- 1Working group “Cellular Interactions of Bacterial Pathogens”, Centre for Biological Threats and Special Pathogens, Highly Pathogenic Microorganisms (ZBS 2), Robert Koch Institute, Berlin, Germany
- 2Department of Infectious Diseases, Respiratory Medicine and Critical Care, Charité - Universitätsmedizin Berlin, Corporate Member of Freie Universität Berlin and Humboldt-Universität zu Berlin, Berlin, Germany
- 3Advanced Light and Electron Microscopy, ZBS 4, Robert Koch Institute, Berlin, Germany
- 4Research Group Zoonoses, Bernhard Nocht Institute for Tropical Medicine, Hamburg, Germany
- 5Department for General and Thoracic Surgery, DRK Clinics, Berlin, Germany
Introduction: Tularemia is mainly caused by Francisella tularensis (Ft) subsp. tularensis (Ftt) and Ft subsp. holarctica (Ftt) in humans and in more than 200 animal species including rabbits and hares. Human clinical manifestations depend on the route of infection and range from flu-like symptoms to severe pneumonia with a mortality rate up to 60% without treatment. So far, only 2D cell culture and animal models are used to study Francisella virulence, but the gained results are transferable to human infections only to a certain extent.
Method: In this study, we firstly established an ex vivo human lung tissue infection model using different Francisella strains: Ftt Life Vaccine Strain (LVS), Ftt LVS ΔiglC, Ftt human clinical isolate A-660 and a German environmental Francisella species strain W12-1067 (F-W12). Human lung tissue was used to determine the colony forming units and to detect infected cell types by using spectral immunofluorescence and electron microscopy. Chemokine and cytokine levels were measured in culture supernatants.
Results: Only LVS and A-660 were able to grow within the human lung explants, whereas LVS ΔiglC and F-W12 did not replicate. Using human lung tissue, we observed a greater increase of bacterial load per explant for patient isolate A-660 compared to LVS, whereas a similar replication of both strains was observed in cell culture models with human macrophages. Alveolar macrophages were mainly infected in human lung tissue, but Ftt was also sporadically detected within white blood cells. Although Ftt replicated within lung tissue, an overall low induction of pro-inflammatory cytokines and chemokines was observed. A-660-infected lung explants secreted slightly less of IL-1β, MCP-1, IP-10 and IL-6 compared to Ftt LVS-infected explants, suggesting a more repressed immune response for patient isolate A-660. When LVS and A-660 were used for simultaneous co-infections, only the ex vivo model reflected the less virulent phenotype of LVS, as it was outcompeted by A-660.
Conclusion: We successfully implemented an ex vivo infection model using human lung tissue for Francisella. The model delivers considerable advantages and is able to discriminate virulent Francisella from less- or non-virulent strains and can be used to investigate the role of specific virulence factors.
1 Introduction
Francisella tularensis is an intracellular Gram-negative bacterium causing tularemia, a life-threatening zoonotic disease occurring in various animals, including vertebrates, invertebrates and humans (Ellis et al., 2002; Foley and Nieto, 2010). More than 200 animal species have been identified to be susceptible for an infection by Ft and, therefore, Ft exhibits a broader host range than any other known zoonotic bacterial pathogen (Foley and Nieto, 2010; Santic et al., 2010). Francisella transmission to humans may occur via direct contact with infected animals (handling of infected animal, ingestion of insufficiently heated meat etc.), arthropod bites or through contaminated water or soil (Ellis et al., 2002; Maurin and Gyuranecz, 2016). The clinical manifestation of tularemia depends on the route of transmission and varies from flu-like symptoms to severe pneumonia with sometimes fatal outcome (Ellis et al., 2002; Maurin and Gyuranecz, 2016). The clinically most relevant Francisella tularensis subspecies are Ft holarctica (Fth) and Ft tularensis (Ftt). The latter is highly virulent (10-20 Ftt bacteria are sufficient to cause tularemia) and only occurs in North America (Saslaw et al., 1961a; Saslaw et al., 1961b; Ellis et al., 2002; Staples et al., 2006; Maurin and Gyuranecz, 2016). In contrast, being spread all over the Northern hemisphere Fth is moderately virulent, although 100-1000 Fth bacteria can already cause tularemia (Ellis et al., 2002; Bandouchova et al., 2009). However, infection with other Francisella species like F. hispaniensis (Whipp et al., 2003; Escudero et al., 2010), F. novicida (Hollis et al., 1989; Birdsell et al., 2009; Brett et al., 2014), F. philomiragia (Hollis et al., 1989; Wenger et al., 1989; Mailman and Schmidt, 2005; Kreitmann et al., 2015; Froböse et al., 2020) and F. salimarina (Hennebique et al., 2022) have been reported in immunocompromised patients. Due to its high infectivity and its ability to be spread by aerosols, Ft is classified as a potential bioterrorism agent of category A by the US Centers for Disease Control and Prevention (CDC) (Dennis et al., 2001; Maurin, 2015).
Ft has been shown to infect and replicate within various cell types including phagocytes (e.g. macrophages (Anthony et al., 1991), dendritic cells (Ben Nasr et al., 2006), neutrophils (Schwartz et al., 2012)) and non-phagocytic cells (e.g. fibroblasts (Horzempa et al., 2010) and epithelial cells (Melillo et al., 2006; Craven et al., 2008; Moreau and Mann, 2013)). For Francisella replication in mammals, macrophages serve as the major cell type (Twenhafel et al., 2009). After phagocytosis, Francisella prevents the fusion of the Francisella-containing phagosome with the lysosome and escapes into host cell cytosol, where a rapid bacterial replication culminates in cell lysis (Clemens et al., 2004; Bröms et al., 2010). During this process, the Francisella pathogenicity island, encoding a type VI secretion system (T6SS), is significantly involved (Lindgren et al., 2004; Santic et al., 2005; Bröms et al., 2010; Clemens et al., 2018). Deletion of the TSS6 tube structure protein IglC (corresponds to canonical TssD) results in a loss of phagosomal escape and intracellular replication culminating in an avirulent phenotype in mice (Golovliov et al., 1997; Golovliov et al., 2003; Lindgren et al., 2004; Santic et al., 2005).
To investigate Francisella infections in vivo different models have been established. For earlier studies, human volunteers were used (Mccrumb, 1961; Saslaw et al., 1961a; Saslaw et al., 1961b); later, different animal models were used, including mice (Bandouchova et al., 2009), rats (Kostiala et al., 1975; Ray et al., 2010) and non-human primates (Eigelsbach et al., 1962; White et al., 1964; Rick Lyons and Wu, 2007; Nelson et al., 2010; Glynn et al., 2015; Roberts et al., 2018). Also, often less pathogenic species, such as F. novicida or Fth live vaccine strain (LVS), were used to investigate Francisella virulence (Owen et al., 1964; Fortier et al., 1991; Rick Lyons and Wu, 2007; Hall et al., 2008). Although the course of infection by Francisella might be comparable between humans, animals and different Francisella strains, there are significant differences regarding host susceptibility and bacterial pathogenicity (Owen et al., 1964; Fortier et al., 1991; Rick Lyons and Wu, 2007; Hall et al., 2008). Hence, the transferability of the results obtained by infection using mice and a less virulent strain might be severely limited. In addition to the attenuated Fth LVS, we used an infectious wild-type Fth A-660 strain obtained from a patient suffering from lung tularemia (Appelt et al., 2019) in this study. As growing ethical concerns regarding the use of animal models emphasize the need for alternative experimental methods to examine Francisella infections, we established an ex vivo infection model using human lung tissue focusing on pulmonary tularemia representing a 3D model with different cell types. This ex vivo infection model has already been used for infection studies of different bacterial and viral pathogens like Streptococcus pneumoniae (Szymanski et al., 2012; Fatykhova et al., 2015; Berg et al., 2017; Peter et al., 2017), Legionella pneumophila (Jäger et al., 2014), Haemophilus influenzae (Wagner et al., 2015), Mycobacterium tuberculosis (Ganbat et al., 2016), Bacillus anthracis (Booth et al., 2016), Coxiella burnetii (Graham et al., 2016), influenza A viruses H5N1 (Weinheimer et al., 2012), H5N8 (Grund et al., 2018), H7N9 (Knepper et al., 2013) and corona viruses (Hocke et al., 2013; Hönzke et al., 2022). The model allows quantification of pathogen replication and identification of cellular tropism, dissemination and tissue interaction including immune response. Here, we present an ex vivo infection model for Francisella using human lung tissue explants and diverse Francisella strains including an Fth wild-type isolate.
2 Materials and methods
2.1 Human lung tissues
Human lung explants were obtained from adult bronchial carcinoma patients (n = 10) undergoing lung resection at local thoracic surgery centers. Peripheral tumour-free lung tissue was used which is far from bronchial tumour and was resected during the surgical intervention for lung anatomical reasons. Written informed consent was obtained from all patients. The study was approved by the ethics committee of the Charité - Universitätsmedizin Berlin, Germany (project EA2/079/13) and performed in accordance with the approved guidelines. The healthy tissue was edited into small pieces (weight app. 0.1-0.2 g/piece) and cultivated in RPMI 1640 medium (RPMI) supplemented with or without 10% fetal calf serum (FCS; Merck, Darmstadt, Germany) at 37°C and 5% CO2, as described before (Fatykhova et al., 2015; Peter et al., 2017).
Motile and leached-out cells of human lung tissue explants were also used for infection studies. This cell suspension was obtained from the bottoms of sample containers and comprised mostly erythrocytes, lymphocytes and primary alveolar macrophages. Erythrocytes were lysed as described by Vuorte et al. (Vuorte et al., 2001). Briefly, the cell suspension was pelleted and resuspended in H2O for 15 sec. PBS was added, samples were centrifuged and the cell pellet was adjusted to 105 cells/mL and seeded into 24-well plates. After over-night incubation cells were challenged with Francisella as described below.
2.2 Isolation of primary human alveolar macrophages and cell culture
Alveolar macrophages (AM) were isolated from human lung tissue as described above (Berg et al., 2017). Briefly, human lung tissue was repeatedly perfused with Hanks’ balanced salt solution (HBSS), and AM were seeded on glass coverslips in 12-well plates, 1x105 cells/well in RPMI medium. After 4 h of adherence (37°C, 5% CO2) remaining erythrocytes were removed by repeated washing with HBSS. AM were cultured in RPMI medium supplemented with 2% FCS for 2 days.
The human macrophage-like cell line U937 (ATCC CRL-1593.2) and leached-out cells of human lung tissue (see above) were cultivated in RPMI medium supplemented with 10% FCS at 37°C and 5% CO2. Prior to the infection assays U937 cells were stimulated with PMA (phorbol-12-myristate-13-acetate, 1 mg/mL in dH2O [Sigma-Aldrich Chemie]) at a concentration of 1:20,000 for 36 h.
2.3 Bacterial strains and growth conditions
Strains used in this study are listed in Table 1. Francisella strains were cultivated in medium T (MT; (Pavlovich and Mishan'kin, 1987; Becker et al., 2016)) or on MT agar plates supplemented with hemoglobin and charcoal (MTKH plates, (Tlapak et al., 2018)).
2.4 Infection of human lung tissue and human cells
Human lung tissue explants were inoculated with 106 CFU overnight grown Francisella strains for 2 h. Explants were washed three times with 2 mL plain RPMI and treated with 50 µg/mL gentamicin for 1 h to eliminate remaining extracellular bacteria. After washing them three-times and applying fresh plain RPMI, the lung explants were incubated up to 72 h. To determine the CFU/mL at various time points of the infection, human lung tissue was homogenized with Lysing Matrix D (MP Biomedicals) and saponin (0.001%; Sigma-Aldrich Chemie) in FastPrep-24 (MP Biomedicals) for 20 sec at 4 m/s and serial dilutions were plated on MTKH agar. Culture supernatants were collected and stored at -80°C until further analysis (see below).
For co-infection of Fth LVS and Fth A-660, human lung tissue explants were simultaneously challenged with both strains for 2 h (106 (LVS) and 105 (A-660) bacteria per explant). After washing and gentamicin treatment (same procedure as above), explants were homogenized and suspension was plated onto MTKH agar plates, half of which were supplemented with erythromycin (Ery), respectively, to distinguish between the two Fth strains. Belonging to biovar II Fth LVS is erythromycin-resistant, whereas Fth A-660, as a biovar I strain, is erythromycin-sensitive. Therefore, only Fth LVS grows on MTKH agar supplemented with Ery (MTKH+Ery; constituting Fth LVS CFU/mL). Fth A-660 CFU/mL values were calculated by subtracting CFU/mL gained from MTKH+Ery plates from total CFU/mL rates received on MTKH plates (on which both strains are able to grow).
For infection of human macrophages (AM, U937), cells were seeded at a concentration of 5 × 105 cells/mL and challenged with overnight grown Francisella strains for 2 h (multiplicity of infection (MOI) of 10). After removing the bacterial suspension, cells were washed and treated with 50 µg/mL gentamicin for 1 h. Subsequently, plain medium was added and cells were incubated up to 72 h. To determine the CFU at various time points of infection, cells were lysed with saponin (0.001%), and serial dilutions were plated on MTKH agar.
For the co-infection assay, U937 cells were seeded at a concentration of 5 × 105 cells/mL and infected with a mixture of Fth LVS and Fth A-660 bacteria (1:1; in a total MOI of 10) for 2 h. Cells were treated with 50 µg/mL gentamicin for 1 h, afterwards washed and incubated up to 72 h (same procedure as for infection of human macrophages, see above). At various time points, U937 cells were lysed with 0.001% saponin, and bacterial suspension was plated on MTKH agar plates, half of which were supplemented with erythromycin (Ery), respectively, to distinguish between the two Fth strains as described above.
2.5 Immunohistochemistry and confocal immunofluorescence
Isolated AM were challenged with Fth A-660 as described in section “Infection of human lung tissue and cells”. After 48 h AM were fixed as described before (Berg et al., 2017) and stained with an established cell marker for AM CD68 (abcam, Cambridge, UK) and anti-Ft LPS-FITC antibody (F11FITC, (Grunow et al., 2000)). Immunofluorescence of AM was analyzed using a LSM 780 [(objectives: Plan Apochromat 63x/1.40 oil DIC M27 and 40x/1.30 oil DIC M27), Carl-Zeiss, Jena, Germany].
For immunohistochemistry of infected human lung tissue, explants were challenged with 106 bacteria/mL for 24 h and 48 h. Afterwards the samples were fixed in 3% paraformaldehyde for 48 h, embedded in paraffin and routinely processed for histology and immunofluorescence staining as described before (Peter et al., 2017; Hönzke et al., 2022). For characterization of infected cells, the specific cell marker CD68 for AM and anti-Ft LPS antibody (Grunow et al., 2000) were used, followed by incubation with corresponding secondary antibodies. Nuclei were subsequently counterstained with DAPI (Sigma Aldrich). Immunofluorescence of human lung slices was analyzed by spectral confocal microscopy using a LSM 780 [(objectives: Plan Apochromat 63x/1.40 oil DIC M27 and Plan Apochromat 40x/1.40 oil DIC M27), Carl-Zeiss, Jena, Germany]. Based on a spectral image lambda stack, linear unmixing of tissue autofluorescence and overlapping spectra of fluorochromes were performed using ZEN 2012 software (Carl-Zeiss, Jena, Germany). To reveal lung and cell morphology, images were combined with Differential Interference Contrast (DIC). All image sets were acquired using optimal configuration regarding resolution and signal to noise ratio. Images were processed using ZEN 2012.
2.6 Electron microscopy
Human lung explants were challenged with 108 Francisella bacteria per mL for up to 48 h. Electron microscopy of human lung explants was done essentially as described before (Weinheimer et al., 2012). Lung tissue was fixed by immersion in 4% Formaldehyde, 2.5% glutaraldehyde (in 50 mMHepes-buffer) and post fixed with 1% OsO4 (1 h), 0.1% tannic acid (in 50 mM Hepes buffer, 30 min) and 2% uranyl acetate (2 h). Samples were dehydrated in ethanol and embedded in epon resin. Thin sections were produced using an ultramicrotome (UC7, Leica, Wetzlar, Germany) and stained with 2% uranyl acetate (20 min) followed by lead citrate (2 min). Sections were examined using a transmission electron microscope (Tecnai Spirit, Thermo Fisher/FEI) operated at 120 kV. Images were recorded using a CCD-camera (Phurona, Emsis, Münster, Germany).
2.7 Cytokine and chemokine measurement
Supernatants of infected human lung tissue explants were collected 24 h, 48 h and 72 h post infection. After centrifugation (10 min, 5000 g, 4°C) 100 µl aliquots were stored at liquid nitrogen until further investigations. Samples were sterilized using 0.2 µm filter (Sartorius, Göttingen, Germany) prior to measurement of cytokines and chemokines. Cytokines and chemokines were analyzed in cell culture supernatants using the LegendPlex assay (BioLegend, USA) according to the manufacturer’s instructions. For the analyzed biomarkers, the detection limits of the LegendPlex assay were, as follows: granulocyte colony-stimulating factor (G-CSF, 33.66 pg/mL), interferon-α (IFNα; 3.11 pg/mL), IFNγ (3.35 pg/mL), interleukin (IL) 1ß (4.23 pg/mL), IL-2 (1.93 pg/mL), IL-4 (2.22 pg/mL), IL-5 (2.7 pg/mL), IL-6 (3.09 pg/mL), IL-8 (2.53 pg/mL), IL-9 (3.12 pg/mL), IL-10 (1.9 pg/mL), IL-12p70 (4.75 pg/mL), IL-13 (3.93 pg/mL), IL-17A (3.39 pg/mL), IL-17F (2.07 pg/mL), IL-21 (4.04 pg/mL), IL-22 (2.16 pg/mL), interferon-γ–induced protein-10 (IP-10 = C-X-C motif chemokine ligand 10 (CXCL-10), 4.48 pg/mL), monocyte chemotactic protein-1 (MCP-1 = CC-chemokine ligand 2 (CCL-2); 6.04 pg/mL), tumor necrosis factor-α (TNFα, 2.41 pg/mL) and vascular endothelial growth factor (VEGF, 31.41 pg/mL).
2.8 Statistics
Statistical analysis was performed with GraphPad Prism 9 software. For comparison between the two analyzed groups (LVS vs. A-660), a two-tailed test t was used. For comparison of three groups (LVS vs. A-660 and A-660 105, respectively), one-way ANOVA and Kruskral-Wallis test with multiple comparison were performed.
3 Results
3.1 Establishment of the human ex vivo infection model for Francisella
In order to determine the growth of intracellular Francisella, represented by the colony forming units (CFU), the human lung explants needed to be homogenized and lysed after infection. To test if Francisella is able to survive the homogenization, 105 Fth LVS bacteria were treated in PBS supplemented with 0.001% saponin for 20 sec with 4 m/s using a FastPrep homogenizer. As shown in Figure S1A, the homogenization with FastPrep did not significantly reduce the bacterial load of the LVS compared to untreated and vortexed samples. In order to monitor a possible extracellular replication of Francisella, we used Fth LVS ΔiglC in all infection assays as a control strain. This mutant strain is unable to escape the phagosome and is therefore, unable to replicate intracellularly (Golovliov et al., 1997; Golovliov et al., 2003; Lindgren et al., 2004; Santic et al., 2005). When U937 macrophages were infected with LVS and LVS ΔiglC for 2 h, washed and incubated for 24 h and 48 h, respectively, CFU/mL of both, LVS and LVS ΔiglC, increased over time (Figure S1B), demonstrating that Fth replicates in co-culture with U937 cells. Treatment by gentamicin inhibited the extracellular replication (Figure S1B). After observing a minimal increase of bacteria (OD600nm) cultivated in RPMI + 10% FCS (data not shown), for all following infection assays, RPMI medium was used without FCS to minimize a possible extracellular replication of Fth in our model.
The experimental procedure of the ex vivo infection model was adapted for Francisella, as follows (Figure 1): 106 Francisella bacteria were injected into human lung tissue explant portioned into three punctures (~ 33 µl each). The infection was performed at 37°C and 5% CO2 for 2 h. Medium was completely removed; lung explants were thoroughly washed and treated with 50 µg/mL gentamicin for 1 h to eliminate the remaining extracellular bacteria. Subsequently, medium was removed, followed by a complete rinsing of explants. Afterwards, fresh plain RMPI medium was added and the lung explants were incubated for up to 72 h. The human lung tissue was further used (1) to determine the intracellular growth and (2) to identify the infected cell types. To achieve this, (1) human lung explants were homogenized, lysed and plated on agar plates to determine the CFU per g lung tissue (Figure 1). (2) The analysis by differential interference contrast and electronic microscopy was conducted after fixing the human lung explants and staining them with antibodies for Fth as well as selected cell markers.
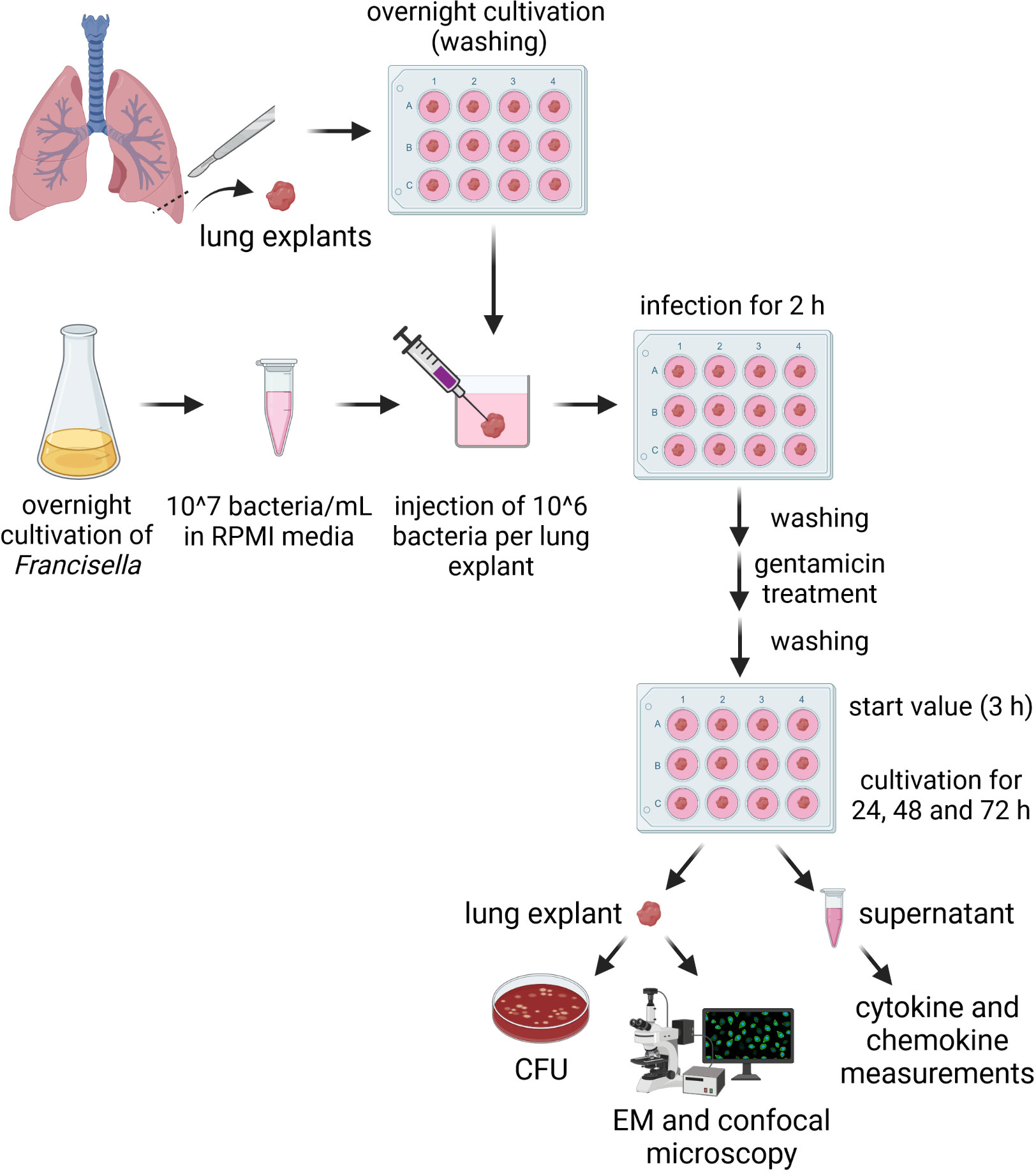
Figure 1 Scheme of the human lung ex vivo infection model for Francisella. 106 Francisella bacteria were injected into human lung tissue explants portioned in three punctures at different lung tissue sections (~ 33 µl each) and incubated for 2 h at 37°C and 5% CO2. Bacterial suspension was completely removed; lung explants were washed and treated with 50 µg/mL gentamicin for 1 h to eliminate remaining extracellular bacteria. Medium was removed; lung explants were washed and fresh plain RPMI was added. Human lung tissue was used to determine the number of intracellular bacteria and to identify the infected cell types using immunofluorescence and electron microscopy. Culture supernatants were used to measure cytokine and chemokine levels. Schema was created with BioRender.com (https://biorender.com).
3.2 Multiplication of Francisella strains in human lung explants.
In this study we used Fth LVS, Fth LVS ΔiglC and a human isolate Fth (Fth A-660) obtained from a tularemia patient exhibiting pneumonia (Appelt et al., 2019), as well as an environmental Francisella species (Francisella sp. strain W12-1067, F-W12) isolated from a cooling tower in Germany (Rydzewski et al., 2014). It is yet not known if the environmental species F-W12 is pathogenic for humans, but the species possesses some well-known virulence factors of Francisella and is able to persist in mouse macrophages and Acanthamoeba lenticulata (Rydzewski et al., 2014; Köppen et al., 2019). By using the human lung ex vivo infection model, an increase of CFU/g could be shown in the lung explants over 72 h for Fth LVS and Fth wild-type A-660. During this process, it became obvious that the human isolate Fth A-660 replicated to a greater extent than Fth LVS leading to a higher CFU count per g lung tissue (Figure 2A). Over a time period of 72 h, a 22-fold CFU-increase/g tissue was observed for Fth LVS and a 49-fold increase for A-660 (A-600: 2.7 × 107 CFU/g; LVS: 2.4 × 105 CFU/g; p = 0.0047). A similar trend was obtained when a lower bacterial load of Fth A-660 was used (105 bacteria instead of 106 per lung explant). Here, we observed an 86-fold CFU-induction/g lung tissue (A-660 105 after 72 h: 5 × 106 CFU/g, LVS: 2.4 × 105 CFU/g; p = 0.3537). In contrast, the bacterial load of Fth LVS ΔiglC did not increase in lung explants over time, instead a minor reduction was obtained (0.56-fold induction from 24 h to 72 h, Figure 2A). Neither replicated the environmental Francisella strain F-W12 in the human lung explants, but the strain persisted more or less stable over a time period of 72 h (1.6-fold induction; Figure 2A). Hence, the human lung ex vivo infection model confirmed the theoretically expected intracellular growth of different Francisella strains. To further underpin the reliability of the observed results, five other Fth isolates (one animal isolate obtained from a Eurasian beaver: A-271; four human isolates obtained from tularemia patients: A-663, A-820, A-981, A-1308) were investigated. All tested isolates showed an increase of CFU per g lung tissue comparable to those observed for Fth A-660 after 48 h (Figure 2B).
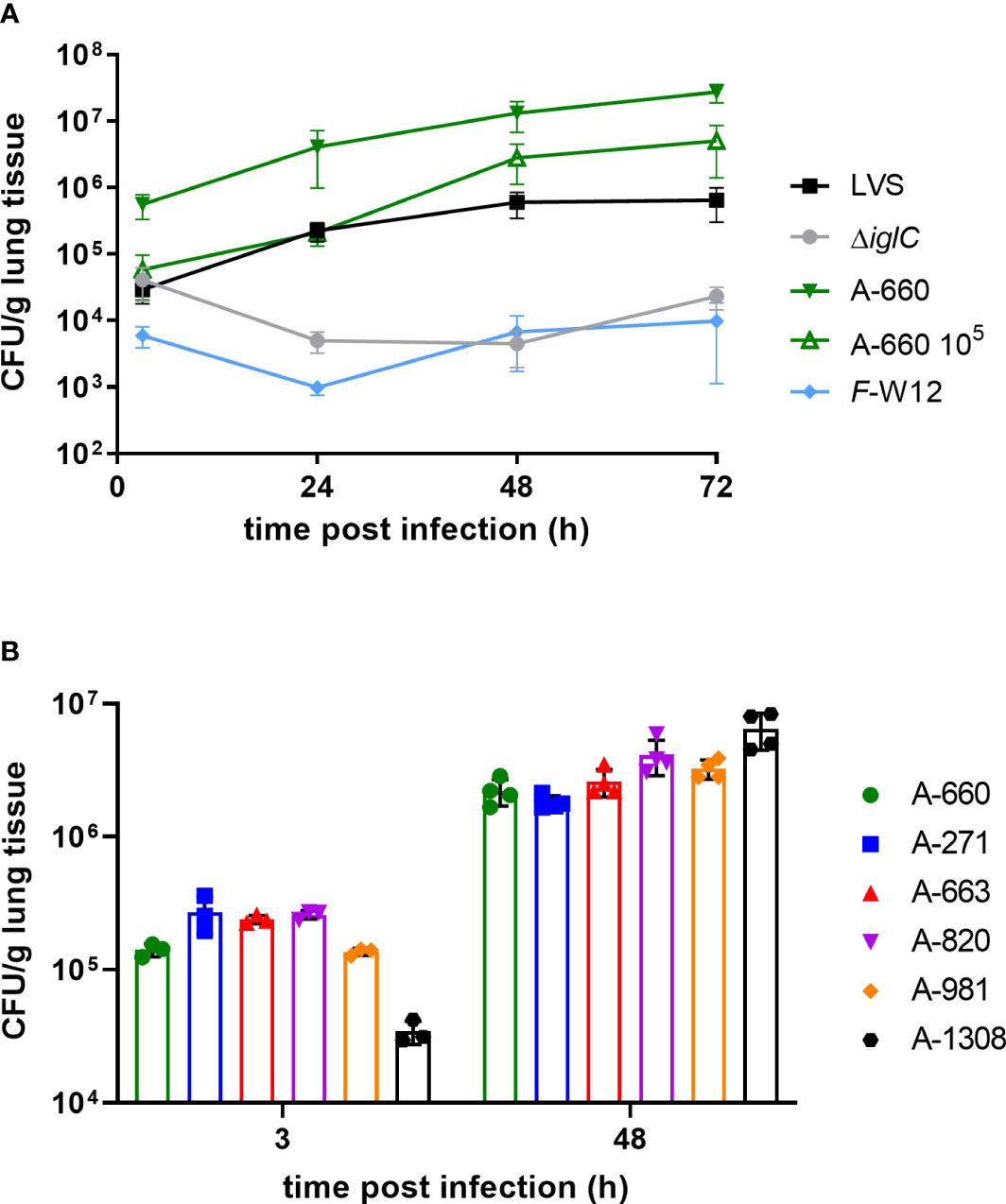
Figure 2 Francisella human lung ex vivo infection model. (A) Lung tissue explants were infected with 106 Fth LVS, Fth LVS ΔiglC, Fth wild-type A-660 and F-W12 bacteria using experimental procedure described in legend of Figure 1 and in material and methods. After 3 h, 24 h, 48 h and 72 h of infection human lung tissue was homogenized. Bacterial suspension was plated onto agar plates to obtain the colony forming units (CFU) per g lung tissue. Human lung explants were additionally infected with 105 bacteria of Fth wild-type A-660 (A-660 105). Means with structural equation modeling of at least five individual experiments are shown. A one-way ANOVA and Kruskral-Wallis test with multiple comparisons were used to compare LVS vs. A-600 and A-660 105, respectively. For comparison of LVS vs. A-600 significances were observed (3 h: p = 0.0076; 24 h: p = 0.0110; 48 h: p = 0.0076; 72 h: p = 0.0047), but comparison of LVS with A-660 105 remained statistically insignificant. (B) Lung tissue explants were infected with six German Fth isolates (A-#). Means with standard deviation of one experiment are shown.
We further aimed to identify the cell types involved in the course of a Francisella infection of the human lung by applying spectral immunofluorescence to Fth A-660-infected lung explants. After 48 h, parts of highly infected areas were found. Especially, the assumed injection spot showed a high concentration of Fth A-660 bacteria, as shown in Figure 3A. Apart from the injection sites, Fth A-660 was primarily detected in human alveolar macrophages in distinct areas (see Figure 3B). These results were confirmed by examining Fth A-660 infected lung explants by electron microscopy (Figure 3C). However, Fth A-660 was not only detected in alveolar macrophages, but also sporadically in other cell types, including lymphocytes, granulocytes and fibrocytes, as well as, extracellular and within the connective lung tissue (Supplemental Figure S2).
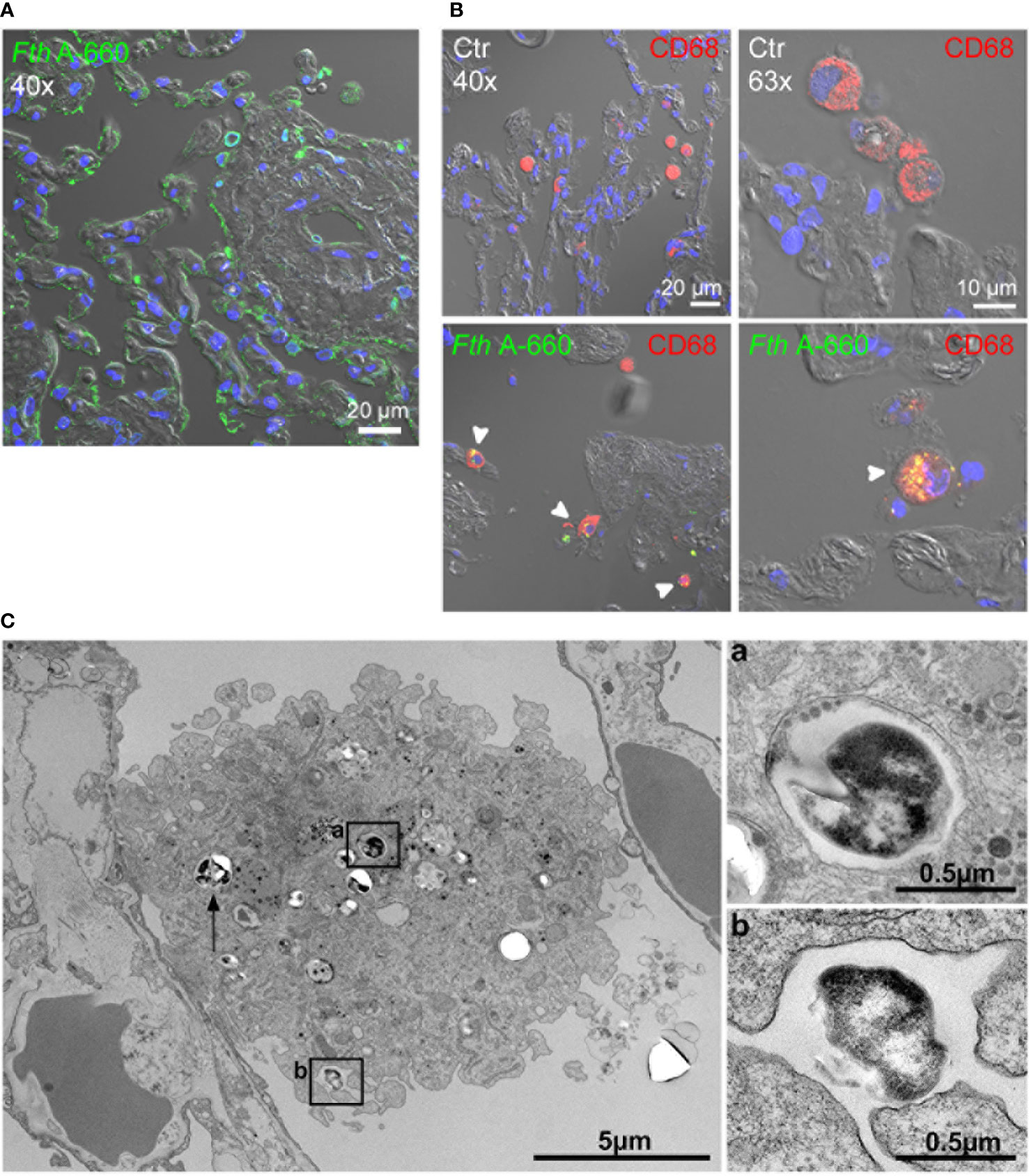
Figure 3 Fth wild-type A-660 (Fth A-660) distribution in human lung tissue explants 48 h post infection. (A, B) Confocal immunofluorescence microscopy of sections from infected lung explants. Fth A-660 bacteria are shown by binding of an antibody against LPS (green), nuclei were counterstained with DAPI (blue) and the tissue was visualized by differential interference contrast. (A) At the putative injection spot of bacteria into the lung explant, the green signal for bacteria is associated with the epithelial lining of the alveoli, the endothelium of a small vessel and within a few cells in the extracellular space and the connective tissue. (B) A region distinct from the putative injection spot, signals for bacteria (green) were mainly found in alveolar macrophages (red; CD68) (C) Electron microscopy of thin sections from infected lung explants shows a macrophage in the alveolar space with contact to the epithelial lining of an alveolus. Two bacterial profiles (box a and arrow) are localized in the cytoplasm of the macrophage within a membrane-bound compartment. One bacterium is associated with a surface niche of the macrophage (box b).
As a next step, we assessed how Francisella activated the host response in ex vivo infected human lungs by quantifying the induced cytokine and chemokine profile. This was performed by collecting and analyzing the culture supernatants of lung explants infected by Fth LVS, Fth LVS ΔiglC, Fth A-660 and F-W12 24 h, 48 h and 72 h post infection to determine the levels of cytokines and chemokines (e.g. IL-1β, IL-6, TNF-α, IL-8, G-CSF, MCP-1, IP-10 and VEGF). Just as the individual immune response differs from human to human, the induced cytokine and chemokine production varied between ex vivo infected lung explants (see Figure S2) and remained not to be statistically significant. Nevertheless, the following tendencies could be observed: The cytokine and chemokine response differed between Fth A-660 and Fth LVS. Fth A-660 seemed to induce a lower secretion of IL-1β (p = 0.0519 after 48 h), MCP-1 and IL-6 compared to Fth LVS (Figure 4). After 24h, a lower concentration of IP-10 seemed to be induced by A-660, but after 72 h, a higher concentration of IP-10 was detected in A-660-infected human lung explant supernatants compared to LVS (Figure 4). We did not identify distinctive differences in secretion of IL-8, G-CSF and VEGF between Fth LVS and Fth A-660 infected lung explants (Figure S2). In general, the environmental F-W12 strain seemed to induce higher levels of cytokines, including TNF-α, in the infected lung explants (Figures 4, S2).Various further chemokines and cytokines, including IL-2, IL-4, IL-5, IL-9, IL-10, IL-12p70, IL-13, IL-17A, IL-17F, IL-21, IL-22, IFN-α and IFN-γ, were investigated but remained below the detection limits (data not shown).
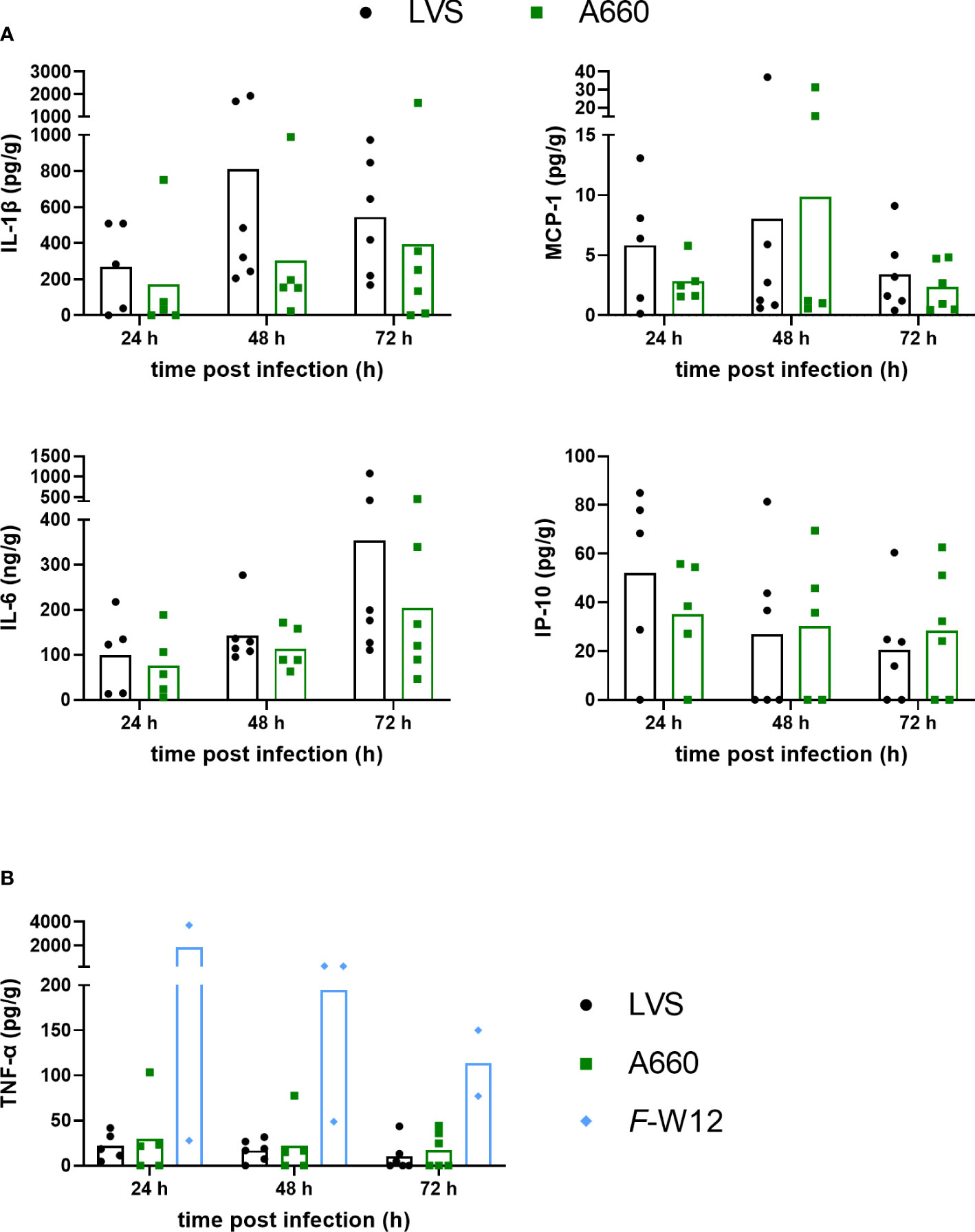
Figure 4 Cytokine levels obtained using the Francisella human lung ex vivo infection model. Lung tissue was infected with A-660 and LVS (A) A-660, LVS and F-W12 (B) using experimental procedure as described in the legends of Figures 1 and 2. After 24 h, 48 h and 72 h of infection, the supernatants were collected and the levels of IL-1β, MCP-1, IL-6, IP-10 (A) and TNF-α (B) were measured. Means are indicated.
3.3 Replication of Francisella strains in cell culture
We next aimed to compare the results obtained by the ex vivo model with “classical” single cell type infection models using U937 macrophages and primarily isolated human alveolar macrophages as well as multicellular primarily isolated mobile human lung cells (Figures 5A–C). Cells were infected with Fth LVS, Fth LVS ΔiglC, Fth wild-type A-660 and F-W12 (MOI = 10) for 2 h and subsequently treated with gentamicin (50 µg/mL for 1 h) to eliminate remaining extracellular bacteria. After 3 h, 24 h, 48 h and 72 h of infection the CFU/mL was determined. Here, as observed in the human lung infection model, only Fth LVS and Fth A-660 were able to replicate within cells tested (Figures 5A–C). In U937 macrophages, both strains similarly replicated (Figure 5A), whereas in primary alveolar macrophages (Figure 5B) and primary mobile lung cells (Figure 5C), the Fth LVS strain showed higher growth rates compared to Fth A-660 (6-fold higher in alveolar macrophages and 19-fold in mobile lung cells, Figures 5C). Hence, these findings differ from the results obtained using the human lung ex vivo infection model, in which Fth A-660 showed a higher replication rate compared to Fth LVS (Figure 2A). However, Fth LVS ΔiglC and F-W12 did not significantly replicate in these cell culture models, except for an increase of CFU/mL observed for ΔiglC in infected primary alveolar macrophages after 72 h, indicating extracellular growth (64-fold induction, Figure 3B). Moreover, the intracellular localization of Fth A-660 in primarily isolated alveolar macrophages was confirmed by spectral immunofluorescence, as shown in Figure 5D.
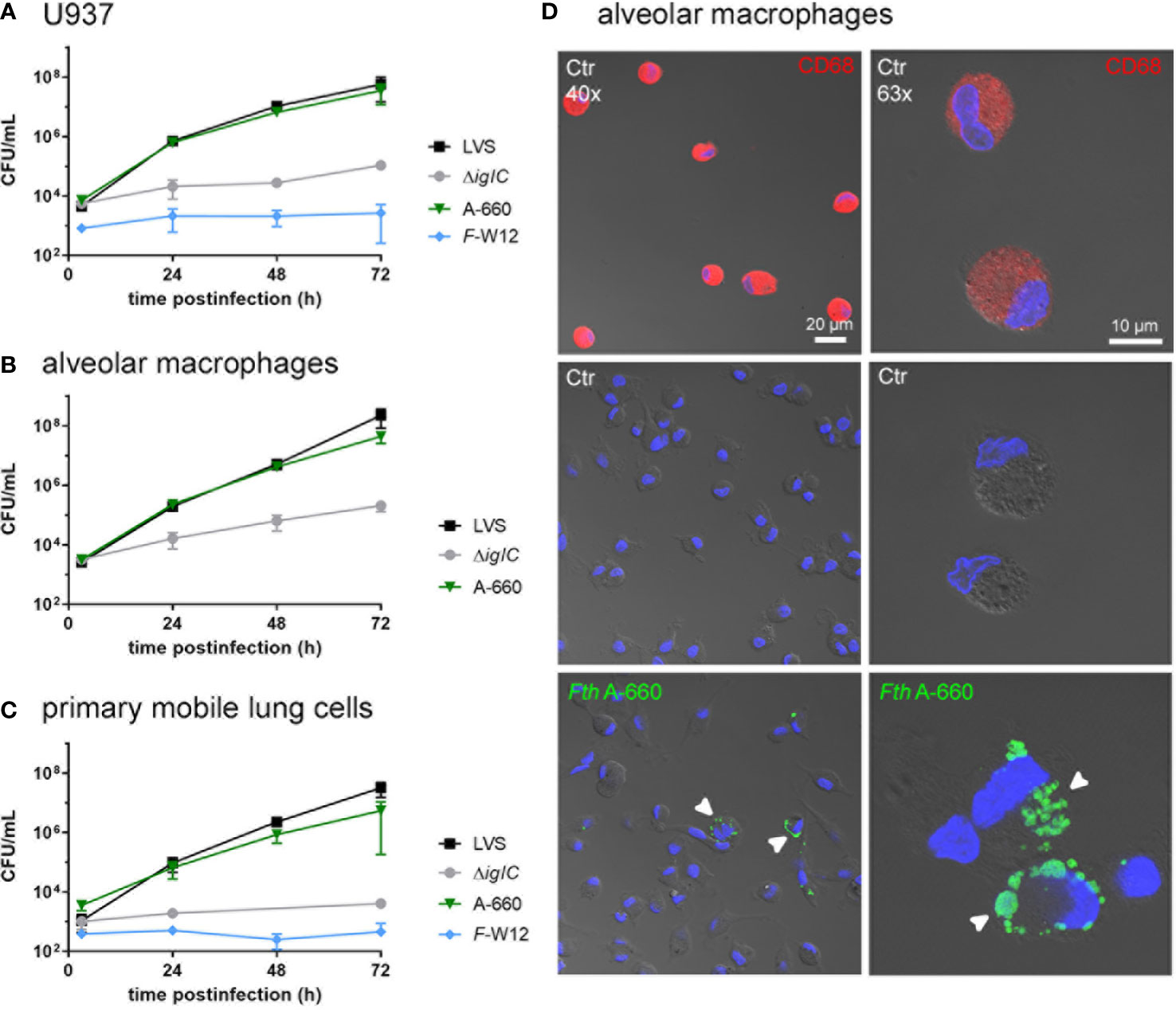
Figure 5 Infection of macrophage-like cell line U937 (A), primarily isolated human alveolar macrophages (B) and primary mobile cells of human lung tissue (C) with Francisella. Cells were infected with Fth LVS, Fth LVS ΔiglC, Fth wild-type A-660 and F-W12 for 2 h (MOI = 10). Gentamicin (50 µg/mL for 1 h) was used to eliminate remaining extracellular bacteria. At various time points of infection, the CFU/mL was determined by lysing the cells and plating suspension onto agar plates. Means with standard deviation are shown. n = 3. (D) Fth wild-type A-660 (Fth A-660) replication in primary human alveolar macrophages (AM). AM, isolated from fresh human lung tissue, were cultivated for 2 days and infected with Fth A-660 (MOI = 10). After 2 hours bacterial suspension was removed and cells were treated with 50 µg/ml gentamicin for 1 h After 48 h of infection, AM were fixed and stained for macrophage specific cell marker CD68 (red), Fth A-660 (green), nuclei were counterstained with DAPI (blue) and cell structure was visualized with differential interference contrast. AM, positive for CD68 (upper panel), control, negative for Fth A-660 (middle panel) and 48 h infected (lower panel). White arrowheads point to Fth A-660. Scale bar represents 10 and 20 µm. Representative figures of three independent experiments are shown.
3.4 Co-infection of U937 macrophages and human lung explants by Fth LVS and Fth A-660
Having observed differences in intracellular growth of Fth LVS and Fth A-660 depending on the infection model used, ex vivo (lung explants) or in vitro (U937), we tested both models by co-infection assays. U937 cells were simultaneously infected with both strains at a ratio of 1:1 representing a MOI of 10 in total. Cells were infected for 2 h and subsequently treated with gentamicin for 1 h. After 3 h, 24 h, 48 h and 72 h of infection, the CFU/mL was determined by lysing cells and plating onto MTKH agar plates partly supplemented with erythromycin to distinguish the two strains. Fth LVS belongs to erythromycin-resistant biovar II, whereas Fth A-660 belongs to erythromycin-sensitive biovar I. In Figure 6A, the CFU percentage of Fth LVS and Fth A-660 per 5 × 105 U937 cells is shown. After infection and gentamicin treatment (3 h), LVS represented 59.9% and A-660 40.1% of bacteria obtained intracellularly in U937 macrophages (p = 0.002). In the course of infection, the percentage of LVS continuously increased up to 93.2% after 72 h (p < 0.000001). Thus, LVS significantly outcompeted A-660 during a co-infection in U937 macrophages. In contrast, the A-660 wild-type strain outcompeted the LVS strain during a co-infection of human lung explants by both strains (Figure 6B). Human lung explants were equally infected with both strains after 3 h (LVS: 50.8%; A-660: 49.2%). After 24 h of co-infection, LVS represented a significantly higher percentage in the explants (69.1%, p = 0.0029), whereas the proportion of A-660 continuously increased up to 73.5% after 48 h and 72 h (72h: p = 0.0231). Hence, the attenuated phenotype (reduced virulence) of Fth LVS is only detectable in co-infections using human lung explants.
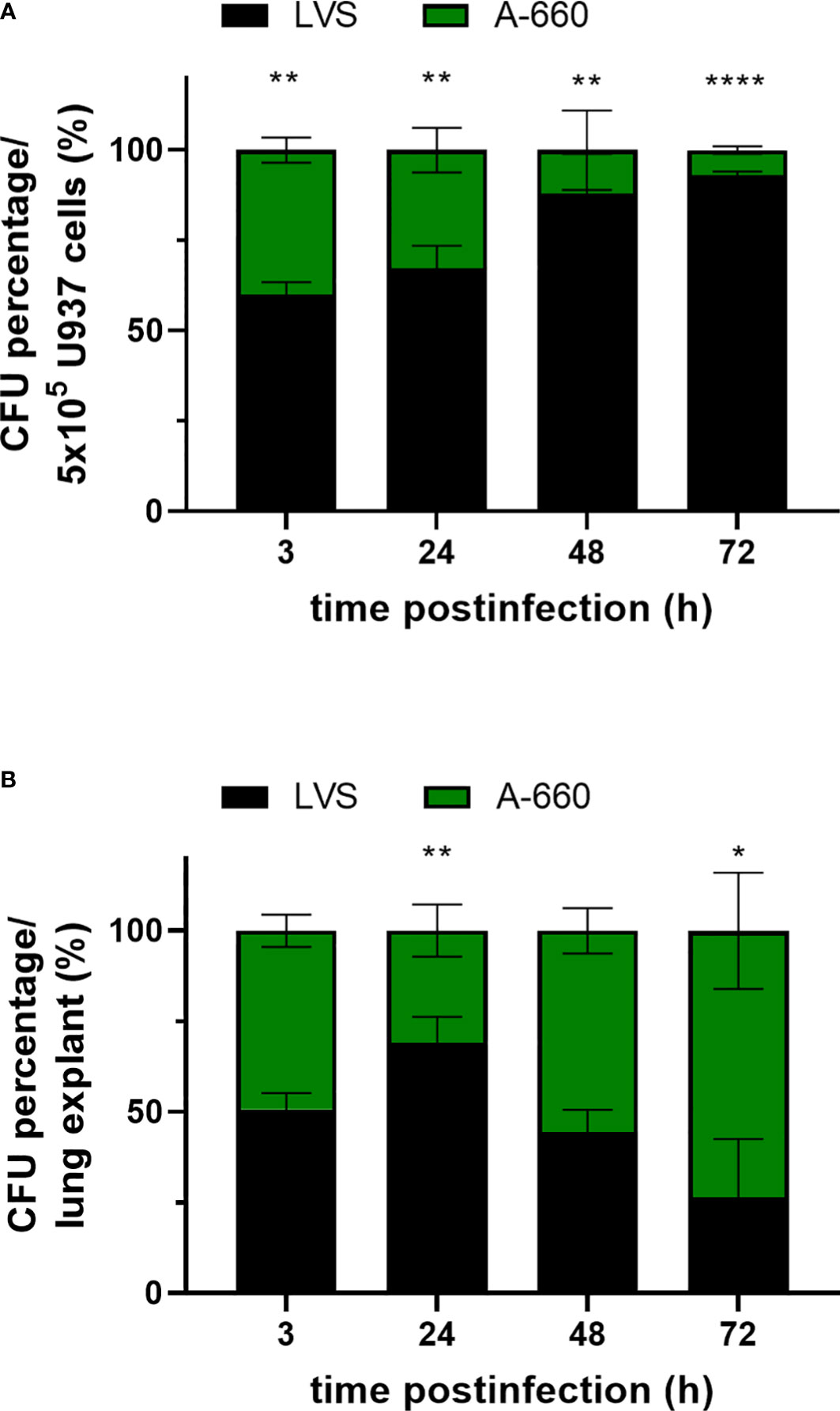
Figure 6 (A) Co-infection assay using U937 cells. Macrophages were competitively infected with Fth LVS and Fth A-660 (MOI = 10) for 2 h and treated afterwards with gentamicin (50 µg/mL for 1 h). At various time points, the CFU was determined by lysing U937 cells and plating onto MTKH agar plates partly supplemented with erythromycin to distinguish between Fth LVS (resistant) and Fth A-660 (sensitive). The percentage proportion of CFU per well (5 × 105cells) is shown for Fth LVS (black) and Fth A-660 (green). (B) Co-infection assay using human lung tissue explants. Explants were competitively infected with Fth LVS and Fth A-660, as described in figure legend 1. The percentage of CFU per lung explant is shown for Fth LVS (black) and Fth A-660 (green). Means with standard deviation of three (A) and four (B) independent experiments, respectively, are shown and statistical analysis were performed using a two-tailed t test with *P < 0.05; **P < 0.001; ***P < 0.0001; ****P < 0.00001.
4 Discussion
In this study, we successfully adapted a human lung ex vivo infection model for analysis of Francisella strains, including Fth and an environmental Francisella strain. In addition to Fth LVS, we used Fth strain A-660 obtained from a patient suffering from pulmonic tularemia (Appelt et al., 2019). Fth LVS is an attenuated laboratory strain and often used to understand virulence and pathogenicity of Francisella (Fortier et al., 1991; Rick Lyons and Wu, 2007; Hall et al., 2008). In contrast, Fth A-660 represents a virulent wild-type strain occurring naturally and having caused tularemia in a patient. However, wild-type strains of Fth have rarely been examined in Francisella studies (Rick Lyons and Wu, 2007). In our ex vivo infection model, Fth LVS and six Fth wild-type strains replicated in human lung explants (Figures 2A, B). The observed replication is assumed to be intracellular, since the Fth LVS ΔiglC mutant strain, which has been shown to be unable to replicate intracellularly (Golovliov et al., 1997; Golovliov et al., 2003; Lindgren et al., 2004; Santic et al., 2005), did not multiply in the model (Figure 2A). An FPI-null mutant strain of F. novicida is able to grow extracellularly, when it is in contact with host cells supporting the conception that intracellular growth of Fth occurs in human lung explants (Rytter et al., 2021). We observed a slightly enhanced growth of A-660 in human lung explants compared to LVS (Figure 2A), but a similar replication of both strains in human U937 macrophages and primary alveolar macrophages (Figures 4A–C). In contrast, an Fth strain-specific virulence has been indicated by other studies using human monocytes (THP-1), murine macrophages (J774A.1; (Matz and Petrosino, 2021)) and a co-culture of human hepatocytes and macrophages (Rennert et al., 2016).These findings can, however, only be considered comparable with our results to a certain extent due to different cell types and experimental procedures used. To investigate Francisella virulence different models has been used so far, including animal models (see above and below), the Fruit Fly (Drosophila melanogaster (Vonkavaara et al., 2008; Ahlund et al., 2010; Asare et al., 2010), the larvae of the Greater Wax Moth (Galleria mellonella, (Aperis et al., 2007; Thelaus et al., 2018; Asai et al., 2023)) and Dictyostelium discoideum (Lampe et al., 2015; Brenz et al., 2018), as well as cell-dependent models such as Drosophila S2 cells (Santic et al., 2009; Asare et al., 2010) and macrophages (Qin and Mann, 2006; Maier et al., 2007; Rasmussen et al., 2015; Matz and Petrosino, 2021). However, it is always the question of transferability of results obtained by animal- or cell-line models to the human host.
The human lung ex vivo infection model clearly underpinned the higher virulence of the Fth wild-type (shown by a higher multiplication rate in human lung explants than LVS). Especially, when human lung explants were simultaneously challenged with both Fth strains, the wild-type A-660 outcompeted LVS demonstrating its higher virulence (Figure 6). Generally, the virulence of Fth depends on the route of infection and the bacterial dose; and is relatively low for humans when the pathogen is inhaled (Saslaw et al., 1961a; Saslaw et al., 1961b; Tigertt, 1962; Hall et al., 2008). After intranasal and aerosol uptake, 500 - 104 Fth bacteria are needed for LD100 in mice and 108 Fth LVS bacteria for a human infection (Saslaw et al., 1961a; Tigertt, 1962; Fortier et al., 1991; Duckett et al., 2005; Wu et al., 2005; Bosio et al., 2007). In our ex vivo model, the bacterial growth of Francisella increased up to 1.5-log levels which is lower compared to growth of other bacterial pathogens, such as e.g. pneumococci, which showed an increase of 3-log levels (Szymanski et al., 2012). Perspectivity, it might be interesting to determine the minimal doses for a successful growth in human lung explants and, thus, to determine the minimal infectious doses of diverse Francisella strains, particularly of highly virulent Ftt.
Using the human lung ex vivo infection model, we demonstrated that the environmental aquatic Francisella species F-W12 was not able to grow in the explants but could survive over three days similarly to Fth LVS ΔiglC strain. In fact, F-W12 does not exhibit the Francisella pathogenicity island encoding a type VI secretion system (Rydzewski et al., 2014). On the other hand, an alternative putative type VI secretion system has been identified in silico within its genome and several virulence factors has been found and experimentally confirmed (Köppen et al., 2019). However, this species does not seem to be as virulent as Fth and its putative pathogenicity for humans still needs to be verified.
In this study, we also aimed to identify the human lung cell types involved in human lung infections caused by Francisella. Spectral immunofluorescence and electron microscopy of Fth A-660 infected lung explants revealed that Fth A-660 was mainly detected in alveolar macrophages. Additionally, lymphocytes, granulocytes and fibrocytes have sporadically been infected by Fth (Figures 3A–C, S3). So far, only mouse (Bosio and Dow, 2005; Bosio et al., 2007; Forestal et al., 2007; Gentry et al., 2007; Hall et al., 2008; Mares et al., 2008; Yu et al., 2008) and primate infection models (Eigelsbach et al., 1962; White et al., 1964; Hall et al., 1973) have been used to identify the cell types involved in Francisella infection. Here, it was shown that the most infected cell types are alveolar macrophages, in particular at an early stage of infection (Hall et al., 2008). In mice, also other cell types are used for replication by Francisella over time, including dendritic cells and neutrophils, whereby the latter become the most-infected cell type in later stages of infection (Hall et al., 2008). The human lung ex vivo infection model does not allow an investigation of adaptive immune response and leukocyte recruitment. Therefore, further experiments are needed to study more closely intracellular replication niches of Francisella in dendritic cells or neutrophils. Furthermore, Francisella is able to infect human and mouse lung alveolar epithelial type II cells in vitro and in vivo (Gentry et al., 2007; Hall et al., 2007; Craven et al., 2008; Faron et al., 2015). Faron et al. hypothesized that after entry of Francisella into lung alveoli, bacteria infect and replicate either in alveolar macrophages or in alveolar epithelial type II cells (Faron et al., 2015). Both events lead to the epithelial barrier being crossed and thus, allow an interaction with surrounding cells, including endothelial cells. Consequently, Francisella bacteria are able to enter the lung blood capillaries and subsequently the blood stream. Although Francisella are not able to grow intracellularly in erythrocytes, the bacteria can use these to spread into the whole body (Horzempa et al., 2011; Schmitt et al., 2017; Cantlay et al., 2022). Francisella has also been shown to exist cell-free and extracellularly in blood and necrotic lesions in the lungs of infected mice (Bosio et al., 2007; Forestal et al., 2007; Yu et al., 2008). We also detected extracellular Francisella bacteria in the connective lung tissue. That partly supports the model of Francisella infection within the lung by Faron et al., although we did neither find infected alveolar epithelial type II cells nor endothelial cells in the investigated lung explants so far.
We also assessed how Francisella activated the host immune response in ex vivo infected human lung tissue. Cytokine and chemokine levels in lung explants from different donors and even from the same donor varied more or less. Moreover, variations were not only observed in supernatants of infected explants, but also of non-infected control explants (see Figure S2). Another variation has been shown in a study investigating cytokine response of naturally acquired tularemia among a group of nine hare hunters (Jacob et al., 2020). Although cytokine response (IL-8, IP-10, MCP-1 and MIP-1α) showed considerable changes during the acute phase of infection, this remained statistically insignificant. Among the tendencies in cytokine response revealed by cytokine measurements conducted in our study, it could be demonstrated that secretion of IL-1β, IL-6, MCP-1 and IP-10, known as proinflammatory stimulators produced by macrophages, seemed to be slightly lower in explants infected by Fth A-660 compared to those infected by Fth LVS (see Figure 4). Our data are consistent with other studies showing an active suppression of the immune response and cytokine release of virulent Francisella strains compared to non- or less-virulent strains in the early phase of infection (Bosio and Dow, 2005; Mares et al., 2008; Gillette et al., 2014). In particular, the upregulation of proinflammatory TNF-α is delayed by an active suppression after Francisella infection. This Francisella-induced active immune repression might explain the generally low levels of cytokine in supernatants of Francisella infected lung explants in our model compared to published data based on diverse pathogens, such as e.g. S. pneumoniae (e.g. TNF-α: max. 30000 pg/g lung tissue; IL-1β: max. of 40000 pg/g lung tissue; IL-8: max. of 5000 ng/g tissue; IL-6: max. of 3000 ng/g tissue (Szymanski et al., 2012; Fatykhova et al., 2015; Berg et al., 2017)). Importantly, the immune response suppression was not observed for the environmental strain F-W12 (Figures 4, S2). Here, we detected cytokine levels that rose up to 40 times higher.
5 Conclusion
For the first time, we successfully implemented an ex vivo infection model using human lung tissue for Francisella. In contrast to a 2D single cell type infection model, this model mimics the 3D in vivo situation with diverse cell types responding to Francisella on a small scale. This enabled us to detect the specific ability of wild-type A-660 to replicate within the cells and tissue, as well as to manipulate the immune response. Thus, the human lung ex vivo model is apt to be used to discriminate virulent from less- or non-virulent Francisella species and to investigate the role of specific virulence factors.
Data availability statement
The original contributions presented in the study are included in the article/Supplementary Material. Further inquiries can be directed to the corresponding authors.
Ethics statement
The studies involving human participants were reviewed and approved by Ethics committee of the Charité - Universitätsmedizin Berlin, Germany (project EA2/079/13). The patients/participants provided their written informed consent to participate in this study.
Author contributions
KH and KK provided the expertise in the field of Francisella. DF, SH and AH provided the expertise in the field of human lung ex vivo infection models. Theoretical and practical advices were given by KH, KK, DF, SH and AH. KH and SH coordinated and supervised the present work. Human lung explants were provided by MG and DF. KK, DF, HG, JR and KR performed the experiments and analyzed the data. KK and KH drafted the manuscript. DF, GH, JR, DT, AH and SH revised the manuscript critically. All authors contributed to the article and approved the submitted version.
Funding
This work received financial support from the Robert Koch Institute. SH and AH were supported by DFG (SFB-TR 84, project A04, B06) and project Z01 (to AH). AH and SH were supported by Charité 3R and by Einstein Foundation EC3R.
Acknowledgments
We would like to thank Dr. Michael Laue and Anna Rohleder for the critical and linguistical revision of the manuscript.
Conflict of interest
The authors declare that the research was conducted in the absence of any commercial or financial relationships that could be construed as a potential conflict of interest.
Publisher’s note
All claims expressed in this article are solely those of the authors and do not necessarily represent those of their affiliated organizations, or those of the publisher, the editors and the reviewers. Any product that may be evaluated in this article, or claim that may be made by its manufacturer, is not guaranteed or endorsed by the publisher.
Supplementary material
The Supplementary Material for this article can be found online at: https://www.frontiersin.org/articles/10.3389/fcimb.2023.1224356/full#supplementary-material
References
Ahlund, M. K., Rydén, P., Sjöstedt, A., Stöven, S. (2010). Directed screen of francisella novicida virulence determinants using drosophila melanogaster. Infect. Immun. 78, 3118–3128. doi: 10.1128/IAI.00146-10
Anthony, L. D., Burke, R. D., Nano, F. E. (1991). Growth of francisella spp. in rodent macrophages. Infect. Immun. 59, 3291–3296. doi: 10.1128/iai.59.9.3291-3296.1991
Aperis, G., Fuchs, B. B., Anderson, C. A., Warner, J. E., Calderwood, S. B., Mylonakis, E. (2007). Galleria mellonella as a model host to study infection by the francisella tularensis live vaccine strain. Microbes Infect. 9, 729–734. doi: 10.1016/j.micinf.2007.02.016
Appelt, S., Köppen, K., Radonic, A., Drechsel, O., Jacob, D., Grunow, R., et al. (2019). Genetic diversity and spatial segregation of francisella tularensis subspecies holarctica in Germany. Front. Cell Infect. Microbiol. 9, 376. doi: 10.3389/fcimb.2019.00376
Asai, M., Li, Y., Newton, S. M., Robertson, B. D., Langford, P. R. (2023). Galleria mellonella-intracellular bacteria pathogen infection models: the ins and outs. FEMS Microbiol. Rev. 47, 1–32. doi: 10.1093/femsre/fuad011
Asare, R., Akimana, C., Jones, S., Abu Kwaik, Y. (2010). Molecular bases of proliferation of francisella tularensis in arthropod vectors. Environ. Microbiol. 12, 2587–2612. doi: 10.1111/j.1462-2920.2010.02230.x
Bandouchova, H., Sedlackova, J., Pohanka, M., Novotny, L., Hubalek, M., Treml, F., et al. (2009). Tularemia induces different biochemical responses in BALB/c mice and common voles. BMC Infect. Dis. 9, 101. doi: 10.1186/1471-2334-9-101
Becker, S., Lochau, P., Jacob, D., Heuner, K., Grunow, R. (2016). Successful re-evaluation of broth medium T for growth of francisella tularensis ssp. and other highly pathogenic bacteria. J. Microbiol. Methods 121, 5–7. doi: 10.1016/j.mimet.2015.11.018
Ben Nasr, A., Haithcoat, J., Masterson, J. E., Gunn, J. S., Eaves-Pyles, T., Klimpel, G. R. (2006). Critical role for serum opsonins and complement receptors CR3 (CD11b/CD18) and CR4 (CD11c/CD18) in phagocytosis of francisella tularensis by human dendritic cells (DC): uptake of francisella leads to activation of immature DC and intracellular survival of the bacteria. J. Leukoc. Biol. 80, 774–786. doi: 10.1189/jlb.1205755
Berg, J., Zscheppang, K., Fatykhova, D., Tonnies, M., Bauer, T. T., Schneider, P., et al. (2017). Tyk2 as a target for immune regulation in human viral/bacterial pneumonia. Eur. Respir. J. 50. doi: 10.1183/13993003.01953-2016
Birdsell, D. N., Stewart, T., Vogler, A. J., Lawaczeck, E., Diggs, A., Sylvester, T. L., et al. (2009). Francisella tularensis subsp. novicida isolated from a human in Arizona. BMC Res. Notes 2, 223. doi: 10.1186/1756-0500-2-223
Booth, J. L., Duggan, E. S., Patel, V. I., Langer, M., Wu, W., Braun, A., et al. (2016). Bacillus anthracis spore movement does not require a carrier cell and is not affected by lethal toxin in human lung models. Microbes Infect. 18, 615–626. doi: 10.1016/j.micinf.2016.06.004
Bosio, C. M., Bielefeldt-Ohmann, H., Belisle, J. T. (2007). Active suppression of the pulmonary immune response by francisella tularensis Schu4. J. Immunol. 178, 4538–4547. doi: 10.4049/jimmunol.178.7.4538
Bosio, C. M., Dow, S. W. (2005). Francisella tularensis induces aberrant activation of pulmonary dendritic cells. J. Immunol. 175, 6792–6801. doi: 10.4049/jimmunol.175.10.6792
Brenz, Y., Winther-Larsen, H. C., Hagedorn, M. (2018). Expanding francisella models: pairing up the soil amoeba dictyostelium with aquatic francisella. Int. J. Med. Microbiol. 308, 32–40. doi: 10.1016/j.ijmm.2017.08.001
Brett, M. E., Respicio-Kingry, L. B., Yendell, S., Ratard, R., Hand, J., Balsamo, G., et al. (2014). Outbreak of francisella novicida bacteremia among inmates at a louisiana correctional facility. Clin. Infect. Dis. 59, 826–833. doi: 10.1093/cid/ciu430
Bröms, J. E., Sjöstedt, A., Lavander, M. (2010). The role of the francisella tularensis pathogenicity island in type VI secretion, intracellular survival, and modulation of host cell signaling. Front. Microbiol. 1, 136. doi: 10.3389/fmicb.2010.00136
Cantlay, S., Kaftanic, C., Horzempa, J. (2022). PdpC, a secreted effector protein of the type six secretion system, is required for erythrocyte invasion by francisella tularensis LVS. Front. Cell Infect. Microbiol. 12, 979693. doi: 10.3389/fcimb.2022.979693
Clemens, D. L., Lee, B. Y., Horwitz, M. A. (2004). Virulent and avirulent strains of francisella tularensis prevent acidification and maturation of their phagosomes and escape into the cytoplasm in human macrophages. Infect. Immun. 72, 3204–3217. doi: 10.1128/IAI.72.6.3204-3217.2004
Clemens, D. L., Lee, B. Y., Horwitz, M. A. (2018). The francisella type VI secretion system. Front. Cell Infect. Microbiol. 8, 121. doi: 10.3389/fcimb.2018.00121
Craven, R. R., Hall, J. D., Fuller, J. R., Taft-Benz, S., Kawula, T. H. (2008). Francisella tularensis invasion of lung epithelial cells. Infect. Immun. 76, 2833–2842. doi: 10.1128/IAI.00043-08
Dennis, D. T., Inglesby, T. V., Henderson, D. A., Bartlett, J. G., Ascher, M. S., Eitzen, E., et al. (2001). Tularemia as a biological weapon: medical and public health management. JAMA 285, 2763–2773. doi: 10.1001/jama.285.21.2763
Duckett, N. S., Olmos, S., Durrant, D. M., Metzger, D. W. (2005). Intranasal interleukin-12 treatment for protection against respiratory infection with the francisella tularensis live vaccine strain. Infect. Immun. 73, 2306–2311. doi: 10.1128/IAI.73.4.2306-2311.2005
Eigelsbach, H. T., Tulis, J. J., Mcgavran, M. H., White, J. D. (1962). LIVE TULAREMIA VACCINE i. : host-parasite relationship in monkeys vaccinated intracutaneously or aerogenically. J. Bacteriol. 84, 1020–1027. doi: 10.1128/jb.84.5.1020-1027.1962
Ellis, J., Oyston, P. C., Green, M., Titball, R. W. (2002). Tularemia. Clin. Microbiol. Rev. 15, 631–646. doi: 10.1128/CMR.15.4.631-646.2002
Escudero, R., Elia, M., Saez-Nieto, J. A., Menendez, V., Toledo, A., Royo, G., et al. (2010). A possible novel francisella genomic species isolated from blood and urine of a patient with severe illness. Clin. Microbiol. Infect. 16, 1026–1030. doi: 10.1111/j.1469-0691.2009.03029.x
Faron, M., Fletcher, J. R., Rasmussen, J. A., Apicella, M. A., Jones, B. D. (2015). Interactions of francisella tularensis with alveolar type II epithelial cells and the murine respiratory epithelium. PloS One 10, e0127458. doi: 10.1371/journal.pone.0127458
Fatykhova, D., Rabes, A., Machnik, C., Guruprasad, K., Pache, F., Berg, J., et al. (2015). Serotype 1 and 8 pneumococci evade sensing by inflammasomes in human lung tissue. PloS One 10, e0137108. doi: 10.1371/journal.pone.0137108
Foley, J. E., Nieto, N. C. (2010). Tularemia. Vet. Microbiol. 140, 332–338. doi: 10.1016/j.vetmic.2009.07.017
Forestal, C. A., Malik, M., Catlett, S. V., Savitt, A. G., Benach, J. L., Sellati, T. J., et al. (2007). Francisella tularensis has a significant extracellular phase in infected mice. J. Infect. Dis. 196, 134–137. doi: 10.1086/518611
Fortier, A. H., Slayter, M. V., Ziemba, R., Meltzer, M. S., Nacy, C. A. (1991). Live vaccine strain of francisella tularensis: infection and immunity in mice. Infect. Immun. 59, 2922–2928. doi: 10.1128/iai.59.9.2922-2928.1991
Froböse, N. J., Masjosthusmann, K., Huss, S., Correa-Martinez, C. L., Mellmann, A., Schuler, F., et al. (2020). A child with soft-tissue infection and lymphadenitis. New Microbes New Infect. 38, 100819. doi: 10.1016/j.nmni.2020.100819
Ganbat, D., Seehase, S., Richter, E., Vollmer, E., Reiling, N., Fellenberg, K., et al. (2016). Mycobacteria infect different cell types in the human lung and cause species dependent cellular changes in infected cells. BMC Pulm. Med. 16, 19. doi: 10.1186/s12890-016-0185-5
Gentry, M., Taormina, J., Pyles, R. B., Yeager, L., Kirtley, M., Popov, V. L., et al. (2007). Role of primary human alveolar epithelial cells in host defense against francisella tularensis infection. Infect. Immun. 75, 3969–3978. doi: 10.1128/IAI.00157-07
Gillette, D. D., Curry, H. M., Cremer, T., Ravneberg, D., Fatehchand, K., Shah, P. A., et al. (2014). Virulent type a francisella tularensis actively suppresses cytokine responses in human monocytes. Front. Cell Infect. Microbiol. 4, 45. doi: 10.3389/fcimb.2014.00045
Glynn, A. R., Alves, D. A., Frick, O., Erwin-Cohen, R., Porter, A., Norris, S., et al. (2015). Comparison of experimental respiratory tularemia in three nonhuman primate species. Comp. Immunol. Microbiol. Infect. Dis. 39, 13–24. doi: 10.1016/j.cimid.2015.01.003
Golovliov, I., Ericsson, M., Sandstrom, G., Tarnvik, A., Sjostedt, A. (1997). Identification of proteins of francisella tularensis induced during growth in macrophages and cloning of the gene encoding a prominently induced 23-kilodalton protein. Infect. Immun. 65, 2183–2189. doi: 10.1128/iai.65.6.2183-2189.1997
Golovliov, I., Sjostedt, A., Mokrievich, A., Pavlov, V. (2003). A method for allelic replacement in francisella tularensis. FEMS Microbiol. Lett. 222, 273–280. doi: 10.1016/S0378-1097(03)00313-6
Graham, J. G., Winchell, C. G., Kurten, R. C., Voth, D. E. (2016). Development of an ex vivo tissue platform to study the human lung response to coxiella burnetii. Infect. Immun. 84, 1438–1445. doi: 10.1128/IAI.00012-16
Grund, C., Hoffmann, D., Ulrich, R., Naguib, M., Schinkothe, J., Hoffmann, B., et al. (2018). A novel European H5N8 influenza a virus has increased virulence in ducks but low zoonotic potential. Emerg. Microbes Infect. 7, 132. doi: 10.1038/s41426-018-0130-1
Grunow, R., Splettstoesser, W., Mcdonald, S., Otterbein, C., O'brien, T., Morgan, C., et al. (2000). Detection of francisella tularensis in biological specimens using a capture enzyme-linked immunosorbent assay, an immunochromatographic handheld assay, and a PCR. Clin. Diagn. Lab. Immunol. 7, 86–90. doi: 10.1128/CDLI.7.1.86-90.2000
Hall, J. D., Craven, R. R., Fuller, J. R., Pickles, R. J., Kawula, T. H. (2007). Francisella tularensis replicates within alveolar type II epithelial cells in vitro and in vivo following inhalation. Infect. Immun. 75, 1034–1039. doi: 10.1128/IAI.01254-06
Hall, W. C., Kovatch, R. M., Schricker, R. L. (1973). Tularaemic pneumonia: pathogenesis of the aerosol-induced disease in monkeys. J. Pathol. 110, 193–201. doi: 10.1002/path.1711100302
Hall, J. D., Woolard, M. D., Gunn, B. M., Craven, R. R., Taft-Benz, S., Frelinger, J. A., et al. (2008). Infected-host-cell repertoire and cellular response in the lung following inhalation of francisella tularensis schu S4, LVS, or U112. Infect. Immun. 76, 5843–5852. doi: 10.1128/IAI.01176-08
Hennebique, A., Caspar, Y., Maurin, M., Boisset, S., Pelloux, I., Gallego-Hernanz, M. P., et al. (2022). Ulceroglandular infection and bacteremia caused by francisella salimarina in immunocompromised patient, France. Emerg. Infect. Dis. 28, 465–467. doi: 10.3201/eid2802.211380
Hocke, A. C., Becher, A., Knepper, J., Peter, A., Holland, G., Tonnies, M., et al. (2013). Emerging human middle East respiratory syndrome coronavirus causes widespread infection and alveolar damage in human lungs. Am. J. Respir. Crit. Care Med. 188, 882–886. doi: 10.1164/rccm.201305-0954LE
Hollis, D. G., Weaver, R. E., Steigerwalt, A. G., Wenger, J. D., Moss, C. W., Brenner, D. J. (1989). Francisella philomiragia comb. nov. (formerly yersinia philomiragia) and francisella tularensis biogroup novicida (formerly francisella novicida) associated with human disease. J. Clin. Microbiol. 27, 1601–1608. doi: 10.1128/jcm.27.7.1601-1608.1989
Hönzke, K., Obermayer, B., Mache, C., Fathykova, D., Kessler, M., Dokel, S., et al. (2022). Human lungs show limited permissiveness for SARS-CoV-2 due to scarce ACE2 levels but virus-induced expansion of inflammatory macrophages. Eur. Respir. J. 60, 210275. doi: 10.1183/13993003.02725-2021
Horzempa, J., O'dee, D. M., Shanks, R. M., Nau, G. J. (2010). Francisella tularensis DeltapyrF mutants show that replication in nonmacrophages is sufficient for pathogenesis in vivo. Infect. Immun. 78, 2607–2619. doi: 10.1128/IAI.00134-10
Horzempa, J., O'dee, D. M., Stolz, D. B., Franks, J. M., Clay, D., Nau, G. J. (2011). Invasion of erythrocytes by francisella tularensis. J. Infect. Dis. 204, 51–59. doi: 10.1093/infdis/jir221
Jacob, D., Barduhn, A., Tappe, D., Rauch, J., Heuner, K., Hierhammer, D., et al. (2020). Outbreak of tularemia in a group of hunters in Germany in 2018-kinetics of antibody and cytokine responses. Microorganisms 8, 1645. doi: 10.3390/microorganisms8111645
Jäger, J., Marwitz, S., Tiefenau, J., Rasch, J., Shevchuk, O., Kugler, C., et al. (2014). Human lung tissue explants reveal novel interactions during legionella pneumophila infections. Infect. Immun. 82, 275–285. doi: 10.1128/IAI.00703-13
Knepper, J., Schierhorn, K. L., Becher, A., Budt, M., Tonnies, M., Bauer, T. T., et al. (2013). The novel human influenza A(H7N9) virus is naturally adapted to efficient growth in human lung tissue. mBio 4, e00601–e00613. doi: 10.1128/mBio.00601-13
Köppen, K., Chen, F., Rydzewski, K., Einenkel, R., Bottcher, T., Morguet, C., et al. (2019). Screen for fitness and virulence factors of francisella sp. strain W12-1067 using amoebae. Int. J. Med. Microbiol. 309, 151341. doi: 10.1016/j.ijmm.2019.151341
Kostiala, A. A., Mcgregor, D. D., Logie, P. S. (1975). Tularaemia in the rat. i. the cellular basis on host resistance to infection. Immunology 28, 855–869.
Kreitmann, L., Terriou, L., Launay, D., Caspar, Y., Courcol, R., Maurin, M., et al. (2015). Disseminated infection caused by francisella philomiragia, France 2014. Emerg. Infect. Dis. 21, 2260–2261. doi: 10.3201/eid2112.150615
Lampe, E. O., Brenz, Y., Herrmann, L., Repnik, U., Griffiths, G., Zingmark, C., et al. (2015). Dissection of francisella-host cell interactions in dictyostelium discoideum. Appl. Environ. Microbiol. 82, 1586–1598. doi: 10.1128/AEM.02950-15
Lindgren, H., Golovliov, I., Baranov, V., Ernst, R. K., Telepnev, M., Sjostedt, A. (2004). Factors affecting the escape of francisella tularensis from the phagolysosome. J. Med. Microbiol. 53, 953–958. doi: 10.1099/jmm.0.45685-0
Maier, T. M., Casey, M. S., Becker, R. H., Dorsey, C. W., Glass, E. M., Maltsev, N., et al. (2007). Identification of francisella tularensis Himar1-based transposon mutants defective for replication in macrophages. Infect. Immun. 75, 5376–5389. doi: 10.1128/IAI.00238-07
Mailman, T. L., Schmidt, M. H. (2005). Francisella philomiragia adenitis and pulmonary nodules in a child with chronic granulomatous disease. Can. J. Infect. Dis. Med. Microbiol. 16, 245–248. doi: 10.1155/2005/486417
Mares, C. A., Ojeda, S. S., Morris, E. G., Li, Q., Teale, J. M. (2008). Initial delay in the immune response to francisella tularensis is followed by hypercytokinemia characteristic of severe sepsis and correlating with upregulation and release of damage-associated molecular patterns. Infect. Immun. 76, 3001–3010. doi: 10.1128/IAI.00215-08
Matz, L. M., Petrosino, J. F. (2021). A study of innate immune kinetics reveals a role for a chloride transporter in a virulent francisella tularensis type b strain. Microbiologyopen 10, e1170. doi: 10.1002/mbo3.1170
Maurin, M. (2015). Francisella tularensis as a potential agent of bioterrorism? Expert Rev. Anti Infect. Ther. 13, 141–144. doi: 10.1586/14787210.2015.986463
Maurin, M., Gyuranecz, M. (2016). Tularaemia: clinical aspects in Europe. Lancet Infect. Dis. 16, 113–124. doi: 10.1016/S1473-3099(15)00355-2
Mccrumb, F. R. (1961). Aerosol infection of man with pasteurella tularensis. Bacteriol. Rev. 25, 262–267. doi: 10.1128/br.25.3.262-267.1961
Melillo, A., Sledjeski, D. D., Lipski, S., Wooten, R. M., Basrur, V., Lafontaine, E. R. (2006). Identification of a francisella tularensis LVS outer membrane protein that confers adherence to A549 human lung cells. FEMS Microbiol. Lett. 263, 102–108. doi: 10.1111/j.1574-6968.2006.00413.x
Moreau, G. B., Mann, B. J. (2013). Adherence and uptake of francisella into host cells. Virulence 4, 826–832. doi: 10.4161/viru.25629
Nelson, M., Lever, M. S., Dean, R. E., Savage, V. L., Salguero, F. J., Pearce, P. C., et al. (2010). Characterization of lethal inhalational infection with francisella tularensis in the common marmoset (Callithrix jacchus). J. Med. Microbiol. 59, 1107–1113. doi: 10.1099/jmm.0.020669-0
Owen, C. R., Buker, E. O., Jellison, W. L., Lackman, D. B., Bell, J. F. (1964). Comparative studies of francisella tularensis and francisella novicida. J. Bacteriol. 87, 676–683. doi: 10.1128/jb.87.3.676-683.1964
Pavlovich, N. V., Mishan'kin, B. N. (1987). [Transparent nutrient medium for culturing francisella tularensis]. Antibiot. Med. Biotekhnol. 32, 133–137.
Peter, A., Fatykhova, D., Kershaw, O., Gruber, A. D., Rueckert, J., Neudecker, J., et al. (2017). Localization and pneumococcal alteration of junction proteins in the human alveolar-capillary compartment. Histochem. Cell Biol. 147, 707–719. doi: 10.1007/s00418-017-1551-y
Qin, A., Mann, B. J. (2006). Identification of transposon insertion mutants of francisella tularensis tularensis strain schu S4 deficient in intracellular replication in the hepatic cell line HepG2. BMC Microbiol. 6, 69. doi: 10.1186/1471-2180-6-69
Rasmussen, J. A., Fletcher, J. R., Long, M. E., Allen, L. A., Jones, B. D. (2015). Characterization of francisella tularensis schu S4 mutants identified from a transposon library screened for O-antigen and capsule deficiencies. Front. Microbiol. 6, 338. doi: 10.3389/fmicb.2015.00338
Ray, H. J., Chu, P., Wu, T. H., Lyons, C. R., Murthy, A. K., Guentzel, M. N., et al. (2010). The Fischer 344 rat reflects human susceptibility to francisella pulmonary challenge and provides a new platform for virulence and protection studies. PloS One 5, e9952. doi: 10.1371/journal.pone.0009952
Rennert, K., Otto, P., Funke, H., Huber, O., Tomaso, H., Mosig, A. S. (2016). A human macrophage-hepatocyte co-culture model for comparative studies of infection and replication of francisella tularensis LVS strain and subspecies holarctica and mediasiatica. BMC Microbiol. 16, 2. doi: 10.1186/s12866-015-0621-3
Rick Lyons, C., Wu, T. H. (2007). Animal models of francisella tularensis infection. Ann. N. Y. Acad. Sci. 1105, 238–265. doi: 10.1196/annals.1409.003
Roberts, L. M., Powell, D. A., Frelinger, J. A. (2018). Adaptive immunity to francisella tularensis and considerations for vaccine development. Front. Cell Infect. Microbiol. 8, 115. doi: 10.3389/fcimb.2018.00115
Rydzewski, K., Schulz, T., Brzuszkiewicz, E., Holland, G., Lück, C., Fleischer, J., et al. (2014). Genome sequence and phenotypic analysis of a first German francisella sp. isolate (W12-1067) not belonging to the species francisella tularensis. BMC Microbiol. 14, 169. doi: 10.1186/1471-2180-14-169
Rytter, H., Jamet, A., Ziveri, J., Ramond, E., Coureuil, M., Lagouge-Roussey, P., et al. (2021). The pentose phosphate pathway constitutes a major metabolic hub in pathogenic francisella. PloS Pathog. 17, e1009326. doi: 10.1371/journal.ppat.1009326
Santic, M., Akimana, C., Asare, R., Kouokam, J. C., Atay, S., Kwaik, Y. A. (2009). Intracellular fate of francisella tularensis within arthropod-derived cells. Environ. Microbiol. 11, 1473–1481. doi: 10.1111/j.1462-2920.2009.01875.x
Santic, M., Al-Khodor, S., Abu Kwaik, Y. (2010). Cell biology and molecular ecology of francisella tularensis. Cell Microbiol. 12, 129–139. doi: 10.1111/j.1462-5822.2009.01400.x
Santic, M., Molmeret, M., Klose, K. E., Jones, S., Kwaik, Y. A. (2005). The francisella tularensis pathogenicity island protein IglC and its regulator MglA are essential for modulating phagosome biogenesis and subsequent bacterial escape into the cytoplasm. Cell Microbiol. 7, 969–979. doi: 10.1111/j.1462-5822.2005.00526.x
Saslaw, S., Eigelsbach, H. T., Prior, J. A., Wilson, H. E., Carhart, S. (1961a). Tularemia vaccine study. II. respiratory challenge. Arch. Intern. Med. 107, 702–714. doi: 10.1001/archinte.1961.03620050068007
Saslaw, S., Eigelsbach, H. T., Wilson, H. E., Prior, J. A., Carhart, S. (1961b). Tularemia vaccine study. i. intracutaneous challenge. Arch. Intern. Med. 107, 689–701. doi: 10.1001/archinte.1961.03620050055006
Schmitt, D. M., Barnes, R., Rogerson, T., Haught, A., Mazzella, L. K., Ford, M., et al. (2017). The role and mechanism of erythrocyte invasion by francisella tularensis. Front. Cell Infect. Microbiol. 7, 173. doi: 10.3389/fcimb.2017.00173
Schulze, C., Heuner, K., Myrtennäs, K., Karlsson, E., Jacob, D., Kutzer, P., et al. (2016). High and novel genetic diversity of francisella tularensis in Germany and indication of environmental persistence. Epidemiol. Infect. 144, 3025–3036. doi: 10.1017/S0950268816001175
Schwartz, J. T., Barker, J. H., Long, M. E., Kaufman, J., Mccracken, J., Allen, L. A. (2012). Natural IgM mediates complement-dependent uptake of francisella tularensis by human neutrophils via complement receptors 1 and 3 in nonimmune serum. J. Immunol. 189, 3064–3077. doi: 10.4049/jimmunol.1200816
Staples, J. E., Kubota, K. A., Chalcraft, L. G., Mead, P. S., Petersen, J. M. (2006). Epidemiologic and molecular analysis of human tularemia, united states 1964-2004. Emerg. Infect. Dis. 12, 1113–1118. doi: 10.3201/eid1207.051504
Szymanski, K. V., Toennies, M., Becher, A., Fatykhova, D., N'guessan, P. D., Gutbier, B., et al. (2012). Streptococcus pneumoniae-induced regulation of cyclooxygenase-2 in human lung tissue. Eur. Respir. J. 40, 1458–1467. doi: 10.1183/09031936.00186911
Thelaus, J., Lundmark, E., Lindgren, P., Sjodin, A., Forsman, M. (2018). Galleria mellonella reveals niche differences between highly pathogenic and closely related strains of francisella spp. Front. Cell Infect. Microbiol. 8, 188. doi: 10.3389/fcimb.2018.00188
Tigertt, W. D. (1962). Soviet viable pasteurella tularensis vaccines. a review of selected articles. Bacteriol. Rev. 26, 354–373. doi: 10.1128/br.26.3.354-373.1962
Tlapak, H., Köppen, K., Rydzewski, K., Grunow, R., Heuner, K. (2018). Construction of a new phage integration vector pFIV-Val for use in different francisella species. Front. Cell Infect. Microbiol. 8, 75. doi: 10.3389/fcimb.2018.00075
Twenhafel, N. A., Alves, D. A., Purcell, B. K. (2009). Pathology of inhalational francisella tularensis spp. tularensis SCHU S4 infection in African green monkeys (Chlorocebus aethiops). Vet. Pathol. 46, 698–706. doi: 10.1354/vp.08-VP-0302-T-AM
Vonkavaara, M., Telepnev, M. V., Ryden, P., Sjostedt, A., Stoven, S. (2008). Drosophila melanogaster as a model for elucidating the pathogenicity of francisella tularensis. Cell Microbiol. 10, 1327–1338. doi: 10.1111/j.1462-5822.2008.01129.x
Vuorte, J., Jansson, S. E., Repo, H. (2001). Evaluation of red blood cell lysing solutions in the study of neutrophil oxidative burst by the DCFH assay. Cytometry 43, 290–296. doi: 10.1002/1097-0320(20010401)43:4<290::AID-CYTO1061>3.0.CO;2-X
Wagner, C., Goldmann, T., Rohmann, K., Rupp, J., Marwitz, S., Rotta Detto Loria, J., et al. (2015). Budesonide inhibits intracellular infection with non-typeable haemophilus influenzae despite its anti-inflammatory effects in respiratory cells and human lung tissue: a role for p38 MAP kinase. Respiration 90, 416–425. doi: 10.1159/000439226
Weinheimer, V. K., Becher, A., Tonnies, M., Holland, G., Knepper, J., Bauer, T. T., et al. (2012). Influenza a viruses target type II pneumocytes in the human lung. J. Infect. Dis. 206, 1685–1694. doi: 10.1093/infdis/jis455
Wenger, J. D., Hollis, D. G., Weaver, R. E., Baker, C. N., Brown, G. R., Brenner, D. J., et al. (1989). Infection caused by francisella philomiragia (formerly yersinia philomiragia). a newly recognized human pathogen. Ann. Intern. Med. 110, 888–892. doi: 10.7326/0003-4819-110-11-888
Whipp, M. J., Davis, J. M., Lum, G., De Boer, J., Zhou, Y., Bearden, S. W., et al. (2003). Characterization of a novicida-like subspecies of francisella tularensis isolated in Australia. J. Med. Microbiol. 52, 839–842. doi: 10.1099/jmm.0.05245-0
White, J. D., Rooney, J. R., Prickett, P. A., Derrenbacher, E. B., Beard, C. W., Griffith, W. R. (1964). Pathogenesis of experimental respiratory tularemia in monkeys. J. Infect. Dis. 114, 277–283. doi: 10.1093/infdis/114.3.277
Wu, T. H., Hutt, J. A., Garrison, K. A., Berliba, L. S., Zhou, Y., Lyons, C. R. (2005). Intranasal vaccination induces protective immunity against intranasal infection with virulent francisella tularensis biovar a. Infect. Immun. 73, 2644–2654. doi: 10.1128/IAI.73.5.2644-2654.2005
Yu, J. J., Raulie, E. K., Murthy, A. K., Guentzel, M. N., Klose, K. E., Arulanandam, B. P. (2008). The presence of infectious extracellular francisella tularensis subsp. novicida in murine plasma after pulmonary challenge. Eur. J. Clin. Microbiol. Infect. Dis. 27, 323–325. doi: 10.1007/s10096-007-0434-x
Keywords: Francisella, intracellular bacteria, human lung, ex vivo, Tularemia, virulence
Citation: Köppen K, Fatykhova D, Holland G, Rauch J, Tappe D, Graff M, Rydzewski K, Hocke AC, Hippenstiel S and Heuner K (2023) Ex vivo infection model for Francisella using human lung tissue. Front. Cell. Infect. Microbiol. 13:1224356. doi: 10.3389/fcimb.2023.1224356
Received: 17 May 2023; Accepted: 23 June 2023;
Published: 10 July 2023.
Edited by:
Vera Kozjak-Pavlovic, Julius Maximilian University of Würzburg, GermanyReviewed by:
Roger Derek Pechous, University of Arkansas for Medical Sciences, United StatesKlara Kubelkova, University of Defence, Czechia
Copyright © 2023 Köppen, Fatykhova, Holland, Rauch, Tappe, Graff, Rydzewski, Hocke, Hippenstiel and Heuner. This is an open-access article distributed under the terms of the Creative Commons Attribution License (CC BY). The use, distribution or reproduction in other forums is permitted, provided the original author(s) and the copyright owner(s) are credited and that the original publication in this journal is cited, in accordance with accepted academic practice. No use, distribution or reproduction is permitted which does not comply with these terms.
*Correspondence: Klaus Heuner, aGV1bmVya0Bya2kuZGU=; Stefan Hippenstiel, c3RlZmFuLmhpcHBlbnN0aWVsQGNoYXJpdGUuZGU=
†These authors have contributed equally to this work and share first authorship