- Department of Laboratory Medicine, Shengjing Hospital of China Medical University, Liaoning Clinical Research Center for Laboratory Medicine, Shenyang, China
Extracellular vesicles (EVs) are nano-sized particles released from cells into the extracellular environment, and are separated from eukaryotic cells, bacteria, and other organisms with cellular structures. EVs alter cell communication by delivering their contents and performing various functions depending on their cargo and release into certain environments or other cells. The cell walls of Gram-positive bacteria have a thick peptidoglycan layer and were previously thought to be unable to produce EVs. However, recent studies have demonstrated that Gram-positive bacterial EVs are crucial for health and disease. In this review, we have summarized the formation, composition, and characteristics of the contents, resistance to external stress, participation in immune regulation, and other functions of Gram-positive bacterial EVs, as well as their application in clinical diagnosis and treatment, to provide a new perspective to further our understanding of Gram-positive bacterial EVs.
1 Introduction
Extracellular vesicles (EVs) are nanoparticles surrounded by a lipid bilayer, secreted by bacteria or eukaryotic cells (Derkus et al., 2017). In 1966, EVs from E. coli were first observed by Work et al. and described as cellular vesicles containing lipopolysaccharides and interstitial membrane proteins secreted by outer membrane protrusions (Work et al., 1966). Subsequently, Gram-negative bacteria, such as Pseudomonas aeruginosa, Salmonella Typhimurium, and Helicobacter pylori, have been shown to produce and secrete EVs (Mullaney et al., 2009; Bai et al., 2014; Sartorio et al., 2021).
EVs are also known as outer membrane vesicles (OMVs) in Gram-negative bacteria and membrane vesicles (MVs) in Gram-positive bacteria. Bacterial EVs have various biological functions, such as exerting effects on bacterial virulence, antibiotic resistance, transfer of virulence genes, helping bacteria survive and evade immune responses, transporting biomolecules to host cells, and assisting in clinical disease treatment (Yaron et al., 2000; Kulp and Kuehn, 2010; Aung et al., 2016; Aytar Çelik et al., 2022). The study of EVs is continuously increasing; however, most studies are currently focused on eukaryotic and Gram-negative bacteria, and studies on EVs in Gram-positive bacteria remain limited.
Gram-positive bacteria, especially Gram-positive cocci, such as coagulase-negative Staphylococcus, Staphylococcus aureus, Enterococcus, and Clostridium difficile, are crucial pathogens in hospitals. According to the 2021 China Antimicrobial Surveillance Network (CHINET) data (Hu et al., 2022), Gram-negative bacteria accounted for 71.4% of the clinical isolates from member hospitals, whereas Gram-positive bacteria accounted for 28.6%, which is approximately half the number of Gram-negative bacterial cases. Although the number of cases is not as high as that of Gram-negative bacteria, they can cause severe infections and increase mortality. In 2016, the number of deaths in 195 countries owing to Streptococcus pneumoniae-induced lower respiratory tract infections alone was approximately 1.19 million (Troeger et al., 2018).
With the widespread use of antibiotics, drug-resistant bacteria have evolved, and the number of antibiotic-resistant Gram-positive bacteria strains has increased in some countries (Lessa et al., 2015; Lakhundi and Zhang, 2018; Watkins et al., 2022). Multi-drug resistant (MDR) bacteria, such as methicillin-resistant Staphylococcus aureus (MRSA), vancomycin-resistant Enterococcus faecalis (VRE), and β-lactamase-producing Streptococcus pneumoniae, often render multiple antibiotic treatments ineffective, resulting in difficult-to-cure chronic infection in patients, and an increased mortality rate. According to the statistics, the in-hospital mortality rate of bloodstream infections caused by Staphylococcus aureus is approximately 32.4% in middle-income countries (Bai et al., 2022). Despite a decrease in the mortality rate over the past 30 years, at least 25% of patients die within three months (Bai et al., 2022).
In this context, MVs are vital in bacterial virulence factor pathogenesis, drug resistance mechanisms, and immune regulation. Therefore, the study of Gram-positive bacterial MVs is of considerable significance in gaining insights into the pathogenesis of Gram-positive bacteria and providing novel therapeutic approaches for clinical practice. Therefore, in this paper, we review the composition and characteristics of Gram-positive bacterial MVs, their functions in auxiliary escape and immunomodulation, and their clinical applications.
2 Formation of Gram-positive bacteria MVs
In Gram-negative bacteria, there are three main hypotheses for vesicle formation: membrane curvature-inducing proteins induce the formation of spherical vesicles by increasing the membrane curvature, dissociation of the crosslinking between the outer membrane and peptidoglycan to produce vesicles, and mutations in the VacJ/Yrb ATP-binding box (ABC) transport system inducing vesicle production (Roier et al., 2016; Toyofuku et al., 2019). Gram-positive bacteria have not been studied as extensively as Gram-negative bacteria, and we lack a complete model for the formation of MVs. It is now believed that the formation of Gram-positive bacterial MVs is primarily mediated by the outgrowth of specific lipid-enriched regions within the cytoplasmic membrane, which subsequently weakens the thick peptidoglycan layer in the presence of endolysins and facilitates MV release (Toyofuku et al., 2019). Furthermore, the formation of MVs is not determined by a small number of genes but is regulated by a complex network of genes (Briaud and Carroll, 2020).
MVs are produced by various Gram-positive bacteria, including Staphylococcus aureus, Streptococcus pneumoniae, Mycobacterium tuberculosis, Lactobacillus, Bacillus subtilis, Bacillus anthracis, Listeria monocytogenes, and Clostridium perfringens (Jiang et al., 2014; White et al., 2018; Choi et al., 2019; Karthikeyan et al., 2020; Abe et al., 2021; Bitto et al., 2021; Mehanny et al., 2022). Their diameters range between 20 and 500 nm (Brown et al., 2015). The differences in the size of MVs may be related to the purification technique and measurement method used (Aytar Çelik et al., 2022), environmental factors of vesicle growth, and the influence of membrane lipid genes (Toyofuku et al., 2019).
For example, Codemo et al. observed Streptococcus pneumoniae MVs in the range of 25–250 nm by electron microscopy using the OptiPrep density gradient media method (Codemo et al., 2018), whereas Mehanny et al. determined MVs in the range of 130–160 nm using size exclusion chromatography (SEC) for vesicle purification and nanoparticle tracking analysis (NTA) (Mehanny et al., 2020). The sizes of bacterial MVs also vary across different growth periods (Mehanny et al., 2022). Bacteria must uptake and utilize nutrients from the environment during growth. The production of EVs is influenced by many environmental factors, such as hypoxia, temperature, iron deficiency, and antibiotic stimulation (Orench-Rivera and Kuehn, 2016). Poor external environmental conditions such as non-growing temperatures and high salt, acid, and iron deficiencies increase the secretion of MVs (Lee T. et al., 2017; Karthikeyan et al., 2020; Wang et al., 2021).
3 Composition and characteristics of Gram-positive bacteria MVs
EVs of Gram-positive bacteria contain fatty acids, phospholipids, cytoplasmic proteins, membrane-associated virulence proteins, lipid acids, peptidoglycans, DNA, and sRNA (Yu et al., 2018) (Figure 1).
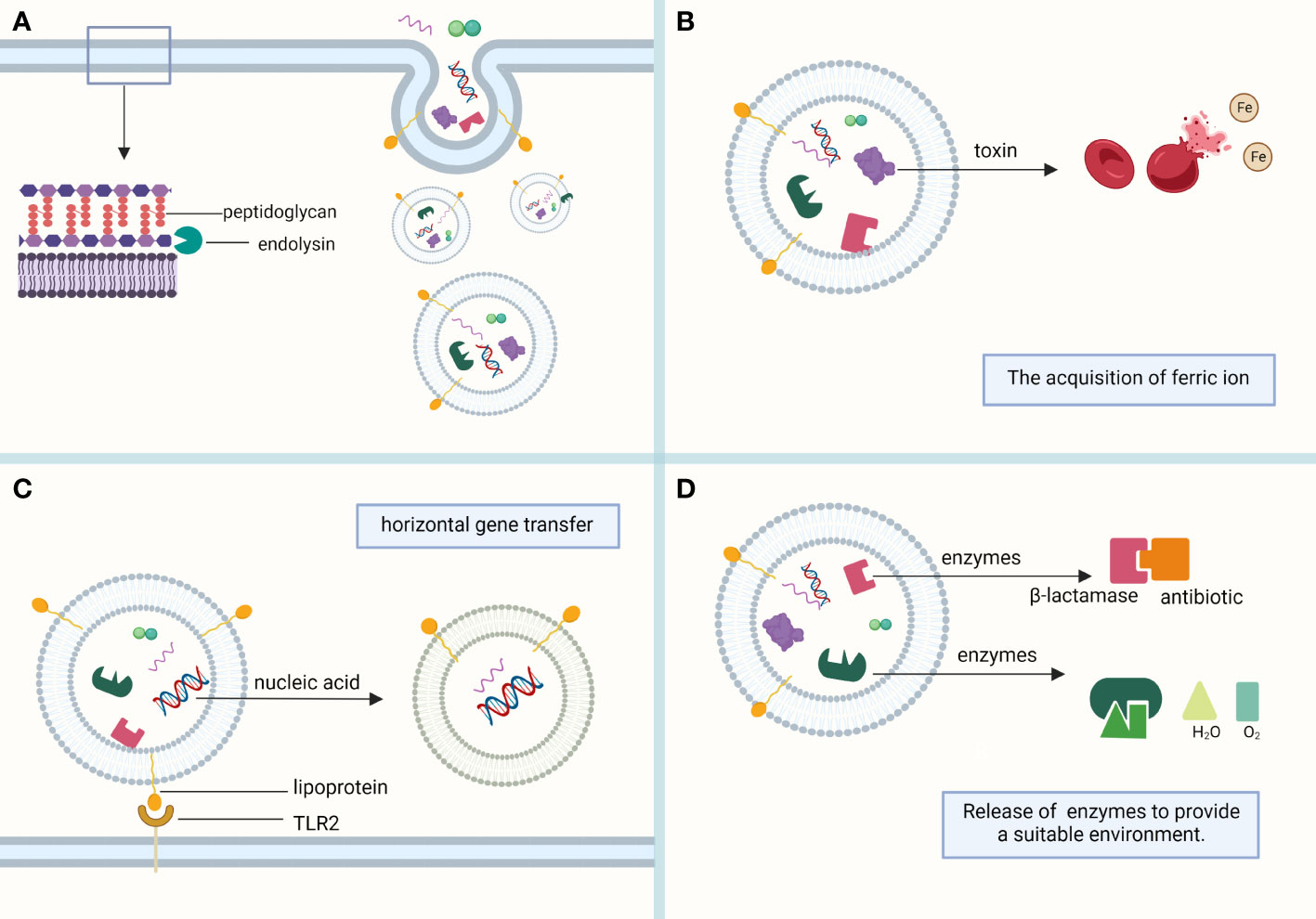
Figure 1 Composition of MVs. (A) MVs sprout in the lipid-rich region of the cell membrane and are formed with the help of the endolysin, which weakens the bacterial peptidoglycan layer. MVs mainly contain phospholipids, proteins, and nucleic acids, which play their respective roles. (B) Toxins in MVs, such as alpha-hemolysin and leukocidins, help lyse red blood cells to obtain the iron required for bacterial survival. (C) Bacterial heritable materials can affect the biological function of bacteria through MV-mediated horizontal gene transfer. Lipoprotein, a membrane protein contained in MVs, is involved in immune response as a Toll-like receptor 2 (TLR2) ligand. (D) The antibiotic-inactivating enzymes and catalases contained in MVs can hydrolyze antibiotics and reactive oxygen species, respectively, to provide a suitable living environment for bacteria. (This figure was created using Biorender.com).
3.1 Proteins
The proteins in bacterial EVs perform various physiological functions. Gram-negative and Gram-positive bacteria both secrete proteins, but the protein composition and quantity within bacterial EVs vary. Notably, the types of proteins detected in the MVs of different strains vary significantly within the same genus (Olaya-Abril et al., 2014; Mehanny et al., 2022). For example, Jeon et al. reported via proteomic analysis that MVs isolated from three S. aureus strains from clinical sources contained 60–85 specific proteins with varying cytotoxicities (Jeon et al., 2016). Nonetheless, core proteins, such as the surface protein PspA, ABC transporter protein substrate-binding protein, and penicillin-binding protein 1 B, are mostly conserved.
Lipoprotein enrichment has also been observed in the proteomic analysis of MVs of most Gram-positive bacteria, such as S. aureus, S. pneumoniae, S. pyogenes, B. subtilis, and M. tuberculosis (Lee et al., 2009; Brown et al., 2014; Olaya-Abril et al., 2014; Biagini et al., 2015; Lee et al., 2015). Bacterial lipoproteins are a group of membrane proteins with many functions and are key ligands of Toll-like receptor 2 (TLR2) in Gram-positive bacteria that play a vital role in host immune responses to bacterial infection (Zähringer et al., 2008; Tomlinson et al., 2014). Lipoprotein deficiency affects bacterial growth, immune activation, and virulence (Stoll et al., 2005; Khandavilli et al., 2008). The absence of pre-prolipoprotein diacylglyceryl transferase (Lgt) in S. pneumoniae delays bacterial growth and reduces its invasion capacity (Chimalapati et al., 2012).
Furthermore, lipoproteins influence the MVs they produced. The absence of lipoproteins significantly affects the host immune response to S. pneumoniae MVs. MVs produced by lipoprotein-deficient S. pneumoniae mutants lead to decreased nuclear factor-kappa B (NF-κB) activity and reduced cytokine production by macrophages in mice, and the levels of immune responses induced by these cytokines are reduced (Yerneni et al., 2021). Similarly, the immunostimulatory ability of MVs produced by Lgt-mutant S. aureus in mice decreased significantly (Kopparapu et al., 2021). In M. tuberculosis MVs, lipoproteins stimulate the TNF-α production by naïve macrophages (Bhatnagar et al., 2007). Thus, lipoproteins are crucial in regulating systemic immune responses to MVs.
3.2 Virulence factors and toxins
The production of virulence factors or toxins is a common survival and attack mechanism of microorganisms, which is necessary for host pathogenesis (Weiser et al., 2018) and is a crucial component of MVs. Pathogenic Gram-negative bacterial vesicles can encapsulate these virulence factors for delivery to host cells (Bomberger et al., 2009), and a similar phenomenon can also be observed in Gram-positive bacteria.
Virulence factors vary among different bacterial MVs and play multiple roles in regulating host responses to bacterial infection and contribute to the colonization and invasion of bacteria (Table 1). The adhesins CapD and PrpA, and collagen-binding proteins Acm and Scm, detected in Enterococcus faecium MVs contribute to the adhesion and colonization of E. faecium (Wagner et al., 2018). Virulence factors, such as α-hemolysin contained in S. aureus MVs, promote the survival of S. aureus in human blood while conferring bacterial resistance to neutrophils (Askarian et al., 2018). Propionibacterium acnes MVs contain hyaluronate lyase, a critical virulence factor that invades host cells (Jeon et al., 2016). Additionally, bacteria can promote infection and induce immunomodulatory responses after delivering virulence factors to the host cells through EVs. B. anthracis MVs contain a variety of toxins, such as protective antigen (PA), lethal factor (LF), edema factor (EF), and anthrolysin (ALO), which immunize mice. The PA causes mice to produce a strong IgM response to the toxin and deliver the toxin to host cells, increasing the potency of the toxin (Rivera et al., 2010). Listeria monocytogenes MVs contain a toxic force protein, listeriolysin O, which induces increased cytotoxicity in the human colon adenocarcinoma Caco-2 cell line, contributes to internalization and participates in interactions with host proteins during bacterial infections (Karthikeyan et al., 2019; Karthikeyan et al., 2020). Toxins in MVs are transported to host cells to elicit their effects and exacerbate damage to the host. For example, staphylococcal protein A (SPA) in S. aureus MVs induces more severe eczematous dermatitis in patients with atopic dermatitis, which may be the result of SPA-induced production of pro-inflammatory cytokines and chemokines, leading to the recruitment of inflammatory cells and local inflammation in atopic dermatitis lesions (Jun et al., 2017).
3.3 Genetic material
Dorward and Garon. (1989) discovered DNA in the vesicles of Neisseria gonorrhoeae (Dorward and Garon, 1989). With the continuous development of vesicle studies, RNA, including sRNA, mRNA, tRNA, and rRNA, has been discovered in the vesicles of Gram-negative bacteria (Quek et al., 2016; Han et al., 2019; Langlete et al., 2019; Diallo et al., 2022). Similar to bacteria, EVs carry genetic material that plays a vital role in their function. In addition to stabilizing and protecting transported proteins from hydrolytic digestion by extracellular proteases, bacterial EVs also protect their nucleic acid cargo from degradation by extracellular nucleases. Therefore, they can deliver numerous bioactive macromolecules to bacterially infected host cells (Doré and Boilard, 2023).
Gram-positive bacterial MVs also possess genetic material (Jiang et al., 2014; Surve et al., 2016; Domínguez Rubio et al., 2017) that can be safely translocated to host cells. For example, miRNAs in Streptococcus sanguinis MVs are protected from degradation by RNase A, and are thus safely transported to host cells (Choi et al., 2016). Group A streptococcal MVs contain RNAs that differ in abundance from the original bacteria, where the release of rRNA and tRNA is influenced by the two-component “control of virulence regulator-sensor” operon (covRS) (Resch et al., 2016).
Furthermore, encapsulated nucleic acid molecules participate in host cell immune responses through different pathways. The DNA and RNA detected in S. aureus MVs can be transported with MVs to host epithelial cells, thereby activating pattern recognition receptors (PRRs) to promote the release of cytokines and chemokines from epithelial cells, followed by autophagy-mediated degradation (Bitto et al., 2021). Transcriptomic sequencing data revealed that Listeria monocytogenes MVs contained various forms of RNA, including tRNA, rRNA, mRNA, and sRNA. rli32 sRNA in MVs correlated with the IFN-β response after incubation with bone marrow macrophages (Frantz et al., 2019). Despite the role of genetic material in Gram-positive bacterial MVs in immune regulation, the exact mechanisms by which these DNA and RNA molecules are involved in host cell immune regulation, and other mechanisms remain unclear.
4 Function of Gram-positive bacteria MVs
OMVs and MVs can be coated with proteins, toxins, nucleic acids, lipids, and other substances for transport to other bacterial species or mammalian cell receptors for their corresponding functions. They are critical for generating immune effects, mediating horizontal gene transfer, generating antimicrobial resistance, nutrient uptake, and transporting virulence factors. They are essential for the survival of both Gram-negative and Gram-positive bacteria. The functions of MVs are heavily mediated by their cargo (Figure 1).
4.1 Strengthening resistance to external pressure
EVs can enhance bacterial resistance to environmental factors, prevent bacterial damage by nutrient uptake from the environment, promote biofilm formation, and produce catalases and antibiotic-inactivating enzymes (Figure 1).
Iron is essential for bacterial survival and growth (Ferreira et al., 2016). Mycobactins are high-affinity iron chelators or siderophores secreted by M. tuberculosis. Iron deficiency results in increased MV production in M. tuberculosis and elevated local concentrations of mycobactins (as part of vesicles), which are subsequently released to provide extracellular iron to support bacterial proliferation (Prados-Rosales et al., 2011). The production of MVs from S. aureus increases under iron-deficient conditions in culture, and α-hemolysin, leukocidin LukED and HlgAB in MVs lyse erythrocytes to release hemoglobin and heme to promote iron acquisition by bacteria (Wang et al., 2021). Similarly, the presence of iron-binding factors in Streptomyces coelicolor contributes to bacterial survival under iron-limited conditions (Schrempf et al., 2011).
Bacterial EVs contain several substances that help bacteria survive external environmental stresses. Antibiotics are the most common environmental stressors encountered by bacteria. Antibiotic-inactivating enzymes can be released into EVs to degrade antibiotics, thereby counteracting the associated damage. MRSA produces MVs that increase in a dose-dependent manner in the presence of ampicillin and contain more β-lactamase enzymes and metallo β-lactamase superfamily proteins, which hydrolyze β-lactam antibiotics. These vesicle-encapsulated proteins can be transported over long distances to prevent degradation and significantly contribute to the development of antibiotic resistance in bacteria (Kim S. et al., 2020). MRSA can also transfer β-lactamases to antimicrobial‐sensitive Escherichia coli via MVs, causing them to secrete several times more β-lactamases than the parental strain to protect against antibiotic pressure (Lee et al., 2022). The expanding application of antibiotics and their excessive use expose bacteria to external stresses more frequently. Further investigation is required to ascertain whether EVs can be stably inherited as a protective mechanism against antibiotics, as well as whether such a mechanism exists in all bacteria.
In addition to producing antibiotic-inactivating enzymes, some MVs harbor substances, such as catalase, to protect bacteria from the disruptive effects of reactive oxygen species. For example, adding MVs to the growth medium of L. monocytogenes induces the production of more MVs under oxidative stress. Superoxide dismutase decomposition and catalase can be detected in these MVs, which may provide a suitable environment for bacteria in oxidative environments (Wang et al., 2021).
4.2 Participation in immune regulation
Immunomodulation is pivotal for the host response to bacterial infections. In addition to the direct interaction between bacteria and host immune cells, MVs released by bacteria can also contribute to host defense by directly or indirectly initiating immune responses and affecting immune cell populations, consequently triggering the recruitment of immune cells and release of pro-inflammatory cytokines.
MVs induce an immune response and participate in innate and adaptive immunity in Gram-positive bacteria. Among the innate immune responses stimulated by MVs in the host, the primary responses involve immune cells, such as macrophages and dendritic cells, as well as TLR molecules, and produce various pro-inflammatory factors. S. pneumoniae MVs induce NF-κB activation in a dose-dependent manner after co-incubation with macrophages, resulting in a significant increase in macrophages in the blood of mice injected with MVs (Yerneni et al., 2021). A similar phenomenon was observed in MVs secreted by Streptococcus suis, which may contribute to increased blood-brain barrier permeability (Haas and Grenier, 2015). M. tuberculosis MVs carry lipoproteins that are essential ligands for TLR2 and activate macrophage responses upon binding with TLR2 (Prados-Rosales et al., 2011).
Furthermore, MVs can mediate different infection outcomes through different intracellular pathways. MVs secreted during Listeria monocytogenes infection can accumulate in lysosomes through endocytosis in non-phagocytic cells and cause an autophagic response by releasing MVs from cells in the phagocytic body (Vdovikova et al., 2017). NOD-, LRR- and pyrin domain-containing 3 (NLRP3) inflammasome plays a vital role in the innate immune response and disease development. Its activation is a host defense mechanism for clearing damaged and infected cells. MVs released by S. aureus mediate the immune response of macrophages through the TLR2 signaling pathway and induce NLRP3 inflammasome activation and production of IL-1β and IL-18 (Wang et al., 2020), which contribute to the establishment of an effective innate immune response to S. aureus infection. Gram-positive bacterial MVs are also involved in adaptive immune responses, primarily through the stimulation of host antibody production, thereby providing immune protection. This property aids vaccines development.
4.3 Assisting bacterial survival and escape
Bacteria typically defend themselves against the immune response of host cells upon entry by producing MVs that release specific components and create a favorable environment for immune escape. For example, lipoglycans from MVs of M. tuberculosis are transported to T cells to stimulate the expression of GRAIL, a marker of T cell anergy, in CD4 + T cells, thus inhibiting T cell responses to facilitate immune escape (Athman et al., 2017). S. pneumoniae MVs can bind to complement C3 in the presence of choline-binding proteins, forming an attack membrane complex that reduces bacterial interactions with the complementary site on phagocytes and contributes to bacterial evasion of host humoral immunity (Codemo et al., 2018).
Neutrophil extracellular traps (NETs), produced after neutrophil death, are another response to bacterial elimination. NETs are DNA networks covered with antimicrobial peptides and histones that can degrade virulence factors and kill bacteria, and belong to a novel intrinsic immune defense mechanism (Brinkmann et al., 2004). During an immune attack by the host, DNase is produced to degrade NETs, which aids bacteria in escaping host killing. The DNase TatD contained in S. pneumoniae MVs helps the bacteria escape NETs; consequently, S. pneumoniae that lack TatD have diminished virulence (Jhelum et al., 2018). A similar phenomenon was observed in S. suis MVs (Haas and Grenier, 2015).
Simultaneously, MVs can help bacteria to resist killing within the host. Various toxins contained in S. aureus MVs increase the resistance of bacteria to neutrophils in human blood and promote bacterial survival (Askarian et al., 2018). MVs evade the killing effect of neutrophils in the host as well as act as molecular decoys to protect bacteria in some cases. To protect human skin and nasal secretions from antimicrobial fatty acids (AFAs), S. aureus releases MVs as decoys to bind to AFA and avoid damage caused by the combination of the bacterial membrane and AFAs (Kengmo Tchoupa and Peschel, 2020).
4.4 Horizontal gene transfer
Microorganisms transfer genetic material via horizontal gene transfer (HGT), which alters or influences biological functions. EVs contain various components, including DNA and various types of RNA, and fusion of these genetic materials encapsulated by EVs mediates the occurrence of HGT (Erdmann et al., 2017). Three main mechanisms underlie HGT: conjugation, transformation, and transduction (Soucy et al., 2015). EVs carrying genetic material may also mediate HGT (Dell’Annunziata et al., 2021). MVs of Ruminococcus spp contain double-stranded DNA and promote HGT in bacteria that can catabolize cellulose; thus, wild transformants also have the heritable cellulolytic ability (Klieve et al., 2005). Furthermore, bacteriophages in B. subtilis can help MVs perform gene transfer and render the recipient bacterial phage sensitive (Tzipilevich et al., 2016).
Bacterial EVs can resist pressure from the external environment by containing antibiotic-inactivating enzymes; concordantly, bacterial EVs can also use HGT to improve antibiotic resistance; however, most studies focus solely on EVs produced by Gram-negative bacteria (Rumbo et al., 2011; Li et al., 2022). Although HGT-mediated antibiotic resistance exists in Gram-positive bacteria of frequent clinical concern, such as E. faecalis, S. aureus, Streptococcus, and M. tuberculosis (Derbyshire and Gray, 2014; Foster, 2017; García-Solache and Rice, 2019), the role of Gram-positive bacterial MVs in HGT, especially in the transfer of resistance genes, remains poorly understood. Therefore, a comprehensive exploration of the association between HGT and MVs could help to address the problem of antibiotic resistance in Gram-positive bacteria.
5 Clinical application of Gram-positive bacterial MVs
Most clinical studies have focused on the hazards of EVs produced by pathogenic bacteria but have neglected the contribution of EVs in diagnosing and treating diseases and maintaining microbial homeostasis in humans (Figure 2).
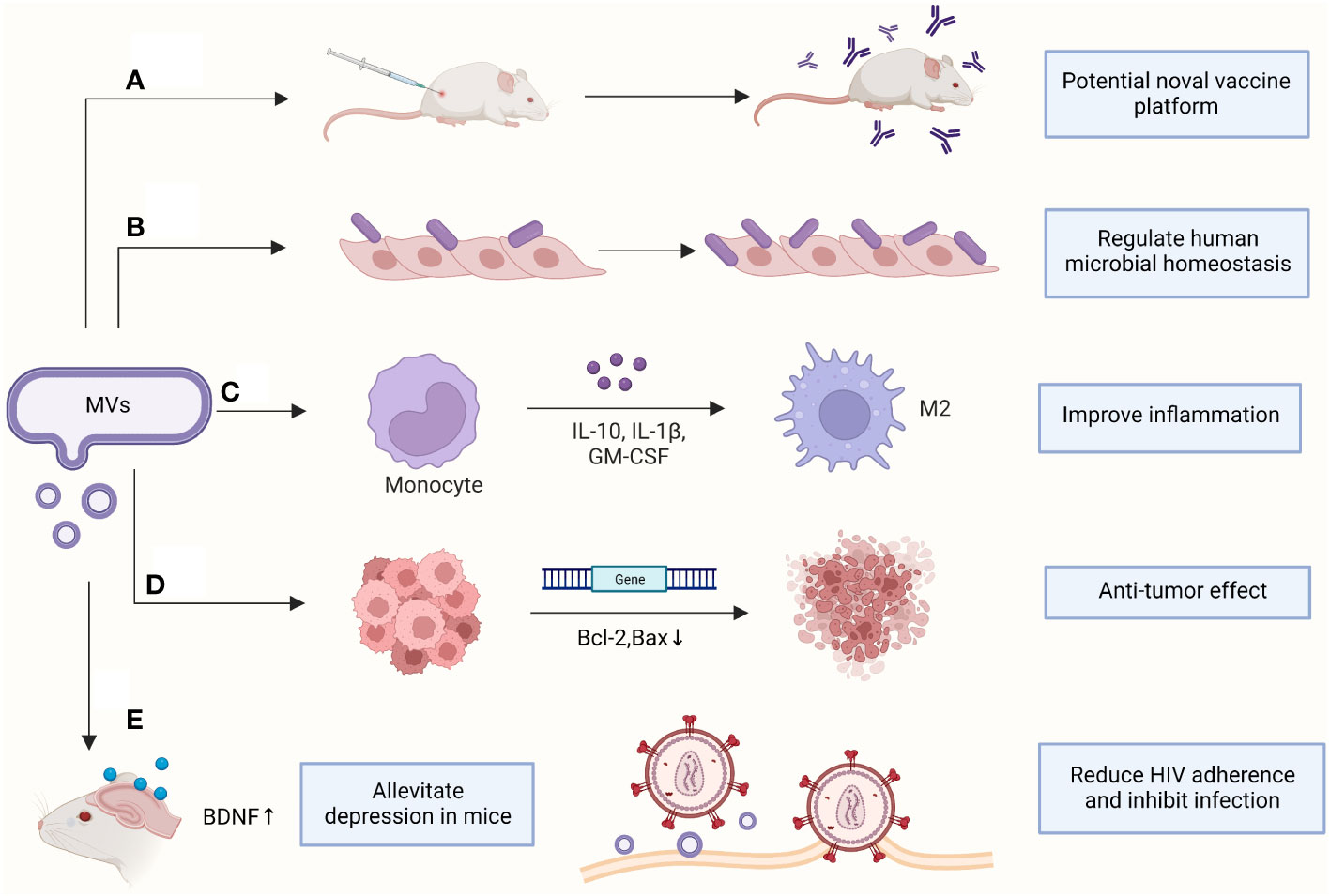
Figure 2 Clinical applications of MVs. (A) Antibodies were produced to protect mice from sepsis; (B) Increasing the adhesion of probiotics to vaginal epithelia; (C) Promoting macrophage polarization and improving inflammatory response; (D) Downregulating genes in cancer cells to induce apoptosis; (E) Assisting clinical disease treatment. BDNF, brain-derived neurotrophic factor; GM-CSF, Granulocyte-macrophage colony-stimulating factor. (This figure was created using Biorender.com).
5.1 Vaccine preparations
In addition to transporting virulence factors and proteins with immune effects to host cells and inducing inflammatory and immune responses, the immunity and stability of EVs highlights their potential as vaccine candidates. Currently, S. pneumoniae and Bacillus Calmette-Guérin (BCG) vaccines are commonly used in clinical practice. However, the capsular polysaccharide vaccine PPSV23 and polysaccharide conjugate vaccine PCV13 in pneumococcal vaccines cover only a limited number of serotypes, and the occurrence of capsular conversion leads to an increase in non-serotype patients. Therefore, there is an urgent need to develop novel vaccines.
Mice vaccinated with nonpathogenic (noncapsular) S. pneumoniae MVs have increased survival under live S. pneumoniae attack compared with that in unvaccinated mice and provide cross-protection against attacks by different S. pneumoniae strains (Choi et al., 2017). Simultaneously, multiple specific immunogenic proteins in S. pneumoniae MVs support their potential as next-generation vaccines (Olaya-Abril et al., 2014). Immunization of mice with MVs produced by S. aureus mutants with suppression of the alpha toxin-encoding gene resulted in increased levels of cytolysin-neutralizing antibodies in the serum of mice and demonstrated protection in a lethal mouse model of sepsis (Wang et al., 2018) (Figure 2).
In addition to their high immunogenicity, MVs have the ability to elicit immune responses in the absence of adjuvants. Vesicles extracted from M. tuberculosis can elicit an immune response comparable with that of BCG without an adjuvant and enhance protective efficacy if injected together with BCG (Prados-Rosales et al., 2014). However, the use of MVs as vaccines presents several challenges that need to be addressed. For example, MVs extracted from bacteria contain lipids, toxins, and other substances that can be toxic to humans or result in adverse effects. Moreover, a limited quantity of MVs can be effectively introduced into the human body without undergoing degradation or inducing significant biological responses. Therefore, further investigation and development are required before MV vaccine formulations can pass adequate clinical trials.
5.2 Assisting disease diagnosis
The release of probiotic MVs can reflect the relationship between the host microbiome, disease, and health conditions, providing a novel detection technique for diagnosis. MVs in the urine and blood of patients with autism spectrum disorder (ASD) can be used to assess the microbiota of patients and thus understand the role of gut microbiota-brain modulation in ASD symptoms (Lee Y. et al., 2017). The current gold standard for the rapid assessment of tuberculosis is mainly by microbial culture and nucleic acid amplification techniques; however, the long cycle time of microbial culture and poor specificity of the latter often cause delays in diagnosis. Schirmer et al. reported that the immunostrips of vesicles isolated from patients with latent and active tuberculosis were consistently higher than that of healthy volunteers, which may contribute to the diagnosis of latent TB (Schirmer et al., 2022). In addition, pneumococcal MVs isolated from the blood or urine of patients with Streptococcus pneumoniae-associated hemolytic uremic syndrome contain highly abundant proteins, which can be used as markers of MVs in Sp-HUS and become the a potential diagnostic indicator for Sp-HUS (Battista et al., 2023).
The use of MVs in the auxiliary diagnosis of diseases is non-invasive and rapid; however, further research must be conducted to determine the accuracy and specificity of MV detection, which would facilitate auxiliary diagnosis of diseases with complex or prolonged diagnoses and deepen our understanding.
5.3 Regulating the disorder of human microbial ecology
Lactobacillus spp. and Bifidobacterium spp. are two commonly used probiotics and Gram-positive bacteria that maintain the healthy state of the human intestine. They are mostly used clinically to regulate intestinal disorders. MVs isolated from Lactobacillus rhamnosus JB-1 are involved in the activation and signaling of multiple PRRs between intestinal epithelial cells and the enteric nervous system (Al-Nedawi et al., 2014). In the presence of MVs, Lactobacillus enhances host cell adhesion and reduces the adhesion of opportunistic pathogens, contributing to the maintenance of homeostasis in the vagina (Croatti et al., 2022). In addition to probiotics, Clostridium butyricum also exhibits a similar effect. Clostridium butyricum MVs not only ameliorated symptoms in mice with ulcerative colitis, but also facilitated the restoration of intestinal ecological balance and reconstruction of gut microbiota (Liang et al., 2022).
5.4 Improving inflammatory response
In addition to inducing host immune response, MVs can use anti-inflammatory factors to enhance the inflammatory response. For example, Lactobacillus plantarum MVs significantly promote the expression of cell surface markers of M2-type macrophages and anti-inflammatory cytokines in human skin tissue cultures, which improve the inflammatory skin state (Kim W. et al., 2020). The blend of Lactobacillus shortus MVs and vitamin D3 lessens H. pylori attachment to AGS human gastric carcinoma cells, strengthens cell-to-cell connections, and diminishes H. pylori-induced inflammation. This combination holds potential as a novel therapy for H. pylori infection (Nabavi-Rad et al., 2023).
Some probiotics can improve allergic diseases by inducing antigen-specific regulatory T cells (Tregs). The MVs secreted by these probiotics can exhibit a similar effect. For example, Bifidobacterium Bifidum MVs are potential therapeutic adjuvants as they can be used to stimulate in vitro dendritic cells to promote Treg cell differentiation and induce proinflammatory factor homeostasis (López et al., 2011). Moreover, MVs produced by Bifidobacterium longum contain bacterial extracellular solute-binding protein (ESBP), which reduces the number of mast cells, thereby reducing allergic reactions to food (Kim et al., 2015). Micrococcus luteus MV-specific IgG1 and IgG4 levels were significantly lower in asthmatic individuals than in healthy subjects. In asthmatic mice, these MVs decreased IL-1β and IL-17 levels, along with the number of group 3 innate lymphoid cells (ILC3s), thus contributing to asthma alleviation (Sim et al., 2023).
5.5 Anti-tumor effect
Currently, the development of cancer treatments is focused on targeted drugs, but other strategies to identify and activate tumor immunity are also being explored. Many bacterial products, such as toxins, peptides, and enzymes, have been increasingly developed for cancer therapy (Soleimani and Javadi, 2022). Some Gram-negative bacterial OMVs have been used as anti-tumor therapeutic vector nanovaccines to inhibit tumor growth based on their potent adjuvant-induced antibody production ability (Huang et al., 2020). Gram-positive bacterial MVs also exhibit properties that could be helpful for the development of cancer treatments. Lactobacillus rhamnosus GG is a probiotic strain commonly used as a probiotic supplement. It produces MVs that have cytotoxic effects on cancer cells at certain concentrations and induce apoptosis in liver cancer cells, primarily by downregulating the expression of bcl-2 and bax genes in cancer cells (Behzadi et al., 2017). Similarly, MVs produced by Lacticaseibacillus paracasei PC-H1 can penetrate colorectal cancer cells, leading to significant suppression of phosphorylation of 3-phosphoinositide-dependent protein kinase-1 (PDK1) and serine/threonine protein kinase (AKT). This, in turn, downregulates Bcl-2 protein expression, ultimately inducing apoptosis in cancer cells and demonstrating antitumor effects (Shi et al., 2022). Kim et al. found that MVs from Lactobacillus acidophilus and S. aureus exhibited anti-tumor effects. The lack of an increase in tumor size in mice treated with these MVs may be related to the induction of interferon (INF)-γ production by MV surface proteins (Kim et al., 2017). The development of Gram-positive EVs, primarily comprising probiotics, is emerging as a promising avenue in anticancer therapy. Compared to Gram-negative bacteria, probiotics aid in the restoration of healthy microbiota, ameliorating complications arising from radiation and chemotherapy while enhancing the efficacy of cancer treatment (Li et al., 2021).
5.6 Other clinical disease treatment
MVs aid in disease treatment in addition to assisting with diagnosis, contributing to the establishment of a normal ecological environment in the intestinal tract, improving inflammatory responses, and exhibiting anti-tumor effects. The expression of brain-derived neurotrophic factor (BDNF) in the hippocampus increased in depressed mice treated with L. plantarum MVs. Depression-like behaviors were alleviated, implying that L. plantarum MVs may have an antidepressant effect on neuronal cells (Choi et al., 2019). In both in vivo and in vitro trials, Lactobacillus plantarum MVs treatment notably boosted microRNA-101a-3p expression. Elevating this miRNA distinctly reduced ischemic neuron apoptosis, offering a new approach for ischemic stroke treatment (Yang et al., 2022). MVs released from Lactobacillus isolated from the vagina of healthy women help protect human T cells against HIV-1 infection by inhibiting viral attachment and entry into target cells (Ñahui Palomino et al., 2019). In addition, Lactobacillus druckerii MVs and Streptococcus epidermidis MVs have potential therapeutic value for dermatological issues such as hyperplastic scars and psoriasis, respectively (Gómez-Chávez et al., 2021; Han et al., 2023).
6 Summary and outlook
Gram-positive bacteria are a vital class of pathogens that cause infections in humans. With the continuous improvement of bacterial EV extraction technology, many studies have demonstrated that Gram-positive bacteria can produce MVs and that these MVs are closely related to the virulence, immune ability, and pathogenic factors of bacteria. MVs perform many functions, including assisting bacteria in evading host killing, eliciting immune responses, and mediating drug-resistance gene transfer. However, these findings are in the primary research phase, and we lack comprehensive information on the molecular mechanisms of MV production, immune response, and gene transfer. Furthermore, several phenomena and problems remain unsolved.
MVs resist the survival mechanism of external environmental stress, such as antibiotic killing. These findings suggest a novel therapeutic approach to counter the threat of antibiotic resistance in bacteria. In addition, the immune response triggered by MVs highlights their potential as candidates for vaccine development. However, there is no clear experimental evidence regarding the safety of MVs or the development of sequelae when they are injected into humans as a vaccine. These issues require further exploration to expand the application of MVs.
Disparate attention has been paid to the deleterious effects of MVs from clinically pathogenic Gram-positive bacteria while ignoring the beneficial effects of MVs on the human body. Some probiotics, specifically Gram-positive bacteria, exhibit important roles in anti-allergy, anti-inflammatory, and disease suppression responses; these characteristics can be utilized to apply probiotic MVs as oral preparations or pharmaceutical supplements to prevent or treat diseases. Moreover, investigating the role of intestinal probiotic MVs in immunomodulation and signaling will offer a new direction in research of the microbe-gut-brain axis. Therefore, further mechanistic research on MVs is essential, and the roles of several Gram-positive bacteria remain to be explored.
Author contributions
YX: Supervision, Validation, Writing – original draft, Writing – review and editing. CX: Writing – review and editing. YL: Writing – review and editing. XQ: Writing – review and editing. JL: Supervision, Validation, Visualization, Writing – review and editing.
Funding
This review was supported by the Special Foundation for the National Science and Technology Basic Research Program of China (grant number 2019FY101200) and the “345 Talent Project” of Shengjing Hospital of China Medical University.
Conflict of interest
The authors declare that the research was conducted in the absence of any commercial or financial relationships that could be construed as a potential conflict of interest.
Publisher’s note
All claims expressed in this article are solely those of the authors and do not necessarily represent those of their affiliated organizations, or those of the publisher, the editors and the reviewers. Any product that may be evaluated in this article, or claim that may be made by its manufacturer, is not guaranteed or endorsed by the publisher.
References
Abe, K., Toyofuku, M., Nomura, N., Obana, N. (2021). Autolysis-mediated membrane vesicle formation in Bacillus subtilis. Environ. Microbiol. 23, 2632–2647. doi: 10.1111/1462-2920.15502
Al-Nedawi, K., Mian, M. F., Hossain, N., Karimi, K., Mao, Y., Forsythe, P., et al. (2014). Gut commensal microvesicles reproduce parent bacterial signals to host immune and enteric nervous systems. FASEB J. 29, 684–695. doi: 10.1096/fj.14-259721
Askarian, F., Lapek, J. D., Dongre, M., Tsai, C.-M., Kumaraswamy, M., Kousha, A., et al. (2018). Staphylococcus aureus membrane-derived vesicles promote bacterial virulence and confer protective immunity in murine infection models. Front. Microbiol. 9. doi: 10.3389/fmicb.2018.00262
Athman, J. J., Sande, O. J., Groft, S. G., Reba, S. M., Nagy, N., Wearsch, P. A., et al. (2017). Mycobacterium tuberculosisMembrane vesicles inhibit T cell activation. J.I 198, 2028–2037. doi: 10.4049/jimmunol.1601199
Aung, K. M., Sjöström, A. E., von Pawel-Rammingen, U., Riesbeck, K., Uhlin, B. E., Wai, S. N. (2016). Naturally Occurring IgG Antibodies Provide Innate Protection against Vibrio cholerae Bacteremia by Recognition of the Outer Membrane Protein U. J. Innate Immun. 8, 269–283. doi: 10.1159/000443646
Aytar Çelik, P., Derkuş, B., Erdoğan, K., Barut, D., Blaise Manga, E., Yıldırım, Y., et al. (2022). Bacterial membrane vesicle functions, laboratory methods, and applications. Biotechnol. Adv. 54, 107869. doi: 10.1016/j.bioteChadv.2021.107869
Bai, J., Kim, S. I., Ryu, S., Yoon, H. (2014). Identification and characterization of outer membrane vesicle-associated proteins in salmonella enterica serovar typhimurium. Infect. Immun. 82, 4001–4010. doi: 10.1128/IAI.01416-13
Bai, A. D., Lo, C. K., Komorowski, A. S., Suresh, M., Guo, K., Garg, A., et al. (2022). Staphylococcus aureus bacteremia mortality across country income groups: A secondary analysis of a systematic review. Int. J. Infect. Dis. 122, 405–411. doi: 10.1016/j.ijid.2022.06.026
Battista, M., Hoffmann, B., Bachelot, Y., Zimmermann, L., Teuber, L., Jost, A., et al. (2023). The role of pneumococcal extracellular vesicles on the pathophysiology of the kidney disease hemolytic uremic syndrome. mSphere 8, e00142–e00123. doi: 10.1128/msphere.00142-23
Behzadi, E., Mahmoodzadeh Hosseini, H., Imani Fooladi, A. A. (2017). The inhibitory impacts of Lactobacillus rhamnosus GG-derived extracellular vesicles on the growth of hepatic cancer cells. Microbial Pathogenesis 110, 1–6. doi: 10.1016/j.micpath.2017.06.016
Bhatnagar, S., Shinagawa, K., Castellino, F. J., Schorey, J. S. (2007). Exosomes released from macrophages infected with intracellular pathogens stimulate a proinflammatory response in vitro and in vivo. Blood 110, 3234–3244. doi: 10.1182/blood-2007-03-079152
Biagini, M., Garibaldi, M., Aprea, S., Pezzicoli, A., Doro, F., Becherelli, M., et al. (2015). The human pathogen streptococcus pyogenes releases lipoproteins as lipoprotein-rich membrane vesicles. Mol. Cell Proteomics 14, 2138–2149. doi: 10.1074/mcp.M114.045880
Bitto, N. J., Cheng, L., Johnston, E. L., Pathirana, R., Phan, T. K., Poon, I. K. H., et al. (2021). Staphylococcus aureus membrane vesicles contain immunostimulatory DNA, RNA and peptidoglycan that activate innate immune receptors and induce autophagy. J. Extracellular Vesicle 10, e12080. doi: 10.1002/jev2.12080
Bomberger, J. M., MacEachran, D. P., Coutermarsh, B. A., Ye, S., O’Toole, G. A., Stanton, B. A. (2009). Long-distance delivery of bacterial virulence factors by pseudomonas aeruginosa outer membrane vesicles. PloS Pathog. 5, e1000382. doi: 10.1371/journal.ppat.1000382
Briaud, P., Carroll, R. K. (2020). Extracellular vesicle biogenesis and functions in gram-positive bacteria. Infect. Immun. 88, e00433–e00420. doi: 10.1128/IAI.00433-20
Brinkmann, V., Reichard, U., Goosmann, C., Fauler, B., Uhlemann, Y., Weiss, D. S., et al. (2004). Neutrophil extracellular traps kill bacteria. Science 303, 1532–1535. doi: 10.1126/science.1092385
Brown, L., Kessler, A., Cabezas-Sanchez, P., Luque-Garcia, J. L., Casadevall, A. (2014). Extracellular vesicles produced by the gram-positive bacterium bacillus subtilis are disrupted by the lipopeptide surfactin. Mol. Microbiol. 93, 183–198. doi: 10.1111/mmi.12650
Brown, L., Wolf, J. M., Prados-Rosales, R., Casadevall, A. (2015). Through the wall: extracellular vesicles in Gram-positive bacteria, mycobacteria and fungi. Nat. Rev. Microbiol. 13, 620–630. doi: 10.1038/nrmicro3480
Chimalapati, S., Cohen, J. M., Camberlein, E., MacDonald, N., Durmort, C., Vernet, T., et al. (2012). Effects of Deletion of the Streptococcus pneumoniae Lipoprotein Diacylglyceryl Transferase Gene lgt on ABC Transporter Function and on Growth In Vivo. PloS One 7, e41393. doi: 10.1371/journal.pone.0041393
Choi, J., Kim, Y.-K., Han, P.-L. (2019). Extracellular vesicles derived from lactobacillus plantarum increase BDNF expression in cultured hippocampal neurons and produce antidepressant-like effects in mice. Exp. Neurobiol. 28, 158–171. doi: 10.5607/en.2019.28.2.158
Choi, J.-W., Kwon, T.-Y., Hong, S.-H., Lee, H.-J. (2016). Isolation and Characterization of a microRNA-size Secretable Small RNA in Streptococcus sanguinis. Cell Biochem. Biophys. 76, 293–301. doi: 10.1007/s12013-016-0770-5
Choi, C.-W., Park, E. C., Yun, S. H., Lee, S.-Y., Kim, S. I., Kim, G.-H. (2017). Potential usefulness of streptococcus pneumoniae extracellular membrane vesicles as antibacterial vaccines. J. Immunol. Res. 2017, 1–8. doi: 10.1155/2017/7931982
Codemo, M., Muschiol, S., Iovino, F., Nannapaneni, P., Plant, L., Wai, S. N., et al. (2018). Immunomodulatory effects of pneumococcal extracellular vesicles on cellular and humoral host defenses. mBio 9, e00559–e00518. doi: 10.1128/mBio.00559-18
Croatti, V., Parolin, C., Giordani, B., Foschi, C., Fedi, S., Vitali, B. (2022). Lactobacilli extracellular vesicles: potential postbiotics to support the vaginal microbiota homeostasis. Microb. Cell Fact 21, 237. doi: 10.1186/s12934-022-01963-6
Dell’Annunziata, F., Folliero, V., Giugliano, R., De Filippis, A., Santarcangelo, C., Izzo, V., et al. (2021). Gene transfer potential of outer membrane vesicles of gram-negative bacteria. IJMS 225985. doi: 10.3390/ijms22115985
Derbyshire, K. M., Gray, T. A. (2014). Distributive conjugal transfer: new insights into horizontal gene transfer and genetic exchange in mycobacteria. Microbiol. Spectr. 2, MGM2-0022–2013. doi: 10.1128/microbiolspec.MGM2-0022-2013
Derkus, B., Emregul, K. C., Emregul, E. (2017). A new approach in stem cell research-Exosomes: Their mechanism of action via cellular pathways. Cell Biol. Int. 41, 466–475. doi: 10.1002/cbin.10742
Diallo, I., Ho, J., Lambert, M., Benmoussa, A., Husseini, Z., Lalaouna, D., et al. (2022). A tRNA-derived fragment present in E. coli OMVs regulates host cell gene expression and proliferation. PloS Pathog. 18, e1010827. doi: 10.1371/journal.ppat.1010827
Domínguez Rubio, A. P., Martínez, J. H., Martínez Casillas, D. C., Coluccio Leskow, F., Piuri, M., Pérez, O. E. (2017). Lactobacillus casei BL23 produces microvesicles carrying proteins that have been associated with its probiotic effect. Front. Microbiol. 8. doi: 10.3389/fmicb.2017.01783
Doré, E., Boilard, E. (2023). Bacterial extracellular vesicles and their interplay with the immune system. Pharmacol. Ther. 247, 108443. doi: 10.1016/j.pharmthera.2023.108443
Dorward, D. W., Garon, C. F. (1989). DNA-binding proteins in cells and membrane blebs of Neisseria gonorrhoeae. J. Bacteriol 171, 4196–4201. doi: 10.1128/jb.171.8.4196-4201.1989
Erdmann, S., Tschitschko, B., Zhong, L., Raftery, M. J., Cavicchioli, R. (2017). A plasmid from an Antarctic haloarchaeon uses specialized membrane vesicles to disseminate and infect plasmid-free cells. Nat. Microbiol. 2, 1446–1455. doi: 10.1038/s41564-017-0009-2
Ferreira, D., Seca, A. M. L., C.G.A., D., Silva, A. M. S. (2016). Targeting human pathogenic bacteria by siderophores: A proteomics review. J. Proteomics 145, 153–166. doi: 10.1016/j.jprot.2016.04.006
Foster, T. J. (2017). Antibiotic resistance in Staphylococcus aureus. Current status and future prospects. FEMS Microbiol. Rev. 41, 430–449. doi: 10.1093/femsre/fux007
Frantz, R., Teubner, L., Schultze, T., La Pietra, L., Müller, C., Gwozdzinski, K., et al. (2019). The secRNome of Listeria monocytogenes Harbors Small Noncoding RNAs That Are Potent Inducers of Beta Interferon. mBio 10, e01223–e01219. doi: 10.1128/mBio.01223-19
García-Solache, M., Rice, L. B. (2019). The enterococcus: a model of adaptability to its environment. Clin. Microbiol. Rev. 32, e00058–e00018. doi: 10.1128/CMR.00058-18
Gómez-Chávez, F., Cedillo-Peláez, C., Zapi-Colín, L. A., Gutiérrez-González, G., Martínez-Torres, I., Peralta, H., et al. (2021). The extracellular vesicles from the commensal staphylococcus epidermidis ATCC12228 strain regulate skin inflammation in the imiquimod-induced psoriasis murine model. IJMS 22, 13029. doi: 10.3390/ijms222313029
Haas, B., Grenier, D. (2015). Isolation, characterization and biological properties of membrane vesicles produced by the swine pathogen streptococcus suis. PloS One 10, e0130528. doi: 10.1371/journal.pone.0130528
Han, E., Choi, S., Lee, Y., Park, J., Hong, S., Lee, H. (2019). Extracellular RNAs in periodontopathogenic outer membrane vesicles promote TNF-α production in human macrophages and cross the blood-brain barrier in mice. FASEB J. 33, 13412–13422. doi: 10.1096/fj.201901575R
Han, F., Wang, K., Shen, K., Wang, J., Han, S., Hu, D., et al. (2023). Extracellular vesicles from Lactobacillus druckerii inhibit hypertrophic scar fibrosis. J. Nanobiotechnol 21, 113. doi: 10.1186/s12951-023-01861-y
Hu, F., Guo, Y., Zhu, D., Wang, F., Jiang, X., Xu, Y., et al. (2022). CHINET surveillance of antimicrobial resistance among the bacterial isolates in 2021. Chin. J. Infect. Chemother. 22, 521–530. doi: 10.16718/j.1009-7708.2022.05.001
Huang, W., Shu, C., Hua, L., Zhao, Y., Xie, H., Qi, J., et al. (2020). Modified bacterial outer membrane vesicles induce autoantibodies for tumor therapy. Acta Biomaterialia 108, 300–312. doi: 10.1016/j.actbio.2020.03.030
Jeon, H., Oh, M. H., Jun, S. H., Kim, S. I., Choi, C. W., Kwon, H. I., et al. (2016). Variation among Staphylococcus aureus membrane vesicle proteomes affects cytotoxicity of host cells. Microbial Pathogenesis 93, 185–193. doi: 10.1016/j.micpath.2016.02.014
Jhelum, H., Sori, H., Sehgal, D. (2018). A novel extracellular vesicle-associated endodeoxyribonuclease helps Streptococcus pneumoniae evade neutrophil extracellular traps and is required for full virulence. Sci. Rep. 8, 7985. doi: 10.1038/s41598-018-25865-z
Jiang, Y., Kong, Q., Roland, K. L., Curtiss, R. (2014). Membrane vesicles of Clostridium perfringens type A strains induce innate and adaptive immunity. Int. J. Med. Microbiol. 304, 431–443. doi: 10.1016/j.ijmm.2014.02.006
Jun, S. H., Lee, J. H., Kim, S. I., Choi, C. W., Park, T. I., Jung, H. R., et al. (2017). Staphylococcus aureus-derived membrane vesicles exacerbate skin inflammation in atopic dermatitis. Clin. Exp. Allergy 47, 85–96. doi: 10.1111/cea.12851
Karthikeyan, R., Gayathri, P., Gunasekaran, P., Jagannadham, M. V., Rajendhran, J. (2019). Comprehensive proteomic analysis and pathogenic role of membrane vesicles of Listeria monocytogenes serotype 4b reveals proteins associated with virulence and their possible interaction with host. Int. J. Med. Microbiol. 309, 199–212. doi: 10.1016/j.ijmm.2019.03.008
Karthikeyan, R., Gayathri, P., Gunasekaran, P., Jagannadham, M. V., Rajendhran, J. (2020). Functional analysis of membrane vesicles of Listeria monocytogenes suggests a possible role in virulence and physiological stress response. Microbial Pathogenesis 142, 104076. doi: 10.1016/j.micpath.2020.104076
Kengmo Tchoupa, A., Peschel, A. (2020). Staphylococcus aureus releases proinflammatory membrane vesicles to resist antimicrobial fatty acids. mSphere 5, e00804–e00820. doi: 10.1128/mSphere.00804-20
Khandavilli, S., Homer, K. A., Yuste, J., Basavanna, S., Mitchell, T., Brown, J. S. (2008). Maturation of Streptococcus pneumoniae lipoproteins by a type II signal peptidase is required for ABC transporter function and full virulence. Mol. Microbiol. 67, 541–557. doi: 10.1111/j.1365-2958.2007.06065.x
Kim, J.-H., Jeun, E.-J., Hong, C.-P., Kim, S.-H., Jang, M. S., Lee, E.-J., et al. (2015). Extracellular vesicle–derived protein from Bifidobacterium longum alleviates food allergy through mast cell suppression. J. Allergy Clin. Immunol. 137, 507–516.e8. doi: 10.1016/j.jaci.2015.08.016
Kim, M. H., Kim, S. Y., Son, J. H., Kim, S. I., Lee, H., Kim, S., et al. (2019). Production of membrane vesicles by enterococcus faecium cultured with or without subinhibitory concentrations of antibiotics and their pathological effects on epithelial cells. Front. Cell. Infect. Microbiol. 9. doi: 10.3389/fcimb.2019.00295
Kim, W., Lee, E. J., Bae, I.-H., Myoung, K., Kim, S. T., Park, P. J., et al. (2020). Lactobacillus plantarum-derived extracellular vesicles induce anti-inflammatory M2 macrophage polarization in vitro. J. Extracell Vesicles 9, 1793514. doi: 10.1080/20013078.2020.1793514
Kim, O. Y., Park, H. T., Dinh, N. T. H., Choi, S. J., Lee, J., Kim, J. H., et al. (2017). Bacterial outer membrane vesicles suppress tumor by interferon-γ-mediated antitumor response. Nat. Commun. 8, 626. doi: 10.1038/s41467-017-00729-8
Kim, S. W., Seo, J.-S., Park, S. B., Lee, A. R., Lee, J. S., Jung, J. W., et al. (2020). Significant increase in the secretion of extracellular vesicles and antibiotics resistance from methicillin-resistant Staphylococcus aureus induced by ampicillin stress. Sci. Rep. 10, 21066. doi: 10.1038/s41598-020-78121-8
Klieve, A. V., Yokoyama, M. T., Forster, R. J., Ouwerkerk, D., Bain, P. A., Mawhinney, E. L. (2005). Naturally occurring DNA transfer system associated with membrane vesicles in cellulolytic ruminococcus spp. of ruminal origin. Appl. Environ. Microbiol. 71, 4248–4253. doi: 10.1128/AEM.71.8.4248-4253.2005
Kopparapu, P. K., Deshmukh, M., Hu, Z., Mohammad, M., Maugeri, M., Götz, F., et al. (2021). Lipoproteins are responsible for the pro-inflammatory property of staphylococcus aureus extracellular vesicles. Int. J. Mol. Sci. 22, 7099. doi: 10.3390/ijms22137099
Kulp, A., Kuehn, M. J. (2010). Biological functions and biogenesis of secreted bacterial outer membrane vesicles. Annu. Rev. Microbiol. 64, 163–184. doi: 10.1146/annurev.micro.091208.073413
Lakhundi, S., Zhang, K. (2018). Methicillin-resistant staphylococcus aureus: molecular characterization, evolution, and epidemiology. Clin. Microbiol. Rev. 31, e00020–e00018. doi: 10.1128/CMR.00020-18
Langlete, P., Krabberød, A. K., Winther-Larsen, H. C. (2019). Vesicles From Vibrio cholerae Contain AT-Rich DNA and Shorter mRNAs That Do Not Correlate With Their Protein Products. Front. Microbiol. 10. doi: 10.3389/fmicb.2019.02708
Lee, E.-Y., Choi, D.-Y., Kim, D.-K., Kim, J.-W., Park, J. O., Kim, S., et al. (2009). Gram-positive bacteria produce membrane vesicles: Proteomics-based characterization of Staphylococcus aureus-derived membrane vesicles. PROTEOMICS 9, 5425–5436. doi: 10.1002/pmic.200900338
Lee, T., Jun, S. H., Choi, C. W., Kim, S. I., Lee, J. C., Shin, J. H. (2017). Salt stress affects global protein expression profiles of extracellular membrane-derived vesicles of Listeria monocytogenes. Microbial Pathogenesis 115, 272–279. doi: 10.1016/j.micpath.2017.12.071
Lee, J., Kim, S.-H., Choi, D.-S., Lee, J. S., Kim, D.-K., Go, G., et al. (2015). Proteomic analysis of extracellular vesicles derived from Mycobacterium tuberculosis. PROTEOMICS 15, 3331–3337. doi: 10.1002/pmic.201500037
Lee, A. R., Park, S. B., Kim, S. W., Jung, J. W., Chun, J. H., Kim, J., et al. (2022). Membrane vesicles from antibiotic-resistant Staphylococcus aureus transfer antibiotic-resistance to antibiotic-susceptible Escherichia coli. J. Appl. Microbiol. 132, 2746–2759. doi: 10.1111/jam.15449
Lee, Y., Park, J.-Y., Lee, E.-H., Yang, J., Jeong, B.-R., Kim, Y.-K., et al. (2017). Rapid assessment of microbiota changes in individuals with autism spectrum disorder using bacteria-derived membrane vesicles in urine. Exp. Neurobiol. 26, 307–317. doi: 10.5607/en.2017.26.5.307
Lessa, F. C., Mu, Y., Bamberg, W. M., Beldavs, Z. G., Dumyati, G. K., Dunn, J. R., et al. (2015). Burden ofClostridium difficileInfection in the United States. N Engl. J. Med. 372, 825–834. doi: 10.1056/NEJMoa1408913
Li, W., Deng, X., Chen, T. (2021). Exploring the modulatory effects of gut microbiota in anti-cancer therapy. Front. Oncol. 11. doi: 10.3389/fonc.2021.644454
Li, P., Luo, W., Xiang, T.-X., Jiang, Y., Liu, P., Wei, D.-D., et al. (2022). Horizontal gene transfer via OMVs co-carrying virulence and antimicrobial-resistant genes is a novel way for the dissemination of carbapenem-resistant hypervirulent Klebsiella pneumoniae. Front. Microbiol. 13. doi: 10.3389/fmicb.2022.945972
Liang, L., Yang, C., Liu, L., Mai, G., Li, H., Wu, L., et al. (2022). Commensal bacteria-derived extracellular vesicles suppress ulcerative colitis through regulating the macrophages polarization and remodeling the gut microbiota. Microb. Cell Fact 21, 88. doi: 10.1186/s12934-022-01812-6
López, P., González-Rodríguez, I., Sánchez, B., Gueimonde, M., Margolles, A., Suárez, A. (2011). Treg-inducing membrane vesicles from Bifidobacterium bifidum LMG13195 as potential adjuvants in immunotherapy. Vaccine 30, 825–829. doi: 10.1016/j.vaccine.2011.11.115
Mehanny, M., Koch, M., Lehr, C.-M., Fuhrmann, G. (2020). Streptococcal extracellular membrane vesicles are rapidly internalized by immune cells and alter their cytokine release. Front. Immunol. 11. doi: 10.3389/fimmu.2020.00080
Mehanny, M., Kroniger, T., Koch, M., Hoppstädter, J., Becher, D., Kiemer, A. K., et al. (2022). Yields and immunomodulatory effects of pneumococcal membrane vesicles differ with the bacterial growth phase. Advanced Healthcare Materials 11, 2101151. doi: 10.1002/adhm.202101151
Mullaney, E., Brown, P. A., Smith, S. M., Botting, C. H., Yamaoka, Y. Y., Terres, A. M., et al. (2009). Proteomic and functional characterization of the outer membrane vesicles from the gastric pathogen Helicobacter pylori. Prot. Clin. Appl. 3, 785–796. doi: 10.1002/prca.200800192
Nabavi-Rad, A., Jamshidizadeh, S., Azizi, M., Yadegar, A., Robinson, K., Monaghan, T. M., et al. (2023). The synergistic effect of Levilactobacillus brevis IBRC-M10790 and vitamin D3 on Helicobacter pylori-induced inflammation. Front. Cell. Infect. Microbiol. 13. doi: 10.3389/fcimb.2023.1171469
Ñahui Palomino, R. A., Vanpouille, C., Laghi, L., Parolin, C., Melikov, K., Backlund, P., et al. (2019). Extracellular vesicles from symbiotic vaginal lactobacilli inhibit HIV-1 infection of human tissues. Nat. Commun. 10, 5656. doi: 10.1038/s41467-019-13468-9
Olaya-Abril, A., Prados-Rosales, R., McConnell, M. J., Martín-Peña, R., González-Reyes, J. A., Jiménez-Munguía, I., et al. (2014). Characterization of protective extracellular membrane-derived vesicles produced by Streptococcus pneumoniae. J. Proteomics 106, 46–60. doi: 10.1016/j.jprot.2014.04.023
Oogai, Y., Matsuo, M., Hashimoto, M., Kato, F., Sugai, M., Komatsuzawa, H. (2011). Expression of virulence factors by staphylococcus aureus grown in serum. Appl. Environ. Microbiol. 77, 8097–8105. doi: 10.1128/AEM.05316-11
Orench-Rivera, N., Kuehn, M. J. (2016). Environmentally controlled bacterial vesicle-mediated export. Cell. Microbiol. 18, 1525–1536. doi: 10.1111/cmi.12676
Prados-Rosales, R., Baena, A., Martinez, L. R., Luque-Garcia, J., Kalscheuer, R., Veeraraghavan, U., et al. (2011). Mycobacteria release active membrane vesicles that modulate immune responses in a TLR2-dependent manner in mice. J. Clin. Invest. 121, 1471–1483. doi: 10.1172/JCI44261
Prados-Rosales, R., Carreño, L. J., Batista-Gonzalez, A., Baena, A., Venkataswamy, M. M., Xu, J., et al. (2014). Mycobacterial membrane vesicles administered systemically in mice induce a protective immune response to surface compartments of mycobacterium tuberculosis. mBio 5, e01921–e01914. doi: 10.1128/mBio.01921-14
Quek, C., Bellingham, S. A., Jung, C.-H., Scicluna, B. J., Shambrook, M. C., Sharples, R. A., et al. (2016). Defining the purity of exosomes required for diagnostic profiling of small RNA suitable for biomarker discovery. RNA Biol. 14, 245–258. doi: 10.1080/15476286.2016.1270005
Resch, U., Tsatsaronis, J. A., Le Rhun, A., Stübiger, G., Rohde, M., Kasvandik, S., et al. (2016). A two-component regulatory system impacts extracellular membrane-derived vesicle production in group A streptococcus. mBio 7, e00207–e00216. doi: 10.1128/mBio.00207-16
Rivera, J., Cordero, R. J. B., Nakouzi, A. S., Frases, S., Nicola, A., Casadevall, A. (2010). Bacillus anthracis produces membrane-derived vesicles containing biologically active toxins. Proc. Natl. Acad. Sci. 107, 19002–19007. doi: 10.1073/pnas.1008843107
Roier, S., Zingl, F. G., Cakar, F., Durakovic, S., Kohl, P., Eichmann, T. O., et al. (2016). A novel mechanism for the biogenesis of outer membrane vesicles in Gram-negative bacteria. Nat. Commun. 7, 10515. doi: 10.1038/ncomms10515
Rumbo, C., Fernández-Moreira, E., Merino, M., Poza, M., Mendez, J. A., Soares, N. C., et al. (2011). Horizontal Transfer of the OXA-24 Carbapenemase Gene via Outer Membrane Vesicles: a New Mechanism of Dissemination of Carbapenem Resistance Genes in Acinetobacter baumannii. Antimicrob. Agents Chemother. 55, 3084–3090. doi: 10.1128/AAC.00929-10
Sartorio, M. G., Pardue, E. J., Feldman, M. F., Haurat, M. F. (2021). Bacterial outer membrane vesicles: from discovery to applications. Annu. Rev. Microbiol. 75, 609–630. doi: 10.1146/annurev-micro-052821-031444
Schirmer, S., Rauh, L., Alebouyeh, S., Delgado-Velandia, M., Salgueiro, V. C., Lerma, L., et al. (2022). Immunogenicity of mycobacterial extracellular vesicles isolated from host-related conditions informs about tuberculosis disease status. Front. Microbiol. 13. doi: 10.3389/fmicb.2022.907296
Schrempf, H., Koebsch, I., Walter, S., Engelhardt, H., Meschke, H. (2011). Extracellular Streptomyces vesicles: amphorae for survival and defence. Microb. Biotechnol. 4, 286–299. doi: 10.1111/j.1751-7915.2011.00251.x
Shi, Y., Meng, L., Zhang, C., Zhang, F., Fang, Y. (2022). Extracellular vesicles of Lacticaseibacillus paracasei PC-H1 induce colorectal cancer cells apoptosis via PDK1/AKT/Bcl-2 signaling pathway. Microbiol. Res. 255, 126921. doi: 10.1016/j.micres.2021.126921
Sim, S., Lee, D.-H., Kim, K., Park, H. J., Kim, Y.-K., Choi, Y., et al. (2023). Micrococcus luteus-derived extracellular vesicles attenuate neutrophilic asthma by regulating miRNAs in airway epithelial cells. Exp. Mol. Med. 55, 196–204. doi: 10.1038/s12276-022-00910-0
Soleimani, N., Javadi, M. M. (2022). Future prospects of bacteria-mediated cancer therapies: Affliction or opportunity? Microbial Pathogenesis 172, 105795. doi: 10.1016/j.micpath.2022.105795
Soucy, S. M., Huang, J., Gogarten, J. P. (2015). Horizontal gene transfer: building the web of life. Nat. Rev. Genet. 16, 472–482. doi: 10.1038/nrg3962
Stoll, H., Dengjel, J., Nerz, C., Götz, F. (2005). Staphylococcus aureus deficient in lipidation of prelipoproteins is attenuated in growth and immune activation. Infect. Immun. 73, 2411–2423. doi: 10.1128/IAI.73.4.2411-2423.2005
Surve, M. V., Anil, A., Kamath, K. G., Bhutda, S., Sthanam, L. K., Pradhan, A., et al. (2016). Membrane vesicles of group B streptococcus disrupt feto-maternal barrier leading to preterm birth. PloS Pathog. 12, e1005816. doi: 10.1371/journal.ppat.1005816
Tomlinson, G., Chimalapati, S., Pollard, T., Lapp, T., Cohen, J., Camberlein, E., et al. (2014). TLR-mediated inflammatory responses to streptococcus pneumoniae are highly dependent on surface expression of bacterial lipoproteins. J. Immunol. 193, 3736–3745. doi: 10.4049/jimmunol.1401413
Toyofuku, M., Nomura, N., Eberl, L. (2019). Types and origins of bacterial membrane vesicles. Nat. Rev. Microbiol. 17, 13–24. doi: 10.1038/s41579-018-0112-2
Troeger, C., Blacker, B., Khalil, I. A., Rao, P. C., Cao, J., Zimsen, S. R. M., et al. (2018). Estimates of the global, regional, and national morbidity, mortality, and aetiologies of lower respiratory infections in 195 countries 1990–2016: a systematic analysis for the Global Burden of Disease Study 2016. Lancet Infect. Dis. 18, 1191–1210. doi: 10.1016/S1473-3099(18)30310-4
Tzipilevich, E., Habusha, M., Ben-Yehuda, S. (2016). Acquisition of phage sensitivity by bacteria through exchange of phage receptors. Cell 168, 186–199.e12. doi: 10.1016/j.cell.2016.12.003
Vdovikova, S., Luhr, M., Szalai, P., Nygård Skalman, L., Francis, M. K., Lundmark, R., et al. (2017). A novel role of listeria monocytogenes membrane vesicles in inhibition of autophagy and cell death. Front. Cell. Infect. Microbiol. 7. doi: 10.3389/fcimb.2017.00154
Wagner, T., Joshi, B., Janice, J., Askarian, F., Škalko-Basnet, N., Hagestad, O. C., et al. (2018). Enterococcus faecium produces membrane vesicles containing virulence factors and antimicrobial resistance related proteins. J. Proteomics 187, 28–38. doi: 10.1016/j.jprot.2018.05.017
Wang, X., Eagen, W. J., Lee, J. C. (2020). Orchestration of human macrophage NLRP3 inflammasome activation by Staphylococcus aureus extracellular vesicles. Proc. Natl. Acad. Sci. U.S.A. 117, 3174–3184. doi: 10.1073/pnas.1915829117
Wang, X., Koffi, P. F., English, O. F., Lee, J. C. (2021). Staphylococcus aureus extracellular vesicles: A story of toxicity and the stress of 2020. Toxins 13, 75. doi: 10.3390/toxins13020075
Wang, X., Thompson, C. D., Weidenmaier, C., Lee, J. C. (2018). Release of Staphylococcus aureus extracellular vesicles and their application as a vaccine platform. Nat. Commun. 9, 1379. doi: 10.1038/s41467-018-03847-z
Watkins, E. R., Kalizang’Oma, A., Gori, A., Gupta, S., Heyderman, R. S. (2022). Factors affecting antimicrobial resistance in Streptococcus pneumoniae following vaccination introduction. Trends Microbiol. 30 (12), 1135–1145. doi: 10.1016/j.tim.2022.06.001
Weiser, J. N., Ferreira, D. M., Paton, J. C. (2018). Streptococcus pneumoniae: transmission, colonization and invasion. Nat. Rev. Microbiol. 16, 355–367. doi: 10.1038/s41579-018-0001-8
White, D. W., Elliott, S. R., Odean, E., Bemis, L. T., Tischler, A. D. (2018). Mycobacterium tuberculosis pst/senX3-regX3 regulates membrane vesicle production independently of ESX-5 activity. mBio 9, e00778–e00718. doi: 10.1128/mBio.00778-18
Work, E., Knox, K. W., Vesk, M. (1966). The chemistry and electron microscopy of an extracellular lipopolysaccharide from Escherichia coli. Ann. N Y Acad. Sci. 133, 438–449. doi: 10.1111/j.1749-6632.1966.tb52382.x
Yang, Z., Gao, Z., Yang, Z., Zhang, Y., Chen, H., Yang, X., et al. (2022). Lactobacillus plantarum-derived extracellular vesicles protect against ischemic brain injury via the microRNA-101a-3p/c-Fos/TGF-β axis. Pharmacol. Res. 182, 106332. doi: 10.1016/j.phrs.2022.106332
Yaron, S., Kolling, G. L., Simon, L., Matthews, K. R. (2000). Vesicle-mediated transfer of virulence genes from escherichia coli O157:H7 to other enteric bacteria. Appl. Environ. Microbiol. 66, 4414–4420. doi: 10.1128/AEM.66.10.4414-4420.2000
Yerneni, S. S., Werner, S., Azambuja, J. H., Ludwig, N., Eutsey, R., Aggarwal, S. D., et al. (2021). Pneumococcal extracellular vesicles modulate host immunity. mBio 12, e01657–e01621. doi: 10.1128/mBio.01657-21
Yu, Y., Wang, X., Fan, G.-C. (2018). Versatile effects of bacterium-released membrane vesicles on mammalian cells and infectious/inflammatory diseases. Acta Pharmacol. Sin. 39, 514–533. doi: 10.1038/aps.2017.82
Keywords: Gram-positive bacteria, membrane vesicles, function, application, virulence
Citation: Xu Y, Xie C, Liu Y, Qin X and Liu J (2023) An update on our understanding of Gram-positive bacterial membrane vesicles: discovery, functions, and applications. Front. Cell. Infect. Microbiol. 13:1273813. doi: 10.3389/fcimb.2023.1273813
Received: 07 August 2023; Accepted: 19 September 2023;
Published: 04 October 2023.
Edited by:
Sheryl S. Justice, The Ohio State University, United StatesReviewed by:
Marcelo Brocchi, State University of Campinas, BrazilFarzam Vaziri, University of California, Davis, United States
Copyright © 2023 Xu, Xie, Liu, Qin and Liu. This is an open-access article distributed under the terms of the Creative Commons Attribution License (CC BY). The use, distribution or reproduction in other forums is permitted, provided the original author(s) and the copyright owner(s) are credited and that the original publication in this journal is cited, in accordance with accepted academic practice. No use, distribution or reproduction is permitted which does not comply with these terms.
*Correspondence: Xiaosong Qin, cWlueHNAc2otaG9zcGl0YWwub3Jn; Jianhua Liu, bGl1amhAc2otaG9zcGl0YWwub3Jn