- 1Department of Paraclinical Sciences, Faculty of Veterinary Medicine, Norwegian University of Life Sciences, Ås, Norway
- 2Department of Pharmacology and Pharmaceutical Biosciences, Department of Pharmacy, University of Oslo, Oslo, Norway
- 3VESO Vikan, Namsos, Norway
Piscirickettsia salmonis, the biological agent of Salmonid Rickettsial Septicemia (SRS), is a facultative intracellular bacterium that can be divided into two genogroups (LF-89 and EM-90) with different virulence levels and patterns. Studies have found co-infection of these genogroups in salmonid farms in Chile, but it is essential to assess whether this interaction within the host is related to virulence and changes in pathogen dynamics. In this study, we studied four isolates from EM-90 and one LF-89 isolate chosen based on their genomic differences. The aim was to evaluate how co-cultivation affects bacterial growth performance and virulence factor expression in Atlantic salmon (Salmo salar) in vitro and in vivo. In vitro results using FN2 medium, showed a similar growth curve between co-cultures of LF-89 and EM-90 compared to EM-90 monocultures. This was explained by the higher ratio of EM-90 to LF-89 in all co-cultures. When evaluating the expression of virulence factors, it was discovered that the luxR gene was expressed only in EM-90-like isolates and that there were significant differences between mono- and co-cultures for flaA and cheA, suggesting a response to cohabitation. Moreover, during in vivo co-cultures, transcriptomic analysis revealed an upregulation of transposases, flagellum-related genes (fliI and flgK), transporters, and permeases that could unveil novel virulence effectors used in the early infection process of P. salmonis. Thus, our work has shown that cohabitation of P. salmonis genogroups can modulate their behavior and virulence effector expression. These data can contribute to new strategies and approaches to improve the current health treatments against this salmonid pathogen.
1 Introduction
Piscirickettsia salmonis is a facultative intracellular Gamma-proteobacteria that in Chile causes the systemic disease Salmonid Rickettsial Septicemia (SRS) in several salmonid species such as Atlantic salmon (Salmo salar), rainbow trout (Oncorhynchus mykiss), and salmon coho (Oncorhynchus kisutch) (SERNAPESCA, 2022a). In other important salmon-farming countries such as Norway (Olsen et al., 1997), Canada (Brocklebank et al., 1992), Scotland (Grant et al., 1996), and Ireland (Rodger and Drinan, 1993), P. salmonis is considered a potential emerging pathogen (Long and Jones, 2021).
In Chile, SRS was the cause of mortality in 54.2% of Atlantic salmon that died during the first half of 2022 (SERNAPESCA, 2022a), resulting in annual economic losses of US$700 million for the aquaculture industry (Maisey et al., 2017). To deal with the SRS, the available treatments consist mainly in the use of antibiotic (e.g., florfenicol) reaching up to 238.2 tons in the same period (SERNAPESCA, 2022b). In addition, 28 commercial vaccines have been approved for use against SRS (SAG, 2022), but missing the expected efficacy by the industry (Figueroa et al., 2022). This could be due to how this pathogen evades the salmon immune response owing to its intracellular nature. When replicating within the cytoplasmic vacuoles of macrophages (Rozas-Serri, 2022), it inhibits phagosome–lysosome fusion through the secretion of virulence effectors using the Dot/Icm secretion system (Gomez et al., 2013) and induces cell apoptosis (Rojas et al., 2010).
P. salmonis is the only species belonging to the genus Piscirickettsia (Fryer et al., 1992) and is closely related to the genera Coxiella, Francisella, and Legionella genera (Wilhelm et al., 2006). Based on several genomic and phylogenetic studies, P. salmonis can be divided into two genogroups or subspecies (LF-89 and EM-90) (Mauel et al., 1999; Casanova et al., 2003; Reid et al., 2004; Bohle et al., 2014; Mandakovic et al., 2016; Otterlei et al., 2016; Nourdin-Galindo et al., 2017; Isla et al., 2019). It has been demonstrated that these two genogroups have different virulence levels and patterns (Rozas-Serri et al., 2017; Saavedra et al., 2017). In addition, recently the co-infection by both genogroups has been proven in Atlantic salmon at farm, fish, and tissue levels (Rozas-Serri et al., 2023).
The high salmon mortality rate caused by SRS in Chile could be related to changes in the disease dynamics of this multiple-genotype infection through competitive interactions (Kotob et al., 2016) and to the selection of strains with higher virulence, as a result of intensive farming conditions (Sundberg et al., 2016). Interestingly, modulation of the dynamics of infections has already been reported in other salmonid pathogens such as Moritella viscosa (etiological agent of winter-ulcer disease), whose transcription profile may be altered during co-cultures with Aliivibrio wodanis (Hjerde et al., 2015). Therefore, evaluating the complex interactions between P. salmonis LF-89 and EM-90 is important, because it could change the approach and strategies to control SRS.
Thus, the aim of this study was to investigate how the cohabitation of both genogroups, LF-89 and EM-90 can modulate their growth performance and virulence factors under in vitro and in vivo conditions in Atlantic salmon. This contributes to a better understanding of the SRS infection process and development of more precise treatments.
2 Materials and methods
2.1 P. salmonis isolates
Five P. salmonis isolates from Atlantic salmon in Chile provided by Prof. Sergio Marshall (Laboratory of Genetics and Molecular Immunology, Pontifical Catholic University of Valparaíso, Chile) were used in this study: one from the LF-89 genogroup (Psal-013) and four from the EM-90 genogroup (Psal-051, Psal-068, Psal-163, and Psal-182) (Table 1). The bacteria were stored at −80°C with glycerol and 20% fetal bovine serum (FBS) stocks and routinely cultured on cysteine heart agar plates (CHAB) supplemented with bovine blood (Norwegian Veterinary Institute, Oslo, Norway) and in FN2 broth medium (Bravo and Martinez, 2016) with shaking at 100 rpm and incubated at 18°C.
2.2 In vitro co-culture of P. salmonis isolates
To measure growth, the FN2 broth medium was used. Freshly grown bacterial cultures were adjusted to an initial OD of 0.02 at 600 nm and transferred into a 250 mL sterile flask with broth medium (25 mL). The flasks were maintained in agitation (100 rpm) at 18°C for 6 days for mono- and co-culture growth. The start point of the genogroup proportion for the co-cultures was a 1:1 ratio.
Each culture assay was performed in four independent replicates. Growth data were measured using a PV4 Visible Spectrophotometer (VWR) every 24 h. In addition, 1 mL samples were taken at the same time to extract total DNA, and at 48 h, 96 h, and 120 h a 500 μL sample was taken for further RNA extraction to monitor changes in P. salmonis gene expression during mono- and co-culture growth.
To check for contamination and viability, 100 μL of each liquid culture were replated on CHAB agar plates and incubated at 18°C for 10 days to evaluate colonies and further DNA extraction to perform an RFLP-PCR analysis specific for P. salmonis (Aravena et al., 2020).
2.3 DNA extraction and growth rate of P. salmonis genogroups
All the samples taken from bacterial growth kinetics were first centrifuged at 10,000×g for 10 min. The pellets were then resuspended in lysis buffer (1 M Tris–HCl, 0.2 M EDTA, 10% Triton X-100) with lysozyme and incubated for 30 min at 37°C. Protein kinase (Qiagen, Hilden, Germany) was added and incubated at 56°C for 30 min. DNA was extracted using QIAcube and QIAGEN DNeasy® Blood & Tissue Kit (Qiagen) according to the manufacturer’s instructions and stored at −20°C until use. A NanoDrop™ ND‐1000 spectrophotometer (Thermo Scientific) was used to determine the concentration and quality of DNA.
To determine the growth rate of P. salmonis genogroups, DNA samples from the co-cultures were used in triplicate for qPCR assays using an AriaMx Real-Time PCR System (Agilent Technologies). Specific primers targeting each genogroup’s unique gene were designed (Table 2). The PCR reaction mixture contained 10 μL of Maxima SYBR Green Master Mix (Thermo Scientific), 300 nM of each specific forward and reverse primer and 15 ng of DNA template in a total volume of 20 μL. The thermal profile started with an initial denaturation at 95°C for 3 min followed by 40 cycles at 95°C for 15 s and 60°C for 60 s, and a final melting curve between 60°C and 95°C. For all primers, efficiencies were established using the equation E = 10(−1/slope). Relative quantification was estimated using threshold cycle (ct) values, normalizing the Ct of the target gene to the housekeeping gene for the same sample (at the same time), and then calculating the ratio between genogroups in fold changes of EM-90 compared to LF-89.
2.4 Gene expression of target biomarkers
To evaluate the gene expression of target biomarkers in P. salmonis at 48 h, 96 h, and 120 h, 500 µL of each sample was centrifuged at 10,000×g for 10 min at 4°C to recover the pellet, which was used for RNA extraction using the Qiagen RNeasy® Mini Kit (Qiagen) according to the manufacturer’s protocol. A NanoDrop™ ND‐1000 spectrophotometer (Thermo Scientific) was used to determine the quality and quantify each RNA sample. Then cDNA was synthesized using the QuantiTect® Reverse Transcription Kit (Qiagen) following the manufacturer’s instructions.
cDNA samples were used in three technical replicates for each of the four biological replicates. Briefly, for RT-qPCR MIX, 15 ng of cDNA was used for each sample along with 10 μL of Maxima SYBR Green Master Mix (Thermo Scientific) and 300 nM of forward and reverse primers in a total volume of 20 μL. The thermal profile started with an initial denaturation at 95°C for 3 min, followed by 40 cycles at 95°C for 15 s and 60°C for 60 s, and a final melting curve between 60°C and 95°C.
Specific primers were used to establish key gene expression changes in the co-cultures compared to the monoculture using glyA as the normalizer (Table 2). For all primers, efficiencies were established using the equation E = 10(−1/slope). Relative gene expression was estimated using threshold cycle (Ct) values and the Livak 2−ΔΔCt method (Livak and Schmittgen, 2001).
2.5 Biofilm assay
Frozen stocks at −80°C from the five isolates were streaked onto cysteine heart agar plates (CHAB) supplemented with bovine blood (Norwegian Veterinary Institute, Oslo, Norway) and incubated at 18°C until growth. Single colonies of the isolates were used to inoculate 3 mL of FN2 broth, which was then incubated at 18°C. After the cultures reached the exponential phase, the optical density (OD) of each culture at 600 nm was adjusted to 0.02 to be used as an inoculum for the 96 well microplates to perform a biofilm formation assay.
Three independent experiments were performed by inoculating eight wells per isolate and co-culturing with 150 µL of the bacterial suspension in FN2 broth. FN2 medium without bacteria was used as the negative control. The microplates were incubated at 18°C with shaking at 100 rpm. After 120 h, all the medium and planktonic bacteria were carefully removed from the inoculated and control wells. Bacteria that adhered to the wells of the microplates were stained with 180 µl of crystal violet solution (0.1%) at room temperature for 10 min. The crystal violet (CV) solution was removed, and the walls were rinsed thoroughly with sterile distilled water. The microplates were turned upside down and dried for 1 h. For CV solubilization, absolute ethanol (200 µL/well) was added and left at room temperature for 10 min. The resulting solutions were homogenized by repeated pipetting before reading the microplates at 620 nm using a PV4 Visible Spectrophotometer (VWR).
2.6 In vivo co-culture of P. salmonis genogroups for RNA isolation and transcriptomics sequencing
One isolate from each genogroup was selected for in vivo cultivation, Psal-013 (LF-89) and Psal-182 (EM-90). Each isolate was cultivated in FN2 broth medium at 18°C with shaking (100 rpm) until obtaining an OD600nm of 0.2 at the beginning of the exponential phase. Thereafter, nine semi-permeable implants were made using 20 cm length dialysis tubes with a 12 kDa–14 kDa molecular weight cut-off (Spectrum Laboratories Inc., LA, CA, USA) as described by Colquhoun and Sørum (1998). One end was sealed and autoclaved in PBS 1X. Then, each tube was inoculated with a total volume of 5 mL for every bacterial culture (0.25 mL + 0.25 mL for the co-cultures) and sealed with a triple knot. Three main groups of bacterial cultures were used: Psal-013 alone, Psal-182 alone, and Psal-013 (0.25 mL) with Psal-182 (0.25 mL) mixed.
For in vivo culturing of P. salmonis isolates, nine Atlantic salmon (S. salar) post-smolts with an average weight of 1 kg were implanted with one dialysis tube (three fish per group). Fish were kept at the Norwegian Institute for Water Research (NIVA, Solbergstrand, Norway) throughout the experiment. The implants were carefully inserted into the abdominal cavity of the fish through a small 4 cm incision along with a pit-tag for marking and later identification. The cut was then sewn along with three to four sutures through all layers of the tissue. The fish were transferred to a common tank containing seawater at an average temperature of 12.5°C. After 6 days, and before any symptoms could be detected, the fish were euthanized with an overdose of benzocaine (Benzoak 200 mg/mL) for implant removal. The implants were washed with PBS 1×, and the bacterial culture was removed and stored on ice until further use. Norwegian Food Safety Authorities (FOTS ID 26316) approved the animal trial in accordance with the laws and regulations for experiments on live animals in the EU (Directive 2010/637EU) and Norway (FOR-2015-06-18-761).
From every fish, the entire content of the dialysis tube was aseptically collected and centrifuged at 5,000×g for 10 min at 4°C for pellet collection. Total RNA was extracted from all samples using the Qiagen RNeasy Mini Kit (Qiagen), according to the manufacturer’s protocol. QIAseq FastSelect−5S/16S/23S (Qiagen) was used according to the manufacturer’s instructions for rRNA depletion. The concentration and quality were measured using a Multiskan Sky Microplate Spectrophotometer (Thermo Fisher Scientific). Library preparation and RNA-seq were performed at the Norwegian Sequencing Center (UiO, Norway) using TruSeq Stranded mRNA library prep and Illumina NovaSeq SP System (150 bp paired-end RNA sequencing).
To process the raw files, the Orion High Performance Computing Cluster at the Norwegian University of Life Sciences (NMBU) was used, according to Morales-Lange et al. (2021). Raw reads from the RNA sequencing were trimmed using BBDuk (v34.56). Then, the clean reads were aligned to P. salmonis genome Psal-013 (ASM970875v1—RefSeq assembly accession: GCF_009708755.1) or Psal-182 genome (ASM970941v1—RefSeq assembly accession: GCF_009709415.1) using HISAT (v2.1.0). The fragments mapped were counted with featureCounts (v1.4.6-1) and differentially expressed genes (DEGs) between groups were estimated using SARTools (v1.7.3) package in Rstudio (v4.2.0). Significant DEGs were identified when the adjusted p-value (padj) was <0.05.
2.7 Statistical analyses
Data from growth rate, gene expression of target biomarkers and biofilm production were analysed and visualized using GraphPad Prism (v8.0.1). One-way ANOVA and Tukey’s multiple comparison test were used to determine significant differences (p <0.05). The growth rate data were correlated using the Corrplot package (v0.84) (Wei and Simko, 2017) in Rstudio (v4.2.0). Correlations were considered significant when the p-value was <0.05.
3 Results
3.1 P. salmonis co-habitation growth
Growth data showed that in monocultures, the LF-89-like isolate (Psal-013) exhibited the lowest growth of all P. salmonis isolates, reaching an OD600 nm of 3.30 after 120 h (Figures 1A–D). In comparison, the EM-90 Psal-051 isolate reached an OD600 nm of 5.46 after 120 h (Figure 1A). Psal-051 exhibited the shortest lag phase of 24 h among the remaining EM-90 isolates. Interestingly, the co-culture of Psal-013 and Psal-051 showed a decrease in growth after 120 h, reaching an OD600 nm of 4.42.
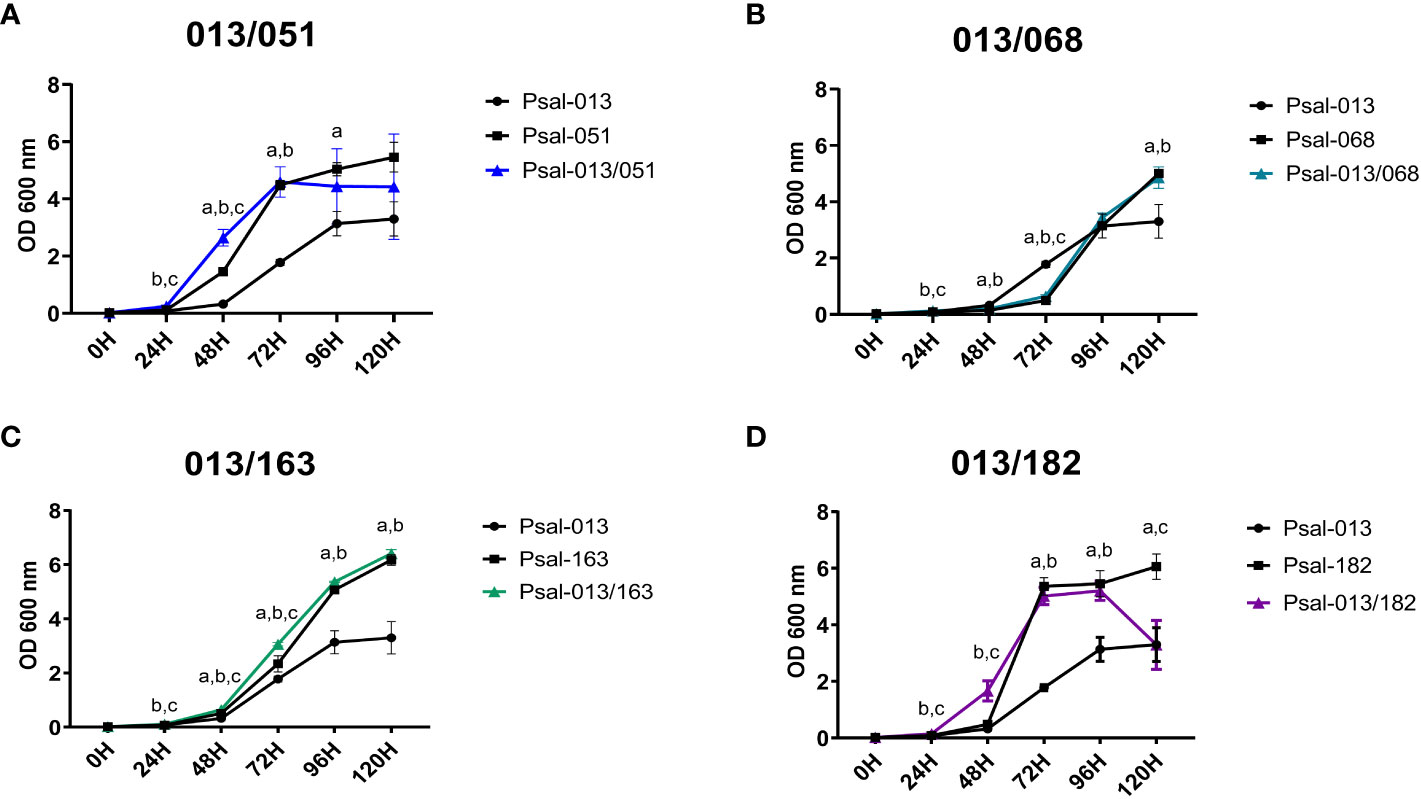
Figure 1 Growth curve of P. salmonis isolates in mono- and co-cultures. (A) Psal-051 and Psal-013, co-culture in blue. (B) Psal-068 and Psal-013, co-culture in light blue. (C) Psal-163 and Psal-013, co-culture in green. (D) Psal-182 and Psal-013, co-culture in purple. Vertical bars indicate the standard deviation. Letters indicate statistical differences at the same time for different cultures: (a) LF-89-like isolate versus EM-90-like isolate, (b) LF-89-like isolate versus co-culture, (c) EM-90-like isolate versus co-culture.
The monoculture of EM-90 isolate Psal-068 reached the logarithmic phase after 72 h of growth (Figure 1B). Psal-068, thereby, had the longest lag phase of all P. salmonis isolates, with a peak growth of 5.01 measured at OD600 nm after 120 h. Similar growth was observed when Psal-068 was co-cultured with Psal-013, following the same curve as Psal-068 alone (Figure 1B).
Moreover, the monoculture growth of the EM-90 isolate Psal-163 (Figure 1C) reached the logarithmic phase after 72 h, and the peak of growth was measured as 6.18 at OD600 nm. In the co-culture with Psal-013, no differences were observed in the growth curve (Figure 1C).
The EM-90 isolate Psal-182 exhibited the fastest logarithmic growth, reaching the stationary phase after 72 h, and a peak growth of 6.06 measured at OD600 nm after 120 h (Figure 1D). In the co-culture experiment with Psal-013, the lag phase was shorter and after 96 h reached a growth of 5.20 at OD600 nm, a decline phase started with an OD600 nm of 3.29. For the re-plating results, all cultures were viable, and no contamination was detected at any time (data not shown).
3.2 EM-90 and LF-89 growth ratio in co-cultures
After determining the effect of co-culturing the LF-89 isolate (Psal-013) with different isolates from EM-90, the growth ratio of the two genotypes in the co-culture was investigated. The results displayed in Figure 2A shows that all EM-90 isolates exhibited improved growth at the expense of LF-89. The Psal-013/051 co-culture showed the lowest difference in fold change. Co-cultures of Psal-013/068 and Psal-013/163 exhibited a similar trend, with a peak in growth after 96 h, with significant differences in time. However, in the co-culture of Psal-013 and Psal-182, the peak was reached at 72 h, after which the ratio between the genogroups began to decline.
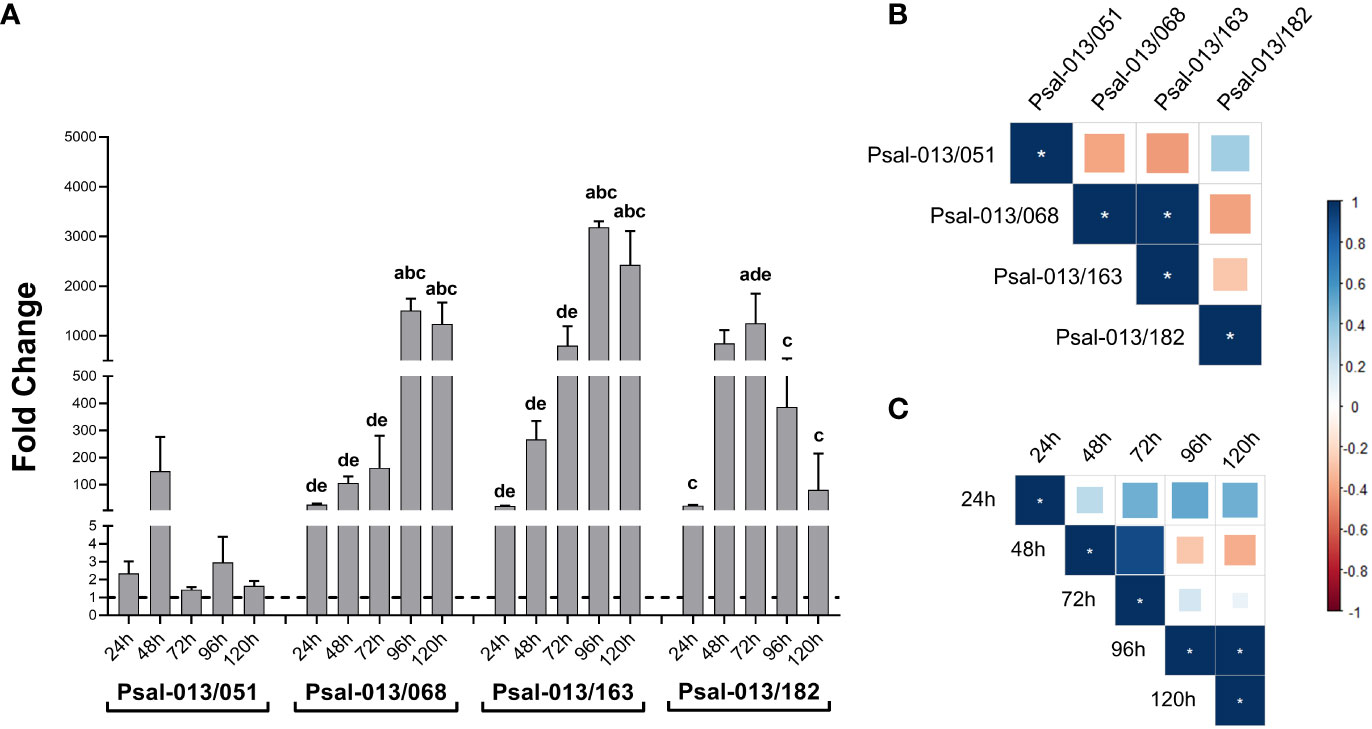
Figure 2 (A) EM-90 and L-89 growth ratio during co-cultures with standard deviation. Groups of different lowercase letters above the bar charts of each co-culture indicate significant differences (p <0.05) compared with different times: 24 h (a), 48 h (b), 96 h (c), 120 h (d). (B) Correlation coefficient between all isolates. (C) Correlation coefficient of co-cultures by time. *: significant values (p <0.05).
When investigating the correlation of all co-cultures, a positive correlation between Psal-013/068 and Psal-013/163 was found (Figure 2B). This finding suggests a similar growth ratio for EM-90 and LF-89 in these two co-cultures. Furthermore, the correlation of co-cultures by time showed a positive correlation between 96 h and 120 h (Figure 2C), which means that the ratio at 96 h and 120 h remained similar.
3.3 Biofilm formation of P. salmonis LF-89 and EM-90 genotype and their co-cultivation
As the establishment of biofilms may influence bacterial pathogenesis, it was of interest to explore differences in biofilm formation between the P. salmonis LF-89 and EM-90 strains, and when the two genotypes were co-cultivated.
The results (Figure 3) showed that the monoculture of Psal-013 (LF-89-like) had the lowest biofilm production (OD620 nm = 0.123 ± 0.08). While Psal-182 reached a higher biofilm production in co-cultures (OD620 nm = 0.70 ± 0.22) than in monoculture (OD620 nm = 0.60 ± 0.08) (Figure 3D). Psal-051 in monocultures, reached a higher production (OD620 nm = 0.507 ± 0.12) than in co-cultures (OD620 nm = 0.45 ± 0.19), but without significant differences (Figure 3A). Psal-068 (Figure 3B) significantly increased biofilm production from monoculture (OD620 nm = 0.17 ± 0.07) to co-culture (OD620 nm = 0.27 ± 0.08). Although, Psal-163 showed the same trend (Figure 3C), a lower biofilm production in monoculture (OD620 nm = 0.41 ± 0.10) than in co-cultures (OD620 nm = 0.48 ± 0.15), there was no significant differences.
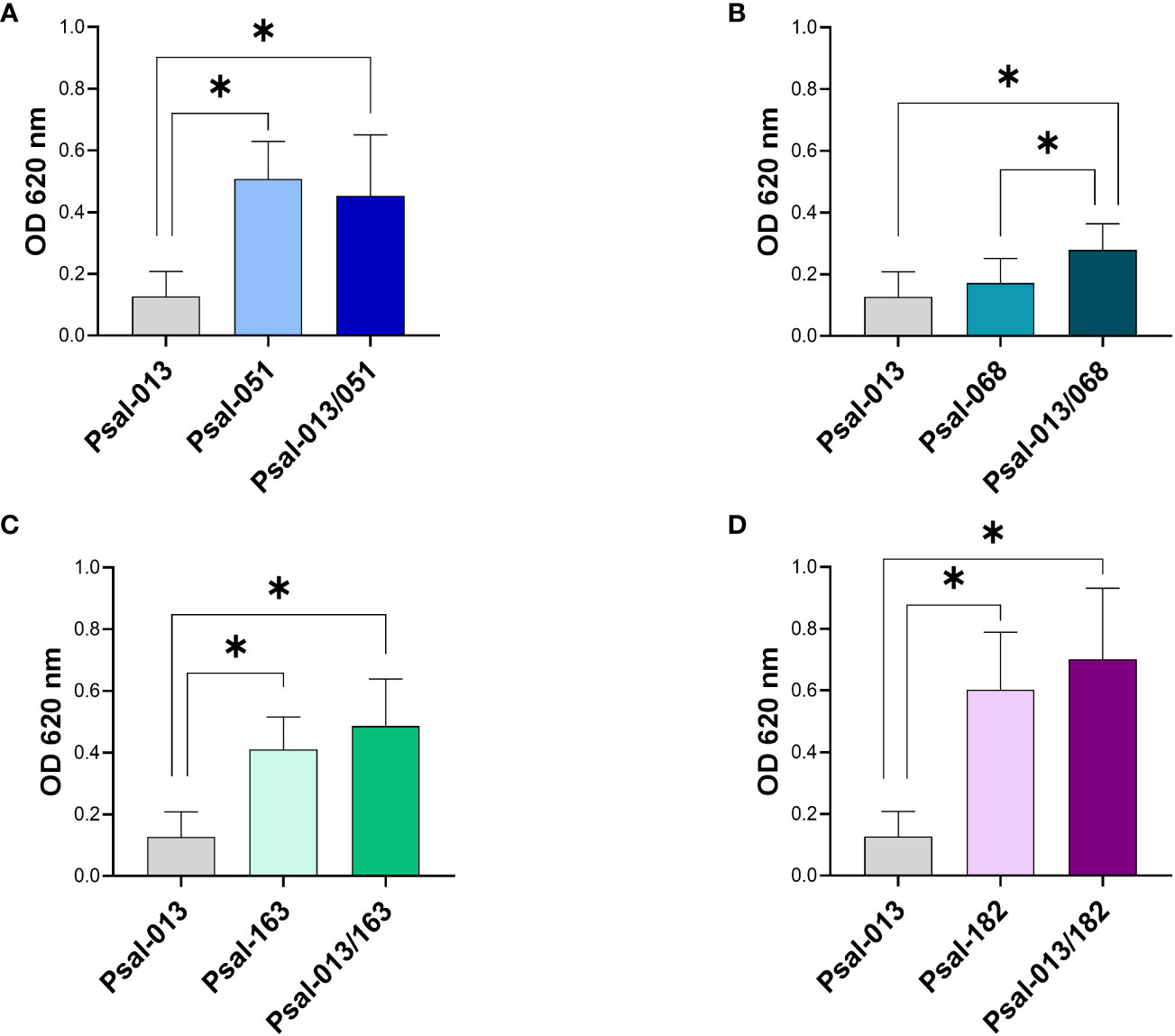
Figure 3 Biofilm production after 120 h of growth. (A) Psal-051 and Psal-013. (B) Psal-068 and Psal-013. (C) Psal-163 and Psal-013. (D) Psal-182 and Psal-013. *: significant values (p <0.05).
3.4 In vitro co-cultivation influence on gene expression
Co-cultivation of P. salmonis LF-89 and EM-90 strains affected bacterial growth phenotypes. Therefore, the next step was to investigate the effects of co-cultivation on gene expression. First we compared the expression of four marker genes (flaA, relA, luxR and cheA genes) after 120 h of growth. flaA encodes the major subunit of flagellin in flagella, while relA activates (p)ppGpp, which is involved in regulating growth and different stress responses in bacteria, luxR is related to communication through N-acylhomoserine lactone (AHL) quorum-sensing (QS) signaling and cheA to chemotaxis.
For flagellin (flaA) (Figure 4A), Psal-182 alone showed the highest expression at 120 h compared with all other cultures, with a significant difference from the co-culture with Psal-013. In contrast, Psal-068 and Psal-013 co-culture showed the lowest expression among all cultures, with a significant difference compared to Psal-068 alone. Psal-051 and Psal-163 in the co-culture presented a similar level of expression to that of Psal-013 grown alone.
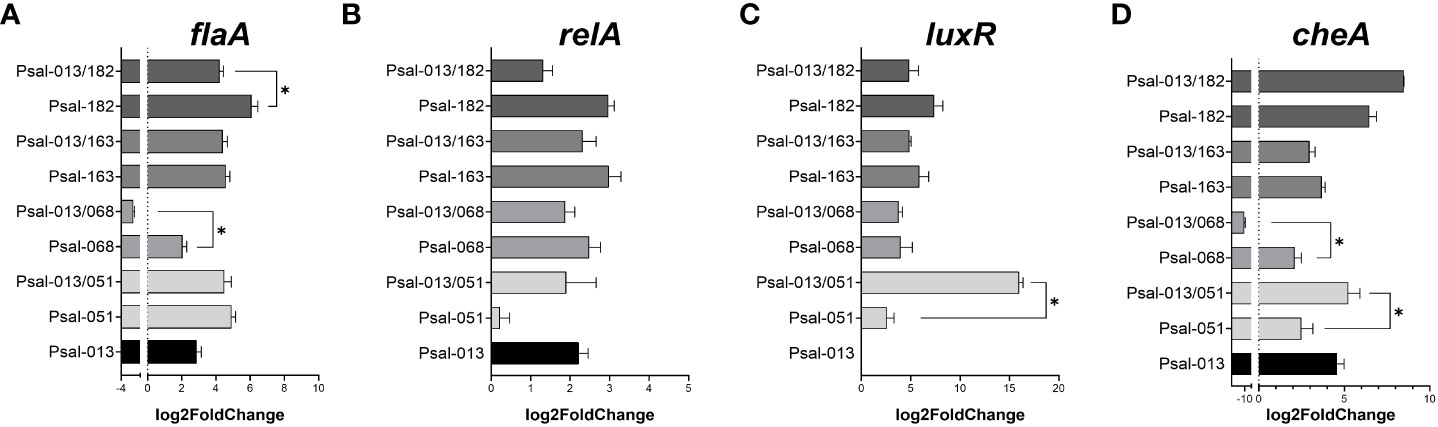
Figure 4 Expression of the marker genes flaA (A), relA (B), luxR (C), and cheA (D) genes during co-culture after 120 h of growth. *: significant values (p <0.05).
RelA gene expression (Figure 4B) was not significantly downregulated in the Psal-051 monoculture. For luxR (Figure 4C), a significant difference was observed in the high expression during the co-culture of Psal-051 and Psal-013 compared to Psal-051 monoculture. However, no expression was observed for the LF-89 isolate Psal-013 alone. Moreover, for Psal-068 and Psal-163, there were no significant differences related to their co-cultures.
Psal-013/182 co-culture showed the highest expression of cheA but was not significantly higher than that in the Psal-182 monoculture (Figure 4D). In addition, Psal-163 in mono- and co-cultures showed no differences in expression related to Psal-013 or between them. In contrast, Psal-051 co-cultured with Psal-013 showed significantly higher expression than Psal-051 monoculture. Finally, the co-culture of Psal-013/068 showed the lowest expression among all the cultures, with a significant difference from the Psal-168 monoculture.
3.5 Differential expression of genes during in vivo co-cultivation
RNA-seq analysis was performed from the co-cultivation of P. salmonis genotypes in vivo (within the dialysis bag in the fish). The DEG distribution from each pairwise comparison is shown in Table 3 (co-culture vs. Psal-182, co-culture vs. Psal-013, and Psal-182 vs. Psal-013). The metadata (normalized gene expression, description, and statistical analysis) are listed in Table S1.
Of the 12 upregulated DEGs in the co-culture compared to Psal-182, 11 belonged to the chromosome and one to plasmid 4. Moreover, of the four downregulated DEGs, three corresponded to the chromosome and one to plasmid 4. In the co-culture, compared to Psal-013, 10 upregulated DEGs corresponded to chromosome and seven to plasmid 1. The unique downregulated DEG belongs to chromosome (Table S1). Psal-182 monoculture compared to Psal-013 showed seven upregulated DEGs belonging to the chromosome, two to plasmid 4, and one to plasmid 2. In the same pairwise comparison, six of the 14 downregulated DEGs belonged to the chromosome, three to plasmid 4, four to plasmid 2 and one to plasmid 1 (Table S1).
Regarding Hypothetical Protein (HP), among the four downregulated DEGs detected in co-cultures compared to Psal-182, two were identified as HP. Similarly, in co-cultures compared with Psal-013, seven upregulated DEGs were identified as HP. In addition, in the Psal-182 monoculture compared to Psal-013, two of the 10 upregulated DEGs were identified as HP and eight out of 14 downregulated DEGs were identified as HP.
During co-culture, compared to Psal-182, the most upregulated gene was IS30 family transposase (Psal182_RS17815) located in plasmid 4, with 3.641 of fold change, followed by IS4 family transposase (helix-turn-helix domain-containing protein) (Psal182_RS12950) 3.478-fold change, IS3 family transposase (Psal182_RS02820) 3.358-fold change, DNA transposase (Psal182_RS01495) 3.085-fold change, IS30 family transposase (Psal182_RS12275) 2.966-fold change, flagellar protein export ATPase FliI (Psal182_RS02060) 2.547-fold change, and flagellar hook-associated protein FlgK (Psal182_RS10205) 2.114-fold change. The downregulated DEGs were P-II family nitrogen regulator (Psal182_RS15510) with a −2.205 of fold change and ATP-dependent Clp protease proteolytic subunit (Psal182_RS08200) with a −1.521-fold change (Figure 5A).
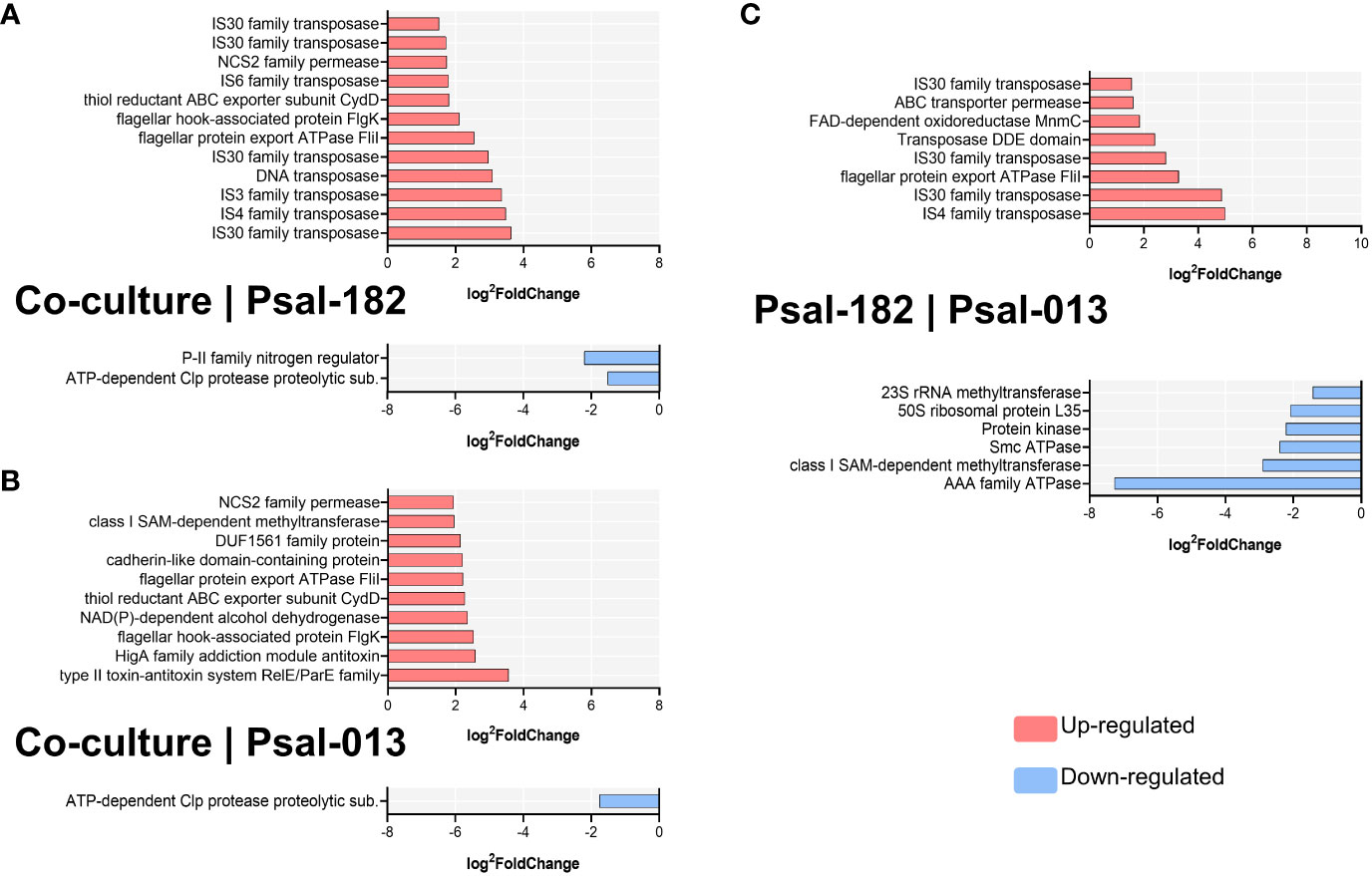
Figure 5 Significant differentially expressed genes (DEGs) per comparison during in vivo culturing. (A) Co-culture versus Psal-182 comparison. (B) Co-culture versus Psal-013 comparison (C) Psal-182 versus Psal-013 comparison (in monocultures). Data sorted by log2foldChange. Upregulated in red; Downregulated in blue.
Compared to Psal-013, during co-culture, the most upregulated gene was the type II toxin–antitoxin system RelE/ParE family (Psal013_RS16670) with 3.558-fold change, followed by HigA family addiction module antitoxin (Psal013_RS16660) with 2.581-fold change, flagellar hook-associated protein FlgK (Psal013_RS06465) with 2.522-fold change, NAD(P)-dependent alcohol dehydrogenase (Psal013_RS16675) with 2.354-fold change, thiol reductant ABC exporter subunit CydD (Psal013_RS11795) with 2.268-fold change, flagellar protein export ATPase FliI (Psal013_RS14360) with 2.22-fold change, and cadherin-like domain-containing protein (Psal013_RS10175) with 2.205-fold change. In contrast, the only downregulated DEG was the ATP-dependent Clp protease proteolytic subunit (Psal013_RS08085) with a fold change of −1.767 (Figure 5B).
In Psal-182 in vivo monocultures compared to Psal-013, the upregulated genes were IS4 family transposase (Psal182_RS12950) with 4.991 of fold change, followed by IS30 family transposase (Psal182_RS17815) (from plasmid 4) 4.86-fold change, flagellar protein export ATPase FliI (Psal182_RS02060) 3.28-fold change, IS30 family transposase (Psal182_RS12275) 2.809-fold change, Transposase DDE domain (Psal182_RS01495) 2.403-fold change, FAD-dependent 5-carboxymethylaminomethyl-2-thiouridine(34) oxidoreductase MnmC (Psal182_RS09240) 1.85-fold change, ABC transporter permease (Psal182_RS11665) 1.609-fold change, and IS30 family transposase (Psal182_RS17360) (located in plasmid 2) 1.552-fold change. The downregulated DEGs were AAA family ATPase (Psal182_RS17775) with a −7.26 fold change, followed by class I SAM-dependent methyltransferase (Psal182_RS01935) with a −2.903-fold change, Smc ATPase (Psal182_RS02460) −2.405-fold change, Protein kinase (Psal182_RS16425) with a−2.225-fold change, and 50S ribosomal protein L35 (Psal182_RS02405) with a −2.087-fold change (Figure 5C).
4 Discussion
Intensive fish farming has led to the increased occurrence of infectious diseases in aquaculture-related species (Sundberg et al., 2016). Moreover, co-infections in aquatic organisms are natural events that should be considered when studying the biology of pathogens for control measures (Kotob et al., 2016), since infection with multiple pathogens may increase the severity of diseases in fish (Long et al., 2019). Nevertheless, in the case of P. salmonis the possibility of a mixed infection of the two genogroups described in Chile has been mostly ignored because the official surveillance program aims to detect the pathogen as a species in salmon farms to prevent and outbreak of SRS, but it does not consider the identification of the genogroups.
In our study, five isolates of P. salmonis were chosen based on their genomic features (Mauel et al., 1999), the sub-phylogenetic organization of the genetically diverged EM-90 genogroup composed by four groups, and the LF-89 monophyletic group (Schober, 2022). To evaluate the relevance of this, one isolate belonging to each subgroup was chosen to present a wider view of P. salmonis genomes and differences among them under in vitro conditions. The results showed evidence of heterogeneity in growth and biofilm production within the EM-90 genogroup. Furthermore, the LF-89 genogroup isolate presented a significantly lower growth rate and biofilm production than all EM-90 isolates. Considering that both genogroups belong to the same species, this supports the differences within the bacterial population of P. salmonis that have been described previously (Bohle et al., 2014).
Using FN2 broth medium for bacterial growth, we achieved a shorter lag phase and a longer logarithmic phase compared with previous studies (Yanez et al., 2012). In that report, they achieved an OD620 nm of 1.8 after 6 days using an EM-90-like isolate, while here we achieved a mean OD600 nm of 5.7 measured after 5 days for the four EM-90 isolates in monocultures and a mean OD600 nm of 4.7 for co-cultures due to the lower growth rate of the LF-89 isolate, which had a mean OD600 nm of 3.3 at the same time. This is in line with the evaluated genogroup ratio of growth during co-cultures using specific primers, where the positive correlation between Psal-013/068 and Psal-013/163 indicated a similarity between these two EM-90 isolates. Thus, to obtain higher P. salmonis biomass, FN2 broth medium is recommended.
In the growth kinetics we observed in Psal-182 co-cultured with Psal-013, a large amount of biofilm was formed at 120 h, which may have interfered with OD measurements as shown in Figure 1D. Biofilm production in P. salmonis is a well-described virulence factor (Marshall et al., 2012; Levipan et al., 2020; Santibanez et al., 2020; Zuniga et al., 2020) that is used as a form of survival strategy and resistance to environmental stressors. The results for Psal-013 (LF-89-like isolate), which had the lowest growth rate and biofilm production, suggest that this is the least virulent isolate. Additionally, three EM-90-like isolates (Psal-068, Psal-163, and Psal-182) exhibited higher biofilm production during the co-cultures. Apart from the biofilm level in the co-culture of Psal-051 (EM-90-like) that could be explained by the higher proportion of LF-89 (Psal-013). These data support the evidence that enhanced biofilm formation may be triggered by the synergistic effect of co-culturing P. salmonis genotypes, which could be indicative of higher virulence during cohabitation (Levipan et al., 2020) as well as the heterogeneity of different P. salmonis isolates (Oliver et al., 2023).
Gene expression of target biomarkers showed that flaA (flagellin) was upregulated in monocultures compared to co-cultures with Psal-013, Psal-182, and Psal-068. This is relevant even though this pathogen is described as non-motile, because the flagellin monomer has been detected using a specific antibody under cell-free conditions and during infection in SHK-1 cells (Carril et al., 2017). This suggests that the transcriptional cascade of flagellar genes responds distinctively to co-culture conditions. Regarding CheA, a gene related to chemotaxis (Tremaroli et al., 2011) and biofilm production in fish pathogens (Yang and Defoirdt, 2015), our data showed the highest expression in Psal-182, which coincided with the higher production of biofilm. These results are consistent with those of previous studies on P. salmonis (Albornoz et al., 2017). Remarkably, luxR, which is related to N-acylhomoserine lactone (AHL) quorum-sensing (QS) signaling for bacterial communication (Subramoni and Venturi, 2009; Ruiz et al., 2021), was not expressed in the LF-89 isolate Psal-013. Neverthelss, even though luxR is found in the Psal-013 genome, apparently under these in vitro conditions is not being expressed.
In in vivo cultures, a lower number of DEGs was observed compared to other transcriptome analyses performed in P. salmonis. However, they used different approaches to study metabolic resistance to antibiotics (Ortiz-Severin et al., 2021) and virulence during biofilm formation (Levipan et al., 2022). In this study, an in vivo culture of P. salmonis was used for the first time to study transcriptional changes, and the RNA-seq data showed what is driving the early infection process. Interestingly, in co-cultures, compared to monocultures of the EM-90-like isolate Psal-182, 50% of the most differentially expressed genes corresponded to transposons that function as mobile elements for DNA rearrangements, transcriptional changes, or gene regulation (De Palmenaer et al., 2008; Lysnyansky et al., 2009). In addition, different transposase families were upregulated simultaneously in plasmids and chromosome, such as in IS30 of Psal-182, which could indicate the importance of the plasmids for P. salmonis virulence, as described previously (Saavedra et al., 2018).
In addition, the IS6 family transposase was upregulated during co-culture compared to Psal-182, which has been described as relevant to the spread of antibiotic resistance by horizontal transference of genes inside bacterial populations (Varani et al., 2021). A similar pattern was detected for the IS4 transposase family, which triggers important rearrangements in prokaryotic genomes as a tool for genome flexibility and evolution (De Palmenaer et al., 2008), and for DNA-binding transposase (part of Class II transposons), in addition to the IS3 family transposase, which catalyzes the movement of transposons to other parts of the genome by a replicative transposition mechanism (Duval-Valentin et al., 2001). This is interesting because genome rearrangements and deletions linked to transposons have improved the ability of bacteria to combat host defenses, likely by changing surface antigens and regulatory circuitry (Siguier et al., 2014), which is in concordance with previous transcriptomic analysis of P. salmonis, where these mobile elements were reported (Nourdin-Galindo et al., 2017; Ortiz-Severin et al., 2019; Valenzuela-Miranda et al., 2020; Levipan et al., 2022). Therefore, the high transcriptional activity of transposases may reflect the stimulus of the environment by using salmon as a living culture broth.
Likewise, the gene encoding the flagellar protein export ATPase FliI was overexpressed during co-culture compared to Psal-182, even though this bacterium is non-motile. However, flagellin monomer synthesis is a well-known virulence effector (Zuniga et al., 2019; Rozas-Serri, 2022). This suggests that FliI can be used for immunomodulation and infection in the host (Carril et al., 2017). Similarly, the flagellar hook-associated protein FlgK, which is required for cell adhesion/invasion in Cronobacter sakazakii was upregulated (Li et al., 2023). Another relevant finding is the thiol reductant ABC exporter subunit cydD used for cysteine/glutathione transporter, which is key in the maintenance of redox homeostasis and for correct disulfide bond folding (Shepherd, 2015). This is required for many extra cytoplasmic virulence factors such as the flagellar P-ring motor protein FlgI (Dailey and Berg, 1993), which should act along with FlgK in flagellar assembly. In addition, FlgK is well-characterized in the establishment of pathogenic bacterial infections, to cope with nitric oxide (NO) (Holyoake et al., 2015). The upregulation of NCS2 family permease requires new hypotheses for its function in P. salmonis. These molecules have nucleobase transmembrane transporter activity, which has been shown to contribute to virulence in Francisella tularensis through attenuated mutants (Meibom and Charbit, 2010), suggesting a role for host colonization during infection.
In co-cultures, compared to Psal-182, the downregulation of the P-II family nitrogen regulator was interesting because this gene is related to nitrogen metabolism control and transcriptional factor activity (Huergo et al., 2013). This suggests a possible mechanism for modulating gene expression during the early stages of infection. In addition, the downregulation of the ATP-dependent Clp protease involved in the proteolysis of defective and misfolded proteins (Aljghami et al., 2022) can be associated with the increased transcription of flagellin-related genes, similar to that described in mutants of S. typhimurium with a clpXP deletion (Tomoyasu et al., 2002). Moreover, it has been found to be modulated in P. salmonis during SHK-1 cell infection (Figueroa et al., 2021).
When comparing the in vivo Psal-182 and Psal-013 monocultures, the ABC transporter permease related to the production of siderophores and iron used by P. salmonis (Pulgar et al., 2015; Calquin et al., 2018) was upregulated, supporting its importance for infection in Atlantic salmon. This has been described in F. tularensis as being directly related to virulence (Meibom and Charbit, 2010). In addition, in the same comparison, FAD-dependent oxidoreductase MnmC was upregulated. This gene is needed for correct post-transcriptional modifications of tRNA that allows accurate reading of specific mRNA codons during translation on the ribosome (Kitamura et al., 2012; Moukadiri et al., 2018), with a transcription regulatory function. This can also be linked to the upregulation of the same family of transposases during co-culture (IS4 and IS30 from chromosome, plasmid 4, and plasmid 2 of Psal-182), contributing to the relevance of plasmids for virulence.
For the LF-89-like isolate (Psal-013) during co-cultures, there was another set of upregulated genes. For instance, the type II toxin-antitoxin system RelE/ParE family, referred to as an mRNA interferase or inhibitor of protein synthesis, has been linked to the reversible inhibition of cellular metabolism in response to cellular stress (Muthuramalingam et al., 2019). The HigA family antitoxin, that functions as a transcriptional regulator related to virulence factors, such as siderophores, and biofilm production in Pseudomonas aeruginosa (Wood and Wood, 2016). Both of these genes are located in plasmid 1 of Psal-013, similar to that described for toxin–antitoxin (TA) systems in other bacteria (Kamruzzaman et al., 2021). This is in concordance with a previous study on P. salmonis LF-89 plasmids, which suggests that TA systems contribute to the heritability of the plasmids and carry virulence factors (Ortiz-Severin et al., 2019). Another upregulated DEG in the same plasmid was the DUF1561 family protein, which was described as part of Virulence-Modifying (VM) proteins in Leptospira, and may be involved in the secretion of exotoxins with virulence effects (Vieira et al., 2023).
The upregulation of cadherin-like domain-containing proteins is relevant, since it is pivotal for adherence to host cells, mediating a well-studied host–pathogen interaction (Dash et al., 2021) for P. salmonis during biofilm formation (Levipan et al., 2022). This could be a key virulence factor for invasion, which was only upregulated by Psal-013 when cohabiting with Psal-182. Additionally, the genes encoding the flagellar hook-associated protein FlgK, the thiol reductant ABC exporter subunit CydD, and the NCS2 family permease were also upregulated for Psal-013 during the in vivo co-culture, along with the downregulation of the Clp protease. Their increased expression in vitro indicates the importance of these virulence effectors in adhesion or early infection processes and is triggered by the cohabitation of both genogroups. Their relevance should be addressed in further studies on the pathogenesis of P. salmonis.
In summary, the gene expression pattern was modulated differently by each genogroup during in vivo co-culture. Psal-013 (LF-89) displayed the expression of more DEGs described as virulence factors than Psal-182 (EM-90) under the co-culture condition, which suggest that the cohabitation might play a role in the pathogenesis of SRS. Therefore, it is relevant to consider these within-host competitive interactions because they are linked to virulence and can also modulate the dynamics of the infection process. The different antigenic epitopes present in this mixed population can trigger a cross-reactive immune response in the host (Balmer and Tanner, 2011; Kinnula et al., 2017), altering the immune efficiency and welfare of fish.
5 Conclusions
In this study, we have shown that the co-habitation of the LF-89 and EM-90 genogroups of P. salmonis induced changes in growth and biofilm production during in vitro co-cultures, indicating a synergistic effect. The differential expression of virulence factors observed under in vivo co-culture may be related to increased pathogenicity to the host. These effects will be studied in further research with co-infection challenges in Atlantic salmon to evaluate the disease dynamics under field conditions. Likewise, we propose new candidate genes used by P. salmonis as virulence effectors (cydD, flgK, and NSC2). Furthermore, we demonstrated that the use of specific primers for RT-qPCR is an efficient strategy for identifying the P. salmonis genogroups. Finally, this approach to SRS as a multiple-genotype infection may offer better insights for proposing novel disease control measures.
Data availability statement
The datasets presented in this study can be found online in Gene Expression Omnibus (NCBI): GSE235725. Supplementary Material can be found in the article.
Ethics statement
The animal study was approved by the Norwegian Food Safety Authorities (Mattilsynet), Norway, FOTS ID 26316. The study was conducted in accordance with the local legislation and institutional requirements.
Author contributions
GC: Conceptualization, Data curation, Formal Analysis, Funding acquisition, Investigation, Methodology, Visualization, Writing – original draft, Writing – review & editing. HW-L: Conceptualization, Methodology, Supervision, Writing – review & editing. ML: Conceptualization, Supervision, Writing – review & editing. HS: Conceptualization, Funding acquisition, Methodology, Project administration, Resources, Supervision, Writing – review & editing.
Funding
This study was funded by the Chilean National Research and Development Agency (ANID) through the Becas Chile program (ANID Scholarship ID 72200497). In addition, Previwo AS (Norway) funded transcriptional studies.
Acknowledgments
The authors thank the NIVA facility staff for their support during the salmon experiment, Kira Salonius (Previwo AS) for her personal contribution on the scientific side, Prof. Sergio Marshall (PUCV) for kindly providing us with the P. salmonis isolates used, and Dr. Byron Morales-Lange (NMBU) for his help during bioinformatics data analysis.
Conflict of interest
The authors declare that the research was conducted in the absence of any commercial or financial relationships that could be construed as a potential conflict of interest.
Publisher’s note
All claims expressed in this article are solely those of the authors and do not necessarily represent those of their affiliated organizations, or those of the publisher, the editors and the reviewers. Any product that may be evaluated in this article, or claim that may be made by its manufacturer, is not guaranteed or endorsed by the publisher.
Supplementary material
The Supplementary Material for this article can be found online at: https://www.frontiersin.org/articles/10.3389/fcimb.2023.1253577/full#supplementary-material
References
Albornoz, R., Valenzuela, K., Pontigo, J. P., Sanchez, P., Ruiz, P., Avendano-Herrera, R., et al. (2017). Identification of chemotaxis operon cheYZA and cheA gene expression under stressful conditions in Piscirickettsia salmonis. Microb. Pathog. 107, 436–441. doi: 10.1016/j.micpath.2017.04.030
Aljghami, M. E., Barghash, M. M., Majaesic, E., Bhandari, V., Houry, W. A. (2022). Cellular functions of the ClpP protease impacting bacterial virulence. Front. Mol. Biosci. 9. doi: 10.3389/fmolb.2022.1054408
Aravena, P., Pulgar, R., Ortiz-Severin, J., Maza, F., Gaete, A., Martinez, S., et al. (2020). PCR-RFLP detection and genogroup identification of piscirickettsia salmonis in field samples. Pathogens 9 (5), 358. doi: 10.3390/pathogens9050358
Balmer, O., Tanner, M. (2011). Prevalence and implications of multiple-strain infections. Lancet Infect. Dis. 11 (11), 868–878. doi: 10.1016/S1473-3099(11)70241-9
Bohle, H., Henriquez, P., Grothusen, H., Navas, E., Sandoval, A., Bustamante, F., et al. (2014). Comparative genome analysis of two isolates of the fish pathogen piscirickettsia salmonis from different hosts reveals major differences in virulence-associated secretion systems. Genome Announc 2 (6), 10–1128. doi: 10.1128/genomeA.01219-14
Bravo, C., Martinez, V. (2016). Whole-genome comparative analysis of the pathogen Piscirickettsia salmonis. Vet. Microbiol. 196, 36–43. doi: 10.1016/j.vetmic.2016.10.015
Brocklebank, J. R., Speare, D. J., Armstrong, R. D., Evelyn, T. (1992). British-columbia - septicemia suspected to be caused by a rickettsia-like agent in farmed atlantic salmon. Can. Veterinary Journal-Revue Veterinaire Can. 33 (6), 407–408.
Calquin, P., Ruiz, P., Oliver, C., Sanchez, P., Haro, R., Oliva, H., et al. (2018). Physiological evidence that Piscirickettsia salmonis produces siderophores and uses iron from different sources. J. Fish Dis. 41 (3), 553–558. doi: 10.1111/jfd.12745
Carril, G. P., Gomez, F. A., Marshall, S. H. (2017). Expression of flagellin and key regulatory flagellar genes in the non-motile bacterium Piscirickettsia salmonis. Dis. Aquat Organ 123 (1), 29–43. doi: 10.3354/dao03079
Casanova, A., Obreque, C. J., Gaggero, A., Landskron, E., Sandino, G. A., Jashes, M. M. (2003). Electrophoretic analysis of ITS from Piscirickettsia salmonis Chilean isolates. FEMS Microbiol. Lett. 225 (2), 173–176. doi: 10.1016/S0378-1097(03)00489-0
Colquhoun, D. J., Sørum, H. N. (1998). Outer membrane protein expression during in vivo cultivation of Vibrio salmonicida. Fish Shellfish Immunol. 8 (5), 367–377. doi: 10.1006/fsim.1998.0147
Dailey, F. E., Berg, H. C. (1993). Mutants in disulfide bond formation that disrupt flagellar assembly in Escherichia coli. Proc. Natl. Acad. Sci. U.S.A. 90 (3), 1043–1047. doi: 10.1073/pnas.90.3.1043
Dash, S., Duraivelan, K., Samanta, D. (2021). Cadherin-mediated host-pathogen interactions. Cell Microbiol. 23 (5), e13316. doi: 10.1111/cmi.13316
De Palmenaer, D., Siguier, P., Mahillon, J. (2008). IS4 family goes genomic. BMC Evol. Biol. 8, 18. doi: 10.1186/1471-2148-8-18
Duval-Valentin, G., Normand, C., Khemici, V., Marty, B., Chandler, M. (2001). Transient promoter formation: a new feedback mechanism for regulation of IS911 transposition. EMBO J. 20 (20), 5802–5811. doi: 10.1093/emboj/20.20.5802
Figueroa, C., Torrealba, D., Morales-Lange, B., Mercado, L., Dixon, B., Conejeros, P., et al. (2022). Commercial Vaccines Do Not Confer Protection against Two Genogroups of Piscirickettsia salmonis, LF-89 and EM-90, in Atlantic Salmon. Biology-Basel 11 (7), 993. doi: 10.3390/biology11070993
Figueroa, J., Villagran, D., Cartes, C., Solis, C., Nourdin-Galindo, G., Haussmann, D. (2021). Analysis of genes encoding for proteolytic enzymes and cytotoxic proteins as virulence factors of Piscirickettsia salmonis in SHK-1 cells. J. Fish Dis. 44 (5), 495–504. doi: 10.1111/jfd.13333
Fryer, J. L., Lannan, C. N., Giovannoni, S. J., Wood, N. D. (1992). Piscirickettsia salmonis gen. nov., sp. nov., the causative agent of an epizootic disease in salmonid fishes. Int. J. Syst. Bacteriol 42 (1), 120–126. doi: 10.1099/00207713-42-1-120
Gomez, F. A., Tobar, J. A., Henriquez, V., Sola, M., Altamirano, C., Marshall, S. H. (2013). Evidence of the presence of a functional Dot/Icm type IV-B secretion system in the fish bacterial pathogen Piscirickettsia salmonis. PloS One 8 (1), e54934. doi: 10.1371/journal.pone.0054934
Grant, A. N., Brown, A. G., Cox, D. I., Birkbeck, T. H., Griffen, A. A. (1996). Rickettsia-like organism in farmed salmon. Veterinary Rec. 138 (17), 423–423.
Hjerde, E., Karlsen, C., Sørum, H., Parkhill, J., Willassen, N. P., Thomson, N. R. (2015). Co-cultivation and transcriptome sequencing of two co-existing fish pathogens Moritella viscosa and Aliivibrio wodanis. BMC Genomics 16, 1–13. doi: 10.1186/s12864-015-1669-z
Holyoake, L. V., Poole, R. K., Shepherd, M. (2015). The cydDC family of transporters and their roles in oxidase assembly and homeostasis. Adv. Microb. Physiol. 66, 1–53. doi: 10.1016/bs.ampbs.2015.04.002
Huergo, L. F., Chandra, G., Merrick, M. (2013). P(II) signal transduction proteins: nitrogen regulation and beyond. FEMS Microbiol. Rev. 37 (2), 251–283. doi: 10.1111/j.1574-6976.2012.00351.x
Isla, A., Saldarriaga-Cordoba, M., Fuentes, D. E., Albornoz, R., Haussmann, D., Mancilla-Schulz, J., et al. (2019). Multilocus sequence typing detects new Piscirickettsia salmonis hybrid genogroup in Chilean fish farms: Evidence for genetic diversity and population structure. J. Fish Dis. 42 (5), 721–737. doi: 10.1111/jfd.12976
Kamruzzaman, M., Wu, A. Y., Iredell, J. R. (2021). Biological functions of type II toxin-antitoxin systems in bacteria. Microorganisms 9 (6), 1276. doi: 10.3390/microorganisms9061276
Kinnula, H., Mappes, J., Sundberg, L. R. (2017). Coinfection outcome in an opportunistic pathogen depends on the inter-strain interactions. BMC Evol. Biol. 17 (1), 77. doi: 10.1186/s12862-017-0922-2
Kitamura, A., Nishimoto, M., Sengoku, T., Shibata, R., Jager, G., Bjork, G. R., et al. (2012). Characterization and structure of the Aquifex aeolicus protein DUF752: a bacterial tRNA-methyltransferase (MnmC2) functioning without the usually fused oxidase domain (MnmC1). J. Biol. Chem. 287 (52), 43950–43960. doi: 10.1074/jbc.M112.409300
Kotob, M. H., Menanteau-Ledouble, S., Kumar, G., Abdelzaher, M., El-Matbouli, M. (2016). The impact of co-infections on fish: a review. Vet. Res. 47 (1), 98. doi: 10.1186/s13567-016-0383-4
Levipan, H. A., Irgang, R., Opazo, L. F., Araya-Leon, H., Avendano-Herrera, R. (2022). Collective behavior and virulence arsenal of the fish pathogen Piscirickettsia salmonis in the biofilm realm. Front. Cell Infect. Microbiol. 12. doi: 10.3389/fcimb.2022.1067514
Levipan, H. A., Irgang, R., Yanez, A., Avendano-Herrera, R. (2020). Improved understanding of biofilm development by Piscirickettsia salmonis reveals potential risks for the persistence and dissemination of piscirickettsiosis. Sci. Rep. 10 (1), 12224. doi: 10.1038/s41598-020-68990-4
Li, P., Zong, W., Zhang, Z., Lv, W., Ji, X., Zhu, D., et al. (2023). Effects and molecular mechanism of flagellar gene flgK on the motility, adhesion/invasion, and desiccation resistance of Cronobacter sakazakii. Food Res. Int. 164, 112418. doi: 10.1016/j.foodres.2022.112418
Livak, K. J., Schmittgen, T. D. (2001). Analysis of relative gene expression data using real-time quantitative PCR and the 2(-Delta Delta C(T)) Method. Methods 25 (4), 402–408. doi: 10.1006/meth.2001.1262
Long, A., Garver, K. A., Jones, S. R. M. (2019). Synergistic osmoregulatory dysfunction during salmon lice (Lepeophtheirus salmonis) and infectious hematopoietic necrosis virus co-infection in sockeye salmon (Oncorhynchus nerka) smolts. J. Fish Dis. 42 (6), 869–882. doi: 10.1111/jfd.12989
Long, A., Jones, S. R. M. (2021). Piscirickettsia salmonis shedding and tissue burden, and hematological responses during cohabitation infections in chum Oncorhynchus keta, pink O. gorbuscha and Atlantic salmon Salmo salar. PloS One 16 (3), e0248098. doi: 10.1371/journal.pone.0248098
Lysnyansky, I., Calcutt, M. J., Ben-Barak, I., Ron, Y., Levisohn, S., Methe, B. A., et al. (2009). Molecular characterization of newly identified IS3, IS4 and IS30 insertion sequence-like elements in Mycoplasma bovis and their possible roles in genome plasticity. FEMS Microbiol. Lett. 294 (2), 172–182. doi: 10.1111/j.1574-6968.2009.01562.x
Maisey, K., Montero, R., Christodoulides, M. (2017). Vaccines for piscirickettsiosis (salmonid rickettsial septicaemia, SRS): the Chile perspective. Expert Rev. Vaccines 16 (3), 215–228. doi: 10.1080/14760584.2017.1244483
Mandakovic, D., Glasner, B., Maldonado, J., Aravena, P., Gonzalez, M., Cambiazo, V., et al. (2016). Genomic-Based Restriction Enzyme Selection for Specific Detection of Piscirickettsia salmonis by 16S rDNA PCR-RFLP. Front. Microbiol. 7. doi: 10.3389/fmicb.2016.00643
Marshall, S. H., Gomez, F. A., Ramirez, R., Nilo, L., Henriquez, V. (2012). Biofilm generation by Piscirickettsia salmonis under growth stress conditions: a putative in vivo survival/persistence strategy in marine environments. Res. Microbiol. 163 (8), 557–566. doi: 10.1016/j.resmic.2012.08.002
Mauel, M. J., Giovannoni, S. J., Fryer, J. L. (1999). Phylogenetic analysis of Piscirickettsia salmonis by 16S, internal transcribed spacer (ITS) and 23S ribosomal DNA sequencing. Dis. Aquat Organ 35 (2), 115–123. doi: 10.3354/dao035115
Meibom, K. L., Charbit, A. (2010). Francisella tularensis metabolism and its relation to virulence. Front. Microbiol. 1. doi: 10.3389/fmicb.2010.00140
Morales-Lange, B., Agboola, J. O., Hansen, J. O., Lagos, L., Oyas, O., Mercado, L., et al. (2021). The spleen as a target to characterize immunomodulatory effects of down-stream processed cyberlindnera jadinii yeasts in atlantic salmon exposed to a dietary soybean meal challenge. Front. Immunol. 12. doi: 10.3389/fimmu.2021.708747
Moukadiri, I., Villarroya, M., Benitez-Paez, A., Armengod, M. E. (2018). Bacillus subtilis exhibits MnmC-like tRNA modification activities. RNA Biol. 15 (9), 1167–1173. doi: 10.1080/15476286.2018.1517012
Muthuramalingam, M., White, J. C., Murphy, T., Ames, J. R., Bourne, C. R. (2019). The toxin from a ParDE toxin-antitoxin system found in Pseudomonas aeruginosa offers protection to cells challenged with anti-gyrase antibiotics. Mol. Microbiol. 111 (2), 441–454. doi: 10.1111/mmi.14165
Nourdin-Galindo, G., Sanchez, P., Molina, C. F., Espinoza-Rojas, D. A., Oliver, C., Ruiz, P., et al. (2017). Comparative Pan-Genome Analysis of Piscirickettsia salmonis Reveals Genomic Divergences within Genogroups. Front. Cell Infect. Microbiol. 7. doi: 10.3389/fcimb.2017.00459
Oliver, C., Cespedes, C., Santibanez, N., Ruiz, P., Romero, A. (2023). Subinhibitory concentrations of florfenicol increase the biofilm formation of Piscirickettsia salmonis. J. Fish Dis. 46 (5), 591–596. doi: 10.1111/jfd.13757
Olsen, A. B., Melby, H. P., Speilberg, L., Evensen, Ø., Håstein, T. (1997). Piscirickettsia salmonis infection in Atlantic salmon Salmo salar in Norway - epidemiological, pathological and microbiological findings. Dis. Aquat. Organisms 31 (1), 35–48. doi: 10.3354/dao031035
Ortiz-Severin, J., Stuardo, C. J., Jimenez, N. E., Palma, R., Cortes, M. P., Maldonado, J., et al. (2021). Nutrient scarcity in a new defined medium reveals metabolic resistance to antibiotics in the fish pathogen piscirickettsia salmonis. Front. Microbiol. 12. doi: 10.3389/fmicb.2021.734239
Ortiz-Severin, J., Travisany, D., Maass, A., Chavez, F. P., Cambiazo, V. (2019). Piscirickettsia salmonis cryptic plasmids: source of mobile DNA and virulence factors. Pathogens 8 (4), 734239. doi: 10.3390/pathogens8040269
Otterlei, A., Brevik, Ø. J., Jensen, D., Duesund, H., Sommerset, I., Frost, P., et al. (2016). Phenotypic and genetic characterization of Piscirickettsia salmonis from Chilean and Canadian salmonids. BMC Vet. Res. 12, 55. doi: 10.1186/s12917-016-0681-0
Pulgar, R., Hodar, C., Travisany, D., Zuniga, A., Dominguez, C., Maass, A., et al. (2015). Transcriptional response of Atlantic salmon families to Piscirickettsia salmonis infection highlights the relevance of the iron-deprivation defence system. BMC Genomics 16, 495. doi: 10.1186/s12864-015-1716-9
Reid, H. I., Griffen, A. A., Birkbeck, T. H. (2004). Isolates of Piscirickettsia salmonis from Scotland and Ireland show evidence of clonal diversity. Appl. Environ. Microbiol. 70 (7), 4393–4397. doi: 10.1128/AEM.70.7.4393-4397.2004
Rodger, H. D., Drinan, E. M. (1993). Observation of a rickettsia-like organism in atlantic salmon, salmo-salar L, in Ireland. J. Fish Dis. 16 (4), 361–369. doi: 10.1111/j.1365-2761.1993.tb00869.x
Rojas, V., Galanti, N., Bols, N. C., Jimenez, V., Paredes, R., Marshall, S. H. (2010). Piscirickettsia salmonis induces apoptosis in macrophages and monocyte-like cells from rainbow trout. J. Cell Biochem. 110 (2), 468–476. doi: 10.1002/jcb.22560
Rozas-Serri, M. (2022). Why does piscirickettsia salmonis break the immunological paradigm in farmed salmon? Biological context to understand the relative control of piscirickettsiosis. Front. Immunol. 13. doi: 10.3389/fimmu.2022.856896
Rozas-Serri, M., Ildefonso, R., Pena, A., Enriquez, R., Barrientos, S., Maldonado, L. (2017). Comparative pathogenesis of piscirickettsiosis in Atlantic salmon (Salmo salar L.) post-smolt experimentally challenged with LF-89-like and EM-90-like Piscirickettsia salmonis isolates. J. Fish Dis. 40 (10), 1451–1472. doi: 10.1111/jfd.12671
Rozas-Serri, M., Pena, A., Gardner, I., Penaloza, E., Maldonado, L., Munoz, A., et al. (2023). Co-infection by LF-89-like and EM-90-like genogroups of piscirickettsia salmonis in farmed atlantic salmon in Chile: implications for surveillance and control of piscirickettsiosis. Pathogens 12 (3), 450. doi: 10.3390/pathogens12030450
Ruiz, P., Sepulveda, D., Vidal, J. M., Romero, R., Contreras, D., Barros, J., et al. (2021). Piscirickettsia salmonis produces a N-acetyl-L-homoserine lactone as a bacterial quorum sensing system-related molecule. Front. Cell Infect. Microbiol. 11. doi: 10.3389/fcimb.2021.755496
Saavedra, J., Grandon, M., Villalobos-Gonzalez, J., Bohle, H., Bustos, P., Mancilla, M. (2018). Isolation, Functional Characterization and Transmissibility of p3PS10, a Multidrug Resistance Plasmid of the Fish Pathogen Piscirickettsia salmonis. Front. Microbiol. 9. doi: 10.3389/fmicb.2018.00923
Saavedra, J., Hernandez, N., Osses, A., Castillo, A., Cancino, A., Grothusen, H., et al. (2017). Prevalence, geographic distribution and phenotypic differences of Piscirickettsia salmonis EM-90-like isolates. J. Fish Dis. 40 (8), 1055–1063. doi: 10.1111/jfd.12581
SAG (2022) PRODUCTOS BIOLÓGICOS INMUNOLÓGICOS CON REGISTRO PROVISIONAL USO EN SALMÓNIDOS. Available at: https://www.sag.gob.cl/sites/default/files/lista_salmonidos_registro_provisional_1.pdf (Accessed April 1, 2023).
Santibanez, N., Vega, M., Perez, T., Yanez, A., Gonzalez-Stegmaier, R., Figueroa, J., et al. (2020). Biofilm produced in vitro by piscirickettsia salmonis generates differential cytotoxicity levels and expression patterns of immune genes in the atlantic salmon cell line SHK-1. Microorganisms 8 (10), 1609. doi: 10.3390/microorganisms8101609
Schober, I. (2022). Characteristics of the genome evolution of the bacterial pathogens piscirickettsia and myroides. doi: 10.24355/dbbs.084-202204051327-0
SERNAPESCA (2022a) INFORME SANITARIO CON INFORMACIÓN SANITARIA DE AGUA DULCE Y MAR. Primer Semestre - Año 2022. Available at: http://www.sernapesca.cl/sites/default/files/informe_sanitario_con_informacion_sanitaria_de_agua_dulce_y_marenero-junio-ano_2022.pdf.
SERNAPESCA (2022b). INFORME SOBRE USO DE ANTIMICROBIANOS EN LA SALMONICULTURA NACIONAL. Primer Semestre - Año 2022. Available at: http://www.sernapesca.cl/sites/default/files/informe_sobre_el_uso_de_antimicrobianos_en_la_salmonicultura_nacional_-_primer_semestre_-_ano_2022_v20221026.pdf.
Shepherd, M. (2015). The CydDC ABC transporter of Escherichia coli: new roles for a reductant efflux pump. Biochem. Soc. Trans. 43 (5), 908–912. doi: 10.1042/BST20150098
Siguier, P., Gourbeyre, E., Chandler, M. (2014). Bacterial insertion sequences: their genomic impact and diversity. FEMS Microbiol. Rev. 38 (5), 865–891. doi: 10.1111/1574-6976.12067
Subramoni, S., Venturi, V. (2009). LuxR-family ‘solos’: bachelor sensors/regulators of signalling molecules. Microbiol. (Reading) 155 (Pt 5), 1377–1385. doi: 10.1099/mic.0.026849-0
Sundberg, L. R., Ketola, T., Laanto, E., Kinnula, H., Bamford, J. K., Penttinen, R., et al. (2016). Intensive aquaculture selects for increased virulence and interference competition in bacteria. Proc. Biol. Sci. 283 (1826), 20153069. doi: 10.1098/rspb.2015.3069
Tomoyasu, T., Ohkishi, T., Ukyo, Y., Tokumitsu, A., Takaya, A., Suzuki, M., et al. (2002). The ClpXP ATP-dependent protease regulates flagellum synthesis in Salmonella enterica serovar typhimurium. J. Bacteriol 184 (3), 645–653. doi: 10.1128/JB.184.3.645-653.2002
Tremaroli, V., Fedi, S., Tamburini, S., Viti, C., Tatti, E., Ceri, H., et al. (2011). A histidine-kinase cheA gene of Pseudomonas pseudoalcaligens KF707 not only has a key role in chemotaxis but also affects biofilm formation and cell metabolism. Biofouling 27 (1), 33–46. doi: 10.1080/08927014.2010.537099
Valenzuela-Miranda, D., Valenzuela-Munoz, V., Nunez-Acuna, G., Gallardo-Escarate, C. (2020). Long-term serial culture of Piscirickettsia salmonis leads to a genomic and transcriptomic reorganization affecting bacterial virulence. Aquaculture 529, 735634. doi: 10.1016/j.aquaculture.2020.735634
Varani, A., He, S., Siguier, P., Ross, K., Chandler, M. (2021). The IS6 family, a clinically important group of insertion sequences including IS26. Mob DNA 12 (1), 11. doi: 10.1186/s13100-021-00239-x
Vieira, D. S., Chaurasia, R., Vinetz, J. M. (2023). Comparison of the PF07598-Encoded Virulence-Modifying Proteins of L. interrogans and L. borgpetersenii. Trop. Med. Infect. Dis. 8 (1), 14. doi: 10.3390/tropicalmed8010014
Wei, T., Simko, V. (2017) R Package “Corrplot”: Visualization of a Correlation Matrix (Version 0.84). Available at: https://github.com/taiyun/corrplot.
Wilhelm, V., Miquel, A., Burzio, L. O., Rosemblatt, M., Engel, E., Valenzuela, S., et al. (2006). A vaccine against the salmonid pathogen Piscirickettsia salmonis based on recombinant proteins. Vaccine 24 (23), 5083–5091. doi: 10.1016/j.vaccine.2006.03.027
Wood, T. L., Wood, T. K. (2016). The HigB/HigA toxin/antitoxin system of Pseudomonas aeruginosa influences the virulence factors pyochelin, pyocyanin, and biofilm formation. Microbiologyopen 5 (3), 499–511. doi: 10.1002/mbo3.346
Yanez, A. J., Valenzuela, K., Silva, H., Retamales, J., Romero, A., Enriquez, R., et al. (2012). Broth medium for the successful culture of the fish pathogen Piscirickettsia salmonis. Dis. Aquat Organ 97 (3), 197–205. doi: 10.3354/dao02403
Yang, Q., Defoirdt, T. (2015). Quorum sensing positively regulates flagellar motility in pathogenic Vibrio harveyi. Environ. Microbiol. 17 (4), 960–968. doi: 10.1111/1462-2920.12420
Zuniga, A., Aravena, P., Pulgar, R., Travisany, D., Ortiz-Severin, J., Chavez, F. P., et al. (2019). Transcriptomic changes of piscirickettsia salmonis during intracellular growth in a salmon macrophage-like cell line. Front. Cell Infect. Microbiol. 9. doi: 10.3389/fcimb.2019.00426
Keywords: intracellular pathogen, Piscirickettsiosis, SRS, co-infection, virulence factor, biofilm, Atlantic salmon
Citation: Carril G, Winther-Larsen HC, Løvoll M and Sørum H (2023) Cohabitation of Piscirickettsia salmonis genogroups (LF-89 and EM-90): synergistic effect on growth dynamics. Front. Cell. Infect. Microbiol. 13:1253577. doi: 10.3389/fcimb.2023.1253577
Received: 05 July 2023; Accepted: 25 September 2023;
Published: 25 October 2023.
Edited by:
Felix Acosta, University of Las Palmas de Gran Canaria, SpainReviewed by:
Kim Dawn Thompson, Moredun Research Institute, United KingdomAudrey Chong, National Institute of Allergy and Infectious Diseases (NIH), United States
Copyright © 2023 Carril, Winther-Larsen, Løvoll and Sørum. This is an open-access article distributed under the terms of the Creative Commons Attribution License (CC BY). The use, distribution or reproduction in other forums is permitted, provided the original author(s) and the copyright owner(s) are credited and that the original publication in this journal is cited, in accordance with accepted academic practice. No use, distribution or reproduction is permitted which does not comply with these terms.
*Correspondence: Gabriela Carril, Z2FicmllbGEucGF6LmNhcnJpbC5sZWl2YUBubWJ1Lm5v; Henning Sørum, aGVubmluZy5zb3J1bUBubWJ1Lm5v