- 1Laboratory of Biomedical Microbiology and Immunology, The University of Veterinary Medicine and Pharmacy, Kosice, Slovakia
- 2Institute of Neuroimmunology of Slovak Academy of Sciences, Bratislava, Slovakia
Background: The blood-brain barrier (BBB), a highly regulated interface between the blood and the brain, prevents blood-borne substances and pathogens from entering the CNS. Nevertheless, pathogens like Neisseria meningitidis and Borrelia bavariensis can breach the BBB and infect the brain parenchyma. The self-assembling BBB-spheroids can simulate the cross talk occurring between the cells of the barrier and neuroinvasive pathogens.
Methods: BBB spheroids were generated by co-culturing human brain microvascular endothelial cells (hBMECs), pericytes and astrocytes. The BBB attributes of spheroids were confirmed by mapping the localization of cells, observing permeability of angiopep2 and non-permeability of dextran. Fluorescent Neisseria, Borrelia or E. coli (non-neuroinvasive) were incubated with spheroids to observe the adherence, invasion and spheroid integrity. Transcriptome analysis with NGS was employed to investigate the response of BBB cells to infections.
Results: hBMECs were localized throughout the spheroids, whereas pericytes and astrocytes were concentrated around the core. Within 1 hr of exposure, Neisseria and Borrelia adhered to spheroids, and their microcolonization increased from 5 to 24 hrs. Integrity of spheroids was compromised by both Neisseria and Borrelia, but not by E. coli infection. Transcriptome analysis revealed a significant change in the expression of 781 genes (467 up and 314 down regulated) in spheroids infected with Neisseria, while Borrelia altered the expression of 621 genes (225 up and 396 down regulated). The differentially expressed genes could be clustered into various biological pathways like cell adhesion, extracellular matrix related, metallothionines, members of TGF beta, WNT signaling, and immune response. Among the differentially expressed genes, 455 (48%) genes were inversely expressed during Neisseria and Borrelia infection.
Conclusion: The self-assembling spheroids were used to perceive the BBB response to neuroinvasive pathogens - Neisseria and Borrelia. Compromised integrity of spheroids during Neisseria and Borrelia infection as opposed to its intactness and non-adherence of E. coli (non-neuroinvasive) denotes the pathogen dependent fate of BBB. Genes categorized into various biological functions indicated weakened barrier properties of BBB and heightened innate immune response. Inverse expression of 48% genes commonly identified during Neisseria and Borrelia infection exemplifies unique response of BBB to varying neuropathogens.
1 Introduction
The blood-brain barrier (BBB) regulating the influx and efflux of ions, oxygen, nutrients, metabolites and cells between the blood and brain parenchyma protects the central nervous system from toxic and pathogenic insults. BBB encompasses a monolayer of specialized endothelial cells lining the cerebral microvessels. Brain microvascular endothelial cells (BMCEs) are interconnected by a paracellular cleft sealed by adherent and tight junctional proteins that aid in producing transendothelial resistance restricting paracellular transfer of molecules into the brain parenchyma. Moreover, high mitochondrial content, limited transcytotic vesicles and absence of fenestrae in the BMCEs synergizes the restriction on blood compounds to gain CNS entry (Abbott et al., 2010). The barrier property of BBB is further supported by pericytes and astrocytes which are in close anatomical proximity with BMECs. Pericytes are the perivascular cells enclosing the BMECs at the basement membrane and share the same extracellular matrix - basal lamina (Abbott et al., 2010; Coureuil et al., 2017). Pericytes are known to regulate the barrier function of BBB by maintaining junctional architecture of endothelium and restrict over production of pinocytotic vesicle in BMECs (Armulik et al., 2010). Astrocytes connect with BMECs through their endfeet projections which surround the capillaries and venules within the brain parenchyma, and perform sieving function on fluid and solute flux (Iliff et al., 2012). The extracellular matrix shared by the BMCEs and astrocyte endfeet projection - basal lamina 2 differs in composition from basal lamina 1 (Abbott et al., 2010). Moreover, the perivascular space between endothelial cells and astrocyte endfeet is filled with the interstitial fluid which is known to possess lymphatic function (Iliff et al., 2012; Coureuil et al., 2017). These three cell types (BMECs, pericytes and astrocytes) along with the smooth muscle cells and neurons are interlinked tightly to the extracellular matrix of basal lamina forming the neurovascular unit (NVU) (Jessen et al., 2015).
The complex architectural characteristics of the neurovascular unit aids in limiting the traffic of both pathogens and host immune cells such as granulocytes and lymphocytes to enter the brain paranchyma. Nevertheless, several pathogens can invade the BBB and cause chronic neurological manifestations (e.g. Borrelia garinii, Borrelia bavariensis), while some cause acute meningitis (like Neisseria meningitidis, Streptococcus Sp., Listeria monocytogenes) as reviewed elsewhere (Bencurova et al., 2011; Pulzova et al., 2011). Transmigration of N. meningitidis and B. bavariensis through human BMECs (hBMECs) occurs through transcellular receptor-mediated endocytosis or by paracellular crossing (Comstock and Thomas, 1989; Grab et al., 2005; Coureuil et al., 2010; Divan et al., 2018). Platelet activating factor receptor (PAFR), laminin receptor, poly-Ig receptor (pIgR), PCAM-1, CD147, β2‐adrenergic receptor and plasminogen activator receptor are some of the well-known receptors on BMCEs targeted for transcellular receptor-mediated endocytosis (Comstock and Thomas, 1989; Grab et al., 2005; Orihuela et al., 2009; Al-Obaidi and Desa, 2018). On the other hand, disruption of inter-cellular tight junctions by enzymes belonging to fibrinolytic system and matrix metalloproteinases (e.g. MMP1 and MMP8) during N. meningitidis and B. burgdorferi infection in hBMECs denotes paracellular passage of bacteria (Schubert-Unkmeir et al., 2010). Permeability of hBMCEs during meningococcal infection has been further confirmed through high throughput sequencing methods, in which transcripts of the molecules involved in cell adhesion, endocytosis, cytoskeletal and extracellular matrix re-organization, cytokine production, pattern recognition receptors dependent signaling (e.g. TLR-2), apoptosis and metabolism were prominently evoked (Nassif et al., 2002; Schubert-Unkmeir et al., 2007; Coureuil et al., 2012; Coureuil et al., 2017; Al-Obaidi and Desa, 2018; Káňová et al., 2019). Interaction of Borrelia with BMECs, microglia, astrocytes, brain tissues of non-human primates and mice has also shown to evoke MMPs, transcription factors and inflammatory and chemokine pathways (Ramesh et al., 2003; Ramesh et al., 2008; Brissette et al., 2013; Carey and Ho, 2017; Casselli et al., 2017). Overexpression of chemokines in astrocytes was suggested to promote infiltration of inflammatory cells causing neuronal damage during neuroborreliosis (Ramesh et al., 2003; Ramesh et al., 2008). Most of the aforementioned studies describing the molecular events in BBB invasion have used single cell types (e.g. BMCEs or glial cells) and the conclusions were drawn towards the BBB permeability. Although, the transwell systems with the monolayer of endothelial cells or choroid plexus epithelial cells are efficient in vitro models to study neuroinvasive bacterial pathogenesis (Grab et al., 2005; Grab et al., 2009; Martins Gomes et al., 2019; Thompson et al., 2020). It is apparent that non-endothelial cells of the NVU are obligatory to generate the complete BBB phenotype.
The co-culture of BMCEs, pericytes, and astrocytes, which forms spontaneous 3D spheroids and simulates the BBB, has recently been standardized (Bergmann et al., 2018). The BBB spheroids bear several distinct features of the barrier like the presence of appropriate junctional proteins and efflux/influx pumps, direct contact between different NVU cell, reduced to nil paracellular permeability and evidence for receptor-mediated transcytosis (Cho et al., 2017; Gastfriend et al., 2018; Kumarasamy and Sosnik, 2021). Thus, in the present study, BBB spheroids produced by co cultivation of human BMECs (hBMECs), human brain vascular pericytes (pericytes) and human astrocytes (astrocytes) were used to observe the infection of N. meningitidis (Neisseria) and B. bavariensis (Borrelia) using time lapse microscopy. Further, differential transcriptome analysis of spheroids exposed to Neisseria or Borrelia was performed through illumina RNAseq technology to elucidate the BBB’s response to invading pathogens.
2 Materials and methods
2.1 Bacterial culture
N. meningitidis (isolate M1/03, MC58 homologue, Serogroup B) was cultured in brain heart infusion broth (BHI; Jena Bioscience, Germany) containing 10 mM MgCl2, (Sigma, Slovakia) at 37 °C in 5% CO2.
To stain Neisseria, culture (OD600 0.6) was centrifuged (3420 x g for 10 min), pellet was redispersed in 2 mL BHI broth with 1x SYBR green I dye (Thermo Fisher Scientific, USA) and incubated for 2 h (37°C, 5% CO2). Bacteria were washed with 1x PBS and redispersed into 1 mL of EBM-2 basal medium (Lonza, Switzerland). Neisseria were enumerated using the flow cytometer (flow rate: slow, 1 min, gate at FL1 533/30nm versus SSC-A threshold of 10,000 on BD Accuri C6, USA).
Neuroinvasive strain of the B. bavariensis sp. nov. strain SKT-7.1 constitutively expressing eGFP (Pulzova et al., 2013) was grown in 40 mL of BSK-II medium till its logarithmic phase, centrifuged at 3420 x g for 10 min, the pellet was washed with 1x PBS (three times) and resuspended in 4 mL of EBM-2 medium. Borrelia were counted using the flow cytometer (flow rate slow, 1 min, core size 10 μm, gate SSC-H threshold 5000 versus FL1 510/15nm threshold 3000).
E. coli strain SG13009 a non-biohazardous laboratory strain [sold by Qiagen, Germany for protein overexpression, (Gottesman et al., 1981)], was modified to express eGFP (Comor et al., 2017). E. coli were grown in 10 mL of LB medium (OD600 0.6) with 50 μg/mL carbenicillin, centrifuged (3420 x g for 10 min) and pellet was resuspended in 2 mL of EBM-2 medium. E. coli were counted on the flow cytometer using the identical parameters used for Borrelia.
2.2 Cell culture conditions
Human brain microvascular endothelial cells (hBMCE/D3, Merck, Czech Republic) were cultured in DMEM-F12 medium as described previously (Hruškovicová et al., 2022). Human brain vascular pericytes (pericytes, ScienCell, USA) were cultured on Poly-L-Lysine (2 µg/cm2, Sigma Aldrich) coated flask in pericyte medium supplemented with 2% FBS, 1x pericyte growth supplements and penicillin-streptomycin (all components from ScienCell). Human astrocytes (ScienCell) were cultured on Poly-L-Lysine (2 µg/cm2) coated flask in DMEM high glucose GlutaMax (Thermo Fisher Scientific) supplemented with 10% FBS, 1x N2-supplement (Thermo Fisher Scientific) and human epidermal growth factor (20 ng/mL, Merck). All cell types were maintained between 3rd- 6th passage and were cultured at 37 °C, 5% CO2 until 80-85% confluency.
2.3 In vitro culture of BBB spheroids
Self-assembling multicellular spheroids were produced as described previously (Bergmann et al., 2018). In brief, 50 µL of molten 1% agarose in PBS (w/v) was dispensed in 96-well plates. After solidification, 200 µL of BBB-working medium (2% human serum (v/v) in EGM-2 Endothelial Cell Growth Medium-2 BulletKit, Lonza, Switzerland) was added in each well. VEGF was omitted from the BBB-working medium to avoid tight junction disruption (Bergmann et al., 2018). hBMECs, pericytes, and astrocytes cultured in T-75 flask (85% confluency) were dislodged (trypsin-EDTA, Biowest, France) and washed once with EBM-2 medium. All cell types were mixed in a ratio 1:1:1 and seeded on agarose coated well (4.5 x 103 cells/well). Cells were incubated at 37 °C in 5% CO2 until spheroid formation.
2.4 Localization of hBMECs, astrocytes and pericytes in BBB spheroids
CellTracker deep red (1µM, 630nmex/650nmem) was used to stain hBMECs, while CellTracker green BODIPY (10µM, 522nmex/529nmem), and CellTracker blue CMHC (10µM, 353nmex/466nmem) were used to stain pericytes and astrocytes, respectively, according the manufacturers’ instructions (all dyes are from Thermo Fisher Scientific). Stained cells were seeded on agarose coated well in 1:1:1 ratio (4.5 x 103/well) and incubated until spheroid formation. Few spheroids were frozen in OCT mounting medium (VWR) and cryosections of 10 microns were cut on LEICA biosystem. The cryosections were mounted on poly-l-lysin coated microscope slides (Thermo Scientific), fixed with cold acetone for 10 min and mounted with Fluoroshield (Sigma Aldrich) prior to their photo-documentation on Cytation 7 (BioTek, USA) or LSM700 confocal microscope (Zeiss, Germany).
2.5 Integrity of self-assembled spheroids governed by tight junctions
Integrity of self-assembled spheroids was examined by comparing the permeability of angiopep-2 and dextran. Ten spheroids were transferred to ultra-low attachment 96 well plate containing 200 µL of BBB-working medium with angiopep-2 5-TAMRA conjugate (10 µg/mL, Lumiprobe, Germany) or 70 kDa dextran-FITC (10 µg/mL, Thermo Fisher Scientific). Spheroids were incubated for 24 h at 37 °C in CO2 incubator with constant rotation (5 rpm).
To confirm the integrity of spheroid governed by tight junctions, three spheroids were incubated with different concentrations of VEGF-A (25, 50 and 100 ng/mL) and dextran-FITC (10 µg/mL) for 24 h at 37 °C in CO2 incubator. Spheroids were then washed with 200 µL of 1x PBS (7 rpm, 10 min; 5 times) and fixed in 4% formalin at room temperature for 1 h. Spheroids were re-washed with PBS, laid on coverslip, air dried and placed within the cavity of glass depression slides. Photo documentation was performed on Cytation 7 using 542nmex/568nmem for angiopep-2-5-TAMRA and 495nmex/518nmem for dextran-FITC.
2.6 Adhesion of Neisseria and Borrelia on spheroids
Spheroids in 200 µL of BBB-working medium devoid of antibiotics were challenged with either 6x103 SYBR green I stained Neisseria (MOI = 1:4; number of hBMECs used to make spheroid: Neisseria) or 1.5x104 constitutively expressing eGFP Borrelia (MOI = 1:10) or non-neuroinvasive E. coli expressing eGFP (MOI = 1:4). Time lapse microscopy was performed to visualize the bacterial adhesion using Cytation 7. Images of infected spheroids were captured at 1, 3, 5 and 24 h post exposure using 495nmex and 518nmem to visualize the green fluorescence of bacteria.
2.7 Integrity of spheroids after exposure to Neisseria or Borrelia
Spheroids were exposed to either Neisseria, Borrelia, or E. coli under the same conditions as described above. Dextran-tetramethylrhodaimine (70 kDa, 10 µg/mL) was immediately added to all wells and incubated in a CO2 incubator at 37°C with rotation (5 rpm). After 3 h, the spheroids were washed with PBS and fixed with the 4% formalin as described above. The passage of dextran-tetramethylrhodaimine in spheroids was analyzed using Cytation 7 at 555 nmex and 580 nmem.
2.8 Cell response of spheroids to Neisseria or Borrelia infection
As previously described, 144 spheroids were generated and transferred to ultra-low attachment plates containing 200 µl of BBB-working medium free of hydrocortisone, GA-100, and ascorbic acid. Simultaneously, Neisseria culture (OD600 0.6) and Borrelia culture in its logarithmic phase was centrifuged (3420 x g for 10 min) and the pellet was resuspended in 2 mL of EBM-2 basal medium. Bacteria were enumerated on flowcytometer as described before. Spheroids (N= 36) were challenged with either Neisseria (6 x 104, MOI 1:4) or Borrelia (1.5 x 105, MOI 1:10). Spheroids without infection served as control group (Supplementary Figure 1A). After 3 h, 12 spheroids per treatment were pooled in a tube and three pools (replicates) for each treatment were designated. Pooled spheroids were rinsed with PBS (in nuclease free water) and the total RNA was extracted using RNeasy Mini Kit (Qiagen), which includes on-column DNase digestion. Concentration of RNA was measured on NanoDrop and the integrity was assessed on 1% agarose gel electrophoresis. RNA (500 ng) was reverse transcribed using oligodT primer included in QuantSeq FWD 3’mRNA Library Prep Kit (Lexogen, Austria). Half the recommended volume of OligodT primer (2.5 µL) was used to minimize the off-target products. RNA removal solution (RS buffer, Lexogen) was used on cDNA to eliminate any remaining RNA. Second strand was synthesized using the UMI Second Strand Synthesis Mix (USS, Lexogen) containing 6 nucleotides long Unique Molecular Identifiers (UMIs). UMI allows detection and removal of PCR duplicates. The double stranded cDNA libraries were amplified in 17-19 PCR cycles using i5 Unique Dual Indexing Add-on Kit (Lexogen). The quality and quantity of libraries was determined on Fragment Analyzer using DNF-474 HS NGS Fragment Kit (Agilent Technologies) and QuantiFluor dsDNA System (Promega), respectively. Illumina NextSeq 500 was employed to sequence the libraries having a read lengths of single-end 75 bp producing about 10 million reads per library (Supplementary Table 1).
2.9 Data analysis
High-throughput RNA-Seq data obtained from sequenced libraries in Bcl file format was converted to Fastq format using bcl2fastq v. 2.20.0.422 (Illumina software for basecalling). The 6-nucelotide long UMIs were extracted and the deduplication of aligned reads was performed by UMI-tools v. 1.1.1. as described elsewhere (Smith et al., 2017). Trimming of UMI and the quality assessment of raw single-end fastq reads was performed using seqtk 1.3 (r106) (https://github.com/lh3/seqtk/releases/tag/v1.3) and FastQC v0.11.9 (http://www.bioinformatics.babraham.ac.uk/projects/fastqc/) respectively. Trimmomatic v0.36 (https://github.com/usadellab/Trimmomatic) was used to trim the adapter sequence and raw fastq reads. The resulting trimmed RNA-Seq reads were mapped to human genome (hg38) and Ensembl GRCh38 v.94 annotation using STAR v2.7.3a (https://github.com/alexdobin/STAR). Quality control on mapped reads (number and percentage of uniquely and multi-mapped reads, rRNA contamination, mapped regions, read coverage distribution, strand specificity, gene biotypes and PCR duplication) was performed using tools: RSeQC v2.6.2 (http://rseqc.sourceforge.net/), Picard v2.18.27 (https://github.com/broadinstitute/picard), Qualimap v.2.2.2 (http://qualimap.conesalab.org/) and BioBloom tools v 2.3.4-6-g433f (https://github.com/bcgsc/biobloom).
To carryout gene expression analysis, Bioconductor R-package - DESeq2 v1.20.0 (Love et al., 2014) was used where in, featureCounts tool v1.6.3 (Liao et al., 2013) was used to enumerate the genes. A minimum threshold of adjusted p-value < 0.05 and log2fold-change (log2FC) = ± 1 was set to considered the gene as differentially expressed (DEG). RNA-seq data was deposited into publicly available EBI Arrayexpress repository (Accession no E-MTAB-13401).
2.10 Bioinformatics analysis of differentially expressed genes
Log2FC values of DEGs were used to create a violin plot (Graphpad Prism v9.3). DEGs were analyzed on Reactome (https://reactome.org/) to segregate them in various biological processes (cutoff p-value < 0.05). Genes unclassified in Reactome, were examined in DAVID Bioinformatics Resources v 6.8 (https://david.ncifcrf.gov/home.jsp) to determine additional clusters (threshold for entry p value set as < 0.05). A Venn diagram was used to determine the relationship between the DEGs found in both infections (Venny 2.1 https://bioinfogp.cnb.csic.es/tools/venny/) and heat maps to visualize the clustered DEGs (GraphPad).
2.11 Validation of DEGs by quantitative real-time polymerase chain reaction
Reverse transcription of RNA (1 µg) into cDNA was performed using random hexamer primers as described previously (Bhide et al., 2022). mRNA levels of DEGs were corroborated with qPCR using 6 ng of cDNA, 10 pmol of gene specific primers (Supplementary Table 2, primers designed with Geneious Pro software) and Sybr green chemistry on StepOnePlus Real-Time PCR System (Bhide et al., 2022). The average of threshold cycle (Ct) values of two reference genes - β2-microtubulin and glyceraldehyde 3-phosphate dehydrogenase was used for normalization. The relative gene expression (2-ΔΔCt) and log2fold change (Log2FC) was determined as described before (Bhide et al., 2022). Pearson correlation coefficient (r) was calculated between the Log2FC values of RNA-seq and qRT-PCR using Graphpad.
3 Results
3.1 Self-assembly of spheroids and their integrity
All the three co-cultured cell types, hBMECs, pericytes and astrocytes, aggregated within 48 h to form a single spheroid (Figures 1A–D). Microscopy of spheroids cultured with cell-tracker-stained cells revealed that hBMECs are present throughout the spheroid, whereas pericytes and astrocytes are concentrated around the core (Figures 1E–H; Supplementary Images 1 and 2). The barrier function of the endothelial cell layer was confirmed by incubating spheroids with dextran-FITC or angiopep-2 5-TAMRA conjugate. Dextran was only found on the periphery, whereas angiopep-2 was observed throughout the spheroid (Figures 2A, B). Incubation of spheroids with VEGF-A increased dextran influx in a concentration-dependent manner (Figures 2C-E). As VEGF is known to disrupt intercellular junctions and increase paracellular permeability (Esser et al., 1998; Antonetti et al., 1999) the findings show that intercellular adherent, tight, and gap junctions were formed properly and spheroids were suitable to use in further experiments.
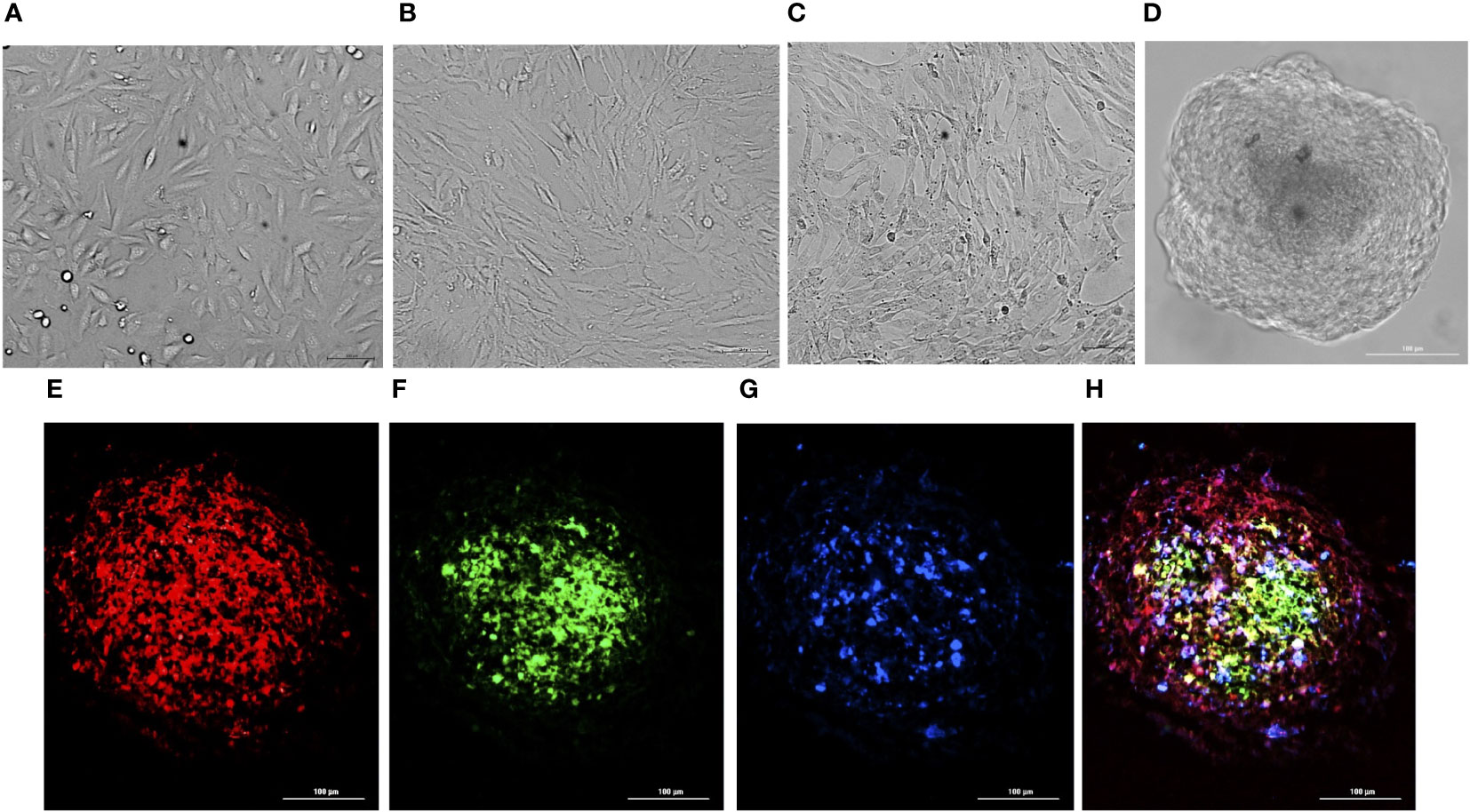
Figure 1 Preparation of BBB spheroids. Bright-field images of hBMECs (A), pericytes (B), and astrocytes (C) self-assembled into BBB spheroids (D). Fluorescence images of cryosection (10 µm) of spheroid generated by co-culturing hBMECs pre-stained with CellTracker Deep Red, captured at 630nmex/650nmem (E), pericytes pre stained with CellTracker Green BODIPY, captured at 522nmex/529nmem (F), astrocytes pre-stained with CellTracker Blue CMHC captured 353nmex/466nmem (G), and a merged image (H) generated by combining (E–G).
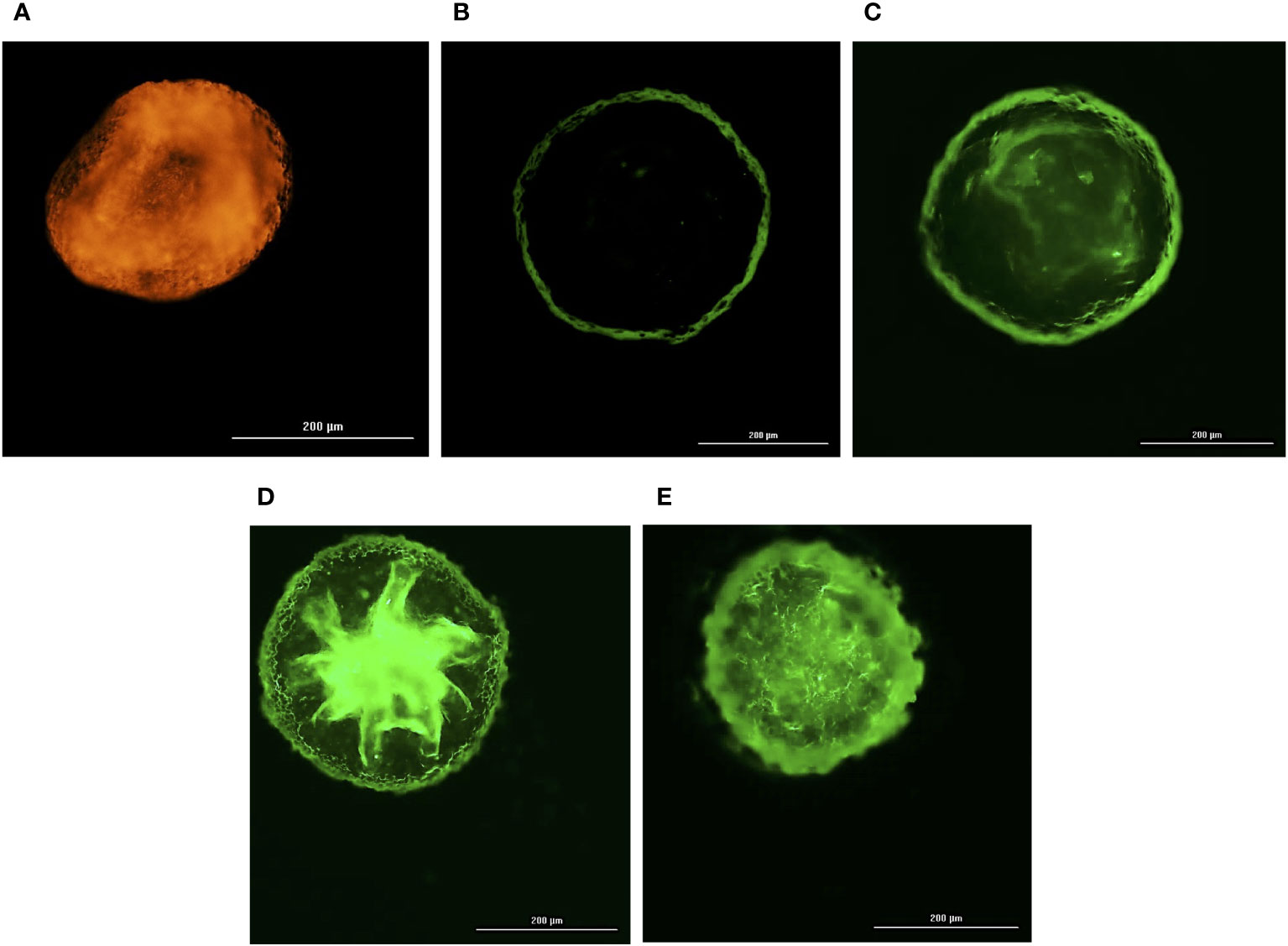
Figure 2 Integrity of self-assembled spheroids. Fluorescence images of a 4% formalin fixed BBB spheroids pre-incubated with 10 µg/mL of angiopep-2 5-TAMRA conjugate (A), 10 µg/mL of 70kDa dextran – FITC (B), 25 ng/mL of VEGF-A and 10 µg/mL of 70kDa dextran – FITC (C), 50 ng/mL of VEGF-A and 10 µg/mL of 70kDa dextran – FITC (D), 100 ng/mL of VEGF-A and 10 µg/mL of 70kDa dextran – FITC (E) for 24h. Orange fluorescence of angiopep-2 5-TAMRA conjugate observed at 542nmex/568nmem (A) denotes permeation of angiopep-2, whereas green fluorescence of FITC observed on the periphery of spheroid captured at 495nmex/518nmem denotes non traversal of 70kDa dextran – FITC (B). However, simultaneous incubation of VEGF-A (25-100 ng/mL) and10 µg/mL of 70kDa dextran – FITC (C–E) shows corresponding increase in green fluorescence of FITC depicting VEGF-A dependent permeability of 70kDa dextran.
3.2 Adhesion of bacteria on spheroids and its effect on integrity
In time-lapse microscopy of spheroids exposed to fluorescent Neisseria and Borrelia, adherence was observed within the 1st hour of incubation, (Figures 3A, F). A marginal increase in their adhesion was observed by 3 hours of incubation (Figures 3B, G). By the 5th hour of exposure, there was micro-colonization of Neisseria and aggregation of Borrelia possibly forming biofilm (Figures 3C, H) (Sapi et al., 2012). By the 24th hour, there was heavy colonization of Neisseria and increased aggregation of Borrelia throughout the spheroids (Figures 3D, I). E. coli, on the other hand, did not adhere the spheroid even after 24 h of incubation (Figures 3K–N). Infection of Borrelia and Neisseria compromised the integrity and barrier properties of spheroids as the influx of the dextran-tetramethylrhodaimine was clearly observed by 3 hours post incubation (Figures 3E, J). On the other hand, spheroids exposed to E. coli, showed the meager presence of dextran at the periphery (Figure 3O).
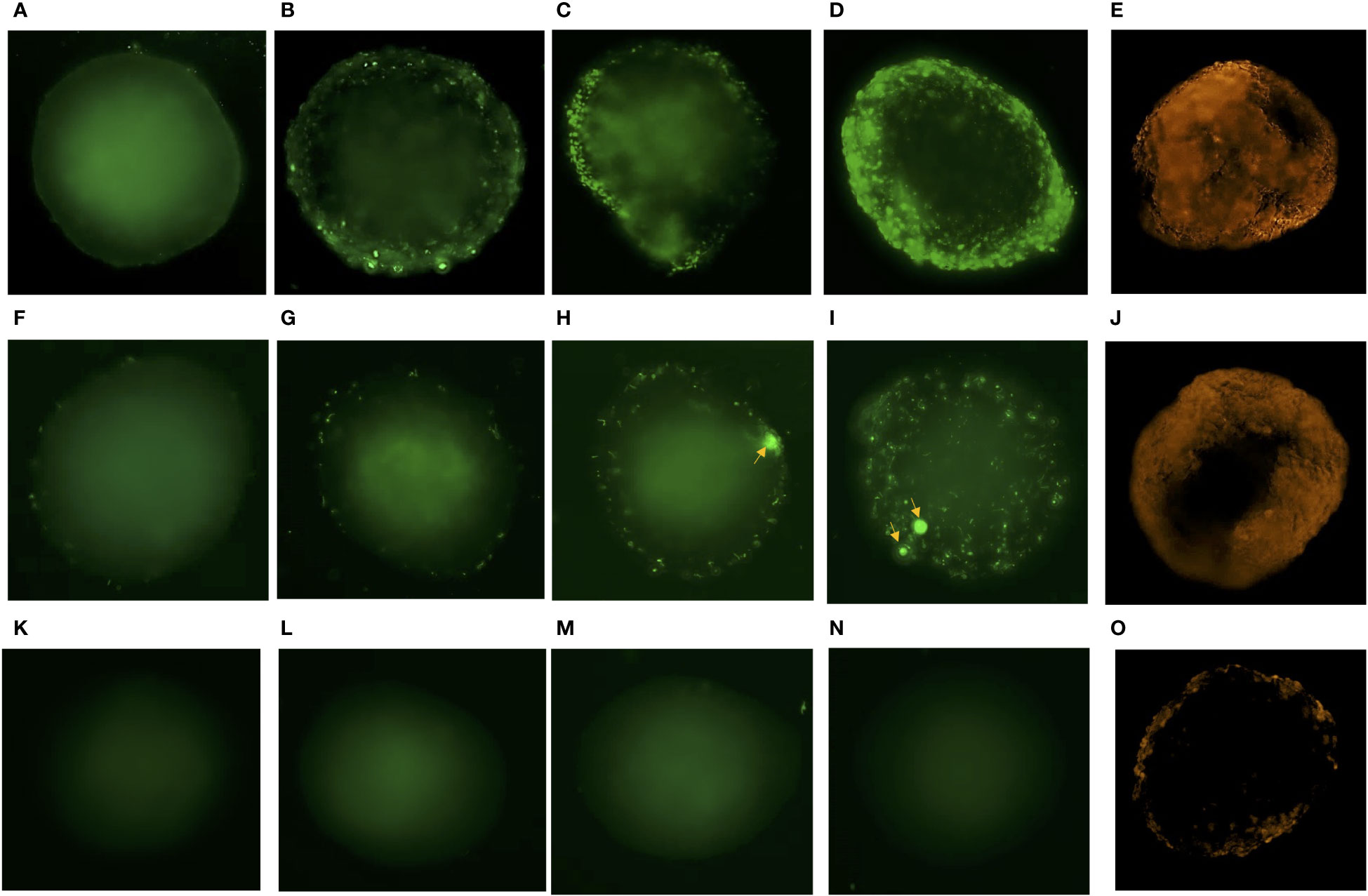
Figure 3 Time lapse microscopy of spheroids exposed to Neisseria, Borrelia, E. coli and evaluation of their integrity. Representative images of BBB spheroids exposed to SYBR green I stained Neisseria captured at 1h (A), 3h (B), 5h (C) and 24h (D) post infection at 495nmex/518nmem is depicted. (E) Infusion of 70 kDa dextran – tetramethylrhodaimine in spheroid exposed to Neisseria for 3h observed as orange fluorescence captured at 555 nmex/580 nmem depicts compromised integrity of BBB spheroids during infection. Similarly, representative images of spheroids exposed to constitutively expressing eGFP Borrelia captured at 1h (F), 3h (G), 5h (H) and 24h (I) post infection and (J) infusion of dextran – tetramethylrhodaimine in spheroids at 3h is depicted. Representative images of spheroids exposed to non-neuroinvasive E. coli expressing eGFP captured at 1h (K), 3h (L), 5h (M) and 24h (N) is depicted. Meager adhesion of 70 kDa dextran – tetramethylrhodaimine on the periphery of spheroids (O) exposed to E. coli for 3h shows optimum integrity of spheroids. Increase in adhesion (B), multiplication (C, D) of Neisseria and aggregation of Borrelia potentially forming biofilm (H, I; indicated by arrows), with the passage of time (1-24h) is evident as opposed to no adhesion of E. coli (K–N).
3.3 RNAseq data analysis, validation and bioinformatic analysis
The response of the BBB spheroid undergoing Neisseria or Borrelia infection was mapped using RNAseq. RNA extracted from the infected and non-infected spheroids had suitable concentration and quality necessary for the synthesis of cDNA libraries (Supplementary Figures 1B, C). The fragment size in all 12 cDNA libraries was about 220 -250 bp (Supplementary Figures 2, 3). Raw reads produced from NGS ranged from 9.3 to 13.0 million. Approximately 73-79% of processed reads were uniquely mapped to the human genome. Supplementary Table 1 contains additional information about the reads and their mapping to the human genome. A total of 30,145 genes were mapped from sequenced libraries with more than one normalized read (Supplementary Data Sheet 1). The expression of 781 genes was significantly altered (DEGs) in spheroids challenged with Neisseria (467 genes with Log2FC ranged from 1.0 to 6.2 and 314 genes with log2FC ranged from -1.0 to -5.7). In spheroids challenged with Borrelia, 225 genes were significantly upregulated (log2FC 1.0 to 5.6) and 396 genes were significantly downregulated (log2FC -1.0 to -6.9) (Figure 4A and Supplementary Data Sheet 2).
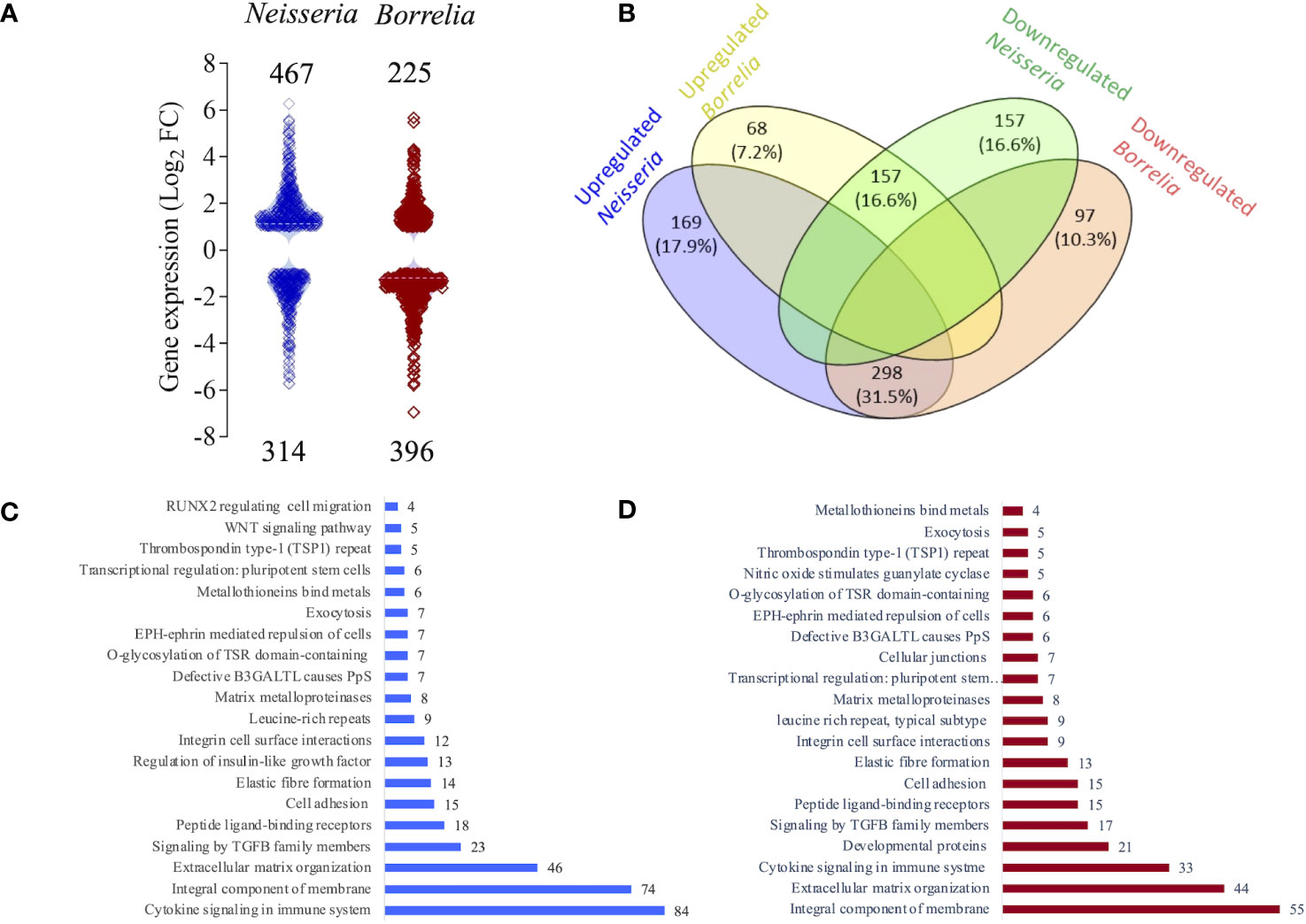
Figure 4 Classification of differentially expressed genes (DEGs). (A) Violin plot depicting the distribution of significantly up (Log2FC >1) or down regulated genes (Log2FC <1) in spheroids infected with either Neisseria (blue) or Borrelia (maroon) identified by RNAseq. Log2FC of each gene is represent by a square and total number of DEGs are mentioned. (B) Venn diagram showing the relationship between the DEGs identified during Neisseria or Borrelia infection. Blue and green ellipse represent number and percentage of up and down regulated DEGs during Neisseria infection respectively. Likewise yellow and orange ellipse represents number and percentage of up and down regulated DEGs during Borrelia infection respectively. Number and percentage of commonly expressed DEGs are mentioned in the intersection of ellipses. Bar graphs showing 20 salient clusters and number of DEGs classified within them during Neisseria (C) or Borrelia (D) infection.
298 genes that were upregulated during Neisseria infection were downregulated during Borrelia infection, whereas 157 genes that were downregulated during Neisseria infection were upregulated during Borrelia infection. 169 genes were exclusively upregulated during Neisseria infection, and during Borrelia infection it was 68 genes. The number of genes that were exclusively downregulated during Neisseria and Borrelia infection was 157 and 97, respectively (Figure 4B and Supplementary Data Sheet 3).
The Pearsons correlation coefficient (r) of 0.805 and p value = 0.005 between the Log2FC values of 10 randomly selected DEGs observed in RNAseq and qRT-PCR validates the differential expression of genes determined by RNAseq (Supplementary Figure 4).
Functional annotation of DEGs was performed using the Reactome and DAVID servers, providing categorization of DEGs into various pathways such as cell adhesion, extracellular matrix organization, cytokine signaling, and so on (Figures 4C, D; Supplementary Sata Sheet 4). In the following part of results, we are presenting the biological process, enriched at BBB that suggests weekended barrier attributes along with the enhanced immune response.
3.4 Cell adhesion molecules
In the infected spheroids, 41 genes belonging to cadherin superfamily, focal adhesion molecules, junctional proteins, and integral components of membrane proteins (all of these pathways govern cell adhesion) were significantly evoked (Figure 5; Supplementary Table 3). All these genes were inversely evoked in BBB infected with Neisseria and Borrelia. Genes encoding cadherins (CDHs), protocadherins (PCDHs), and the protocadherin gamma gene cluster - A10 and B7 were upregulated during Neisseria infection, whereas the same genes were downregulated during Borrelia infection, with an exception of CDH15. Among junctional proteins, the bicellular tight junction genes F11 receptor (F11R) and claudin 7 (CLDN7) were downregulated during Neisseria infection but upregulated during Borrelia infection (Figure 5; Supplementary Table 3). Genes encoding integral components of cell membrane (ICAM, VCAM and NCAM-1) were also inversely expressed. However, the pattern of expression for genes related to focal adhesion was not consistent during either of the bacterial infections (Figure 5; Supplementary Table 3).
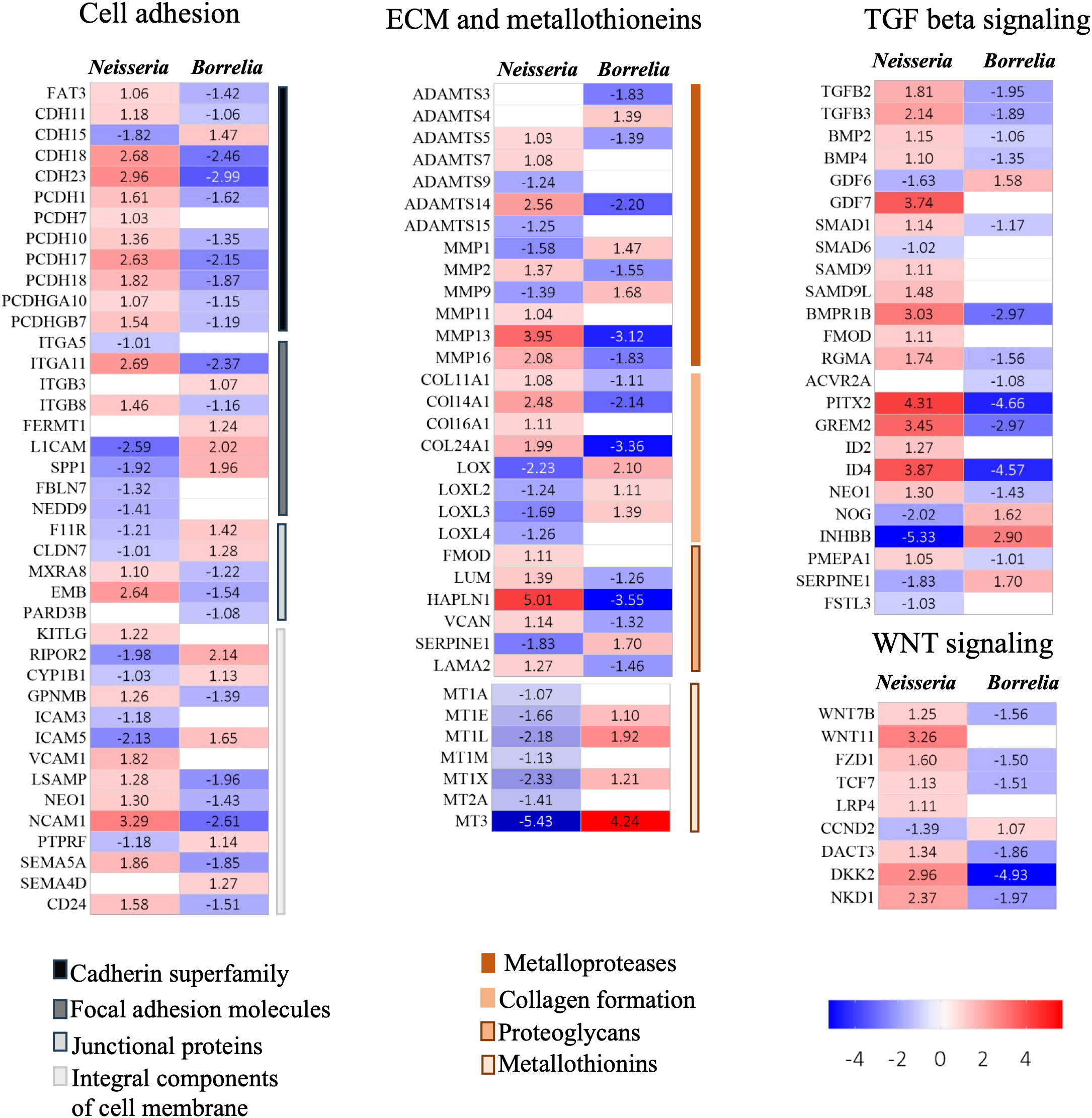
Figure 5 Heatmaps for DEGs. Heatmaps depicting Log2FC values of DEGs identified in BBB spheroids undergoing Neisseria or Borrelia infection. DEGs are categorized into biological functions – cell adhesion, ECM and metallothionins, TGF beta signaling and WNT signaling. Color scale presents Log2FC values.
Surprisingly, E-selectin’s expression levels remained unchanged in infected spheroids, despite the fact that it was one of the highly expressed DEGs observed previously during Neisseria or Borrelia infection to hBMECs.
3.5 Extracellular matrix related genes and metallothioneins
Several DEGs involved in extracellular matrix reorganization were activated in response to Neisseria and Borrelia infection (Figure 5; Supplementary Table 3). Among the metalloproteases, seven ADAMTS (a disintegrin and metalloproteinase with thrombospondin motifs) and six matrix metalloproteinases (MMPs), were differentially expressed. Both ADAMTS and MMPs, are extracellular metalloenzymes that can degrade extracellular matrix (ECM) proteins. Besides degrading the components of ECM, MMPs can cleave various, basement membrane proteins, tight junction proteins, cytokines, chemokines and growth factors (Schubert-Unkmeir et al., 2010). Four Collagens (COL11A1, COL14A1, COL16A1, and COL24A1) mediating fibrillogenesis, were upregulated in response to Neisseria, but downregulated in during Borrelia infection (Figure 5; Supplementary Table 3). The genes encoding the members of the lysyl oxidase family (Lox, LoxL2, LoxL3 and LoxL4) also showed an opposite pattern of expression during Neisseria and Borrelia infection. Lysyl oxidases are critical for the crosslinking of elastin and collagen in extracellular matrix during the repair of ECM. Fibromodulin, which interacts with Lox to modulate collagen crosslinking and prevent collaginolysis, was upregulated in Neisseria infections. In addition to fibromodulin, Neisseria induced the expression of five other ECM-proteoglycans, viz., lumican (which stimulates endothelial cell growth and vascular sprouting), hyaluronan, proteoglycan link protein (Hapln-1) and versican (Figure 5; Supplementary Table 3). Hapln-1 was the only EMC proteoglycan that was downregulated in response to Borrelia.
Metallothionins, regulating intracellular zinc and copper trafficking in response to endogenous or exogenous stimuli (such as infection, cytokine release, and oxidative stress), were downregulated during neisserial infection. The list includes - metallothionin 1 (MT1A, MT1E, MT1M, and MT1X), the MT1L pseudogene, and metallothionin 2 and 3. On the other hand four metallothionins - MT1E, MT1L, MT1X, and MT3, were upregulated during Borrelia infection (Figure 5; Supplementary Table 3). In the presence of lipopolysaccharide, metallothionins can aid in the induction of TNF-α and NF-kB, whereas metallothionin knockout causes vacuolar damage in endothelial cell and focal loss of basement membrane. MT2A specifically influences the MMP9 expression and MT3 increases VEGF expression in BMECs (Kanekiyo et al., 2002; Takano et al., 2004).
3.6 Signaling by Transforming growth factor beta family members
TGF-β, a multifunctional cytokine, regulates a variety of cellular functions namely cell proliferation, differentiation, adhesion, and migration, all of which are critical for maintaining BBB integrity. There are atleast 30 proteins associated with TGF-β, which form a superfamily, and many of them were differentially expressed in infected spheroids viz., ligands of TGF-β (TGFB2, TGFB3, bone morphogenetic protein 2, growth factors - germalin 2, inhibin subunit beta B, and growth differentiation factor 6 and 7), receptors (BMP receptor type 1B, activin A receptor type 2A and neogenin 1), signal transducers (receptor regulated SMADS 1 and 9, and inhibitory SMAD 6) and transcriptional regulators (paired like homeodomain 2(PITX2) and inhibitor of DNA binding 2 and 4) (Figure 5; Supplementary Table 3). TGF-β signaling occurs in two branches, the TGF-β branch and the BMP branch. Both the branches appeared to be activated during Neisseria infection. Three inhibitory molecules were downregulated viz. SMAD 6 (Log2FC -1.02), noggin (NOG; Log2FC -2.02) and inhibin subunit beta b (INHBB; Log2FC -5.32). As opposed to Neisseria infection, the TGF-β signaling pathway was not activated during borrelial infection as several inhibitory molecules were induced.
3.7 WNT signaling pathway
Canonical Wnt/β -catenin signaling pathway can regulate the formation of the BBB (Stenman et al., 2008). In our study, at least 9 DEGs belonging to Wnt/β-catenin signaling are identified, which includes Wnt7b, Wnt11, FZD1, TCF7, LRP4, DKK2, DACT3, NKD1 and CCND2. FZD1 is receptor for Wnt glycoproteins, TCF7 represses transcription of β-catenin target genes, LRP4 is a negative regulator of the Wnt signaling pathway, DACT3 is a dishevelled binding antagonist of β-catenin 3, NKD1 is inhibitor of WNT signaling pathway and CCND2 is an effector of cell cycle progression. Although Neisseria infection upregulated Wnt7b, Wnt11, and FZD1, four inhibitors (TCF7, LRP4, DKK2, DACT3 and NKD1) were upregulated and CCND2 which favors cell cycle progression was downregulated. Borrelia infection resulted in the inverse expression of the aforementioned genes (Figure 5; Supplementary Table 3).
3.8 Intracellular trafficking
Eighteen genes associated with endocytosis and intracellular trafficking were differentially expressed in infected spheroids (Figure 6; Supplementary Table 3). Dynamin 1 (DNM1), a GTPase involved in clathrin-mediated and clathrin-independent endocytic pathways was upregulated during Neisseria infection. Surface proteins of Neisseria can induce the expression of DNM1 to gain entry into the endothelial cells (Herold et al., 2021). Another GTPase, RAC3 was downregulated only in Neisseria infection. Members of RAB GTPse (RAB 6B, 7b, 9b, 20 and 27b) and their effector molecules like synaptotagmin (SYT1, 5, 7, and 14) and exophilins (SYTL 3 and 5) were differentially expressed in infected spheroids that are known to participate in the endocytosis and exocytosis pathways.
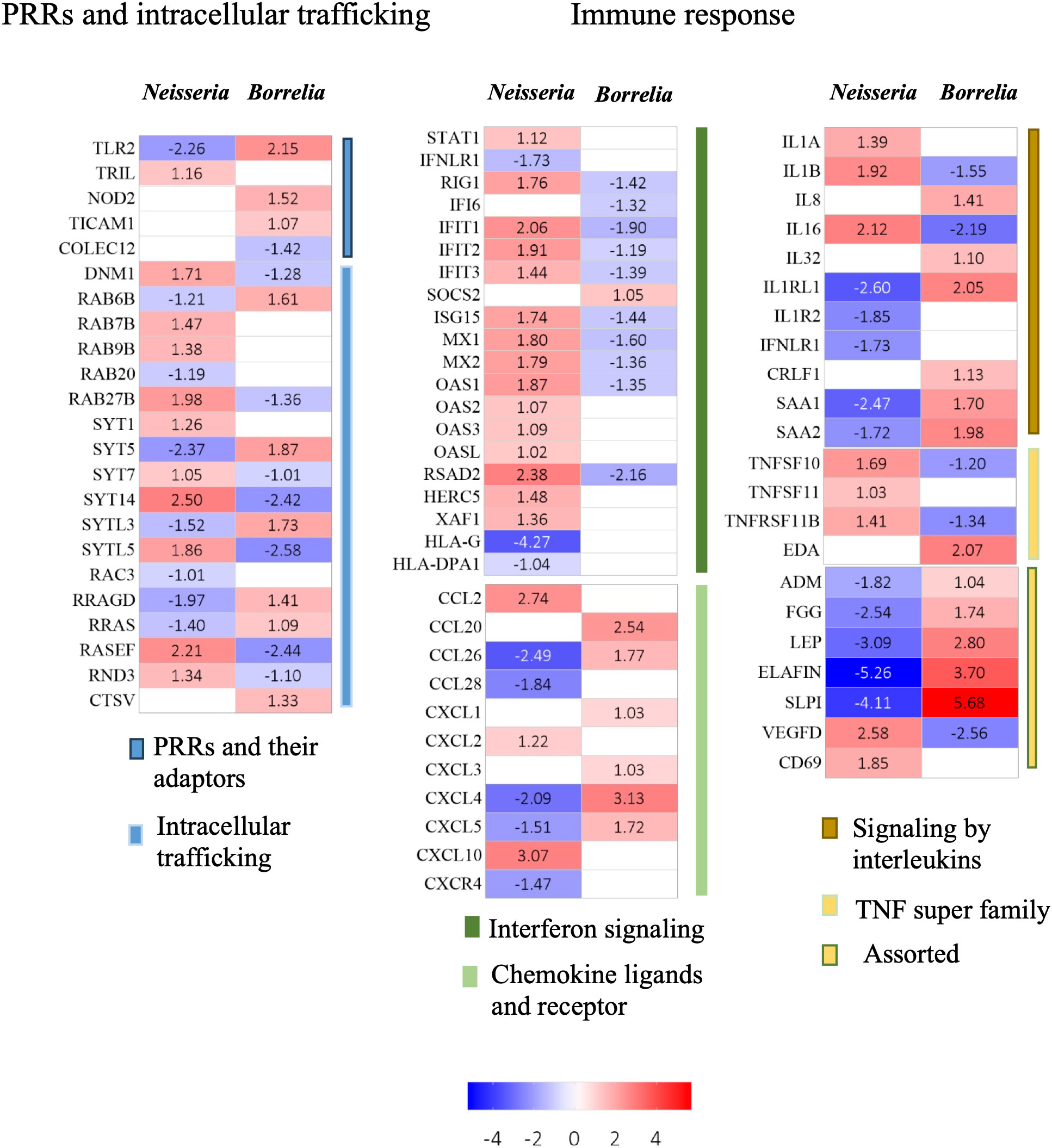
Figure 6 Heatmaps for DEGs. Heatmaps depicting Log2FC values of DEGs identified in BBB spheroids undergoing Neisseria or Borrelia infection. DEGs are categorized into biological functions – PRRS and intracellular trafficking, and immune response. Color scale presents Log2FC values.
3.9 Immune response
At least 56 genes differentially evoked in infected spheroids were related to immune response (Figure 6; Supplementary Table 3).
3.9.1 Pattern recognition receptors
Molecular patterns of both Neisseria and Borrelia are recognized by members of the family of Toll like receptors, Nod like receptors, C type of lectins, scavenger receptors and integrins (Petnicki-Ocwieja and Kern, 2014) Across all of these receptors and their associated molecules, only five genes changed their expression significantly viz., TLR2, TLR4 interactor with leucine-rich repeats (TRIL4), nucleotide binding oligomerization domain containing 2 (NOD2), TIR domain containing adaptor molecule 1 (TICAM1), and collectin 12 (COLEC12). Surprisingly, the two subunits of NFKB – NFkB1 and NFkB 2 were not differentially expressed in spheroids undergoing Neisseria (Log2FC 0.61, -0.26) and Borrelia (Log2FC -0.030, 0.44) infection.
3.9.2 Interleukins and chemokines
In response to infection, four interleukins (IL1A, IL1B, IL8 and IL16), their receptors (IL1 receptor like 1, IL1 receptor 2, IFN-lambda receptor 1, cytokine receptor like factor 1) and two acute phase proteins (Serum amyloid A 1 and 2) were differentially expressed. IL-1A can induce the pro-angiogenic cytokines e.g., CXCL1 in brain endothelial cells, while IL1B can induces expression of C-X-C chemokines, adhesion molecules, and increases vascular permeability (Makó et al., 2010; Dinarello, 2018). Overexpressed IL1A (Log2FC 1.39) and IL1B (Log2FC 1.92) in spheroids infected with Neisseria indicates inflammatory response that could compromise the BBB integrity. In the case of Borrelia infection, IL1B was downregulated (Log2FC -1.55), however, a proinflammatory cytokine IL32 (Log2FC 1.09), a receptor IL1RL1 receptor (Log2FC 2.05) and IL8 (Log2FC 1.40) were upregulated. The acute phase SAA1 and SAA2 proteins, which also possess cytokine-like properties, had Log2FC as 1.70 and 1.98 in Borrelia infected spheroids respectively. SAA may stimulate the production and release of matrix metalloproteases (like MMP9 Log2FC 1.68), cytokines and chemokines (such as IL8, Log2FC 1.69). On the other hand, both SAA1 (Log2FC -2.47) and SAA2 (Log2FC -1.72) were downregulated during Neisseria infection.
Some of members of CC and CXC chemokines were differentially expressed in infected spheroids. Upregulated C-X-C motif chemokine ligand 2 (CXCL2, Log2FC 1.21), CXCL10 (Log2FC 3.06) and C-C motif chemokine ligand (CCL2, Log2FC 2.74) during Neisseria infection are important to encourage chemotaxis of neutrophiles, Th1 cells, and monocytes, respectively. Similarly, in spheroids infected with Borrelia, upregulated CCL20 (Log2FC 2.54), CXCL1 (Log2FC 1.02), CXCL3 (Log2FC 1.03), CCL26 (Log2FC 1.77), CXCL5 (Log2FC 1.71), and PF4 (Log2FC 3.13) can increase the chemotaxics of dendritic cells, lymphocytes, neutrophils, and eosinophils. Due to the presence of an amphipathic -helix domain or a large patch of positively charged amino acids at the C-terminus, chemokines CXCL10, CCL20, and CCL26 can also act as broad spectrum anti-microbials.
CXCR4 (Log2FC -1.46) was the only chemokine receptor downregulated during Neisseria infection. As a result, CXCR4 mediated signaling cascade leading to cell proliferation, differentiation, apoptosis and angiogenesis could be hampered during Neisseria infection.
3.9.3 Members of TNF superfamily
TNF superfamily members (TNFSF10, 11, 11b) and ectodysplasin A (EDA) were differentially expressed during Neisseria or Borrelia infection. Upregulation of TNFSF10 (Log2FC 1.69) during Neisseria infection could promote the induction of JNK or NF-kB mediated inflammatory response or apoptosis. On the other hand, TNFSF10, was downregulated during Borrelia infection (Log2FC -1.20). TNFSF11 (Log2FC 1.03) and TNFRSF11B (Log2FC 1.41) upregulated during Neisseria infection, could induce CCL20 in astrocytes and promote endothelial cell angiogenesis. In spheroids infected with Borrelia, TNFRSF11B was downregulated (Log2FC -1.33) and ectodysplasin A (EDA; Log2FC 2.07), was upregulated. Ectodysplasin A can positively regulate the expression of ZO-1 and claudin-1, which is necessary to maintain the barrier function. Whether the upregulated EDA (Log2FC 2.07) and overexpressed tight junction proteins – F11R (Log2FC 1.42) and CLDN7 (Log2FC 1.28) during borrelial infection are interdependent to limit the endothelial barrier disfunction needs further confirmation.
3.9.4 Interferon signaling
Although interferons are produced in response to viral infections, they can also have a limited antibacterial effect, such as inhibiting bacterial migration across endothelial and epithelial barriers (LeMessurier et al., 2013) Prolonged interferon response, on the other hand, can impair cell barrier function (Major et al., 2020). In the present study, signal transducer STAT1, DExD/H-box helicase 58 (RIG1) and several IFN stimulated genes (ISGs) namely IFNα inducible protein 6 (IFI6), Interferon induced protein with tetratricopeptide repeats (IFIT) 1, 2 and 3, ISG15 ubiquitin like modifier (ISG15), HECT and RLD domain containing E3 ubiquitin protein ligase 5 (HERC5), MX dynamin like GTPases (MX 1 and 2), 2’-5’-oligoadenylate synthetase (OAS 1,2 and 3) and OAS-like protein (OASL) were upregulated during Neisseria infection and their expression was downregulated during borrelial infection (Figure 6; Supplementary Table 3). On the other hand, the receptor for type III IFN, Interferon lambda receptor 1 (IFNLR1; Log2FC -1.73) was downregulated during Neisseria infection which suggests the repression of IFN -λ signaling. The nonclassical human leukocyte antigen G (HLA-G) was strongly downregulated during neisserial infection (Log2FC -4.27) (Figure 6; Supplementary Table 3).
3.9.5 Other important genes
VEGFs, specifically VEGF-A, VEGF-B, VEGF-C, and VEGF-D, as well as their receptors (tyrosin kinase VEGF-R1-3), are important regulators of angiogenesis. VEGF-D expression was found to be upregulated (Log2FC 2.58) during Neisseria infection but downregulated (Log2FC -2.56) during Borrelia infection. Because VEGF-D induces the formation of intercellular gaps (Wong et al., 2011), it is possible that the barrier function might be compromised during Neisseria infection. Adrenomedullin (ADM) and leptin, both required for endothelial barrier maintenance, were downregulated during Neisseria infection (ADM Log2FC -1.81, leptin Log2FC -3.08), however their expression levels were increased during Borrelia infection (ADM Log2FC 1.04, leptin Log2FC 2.80) (Figure 6; Supplementary Table 3).
4 Discussion
A plethora of molecular cross talk occurs between the pathogens and the cells of BBB during its invasion. The Cross talk includes ligand-receptor interactions, cell signaling, host cell responses to resist pathogen invasion, etc. (Kánová et al., 2018; Káňová et al., 2019; Thompson et al., 2020; Tkáčová et al., 2020; Tkáčová et al., 2021). Earlier studies describing the BBB response to invading neuropathogens have focused on BMECs, the barrier’s frontline cells. Nonetheless, it is clear that other cells of the neurovascular unit (pericytes and astrocytes) are equally important in maintaining cell-cell/cell-extracellular matrix interactions and governing the BBB functions. Moreover, studies in the recent past have shown that BBB spheroids are convenient to study brain infections like - cerebral malaria (Adams et al., 2021), fungal meningitis (Kim et al., 2021) and toxoplasmosis (Correa Leite et al., 2021). In the current study, spheroids composed of hBMCEs, pericytes, and astrocytes were used to understand the barrier’s response (via transcriptomic analysis) to neuroinvasive Neisseria or Borrelia at 3 h post infection in order to capture the events leading to BBB breakdown (Figures 3E, J).
Although both Neisseria and Borrelia are neuroinvasive, they differ significantly in terms of latency time, virulence factors, strategies used to breach the BBB, etc. Capsular polysaccharides, lipo-oligosaccharides, iron-binding proteins, porins, major adhesins (pili, outer-membrane proteins), and phase variable minor adhesins are virulence factors for Neisseria (Herold et al., 2019). Borrelia, on the other hand, lacks an LPS and iron uptake system but does possess ECM binding, ECM degrading and complement evading lipoproteins that can undergo antigenic variation (Johnson, 2018). While crossing the BBB, Neisseria promotes formation of cortical plaques and delocalizes adherence and tight junction proteins (Miller et al., 2013), whereas Borrelia activates CD40-mediated signaling cascade and the host’s fibrinolytic system, metalloproteases and proinflammatory cytokines, which may result in fenestrations in hBMECs and transient degradation of tight junction proteins (Grab et al., 2005; Pulzova et al., 2011). Given the differences, we hypothesized that the BBB’s response to both pathogens would differ significantly. The inverse expression of 455 genes in spheroids infected with Neisseria or Borrelia (Figure 4D) supports our assertion.
TLRs, NOD-like receptors, C type lectins, scavenger receptors, integrins, and other PRRs should be activated by Neisseria and Borrelia (Petnicki-Ocwieja and Kern, 2014; Johswich, 2017). However, only 5 PPR-related genes were found to be differentially expressed (Figure 6), namely TLR2, NOD2, and COLEC12 (receptors), TRIL (the modulator of TLR3/4 signaling), and TICAM-1 (the adaptor molecule of TLR3 signaling). In contrast to previous findings (Káňová et al., 2019; Tkáčová et al., 2021), the TLR2 adaptor molecule MyD88 and the transcription regulator NFĸB were not found to be significantly expressed in the current study. The inclusion of pericytes and astrocytes in this study, as opposed to only hBMECs in previous studies, may explain the variation. The same reason could be behind the elevated expression of ICAMs, VCAMS, CD44, E-selectin, and F11R in the monolayer of hBMEC challenged with Neisseria (Log2FC ≥ 5) (Káňová et al., 2019) as oppose to marginal upregulation of VCAM1 (Log2FC 1.82) and rather down regulation of ICAM 3 (Log2FC -1.18), ICAM 5 (Log2FC -2.13), and F11R (Log2FC -1.21) in the present study. Initial colonization of Neisseria on hBMECs (Figure 3B) may result in abnormal recruitment of polarity complex molecules and adherence junction proteins beneath the bacteria (Miller et al., 2013). Cadherins, ICAMs, and VCAMS, adhesion junction proteins, relocate to the apical regions of hBMECs to form docking structures or transmigratory cups (Doulet et al., 2006). The upregulation of cadherins and VCAM1 in spheroids infected with Neisseria may be in response to abnormal translocation (Figure 5 and Supplementary Table 3). No such translocation is expected during Borrelia infection as the same cadherin genes were under expressed (Figure 5 and Supplementary Table 3). Tight junction proteins like F11R and CLDN7 are required to maintain barrier function, and their downregulation during Neisseria infection may result in transient intercellular junction opening. Despite the fact that F11R and CLDN7 were upregulated during Borrelia infection, the other three tight junction proteins, MXRA8, PARD3B, and EMB, were downregulated. Along with the dysregulation of tight junction proteins, focal adhesion molecules like integrins that are required to anchor cells to the extracellular matrix had altered expression. Integrin subunits such as L1CAM, SPP1, and FBLN7, for example, were downregulated during Neisseria infection. The downregulation of integrins may have an adverse effect on cell attachment to ECM. Compromised BBB integrity was observed in both Neisseria and Borrelia infections (Figures 3E, J).
Extracellular matrix related protein like MMPs and ADAMTS are secreted in response to the exogenous insults such as invading pathogens. ADAMTS and MMPs can degrade glycocalyx and ECM components, which can result in BBB breakdown, inflammatory cell infiltration, and cytokine signalling dysregulation (Elkington et al., 2005; Muri et al., 2019). In the context of increased permeability of infected spheroids, upregulation of MMPs (MMP 2, 11,13 and 16 in Neisseria and MMP1 and 9 in Borrelia) and ADAMTS (ADAMTS5, 7 and 14 in Neisseria and ADAMTS1, 5 and 14 in Borrelia) is relevant observation. It is also important to note the reduced expression of some of the members of MMP family especially MMP1 and 9 in Neisseria and MMP2 and 13 in Borrelia infected spheroids. The reduced expression of metalloproteinases during pathogen invasion may be harmful to the host. Other ECM related genes such as collagen synthesizers and lysyl oxidase family members are known to maintain the homeostasis of ECM by stabilizing the elastin and collagen fibres. Additionally they act as chemoattractant for peripheral blood mononuclear cells and inhibit the nuclear translocation of NF-κB (Lucero and Kagan, 2006). It would be interesting to determine if, downregulation of LOX and LOXL genes observed during Neisseria infection signals for enhancement in NF-κB dependent inflammatory response at BBB. Since Borrelia can damage the collagen and elastic fibres it is necessary to determine if overexpressed LOX and LOXL genes during Borrelia infection is host response to damage.
Genes encoding ligands, receptors, signal transducers and transcription factors of TGF-β were mostly upregulated during Neisseria infection. However, the same set of genes were downregulated during borrelial infection (Figure 5; Supplementary Table 3). TGF-β signaling is a context dependent mechanism that can regulate essential biological process such as proliferation and ECM synthesis/degradation (Santibañez et al., 2011). In the context of present study, we predict that TGF-β signaling may have influenced the expression of MMPs as described previously (Schumacher et al., 2023). Several MMPs (at least MMP1, 7, 9, 13 and 14) are known to possess two regulatory domains - TGF-β inhibitory element and Smad binding element (SBE) and therefore, TGF-β can regulate the expression and secretion MMPs. Additionally, TGF-β can activate the transcription factors like AP1, PEA3 and NFκB that can control the MMP expression (Krstic and Santibanez, 2014). In short, the genes involved in TGF signalling are worth investigating in the context of BBB integrity. Another signalling pathway, the Wnt signalling, is important as far as BBB homeostasis is concern. It has been demonstrate that inhibiting Wnt/β-catenin signalling can result in severe defects in brain endothelial cell angiogenesis and breakdown of BBB caused by under expression of tight junction proteins such as claudin (Liebner et al., 2008; Daneman et al., 2009). Several molecules in this pathway including WNT7A, WNT7B, WNT3A, β-catenin, and others, are important in maintaining endothelial cell phenotype (Liebner et al., 2008; Daneman et al., 2009). During Neisseria infection, the Wnt signalling pathway appears to be inhibited, as we observed upregulation of 5 inhibitors and downregulation of cycline D2, a cell cycle progression effector molecule (Figure 5). It appears that Neisseria and Borrelia elicit Wnt signaling pathways differently, as the same set of Wnt/β -catenin inhibitors were downregulated during borrelial infection.
Both Neisseria and Borrelia infections elicited proinflammatory response in spheroids (Figure 6) in line with previous findings in BMECs (Káňová et al., 2019; Tkáčová et al., 2021), pericytes (Butsabong et al., 2021; Gil et al., 2022) and astrocytes (Casselli et al., 2017; Geyer et al., 2019). Upregulation of interferon signaling genes (e.g. STAT1, IFIT 1-3 and ISG15, MX 1 - 2 and OAS 1-3 etc), chemokines (eg. CXCL2, CXCL10 and CCL2), interleukins (eg. IL1A, IL1B, IL16) and members of TNF superfamily (TNFSF10 and TNFSF11) can promote secretion of proinflammatory cytokines and MMPs (Kim et al., 2005; Ovstebø et al., 2008; Madhvi et al., 2022), interfere in pathogen replication by either inducing apoptosis or ubiquitin mediated proteasomal degradation (Zhang et al., 2021), induce chemotaxis of leucocytes (Dinarello, 2018) and directly act as antimicrobials (Yang et al., 2003). To contain the bacterial infection, which has a short doubling time (Neisseria has only 30 min), an aggravated inflammatory response is essential; however, at the BBB level, such response may compromise barrier function. Along with other proinflammatory genes, elevated levels of VEGFD (Log2FC 2.57), IL1B (Log2FC 1.92), MMP-2 (Log2FC 1.37) and MMP13 (Log2FC 3.94) indicate that these molecules may have contributed in increased BBB permeability observed in spheroids. Acute meningitis and altered BBB permeability are common in meningococcal infection patients, but not in neuroborreliosis patients. In spheroids challenged with Borrelia interferon related genes (IFIT1-3, IFI6, MX1-2, ISG15) and IL1B were downregulated, while expression of suppressors of cytokine signalling, such as SOCS2, was elevated, which clearly denotes the difference in interferon signalling elicitation by two pathogens (Figure 6).
5 Conclusion
Self-assembling spheroids comprised of hBMECs, pericytes and astrocytes were cultured to perceive infection of BBB by neuroinvasive Neisseria and Borrelia. Both the pathogens invaded the BBB spheroids showing adherence with in 1h, followed by massive aggregation until 24 h after exposure. Non-neuroinvasive E. coli, on the other hand, did not adhere to the spheroids. The invasion of Neisseria and Borrelia resulted in complete loss of spheroid integrity within 3 h. The transcriptome analysis revealed underlying molecular events that are responsible for the weakening of the barrier function during Neisseria and Borrelia infection. Although both Neisseria and Borrelia increased barrier permeability and altered overall gene expression significantly, 48% of the DEGs were inversely expressed in spheroids infected with Neisseria and Borrelia. The findings show that, while both Neisseria and Borrelia can successfully cross the BBB, they elicit the response in the neurovascular unit in very distinct ways. Some of the highly expressed genes (Log2 FC > 3.5) in inverse manner during Neisseria and Borrelia like HAPLN1, MT3, PITX2, ID4, ELAFIN, SLPI, etc., could be targeted to know their relevance in favoring or resisting the infection. Likewise, role of TGF-β and WNT signaling pathways during Neisseria and Borrelia infection at BBB needs further exploration.
Data availability statement
The data presented in the study are deposited in the EBI Arrayexpress repository, accession number E-MTAB-13401.
Ethics statement
Ethical approval was not required for the studies on humans in accordance with the local legislation and institutional requirements because only commercially available established cell lines were used. Ethical approval was not required for the studies on animals in accordance with the local legislation and institutional requirements because only commercially available established cell lines were used.
Author contributions
AK: Conceptualization, Funding acquisition, Methodology, Visualization, Writing – original draft. JJ: Methodology, Writing – review & editing. KB: Methodology, Writing – review & editing. EM: Funding acquisition, Writing – review & editing. MB: Funding acquisition, Conceptualization, Supervision, Writing – review & editing.
Funding
The author(s) declare financial support was received for the research, authorship, and/or publication of this article. Authors AK and EM are supported by EURONANOMED2021-105 (Antineuropatho) and OPENMED-NFP313010V455, respectively. Research was funded from the projects APVV-22-0084, VEGA 1/0348/22, 2/0128/21 and 1/0381/23.
Acknowledgments
We thank the CF Genomics CEITEC MU supported by the NCMG research infrastructure (LM2015091 funded by MEYS CR) for their support to obtain RNAseq data. We acknowledge, Prof. Zuzana Ševčiková, Department of Morphological Disciplines, UVLF, Košice, Slovakia for their support to obtain cryosections of spheroids.
Conflict of interest
The authors declare that the research was conducted in the absence of any commercial or financial relationships that could be construed as a potential conflict of interest.
Publisher’s note
All claims expressed in this article are solely those of the authors and do not necessarily represent those of their affiliated organizations, or those of the publisher, the editors and the reviewers. Any product that may be evaluated in this article, or claim that may be made by its manufacturer, is not guaranteed or endorsed by the publisher.
Supplementary material
The Supplementary Material for this article can be found online at: https://www.frontiersin.org/articles/10.3389/fcimb.2023.1326578/full#supplementary-material
Supplementary Image 1 | 3D confocal images of spheroid showing the localization of three cell types. Cryosection of spheroid used to generate Figure 1, panel E-H was scanned by LSM700 to depict the localization of prestained hBMEC (red fluorescence), pericytes (green fluorescence) and astrocytes (blue fluroscence). The Z-stack images are arranged in 3D format. It is advised to use ImageJ (https://imagej.net/ij/) for proper visualization of fluorescent signals.
Supplementary Image 2 | Confocal microscopy image of spheroid generated by co-culturing prestained cells. Cryosection of spheroid used to generate Figure 1, panel E-H was scanned by LSM700 to depict the localization of prestained hBMEC (red fluorescence), pericytes (green fluorescence) and astrocytes (blue fluroscence). The Z projections in X-Z directions are shown in top and right panel. The orthogonal planes of the X–Z and Y–Z projection are shown by the green and red lines respectively. It is advised to use ImageJ (https://imagej.net/ij/) for proper visualization of fluorescent signals.
References
Abbott, N. J., Patabendige, A. A. K., Dolman, D. E. M., Yusof, S. R., Begley, D. J. (2010). Structure and function of the blood–brain barrier. Neurobiol. Dis. 37 (1), 13–25. doi: 10.1016/j.nbd.2009.07.030
Adams, Y., Olsen, R. W., Bengtsson, A., Dalgaard, N., Zdioruk, M., Satpathi, S., et al. (2021). Plasmodium falciparum erythrocyte membrane protein 1 variants induce cell swelling and disrupt the blood–brain barrier in cerebral malaria. J. Exp. Med. 218 (3), e20201266. doi: 10.1084/jem.20201266
Al-Obaidi, M. M. J., Desa, M. N. M. (2018). Mechanisms of blood brain barrier disruption by different types of bacteria, and bacterial–host interactions facilitate the bacterial pathogen invading the brain. Cell. Mol. Neurobiol. 38 (7), 1349–1368. doi: 10.1007/s10571-018-0609-2
Antonetti, D. A., Barber, A. J., Hollinger, L. A., Wolpert, E. B., Gardner, T. W. (1999). Vascular endothelial growth factor induces rapid phosphorylation of tight junction proteins occludin and zonula occluden 1. J. Biol. Chem. 274 (33), 23463–23467. doi: 10.1074/jbc.274.33.23463
Armulik, A., Genové, G., Mäe, M., Nisancioglu, M. H., Wallgard, E., Niaudet, C., et al. (2010). Pericytes regulate the blood–brain barrier. Nature 468 (7323), 557–561. doi: 10.1038/nature09522
Bencurova, E., Mlynarcik, P., Bhide, M. (2011). An insight into the ligand–receptor interactions involved in the translocation of pathogens across blood–brain barrier. FEMS Immunol. Med. Microbiol. 63 (3), 297–318. doi: 10.1111/j.1574-695x.2011.00867.x
Bergmann, S., Lawler, S. E., Qu, Y., Fadzen, C. M., Wolfe, J. M., Regan, M. S., et al. (2018). Blood-brain-barrier organoids for investigating the permeability of CNS therapeutics. Nat. Protoc. 13 (12), 2827–2843. doi: 10.1038/s41596-018-0066-x
Bhide, K., Mochnáčová, E., Tkáčová, Z., Petroušková, P., Kulkarni, A., Bhide, M. (2022). Signaling events evoked by domain III of envelop glycoprotein of tick-borne encephalitis virus and West Nile virus in human brain microvascular endothelial cells. Sci. Rep. 12 (1), 8863–8863. doi: 10.1038/s41598-022-13043-1
Brissette, C. A., Kees, E. D., Burke, M. M., Gaultney, R. A., Floden, A. M., Watt, J. A. (2013). The multifaceted responses of primary human astrocytes and brain microvascular endothelial cells to the Lyme disease spirochete, Borrelia burgdorferi. ASN Neuro 5 (3), 221–229. doi: 10.1042/AN20130010
Butsabong, T., Felippe, M., Campagnolo, P., Maringer, K. (2021). The emerging role of perivascular cells (pericytes) in viral pathogenesis. J. Gen. Virol. 102 (8), 1634. doi: 10.1099/jgv.0.001634
Carey, M. A., Ho, E. S. (2017). A transcriptome study of Borrelia burgdorferi infection in murine heart and brain tissues. J. Young Invest. 33 (1), 28–41. doi: 10.22186/jyi.33.1.28-41
Casselli, T., Qureshi, H., Peterson, E., Perley, D., Blake, E., Jokinen, B., et al. (2017). MicroRNA and mRNA transcriptome profiling in primary human astrocytes infected with Borrelia burgdorferi. PloS One 12 (1), e0170961–e0170961. doi: 10.1371/journal.pone.0170961
Cho, C.-F., Wolfe, J. M., Fadzen, C. M., Calligaris, D., Hornburg, K., Chiocca, E. A., et al. (2017). Blood-brain-barrier spheroids as an in vitro screening platform for brain-penetrating agents. Nat. Commun. 8, 15623–15623. doi: 10.1038/ncomms15623
Comor, L., Dolinska, S., Bhide, K., Pulzova, L., Jiménez-Munguía, I., Bencurova, E., et al. (2017). Joining the in vitro immunization of alpaca lymphocytes and phage display: rapid and cost effective pipeline for sdAb synthesis. Microb. Cell Factories 16 (1), 13–13. doi: 10.1186/s12934-017-0630-z
Comstock, L. E., Thomas, D. D. (1989). Penetration of endothelial cell monolayers by Borrelia burgdorferi. Infect. Immun. 57 (5), 1626–1628. doi: 10.1128/iai.57.5.1626-1628.1989
Correa Leite, P. E., de Araujo Portes, J., Pereira, M. R., Russo, F. B., Martins-Duarte, E. S., Almeida dos Santos, N., et al. (2021). Morphological and biochemical repercussions of Toxoplasma gondii infection in a 3D human brain neurospheres model. Brain Behav. Immun. Health 11, 100190. doi: 10.1016/j.bbih.2020.100190
Coureuil, M., Join-Lambert, O., Lécuyer, H., Bourdoulous, S., Marullo, S., Nassif, X. (2012). Mechanism of meningeal invasion by Neisseria meningitidis. Virulence 3 (2), 164–172. doi: 10.4161/viru.18639
Coureuil, M., Lécuyer, H., Bourdoulous, S., Nassif, X. (2017). A journey into the brain: insight into how bacterial pathogens cross blood–brain barriers. Nat. Rev. Microbiol. 15 (3), 149–159. doi: 10.1038/nrmicro.2016.178
Coureuil, M., Lécuyer, H., Scott, M. G. H., Boularan, C., Enslen, H., Soyer, M., et al. (2010). Meningococcus hijacks a β2-adrenoceptor/β-arrestin pathway to cross brain microvasculature endothelium. Cell 143 (7), 1149–1160. doi: 10.1016/j.cell.2010.11.035
Daneman, R., Agalliu, D., Zhou, L., Kuhnert, F., Kuo, C. J., Barres, B. A. (2009). Wnt/beta-catenin signaling is required for CNS, but not non-CNS, angiogenesis. Proc. Natl. Acad. Sci. U. States America 106 (2), 641–646. doi: 10.1073/pnas.0805165106
Dinarello, C. A. (2018). Overview of the IL-1 family in innate inflammation and acquired immunity. Immunol. Rev. 281 (1), 8–27. doi: 10.1111/imr.12621
Divan, A., Casselli, T., Narayanan, S. A., Mukherjee, S., Zawieja, D. C., Watt, J. A., et al. (2018). Borrelia burgdorferi adhere to blood vessels in the dura mater and are associated with increased meningeal T cells during murine disseminated borreliosis. PloS One 13 (5), e0196893–e0196893. doi: 10.1371/journal.pone.0196893
Doulet, N., Donnadieu, E., Laran-Chich, M.-P., Niedergang, F., Nassif, X., Couraud, P. O., et al. (2006). Neisseria meningitidis infection of human endothelial cells interferes with leukocyte transmigration by preventing the formation of endothelial docking structures. J. Cell Biol. 173 (4), 627–637. doi: 10.1083/jcb.200507128
Elkington, P. T. G., O’Kane, C. M., Friedland, J. S. (2005). The paradox of matrix metalloproteinases in infectious disease. Clin. Exp. Immunol. 142 (1), 12–20. doi: 10.1111/j.1365-2249.2005.02840.x
Esser, S., Lampugnani, M. G., Corada, M., Dejana, E., Risau, W. (1998). Vascular endothelial growth factor induces VE-cadherin tyrosine phosphorylation in endothelial cells. J. Cell Sci. 111 (13), 1853–1865. doi: 10.1242/jcs.111.13.1853
Gastfriend, B. D., Palecek, S. P., Shusta, E. V. (2018). Modeling the blood-brain barrier: beyond the endothelial cells. Curr. Opin. Biomed. Eng. 5, 6–12. doi: 10.1016/j.cobme.2017.11.002
Geyer, S., Jacobs, M., Hsu, N.-J. (2019). Immunity against bacterial infection of the central nervous system: an astrocyte perspective. Front. Mol. Neurosci. 12. doi: 10.3389/fnmol.2019.00057
Gil, E., Venturini, C., Stirling, D., Turner, C., Tezera, L. B., Ercoli, G., et al. (2022). Pericyte derived chemokines amplify neutrophil recruitment across the cerebrovascular endothelial barrier. Front. Immunol. 13. doi: 10.3389/fimmu.2022.935798
Gottesman, S., Halpern, E., Trisler, P. (1981). Role of sulA and sulB in filamentation by lon mutants of Escherichia coli K-12. J. Bacteriol. 148 (1), 265–273. doi: 10.1128/jb.148.1.265-273.1981
Grab, D. J., Nyarko, E., Nikolskaia, O. V., Kim, Y. V., Dumler, J. S. (2009). Human brain microvascular endothelial cell traversal by Borrelia burgdorferi requires calcium signaling. Clin. Microbiol. Infect. 15 (5), 422–426. doi: 10.1111/j.1469-0691.2009.02869.x
Grab, D. J., Perides, G., Dumler, J. S., Kim, K. J., Park, J., Kim, Y. V., et al. (2005). Borrelia burgdorferi, host-derived proteases, and the blood-brain barrier. Infect. Immun. 73 (2), 1014–1022. doi: 10.1128/IAI.73.2.1014-1022.2005
Herold, R., Schroten, H., Schwerk, C. (2019). Virulence factors of meningitis-causing bacteria: enabling brain entry across the blood-brain barrier. Int. J. Mol. Sci. 20 (21), 5393. doi: 10.3390/ijms20215393
Herold, R., Sünwoldt, G., Stump-Guthier, C., Weiss, C., Ishikawa, H., Schroten, H., et al. (2021). Invasion of the choroid plexus epithelium by Neisseria meningitidis is differently mediated by Arp2/3 signaling and possibly by dynamin dependent on the presence of the capsule. Pathog. Dis. 79 (7), ftab042. doi: 10.1093/femspd/ftab042
Hruškovicová, J., Bhide, K., Petroušková, P., Tkáčová, Z., Mochnáčová, E., Čurlík, J., et al. (2022). Engineering the single domain antibodies targeting receptor binding motifs within the domain III of West Nile virus envelope glycoprotein. Front. Microbiol. 13. doi: 10.3389/fmicb.2022.801466
Iliff, J. J., Wang, M., Liao, Y., Plogg, B. A., Peng, W., Gundersen, G. A., et al. (2012). A paravascular pathway facilitates CSF flow through the brain parenchyma and the clearance of interstitial solutes, including amyloid β. Sci. Trans. Med. 4 (147), 147ra111–147ra111. doi: 10.1126/scitranslmed.3003748
Jessen, N. A., Munk, A. S. F., Lundgaard, I., Nedergaard, M. (2015). The glymphatic system: a beginner’s guide. Neurochem. Res. 40 (12), 2583–2599. doi: 10.1007/s11064-015-1581-6
Johnson, D. I. (2018). “Borrelia spp,” in Bacterial pathogens and their virulence factors. Ed. Johnson, D. I. (Cham: Springer International Publishing), 191–197.
Johswich, K. (2017). Innate immune recognition and inflammation in Neisseria meningitidis infection. Pathog. Dis. 75 (2), ftx022. doi: 10.1093/femspd/ftx022
Kanekiyo, M., Itoh, N., Kawasaki, A., Matsuyama, A., Matsuda, K., Nakanishi, T., et al. (2002). Metallothionein modulates lipopolysaccharide-stimulated tumour necrosis factor expression in mouse peritoneal macrophages. Biochem. J. 361 (2), 363–369. doi: 10.1042/bj3610363
Kánová, E., Jiménez-Munguía, I., Majerová, P., Tkáčová, Z., Bhide, K., Mertinková, P., et al. (2018). Deciphering the interactome of Neisseria meningitidis with human brain microvascular endothelial cells. Front. Microbiol. 9. doi: 10.3389/fmicb.2018.02294
Káňová, E., Tkáčová, Z., Bhide, K., Kulkarni, A., Jiménez-Munguía, I., Mertinková, P., et al. (2019). Transcriptome analysis of human brain microvascular endothelial cells response to Neisseria meningitidis and its antigen MafA using RNA-seq. Sci. Rep. 9 (1), 18763–18763. doi: 10.1038/s41598-019-55409-y
Kim, J., Lee, K.-T., Lee, J. S., Shin, J., Cui, B., Yang, K., et al. (2021). Fungal brain infection modelled in a human-neurovascular-unit-on-a-chip with a functional blood–brain barrier. Nat. Biomed. Eng. 5 (8), 830–846. doi: 10.1038/s41551-021-00743-8
Kim, K. I., Malakhova, O. A., Hoebe, K., Yan, M., Beutler, B., Zhang, D.-E. (2005). Enhanced Antibacterial Potential in UBP43-deficient mice against Salmonella typhimurium infection by up-regulating type I IFN signaling. J. Immunol. 175 (2), 847–854. doi: 10.4049/jimmunol.175.2.847
Krstic, J., Santibanez, J. F. (2014). Transforming growth factor-beta and matrix metalloproteinases: functional interactions in tumor stroma-infiltrating myeloid cells. Sci. World J. 2014, 521754–521754. doi: 10.1155/2014/521754
Kumarasamy, M., Sosnik, A. (2021). Heterocellular spheroids of the neurovascular blood-brain barrier as a platform for personalized nanoneuromedicine. iScience 24 (3), 102183–102183. doi: 10.1016/j.isci.2021.102183
LeMessurier, K. S., Häcker, H., Chi, L., Tuomanen, E., Redecke, V. (2013). Type I interferon protects against pneumococcal invasive disease by inhibiting bacterial transmigration across the lung. PloS Pathog. 9 (11), e1003727–e1003727. doi: 10.1371/journal.ppat.1003727
Liao, Y., Smyth, G. K., Shi, W. (2013). featureCounts: an efficient general purpose program for assigning sequence reads to genomic features. Bioinformatics 30 (7), 923–930. doi: 10.1093/bioinformatics/btt656
Liebner, S., Corada, M., Bangsow, T., Babbage, J., Taddei, A., Czupalla, C. J., et al. (2008). Wnt/beta-catenin signaling controls development of the blood-brain barrier. J. Cell Biol. 183 (3), 409–417. doi: 10.1083/jcb.200806024
Love, M. I., Huber, W., Anders, S. (2014). Moderated estimation of fold change and dispersion for RNA-seq data with DESeq2? Genome Biol. 15 (12), 1–21. doi: 10.1186/s13059-014-0550-8
Lucero, H. A., Kagan, H. M. (2006). Lysyl oxidase: an oxidative enzyme and effector of cell function. Cell. Mol. Life Sci. 63 (19-20), 2304–2316. doi: 10.1007/s00018-006-6149-9
Madhvi, A., Mishra, H., Chegou, N. N., Baker, B. (2022). Increased interferon-induced protein with tetracopeptides (IFITs) reduces mycobacterial hrowth. Front. Cell. Infect. Microbiol. 12. doi: 10.3389/fcimb.2022.828439
Major, J., Crotta, S., Llorian, M., McCabe, T. M., Gad, H. H., Priestnall, S. L., et al. (2020). Type I and III interferons disrupt lung epithelial repair during recovery from viral infection. Sci. (New York N.Y.) 369 (6504), 712–717. doi: 10.1126/science.abc2061
Makó, V., Czúcz, J., Weiszhár, Z., Herczenik, E., Matkó, J., Prohászka, Z., et al. (2010). Proinflammatory activation pattern of human umbilical vein endothelial cells induced by IL-1β, TNF-α, and LPS. Cytometry Part A 77A (10), 962–970. doi: 10.1002/cyto.a.20952
Martins Gomes, S. F., Westermann, A. J., Sauerwein, T., Hertlein, T., Förstner, K. U., Ohlsen, K., et al. (2019). Induced pluripotent stem cell-derived brain endothelial cells as a cellular model to study Neisseria meningitidis infection. Front. Microbiol. 10. doi: 10.3389/fmicb.2019.01181
Miller, F., Lécuyer, H., Join-Lambert, O., Bourdoulous, S., Marullo, S., Nassif, X., et al. (2013). Neisseria meningitidis colonization of the brain endothelium and cerebrospinal fluid invasion. Cell. Microbiol. 15 (4), 512–519. doi: 10.1111/cmi.12082
Muri, L., Leppert, D., Grandgirard, D., Leib, S. L. (2019). MMPs and ADAMs in neurological infectious diseases and multiple sclerosis. Cell. Mol. Life Sci.: CMLS 76 (16), 3097–3116. doi: 10.1007/s00018-019-03174-6
Nassif, X., Bourdoulous, S., Eugène, E., Couraud, P.-O. (2002). How do extracellular pathogens cross the blood–brain barrier? Trends Microbiol. 10 (5), 227–232. doi: 10.1016/s0966-842x(02)02349-1
Orihuela, C. J., Mahdavi, J., Thornton, J., Mann, B., Wooldridge, K. G., Abouseada, N., et al. (2009). Laminin receptor initiates bacterial contact with the blood brain barrier in experimental meningitis models. J. Clin. Invest. 119 (6), 1638–1646. doi: 10.1172/JCI36759
Ovstebø, R., Olstad, O. K., Brusletto, B., Møller, A. S., Aase, A., Haug, K. B. F., et al. (2008). Identification of genes particularly sensitive to lipopolysaccharide (LPS) in human monocytes induced by wild-type versus LPS-deficient Neisseria meningitidis strains. Infect. Immun. 76 (6), 2685–2695. doi: 10.1128/IAI.01625-07
Petnicki-Ocwieja, T., Kern, A. (2014). Mechanisms of Borrelia burgdorferi internalization and intracellular innate immune signaling. Front. Cell. Infect. Microbiol. 4. doi: 10.3389/fcimb.2014.00175
Pulzova, L., Bencurova, E., Csank, T., Mlynarcik, P., Bhide, M. R. (2013). “In situ assessment of differential adhesion of neuroinvasive and non-neuroinvasive Borrelia strains to BMECs,” in Farm animal proteomics 2013 (Wageningen: Wageningen Academic Publishers).
Pulzova, L., Kovac, A., Mucha, R., Mlynarcik, P., Bencurova, E., Madar, M., et al. (2011). OspA-CD40 dyad: ligand-receptor interaction in the translocation of neuroinvasive Borrelia across the blood-brain barrier. Sci. Rep. 1, 86–86. doi: 10.1038/srep00086
Ramesh, G., Alvarez, A. L., Roberts, E. D., Dennis, V. A., Lasater, B. L., Alvarez, X., et al. (2003). Pathogenesis of Lyme neuroborreliosis:Borrelia burgdorferi lipoproteins induce both proliferation and apoptosis in rhesus monkey astrocytes. Eur. J. Immunol. 33 (9), 2539–2550. doi: 10.1002/eji.200323872
Ramesh, G., Borda, J. T., Dufour, J., Kaushal, D., Ramamoorthy, R., Lackner, A. A., et al. (2008). Interaction of the Lyme disease spirochete Borrelia burgdorferi with brain parenchyma elicits inflammatory mediators from glial cells as well as glial and neuronal apoptosis. Am. J. Pathol. 173 (5), 1415–1427. doi: 10.2353/ajpath.2008.080483
Santibañez, J. F., Quintanilla, M., Bernabeu, C. (2011). TGF-β/TGF-β receptor system and its role in physiological and pathological conditions. Clin. Sci. 121 (6), 233–251. doi: 10.1042/cs20110086
Sapi, E., Bastian, S. L., Mpoy, C. M., Scott, S., Rattelle, A., Pabbati, N., et al. (2012). Characterization of biofilm formation by Borrelia burgdorferi in vitro. PloS One 7 (10), e48277. doi: 10.1371/journal.pone.0048277
Schubert-Unkmeir, A., Konrad, C., Slanina, H., Czapek, F., Hebling, S., Frosch, M. (2010). Neisseria meningitidis induces brain microvascular endothelial cell detachment from the matrix and cleavage of occludin: a role for MMP-8. PloS Pathog. 6 (4), e1000874–e1000874. doi: 10.1371/journal.ppat.1000874
Schubert-Unkmeir, A., Sokolova, O., Panzner, U., Eigenthaler, M., Frosch, M. (2007). Gene expression pattern in human brain endothelial cells in response to Neisseria meningitidis. Infect. Immun. 75 (2), 899–914. doi: 10.1128/IAI.01508-06
Schumacher, L., Slimani, R., Zizmare, L., Ehlers, J., Kleine Borgmann, F., Fitzgerald, J. C., et al. (2023). TGF-Beta modulates the integrity of the blood brain barrier in vitro, and is associated with metabolic alterations in pericytes. Biomedicines 11 (1), 214. doi: 10.3390/biomedicines11010214
Smith, T., Heger, A., Sudbery, I. (2017). UMI-tools: modeling sequencing errors in unique molecular identifiers to improve quantification accuracy? Genome Res. 27 (3), 491–499. doi: 10.1101/gr.209601.116
Stenman, J. M., Rajagopal, J., Carroll, T. J., Ishibashi, M., McMahon, J., McMahon, A. P. (2008). Canonical Wnt signaling regulates organ-specific assembly and differentiation of CNS vasculature. Science 322 (5905), 1247–1250. doi: 10.1126/science.1164594
Takano, H., Inoue, K., Yanagisawa, R., Sato, M., Shimada, A., Morita, T., et al. (2004). Protective role of metallothionein in acute lung injury induced by bacterial endotoxin. Thorax 59 (12), 1057–1062. doi: 10.1136/thx.2004.024232
Thompson, D., Sorenson, J., Greenmyer, J., Brissette, C. A., Watt, J. A. (2020). The Lyme disease bacterium, Borrelia burgdorferi, stimulates an inflammatory response in human choroid plexus epithelial cells. PloS One 15 (7), e0234993–e0234993. doi: 10.1371/journal.pone.0234993
Tkáčová, Z., Bhide, K., Mochnáčová, E., Petroušková, P., Hruškovicová, J., Kulkarni, A., et al. (2021). Comprehensive mapping of the cell response to Borrelia bavariensis in the brain microvascular endothelial cells in vitro using RNA-seq. Front. Microbiol. 12. doi: 10.3389/fmicb.2021.760627
Tkáčová, Z., Pulzová, L. B., Mochnáčová, E., Jiménez-Munguía, I., Bhide, K., Mertinková, P., et al. (2020). Identification of the proteins of Borrelia garinii interacting with human brain microvascular endothelial cells. Ticks Tick-Borne Dis. 11 (4), 101451. doi: 10.1016/j.ttbdis.2020.101451
Wong, B. W., Wong, D., Luo, H., McManus, B. M. (2011). Vascular endothelial growth factor-D is overexpressed in human cardiac allograft vasculopathy and diabetic atherosclerosis and induces endothelial permeability to low-density lipoproteins in vitro? J Heart Lung Transplant 30 (8), 955–962. doi: 10.1016/j.healun.2011.04.00
Yang, D., Chen, Q., Hoover, D. M., Staley, P., Tucker, K. D., Lubkowski, J., et al. (2003). Many chemokines including CCL20/MIP-3α display antimicrobial activity. J. Leukocyte Biol. 74 (3), 448–455. doi: 10.1189/jlb.0103024
Keywords: blood-brain barrier, BBB-spheroids, Neisseria meningitidis, Borrelia bavariensis, transcriptome analysis, human BMECs, pericytes, astrocytes
Citation: Kulkarni A, Jozefiaková J, Bhide K, Mochnaćová E and Bhide M (2023) Differential transcriptome response of blood brain barrier spheroids to neuroinvasive Neisseria and Borrelia. Front. Cell. Infect. Microbiol. 13:1326578. doi: 10.3389/fcimb.2023.1326578
Received: 23 October 2023; Accepted: 30 November 2023;
Published: 19 December 2023.
Edited by:
Xiangru Wang, Huazhong Agricultural University, ChinaReviewed by:
Bin Gong, University of Texas Medical Branch at Galveston, United StatesE. Diane Williamson, Defence Science and Technology Laboratory, United Kingdom
Copyright © 2023 Kulkarni, Jozefiaková, Bhide, Mochnaćová and Bhide. This is an open-access article distributed under the terms of the Creative Commons Attribution License (CC BY). The use, distribution or reproduction in other forums is permitted, provided the original author(s) and the copyright owner(s) are credited and that the original publication in this journal is cited, in accordance with accepted academic practice. No use, distribution or reproduction is permitted which does not comply with these terms.
*Correspondence: Mangesh Bhide, YmhpZGVtYW5nZXNoQGdtYWlsLmNvbQ==