- 1Jilin Provincial Key Laboratory of Tooth Development and Bone Remodeling, Department of Prosthodontics, Hospital of Stomatology, Jilin University, Changchun, Jilin, China
- 2Department of Prosthodontics, Hospital of Stomatology, Jilin University, Changchun, Jilin, China
Polymethyl methacrylate (PMMA) frequently features in dental restorative materials due to its favorable properties. However, its surface exhibits a propensity for bacterial colonization, and the material can fracture under masticatory pressure. This study incorporated commercially available RHA-1F-II nano-silver loaded zirconium phosphate (Ag-ZrP) into room-temperature cured PMMA at varying mass fractions. Various methods were employed to characterize Ag-ZrP. Subsequently, an examination of the effects of Ag-ZrP on the antimicrobial properties, biosafety, and mechanical properties of PMMA materials was conducted. The results indicated that the antibacterial rate against Streptococcus mutans was enhanced at Ag-ZrP additions of 0%wt, 0.5%wt, 1.0%wt, 1.5%wt, 2.0%wt, 2.5%wt, and 3.0%wt, achieving respective rates of 53.53%, 67.08%, 83.23%, 93.38%, 95.85%, and 98.00%. Similarly, the antibacterial rate against Escherichia coli registered at 31.62%, 50.14%, 64.00%, 75.09%, 86.30%, 92.98%. When Ag-ZrP was introduced at amounts ranging from 1.0% to 1.5%, PMMA materials exhibited peak mechanical properties. However, mechanical strength diminished beyond additions of 2.5%wt to 3.0%wt, relative to the 0%wt group, while PMMA demonstrated no notable cytotoxicity below a 3.0%wt dosage. Thus, it is inferred that optimal antimicrobial and mechanical properties of PMMA materials are achieved with nano-Ag-ZrP (RHA-1F-II) additions of 1.5%wt to 2.0%wt, without eliciting cytotoxicity.
1 Introduction
Polymethyl methacrylate (PMMA), recognized for its commendable biological, physical, and chemical properties along with superior machinability, has ascended as a predominant material in the field of stomatology (Rao et al., 2022). Employed as a material for denture reconstruction, orthodontic mobile appliances, periodontal splints, and jaw cystoccluders, room-temperature curing PMMA, while boasting numerous advantages, also manifests conspicuous disadvantages. Primarily, the rough surface and porosity of PMMA materials facilitate the colonization of microorganisms, such as Candida albicans, Streptococcus sanguineus, and Streptococcus mutans, thereby propelling the incidence of various oral diseases (An et al., 2022; Moreno-Maldonado et al., 2012; Quassem and Mahross, 2018; Sahm et al., 2022; Pourhajibagher et al., 2023; Schubert et al., 2019; Al-Dwairi et al., 2019; Patil et al., 2020; Dantas et al., 2016). Moreover, PMMA material exhibits a challenge regarding mechanical strength, proving susceptible to breakage under masticatory pressures ( Zafar, 2020; Ali Sabri et al., 2021; Alkordy and Alsaadi, 2014; Mohd Farid et al., 2022; Khan et al., 2022; Oleiwi et al., 2019; Zafar, 2018; Rana et al., 2021). Considering the myriad and multifaceted bacteria present in the human oral cavity, alongside the insufficient physical and mechanical strength of PMMA materials, an imperative arises to enhance the antibacterial properties of PMMA materials without compromising their physical and mechanical attribute.
In recent years, nano-antibacterial materials, notably nano-silver, have garnered considerable attention due to their broad-spectrum and enduring antibacterial effects without inducing bacterial resistance (Y. Liu et al., 2023;Y.-M. Wu et al., 2022). Research indicates that the incorporation of nanoparticles, which contain Ag, into PMMA materials can augment not only the antibacterial prowess of the PMMA materials but also enhance their mechanical properties, thus aptly fulfilling clinical requirements ( Bacali et al., 2020; Oyar et al., 2018; Garcia et al., 2021; Chen et al., 2017; Alhotan et al., 2023). Recent studies indicate that incorporating metal nanoparticles, such as silver nanoparticles, into inorganic carriers can enhance nanoparticle dispersion in polymers and mitigate the aggregation of these nanoparticles due to surface effects. This improvement facilitates the optimal utilization of the antibacterial properties of silver nanoparticles (Liu et al., 2019). Common carriers for nano-silver include zinc oxide (Pham et al., 2021), silica (Wong et al., 2017), montmorillonite (Fernández Solarte et al., 2019), zeolite (Youssef et al., 2019), graphene oxide (Huong et al., 2020), and zirconium phosphate (Liu et al., 2022). Researchers have investigated the incorporation of zinc oxide (Mousavi et al., 2020), silicon dioxide (Aati et al., 2022), graphene oxide (Arslan et al., 2023), and zeolite (Malic et al., 2019), all containing nano-silver, into PMMA materials. These studies explore the influence on the antimicrobial and mechanical properties of PMMA, offering new insights for clinical enhancements of these properties. However, zirconium α-phosphate, distinct from the carriers mentioned earlier, exhibits a two-dimensional layered nanosheet structure. It possesses exceptional ion exchange capabilities, catalytic activity, stable physicochemical properties, and biosafety, making it a versatile carrier for various medicines with significant potential in biomedicine (Saxena et al., 2013; Ramos-Garcés et al., 2021; Nocchetti et al., 2022). Moreover, the layered structure of zirconium phosphate nanosheets allows for increased surface area upon exfoliation, enabling a higher loading capacity for silver nanoparticles and thus enhancing the material’s antibacterial efficacy (Nocchetti et al., 2022). Despite these advances, research on the effects of nano-Ag-ZrP in PMMA materials, particularly regarding their antibacterial and mechanical properties, remains limited, suggesting an area ripe for further investigation.
Consequently, this study employs commercially available nano Ag-ZrP (RHA-1F-II) as the research subject, integrating it with room temperature curing PMMA material for material characterization analysis, and examines the impacts of nano Ag-ZrP antibacterial agent on the antibacterial attributes, mechanical properties, and biosafety of the room temperature curing PMMA material.
2 Materials and methods
2.1 Materials
Self-setting denture base polymer (NISSIN, Japan); self-setting denture water (NISSIN, Japan); Nano-Ag-ZrP (RHA-1F-II, China); BHI broth medium (Hopebio, China); LB broth medium (Hopebio, China); Nutritional Agar (Sigma, USA); SYTO 9/PI Live/Dead Bacterial Staining Kit (Thermo Fisher, USA); DMEM culture medium (Gibco, USA); Fetal Bovine Serum (FBS; Gibco, USA); 0.25% trypsin (Gibco, USA); Cell Counting Kit-8 (CCK-8); Calcein-AM/PI Live/Dead Cell Staining Kit (Beyotime, China); Annexin V-FITC/PI Apoptosis Detection Kit (Beyotime, China).
2.2 Preparation of specimen
PMMA powder and nano-Ag-ZrP were amalgamated in predefined mass fractions, subsequently subjected to an 8-hour processing in a planetary ball mill to procure the mixed powder.The material of the ball milling tank is zirconia, with a volume of 50 ml and a working volume of 15 ml;The material of the grinding ball is zirconia, with a total mass of 50 g, a diameter of 5 mm, and a ball to material ratio of 5:1; The working speed of the ball mill is 180 rpm/min. The requisite mass fractions of nano-Ag-ZrP for experimentation were defined as 0wt%, 0.5wt%, 1.0wt%, 1.5wt%, 2.0wt%, 2.5wt%, and 3.0wt%. Through combining the mixed powders with denture water, samples of disparate shapes and sizes were fabricated, culminating in the formation of nano-Ag-ZrP/PMMA materials. Conforming to relevant standards (ISO 22196:2007; ISO 20795-1:2008; ISO 527:2012), the antimicrobial specimen dimensions were set at (50 ± 2) mm × (50 ± 2) mm × (2 ± 0.5) mm; the three-point bending specimen at 64 mm × (10.0 ± 0.2) mm × (3.3 ± 0.2) mm; the modified three-point bending specimen at 39 mm × (8.0 ± 0.2) mm × (4.0 ± 0.2) mm, featuring a central notch measuring (3.0 ± 0.2) mm in length and 0.5 mm in width. The tensile test specimen adhered to a 5B dumbbell shape, with an overall length of 35.0 mm, end width of (6.0 ± 0.5) mm, narrow section width of (2.0 ± 0.1) mm, and a thickness of 1.0 mm.
2.3 Nano Ag - ZrP characterization
Surface morphology and elemental mapping of nano-Ag-ZrP were scrutinized utilizing field emission scanning electron microscopy (SEM;FEI, nova nanosem650,America) and transmission electron microscopy(TEM;FEI,F20,America). Additionally, the functional groups, crystal compositions, and valence states of Ag-ZrP were characterized through Fourier transform infrared spectroscopy(FT-IR;Nicolet,iS10,America),X-ray diffraction(XRD;Bruker,D8,Germany), and X-ray photoelectron spectroscopy(XPS; Thermo Fisher, ESCA LAB 350Xi,America).
2.4 The separation, culture and characterization of primary HGFs
Ethical approval was obtained from the Ethics Committee of the Stomatology Hospital of Jilin University. Gingival tissue, procured from patients aged 18-28 undergoing mandibular third molar extraction, necessitated the adjacent mandibular third molar to be healthy. Upon acquiring patient consent, samples were harvested around the extraction wound. Primary Human Gingival Fibroblasts (HGFs) were isolated and cultured utilizing the tissue block method. Optimal efforts were exerted to remove the epithelial tissue utilizing ophthalmic scissors. Gingival tissue, post-epithelial removal, was subjected to 50 repeat washes with DMEM medium, supplemented with 400 U/mL penicillin and 0.4 mg/mL streptomycin, until overt blood stains and impurities were absent. The tissue was then dissected into approximately 1 mm³ tissue blocks using ophthalmic scissors within a 60 mm culture dish. Subsequently, the tissue blocks were uniformly seeded into the base of T25 culture flasks with an approximate spacing of 0.5 cm between each block. Each flask was inoculated with about 10 tissue blocks, complemented by 5 ml of DMEM medium enriched with 20% Fetal Bovine Serum (FBS). The culture flasks were then incubated at 37°C, 5% CO2 for 4 hours, following which, the flasks were gently inverted and returned to the incubator. Minimal disturbance of the culture flasks was endeavored for the initial 3 days, with medium changes executed every 3 days. Once the primary cells occupied 60~70% of the flask bottom, the first passage was conducted. HGFs from passages 4~6 were designated for experimental use. Characterization of the isolated HGFs was accomplished through immunohistochemistry and immunofluorescence.
2.5 Detection of biosafety
2.5.1 Preparation of specimen extract
The specimen was fashioned into a 10 mm x 10 mm x 2 mm round sheet. Adhering to ISO 10993-12:2012, extracts were formulated at an extraction ratio of 3.0 cm²/mL. For each group, samples were positioned within centrifuge tubes, accompanied by 733 μl of DMEM culture medium, which contained 10% FBS and 1% dual antibodies. Subsequently, the centrifuge tubes were incubated at 37°C for a duration of 72 hours. Extracts were thereafter collected and reserved for subsequent utilization.
2.5.2 Detection of cytotoxicity by CCK-8
Three 96-well culture plates were seeded with 1.0×104 cells per well and incubated at 37° for 24 hours. Subsequently, the culture medium was aspirated, and wells were rinsed with PBS before the addition of 100 μl of extract from each specimen group to the respective wells. On the conclusion of the 1st, 2nd, and 3rd days, a well plate was selected, the spent culture medium was discarded, and 100 μl of CCK-8 culture medium was introduced to each well. Following a 1-hour incubation period, absorbance (OD) values were measured using an enzyme-linked immunosorbent assay apparatus, and the relative cell proliferation rate (RGR) was calculated utilizing the following formula:
where ODsamples represents the absorbance value of each extract group, and ODcontrol denotes the absorbance value of the negative control group.
2.5.3 Live and dead cell staining test
Cells were seeded into 6-well plates at a density of 1.0x105 cells per well and incubated for 24h. Subsequently, the culture medium was aspirated, and 2 ml of extract for each group was added for further culturing. After an additional incubation of 72h, the extract was removed from each well, followed by the addition of Calcein-AM/PI staining solution, and a subsequent 30-minute incubation at room temperature, protected from light, ensued. Observations were conducted using a fluorescence microscope.
2.5.4 Annexin V-FITC/PI flow cytometry was used to detect apoptosis
Cells were cultured in 6-well plates at a density of 1.0x105 cells per well and incubated for 24h. Following incubation, the culture medium was aspirated and wells were washed with PBS; subsequently, extracts for each group were introduced and maintained for an additional 72h. Cells were then harvested by centrifugation, and Binding Buffer was utilized to resuspend the cells, adjusting the cell concentration to 1.0x106/mL. A 100 μl aliquot of cell suspension was transferred to a centrifuge tube, and Annexin V-FITC and PI dyes were added according to the manufacturer’s instructions. The mixture was incubated for 20 min in dark conditions, followed by analysis via flow cytometry.
2.6 Detection of antibacterial property
2.6.1 Preparation of bacterial suspensions
S. mutans (ATCC 700610) and E. coli (ATCC 25922) were revitalized. Individual colonies of S. mutans and E. coli were isolated and cultured in Brain Heart Infusion (BHI) and Lysogeny Broth (LB) media, respectively, and incubated at 37°. Following an 8-hour incubation period, the optical density (OD) of the bacterial solutions was ascertained using a spectrophotometer. Subsequently, the concentrations of the bacterial suspensions were standardized to 1.0×106 CFU/ml to prepare working bacterial suspensions.
2.6.2 The antimicrobial rate of each group was determined by film coating method
Both sides of the specimens were exposed to ultraviolet light for 12 hours, followed by transfer to sterile petri dishes on an ultra-clean workbench. Working bacterial droplets of S. mutans and E. coli, each measuring 100 μl, were absorbed and introduced to the surface of each specimen group. Polyethylene film was applied to the surface of each specimen group, ensuring close contact with the specimen, and then incubated at 37° for 24 hours. Subsequently, the specimens and films were cleaned with 20 ml of 0.85% NaCl solution, and the collected eluent was vigorously shaken and diluted in a two-fold ratio. The diluted eluent, 100 μl, was plated and incubated at 37°. After 36 hours, colonies were enumerated on the agar plates. The antimicrobial rate was calculated using the formula R=(B-C)/B, where R represents the antibacterial rate, B is the average colony count of specimens in the 0%wt group (CFU/ml), and C denotes the average colony count in the experimental group (CFU/ml).
2.6.3 SYTO 9/PI staining of live and dead bacteria
The size of the specimens adhered to the dimensions specified in Section 2.5.1. Specimens from each group were positioned in 48-well plates, with each well receiving 500 μL of a working bacterial suspension of S. mutans and E. coli for a 24-hour incubation period. Subsequently, the bacterial solution from each well was aspirated and the specimens were rinsed with 0.85% NaCl solution. Specimens from each group were then transferred to centrifuge tubes, combined with 3 ml of 0.85% NaCl solution, and the bacteria from the specimen surfaces were collected following an ultrasonic shock for 5 minutes. Bacterial solutions for each group were centrifuged at 10000 g for 10 minutes; thereafter, the supernatant was discarded, and 200 μL of staining solution was added to suspend the bacterial pellet, followed by incubation in the dark at room temperature for 15 minutes. Finally, 5 μL of the bacterial solution was extracted from each tube, applied to a slide, and observed via a fluorescent inverted microscope.
2.6.4 Bacterial adhesion test
The specimen size and the co-culture methodology with the bacterial solution adhered to the parameters set in Section 2.6.3. Following a 24-hour period, the 48-well plate was removed, rinsed with 0.85% NaCl solution, and subsequently exposed to 2.5% glutaraldehyde, being fixed at 4° for 1 hour in light-protected conditions. Each well was aspirated of the glutaraldehyde solution and sequentially dehydrated with ethanol solutions of increasing concentrations (30%, 50%, 70%, 90%, and 100%) for 10 minutes each. Subsequently, the surface of the specimens in each group underwent gold sputter coating, and bacterial adhesion on the surfaces was observed using Scanning Electron Microscopy (SEM).
2.7 Mechanical property test
2.7.1 Three-point bending test
In adherence to the YY 0270.1-2011 standard, the test span was defined as (50 ± 0.1 mm) and the indenter speed was established at 5.0 mm/min. The bending strength, σ, is calculated as follows:
where: σ represents the bending strength in MPa; F signifies the maximum force exerted on the specimen, with a unit of N; L indicates the span and is measured in mm; b denotes the width of the specimen in mm; h defines the height of the specimen in mm. Similarly, the bending elastic modulus, E, can be calculated using the formula:
where: E represents the bending elastic modulus in MPa; K signifies the maximum slope in the stress-strain curve, expressed in N/mm; L, b, and h retain their previously defined meanings.
2.7.2 Improved three-point bending test
The span was established at (32.0 ± 0.1) mm, and the indenter speed was set to 1.0 mm/min. The formulas used to calculate the maximum stress intensity factor, Kmax, and the total breaking work, Wt, are as follows:
Pmax represents the maximum load exerted on the specimen and is measured in N; a delineates the total length of the specimen notch, with units in mm; ht is the specimen’s height, in mm. Additionally, bt signifies the specimen’s width and is also measured in mm, while lt designates the test span, expressed in mm.
U represents the area documented under the load/deflection curve, measured in N·mm. The total length of the specimen notch is denoted by a and is expressed in mm. The height and width of the specimen are represented by ht and bt respectively, both quantified in mm.
2.7.3 Tensile strength test
Conforming to ISO 527:2012, a 5B sample was utilized, establishing a fixture distance of (20 ± 2)mm and a standard distance of (10 ± 0.2)mm, with a test speed set at 1 mm/min. The tensile strength, β, is defined as β=F/A, where β is the tensile strength in MPa; F signifies the critical failure force value of the specimen, measured in N; and A represents the cross-sectional area of the sample, expressed in mm2.
2.8 Statistical analysis
Data from each group underwent statistical analysis utilizing GraphPad Prism. Here,`X denotes the mean of the data, while S signifies the standard deviation. Subsequent to the normal distribution test and variance homogeneity test, a one-way analysis of variance, coupled with the LSD-t test, was employed for analyzing statistical differences. The P-value threshold was established at 0.05, with P< 0.05 indicating statistical significance in the observed differences.
3 Results and discussion
3.1 Characterization of nano-borne Ag-ZrP
Figure 1 shows the synthesis of nano-Ag-ZrP/PMMA composites and the antibacterial mechanism of AgNp. SEM (Loaded voltage is 3kV,and sputtered with Au) reveals that nano-Ag-ZrP exhibits a layered cubic structure (Figure 2A), while Figures 2B-D sequentially depict PMMA materials with the incorporation of 0%wt, 1.5%wt, and 3.0%wt Ag-ZrP, respectively. Notably, in the 1.5%wt group, nano-Ag-ZrP minimally agglomerates within PMMA, showcasing a relatively uniform distribution, whereas the 3.0%wt group experiences an elevation in Ag-ZrP aggregation. It is speculated that the agglomeration of nano-Ag-ZrP in the 3.0%wt group was increased because the excessive addition of nano-Ag-ZrP led to the increase of the distribution density of nano-Ag-ZrP in PMMA materials, and the enhancement of the electrostatic attraction and van der Waals force, which led to the increase of the agglomeration of nano-Ag-ZrP particles (Sun et al., 2019; Kignelman et al., 2021; Kumar et al., 2022). Figures 2E-H present images of Ag-ZrP, as observed through TEM (Loaded voltage is 200KV,and loaded on copper mesh), at magnifications of 2 μm, 1 μm, 500 nm, and 200 nm, respectively. The ZrP crystal, featuring a sheet structure, is indicated by a blue arrow, and nano silver, exhibiting a black spherical shape, is indicated by a red arrow. Observable from the figure is the loading of nano-Ag particles onto the ZrP surface, bearing substantial morphological resemblance to the nano-Ag-ZrP detailed in existing literature (Qin et al., 2023; Yoshihara et al., 2021; Nocchetti et al., 2022). According to the TEM-Energy Dispersive Spectroscopy (TEM-EDS) element mapping analysis (Figures 2I-N) for P, O, Ag, and Zr elements, an intuitive reflection of each element’s distribution within nano-Ag-ZrP is provided, illustrating a uniform dispersion of nano-silver.
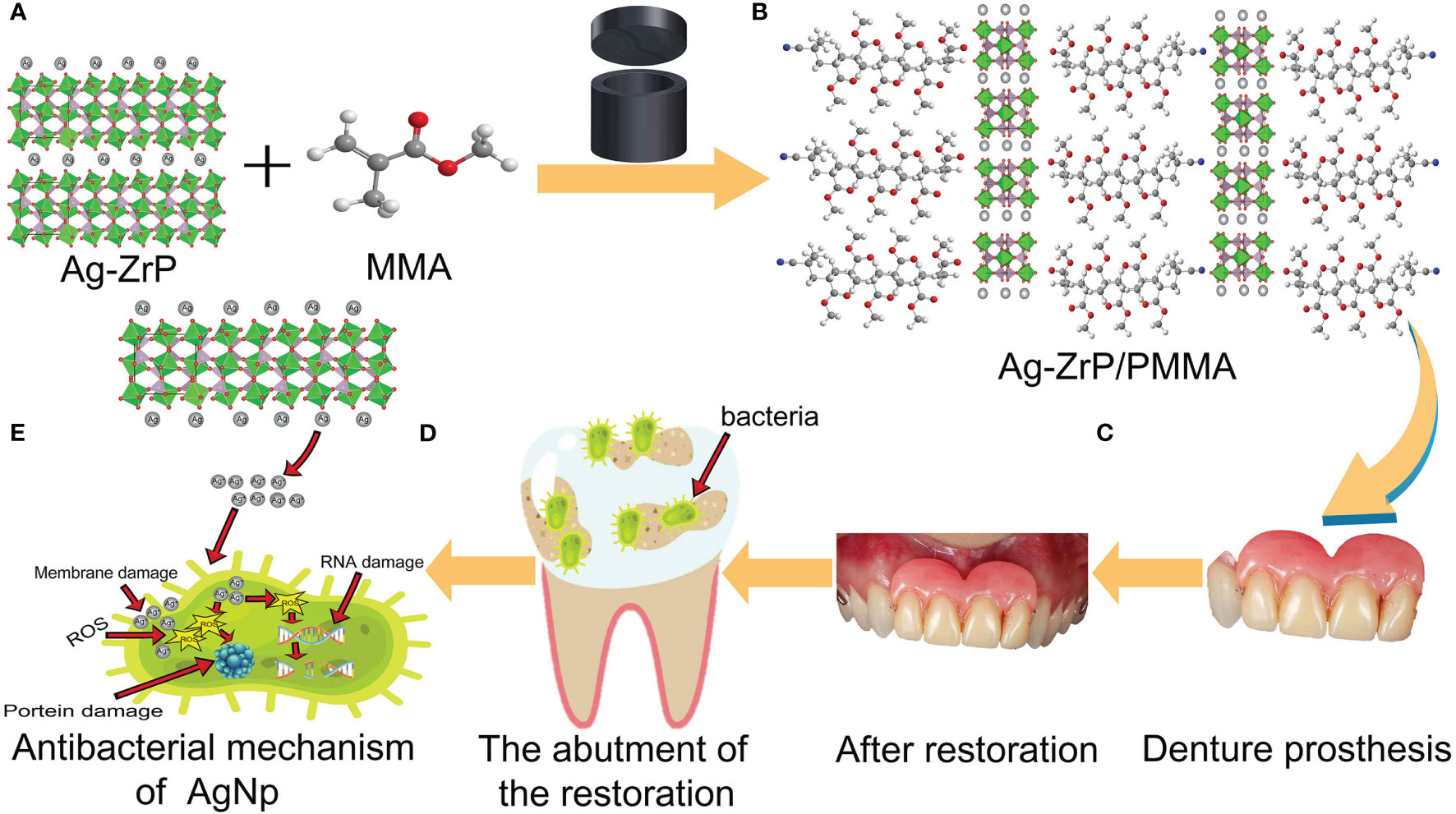
Figure 1 (A, B) is the schematic diagram of adding nano-Ag-ZrP to PMMA material; (C) is a denture prosthesis made of nano-Ag-ZrP/PMMA material; (D) for restoration abutments that are not easy to clean and adhere to bacteria; (E) is release Ag+ for nano-Ag-ZrP and produces antibacterial effect.
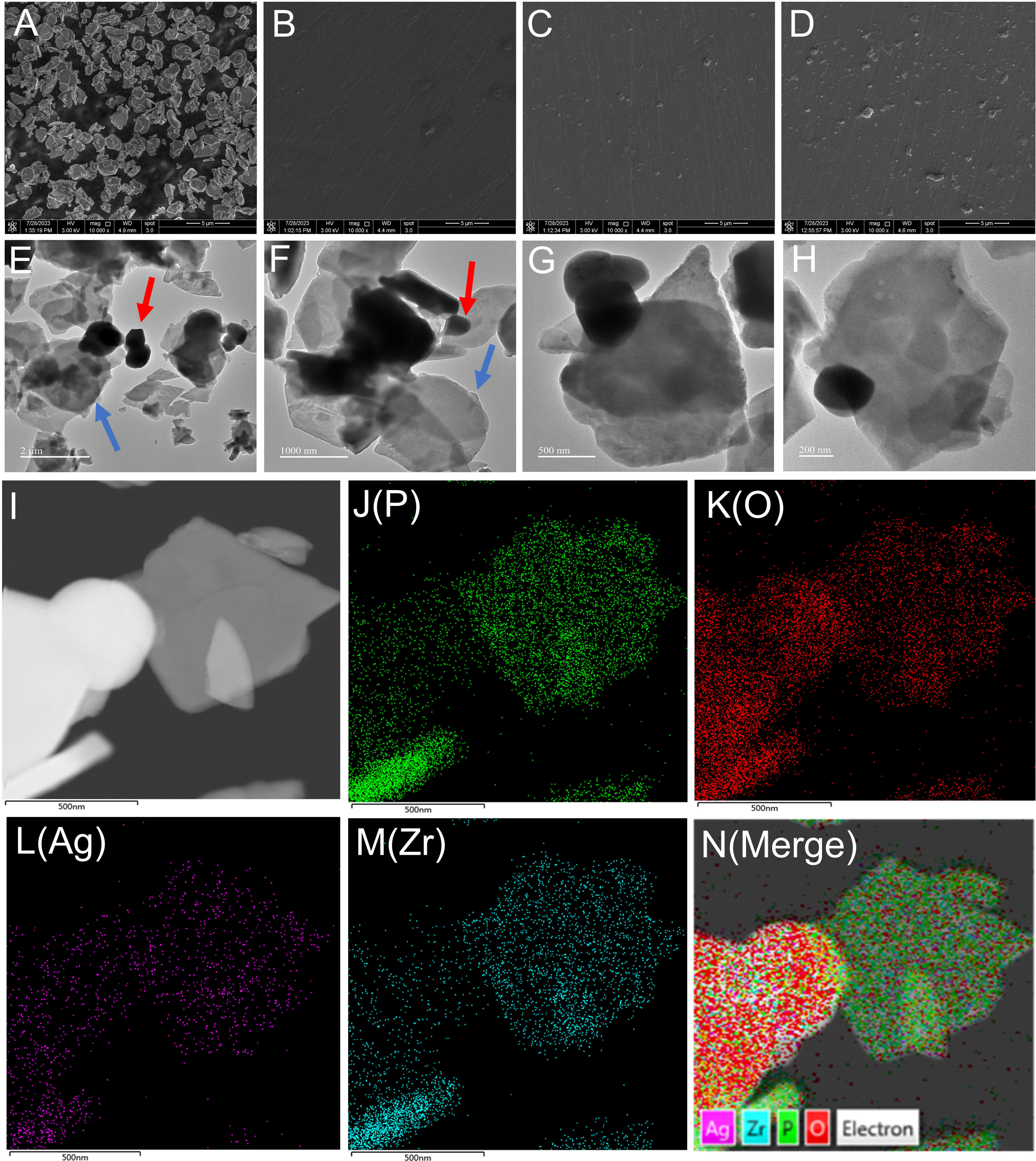
Figure 2 (A) is the SEM image of nano-Ag-ZrP; (B-D) are the SEM images of nano-Ag-ZrP/PMMA materials with 0%wt, 1.5%wt and 3.0%wt added in sequence. (E-H) is TEM image of nano-Ag-ZrP; (I-N) is the TEM-EDS element mapping of P, O, Ag and Zr of nano-Ag-ZrP.
Figure 3A displays the FT-IR (400 cm-1~ 4000 cm-1) spectroscopy analysis of Ag-ZrP, where the peak identified at 3453.78 cm-1 corresponds to the symmetric stretching vibration of OH, and the peak at 1637.66 cm-1 is attributed to the stretching vibration of OH from water molecules situated between crystal layers ( Bhatt et al., 2019). Peaks detected at 1207.58 cm-1 and 980.27 cm-1 associate with in-plane and out-of-plane vibrations of P-OH groups, respectively ( Safari et al., 2016), while those at 1020.94 cm-1 and 1105.65 cm-1 represent symmetric stretching vibrations of P-O bonds (Yan-Qing et al., 2015). The peak at 746.66 cm-1 pertains to P-O-P stretching vibrations(Sinhamahapatra et al., 2010) whereas those at 646.45 cm-1 and 548.70 cm-1 denote tensile vibrations of Zr-O bonds (Yan-Qing et al., 2015; Xu et al., 2020). Nano-Ag-ZrP was incorporated into PMMA materials in varying mass fractions. The FT-IR spectra for each nano-Ag-ZrP/PMMA composite are presented in Figure 3B. The spectra exhibit peaks at 2995.77 cm¹ and 2951.13 cm¹, corresponding to the asymmetric vibration of CH3 groups (Abutalib and Rajeh, 2020). Additional characteristic peaks include 985.49 cm¹ for C-C bond stretching, 1143.98 cm¹ for CH3 bending vibration, 1237.56 cm¹ for C-O bond stretching, 1447.97 cm¹ for CH3 deformation, and 1723.04 cm¹ for C=O bond stretching (Duan et al., 2008). The peak at 842.16 cm¹ is identified as the C=O bond (Abutalib and Rajeh, 2020), with in-plane and out-of-plane flexural vibrations of the C=O bond at 749.24 cm¹ (Singhal et al., 2013). Notably, a peak at 550.34 cm¹, attributed to the Zr-O bond stretching, appears in the spectra (Xu et al., 2020). For compositions with nano-Ag-ZrP, a new peak at 550.34 cm¹ emerges, becoming increasingly prominent with higher nano-Ag-ZrP concentrations, reaching its maximum at 3.0%wt. These results confirm the successful integration of nano-Ag-ZrP into PMMA materials, with a gradual increase in nano-Ag-ZrP content from 0%wt to 3.0%wt.XRD analysis (The XRD test uses copper targets with a scanning range of 5~70 degrees and a speed of 10 degrees per minute) of nano-Ag-ZrP and each group of nano-Ag-ZrP/PMMA composites (Figure 3C) indicates that peaks at 2θ values of 18.59°, 21.53°, 24.09°, 26.43°, 30.95°, 36.04°, and 39.28° correspond to ZrP2O7 (PDF No. 00-010-0004). Characteristic peaks at 20.12°, 23.27°, 33.38°, and 35.24° are aligned with NaHZr(PO4)2 (PDF No. 00-055-0206), while those at 37.72° and 44.57° correspond to characteristic peaks of nano silver (PDF No. 04-003-1659). The XRD results for the nano-Ag-ZrP/PMMA composites across different groups reveal a correlation between the concentration of nano-Ag-ZrP and the prominence of characteristic diffraction peaks. Specifically, as the nano-Ag-ZrP content increases, the distinct diffraction peaks corresponding to ZrP2O7, NaHZr(PO4)
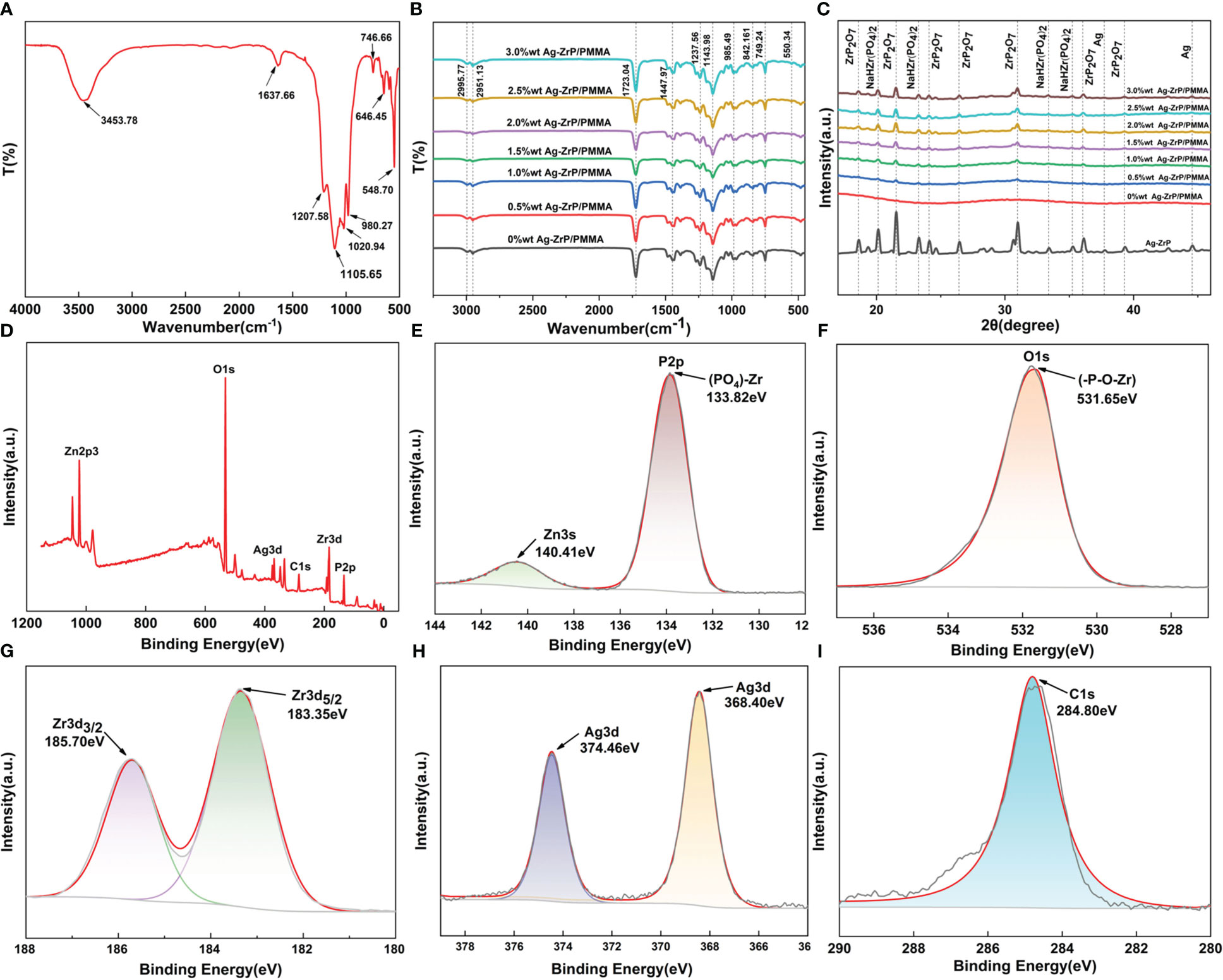
Figure 3 (A) is the FT-IR diagram of nano-Ag-ZrP; (B) is the FT-IR diagram of nano-Ag-ZrP/PMMA composites across different groups. (C) is the XRD analysis diagram of nano-Ag-ZrP and different groups of nano-Ag-ZrP/PMMA composites; (D) is the total XPS spectrum of nano-Ag-ZrP; (E-I) are the XPS spectra of P, O, Zr, Ag and C elements.
3.2 Characterization and identification of primary HGFs
On day 8, primary HGFs were observed to migrate from the gingival tissue (Figure 4A), and by day 15, the cells exhibited a swirl-like arrangement (Figure 4B). Figure 4C illustrates the morphology of fifth-generation HGFs under the microscope (40x magnification), revealing spindle- or star-shaped cells with centrally located nuclei, clear nucleoli, and round or oval nuclear shapes, consistent with typical fibroblast characteristics. Immunostaining was performed on the fifth-generation HGFs utilizing anti-vimentin antibody and anti-cytokeratin antibody (Cytokeratin 8). Figures 4D-I display immunofluorescence staining, while Figures 4J, K depict immunohistochemical staining. Staining for Vimentin was positive, whereas Cytokeratin 8 staining was negative. These results align with the staining findings for HGFs by Xuqian Liu, Kai Yang et al., confirming the cells to be mesodermal-origin fibroblasts (Liu et al., 2016; Yang et al., 2021).
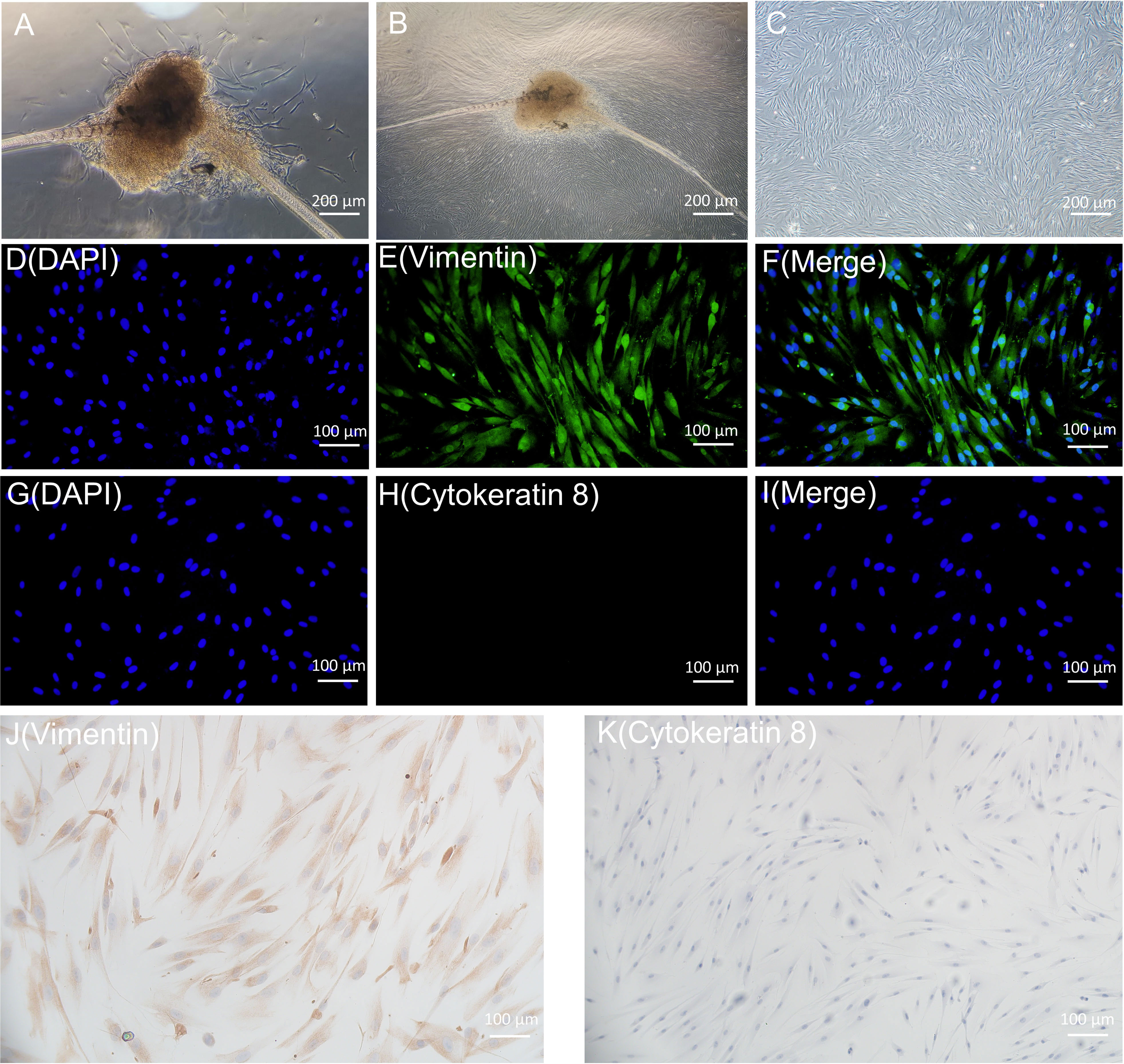
Figure 4 (A, B) are the primary HGFs flowing out of tissue blocks. C is the microscopic morphology of 5th generation HGFs. (D-I) is the result of immunofluorescence staining of HGFs; (J, K) were immunohistochemical staining results of HGFs.
3.3 Effect of nano-Ag-ZrP on biosafety of room-temperature cured PMMA materials
AgNP has been documented to exert specific impacts on cell activity, oxidative stress, and cytoskeletal protein among other factors (Shimizu et al., 2022; Perde-Schrepler et al., 2019) necessitating further investigation into the biosafety of Ag-ZrP/PMMA materials. Figure 5A illustrates the outcomes of apoptosis detection via Annexin V-FITC/PI double staining, following a 3-day culture of HGFs with the extract from each sample group. Subsequent statistical analysis of the apoptosis findings (Figure 5C) revealed a notable increase in the early apoptosis rate of HGF-s in the 3.0%wt group compared to the 0%wt group (P< 0.05). In comparison to the 0.5%wt group, the late apoptosis/necrosis rates of HGFs in the 2.5%wt and 3.0%wt groups notably escalated (P< 0.05), yet remained statistically indistinguishable among other groups. A plausible reason for the augmented apoptosis rate in 2.5%wt and 3.0%wt groups could be attributed to a minor release of AgNp with the elevation of Ag-ZrP supplemental level, thereby inducing a modest cytotoxicity. Despite this, the majority of cells maintained normal activity, and the overarching apoptosis rate did not reach substantial levels, warranting the incorporation of additional detection methods for accurate determination. Figure 5B portrays the staining results of live and dead cells post a 3-day culture of HGFs in each group’s extract solution, demonstrating that an uptick in nano-Ag-ZrP supplemental level correlated with an elevation in the count of live cells across all experimental groups and a reduction in the proportion of dead cells, without overt cell morphological alterations across groups. The CCK-8 method, employed to ascertain the activity of HGFs post a 3-day culture in extracts from each group (Figure 5D), yielded no significant discre-pancy in the Relative Growth Rate (RGR) of HGFs among extract groups (P > 0.05), with an RGR of HGFs exceeding 85% following 24h, 48h, and 72h of culture. According to the cytoto-xicity grading evaluation criteria stipulated by ISO 10993-5:2009, the cytotoxicity of extracts from each group post 24h, 48h, and 72h of cultured HGFs consecutively could be classified as less than or equal to grade 1, with no discernible cytotoxicity detected. Erkose and Jie SUN et al. incorporated AgNP into PMMA denture base materials, while Cecilia et al. introduced AgNP-GO into PMMA denture base materials, with neither finding substantial cytotoxicity in PMMA materials containing a low mass fraction of AgNP (Bacali et al., 2020; Kurt et al., 2017; Sun et al., 2021). Consequently, it may be inferred that the biosafety of roomtemperatur-e cured PMMA materials remains favorable when the addition of nano-Ag-ZrP is restrained below 3.0%wt.
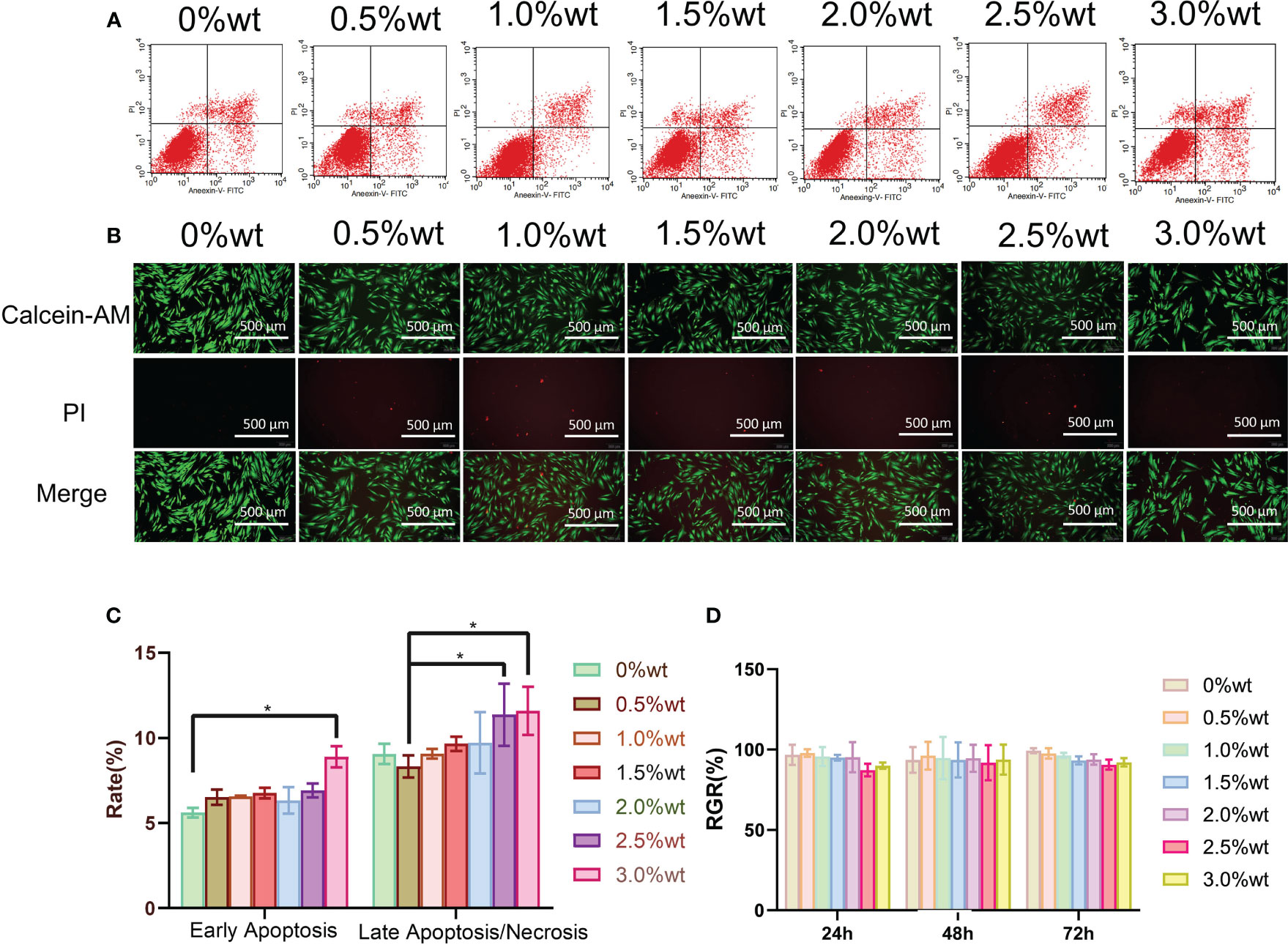
Figure 5 (A) is the Annexin V-FITC/PI double staining was used to detect apoptosis. (B) is the staining results of live and dead cells after incubation with HGFs 3 days in the extract solution of each group. (C) is the analysis of apoptosis detection results; (D) is the detection result of CCK-8 method. * symbol 0.01<P-value<0.05,it is indicating significant differences.
3.4 Effect of nano-Ag-ZrP on antibacterial properties of room-temperature cured PMMA
Table 1 illustrates the colony recovery number and antibacterial rate of S. mutans and E. coli across varied sample groups. The colony-counting results for S. mutans (Figure 6A) and E. coli (Figure 6B) elucidate that an elevation in nano-Ag-ZrP content correspondingly reduces the colony number of sample elutes across all groups. A diminution to the least colonies of S. mutans and E. coli is observed at 3.0%wt. Figure 6E reveals the statistical analysis results of S. mutans recovered colony numbers across different groups. A substantial reduction in the S. mutans colony number is observed in all groups compared to the control group (P< 0.05). Pairwise comparison among experimental groups reveals statistically significant discrepancies (P< 0.05) among them, save for the comparisons between 2.0%wt and 2.5%wt, 2.0%wt and 3.0%wt, and 2.5%wt and 3.0%wt (P > 0.05). For E. coli, statistical results of recovered colony numbers (Figure 6F) demonstrate a notable reduction in colony numbers across all experimental groups compared to the 0%wt control group (P< 0.05), with all experimental group pairwise comparisons showcasing statistically significant disparities (P< 0.05). Research by Shenggui Chen et al. involved the addition of 0.1-0.25%wt AgNP-CNCs (nanocellulose) to PMMA materials (Chen et al., 2018), Souza Neto et al. introduced 0.05%wt AgNP to PMMA resin material (Souza Neto et al., 2019), and Sultan Aati et al. incorporated silver-carrying mesoporous SO2 nanoparticles into the denture base (Aati et al., 2022). All studies affirm that materials embedded with AgNP can substantially enhance the antibacterial capability of PMMA materials, aligning with the findings obtained in this study that an increased AgNP content improves antibacterial effects. SEM images depicting S. mutans adherence to the surface of specimens in each group are presented in Figure 6C. S. mutans in the 0%wt∼0.5%wt group exhibits a smooth surface and a continuous, intact envelope(indicated by white arrows), contrasted by the 1.0%wt group where the envelope appears wrinkled and sunken. Within the 1.5% ~ 3.0%wt groups, the membrane of S. mutans evidences varied degrees of perforation and damage (indicated by white arrows). Figure 6D discloses SEM observation images of E. coli on specimen surfaces across groups. As you can see from the arrows,E. coli in the 0%wt∼0.5%wt group manifests well-preserved morphology, with a predominantly smooth surface and negligible folding and collapse. However, in the 1.0%wt and 1.5%wt groups, the E. coli cell membrane surface becomes notably rough and wrinkled. A further increase in the nano-Ag-ZrP antibacterial agent leads to an escalation in E. coli surface folds, cell membrane rupture, and exposure of bacterial contents in the 2.0% to 3.0%wt group. The SEM images of S. mutans and E. coli on the surface adhesion of specimens of each group showed that the damage degree of S. mutans and E. coli on the surface adhesion of specimens of each group was gradually serious with the increase of nano-Ag-ZrP addition. It is shown that the antibacterial properties of PMMA materials can be improved by adding nano-Ag-ZrP to PMMA materials. The presumed antibacterial mechanism may be attributed to direct contact between AgNP and the bacterial cell membrane, Ag+ release, bacterial cell wall and cell membrane disruption, reactive oxygen species (ROS) generation, bacterial substance leakage, and subsequent bacterial internal structure obliteration, culminating in a bactericidal effect (Ji et al., 2020; Hajipour et al., 2012; Dakal et al., 2016; Estevez et al., 2021).
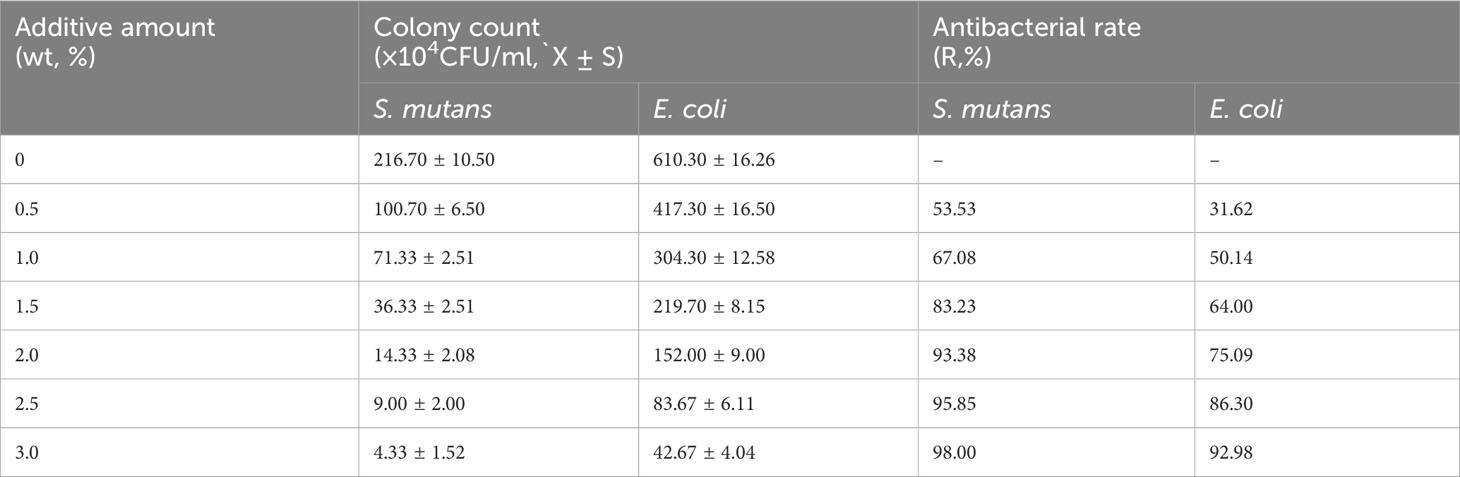
Table 1 The number of S. mutans and E. coli colonies recovered and the antibacterial rate of each group.
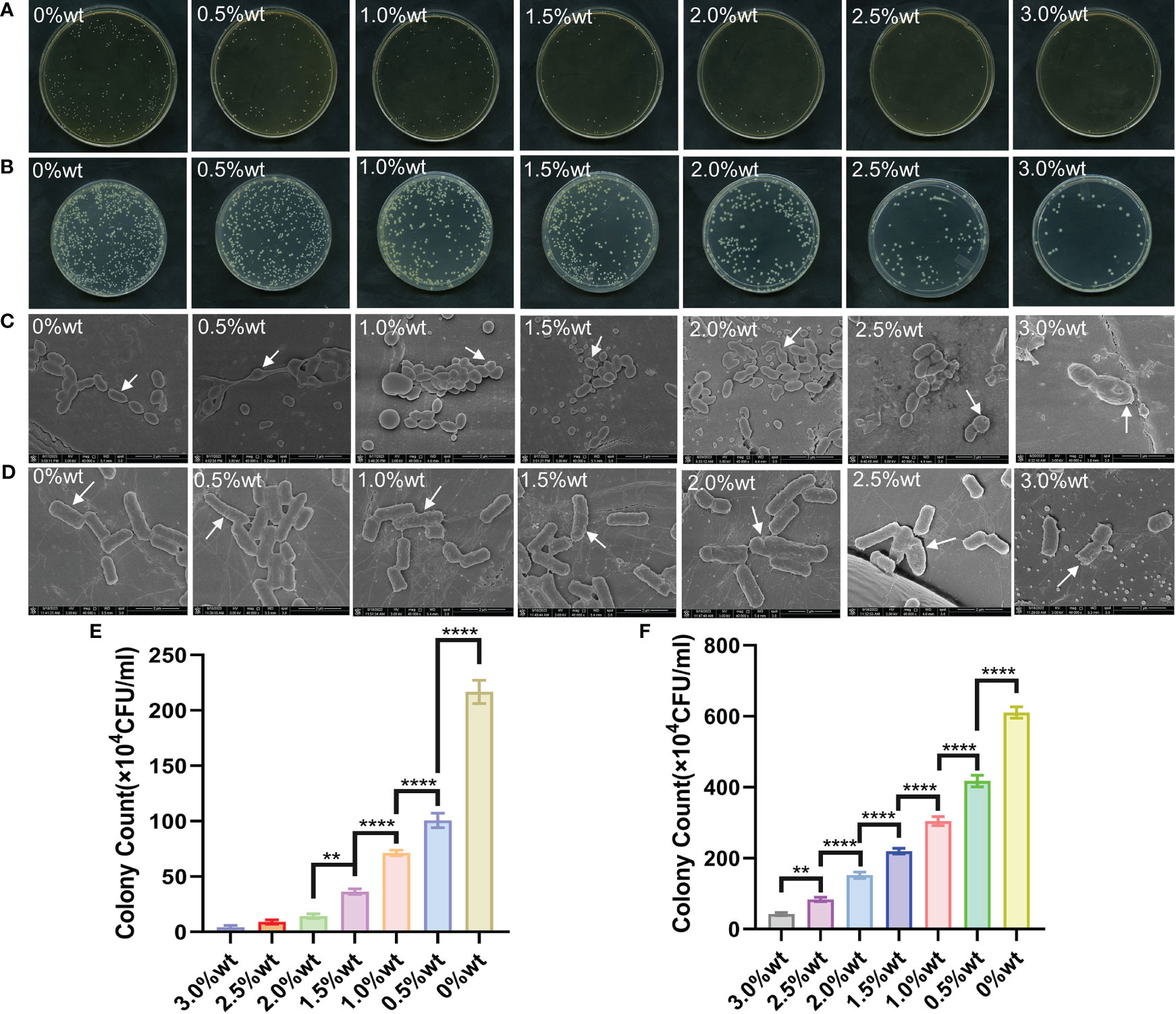
Figure 6 (A) is the plate colony count result of S. mutans. (B) is the colony count result of E. coli. (C) is the SEM image of S. mutans on the surface of each group of specimens. (D) is the SEM image of E. coli on the surface of each group of specimens; (E) is the results of statistical analysis of the colony count of S. mutans. (F) is the result of statistical analysis of E. coli colony count. * symbol 0.01<P-value<0.05, it is indicating significant differences; ** symbol 0.001<P-value<0.01, it is indicating that the difference is very significant; **** symbol P-value<0.0001, it is indicating that the significance of the difference is the highest.
SYTO 9 has the capability to stain the nucleic acid of live bacteria, or bacteria possessing damaged cell membranes, resulting in green fluorescence emission, while propidium iodide (PI) can stain the nucleic acid of dead bacteria, or bacteria with compromised cell membranes, to emit red fluorescence. Upon concurrent application, bacteria with impaired cell membranes exhibit yellow fluorescence. Figure 7 illustrates the staining results for live and dead bacteria of S. mutans and E. coli. It is evident from the figure that, concomitant with the augmentation of nano-Ag-ZrP antibacterial agent content, the quantity of live S. mutans and E. coli bacteria on the specimen surface progressively diminished, whereas the number of dead bacteria or bacteria with damaged cell membranes progressively escalated. When the addition of Ag-ZrP antibacterial agent reached 2.5%wt, and subsequently 3.0%wt, the dead bacteria or bacteria with compromised cell membranes pervaded almost the entire visual field on the specimen surface, aligning with the outcomes from the film coating experiment.
3.5 Effect of nano-Ag-ZrP on mechanical properties of PMMA materials
The fracture morphology of the sample, post-three-point bending test fracture, as visualized in the SEM image (Figure 8A), reveals that the cross-section of the sample in the 0%wt group is predominantly smooth with a flat, continuous fracture line, indicative of typical brittle fracture characteristics. With the escalation of nano-Ag-ZrP content, a gradual increase in the number of nano-Ag-ZrP crystals on the fracture surface of the 0.5%wt ~ 3.0%wt group is observable, and the evident rough stratification of the fracture surface displays dimple formation. Nano-Ag-ZrP particles were identified within the dimple, exhibiting classic ductile fracture traits. A notably deep and large dimple is present on the fracture surface of the 0.5%wt ~ 2.00%wt group. Conversely, the dimple on the fracture surface of the 2.5wt ~ 3.0%wt group is shallow and small, with Ag-ZrP crystal particles appearing more agglomerated. Figure 8B illustrates the shape of the mechanical properties test specimen and the universal mechanical testing machine. Three-point bending test results (Figures 8C, D) 1.5%wt, the bending strength and bending elastic modulus of the PMMA material incrementally rise, subsequently diminishing with a continuous increase of Ag-ZrP content. Both the bending strength and bending elastic modulus of the 1.5%wt group reached peak values. When compared with the 1.5%wt group, the 2.0%wt, 2.5%wt, and 3.0%wt groups exhibit significantly reduced bending strength and bending elastic modulus, with the difference being statistically significant (P< 0.05). Figures 8E, F depict the outcomes from the modified three-point bending test, illustrating a notable trend in the Wt and Kmax of each group with varying nano-Ag-ZrP content. Specifically, in the range of 0%wt to 1.0%wt nano-Ag-ZrP, both Wt and Kmax exhibit a gradual increment, showcasing a statistically significant difference in comparison to the 0%wt control group (P< 0.05). Conversely, in the 1.0%wt to 3.0%wt nano-Ag-ZrP range, a gradual decline in the Wt and Kmax of the sample is observed, with the zenith being at the 1.0%wt group. The tensile test results from each group’s specimens (Figure 8G) demonstrate a gradual ascending trend in the tensile strength (TS) within the 0%wt to 1.5%wt group, peaking at 1.5%wt. Subsequently, a gradual descending trend in TS is exhibited by the experimental groups, nadiring at 3.0%wt. Rongrong CHEN et al. integrated 3.0wt% nano-Ag-ZrP (Novaron) into a blend comprising PMMA powder, MMA monomer, silanized nano-zirconia, and silanized aluminum borate whisker, determining that an enhancement in the bending strength and hardness of PMMA material was realized exclusively post nano-Ag-ZrP addition(R. Chen et al., 2017). Wenbo Liao et al., found that the addition of silanized nano-Ag-ZrP to PMMA material resulted in an initial increase followed by a decrease in the bending strength as the mass fraction of Ag-ZrP augmented from 0wt% to 3.0wt%. A pinnacle in the bending strength of PMMA material was achieved when the nano-silver zirconium phosphate was introduced at 1.0%wt (Liao et al., 2020). Incorporation of nanoparticles, such as zirconium dioxide (ZrO2), graphene oxide (GO), and silicon dioxide (SiO2), into PMMA materials has been investigated by various scholars, revealing a general tendency: an initial enhancement in mechanical properties bending strength, bending elastic modulus, and tensile strength of PMMA materials, followed by a decrement (Checinska et al., 2022; Sahm et al., 2023; Ahamad Said et al., 2022; Alzayyat et al., 2022; Wang et al., 2019). Literature elucidates that the mechanism underpinning the nanoparticle-induced enhancement of mechanical strength in PMMA materials involves the induction of stress aggregation by the nanoparticle crystal particles. Upon integration into PMMA materials, these particles promote silver patterns in the surrounding matrix, instigating plastic deformation of the adjacent matrix, thereby absorbing external energy and generating toughness. Concurrently, nanoparticles are also capable of terminating the silver lines, yielding, and imparting a toughening effect. However, an incessant increase in the nano-Ag-ZrP crystal content compromises the dispersion of nanoparticle crystals in PMMA material, initiating agglomeration. Transformed from small to large particulate matter through agglomeration, the nanoparticle crystals reduce in surface area, forming a stress concentration zone, and their adhesion to PMMA material deteriorates. The manifestation of numerous defective structures within the material consequently undermines the mechanical properties of PMMA materials (Daelemans et al., 2016; Zeidi et al., 2023; Wang et al., 2017; He et al., 2018). In the current experiment, SEM observation of the fracture surface of each group of specimens, coupled with analysis post-mechanical properties test, affords a viewpoint aligning with preceding studies.
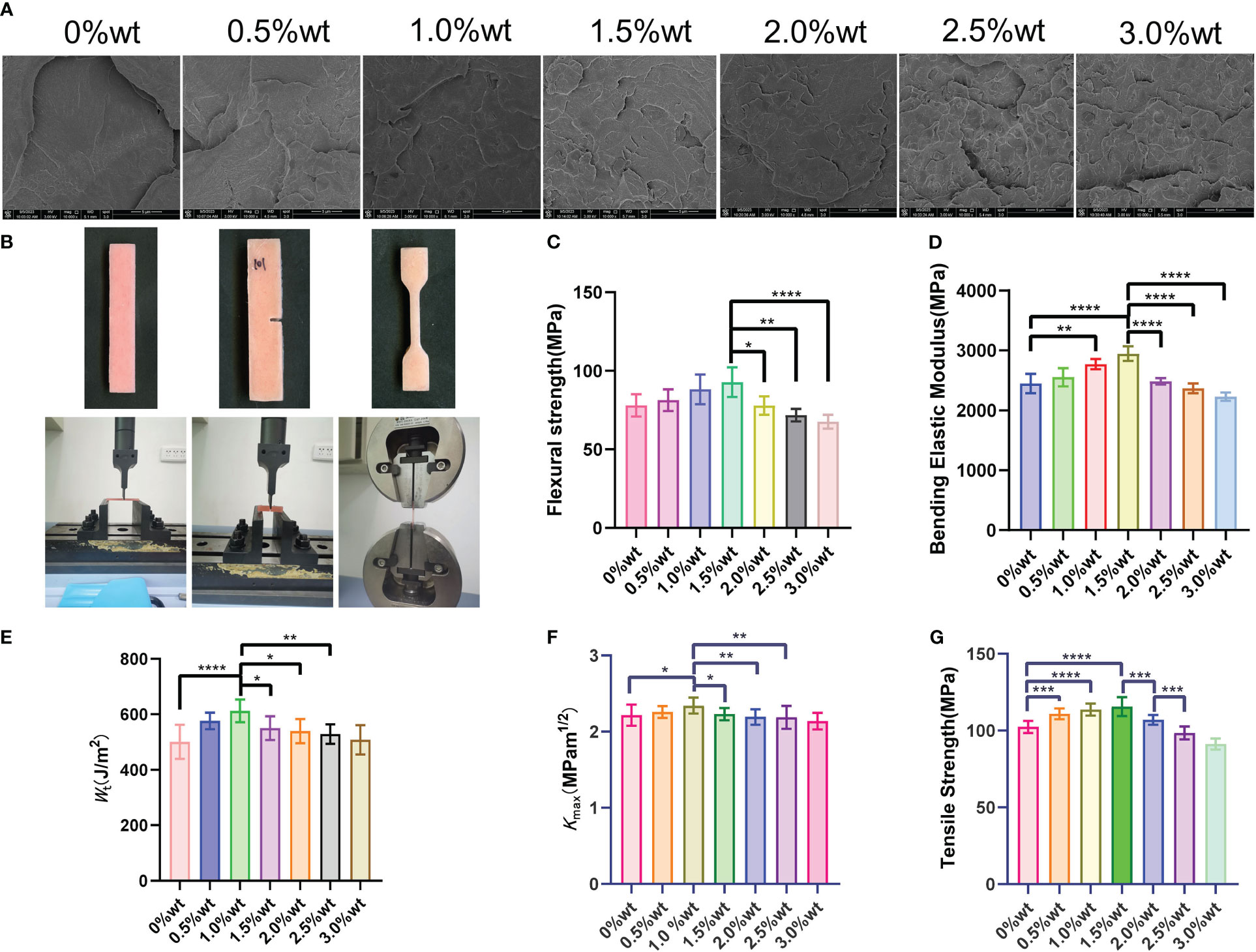
Figure 8 (A) is SEM observation of three-point bending specimen; (B) is the specimen shape and universal mechanical testing machine; (C, D) are the results of three-point curve test; (E, F) are the results of improved three-point bending test; (G) is the result of tensile test. * symbol 0.01<P-value<0.05, it is indicating significant differences; ** symbol 0.001<P-value<0.01, it is indicating that the difference is very significant; *** symbol 0.0001<P-value<0.001, it is indicating difference is more significant; **** symbol P-value<0.0001, it is indicating that the significance of the difference is the highest.
4 Conclusion
The morphological characteristics, functional groups, crystal structures, and elemental composition of commercially available nano-Ag-ZrP were analyzed employing SEM, TEM, FT-IR, XRD, and XPS. The material, initially presumed to be nano-Ag-ZrP, demonstrated optimal antibacterial activity against S. mutans and E. coli when incorporated at a 3.0%wt into the room-temperature cured PMMA material, achieving antibacterial rates of 98.00% and 92.98%, respectively. A concentration of 2.0%wt resulted in antibacterial rates of 93.38% against S. mutans and 75.09% against E. coli in the PMMA material. Mechanical property testing revealed that room-temperature-curing PMMA materials exhibited peak mechanical properties when the concentration of nano-Ag-ZrP antibacterial agent was within 1.0%~1.5%wt. When the antimicrobial agent concentration was increased to 2.0%wt, the mechanical strength of the PMMA material did not diminish compared to the 0%wt group, while concentrations starting from 2.5%wt resulted in reduced mechanical strength relative to the 0%wt group. Furthermore, cytotoxicity was not detected at nano-Ag-ZrP concentrations below 3.0%wt, indicating commendable biosafety. Consequently, future research aiming to enhance the antimicrobial and mechanical properties of PMMA materials should prioritize augmenting antimicrobial properties without compromising the mechanical integrity of the PMMA materials. In summary, it is recommended that the incorporation of nano-Ag-ZrP (RHA-1F-II) in room-temperature curing PMMA material be maintained within 1.5%wt ~ 2.0%wt.
Data availability statement
The raw data supporting the conclusions of this article will be made available by the authors, without undue reservation.
Ethics statement
The studies involving humans were approved by Stomatology Hospital of Jilin University. The studies were conducted in accordance with the local legislation and institutional requirements. Written informed consent for participation in this study was provided by the participants’ legal guardians/next of kin.
Author contributions
XC: Writing – original draft, Writing – review & editing. TY: Data curation, Methodology, Supervision, Writing – review & editing. SS: Methodology, Writing – review & editing. AL: Conceptualization, Data curation, Writing – review & editing. XW: Formal Analysis, Funding acquisition, Methodology, Resources, Supervision, Writing – review & editing.
Funding
The author(s) declare financial support was received for the research, authorship, and/or publication of this article. This research was funded by Project of Development Plan of Science and Technology of Jilin Province (no. 20220401092YY).
Conflict of interest
The authors declare that the research was conducted in the absence of any commercial or financial relationships that could be construed as a potential conflict of interest.
Publisher’s note
All claims expressed in this article are solely those of the authors and do not necessarily represent those of their affiliated organizations, or those of the publisher, the editors and the reviewers. Any product that may be evaluated in this article, or claim that may be made by its manufacturer, is not guaranteed or endorsed by the publisher.
References
Aati, S., Aneja, S., Kassar, M., Leung, R., Nguyen, A., Tran, S., et al. (2022). Silver-loaded mesoporous silica nanoparticles enhanced the mechanical and antimicrobial properties of 3D printed denture base resin. J. Mech. Behav. BioMed. Mater 134, 105421. doi: 10.1016/j.jmbbm.2022.105421
Abutalib, M. M., Rajeh, A. (2020). Influence of Fe3O4 nanoparticles on the optical, magnetic and electrical properties of PMMA/PEO composites: Combined FT-IR/DFT for electrochemical applications. J. Organometallic Chem. 920, 121348. doi: 10.1016/j.jorganchem.2020.121348
Ahamad Said, M. N., Hasbullah, N. A., Rosdi, M. R. H., Musa, M. S., Rusli, A., Ariffin, A., et al. (2022). Polymerization and applications of poly(methyl methacrylate)-graphene oxide nanocomposites: A review. ACS Omega 7 (51), 47490–47503. doi: 10.1021/acsomega.2c04483
Ajaj-Alkordy, N. M., Alsaadi, M. H. (2014). Elastic modulus and flexural strength comparisons of high-impact and traditional denture base acrylic resins. Saudi Dent. J. 26 (1), 15–18. doi: 10.1016/j.sdentj.2013.12.005
Al-Dwairi, Z. N., Tahboub, K. Y., Baba, N. Z., Goodacre, C. J., Ozcan, M. (2019). A comparison of the surface properties of CAD/CAM and conventional polymethylmethacrylate (PMMA). J. Prosthodont 28 (4), 452–457. doi: 10.1111/jopr.13033
Alhotan, A., Abdelraouf, R. M., El-Korashy, S. A., Labban, N., Alotaibi, H., Matinlinna, J. P., et al. (2023). Effect of adding silver-doped carbon nanotube fillers to heat-cured acrylic denture base on impact strength, microhardness, and antimicrobial activity: A preliminary study. Polymers (Basel) 15 (13), 2976. doi: 10.3390/polym15132976
Ali Sabri, B., Satgunam, M., Abreeza, N. M., N. Abed, A., Jones, I. P. (2021). A review on enhancements of PMMA Denture Base Material with Different Nano-Fillers. Cogent Eng. 8 (1), 1875968. doi: 10.1080/23311916.2021.1875968
Alzayyat, S. T., Almutiri, G. A., Aljandan, J. K., Algarzai, R. M., Khan, S. Q., Akhtar, S., et al. (2022). Effects of siO2 incorporation on the flexural properties of a denture base resin: an in vitro study. Eur. J. Dent. 16 (1), 188–194. doi: 10.1055/s-0041-1732806
An, J., Ding, N., Zhang, Z. (2022). Mechanical and antibacterial properties of polymethyl methacrylate modified with zinc dimethacrylate. J. Prosthet Dent. 128 (1), 100 e1–100.e8. doi: 10.1016/j.prosdent.2022.04.029
Arslan, S., Ekrikaya, S., Ildiz, N., Yusufbeyoglu, S., Ocsoy, I. (2023). Evaluation of the antibacterial activity of dental adhesive containing biogenic silver nanoparticles decorated nanographene oxide nanocomposites (Ag@nGO NCs) and effect on bond strength to dentine. Odontology. doi: 10.1007/s10266-023-00836-7
Bacali, C., Baldea, I., Moldovan, M., Carpa, R., Olteanu, D. E., Filip, G. A., et al. (2020). Flexural strength, biocompatibility, and antimicrobial activity of a polymethyl methacrylate denture resin enhanced with graphene and silver nanoparticles. Clin. Oral. Investig. 24 (8), 2713–2725. doi: 10.1007/s00784-019-031332
Bhatt, R., Ageetha, V., Rathod, S. B., Padmaja, P. (2019). Self-assembled chitosan-zirconium phosphate nanostructures for adsorption of chromium and degradation of dyes. Carbohydr Polym 208, 441–450. doi: 10.1016/j.carbpol.2018.12.077
Biswal, N., Martha, S., Subudhi, U., Parida, K. (2011). Incorporation of Silver Ions into Zirconium Titanium Phosphate: A Novel Approach toward Antibacterial Activity. Ind. Eng. Chem. Res. 50 (16), 9479–9486. doi: 10.1021/ie102199b
Checinska, K., Checinski, M., Sikora, M., Nowak, Z., Karwan, S., Chlubek, D. (2022). The effect of zirconium dioxide (ZrO(2)) nanoparticles addition on the mechanical parameters of polymethyl methacrylate (PMMA): A systematic review and meta-analysis of experimental studies. Polymers (Basel) 14 (5). doi: 10.3390/polym14051047
Chen, R., Han, Z., Huang, Z., Karki, J., Wang, C., Zhu, B., et al. (2017). Antibacterial activity, cytotoxicity and mechanical behavior of nano-enhanced denture base resin with different kinds of inorganic antibacterial agents. Dental Materials J. 36 (6), 693–699. doi: 10.4012/dmj.2016-301
Chen, Y., Sun, X., Zhang, T., Han, C., Ye, X., Liu, X., et al. (2022). Tribological performance study of zirconium phosphate as an additive in titanium complex gr-ease. Materials Lett. 321, 132402. doi: 10.1016/j.matlet.2022.132402
Chen, S., Yang, J., Jia, Y. G., Lu, B., Ren, L. (2018). A study of 3D-printable reinforced composite resin: PMMA modified with silver nanoparticles loaded cellulose nanocrystal. Materials (Basel) 11 (12). doi: 10.3390/ma11122444
Daelemans, L., van der Heijden, S., De Baere, I., Rahier, H., Van Paepegem, W., De Clerck, K. (2016). Damage-resistant composites using electrospun nanofibers: A multiscale analysis of the toughening mechanisms. ACS Appl. Mater Interfaces 8 (18), 11806–11818. doi: 10.1021/acsami.6b02247
Dakal, T. C., Kumar, A., Majumdar, R. S., Yadav, V. (2016). Mechanistic basis of antimicrobial actions of silver nanoparticles. Front. Microbiol. 7. doi: 10.3389/fmicb.2016.01831
Dantas, L. C., da Silva-Neto, J. P., Dantas, T. S., Naves, L. Z., das Neves, F. D., da Mota, A. S. (2016). Bacterial adhesion and surface roughness for different clinical techniques for acrylic polymethyl methacrylate. Int. J. Dent. 2016, 8685796. doi: 10.1155/2016/8-685796
Duan, G., Zhang, C., Li, A., Yang, X., Lu, L., Wang, X. (2008). Preparation and characterization of mesoporous zirconia made by using a poly (methyl methacrylate) template. Nanoscale Res. Lett. 3 (3), 118–122. doi: 10.1007/s11671-008-9123-7
Estevez, M. B., Casaux, M. L., Fraga, M., Faccio, R., Albores, S. (2021). Biogenic silver nanoparticles as a strategy in the fight against multi-resistant salmonella enterica isolated from dairy calves. Front. Bioeng Biotechnol. 9. doi: 10.3389/fbioe.2021.644014
Fernández Solarte, A. M., Villarroel-Rocha, J., Morantes, C. F., Montes, M. L., Sapag, K., Curutchet, G., et al. (2019). Insight into surface and structural changes of montmorillonite and organomontmorillonites loaded with Ag. Comptes Rendus Chimie 22 (2-3), 142–153. doi: 10.1016/j.crci.2018.09.006
Garcia, A., Sugio, C. Y. C., de Azevedo-Silva, L. J., Gomes, A. C. G., Batista, A. U. D., Porto, V. C., et al. (2021). Nanoparticle-modified PMMA to prevent denture stomatitis: a systematic review. Arch. Microbiol. 204 (1), 75. doi: 10.1007/s00203-021-026534
Hajipour, M. J., Fromm, K. M., Ashkarran, A. A., Jimenez de Aberasturi, D., de Larramendi, I. R., Rojo, T., et al. (2012). Antibacterial properties of nanoparti-cles. Trends Biotechnol. 30 (10), 499–511. doi: 10.1016/j.tibtech.2012.06.004
He, S., Qian, Y., Liu, K., Macosko, C. W., Stein, A. (2018). Effects of inorganic fillers on toughening of vinyl ester resins by modified graphene oxide. Ind. Eng. Chem. Res. 57 (13), 4592–4599. doi: 10.1021/acs.iecr.8b00362
Huong, N. T., Dat, N. M., Thinh, D. B., Minh Anh, T. N., Nguyet, D. M., Quan, T. H., et al. (2020). Optimization of the antibacterial activity of silver nanoparticles-decorated graphene oxide nanocomposites. Synthetic Metals 268, 116492. doi: 10.1016/j.synthmet.2020.116492
Ji, H., Zhou, S., Fu, Y., Wang, Y., Mi, J., Lu, T., et al. (2020). Size-controllable preparation and antibacterial mechanism of thermo-responsive copolymer-stabilized silver nanoparticles with high antimicrobial activity. Mater Sci. Eng. C Mater Biol. Appl. 110, 110735. doi: 10.1016/j.msec.2020.110735
Khan, A. A., Fareed, M. A., Alshehri, A. H., Aldegheishem, A., Alharthi, R., Saadaldin, S. A., et al. (2022). Mechanical properties of the modified denture base materials and polymerization methods: A systematic review. Int. J. Mol. Sci. 23 (10), 5737. doi: 10.3390/ijms23105737
Kignelman, G., Eyley, S., Zhou, C., Tunca, B., Gonon, M., Lahem, D., et al. (2021). Colloidal stability and aggregation mechanism in aqueous suspensions of TiO2 nanoparticles prepared by sol-gel synthesis. Langmuir 37 (51), 14846–14855. doi: 10.1021/acs.langmuir.1c02533
Kumar, S., Saha, D., Kohlbrecher, J., Aswal, V. K. (2022). Interplay of interactions for different pathways of the fractal aggregation of nanoparticles. Chem. Phys. Lett. 803, 139808. doi: 10.1016/j.cplett.2022.139808
Kurt, A., Erkose-Genc, G., Uzun, M., Emrence, Z., Ustek, D., Isik-Ozkol, G. (2017). The antifungal activity and cytotoxicity of silver containing denture base material. Niger J. Clin. Pract. 20 (3), 290–295. doi: 10.4103/1119-3077.181362
Li, H., He, Y., Luo, P., Fan, Y., Gou, L., Li, Z., et al. (2021). Preparation of laminar α-ZrP nanosheets enhanced Ni W nanocomposite coating and investigation of its mechanical and anti-corrosion properties. Surface Coatings Te-chnology 423, 127590. doi: 10.1016/j.surfcoat.2021.127590
Liao, W., Zheng, S., Chen, S., Zhao, L., Huang, X., Huang, L., et al. (2020). Surface silanization and grafting reaction of nano-silver loaded zirconium phosphate and properties strengthen in 3D-printable dental base composites. J. Mech. Behav. BioMed. Mater 110, 103864. doi: 10.1016/j.jmbbm.2020.103864
Liu, X., Chen, L., Dong, Q., Wang, Z., Zhang, D., He, J., et al. (2022). Emerging starch composite nanofibrous films for food packaging: Facile construction, hydrophobic property, and antibacterial activity enhancement. Int. J. Biol. Macromol 222 (Pt A), 868–879. doi: 10.1016/j.ijbiomac.2022.09.187
Liu, Y., Wan, Y., Zeng, Z., Yan, Z., Liu, Y. (2023). Nanosilver-decorated covalent organic frameworks for enhanced photodynami-c, photothermal, and antibacterial properties. Materials Chem. Phys. 307, 128158. doi: 10.1016/j.matchemphys.2023.128158
Liu, X., Wang, J., Dong, F., Li, H., Hou, Y. (2016). Human gingival fibroblasts induced and differentiated into vascular endothelial-like cells. Dev. Growth Differ 58 (9), 702–713. doi: 10.1111/dgd.123-27
Liu, T., Zeng, X., Lai, X., Li, H., Wu, T. (2019). Improvement of platinum nanoparticles-immobilized α-zirconium phosphate sheets on tracking and erosion resistance of silicone rubber. Composites Part B: Eng. 176, 107203. doi: 10.1016/j.compositesb.2019.107203
Mahakal, S., Pathan, H. M., Prasad, M., Rondiya, S., Patole, S. P., Jadkar, S. R. (2023). Modification in Toxicity of l-Histidine-Incorporated ZnO Nanoparticles toward Escherichia coli. ACS Omega 8 (38), 34354–34363. doi: 10.1021/acsomega.3c01183
Malic, S., Rai, S., Redfern, J., Pritchett, J., Liauw, C. M., Verran, J., et al. (2019). Zeolite-embedded silver extends antimicrobial activity of dental acrylics. Colloids Surf B Biointerfaces 173, 52–57. doi: 10.1016/j.colsurfb.2018.09.043
Mohd Farid, D. A., Zahari, N. A. H., Said, Z., Ghazali, M. I. M., Hao-Ern, L., Mohamad Zol, S., et al. (2022). Modification of polymer based dentures on biological properties: current update, status, and findings. Int. J. Mol. Sci. 23 (18), 10426. doi: 10.3390/ijms231810426
Moreno-Maldonado, V., Acosta-Torres, L. S., Barceló-Santana, F. H., Vanegas-Lancón, R. D., Plata-Rodríguez, M. E., Castaño, V. M. (2012). Fiber-reinforced nanopigmented poly(methyl methacrylate) as improved denture base. J. Appl. Polymer Sci. 126 (1), 289–296. doi: 10.1002/app.36913
Mousavi, S. A., Ghotaslou, R., Khorramdel, A., Akbarzadeh, A., Aeinfar, A. (2020). Antibacterial and antifungal impacts of combined silver, zinc oxide, and chitosan nanoparticles within tissue conditioners of complete dentures in vitro. Ir J. Med. Sci. 189 (4), 1343–1350. doi: 10.1007/s11845-020-02243-1
Nocchetti, M., Donnadio, A., Vischini, E., Posati, T., Albonetti, C., Campoccia, D., et al. (2022). Synthesis, crystal structure, and antibacterial properties of silver-functionalized low-dimensional layered zirconium phosphonates. Inorg Chem. 61 (4), 2251–2264. doi: 10.1021/acs.inorgchem.1c03565
Oleiwi, J., Hamad, Q., Kadhim, N. (2019). Study Compression, Hardness and Density properties of PMMA Reinforced by Natural Powder Used in Denture Base applications. Eng. Technol. J. 37 (12A), 522–527. doi: 10.30684/etj.37.12A.5
Oyar, P., Asghari Sana, F., Durkan, R. (2018). Comparison of mechanical properties of heat-polymerized acrylic resin with silver nanoparticles added at different concentrations and sizes. J. Appl. Polymer Sci. 135 (6), 45807. doi: 10.1002/app.45807
Patil, S. S., Patil, A. G., Benakatti, V., Patil, R. (2020). Comparative Evaluation of Adhesion of Candida albicans to Heat Cure Polymethyl Methacrylate, Self-Cure Polymethyl Methacrylate and Vacuum formed Thermoplastic Resin - An In Vitro Study. J. Evol. Med. Dental Sci. 9 (52), 3935–3938. doi: 10.14260/jemds/2020/861
Perde-Schrepler, M., Florea, A., Brie, I., Virag, P., Fischer-Fodor, E., Vâlcan, A., et al. (2019). Size-dependent cytotoxicity and genotoxicity of silver nanoparticles in cochlear cells in vitro. J. Nanomaterials 2019, 1–12. doi: 10.1155/2019/6090259
Pham, T. N., Thi Hue, N., Lee, Y. C., Huy, T. Q., Thi Thu Thuy, N., Tuan, H. V., et al. (2021). A hybrid design of Ag-decorated ZnO on layered nanomaterials (MgAC) with photocatalytic and antibacterial dual-functional abilities. RSC Adv. 11 (61), 38578–38588. doi: 10.1039/d1ra08365a
Pourbeyram, S. (2016). Effective removal of heavy metals from aqueous solutions by graphene oxide–zirconium phosphate (GO–zr-P) nanocomposite. Ind. Eng. Chem. Res. 55 (19), 5608–5617. doi: 10.1021/acs.iecr.6b00728
Pourhajibagher, M., Bahrami, R., Bazarjani, F., Bahador, A. (2023). Anti-multispecies microbial biofilms and anti-inflammatory effects of antimicrobial photo-sonodynamic therapy based on acrylic resin containing nano-resveratrol. Photodiagnosis Photodyn. Ther. 43, 103669. doi: 10.1016/j.pdpdt.2023.103669
Qin, Y., Jiang, Y., Wei, X., Ma, Y., Liao, H., Peng, Q., et al. (2023). Zirconium phosphate supported-silver nanoparticles for selective hydrogenation of nitrobenzene into azoxybenzene compounds. New J. Chem. 47 (30), 14380–14394. doi: 10.1039/d3nj00518f
Quassem, M. A., Mahross, H. Z. (2018). Comparative evaluation of porosities and solubility for different non-metallic denture base material. J. Clin. Diagn. Res 12 (10), ZC18-ZC22,18–22. doi: 10.7860/jcdr/2018/36219.12132
Ramos-Garcés, M. V., González-Villegas, J., López-Cubero, A., Colón, J. L. (2021). New applications of zirconium phosphate nanomaterials. Accounts Materials Res. 2 (9), 793–803. doi: 10.1021/accountsmr.1c00102
Rana, M. H., Shaik, S., Hameed, M. S., Al-Saleh, S., AlHamdan, E. M., Alshahrani, A., et al. (2021). Influence of dental glass fibers and orthopedic mesh on the failure loads of polymethyl methacrylate dent-ure base resin. Polymers (Basel) 13 (16), 2793. doi: 10.3390/polym13162793
Rao, S., B, T. N., Preman, N. K., Johnson, R. P., Ginjupalli, K., P, P., Prabhu, A., Das, R., K, J., et al. (2022). Synthesis, characterization, and evaluation of quaternary ammonium-based polymerizable antimicrobial monomers for prosthodontic applications. Heliy-on 8 (8), e10374. doi: 10.1016/j.heliyon.2022.e10374
Safari, M., Ghiaci, M., Rahimi, F. (2016). Preparation of rGO/ZrP as a new adsorbent in dibenzothiophene removal from n-decane with high capacities and good regenerability. RSC Adv. 6 (72), 68445–68453. doi: 10.1039/c6ra15305d
Sahm, B. D., Botelho, A. L., Agnelli, J. A. M., dos Reis, A. C. (2022). Relation of physicochemical properties and accumulation of microorganisms in acrylic resins with antimicrobial properties: a systematic review. Polymer Bull 80 (11), 11701–11713. doi: 10.1007/s00289-022-046594
Sahm, B. D., Teixeira, A. B. V., Dos Reis, A. C. (2023). Graphene loaded into dental polymers as reinforcement of mechanical properties: A systematic review. Jpn Dent. Sci. Rev. 59, 160–166. doi: 10.1016/j.jdsr.2023.06.003
Saxena, V., Diaz, A., Clearfield, A., Batteas, J. D., Hussain, M. D., Kignelman, G., et al. (2013). Zirconium phosphate nanoplatelets: a biocompatible nanomaterial for drug delivery to cancer Colloidal stability and aggregation mechanism in aqueous suspensions of tiO2 nanoparticles prepared by sol-gel synthesis. Nanoscale Langmuir 5 (51), 14846–14855. doi: 10.1021/acs.langmuir.1c02533
Schubert, A., Wassmann, T., Holtappels, M., Kurbad, O., Krohn, S., Bürgers, R. (2019). Predictability of microbial adhesion to dental materials by roughness parameters. Coatings 9 (7), 456. doi: 10.3390/coatings9070456
Shimizu, K., Kashiwada, S., Horie, M. (2022). Cellular effects of silver nanoparticle suspensions on lung epithelial cells and macrophages. Appl. Sci. 12 (7), 3554. doi: 10.3390/app12073554
Singhal, A., Dubey, K. A., Bhardwaj, Y. K., Jain, D., Choudhury, S., Tyagi, A. K. (2013). UV-shielding transparent PMMA/In2O3 nanocomposite films based on In2O3 nanoparticles. RSC Adv. 3 (43), 20913–20921. doi: 10.1039/c3ra42244e
Sinhamahapatra, A., Sutradhar, N., Roy, B., Tarafdar, A., Bajaj, H. C., Panda, A. B. (2010). Mesoporous zirconium phosphate catalyzed reactions: Synthesis of industrially important chemicals in solvent-free conditions. Appl. Catalysis A: Gen. 385 (1-2), 22–30. doi: 10.1016/j.apcata.2010.06.016
Souza Neto, F. N., Sala, R. L., Fernandes, R. A., Oliveira Xavier, T. P., Cruz, S. A., Paranhos, C. M., et al. (2019). and camargo, E Effect of synthetic colloidal nanoparticles in acrylic resin of dental use. R. d. Eur. Polymer J. 112, 531–538. doi: 10.1016/j.eurpolymj.2018.10.009
Sun, H., Jiao, R., Xu, H., An, G., Wang, D. (2019). The influence of particle size and concentration combined with pH on coagulation mechanisms. J. Environ. Sci. (China) 82, 39–46.doi:10.1016/j.jes.2019.02.021. doi: 10.1016/j.jes.2019.02.021
Sun, J., Wang, L., Wang, J., Li, Y., Zhou, X., Guo, X., et al. (2021). Characterization and evaluation of a novel silver nanoparticles-loaded polymethyl methacrylate denture base: In vitro and in vivo animal study. Dent. Mater J. 40 (5), 1100–1108. doi: 10.4012/dmj.2020-129
Wang, F., Guo, J., Li, K., Sun, J., Zeng, Y., Ning, C. (2019). High strength polymer/silicon nitride composites for dental restoration-s. Dent. Mater 35 (9), 1254–1263. doi: 10.1016/j.dental.2019.05.022
Wang, Y., Zhang, L., Sun, J., Bao, J.-B., Wang, Z., Ni, L. (2017). Dramatic toughness enhancement of polydicyclopentadiene co-mposites by incorporating low amounts of vinyl-functionalized siO2. Ind. Eng. Chem. Res. 56 (16), 4750–4757. doi: 10.1021/acs.iecr.7b00093
Wong, A., Liu, Q., Griffin, S., Nicholls, A., Regalbuto, J. R. (2017). Synthesis of ultrasmall, homogeneously alloyed, bimetallic nanoparticles on silica supports. Science 358, 1427–1430. doi: 10.1126/science.aao6538
Wu, Y.-M., Zhao, P.-C., Jia, B., Li, Z., Yuan, S., Li, C.-H. (2022). A silver-functionalized metal–organic framework with effective antibacterial activity. New J. Chem. 46 (13), 5922–5926. doi: 10.1039/d1nj06183f
Xu, Y., Zhou, F., Chen, M., Hu, H., Lin, L., Wu, J., et al. (2020). Facile assembly of 2D α-zirconium phosphate supported silver nanoparticles: superior and recyclable catalysis. New J. Chem. 44 (23), 9793–9801. doi: 10.1039/d0nj01378a
Yang, K., Xu, S., Zhao, H., Liu, L., Lv, X., Hu, F., et al. (2021). Hypoxia and Porphyromonas gingivalis-lipopolysaccharide synergistically induce NLRP3 inflammasome activation in human gingival fibroblasts. Int. Immunopharmacol 94, 107456. doi: 10.1016/j.intimp.2021.107456
Yan-Qing, W., Zhao-Jia, L., Ming-Lin, G. (2015). Hydrotherma-l synthesized microflower sodium zirconium phosphate and its tra-nsformation to zirconium hydrogen phosphate. Materials Lett. 15-9, 197–199. doi: 10.1016/j.matlet.2015.06.114
Yoshihara, K., Nagaoka, N., Umeno, A., Sonoda, A., Obika, H., Yoshida, Y., et al. (2021). Antibacterial effect of amino acid-silver complex loaded montmorillonite incorporated in dental acrylic resin. Materials (Basel) 14 (6), 1442. doi: 10.3390/ma14061442
Youssef, H. F., El-Naggar, M. E., Fouda, F. K., Youssef, A. M. (2019). Antimicrobial packaging film based on biodegradable CMC/PVA-zeolite doped with noble metal cations. Food Packaging Shelf Life 22, 100378. doi: 10.1016/j.fpsl.2019.100378
Yuan, Z.-Y., Ren, T.-Z., Azioune, A., Pireaux, J.-J., Su, B.-L. (2005). Marvelous self-assembly of hierarchically nanostructured porous zirconium phosphate solid acids with high thermal stability. Catalysis Today 105 (3-4), 647–654. doi: 10.1016/j.cattod.2005.06.038
Zafar, M. S. (2018). Wear behavior of various dental restorative materials. Materials Technol. 34 (1), 25–31. doi: 10.1080/10667857.2018.1462978
Zafar, M. S. (2020). Prosthodontic applications of polymethyl methacrylate (PMMA): an update. Polymers (Basel) 12 (10), 2229. doi: 10.3390/polym12102299
Keywords: PMMA, AgNP Ag nanoparticle, mechanical properties, antibacterial properties, biosafety
Citation: Chen X, Yan T, Sun S, Li A and Wang X (2023) The effects of nano-silver loaded zirconium phosphate on antibacterial properties, mechanical properties and biosafety of room temperature curing PMMA materials. Front. Cell. Infect. Microbiol. 13:1325103. doi: 10.3389/fcimb.2023.1325103
Received: 20 October 2023; Accepted: 04 December 2023;
Published: 20 December 2023.
Edited by:
Ana Oliveira, University of Minho, PortugalReviewed by:
Payal Gupta, Graphic Era University, IndiaPurusottam Mishra, Central University of Rajasthan, India
Copyright © 2023 Chen, Yan, Sun, Li and Wang. This is an open-access article distributed under the terms of the Creative Commons Attribution License (CC BY). The use, distribution or reproduction in other forums is permitted, provided the original author(s) and the copyright owner(s) are credited and that the original publication in this journal is cited, in accordance with accepted academic practice. No use, distribution or reproduction is permitted which does not comply with these terms.
*Correspondence: Tongtong Yan, NDE5NDEzOTg0QHFxLmNvbQ==; Xiaorong Wang, d2FuZ3hyMTM1QGhvdG1haWwuY29t