- Division of Agricultural Microbiology, National Institute of Agricultural Sciences, Rural Development Administration, Wanju, Republic of Korea
Acidovorax citrulli is a seed-borne bacterial pathogen that causes bacterial fruit blotch in cucurbits and severely affects the production of cucumbers and watermelons globally. In this study, we investigated the effects of di-(2-ethylhexyl) phthalate (DEHP) on the growth, metabolism, and virulence of A. citrulli. Bacterial population was not affected by DEHP exposure; moreover, significant changes were not observed in lipid peroxidation, membrane permeability, and nucleic acid leakage. However, palmitoleic acid content was increased in the cell membrane of DEHP-exposed A. citrulli. Further, DEHP exposure increased the activity of TCA cycle-related enzymes, including α-ketoglutarate dehydrogenase and succinyl-CoA synthetase, along with increase in the content of glutamate, succinate, fumarate, and malate in TCA cycle. Additionally, total 270 genes were differentially expressed by the treatment, of which 28 genes were upregulated and 242 genes, including those related to translation, flagellum-dependent cell motility, and flagellum assembly, were downregulated. Regarding virulence traits, swimming activity was decreased in DEHP-exposed A. citrulli; however, biofilm formation was not affected in in vitro assay. Moreover, relative expression of pathogenicity genes, including hrpX and hrpG, were decreased in DEHP-exposed A. citrulli compared to that of unexposed A. citrulli. Therefore, these results suggest that DEHP accumulation in soil could potentially influence the metabolism and virulence traits of A. citrulli.
Introduction
Phthalates are a group of chemical compounds that are commonly used as plasticizers for flexibility and durability in various other products, including adhesives, sealants, and personal care products (Gao and Wen, 2016). Phthalates are toxic to the environment because they are non-biodegradable and persist in the environment for a long time. Owing to their extensive presence, they can accumulate in the food chain and cause potentially harmful effects on wildlife and humans (He et al., 2013; Groh et al., 2019; Li et al., 2021). Studies have shown that phthalates can disrupt hormone function in both animals and humans, leading to developmental and reproductive problems. For example, exposure to phthalates during pregnancy is associated with various adverse effects on fetal development, including low birth weight, developmental delays, and changes in genital development. In addition to their effects on human health, phthalates have negative impact on the environment (Groh et al., 2019). They can leach from plastic products, contaminate soil and water, and have been found at high levels in some aquatic ecosystems (Li et al., 2021). Studies have shown that phthalates affect the growth and survival of certain organisms (Liu et al., 2009; Kim et al., 2019; Li et al., 2021). Owing to their potentially harmful effects on human health and environment, some phthalates have been restricted or banned in certain countries. However, they are still widely used in many products globally.
Di-(2-ethylhexyl) phthalate (DEHP) is commonly used in various products, including vinyl flooring, medical devices, food packaging, and toys. However, DEHP has been found to have toxic effects on both humans and environment. Studies have shown that DEHP exposure can cause reproductive and developmental problems as well as liver and kidney damage. In addition, DEHP has a negative impact on the environment (Chen et al., 2011; Shen et al., 2015) because it is non-biodegradable and can persist in the environment for a long time. Additionally, it can contaminate soil and water, and has been found at high levels in some ecosystems.
Furthermore, DEHP can negatively affect soil biology (Wang et al., 2017; Gao et al., 2020b). When it is released into the soil, it can remain and accumulate the soil over long time, leading to potentially harmful effects on soil microorganisms and other biota (Jiang et al., 2022). Studies have shown that DEHP can affect the soil microbial activity, diversity, and composition of soil microbial community (Gao et al., 2020a). For example, Wang et al. (2009) found that DEHP exposure led to a decrease in soil microbial biomass and activity as well as changes in the relative abundance of certain bacterial groups; Gao et al. (2020b) suggested that DEHP could potentially affect soil nutrient cycling and plant growth, thereby indirectly affecting the soil biota. Additionally, DEHP exposure reduced soil nitrogen availability, leading to decreased plant growth and biomass (Tao et al., 2021). These studies demonstrate that DEHP exposure can have significant effects on soil bacterial biology, potentially altering soil nutrient cycling and affecting plant growth and health.
Acidovorax citrulli is a gram-negative bacterium that causes bacterial fruit blotch (BFB) disease in cucurbit plants such as watermelon, cantaloupe, and cucumber (Burdman and Walcott, 2012). This disease is frequently outbreaks around the world, resulting in significant economic loss by reducing crop yields, decreasing fruit quality, and increasing postharvest loss (Burdman and Walcott, 2012; Zhao et al., 2020). BFB is characterized by water-soaked lesions on fruits and leaves, which can progress into brown, necrotic areas (Burdman and Walcott, 2012). This bacterium can also infect seeds, leading to seedling rot, and eventually plant death. Infected fruits become inedible because of the presence of bacteria and the development of secondary fungal infections. A. citrulli possesses several virulence factors, including exopolysaccharides (EPS), which are sticky, carbohydrate-based substances that enable it to adhere to the plant, colonize host plant tissues, and cause disease in host plants (Burdman and Walcott, 2012). EPS also protects the bacterium from the host plant immune system and helps it form biofilm on plant surfaces, which can increase its resistance to environmental stressors. The type III secretion system (T3SS), a specialized protein secretion system, is used by the bacterium to inject effector proteins into the host plant cells. These effector proteins can manipulate the host cell signaling pathways, suppress the host plant immune response, and enable bacteria to extract nutrients from the host. Moreover, A. citrulli produces extracellular enzymes, such as pectinases and cellulases, which allow the bacterium to break down plant cell wall components and access nutrients. Additionally, the bacterium can produce siderophores, specialized molecules that enable it to scavenge iron from the host plant. These virulence factors enable A. citrulli to colonize and cause disease in the host plants, making it an important pathogen in cucurbit crops.
There is currently no evidence of a direct relationship between DEHP and phytopathogenic bacteria. However, DEHP persists in soils, and exposure may affect phytopathogens, including their population, metabolism, and virulence. Therefore, this study was aimed at evaluating the toxic effects of DEHP to A. citrulli, exploring its effects on metabolism and virulence of the bacterium, and investigating changes in gene expression following DEHP exposure. Understanding the potential impact of DEHP exposure on the seed-borne plant pathogenic bacteria A. citrulli is important for assessing the risk and control of BFB in agriculture.
Materials and methods
Bacterial strain preparation
The bacterial strain Acidovorax citrulli (KACC no. 17002) used in this study was provided by the Korean Agricultural Culture Collection. To prepare the bacterial suspension, A. citrulli was grown on nutrient agar (NA, Difco, Detroit, MI, USA) at 28°C for 48 h, followed by culture of single colony in nutrient broth (NB) at 28°C for 48 h.
Bacterial culture and population in a medium containing di-(2-ethylhexyl) phthalate
The DEHP (200 mg/10 mL in 80% acetone, as a stock) was amended with diluted NB (1:10) media at 0.5% of the final volume. A. citrulli (3.6 × 108 colony forming units (CFU)/mL, 1 mL) was grown in 100 mL of diluted NB supplemented with 0.01% Tween 20 and various concentrations (10, 20, 40, 60, 80, and 100 mg/L) of DEHP at 160 rpm and 28°C for 48 h. Diluted NB supplemented with 0.01% (v/v) of Tween 20 and 0.5% (v/v) of 80% acetone was used as control. After incubation, cell cultures were serially diluted in 0.85% saline solution and incubated onto diluted NA. After incubation at 28°C for 48 h, bacterial CFU were counted. The experiment was conducted twice with five replicates each. For further study, A. citrulli was cultured in diluted NB (1:10) supplemented with 0.01% Tween 20 and DEHP at a final concentration of 20 mg/L based on the amount remaining in the soil (Liu et al., 2019) at 160 rpm and 28°C for 48 h.
Lipid peroxidation assay
Bacterial lipid peroxidation after DEHP exposure was measured using a lipid hydroperoxide assay kit according to the manufacturer’s instructions (Cayman Chemical Co., MI, USA). A. citrulli suspension was prepared as described above; the cells were harvested by centrifugation at 13,000 rpm for 10 min at 4°C and used for the lipid peroxidation assay. The absorbance was measured at 500 nm using a microplate reader (Infinite M200 Pro, Tecan, Switzerland). The experiment was conducted twice with ten replicates each.
Membrane permeability
Bacterial membrane permeability assay was performed according to the method described by Loh et al. (1984). The bacterial cells were prepared as described above; cell pellets were resuspended in 5 mM HEPES buffer (pH 7.2), and the concentration was adjusted to an optical density (OD)600 of 0.5. Further, 50 µL of 1-N-phenylnaphthylamine (NPN; 40 µM stock) and 50 µL of 5 mM HEPES buffer (pH 7.2) containing 5 mM glucose and 1 mM sodium azide were added to 100 µL of bacterial suspension (A1). A solution containing 50 µL of NPN (40 μM stock) and 150 µL of 5 mM HEPES buffer (pH 7.2) (A0) was used as background. The fluorescence was immediately measured using Hidex Sense microplate reader (Hidex, Finland) at an excitation and emission wavelength of 355 nm and 405 nm, respectively; slit width was set to 5 nm. The experiment was conducted twice with ten replicates each.
Nucleic acid leakage
The bacterial cells were harvested in the logarithmic phase by centrifugation at 4,000 rpm for 10 min, washed twice with 0.85% saline solution, resuspended in 0.85% saline solution, and added to 20 mg/L of DEHP at 160 rpm and 28°C for 3 h. The samples were harvested by centrifugation at 4,000 rpm for 10 min, and absorbance of the supernatant was measured at 260 nm. The experiment was conducted twice with three replicates each (Fu et al., 2008).
Fatty acid methyl esters
The bacterial cells were harvested by centrifugation at 13,000 rpm for 10 min, and the sample and internal standard (pentadecanoic acid; Sigma, USA) were placed in tubes with teflon-lined caps. A methylation mixture containing methanol: benzene: 2,2-dimethoxy-propane (DMP): H2SO4 (39: 20: 5: 2) was used, and a mixture of heptane was added to the sample. Afterwards, the tube was placed in a water bath at 80°C. The supernatants were determined by gas chromatography (Agilent 7890A, Agilent Technologies, USA) equipped with a column (DB-23, 120 mm × 0.25 mm × 0.25 μm) and a flame ionization detector. The temperature of the injector and detector was set at 250°C and 280°C, respectively. The experiment was conducted in triplicate (Garcés and Mancha, 1993).
ATPase activity
ATPase activity assay was performed using an ATPase/GTPase activity assay kit according to the manufacturer’s instructions (MAK113, Sigma). A. citrulli was prepared as described above; the cells were harvested by centrifugation at 13,000 rpm for 10 min at 4°C, and the pellets were suspended in assay buffer [40 mM Tris buffer (pH 7.5), 80 mM NaCl, 8 mM MgAc2, 1 mM EDTA]. Ten µL of 4 mM ATP was added to 20 µL of cell suspension, and the cells were incubated for 30 min at 25°C. Subsequently, 200 µL of reagent was added to each well, and the samples were incubated for another 30 min at room temperature. Their absorbance was measured at 620 nm using a microplate reader. The experiment was conducted twice with five replicates each.
Tricarboxylic acid cycle
The bacterial cells were cultured and harvested as described above. Quantitative analyses of citrate, glutamate, oxaloacetate, pyruvate, isocitrate, malate, fumarate, succinate, and alpha-ketoglutarate (α-KG) were performed using citrate (MAK057, Sigma), glutamate (MAK004, Sigma), oxaloacetate (MAK070, Sigma), pyruvate (MAK071, Sigma), isocitrate (MAK061, Sigma), malate (MAK067, Sigma), fumarate (MAK060, Sigma), succinate colorimetric (MAK184, Sigma), and α-KG (MAK054, Sigma) assay kit, respectively. Succinate dehydrogenase activity was analyzed using a succinate dehydrogenase assay kit (MAK197; Sigma-Aldrich). The experiment was conducted twice with five replicates each.
RNA sequencing and quantitative real-time polymerase chain reaction
A. citrulli was prepared as described above; the cell pellets were harvested by centrifugation at 13,000 rpm for 10 min at 4°C. For RNA extraction, total RNA was extracted by using Total RNA extraction kit (iNtRON, South Korea). After quantification using Nanodrop (ND-1000, Thermo Scientific, USA), total RNA was analyzed by Macrogen Inc. (South Korea) for RNA-seq. Differentially expressed genes (DEGs) between DEHP-treated and untreated cells were analyzed using the DESeq2 R library. DEG gene lists were independently submitted for functional annotation using the Database for Annotation Visualization and Integrated Discovery (DAVID). Each sample was assayed in triplicate. Raw reads were deposited in the Sequence Read Archive (SRA) database of NCBI under BioProject accession number PRJNA1002002. For quantitative real-time PCR validation of RNA-seq, 0.6 μg of total RNA was synthesized to complementary DNA using TOPscript RT DryMIX (Enzynomics, South Korea), and a CFX96 Real-time PCR Detection system (Bio-Rad, USA) and primers were used (Supplementary Table S1). The real-time PCR was conducted as follows: 95°C for 10 min; 40 cycles of 95°C for 15 s, 58°C for 20 s, and 72°C for 18 s. As reference primer, rpoB was used. For relative expression of pathogenicity-related genes using qRT-PCR, the synthesized cDNA was used, and relative quantities of specific transcripts were determined using SYBR Green RT-PCR reagents. The primers used for qRT-PCR are listed in Table 1, and the qRT-PCR was performed as follows: 95°C for 10 min, followed by 40 cycles at 95°C for 15 s, 56°C for 30 s, and 72°C for 30 s. The relative expression of genes of interest was calculated using the 2-△△CT method (Livak and Schmittgen, 2001). The experiment was conducted twice with five replicates each.
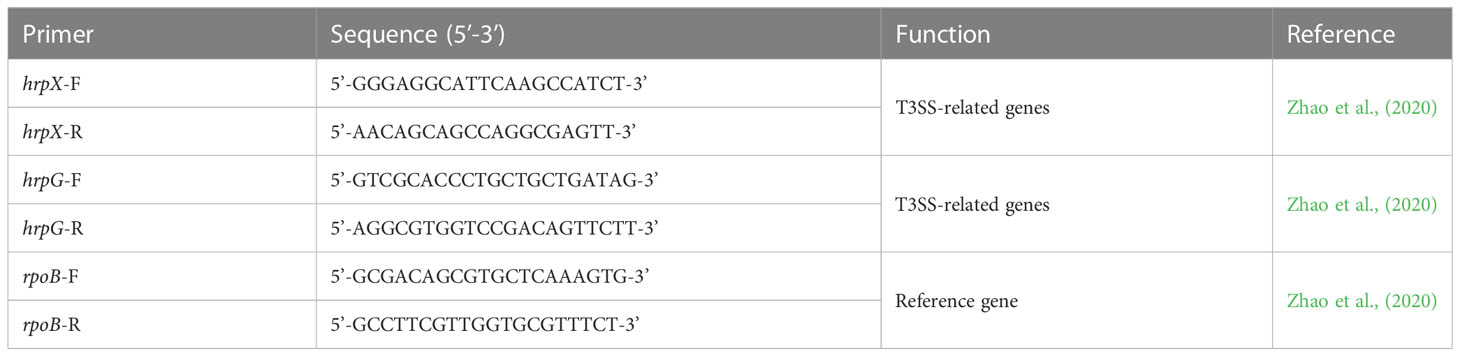
Table 1 Primers used in this study for evaluating relative gene expression of virulence genes of Acidovorax citrulli.
Swimming motility assay and biofilm formation
Swimming motility assay was performed on NB containing 0.2% agar (SHOWA, Japan) using the method described by Rashid and Kornberg (2000) with slight modification. The bacterial cells were prepared as described above, suspended in 0.85% NaCl, and 2 µL of bacterial suspension (104 CFU/mL) was inoculated into NB. The cells were incubated at 28°C, and the colony diameter was measured for consecutive three days after inoculation. The experiment was conducted twice with five replicates each.
For biofilm formation assay, the bacterial cell pellets, prepared as described above, were adjusted to 104 CFU/mL by dilution with NB. The cell suspension (130 µL) was added into a 96-well polyvinyl chloride plate and incubated (Falcon 353911, Corning, USA) at 28°C for three days; subsequently, the bacterial suspension was removed and washed three times with sterile distilled water. Crystal violet (0.1%) was added to the wells for staining the cells, which were then incubated for 30 min at room temperature, and then washed with sterile distilled water. The stained cells were suspended in 95% ethanol for 30 min, and their absorbance was measured at 590 nm using a microplate reader (Coffey and Anderson, 2014). The experiment was conducted twice with five replicates each; each replicate was performed in five wells.
Statistical analyses
Data analyses, including the calculation of average values, standard errors, analysis of variance (ANOVA), and least significant difference (LSD) test at P < 0.05, were performed using R (version 1.4.1106, USA).
Results
Effect of di-(2-ethylhexyl) phthalate on the bacterial population and membrane of Acidovorax citrulli
The population of A. citrulli was not affected by various concentrations (0, 10, 20, 40, 60, 80, and 100 mg/L) of DEHP present in the NB (Figure 1). To examine the effects of DEHP exposure on the membrane of A. citrulli, malondialdehyde (MDA) content, membrane permeability, and nucleic acid leakage were evaluated (Table 2). DEHP treatment tended to increase lipid peroxidation, membrane permeability, and nucleic acid leakage, however, significant differences were not observed (Table 2). The membrane of A. citrulli is mainly comprised of saturated fatty acids, including C12:0 (lauric acid), C14:0 (myristic acid), C16:0 (palmitic acid), C17:0 (margaric acid), and C18:0 (stearic acid), and unsaturated fatty acids, including C16:1 (palmitoleic acid) and C18:1n9c (oleic acid); the amount of fatty acids in the membrane of DEHP-exposed A. citrulli increased compared to that of unexposed, and therefore, the ratio of fatty acid content of exposed and unexposed cells was more than 1.0 (Table 3). Notably, the content of C16:0 and C16:1 were increased, whereas significant increase was observed in only that of C16:1 in response to DEHP exposure (Table 3).
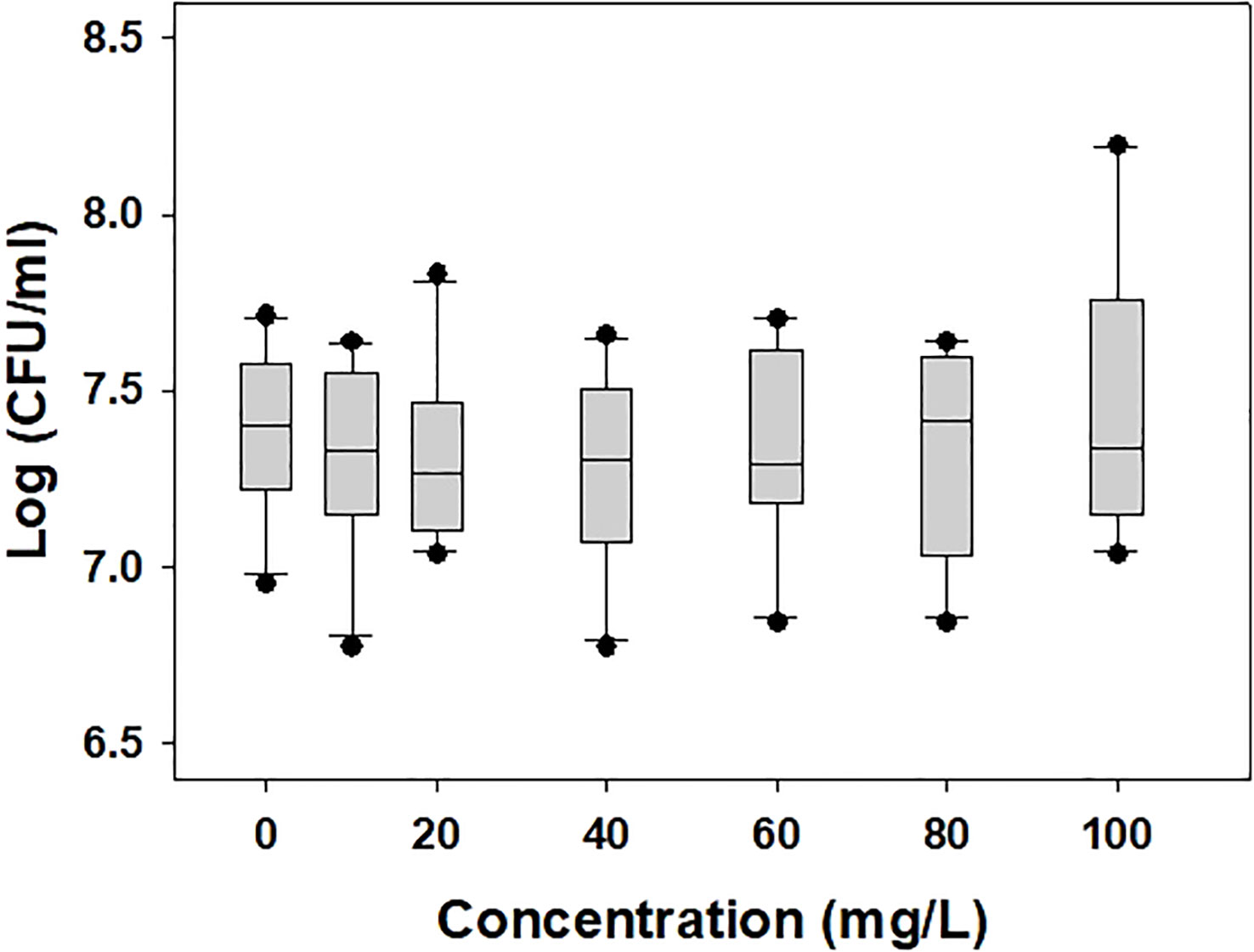
Figure 1 Bacterial population of di-(2-ethylhexyl) phthalate-exposed Acidovorax citrulli. No statistically significant differences were observed in LSD test (P < 0.05).

Table 2 Lipid peroxidation, membrane permeability, and nucleic acid leakage in di(2-ethylhexyl) phthalate-exposed and unexposed Acidovorax citrulli.
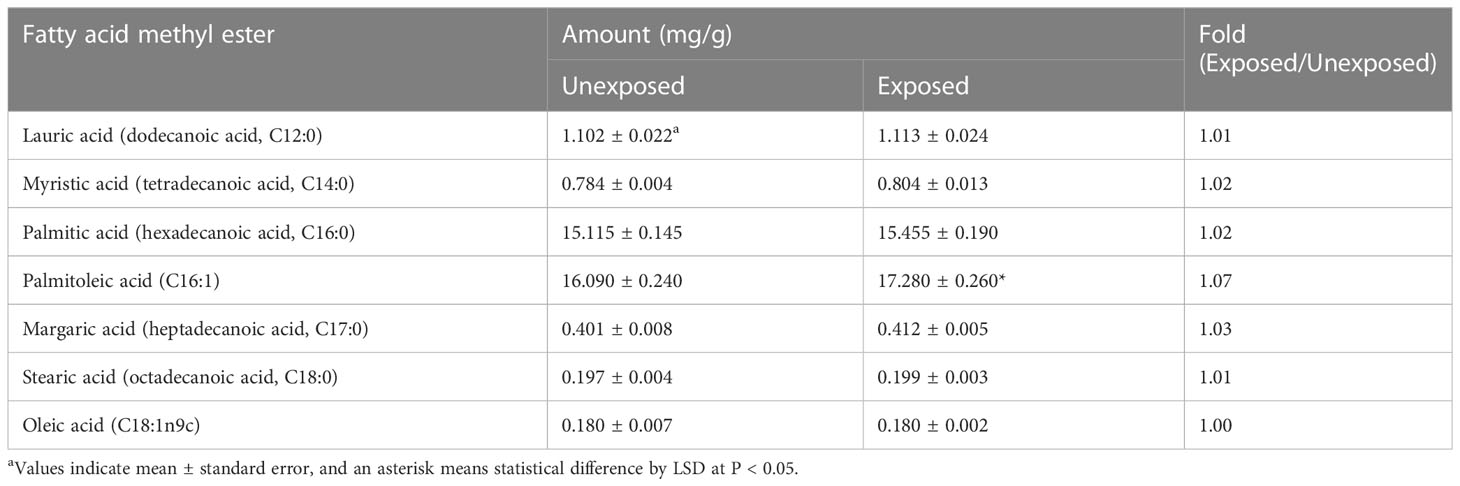
Table 3 Fatty acid methyl ester (FAME) composition of di(2-ethylhexyl) phthalate-exposed and unexposed Acidovorax citrulli.
Influence of di-(2-ethylhexyl) phthalate on metabolism of Acidovorax citrulli
To examine the effects of DEHP on the metabolism of A. citrulli, ATPase (Table 4) and TCA cycle-related components and enzymes were investigated (Figures 2, 3). DEHP treatment did not influence ATPase activity; however, the production of glutamate, malate, succinate, and fumarate in the TCA cycle of A. citrulli was significantly increased compared to that in the unexposed control (Figure 2). As a result, TCA cycle-related enzyme activity, including α-ketoglutarate dehydrogenase and succinyl-CoA synthetase, in DEHP-exposed A. citrulli was effectively increased compared to that in the unexposed control (Figure 3).
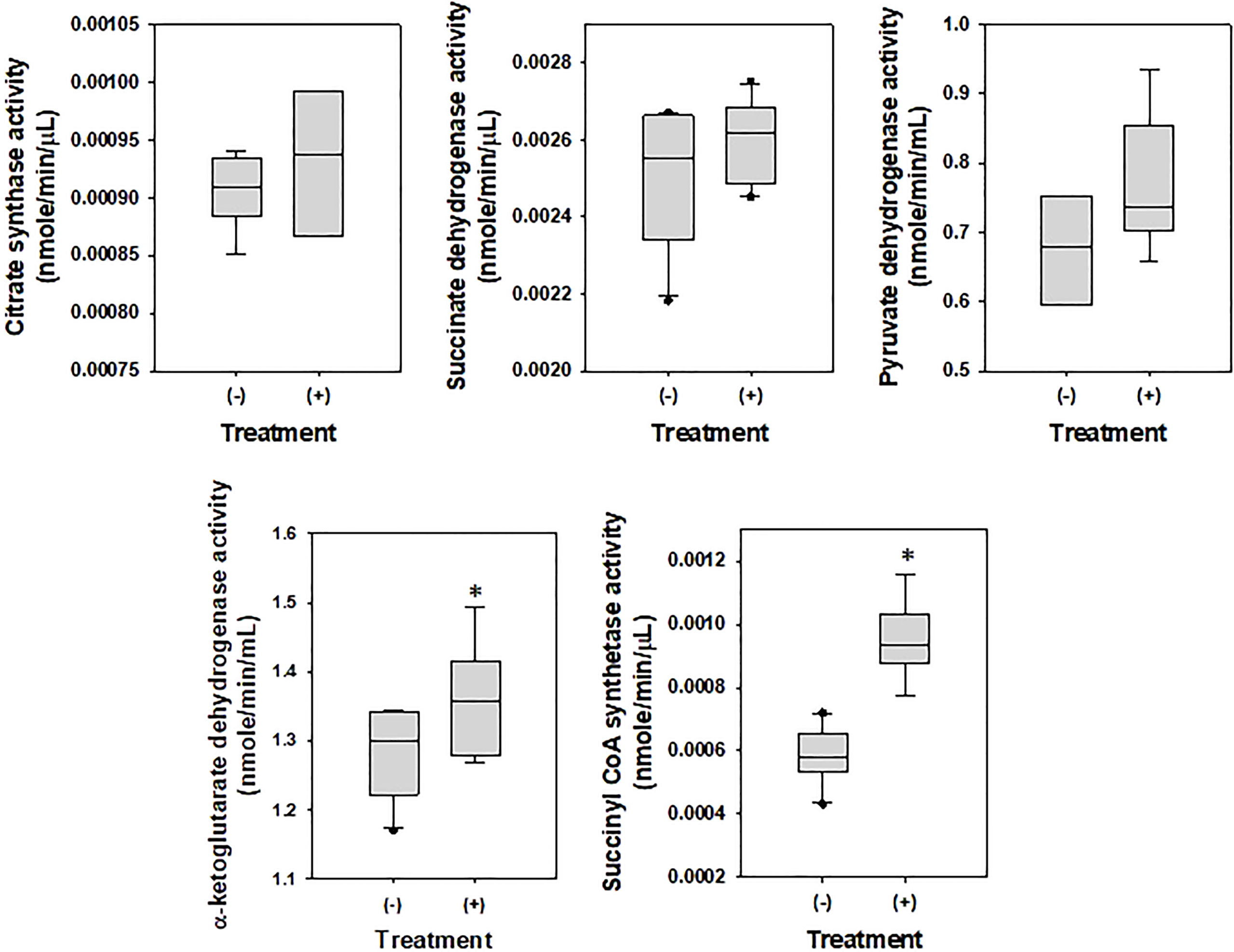
Figure 2 Effects of di-(2-ethylhexyl) phthalate on enzymes related to glycolysis and TCA cycle. (-) indicates DEHP-unexposed Acidovorax citrulli, and (+) indicates DEHP-exposed A. citrulli. An asterisk indicates a statistically significant difference as determined by LSD test (P < 0.05).
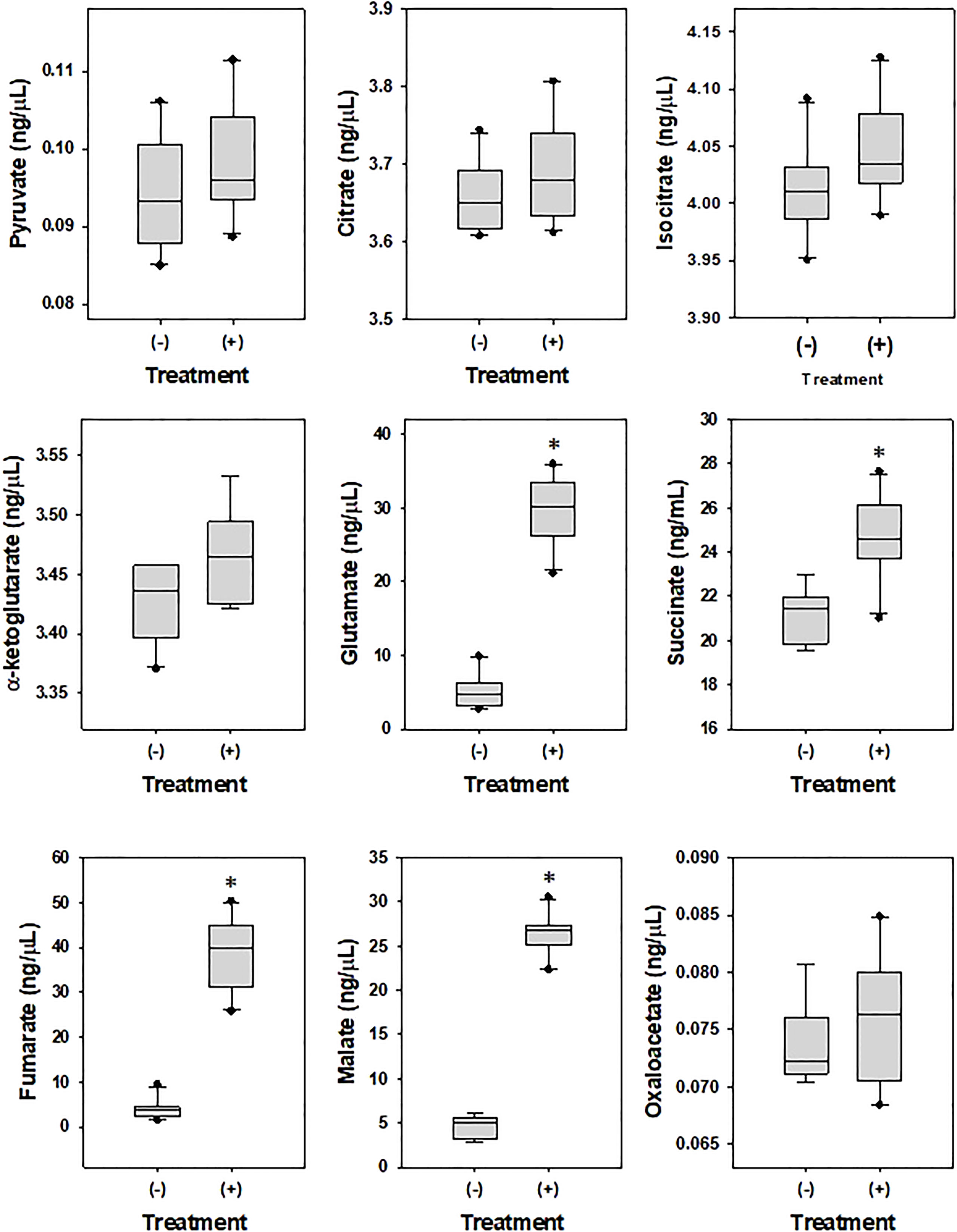
Figure 3 Effects of di-(2-ethylhexyl) phthalate (DEHP) on metabolites in TCA cycle. (-) indicates DEHP-unexposed Acidovorax citrulli, and (+) indicates DEHP-exposed A. citrulli. An asterisk indicates a statistically significant difference as determined by LSD test (P < 0.05).
Transcriptome analysis using RNA sequencing
The gene expression of DEHP-exposed A. citrulli was compared with that of the unexposed control using transcriptome and gene ontology (GO) analyses. RNA-seq results showed that 270 genes were differentially expressed in DEHP-exposed A. citrulli compared to the unexposed control; 28 genes were upregulated and 242 genes were downregulated (Figure 4). GO analysis divided DEGs into genes related to biological processes (BP), molecular functions (MF), and cellular components (CC); among BP, genes related to translation (GO:0006412), flagellum-dependent cell motility (GO:0071973), and flagellum assembly (GO:0044780) were significantly downregulated (Figure 4). In addition, DAVID analysis confirmed that the genes downregulated by DEHP treatment were mainly associated with cellular components (bacterial flagellum and cilium) (Figure 4). Among upregulated 28 genes by DEHP-exposure, 14 genes were hypothetical protein, and the others belonged to various categories such as ATP-binding cassette domain-containing protein, ABC transporter ATP-binding protein, the others were belonging to SDR family NAD(P)-dependent oxidoreductase, outer membrane beta-barrel protein and so on. The similar result to RNA-seq data were validated by qRT-PCR in Supplementary Table S1. Overall, most DEGs associated with translation, the flagellum assembly and flagellum-dependent motility were downregulated in DEHP-exposed A. citrulli, which may lead to a loss of motility.
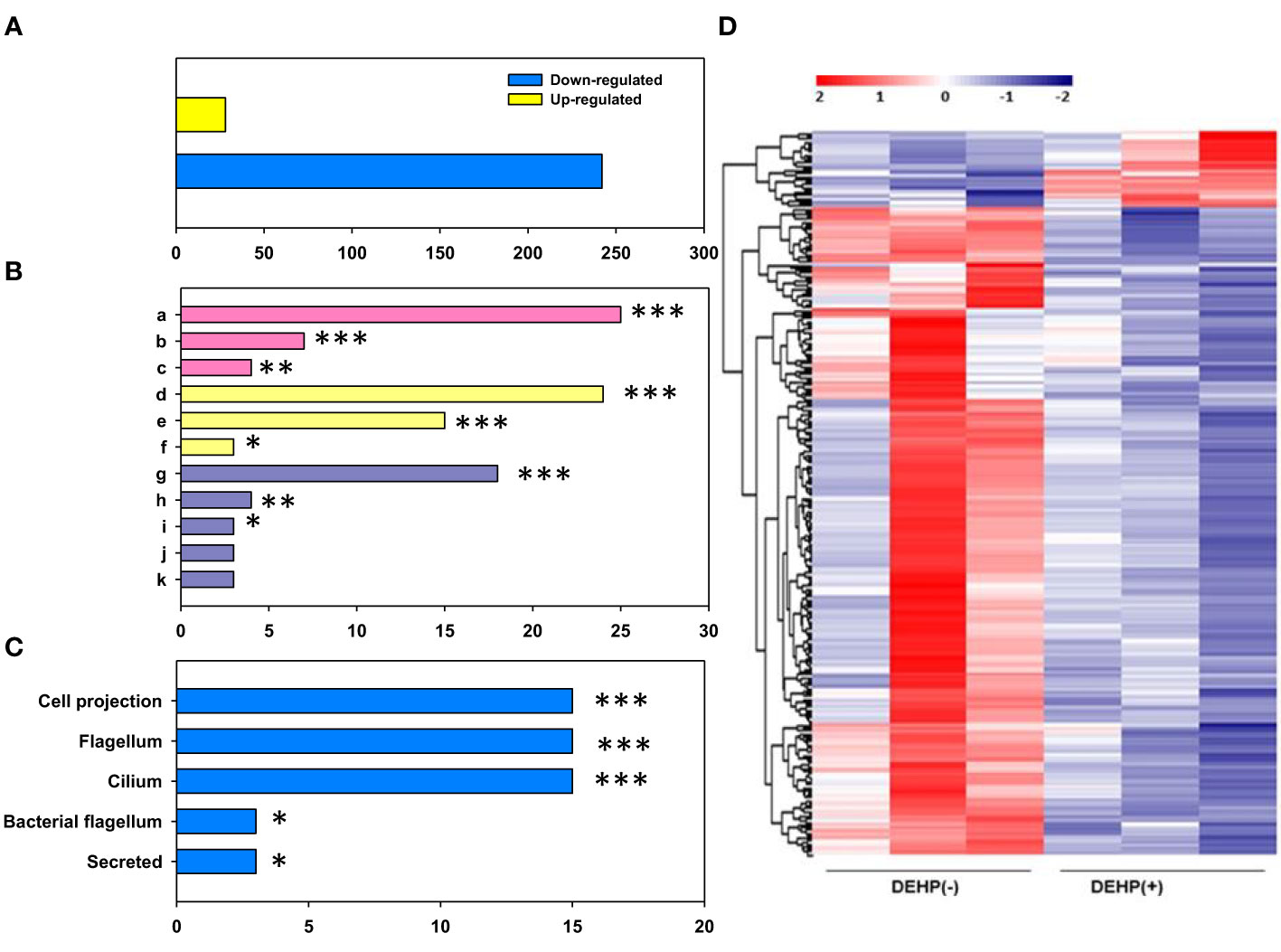
Figure 4 RNA sequencing of Acidovorax citrulli. (A) Significant count by fold change and p-value; Number of upregulated and downregulated genes. (B) Gene ontology categories: Biological Process (BP, pink), Molecular Function (MF, yellow), and Cellular Component (CC, purple); a: translation, b: bacterial-type flagellum-dependent cell motility, c: bacterial-type flagellum assembly, d: structural constituent of ribosome, e: rRNA binding, f: structural molecule activity, g: ribosome, h: extracellular region, i: bacterial-type flagellum hook, j: small ribosomal subunit, k: large ribosomal subunit. (C) Functional annotation. (D) Heat map of the expression patterns of di-(2-ethylhexyl) phthalate (DEHP)-exposed and unexposed A. citrulli; Red and blue colors represent the maximum and minimum values, respectively. (B, C); DEHP-exposed A. citrulli showed a significant decrease in differentially expressed genes compared to the unexposed control. *, **, and *** indicate statistically significant differences at P < 0.05, P < 0.01, and P < 0.001, respectively.
Impact of di-(2-ethylhexyl) phthalate on virulence traits of Acidovorax citrulli in vitro
To investigate changes in the virulence of A. citrulli caused by DEHP exposure, pathogenicity-related gene expression and virulence traits, including swimming motility and biofilm formation, were tested. The relative gene expression of hrpX and hrpG in DEHP-exposed A. citrulli was significantly decreased by 0.67- and 0.82-folds, respectively, compared to that in the unexposed control (Figure 5). In addition, DEHP treatment significantly decreased the swimming motility of A. citrulli; however, biofilm formation was not affected (Table 5).
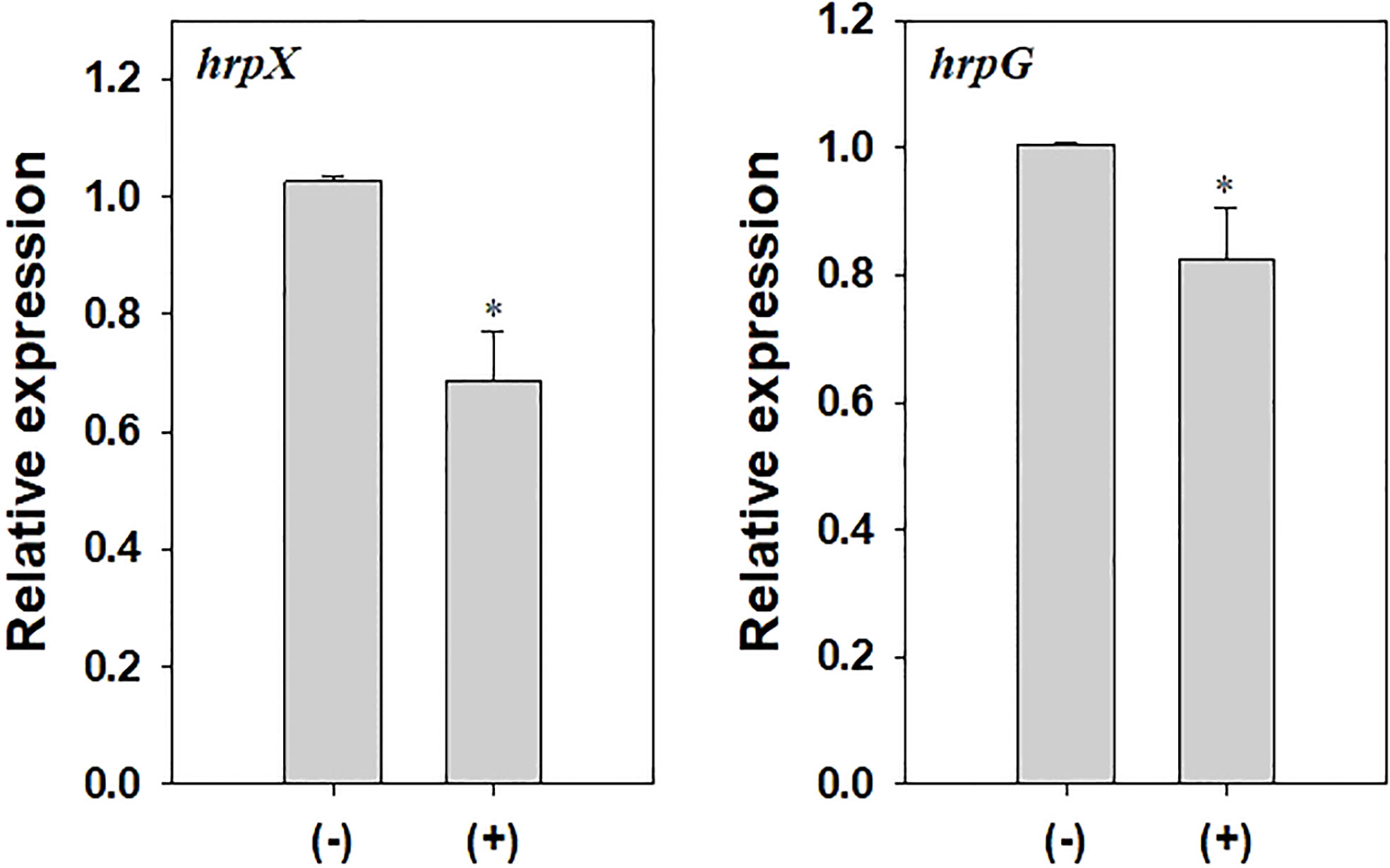
Figure 5 Relative expression of hrpX and hrpG in Acidovorax citrulli. (-) indicates di-(2-ethylhexyl) phthalate (DEHP)-unexposed A. citrulli, and (+) indicates DEHP-exposed A. citrulli. An asterisk indicates a statistically significant difference as indicated by LSD test (P < 0.05); error bars indicate standard error.

Table 5 Swimming motility and biofilm formation of di(2-ethylhexyl) phthalate-exposed or unexposed Acidovorax citrulli.
Discussion
In this study, we evaluated the effects of DEHP on bacterial population and metabolism of A. citrulli and the resulting changes in its virulence traits. Pollutants have been known to affect bacterial cell structure, including permeability and membrane stability, and physiological activity (Qian et al., 2016; Chen et al., 2018). Wang et al. (2019a) reported that the treatment with 20, 40, and 80 mg/L of dimethyl phthalate inhibited the growth of Escherichia coli along with severe cell membrane disruption and increased oxidative stress. In the present study, 20 mg/L DEHP treatment did not affect the population of A. citrulli in vitro; however, it tended to increase lipid peroxidation, membrane permeability, and nucleic acid leakage. The results indicated the absence of adverse effects on the survival of A. citrulli treated with all tested concentrations of DEHP. Similarly, Kim et al. (2019) used DEHP solution (10–20,000 mg in 1 kg of dried soil) for evaluation of soil ecotoxicity through assay on plant, earthworm, soil algae, Collembola, and nematodes; they observed low toxicity at only high concentrations of DEHP, and no effect on soil eukaryotic species at environmentally relevant concentrations. Liu et al. (2019) reported that the amount of six phthalic acid esters, including benzyl butyl phthalate, dubutyl phthalate, di-(2-ethylhexyl) phthalate, diethyl phthalate, dimethyl phthalate, and di-n-octyl phthalate, ranged from 1.2 to 7.3 mg/kg in vegetable fields; in particular, high concentration of DEHP was detected (0.48–15.34 mg/kg) in cultivated soils of China. The diverse range of accumulated amounts might be dependent on soil characteristics, samples, regions, and types of phthalate. Thus, the 20 mg/L of DEHP used in this study was assumed as highly relevant concentration in soils, the concentration of DEHP could be less influence on survival of A. citrulli.
However, DEHP caused changes in the FAME membrane composition of A. citrulli. Exposure to pollutants leads to change in the permeability and hydrophobicity of bacterial cell membranes (Al-Tahhan et al., 2000; Wang et al., 2019b), and remodeling of membrane lipid composition is essential to adapt in response to environmental changes (Čertík et al., 2003). Chlorophenol, a soil and water contaminant, affected the growth, lipid content, and fatty acid composition of the membrane of the bacterium Kocuria varians. In particular, it markedly enhanced the accumulation of palmitoleic and oleic acids; however, the content of phosphatidylcholine and phosphatidylethanolamine, two main unsaturated lipids, decreased (Dercová et al., 2004). The current study findings indicate that the presence of DEHP caused changes in fatty acid composition of A. citrulli, and that palmitoleic acid (C16:1) was mainly sensitive to DEHP.
During analysis of TCA cycle-related components and enzymes, DEHP-exposed A. citrulli showed increased production of glutamate, malate, succinate, and fumarate and induced activation of α-ketoglutarate dehydrogenase and succinyl-CoA synthetase in TCA cycle. TCA cycle, also known as the citric acid cycle, is a metabolic pathway that connects carbohydrate, fat, and protein metabolism and plays a major role in energy production. Fumarate (Zheng et al., 2015), succinate (Dalla Pozza et al., 2020), α-ketoglutarate dehydrogenase (Kehinde et al., 2022), and succinyl-CoA synthetase (Elpeleg et al., 2005) play important roles in TCA cycle. TCA cycle is generally activated during biodegradation of organic pollutant (Seo et al., 2013); for example, succinate accumulation was observed in Pseudomonas sp. strain HF-1 during nicotine degradation (Ye et al., 2012) and in Rhodococcus sp. YYL during tetrahydrofuran degradation (He et al., 2014). A. citrulli significantly accumulated succinate, fumarate, and malate, which are serially degraded in TCA cycle, and activated α-ketoglutarate dehydrogenase and succinyl-CoA synthetase in response to DEHP treatment.
Bacterial flagella are involved in motility, adherence, colonization, biofilm formation, and virulence (Bahar et al., 2010; Soria-Bustos et al., 2022). The flagellum is primarily composed of basal body rings and tubular axial structures (hooks, filaments, and rods), which produce thrust for the cells to swim in viscous environment and cause smooth transmission of motor torque to the filament regardless of its orientation (Imada, 2018). The flagellar motor switch protein (Flig) is one of the three proteins encoded by filM in certain bacteria. The protein complex regulates the direction of flagellar rotation and hence controls swimming behavior. The switch protein is a complex protein that responds to signals transduced by chemotactic sensory signaling system during chemotactic behavior (Roman et al., 1993). In this study, all DEGs involved in flagellar assembly, including fliM, fliS, fliD, flhA, flgA, and flgG, were downregulated in DEHP-treated A. citrulli. This suggests that DEHP exposure reduced flagellar assembly. In contrast, 28 proteins were upregulated in DEHP-treated cells compared to that of the untreated control, 14 of which were hypothetical proteins; elevated expression was observed for one protein associated with sugar transporter system, ABC transporter protein, and ATP-binding protein. ABC transporters present in bacteria take up various nutrients, vitamins, and ions and secrete toxic compounds (Moussatova et al., 2008). Pollutants alter the microbial community and increase the abundance of genes encoding ABC transporters and other proteins (Xu et al., 2018; Chen et al., 2020). Similarly, ABC transporter and ATP-binding protein gene expression was likely increased in response to toxin release by DEHP.
Biofilm formation and swimming motility are important virulence factors of A. citrulli. Our study revealed that the swimming motility of A. citrulli was decreased by DEHP exposure, whereas no difference was observed in biofilm formation. Many plant pathogenic bacteria secrete protein effectors into the host cells through T3SS (Buttner and He, 2009), which causes disease in susceptible plants and induces hypersensitivity reactions in resistant plants. T3SS is encoded by hrp (hypersensitive reaction and pathogenicity) genes. hrpG is a key regulatory gene of T3SS in A. citrulli and an OmpR-type regulator that can activate the transcription of the AraC-type activator hrpX (Zhang et al., 2018). Deletion of hrpG and hrpX in A. citrulli reduced pathogenicity in watermelon seedlings. We showed that hrpX and hrpG gene expression of A. citrulli was decreased after DEHP treatment. These results suggest that DEHP could affect the virulence factors of A. citrulli in laboratory conditions.
Our study revealed that exposure to DEHP in tested concentration did not significantly affect the survival of A. citrulli; however, it led to altered fatty acid composition of the bacterial membrane. DEHP exposure increased the production of certain metabolites in TCA cycle and activated TCA-related enzymes. Additionally, DEHP exposure reduced gene expression related to flagellar assembly, and increased the expression of genes encoding ABC transporter and ATP-binding proteins, which are involved in the uptake of nutrients and secretion of toxic compounds. Moreover, it influenced virulence traits, including swimming activity, and expression of pathogenicity genes, including hrpX and hrpG. Taken together, these results suggest that accumulation or release of DEHP in soil environment could potentially affect the metabolism and virulence traits of A. citrulli.
Data availability statement
The original contributions presented in the study are included in the article/Supplementary Materials. Further inquiries can be directed to the corresponding author.
Author contributions
Y-RK: investigation, writing-original draft preparation, and analysis. MS: conceptualization, data curation, analysis, and writing-review & editing. All authors contributed to the article and approved the submitted version
Funding
This work was supported by the National Institute of Agricultural Sciences (Project No. PJ01475802) of Rural Development Administration, Republic of Korea.
Conflict of interest
The authors declare that the research was conducted in the absence of any commercial or financial relationships that could be construed as a potential conflict of interest.
Publisher’s note
All claims expressed in this article are solely those of the authors and do not necessarily represent those of their affiliated organizations, or those of the publisher, the editors and the reviewers. Any product that may be evaluated in this article, or claim that may be made by its manufacturer, is not guaranteed or endorsed by the publisher.
Supplementary material
The Supplementary Material for this article can be found online at: https://www.frontiersin.org/articles/10.3389/fcimb.2023.1228713/full#supplementary-material
References
Al-Tahhan, R. A., Sandrin, T. R., Bodour, A. A., Maier, R. M. (2000). Rhamnolipid-induced removal of lipopolysaccharide from Pseudomonas aeruginosa: effect on cell surface properties and interaction with hydrophobic substrates. Appl. Environ. Microbiol. 66 (8), 3262–3268. doi: 10.1128/AEM.66.8.3262-3268.2000
Bahar, O., de la Fuente, L., Burdman, S. (2010). Assessing adhesion, biofilm formation and motility of Acidovorax citrulli using microfluidic flow chambers. FEMS Microbiol. Lett. 312 (1), 33–39. doi: 10.1111/j.1574-6968.2010.02094.x
Burdman, S., Walcott, R. (2012). Acidovorax citrulli: generating basic and applied knowledge to tackle a global threat to the cucurbit industry. Mol. Plant Pathol. 13, 805–815. doi: 10.1111/j.1364-3703.2012.00810.x
Buttner, D., He, S. Y. (2009). Type III protein secretion in plant pathogenic bacteria. Plant Physiol. 150 (4), 1656–1664. doi: 10.1104/pp.109.139089
Čertík, M., Dercová, K., Sejáková, Z., Findová, M., Jakubík, T. (2003). Effect of polyaromatic hydrocarbons (PAHs) on the membrane lipids of bacterial cell. Biology 58 (6), 1111–1117.
Chen, X., An, H., Ao, L., Sun, L., Liu, W., Zhou, Z., et al. (2011). The combined toxicity of dibutyl phthalate and benzo (a) pyrene on the reproductive system of male Sprague Dawley rats in vivo. J. Hazard. Mater. 186 (1), 835–841. doi: 10.1016/j.jhazmat.2010.11.078
Chen, X., Song, D., Xu, J., Li, E., Sun, G., Xu, M. (2018). Role and mechanism of cell-surface hydrophobicity in the adaptation of Sphingobium hydrophobicum to electronic-waste contaminated sediment. Appl. Microbiol. Biotechnol. 102 (6), 2803–2815. doi: 10.1007/s00253-017-8734-2
Chen, W., Wang, Z., Xu, W., Tian, R., Zeng, J. (2020). Dibutyl phthalate contamination accelerates the uptake and metabolism of sugars by microbes in black soil. Environ. pollut. 262, 114332. doi: 10.1016/j.envpol.2020.114332
Coffey, B. M., Anderson, G. G. (2014). Biofilm formation in the 96-well microtiter plate. Pseudomonas methods and protocols Methods Mol. Biol. 1149, 631–641. doi: 10.1007/978-1-4939-0473-0_48
Dalla Pozza, E., Dando, I., Pacchiana, R., Liboi, E., Scupoli, M. T., Donadelli, M., et al. (2020). Regulation of succinate dehydrogenase and role of succinate in cancer. .Academic Press 98, 4–14. doi: 10.1016/j.semcdb.2019.04.013
Dercová, K., Čertík, M., Malová, A., Sejáková, Z. (2004). ). Effect of chlorophenols on the membrane lipids of bacterial cells. Int. Biodeterior. Biodegradation. 54 (4), 251–254. doi: 10.1016/j.ibiod.2004.01.002
Elpeleg, O., Miller, C., Hershkovitz, E., Bitner-Glindzicz, M., Bondi-Rubinstein, G., Rahman, S., et al. (2005). Deficiency of the ADP-forming succinyl-CoA synthase activity is associated with encephalomyopathy and mitochondrial DNA depletion Am. J. Hum. Genet 76 (6), 1081–1086. doi: 10.1086/430843
Fu, X. Y., Sotani, T., Matsuyama, H. (2008). Effect of membrane preparation method on the outer surface roughness of cellulose acetate butyrate hollow fiber membrane. Desalination 233 (1-3), 10–18. doi: 10.1016/j.desal.2007.09.022
Gao, M., Dong, Y., Zhang, Z., Song, Z. (2020a). Effect of dibutyl phthalate on microbial function diversity and enzyme activity in wheat rhizosphere and non-rhizosphere soils. Environ. pollut. 265, 114800. doi: 10.1016/j.envpol.2020.114800
Gao, D. W., Wen, Z. D. (2016). Phthalate esters in the environment: a critical review of their occurrence, biodegradation, and removal during wastewater treatment processes. Sci. Total. Environ. 541, 986–1001. doi: 10.1016/j.scitotenv.2015.09.148
Gao, M., Zhang, Z., Dong, Y., Song, Z., Dai, H. (2020b). Responses of bacterial communities in wheat rhizospheres in different soils to di-n-butyl and di(2-ethylhexyl) phthalate contamination. Geoderma 362, 114126. doi: 10.1016/j.geoderma.2019.114126
Garcés, R., Mancha, M. (1993). One-step lipid extraction and fatty acid methyl esters preparation from fresh plant tissues. Anal. Biochem. 211 (1), 139–143. doi: 10.1006/abio.1993.1244
Groh, K. J., Backhaus, T., Carney-Almroth, B., Geueke, B., Inostroza, P. A., Lennquist, A., et al. (2019). Overview of known plastic packaging-associated chemicals and their hazards. Sci. Total. Environ. 651, 3253–3268. doi: 10.1016/j.scitotenv.2018.10.015
He, W., Qin, N., Kong, X., Liu, W., He, Q., Ouyang, H., et al. (2013). Spatio-temporal distributions and the ecological and health risks of phthalate esters(PAEs) in the surface water of a large, shallow Chinese lake. Sci. Total Environ. 461, 672–680. doi: 10.1016/j.scitotenv.2013.05.049
He, Z., Yao, Y., Lu, Z., Ye, Y. (2014). Dynamic metabolic and transcriptional profiling of Rhodococcus sp. strain YYL during the degradation of tetrahydrofuran. Appl. Environ. Microb. 80 (9), 2656–2664. doi: 10.1128/AEM.04131-13
Imada, K. (2018). Bacterial flagellar axial structure and its construction. Biophys. Rev. 10 (2), 559–570. doi: 10.1007/s12551-017-0378-z
Jiang, L., Zhu, X., Luo, C., Song, D., Song, M. (2022). The synergistic toxicity effect of di (2-ethylhexyl) phthalate and plant growth disturbs the structure and function of soil microbes in the rhizosphere. Environ. Int. 170, 107629. doi: 10.1016/j.envint.2022.107629
Kehinde, S. A., Ore, A., Olajide, A. T., Ajagunna, I. E., Oloyede, F. A., Faniyi, T. O., et al. (2022). Diisononyl phthalate inhibits cardiac glycolysis and oxidative phosphorylation by down-regulating cytosolic and mitochondrial energy metabolizing enzymes in murine model. Adv. Redox Res. 6, 100041. doi: 10.1016/j.arres.2022.100041
Kim, H., Nam, K., Oh, S., Son, S., Jeon, D., Gye, M. C., et al. (2019). Toxicological assessment of phthalates and their alternatives using human keratinocytes. Environ. Res. 175, 316–322. doi: 10.1016/j.envres.2019.05.007
Li, X., Zhang, W., L, J., Liu, W., Sun, S., Guo, C., et al. (2021). Distribution, source apportionment, and health risk assessment of phthalate esters in door dust samples across China. Environ. Sci. Eur. 33, 19. doi: 10.1186/s12302-021-00457-3
Liu, Y., Guan, Y., Yang, Z., Cai, Z., Mizuno, T., Tsuno, H., et al. (2009). Toxicity of seven phthalate esters to embryonic development of the abalone Haliotis diversicolor supertexta. Ecotoxicology 18, 293–303. doi: 10.1007/s10646-008-0283-0
Liu, S., Peng, Y., Lin, Q., Xiao, R., Luo, H., Liao, X., et al. (2019). Di-(2-ethylhexyl) phthalate as a chemical indicator for phthalic acid esters: an investigation into phthalic acid esters in cultivated fields and E-waste dismantling sites. Environ. Toxicol. Chem. 38 (5), 1132–1141. doi: 10.1002/etc.4402
Livak, K. J., Schmittgen, T. D. (2001). Analysis of relative gene expression data using real-time quantitative PCR and the 2–ΔΔCT method. Methods 25, 402–408. doi: 10.1006/meth.2001.1262
Loh, B., Grant, C., Hancock, R. (1984). Use of the fluorescent probe 1-N-phenylnaphthylamine to study the interactions of aminoglycoside antibiotics with the outer membrane of Pseudomonas aeruginosa. Antimicrob. Agents Chemother. 26, 546–551. doi: 10.1128/AAC.26.4.546
Moussatova, A., Kandt, C., O’Mara, M. L., Tieleman, D. P. (2008). ATP-binding cassette transporters in Escherichia coli. Biochim. Biophys. Acta Biomembr. 1778 (9), 1757–1771. doi: 10.1016/j.bbamem.2008.06.009
Qian, J., Zhou, C., Ma, H., Li, S., Yagoub, A. E. A., Abdualrahman, M. A. Y. (2016). Biological effect and inactivation mechanism of Bacillus subtilis exposed to pulsed magnetic field: morphology, membrane permeability and intracellular contents. Food Biophys. 11 (4), 429–435. doi: 10.1007/s11483-016-9442-7
Rashid, M. H., Kornberg, A. (2000). Kornberg. Inorganic polyphosphate is needed for swimming, swarming, and twitching motilities of Pseudomonas aeruginosa Proc. Natl. Acad. Sci. U.S.A. 97 (9), 4885–4890. doi: 10.1073/pnas.060030097
Roman, S. J., Frantz, B. B., Matsumura, P. (1993). Gene sequence, overproduction, purification and determination of the wild-type level of the Escherichia coli flagellar switch protein FliG. Gene 133 (1), 103–108. doi: 10.1016/0378-1119(93)90232-R
Seo, J. S., Keum, Y. S., Li, O. X. (2013). Metabolomic and proteomic insights into carbaryl catabolism by Burkholderia sp. C3 and degradation of ten N-methylcarbamates. Biodegradation 24, 795–811. doi: 10.1007/s10532-013-9629-2
Shen, Q., Shi, H., Zhang, Y., Cao, Y. (2015). Dietary intake and phthalates body burden in boys and girls. Arch. Public Health 73 (1), 1–5. doi: 10.1186/2049-3258-73-5
Soria-Bustos, J., Saldaña-Ahuactzi, Z., Samadder, P., Yañez-Santos, J. A., Laguna, Y. M., Cedillo-Ramírez, M. L., et al. (2022). The assembly of flagella in enteropathogenic Escherichia coli requires the presence of a functional type III secretion system. Int. J. Mol. Sci. 23 (22), 13705. doi: 10.3390/ijms232213705
Tao, R., Zhang, H., Gu, X., Hu, B., Li, J., Chu, G. (2021). Di-(2-ethylhexyl) phthalate (DEHP) exposure suppressed the community diversity and abundance of ammonia-oxidizers and mitigated N2O emissions in an alkaline soil. Ecotoxicol. Environ. Saf. 227, 112910. doi: 10.1016/j.ecoenv.2021.112910
Wang, L., Wang, L. H., Chang, Q., Dong, J. W., Sun, R. X., Yang, S. S., et al. (2017). Effects of di-(2-ethylhexyl) phthalate on microbial biomass carbon and microbial community structural diversity in a Mollisol. Eur. J. Soil Sci. 68 (6), 897–908. doi: 10.1111/ejss.12471
Wang, Z., Wang, C., You, Y., Xu, W., Lv, Z., Liu, Z., et al. (2019b). Response of Pseudomonas fluorescens to dimethyl phthalate. Ecotoxicol. Environ. Saf. 167, 36–43. doi: 10.1016/j.ecoenv.2018.09.078
Wang, X., Yuan, X., Hou, Z., Miao, J., Zhu, H., Song, C. (2009). ). Effect of di-(2-ethylhexyl) phthalate (DEHP) on microbial biomass C and enzymatic activities in soil. Eur. J. Soil Biol. 45 (4), 370–376. doi: 10.1016/j.ejsobi.2009.05.002
Wang, Z., Zhu, X., Su, Y., Xu, W., Liu, H., Liu, Z., et al. (2019a). Dimethyl phthalate damaged the cell membrane of Escherichia coli K12. Ecotoxicol. Environ. Saf. 180, 208–214. doi: 10.1016/j.ecoenv.2019.05.009
Xu, W., You, Y., Wang, Z., Chen, W., Zeng, J., Zhao, X., et al. (2018). Dibutyl phthalate alters the metabolic pathways of microbes in black soils. Sci. Rep. 8 (1), 2605. doi: 10.1038/s41598-018-21030-8
Ye, Y., Wang, X., Zhang, L., Lu, Z., Yan, X. (2012). Unraveling the concentration-dependent metabolic response of Pseudomonas sp. HF-1 to nicotine stress by H NMR-based metabolomics. Ecotoxicology 21, 1314–1324. doi: 10.1007/s10646-012-0885-4
Zhang, X., Zhao, M., Yan, J., Yang, L., Yang, Y., Guan, W., et al. (2018). Involvement of hrpX and hrpG in the virulence of Acidovorax citrulli strain Aac5, causal agent of bacterial fruit blotch in cucurbits. Front. Microbiol. 9. doi: 10.3389/fmicb.2018.00507
Zheng, L., Cardaci, S., Jerby, L., MacKenzie, E. D., Sciacovelli, M., Johnson, T. I., et al. (2015). Fumarate induces redox-dependent senescence by modifying glutathione metabolism. Nat. Commun. 6 (1), 6001. doi: 10.1038/ncomms7001
Keywords: di-(2-ethylhexyl) phthalate (DEHP), phthalate, Acidovorax citrulli, metabolism, virulence
Citation: Kim Y-R and Sang MK (2023) Effects of di-(2-ethylhexyl) phthalate on growth, metabolism, and virulence of the plant pathogenic bacterium Acidovorax citrulli. Front. Cell. Infect. Microbiol. 13:1228713. doi: 10.3389/fcimb.2023.1228713
Received: 30 May 2023; Accepted: 07 August 2023;
Published: 25 August 2023.
Edited by:
Sang-Wook Han, Chung-Ang University, Republic of KoreaReviewed by:
Youn-Sig Kwak, Gyeongsang National University, Republic of KoreaBei Bei Ge, Chinese Academy of Agricultural Sciences, China
Copyright © 2023 Kim and Sang. This is an open-access article distributed under the terms of the Creative Commons Attribution License (CC BY). The use, distribution or reproduction in other forums is permitted, provided the original author(s) and the copyright owner(s) are credited and that the original publication in this journal is cited, in accordance with accepted academic practice. No use, distribution or reproduction is permitted which does not comply with these terms.
*Correspondence: Mee Kyung Sang, bWtzYW5nQGtvcmVhLmty