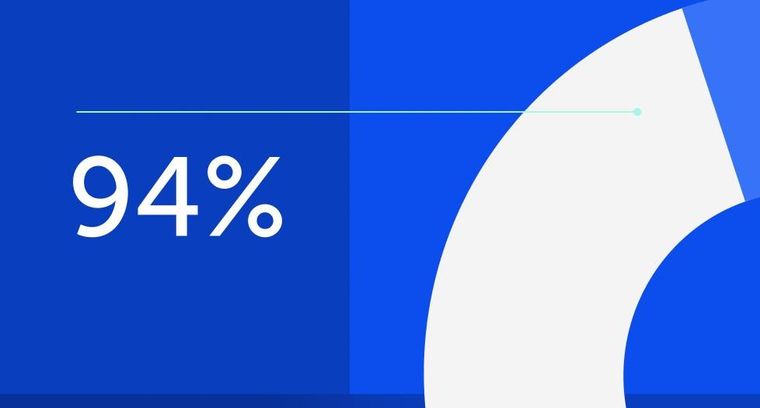
94% of researchers rate our articles as excellent or good
Learn more about the work of our research integrity team to safeguard the quality of each article we publish.
Find out more
ORIGINAL RESEARCH article
Front. Cell. Infect. Microbiol., 27 November 2023
Sec. Bacteria and Host
Volume 13 - 2023 | https://doi.org/10.3389/fcimb.2023.1257433
Introduction: Bacterial persistence is considered one of the main causal factors for regenerative endodontic treatment (RET) failure in immature permanent teeth. This interference is claimed to be caused by the interaction of bacteria that reside in the root canal with the stem cells that are one of the essentials for RET. The aim of the study was to investigate whether prolonged exposure of stem cells from the apical papilla (SCAP) to bacterial remnants of Fusobacterium nucleatum, Actinomyces gerensceriae, Slackia exigua, Enterococcus faecalis, Peptostreptococcaceae yurii, commonly found in infected traumatized root canals, and the probiotic bacteria Lactobacillus gasseri and Limosilactobacillus reuteri, can alter SCAP’s inflammatory response and mineralization potential.
Methods: To assess the effect of bacterial remnants on SCAP, we used UV-C–inactivated bacteria (as cell wall-associated virulence factors) and bacterial DNA. Histochemical staining using Osteoimage Mineralization Assay and Alizarin Red analysis was performed to study SCAP mineralization, while inflammatory and osteo/odontogenic-related responses of SCAPs were assessed with Multiplex ELISA.
Results: We showed that mineralization promotion was greater with UV C–inactivated bacteria compared to bacterial DNA. Immunofluorescence analysis detected that the early mineralization marker alkaline phosphatase (ALP) was increased by the level of E. coli lipopolysaccharide (LPS) positive control in the case of UV-C–inactivated bacteria; meanwhile, DNA treatment decreased the level of ALP compared to the positive control. SCAP’s secretome assessed with Multiplex ELISA showed the upregulation of pro-inflammatory factors IL-6, IL-8, GM-CSF, IL-1b, neurotrophic factor BDNF, and angiogenic factor VEGF, induced by UV-C–killed bacteria.
Discussion: The results suggest that long term stimulation (for 21 days) of SCAP with UV-C–inactivated bacteria stimulate their mineralization and inflammatory response, while DNA influence has no such effect, which opens up new ideas about the nature of RET failure.
Violations of the integrity of the tooth by caries or traumatic dental injuries (TDI) increases the risk of developing pulp tissue inflammation, pulp necrosis, and periapical bone destruction (Fouad, 2019). In this regard, polymicrobial oral microflora can invade normally sterile tissues like dental pulp and cause severe tooth damage. Indeed, in young children with immature teeth, infection will lead to the death of odontoblast cells and consequent interruption of root maturation (Hecova et al., 2010). Regenerative endodontic treatment (RET), a biological-based procedure, has shown promising clinical results evidencing root development (Wikström et al., 2022) and pulp tissue regeneration (Xuan et al., 2018). Although promising, the successful outcome of RET has been jeopardized by bacterial persistence in the root canal, affecting the viability, proliferation, immune response, and differentiation potential of stem cells from the apical papilla (SCAP) (Petridis et al., 2018a).
The microbiota of infected root canals housed in closed environments is comprised of bacteria that belong to the phyla Bacillota, Bacteroidota, Fusobacteriota, Actinomycetota, and Pseudomonadota (Sakamoto et al., 2006; Siqueira et al., 2011; Manoharan et al., 2020) with a high prevalence of anaerobic species of the genus Bacteroides, Corynebacterium, Peptostreptococcus, and Fusobacterium (Bergenholtz, 1974; Sundqvist and Figdor, 2003). Noteworthy, residual bacterial biofilms and/or their components can persist in dentine tubules even after RET disinfection protocols and could potentially influence RET outcomes (Verma et al., 2017). Certainly, regardless of bacteria’s pathogenicity to the host, all bacteria produce microbe-associated molecular patterns (MAMPs) or pathogen-associated molecular patterns (PAMPs) that are recognizable to the host cell pattern-recognition receptors (PRRs) (Zhong et al., 2017). The influence of bacteria on host cells is mediated by bacterial antigens and structural components (modulins), as well as lipopolysaccharides (LPS or endotoxins), peptidoglycans, lipoteichoic acids (LTA), fimbriae, flagella, outer membrane proteins, and exopolysaccharides, which altogether comprise MAMPs and determine bacterial virulence (J. Siqueira, 2019). It was shown that LPS of gram-negative bacteria, LTA of gram-positive, and bacterial lipoproteins can stimulate mesenchymal stem cells inflammatory response, and reduce their osteogenic potential (Tom-Kun Yamagishi et al., 2011; Morsczeck et al., 2012; Kato et al., 2014; Nguyen and Götz, 2016; Yu et al., 2019; Lei et al., 2020; Yin et al., 2020; Casey et al., 2022).
Unlike most virulence factors, which are secreted by bacteria as metabolites or are components of their membranes or cell wall, bacterial DNA normally remains inside the bacterial cell. However, if bacteria undergo stress or lysis, bacterial DNA can be released into the extracellular environment and stimulate a cellular response (Nielsen et al., 2007). Bacterial DNA, or rather the specific sequence prominent in bacterial DNA—unmethylated CpG (Cytosine-phosphate-guanine)—is capable of stimulating the TLR9 receptor resulting in the secretion of IL-12, IFN-α, TNF-α and IL-6 (Hemmi et al., 2003). Today, the advent of next generation sequencing techniques (NGS) allows the identification of bacteria that cannot be cultivated, and the accurate determination of which bacterial species are presented in the root canal and their proportions (Winand et al., 2019; Sharaf et al., 2022). Using NGS methods, it was shown that DNA of various bacterial species was found in infected, traumatized root canals (Manoharan et al., 2020). However, the question arises, were these bacteria alive and one of the reasons for the development of the inflammatory process and disease, or was only their bacterial DNA present?
Previously we showed that bacteria, which are mostly associated with traumatized infected root canals (Manoharan et al., 2020), mainly Fusobacterium nucleatum and Enterococcus faecalis, can upregulate inflammatory response and negatively regulate the expression of genes that are important for osteo- and dentinogenesis in human SCAPs after 24 hours of co-cultivation (Razghonova et al., 2022; Zymovets et al., 2022). Notwithstanding the results indicating the primary response of SCAP to bacterial stimuli after 24 hours, longer exposure time is necessary to see whether bacterial interaction with SCAP can alter their differentiation potential. However, the co-cultivation of SCAPs with live bacteria for 21 days (the time required to see differentiation of SCAP towards osteoblasts or odontoblasts) (Abuarqoub et al., 2015) cannot be implemented due to the rapid death of cells after more than 24 hours of co-cultivation with bacteria (Zymovets et al., 2022).
Given the inability of SCAP to remain viable for more than 24h under the bacterial influence, and the need for a long-term exposure of SCAP with bacteria simulating real infection conditions, various types of bacterial inactivations such as heat inactivation of various temperature points, freezing, and exposure to UV irradiation are used to inactivate bacteria while maintaining their surface structural components (Gao et al., 2009; Rose and O’Connell, 2009; Smelt and Brul, 2014; Tomb et al., 2018). In comparison to heat inactivation, bacterial UV irradiation affects mostly bacterial DNA by forming thymine dimers and partially blocking DNA synthesis, subsequently causing cell death (Coohill and Sagripanti, 2008), therefore was chosen as an inactivation method in our study.
Our study aimed to simulate the effects of direct bacterial influence on cells in a long-term co-culture by using UV-C–inactivated bacteria or their DNA. Through this approach, we were able to investigate, how in the absence of osteo/odontogenic medium, these UV-C–inactivated bacteria or their DNA, impact the mineralization and inflammatory response of SCAPs, which are factors closely linked to the outcome of RET.
In this study, five strains of opportunistic pathogenic bacteria (Fusobacterium nucleatum subsp. polymorphum R9(50)A, Actinomyces gerensceriae 46B, Slackia exigua N51A, Enterococcus faecalis Tand-4F, Peptostreptococcaceae [Eubacteria] yurii subsp. yurii & margaretiae 30G1), and two species of probiotic bacteria (Lactobacillus gasseri B6, Limosilactobacillus reuteri DSM 17938) were used. All opportunistic species used in this study were clinical isolates from the root-canal–traumatized teeth of patients of the Endodontic Department, Region of Västerbotten, Sweden (Reg. no. 2016/520-31). All isolates were identified by comparing 16S rRNA gene sequences to databases (HOMD), as previously described (Manoharan et al., 2020). L. gasseri was cultured from the saliva of breastfed infants and showed probiotic characteristics (Romani Vestman et al., 2013), while L. reuteri is considered a commercial probiotic that tends to balance oral microbiology (Romani Vestman et al., 2015). F. nucleatum and S. exigua were the most prevalent isolates from traumatized infected root canals, (Manoharan et al., 2020), while A. gerensceriae and E. faecalis were chosen for their role in root canal treatment failure (Siqueira and Rôças, 2008).
All strains were stored at −80°C. The opportunistic strains were plated on fastidious anaerobic agar (FAA) plates (Svenska LABFAB, ACU-7531A), supplemented with synthetic water-soluble analogues of vitamin K1 (MERCK, M5750) and Sterile Defibrinated House Blood (Håtunalab, Sweden). Lactobacillus strains were grown on MRS agar plates (Sigma Aldrich, 69964-500G). All strains were grown in an anaerobic atmosphere (5% CO2, 10% H2, 85% N2) at 37°C for 5–7 days. Cultivated bacteria were individually resuspended in the antibiotic-free cell culture media Minimum Essential Medium alpha (MEM α, GlutaMAX™ Supplement, no nucleosides, ThermoFisher, 32561-029) supplemented with 10% Fetal Bovine Serum (FBS; Sigma Aldrich, F7524). The optical density of each suspension was adjusted by a spectrophotometer (Ultrospec 2100 pro, Amersham Biosciences) at 600nm to OD= 1.0; after which, suspensions were aliquoted and exposed to different inactivation protocols.
Based on the experience in a previous study (Rakhimova et al., 2021) of bacterial overgrowth and subsequent cell death, UV-C irradiation was used, to ensure termination of bacterial growth with maximum preservation of bacterial cell integrity. Prior to irradiation by UV-light with the spectral range of 205–315nm, 10ml of bacterial suspensions were transferred to the cell culture plates (Sigma-Aldrich, P5981), plates were left open for 60 minutes at a 10cm distance from the portable UV lamp (Ninolab AB, 2007-29-900) in the laminar hood (Holten Laminar Air HB 2448).
The viability of bacteria after UV-C irradiation was determined by CFU determination by preparing a 10-fold serial dilution; three drops (10μl each) from each dilution were placed on appropriate agar plates and cultured at the appropriate conditions for one week.
The integrity of bacterial cells was confirmed by measuring the extracellular DNA concentration. Briefly, suspensions with inactivated bacteria were centrifuged at 16,000 × g for 10 minutes, and the DNA 260nm/280nm ratio was measured from the top fraction of the supernatant using NanoDrop ND-1000 (Thermo Fisher Scientific).
Since the DNA of the UV-C–killed bacteria used in this study lost their native structure and functionality, the native and non-damaged genomic DNA from corresponding bacterial species used in the study were purified from these species with Sigma Aldrich Kit (NA2120) according to the manufacturer’s protocol, extracted bacterial DNA were used for SCAP treatment. The concentration of purified DNA was measured by Nanodrop One (Thermo Scientific), aliquoted, and saved in a –20 °C freezer until the experiment.
SCAP isolated from three healthy donors was used in this study: donor I, donor II, and donor III as previously described (Kolar et al., 2017; Pettersson et al., 2017) Only cells within 5–8 passages were used in the experiment. SCAPs were taken out from the cryostocks and cultured on MEM-alpha medium enriched with 10% FBS and 1% Penicillin–Streptomycin solution (ThermoFisher, P0781) at 37°C in a 5% CO2 atmosphere.
SCAPs were harvested by trypsinization and counted by automated cell counter Countess-II (ThermoFisher, AMQAX1000) with 0.4% trypan blue solution (ThermoFisher, 15250061) in the Countess Cell Counting chamber sample slide (ThermoFisher, C10228). Then SCAPs were quantitatively seeded and cultured overnight to let the cells adhere to the plates. The next day cells were examined under the phase contrast microscope (Nikon TMS Inverted Phase Contrast Microscope), then the cell media was exchanged by cell culture media lacking the antibiotic mixture. Individual suspensions of inactivated bacteria were quantitatively applied to the cells at a to obtain a ratio of 100 bacteria per 1 cell (100 MOI-multiplicity of infection), to follow the same MOI that was determined as sub-lethal for SCAPs in case of live bacteria in the previous study (Rakhimova et al., 2021). As the positive control, SCAP were treated with LPS from E. coli (#L8274, Sigma-Aldrich, MO, USA), in dilution 1:1000. Suspensions of inactivated bacteria were prepared in advance (as described above) and stored in the freezer.
The inactivation of bacteria by UV-C irradiation was chosen because it is highly reliable and the most structure-preserving (except for the structure of DNA) method. The effectivity of ultraviolet (UV) light is based on its phenomenon of forming dimers in DNA, which lead to the accumulation of DNA damage that impede transcription and replication, subsequently causing bacterial cell death (Goosen and Moolenaar, 2008; Cutler and Zimmerman, 2011). For treatment of cells with the bacterial DNA, non-damaged genomic DNA, extracted from corresponding bacterial species were used in the study for SCAP treatment.
SCAPs from three different donors were taken out of the cryostocks and cultured as described above until reaching 90% confluency, after which cells were harvested with 0.05% trypsin-EDTA and seeded onto the cell culture plates at a concentration of 20,000 cells/ml and placed overnight in the CO2 incubator for cell adherence. Next day, the cell culture medium containing the non-adherent cells was exchanged with the fresh cell culture medium and UV-C–inactivated bacterial suspensions were applied individually to each of the three SCAPs to get the 100 MOI. In parallel, the DNA purified from corresponding bacterial species was applied to SCAPs in an amount equivalent to 100 MOI. SCAPs with UV-C–inactivated bacteria/DNA were cultured in an anaerobic atmosphere (10% CO2, 10% H2, 80% N2) at 37 ◦C for 21 days in the cell culture medium and subsequently medium (not treatment) were refreshed every second day.
On the twenty-first day of the culture period, cells were harvested with trypsin-EDTA. The number of viable cells in each variant of SCAP culture was determined by the automated cell counter—Countess-II in trypan blue dye exclusion assay. In brief, one volume of the cell suspension is mixed with an equal volume of trypan blue dye (ThermoFisher Scientific, 15250061) and then examined to determine whether cells take up or exclude dye. Viable cells will have a clear cytoplasm, whereas dead cells will have a blue cytoplasm. The number of viable and/or dead cells per unit volume is determined as a percentage of untreated control cells.
Histochemical staining was performed directly in culture well plates to check the changes in mineralization of SCAPs cultured in presence of UV-C-inactivated bacteria/DNA. Three different types of histochemistry staining were used: detection of hydroxyapatite (HA), Ca2+ deposits, and alkaline phosphatase detection in SCAPs.
Osteoimage Mineralization Assay (Lonza, PA-1503) is based on the specific binding of the fluorescent OsteoImage™ Staining Reagent to the HA portion of the bone-like nodules deposited by cells. This staining was performed following the manufacturer´s instruction, adapted for small-size specimens, and quantitatively assessed by the Fluorescent plate reader (Safire 2 with Magellan Tracker v7.0 software, Tecan, Switzerland) at the excitation/emission wavelengths of 492/520nm respectively.
Ca2+ deposits were assessed by pre-filtered 1% Alizarin Red (Thermo Fisher Scientific, Gothenburg, Sweden) solution for 3 minutes to previously washed (PBS), fixed (60% isopropanol) and rehydrated (deionized water) cell layers. The dye excess was washed with distilled water before cells were investigated under the microscope (Evident Olympus Stand column SZX2-ILLTQ). Images of each SCAP’s culture variant were analyzed with the help of the Trainable Weka Segmentation Plugin in Fiji for the calculation of the percentage of area occupied by the Alizarin Red-positive stained cells.
Alkaline phosphatase (ALP) presence was visualized in SCAPs (preliminary fixed in 4% formalin) with ALP staining by using Naphthol AS-MX phosphate disodium salt (Sigma Aldrich, 855-25ML) and Fast Blue BB (Sigma Aldrich, F0500-25G), according to standard histochemical procedure. After staining, samples were examined under the microscope (Evident Olympus Stand column SZX2-ILLTQ), and analyzed with the help of the Trainable Weka Segmentation Plugin in Fiji. Data were expressed as the percentage of area occupied by ALP-positive stained cells.
Cells were seeded, grown on the coverslips, and treated as described above prior to application of UV-C-inactivated bacteria or DNA. After cells were cultured for the indicated time, the coverslips were washed 3 times in ice-cold PBS, fixed in a 4% formaldehyde buffer (4% formaldehyde solution, 1.4% methanol, water, buffered with sodium-di-phosphate and potassium-di-phosphate, at pH 7.4), permeabilized with 0.2% Triton X-100 and blocked in 10mM glycine (pH 7.4). Incubation with mouse anti-ALP monoclonal antibodies (Bio-Techne (R&D), MAB1448) was performed for 1 hour at room temperature and followed by incubation with anti-mouse Alexa Fluor 647-conjugated antibodies (Jackson Immuno Research, 715-136-150). To detect nuclei of the cells on the slides, the mounting medium 4’,6–Diamino-2-Phenylinsole, Dihydrochloride (DAPI) was used (Vector Laboratories, H-1500).
The slides were examined with a Zeiss LSM 710 confocal microscope equipped with a Zeiss AXIO 6. For analysis, each image was imported into ImageJ v.1.47 software (http://imagej.nih.gov/ij)—a public domain image processing program from the National Institutes of Health, Maryland, USA. The intensity of the fluorescence was assessed with the ImageJ program and expressed as a mean value per cell with standard deviation. An intensity threshold was set to discriminate the detected fluorescent signal from the background. Representative results are shown from at least three independent images.
After 21 days of cultivation of SCAPs with inactivated bacteria or SCAPs with DNA, as well as SCAP with commercial LPS from E. coli (#L8274, Sigma-Aldrich, MO, USA), the conditioned medium samples were taken from each variant SCAPs culture, aliquoted by 1ml into the cryotubes and kept in the –80°C freezer until investigation. The conditioned medium from three SCAP donors was pooled for each UV-C–inactivated bacteria treatment, while for the DNA treatment, only cell supernatants from two donors were pooled. The human brain-derived neurotrophic factor (BDNF), granulocyte-macrophage colony-stimulating factor (GM-CSF), Interleukin 6 (IL-6), Interleukin 8 (IL-8), Interleukin 17A (IL-17A), interleukin-1α (IL-1α), interleukin-1β (IL-1β), monocyte chemoattractant protein-1 (MCP-1), and vascular endothelial growth factor A (VEGF-A) concentrations were measured in the conditioned pooled media samples by using a commercially-customised U-PLEX human biomarkers (Meso Scale Diagnostics, K151ACL-1) multiplex assay. The BDNF, GM-CSF, and VEGF-A, were chosen for their important role in osteo/odontogenic cells differentiation and regeneration (Saito et al., 2011; Kolar et al., 2017; Liu et al., 2018)). The pro-inflammatory markers—IL-8, IL-6, MCP-1, and IL-17A were selected based on the results of previous studies, indicating the upregulation of these markers in SCAPs under bacterial influence (Razghonova et al., 2022; Zymovets et al., 2022) as well as IL-1a and IL-1β, as they are involved in innate pro-inflammatory response (Dinarello, 2018).
Cell index (CI) for real-time proliferation assessment, normalization, and slope calculations assessments were calculated automatically by the RTCA Software Package 1.2 of the RTCA system. Numerical data were expressed as a mean ± standard deviation. The normal distribution of data was confirmed with Shapiro-Wilk test. Statistical differences between the means for the different groups were evaluated with Prism 5.0 (GraphPad Software, La Jolla, CA, USA) using the Student’s t-test with a level of significance at p <0.05. One-way ANOVA with Bonferroni correction p values: * p <0.05, ** p 0.01, *** p <0.001 in the analysis of CI measurements.
The experiments were performed with three biological replicas and two technical replicas in each variant of treatment. The results are expressed as a mean ± standard deviation (SD). Graph Pad Prism 7.0 (GraphPad Software Inc. San Diego, USA) software package was used for the statistical analysis. The normal distribution of the data for Osteoimage Mineralization Assay, Alizarin Red analysis and Alkaline phosphatase detection was verified with a Shapiro-Wilk test. The normal distribution of data in Multiplex ELISA experiments was tested using the Kolmogorov-Smirnov test. The level of significance was established as p <0.05. Normally distributed data was analyzed by 2-way ANOVA, followed by the Dunnett’s Multiple comparison tests, to examine the difference between non-treated SCAPs and each variant of SCAP treatment. Datasets which did not pass the normality test were analysed using a Kruskal-Wallis test. Statistical differences with p < 0.05 were considered significant. The influence of the SCAPs donor on the variations in results of Osteoimage Assay and Alizarin Red analysis was assessed using 2-way ANOVA.
SCAPs were co-cultivated with UV-C–inactivated species of F. nucleatum, A. gerensceriae, Slackia exigua, Enterococcus faecalis, Peptostreptococcaceae yurii, Lactobacillus gasseri or Limosilactobacillus reuteri and their DNA for 21 days. It was shown that SCAPs viability was not significantly affected by UV-C–inactivated bacteria (Figure 1A). Interestingly, the highest numbers of viable cells were detected in cases of co-culture with UV-C–inactivated probiotic species: Lactobacillus gasseri and Limosilactobacillus reuteri. The viability of cultured SCAPs in presence of DNA from different bacterial species did not vary statistically significantly from non-treated controls (Figure 1B). The lowest numbers of viable cells were detected in the case of co-culture with DNA isolated from E. faecalis.
Figure 1 Viability of SCAPs after 3 weeks of co-culture with (A) UV-C–inactivated bacteria or with (B) bacterial DNA (Mean ± SD). The viability of cells was measured by trypan blue dye exclusion assay. UV-C–inactivated bacterial influence resulted in the viability variation on 15.42% (p =0.1541) caused by SCAP donor and for 32.92% (p = 0.3965) by treatment. Bacterial DNA treatment led to viability variation on 7.665% (p =0.4223) caused by SCAP donor and for 24.95% (p =0.6562) by treatment. Data analyzed by 2-way ANOVA. Dunnett’s multiple comparison did not reveal any statistically significant difference between non-treated cells and cells with each variant of treatment.
Differentiation of dental stem cells towards osteo- or odontoblasts is accompanied by deposition of calcium and hydroxyapatite (HA) (Volponi et al., 2015; Blair et al., 2017). Accordingly, SCAP co-cultured with different bacterial treatment type was analyzed with specific staining, determining HA and calcium levels. For this, a fluorescent reagent was used (Osteoimage Mineralization Assay) that specifically binds to HA (Figure 2A, B). The results showed that UV-C–inactivated bacteria increased the level of HA in SCAPs for all bacterial species, compared to the untreated control. However, only co-cultivation with UV-C–inactivated A. gerensceriae, S. exigua and P. yurii resulted in a statistically significant increase in HA content (Figure 2A). Accordingly, the bacterial DNA of almost all bacteria, except for L. reuteri and P. yurii, statistically significantly increased the content of HA in SCAP compared to the untreated control (Figure 2B). Nevertheless, it should be noted that, when comparing the influence of UV-C–inactivated bacteria with bacterial DNA, the content of HA in SCAP was several times lower for most bacteria in the latter case.
Figure 2 Mineralization of SCAPs co-cultured with UV-C–inactivated bacteria (A, C) or co-cultured with DNA (B, D) for 21 days (Mean ± SD). (A, B)—histochemical staining with Osteoimage (HA-specific staining); (C, D)—histochemical staining with Alizarin Red. The levels of HA and Ca2+ deposition are expressed via the control. Statistical significance is marked as: * p <0.05, ** p 0.01.
Alizarin Red staining of SCAPs detecting the deposits of Ca2+, did not reveal significant difference between variants of SCAP bacterial and DNA co-culture and their respective non-treated controls (Figures 2C, D). However, a concordant detection between HA and Alizarin red staining for UV-C–inactivated bacteria (Figures 2A, C) and bacterial DNA (Figures 2B, D) treatments is clearly evident. Overall, we can see that inactivated bacteria influence on SCAP led to the highest levels of calcium deposition for several bacterial species compared to bacterial DNA treatment.
To estimate whether the variations in the SCAPs donor influenced the level of HA or Ca2+ deposition in SCAPs after different treatments, the data were analyzed using 2-way ANOVA. For SCAPs co-cultured with UV-C–inactivated bacteria, the donor variations accounted for 45.77% (p**** <0.0001) in the changes of mineralization for HA-specific staining and 29.14% (p* =0.0146) for Ca2+ specific staining. The treatment itself accounted for 39.11% (p** =0.0044) in the changes of mineralization in case of HA-specific staining and 35.73% (pns =0.1223) in the case of Ca2+ specific staining. These finding suggests that both SCAPs donor and inactivated bacteria influence SCAPs HA and Ca2+ deposition.
In contrast, for SCAPs co-cultured with DNA, the donor variation showed a statistically non-significant contribution to the changes in mineralization, both for HA-specific staining (14.52% pns =0.0645) and Ca2+ specific staining (1.955% pns =0.6589). This indicates that DNA treatment is the primary and statistically significant source of alterations in SCAPs mineralization for HA-specific staining (55.19% p* =0.0189) and Ca2+ specific staining (66.21% p* =0.0112).
To check whether comparable numbers of cells were present in each variant of treatment and confirm the fluorescent measurements obtained by a Fluorescent ELISA reader, all samples were examined under the fluorescent microscope. Representative images are shown in Figure 3. Likewise, SCAPs treated by different methods and analyzed for Ca2+ with Alizarin Red staining are shown in Figure 4.
Figure 3 Hydroxyapatites deposits detected with green fluorescence reagent (200x magnification) with Osteoimage® (HA-specific staining), and 4′,6-diamidino-2-phenylindole (DAPI) for cells nuclei. Shown is a set of representative images: One SCAP donor co-cultured with UV-C–inactivated S. exigua or with DNA from S. exigua in comparison with non-treated cells.
Figure 4 Calcium deposits detected by Alizarin Red staining (200x magnification). Shown is a set of representative images of from one SCAP donor, co-cultured with the UV-C–killed S. exigua, E. faecalis, and L. reuteri bacteria versus or their corresponding DNA in comparison with non-treated control.
Alkaline phosphatase (ALP), which plays an important role in the osteo/odontogenic differentiation of stem cells, is also actively produced by cells to accelerate the detoxification of LPS (Tuin, 2007; Lei et al., 2015; Komazin et al., 2019). This is the reason why LPS treatment was used as a positive control for our experiments and non-treated SCAPs were used as a negative control (Figures 5A–C).
Figure 5 Level of ALP in SCAPs co-cultured (A) with UV-C–inactivated bacterial species or treated with; * Data for treatment of SCAPs with UV-C–inactivated A. gerensceriae is absent. (B) DNA from corresponding bacterial species – immunohistochemical staining images, scale bar 46 µm. ALP labeled with Alexa Fluor® 647 conjugates and SCAPs nuclei labeled with DAPI. (C) The fluorescent intensity that reflects the amount of detected ALP was estimated with the ImageJ program. The boxes for each variant of treatment indicate the spread of data from min to max; the line in each box indicates the median value; data compared by the unpaired t-test; statistically significant difference marked by *(<0.05), **(<0.01) or “ns”, in case a statistically significant difference between compared data was not found.
Various inactivated bacterial species affected SCAP ALP production in a species-specific manner (Figures 5A, B), in contrast to non-treated and E. coli LPS treated positive controls. The UV-C–inactivated F. nucleatum, A. gerensceriae, S. exigua, and E. faecalis induced an increase in ALP production in SCAPs, that was not significantly different from the LPS positive control. The treatment of cells with bacterial DNA resulted in significantly decreased ALP levels for all bacterial species compared to the positive LPS control. These findings can be interpreted that long-term influence of UV-C–inactivated bacteria can increase mineralization of SCAP, while bacterial DNA has no influence on SCAPs mineralization.
Using Multiplex ELISA, we were able to detect which of proteins are secreted by SCAPs as osteo/odontogenic or inflammatory promoters, under the influence of UV-C–inactivated bacteria or their DNA.
For this, the same set of UV-C–killed bacteria and corresponding DNA were co-cultured with SCAP for 21 days, and a set of osteo/odontogenic and inflammation related markers was detected afterwards in the obtained conditioned medium. The set of markers included – BDNF, GM-CSF, VEGF-A, IL-8, IL-6, MCP-1, IL-17A, IL-1α and IL-1β. To investigate the impact of UV-C–killed bacteria/bacterial DNA on SCAPs, we compared the levels of secreted cytokines in conditioned medium from treated SCAPs with untreated ones. The results show that the levels of BDNF (Figure 6A), GM-CSF (Figure 6B), IL-6 (Figure 6D), IL-8 (Figure 6E) and IL-1β (Figure 6I) were significantly upregulated when SCAPs were influenced by inactivated F. nucleatum or P. yurii. Levels of IL-17A (Figure 6C), MCP-1 (Figure 6F) and IL-1α (Figure 6H) did not exibit statistically significant observed differences, compared to non-treated controls. Interestingly, the level of VEGF-A (Figure 6G) was significantly reduced, in comparison to non-treated controls, under stimulation of all represented bacterial species DNA or UV-C–killed bacteria, except UV-C–inactivated S. exigua and L. reuteri.
Figure 6 Level of selected markers in different variants of SCAPs co-cultures (A) BDNF, (B) GM-CSF, (C) IL-17A, (D) IL-6, (E) IL-8, (F) MCP-1, (G) VEGF, (H) IL-1α, (I) IL-1β, (Mean ± SD). A conditioned medium of SCAP cultured for 21 days without any treatment was used as a negative control. For positive control, SCAPs were co-cultured with LPS of E. coli. Statistical significance is marked as: * p <0.05, ** p 0.01, *** p <0.001, **** p <0.0001.
Dependent on the niche, human stem cells can be in constant interaction with commensal or pathogenic bacteria, which can determine cells’ differentiation and proliferation potential (Globus et al., 1998). In this regard, dental stem cells represent valuable examples of this interaction. In the event of trauma, cells that reside in the root canal and pulpal space of immature permanent teeth can be directly exposed to the bacteria that inhabit the oral cavity (Petridis et al., 2018b). In this case, regenerative endodontic treatment using dental stem cells, such as SCAP, may be hazarded due to the possible impact of bacteria not only on SCAP proliferation, but their odontogenic differentiation (Diogenes and Hargreaves, 2017).
In our past studies, we have shown how short-term modulation of SCAP by bacteria can affect their viability, immune response, and gene expression for osteogenesis and dentinogenesis (Razghonova et al., 2022; Zymovets et al., 2022). However, to assess whether bacterial influence alone, without the presence of osteo/dentinogenic induction medium, can stimulate SCAPs mineralization and thus influence their differentiation potential, a minimum of 21 days of bacterial exposure is required, which is the necessary duration for cell differentiation (Abuarqoub et al., 2015).
In this study, we chose UV-C irradiation for bacterial inactivation, as the most optimal method to mimic the effect of live bacteria on SCAP for long-term cultivation. Compared to other types of UVR based on spectral regions such as UVA (320–400nm) and UVB (280–320nm), UV-C irradiation (100–280nm) is the most commonly used method for bacterial inactivation (Coohill and Sagripanti, 2008). While UV-A have a mostly indirect effect, resulting in influence on bacterial proteins and lipids thereby modifying membrane permeability (Bose and Chatterjee, 1995; Futsaether et al., 1995), and UV-B experience both direct and indirect damage (Moran, 2000), UV-C irradiation is directly absorbed by bacterial DNA, subsequently disrupting it natural structure by forming dimers and breaking the DNA’s phosphate base (Pfeifer, 1997). The treatment of bacteria by this method allows for killing the bacteria, while at the same time preserving the integrity of the bacterial cell, and thus the pathogen-associated molecular patterns (PAMPs), that bind to the SCAP pattern-recognition receptors (PRRs) and cause their response to the stimuli. Along with UV-C–inactivated bacteria influence, we have also investigated whether DNA of different bacterial species impact SCAP viability and mineralization, since bacterial DNA is a ligand for TLR9 (Dalpke et al., 2006), that is also expressed by SCAP, along with other PRRs (Fehrmann et al., 2020).
To date, there are very few studies on the co-cultivation of UV-C–inactivated bacteria with human cells, particularly with dental stem cells. Most of the available studies describe the effects of inactivated probiotic bacterial species in a new approach—paraprobiotics (de Almada et al., 2016). This allows us to compare that our results showed that the probiotic bacterial species Limosilactobacillus reuteri and Lactobacillus gasseri did upregulate a number of viable SCAPs, although not reaching statistical significance. Han et al. (2019) also showed that the bacterial extract of L. reuteri ATCC 11284 derived by ultrasonication promoted the proliferation of gingival mesenchymal stem cells (MSCs) in mice models. Much remains unknown regarding the effect of bacterial DNA on mammalian cells; especially in relation to mesenchymal stem cells, since most studies show the effect of bacterial DNA on the activation of immune cells (Wagner, 1999; Lipford et al., 2000; Krieg, 2002), as it has been shown that the proliferation of human B cells are stimulated by Escherichia coli DNA (Bauer et al., 2001).
Given that the viability of SCAPs was not significantly affected by inactivated bacteria or bacterial DNA compared to the control, it was possible to carry out long-term cultivation and establish the effect of these types of treatments on SCAP mineralization.
Biomineralization, an integral part of dentinogenesis, is impossible without the accumulation of hydroxyapatite (HA) crystals by odontoblasts (Boskey, 2003). Therefore, as SCAP exert differentiation potential towards odontoblast-like cells (Zhang et al., 2014), the deposits of HA crystals in SCAPs is an important marker of mineralization (Wang et al., 2012). It is indicated that SCAP stimulated by osteogenic induced medium for five weeks, exert a drastic increase on the level of HA compared to non-treated controls (Pettersson et al., 2017). Whereas in our study, despite a shorter culture time of three weeks, as well as the absence of an inducible osteo/dentinogenic medium, HA levels were increased in both inactivated bacteria and DNA treatments. However, it is still worth noting that the treatment of cells with inactivated bacteria resulted in a higher percentage of HA deposition in the cells, especially after treatment with Actinomyces gerensceriae, Slackia exigua and Peptostreptococcaceae yurii. To date, there is no evidence of how bacteria stimuli influence the accumulation of mineral deposits in dental stem cells, and further studies are needed to achieve increased knowledge about the nature of this phenomena.
Another important marker for evaluating osteogenic/odontogenic potential is calcium deposition by cells, which indicates the production of calcified matrix and mineralization and is usually estimated by Alizarin Red (Puchtler et al., 1969; Lievremont et al., 1982; Stanford et al., 1995). Multiple studies show that SCAP exhibit strong mineralization potential when induced with osteogenic differentiation media (Sonoyama et al., 2006; Bakopoulou et al., 2011; Pettersson et al., 2017; Smeda et al., 2022). In our study, we could not observe a statistically significant result of UV-C–inactivated bacterial influence on SCAPs production of bone-like nodules, evaluated by Alizarin Red assay. Nevertheless, the difference between control and UV-C–inactivated bacteria is clearly observed in Figure 4, with an increased level of Ca2+ deposition, as well as the tendency to increased percentage of Alizarin Red positive cells, in comparison to control and bacterial DNA treatment. Unfortunately, there are few studies showing how oral opportunistic bacteria influence SCAP mineralization potential. However it was shown, by (Petridis et al., 2018) that the conditioned medium of clinical isolates of Streptococcus oralis J22 and A. naeslundii T14V-J1 decreased SCAP calcium deposition after 14 days of exposure.
The increase of alkaline phosphatase (ALP)—which can be evaluated by transcriptome analysis, enzymatically or with help of histochemistry analysis—is the indicator of positive differentiation towards an osteogenic or odontogenic pathway. Importantly, ALP secretion on a cell surface is considered an early marker of mineralization, allowing to detect the initial stages of mineralization (Golub and Boesze-Battaglia, 2007). In many studies, together with the detection of osteo/odontogenic genes, the detection of ALP is used to determine the effect of bacteria on the mineralization of dental stem cells. For example, the influence of sonicated extracts of S. mutans and P. gingivalis on human dental pulp cells revealed an increase in ALP activity. Interestingly, the increase was only observed when cells were exposed to low concentrations of P. gingivalis (0.01 µg/ml), while higher concentrations (10 µg/ml) decreased the level of ALP (Abe et al., 2010). Our results demonstrated that co-cultivation with UV-C–inactivated F. nucleatum, A. gerensceriae, S. exigua, and E. faecalis induced an ALP production that was not significantly different from the lipopolysaccharide (LPS) positive control, which is in opposition with numerous studies, showing the inhibiting effect of LPS on dental stem cells (Andrukhov, 2021). For example, Li. et al. showed, that 10 μg/mL of E. coli LPS, decrease the osteogenic differentiation of PDLSCs, with TLR4 regulated nuclear factor (NF)-κB pathway (Li C. et al., 2014). In SCAPs, 5 μg/mL LPS of P. gingivalis induced autophagy and accordingly inhibited expression of ALP, dentin matrix acidic phosphoprotein 1 (DMP-1) and RUNX2 (Lei et al., 2020). However, in this regards it should be noted, that the inhibition of osteo/odontogenic markers (such as ALP) under the LPS influence, is connected with high dosages, and depends on the dental stem cells type and chosen bacteria. Some studies also evaluated isolated bacterial MAMPs’ influence on stem cells osteogenic differentiation: it was shown that LPS from Escherichia coli and lipoteichoic acid (LTA) from S. pyogenes increased alkaline phosphatase activity and calcium deposition in bone marrow mesenchymal stem cells (Mo et al., 2008).
The full process of bacterial stimulation of dental stem cells towards mineralization still remains unknown. However, we could assume that an inflammation provoked by bacterial PAMPs through stimulation of different TLRs may play a role (Kawasaki and Kawai, 2014). Bacterial peptidoglycan and LPS, which are ligands for TLR-2 and TLR-4, activate MyD88-dependent signaling, resulting in activation of the NF-κB pathway. The NF-κB pathway, in turn, is responsible for inducing the expression of genes for pro-inflammatory cytokines like IL-6, IL-8 and TNF-α (Mogensen, 2009). Several studies indicate that under the influence of these pro-inflammatory cytokines, MSCs of different origin demonstrate increase of ALP and mineralization (Croes et al., 2015; Bastidas-Coral et al., 2016; Bastidas-Coral et al., 2019). Interesting results were published by (Li J. et al., 2014) showing that SCAPs’ levels of ALP and calcium deposition were increased after stimulation of the NF-κB pathway by its activator TNF-α. In line with this data, with the use of Multiplex ELISA (using commercially-customized U-PLEX human biomarkers) in our study, we showed that UV-C–killed bacteria significantly induced pro-inflammatory cytokines secretion—IL-6, IL-8, IL-1β and granulocyte macrophage colony-stimulating factor (GM-CSF)—especially by gram-negative F. nucleatum and gram-positive P. yurii. Consistent with our results, several studies showed that LPS of periodontal pathogen P. gingivalis and LPS from E. coli can stimulate the secretion of the same pro-inflammatory cytokines IL-6 and IL-8 in periodontal-ligament–derived, gingiva-derived MSCs and SCAP (Wang and Ohura, 2002; Jönsson et al., 2008; Trubiani et al., 2012; Herath et al., 2013; Wang et al., 2013; Kato et al., 2014; Andrukhov et al., 2016; Diomede et al., 2017). This pro-inflammatory cytokines secretion was also observed for gram-positive P. yurii, which exerts the LTA ligand for TLR2, and it was shown by several studies that induction of TLR2 in human periodontal-ligament–derived stem cells also resulted in the upregulation of IL-8, IL-6, and MCP-1 (in our study we did not observe the statistically significant increase of the MCP-1, except the trend towards it) (Blufstein et al., 2018; Behm et al., 2019). Compatible with our study, the increase of GM-CSF by human monocytes was observed after LPS treatment of human monocytes culture (Meja et al., 2000), as well by Staphylococcus aureus LTA in human neutrophils (Saba et al., 2002). Regarding IL-1β, it has been observed that it is significantly upregulated when exposed to gram-negative F. nucleatum and gram-positive P. yurii. This finding was demonstrated in inflamed tissues of patients with caries and chronic periodontitis, where it was shown that the presence of IL-1β has the potential to contribute to pulp inflammation by regulating the level of IL-8 (Chang et al., 2019). Based on our previous results in short-term co-culturing (Zymovets et al., 2022), we can hypothesize that the pro-inflammatory environment mediated by F. nucleatum and P. yurii persists even with long-term co-cultivation of 21 days, which may negatively affect the osteo- and dentinogenic potential of SCAP.
Furthermore, the cytokine that is important for restoration of the angiogenesis during RET—vascular endothelial growth factor (VEGF-A) (Saghiri et al., 2015)—was significantly decreased by all bacterial species (Figure 6G). The results of some studies indicate that E. coli LPS is capable of both increasing the secretion of VEGF and its inhibition (Palaska et al., 2016; Keong et al., 2020). Notably, bacterial DNA influence had the greatest impact on the decrease of VEGF. Several different study models showed a decrease in the VEGF-C level after influence of CpG-ODN (which is present in bacterial DNA and a ligand for TLR-9) (Yan et al., 2015; Wu et al., 2016), while a study conducted by Zhou et al. showed that pre-conditioning the cardiomyocytes with CpG-ODN induce VEGF increase (Zhou et al., 2018). The observed effect might be due to the reason that short-term influence, as pre-conditioning, may induce VEGF, while longer exposure, as we observed, may led to a downregulation of this marker. Hence, decrease of the VEGF in the presence of bacteria MAMPs can impede revascularization and odontogenesis; both of which are crucial for successful endodontic treatment (Saghiri et al., 2015). An equally important cytokine for vascularization which regulates the formation of vessels by VEGF-A, is brain-derived neurotrophic factor (BDNF) (Blais et al., 2013). As inflammation can increase the level of BDNF, consistent with our results, it was shown that both LPS and LTA can induce the secretion of BDNF by DPSCs or dental pulp fibroblasts (Chmilewsky et al., 2016; Irfan et al., 2022).
However, the results of the Multiplex ELISA not only showed that UV-C–inactivated bacteria stimulate the secretion of pro-inflammatory cytokines by SCAP, but also showed the absence of the stimulating effect of bacterial DNA on cells’ pro-inflammatory markers and even demonstrated a decrease of angiogenic markers. This is in contrast with a study showing that, when stimulated TLR-9 by ligand CpG-DNA, human gingival fibroblasts exhibited strong IL-6, IL-8, and MCP-1 secretion (Uehara and Takada, 2007).
The biggest problem that arises in RET is the presence of bacteria, even after disinfection treatment, which can significantly affect the success of RET through the influence on stem cells important for regenerative procedures (Fouad et al., 2022). In our study, in order to see if long-term bacterial modulation change SCAPs mineralization potential as well as their inflammatory response, we suggested a model of using UV-C–inactivated bacteria and bacterial DNA (of bacteria commonly implicated in root canal treatment failure) to mimic the in vivo infection situation. We showed that UV-C–inactivated bacteria over the course of 21 days, resulted in an increase of hydroxyapatite and calcium deposition by SCAPs compared to bacterial DNA influence as well as to that of a non-treated control. Moreover, the trend toward increased ALP expression was observed when SCAPs were treated with UV-C–inactivated bacteria. Aligned with our previous studies, in short-term exposure to SCAP in live bacteria, Multiplex ELISA exhibited that even after 21 days of stimulation with non-active oral opportunistic bacteria, the levels of pro-inflammatory IL-6, IL-8, and GM-CSF were significantly increased; however, DNA treatment did not result in the same outcome. Therefore, the treatment of cells with bacterial DNA did not lead to increased mineralization and secretion of the pro-inflammatory SCAP response.
It is worth noting that the observed effect of SCAP mineralization was only achieved when cells were influenced by inactivated bacteria or their DNA, in the absence of the osteo/odontogenic medium stimulus. Our results are consistent with those of Zhang et al., who demonstrated that planktonic P. gingivalis and E. faecalis altered the stemness of dental pulp stem cells, leading to cells exerting markers of mineralized-like and fibroblast-like characteristics (Zhang et al., 2023). The observed mineralization effect in cells—induced not by a mineralization-inducing medium but rather by inflammation provoked by bacterial remnants—may serve as an indicator of the cellular response towards repair rather than regeneration. (Kim, 2016).
However, as the result of our work, we can draw attention to one important aspect in the microbiological cause of RET failure: the identification of bacteria in the root canal using the detection of bacterial DNA, as the most relevant method today, is not a guarantee that exactly the identified bacteria were a possible reason for the failure of RET. Moreover, it is shown that bacterial DNA of dead bacteria can be still identifiable in root canals at 6–24 months (Brundin et al., 2010, 2015). Therefore, based on our results, we can assume, that the isolated bacterial DNA by itself does not affect the inflammatory response and mineralization of SCAP, and therefore is unlikely to affect the success of RET. Accordingly, new methods are needed to determine whether isolated bacteria were alive at the time of the study. At the same time, based on our results, we may assume that the presence of bacterial remnants in the root canal during RET—i.e., after direct interaction with SCAP—may cause a significant change in SCAPs’ mineralization potential or in their inflammatory response.
Despite the limitations of the in vitro study, in this work we were able to elucidate the influence of opportunistic oral bacteria and their DNA on the differentiation potential of SCAPs, which is necessary to improve understanding of the causes of RET failure.
The original contributions presented in the study are included in the article/Supplementary Materials, further inquiries can be directed to the corresponding author/s.
The studies involving humans were approved by The Research Ethics Committee at Umeå University. The studies were conducted in accordance with the local legislation and institutional requirements. Written informed consent for participation in this study was provided by the participants’ legal guardians/next of kin.
VZ: Methodology, Writing – original draft. OR: Conceptualization, Data curation, Formal analysis, Methodology, Writing – original draft. PW: Methodology, Writing – original draft. AS: Methodology, Writing – review & editing. MB: Funding acquisition, Writing – review & editing. PK: Writing – review & editing. ML: Funding acquisition, Writing – review & editing. NV: Conceptualization, Funding acquisition, Project administration, Supervision, Writing – review & editing.
The author(s) declare financial support was received for the research, authorship, and/or publication of this article. This research was funded by the Knut and AliceWallenberg Foundation (project number 7003503, the Region of Vasterbotten (Sweden) via ALF grant number 7004361(NV) and 98263 (ML), Kempestiftelserna Kempe SMK-1966 and the research fund of the County Council of Vasterbotten (Project Number: 7003459 and 7003589).
We gratefully acknowledge Dr. Igor Iashchishyn from the Department of Medical Biochemistry and Biophysics, Umeå University, for his expertise and assistance with the statistical analysis conducted in this publication. We acknowledge the Biochemical Imaging Center at Umeå University and the National Microscopy Infrastructure, NMI (VR-RFI 2019-00217) for providing assistance in microscopy.
The authors declare that the research was conducted in the absence of any commercial or financial relationships that could be construed as a potential conflict of interest.
All claims expressed in this article are solely those of the authors and do not necessarily represent those of their affiliated organizations, or those of the publisher, the editors and the reviewers. Any product that may be evaluated in this article, or claim that may be made by its manufacturer, is not guaranteed or endorsed by the publisher.
Abe, S., Imaizumi, M., Mikami, Y., Wada, Y., Tsuchiya, S., Irie, S., et al. (2010). Oral bacterial extracts facilitate early osteogenic/dentinogenic differentiation in human dental pulp–derived cells. Oral. Surgery Oral. Med. Oral. Pathol. Oral. Radiol. Endodontology 109, 149–154. doi: 10.1016/j.tripleo.2009.08.028
Abuarqoub, D., Awidi, A., Abuharfeil, N. (2015). Comparison of osteo/odontogenic differentiation of human adult dental pulp stem cells and stem cells from apical papilla in the presence of platelet lysate. Arch. Oral. Biol. 60, 1545–1553. doi: 10.1016/j.archoralbio.2015.07.007
Andrukhov, O. (2021). Toll-like receptors and dental mesenchymal stromal cells. Front. Oral. Heal. 2. doi: 10.3389/froh.2021.648901
Andrukhov, O., Andrukhova, O., Özdemir, B., Haririan, H., Müller-Kern, M., Moritz, A., et al. (2016). Soluble CD14 enhances the response of periodontal ligament stem cells to P. gingivalis lipopolysaccharide. PloS One 11, e0160848. doi: 10.1371/journal.pone.0160848
Bakopoulou, A., Leyhausen, G., Volk, J., Tsiftsoglou, A., Garefis, P., Koidis, P., et al. (2011). Comparative analysis of in vitro osteo/odontogenic differentiation potential of human dental pulp stem cells (DPSCs) and stem cells from the apical papilla (SCAP). Arch. Oral. Biol. 56, 709–721. doi: 10.1016/j.archoralbio.2010.12.008
Bastidas-Coral, A. P., Bakker, A. D., Zandieh-Doulabi, B., Kleverlaan, C. J., Bravenboer, N., Forouzanfar, T., et al. (2016). Cytokines TNF- α, IL-6, IL-17F, and IL-4 differentially affect osteogenic differentiation of human adipose stem cells. Stem Cells Int. 2016, 1–9. doi: 10.1155/2016/1318256
Bastidas-Coral, A. P., Hogervorst, J. M. A., Forouzanfar, T., Kleverlaan, C. J., Koolwijk, P., Klein-Nulend, J., et al. (2019). IL-6 counteracts the inhibitory effect of IL-4 on osteogenic differentiation of human adipose stem cells. J. Cell. Physiol. 234, 20520–20532. doi: 10.1002/jcp.28652
Bauer, S., Kirschning, C. J., Häcker, H., Redecke, V., Hausmann, S., Akira, S., et al. (2001). Human TLR9 confers responsiveness to bacterial DNA via species-specific CpG motif recognition. Proc. Natl. Acad. Sci. 98, 9237–9242. doi: 10.1073/pnas.161293498
Behm, C., Blufstein, A., Gahn, J., Noroozkhan, N., Moritz, A., Rausch-Fan, X., et al. (2019). Soluble CD14 enhances the response of periodontal ligament stem cells to toll-like receptor 2 agonists. Mediators Inflamm. 2019, 1–13. doi: 10.1155/2019/8127301
Bergenholtz, G. (1974). Micro-organisms from necrotic pulp of traumatized teeth. Odontol. Revy 25, 347–358.
Blair, H. C., Larrouture, Q. C., Li, Y., Lin, H., Beer-Stoltz, D., Liu, L., et al. (2017). Osteoblast differentiation and bone matrix formation in vivo and in vitro. Tissue Eng. Part B Rev. 23, 268–280. doi: 10.1089/ten.teb.2016.0454
Blais, M., Lévesque, P., Bellenfant, S., Berthod, F. (2013). Nerve growth factor, brain-derived neurotrophic factor, neurotrophin-3 and glial-derived neurotrophic factor enhance angiogenesis in a tissue-engineered in vitro model. Tissue Eng. Part A 19, 1655–1664. doi: 10.1089/ten.tea.2012.0745
Blufstein, A., Behm, C., Nguyen, P. Q., Rausch-Fan, X., Andrukhov, O. (2018). Human periodontal ligament cells exhibit no endotoxin tolerance upon stimulation with Porphyromonas gingivalis lipopolysaccharide. J. Periodontal Res. 53, 589–597. doi: 10.1111/jre.12549
Bose, B., Chatterjee, S. N. (1995). Correlation between UVA-induced changes in microviscosity, permeability and malondialdehyde formation in liposomal membrane. J. Photochem. Photobiol. B Biol. 28, 149–153. doi: 10.1016/1011-1344(94)07102-T
Boskey, A. L. (2003). Biomineralization: an overview. Connect. Tissue Res. 44 Suppl 1, 5–9. doi: 10.1080/03008200390152007
Brundin, M., Figdor, D., Roth, C., Davies, J. K., Sundqvist, G., Sjögren, U. (2010). Persistence of dead-cell bacterial DNA in ex vivo root canals and influence of nucleases on DNA decay in vitro. Oral. Surgery Oral. Med. Oral. Pathol. Oral. Radiol. Endodontology 110, 789–794. doi: 10.1016/j.tripleo.2010.07.010
Brundin, M., Figdor, D., Sundqvist, G., Sjögren, U. (2015). Preservation of fusobacterium nucleatum and peptostreptococcus anaerobius DNA after loss of cell viability. Int. Endod. J. 48, 37–45. doi: 10.1111/iej.12273
Casey, S. M., Fox, D., Duong, W., Bui, N., Latifi, N., Ramesh, V., et al. (2022). Patient centered outcomes among a cohort receiving regenerative endodontic procedures or apexification treatments. J. Endod. 48, 345–354. doi: 10.1016/j.joen.2021.11.013
Chang, M.-C., Lin, S.-I., Pan, Y.-H., Lin, L.-D., Wang, Y.-L., Yeung, S.-Y., et al. (2019). IL-1β-induced ICAM-1 and IL-8 expression/secretion of dental pulp cells is differentially regulated by IRAK and p38. J. Formos. Med. Assoc. 118, 1247–1254. doi: 10.1016/j.jfma.2018.11.015
Chmilewsky, F., About, I., Chung, S.-H. (2016). Pulp fibroblasts control nerve regeneration through complement activation. J. Dent. Res. 95, 913–922. doi: 10.1177/0022034516643065
Coohill, T. P., Sagripanti, J.-L. (2008). Overview of the inactivation by 254nm ultraviolet radiation of bacteria with particular relevance to biodefense. Photochem. Photobiol. 84 (5), 1084–1090. doi: 10.1111/j.1751-1097.2008.00387.x
Croes, M., Oner, F. C., Kruyt, M. C., Blokhuis, T. J., Bastian, O., Dhert, W. J. A., et al. (2015). Proinflammatory mediators enhance the osteogenesis of human mesenchymal stem cells after lineage commitment. PloS One 10, e0132781. doi: 10.1371/journal.pone.0132781
Cutler, T. D., Zimmerman, J. J. (2011). Ultraviolet irradiation and the mechanisms underlying its inactivation of infectious agents. Anim. Heal. Res. Rev. 12, 15–23. doi: 10.1017/S1466252311000016
Dalpke, A., Frank, J., Peter, M., Heeg, K. (2006). Activation of toll-like receptor 9 by DNA from different bacterial species. Infect. Immun. 74, 940–946. doi: 10.1128/IAI.74.2.940-946.2006
de Almada, C. N., Almada, C. N., Martinez, R. C. R., Sant’Ana, A. S. (2016). Paraprobiotics: Evidences on their ability to modify biological responses, inactivation methods and perspectives on their application in foods. Trends Food Sci. Technol. 58, 96–114. doi: 10.1016/j.tifs.2016.09.011
Dinarello, C. A. (2018). Overview of the IL-1 family in innate inflammation and acquired immunity. Immunol. Rev. 281, 8–27. doi: 10.1111/imr.12621
Diogenes, A., Hargreaves, K. M. (2017). Microbial modulation of stem cells and future directions in regenerative endodontics. J. Endod. 43, S95–S101. doi: 10.1016/j.joen.2017.07.012
Diomede, F., Zingariello, M., Cavalcanti, M. F. X. B., Merciaro, I., De Isla, N., Caputi, S., et al. (2017). MyD88/ERK/NFkB pathways and pro-inflammatory cytokines release in periodontal ligament stem cells stimulated by Porphyromonas gingivalis. Eur. J. Histochem. 61, 112–117. doi: 10.4081/ejh.2017.2791
Fehrmann, C., Dörfer, C. E., Fawzy El-Sayed, K. M. (2020). Toll-like receptor expression profile of human stem/progenitor cells form the apical papilla. J. Endod. 46, 1623–1630. doi: 10.1016/j.joen.2020.08.017
Fouad, A. F. (2019). Microbiological aspects of traumatic injuries. Dent. Traumatol. 35, 324–332. doi: 10.1111/edt.12494
Fouad, A. F., Diogenes, A. R., Torabinejad, M., Hargreaves, K. M. (2022). Microbiome changes during regenerative endodontic treatment using different methods of disinfection. J. Endod. 48, 1273–1284. doi: 10.1016/j.joen.2022.07.004
Futsaether, C. M., Kjeldstad, B., Johnsson, A. (1995). Intracellular pH changes induced in Propionibacterium acnes by UVA radiation and blue light. J. Photochem. Photobiol. B Biol. 31, 125–131. doi: 10.1016/1011-1344(95)07102-4
Gao, W., Leung, K., Hawdon, N. (2009). Freezing inactivation of escherichia coli and enterococcus faecalis in water: response of different strains. Water Environ. Res. 81, 824–830. doi: 10.2175/106143009X407348
Globus, R. K., Doty, S. B., Lull, J. C., Holmuhamedov, E., Humphries, M. J., Damsky, C. H. (1998). Fibronectin is a survival factor for differentiated osteoblasts. J. Cell Sci. 111, 1385–1393. doi: 10.1242/jcs.111.10.1385
Golub, E. E., Boesze-Battaglia, K. (2007). The role of alkaline phosphatase in mineralization. Curr. Opin. Orthop. 18, 444–448. doi: 10.1097/BCO.0b013e3282630851
Goosen, N., Moolenaar, G. F. (2008). Repair of UV damage in bacteria. DNA Repair (Amst). 7, 353–379. doi: 10.1016/j.dnarep.2007.09.002
Han, N., Jia, L., Su, Y., Du, J., Guo, L., Luo, Z., et al. (2019). Lactobacillus reuteri extracts promoted wound healing via PI3K/AKT/β-catenin/TGFβ1 pathway. Stem Cell Res. Ther. 10, 243. doi: 10.1186/s13287-019-1324-8
Hecova, H., Tzigkounakis, V., Merglova, V., Netolicky, J. (2010). A retrospective study of 889 injured permanent teeth. Dent. Traumatol. 26, 466–475. doi: 10.1111/j.1600-9657.2010.00924.x
Hemmi, H., Kaisho, T., Takeda, K., Akira, S. (2003). The roles of toll-like receptor 9, myD88, and DNA-dependent protein kinase catalytic subunit in the effects of two distinct CpG DNAs on dendritic cell subsets. J. Immunol. 170, 3059–3064. doi: 10.4049/jimmunol.170.6.3059
Herath, T. D. K., Darveau, R. P., Seneviratne, C. J., Wang, C.-Y., Wang, Y., Jin, L. (2013). Tetra- and penta-acylated lipid A structures of porphyromonas gingivalis LPS differentially activate TLR4-mediated NF-κB signal transduction cascade and immuno-inflammatory response in human gingival fibroblasts. PloS One 8, e58496. doi: 10.1371/journal.pone.0058496
Irfan, M., Kim, J. H., Druzinsky, R. E., Ravindran, S., Chung, S. (2022). Complement C5aR/LPS-induced BDNF and NGF modulation in human dental pulp stem cells. Sci. Rep. 12, 2042. doi: 10.1038/s41598-022-06110-0
Jönsson, D., Nebel, D., Bratthall, G., Nilsson, B.-O. (2008). LPS-induced MCP-1 and IL-6 production is not reversed by oestrogen in human periodontal ligament cells. Arch. Oral. Biol. 53, 896–902. doi: 10.1016/j.archoralbio.2008.05.001
Kato, H., Taguchi, Y., Tominaga, K., Umeda, M., Tanaka, A. (2014). Porphyromonas gingivalis LPS inhibits osteoblastic differentiation and promotes pro-inflammatory cytokine production in human periodontal ligament stem cells. Arch. Oral. Biol. 59, 167–175. doi: 10.1016/j.archoralbio.2013.11.008
Kawasaki, T., Kawai, T. (2014). Toll-like receptor signaling pathways. Front. Immunol. 5. doi: 10.3389/fimmu.2014.00461
Keong, J. Y., Low, L. W., Chong, J. M., Ong, Y. Y., Pulikkotil, S. J., Singh, G., et al. (2020). Effect of lipopolysaccharide on cell proliferation and vascular endothelial growth factor secretion of periodontal ligament stem cells. Saudi Dent. J. 32, 148–154. doi: 10.1016/j.sdentj.2019.08.001
Kolar, M. K., Itte, V. N., Kingham, P. J., Novikov, L. N., Wiberg, M., Kelk, P. (2017). The neurotrophic effects of different human dental mesenchymal stem cells. Sci. Rep. 7, 12605. doi: 10.1038/s41598-017-12969-1
Komazin, G., Maybin, M., Woodard, R. W., Scior, T., Schwudke, D., Schombel, U., et al. (2019). Substrate structure-activity relationship reveals a limited lipopolysaccharide chemotype range for intestinal alkaline phosphatase. J. Biol. Chem. 294, 19405–19423. doi: 10.1074/jbc.RA119.010836
Krieg, A. M. (2002). CpG motifs in bacterial DNA and their immune effects. Annu. Rev. Immunol. 20, 709–760. doi: 10.1146/annurev.immunol.20.100301.064842
Lei, S., Liu, X.-M., Liu, Y., Bi, J., Zhu, S., Chen, X. (2020). Lipopolysaccharide downregulates the osteo-/odontogenic differentiation of stem cells from apical papilla by inducing autophagy. J. Endod. 46, 502–508. doi: 10.1016/j.joen.2020.01.009
Lei, W., Ni, H., Herington, J., Reese, J., Paria, B. C. (2015). Alkaline phosphatase protects lipopolysaccharide-induced early pregnancy defects in mice. PloS One 10, e0123243. doi: 10.1371/journal.pone.0123243
Li, C., Li, B., Dong, Z., Gao, L., He, X., Liao, L., et al. (2014). Lipopolysaccharide differentially affects the osteogenic differentiation of periodontal ligament stem cells and bone marrow mesenchymal stem cells through Toll-like receptor 4 mediated nuclear factor κB pathway. Stem Cell Res. Ther. 5, 67. doi: 10.1186/scrt456
Li, J., Yan, M., Wang, Z., Jing, S., Li, Y., Liu, G., et al. (2014). Effects of canonical NF-κB signaling pathway on the proliferation and odonto/osteogenic differentiation of human stem cells from apical papilla. BioMed. Res. Int. 2014, 319651. doi: 10.1155/2014/319651
Lievremont, M., Potus, J., Guillou, B. (1982). Use of alizarin red S for histochemical staining of Ca2+ in the mouse; some parameters of the chemical reaction in vitro. Cells Tissues Organs 114, 268–280. doi: 10.1159/000145596
Lipford, G. B., Bendigs, S., Heeg, K., Wagner, H. (2000). Poly-guanosine motifs costimulate antigen-reactive CD8 T cells while bacterial CpG-DNA affect T-cell activation via antigen-presenting cell-derived cytokines. Immunology 101, 46–52. doi: 10.1046/j.1365-2567.2000.00077.x
Liu, Q., Lei, L., Yu, T., Jiang, T., Kang, Y. (2018). Effect of brain-derived neurotrophic factor on the neurogenesis and osteogenesis in bone engineering. Tissue Eng. Part A 24, 1283–1292. doi: 10.1089/ten.tea.2017.0462
Manoharan, L., Brundin, M., Rakhimova, O., Chávez de Paz, L., Romani Vestman, N. (2020). New insights into the microbial profiles of infected root canals in traumatized teeth. J. Clin. Med. 9, 3877. doi: 10.3390/jcm9123877
Meja, K. K., Seldon, P. M., Nasuhara, Y., Ito, K., Barnes, P. J., Lindsay, M. A., et al. (2000). p38 MAP kinase and MKK-1 co-operate in the generation of GM-CSF from LPS-stimulated human monocytes by an NF-κB-independent mechanism. Br. J. Pharmacol. 131, 1143–1153. doi: 10.1038/sj.bjp.0703684
Mo, I. F. Y., Yip, K. H. K., Chan, W. K., Law, H. K. W., Lau, Y. L., Chan, G. C. F. (2008). Prolonged exposure to bacterial toxins downregulated expression of toll-like receptors in mesenchymal stromal cell-derived osteoprogenitors. BMC Cell Biol. 9, 52. doi: 10.1186/1471-2121-9-52
Mogensen, T. H. (2009). Pathogen recognition and inflammatory signaling in innate immune defenses. Clin. Microbiol. Rev. 22, 240–273. doi: 10.1128/CMR.00046-08
Moran, M. A. Z. R. G. (2000). “UV RADIATION EFFECTS ON MICROBES AND MICROBIAL PROCESSES,” in Microbial ecology of the oceans. Ed. Kirchman, D. L. (New York: John Wiley & Sons Incorporated), 201–228.
Morsczeck, C. O., Dress, J., Gosau, M. (2012). Lipopolysaccharide from Escherichia coli but not from Porphyromonas gingivalis induce pro-inflammatory cytokines and alkaline phosphatase in dental follicle cells. Arch. Oral. Biol. 57, 1595–1601. doi: 10.1016/j.archoralbio.2012.07.016
Nguyen, M. T., Götz, F. (2016). Lipoproteins of gram-positive bacteria: key players in the immune response and virulence. Microbiol. Mol. Biol. Rev. 80, 891–903. doi: 10.1128/MMBR.00028-16
Nielsen, K. M., Johnsen, P. J., Bensasson, D., Daffonchio, D. (2007). Release and persistence of extracellular DNA in the environment. Environ. Biosafety Res. 6, 37–53. doi: 10.1051/ebr:2007031
Palaska, I., Gagari, E., Theoharides, T. C. (2016). The effects of P. gingivalis and E. coli LPS on the expression of proinflammatory mediators in human mast cells and their relevance to periodontal disease. J. Biol. Regul. Homeost. Agents 30, 655–664.
Petridis, X., van der Sluis, L. W. M., Dijkstra, R. J. B., Brinker, M. G. L., van der Mei, H. C., Harmsen, M. C. (2018). Secreted products of oral bacteria and biofilms impede mineralization of apical papilla stem cells in TLR-, species-, and culture-dependent fashion. Sci. Rep. 8, 12529. doi: 10.1038/s41598-018-30658-5
Pettersson, L. F., Kingham, P. J., Wiberg, M., Kelk, P. (2017). In vitro osteogenic differentiation of human mesenchymal stem cells from jawbone compared with dental tissue. Tissue Eng. Regen. Med. 14, 763–774. doi: 10.1007/s13770-017-0071-0
Pfeifer, G. P. (1997). Formation and processing of UV photoproducts: effects of DNA sequence and chromatin environment. Photochem. Photobiol. 65, 270–283. doi: 10.1111/j.1751-1097.1997.tb08560.x
Puchtler, H., Meloan, S. N., Terry, M. S. (1969). On the history and mechanism of alizarin and alizarin red S stains for calcium. J. Histochem. Cytochem. 17, 110–124. doi: 10.1177/17.2.110
Rakhimova, O., Schmidt, A., Landström, M., Johansson, A., Kelk, P., Romani Vestman, N. (2021). Cytokine secretion, viability, and real-time proliferation of apical-papilla stem cells upon exposure to oral bacteria. Front. Cell. Infect. Microbiol. 10. doi: 10.3389/fcimb.2020.620801
Razghonova, Y., Zymovets, V., Wadelius, P., Rakhimova, O., Manoharan, L., Brundin, M., et al. (2022). Transcriptome analysis reveals modulation of human stem cells from the apical papilla by species associated with dental root canal infection. Int. J. Mol. Sci. 23, 14420. doi: 10.3390/ijms232214420
Romani Vestman, N., Chen, T., Lif Holgerson, P., Öhman, C., Johansson, I. (2015). Oral microbiota shift after 12-week supplementation with lactobacillus reuteri DSM 17938 and PTA 5289; a randomized control trial. PloS One 10 (5), e0125812. doi: 10.1371/journal.pone.0125812
Romani Vestman, N., Timby, N., Holgerson, P. L. (2013). Characterization and in vitro properties of oral lactobacilli in breastfed infants. BMC Microbiol. 13, 193. doi: 10.1186/1471-2180-13-193
Rose, L. J., O’Connell, H. (2009). UV light inactivation of bacterial biothreat agents. Appl. Environ. Microbiol. 75, 2987–2990. doi: 10.1128/AEM.02180-08
Saba, S., Soong, G., Greenberg, S., Prince, A. (2002). Bacterial stimulation of epithelial G-CSF and GM-CSF expression promotes PMN survival in CF airways. Am. J. Respir. Cell Mol. Biol. 27, 561–567. doi: 10.1165/rcmb.2002-0019OC
Saghiri, M. A., Asatourian, A., Sorenson, C. M., Sheibani, N. (2015). Role of angiogenesis in endodontics: contributions of stem cells and proangiogenic and antiangiogenic factors to dental pulp regeneration. J. Endod. 41, 797–803. doi: 10.1016/j.joen.2014.12.019
Saito, K., Nakatomi, M., Ida-Yonemochi, H., Kenmotsu, S., Ohshima, H. (2011). The expression of GM-CSF and osteopontin in immunocompetent cells precedes the odontoblast differentiation following allogenic tooth transplantation in mice. J. Histochem. Cytochem. 59, 518–529. doi: 10.1369/0022155411403314
Sakamoto, M., Rocas, I. N., Siqueira, J. F., Benno, Y. (2006). Molecular analysis of bacteria in asymptomatic and symptomatic endodontic infections. Oral. Microbiol. Immunol. 21, 112–122. doi: 10.1111/j.1399-302X.2006.00270.x
Sharaf, P. H., El Backly, R. M., Sherif, R. A., Zaazou, A. M., Hafez, S. F. (2022). Microbial identification from traumatized immature permanent teeth with periapical lesions using matrix-assisted laser desorption/ionization time-of-flight mass spectrometry. BMC Oral. Health 22, 661. doi: 10.1186/s12903-022-02562-y
Siqueira, J. F., Alves, F. R. F., Rôças, I. N. (2011). Pyrosequencing analysis of the apical root canal microbiota. J. Endod. 37, 1499–1503. doi: 10.1016/j.joen.2011.08.012
Siqueira, J. F., Jr., Rôças, I. N. (2019). "Microbiology of apical periodontitis" in Essential Endodontology. Prevention and Treatment of Apical Periodontitis. 3rd ed. Ed. D. Ørstavik (John Wiley & Sons, Ltd.), 91–142. doi: 10.1002/9781119272014.ch4
Siqueira, J. F., Rôças, I. N. (2008). Clinical implications and microbiology of bacterial persistence after treatment procedures. J. Endod. 34, 1291–1301.e3. doi: 10.1016/j.joen.2008.07.028
Smeda, M., Galler, K. M., Woelflick, M., Rosendahl, A., Moehle, C., Lenhardt, B., et al. (2022). Molecular biological comparison of dental pulp- and apical papilla-derived stem cells. Int. J. Mol. Sci. 23, 2615. doi: 10.3390/ijms23052615
Smelt, J. P. P. M., Brul, S. (2014). Thermal inactivation of microorganisms. Crit. Rev. Food Sci. Nutr. 54, 1371–1385. doi: 10.1080/10408398.2011.637645
Sonoyama, W., Liu, Y., Fang, D., Yamaza, T., Seo, B.-M., Zhang, C., et al. (2006). Mesenchymal stem cell-mediated functional tooth regeneration in swine. PloS One 1, e79. doi: 10.1371/journal.pone.0000079
Stanford, C. M., Jacobson, P. A., Eanes, E. D., Lembke, L. A., Midura, R. J. (1995). Rapidly forming apatitic mineral in an osteoblastic cell line (UMR 106—01 BSP). J. Biol. Chem. 270, 9420–9428. doi: 10.1074/jbc.270.16.9420
Sundqvist, G., Figdor, D. (2003). Life as an endodontic pathogen. Ecological differences between the untreated and root-filled root canals. Endod. Top. 6, 3–28. doi: 10.1111/j.1601-1546.2003.00054.x
Tomb, R. M., White, T. A., Coia, J. E., Anderson, J. G., MacGregor, S. J., Maclean, M. (2018). Review of the comparative susceptibility of microbial species to photoinactivation using 380-480 nm violet-blue light. Photochem. Photobiol. 94, 445–458. doi: 10.1111/php.12883
Tom-Kun Yamagishi, V., Torneck, C. D., Friedman, S., Huang, G. T.-J., Glogauer, M. (2011). Blockade of TLR2 inhibits porphyromonas gingivalis suppression of mineralized matrix formation by human dental pulp stem cells. J. Endod. 37, 812–818. doi: 10.1016/j.joen.2011.03.013
Trubiani, O., Ballerini, P., Murmura, G., Pizzicannella, J., Giuliani, P., Buccella, S., et al. (2012). Toll-like receptor 4 expression, interleukin-6, -8 and Ccl-20 release, and NF-KB translocation in human periodontal ligament mesenchymal stem cells stimulated with LPS- P. Gingivalis. Eur. J. Inflamm. 10, 81–89. doi: 10.1177/1721727X1201000109
Tuin, A. (2007). Detoxification of LPS by alkaline phosphatase : application of a new concept in sepsis and inflammatory bowel disease. [Doctoral thesis] (University of Groningen). Retrieved from https://pure.rug.nl/ws/portalfiles/portal/2772217/thesis.pdf.
Uehara, A., Takada, H. (2007). Functional TLRs and NODs in human gingival fibroblasts. J. Dent. Res. 86, 249–254. doi: 10.1177/154405910708600310
Verma, P., Nosrat, A., Kim, J. R., Price, J. B., Wang, P., Bair, E., et al. (2017). Effect of residual bacteria on the outcome of pulp regeneration in vivo. J. Dent. Res. 96, 100–106. doi: 10.1177/0022034516671499
Volponi, A. A., Gentleman, E., Fatscher, R., Pang, Y. W. Y., Gentleman, M. M., Sharpe, P. T. (2015). Composition of mineral produced by dental mesenchymal stem cells. J. Dent. Res. 94, 1568–1574. doi: 10.1177/0022034515599765
Wagner, H. (1999). Bacterial CpG DNA activates immune cells to signal infectious danger. Adv. Immunol. 73, 329–368. doi: 10.1016/S0065-2776(08)60790-7
Wang, J., Dai, J., Liu, B., Gu, S., Cheng, L., Liang, J. (2013). Porphyromonas gingivalis Lipopolysaccharide Activates Canonical Wnt/β-Catenin and p38 MAPK Signalling in Stem Cells from the Apical Papilla. Inflammation 36, 1393–1402. doi: 10.1007/s10753-013-9679-y
Wang, S., Mu, J., Fan, Z., Yu, Y., Yan, M., Lei, G., et al. (2012). Insulin-like growth factor 1 can promote the osteogenic differentiation and osteogenesis of stem cells from apical papilla. Stem Cell Res. 8, 346–356. doi: 10.1016/j.scr.2011.12.005
Wang, P.-L., Ohura, K. (2002). Porphyromonas gingivalis L ipopolysaccharide S ignaling in G ingival F ibroblasts –CD14 and T oll-like R eceptors. Crit. Rev. Oral. Biol. Med. 13, 132–142. doi: 10.1177/154411130201300204
Wikström, A., Brundin, M., Romani Vestman, N., Rakhimova, O., Tsilingaridis, G. (2022). Endodontic pulp revitalization in traumatized necrotic immature permanent incisors: Early failures and long-term outcomes—A longitudinal cohort study. Int. Endod. J. 55, 630–645. doi: 10.1111/iej.13735
Winand, R., Bogaerts, B., Hoffman, S., Lefevre, L., Delvoye, M., Van Braekel, J., et al. (2019). Targeting the 16S rRNA gene for bacterial identification in complex mixed samples: comparative evaluation of second (Illumina) and third (Oxford nanopore technologies) generation sequencing technologies. Int. J. Mol. Sci. 21, 298. doi: 10.3390/ijms21010298
Wu, J., Su, W., Powner, M. B., Liu, J., Copland, D. A., Fruttiger, M., et al. (2016). Pleiotropic action of CpG-ODN on endothelium and macrophages attenuates angiogenesis through distinct pathways. Sci. Rep. 6, 31873. doi: 10.1038/srep31873
Xuan, K., Li, B., Guo, H., Sun, W., Kou, X., He, X., et al. (2018). Deciduous autologous tooth stem cells regenerate dental pulp after implantation into injured teeth. Sci. Transl. Med. 10. doi: 10.1126/scitranslmed.aaf3227
Yan, W., Sun, T.-Y., Yang, C.-M., Jia, M., Li, J., Tang, H.-L., et al. (2015). CpG ODN 1826 enhances radiosensitivity of the human lung cancer cell line A549 in a rat model. Genet. Mol. Res. 14, 9804–9812. doi: 10.4238/2015.August.19.13
Yin, W., Liu, S., Dong, M., Liu, Q., Shi, C., Bai, H., et al. (2020). A new NLRP3 inflammasome inhibitor, dioscin, promotes osteogenesis. Small 16, 1905977. doi: 10.1002/smll.201905977
Yu, B., Li, Q., Zhou, M. (2019). LPS−induced upregulation of the TLR4 signaling pathway inhibits osteogenic differentiation of human periodontal ligament stem cells under inflammatory conditions. Int. J. Mol. Med. 43 (6), 2341–2351. doi: 10.3892/ijmm.2019.4165
Zhang, W., Xu, T., Li, X., Zhang, Y., Zou, X., Chen, F., et al. (2023). Single-cell atlas of dental pulp stem cells exposed to the oral bacteria Porphyromonas gingivalis and Enterococcus faecalis. Front. Cell Dev. Biol. 11. doi: 10.3389/fcell.2023.1166934
Zhang, W., Zhang, X., Ling, J., Liu, W., Zhang, X., Ma, J., et al. (2014). Proliferation and odontogenic differentiation of BMP2 gene-transfected stem cells from human tooth apical papilla: An in vitro study. Int. J. Mol. Med. 34, 1004–1012. doi: 10.3892/ijmm.2014.1862
Zhong, M., Yan, H., Li, Y. (2017). Flagellin: a unique microbe-associated molecular pattern and a multi-faceted immunomodulator. Cell. Mol. Immunol. 14, 862–864. doi: 10.1038/cmi.2017.78
Zhou, D., Su, Y., Jiang, F., Xia, J., Wu, H., Chang, Z., et al. (2018). CpG oligodeoxynucleotide preconditioning improves cardiac function after myocardial infarction via modulation of energy metabolism and angiogenesis. J. Cell. Physiol. 233, 4245–4257. doi: 10.1002/jcp.26243
Keywords: SCAP, mineralization, oral bacteria, inflammation, bacterial DNA, bacterial remnants
Citation: Zymovets V, Rakhimova O, Wadelius P, Schmidt A, Brundin M, Kelk P, Landström M and Vestman NR (2023) Exploring the impact of oral bacteria remnants on stem cells from the Apical papilla: mineralization potential and inflammatory response. Front. Cell. Infect. Microbiol. 13:1257433. doi: 10.3389/fcimb.2023.1257433
Received: 12 July 2023; Accepted: 08 November 2023;
Published: 27 November 2023.
Edited by:
Li Zhang, University of New South Wales, AustraliaReviewed by:
Oleh Andrukhov, Medical University of Vienna, AustriaCopyright © 2023 Zymovets, Rakhimova, Wadelius, Schmidt, Brundin, Kelk, Landström and Vestman. This is an open-access article distributed under the terms of the Creative Commons Attribution License (CC BY). The use, distribution or reproduction in other forums is permitted, provided the original author(s) and the copyright owner(s) are credited and that the original publication in this journal is cited, in accordance with accepted academic practice. No use, distribution or reproduction is permitted which does not comply with these terms.
*Correspondence: Valeriia Zymovets, dmFsZXJpaWEuenltb3ZldHNAdW11LnNl
†These authors have contributed equally to this work
Disclaimer: All claims expressed in this article are solely those of the authors and do not necessarily represent those of their affiliated organizations, or those of the publisher, the editors and the reviewers. Any product that may be evaluated in this article or claim that may be made by its manufacturer is not guaranteed or endorsed by the publisher.
Research integrity at Frontiers
Learn more about the work of our research integrity team to safeguard the quality of each article we publish.