- 1Division of Musculoskeletal and Dermatological Sciences, School of Biological Sciences, Faculty of Biology, Medicine and Health, University of Manchester, Manchester, United Kingdom
- 2TNO, Holst Centre, Eindhoven, Netherlands
Cutaneous diseases (such as atopic dermatitis, acne, psoriasis, alopecia and chronic wounds) rank as the fourth most prevalent human disease, affecting nearly one-third of the world’s population. Skin diseases contribute to significant non-fatal disability globally, impacting individuals, partners, and society at large. Recent evidence suggests that specific microbes colonising our skin and its appendages are often overrepresented in disease. Therefore, manipulating interactions of the microbiome in a non-invasive and safe way presents an attractive approach for management of skin and hair follicle conditions. Due to its proven anti-microbial and anti-inflammatory effects, blue light (380 – 495nm) has received considerable attention as a possible ‘magic bullet’ for management of skin dysbiosis. As humans, we have evolved under the influence of sun exposure, which comprise a significant portion of blue light. A growing body of evidence indicates that our resident skin microbiome possesses the ability to detect and respond to blue light through expression of chromophores. This can modulate physiological responses, ranging from cytotoxicity to proliferation. In this review we first present evidence of the diverse blue light-sensitive chromophores expressed by members of the skin microbiome. Subsequently, we discuss how blue light may impact the dialog between the host and its skin microbiome in prevalent skin and hair follicle conditions. Finally, we examine the constraints of this non-invasive treatment strategy and outline prospective avenues for further research. Collectively, these findings present a comprehensive body of evidence regarding the potential utility of blue light as a restorative tool for managing prevalent skin conditions. Furthermore, they underscore the critical unmet need for a whole systems approach to comprehend the ramifications of blue light on both host and microbial behaviour.
Introduction
The integumentary system is home to a diverse and delicately balanced ecosystem, termed the microbiome. It comprises a dynamic community of fungi, bacteria, viruses, archaea and mites, the abundance of which varies considerably across sebaceous (e.g. forehead), moist (e.g. toe webs) and dry (e.g. volar forearm) sites. Collectively, the microbiome participates in a symbiotic dialog with its host, modulating host innate immune responses, maintaining immune homeostasis, protecting against oxidative stress and invading pathogens and, metabolising host products, as well as promoting barrier repair and recovery following insult (Byrd et al., 2018). Tipping this balance can result in dysbiosis, a phenotype often characterised by reduced microbial diversity or overrepresentation of certain species. This dysbiosis is increasingly prevalent in integumentary system conditions, especially in acne vulgaris, atopic dermatitis, seborrheic dermatitis, alopecia areata, psoriasis and non-healing chronic wounds (Kong et al., 2012; Constantinou et al., 2021; Carmona-Cruz et al., 2022; Lawrence et al., 2022). The changes to the microbiome observed range from an elevated abundance of at least Staphylococcus aureus in atopic dermatitis to an overrepresentation of specific Cutibacterium acnes phylotypes in acne vulgaris (Kong et al., 2012; Lee et al., 2019; Versey et al., 2021).
UV light has anti-microbial action (Marasini et al., 2021), but its carcinogenicity must be considered. Recently, described as a potential ‘magic bullet’ for the 21st century, blue light (400 – 470nm) has proven to be a particularly enticing non-invasive and drug free modality for the management of dysbiosis (Leanse et al., 2022). These antimicrobial effects are often attributed to the expression of porphyrins - heterocyclic compounds with a Soret band at 405nm, by microbes (Serrage et al., 2019). Photo-excitation of porphyrins results in the production of reactive oxygen species (ROS). Optimal dosimetry results in lethal ROS accumulation above a threshold for Cutibacterium acnes, a contributor to acne vulgaris (Spittaels et al., 2021).
These findings led to an upsurge of literature suggesting that microbial species respond uniformly to 405nm light, irrespective of the presence or indeed absence of porphyrins. However, such an assumption ignores a myriad of prospective chromophores expressed across the skin and hair follicle microbiome (both in health and disease) that all may exhibit wavelength- and perhaps dose dependent responses to blue light. Indeed, members of the skin microbiome expressing blue light absorbing chromophores are common. Mapping of prospective chromophore’s onto genomes of the top 10 most abundant bacteria of the skin microbiome showcases flavins, porphyrins and carotenoid pigments capable of detecting and responding to the blue component of the visible spectrum (Table 1). Expression of such pigments and proteins has been observed from members of the healthy skin microbiome and in species overrepresented in skin conditions including the carotenoid pigment staphyloxanthin (460nm) in Staphylococcus aureus (Dong et al., 2019) and the phenazine pyocyanin (380nm) from Pseudomonas aeruginosa ( (El-Fouly et al., 2015) Figure 1). Wavelength-dependent excitation of these chromophores can lead to a plurality of versatile responses (Harris, 2023) ranging from phototoxicity, to elevated motility, growth and even invasion of eukaryotic cells (Mussi et al., 2010; Halstead et al., 2019).
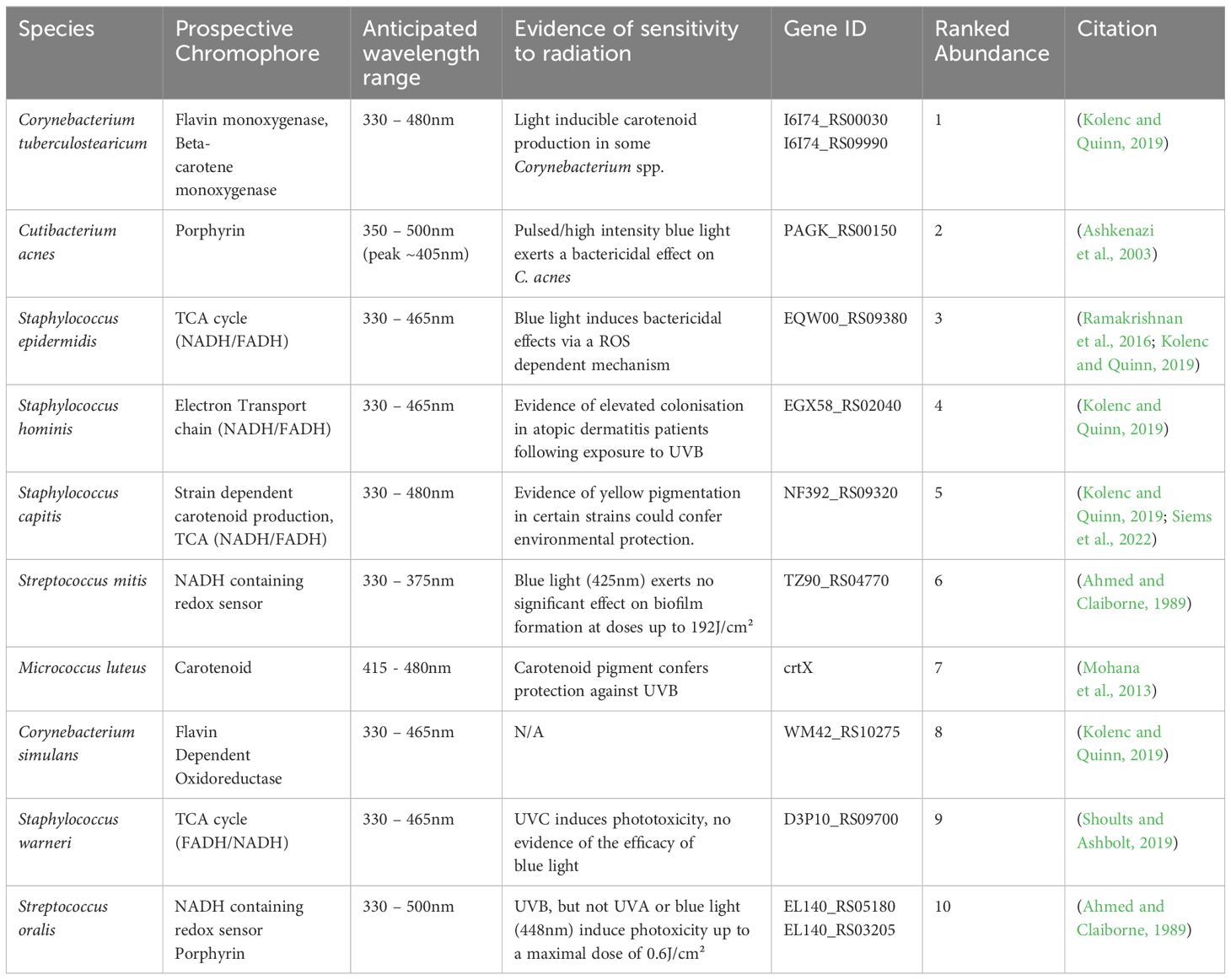
Table 1 Mapping of prospective chromophore’s onto genomes of the top 10 most abundant bacterial members of our skins diverse microbiome.
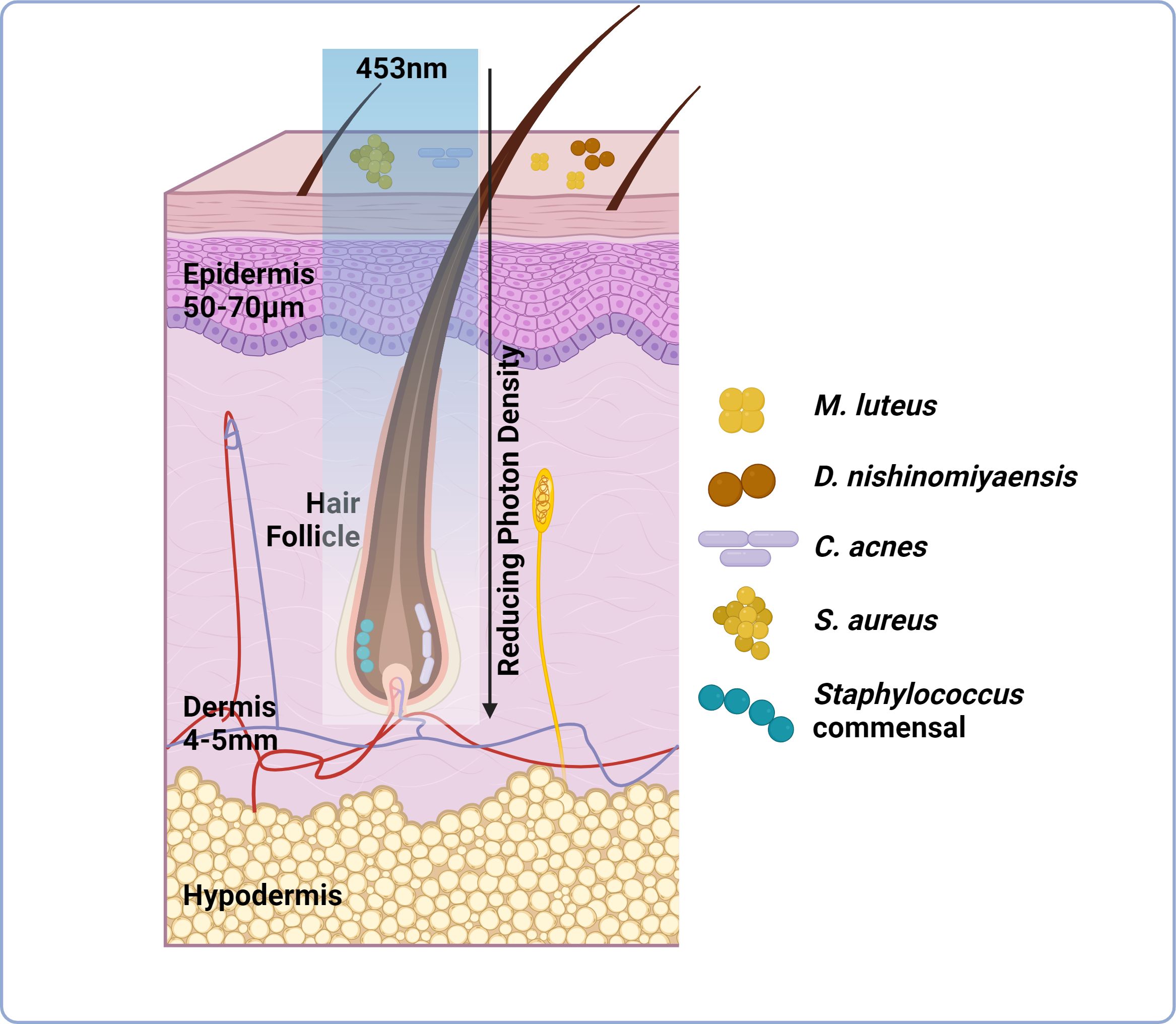
Figure 1 Light transmission through skin is attenuated but can be manipulated through increased irradiance and exposure times. Skin can be subdivided into three layers; epidermis, dermis and hypodermis. Skin microbiome composition is variable these layers Certain species preferentially colonise the skin surface including M. luteus and S. aureus, whilst others prefer anaerobic environments (hair follicle, dermis) including P. acnes. This niche specific colonisation potentially dictates the capacity of light to manipulate skin microbiome composition as well as host responses. Light delivery at 453nm is attenuated through skin by a factor of 2.5-10x at a depth of 0.5-1mm from the skin surface. Light delivery can be improved through increased irradiance and exposure times to a maximum safe exposure of up to 250mW/cm². However, delivery still reduces as the light penetrates through the multilayered structure of skin (as indicated by the reducing photon density observed when passing 453nm light through tissue), therefore diminishing the possibility of manipulating microbial behaviour/viability (image created using BioRender.com).
Having at hand a ‘magic bullet’ with anti-bacterial action may seem a very efficacious solution. However, as human skin and hair follicle microbiota is a universe of many different species, one needs to restore the ecosystem balance instead of eradicating ‘‘all life’’. This approach brings a challenge of identifying molecular targets for light therapy, suitable wavelength, and treatment regimes.
In this review we first present evidence of the diverse blue light-sensitive chromophores expressed by members of the skin microbiome. Subsequently, we discuss how blue light may impact the dialog between the host and its skin microbiome in prevalent skin and hair follicle conditions. Finally, we examine the constraints of this non-invasive treatment strategy and outline prospective avenues for further research.
Microbial chromophores
Historically, light sensing systems were thought to be exclusive to photosynthetic organisms However, a growing body of evidence points to a plethora of pigments as well as flavin and porphyrin containing sensing systems in non- photosynthetic bacteria. Here, we summarise these characterised systems and potential implications for future investigations using blue light.
Flavins
Flavins, including flavin mononucleotide (FMN) and flavin adenine dinucleotide (FAD) can be excited by blue light (Losi and Gärtner, 2011). Herein, we detail two bacterial flavin-containing systems.
LOV and blue light-dependent transcription regulation
In 2007 Swartz et al. made the discovery that Brucella abortis, a gram-negative bacterium implicated in cutaneous infections in humans (Karaali et al., 2011), expressed a blue light activated, two component system; the light, oxygen or voltage (LOV) histidine kinase. LOV senses blue light via a non-covalently bound flavin co-factor, its photoactivation results in elevated B. abortis proliferation and stimulation of macrophage invasion (Kennis and Crosson, 2007; Swartz et al., 2007).
Curiously, LOV domains are encoded in 16% of sequenced bacterial genomes and appear ubiquitous across the Pseudomonas genus, with the wound associated pathogen Pseudomonas aeruginosa possessing a “short” LOV domain (Glantz et al., 2016). Indeed, blue light was reported effective in photo disinfection of P. aeruginosa associated biofilm (Kahl et al., 2022). However, uncertainties persist regarding suitable irradiation parameters.
BLUF
Blue-light sensing using flavin (BLUF) domains were first observed ~20 years ago in the single celled algae Euglena gracilis and facultative purple photosynthetic bacterium Rhodobacter sphaeroides (Park and Tame, 2017). Like the LOV domain, BLUF isn’t exclusive to photosynthetic/phototrophic organisms and it has been observed within the small blue-light-sensing protein A (BlsA). BlsA is expressed by the nosocomially acquired pathogen Acinetobacter baumannii (Mussi et al., 2010; Brust et al., 2014). This species has proven an increasing problem in hospitals due to its growing recalcitrance to antibiotic treatment, capacity to form biofilm and cause soft-tissue infections (Dijkshoorn et al., 2007).
Upon excitation at 470 nm, BLUF undergoes a conformational change resulting in the binding of flavin adenine dinucleotide (FAD) between two α-helices (Tokonami et al., 2022). In the strain where the BLUF domain was originally observed, A. baumannii ATCC 17978, this small protein can drastically impact biofilm formation, virulence and even motility. BLUF domains are observed across a diverse array of microbial species including Escherichia coli which is frequently isolated from skin and soft tissue infections (Petkovsek et al., 2009). E. coli possesses a BLUF-EAL protein (YcgF) which binds the MerR-like repressor (YcgE) upon illumination, resulting in dissociation from its operator DNA and regulation of two component systems that diminish biofilm formation and adhesion to surfaces (Tschowri et al., 2009). Details of BLUF diversity, structure and function are discussed in detail elsewhere (Kaushik et al., 2019).
Porphyrins
Porphyrins are a highly abundant group of macromolecules, implicated in the biosynthesis of heme and chlorophyll (Bonkovsky et al., 2013). This group of heterocyclic organic compounds act as pigments, exhibiting a Soret band at 400 - 420nm (Gouterman, 1978; Koren et al., 2011). Excitation of this Soret band results in ROS accumulation and ultimately cell lysis (Wu et al., 2018). Biosynthesis of porphyrins includes generation of δ-aminolevulinic acid from glutamyl-tRNA (Jones et al., 2020), ultimately resulting in downstream accumulation of protoporphyrin IX, uroporphyrin III and coproporphyrin III (Shu et al., 2013).
The abundant skin commensal Cutibacterium acnes synthesises a range of porphyrins (Johnson et al., 2016). This has been exploited for utilizing blue light for management of the C. acnes in acne vulgaris (Sorbellini et al., 2018). However, success of this treatment depends upon a host of factors including the propensity of C. acnes strains to produce porphyrins. The latter highly varies and depends upon the presence of the porphyrin biosynthesis repressor gene; deoR (Spittaels et al., 2021). This may explain subsets of acne patients proving recalcitrant to blue light treatment (Diogo et al., 2021). However, other factors including abnormalities in follicular keratinisation and sebum overproduction contribute to onset (Cruz et al., 2023). Therefore, failing to address other underlying causes may also contribute to the persistence of this prevalent condition.
Intriguingly, the expression of porphyrins occurs across a host of wound-associated pathogens. Jones et al. surveyed the porphyrin producing and autofluorescence of 32 bacterial members of the wound microbiome and found 88% exhibited porphyrin expression and excitation with blue light (Jones et al., 2020). This suggests that violet-blue light could be a promising approach for infection management.
Pigments
Pigments are expressed by a diverse range of both environmental and skin-resident microbes. Expression of such pigments is hypothesised to confer potential selective advantages including both photo- and thermal- stability (Celedón and Díaz, 2021; Agarwal et al., 2023). However, one still needs to comprehend how we might exploit these traits to manage microbial adherence and biofilm formation in both health and disease scenarios (Narsing Rao et al., 2017).
Yellow pigmented photoreceptors
Bacteria displaying yellow pigmentation are common across the skin microbiome. Commensals including Micrococcus luteus (Anwar and Prebble, 1977), Kocuria rizophila (Govindan et al., 2020), Dermacoccus nishinomiyaensis (Klein et al., 2017) and Staphylococcus aureus (Zhang et al., 2018) exhibit a golden yellow colour which is derived from a carotenoid pigment, a molecule with a profound antioxidant function. Its absorption displays peaks across 415 – 485nm, indicating photosensitivity to blue light (Darvin et al., 2022). Similarly, group B streptococci (Streptococcus agalactiae, gastrointestinal tract commensal often associated with skin and soft tissue infections including diabetic foot ulcers (Raabe and Shane, 2019),) express granadaene, a yellow/orange pigment displaying an absorption spectra from 435-485nm (Rosa-Fraile et al., 2014).
Phenazines
Phenazines form part of a group of nitrogen containing heterocyclic compounds (Pierson and Pierson, 2010). They shuttle electrons, ultimately modifying cellular redox status thereby regulating gene expression, biofilm formation and even architecture (Wang et al., 2011; Jo et al., 2020). The colour of this metabolite varies from yellow to blue, depending upon pH or redox state with absorption band ~385 – 500nm across the UVA-green range (Ryazanova et al., 2007).
One well-characterised derivative of a phenazine is pyocyanin, a blue metabolite expressed by the pathogen Pseudomonas aeruginosa, proven to facilitate aggregation via extracellular DNA (Das et al., 2013), promote biofilm formation and maintain bacterial fitness (Jayaseelan et al., 2014). The absorption spectrum of pyocyanin is dependent upon pH and redox state, with a maximum occurring in the UVA range (~380nm) (El-Fouly et al., 2015). However, purified pyocyanin can potentiate the bactericidal properties of 405nm wavelength against methicillin resistant S. aureus, indicating its capacity to elicit a response to blue light (Leanse et al., 2022).
Blue light and cutaneous conditions
Dysbiosis in skin and hair follicle conditions is common, demanding effective strategies to address microbiome composition imbalances. Here, we highlight how blue light may manipulate microbial behaviour in highly prevalent skin and hair conditions, where there remains a deficit in treatments that effectively restore skin health. Moreover, we explore how light exposure may exert dual effects through photoexcitation of host derived chromophores.
Acne
Acne is a highly prevalent dermatosis affecting up to 95% of 11-30 year olds, characterised by an elevated abundance of Cutibacterium spp residing upon affected sites (Golchai et al., 2010). Blue light therapy is a well-established drug-free strategy for management of acne vulgaris (Gold, 2007). The efficacy of blue light treatment is thought to centre on photoexcitation of porphyrin containing Cutibacterium spp, resulting in bacterial lysis and resolution of acne vulgaris. Indeed, the bactericidal effects of blue light on Cutibacterium acnes have been widely reported (Masson-Meyers et al., 2020; Nakayama et al., 2023), and porphyrin containing phylotypes appear more abundant in acne vulgaris (Huang et al., 2023).
Advances in a metagenomic sequencing have revealed that next to C. acnes, as the key player in acne pathology, other species may play an active role in promoting disease pathogenesis. The abundance of Staphylococcus spp. increases with severity of acne (Dreno et al., 2017). In particular, S. epidermidis has been cited as a new player in acne pathogenesis. Staphylococcus epidermidis is abundant in acne lesions and utilises glycerol as a shared carbon source, resulting in production of short chain fatty acids (SCFA) (Wang et al., 2014). These SCFAs facilitate competition between S. epidermidis and C. acnes, indicating a delicate balance between these two species could be important in the resolution of inflammation associated with acne vulgaris. S. epidermidis possesses a NADH:flavinoxidoreductase, which may be excited by blue light (Wu et al., 2015) and Ramarkrishnan et al. indicated exposure to 405nm light resulted in cell cytotoxicity via a ROS dependent mechanism (Ramakrishnan et al., 2016). However, studies also indicate S. epidermidis possesses mechanisms by which it can deactivate ROS (Balasubramaniam et al., 2020; Smith et al., 2023). Understanding of how these protective responses may defend against blue light exposure and modulate imbalances in microbiota composition remains to be elucidated.
Psoriasis
Psoriasis is a long-term, chronic immune-mediated skin disease with no cure. It is linked to comorbidities like psoriatic arthritis, cardiometabolic issues, and mental health conditions with a prevalence of 3% in adults in the USA (Armstrong et al., 2021). In the 1990s, researchers discovered psoriatic plaques in 45% of patients emitted red fluorescence when excited with blue (442nm) light (Bissonnette et al., 1998). Autofluorescence was attributed to protoporphyrin IX, elevated in this subset of patients (Bissonnette et al., 1998). Auto-fluorescence positive lesions have proven to correlate with psoriasis severity and identified as a novel approach for psoriasis diagnosis (Wang et al., 2017). It was hypothesised that, like with acne vulgaris, protoporphyrin IX may be of microbial origin, indicating inter-individual differences in skin microbiome may predispose towards porphyrin accumulation. Presently, literature citing psoriasis community composition remains variable (Mazur et al., 2021) and no discernible link between microbiome composition and protoporphyrin accumulation was identified. Yet, a growing body of literature supports the use of blue light for treating psoriasis, where efficacy varies from improved disease severity (Lesiak et al., 2021) to no significant effect, specifically in a subset of patients with elevated endogenous protoporphyrin’s (Maari and Bissonnette, 2002). Favourable patient outcomes were attributed to antiproliferative and pro-differentiative effect of blue light on keratinocytes shown in vitro and to nitric oxide-mediated mechanism shown in vivo, overall resulting in resolution of plaques (Sadowska et al., 2021).
Perturbations in the microbiome associated with psoriasis also include enrichment in members of the Pseudomonas genus, particularly sensitive to blue light exposure (Chen et al., 2020). Future studies shall characterise the cross-talk between the microbiome and skin in this multi-faceted and highly prevalent condition.
Atopic dermatitis
Atopic dermatitis (AD) is one of the most common chronic, relapsing inflammatory skin disorders affecting up to 3% of adults worldwide (Kapur et al., 2018). It is characterised by an itch-flare cycle that can lead to lichenification (hyperpigmentation, skin thickening and exaggerated skin lines) AD pathogenesis is characterized by impaired epidermal barrier function and immune dysregulation some of which may be driven by S. aureus, which is over-abundant in the skin of patients with AD (Weidinger et al., 2018).. Current treatments both topical (tacrolimus, corticosteroids and antibiotics) and systemic (cyclosporine) act to supress immune responses and diminish S. aureus abundance (Belloni Fortina and Neri, 2015; Avena-Woods, 2017). However, these first line treatments are often ineffective and there remain cohorts of patients recalcitrant to mainstay treatments for AD (Lewis and Feldman, 2018).
A growing body of literature has endeavoured to evaluate the efficacy of visible – near infra-red (NIR) light in the management of AD. Blue light has garnered considerable attention due to its capacity to manipulate dendritic cell activation, resulting in modulation of cell proliferation and inflammatory responses in vitro (Liebmann et al., 2010; Fischer et al., 2013). In vivo studies demonstrated that full body irradiation with blue light (400 - 500 nm) resulted in improved pruritus (urge to itch), quality of life and reduced hydrocortisone use (Uzunbajakava et al., 2023).
It is yet to be understood how blue light manipulates the AD microbiota. Staphylococcus aureus is enriched in AD flares (lesional skin) (Kong et al., 2012) and is capable of manipulating dendritic cell activation and pruritus via induction of IL-31, ultimately promoting skin barrier dysfunction (Wu and Xu, 2014; Blicharz et al., 2019). Staphylococcus aureus exhibits a golden yellow colour due to the expression of carotenoid pigment; staphyloxanthin, which displays characteristic absorption peaks at 408nm and 486nm (Harris, 2023). The expression of this pigment promotes bacterial survival, where in environments with high levels of oxidative stress staphyloxanthin acts as an antioxidant, detoxifying free radicals facilitating enhanced survival both in vitro (Clauditz et al., 2006) and in murine models for chronic wounds (Campbell et al., 2023), an environment associated with high levels of oxidative stress (Cano Sanchez et al., 2018).
This potential carotenoid-dependent tolerance to radiation is not exclusive to S. aureus. For example, skin commensals M. luteus, Kocuria spp. and D. nishinomiyaensis exhibit carotenoid pigment expression (Koshti et al., 2022). M. luteus, a skin commensal with probiotic properties, has proven highly tolerant to UV exposure due to carotenoid expression but responses to blue light are yet to be evaluated (Anwar and Prebble, 1977). Future steps will be required to understand how blue light manipulates pigment producing bacteria and in turn, how we might exploit these characteristics for management of prevalent conditions including AD.
Alopecia areata
Alopecia areata (AA) is an autoimmune disease with a lifetime incidence of 2.1% characterised by the collapse of immune privilege in the hair follicle which causes hair loss and has drastic impacts on patient quality of life, often causing psychological distress (Pratt et al., 2017).
Since early discovery of positive effect of red light on hair regrowth (Hamblin, 2016), strides have been taken to understand whether red light could prove a strategy for management of alopecia areata as well as to unravel the fundamental mechanisms behind photobiomodulation of the hair cycle. Blue light has been reported to prolong anagen hair growth phase via sustained proliferation in the hair follicle matrix (Buscone et al., 2017). The effect has been attributed to interaction of blue light with putative photoreceptors, cryptochromes and opsins (Buscone et al., 2017; Buscone et al., 2021). However, so far to the best or our knowledge no research has been conducted to unravel a potential blue-light-dependent hair growth modulation via modulation of the HF microbiome (Lodi et al., 2021).
The collapse of immune privilege is considered a pathogenic event in AA and patients exhibit an elevated abundance of Cutibacterium spp. and Mallasezia spp, and reduced abundance of Staphylococcus spp (Huang et al., 2019; Sánchez-Pellicer et al., 2022). Increasingly, researchers are posing the question of whether the scalp microbiome can influence hair regrowth. The antibiotic roxithromycin elevates hair growth, but is also toxic to C. acnes (Sadowska et al., 2021). The fungicidal and bactericidal effects of blue light on both Cutibacterium and Mallasezia spp. have been reported (Wi et al., 2012; Nakayama et al., 2023). Therefore, understanding of how blue light manipulates microbiome communities and markers for AA pathogenesis warrants investigation.
Chronic wounds
Prevalence of chronic nonhealing wounds represent an immense and ever-growing global pandemic, with incidence and mortality rates exceeding those of many common cancers (Weigelt et al., 2022). Recently, researchers started exploiting light to understand wound microbiome composition. Excitation using a violet-blue light (~405nm) resulted in detection of auto-fluorescence in the orange, red and green spectral regions, denoting the presence of S. aureus, A. baumannii and P. aeruginosa, respectively (DaCosta et al., 2015; Jung et al., 2022; Rahma et al., 2022). This auto-fluorescence has been observed in >50% of patients, and is increasingly utilised to detect infection. Violet-blue light can be absorbed by several microbiome species and could be potentially applied for photo-disinfection (Bayat et al., 2022).
Studies assessing blue light mediated photodisinfection addressed readily isolated wound pathogens; P. aeruginosa and S. aureus. Mechanisms of blue light dependent manipulation of P. aeruginosa viability and biofilm formation have been assessed in single species biofilm models, reporting blue light-dependent manipulation of membrane potentials in P. aeruginosa, resulting in altered biofilm formation (Kahl et al., 2022). Blue light induction of membrane depolarisation/potential has proven both dose and biofilm formation stage dependent: P. aeruginosa exhibits heightened sensitivity to blue light in the adherence (1h) and proliferation (3h) stages of biofilm formation. Cells in the proliferative stage are particularly sensitive and doses <20mJ/cm² have been exploited to disperse biofilm (Blee et al., 2020). This poses the question; could blue light be applied as a preventative measure following injury to prevent colonisation by P. aeruginosa alongside treatment of chronic wounds?
Clinical studies on blue light efficacy in non-healing wounds are scars, with only one study using blue light to accelerate wound healing (Fraccalvieri et al., 2022). Therefore, future studies should endeavour to evaluate promising bactericidal and wound healing properties of blue light (Bayat et al., 2022).
The future of blue light in skin health: potential therapeutic insights and challenges
Exposure of skin to blue light has become a contentious topic, sparking discussions over its benefits and risks, implications in disease management, circadian clock, pigmentation but also photodamage (Campbell et al., 2017; Stern et al., 2018). Blue light could be a promising therapy for a diverse range of cutaneous and systemic conditions, from blood pressure reduction to management of seasonal affective disorder (Meesters et al., 2016; Stern et al., 2018).
However, reports of adverse effects have also proven abundant, even from exposure to environmental levels of blue part of electromagnetic spectrum (Diogo et al., 2021; Kumari et al., 2023). These potentially undesired effects span erythema (sunburn), photoaging, decreased skin hydration, elevated melanin production and subsequent hyperpigmentation (Mahmoud et al., 2010; Duteil et al., 2014; Duteil et al., 2020; Kumari et al., 2023). However, the latter has also been hypothesized to carry a photoprotective effect against subsequent UV irradiation by some groups (Uzunbajakava et al., 2023). The exposure to blue light has only increased as technological advances have resulted in our constant exposure to blue light from screens and mobile devices. The actual irradiance and dose emitted by these appliances is far lower than those found in natural lighting and hence the impact of such appliances on the skin is minimal, if present at all (Arjmandi et al., 2018; Duteil et al., 2020; Kumari et al., 2023; Uzunbajakava et al., 2023). Nevertheless, exposure to such devices, peaking around ~440nm via visual pathways, has been shown to manipulate circadian rhythm through excitation of melanopsin located within retinal ganglion cells (Wahl et al., 2019). Despite increased understanding of the dose dependent effects of blue light on skin, there remains limited literature exploring how blue light might manipulate the healthy skin microbiome. In one study, Willmott et al. evidenced sunlight exposure dependent shifts in microbiome composition in holidaymakers. It is hypothesised blue light could play an important role in mediating these shifts, due to the application of sunscreens by holiday makers that may mitigate prospective effects of other forms of radiation (UVA/UVB (Willmott et al., 2023)).
Notably, as humans, we have evolved under the sun, and under the influence of the blue spectral band, where the skin is directly exposed to photons of light. The intensity of the blue band varies considerably depending upon the time of day (Bird and Riordan, 1984). It’s highest intensity relative to other spectral components occurs at noon, but reduces significantly at dusk. Hence it is not surprising that blue light entrains a circadian rhythm that is controlled via central control mechanism (CNS) but perhaps also locally via our cutaneous system of clock genes (Lyons et al., 2019). These circadian rhythms are intrinsically linked to skin diseases. For example, symptoms of psoriasis exhibit a diurnal pattern with a more severe itch in the evening for 70% of patients (Duan et al., 2021). Circadian oscillations also affect mast cells (Baumann et al., 2013), participating in various immune and inflammatory reactions, including allergies, infections, wound healing and skin cancer. In line with that, patients with mastocytosis present higher plasma histamine levels in the morning (Friedman et al., 1989) and patients with chronic urticaria often have symptoms in the evening (Maurer et al., 2009).
In vivo studies have revealed deletion of Clock genes in mouse models for atopic dermatitis result in more severe delayed-type allergic reactions and elevated ‘nocturnal pruritus’ in patients (Lavery et al., 2016; Duan et al., 2021). These diurnal cycles are certainty not restricted to the skin cells only, as the skin together with its microbiome evolved under the sunlight. So, it is not surprising that the relative abundance of members of the skin microbiome vary with the time of day. For example, evidence suggests that the abundance of acne associated species C. acnes increases in the evening (Wilkins et al., 2021). This highlights a key question surrounding blue light therapy: could efficacy depend upon the time of exposure?
Perhaps the most promising trait of blue light is its potential to address dysbiosis in skin and hair follicle conditions, while exerting little or no impact on bacterial resistance following repeated exposures (Haridas and Atreya, 2022). The rising prevalence of skin diseases urgently demands a comprehensive understanding of the dialog between the host and microbiome in response to blue light to develop adequate therapies for relevant conditions. Future studies are needed as current focus remains upon nosocomial acquired pathogenic species only, with little regard for the contribution of commensals to light dependent responses. For example, abundant members of the commensal microbiome express carotenoid pigments, that exhibit antioxidant properties (Mohana et al., 2013). It could be hypothesised that strains may evolve to elevate expression of carotenoid synthesis genes as a protective mechanism against blue light exposure. Another aspect to consider is virulence, where reports cite blue light enhanced virulence of S. aureus and A. baumannii (Tuttobene et al., 2021).
To achieve favourable patient outcomes, effective blue light delivery systems will be required. Blue light is strongly attenuated by the skin haemoglobin and melanin in comparison to wavelengths within the red and near-infrared spectra. Still blue light can reach relatively deep targets in the skin, including the hair follicle bulge at a depth of approximately 0.5-1mm relative to the skin surface, while its intensity will be highly attenuated versus the photon density administered at the skin surface (Figure 2) (Uzunbajakava et al., 2023). While blue light may be most effective in manipulating surface level microbes, its efficacy in influencing sub-epidermal layers including sebaceous gland and entire hair follicles will be modulated by light attenuation by the skin. Methods to boost photon concentration at deeper-located targets include usage of a larger spot size, pulsing along with increasing the applied dose within the safety limits, and perhaps usage of light guides along the hair follicle shafts for light delivery to a deeper targets (Ash et al., 2017). Therefore, critically the relationship between the location of the target, penetration depth, dose, irradiance and pulsing in relation with efficacy and potential adverse effects must be considered when designing blue light devices.
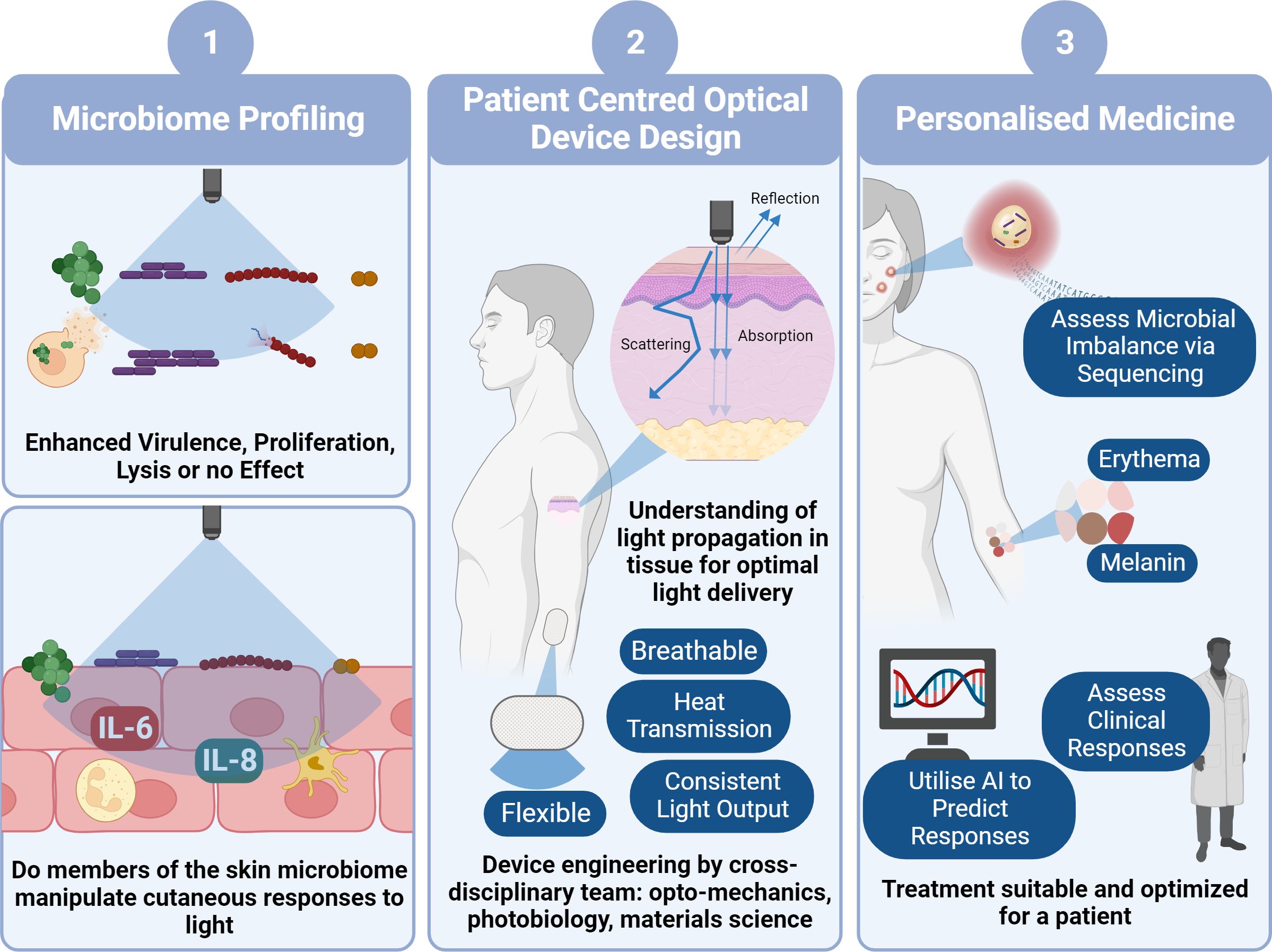
Figure 2 Pathway to Clinical Implementation of Blue Light for Management of Skin Conditions. To determine the viability of blue light as a potential treatment option for skin conditions, firstly we need to decipher how blue light impacts microbial behaviour and in turn how theses microbes manipulate host responses to blue light utilising laboratory characterisation techniques (1). This will involve development of increasingly physiologically relevant models with the eventual use of ex vivo models and microbes derived directly from individuals. In parallel with this we also must ensure device design (2) is central to clinical application through thorough characterisation of light transmission through skin and patient centred design of photonics devices. This will involve a combination of photonics experts, biologists and engineers to reliably characterise a device convenient for everyday use. Ultimately, design of a device and application of irradiation of parameters will also rely upon personalised approach to medicine (3). For example, determining if there is a microbial imbalance in tissue and in turn anticipating whether the microbiome will elicit a favourable response to blue light, without elevating virulence and promoting host cell tissue apoptosis. We all must consider the dose dependent response where some individuals may experience a ‘sunburn’ response to blue light, whilst others may experience hyperpigmentation through elevated melanin expression. Such responses may be predicted through clinical consultation or perhaps development of AI platforms that automatically predict responses. Collectively this pathway will facilitate prediction of responses and suitability for clinical applications (image created using BioRender.com).
It is apparent that an interdisciplinary and whole systems approach is required for the effective translation of blue light to clinics (Figure 3). However, alongside understanding individual microbial responses to blue light, development of co-culture models to assess the impact of microbes on host-responses and an understanding of light transmission through tissue, the patient must be at the centre of light delivery. Device design must be carefully considered, and recent technological advances have facilitated the development of flexible, breathable, and convenient devices capable of effective light delivery (Matsuhisa et al., 2021). Such designs should be incorporated into a personalised approach to blue light exposure. In which a combination of microbiome composition, skin condition severity and survey of pre-existing conditions (e.g., cancer) should be taken into consideration prior to exposure.
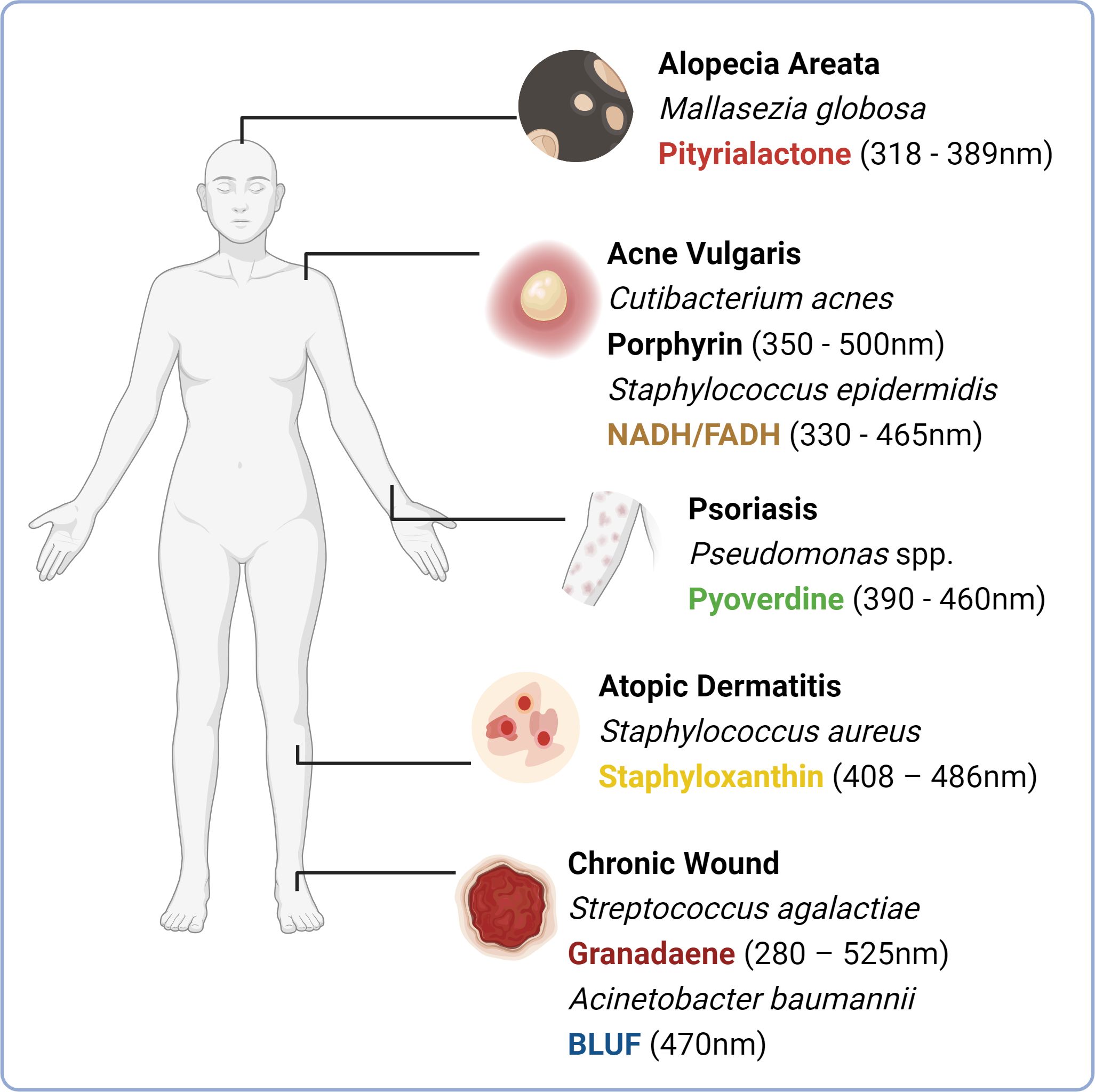
Figure 3 Chromophores expressed by microbial species implicated in skin and hair conditions. An imbalance in skin microbiome composition is often observed in skin and hair follicle conditions including alopecia areata (elevated abundance of Mallasezia globosa), acne vulgaris (increased abundance of porphyrin producing phylotypes of Cutibacterum acnes), psoriasis (possible association with the elevated abundance of Pseudomonas aeruginosa) and atopic dermatitis (increased abundance of Staphylococcus aureus in atopic dermatitis flares). Soft tissue infections that can ultimately develop into chronic wounds are often colonised by environmentally acquired isolates including Streptococcus agalactiae and Acinetobacter baumannii, which express blue light absorbing chromophores granadaene and blue light sensing using flavin (BLUF) respectively. This figure depicts these species commonly overrepresented, alongside their prospective chromophores. (Image created with Biorender.com).
Conclusion
Blue light represents an attractive non-invasive, drug-free approach for management of cutaneous conditions where there is an imbalance in microbial community composition. However, we have only scratched the surface in understanding the relative abundance of blue light sensing systems across the skin microbiome, and in turn how this impacts microbial viability, motility and virulence. It is apparent that steps must be taken in this nascent field to understand how presence or absence of these systems manipulates microbial community composition and in turn how photosensitive chromophores expressed by the host contribute to these responses.
Data availability statement
The raw data supporting the conclusions of this article will be made available by the authors, without undue reservation.
Author contributions
HS: Conceptualization, Investigation, Methodology, Project administration, Writing – original draft, Writing – review & editing. CO’N: Writing – review & editing. NU: Writing – review & editing.
Funding
The author(s) declare financial support was received for the research, authorship, and/or publication of this article. Thanks to the University of Manchester and Skin BioTherapeutics PLC (R126061) for funding this project.
Acknowledgments
The authors would like to thank the School of Biological Sciences, University of Manchester.
Conflict of interest
The authors declare that the research was conducted in the absence of any commercial or financial relationships that could be construed as a potential conflict of interest.
The author(s) declared that they were an editorial board member of Frontiers, at the time of submission. This had no impact on the peer review process and the final decision.
Publisher’s note
All claims expressed in this article are solely those of the authors and do not necessarily represent those of their affiliated organizations, or those of the publisher, the editors and the reviewers. Any product that may be evaluated in this article, or claim that may be made by its manufacturer, is not guaranteed or endorsed by the publisher.
References
Agarwal, H., Bajpai, S., Mishra, A., Kohli, I., Varma, A., Fouillaud, M., et al. (2023). Bacterial pigments and their multifaceted roles in contemporary biotechnology and pharmacological applications. Microorganisms 11. doi: 10.3390/microorganisms11030614
Ahmed, S. A., Claiborne, A. (1989). The streptococcal flavoprotein NADH oxidase. II. Interactions of pyridine nucleotides with reduced and oxidized enzyme forms. J. Biol. Chem. 264, 19863–19870. doi: 10.1016/S0021-9258(19)47190-1
Anwar, M., Prebble, J. (1977). The photoinactivation of the respiratory chain in sarcina luteus (Micrococcus luteus) and protection by endogenous carotenoid. Photochem. Photobiol. 26, 475–481. doi: 10.1111/j.1751-1097.1977.tb07517.x
Arjmandi, N., Mortazavi, G., Zarei, S., Faraz, M., Mortazavi, S. A. R. (2018). Can light emitted from smartphone screens and taking selfies cause premature aging and wrinkles? J. BioMed. Phys. Eng. 8, 447–452.
Armstrong, A. W., Mehta, M. D., Schupp, C. W., Gondo, G. C., Bell, S. J., Griffiths, C. E. M. (2021). Psoriasis prevalence in adults in the United States. JAMA Dermatol. 157, 940–946. doi: 10.1001/jamadermatol.2021.2007
Ash, C., Dubec, M., Donne, K., Bashford, T. (2017). Effect of wavelength and beam width on penetration in light-tissue interaction using computational methods. Lasers Med. Sci. 32, 1909–1918. doi: 10.1007/s10103-017-2317-4
Ashkenazi, H., Malik, Z., Harth, Y., Nitzan, Y. (2003). Eradication of Propionibacterium acnes by its endogenic porphyrins after illumination with high intensity blue light. FEMS Immunol. Med. Microbiol. 35, 17–24. doi: 10.1111/fim.2003.35.issue-1
Balasubramaniam, A., Adi, P., Do Thi, T. M., Yang, J. H., Labibah, A. S., Huang, C. M. (2020). Skin bacteria mediate glycerol fermentation to produce electricity and resist UV-B. Microorganisms 8, 1092. doi: 10.3390/microorganisms8071092
Baumann, A., Gönnenwein, S., Bischoff, S. C., Sherman, H., Chapnik, N., Froy, O., et al. (2013). The circadian clock is functional in eosinophils and mast cells. Immunology 140, 465–474. doi: 10.1111/imm.12157
Bayat, M., Albright, R., Hamblin, M. R., Chien, S. (2022). Impact of blue light therapy on wound healing in preclinical and clinical subjects: A systematic review. J. Lasers Med. Sci. 13, e69. doi: 10.34172/jlms.2022.69
Belloni Fortina, A., Neri, L. (2015). Antibiotic therapy in the management of atopic dermatitis. G Ital Dermatol. Venereol. 150, 321–325.
Bird, R. E., Riordan, C. (1984). Simple solar spectral model for direct and diffuse irradiance on horizontal and tilted planes at the earth's surface for cloudless atmospheres. J. Appl. Meteorol. Climatol. 25, 87–97. doi: 10.2172/5986936
Bissonnette, R., Zeng, H., McLean, D. I., Schreiber, W. E., Roscoe, D. L., Lui, H. (1998). Psoriatic plaques exhibit red autofluorescence that is due to protoporphyrin IX. J. Invest. Dermatol. 111, 586–591. doi: 10.1046/j.1523-1747.1998.00345.x
Blee, J. A., Roberts, I. S., Waigh, T. A. (2020). Membrane potentials, oxidative stress and the dispersal response of bacterial biofilms to 405 nm light. Phys. Biol. 17, 036001. doi: 10.1088/1478-3975/ab759a
Blicharz, L., Rudnicka, L., Samochocki, Z. (2019). Staphylococcus aureus: an underestimated factor in the pathogenesis of atopic dermatitis? Postepy Dermatol. Alergol 36, 11–17. doi: 10.5114/ada.2019.82821
Bonkovsky, H. L., Guo, J. T., Hou, W., Li, T., Narang, T., Thapar, M. (2013). Porphyrin and heme metabolism and the porphyrias. Compr. Physiol. 3, 365–401. doi: 10.1002/cphy.c120006
Brust, R., Haigney, A., Lukacs, A., Gil, A., Hossain, S., Addison, K., et al. (2014). Ultrafast structural dynamics of blsA, a photoreceptor from the pathogenic bacterium acinetobacter baumannii. J. Phys. Chem. Letters 5, 220–224. doi: 10.1021/jz4023738
Buscone, S., Mardaryev, A. N., Raafs, B., Bikker, J. W., Sticht, C., Gretz, N., et al. (2017). A new path in defining light parameters for hair growth: Discovery and modulation of photoreceptors in human hair follicle. Lasers Surg. Med. 49, 705–718. doi: 10.1002/lsm.22673
Buscone, S., Mardaryev, A. N., Westgate, G. E., Uzunbajakava, N. E., Botchkareva, N. V. (2021). Cryptochrome 1 is modulated by blue light in human keratinocytes and exerts positive impact on human hair growth. Exp. Dermatol. 30, 271–277. doi: 10.1111/exd.14231
Byrd, A. L., Belkaid, Y., Segre, J. A. (2018). The human skin microbiome. Nat. Rev. Microbiol. 16, 143–155. doi: 10.1038/nrmicro.2017.157
Campbell, A. E., McCready-Vangi, A. R., Uberoi, A., Murga-Garrido, S. M., Lovins, V. M., White, E. K., et al. (2023). Variable staphyloxanthin production by Staphylococcus aureus drives strain-dependent effects on diabetic wound-healing outcomes. Cell Rep. 42, 113281. doi: 10.1016/j.celrep.2023.113281
Campbell, P. D., Miller, A. M., Woesner, M. E. (2017). Bright light therapy: seasonal affective disorder and beyond. Einstein J. Biol. Med. 32, E13–e25. doi: 10.1016/j.celrep.2023.113281
Cano Sanchez, M., Lancel, S., Boulanger, E., Neviere, R. (2018). Targeting oxidative stress and mitochondrial dysfunction in the treatment of impaired wound healing: A systematic review. Antioxidants (Basel) 7, 98. doi: 10.3390/antiox7080098
Carmona-Cruz, S., Orozco-Covarrubias, L., Sáez-de-Ocariz, M. (2022). The human skin microbiome in selected cutaneous diseases. Front. Cell. Infection Microbiol. 12. doi: 10.3389/fcimb.2022.834135
Celedón, R. S., Díaz, L. B. (2021). Natural pigments of bacterial origin and their possible biomedical applications. Microorganisms 9, 739. doi: 10.3390/microorganisms9040739
Chen, L., Li, J., Zhu, W., Kuang, Y., Liu, T., Zhang, W., et al. (2020). Skin and gut microbiome in psoriasis: gaining insight into the pathophysiology of it and finding novel therapeutic strategies. Front. Microbiol. 11. doi: 10.3389/fmicb.2020.589726
Clauditz, A., Resch, A., Wieland, K. P., Peschel, A., Götz, F. (2006). Staphyloxanthin plays a role in the fitness of Staphylococcus aureus and its ability to cope with oxidative stress. Infect. Immun. 74, 4950–4953. doi: 10.1128/IAI.00204-06
Constantinou, A., Kanti, V., Polak-Witka, K., Blume-Peytavi, U., Spyrou, G. M., Vogt, A. (2021). The potential relevance of the microbiome to hair physiology and regeneration: the emerging role of metagenomics. Biomedicines 9, 236. doi: 10.3390/biomedicines9030236
Cruz, S., Vecerek, N., Elbuluk, N. (2023). Targeting inflammation in acne: current treatments and future prospects. Am. J. Clin. Dermatol. 24, 681–694. doi: 10.1007/s40257-023-00789-1
DaCosta, R. S., Kulbatski, I., Lindvere-Teene, L., Starr, D., Blackmore, K., Silver, J. I., et al. (2015). Point-of-care autofluorescence imaging for real-time sampling and treatment guidance of bioburden in chronic wounds: first-in-human results. PloS One 10, e0116623. doi: 10.1371/journal.pone.0116623
Darvin, M. E., Lademann, J., von Hagen, J., Lohan, S. B., Kolmar, H., Meinke, M. C., et al. (2022). Carotenoids in human skin in vivo: antioxidant and photo-protectant role against external and internal stressors. Antioxidants (Basel) 11, 1451. doi: 10.3390/antiox11081451
Das, T., Kutty, S. K., Kumar, N., Manefield, M. (2013). Pyocyanin facilitates extracellular DNA binding to pseudomonas aeruginosa influencing cell surface properties and aggregation. PloS One 8, e58299. doi: 10.1371/journal.pone.0058299
Dijkshoorn, L., Nemec, A., Seifert, H. (2007). An increasing threat in hospitals: multidrug-resistant Acinetobacter baumannii. Nat. Rev. Microbiol. 5, 939–951. doi: 10.1038/nrmicro1789
Diogo, M. L. G., Campos, T. M., Fonseca, E. S. R., Pavani, C., Horliana, A., Fernandes, K. P. S., et al. (2021). Effect of blue light on acne vulgaris: A systematic review. Sensors (Basel) 21, 6943. doi: 10.3390/s21206943
Dong, P. T., Mohammad, H., Hui, J., Leanse, L. G., Li, J., Liang, L., et al. (2019). Photolysis of staphyloxanthin in methicillin-resistant staphylococcus aureus potentiates killing by reactive oxygen species. Adv. Sci. (Weinh) 6, 1900030. doi: 10.1002/advs.201900030
Dreno, B., Martin, R., Moyal, D., Henley, J. B., Khammari, A., Seité, S. (2017). Skin microbiome and acne vulgaris: Staphylococcus, a new actor in acne. Exp. Dermatol. 26, 798–803. doi: 10.1111/exd.13296
Duan, J., Greenberg, E. N., Karri, S. S., Andersen, B. (2021). The circadian clock and diseases of the skin. FEBS Letters 595, 2413–2436. doi: 10.1002/1873-3468.14192
Duteil, L., Cardot-Leccia, N., Queille-Roussel, C., Maubert, Y., Harmelin, Y., Boukari, F., et al. (2014). Differences in visible light-induced pigmentation according to wavelengths: a clinical and histological study in comparison with UVB exposure. Pigment Cell Melanoma Res. 27, 822–826. doi: 10.1111/pcmr.12273
Duteil, L., Queille-Roussel, C., Lacour, J. P., Montaudié, H., Passeron, T. (2020). Short-term exposure to blue light emitted by electronic devices does not worsen melasma. J. Am. Acad. Dermatol. 83, 913–914. doi: 10.1016/j.jaad.2019.12.047
El-Fouly, M. Z., Sharaf, A. M., Shahin, A. A. M., El-Bialy, H. A., Omara, A. M. A. (2015). Biosynthesis of pyocyanin pigment by Pseudomonas aeruginosa. J. Radiat. Res. Appl. Sci. 8, 36–48. doi: 10.1016/j.jrras.2014.10.007
Fischer, M. R., Abel, M., Lopez Kostka, S., Rudolph, B., Becker, D., von Stebut, E. (2013). Blue light irradiation suppresses dendritic cells activation in vitro. Exp. Dermatol. 22, 558–560. doi: 10.1111/exd.12193
Fraccalvieri, M., Amadeo, G., Bortolotti, P., Ciliberti, M., Garrubba, A., Mosti, G., et al. (2022). Effectiveness of Blue light photobiomodulation therapy in the treatment of chronic wounds. Results of the Blue Light for Ulcer Reduction (B.L.U.R.) Study. Ital J. Dermatol. Venerol. 157, 187–194. doi: 10.23736/S2784-8671.21.07067-5
Friedman, B. S., Steinberg, S. C., Meggs, W. J., Kaliner, M. A., Frieri, M., Metcalfe, D. D. (1989). Analysis of plasma histamine levels in patients with mast cell disorders. Am. J. Med. 87, 649–654. doi: 10.1016/S0002-9343(89)80398-5
Glantz, S. T., Carpenter, E. J., Melkonian, M., Gardner, K. H., Boyden, E. S., Wong, G. K., et al. (2016). Functional and topological diversity of LOV domain photoreceptors. Proc. Natl. Acad. Sci. U S A. 113, E1442–E1451. doi: 10.1073/pnas.1509428113
Golchai, J., Khani, S. H., Heidarzadeh, A., Eshkevari, S. S., Alizade, N., Eftekhari, H. (2010). Comparison of anxiety and depression in patients with acne vulgaris and healthy individuals. Indian J. Dermatol. 55, 352–354. doi: 10.4103/0019-5154.74539
Gold, M. H. (2007). Acne and PDT: new techniques with lasers and light sources. Lasers Med. Sci. 22, 67–72. doi: 10.1007/s10103-006-0420-z
Gouterman, M. (1978). “1 - Optical Spectra and Electronic Structure of Porphyrins and Related Rings,” in The Porphyrins. Ed. Dolphin, D. (Cambridge, Massachusetts: Academic Press), 1–165. doi: 10.1016/B978-0-12-220103-5.50008-8
Govindan, J. A., Elamparithi, J., Victor, L., Jack, S., Gary, R. (2020). Bacterial carotenoids suppress Caenorhabditis elegans surveillance and defence of translational dysfunction. bioRxiv. doi: 10.1101/2020.01.08.898668
Halstead, F. D., Ahmed, Z., Bishop, J. R. B., Oppenheim, B. A. (2019). The potential of visible blue light (405 nm) as a novel decontamination strategy for carbapenemase-producing enterobacteriaceae (CPE). Antimicrobial Resistance Infection Control. 8, 14. doi: 10.1186/s13756-019-0470-1
Hamblin, M. R. (2016). Photobiomodulation or low-level laser therapy. J. Biophotonics 9, 1122–1124. doi: 10.1002/jbio.201670113
Haridas, D., Atreya, C. D. (2022). The microbicidal potential of visible blue light in clinical medicine and public health. Front. Med. 9. doi: 10.3389/fmed.2022.905606
Harris, D. M. (2023). Spectra of pathogens predict lethality of blue light photo-inactivation. Laser Ther. 30. doi: 10.4081/ltj.2023.314
Huang, J., Ran, Y., Pradhan, S., Yan, W., Dai, Y. (2019). Investigation on microecology of hair root fungi in androgenetic alopecia patients. Mycopathologia 184, 505–515. doi: 10.1007/s11046-019-00345-8
Huang, C., Zhuo, F., Han, B., Li, W., Jiang, B., Zhang, K., et al. (2023). The updates and implications of cutaneous microbiota in acne. Cell Biosci. 13, 113. doi: 10.1186/s13578-023-01072-w
Jayaseelan, S., Ramaswamy, D., Dharmaraj, S. (2014). Pyocyanin: production, applications, challenges and new insights. World J. Microbiol. Biotechnol. 30, 1159–1168. doi: 10.1007/s11274-013-1552-5
Jo, J., Price-Whelan, A., Cornell, W. C., Dietrich, L. E. P. (2020). Interdependency of respiratory metabolism and phenazine-associated physiology in pseudomonas aeruginosa PA14. J. Bacteriol. 202, 10. doi: 10.1128/JB.00700-19
Johnson, T., Kang, D., Barnard, E., Li, H. (2016). Strain-level differences in porphyrin production and regulation in propionibacterium acnes elucidate disease associations. mSphere 1, e00023–15. doi: 10.1128/mSphere.00023-15
Jones, L. M., Dunham, D., Rennie, M. Y., Kirman, J., Lopez, A. J., Keim, K. C., et al. (2020). In vitro detection of porphyrin-producing wound bacteria with real-time fluorescence imaging. Future Microbiol. 15, 319–332. doi: 10.2217/fmb-2019-0279
Jung, E.-H., Yang, J.-H., Mun, S.-J., Han, S.-Y. (2022). Detection of nosocomial pneumonia pathogens using a fluorescence-based device. Photodiagnosis Photodyn. Ther. 37, 102621. doi: 10.1016/j.pdpdt.2021.102621
Kahl, L. J., Eckartt, K. N., Morales, D. K., Price-Whelan, A., Dietrich, L. E. P. (2022). Light/dark and temperature cycling modulate metabolic electron flow in pseudomonas aeruginosa biofilms. mBio 13, e0140722. doi: 10.1128/mbio.01407-22
Kapur, S., Watson, W., Carr, S. (2018). Atopic dermatitis. Allergy Asthma Clin. Immunol. 14, 52. doi: 10.1186/s13223-018-0281-6
Karaali, Z., Baysal, B., Poturoglu, S., Kendir, M. (2011). Cutaneous manifestations in brucellosis. Indian J. Dermatol. 56, 339–340. doi: 10.4103/0019-5154.82505
Kaushik, M. S., Sharma, R., Veetil, S. K., Srivastava, S. K., Kateriya, S. (2019). Modular diversity of the BLUF proteins and their potential for the development of diverse optogenetic tools. Appl. Sci. [Internet] 9, 3924. doi: 10.3390/app9183924
Kennis, J. T., Crosson, S. (2007). Microbiology. A bacterial pathogen sees the light. Science 317, 1041–1042. doi: 10.1126/science.1147609
Klein, B. A., Lemon, K. P., Gajare, P., Jospin, G., Eisen, J. A., Coil, D. A. (2017). Draft genome sequences of dermacoccus nishinomiyaensis strains UCD-KPL2534 and UCD-KPL2528 isolated from an indoor track facility. Genome Announc. 5, e01652–16. doi: 10.1128/genomeA.01652-16
Kolenc, O. I., Quinn, K. P. (2019). Evaluating cell metabolism through autofluorescence imaging of NAD(P)H and FAD. Antioxid Redox Signal. 30, 875–889. doi: 10.1089/ars.2017.7451
Kong, H. H., Oh, J., Deming, C., Conlan, S., Grice, E. A., Beatson, M. A., et al. (2012). Temporal shifts in the skin microbiome associated with disease flares and treatment in children with atopic dermatitis. Genome Res. 22, 850–859. doi: 10.1101/gr.131029.111
Koren, K., Borisov, S. M., Saf, R., Klimant, I. (2011). Strongly phosphorescent iridium(III)-porphyrins - new oxygen indicators with tuneable photophysical properties and functionalities. Eur. J. Inorg Chem. 2011, 1531–1534. doi: 10.1002/ejic.201100089
Koshti, R., Jagtap, A., Noronha, D., Patkar, S., Nazareth, J., Paulose, R., et al. (2022). Evaluation of antioxidant potential and UV protective properties of four bacterial pigments. Microbiol. Biotechnol. Letters 50, 375–386. doi: 10.48022/mbl.2205.05010
Kumari, J., Das, K., Babaei, M., Rokni, G. R., Goldust, M. (2023). The impact of blue light and digital screens on the skin. J. Cosmetic Dermatol. 22, 1185–1190. doi: 10.1111/jocd.15576
Lavery, M. J., Stull, C., Kinney, M. O., Yosipovitch, G. (2016). Nocturnal pruritus: the battle for a peaceful night's sleep. Int. J. Mol. Sci. 17, 425. doi: 10.3390/ijms17030425
Lawrence, K., Myrissa, K., Toribio-Mateas, M., Minini, L., Gregory, A. M. (2022). Trialling a microbiome-targeted dietary intervention in children with ADHD-the rationale and a non-randomised feasibility study. Pilot Feasibility Stud. 8, 108. doi: 10.1186/s40814-022-01058-4
Leanse, L. G., dos Anjos, C., Mushtaq, S., Dai, T. (2022). Antimicrobial blue light: A ‘Magic Bullet’ for the 21st century and beyond? Advanced Drug Delivery Rev. 180, 114057. doi: 10.1016/j.addr.2021.114057
Lee, Y. B., Byun, E. J., Kim, H. S. (2019). Potential role of the microbiome in acne: A comprehensive review. J. Clin. Med. 8, 987. doi: 10.3390/jcm8070987
Lesiak, A., Bednarski, I. A., Narbutt, J. (2021). Prospective 3-month study on the efficacy of UV-free blue light in mild psoriasis vulgaris treatment. Postepy Dermatologii i Alergologii. 38, 446–449. doi: 10.5114/ada.2021.107931
Lewis, D. J., Feldman, S. R. (2018). Rapid, successful treatment of atopic dermatitis recalcitrant to topical corticosteroids. Pediatr. Dermatol. 35, 278–279. doi: 10.1111/pde.13376
Liebmann, J., Born, M., Kolb-Bachofen, V. (2010). Blue-light irradiation regulates proliferation and differentiation in human skin cells. J. Invest. Dermatol. 130, 259–269. doi: 10.1038/jid.2009.194
Lodi, G., Sannino, M., Cannarozzo, G., Giudice, A., Del Duca, E., Tamburi, F., et al. (2021). Blue light-emitting diodes in hair regrowth: the first prospective study. Lasers Med. Sci. 36, 1719–1723. doi: 10.1007/s10103-021-03327-9
Losi, A., Gärtner, W. (2011). Old chromophores, new photoactivation paradigms, trendy applications: flavins in blue light-sensing photoreceptors. Photochem. Photobiol. 87, 491–510. doi: 10.1111/j.1751-1097.2011.00913.x
Lyons, A. B., Moy, L., Moy, R., Tung, R. (2019). Circadian rhythm and the skin: A review of the literature. J. Clin. Aesthet Dermatol. 12, 42–45.
Maari, C., Bissonnette, R. (2002). 002Multiple exposures to blue light does not improve psoriasis in patients with elevated endogenous levels of protoporphyrin IX. Photodermatol. Photoimmunol. Photomed. 18, 104. doi: 10.1034/j.1600-0781.2002.180208_2.x
Mahmoud, B. H., Ruvolo, E., Hexsel, C. L., Liu, Y., Owen, M. R., Kollias, N., et al. (2010). Impact of long-wavelength UVA and visible light on melanocompetent skin. J. Invest. Dermatol. 130, 2092–2097. doi: 10.1038/jid.2010.95
Marasini, S., Zhang, A. C., Dean, S. J., Swift, S., Craig, J. P. (2021). Safety and efficacy of UV application for superficial infections in humans: A systematic review and meta-analysis. Ocular Surface. 21, 331–344. doi: 10.1016/j.jtos.2021.03.002
Masson-Meyers, D. S., Bumah, V. V., Castel, C., Castel, D., Enwemeka, C. S. (2020). Pulsed 450 nm blue light significantly inactivates Propionibacterium acnes more than continuous wave blue light. J. Photochem. Photobiol. B: Biol. 202, 111719. doi: 10.1016/j.jphotobiol.2019.111702
Matsuhisa, N., Niu, S., O'Neill, S. J. K., Kang, J., Ochiai, Y., Katsumata, T., et al. (2021). High-frequency and intrinsically stretchable polymer diodes. Nature 600, 246–252. doi: 10.1038/s41586-021-04053-6
Maurer, M., Ortonne, J. P., Zuberbier, T. (2009). Chronic urticaria: an internet survey of health behaviours, symptom patterns and treatment needs in European adult patients. Br. J. Dermatol. 160, 633–641. doi: 10.1111/bjd.2009.160.issue-3
Mazur, M., Tomczak, H., Lodyga, M., Czajkowski, R., Żaba, R., Adamski, Z. (2021). The microbiome of the human skin and its variability in psoriasis and atopic dermatitis. Postepy Dermatol. Alergol. 38, 205–209. doi: 10.5114/ada.2021.106197
Meesters, Y., Winthorst, W. H., Duijzer, W. B., Hommes, V. (2016). The effects of low-intensity narrow-band blue-light treatment compared to bright white-light treatment in sub-syndromal seasonal affective disorder. BMC Psychiatry 16, 27. doi: 10.1186/s12888-016-0729-5
Mohana, D., Thippeswamy, S., Abhishek, R. (2013). Antioxidant, antibacterial, and ultraviolet-protective properties of carotenoids isolated from Micrococcus spp. Radiat. Prot. Environment 36, 168–174. doi: 10.4103/0972-0464.142394
Mussi, M. A., Gaddy, J. A., Cabruja, M., Arivett, B. A., Viale, A. M., Rasia, R., et al. (2010). The opportunistic human pathogen Acinetobacter baumannii senses and responds to light. J. Bacteriol. 192, 6336–6345. doi: 10.1128/JB.00917-10
Nakayama, E., Kushibiki, T., Mayumi, Y., Fushuku, S., Nakamura, T., Kiyosawa, T., et al. (2023). Optimal blue light irradiation conditions for the treatment of acne vulgaris in a mouse model. J. Photochem. Photobiol. B: Biol. 239, 112651. doi: 10.1016/j.jphotobiol.2023.112651
Narsing Rao, M. P., Xiao, M., Li, W. J. (2017). Fungal and bacterial pigments: secondary metabolites with wide applications. Front. Microbiol. 8, 1113. doi: 10.3389/fmicb.2017.01113
Park, S. Y., Tame, J. R. H. (2017). Seeing the light with BLUF proteins. Biophys. Rev. 9, 169–176. doi: 10.1007/s12551-017-0258-6
Petkovsek, Z., Elersic, K., Gubina, M., Zgur-Bertok, D., Starcic Erjavec, M. (2009). Virulence potential of Escherichia coli isolates from skin and soft tissue infections. J. Clin. Microbiol. 47, 1811–1817. doi: 10.1128/JCM.01421-08
Pierson, L. S., 3rd, Pierson, E. A. (2010). Metabolism and function of phenazines in bacteria: impacts on the behaviour of bacteria in the environment and biotechnological processes. Appl. Microbiol. Biotechnol. 86, 1659–1670. doi: 10.1007/s00253-010-2509-3
Pratt, C. H., King, L. E., Jr., Messenger, A. G., Christiano, A. M., Sundberg, J. P. (2017). Alopecia areata. Nat. Rev. Dis. Primers 3, 17011. doi: 10.1038/nrdp.2017.11
Raabe, V. N., Shane, A. L. (2019). Group B streptococcus (Streptococcus agalactiae). Microbiol. Spectr. 7, 10.1128. doi: 10.1128/microbiolspec.GPP3-0007-2018
Rahma, S., Woods, J., Brown, S., Nixon, J., Russell, D. (2022). The use of point-of-care bacterial autofluorescence imaging in the management of diabetic foot ulcers: A pilot randomized controlled trial. Diabetes Care 45, 1601–1609. doi: 10.2337/dc21-2218
Ramakrishnan, P., Maclean, M., MacGregor, S. J., Anderson, J. G., Grant, M. H. (2016). Cytotoxic responses to 405nm light exposure in mammalian and bacterial cells: Involvement of reactive oxygen species. Toxicol. Vitro 33, 54–62. doi: 10.1016/j.tiv.2016.02.011
Rosa-Fraile, M., Dramsi, S., Spellerberg, B. (2014). Group B streptococcal haemolysin and pigment, a tale of twins. FEMS Microbiol. Rev. 38, 932–946. doi: 10.1111/1574-6976.12071
Ryazanova, O. A., Voloshin, I. M., Makitruk, V. L., Zozulya, V. N., Karachevtsev, V. A. (2007). pH-Induced changes in electronic absorption and fluorescence spectra of phenazine derivatives. Spectrochimica Acta Part A: Mol. Biomolecular Spectrosc. 66, 849–859. doi: 10.1016/j.saa.2006.04.027
Sadowska, M., Narbutt, J., Lesiak, A. (2021). Blue light in dermatology. Life (Basel) 11, 670. doi: 10.3390/life11070670
Sánchez-Pellicer, P., Navarro-Moratalla, L., Núñez-Delegido, E., Agüera-Santos, J., Navarro-López, V. (2022). How our microbiome influences the pathogenesis of alopecia areata. Genes (Basel) 13, 1860. doi: 10.3390/genes13101860
Serrage, H., Heiskanen, V., Palin, W. M., Cooper, P. R., Milward, M. R., Hadis, M., et al. (2019). Under the spotlight: mechanisms of photobiomodulation concentrating on blue and green light. Photochem. Photobiol. Sci. 18, 1877–1909. doi: 10.1039/c9pp00089e
Shoults, D. C., Ashbolt, N. J. (2019). Decreased efficacy of UV inactivation of Staphylococcus aureus after multiple exposure and growth cycles. Int. J. Hygiene Environ. Health 222, 111–116. doi: 10.1016/j.ijheh.2018.08.007
Shu, M., Kuo, S., Wang, Y., Jiang, Y., Liu, Y. T., Gallo, R. L., et al. (2013). Porphyrin metabolisms in human skin commensal Propionibacterium acnes bacteria: potential application to monitor human radiation risk. Curr. Med. Chem. 20, 562–568. doi: 10.2174/0929867311320040007
Siems, K., Runzheimer, K., Rehm, A., Schwengers, O., Heidler von Heilborn, D., Kaser, L., et al. (2022). Phenotypic and genomic assessment of the potential threat of human spaceflight-relevant Staphylococcus capitis isolates under stress conditions. Front. Microbiol. 13, 1007143. doi: 10.3389/fmicb.2022.1007143
Smith, M. L., O’Neill, C. A., Dickinson, M. R., Chavan, B., McBain, A. J. (2023). Exploring associations between skin, the dermal microbiome, and ultraviolet radiation: advancing possibilities for next-generation sunscreens. Front. Microbiomes 2. doi: 10.3389/frmbi.2023.1102315
Sorbellini, E., Rucco, M., Rinaldi, F. (2018). Photodynamic and photobiological effects of light-emitting diode (LED) therapy in dermatological disease: an update. Lasers Med. Sci. 33, 1431–1439. doi: 10.1007/s10103-018-2584-8
Spittaels, K. J., van Uytfanghe, K., Zouboulis, C. C., Stove, C., Crabbé, A., Coenye, T. (2021). Porphyrins produced by acneic Cutibacterium acnes strains activate the inflammasome by inducing K(+) leakage. iScience 24, 102575. doi: 10.1016/j.isci.2021.102575
Stern, M., Broja, M., Sansone, R., Gröne, M., Skene, S. S., Liebmann, J., et al. (2018). Blue light exposure decreases systolic blood pressure, arterial stiffness, and improves endothelial function in humans. Eur. J. Prev. Cardiol. 25, 1875–1883. doi: 10.1177/2047487318800072
Swartz, T. E., Tseng, T. S., Frederickson, M. A., Paris, G., Comerci, D. J., Rajashekara, G., et al. (2007). Blue-light-activated histidine kinases: two-component sensors in bacteria. Science 317, 1090–1093. doi: 10.1126/science.1144306
Tokonami, S., Onose, M., Nakasone, Y., Terazima, M. (2022). Slow conformational changes of blue light sensor BLUF proteins in milliseconds. J. Am. Chem. Society 144, 4080–4090. doi: 10.1021/jacs.1c13121
Tschowri, N., Busse, S., Hengge, R. (2009). The BLUF-EAL protein YcgF acts as a direct anti-repressor in a blue-light response of Escherichia coli. Genes Dev. 23, 522–534. doi: 10.1101/gad.499409
Tuttobene, M. R., Pérez, J. F., Pavesi, E. S., Mora, B. P., Biancotti, D., Cribb, P., et al. (2021). Light Modulates Important Pathogenic Determinants and Virulence in ESKAPE Pathogens Acinetobacter baumannii, Pseudomonas aeruginosa, and Staphylococcus aureus. J. Bacteriol. 203, e00566–20. doi: 10.1128/JB.00566-20
Uzunbajakava, N. E., Tobin, D. J., Botchkareva, N. V., Dierickx, C., Bjerring, P., Town, G. (2023). Highlighting nuances of blue light phototherapy: Mechanisms and safety considerations. J. Biophotonics 16, e202200257. doi: 10.1002/jbio.202200257
Versey, Z., da Cruz Nizer, W. S., Russell, E., Zigic, S., DeZeeuw, K. G., Marek, J. E., et al. (2021). Biofilm-innate immune interface: contribution to chronic wound formation. Front. Immunol. 12. doi: 10.3389/fimmu.2021.648554
Wahl, S., Engelhardt, M., Schaupp, P., Lappe, C., Ivanov, I. V. (2019). The inner clock-Blue light sets the human rhythm. J. Biophotonics 12, e201900102. doi: 10.1002/jbio.201900102
Wang, Y., Kuo, S., Shu, M., Yu, J., Huang, S., Dai, A., et al. (2014). Staphylococcus epidermidis in the human skin microbiome mediates fermentation to inhibit the growth of Propionibacterium acnes: implications of probiotics in acne vulgaris. Appl. Microbiol. Biotechnol. 98, 411–424. doi: 10.1007/s00253-013-5394-8
Wang, Y., Wilks, J. C., Danhorn, T., Ramos, I., Croal, L., Newman, D. K. (2011). Phenazine-1-carboxylic acid promotes bacterial biofilm development via ferrous iron acquisition. J. Bacteriol. 193, 3606–3617. doi: 10.1128/JB.00396-11
Wang, B., Xu, Y. T., Zhang, L., Zheng, J., Sroka, R., Wang, H. W., et al. (2017). Protoporphyrin IX fluorescence as potential indicator of psoriasis severity and progression. Photodiagnosis Photodyn. Ther. 19, 304–307. doi: 10.1016/j.pdpdt.2017.05.016
Weidinger, S., Beck, L. A., Bieber, T., Kabashima, K., Irvine, A. D. (2018). Atopic dermatitis. Nat. Rev. Dis. Primers 4, 1. doi: 10.1038/s41572-018-0001-z
Weigelt, M. A., Lev-Tov, H. A., Tomic-Canic, M., Lee, W. D., Williams, R., Strasfeld, D., et al. (2022). Advanced wound diagnostics: toward transforming wound care into precision medicine. Adv. Wound Care (New Rochelle) 11, 330–359. doi: 10.1089/wound.2020.1319
Wi, H. S., Na, E. Y., Yun, S. J., Lee, J.-B. (2012). The antifungal effect of light emitting diode on Malassezia yeasts. J. Dermatol. Sci. 67, 3–8. doi: 10.1016/j.jdermsci.2012.04.001
Wilkins, D., Tong, X., Leung, M. H. Y., Mason, C. E., Lee, P. K. H. (2021). Diurnal variation in the human skin microbiome affects accuracy of forensic microbiome matching. Microbiome 9, 129. doi: 10.1186/s40168-021-01082-1
Willmott, T., Campbell, P. M., Griffiths, C. E. M., O’Connor, C., Bell, M., Watson, R. E. B., et al. (2023). Behaviour and sun exposure in holidaymakers alters skin microbiota composition and diversity. Front. Aging 4. doi: 10.3389/fragi.2023.1217635
Wu, J., Chu, Z., Ruan, Z., Wang, X., Dai, T., Hu, X. (2018). Changes of intracellular porphyrin, reactive oxygen species, and fatty acids profiles during inactivation of methicillin-resistant staphylococcus aureus by antimicrobial blue light. Front. Physiol. 9, 1658. doi: 10.3389/fphys.2018.01658
Wu, Y., Wu, Y., Zhu, T., Han, H., Liu, H., Xu, T., et al. (2015). Staphylococcus epidermidis SrrAB regulates bacterial growth and biofilm formation differently under toxic and microaerobic conditions. J. Bacteriol. 197, 459–476. doi: 10.1128/JB.02231-14
Wu, X., Xu, F. (2014). Dendritic cells during Staphylococcus aureus infection: subsets and roles. J. Transl. Med. 12, 358. doi: 10.1186/s12967-014-0358-z
Keywords: blue light, microbiome, cutaneous, skin, photobiomodulation, photodisinfection, chromophore, atopic dermatitis
Citation: Serrage HJ, O’ Neill CA and Uzunbajakava NE (2024) Illuminating microflora: shedding light on the potential of blue light to modulate the cutaneous microbiome. Front. Cell. Infect. Microbiol. 14:1307374. doi: 10.3389/fcimb.2024.1307374
Received: 05 October 2023; Accepted: 26 March 2024;
Published: 10 April 2024.
Edited by:
Xin Xu, Sichuan University, ChinaReviewed by:
Cristina de Guzman Strong, Henry Ford Health System, United StatesVijaykumar Patra, Research And Innovation, L’Oreal, France
Copyright © 2024 Serrage, O’ Neill and Uzunbajakava. This is an open-access article distributed under the terms of the Creative Commons Attribution License (CC BY). The use, distribution or reproduction in other forums is permitted, provided the original author(s) and the copyright owner(s) are credited and that the original publication in this journal is cited, in accordance with accepted academic practice. No use, distribution or reproduction is permitted which does not comply with these terms.
*Correspondence: Hannah J. Serrage, Hannah.serrage@manchester.ac.uk