Kim, D.-H. (2013). Comparative analysis of the gut microbiota in people with different levels of ginsenoside Rb1 degradation to compound K. PloS one 8. doi: 10.1371/journal.pone.0062409
Kim, M., Kim, N., Han, J. (2014). Metabolism of Kaempferia parviflora polymethoxyflavones by human intestinal bacterium Bautia sp. MRG-PMF1. J. Agric. Food Chem. 62, 12377–12383. doi: 10.1021/jf504074n
Kim, J.-S., Lee, H., Nirmala, F. S., Jung, C. H., Kim, M. J., Jang, Y.-J., et al. (2019). Dihydrodaidzein and 6-hydroxydaidzein mediate the fermentation-induced increase of antiosteoporotic effect of soybeans in ovariectomized mice. FASEB J. 33, 3252–3263. doi: 10.1096/fj.201800953R
Knights, D., Silverberg, M. S., Weersma, R. K., Gevers, D., Dijkstra, G., Huang, H., et al. (2014). Complex host genetics influence the microbiome in inflammatory bowel disease. Genome Med. 6, 1–11. doi: 10.1186/s13073-014-0107-1
Koppel, N., Maini Rekdal, V., Balskus, E. P. (2017). Chemical transformation of xenobiotics by the human gut microbiota. Science 356. doi: 10.1126/science.aag2770
Kubinak, J. L., Stephens, W. Z., Soto, R., Petersen, C., Chiaro, T., Gogokhia, L., et al. (2015). MHC variation sculpts individualized microbial communities that control susceptibility to enteric infection. Nat. Commun. 6, 8642. doi: 10.1038/ncomms9642
Kumar, K., Jaiswal, S. K., Dhoke, G. V., Srivastava, G. N., Sharma, A. K., Sharma, V. K. (2018). Mechanistic and structural insight into promiscuity based metabolism of cardiac drug digoxin by gut microbial enzyme. J. Cell. Biochem. 119, 5287–5296. doi: 10.1002/jcb.26638
Kwan, B. C.-H., Chow, K.-M., Leung, C.-B., Law, M.-C., Cheng, P. M.-S., Yu, V., et al. (2013). Circulating bacterial-derived DNA fragments as a marker of systemic inflammation in peritoneal dialysis. Nephrol. Dial. Transplant. 28, 2139–2145. doi: 10.1093/ndt/gft100
Lakhdari, O., Cultrone, A., Tap, J., Gloux, K., Bernard, F., Ehrlich, S. D., et al. (2010). Correction: functional metagenomics: A high throughput screening method to decipher microbiota-driven NF-κB modulation in the human gut. PloS one 5, e13092. doi: 10.1371/annotation/4ea12169-7c97-497c-a45f-52203543065f
Lannes-Costa, P., De Oliveira, J., da Silva Santos, G., Nagao, P. E. (2021). A current review of pathogenicity determinants of Streptococcus sp. J. Appl. Microbiol. 131, 1600–1620. doi: 10.1111/jam.15090
Law, J. W.-F., Law, L. N.-S., Letchumanan, V., Tan, L. T.-H., Wong, S. H., Chan, K.-G., et al. (2020). Anticancer drug discovery from microbial sources: The unique mangrove streptomycetes. Molecules 25, 5365. doi: 10.3390/molecules25225365
Lepage, P., Häsler, R., Spehlmann, M. E., Rehman, A., Zvirbliene, A., Begun, A., et al. (2011). Twin study indicates loss of interaction between microbiota and mucosa of patients with ulcerative colitis. Gastroenterology 141, 227–236. doi: 10.1053/j.gastro.2011.04.011
Leung, R. K.-K., Wu, Y.-K. (2015). Circulating microbial RNA and health. Sci. Rep. 5, 16814. doi: 10.1038/srep16814
Lewis, K., Epstein, S., D’onofrio, A., Ling, L. L. (2010). Uncultured microorganisms as a source of secondary metabolites. J. Antibiot. 63, 468–476. doi: 10.1038/ja.2010.87
Ley, R. E., Hamady, M., Lozupone, C., Turnbaugh, P. J., Ramey, R. R., Bircher, J. S., et al. (2008). Evolution of mammals and their gut microbes. Science 320, 1647–1651. doi: 10.1126/science.1155725
Ley, R. E., Turnbaugh, P. J., Klein, S., Gordon, J. I. (2006). Human gut microbes associated with obesity. Science 444, 1022–1023. doi: 10.1038/4441022a
Li, D., Shi, G., Wang, J., Zhang, D., Pan, Y., Dou, H., et al. (2019). and Therapy, Baicalein ameliorates pristane-induced lupus nephritis via activating Nrf2/HO-1 in myeloid-derived suppressor cells. Arthritis Res. Ther. 21, 1–14. doi: 10.1186/s13075-019-1876-0
Li, B.-C., Zhang, T., Li, Y.-Q., Ding, G.-B. (2019). Target discovery of novel α-L-rhamnosidases from human fecal metagenome and application for biotransformation of natural flavonoid glycosides. Appl. Biochem. Biotechnol. 189, 1245–1261. doi: 10.1007/s12010-019-03063-5
Liang, D., Leung, R. K.-K., Guan, W., Au, W. W. (2018). Involvement of gut microbiome in human health and disease: brief overview, knowledge gaps and research opportunities. Gut Pathog. 10, 1–9. doi: 10.1186/s13099-018-0230-4
Liang, C., Tseng, H.-C., Chen, H.-M., Wang, W.-C., Chiu, C.-M., Chang, J.-Y., et al. (2017). Diversity and enterotype in gut bacterial community of adults in Taiwan. BMC Genomics 18, 1–11. doi: 10.1186/s12864-016-3261-6
Lindell, A. E., Zimmermann-Kogadeeva, M., Patil, K. R. (2022). Multimodal interactions of drugs, natural compounds and pollutants with the gut microbiota. Nat. Rev. Microbiol. 20, 431–443. doi: 10.1038/s41579-022-00681-5
Liu, M.-Y., Li, M., Wang, X.-L., Liu, P., Hao, Q.-H., Yu, X.-M. (2013). Study on human intestinal bacterium Blautia sp. AUH-JLD56 for the conversion of arctigenin to (–)-3′-desmethylarctigenin. J. Agric. Food Chem. 61, 12060–12065. doi: 10.1021/jf403924c
Liu, J., Xu, Y., Yan, M., Yu, Y., Guo, Y. (2022). 18β-Glycyrrhetinic acid suppresses allergic airway inflammation through NF-κB and Nrf2/HO-1 signaling pathways in asthma mice. Sci. Rep. 12, 3121. doi: 10.1038/s41598-022-06455-6
Lloyd-Price, J., Abu-Ali, G., Huttenhower, C. (2016). The healthy human microbiome. Genome Med. 8, 1–11. doi: 10.1186/s13073-016-0307-y
Lu, B., Chen, X., Chen, H., Li, Q., Li, H., Xu, Y., et al. (2022). Demethoxycurcumin mitigates inflammatory responses in lumbar disc herniation via MAPK and NF-κB pathways in vivo and in vitro. Int. Immunopharmacol. 108, 108914. doi: 10.1016/j.intimp.2022.108914
Manor, O., Dai, C. L., Kornilov, S. A., Smith, B., Price, N. D., Lovejoy, J. C., et al. (2020). Health and disease markers correlate with gut microbiome composition across thousands of people. Nat. Commun. 11, 5206. doi: 10.1038/s41467-020-18871-1
Marchesi, J. R., Adams, D. H., Fava, F., Hermes, G. D., Hirschfield, G. M., Hold, G., et al. (2016). The gut microbiota and host health: a new clinical frontier. Gut 65, 330–339. doi: 10.1136/gutjnl-2015-309990
Marchetti, G., Tincati, C., Silvestri, G. (2013). Microbial translocation in the pathogenesis of HIV infection and AIDS. Clin. Microbiol. Rev. 26, 2–18. doi: 10.1128/CMR.00050-12
Marsh, J. W., Kirk, C., Ley, R. E. (2023). Toward Microbiome Engineering: expanding the repertoire of genetically tractable members of the human gut Microbiome. Annu. Rev. Microbiol. 77, 427–449. doi: 10.1146/annurev-micro-032421-112304
Maslowski, K. M., Vieira, A. T., Ng, A., Kranich, J., Sierro, F., Yu, D., et al. (2009). Regulation of inflammatory responses by gut microbiota and chemoattractant receptor GPR43. Nature 461, 1282–1286. doi: 10.1038/nature08530
McIntosh, K., Reed, D. E., Schneider, T., Dang, F., Keshteli, A. H., De Palma, G., et al. (2017). FODMAPs alter symptoms and the metabolome of patients with IBS: a randomised controlled trial. Gut 66, 1241–1251. doi: 10.1136/gutjnl-2015-311339
McKnite, A. M., Perez-Munoz, M. E., Lu, L., Williams, E. G., Brewer, S., Andreux, P. A., et al. (2012). Murine gut microbiota is defined by host genetics and modulates variation of metabolic traits. PloS one 7. doi: 10.1371/journal.pone.0039191
Medema, M. H., Fischbach, M. A. (2015). Computational approaches to natural product discovery. Nat. Chem. Biol. 11, 639–648. doi: 10.1038/nchembio.1884
Medema, M. H., Takano, E., Breitling, R. (2013). Detecting sequence homology at the gene cluster level with MultiGeneBlast. Mol. Biol. Evol. 30, 1218–1223. doi: 10.1093/molbev/mst025
Meng, X., Zhang, G., Cao, H., Yu, D., Fang, X., de Vos, W. M., et al. (2020). Gut dysbacteriosis and intestinal disease: mechanism and treatment. J. Appl. Microbiol. 129, 787–805. doi: 10.1111/jam.14661
Methé, BA, Nelson, KE, Pop, M, Creasy, HH, Giglio, MG, Huttenhower, C, et al (2012). A framework for human microbiome research. Nature 486, 215–221. doi: 10.1038%2Fnature11209
Milshteyn, A., Colosimo, D. A., Brady, S. F. (2018). Accessing bioactive natural products from the human microbiome. Cell Host Microbe 23, 725–736. doi: 10.1016/j.chom.2018.05.013
Miyake, S., Ngugi, D. K., Stingl, U. (2015). Diet strongly influences the gut microbiota of surgeonfishes. Mol. Ecol. 24, 656–672. doi: 10.1111/mec.13050
Moeller, A. H., Degnan, P. H., Pusey, A. E., Wilson, M. L., Hahn, B. H., Ochman, H. (2012). Chimpanzees and humans harbour compositionally similar gut enterotypes. Nat. Commun. 3, 1179. doi: 10.1038/ncomms2159
Morgan, E. W., Perdew, G. H., Patterson, A. D. (2022). Multi-omics strategies for investigating the microbiome in toxicology research. Toxicol. Sci. 187, 189–213. doi: 10.1093/toxsci/kfac029
Mousa, W. K., Athar, B., Merwin, N. J., Magarvey, N. A. (2017). Antibiotics and specialized metabolites from the human microbiota. Nat. Prod. Rep. 34, 1302–1331. doi: 10.1039/C7NP00021A
Mukherjee, A., Lordan, C., Ross, R. P., Cotter, P. D. (2020). Gut microbes from the phylogenetically diverse genus Eubacterium and their various contributions to gut health. Gut Microbes 12, 1802866. doi: 10.1080/19490976.2020.1802866
Mullane, K., Lee, C., Bressler, A., Buitrago, M., Weiss, K., Dabovic, K., et al. (2015). Multicenter, randomized clinical trial to compare the safety and efficacy of LFF571 and vancomycin for Clostridium difficile infections. Antimicrob. Agents Chemother. 59, 1435–1440. doi: 10.1128/AAC.04251-14
Mutlu, E. A., Gillevet, P. M., Rangwala, H., Sikaroodi, M., Naqvi, A., Engen, P. A., et al. (2012). Colonic microbiome is altered in alcoholism. Am. J. Physiol. Gastrointest. Liver Physiol. 302, G966–G978. doi: 10.1152/ajpgi.00380.2011
Mutlu, E., Keshavarzian, A., Engen, P., Forsyth, C. B., Sikaroodi, M., Gillevet, P. (2009). Intestinal dysbiosis: a possible mechanism of alcohol-induced endotoxemia and alcoholic steatohepatitis in rats. Alcohol.: Clin. Exp. Res. 33, 1836–1846. doi: 10.1111/j.1530-0277.2009.01022.x
Nam, Y.-D., Jung, M.-J., Roh, S. W., Kim, M.-S., Bae, J.-W. (2011). Comparative analysis of Korean human gut microbiota by barcoded pyrosequencing. PloS one 6. doi: 10.1371/journal.pone.0022109
Newman, D. J., Cragg, G. M. (2016). Natural products as sources of new drugs from 1981 to 2014. J. Nat. Prod. 79, 629–661. doi: 10.1021/acs.jnatprod.5b01055
O’Dwyer, D. N., Dickson, R. P., Moore, B. B. (2016). The lung microbiome, immunity, and the pathogenesis of chronic lung disease. J. Immun. 196, 4839–4847. doi: 10.4049/jimmunol.1600279
Oehmcke-Hecht, S., Mandl, V., Naatz, L. T., Dühring, L., Köhler, J., Kreikemeyer, B., et al. (2020). Streptococcus gallolyticus abrogates anti-carcinogenic properties of tannic acid on low-passage colorectal carcinomas. Sci. Rep. 10, 4714. doi: 10.1038/s41598-020-61458-5
Pang, C., Zheng, Z., Shi, L., Sheng, Y., Wei, H., Wang, Z., et al. (2016). Caffeic acid prevents acetaminophen-induced liver injury by activating the Keap1-Nrf2 antioxidative defense system. Free Radical Biol. Med. 91, 236–246. doi: 10.1016/j.freeradbiomed.2015.12.024
Peisl, L., Schymanski, E. L., Wilmes, P. (2018). Dark matter in host-microbiome metabolomics: tackling the unknowns–a review. Anal. Chim. Acta 1037, 13–27. doi: 10.1016/j.aca.2017.12.034
Pereira-Caro, G., Fernández-Quirós, B., Ludwig, I. A., Pradas, I., Crozier, A., Moreno-Rojas, J. M. (2018). Catabolism of citrus flavanones by the probiotics Bifidobacterium longum and Lactobacillus rhamnosus. Eur. J. Nutr. 57, 231–242. doi: 10.1007/s00394-016-1312-z
Petrof, E. O., Claud, E. C., Sun, J., Abramova, T., Guo, Y., Waypa, T. S., et al. (2009). Bacteria-free solution derived from Lactobacillus plantarum inhibits multiple NF-kappaB pathways and inhibits proteasome function. Inflamm. Bowel Dis. 15, 1537–1547. doi: 10.1002/ibd.20930
Proal, A. D., Albert, P. J., Marshall, T. G. (2014). Inflammatory disease and the human microbiome. Discov. Med. 22, 257–265.
Qin, J., Li, R., Raes, J., Arumugam, M., Burgdorf, K. S., Manichanh, C., et al. (2010). A human gut microbial gene catalogue established by metagenomic sequencing. Nature 464, 59–65. doi: 10.1038/nature08821
Qin, J., Li, Y., Cai, Z., Li, S., Zhu, J., Zhang, F., et al. (2012). A metagenome-wide association study of gut microbiota in type 2 diabetes. Nature 490, 55–60. doi: 10.1038/nature11450
Queipo-Ortuño, M. I., Boto-Ordóñez, M., Murri, M., Gomez-Zumaquero, J. M., Clemente-Postigo, M., Estruch, R., et al. (2012). Influence of red wine polyphenols and ethanol on the gut microbiota ecology and biochemical biomarkers. Am. J. Clin. Nutr. 95, 1323–1334. doi: 10.3945/ajcn.111.027847
Raimondi, S., Anighoro, A., Quartieri, A., Amaretti, A., Tomás-Barberán, F. A., Rastelli, G., et al. (2015). Role of bifidobacteria in the hydrolysis of chlorogenic acid. Microbiologyopen 4, 41–52. doi: 10.1002/mbo3.219
Reddy, B. V. B., Milshteyn, A., Charlop-Powers, Z., Brady, S. F. (2014). eSNaPD: a versatile, web-based bioinformatics platform for surveying and mining natural product biosynthetic diversity from metagenomes. Chem. Biol. 21, 1023–1033. doi: 10.1016/j.chembiol.2014.06.007
Relman, D. A. (2012). The human microbiome: ecosystem resilience and health. Nutr. Rev. 70, S2–S9. doi: 10.1111/j.1753-4887.2012.00489.x
Reverón, I., de las Rivas, B., Matesanz, R., Muñoz, R., López de Felipe, F. (2015). Molecular adaptation of Lactobacillus plantarum WCFS1 to gallic acid revealed by genome-scale transcriptomic signature and physiological analysis. Microb. Cell Fact. 14, 1–12. doi: 10.1186/s12934-015-0345-y
Reverón, I., Jiménez, N., Curiel, J. A., Peñas, E., Lopez de Felipe, F., de Las Rivas, B., et al. (2017). Differential gene expression by Lactobacillus plantarum WCFS1 in response to phenolic compounds reveals new genes involved in tannin degradation. Appl. Environ. Microbiol. 83. doi: 10.1128/AEM.03387-16
Ridaura, V. K., Faith, J. J., Rey, F. E., Cheng, J., Duncan, A. E., Kau, A. L., et al. (2013). Gut microbiota from twins discordant for obesity modulate metabolism in mice. Science 341, 1241214. doi: 10.1126/science.1241214
Rinninella, E., Raoul, P., Cintoni, M., Franceschi, F., Miggiano, G. A. D., Gasbarrini, A., et al. (2019). What is the healthy gut microbiota composition? A changing ecosystem across age, environment, diet, and diseases. Microorganisms 7, 14. doi: 10.3390/microorganisms7010014
Rocchetti, G., Gregorio, R. P., Lorenzo, J. M., Barba, F. J., Oliveira, P. G., Prieto, M. A., et al. (2022). Safety, Functional implications of bound phenolic compounds and phenolics–food interaction: A review. Compr. Rev. Food Sci. Food Saf. 21, 811–842. doi: 10.1111/1541-4337.12921
Rodríguez-Daza, M. C., Pulido-Mateos, E. C., Lupien-Meilleur, J., Guyonnet, D., Desjardins, Y., Roy, D. (2021). Polyphenol-mediated gut microbiota modulation: Toward prebiotics and further. Front. Nutr. 8, 689456. doi: 10.3389/fnut.2021.689456
Rowland, I., Gibson, G., Heinken, A., Scott, K., Swann, J., Thiele, I., et al. (2018). Gut microbiota functions: metabolism of nutrients and other food components. Eur. J. Nutr. 57, 1–24. doi: 10.1007/s00394-017-1445-8
Santos, E. A. A., Schieber, A., Weber, F. (2018). Site-specific hydrolysis of chlorogenic acids by selected Lactobacillus species. Int. Food Res. 109, 426–432. doi: 10.1016/j.foodres.2018.04.052
Satti, M., Modesto, M., Endo, A., Kawashima, T., Mattarelli, P., Arita, M. (2021). Host-diet effect on the metabolism of bifidobacterium. Genes 12, 609. doi: 10.3390/genes12040609
Schnabl, B., Brenner, D. A. (2014). Interactions between the intestinal microbiome and liver diseases. Gastroenterology 146, 1513–1524. doi: 10.1053/j.gastro.2014.01.020
Schnorr, S. L., Candela, M., Rampelli, S., Centanni, M., Consolandi, C., Basaglia, G., et al. (2014). Gut microbiome of the Hadza hunter-gatherers. Nat. Commun. 5, 3654. doi: 10.1038/ncomms4654
Shoaie, S., Karlsson, F., Mardinoglu, A., Nookaew, I., Bordel, S., Nielsen, J. (2013). Understanding the interactions between bacteria in the human gut through metabolic modeling. Sci. Rep. 3, 2532. doi: 10.1038/srep02532
Sidebottom, A. M., Carlson, E. E. (2015). A reinvigorated era of bacterial secondary metabolite discovery. Curr. Opin. Chem. Biol. 24, 104–111. doi: 10.1016/j.cbpa.2014.10.014
Smith, P. A. (2015). The tantalizing links between gut microbes and the brain. Nature 526, 312–314. doi: 10.1038/526312a
Smith, M. I., Yatsunenko, T., Manary, M. J., Trehan, I., Mkakosya, R., Cheng, J., et al. (2013). Gut microbiomes of Malawian twin pairs discordant for kwashiorkor. Science 339, 548–554. doi: 10.1126/science.1229000
Snijders, A. M., Langley, S. A., Kim, Y.-M., Brislawn, C. J., Noecker, C., Zink, E. M., et al. (2016). Influence of early life exposure, host genetics and diet on the mouse gut microbiome and metabolome. Nat. Microbiol. 2, 1–8. doi: 10.1038/nmicrobiol.2016.221
Spanogiannopoulos, P., Bess, E. N., Carmody, R. N., Turnbaugh, P. J. (2016). The microbial pharmacists within us: a metagenomic view of xenobiotic metabolism. Nat. Rev. Microbiol. 14, 273–287. doi: 10.1038/nrmicro.2016.17
Tai, N., Wong, F. S., Wen, L. (2015). The role of gut microbiota in the development of type 1, type 2 diabetes mellitus and obesity. Rev. Endocr. Metab. Disord. 16, 55–65. doi: 10.1007/s11154-015-9309-0
Tan, S., Rupasinghe, T. W., Tull, D. L., Boughton, B., Oliver, C., McSweeny, C., et al. (2014). Degradation of curcuminoids by in vitro pure culture fermentation. J. Agric. Food Chem. 62, 11005–11015. doi: 10.1021/jf5031168
Tanaka, H., Hashiba, H., Kok, J., Mierau, I. (2000). Bile salt hydrolase of Bifidobacterium longum—biochemical and genetic characterization. Appl. Environ. Microbiol. 66, 2502–2512. doi: 10.1128/AEM.66.6.2502-2512.2000
Tanaka, M., Kishimoto, Y., Sasaki, M., Sato, A., Kamiya, T., Kondo, K., et al. (2018). Terminalia bellirica (Gaertn.) Roxb. extract and gallic acid attenuate LPS-induced inflammation and oxidative stress via MAPK/NF-κB and Akt/AMPK/Nrf2 pathways. Oxid. Med. Cell. Longev. 2018, 9364364. doi: 10.1155/2018/9364364
Tang, W. W., Kitai, T., Hazen, S. L. (2017). Gut microbiota in cardiovascular health and disease. Circ. Res. 120, 1183–1196. doi: 10.1161/CIRCRESAHA.117.309715
Tang, J., Tan, X., Huang, X., Zhang, J., Chen, L., Li, A., et al. (2021). Dual targeting of autophagy and NF-κB pathway by PPARγ contributes to the inhibitory effect of demethoxycurcumin on NLRP3 inflammasome priming. Curr. Mol. Pharmacol. 14, 914–921. doi: 10.2174/1874467214666210301121020
Tremaroli, V., Bäckhed, F. (2012). Functional interactions between the gut microbiota and host metabolism. Nature 489, 242–249. doi: 10.1038/nature11552
Turnbaugh, P. J., Gordon, J. I. (2009). The core gut microbiome, energy balance and obesity. J. Physiol. 587, 4153–4158. doi: 10.1113/jphysiol.2009.174136
Turroni, F., Marchesi, J. R., Foroni, E., Gueimonde, M., Shanahan, F., Margolles, A., et al. (2009). Microbiomic analysis of the bifidobacterial population in the human distal gut. ISME J. 3, 745–751. doi: 10.1038/ismej.2009.19
Turroni, F., Ribbera, A., Foroni, E., van Sinderen, D., Ventura, M. (2008). Human gut microbiota and bifidobacteria: from composition to functionality. Antonie Van Leeuwenhoek 94, 35–50. doi: 10.1007/s10482-008-9232-4
Ursell, L. K., Metcalf, J. L., Parfrey, L. W., Knight, R. (2012). Defining the human microbiome. Nutr. Rev. 70, S38–S44. doi: 10.1111/j.1753-4887.2012.00493.x
Van Baarlen, P., Troost, F. J., van Hemert, S., van der Meer, C., de Vos, W. M., de Groot, P. J., et al. (2009). Differential NF-κB pathways induction by Lactobacillus plantarum in the duodenum of healthy humans correlating with immune tolerance. Proc. Natl. Acad. Sci. 106, 2371–2376. doi: 10.1073/pnas.0809919106
Vinarov, Z., Abrahamsson, B., Artursson, P., Batchelor, H., Berben, P., Bernkop-Schnürch, A., et al. (2021). Current challenges and future perspectives in oral absorption research: An opinion of the UNGAP network. Adv. Drug Deliv. Rev. 171, 289–331. doi: 10.1016/j.addr.2021.02.001
Walker, A. W., Hoyles, L. (2023). Human microbiome myths and misconceptions. Nat. Microbiol. 8, 1392–1396. doi: 10.1038/s41564-023-01426-7
Wang, X.-L., Kim, H.-J., Kang, S.-I., Kim, S.-I., Hur, H.-G. (2007). Production of phytoestrogen S-equol from daidzein in mixed culture of two anaerobic bacteria. Arch. Microbiol. 187, 155–160. doi: 10.1007/s00203-006-0183-8
Wang, J., Linnenbrink, M., Künzel, S., Fernandes, R., Nadeau, M.-J., Rosenstiel, P., et al. (2014). Dietary history contributes to enterotype-like clustering and functional metagenomic content in the intestinal microbiome of wild mice. Proc. Natl. Acad. Sci. 111, E2703–E2710. doi: 10.1073/pnas.1402342111
Wardman, J. F., Bains, R. K., Rahfeld, P., Withers, S. G. (2022). Carbohydrate-active enzymes (CAZymes) in the gut microbiome. Nat. Rev. Microbiol. 20, 542–556. doi: 10.1038/s41579-022-00712-1
Weinstock, G. M. (2012). Genomic approaches to studying the human microbiota. Nature 489, 250–256. doi: 10.1038/nature11553
Wikoff, W. R., Anfora, A. T., Liu, J., Schultz, P. G., Lesley, S. A., Peters, E. C., et al. (2009). Metabolomics analysis reveals large effects of gut microflora on mammalian blood metabolites. Proc. Natl. Acad. Sci. 106, 3698–3703. doi: 10.1073/pnas.0812874106
Wilson, I. D., Nicholson, J. K. (2017). Gut microbiome interactions with drug metabolism, efficacy, and toxicity. Transl. Res. 179, 204–222. doi: 10.1016/j.trsl.2016.08.002
Wilson, M. C., Piel, J. (2013). Metagenomic approaches for exploiting uncultivated bacteria as a resource for novel biosynthetic enzymology. Chem. Biol. 20, 636–647. doi: 10.1016/j.chembiol.2013.04.011
Wilson, M. R., Zha, L., Balskus, E. P. (2017). Natural product discovery from the human microbiome. J. Biol. Chem. 292, 8546–8552. doi: 10.1074/jbc.R116.762906
Wlodarska, M., Luo, C., Kolde, R., d’Hennezel, E., Annand, J. W., Heim, C. E., et al. (2017). Indoleacrylic acid produced by commensal peptostreptococcus species suppresses inflammation. Cell Host Microbe 22, 25–37. e6. doi: 10.1016/j.chom.2017.06.007
Wu, G. D., Chen, J., Hoffmann, C., Bittinger, K., Chen, Y.-Y., Keilbaugh, S. A., et al. (2011). Linking long-term dietary patterns with gut microbial enterotypes. Science 334, 105–108. doi: 10.1126/science.1208344
Wu, G. D., Compher, C., Chen, E. Z., Smith, S. A., Shah, R. D., Bittinger, K., et al. (2016). Comparative metabolomics in vegans and omnivores reveal constraints on diet-dependent gut microbiota metabolite production. Gut. 65, 63–72. doi: 10.1136/gutjnl-2014-308209
Wu, H., Kim, M., Han, J. (2016). Icariin metabolism by human intestinal microflora. Molecules 21, 1158. doi: 10.3390/molecules21091158
Xie, Y., Hu, F., Xiang, D., Lu, H., Li, W., Zhao, A., et al. (2020). The metabolic effect of gut microbiota on drugs. Drug Metab. Rev. 52, 139–156. doi: 10.1080/03602532.2020.1718691
Yan, A. W., Fouts, D. E., Brandl, J., Stärkel, P., Torralba, M., Schott, E., et al. (2011). Enteric dysbiosis associated with a mouse model of alcoholic liver disease. Hepatology 53, 96–105. doi: 10.1002/hep.24018
Ye, M., Yu, J., Shi, X., Zhu, J., Gao, X., Liu, W. (2021). and Nutrition, Polysaccharides catabolism by the human gut bacterium-Bacteroides thetaiotaomicron: Advances and perspectives. Crit. Rev. Food Sci. Nutr. 61, 3569–3588. doi: 10.1080/10408398.2020.1803198
Yu, Q., Liu, Y., Wu, Y., Chen, Y. (2018). Dihydrocurcumin ameliorates the lipid accumulation, oxidative stress and insulin resistance in oleic acid-induced L02 and HepG2 cells. Biomed. Pharmacother. 103, 1327–1336. doi: 10.1016/j.biopha.2018.04.143
Zhang, Q., Lenardo, M. J., Baltimore, D. (2017). 30 years of NF-κB: a blossoming of relevance to human pathobiology. Cell 168, 37–57. doi: 10.1016/j.cell.2016.12.012
Zhang, R., Huang, X.-M., Yan, H.-J., Liu, X.-Y., Zhou, Q., Luo, Z.-Y., et al. (2019). Highly selective production of compound K from ginsenoside Rd by hydrolyzing glucose at C-3 glycoside using β-glucosidase of Bifidobacterium breve ATCC. J. Microbiol. Biotechnol. 15700, 410–418. doi: 10.4014/jmb.1808.08059
Zhao, Y., Zhong, X., Yan, J., Sun, C., Zhao, X., Wang, X. (2022). Potential roles of gut microbes in biotransformation of natural products: An overview. Front. Microbiol. 13, 956378. doi: 10.3389/fmicb.2022.956378
Zhong, F.-L., Ma, R., Jiang, M., Dong, W.-W., Jiang, J., Wu, S., et al. (2016). Cloning and characterization of ginsenoside-hydrolyzing β-glucosidase from Lactobacillus brevis that transforms ginsenosides Rb1 and F2 into ginsenoside Rd and compound K. J. Microbiol. Biotechnol. 26, 1661–1667. doi: 10.4014/jmb.1605.05052
Zoetendal, E. G., Raes, J., Van Den Bogert, B., Arumugam, M., Booijink, C. C., Troost, F. J., et al. (2012). The human small intestinal microbiota is driven by rapid uptake and conversion of simple carbohydrates. ISME J. 6, 1415–1426. doi: 10.1038/ismej.2011.212
Zupancic, M. L., Cantarel, B. L., Liu, Z., Drabek, E. F., Ryan, K. A., Cirimotich, S., et al. (2012). Analysis of the gut microbiota in the old order Amish and its relation to the metabolic syndrome. Plos one. doi: 10.1371/journal.pone.0043052
Keywords: microbiota, gut microbiome, anaerobic bacteria, microbial transformation, natural products, human health
Citation: Qadri H, Shah AH, Almilaibary A and Mir MA (2024) Microbiota, natural products, and human health: exploring interactions for therapeutic insights. Front. Cell. Infect. Microbiol. 14:1371312. doi: 10.3389/fcimb.2024.1371312
Received: 16 January 2024; Accepted: 03 June 2024;
Published: 05 July 2024.
Edited by:
Ajaz Bhat, Sidra Medicine, QatarReviewed by:
Vijay Antharam, Methodist University, United StatesJavaid Ahmad Sheikh, Jamia Hamdard University, India
Copyright © 2024 Qadri, Shah, Almilaibary and Mir. This is an open-access article distributed under the terms of the Creative Commons Attribution License (CC BY). The use, distribution or reproduction in other forums is permitted, provided the original author(s) and the copyright owner(s) are credited and that the original publication in this journal is cited, in accordance with accepted academic practice. No use, distribution or reproduction is permitted which does not comply with these terms.
*Correspondence: Manzoor Ahmad Mir, ZHJtYW56b29yQGthc2htaXJ1bml2ZXJzaXR5LmFjLmlu; Abdul Haseeb Shah, YWJkdWxoYXNlZWJAa2FzaG1pcnVuaXZlcnNpdHkuYWMuaW4=