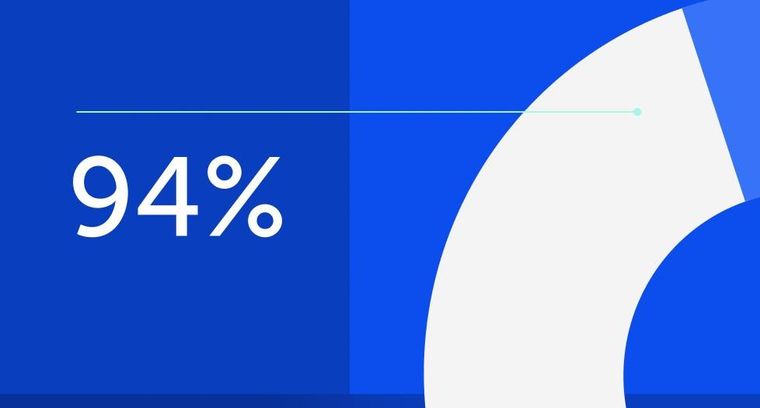
94% of researchers rate our articles as excellent or good
Learn more about the work of our research integrity team to safeguard the quality of each article we publish.
Find out more
REVIEW article
Front. Cell. Infect. Microbiol., 15 December 2023
Sec. Intestinal Microbiome
Volume 13 - 2023 | https://doi.org/10.3389/fcimb.2023.1296713
This article is part of the Research TopicNew Techniques in Microbiome Research - Vol II – Host-Microbiome Interactions using ‘meta-omics’ techniquesView all 19 articles
The human gastrointestinal tract is inhabited by a diverse range of microorganisms, collectively known as the gut microbiota, which form a vast and complex ecosystem. It has been reported that the microbiota-gut-brain axis plays a crucial role in regulating host neuroprotective function. Studies have shown that patients with Parkinson’s disease (PD) have dysbiosis of the gut microbiota, and experiments involving germ-free mice and fecal microbiota transplantation from PD patients have revealed the pathogenic role of the gut microbiota in PD. Interventions targeting the gut microbiota in PD, including the use of prebiotics, probiotics, and fecal microbiota transplantation, have also shown efficacy in treating PD. However, the causal relationship between the gut microbiota and Parkinson’s disease remains intricate. This study reviewed the association between the microbiota-gut-brain axis and PD from the perspectives of humoral pathway, cellular immune pathway and neuronal pathway. We found that the interactions among gut microbiota and PD are very complex, which should be “multidirectional”, rather than conventionally regarded “bidirectional”. To realize application of the gut microbiota-related mechanisms in the clinical setting, we propose several problems which should be addressed in the future study.
Parkinson’s disease (PD) is the leading movement disorders and the second leading neurodegenerative disease (NDD), with which patients are expected to be over doubled in the next 20 years (Asakawa et al., 2016b; Qin et al., 2022). The activity of daily living (ADL) and quality of life (QOL) in the advanced patients are significantly affected, however, more officious treatments are under developing. Till now, dopaminergic medicine and surgical therapy like deep brain stimulation (DBS) remain the mainstay treatments against PD (Qin et al., 2022). There has been no revolutionary breakthrough in terms of new therapy since 1967 levodopa and 1993 DBS emerged. It is difficult to obtain a novel but satisfactory treatment against PD because of the extreme complex and multifold pathophysiological mechanisms of PD, which remains unclear by far (Qin et al., 2022). Hence, clarifying the PD related mechanisms has been an urgent task faced by the global PD researchers. Although many researchers have attempted to interpret PD related mechanisms from multidimensions, the substantial pathogenesis (we call it “neurotoxic environment”) to cause apoptosis of the dopaminergic neurons in the substantial nigra remains intricate. Aging related neurodegeneration seems to be a reasonable interpretation for many PD related changes. However, the factor of aging cannot elucidate those patients with PD onset in a young age. Many animal models were established according to the known neurotoxins. Unfortunately, none of these models can completely imitate the human’ PD (Asakawa et al., 2016a), which implies that neurotoxin might not be a main cause of this “neurotoxic environment”. It seems to indicate that the “neurotoxic environment” is made from many factors. PD is the final comprehensive result of the complicated interaction/crosstalk among these factors. Other than the conventional environmental and genetic pathogenic factors, recently, the roles of gut microbiota and the related microbiota-gut-brain axis come into the picture, particularly with the development of the high-throughput sequencing (Tan et al., 2022).
The relationship between gastrointestinal (GI) dysfunction and PD was noticed even by James Parkinson, who first reported PD in 1817 (Tan et al., 2022). It is reported that GI dysfunction is consistently found in 80% early PD patients (Fasano et al., 2015; Killinger et al., 2018). Gut microbiota represents a huge community of bacteria residing in the GI tract (Jia et al., 2021). The total amount of gut microbiota genes is approximately 100 times than that of human genes and is known as the “second genome” of humans (Moeller et al., 2012; Komaroff, 2018). Using the technology like high-throughput sequencing, investigation regarding the microbiota-gut-brain axis stepped into a new era. Recently, a growing number of studies have elucidated the PD-related metabolic changes of gut microbiota are closely associated with the occurrence and development of PD (Shen et al., 2021; Tan et al., 2021). These “impaired” gut microbiota along with their relevant metabolites are believed to interact with the host, mainly via the microbiota-gut-brain axis, and finally become a pathogenic factor of PD (Mayer et al., 2015). Additionally, many later studies suggested that gut microbiota can be considered as the diagnostic/therapeutic biomarkers for PD, or biomarkers to display the PD progression (Schapira, 2013; Neurology, 2016; Nair et al., 2018). However, due to the characteristics of gut microbiota, somewhile it is difficult to make a directed interaction to change the behaviors of the gut microbiota. Hence, development of a medication against PD on the basis of the gut microbiota related mechanisms are quite difficult. Recently, Tan and her colleagues published an insightful study discussing the available evidence regarding the microbiome–gut–brain axis and PD (Tan et al., 2022). This study critically reviewed the complex interaction/crosstalk between the brain and gut via the microbiome–gut–brain axis. Based on this study, we motivated this review focusing on the association between gut microbiota and PD from the perspectives of humoral pathway, cellular immune pathway and neuronal pathway. We attempted to provide the wider and update information on this topic. We believe these take-home- messages will deepen the understanding the roles of gut microbiota in the PD scenario, and will be helpful for developing novel diagnostic/therapeutic targets against PD based on the gut microbiota.
Clinically, many PD patients suffer from GI dysfunction, this might be the first noteworthy symptoms which drew the attention of the clinicians to investigate the role of GI in PD. Indeed, whether it is the GI dysfunction causes PD, or conversely PD causes GI dysfunction is a chicken-and-egg problem. Although growing evidence elucidated that dysbiosis of gut microbiota plays a pathogenic role in development of PD, where the microbiota-gut-brain axis plays a key role, nowadays, the mainstream opinion is that the association between PD and Gut microbiota is bidirectional (Tan et al., 2022): Certainly, dysbiosis of gut microbiota may lead PD development, and GI dysfunction may be a non-motor symptom in PD. Importantly, dysbiosis of gut microbiota exhibits complicated interactions with PD pathophysiology, and comprehensively drives the PD progress.
PD patients may experience many symptoms of GI dysfunction, such as constipation, delayed gastric emptying, altered bowel habits, and nausea (Marrinan et al., 2014; Pellegrini et al., 2015; Mrabet et al., 2016). Indeed, these symptoms have been noticed as non-motor symptoms in PD. It has been reported that PD patients are commonly accompanied with and infection of Helicobacter pylori infection and peptic ulcer (Rees et al., 2011; Tan et al., 2020). Interestingly, when the patient-derived α-synuclein (α-syn) were stereotactically injected into the striatum or enteric nerves in a non-human primate animal, both injections would cause lesions in the nigrostriatal pathway and enteric nervous system (Arotcarena et al., 2020). This finding indicates that exposure of α-syn may results in pathological changes, not only in brain, but also in GI. Numerous studies revealed the abnormality of the gut microbiota in PD. Structure and function of the gut microbiota in PD patients are quite different in comparison with the healthy subjects among these studies (Table 1). The heterogeneous results indicate that the changes of gut microbiota are affects by many complicated factors such as age, geographic provenance, dietary habit, research protocols and sequencing methods (Maier et al., 2018; Boertien et al., 2019). Of those, Akkermansia was reportedly increased in PD patients (Gerhardt and Mohajeri, 2018; Baldini et al., 2020; Nishiwaki et al., 2020; Vascellari et al., 2020; Zhang et al., 2020). Meanwhile, abundance of Bifidobacterium was reportedly increased (Vascellari et al., 2020; Li et al., 2021; Tan et al., 2021; Yan et al., 2021b). Roseburia and Prevotella were observed to be significantly decreased in PD patients (Nishiwaki et al., 2020; Vascellari et al., 2020; Zhang et al., 2020; Li et al., 2021), and a decrease in Prevotella was observed, which was negatively correlated with PD pathogenesis (Hasegawa et al., 2015). Additionally, many studies indicated close association between dysbiosis of gut microbiota and PD-related clinical manifestations like PD severity, duration, motor and nonmotor symptoms (Ren et al., 2020; Zheng et al., 2021).
Put another way, dysbiosis of gut microbiota is also observed in PD animal models (Table 2), albeit all the animal models available nowadays cannot completely imitate the pathophysiological changes in PD patients (Asakawa et al., 2016a). Similar to PD patients, Akkermansia was also increased (Gorecki et al., 2019; Yan et al., 2021a; Zhao et al., 2021), whereas abundance of Prevotella was reportedly decrease in these animal models (Sampson et al., 2016; Joers et al., 2020; Yan et al., 2021a). Similar to the scenario in Human patients, the changes of gut microbiota in animal models are also influenced by many factors such as neurotoxin used, and administrating approaches, dose, and timing.
Some authors believed that specific gut microbiota might be considered as a potential marker and therapeutic target for PD because the changes of PD-related gut microbiota are closely related to the occurrence and development of PD (Ji et al., 2021). However, due to the heterogeneous nature of these results, no matter in human patients and in animal models, till now, there remains no compelling evidence supporting which gut microbiota can be served as a diagnostic/therapeutic target against PD. In addition, the aforementioned results are derived from investigations with small samples in different experimental conditions. Moreover, they cannot answer the chicken-and-egg problem, namely whether the pathophysiological changes of PD cause dysbiosis of gut microbiota, or vice versa. Hence, before we can conclude the roles of gut microbiota in PD, much more deep and rigorous investigations are desired.
The interaction/crosstalk between brain and gut microbiota is increasingly attended. Gut microbiota is living in a relatively balanced environment in a normal human, which may contribute to modulation of the neurological functions such as learning, cognitive and memory (Parashar and Udayabanu, 2017; Cryan et al., 2020). Cryan et al. reported that gut microbiota can regulate intellectual and behavioral development, and is associated with some neurological disorders (Cryan et al., 2019). A number of investigations recently documented that complete absence or severe depletion of the gut microbiota may result in impairments of learning and memory in the hosts (Chu et al., 2019; Yu et al., 2019; Liu et al., 2020). Olson et al. found that co-treatment of ketogenic diet and hypoxia can change the gut microbiota with bilophila wadsworthia enriched, then promote cognitive impairment in germfree (GF) mice (Olson et al., 2021). Wu et al. reported that enterococcus faecalis can affect social behavior through discrete neuronal circuits and mediate stress response in GF mice (Wu et al., 2021c).
In the scenario of PD, many studies also elucidated a close association between PD and gut microbiota. Sampson et al. reported that gut microbiota regulates motor deficits and neuroinflammation in α-syn overexpressed mice. Importantly, once the gut microbiota derived from PD patients was transplanted into these models, the neurological deficits were remarkably enhanced (Sampson et al., 2016). Wekerle reported that Escherichia coli and Salmonella typhimurium may induce secretion of the Curli protein and trigger accumulation of α-syn in aged Fischer 344 rats and Caenorhabditis elegans (Wekerle, 2017). Later, Sun et al. found that fecal microbiota transplantation (FMT) resulted in attenuation of microglial activation, decrease of short-chain fatty acids (SCFAs) and caused motor deficits (Sun et al., 2018). Clinically, it has been documented that gut microbiota can influence PD severity (Malkki, 2017; Rani and Mondal, 2021).
These aforementioned reports suggested a close link between PD and gut microbiota from multidimensions. However, direct evidence elucidating PD and gut microbiota remains insufficient. In this regard, it is indispensable to discuss the potential roles of several factors involved in the interactions between PD and gut microbiota.
Recently, Tan et al. reviewed the potential roles of microbiota-gut-brain in the PD scenario (Tan et al., 2022). Indeed, microbiota-gut-brain axis plays a key role in the interactions between gut microbiota and brain. It is known that at least four systems involved in the modulation of the microbiota-gut-brain axis, namely central nervous system (CNS), autonomic nervous system (ANS), hypothalamic pituitary adrenal (HPA) axis, and enteric nervous system (ENS) (Cryan et al., 2019; Heiss and Olofsson, 2019; Rutsch et al., 2020; Doroszkiewicz et al., 2021). Delivery of inflammatory signals plays a key role in the interaction between brain and gut microbiota (Agirman et al., 2021). A conventional theory is that dysbiosis of gut may induce peripheral inflammation, the inflammatory signals can be delivered into the brain via these pathways thereby cause the inflammatory response in CNS, ultimately form the “neurotoxic environment” in the brain, which might be a pathogenic factor causing PD. However, there is no direct evidence proving that dysbiosis of gut microbiota might cause PD. Several hypothetic pathways were introduced contributing the inflammatory interactions among brain, gut and microbiota, including humoral pathway, cellular immune pathway and neuronal pathway (Agirman et al., 2021). However, these pathways are also problematic in terms of PD pathophysiology (Figure 1).
Figure 1 Microbiota-gut-brain axis-related PD pathology. Intestinal microbiota along with its derivatives play a role in the pathology of PD. The emerging mechanisms include modulation of the gut environment, maintenance of the ecological balance among the gut microbiota, and effects on the neurotransmitters, inflammatory factors, and related metabolites within the gut. The potential influence pathways were i) the vagus nerve conveys α-syn; ii) the neuroendocrine pathways primarily involve the HPA axis, and the circulation-mediated neurotransmitters such as serotonin (5-HT) and gamma-aminobutyric acid (GABA); iii) the immune system regulates immune cells and mediates the release of inflammatory factors; and iv) the circulation system transports BAS, SCFAs, LPS, and hydrogen.
Agirman et al. introduced that dysbiosis of gut microbiota may trigger release of pro-inflammatory cytokines and disrupt the blood brain barrier (BBB) integrity, then make a gateway which may introduce peripheral toxins, and pathogens into brain and cause neuroinflammation (Agirman et al., 2021). Meanwhile, such neuroinflammation may trigger release of glucocorticoid and deteriorate the environment of gut microbiota. These bidirectional regulations create a vicious circle. However, this pathway is mainly based on the BBB damage. If the BBB is relative unbroken, the interactions between CNS and peripheral blood are limited. Albeit a recent report indicated that BBB damage was found in PD with dementia (Wong et al., 2022), there is no direct clinical evidence showing the BBB damage in PD without cognitive impairment, particularly in early stage of PD. Hence, other than the cytokines, liposoluble small molecules might play a more important role in the humoral pathway, such as metabolites in the tryptophan–kynurenine pathway (KP), SCFAs, and bile acids (BAs) (Qin et al., 2022).
Tryptophan is an essential amino acid which approximately over 95% is metabolized through KP whereas less than 5% is generates 5-HT. Importantly, both metabolites in KP and 5-HT are proven to be closely associated with the PD pathophysiology.
Recently, we reviewed the role of KP in the pathogenicity of PD (Qin et al., 2022). Both aging and dysbiosis of gut microbiota may activate some rate-limiting enzymes in KP, such as indoleamine 2,3-dioxygenase 1 (IDO1) and tryptophan 2,3-dioxygenase (TDO). Activation of KP will result in enhancement of the downstream toxins, such as 3-hydroxykynurenine, 3-hydroxyanthranilic acid quinolinic acid. Although these toxins are mainly produced peripherally, the liposoluble nature with small molecular weight make they are easily cross the BBB and accumulate in the CNS, thereby exhibiting neurotoxic effects. Hence, at the state of BBB remains unbroken, KP may act as an “aging signal deliverer”, particularly deliver inflammatory signal from the periphery to the CNS. Close association among KP, α-syn, and PD has been well documented at this review (Qin et al., 2022). However, there remains no direct evidence supporting the activation of KP will cause α-syn deposition and ultimately cause PD (Qin et al., 2022).
As a minor production of the tryptophan metabolism, 5-HT cannot directly cross BBB. Hence, the 5-HT in CNS is generated in the CNS in case of BBB is unbroken. Many studies reported the close association between 5-HT and gut microbiota. It is documented that the hippocampal 5-HT and its’ main catabolic product levels increase whereas plasma tryptophan, and tryptophan metabolism via KP decrease in the GF mice (Wikoff et al., 2009; Diaz Heijtz et al., 2011). Animal experiments demonstrated that after administration of antibiotics in GF mice, the levels of 5-HT in colon and feces decreased, nevertheless reconstruction of gut microbiota restored 5-HT levels in these animals (Yano et al., 2015; Ge et al., 2017). Desbonnet et al. found that Bifidobacterium infantis can increase plasma tryptophan level, which may influence central 5-HT transmission (Desbonnet et al., 2010). These results imply that 5-HT is remarkably affected by the gut microbiota. Some gut microbiota may enhance the peripheral tryptophan level, which can cross BBB, and subsequently increase the tryptophan level in CNS, and finally increase the 5-HT level in CNS. In terms of the connection of 5-HT and PD, Tong et al. found that plasma 5-HT levels in PD patients were lower than those in healthy control, and 5-HT levels were negatively correlated with the severity of non-motor symptoms like depression and pain (Tong et al., 2015). Kotagal et al. found that 5-HT may change Aβ metabolism and reduce the risk of PD related cognitive impairment (Kotagal et al., 2018). Lelieveld et al. reported that 5-HT may influence the sleep disordered breathing in PD (Lelieveld et al., 2012). These findings seem to imply the potential associations between 5-HT and the non-motor symptoms in PD, however, require further verification.
SCFAs, such as acetic, propionic, butyric, isobutyric, valeric, and isovaleric are commonly produced by gut microorganisms from fermentation of dietary fiber by gut microorganisms (Cummings et al., 1987; Bergman, 1990; Miller and Wolin, 1996). It was documented that Bifidobacterium, Clostridia, Lactobacillus, Bacteroides, and Faecalibacterium can produce SCFA (Hold et al., 2003; Chambers et al., 2015; Koh et al., 2016; Yang et al., 2020). SCFAs, serve as the primary nutrients of the colonocyte, play a fundamental role in maintaining intestinal homeostasis and regulating energy metabolism (Cummings and Macfarlane, 1997; Priyadarshini et al., 2018). Recently, the SCFAs were proposed as key mediators of microbiota-gut-brain interactions (Dalile et al., 2019). SCFAs are commonly produced in the gut. They can cross BBB, thereby can regulator the inflammation reactions in both periphery and CNS. Peripherally, SCFAs contribute to regulating intestinal permeability and enhancing the integrity of intestinal barrier. Kim et al. found that SCFAs activate G protein coupled receptor (GPR) 41 and GPR43 on intestinal epithelial cells, lead to the production of chemokines and cytokines, and mediate protective immunity and tissue inflammation in mice (Kim et al., 2013). Butyrate administration can affect the expression of tight junction proteins thereby maintain the integrity of intestinal barrier (Plöger et al., 2012; Wang et al., 2012). Additionally, butyrate ministration can increase the levels of tight junction protein and improve intestinal permeability via GPR109A (a G-protein coupled receptor) (Karunaratne et al., 2020). In CNS, SCFAs display the analogous protective effects on BBB. Braniste et al. reported that administration of Clostridium butyricum (mainly produces butyrate) and Bacteroides thetaiotaomicron (mainly produces acetate and propionate) resulted in decrease of the BBB permeability in the frontal cortex, hippocampus, and striatum, along with upregulation of the occludin expression in GF mice, indicating a protective effect on BBB (Braniste et al., 2014). In addition, SCFAs interacting with immune cells, contribute to reduction of the systemic inflammation, subsequently alleviation of the neuroinflammation (Segerstrom and Miller, 2004; Miller and Raison, 2016; Parada Venegas et al., 2019). Moreover, SCFAs constantly modulate maturation and function of microglia in CNS (Smith et al., 2013; Borre et al., 2014). These findings suggest a neuroprotective effect of SCFAs.
Numerous studies elucidated the effects of SCFAs on PD. Aho et al. found the SCFAs levels in PD patients were lower in comparison with healthy people, which were associated with gastrointestinal inflammation and the development of PD (Aho et al., 2021). Another study found that anti-inflammatory butyrate-producing bacteria such as genera Blautia, Coprococcus, and Roseburia were lower, whereas pro-inflammatory Proteobacteria of the genus Ralstonia were higher in the sigmoid mucosa of the PD patients (vs healthy controls). They therefore verified the involvement of pro-inflammatory dysbiosis of gut microbiota in the PD pathophysiology, particularly in the inflammation-induced α-syn misfolding (Keshavarzian et al., 2015). These human results seem to imply the neuroprotective effects of SCFAs on PD, which were also verified by several in vitro studies (Chen et al., 2006; Sechi et al., 2008; Fu et al., 2015; Jang et al., 2017). Evidence from the current animal studies also proved the significant effects of SCFAs on PD subjects. However, the results are controversial among studies. Hou et al. found that administration of sodium butyrate achieved suppression of the neuroinflammation and alleviation of the neurological deficits in 1-Methyl-4-phenyl-1,2,3,6-tetrahydropyridine (MPTP) treated mouse PD models (Hou et al., 2021a). Later, they also found that oral administration of gut microbiota-derived propionate resulted in amelioration of the motor deficits and depletion of the dopamine neurons in 6-hydroxydopamine (6-OHDA) induced mouse PD models (Hou et al., 2021b). Conversely, Cannon et al. found the enhancement of butyric acid level and reduction of isobutyric acid level in a Pink1−/- PD mouse (Cannon et al., 2020). Qiao et al. found that administration of sodium butyrate resulted in deterioration of the motor deficits and neuroinflammatory by increasing the number of microglia and activated astrocytes (Qiao et al., 2020). These contradictory results imply an extremely complex nature of SCFAs under a PD condition. The problem whether SCFAs play a neuroprotective or neurotoxic role remains still requires further investigation.
BAs are synthesized by the hepatic cells and secreted to the intestine through the bile duct. Primary BAs are transformed into secondary BAs under the action of gut microbiota in the terminal ileum and colon (Russell and Setchell, 1992; Ridlon et al., 2006). BAs can cross the BBB. It has been reported that BAs are distributed in the brain in human and animals, of which approximately 20 BAs were identified in the rat brain (Xie et al., 2013; Zheng et al., 2016; Pan et al., 2017). Chenodeoxycholic acid (CDCA) and cholic acid (CA) are the major BAs in the rat’s brain (Mano et al., 2004; Zheng et al., 2016). BAs can affect the immune regulation in CNS (Fiorucci et al., 2010). Currently, many studies proved the potential neuroprotective effects of BAs in several neurological diseases. Many studies have demonstrated the potential neuroprotective effects of BAs in various neurological diseases. Furthermore, BAs have been found to be involved in cognitive functions such as learning and memory (Abrams et al., 2006). For instance, intracerebroventricular administration of tauroursodeoxycholic acid (TUDCA) in adult rats significantly enhanced early neurogenesis (Soares et al., 2018). In a mouse model of PD, TUDCA prevented JNK phosphorylation in the midbrain and striatum by regulating Akt signaling and prevented MPTP-induced dopaminergic cell death (Castro-Caldas et al., 2012). Additionally, TUDCA was found to activate Nrf2 to prevent oxidative stress induced by 1-methyl-4-phenylpyridinium and α-syn in an in vitro experiment using SH-SY5Y cells (Moreira et al., 2017). TUDCA was also reported to induce mitochondrial autophagy by increasing the expression of PINK1, parkin, and LC3-II/LC3-I in another experiment (Rosa et al., 2017). In a transgenic mouse model of PD, TUDCA reduced striatal cell apoptosis and improved locomotor ability (Keene et al., 2002). Interestingly, TUDCA administration improved cognitive impairment in db/db mice (Liu et al., 2020). Moreover, UDCA was found to have anti-inflammatory potential in the rotenone model of PD, reducing rotenone-induced NF-κB expression and TNF-α levels (Abdelkader et al., 2016). In the study of major depressive disorder, activating the bile acid receptor FXR (farnesoid X receptor) was reported to regulate neuroimmunity and inhibit brain inflammation (Bao et al., 2021). The latest research shows that obeticholic acid (OCA), a derivative of BAs, reduced the expression of neuroinflammatory microglia and IL-1 in the hippocampus (Wu et al., 2021b). Several studies have suggested that BAs may play a role in cognitive dysfunction (Chen et al., 2020b; Jena et al., 2020). In summary, BAs may exert their effects on PD through anti-inflammatory and neuroimmunomodulatory mechanisms.
It is known that BAs and gut microbiota affect mutually. Commonly, BAs are modified by the microorganisms via certain enzymatic reactions. It is easy to understand that the abnormal state of gut microbiota in PD context may affect the modification of BAs. However, the direct evidence remains insufficient. Previous studies reported that several bile salt hydrolase-containing bacteria, such as Bacteroides spp., Bifidobacterium, and Lactobacillus, were enhanced in PD patients (Spichak et al., 2021; Ghezzi et al., 2022), indicating a complicated association among PD, microbiota, and BAs (Kiriyama and Nochi, 2023), which is still unclear. Wang et al. documented that BAs might play a role as a “star molecule” in the mechanisms of microbiota-gut-brain axis, which involved in the interactions between gut microbiota and PD (Wang et al., 2023). (5) The roles of tryptophan metabolites γ- Aminobutyric acid.
GABA (γ-Aminobutyric acid), also known as 4-aminobutanoic acid, is a non-protein amino acid and the primary inhibitory neurotransmitter in the mammalian central nervous system(Hyland and Cryan, 2010; Vithlani et al., 2011; Muthukumar et al., 2014). Besides the brain tissue, GABA is present in peripheral tissues such as the heart, stomach, small intestine, liver, and kidney (Akinci and Schofield, 1999; Lakhani et al., 2014). GABA possesses antioxidant, anticonvulsant, anti-inflammatory, analgesic, sedative, and anxiolytic properties (Rorsman et al., 1989; Adeghate and Ponery, 2002; Lacerda et al., 2003; Menezes and Fontes, 2007; Ben-Othman et al., 2017), and has been reported to enhance brain and nervous system function, as well as cognitive and memory functions (Yamatsu et al., 2016; Kim et al., 2019a; Inotsuka et al., 2021). Recent studies have found that GABA produced by gut microbiota (Bacteroides, Parabacteroides, Escherichia species, Lactobacilli, and Bifidobacteria) can participate in neural activities through the microbiota-gut-brain axis (Yunes et al., 2016; Strandwitz et al., 2019). An animal experiment using probiotics found that L. rhamnosus (JB-1) can induce region-dependent changes in GABA mRNA expression in the brain through the vagus nerve, and reduce stress-induced corticosterone and anxiety- and depression-related behavior (Bravo et al., 2011). GF mice were found to have significantly reduced levels of GABA in feces and blood, and antibiotics were found to change the level of GABA in feces, suggesting that the microbiota may contribute to the circulating level of GABA (Matsumoto et al., 2017; Fujisaka et al., 2018). A study on Caenorhabditis elegans found that GABA produced by Escherichia coli HT115 has a protective effect on neurons (Urrutia et al., 2020). while a previous study on learning in bees reported that the brains of bees colonized with the heritable Bifidobacterium strain displayed an increased level of GABA (Wu et al., 2021a). It has been reported in the literature that GABA plays a crucial role in protecting the intestinal barrier and preventing gastrointestinal mucosal inflammation (Xie et al., 2017; Chen et al., 2019). GABA may contribute to regulation of the population and diversity of gut microbiota, such as enhancement of the dominant microorganism populations, enrichment of the microbial community (Chen et al., 2019). In addition, GABA may improve the colon health by enhancement of the levels of acetate, propionate, butyrate and total short-chain fatty acids, along with decrease of colonic and cecal pH values (Xie et al., 2017). Recent clinical trials and animal studies have shown that GABA plays an important role in the pathogenesis of PD. An experiment examining the GABA levels of PD patients and healthy controls found that the GABA levels in the upper brainstem of PD patients were significantly lower than those of healthy controls (Song et al., 2021b). while a preliminary study also found that a single dose of L-dopa increases upper brainstem GABA in PD (Song et al., 2021c). Some experts suggest that the reduced GABA levels in PD patients may be associated with GABAergic dysfunction (Elmaki et al., 2018). Animal experiments have also reported that polymannuronic acid regulates GABA levels through the microbiota-gut-brain axis in PD mice to prevent the loss of dopaminergic neurons (Dong et al., 2020). Based on the aforementioned information, we can hypothesize that GABA plays an important role in the pathogenesis of PD mediated by the microbiota-gut-brain axis.
LPS, also known as endotoxin, is an integral structural component of the outer membrane of gram-negative bacteria (Raetz and Whitfield, 2002). Dysbiosis of the microbiota leads to increased intestinal permeability, allowing LPS products to translocate from the intestinal lumen into the host circulation (Rietschel et al., 1994; Tilg et al., 2016). Several studies have found a significant increase in gram-negative bacteria in PD patients, which is closely related to intestinal inflammation (Guo et al., 2015; Nighot et al., 2017; Gorecki et al., 2019). Furthermore, a study found a positive association between the relative abundance of gram-negative Enterobacteriaceae and the severity of postural instability and gait difficulty (Scheperjans et al., 2015). An animal experiment demonstrated that injecting LPS into the rat substantia nigra can activate microglia, trigger the inflammatory response, increase the expression of pro-inflammatory cytokines, and change the activity of oxidative stress markers, inducing the characterization of a PD model (Sharma and Nehru, 2015). Different routes and modes of LPS administration can cause various parkinsonian symptoms. Intraperitoneal injection of LPS can increase α-Syn expression in the large intestine and intestinal permeability (Kelly et al., 2014). The PD model induced by endotoxin can be established by unilateral intranasal drip of LPS. These mice showed progressive hypokinesia, selective loss of dopaminergic neurons (He et al., 2013), decreased dopamine (DA) content in the striatum, and α-Syn aggregates without systemic inflammation and immune response (He et al., 2013). Intra-rectal injection of LPS derived from P. mirabilis can induce inflammation in the nigrostriatal regions of the brain (Choi et al., 2018). Intestinal biopsy of PD patients revealed a close relationship between increased intestinal permeability and intestinal α-syn and LPS (Forsyth et al., 2011). Moreover, a series of in vitro and in vivo experiments proved the relationship between LPS and intestinal permeability and the associated inflammatory signals (Guo et al., 2013; Nighot et al., 2017; Nighot et al., 2019). Previous research has shown that inflammatory monocytes primed with LPS can internalize α-syn, which in turn facilitates its dissemination from the periphery towards the brain and spinal cord (Peralta Ramos et al., 2019). In conclusion, it is speculated that LPS releases inflammatory factors and induces α-syn accumulation in the intestine, which then reaches the brain through the circulatory system, resulting in PD-related pathological changes.
Hydrogen is a metabolite of bacterial fermentation in the gut and has been shown to have selective antioxidant and anti-inflammatory effects (Levitt, 1969; Ohsawa et al., 2007). Furthermore, it has been found to possess anti-apoptotic and cytoprotective properties, contributing significantly to cell protection (Xie et al., 2010; Dixon et al., 2013). In a rat model of PD, treatment with hydrogen was reportedly neuroprotective and prevented dopaminergic cell loss (Fu et al., 2009). Mice models and PD patients also showed similar findings (Fujita et al., 2009; Yoritaka et al., 2013). An animal experiment demonstrated that drinking hydrogen water and intermittent hydrogen exposure can prevent 6-hydroxydopamine-induced PD in rats (Ito et al., 2012). Moreover, evidence has shown that the generation and oxidation of H2 help maintain the diversity of the gut microbiome (Hylemon et al., 2018). A study discovered that probiotics can promote the intestinal production of hydrogen and improve metabolic syndrome in C57BL/6J mice (Zhao et al., 2019). Analysis of 16S rRNA gene isolates indicated a low abundance of hydrogen-producing bacteria in PD patients (Hasegawa et al., 2015). Gas chromatography measurements showed that compared to the control group, the intestinal hydrogen production of PD patients was 2.2 times lower (Suzuki et al., 2018). A clinical trial found that oral intake of lactulose can increase the concentration of hydrogen in PD patients (Ito et al., 2012). Subsequent studies provided evidence that lactulose may increase hydrogen production by the intestinal microbiota (Ohno et al., 2012). These findings suggest that the abundance of intestinal hydrogen-producing bacteria may be related to the occurrence and development of PD. Therefore, intestinal-derived hydrogen may serve as a novel candidate non-invasive biomarker for diagnosis, prognosis, and treatment response in PD.
It is known that the immune system, no matter innate immune system and adaptive immune system, is closely associated with the gut microbiota. Gut microbiota plays a key role in maintaining intestinal mucosal barrier and contributes to maintenance and development of the immune system (Fernández et al., 2003; Candela et al., 2008; Olszak et al., 2012). Immune function of gut microbiota initiates in the intestine and then regulates systemic immunity during the immune response (Dai et al., 2020). A number of early studies documented that implantation of segmented filamentous bacteria from the small intestine resulted in recovery of the immune function of intestinal B and T lymphocytes (Umesaki et al., 1995; Talham et al., 1999; Umesaki et al., 1999). Later, Forsythe found that implantation of the intestinal microorganism achieved reconstruction of the immune function, which is deficient in a GF mouse (Forsythe et al., 2010). Nevertheless, immunoreactions in CNS and the peripheral organs are relatively independent under the condition that BBB is unbroken. Due to aforementioned BBB state in PD, here, we only discuss the inherent cellular immune pathway in CNS.
Microglia are key immune cells in the CNS, which contribute to presentation of antigen, production of cytokines, and activation/regulation of inflammation (Chen et al., 2020a; Jiang et al., 2020). Numerous studies have elucidated that microglia are remarkably affected by changes of gut microbiota. Erny et al. found that the proportions of immature microglia in GF mice increased in GF mice. Interestingly, once the gut microbiota in these GF mice was recovered, the features of microglia were accordingly recovered (Erny et al., 2015). Alternatively, knockout of the microglia-specific gene MafB upregulated the expression of the inflammatory pathways in adult GF mice (Matcovitch-Natan et al., 2016). Song et al. found that dysbiosis of gut microbiota caused suppression of the microglia activation and upregulation of the TLR4/NF-κB inflammatory pathway in the hippocampus of aging rats (Song et al., 2021a). Accordingly, treatment of FMT can alleviate the dysbiosis of gut microbiota and resulted in suppression of the microglia activation (Sun et al., 2018). These results reveal a close interaction between microglia and gut microbiota, even the aforementioned experiments were using the animals which BBB seems to be unbroken. Many authors believed that the bidirectional interactions between microglia and gut microbiota might play a role in the etiology of neurodegenerative diseases (Balducci and Forloni, 2018; Davoli-Ferreira et al., 2021; Wang et al., 2021b; Zheng et al., 2023). In the PD scenario, this hypothesis is also supported by many experiments. Sampson et al. proved that gut microbiota plays a role in the motor deficits, microglia activation and α-syn pathology in a α-syn overexpressed mouse (Sampson et al., 2016). Fang et al. found that using the mouse strain MG1363-pMG36e-GLP-1 expressing glucagon-like peptide-1 to treat MPTP-treated mice resulted in amelioration of the motor deficits, suppression of the microglia activation and inflammation, reduction of the pathogen Enterobacteriaceae, and enhancement of the probiotics Lactobacillus and Akkermansia (Fang et al., 2019). Joers et al. treated the PD monkey models with a novel TNF inhibitor, XPro1595. They found that the diversity of gut microbiota, inflammatory, and the microglial behavior were correspondingly changed (Joers et al., 2020). Sun et al. found that oral administration of Clostridium butyricum achieved improvement of the motor deficits, dopamine neuron depletion, synaptic dysfunction and microglia activation in MPTP treated mice (Sun et al., 2021a). All these experiments verified the close relationship between microglia and gut microbiota under the PD condition from various treatments and animal models. Hence, Claudino Dos Santos et al. recently commented that inflammatory reactions may activate microglia and induce aggregation of α-syn, ultimately cause damage of dopaminergic neurons, where dysbiosis of gut microbiota plays a key role (Claudino Dos Santos et al., 2023). There is no clinical evidence investigating the roles of microglia during the intervention of gut microbiota in PD patients. Importantly, there is no study observing changes of the gut microbiota once the microglia in PD are intervened. The complicated interactions among microglia, PD, and gut microbiota are still intricate, which require further investigation in the future.
As the most abundant cells in CNS, astrocytes also play a role in the regulation of the inflammatory and immune reactivity. Interactions between astrocytes and gut microbiota are also reported. Rothhammer et al. reported that dietary tryptophan is metabolized into aryl hydrocarbon receptor agonists by the gut microbiota, and then act on astrocytes to suppress CNS inflammation (Rothhammer et al., 2016). Zhang et al. found that FMT from the NLRP3 KO gut microbiota significantly ameliorated the depression-like behaviors along with astrocyte dysfunction via suppression of the circular RNA HIPK2 (circHIPK2) expression in animal depression models. They hence proposed a gut microbiota-circHIPK2-astrocyte axis in depression (Zhang et al., 2019). Sanmarco et al. found an astrocyte subset, LAMP1+TRAIL+ astrocytes suppressed the CNS inflammation by inducing T cell apoptosis, while these LAMP1+TRAIL+ astrocytes are maintained by the microbiome-licensed meningeal IFN+ NK cells (Sanmarco et al., 2021). Margineanu et al. reported that the hippocampal expression of astrocyte-neuron lactate shuttle related genes was regulated by the gut microbiota (Margineanu et al., 2020). Another study indicated that greater level of gut microbiome-derived metabolite trimethylamine N-oxide was associated with higher expression of brain pro-inflammatory cytokines and astrocyte activation markers (Brunt et al., 2021). These studies also elucidate the crucial role of astrocytes in the immune modulation in the CNS. In terms of PD, Sun et al. found that FMT protected the neuronal function by inhibition of the neuroinflammation and reduction of astrocytes activation in the substantia nigra (Sun et al., 2018). Another acupunctural study found that acupuncture on PD mice regulated the abundance of gut microbiota and restored the overexpression of astrocytes in striatum and substantia nigra (Jang et al., 2020). By far, the evidence between astrocytes and gut microbiota in PD remains limited.
To the extent known, the modulations between PD and dysbiosis of the gut microbiota are bidirectional. i) Dysbiosis of gut microbiota may cause PD-related abnormal inflammatory response. Gut microbiota may regulate immune-related inflammatory factors in the intestine, and subsequently affect the CNS (Shimizu et al., 2011; Huang et al., 2019c; Claudino Dos Santos et al., 2023). Microbiota along with their products can stimulate intestinal epithelial cells and macrophages in the gut, resulting in activation of immune response and the release of inflammatory cytokines, and they can reach the CNS (Banks, 2009; Mayer et al., 2014; Gorky and Schwaber, 2016). At autopsy, it was found that the expression of pro-inflammatory cytokines and chemokines were upregulated in the brain tissue and cerebrospinal fluid of patients with PD, including TNF-α, IL-1β, IL-6 and IFN-γ (Mogi et al., 1994a; Mogi et al., 1994b). Knockout of the TNF-α receptor gene exhibited neuroprotective effects in mice (Bhaskar et al., 2014). Under the physiological conditions, gut microbiota downregulates IFN-γ expression in astrocytes while upregulates TRAIL expression in astrocytes (Sanmarco et al., 2021). The gut microbiota can produce various toll like receptor (TLR) ligands that can play a pro-inflammatory role in a specific environment (Caputi and Giron, 2018). Thus, any changes of gut microbiota and destruction of the gut epithelial barrier may activate TLRs, and promote inflammatory response in both the gut and brain of PD patients (Terán-Ventura et al., 2014; Dheer et al., 2016; Sun et al., 2018). It has been known that the signal regulation of TLR2 and TLR4 can affect the development of PD (Harms et al., 2018; Pascual et al., 2021). In the intestines of PD patients, enhancement of CD3+ T cells in colon tissue was found along with the immune interactions among TLR4, cytokines, and chemokines (Terán-Ventura et al., 2014). Epidemiological studies also suggested that inflammatory bowel disease is a significant risk factor for the development of PD (Lin et al., 2016; Villumsen et al., 2019; Weimers et al., 2019). Some scholars hypothesized that the alteration of gut microbial flora leads to gastrointestinal system disturbance which cause neuroinflammation by prion α-syn expression and produces PD like symptoms (Sharma et al., 2019). ii) PD may have GI dysfunction, which worsen the state of gut microbiota. In PD patients at the early stage, mild intestinal inflammation is commonly developed that triggers a low-level immune response and increases intestinal permeability (Houser and Tansey, 2017). Accordingly, intestinal inflammation and enhanced intestinal permeability are the intestinal characteristics of PD patients (Houser and Tansey, 2017; Perez-Pardo et al., 2019). These issues markedly break the balance of gut microbiota and deteriorate their ecology. Thus, the imbalance of intestinal microecology increases the pro-inflammatory environment in the intestine, which promotes the neuroinflammation of the central nervous system and leads to the development of PD. In this regard, PD and dysbiosis of the gut microbiota may generate a bidirectional vicious circle, and promote the development and progression of PD.
It has been reported that α-syn misfolding might initiate in the gut, and spread to the brain via the vagus nerve (Claudino Dos Santos et al., 2023). This hypothesis provided a plausible “pathway” of the impacts of gut microbiota to the α-syn-based PD pathogenesis (see next section). However, due to the complex nature of interactions among microglia, astrocytes, gut microbiota and enteric glia, we believe that the actual interactions between PD and gut microbiota -related inflammatory reactions are far more complex than “bidirectional”. It might be “multidirectional” involved by many known and unknown factors, which indeed warrant further investigation.
The vagus nerve, comprising the tenth pair of cranial nerves, is the longest and most widely distributed pair of cranial nerves, containing sensory, motor, and parasympathetic nerve fibers (Rosas-Ballina et al., 2011; McVey Neufeld et al., 2019; van der Meij and Wermer, 2021). It serves as a crucial link between gut bacteria and the brain, transmitting signals from various intestinal tracts and being associated with numerous gastrointestinal tracts, nervous system, and immune system diseases (Berthoud and Neuhuber, 2000; Goehler et al., 2000; Powley et al., 2011; Ahmed et al., 2019). The vagus nerve communicates with the brain through multiple synaptic connections with the nucleus tractus solitarius of the brain stem (Biaggioni, 2017). According to reports, when gut microbiota stimulate the afferent neurons of ENS, the ENS and vagus nerve form a synaptic connection and an information transmission pathway, enabling mutual communication and regulation between gut microbiota and the brain (Yu et al., 2020). Studies have also found that sensory neurons of the vagus nerve form various mechanical and chemical sensory terminals along the gastrointestinal tract to receive intestinal-brain signals (Bravo et al., 2011; Perez-Burgos et al., 2013). Additionally, enteroendocrine cells can form synapses with neighboring nerves to assist the vagus nerve in receiving signals from the intestine (Bohórquez et al., 2015; Bellono et al., 2017; Fülling et al., 2019). A large prospective cohort study found that subjects who have undergone complete vagotomy have a low risk of PD (Svensson et al., 2015). A post-mortem study has shown that PD pathology may start in the gastrointestinal tract and then spread through the vagus nerve to the brain (Braak et al., 2006). An animal study found that the central role of Lactobacillus rhamnosus was eliminated after vagus nerve amputation (Fung et al., 2017). Recently, α-syn has emerged as a critical factor contributing to PD pathogenesis (Mor et al., 2017). More evidence has indicated that the vagus nerve is a route by which α-syn pathology can spread both to and from the brain (Pan-Montojo et al., 2012; Matteoli and Boeckxstaens, 2013; Gray et al., 2014; Liu et al., 2017). A new mouse model of PD demonstrates α-syn pathology spreading from the gut to the brain via the vagus nerve (Kim et al., 2019b). Recent advances have suggested that gut bacteria enhance the aggregation of α-syn and other similar proteins, a mechanism that might promote the ability of pathogens to reach the CNS through the vagus nerve (Sampson et al., 2016; Choudhry and Perlmuter, 2017). However, some scholars denied the connection between PD and the vagus nerve (Tysnes et al., 2015). A study using baboons as a model found a possible systemic mechanism in which the general circulation would act as a route for long-distance bidirectional transmission of endogenous α-syn between the enteric and the central nervous systems (Arotcarena et al., 2020). Although accumulated and convincing evidence supports this pathway, its specific mechanisms remains unclear and requires further exploration.
The HPA axis is a crucial component of the neuroendocrine system that regulates various body processes to cope with stress (Smith and Vale, 2006). Under stress, Corticotropin-releasing factor (CRF) is released from the paraventricular nucleus (PVN) of the hypothalamus, which is the main regulator of the HPA axis and induces the release of adrenocorticotropic hormone (ACTH) into systemic circulation (Mastorakos and Zapanti, 2004). Subsequently, the adrenal cortex is stimulated by ACTH and secretes glucocorticoids (Smith and Vale, 2006). The released glucocorticoids then bind to glucocorticoid receptors (GR), resulting in feedback inhibition (Keller-Wood and Dallman, 1984). In recent years, more and more studies have been conducted to discover a bidirectional communication between the neuroendocrine system and gut microbiota through the HPA axis (Farzi et al., 2018). The CNS can regulate intestinal function through the HPA axis, and the gut microbiota can also regulate gut hormone levels, which affect CNS function through the HPA axis (Sun et al., 2020). An animal experiment using GF mice found that Enterococcus faecalis can inhibit excessive stress response in social interaction by limiting the production of corticosterone levels mediated by the HPA axis, thereby promoting the social activity of mice (Wu et al., 2021c). An experiment using GF and pathogen-free mice demonstrated that maternal isolated early life stress altered the HPA axis in a microbiota-independent fashion (Jiang et al., 2015; Kelly et al., 2016). Additionally, evidence suggests that GF mice can overstimulate the HPA axis under stress, while probiotics can alleviate the response state of the HPA axis in mice (Heym et al., 2019; Huang et al., 2019b). A study from 2013 found that DBS of the subthalamic nucleus can reduce cortisol levels in patients with advanced PD (Seifried et al., 2013). Psychological stress and changes in the hypothalamic-pituitary-adrenal axis were also present in patients with “de novo” PD (Ibrahimagic et al., 2016). Increasing numbers of clinical trials and animal experiments showed that the HPA axis was altered and unbalanced in PD patients, leading to a significant increase in cortisol levels (Höschl and Hajek, 2001; Zhang et al., 2017; Grigoruţă et al., 2020). Interestingly, one study found that non-motor symptoms of PD (e.g., mood and anxiety) were significantly associated with hair cortisone levels (van den Heuvel et al., 2020). Hair glucocorticoid levels reflect longer-term HPA-axis function and can provide additional insights into the role of a dysregulated HPA-axis in PD (Wosu et al., 2013; Stalder et al., 2017). In conclusion, although there are few studies on the role of the HPA axis in gut microbiota and PD, we cannot ignore the roles of the HPA axis in the microbiota-gut-brain axis of PD.
In the clinical setting, the primary treatment for PD remains dopaminergic medication. Currently, there is no known cure for PD. Although growing evidence supporting the role of gut microbiota in PD, the gut microbiota-based therapy is still under investigation. This section summarizes the emerging preventive and therapeutic interventions that modulate the gut microbiome (Table 3).
Prebiotics are organic substances that are not digested and absorbed by the host, but selectively promote the metabolism and proliferation of beneficial bacteria in the body, thereby improving host health. Once they reach the colon, prebiotics are decomposed and utilized, promoting the growth of colonic microbiota, where they play an important role in improving the intestinal microecology and regulating lipid metabolism. Different types of prebiotics include isomalto-oligosaccharide, fructooligosaccharide, galacto-oligosaccharide (GOS), xylooligosaccharide, lactulooligosaccharide, soybean oligosaccharide, and inulin (Slavin, 2013). Although there are only few studies using prebiotics in the treatment of PD, neuroprotective effects of prebiotics were verified. Feeding GOS to rats could elevate the expression of central brain-derived neurotrophic factor (BDNF), N-methyl-D-aspartate receptor subunit (NMDAR), and D-serine (Savignac et al., 2013). In another experiment, neonatal prebiotic (BGOS) supplementation increased the levels of synaptophysin, GluN2A-subunits, and BDNF proteins in the adult rat hippocampus (Williams et al., 2016). Additionally, it was reported that xylooligosaccharide could effectively restore the cognitive function of obese-insulin resistant rats by reducing the activation of microglia through the gut-brain axis (Chunchai et al., 2018). Lactulose was also found to contribute to repairing intestinal barrier injuries, improving gut microbiota imbalances, and reducing post-stroke inflammatory responses in the stroke mice (Yuan et al., 2021). Findings of these studies demonstrated that prebiotics may play an important role in the improvement of neurological functions. Moreover, prebiotics could improve immune function and regulate bowel defecation habits (Jeurink et al., 2013; Meksawan et al., 2016). We speculate that this may be associated with amelioration of the inflammation and gastrointestinal dysfunction in PD. A clinical trial found that a fermented milk containing prebiotic fiber was superior to placebo in improving constipation in PD patients (Barichella et al., 2016). In PD striatum rotenone model mice, prebiotic fibers were found to partially alleviate the motor and non-motor problems caused by rotenone (Perez-Pardo et al., 2017). Additionally, it restored the level of striatal dopamine transporter in PD mice, indicating its neurorestorative properties (Perez-Pardo et al., 2017). In summary, the prebiotics-related mechanisms might lie in improving the composition of the gut microbiota, improving intestinal barrier function, and showing potential beneficial effects on the regulation of the microbiota-gut-brain axis.
Probiotics are microorganisms that can improve the balance of gut microflora thereby exhibit beneficial effects in the host. The majority of available studies reported that administration of probiotic achieved neuroprotective benefits and alleviation of cognitive impairments (Ji and Shen, 2021). Probiotics can exert beneficial effects via decline of neurotransmitter levels, chronic inflammation, oxidative stress, and apoptosis in NDDs (Westfall et al., 2017). An early study found that regular intake of probiotics significantly improved stool consistency and defecation habits in patients with PD (Cassani et al., 2011). A clinical trial found that a mixture of probiotics (Lactobacillus acidophilus, Bifidobacterium bifidum, Lactobacillus reuteri, and Lactobacillus fermentum) ameliorated the clinical symptoms and reduced inflammatory and oxidative responses in PD patients (Tamtaji et al., 2019). In vitro experiments found that probiotics (Lactobacillus and Bifidobacterium) inhibited the production of inflammatory cytokines and ROS in PD patients (Magistrelli et al., 2019). In line with the clinical studies, it was documented that long-term administration of probiotics achieved protection of dopamine neurons and amelioration of the motor dysfunction in PD mice (Hsieh et al., 2020). In the rat model of PD induced by 6-hydroxydopamine, administration of probiotics could improve the rotational behavior, cognitive function, lipid peroxidation, and neuronal damage (Alipour Nosrani et al., 2021). In another experiment, it was found that a mixture of probiotics could protect dopaminergic neurons against neurotoxic effects induced by MPTP or rotenone exposure (Srivastav et al., 2019). In vivo and in vitro experiments demonstrated that SLAB51, a novel probiotic agent, could regulate the BDNF pathway, increase the level of neuroprotective proteins, and reduce neuronal death proteins. Thus, the neuroprotective effects of probiotics were verified (Castelli et al., 2020). In addition, probiotics (Bifidobacterium longum BB536 and Lactobacillus rhamnosus HN001) were found to have tendency to improve the swimming performance and reduce the oxidative stress in a rotenone-induced PD zebrafish model (Ilie et al., 2021). Interestingly, the probiotic Bacillus subtilis was reportedly to prevent α-syn aggregation in Caenorhabditis elegans models (Goya et al., 2020). Accordingly, probiotics can be considered as an emerging treatment for PD subjects.
FMT refers to transfer of functional bacteria from feces of healthy individuals to the intestinal tracts of patients in a specialized manner to regulate the gut microbiota. The objective of FMT is to restore the healthy diversity of gut microbiota and provide successful therapy for certain diseases both within and outside the intestinal tract. Recently, FMT has also been considered as a potential therapy for the treatment of NDDs, particularly for PD (Sun et al., 2021b; Wang et al., 2021a). Kuai et al. found that the abundance of Blautia and Prevotella increased after undergoing FMT in PD patients, whereas the abundance of Bacteroides decreased sharply, and the PAC-QOL scores and Wexner constipation scores significantly decreased (Kuai et al., 2021). Huang et al. found that constipation and tremors were effectively relieved after undergoing the FMT treatments (Huang et al., 2019a). Additionally, Xue et al. found that FMT alleviated the motor and non-motor symptoms in PD patients, and colonic FMT was more preferable than nasointestinal FMT (Xue et al., 2020). Oral administration of specific microbial metabolites to GF mice promoted neuroinflammation and motor symptoms, meanwhile, colonization of α-syn-overexpressing mice with feces from PD patients deteriorated the physical impairments in animals (Sampson et al., 2016). In MPTP-induced PD mice, FMT was found to achieve reducing dysbiosis of gut microbial, decreasing fecal SCFAs, alleviating physical impairments, and increasing striatal DA and 5-HT levels (Sun et al., 2018). These results suggest that FMT the feces of healthy subjects could change the microecology of the intestine, thereby exhibit therapeutic effects in PD subjects.
The refractory nature of PD treatments, particularly for advanced PD so far, requires better exploration of the novel strategies other than dopaminergic-based treatments. Undoubtedly the gut microbiota-related pathogenesis has been highlighted and become a beacon for the drug development. Although advances in the technologies like high-throughput sequencing, GF animals and GMT make deep investigation of gut microbiota become feasible, the associations between gut microbiota and PD are still intricate due to the complex nature of interactions among gut microbiota as well as the relative influence factors. The present review summarized the potential the gut microbiota-related mechanisms from the perspectives of humoral pathway, cellular immune pathway and neuronal pathway. Numerous studies have elucidated the potential mechanisms from these different angles. It seems a plausible hypothesis that α-syn might initiate in the gut induced by the dysbiosis of gut microbiota, and then transport into CNS with a “prion-like” manner via several pathways (such as the vagus nerve pathway) (Claudino Dos Santos et al., 2023). However, we still believe the association between gut microbiota and PD is far more complex than “bidirectional”, it must be “multidirectional”, which require further investigation. So far, there is still a long way before the gut microbiota-related strategy against PD can be actually application in the clinical setting. Future investigation should focus on the following issues: i) Clarification of delivery mechanisms of the gut-original α-syn into brain. How to intervene such delivery processes (prevention of PD)? ii) Other than the α-syn-related mechanisms, are there any other PD related pathogenic factors involved in the dysbiosis of gut microbiota (such as KP-related liposoluble small-molecule neurotoxins)? iii) Development of gut microbiota-based PD biomarkers with low-cost, low-invasion and high specificity is indispensable. iv) Establishment of GF animal models in large animals, such as non-human primates, is needed. v) Implementation of well-designed clinical trials of GMT, prebiotics and probiotics. More investigations with comprehensive and rigorous experimental design are therefore highly anticipated.
XJ: Conceptualization, Formal analysis, Software, Writing – original draft, Writing – review & editing. QC: Funding acquisition, Writing – original draft. YZ: Funding acquisition, Writing – original draft. TA: Conceptualization, Funding acquisition, Writing – review & editing, Supervision.
The author(s) declare financial support was received for the research, authorship, and/or publication of this article. This work was supported by the Shenzhen High-level Hospital Construction Fund (23274G1001), the Guangdong Provincial Administration of Traditional Chinese Medicine (20231104), Jointly funded project by the city, schools (institutes), and enterprises of Guangzhou Science and Technology Bureau (SL2022A03J00212), Young Talent Support Project of Guangzhou Association for Science and Technology (QT-2023-027), National Natural Science Foundation Youth Fund (82305068), and Youth Science and Technology Talent Project of The Affiliated TCM Hospital of Guangzhou Medical University (2022RC08).
The authors declare that the research was conducted in the absence of any commercial or financial relationships that could be construed as a potential conflict of interest.
All claims expressed in this article are solely those of the authors and do not necessarily represent those of their affiliated organizations, or those of the publisher, the editors and the reviewers. Any product that may be evaluated in this article, or claim that may be made by its manufacturer, is not guaranteed or endorsed by the publisher.
Abdelkader, N. F., Safar, M. M., Salem, H. A. (2016). Ursodeoxycholic acid ameliorates apoptotic cascade in the rotenone model of parkinson's disease: modulation of mitochondrial perturbations. Mol. Neurobiol. 53 (2), 810–817. doi: 10.1007/s12035-014-9043-8
Abrams, P., Andersson, K. E., Buccafusco, J. J., Chapple, C., de Groat, W. C., Fryer, A. D., et al. (2006). Muscarinic receptors: their distribution and function in body systems, and the implications for treating overactive bladder. Br. J. Pharmacol. 148 (5), 565–578. doi: 10.1038/sj.bjp.0706780
Adeghate, E., Ponery, A. S. (2002). GABA in the endocrine pancreas: cellular localization and function in normal and diabetic rats. Tissue Cell 34 (1), 1–6. doi: 10.1054/tice.2002.0217
Agirman, G., Yu, K. B., Hsiao, E. Y. (2021). Signaling inflammation across the gut-brain axis. Science 374 (6571), 1087–1092. doi: 10.1126/science.abi6087
Ahmed, S., Busetti, A., Fotiadou, P., Vincy Jose, N., Reid, S., Georgieva, M., et al. (2019). In vitro characterization of gut microbiota-derived bacterial strains with neuroprotective properties. Front. Cell Neurosci. 13. doi: 10.3389/fncel.2019.00402
Aho, V. T. E., Houser, M. C., Pereira, P. A. B., Chang, J., Rudi, K., Paulin, L., et al. (2021). Relationships of gut microbiota, short-chain fatty acids, inflammation, and the gut barrier in Parkinson's disease. Mol. Neurodegener. 16 (1), 6. doi: 10.1186/s13024-021-00427-6
Akinci, M. K., Schofield, P. R. (1999). Widespread expression of GABA(A) receptor subunits in peripheral tissues. Neurosci. Res. 35 (2), 145–153. doi: 10.1016/s0168-0102(99)00078-4
Alipour Nosrani, E., Tamtaji, O. R., Alibolandi, Z., Sarkar, P., Ghazanfari, M., Azami Tameh, A., et al. (2021). Neuroprotective effects of probiotics bacteria on animal model of Parkinson's disease induced by 6-hydroxydopamine: A behavioral, biochemical, and histological study. J. Immunoassay. Immunochem. 42 (2), 106–120. doi: 10.1080/15321819.2020.1833917
Arotcarena, M. L., Dovero, S., Prigent, A., Bourdenx, M., Camus, S., Porras, G., et al. (2020). Bidirectional gut-to-brain and brain-to-gut propagation of synucleinopathy in non-human primates. Brain 143 (5), 1462–1475. doi: 10.1093/brain/awaa096
Asakawa, T., Fang, H., Sugiyama, K., Nozaki, T., Hong, Z., Yang, Y., et al. (2016a). Animal behavioral assessments in current research of Parkinson's disease. Neurosci. Biobehav. Rev. 65, 63–94. doi: 10.1016/j.neubiorev.2016.03.016
Asakawa, T., Fang, H., Sugiyama, K., Nozaki, T., Kobayashi, S., Hong, Z., et al. (2016b). Human behavioral assessments in current research of Parkinson's disease. Neurosci. Biobehav. Rev. 68, 741–772. doi: 10.1016/j.neubiorev.2016.06.036
Baldini, F., Hertel, J., Sandt, E., Thinnes, C. C., Neuberger-Castillo, L., Pavelka, L., et al. (2020). Parkinson's disease-associated alterations of the gut microbiome predict disease-relevant changes in metabolic functions. BMC Biol. 18 (1), 62. doi: 10.1186/s12915-020-00775-7
Balducci, C., Forloni, G. (2018). Novel targets in Alzheimer's disease: A special focus on microglia. Pharmacol. Res. 130, 402–413. doi: 10.1016/j.phrs.2018.01.017
Banks, W. A. (2009). The blood-brain barrier in psychoneuroimmunology. Immunol. Allergy Clin. North Am. 29 (2), 223–228. doi: 10.1016/j.iac.2009.02.001
Bao, H., Li, H., Jia, Y., Xiao, Y., Luo, S., Zhang, D., et al. (2021). Ganoderic acid A exerted antidepressant-like action through FXR modulated NLRP3 inflammasome and synaptic activity. Biochem. Pharmacol. 188, 114561. doi: 10.1016/j.bcp.2021.114561
Barichella, M., Pacchetti, C., Bolliri, C., Cassani, E., Iorio, L., Pusani, C., et al. (2016). Probiotics and prebiotic fiber for constipation associated with Parkinson disease: An RCT. Neurology 87 (12), 1274–1280. doi: 10.1212/wnl.0000000000003127
Bellono, N. W., Bayrer, J. R., Leitch, D. B., Castro, J., Zhang, C., O'Donnell, T. A., et al. (2017). Enterochromaffin cells are gut chemosensors that couple to sensory neural pathways. Cell 170 (1), 185–198.e116. doi: 10.1016/j.cell.2017.05.034
Ben-Othman, N., Vieira, A., Courtney, M., Record, F., Gjernes, E., Avolio, F., et al. (2017). Long-term GABA administration induces alpha cell-mediated beta-like cell neogenesis. Cell 168 (1-2), 73–85.e11. doi: 10.1016/j.cell.2016.11.002
Bergman, E. N. (1990). Energy contributions of volatile fatty acids from the gastrointestinal tract in various species. Physiol. Rev. 70 (2), 567–590. doi: 10.1152/physrev.1990.70.2.567
Berthoud, H. R., Neuhuber, W. L. (2000). Functional and chemical anatomy of the afferent vagal system. Auton. Neurosci. 85 (1-3), 1–17. doi: 10.1016/s1566-0702(00)00215-0
Bhaskar, K., Maphis, N., Xu, G., Varvel, N. H., Kokiko-Cochran, O. N., Weick, J. P., et al. (2014). Microglial derived tumor necrosis factor-α drives Alzheimer's disease-related neuronal cell cycle events. Neurobiol. Dis. 62, 273–285. doi: 10.1016/j.nbd.2013.10.007
Biaggioni, I. (2017). The pharmacology of autonomic failure: from hypotension to hypertension. Pharmacol. Rev. 69 (1), 53–62. doi: 10.1124/pr.115.012161
Boertien, J. M., Pereira, P. A. B., Aho, V. T. E., Scheperjans, F. (2019). Increasing comparability and utility of gut microbiome studies in parkinson's disease: A systematic review. J. Parkinsons. Dis. 9 (s2), S297–s312. doi: 10.3233/jpd-191711
Bohórquez, D. V., Shahid, R. A., Erdmann, A., Kreger, A. M., Wang, Y., Calakos, N., et al. (2015). Neuroepithelial circuit formed by innervation of sensory enteroendocrine cells. J. Clin. Invest. 125 (2), 782–786. doi: 10.1172/jci78361
Borre, Y. E., O'Keeffe, G. W., Clarke, G., Stanton, C., Dinan, T. G., Cryan, J. F. (2014). Microbiota and neurodevelopmental windows: implications for brain disorders. Trends Mol. Med. 20 (9), 509–518. doi: 10.1016/j.molmed.2014.05.002
Braak, H., de Vos, R. A., Bohl, J., Del Tredici, K. (2006). Gastric alpha-synuclein immunoreactive inclusions in Meissner's and Auerbach's plexuses in cases staged for Parkinson's disease-related brain pathology. Neurosci. Lett. 396 (1), 67–72. doi: 10.1016/j.neulet.2005.11.012
Braniste, V., Al-Asmakh, M., Kowal, C., Anuar, F., Abbaspour, A., Tóth, M., et al. (2014). The gut microbiota influences blood-brain barrier permeability in mice. Sci. Transl. Med. 6 (263), 263ra158. doi: 10.1126/scitranslmed.3009759
Bravo, J. A., Forsythe, P., Chew, M. V., Escaravage, E., Savignac, H. M., Dinan, T. G., et al. (2011). Ingestion of Lactobacillus strain regulates emotional behavior and central GABA receptor expression in a mouse via the vagus nerve. Proc. Natl. Acad. Sci. U.S.A. 108 (38), 16050–16055. doi: 10.1073/pnas.1102999108
Brunt, V. E., LaRocca, T. J., Bazzoni, A. E., Sapinsley, Z. J., Miyamoto-Ditmon, J., Gioscia-Ryan, R. A., et al. (2021). The gut microbiome-derived metabolite trimethylamine N-oxide modulates neuroinflammation and cognitive function with aging. Geroscience 43 (1), 377–394. doi: 10.1007/s11357-020-00257-2
Candela, M., Perna, F., Carnevali, P., Vitali, B., Ciati, R., Gionchetti, P., et al. (2008). Interaction of probiotic Lactobacillus and Bifidobacterium strains with human intestinal epithelial cells: adhesion properties, competition against enteropathogens and modulation of IL-8 production. Int. J. Food Microbiol. 125 (3), 286–292. doi: 10.1016/j.ijfoodmicro.2008.04.012
Cannon, T., Sinha, A., Trudeau, L. E., Maurice, C. F., Gruenheid, S. (2020). Characterization of the intestinal microbiota during Citrobacter rodentium infection in a mouse model of infection-triggered Parkinson's disease. Gut. Microbes 12 (1), 1–11. doi: 10.1080/19490976.2020.1830694
Caputi, V., Giron, M. C. (2018). Microbiome-gut-brain axis and toll-like receptors in parkinson's disease. Int. J. Mol. Sci. 19 (6), 1689. doi: 10.3390/ijms19061689
Cassani, E., Privitera, G., Pezzoli, G., Pusani, C., Madio, C., Iorio, L., et al. (2011). Use of probiotics for the treatment of constipation in Parkinson's disease patients. Minerva. Gastroenterol. Dietol. 57 (2), 117–121.
Castelli, V., d'Angelo, M., Lombardi, F., Alfonsetti, M., Antonosante, A., Catanesi, M., et al. (2020). Effects of the probiotic formulation SLAB51 in in vitro and in vivo Parkinson's disease models. Aging (Albany. NY). 12 (5), 4641–4659. doi: 10.18632/aging.102927
Castro-Caldas, M., Carvalho, A. N., Rodrigues, E., Henderson, C. J., Wolf, C. R., Rodrigues, C. M., et al. (2012). Tauroursodeoxycholic acid prevents MPTP-induced dopaminergic cell death in a mouse model of Parkinson's disease. Mol. Neurobiol. 46 (2), 475–486. doi: 10.1007/s12035-012-8295-4
Chambers, E. S., Morrison, D. J., Frost, G. (2015). Control of appetite and energy intake by SCFA: what are the potential underlying mechanisms? Proc. Nutr. Soc. 74 (3), 328–336. doi: 10.1017/s0029665114001657
Chen, P. S., Peng, G. S., Li, G., Yang, S., Wu, X., Wang, C. C., et al. (2006). Valproate protects dopaminergic neurons in midbrain neuron/glia cultures by stimulating the release of neurotrophic factors from astrocytes. Mol. Psychiatry 11 (12), 1116–1125. doi: 10.1038/sj.mp.4001893
Chen, Q., Liu, Y., Zhang, Y., Jiang, X., Zhang, Y., Asakawa, T. (2020a). An in vitro verification of the effects of paeoniflorin on lipopolysaccharide-exposed microglia. Evid. Based. Complement. Alternat. Med. 2020, 5801453. doi: 10.1155/2020/5801453
Chen, Z., Ruan, J., Li, D., Wang, M., Han, Z., Qiu, W., et al. (2020b). The role of intestinal bacteria and gut-brain axis in hepatic encephalopathy. Front. Cell Infect. Microbiol. 10. doi: 10.3389/fcimb.2020.595759
Chen, S., Tan, B., Xia, Y., Liao, S., Wang, M., Yin, J., et al. (2019). Effects of dietary gamma-aminobutyric acid supplementation on the intestinal functions in weaning piglets. Food Funct. 10 (1), 366–378. doi: 10.1039/c8fo02161a
Choi, J. G., Kim, N., Ju, I. G., Eo, H., Lim, S. M., Jang, S. E., et al. (2018). Oral administration of Proteus mirabilis damages dopaminergic neurons and motor functions in mice. Sci. Rep. 8 (1), 1275. doi: 10.1038/s41598-018-19646-x
Choudhry, H., Perlmuter, L. C. (2017). Non-CNS pathogenic origin of Parkinson's disease. J. Neurol. 264 (9), 2027–2030. doi: 10.1007/s00415-017-8588-7
Chu, C., Murdock, M. H., Jing, D., Won, T. H., Chung, H., Kressel, A. M., et al. (2019). The microbiota regulate neuronal function and fear extinction learning. Nature 574 (7779), 543–548. doi: 10.1038/s41586-019-1644-y
Chunchai, T., Thunapong, W., Yasom, S., Wanchai, K., Eaimworawuthikul, S., Metzler, G., et al. (2018). Decreased microglial activation through gut-brain axis by prebiotics, probiotics, or synbiotics effectively restored cognitive function in obese-insulin resistant rats. J. Neuroinflamm. 15 (1), 11. doi: 10.1186/s12974-018-1055-2
Claudino Dos Santos, J. C., Lima, M. P. P., Brito, G. A. C., Viana, G. S. B. (2023). Role of enteric glia and microbiota-gut-brain axis in parkinson disease pathogenesis. Ageing Res. Rev. 84, 101812. doi: 10.1016/j.arr.2022.101812
Cryan, J. F., O'Riordan, K. J., Cowan, C. S. M., Sandhu, K. V., Bastiaanssen, T. F. S., Boehme, M., et al. (2019). The microbiota-gut-brain axis. Physiol. Rev. 99 (4), 1877–2013. doi: 10.1152/physrev.00018.2018
Cryan, J. F., O'Riordan, K. J., Sandhu, K., Peterson, V., Dinan, T. G. (2020). The gut microbiome in neurological disorders. Lancet Neurol. 19 (2), 179–194. doi: 10.1016/s1474-4422(19)30356-4
Cummings, J. H., Macfarlane, G. T. (1997). Colonic microflora: nutrition and health. Nutrition 13 (5), 476–478. doi: 10.1016/s0899-9007(97)00114-7
Cummings, J. H., Pomare, E. W., Branch, W. J., Naylor, C. P., Macfarlane, G. T. (1987). Short chain fatty acids in human large intestine, portal, hepatic and venous blood. Gut 28 (10), 1221–1227. doi: 10.1136/gut.28.10.1221
Dai, Z., Zhang, J., Wu, Q., Fang, H., Shi, C., Li, Z., et al. (2020). Intestinal microbiota: a new force in cancer immunotherapy. Cell Commun. Signal 18 (1), 90. doi: 10.1186/s12964-020-00599-6
Dalile, B., Van Oudenhove, L., Vervliet, B., Verbeke, K. (2019). The role of short-chain fatty acids in microbiota-gut-brain communication. Nat. Rev. Gastroenterol. Hepatol. 16 (8), 461–478. doi: 10.1038/s41575-019-0157-3
Davoli-Ferreira, M., Thomson, C. A., McCoy, K. D. (2021). Microbiota and microglia interactions in ASD. Front. Immunol. 12. doi: 10.3389/fimmu.2021.676255
Desbonnet, L., Garrett, L., Clarke, G., Kiely, B., Cryan, J. F., Dinan, T. G. (2010). Effects of the probiotic Bifidobacterium infantis in the maternal separation model of depression. Neuroscience 170 (4), 1179–1188. doi: 10.1016/j.neuroscience.2010.08.005
Dheer, R., Santaolalla, R., Davies, J. M., Lang, J. K., Phillips, M. C., Pastorini, C., et al. (2016). Intestinal epithelial toll-like receptor 4 signaling affects epithelial function and colonic microbiota and promotes a risk for transmissible colitis. Infect. Immun. 84 (3), 798–810. doi: 10.1128/iai.01374-15
Diaz Heijtz, R., Wang, S., Anuar, F., Qian, Y., Björkholm, B., Samuelsson, A., et al. (2011). Normal gut microbiota modulates brain development and behavior. Proc. Natl. Acad. Sci. U.S.A. 108 (7), 3047–3052. doi: 10.1073/pnas.1010529108
Dixon, B. J., Tang, J., Zhang, J. H. (2013). The evolution of molecular hydrogen: a noteworthy potential therapy with clinical significance. Med. Gas. Res. 3 (1), 10. doi: 10.1186/2045-9912-3-10
Dong, X. L., Wang, X., Liu, F., Liu, X., Du, Z. R., Li, R. W., et al. (2020). Polymannuronic acid prevents dopaminergic neuronal loss via brain-gut-microbiota axis in Parkinson's disease model. Int. J. Biol. Macromol. 164, 994–1005. doi: 10.1016/j.ijbiomac.2020.07.180
Doroszkiewicz, J., Groblewska, M., Mroczko, B. (2021). The role of gut microbiota and gut-brain interplay in selected diseases of the central nervous system. Int. J. Mol. Sci. 22 (18), 10028. doi: 10.3390/ijms221810028
Elmaki, E. E. A., Gong, T., Nkonika, D. M., Wang, G. (2018). Examining alterations in GABA concentrations in the basal ganglia of patients with Parkinson's disease using MEGA-PRESS MRS. Jpn. J. Radiol. 36 (3), 194–199. doi: 10.1007/s11604-017-0714-z
Erny, D., Hrabě de Angelis, A. L., Jaitin, D., Wieghofer, P., Staszewski, O., David, E., et al. (2015). Host microbiota constantly control maturation and function of microglia in the CNS. Nat. Neurosci. 18 (7), 965–977. doi: 10.1038/nn.4030
Fang, X., Tian, P., Zhao, X., Jiang, C., Chen, T. (2019). Neuroprotective effects of an engineered commensal bacterium in the 1-methyl-4-phenyl-1, 2, 3, 6-tetrahydropyridine Parkinson disease mouse model via producing glucagon-like peptide-1. J. Neurochem. 150 (4), 441–452. doi: 10.1111/jnc.14694
Farzi, A., Fröhlich, E. E., Holzer, P. (2018). Gut microbiota and the neuroendocrine system. Neurotherapeutics 15 (1), 5–22. doi: 10.1007/s13311-017-0600-5
Fasano, A., Visanji, N. P., Liu, L. W., Lang, A. E., Pfeiffer, R. F. (2015). Gastrointestinal dysfunction in Parkinson's disease. Lancet Neurol. 14 (6), 625–639. doi: 10.1016/s1474-4422(15)00007-1
Fernández, M. F., Boris, S., Barbés, C. (2003). Probiotic properties of human lactobacilli strains to be used in the gastrointestinal tract. J. Appl. Microbiol. 94 (3), 449–455. doi: 10.1046/j.1365-2672.2003.01850.x
Fiorucci, S., Cipriani, S., Mencarelli, A., Renga, B., Distrutti, E., Baldelli, F. (2010). Counter-regulatory role of bile acid activated receptors in immunity and inflammation. Curr. Mol. Med. 10 (6), 579–595. doi: 10.2174/1566524011009060579
Forsyth, C. B., Shannon, K. M., Kordower, J. H., Voigt, R. M., Shaikh, M., Jaglin, J. A., et al. (2011). Increased intestinal permeability correlates with sigmoid mucosa alpha-synuclein staining and endotoxin exposure markers in early Parkinson's disease. PloS One 6 (12), e28032. doi: 10.1371/journal.pone.0028032
Forsythe, P., Sudo, N., Dinan, T., Taylor, V. H., Bienenstock, J. (2010). Mood and gut feelings. Brain Behav. Immun. 24 (1), 9–16. doi: 10.1016/j.bbi.2009.05.058
Fu, Y., Ito, M., Fujita, Y., Ito, M., Ichihara, M., Masuda, A., et al. (2009). Molecular hydrogen is protective against 6-hydroxydopamine-induced nigrostriatal degeneration in a rat model of Parkinson's disease. Neurosci. Lett. 453 (2), 81–85. doi: 10.1016/j.neulet.2009.02.016
Fu, S. P., Wang, J. F., Xue, W. J., Liu, H. M., Liu, B. R., Zeng, Y. L., et al. (2015). Anti-inflammatory effects of BHBA in both in vivo and in vitro Parkinson's disease models are mediated by GPR109A-dependent mechanisms. J. Neuroinflamm. 12, 9. doi: 10.1186/s12974-014-0230-3
Fujisaka, S., Avila-Pacheco, J., Soto, M., Kostic, A., Dreyfuss, J. M., Pan, H., et al. (2018). Diet, genetics, and the gut microbiome drive dynamic changes in plasma metabolites. Cell Rep. 22 (11), 3072–3086. doi: 10.1016/j.celrep.2018.02.060
Fujita, K., Seike, T., Yutsudo, N., Ohno, M., Yamada, H., Yamaguchi, H., et al. (2009). Hydrogen in drinking water reduces dopaminergic neuronal loss in the 1-methyl-4-phenyl-1,2,3,6-tetrahydropyridine mouse model of Parkinson's disease. PloS One 4 (9), e7247. doi: 10.1371/journal.pone.0007247
Fülling, C., Dinan, T. G., Cryan, J. F. (2019). Gut microbe to brain signaling: what happens in vagus…. Neuron 101 (6), 998–1002. doi: 10.1016/j.neuron.2019.02.008
Fung, T. C., Olson, C. A., Hsiao, E. Y. (2017). Interactions between the microbiota, immune and nervous systems in health and disease. Nat. Neurosci. 20 (2), 145–155. doi: 10.1038/nn.4476
Ge, X., Ding, C., Zhao, W., Xu, L., Tian, H., Gong, J., et al. (2017). Antibiotics-induced depletion of mice microbiota induces changes in host serotonin biosynthesis and intestinal motility. J. Transl. Med. 15 (1), 13. doi: 10.1186/s12967-016-1105-4
Gerhardt, S., Mohajeri, M. H. (2018). Changes of colonic bacterial composition in parkinson's disease and other neurodegenerative diseases. Nutrients 10 (6), 708. doi: 10.3390/nu10060708
Ghezzi, L., Cantoni, C., Rotondo, E., Galimberti, D. (2022). The gut microbiome-brain crosstalk in neurodegenerative diseases. Biomedicines 10 (7), 1486. doi: 10.3390/biomedicines10071486
Goehler, L. E., Gaykema, R. P., Hansen, M. K., Anderson, K., Maier, S. F., Watkins, L. R. (2000). Vagal immune-to-brain communication: a visceral chemosensory pathway. Auton. Neurosci. 85 (1-3), 49–59. doi: 10.1016/s1566-0702(00)00219-8
Gorecki, A. M., Preskey, L., Bakeberg, M. C., Kenna, J. E., Gildenhuys, C., MacDougall, G., et al. (2019). Altered gut microbiome in parkinson's disease and the influence of lipopolysaccharide in a human α-synuclein over-expressing mouse model. Front. Neurosci. 13. doi: 10.3389/fnins.2019.00839
Gorky, J., Schwaber, J. (2016). The role of the gut-brain axis in alcohol use disorders. Prog. Neuropsychopharmacol. Biol. Psychiatry 65, 234–241. doi: 10.1016/j.pnpbp.2015.06.013
Goya, M. E., Xue, F., Sampedro-Torres-Quevedo, C., Arnaouteli, S., Riquelme-Dominguez, L., Romanowski, A., et al. (2020). Probiotic Bacillus subtilis Protects against α-Synuclein Aggregation in C. elegans. Cell Rep. 30 (2), 367–380.e367. doi: 10.1016/j.celrep.2019.12.078
Gray, M. T., Munoz, D. G., Gray, D. A., Schlossmacher, M. G., Woulfe, J. M. (2014). Alpha-synuclein in the appendiceal mucosa of neurologically intact subjects. Mov. Disord. 29 (8), 991–998. doi: 10.1002/mds.25779
Grigoruţă, M., Martínez-Martínez, A., Dagda, R. Y., Dagda, R. K. (2020). Psychological stress phenocopies brain mitochondrial dysfunction and motor deficits as observed in a parkinsonian rat model. Mol. Neurobiol. 57 (4), 1781–1798. doi: 10.1007/s12035-019-01838-9
Guo, S., Al-Sadi, R., Said, H. M., Ma, T. Y. (2013). Lipopolysaccharide causes an increase in intestinal tight junction permeability in vitro and in vivo by inducing enterocyte membrane expression and localization of TLR-4 and CD14. Am. J. Pathol. 182 (2), 375–387. doi: 10.1016/j.ajpath.2012.10.014
Guo, S., Nighot, M., Al-Sadi, R., Alhmoud, T., Nighot, P., Ma, T. Y. (2015). Lipopolysaccharide regulation of intestinal tight junction permeability is mediated by TLR4 signal transduction pathway activation of FAK and myD88. J. Immunol. 195 (10), 4999–5010. doi: 10.4049/jimmunol.1402598
Harms, A. S., Thome, A. D., Yan, Z., Schonhoff, A. M., Williams, G. P., Li, X., et al. (2018). Peripheral monocyte entry is required for alpha-Synuclein induced inflammation and Neurodegeneration in a model of Parkinson disease. Exp. Neurol. 300, 179–187. doi: 10.1016/j.expneurol.2017.11.010
Hasegawa, S., Goto, S., Tsuji, H., Okuno, T., Asahara, T., Nomoto, K., et al. (2015). Intestinal dysbiosis and lowered serum lipopolysaccharide-binding protein in parkinson's disease. PloS One 10 (11), e0142164. doi: 10.1371/journal.pone.0142164
He, Q., Yu, W., Wu, J., Chen, C., Lou, Z., Zhang, Q., et al. (2013). Intranasal LPS-mediated Parkinson's model challenges the pathogenesis of nasal cavity and environmental toxins. PloS One 8 (11), e78418. doi: 10.1371/journal.pone.0078418
Heiss, C. N., Olofsson, L. E. (2019). The role of the gut microbiota in development, function and disorders of the central nervous system and the enteric nervous system. J. Neuroendocrinol. 31 (5), e12684. doi: 10.1111/jne.12684
Heym, N., Heasman, B. C., Hunter, K., Blanco, S. R., Wang, G. Y., Siegert, R., et al. (2019). The role of microbiota and inflammation in self-judgement and empathy: implications for understanding the brain-gut-microbiome axis in depression. Psychopharmacol. (Berl). 236 (5), 1459–1470. doi: 10.1007/s00213-019-05230-2
Hold, G. L., Schwiertz, A., Aminov, R. I., Blaut, M., Flint, H. J. (2003). Oligonucleotide probes that detect quantitatively significant groups of butyrate-producing bacteria in human feces. Appl. Environ. Microbiol. 69 (7), 4320–4324. doi: 10.1128/aem.69.7.4320-4324.2003
Höschl, C., Hajek, T. (2001). Hippocampal damage mediated by corticosteroids–a neuropsychiatric research challenge. Eur. Arch. Psychiatry Clin. Neurosci. 251 Suppl 2, Ii81–Ii88. doi: 10.1007/bf03035134
Hou, Y., Li, X., Liu, C., Zhang, M., Zhang, X., Ge, S., et al. (2021a). Neuroprotective effects of short-chain fatty acids in MPTP induced mice model of Parkinson's disease. Exp. Gerontol. 150, 111376. doi: 10.1016/j.exger.2021.111376
Hou, Y. F., Shan, C., Zhuang, S. Y., Zhuang, Q. Q., Ghosh, A., Zhu, K. C., et al. (2021b). Gut microbiota-derived propionate mediates the neuroprotective effect of osteocalcin in a mouse model of Parkinson's disease. Microbiome 9 (1), 34. doi: 10.1186/s40168-020-00988-6
Houser, M. C., Tansey, M. G. (2017). The gut-brain axis: is intestinal inflammation a silent driver of Parkinson's disease pathogenesis? NPJ Parkinsons. Dis. 3, 3. doi: 10.1038/s41531-016-0002-0
Hsieh, T. H., Kuo, C. W., Hsieh, K. H., Shieh, M. J., Peng, C. W., Chen, Y. C., et al. (2020). Probiotics alleviate the progressive deterioration of motor functions in a mouse model of parkinson's disease. Brain Sci. 10 (4), 206. doi: 10.3390/brainsci10040206
Huang, T. T., Lai, J. B., Du, Y. L., Xu, Y., Ruan, L. M., Hu, S. H. (2019b). Current understanding of gut microbiota in mood disorders: an update of human studies. Front. Genet. 10. doi: 10.3389/fgene.2019.00098
Huang, Y., Li, M., Zhou, L., Xu, D., Qian, F., Zhang, J., et al. (2019c). Effects of qingluo tongbi decoction on gut flora of rats with adjuvant-induced arthritis and the underlying mechanism. Evid. Based. Complement. Alternat. Med. 2019, 6308021. doi: 10.1155/2019/6308021
Huang, H., Xu, H., Luo, Q., He, J., Li, M., Chen, H., et al. (2019a). Fecal microbiota transplantation to treat Parkinson's disease with constipation: A case report. Med. (Baltimore). 98 (26), e16163. doi: 10.1097/md.0000000000016163
Hyland, N. P., Cryan, J. F. (2010). A gut feeling about GABA: focus on GABA(B) receptors. Front. Pharmacol. 1. doi: 10.3389/fphar.2010.00124
Hylemon, P. B., Harris, S. C., Ridlon, J. M. (2018). Metabolism of hydrogen gases and bile acids in the gut microbiome. FEBS Lett. 592 (12), 2070–2082. doi: 10.1002/1873-3468.13064
Ibrahimagic, O. C., Jakubovic, A. C., Smajlovic, D., Dostovic, Z., Kunic, S., Iljazovic, A. (2016). Psychological stress and changes of hypothalamic-pituitary-adrenal axis in patients with "De novo" Parkinson's disease. Med. Arch. 70 (6), 445–448. doi: 10.5455/medarh.2016.70.445-448
Ilie, O. D., Paduraru, E., Robea, M. A., Balmus, I. M., Jijie, R., Nicoara, M., et al. (2021). The Possible Role of Bifidobacterium longum BB536 and Lactobacillus rhamnosus HN001 on Locomotor Activity and Oxidative Stress in a Rotenone-Induced Zebrafish Model of Parkinson's Disease. Oxid. Med. Cell Longev. 2021, 9629102. doi: 10.1155/2021/9629102
Inotsuka, R., Udono, M., Yamatsu, A., Kim, M., Katakura, Y. (2021). Exosome-mediated activation of neuronal cells triggered by γ-aminobutyric acid (GABA). Nutrients 13 (8), 2544. doi: 10.3390/nu13082544
Ito, M., Hirayama, M., Yamai, K., Goto, S., Ito, M., Ichihara, M., et al. (2012). Drinking hydrogen water and intermittent hydrogen gas exposure, but not lactulose or continuous hydrogen gas exposure, prevent 6-hydorxydopamine-induced Parkinson's disease in rats. Med. Gas. Res. 2 (1), 15. doi: 10.1186/2045-9912-2-15
Jang, H., Kim, S., Lee, J. M., Oh, Y. S., Park, S. M., Kim, S. R. (2017). Montelukast treatment protects nigral dopaminergic neurons against microglial activation in the 6-hydroxydopamine mouse model of Parkinson's disease. Neuroreport 28 (5), 242–249. doi: 10.1097/wnr.0000000000000740
Jang, J. H., Yeom, M. J., Ahn, S., Oh, J. Y., Ji, S., Kim, T. H., et al. (2020). Acupuncture inhibits neuroinflammation and gut microbial dysbiosis in a mouse model of Parkinson's disease. Brain Behav. Immun. 89, 641–655. doi: 10.1016/j.bbi.2020.08.015
Jena, P. K., Sheng, L., Nguyen, M., Di Lucente, J., Hu, Y., Li, Y., et al. (2020). Dysregulated bile acid receptor-mediated signaling and IL-17A induction are implicated in diet-associated hepatic health and cognitive function. biomark. Res. 8 (1), 59. doi: 10.1186/s40364-020-00239-8
Jeurink, P. V., van Esch, B. C., Rijnierse, A., Garssen, J., Knippels, L. M. (2013). Mechanisms underlying immune effects of dietary oligosaccharides. Am. J. Clin. Nutr. 98 (2), 572s–577s. doi: 10.3945/ajcn.112.038596
Ji, T., Huang, H., Liu, J., Peng, T., Zhou, X., Tan, Q., et al. (2021). Leveraging sequence-based faecal microbial community survey data to identify alterations in gut microbiota among patients with Parkinson's disease. Eur. J. Neurosci. 53 (2), 687–696. doi: 10.1111/ejn.14952
Ji, H. F., Shen, L. (2021). Probiotics as potential therapeutic options for Alzheimer's disease. Appl. Microbiol. Biotechnol. 105 (20), 7721–7730. doi: 10.1007/s00253-021-11607-1
Jia, X., Xu, W., Zhang, L., Li, X., Wang, R., Wu, S. (2021). Impact of gut microbiota and microbiota-related metabolites on hyperlipidemia. Front. Cell Infect. Microbiol. 11. doi: 10.3389/fcimb.2021.634780
Jiang, H., Ling, Z., Zhang, Y., Mao, H., Ma, Z., Yin, Y., et al. (2015). Altered fecal microbiota composition in patients with major depressive disorder. Brain Behav. Immun. 48, 186–194. doi: 10.1016/j.bbi.2015.03.016
Jiang, B., Yang, W., Chen, L., Wang, S., Chen, S., Bao, Y., et al. (2020). In vitro effects of Pueraria extract on ethanol-exposed microglia and neurons. BioMed. Pharmacother. 127, 110163. doi: 10.1016/j.biopha.2020.110163
Joers, V., Masilamoni, G., Kempf, D., Weiss, A. R., Rotterman, T. M., Murray, B., et al. (2020). Microglia, inflammation and gut microbiota responses in a progressive monkey model of Parkinson's disease: A case series. Neurobiol. Dis. 144, 105027. doi: 10.1016/j.nbd.2020.105027
Karunaratne, T. B., Okereke, C., Seamon, M., Purohit, S., Wakade, C., Sharma, A. (2020). Niacin and butyrate: nutraceuticals targeting dysbiosis and intestinal permeability in parkinson's disease. Nutrients 13 (1), 28. doi: 10.3390/nu13010028
Keene, C. D., Rodrigues, C. M., Eich, T., Chhabra, M. S., Steer, C. J., Low, W. C. (2002). Tauroursodeoxycholic acid, a bile acid, is neuroprotective in a transgenic animal model of Huntington's disease. Proc. Natl. Acad. Sci. U.S.A. 99 (16), 10671–10676. doi: 10.1073/pnas.162362299
Keller-Wood, M. E., Dallman, M. F. (1984). Corticosteroid inhibition of ACTH secretion. Endocr. Rev. 5 (1), 1–24. doi: 10.1210/edrv-5-1-1
Kelly, J. R., Borre, Y., O' Brien, C., Patterson, E., El Aidy, S., Deane, J., et al. (2016). Transferring the blues: Depression-associated gut microbiota induces neurobehavioural changes in the rat. J. Psychiatr. Res. 82, 109–118. doi: 10.1016/j.jpsychires.2016.07.019
Kelly, L. P., Carvey, P. M., Keshavarzian, A., Shannon, K. M., Shaikh, M., Bakay, R. A., et al. (2014). Progression of intestinal permeability changes and alpha-synuclein expression in a mouse model of Parkinson's disease. Mov. Disord. 29 (8), 999–1009. doi: 10.1002/mds.25736
Keshavarzian, A., Green, S. J., Engen, P. A., Voigt, R. M., Naqib, A., Forsyth, C. B., et al. (2015). Colonic bacterial composition in Parkinson's disease. Mov. Disord. 30 (10), 1351–1360. doi: 10.1002/mds.26307
Killinger, B. A., Madaj, Z., Sikora, J. W., Rey, N., Haas, A. J., Vepa, Y., et al. (2018). The vermiform appendix impacts the risk of developing Parkinson's disease. Sci. Transl. Med. 10 (465), eaar5280. doi: 10.1126/scitranslmed.aar5280
Kim, S., Jo, K., Hong, K. B., Han, S. H., Suh, H. J. (2019a). GABA and l-theanine mixture decreases sleep latency and improves NREM sleep. Pharm. Biol. 57 (1), 65–73. doi: 10.1080/13880209.2018.1557698
Kim, M. H., Kang, S. G., Park, J. H., Yanagisawa, M., Kim, C. H. (2013). Short-chain fatty acids activate GPR41 and GPR43 on intestinal epithelial cells to promote inflammatory responses in mice. Gastroenterology 145 (2), 396–406.e391-310. doi: 10.1053/j.gastro.2013.04.056
Kim, S., Kwon, S. H., Kam, T. I., Panicker, N., Karuppagounder, S. S., Lee, S., et al. (2019b). Transneuronal propagation of pathologic α-synuclein from the gut to the brain models parkinson's disease. Neuron 103 (4), 627–641.e627. doi: 10.1016/j.neuron.2019.05.035
Kiriyama, Y., Nochi, H. (2023). Role of microbiota-modified bile acids in the regulation of intracellular organelles and neurodegenerative diseases. Genes (Basel). 14 (4), 825. doi: 10.3390/genes14040825
Koh, A., De Vadder, F., Kovatcheva-Datchary, P., Bäckhed, F. (2016). From dietary fiber to host physiology: short-chain fatty acids as key bacterial metabolites. Cell 165 (6), 1332–1345. doi: 10.1016/j.cell.2016.05.041
Komaroff, A. L. (2018). The microbiome and risk for atherosclerosis. Jama 319 (23), 2381–2382. doi: 10.1001/jama.2018.5240
Kotagal, V., Spino, C., Bohnen, N. I., Koeppe, R., Albin, R. L. (2018). Serotonin, β-amyloid, and cognition in Parkinson disease. Ann. Neurol. 83 (5), 994–1002. doi: 10.1002/ana.25236
Kuai, X. Y., Yao, X. H., Xu, L. J., Zhou, Y. Q., Zhang, L. P., Liu, Y., et al. (2021). Evaluation of fecal microbiota transplantation in Parkinson's disease patients with constipation. Microb. Cell Fact. 20 (1), 98. doi: 10.1186/s12934-021-01589-0
Lacerda, J. E., Campos, R. R., Araujo, G. C., Andreatta-Van Leyen, S., Lopes, O. U., Guertzenstein, P. G. (2003). Cardiovascular responses to microinjections of GABA or anesthetics into the rostral ventrolateral medulla of conscious and anesthetized rats. Braz. J. Med. Biol. Res. 36 (9), 1269–1277. doi: 10.1590/s0100-879x2003000900019
Lai, F., Jiang, R., Xie, W., Liu, X., Tang, Y., Xiao, H., et al. (2018). Intestinal pathology and gut microbiota alterations in a methyl-4-phenyl-1,2,3,6-tetrahydropyridine (MPTP) mouse model of parkinson's disease. Neurochem Res 43 (10), 1986–1999. doi: 10.1007/s11064-018-2620-x
Lakhani, R., Vogel, K. R., Till, A., Liu, J., Burnett, S. F., Gibson, K. M., et al. (2014). Defects in GABA metabolism affect selective autophagy pathways and are alleviated by mTOR inhibition. EMBO Mol. Med. 6 (4), 551–566. doi: 10.1002/emmm.201303356
Lelieveld, I. M., Müller, M. L., Bohnen, N. I., Koeppe, R. A., Chervin, R. D., Frey, K. A., et al. (2012). The role of serotonin in sleep disordered breathing associated with Parkinson disease: a correlative [11C]DASB PET imaging study. PloS One 7 (7), e40166. doi: 10.1371/journal.pone.0040166
Levitt, M. D. (1969). Production and excretion of hydrogen gas in man. N. Engl. J. Med. 281 (3), 122–127. doi: 10.1056/nejm196907172810303
Li, Z., Lu, G., Li, Z., Wu, B., Luo, E., Qiu, X., et al. (2021). Altered actinobacteria and firmicutes phylum associated epitopes in patients with parkinson's disease. Front. Immunol. 12. doi: 10.3389/fimmu.2021.632482
Lin, J. C., Lin, C. S., Hsu, C. W., Lin, C. L., Kao, C. H. (2016). Association between parkinson's disease and inflammatory bowel disease: a nationwide Taiwanese retrospective cohort study. Inflammation Bowel. Dis. 22 (5), 1049–1055. doi: 10.1097/mib.0000000000000735
Liu, Z., Dai, X., Zhang, H., Shi, R., Hui, Y., Jin, X., et al. (2020). Gut microbiota mediates intermittent-fasting alleviation of diabetes-induced cognitive impairment. Nat. Commun. 11 (1), 855. doi: 10.1038/s41467-020-14676-4
Liu, B., Fang, F., Pedersen, N. L., Tillander, A., Ludvigsson, J. F., Ekbom, A., et al. (2017). Vagotomy and Parkinson disease: A Swedish register-based matched-cohort study. Neurology 88 (21), 1996–2002. doi: 10.1212/wnl.0000000000003961
Magistrelli, L., Amoruso, A., Mogna, L., Graziano, T., Cantello, R., Pane, M., et al. (2019). Probiotics may have beneficial effects in parkinson's disease: in vitro evidence. Front. Immunol. 10. doi: 10.3389/fimmu.2019.00969
Maier, L., Pruteanu, M., Kuhn, M., Zeller, G., Telzerow, A., Anderson, E. E., et al. (2018). Extensive impact of non-antibiotic drugs on human gut bacteria. Nature 555 (7698), 623–628. doi: 10.1038/nature25979
Malkki, H. (2017). Parkinson disease: Could gut microbiota influence severity of Parkinson disease? Nat. Rev. Neurol. 13 (2), 66–67. doi: 10.1038/nrneurol.2016.195
Mano, N., Goto, T., Uchida, M., Nishimura, K., Ando, M., Kobayashi, N., et al. (2004). Presence of protein-bound unconjugated bile acids in the cytoplasmic fraction of rat brain. J. Lipid Res. 45 (2), 295–300. doi: 10.1194/jlr.M300369-JLR200
Margineanu, M. B., Sherwin, E., Golubeva, A., Peterson, V., Hoban, A., Fiumelli, H., et al. (2020). Gut microbiota modulates expression of genes involved in the astrocyte-neuron lactate shuttle in the hippocampus. Eur. Neuropsychopharmacol. 41, 152–159. doi: 10.1016/j.euroneuro.2020.11.006
Marrinan, S., Emmanuel, A. V., Burn, D. J. (2014). Delayed gastric emptying in Parkinson's disease. Mov. Disord. 29 (1), 23–32. doi: 10.1002/mds.25708
Mastorakos, G., Zapanti, E. (2004). The hypothalamic-pituitary-adrenal axis in the neuroendocrine regulation of food intake and obesity: the role of corticotropin releasing hormone. Nutr. Neurosci. 7 (5-6), 271–280. doi: 10.1080/10284150400020516
Matcovitch-Natan, O., Winter, D. R., Giladi, A., Vargas Aguilar, S., Spinrad, A., Sarrazin, S., et al. (2016). Microglia development follows a stepwise program to regulate brain homeostasis. Science 353 (6301), aad8670. doi: 10.1126/science.aad8670
Matsumoto, M., Ooga, T., Kibe, R., Aiba, Y., Koga, Y., Benno, Y. (2017). Colonic absorption of low-molecular-weight metabolites influenced by the intestinal microbiome: A pilot study. PloS One 12 (1), e0169207. doi: 10.1371/journal.pone.0169207
Matteoli, G., Boeckxstaens, G. E. (2013). The vagal innervation of the gut and immune homeostasis. Gut 62 (8), 1214–1222. doi: 10.1136/gutjnl-2012-302550
Mayer, E. A., Knight, R., Mazmanian, S. K., Cryan, J. F., Tillisch, K. (2014). Gut microbes and the brain: paradigm shift in neuroscience. J. Neurosci. 34 (46), 15490–15496. doi: 10.1523/jneurosci.3299-14.2014
Mayer, E. A., Tillisch, K., Gupta, A. (2015). Gut/brain axis and the microbiota. J. Clin. Invest. 125 (3), 926–938. doi: 10.1172/jci76304
McVey Neufeld, K. A., Bienenstock, J., Bharwani, A., Champagne-Jorgensen, K., Mao, Y., West, C., et al. (2019). Oral selective serotonin reuptake inhibitors activate vagus nerve dependent gut-brain signalling. Sci. Rep. 9 (1), 14290. doi: 10.1038/s41598-019-50807-8
Meksawan, K., Chaotrakul, C., Leeaphorn, N., Gonlchanvit, S., Eiam-Ong, S., Kanjanabuch, T. (2016). Effects of fructo-oligosaccharide supplementation on constipation in elderly continuous ambulatory peritoneal dialysis patients. Perit. Dial. Int. 36 (1), 60–66. doi: 10.3747/pdi.2014.00015
Menezes, R. C., Fontes, M. A. (2007). Cardiovascular effects produced by activation of GABA receptors in the rostral ventrolateral medulla of conscious rats. Neuroscience 144 (1), 336–343. doi: 10.1016/j.neuroscience.2006.08.062
Miller, A. H., Raison, C. L. (2016). The role of inflammation in depression: from evolutionary imperative to modern treatment target. Nat. Rev. Immunol. 16 (1), 22–34. doi: 10.1038/nri.2015.5
Miller, T. L., Wolin, M. J. (1996). Pathways of acetate, propionate, and butyrate formation by the human fecal microbial flora. Appl. Environ. Microbiol. 62 (5), 1589–1592. doi: 10.1128/aem.62.5.1589-1592.1996
Moeller, A. H., Degnan, P. H., Pusey, A. E., Wilson, M. L., Hahn, B. H., Ochman, H. (2012). Chimpanzees and humans harbour compositionally similar gut enterotypes. Nat. Commun. 3, 1179. doi: 10.1038/ncomms2159
Mogi, M., Harada, M., Kondo, T., Riederer, P., Inagaki, H., Minami, M., et al. (1994a). Interleukin-1 beta, interleukin-6, epidermal growth factor and transforming growth factor-alpha are elevated in the brain from parkinsonian patients. Neurosci. Lett. 180 (2), 147–150. doi: 10.1016/0304-3940(94)90508-8
Mogi, M., Harada, M., Riederer, P., Narabayashi, H., Fujita, K., Nagatsu, T. (1994b). Tumor necrosis factor-alpha (TNF-alpha) increases both in the brain and in the cerebrospinal fluid from parkinsonian patients. Neurosci. Lett. 165 (1-2), 208–210. doi: 10.1016/0304-3940(94)90746-3
Mor, D. E., Tsika, E., Mazzulli, J. R., Gould, N. S., Kim, H., Daniels, M. J., et al. (2017). Dopamine induces soluble α-synuclein oligomers and nigrostriatal degeneration. Nat. Neurosci. 20 (11), 1560–1568. doi: 10.1038/nn.4641
Moreira, S., Fonseca, I., Nunes, M. J., Rosa, A., Lemos, L., Rodrigues, E., et al. (2017). Nrf2 activation by tauroursodeoxycholic acid in experimental models of Parkinson's disease. Exp. Neurol. 295, 77–87. doi: 10.1016/j.expneurol.2017.05.009
Mrabet, S., Ben Ali, N., Achouri, A., Dabbeche, R., Najjar, T., Haouet, S., et al. (2016). Gastrointestinal dysfunction and neuropathologic correlations in parkinson disease. J. Clin. Gastroenterol. 50 (9), e85–e90. doi: 10.1097/mcg.0000000000000606
Muthukumar, A. K., Stork, T., Freeman, M. R. (2014). Activity-dependent regulation of astrocyte GAT levels during synaptogenesis. Nat. Neurosci. 17 (10), 1340–1350. doi: 10.1038/nn.3791
Nair, A. T., Ramachandran, V., Joghee, N. M., Antony, S., Ramalingam, G. (2018). Gut microbiota dysfunction as reliable non-invasive early diagnostic biomarkers in the pathophysiology of parkinson's disease: A critical review. J. Neurogastroenterol. Motil. 24 (1), 30–42. doi: 10.5056/jnm17105
Neurology, L. (2016). Building on 50 years of levodopa therapy. Lancet Neurol. 15 (1), 1. doi: 10.1016/s1474-4422(15)00349-x
Nighot, M., Al-Sadi, R., Guo, S., Rawat, M., Nighot, P., Watterson, M. D., et al. (2017). Lipopolysaccharide-induced increase in intestinal epithelial tight permeability is mediated by toll-like receptor 4/myeloid differentiation primary response 88 (MyD88) activation of myosin light chain kinase expression. Am. J. Pathol. 187 (12), 2698–2710. doi: 10.1016/j.ajpath.2017.08.005
Nighot, M., Rawat, M., Al-Sadi, R., Castillo, E. F., Nighot, P., Ma, T. Y. (2019). Lipopolysaccharide-induced increase in intestinal permeability is mediated by TAK-1 activation of IKK and MLCK/MYLK gene. Am. J. Pathol. 189 (4), 797–812. doi: 10.1016/j.ajpath.2018.12.016
Nishiwaki, H., Hamaguchi, T., Ito, M., Ishida, T., Maeda, T., Kashihara, K., et al. (2020). Short-chain fatty acid-producing gut microbiota is decreased in parkinson's disease but not in rapid-eye-movement sleep behavior disorder. mSystems 5 (6), e00797–20. doi: 10.1128/mSystems.00797-20
Ohno, K., Ito, M., Ichihara, M., Ito, M. (2012). Molecular hydrogen as an emerging therapeutic medical gas for neurodegenerative and other diseases. Oxid. Med. Cell Longev. 2012, 353152. doi: 10.1155/2012/353152
Ohsawa, I., Ishikawa, M., Takahashi, K., Watanabe, M., Nishimaki, K., Yamagata, K., et al. (2007). Hydrogen acts as a therapeutic antioxidant by selectively reducing cytotoxic oxygen radicals. Nat. Med. 13 (6), 688–694. doi: 10.1038/nm1577
Olson, C. A., Iñiguez, A. J., Yang, G. E., Fang, P., Pronovost, G. N., Jameson, K. G., et al. (2021). Alterations in the gut microbiota contribute to cognitive impairment induced by the ketogenic diet and hypoxia. Cell Host Microbe 29 (9), 1378–1392.e1376. doi: 10.1016/j.chom.2021.07.004
Olszak, T., An, D., Zeissig, S., Vera, M. P., Richter, J., Franke, A., et al. (2012). Microbial exposure during early life has persistent effects on natural killer T cell function. Science 336 (6080), 489–493. doi: 10.1126/science.1219328
Pan, X., Elliott, C. T., McGuinness, B., Passmore, P., Kehoe, P. G., Hölscher, C., et al. (2017). Metabolomic profiling of bile acids in clinical and experimental samples of alzheimer's disease. Metabolites 7 (2), 28. doi: 10.3390/metabo7020028
Pan-Montojo, F., Schwarz, M., Winkler, C., Arnhold, M., O'Sullivan, G. A., Pal, A., et al. (2012). Environmental toxins trigger PD-like progression via increased alpha-synuclein release from enteric neurons in mice. Sci. Rep. 2, 898. doi: 10.1038/srep00898
Parada Venegas, D., de la Fuente, M. K., Landskron, G., González, M. J., Quera, R., Dijkstra, G., et al. (2019). Short chain fatty acids (SCFAs)-mediated gut epithelial and immune regulation and its relevance for inflammatory bowel diseases. Front. Immunol. 10. doi: 10.3389/fimmu.2019.00277
Parashar, A., Udayabanu, M. (2017). Gut microbiota: Implications in Parkinson's disease. Parkinsonism. Relat. Disord. 38, 1–7. doi: 10.1016/j.parkreldis.2017.02.002
Pascual, M., Calvo-Rodriguez, M., Núñez, L., Villalobos, C., Ureña, J., Guerri, C. (2021). Toll-like receptors in neuroinflammation, neurodegeneration, and alcohol-induced brain damage. IUBMB Life 73 (7), 900–915. doi: 10.1002/iub.2510
Pellegrini, C., Antonioli, L., Colucci, R., Ballabeni, V., Barocelli, E., Bernardini, N., et al. (2015). Gastric motor dysfunctions in Parkinson's disease: Current pre-clinical evidence. Parkinsonism. Relat. Disord. 21 (12), 1407–1414. doi: 10.1016/j.parkreldis.2015.10.011
Peralta Ramos, J. M., Iribarren, P., Bousset, L., Melki, R., Baekelandt, V., van der Perren, A. (2019). Peripheral inflammation regulates CNS immune surveillance through the recruitment of inflammatory monocytes upon systemic α-synuclein administration. Front. Immunol. 10. doi: 10.3389/fimmu.2019.00080
Perez-Burgos, A., Wang, B., Mao, Y. K., Mistry, B., McVey Neufeld, K. A., Bienenstock, J., et al. (2013). Psychoactive bacteria Lactobacillus rhamnosus (JB-1) elicits rapid frequency facilitation in vagal afferents. Am. J. Physiol. Gastrointest. Liver. Physiol. 304 (2), G211–G220. doi: 10.1152/ajpgi.00128.2012
Perez-Pardo, P., de Jong, E. M., Broersen, L. M., van Wijk, N., Attali, A., Garssen, J., et al. (2017). Promising effects of neurorestorative diets on motor, cognitive, and gastrointestinal dysfunction after symptom development in a mouse model of parkinson's disease. Front. Aging Neurosci. 9. doi: 10.3389/fnagi.2017.00057
Perez-Pardo, P., Dodiya, H. B., Engen, P. A., Forsyth, C. B., Huschens, A. M., Shaikh, M., et al. (2019). Role of TLR4 in the gut-brain axis in Parkinson's disease: a translational study from men to mice. Gut 68 (5), 829–843. doi: 10.1136/gutjnl-2018-316844
Perez-Pardo, P., Dodiya, H. B., Engen, P. A., Naqib, A., Forsyth, C. B., Green, S. J., et al. (2018). Gut bacterial composition in a mouse model of Parkinson's disease. Benef Microbes 9 (5), 799–814. doi: 10.3920/bm2017.0202
Plöger, S., Stumpff, F., Penner, G. B., Schulzke, J. D., Gäbel, G., Martens, H., et al. (2012). Microbial butyrate and its role for barrier function in the gastrointestinal tract. Ann. N. Y. Acad. Sci. 1258, 52–59. doi: 10.1111/j.1749-6632.2012.06553.x
Powley, T. L., Spaulding, R. A., Haglof, S. A. (2011). Vagal afferent innervation of the proximal gastrointestinal tract mucosa: chemoreceptor and mechanoreceptor architecture. J. Comp. Neurol. 519 (4), 644–660. doi: 10.1002/cne.22541
Priyadarshini, M., Kotlo, K. U., Dudeja, P. K., Layden, B. T. (2018). Role of short chain fatty acid receptors in intestinal physiology and pathophysiology. Compr. Physiol. 8 (3), 1091–1115. doi: 10.1002/cphy.c170050
Qian, Y., Yang, X., Xu, S., Huang, P., Li, B., Du, J., et al. (2020). Gut metagenomics-derived genes as potential biomarkers of Parkinson's disease. Brain 143 (8), 27474–2489. doi: 10.1093/brain/awaa201
Qiao, C. M., Sun, M. F., Jia, X. B., Li, Y., Zhang, B. P., Zhao, L. P., et al. (2020). Sodium butyrate exacerbates parkinson's disease by aggravating neuroinflammation and colonic inflammation in MPTP-induced mice model. Neurochem. Res. 45 (9), 2128–2142. doi: 10.1007/s11064-020-03074-3
Qin, W., Shi, Y., Chen, W., Jia, X., Asakawa, T. (2022). Can kynurenine pathway be considered as a next-generation therapeutic target for Parkinson's disease? An update information. Biosci. Trends 16 (4), 249–256. doi: 10.5582/bst.2022.01352
Raetz, C. R., Whitfield, C. (2002). Lipopolysaccharide endotoxins. Annu. Rev. Biochem. 71, 635–700. doi: 10.1146/annurev.biochem.71.110601.135414
Rani, L., Mondal, A. C. (2021). Unravelling the role of gut microbiota in Parkinson's disease progression: Pathogenic and therapeutic implications. Neurosci. Res. 168, 100–112. doi: 10.1016/j.neures.2021.01.001
Rees, K., Stowe, R., Patel, S., Ives, N., Breen, K., Clarke, C. E., et al. (2011). Helicobacter pylori eradication for Parkinson's disease. Cochrane Database Syst. Rev. 11), Cd008453. doi: 10.1002/14651858.CD008453.pub2
Ren, T., Gao, Y., Qiu, Y., Jiang, S., Zhang, Q., Zhang, J., et al. (2020). Gut microbiota altered in mild cognitive impairment compared with normal cognition in sporadic parkinson's disease. Front. Neurol. 11. doi: 10.3389/fneur.2020.00137
Ridlon, J. M., Kang, D. J., Hylemon, P. B. (2006). Bile salt biotransformations by human intestinal bacteria. J. Lipid Res. 47 (2), 241–259. doi: 10.1194/jlr.R500013-JLR200
Rietschel, E. T., Kirikae, T., SChade, F. U., Mamat, U., Schmidt, G., Loppnow, H., et al. (1994). Bacterial endotoxin: molecular relationships of structure to activity and function. FASEB J. 8 (2), 217–225. doi: 10.1096/fasebj.8.2.8119492
Rorsman, P., Berggren, P. O., Bokvist, K., Ericson, H., Möhler, H., Ostenson, C. G., et al. (1989). Glucose-inhibition of glucagon secretion involves activation of GABAA-receptor chloride channels. Nature 341 (6239), 233–236. doi: 10.1038/341233a0
Rosa, A. I., Fonseca, I., Nunes, M. J., Moreira, S., Rodrigues, E., Carvalho, A. N., et al. (2017). Novel insights into the antioxidant role of tauroursodeoxycholic acid in experimental models of Parkinson's disease. Biochim. Biophys. Acta Mol. Basis. Dis. 1863 (9), 2171–2181. doi: 10.1016/j.bbadis.2017.06.004
Rosas-Ballina, M., Olofsson, P. S., Ochani, M., Valdés-Ferrer, S. I., Levine, Y. A., Reardon, C., et al. (2011). Acetylcholine-synthesizing T cells relay neural signals in a vagus nerve circuit. Science 334 (6052), 98–101. doi: 10.1126/science.1209985
Rothhammer, V., Mascanfroni, I. D., Bunse, L., Takenaka, M. C., Kenison, J. E., Mayo, L., et al. (2016). Type I interferons and microbial metabolites of tryptophan modulate astrocyte activity and central nervous system inflammation via the aryl hydrocarbon receptor. Nat. Med. 22 (6), 586–597. doi: 10.1038/nm.4106
Russell, D. W., Setchell, K. D. (1992). Bile acid biosynthesis. Biochemistry 31 (20), 4737–4749. doi: 10.1021/bi00135a001
Rutsch, A., Kantsjö, J. B., Ronchi, F. (2020). The gut-brain axis: how microbiota and host inflammasome influence brain physiology and pathology. Front. Immunol. 11. doi: 10.3389/fimmu.2020.604179
Sampson, T. R., Debelius, J. W., Thron, T., Janssen, S., Shastri, G. G., Ilhan, Z. E., et al. (2016). Gut microbiota regulate motor deficits and neuroinflammation in a model of parkinson's disease. Cell 167 (6), 1469–1480.e1412. doi: 10.1016/j.cell.2016.11.018
Sanmarco, L. M., Wheeler, M. A., Gutiérrez-Vázquez, C., Polonio, C. M., Linnerbauer, M., Pinho-Ribeiro, F. A., et al. (2021). Gut-licensed IFNγ(+) NK cells drive LAMP1(+)TRAIL(+) anti-inflammatory astrocytes. Nature 590 (7846), 473–479. doi: 10.1038/s41586-020-03116-4
Savignac, H. M., Corona, G., Mills, H., Chen, L., Spencer, J. P., Tzortzis, G., et al. (2013). Prebiotic feeding elevates central brain derived neurotrophic factor, N-methyl-D-aspartate receptor subunits and D-serine. Neurochem. Int. 63 (8), 756–764. doi: 10.1016/j.neuint.2013.10.006
Schapira, A. H. (2013). Recent developments in biomarkers in Parkinson disease. Curr. Opin. Neurol. 26 (4), 395–400. doi: 10.1097/WCO.0b013e3283633741
Scheperjans, F., Aho, V., Pereira, P. A., Koskinen, K., Paulin, L., Pekkonen, E., et al. (2015). Gut microbiota are related to Parkinson's disease and clinical phenotype. Mov. Disord. 30 (3), 350–358. doi: 10.1002/mds.26069
Sechi, G. P., Conti, M., Sau, G. F., Cocco, G. A. (2008). Valproate-induced parkinsonism, glial cells and Alexander's disease. Prog. Neuropsychopharmacol. Biol. Psychiatry 32 (5), 1351–1352. doi: 10.1016/j.pnpbp.2008.03.022
Segerstrom, S. C., Miller, G. E. (2004). Psychological stress and the human immune system: a meta-analytic study of 30 years of inquiry. Psychol. Bull. 130 (4), 601–630. doi: 10.1037/0033-2909.130.4.601
Seifried, C., Boehncke, S., Heinzmann, J., Baudrexel, S., Weise, L., Gasser, T., et al. (2013). Diurnal variation of hypothalamic function and chronic subthalamic nucleus stimulation in Parkinson's disease. Neuroendocrinology 97 (3), 283–290. doi: 10.1159/000343808
Sharma, S., Awasthi, A., Singh, S. (2019). Altered gut microbiota and intestinal permeability in Parkinson's disease: Pathological highlight to management. Neurosci. Lett. 712, 134516. doi: 10.1016/j.neulet.2019.134516
Sharma, N., Nehru, B. (2015). Characterization of the lipopolysaccharide induced model of Parkinson's disease: Role of oxidative stress and neuroinflammation. Neurochem. Int. 87, 92–105. doi: 10.1016/j.neuint.2015.06.004
Shen, T., Yue, Y., He, T., Huang, C., Qu, B., Lv, W., et al. (2021). The association between the gut microbiota and parkinson's disease, a meta-analysis. Front. Aging Neurosci. 13. doi: 10.3389/fnagi.2021.636545
Shimizu, K., Ogura, H., Tomono, K., Tasaki, O., Asahara, T., Nomoto, K., et al. (2011). Patterns of Gram-stained fecal flora as a quick diagnostic marker in patients with severe SIRS. Dig. Dis. Sci. 56 (6), 1782–1788. doi: 10.1007/s10620-010-1486-9
Slavin, J. (2013). Fiber and prebiotics: mechanisms and health benefits. Nutrients 5 (4), 1417–1435. doi: 10.3390/nu5041417
Smith, P. M., Howitt, M. R., Panikov, N., Michaud, M., Gallini, C. A., Bohlooly, Y. M., et al. (2013). The microbial metabolites, short-chain fatty acids, regulate colonic Treg cell homeostasis. Science 341 (6145), 569–573. doi: 10.1126/science.1241165
Smith, S. M., Vale, W. W. (2006). The role of the hypothalamic-pituitary-adrenal axis in neuroendocrine responses to stress. Dialogues. Clin. Neurosci. 8 (4), 383–395. doi: 10.31887/DCNS.2006.8.4/ssmith
Soares, R., Ribeiro, F. F., Xapelli, S., Genebra, T., Ribeiro, M. F., Sebastião, A. M., et al. (2018). Tauroursodeoxycholic acid enhances mitochondrial biogenesis, neural stem cell pool, and early neurogenesis in adult rats. Mol. Neurobiol. 55 (5), 3725–3738. doi: 10.1007/s12035-017-0592-5
Song, Y., Gong, T., Saleh, M. G., Mikkelsen, M., Wang, G., Edden, R. A. E. (2021b). Upper brainstem GABA levels in Parkinson's disease. Magma 34 (5), 689–696. doi: 10.1007/s10334-021-00910-7
Song, Y., Gong, T., Xiang, Y., Mikkelsen, M., Wang, G., Edden, R. A. E. (2021c). Single-dose L-dopa increases upper brainstem GABA in Parkinson's disease: A preliminary study. J. Neurol. Sci. 422, 117309. doi: 10.1016/j.jns.2021.117309
Song, C., Zhang, Y., Cheng, L., Shi, M., Li, X., Zhang, L., et al. (2021a). Tea polyphenols ameliorates memory decline in aging model rats by inhibiting brain TLR4/NF-κB inflammatory signaling pathway caused by intestinal flora dysbiosis. Exp. Gerontol. 153, 111476. doi: 10.1016/j.exger.2021.111476
Spichak, S., Bastiaanssen, T. F. S., Berding, K., Vlckova, K., Clarke, G., Dinan, T. G., et al. (2021). Mining microbes for mental health: Determining the role of microbial metabolic pathways in human brain health and disease. Neurosci. Biobehav. Rev. 125, 698–761. doi: 10.1016/j.neubiorev.2021.02.044
Srivastav, S., Neupane, S., Bhurtel, S., Katila, N., Maharjan, S., Choi, H., et al. (2019). Probiotics mixture increases butyrate, and subsequently rescues the nigral dopaminergic neurons from MPTP and rotenone-induced neurotoxicity. J. Nutr. Biochem. 69, 73–86. doi: 10.1016/j.jnutbio.2019.03.021
Stalder, T., Steudte-Schmiedgen, S., Alexander, N., Klucken, T., Vater, A., Wichmann, S., et al. (2017). Stress-related and basic determinants of hair cortisol in humans: A meta-analysis. Psychoneuroendocrinology 77, 261–274. doi: 10.1016/j.psyneuen.2016.12.017
Strandwitz, P., Kim, K. H., Terekhova, D., Liu, J. K., Sharma, A., Levering, J., et al. (2019). GABA-modulating bacteria of the human gut microbiota. Nat. Microbiol. 4 (3), 396–403. doi: 10.1038/s41564-018-0307-3
Sun, J., Li, H., Jin, Y., Yu, J., Mao, S., Su, K. P., et al. (2021a). Probiotic Clostridium butyricum ameliorated motor deficits in a mouse model of Parkinson's disease via gut microbiota-GLP-1 pathway. Brain Behav. Immun. 91, 703–715. doi: 10.1016/j.bbi.2020.10.014
Sun, L. J., Li, J. N., Nie, Y. Z. (2020). Gut hormones in microbiota-gut-brain cross-talk. Chin. Med. J. (Engl). 133 (7), 826–833. doi: 10.1097/cm9.0000000000000706
Sun, P., Su, L., Zhu, H., Li, X., Guo, Y., Du, X., et al. (2021b). Gut microbiota regulation and their implication in the development of neurodegenerative disease. Microorganisms 9 (11), 2281. doi: 10.3390/microorganisms9112281
Sun, M. F., Zhu, Y. L., Zhou, Z. L., Jia, X. B., Xu, Y. D., Yang, Q., et al. (2018). Neuroprotective effects of fecal microbiota transplantation on MPTP-induced Parkinson's disease mice: Gut microbiota, glial reaction and TLR4/TNF-α signaling pathway. Brain Behav. Immun. 70, 48–60. doi: 10.1016/j.bbi.2018.02.005
Suzuki, A., Ito, M., Hamaguchi, T., Mori, H., Takeda, Y., Baba, R., et al. (2018). Quantification of hydrogen production by intestinal bacteria that are specifically dysregulated in Parkinson's disease. PloS One 13 (12), e0208313. doi: 10.1371/journal.pone.0208313
Svensson, E., Horváth-Puhó, E., Thomsen, R. W., Djurhuus, J. C., Pedersen, L., Borghammer, P., et al. (2015). Vagotomy and subsequent risk of Parkinson's disease. Ann. Neurol. 78 (4), 522–529. doi: 10.1002/ana.24448
Talham, G. L., Jiang, H. Q., Bos, N. A., Cebra, J. J. (1999). Segmented filamentous bacteria are potent stimuli of a physiologically normal state of the murine gut mucosal immune system. Infect. Immun. 67 (4), 1992–2000. doi: 10.1128/iai.67.4.1992-2000.1999
Tamtaji, O. R., Taghizadeh, M., Daneshvar Kakhaki, R., Kouchaki, E., Bahmani, F., Borzabadi, S., et al. (2019). Clinical and metabolic response to probiotic administration in people with Parkinson's disease: A randomized, double-blind, placebo-controlled trial. Clin. Nutr. 38 (3), 1031–1035. doi: 10.1016/j.clnu.2018.05.018
Tan, A. H., Chong, C. W., Lim, S. Y., Yap, I. K. S., Teh, C. S. J., Loke, M. F., et al. (2021). Gut microbial ecosystem in parkinson disease: new clinicobiological insights from multi-omics. Ann. Neurol. 89 (3), 546–559. doi: 10.1002/ana.25982
Tan, A. H., Lim, S. Y., Lang, A. E. (2022). The microbiome-gut-brain axis in Parkinson disease - from basic research to the clinic. Nat. Rev. Neurol. 18 (8), 476–495. doi: 10.1038/s41582-022-00681-2
Tan, A. H., Lim, S. Y., Mahadeva, S., Loke, M. F., Tan, J. Y., Ang, B. H., et al. (2020). Helicobacter pylori eradication in parkinson's disease: A randomized placebo-controlled trial. Mov. Disord. 35 (12), 2250–2260. doi: 10.1002/mds.28248
Terán-Ventura, E., Aguilera, M., Vergara, P., Martínez, V. (2014). Specific changes of gut commensal microbiota and TLRs during indomethacin-induced acute intestinal inflammation in rats. J. Crohns. Colitis. 8 (9), 1043–1054. doi: 10.1016/j.crohns.2014.02.001
Tilg, H., Cani, P. D., Mayer, E. A. (2016). Gut microbiome and liver diseases. Gut 65 (12), 2035–2044. doi: 10.1136/gutjnl-2016-312729
Tong, Q., Zhang, L., Yuan, Y., Jiang, S., Zhang, R., Xu, Q., et al. (2015). Reduced plasma serotonin and 5-hydroxyindoleacetic acid levels in Parkinson's disease are associated with nonmotor symptoms. Parkinsonism. Relat. Disord. 21 (8), 882–887. doi: 10.1016/j.parkreldis.2015.05.016
Tysnes, O. B., Kenborg, L., Herlofson, K., Steding-Jessen, M., Horn, A., Olsen, J. H., et al. (2015). Does vagotomy reduce the risk of Parkinson's disease? Ann. Neurol. 78 (6), 1011–1012. doi: 10.1002/ana.24531
Umesaki, Y., Okada, Y., Matsumoto, S., Imaoka, A., Setoyama, H. (1995). Segmented filamentous bacteria are indigenous intestinal bacteria that activate intraepithelial lymphocytes and induce MHC class II molecules and fucosyl asialo GM1 glycolipids on the small intestinal epithelial cells in the ex-germ-free mouse. Microbiol. Immunol. 39 (8), 555–562. doi: 10.1111/j.1348-0421.1995.tb02242.x
Umesaki, Y., Setoyama, H., Matsumoto, S., Imaoka, A., Itoh, K. (1999). Differential roles of segmented filamentous bacteria and clostridia in development of the intestinal immune system. Infect. Immun. 67 (7), 3504–3511. doi: 10.1128/iai.67.7.3504-3511.1999
Urrutia, A., García-Angulo, V. A., Fuentes, A., Caneo, M., Legüe, M., Urquiza, S., et al. (2020). Bacterially produced metabolites protect C. elegans neurons from degeneration. PloS Biol. 18 (3), e3000638. doi: 10.1371/journal.pbio.3000638
van den Heuvel, L. L., du Plessis, S., Stalder, T., Acker, D., Kirschbaum, C., Carr, J., et al. (2020). Hair glucocorticoid levels in Parkinson's disease. Psychoneuroendocrinology 117, 104704. doi: 10.1016/j.psyneuen.2020.104704
van der Meij, A., Wermer, M. J. H. (2021). Vagus nerve stimulation: a potential new treatment for ischaemic stroke. Lancet 397 (10284), 1520–1521. doi: 10.1016/s0140-6736(21)00667-x
Vascellari, S., Palmas, V., Melis, M., Pisanu, S., Cusano, R., Uva, P., et al. (2020). Gut microbiota and metabolome alterations associated with parkinson's disease. mSystems 5 (5), e00561–20. doi: 10.1128/mSystems.00561-20
Villumsen, M., Aznar, S., Pakkenberg, B., Jess, T., Brudek, T. (2019). Inflammatory bowel disease increases the risk of Parkinson's disease: a Danish nationwide cohort study 1977-2014. Gut 68 (1), 18–24. doi: 10.1136/gutjnl-2017-315666
Vithlani, M., Terunuma, M., Moss, S. J. (2011). The dynamic modulation of GABA(A) receptor trafficking and its role in regulating the plasticity of inhibitory synapses. Physiol. Rev. 91 (3), 1009–1022. doi: 10.1152/physrev.00015.2010
Wang, Q., Davis, P. B., Qi, X., Chen, S. G., Gurney, M. E., Perry, G., et al. (2021b). Gut-microbiota-microglia-brain interactions in Alzheimer's disease: knowledge-based, multi-dimensional characterization. Alzheimers Res. Ther. 13 (1), 177. doi: 10.1186/s13195-021-00917-1
Wang, H. B., Wang, P. Y., Wang, X., Wan, Y. L., Liu, Y. C. (2012). Butyrate enhances intestinal epithelial barrier function via up-regulation of tight junction protein Claudin-1 transcription. Dig. Dis. Sci. 57 (12), 3126–3135. doi: 10.1007/s10620-012-2259-4
Wang, S., Xu, C., Liu, H., Wei, W., Zhou, X., Qian, H., et al. (2023). Connecting the gut microbiota and neurodegenerative diseases: the role of bile acids. Mol. Neurobiol. 60 (8), 4618–4640. doi: 10.1007/s12035-023-03340-9
Wang, H., Yang, F., Zhang, S., Xin, R., Sun, Y. (2021a). Genetic and environmental factors in Alzheimer's and Parkinson's diseases and promising therapeutic intervention via fecal microbiota transplantation. NPJ Parkinsons. Dis. 7 (1), 70. doi: 10.1038/s41531-021-00213-7
Weimers, P., Halfvarson, J., Sachs, M. C., Saunders-Pullman, R., Ludvigsson, J. F., Peter, I., et al. (2019). Inflammatory bowel disease and parkinson's disease: A nationwide swedish cohort study. Inflammation Bowel. Dis. 25 (1), 111–123. doi: 10.1093/ibd/izy190
Wekerle, H. (2017). Brain autoimmunity and intestinal microbiota: 100 trillion game changers. Trends Immunol. 38 (7), 483–497. doi: 10.1016/j.it.2017.03.008
Westfall, S., Lomis, N., Kahouli, I., Dia, S. Y., Singh, S. P., Prakash, S. (2017). Microbiome, probiotics and neurodegenerative diseases: deciphering the gut brain axis. Cell Mol. Life Sci. 74 (20), 3769–3787. doi: 10.1007/s00018-017-2550-9
Wikoff, W. R., Anfora, A. T., Liu, J., Schultz, P. G., Lesley, S. A., Peters, E. C., et al. (2009). Metabolomics analysis reveals large effects of gut microflora on mammalian blood metabolites. Proc. Natl. Acad. Sci. U.S.A. 106 (10), 3698–3703. doi: 10.1073/pnas.0812874106
Williams, S., Chen, L., Savignac, H. M., Tzortzis, G., Anthony, D. C., Burnet, P. W. (2016). Neonatal prebiotic (BGOS) supplementation increases the levels of synaptophysin, GluN2A-subunits and BDNF proteins in the adult rat hippocampus. Synapse 70 (3), 121–124. doi: 10.1002/syn.21880
Wong, Y. Y., Wu, C. Y., Yu, D., Kim, E., Wong, M., Elez, R., et al. (2022). Biofluid markers of blood-brain barrier disruption and neurodegeneration in Lewy body spectrum diseases: A systematic review and meta-analysis. Parkinsonism. Relat. Disord. 101, 119–128. doi: 10.1016/j.parkreldis.2022.06.004
Wosu, A. C., Valdimarsdóttir, U., Shields, A. E., Williams, D. R., Williams, M. A. (2013). Correlates of cortisol in human hair: implications for epidemiologic studies on health effects of chronic stress. Ann. Epidemiol. 23 (12), 797–811.e792. doi: 10.1016/j.annepidem.2013.09.006
Wu, W. L., Adame, M. D., Liou, C. W., Barlow, J. T., Lai, T. T., Sharon, G., et al. (2021c). Microbiota regulate social behaviour via stress response neurons in the brain. Nature 595 (7867), 409–414. doi: 10.1038/s41586-021-03669-y
Wu, L., Han, Y., Zheng, Z., Zhu, S., Chen, J., Yao, Y., et al. (2021b). Obeticholic acid inhibits anxiety via alleviating gut microbiota-mediated microglia accumulation in the brain of high-fat high-sugar diet mice. Nutrients 13 (3), 940. doi: 10.3390/nu13030940
Wu, J., Lang, H., Mu, X., Zhang, Z., Su, Q., Hu, X., et al. (2021a). Honey bee genetics shape the strain-level structure of gut microbiota in social transmission. Microbiome 9 (1), 225. doi: 10.1186/s40168-021-01174-y
Xie, M., Chen, H. H., Nie, S. P., Yin, J. Y., Xie, M. Y. (2017). Gamma-aminobutyric acid increases the production of short-chain fatty acids and decreases pH values in mouse colon. Molecules 22 (4), 653. doi: 10.3390/molecules22040653
Xie, K., Yu, Y., Pei, Y., Hou, L., Chen, S., Xiong, L., et al. (2010). Protective effects of hydrogen gas on murine polymicrobial sepsis via reducing oxidative stress and HMGB1 release. Shock 34 (1), 90–97. doi: 10.1097/SHK.0b013e3181cdc4ae
Xie, G., Zhong, W., Li, H., Li, Q., Qiu, Y., Zheng, X., et al. (2013). Alteration of bile acid metabolism in the rat induced by chronic ethanol consumption. FASEB J. 27 (9), 3583–3593. doi: 10.1096/fj.13-231860
Xue, L. J., Yang, X. Z., Tong, Q., Shen, P., Ma, S. J., Wu, S. N., et al. (2020). Fecal microbiota transplantation therapy for Parkinson's disease: A preliminary study. Med. (Baltimore). 99 (35), e22035. doi: 10.1097/md.0000000000022035
Yamatsu, A., Yamashita, Y., Pandharipande, T., Maru, I., Kim, M. (2016). Effect of oral γ-aminobutyric acid (GABA) administration on sleep and its absorption in humans. Food Sci. Biotechnol. 25 (2), 547–551. doi: 10.1007/s10068-016-0076-9
Yan, Y., Ren, S., Duan, Y., Lu, C., Niu, Y., Wang, Z., et al. (2021a). Gut microbiota and metabolites of α-synuclein transgenic monkey models with early stage of Parkinson's disease. NPJ Biofilms. Microbiomes. 7 (1), 69. doi: 10.1038/s41522-021-00242-3
Yan, Z., Yang, F., Cao, J., Ding, W., Yan, S., Shi, W., et al. (2021b). Alterations of gut microbiota and metabolome with Parkinson's disease. Microb. Pathog. 160, 105187. doi: 10.1016/j.micpath.2021.105187
Yang, W., Yu, T., Huang, X., Bilotta, A. J., Xu, L., Lu, Y., et al. (2020). Intestinal microbiota-derived short-chain fatty acids regulation of immune cell IL-22 production and gut immunity. Nat. Commun. 11 (1), 4457. doi: 10.1038/s41467-020-18262-6
Yano, J. M., Yu, K., Donaldson, G. P., Shastri, G. G., Ann, P., Ma, L., et al. (2015). Indigenous bacteria from the gut microbiota regulate host serotonin biosynthesis. Cell 161 (2), 264–276. doi: 10.1016/j.cell.2015.02.047
Yoritaka, A., Takanashi, M., Hirayama, M., Nakahara, T., Ohta, S., Hattori, N. (2013). Pilot study of H2 therapy in Parkinson's disease: a randomized double-blind placebo-controlled trial. Mov. Disord. 28 (6), 836–839. doi: 10.1002/mds.25375
Yu, F., Han, W., Zhan, G., Li, S., Xiang, S., Zhu, B., et al. (2019). Abnormal gut microbiota composition contributes to cognitive dysfunction in streptozotocin-induced diabetic mice. Aging (Albany. NY). 11 (10), 3262–3279. doi: 10.18632/aging.101978
Yu, C. D., Xu, Q. J., Chang, R. B. (2020). Vagal sensory neurons and gut-brain signaling. Curr. Opin. Neurobiol. 62, 133–140. doi: 10.1016/j.conb.2020.03.006
Yuan, Q., Xin, L., Han, S., Su, Y., Wu, R., Liu, X., et al. (2021). Lactulose improves neurological outcomes by repressing harmful bacteria and regulating inflammatory reactions in mice after stroke. Front. Cell Infect. Microbiol. 11. doi: 10.3389/fcimb.2021.644448
Yunes, R. A., Poluektova, E. U., Dyachkova, M. S., Klimina, K. M., Kovtun, A. S., Averina, O. V., et al. (2016). GABA production and structure of gadB/gadC genes in Lactobacillus and Bifidobacterium strains from human microbiota. Anaerobe 42, 197–204. doi: 10.1016/j.anaerobe.2016.10.011
Zhang, Y., Huang, R., Cheng, M., Wang, L., Chao, J., Li, J., et al. (2019). Gut microbiota from NLRP3-deficient mice ameliorates depressive-like behaviors by regulating astrocyte dysfunction via circHIPK2. Microbiome 7 (1), 116. doi: 10.1186/s40168-019-0733-3
Zhang, F., Yue, L., Fang, X., Wang, G., Li, C., Sun, X., et al. (2020). Altered gut microbiota in Parkinson's disease patients/healthy spouses and its association with clinical features. Parkinsonism. Relat. Disord. 81, 84–88. doi: 10.1016/j.parkreldis.2020.10.034
Zhang, B., Zhang, Y., Wu, W., Xu, T., Yin, Y., Zhang, J., et al. (2017). Chronic glucocorticoid exposure activates BK-NLRP1 signal involving in hippocampal neuron damage. J. Neuroinflamm. 14 (1), 139. doi: 10.1186/s12974-017-0911-9
Zhao, Z., Ning, J., Bao, X. Q., Shang, M., Ma, J., Li, G., et al. (2021). Fecal microbiota transplantation protects rotenone-induced Parkinson's disease mice via suppressing inflammation mediated by the lipopolysaccharide-TLR4 signaling pathway through the microbiota-gut-brain axis. Microbiome 9 (1), 226. doi: 10.1186/s40168-021-01107-9
Zhao, L., Wang, Y., Zhang, G., Zhang, T., Lou, J., Liu, J. (2019). L-arabinose elicits gut-derived hydrogen production and ameliorates metabolic syndrome in C57BL/6J mice on high-fat-diet. Nutrients 11 (12), 3054. doi: 10.3390/nu11123054
Zheng, X., Chen, T., Zhao, A., Wang, X., Xie, G., Huang, F., et al. (2016). The brain metabolome of male rats across the lifespan. Sci. Rep. 6, 24125. doi: 10.1038/srep24125
Zheng, S. Y., Li, H. X., Xu, R. C., Miao, W. T., Dai, M. Y., Ding, S. T., et al. (2021). Potential roles of gut microbiota and microbial metabolites in Parkinson's disease. Ageing Res. Rev. 69, 101347. doi: 10.1016/j.arr.2021.101347
Keywords: gut microbiota, Parkinson’s disease, microbiota-gut-brain axis, inflammatory reaction, neuronal pathway
Citation: Jia X, Chen Q, Zhang Y and Asakawa T (2023) Multidirectional associations between the gut microbiota and Parkinson’s disease, updated information from the perspectives of humoral pathway, cellular immune pathway and neuronal pathway. Front. Cell. Infect. Microbiol. 13:1296713. doi: 10.3389/fcimb.2023.1296713
Received: 19 September 2023; Accepted: 27 November 2023;
Published: 15 December 2023.
Edited by:
Tao Lin, Baylor College of Medicine, United StatesReviewed by:
Almagul Kushugulova, Nazarbayev University, KazakhstanCopyright © 2023 Jia, Chen, Zhang and Asakawa. This is an open-access article distributed under the terms of the Creative Commons Attribution License (CC BY). The use, distribution or reproduction in other forums is permitted, provided the original author(s) and the copyright owner(s) are credited and that the original publication in this journal is cited, in accordance with accepted academic practice. No use, distribution or reproduction is permitted which does not comply with these terms.
*Correspondence: Yuanyuan Zhang, Z3p6anp5eTgwMzRAMTYzLmNvbQ==; Tetsuya Asakawa, YXNha2F3YXQxOTcxQGdtYWlsLmNvbQ==
†ORCID: Tetsuya Asakawa, orcid.org/0000-0002-2300-3509
Disclaimer: All claims expressed in this article are solely those of the authors and do not necessarily represent those of their affiliated organizations, or those of the publisher, the editors and the reviewers. Any product that may be evaluated in this article or claim that may be made by its manufacturer is not guaranteed or endorsed by the publisher.
Research integrity at Frontiers
Learn more about the work of our research integrity team to safeguard the quality of each article we publish.