- Department of Obstetrics and Gynecology, Shengjing Hospital of China Medical University, Shenyang, China
Background: Preeclampsia (PE) is a common pregnancy-related disorder characterized by disrupted maternal-fetal immune tolerance, involving diffuse inflammatory responses and vascular endothelial damage. Alterations in the gut microbiota (GM) during pregnancy can affect intestinal barrier function and immune balance.
Aims and purpose: This comprehensive review aims to investigate the potential role of short-chain fatty acids (SCFAs), essential metabolites produced by the GM, in the development of PE. The purpose is to examine their impact on colonic peripheral regulatory T (Treg) cells, the pathogenic potential of antigen-specific helper T (Th) cells, and the inflammatory pathways associated with immune homeostasis.
Key insights: An increasing body of evidence suggests that dysbiosis in the GM can lead to alterations in SCFA levels, which may significantly contribute to the development of PE. SCFAs enhance the number and function of colonic Treg cells, mitigate the pathogenic potential of GM-specific Th cells, and inhibit inflammatory progression, thereby maintaining immune homeostasis. These insights highlight the potential significance of GM dysregulation and SCFAs produced by GM in the pathogenesis of PE. While the exact causes of PE remain elusive, and definitive clinical treatments are lacking, the GM and SCFAs present promising avenues for future clinical applications related to PE, offering a novel approach for prophylaxis and therapy.
1 Introduction
Preeclampsia (PE) is a pregnancy-specific disorder characterized by hypertension (Mol et al., 2016). It is a subtype of metabolic disease, with most cases occurring in late pregnancy, while early onset is associated with worse prognosis (Malik and Kumar, 2017). The causes of PE remain unknown, but potential pathogenic factors may involve thrombophilia, inflammation, oxidative stress, and genetic variants of the renin-angiotensin system (Goetzinger et al., 2014; Mol et al., 2016; Rana et al., 2019). Recently, some researchers have hypothesized that changes in the microbiota, microbial metabolites, and their interactions with the immune and endocrine systems may be associated with various diseases (Gilbert et al., 2018) that can lead to obesity, elevated blood pressure, and other comorbidities, which in turn increase the probability of illness (Poon et al., 2019; Ishimwe, 2021). The gut microbiota (GM) plays a role in maintaining intestinal and immune homeostasis through processes like carbohydrate digestion and the production of metabolites. However, disruptions in the stable relationship between the host and its microbiota, known as GM dysregulation, can lead to immune system disorders and the development of diseases, such as hypertension, obesity, and diabetes (Delhaes et al., 2018; Robles-Vera et al., 2020; Jin et al., 2022). However, the mechanisms by which the GM influences the progression of PE have not been fully understood to date.
In 2012, Omry et al. conducted a pioneering study investigating changes in GM and their association with metabolic disorders. They observed a significant alteration in GM composition, i.e., reduced diversity, in women during the third trimester of pregnancy compared to early pregnancy. Additionally, the progression of pregnancy was found to lead to low-level inflammation on the gut mucosal surface, indicated by elevated levels of pro-inflammatory cytokines and leukocytes, which in turn could contribute to GM disorders (Lv et al., 2019; Wang et al., 2020). Moreover, when GM from women in the third trimester was transferred to germ-free mice, metabolic changes such as significant fat accumulation and insulin resistance were induced, suggesting the relevance of GM to inflammatory metabolic diseases (Koren et al., 2012). In a study by Chen et al., third trimester PE patients, along with normotensive pregnant women, were recruited. The researchers observed that when the GM of patients was transferred to germ-free mice, the mice developed PE-like symptoms, including elevated blood pressure and increased urinary protein (Liu et al., 2017; Yong et al., 2022). These findings suggest a potential correlation between GM dysregulation and the development of PE (Huang et al., 2021). Further analysis comparing splenocytes and lymphocytes in the lamina propria of the small intestine using flow cytometry showed a significant decrease in Treg cells and a significant increase in Th17 cells in PE patients (Eghbal-Fard et al., 2019), suggesting that GM may play a role in the pathogenetic progression of PE by disrupting mucosal and systemic immune responses (Pickard et al., 2017; Chen et al., 2020).
In recent years, emerging research has highlighted the potential of short-chain fatty acids (SCFAs) (Dalile et al., 2019), a prominent metabolite of the gut microbiota (GM), in the progression of GM-associated PE (Jin et al., 2022). Smith et al. conducted a study demonstrating that SCFAs can restore the number of colonic regulatory T cells in GM-deficient mice, indicating their influence on Treg cell function and immune modulation (Smith et al., 2013). Moreover, SCFAs have been shown to regulate cytokine expression in macrophages through G protein-coupled receptors (GPCRs), promoting M2 macrophage polarization and inhibiting lipopolysaccharide (LPS)-induced M1 macrophage polarization, ultimately reducing the inflammatory response and acting as anti-inflammatory agents (Ji et al., 2016; Jiang et al., 2020). Furthermore, Jin et al. conducted experiments in PE rats and found that oral administration of propionic acid or butyric acid significantly lowered blood pressure, improved placental function, and enhanced embryonic development, suggesting a potential therapeutic role for SCFAs in PE (Jin et al., 2022). The dysregulation of SCFAs resulting from GM alterations may contribute to the pathogenesis of PE by affecting various immune cells. The roles of GM and SCFAs in the pathogenesis of PE progression are summarized in Figure 1. Exploring the specific mechanisms and roles by which SCFAs regulate PE pathogenesis in the context of GM dysregulation may provide a promising avenue for the prevention and treatment of PE.
2 Methods
The aim of this narrative review is to explore the role played by GM in PE progression and the relationship between SCFAs produced by GM metabolism and PE, respectively, in terms of anti-inflammatory effects, reduction of GDM, and PE comorbidities such as obesity and hypertension. A literature search was conducted using the PubMed/Web Of Science database to retrieve relevant English language studies published in the past 20 years. The authors independently identified the most relevant studies, including randomized controlled trials (RCTs) (Table 1), experimental and observational studies. The inclusion criteria focused on RCTs, human cohort studies, and in vivo/in vitro studies with clear experimental methods and relevant outcomes. Studies with unclear methods, experimental subjects, or negative/ambiguous results were excluded. The search utilized keywords such as gut microbiota, intestinal inflammation, PE, gestational hypertension, hypertension, gestational diabetes, obesity, diabetes, SCFAs, metabolism, pregnancy and gestational disorders.
3 Relationship between SCFAs and GM alterations
3.1 GM metabolism produces SCFAs
SCFAs are major metabolites produced through the fermentation of carbohydrates by the GM and are important for maintaining gut homeostasis (Parada Venegas et al., 2019). They have several beneficial effects in the human body (Macfarlane and Macfarlane, 2003; Yan and Ajuwon, 2015), including improving gut hormone secretion, regulating inflammatory homeostasis and the composition of GM, as well as having protective effects on gastrointestinal barrier function (Lee et al., 2017), as well as regulating blood pressure by affecting epithelial, nervous, immune system, and vascular functions. It is speculated that SCFAs may affect the progress of PE. SCFAs are saturated aliphatic organic acids consisting of one to six carbons, of which acetate, propionate, and butyrate are the most abundant (≥95%). The molar ratio of propionate, butyrate, and acetate in the colon and feces is approximately 20:20:60, and a series of postmortem examinations conducted on individuals who passed away unexpectedly (n = 6) indicated that the ratios of propionate, butyrate, and acetate were similar in the proximal and distal zones of the large intestine (Cummings et al., 1987). In the cecum and large intestine, approximately 95% of SCFAs are quickly taken up by colonic cells, with the other 5% excreted in the feces (Ruppin et al., 1980; Den Besten et al., 2013). The main butyrate-producing bacteria in the human gut belong to the phylum Firmicutes, including the genera Clostridium faecium and Eubacterium. Members of other phyla, especially the Bacteroidetes like Odoribacter and Alistipes, primarily produce acetate and propionate (Duncan et al., 2002; Gomez-Arango et al., 2016).
GM hydrolyzes carbohydrates into monosaccharides, which are subsequently fermented in the gut’s anaerobic environment. The main metabolic pathways of bacteria include the glycolytic pathways for the six carbon sugars (Embden–Meyerhof–Parnas pathway) and the five carbon sugars (pentose phosphate pathway), which transform monosaccharides into phosphoenolpyruvate (PEP). Thereafter, PEP is transformed into various products of fermentation, like organic acids or alcohols. During the glyceraldehyde-3-phosphate dehydrogenase (GAPDH) step, reduced coenzyme I (nicotinamide adenine dinucleotide, NADH) is produced. Under anaerobic conditions, excess reducing equivalents can be eliminated through the following mechanisms (Figure 2). In the first classical fermentation pathway, NADH is oxidized to reduce pyruvate to lactic acid or ethanol. Additionally, the pathway generates molecular hydrogen (H2) from the excess reducing equivalents through the pyruvate pathway or the endomembrane pathway (Fischbach and Sonnenburg, 2011). The main end products of the above fermentation pathway are SCFAs (Den Besten et al., 2013) (Figure 2). Acetate can be formed directly from acetyl-coenzyme A (acetyl-CoA) or via the Wood–Ljungdahl pathway, which involves the utilization of formate (Ragsdale and Pierce, 2008). Propionate can be formed via the succinate decarboxylation pathway or through the conversion of PEP via the acrylate pathway (Miller and Wolin, 1996). The condensation of two acetyl-CoA molecules produces butyrate via butyrate kinase (buk) or using butyryl-coenzyme, acetate-coenzyme A transferase to produce butyrate using exogenously derived acetate (Duncan et al., 2002).
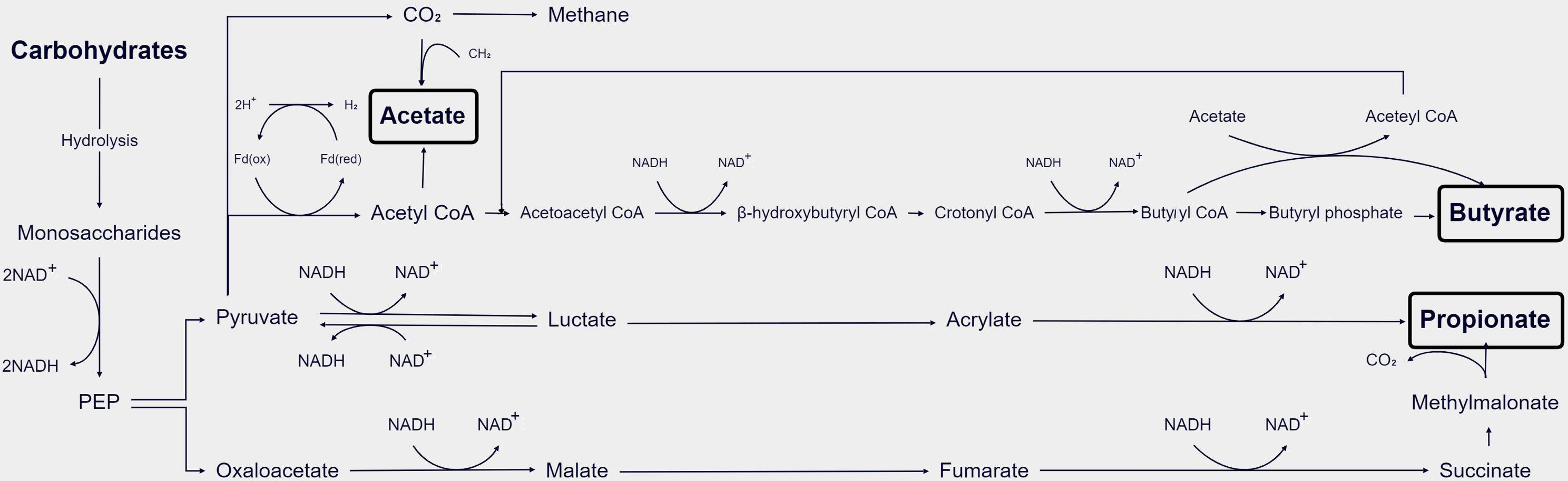
Figure 2 Schematic diagram of the process of GM metabolizing SCFAs in the body (Den Besten et al., 2013).
3.2 Effect of altered GM abundance of PE on SCFAs
Altemani et al. discovered that patients with late-onset preeclampsia (DPE), specifically those with >34 weeks of gestation, exhibited reduced serum levels of butyric acid. Furthermore, a decrease in the gene abundance of butyryl-coenzyme A, acetate-coenzyme A transferase, and buk was observed in the GM, indicating a correlation between reduced levels of butyric acid produced by the GM and PE (Altemani et al., 2021; Li et al., 2022). Additionally, alterations in the composition of GM species are associated with the pathophysiological and immune status of patients, and these changes are significantly correlated with SCFA levels (Yan et al., 2017; Ahmadian et al., 2020; Jin et al., 2022). Studies isolating bacteria from human clarified rumen fluid and fecal samples have identified major butyrate producers in the human intestine, including members of the Firmicutes phylum, such as Clostridium, Faecalibacterium from the Rumenobacteriaceae family, and Eubacterium Rectale from the Lachnospiraceae family (Louis and Flint, 2017). Other bacteria, such as Actinomycetes, Bacteroides, Clostridium, Proteus, and Thermotogae, may also have the capacity to produce butyrate depending on the genes they express (Duncan et al., 2002; Gomez-Arango et al., 2016). Within the Bacteroidetes phylum, Odoribacter and Alistipes species are known to primarily produce acetate and butyrate (Macfarlane and Macfarlane, 2003). Bifidobacterium, belonging to the Actinomycetes phylum, is mainly responsible for acetate and lactic acid production, while the mucin-degrading bacterium Akkermansia muciniphila can produce propionate and acetate (Parada Venegas et al., 2019).
The researchers utilized fecal specimens from severe PE patients and pregnant healthy controls to assess the abundance of GM and the content of fecal SCFAs through 16SrRNA sequencing and gas chromatography. Heat maps were generated to visualize the correlations between GM abundance differences and SCFAs levels based on the data results. Analysis revealed that the most abundant SCFAs in the feces were acetic, propionic, and butyric acid, while valeric, isovaleric, and isobutyric acids constituted a smaller fraction of the total SCFAs. Remarkably, reduced concentrations of butyric and valeric acids were observed in individuals with PE (Chang et al., 2020).
On the genus level, several bacteria showed positive correlations with fecal SCFAs levels, particularly pentanoic and butyric acids. These bacteria included Alistipes, Blautia, Collinsella, Eubacterium Rectale, and Streptococcus, belonging to the phyla Actinomycetes, Bacteroidetes, or Firmicutes (Yu et al., 2018; Chang et al., 2020). Notably, a negative association was found between systolic blood pressure and the abundance of Eubacterium Rectale and Blautia in the gut, indicating a decrease in the relative abundance of these bacteria in hypertensive patients (Saji et al., 2019; Toral et al., 2019a). Conversely, bacteria such as Escherichia, Shigella (both belonging to Proteobacteria), and Enterobacter (also belonging to Proteobacteria) exhibited negative associations with fecal concentrations of acetic, propionic, butyric, and valeric acids. It has been reported that Escherichia and Enterobacter, enriched in the gut of PE patients, can lead to excessive inflammatory responses (Reuschel et al., 2020). These findings suggest that changes in fecal SCFA levels align with variations in GM abundance in the PE group (Chang et al., 2020).
Additionally, Altemani et al. identified bacterial species associated with butyrate production that were present in low numbers at 28 weeks of pregnancy, before the onset of PE symptoms. This reduction in specific butyrate-producing bacterial species was observed to persist in individuals who later developed PE. The study also found significantly lower levels of butyrate in the blood of individuals with late-onset preeclampsia compared to the control group. These findings suggest that a decrease in the relative abundance of specific bacterial species involved in butyrate production may contribute to the risk of developing PE in the third trimester of pregnancy, particularly in obese pregnant women (Altemani et al., 2021).
4 Correlation between GM changes and PE
4.1 Inflammatory response due to maternal GM alterations and effects on fetal health
Through the collection of stool samples from PE patients and healthy individuals (2020), researchers conducted 16S rRNA sequencing and discovered notable differences in the abundance of Bacteroidetes, Proteobacteria, and Fusobacterium in PE patients, indicating an altered microbial diversity between the two groups (Wang et al., 2019; Meijer et al., 2023). Additionally, PE patients exhibited a significant increase in inflammatory factors, including IL-6 and TNF-α, in their plasma (Black and Horowitz, 2018; Zhao et al., 2022). These findings suggest that patients with PE experience a characteristic activation of the inflammatory response (Lavelle and Sokol, 2020). Fecal microbiota transplantation experiments (Figure 3) demonstrated that this inflammatory response is linked to GM alterations (Kim et al., 2017; Michaudel and Sokol, 2020). The utilization of fecal microbiota transplantation (FMT) from PE patients in an antibiotic-treated mouse model triggered a PE-like phenotype in mice (PE-FMT mice). Translocation of microbiota and immune alterations in human and mouse placental specimens can be identified by 16SrRNA sequencing and quantitative PCR (Chen et al., 2020). PE-FMT mice exhibited decreased Treg cell number and function and increased Th17 cells (Darmochwal-Kolarz et al., 2012; Eviston et al., 2012). In a rat model of PE with reduced uterine perfusion pressure (RUPP), chronic inflammation was found to be associated with an imbalance between T cell subtypes (Cornelius et al., 2016). Much of the work on GM and immunity in PE patients is focused on imbalances in T cell homeostasis when GM, through interaction with T cells, results in impaired gut barrier function and microbial molecular translocation. However, T cells may also be involved in the peri-implantation period, impacting both the embryo and mother (Hu et al., 2019).
Furthermore, the inflammatory response mediated by the GM is not limited to specific bacterial species but also involves other bacterial metabolites, such as endotoxin and LPS, which possess high inflammatory potential (Robles-Vera et al., 2018). These molecules can activate the innate immune response, thereby exacerbating the overall inflammatory effects (Kell and Kenny, 2016; Armani et al., 2017). The sustained presence of such inflammatory stimuli can contribute to chronic inflammation (Kim et al., 2017), leading to an increase in inflammatory cytokines, oxidative stress in the placenta, impaired vascular function, and ultimately the progression of fetal immune rejection and hypertension (Chen et al., 2020).
Moreover, emerging evidence suggests that alterations in maternal GM can influence the composition of the fetal microbiota, and the maternal gut environment may have long-term effects on the health of offspring through mechanisms such as developmental programming (Alexander, 2006; Torres et al., 2020). Studies in animal models have demonstrated that prenatal modulation of maternal microbiota through the administration of probiotics or prebiotics can confer protection against cardiovascular diseases, including hypertension, in the offspring (Hsu et al., 2018). Additionally, maternal GM has been shown to shape the immune system of offspring, as pups born to pregnant mice with transiently modified GM during pregnancy exhibit enhanced ability to modulate inflammatory responses to GM (Gomez De Agüero et al., 2016; Koch et al., 2016). Disruption of maternal GM can also impact fetal immune mechanisms, potentially leading to immune disorders such as asthma (Zhao et al., 2015; Nyangahu et al., 2018). Collectively, these findings suggest that GM may play a role in the progression of PE and have implications for fetal health by influencing immune homeostasis and triggering inflammatory responses.
4.2 Association of GM alterations with common PE comorbidities hypertension, obesity, and diabetes
PE is characterized by hypertension and studies have indicated a potential association between blood pressure and the GM (Yang et al., 2018; Tang et al., 2019). Transplantation of fecal material from hypertensive human donors into germ-free mice has shown that bacterial transfer can induce an increase in blood pressure in the recipient mice (Muralitharan et al., 2020), suggesting a direct impact of GM on host blood pressure regulation (Yang et al., 2015; Li et al., 2017). Several mechanisms have been proposed to explain how GM may influence blood pressure in the host. Certain bacteria, such as Bifidobacterium, Escherichia, Lactobacillus and Streptococcus have, been implicated in neurotransmitter synthesis within the autonomic nervous system, and alterations in their abundance can consequently affect vascular tone and contribute to the development of hypertension (El Aidy et al., 2014). GM can also influence blood pressure by modulating inflammatory reactions in the body, affecting vascular endothelial function, and subsequently influencing blood pressure regulation (Gomez-Arango et al., 2016). Furthermore, GM can produce bioactive metabolites, including trimethylamine (TMA), trimethylamine N-oxide (TMAO) and SCFAs (Tang et al., 2019). TMAO, GM-derived metabolite associated with increased levels of choline and carnitine in the gut, has been found to be significantly elevated in the peripheral circulation of PE patients (Cornelius et al., 2016). Observational studies have suggested that TMAO may contribute to the development of cardiovascular disease (Wang et al., 2019). Additionally, Koeth et al. demonstrated that TMAO plays a significant role in atherosclerosis progression by regulating sterol and cholesterol metabolism within the host (Koeth et al., 2013), and atherosclerotic-like lesions have been observed in the placenta of PE patients, characterized by lipid deposition in the walls of spiral arteries (Hu et al., 2019). Therefore, it is hypothesized that TMAO might accelerate the formation of atherosclerotic lesions, thus contributing to the development of PE (Wang et al., 2019). These results support a potential correlation between GM dysregulation and elevated blood pressure.
Numerous studies conducted on both human cohorts and animal models have demonstrated alterations in GM abundance and diversity in the context of obesity (Gérard, 2016). Ley et al. reported a significant increase in Firmicutes and a decrease in Bacteroides in obese mice compared to non-obese mice (Ley et al., 2005). Collado et al. conducted a comparison of GM composition between obese and healthy weight pregnant women using qPCR and other techniques, revealing a significant increase in Bacteroides and Staphylococcus in overweight and obese females (Collado et al., 2008). Furthermore, an elevated number of Staphylococcus was positively correlated with increased plasma cholesterol levels, which could be attributed to the production of distinct SCFAs and the modulation of host gene expression influencing lipid metabolism (Pouteau et al., 2003; Turnbaugh et al., 2006). In an experiment conducted on SD rats, prebiotic treatment selectively modified the GM composition of obese dams and enhanced the secretion of intestinal satiety hormones such as glucagon-like peptide-1 (GLP-1) (Bell et al., 2005). This resulted in a reduction in maternal energy intake, a decrease in body weight gained during pregnancy, and a decrease in the probability of excessive weight gain in the mother and her offspring (Paul et al., 2016). Additionally, GM alterations have the potential to predict changes in metabolic pathways such as glycolysis and lipid metabolism (Gohir et al., 2015). Through 16S rRNA sequencing of fecal samples from individuals with gestational diabetes and normal pregnancies, it was discovered that Akkermansia was associated with lower systemic insulin sensitivity (Hansen et al., 2012). In rodent studies, supplementation with the probiotic Akkermansia improved glucose tolerance and insulin sensitivity (Zhao et al., 2017; Crusell et al., 2018). These findings indicate the potential metabolic benefits of probiotics, including improvements in obesity, hypertension, and diabetes, which are commonly observed comorbidities of PE. Therefore, probiotic interventions may also hold promise for mitigating the progression of PE.
5 Mechanism of action of SCFAs in PE
5.1 Anti-inflammatory effect of SCFAs in the progression of PE
SCFAs play a multifaceted role in immune regulation. They not only affect the production of interleukins and leukocyte migration but also induce apoptosis in macrophages, lymphocytes (Bailón et al., 2010), and neutrophils (Vinolo et al., 2011). Additionally, SCFAs can inhibit the expression of adhesion molecules induced by stimuli and the production of chemokines, as well as their interactions with endothelial cells. This includes selectins, integrins (such as macrophage antigen-1 [Mac-1] and lymphocyte function-associated antigen-1 [LFA-1]), anti-ligand molecules like intercellular adhesion molecule-1 (ICAM-1), specific carbohydrates, and vascular cell adhesion molecule-1 (VCAM-1) (Vinolo et al., 2009) (Figure 4). These cytoadhesion factors and chemokines play crucial roles in leukocyte rolling along vascular endothelium, stable adhesion, and trans-endothelial migration (Meijer et al., 2010). SCFAs can suppress the recruitment of neutrophils, monocytes, and macrophages through the aforementioned cytokines, exerting anti-inflammatory effects. Furthermore, SCFAs also have the ability to regulate gut cell renewal, maintain barrier function, and modulate responses to inflammatory or infectious stimuli, thus playing an essential role in intestinal epithelial physiology (Corrêa-Oliveira et al., 2016).
Among the metabolites produced by the fermentation of dietary fiber by gut microbiota, SCFAs also include the pro-inflammatory metabolite lactic acid. The inflammatory effects of lactic acid are associated with bile acids, and disturbances in bile acid metabolism can lead to abnormal inflammatory responses. Certain bile acids, due to their hydrophobic properties, can disrupt cell membranes (Hang et al., 2019). Consequently, the resulting increase in lactate levels has been shown to be associated with hypertension and inflammatory response (Durgan et al., 2016; Ishimwe et al., 2021).
Furthermore, Yu et al. constructed a mouse model utilizing the T-cell over-transfer colitis method, whereby Th1 cells or naive CD4+ T cells were injected intravenously into mice and subjected to butyrate treatment and flow cytometry analysis. SCFAs were shown to promote IL-10 generation by GM antigen-specific Th1 cells, a process mediated through GPR3 (Sun et al., 2018). Mechanistically, this relies on the activation of STAT3 and mTOR, which promote the expression of the transcription factor Blimp-1 in Th1 cells. Consequently, SCFAs attenuate GM-specific Th1 cell-induced inflammation in the intestine (Heinemann et al., 2014; Sun et al., 2018) (Figure 4). In addition, the offspring of PE patients have been found to exhibit persistent alterations in fetal thymus size and structure and reduced output of thymic regulatory T cells, which can be observed until the age of 4 years (Sun et al., 2018). In studies using germ-free mice, researchers observed that the growth and development of fetal thymus Treg cells and CD4+ T cells were similarly impaired, and this impairment could be reversed by maternal supplementation with acetate. The administration of acetate, one of the SCFAs, induced an increased expression of autoimmune regulator (AIRE), a transcription factor involved in facilitating Treg cell production (Heinemann et al., 2014). These findings suggest that maternal acetate supplementation may have the potential to pass through the placenta and influence fetal T cell immunity, thereby impacting the altered development of T cells and immune function observed in the offspring of PE patients (Hu et al., 2019).
Moreover, Yong et al. induced a PE-like phenotype in pregnant rats using Nω-nitro- L-arginine methyl ester hydrochloride, and found that sodium butyrate significantly decreased blood pressure, 24-hour proteinuria, and the expression levels of inflammatory factors including TGF-β, IL- 1, and IL- 6, as well as increasing placental weight and expression of gut barrier markers such as claudin-5, ocludin, and ZO-1 (Chang et al., 2020; Yong et al., 2022). Meanwhile, a decrease in Treg/Th17 cell ratios and an increase in pregnancy-associated dimethylamine oxidase levels in spleen and small intestine immune cells were also observed in sodium butyrate-treated pregnant rats. In addition, sodium butyrate increased the expression of antimicrobial peptides such as LL-37 and CAP-18, in rabbit and human colonic epithelial cells (Raqib et al., 2012). These antimicrobial peptides act as reservoirs for antimicrobial agents against a wide range of pathogens at the host-microbial interface (Zasloff, 2002; Heinemann et al., 2014). Notably, the administration of sodium butyrate to PE rats also improved the abundance of GM, particularly Firmicutes and Bacteroides, and significantly increased the levels of butyrate and valeric acid (Yong et al., 2022). This provides further evidence that SCFAs can improve inflammatory function and may help alleviate PE in pregnant rats.
5.2 Regulation of blood pressure by SCFAs
In a rat model utilizing spontaneously hypertensive rats (SHR), the GM is less diverse and richer, with a higher ratio of Firmicutes in contrast with Bacteroidetes (Ley et al., 2006; Gomez-Arango et al., 2016). Supplementation with acetate and propionate was observed to dramatically reduce blood pressure levels (Pluznick et al., 2013; Marques et al., 2017; Kim et al., 2018). In hypertensive patients, increased levels of LPS, intestinal fatty acid binding protein (I-FABP), and Th17 cells were observed, indicating heightened intestinal inflammation and permeability (Qi et al., 2017; Kim et al., 2018). Robles et al. found that, in SHR rats, alterations in gut microbiota contribute to elevated blood pressure by activating and accumulating T cells (Itani et al., 2016; Toral et al., 2019b). Furthermore, propionate has been found to significantly mitigate cardiac hypertrophy, fibrosis, and vascular dysfunction in hypertensive mice. It accomplishes this by reducing systemic inflammation, decreasing the frequency of splenic T cells, and suppressing Th17 cell activity (Bartolomaeus et al., 2019).
In SHR rats, compared to normal Wistar Kyoto rats (WKY rats), a decrease of approximately 30% in the T cell ratio was observed in the spleen, while an increase in the number of Treg cells in the intestinal lymph nodes and spleen was observed after treatment with probiotics or oral butyrate, which increased fecal butyrate content. These treatments also reduced Th17 cells to levels similar to those in the secondary lymphoid organs of WKY rats. These findings suggest that T cells play a crucial role in the hypertensive response triggered by fecal microbiota transplantation in SHR rats, and that SCFAs can reverse this effect (Robles-Vera et al., 2020).
In addition, bacteria that produce SCFAs may influence host blood pressure, either via direct vasodilatory effects or via plasminogen activator inhibitor-1 (PAI-1) (Pluznick, 2014; Li et al., 2022). Activation of GPR41 has been associated with the regulation of blood pressure. Butyric acid, an SCFA, can directly activate colonic vagal signal transduction through GPR41/43 receptors, leading to vasodilation and ultimately lowering blood pressure. GPR41 is expressed in various tissues, including the colon and blood vessels, and its activation by butyric acid promotes vasodilation and relaxation of smooth muscle cells, contributing to the reduction of systemic blood pressure (Onyszkiewicz et al., 2019). In addition, n-butyric acid increases the mRNA expression of PAI-1 in liver cells, and the enema of SCFAs stimulates increased rectal microcirculation (Gomez-Arango et al., 2016). In a meta-analysis, PAI-1 gene diversity was linked to an enhanced risk of PE (Morgan et al., 2013); the analysis found that pregnant women with hypertension, such as PE, showed increased placental PAI-1 expression and elevated levels of PAI-1 in their circulating blood. This is because hypertension can lead to vascular endothelial damage, triggering an inflammatory response, which in turn increases C-reactive protein and thus induces increased PAI-1 expression. Therefore, the inhibition of PAI-1 expression may inhibit the progression of hypertension. PAI-1 inhibition may also attenuate nitric oxide synthase-induced hypertension (Gomez-Arango et al., 2016). Therefore, we hypothesize that by manipulating the GM metabolites, SCFAs could reduce PAI-1 levels and thus alleviate hypertension in PE (Figure 4).
5.3 SCFAs may improve obesity and PE comorbidities such as diabetes
PE, as a metabolic disease, is influenced by factors such as obesity, diabetes, and other metabolic disorders, which are known to increase its prevalence (Pinheiro et al., 2019; Poon et al., 2019). SCFAs have been implicated in glucose and lipid metabolism as well as obesity (He et al., 2020; Litwin and Kułaga, 2021; Wu et al., 2021). They serve as an energy source and can activate intestinal gluconeogenesis, which is crucial for maintaining normal blood glucose levels and metabolic homeostasis (De Vadder et al., 2014; Machate et al., 2020). SCFAs also impact intestinal transit, function, and food intake by stimulating the release of peptide YY (PYY), an enteroendocrine hormone that reduces intestinal motility (Forbes et al., 2015), enhances glucose absorption in muscle and adipose tissue, and decreases food intake (Batterham et al., 2002; Van Den Hoek et al., 2004). Moreover, butyrate and propionate have been shown to inhibit the secretion of pro-inflammatory cytokines and chemokines in the placenta and visceral adipose tissue, improve insulin sensitivity, and regulate glucose uptake (Heimann et al., 2015; Roy et al., 2020). SCFAs also influence leptin secretion from adipocytes, thereby modulating food intake, body weight, and energy metabolism through the central nervous system (Sakakibara et al., 2006; Fujikawa et al., 2010; Wang et al., 2020; Alsharairi, 2021). Studies using non-pregnant animal models of obesity and diabetes have demonstrated that the inclusion of butyrate and propionate in the diet leads to reduced body weight, obesity, fasting glucose and insulin levels, improved insulin tolerance, and amelioration of diabetes symptoms (Gao et al., 2009; Henagan et al., 2015) (Figure 4).
In previous studies, GDM and PE were both common complications of pregnancy and had similar pathological changes, such as the elevated levels of pro-inflammatory cytokine TNF-αand IL-6, endothelial dysfunction, etc (Mcelwain et al., 2020; Phoswa and Khaliq, 2021), therefore there may be a correlation (Yang and Wu, 2022). For example, in a large international prospective Cohort study, it was found that after adjusting for age, BMI, urinary tract infection, OGTT, smoking and drinking status, family history and other factors, the occurrence of PE was still positively correlated with blood glucose level (Metzger et al., 2008). In term infants born to GDM women, GM, such as Bifidobacterium, is related to the decrease of SCFAs content in feces (Soderborg et al., 2020). Bifidobacterium can utilize human milk oligosaccharides (HMOs) through related degradation enzymes, thereby increasing the production of butyrate, propionate and other SCFAs (Gopalsamy et al., 2019; Alsharairi, 2023). The results of a control trial showed that butyrate could reduce intestinal inflammation in mice, and the expression of M2 macrophage related protein Arg1 was significantly increased. Then the treatment of M2 macrophage with butyrate increased the expression of H3K9/STAT6 signaling pathway (Hunter et al., 2010; Ji et al., 2016), which was related to reducing intestinal mucosal damage and the level of proinflammatory cytokines (Mosser and Edwards, 2008). In addition, the classic pathway of IL-4 inducing polarization of M2 Macrophage polarization involves STAT6 signaling pathway, which leads to the expression of Fizz1, Ym-1, Arg1 and other cytokines in macrophages (Mikita et al., 1996; Dong et al., 2011). SCFAs can also regulate the function of the Treg cells pool in the colon and prevent intestinal inflammation in mice in a GPR43 dependent manner (Furusawa et al., 2013; Smith et al., 2013). These results provide a molecular mechanism for SCFAs mediated protection against PE and comorbidities such as GDM, possibly by reducing intestinal inflammatory response.
6 Conclusions and recommendations
SCFAs, such as acetic acid, propionic acid, and butyric acid, are SCFAs, such as acetic acid, propionic acid, and butyric acid, are important metabolites of gut microbiota that play a significant role in immune homeostasis, particularly in the gut (Mell et al., 2015). They have potent anti-inflammatory actions and can enhance the function of Treg cells in the colon (Robles-Vera et al., 2020). Abnormal gut microbiota metabolism of SCFAs is associated with the pathogenesis of PE. Treating PE rats with sodium butyrate improved their gut microbiota composition, increased levels of butyric and valeric acid, and alleviated PE symptoms (Yong et al., 2022). Probiotic supplementation in the third trimester of pregnancy may reduce the risk of PE. Microecological regulators, including probiotics, prebiotics, and synbiotics, have shown promise in improving gut health and reducing PE incidence (Boe et al., 2013; Gomez-Arango et al., 2016). Prebiotics stimulate beneficial bacteria, such as Bifidobacterium and Colonococcus, which can help reduce blood pressure and glucose levels (Zhou and Xiao, 2018; Wu et al., 2021). Future, research should investigate the therapeutic role of special diets with probiotic supplements in preventing PE development (Huang et al., 2021). The correlation between GM dysregulation and PE highlights the potential for targeted treatments. Early detection and diagnosis of PE remain challenging, and further exploration of SCFAs and GM markers is warranted for more effective diagnosis and treatment.
While numerous microbiota environments, such as oral, gut, placental, and vaginal, are thought to contribute to PE, the role of GM in PE remains poorly understood. Moreover, evidence of a specific mechanism of GM in the gut is lacking. In addition to GM, other factors, such as dietary factors and daily sodium intake, can also influence the progression of PE. Therefore, a larger sample size and more detailed experiments should be conducted to eliminate other confounding factors and investigate the possible correlation between GM and PE progression.
A complete fecal sample includes bacteria, fungi, viruses, bacteriophages, and other metabolites from hosts and bacteria. To understand the role of different components in PE, it is necessary to employ multi-omics technologies, analyze deactivated fecal samples, and investigate specific bacterial metabolites. This approach can help identify critical components involved in PE development. Additionally, screening potential positive indicators and conducting pathway enrichment analysis can shed light on the regulatory metabolites in PE. Further research is needed to obtain detailed data on the microbiota composition of pregnant women following probiotic interventions. Determining the optimal dose and timing of probiotic interventions, such as before pregnancy, in the second trimester, or in the third trimester, requires more investigation. Addressing these questions will contribute to a better understanding of the effects of probiotics on GM and PE.
Further research is needed to identify the specific mechanisms by which SCFAs derived from GM act on different inflammatory cells in the context of PE. Longitudinal, multi-regional cohort studies are warranted to gain a comprehensive understanding of the microbial ecosystem in the treatment of PE and investigate the metabolic pathways involved. Functional experiments involving SCFAs and various cellular and animal models are necessary to observe phenotypic changes and elucidate the diverse effects and mechanisms of SCFAs at different stages of PE development. This research will help identify the specific SCFAs, optimal dosages, and intervention strategies that can effectively improve PE symptoms and provide valuable insights for clinical treatment.
The investigation of GM alteration and the role of SCFAs in the pathogenesis of PE holds promise for the prevention and treatment of this condition. However, further research is necessary to fully understand these relationships and validate the potential of probiotics or SCFAs as diagnostic and therapeutic approaches for PE. Large-scale studies involving diverse patient cohorts are needed to confirm the efficacy and safety of these interventions. Such endeavors will contribute to the development of innovative strategies for the prevention and treatment of PE.
Author contributions
All authors contributed to data analysis, drafting, or revising the article, have agreed on the journal to which the article will be submitted, gave final approval of the version to be published, and agree to be accountable for all aspects of the work.
Funding
This work was supported by the Natural Science Foundation of Liaoning Province (No. 2020-MS-03), the Applied basic research program of Liaoning Provincial Department of science and technology (No. 2022020796-JH2/1015), the Wu Jieping Medical Foundation (No. 320.6750.2022-6-12) and the 345 Talent Project of Shengjing hospital.
Conflict of interest
The authors declare that the research was conducted in the absence of any commercial or financial relationships that could be construed as a potential conflict of interest.
Publisher’s note
All claims expressed in this article are solely those of the authors and do not necessarily represent those of their affiliated organizations, or those of the publisher, the editors and the reviewers. Any product that may be evaluated in this article, or claim that may be made by its manufacturer, is not guaranteed or endorsed by the publisher.
Abbreviations
PE, Preeclampsia; GM, Gut microbiota; FMT, Fecal microbiota transplantation; DPE, Late-onset (>34 weeks gestation) PAI-1, Plasminogen activator inhibitor-1; GPCRs, G protein-coupled receptors; LPS, Lipopolysaccharide; GDM, Gestational diabetes mellitus; T1, First trimester; T2, Second trimester; T3, Third trimester; PIGF, Placental growth factor in early pregnancy; WKY, Wistar Kyoto rats; SHR rats, Spontaneous hypertension rats; SPF mice, Pathogen free mice; GF mice, Sterile mice; SD rats, Sprague–Dawley rats; I-FABP, Intestinal fatty acid binding protein; SCFAs, Short-chain fatty acids ; Treg cells, Regulatory T cells; Th cells, Helper T (Th) cells.
References
(2020). Gestational hypertension and preeclampsia: ACOG practice bulletin summary, number 222. Obstet Gynecol 135 (6), 1492–1495. doi: 10.1097/AOG.0000000000003892
Ahmadian, E., Rahbar Saadat, Y., Hosseiniyan Khatibi, S. M., Nariman-Saleh-Fam, Z., Bastami, M., Zununi Vahed, F., et al. (2020). Pre-eclampsia: microbiota possibly playing a role. Pharmacol. Res. 155, 104692. doi: 10.1016/j.phrs.2020.104692
Alexander, B. T. (2006). Fetal programming of hypertension. Am. J. Physiol. Regul. Integr. Comp. Physiol. 290 (1), R1–r10. doi: 10.1152/ajpregu.00417.2005
Alsharairi, N. A. (2021). The role of short-chain fatty acids in mediating very low-calorie ketogenic diet-infant gut microbiota relationships and its therapeutic potential in obesity. Nutrients 13 (11), 3702. doi: 10.3390/nu13113702
Alsharairi, N. A. (2023). Therapeutic potential of gut microbiota and its metabolite short-chain fatty acids in neonatal necrotizing enterocolitis. Life (Basel) 13 (2), 561. doi: 10.3390/life13020561
Altemani, F., Barrett, H. L., Gomez-Arango, L., Josh, P., David McIntyre, H., Callaway, L. K., et al. (2021). Pregnant women who develop preeclampsia have lower abundance of the butyrate-producer coprococcus in their gut microbiota. Pregnancy Hypertens. 23, 211–219. doi: 10.1016/j.preghy.2021.01.002
Armani, R. G., Ramezani, A., Yasir, A., Sharama, S., Canziani, M. E.F., Raj, D. S., et al. (2017). Gut microbiome in chronic kidney disease. Curr. Hypertens. Rep. 19 (4), 29. doi: 10.1007/s11906-017-0727-0
Bailón, E., Cueto-Sola, M., Utrilla, P., Rodríguez-Cabezas, M. E., Garrido-Mesa, N., Zarzuelo, A., et al. (2010). Butyrate in vitro immune-modulatory effects might be mediated through a proliferation-related induction of apoptosis. Immunobiology 215 (11), 863–873. doi: 10.1016/j.imbio.2010.01.001
Bartolomaeus, H., Balogh, A., Yakoub, M., Homann, S., Markó, L., Höges, S., et al. (2019). Short-chain fatty acid propionate protects from hypertensive cardiovascular damage. Circulation 139 (11), 1407–1421. doi: 10.1161/CIRCULATIONAHA.118.036652
Batterham, R. L., Cowley, M. A., Small, C. J., Herzog, H., Cohen, M. A., Dakin, C. L., et al. (2002). Gut hormone PYY(3-36) physiologically inhibits food intake. Nature 418 (6898), 650–654. doi: 10.1038/nature00887
Bell, C. G., Walley, A. J., Froguel, P. (2005). The genetics of human obesity. Nat. Rev. Genet. 6 (3), 221–234. doi: 10.1038/nrg1556
Black, K. D., Horowitz, J. A. (2018). Inflammatory markers and preeclampsia: a systematic review. Nurs. Res. 67 (3), 242–251. doi: 10.1097/NNR.0000000000000285
Boe, A. E., Eren, M., Murphy, S. B., Kamide, C. E., Ichimura, A., Terry, D., et al. (2013). Plasminogen activator inhibitor-1 antagonist TM5441 attenuates nω-nitro-L-arginine methyl ester-induced hypertension and vascular senescence. Circulation 128 (21), 2318–2324. doi: 10.1161/CIRCULATIONAHA.113.003192
Chang, Y., Chen, Y., Zhou, Q., Wang, C., Chen, L., Di, W., et al. (2020). Short-chain fatty acids accompanying changes in the gut microbiome contribute to the development of hypertension in patients with preeclampsia. Clin. Sci. (Lond) 134 (2), 289–302. doi: 10.1042/CS20191253
Chen, X., Li, P., Liu, M., Zheng, H., He, Y., Chen, M. X., et al. (2020). Gut dysbiosis induces the development of pre-eclampsia through bacterial translocation. Gut 69 (3), 513–522. doi: 10.1136/gutjnl-2019-319101
Collado, M. C., Isolauri, E., Laitinen, K., Salminen, S. (2008). Distinct composition of gut microbiota during pregnancy in overweight and normal-weight women. Am. J. Clin. Nutr. 88 (4), 894–899. doi: 10.1093/ajcn/88.4.894
Cornelius, D. C., Amaral, L. M., Wallace, K., Campbell, N., Thomas, A. J., Scott, J., et al. (2016). Reduced uterine perfusion pressure T-helper 17 cells cause pathophysiology associated with preeclampsia during pregnancy. Am. J. Physiol. Regul. Integr. Comp. Physiol. 311 (6), R1192–r1199. doi: 10.1152/ajpregu.00117.2016
Corrêa-Oliveira, R., Fachi, J. L., Vieira, A., Sato, F. T., Vinolo, M. A. (2016). Regulation of immune cell function by short-chain fatty acids. Clin. Transl. Immunol. 5 (4), e73. doi: 10.1038/cti.2016.17
Crusell, M. K. W., Hansen, T. H., Nielsen, T., Allin, K. H., Rühlemann, M. C., Damm, P., et al. (2018). Gestational diabetes is associated with change in the gut microbiota composition in third trimester of pregnancy and postpartum. Microbiome 6 (1), 89. doi: 10.1186/s40168-018-0472-x
Cummings, J. H., Pomare, E. W., Branch, W. J., Naylor, C. P., Macfarlane, G. T. (1987). Short chain fatty acids in human large intestine, portal, hepatic and venous blood. Gut 28 (10), 1221–1227. doi: 10.1136/gut.28.10.1221
Dalile, B., Van Oudenhove, L., Vervliet, B., Verbeke, K. (2019). The role of short-chain fatty acids in microbiota-gut-brain communication. Nat. Rev. Gastroenterol. Hepatol. 16 (8), 461–478. doi: 10.1038/s41575-019-0157-3
Darmochwal-Kolarz, D., Kludka-Sternik, M., Tabarkiewicz, J., Kolarz, B., Rolinski, J., Leszczynska-Gorzelak, B., et al. (2012). The predominance of Th17 lymphocytes and decreased number and function of treg cells in preeclampsia. J. Reprod. Immunol. 93 (2), 75–81. doi: 10.1016/j.jri.2012.01.006
Delhaes, F., Giza, S. A., Koreman, T., Eastabrook, G., McKenzie, C. A., Bedell, S., et al. (2018). Altered maternal and placental lipid metabolism and fetal fat development in obesity: current knowledge and advances in non-invasive assessment. Placenta 69, 118–124. doi: 10.1016/j.placenta.2018.05.011
Den Besten, G., Van Eunen, K., Groen, A. K., Venema, K., Reijngoud, D. J., Bakker, B. M., et al. (2013). The role of short-chain fatty acids in the interplay between diet, gut microbiota, and host energy metabolism. J. Lipid Res. 54 (9), 2325–2340. doi: 10.1194/jlr.R036012
De Vadder, F., Kovatcheva-Datchary, P., Goncalves, D., Vinera, J., Zitoun, C., Duchampt, A., et al. (2014). Microbiota-generated metabolites promote metabolic benefits via gut-brain neural circuits. Cell 156 (1-2), 84–96. doi: 10.1016/j.cell.2013.12.016
Dong, L., Zhang, X., Fu, X., Zhang, X., Gao, X., Zhu, M., et al. (2011). PTB-associated splicing factor (PSF) functions as a repressor of STAT6-mediated ig epsilon gene transcription by recruitment of HDAC1. J. Biol. Chem. 286 (5), 3451–3459. doi: 10.1074/jbc.M110.168377
Duncan, S. H., Barcenilla, A., Stewart, C. S., Pryde, S. E., Flint, H. J. (2002). Acetate utilization and butyryl coenzyme a (CoA):acetate-CoA transferase in butyrate-producing bacteria from the human large intestine. Appl. Environ. Microbiol. 68 (10), 5186–5190. doi: 10.1128/AEM.68.10.5186-5190.2002
Durgan, D. J., Ganesh, B. P., Cope, J. L., Ajami, N. J., Phillips, S. C., Petrosino, J. F., et al (2016). Role of the gut microbiome in obstructive sleep apnea-induced hypertension. Hypertension 67 (2), 469–474. doi: 10.1161/HYPERTENSIONAHA.115.06672
Eghbal-Fard, S., Yousefi, M., Heydarlou, H., Ahmadi, M., Taghavi, S., Movasaghpour, A., et al. (2019). The imbalance of Th17/Treg axis involved in the pathogenesis of preeclampsia. J. Cell Physiol. 234 (4), 5106–5116. doi: 10.1002/jcp.27315
El Aidy, S., Dinan, T. G., Cryan, J. F. (2014). Immune modulation of the brain-gut-microbe axis. Front. Microbiol. 5, 146. doi: 10.3389/fmicb.2014.00146
Eviston, D. P., Quinton, A. E., Benzie, R. J., Peek Michael, J., Martin Andrew., , Nanan Ralph, K., et al. (2012). Impaired fetal thymic growth precedes clinical preeclampsia: a case–control study. J. Reprod. Immunol. 94 (2), 183–189. doi: 10.1016/j.jri.2012.04.001
Fischbach, M. A., Sonnenburg, J. L. (2011). Eating for two: how metabolism establishes interspecies interactions in the gut. Cell Host Microbe 10 (4), 336–347. doi: 10.1016/j.chom.2011.10.002
Forbes, S., Stafford, S., Coope, G., Heffron, H., Real, K., Newman, R., et al. (2015). Selective FFA2 agonism appears to act via intestinal PYY to reduce transit and food intake but does not improve glucose tolerance in mouse models. Diabetes 64 (11), 3763–3771. doi: 10.2337/db15-0481
Fujikawa, T., Chuang, J. C., Sakata, I., Ramadori, G., Coppari, R. (2010). Leptin therapy improves insulin-deficient type 1 diabetes by CNS-dependent mechanisms in mice. Proc. Natl. Acad. Sci. U.S.A. 107 (40), 17391–17396. doi: 10.1073/pnas.1008025107
Furusawa, Y., Obata, Y., Fukuda, S., Endo, T. A., Nakato, G., Takahashi, D., et al. (2013). Commensal microbe-derived butyrate induces the differentiation of colonic regulatory T cells. Nature 504 (7480), 446–450. doi: 10.1038/nature12721
Gao, Z., Yin, J., Zhang, J., Ward, R. E., Martin, R. J., Lefevre, M., et al. (2009). Butyrate improves insulin sensitivity and increases energy expenditure in mice. Diabetes 58 (7), 1509–1517. doi: 10.2337/db08-1637
Gérard, P. (2016). Gut microbiota and obesity. Cell Mol. Life Sci. 73 (1), 147–162. doi: 10.1007/s00018-015-2061-5
Gilbert, J. A., Blaser, M. J., Caporaso, J. G., Jansson, J. K., Lynch, S. V., Knight, R., et al. (2018). Current understanding of the human microbiome. Nat. Med. 24 (4), 392–400. doi: 10.1038/nm.4517
Goetzinger, K. R., Tuuli, M. G., Cahill, A. G., Macones, G. A., Odibo, A. O. (2014). Development and validation of a risk factor scoring system for first-trimester prediction of preeclampsia. Am. J. Perinatol 31 (12), 1049–1056. doi: 10.1055/s-0034-1371705
Gohir, W., Whelan, F. J., Surette, M. G., Moore, C., Schertzer, J. D., Sloboda, D. M., et al. (2015). Pregnancy-related changes in the maternal gut microbiota are dependent upon the mother's periconceptional diet. Gut Microbes 6 (5), 310–320. doi: 10.1080/19490976.2015.1086056
Gomez-Arango, L. F., Barrett, H. L., Mcintyre, H. D., Callaway, L. K., Morrison, M., Dekker Nitert, M., et al. (2016). Increased systolic and diastolic blood pressure is associated with altered gut microbiota composition and butyrate production in early pregnancy. Hypertension 68 (4), 974–981. doi: 10.1161/HYPERTENSIONAHA.116.07910
Gomez De Agüero, M., Ganal-Vonarburg, S. C., Fuhrer, T., Rupp, S., Uchimura, Y., Li, H., et al. (2016). The maternal microbiota drives early postnatal innate immune development. Science 351 (6279), 1296–1302. doi: 10.1126/science.aad2571
Gopalsamy, G., Mortimer, E., Greenfield, P., Bird, A. R., Young, G. P., Christophersen, C. T., et al. (2019). Resistant starch is actively fermented by infant faecal microbiota and increases microbial diversity. Nutrients 11 (6), 1345. doi: 10.3390/nu11061345
Hang, S., Paik, D., Yao, L., Kim, E., Trinath, J., Lu, J., et al. (2019). Bile acid metabolites control T(H)17 and t(reg) cell differentiation. Nature 576 (7785), 143–148. doi: 10.1038/s41586-019-1785-z
Hansen, C. H., Krych, L., Nielsen, D. S., Vogensen, F. K., Hansen, L. H., Sørensen, S. J., et al. (2012). Early life treatment with vancomycin propagates akkermansia muciniphila and reduces diabetes incidence in the NOD mouse. Diabetologia 55 (8), 2285–2294. doi: 10.1007/s00125-012-2564-7
He, J., Zhang, P., Shen, L., Niu, L., Tan, Y., Chen, L., et al. (2020). Short-chain fatty acids and their association with signalling pathways in inflammation, glucose and lipid metabolism. Int. J. Mol. Sci. 21 (17), 6356. doi: 10.3390/ijms21176356
Heimann, E., Nyman, M., Degerman, E. (2015). Propionic acid and butyric acid inhibit lipolysis and de novo lipogenesis and increase insulin-stimulated glucose uptake in primary rat adipocytes. Adipocyte 4 (2), 81–88. doi: 10.4161/21623945.2014.960694
Heinemann, C., Heink, S., Petermann, F., Vasanthakumar, A., Rothhammer, V., Doorduijn, E., et al. (2014). IL-27 and IL-12 oppose pro-inflammatory IL-23 in CD4+ T cells by inducing Blimp1. Nat. Commun. 5, 3770. doi: 10.1038/ncomms4770
Henagan, T. M., Stefanska, B., Fang, Z., Navard, A. M., Ye, J., Lenard, N. R., et al. (2015). Sodium butyrate epigenetically modulates high-fat diet-induced skeletal muscle mitochondrial adaptation, obesity and insulin resistance through nucleosome positioning. Br. J. Pharmacol. 172 (11), 2782–2798. doi: 10.1111/bph.13058
Hsu, C. N., Lin, Y. J., Hou, C. Y., Tain, Y. L. (2018). Maternal administration of probiotic or prebiotic prevents Male adult rat offspring against developmental programming of hypertension induced by high fructose consumption in pregnancy and lactation. Nutrients 10 (9), 1229. doi: 10.3390/nu10091229
Hu, M., Eviston, D., Hsu, P., Mariño, E., Chidgey, A., Santner-Nanan, B., et al. (2019). Decreased maternal serum acetate and impaired fetal thymic and regulatory T cell development in preeclampsia. Nat. Commun. 10 (1), 3031. doi: 10.1038/s41467-019-10703-1
Huang, L., Cai, M., Li, L., Zhang, X., Xu, Y., Xiao, J., et al. (2021). Gut microbiota changes in preeclampsia, abnormal placental growth and healthy pregnant women. BMC Microbiol. 21 (1), 265. doi: 10.1186/s12866-021-02327-7
Hunter, M. M., Wang, A., Parhar, K. S., Johnston, M. J., Van Rooijen, N., Beck, P. L., et al. (2010). In vitro-derived alternatively activated macrophages reduce colonic inflammation in mice. Gastroenterology 138 (4), 1395–1405. doi: 10.1053/j.gastro.2009.12.041
Ishimwe, J. A. (2021). Maternal microbiome in preeclampsia pathophysiology and implications on offspring health. Physiol. Rep. 9 (10), e14875. doi: 10.14814/phy2.14875
Ishimwe, J. A., Akinleye, A., Johnson, A. C., Garrett, M. R., Sasser, J. M. (2021). Gestational gut microbial remodeling is impaired in a rat model of preeclampsia superimposed on chronic hypertension. Physiol. Genomics 53 (3), 125–136. doi: 10.1152/physiolgenomics.00121.2020
Itani, H. A., Xiao, L., Saleh, M. A., Wu, J., Pilkinton, M. A., Dale, B. L., et al. (2016). CD70 exacerbates blood pressure elevation and renal damage in response to repeated hypertensive stimuli. Circ. Res. 118 (8), 1233–1243. doi: 10.1161/CIRCRESAHA.115.308111
Ji, J., Shu, D., Zheng, M., Wang, J., Luo, C., Wang, Y., et al. (2016). Microbial metabolite butyrate facilitates M2 macrophage polarization and function. Sci. Rep. 6, 24838. doi: 10.1038/srep24838
Jiang, X., Huang, X., Tong, Y., Gao, H. (2020). Butyrate improves cardiac function and sympathetic neural remodeling following myocardial infarction in rats. Can. J. Physiol. Pharmacol. 98 (6), 391–399. doi: 10.1139/cjpp-2019-0531
Jin, J., Gao, L., Zou, X., Zhang, Y., Zheng, Z., Zhang, X., et al. (2022). Gut dysbiosis promotes preeclampsia by regulating macrophages and trophoblasts. Circ. Res. 131 (6), 492–506. doi: 10.1161/CIRCRESAHA.122.320771
Kell, D. B., Kenny, L. C. (2016). A dormant microbial component in the development of preeclampsia. Front. Med. (Lausanne) 3, 60. doi: 10.3389/fmed.2016.00060
Kim, S., Goel, R., Kumar, A., Qi, Y., Lobaton, G., Hosaka, K., et al. (2018). Imbalance of gut microbiome and intestinal epithelial barrier dysfunction in patients with high blood pressure. Clin. Sci. (Lond) 132 (6), 701–718. doi: 10.1042/CS20180087
Kim, D., Zeng, M. Y., Núñez, G. (2017). The interplay between host immune cells and gut microbiota in chronic inflammatory diseases. Exp. Mol. Med. 49 (5), e339. doi: 10.1038/emm.2017.24
Koch, M. A., Reiner, G. L., Lugo, K. A., Kreuk, L. S., Stanbery, A. G., Ansaldo, E., et al. (2016). Maternal IgG and IgA antibodies dampen mucosal T helper cell responses in early life. Cell 165 (4), 827–841. doi: 10.1016/j.cell.2016.04.055
Koeth, R. A., Wang, Z., Levison, B. S., Buffa, J. A., Org, E., Sheehy, B. T., et al. (2013). Intestinal microbiota metabolism of l-carnitine, a nutrient in red meat, promotes atherosclerosis. Nat. Med. 19 (5), 576–585. doi: 10.1038/nm.3145
Koren, O., Goodrich, J. K., Cullender, T. C., Spor, A., Laitinen, K., Bäckhed, H. K., et al. (2012). Host remodeling of the gut microbiome and metabolic changes during pregnancy. Cell 150 (3), 470–480. doi: 10.1016/j.cell.2012.07.008
Lavelle, A., Sokol, H. (2020). Gut microbiota-derived metabolites as key actors in inflammatory bowel disease. Nat. Rev. Gastroenterol. Hepatol. 17 (4), 223–237. doi: 10.1038/s41575-019-0258-z
Lee, C., Kim, B. G., Kim, J. H., Chun, J., Im, J. P., Kim, J. S., et al. (2017). Sodium butyrate inhibits the NF-kappa b signaling pathway and histone deacetylation, and attenuates experimental colitis in an IL-10 independent manner. Int. Immunopharmacol 51, 47–56. doi: 10.1016/j.intimp.2017.07.023
Ley, R. E., Bäckhed, F., Turnbaugh, P., Lozupone, C. A., Knight, R. D., Gordon, J. I., et al. (2005). Obesity alters gut microbial ecology. Proc. Natl. Acad. Sci. U.S.A. 102 (31), 11070–11075. doi: 10.1073/pnas.0504978102
Ley, R. E., Turnbaugh, P. J., Klein, S., Gordon, J. I. (2006). Microbial ecology: human gut microbes associated with obesity. Nature 444 (7122), 1022–1023. doi: 10.1038/4441022a
Li, P., Wang, H., Guo, L., Gou, X., Chen, G., Lin, D., et al. (2022). Association between gut microbiota and preeclampsia-eclampsia: a two-sample mendelian randomization study. BMC Med. 20 (1), 443. doi: 10.1186/s12916-022-02657-x
Li, J., Zhao, F., Wang, Y., Chen, J., Tao, J., Tian, G., et al. (2017). Gut microbiota dysbiosis contributes to the development of hypertension. Microbiome 5 (1), 14. doi: 10.1186/s40168-016-0222-x
Litwin, M., Kułaga, Z. (2021). Obesity, metabolic syndrome, and primary hypertension. Pediatr. Nephrol. 36 (4), 825–837. doi: 10.1007/s00467-020-04579-3
Liu, J., Yang, H., Yin, Z., Jiang, X., Zhong, H., Qiu, D., et al. (2017). Remodeling of the gut microbiota and structural shifts in preeclampsia patients in south China. Eur. J. Clin. Microbiol. Infect. Dis. 36 (4), 713–719. doi: 10.1007/s10096-016-2853-z
Louis, P., Flint, H. J. (2017). Formation of propionate and butyrate by the human colonic microbiota. Environ. Microbiol. 19 (1), 29–41. doi: 10.1111/1462-2920.13589
Lv, L.-J., Li, S.-H., Li, S.-C., Zhong, Z-C., Duan, H-L., Tian, C., et al. (2019). Early-onset preeclampsia is associated with gut microbial alterations in antepartum and postpartum women. Front. Cell Infect. Microbiol. 9, 224. doi: 10.3389/fcimb.2019.00224
Macfarlane, S., Macfarlane, G. T. (2003). Regulation of short-chain fatty acid production. Proc. Nutr. Soc. 62 (1), 67–72. doi: 10.1079/PNS2002207
Machate, D. J., Figueiredo, P. S., Marcelino, G., Guimarães, R. C.A., Hiane, P. A., Bogo, D., et al. (2020). Fatty acid diets: regulation of gut microbiota composition and obesity and its related metabolic dysbiosis. Int. J. Mol. Sci. 21 (11), 4093. doi: 10.3390/ijms21114093
Malik, R., Kumar, V. (2017). Hypertension in pregnancy. Adv. Exp. Med. Biol. 956, 375–393. doi: 10.1007/5584_2016_150
Marques, F. Z., Nelson, E., Chu, P. Y., Horlock, D., Fiedler, A., Ziemann, M., et al. (2017). High-fiber diet and acetate supplementation change the gut microbiota and prevent the development of hypertension and heart failure in hypertensive mice. Circulation 135 (10), 964–977. doi: 10.1161/CIRCULATIONAHA.116.024545
Mcelwain, C. J., Tuboly, E., Mccarthy, F. P., McCarthy, C. M. (2020). Mechanisms of endothelial dysfunction in pre-eclampsia and gestational diabetes mellitus: windows into future cardiometabolic health? Front. Endocrinol. (Lausanne) 11, 655. doi: 10.3389/fendo.2020.00655
Meijer, K., De Vos, P., Priebe, M. G. (2010). Butyrate and other short-chain fatty acids as modulators of immunity: what relevance for health? Curr. Opin. Clin. Nutr. Metab. Care 13 (6), 715–721. doi: 10.1097/MCO.0b013e32833eebe5
Meijer, S., Pasquinelli, E., Renzi, S., Lavasani, S., Nouri, M., Erlandsson, L., et al. (2023). Gut micro- and mycobiota in preeclampsia: bacterial composition differences suggest role in pathophysiology. Biomolecules 13 (2), 346. doi: 10.3390/biom13020346
Mell, B., Jala, V. R., Mathew, A. V., Byun, J., Waghulde, H., Zhang, Y., et al. (2015). Evidence for a link between gut microbiota and hypertension in the Dahl rat. Physiol. Genomics 47 (6), 187–197. doi: 10.1152/physiolgenomics.00136.2014
Metzger, B. E., Lowe, L. P., Dyer, A. R., Trimble, E. R., Chaovarindr, U., Coustan, D. R., et al. (2008). Hyperglycemia and adverse pregnancy outcomes. N Engl. J. Med. 358 (19), 1991–2002. doi: 10.1056/NEJMoa0707943
Michaudel, C., Sokol, H. (2020). The gut microbiota at the service of immunometabolism. Cell Metab. 32 (4), 514–523. doi: 10.1016/j.cmet.2020.09.004
Mikita, T., Campbell, D., Wu, P., Williamson, K., Schindler, U. (1996). Requirements for interleukin-4-induced gene expression and functional characterization of Stat6. Mol. Cell Biol. 16 (10), 5811–5820. doi: 10.1128/MCB.16.10.5811
Miller, T. L., Wolin, M. J. (1996). Pathways of acetate, propionate, and butyrate formation by the human fecal microbial flora. Appl. Environ. Microbiol. 62 (5), 1589–1592. doi: 10.1128/aem.62.5.1589-1592.1996
Mol, B. W. J., Roberts, C. T., Thangaratinam, S., Magee, L. A., de Groot, C. J.M., Hofmeyr, G. J., et al. (2016). Pre-eclampsia. Lancet 387 (10022), 999–1011. doi: 10.1016/S0140-6736(15)00070-7
Morgan, J. A., Bombell, S., Mcguire, W. (2013). Association of plasminogen activator inhibitor-type 1 (-675 4G/5G) polymorphism with pre-eclampsia: systematic review. PloS One 8 (2), e56907. doi: 10.1371/journal.pone.0056907
Mosser, D. M., Edwards, J. P. (2008). Exploring the full spectrum of macrophage activation. Nat. Rev. Immunol. 8 (12), 958–969. doi: 10.1038/nri2448
Muralitharan, R. R., Jama, H. A., Xie, L., Peh, A., Snelson, M., Marques, F. Z., et al. (2020). Microbial peer pressure: the role of the gut microbiota in hypertension and its complications. Hypertension 76 (6), 1674–1687. doi: 10.1161/HYPERTENSIONAHA.120.14473
Nyangahu, D. D., Lennard, K. S., Brown, B. P., Darby, M. G., Wendoh, J. M., Havyarimana, E., et al. (2018). Disruption of maternal gut microbiota during gestation alters offspring microbiota and immunity. Microbiome 6 (1), 124. doi: 10.1186/s40168-018-0511-7
Onyszkiewicz, M., Gawrys-Kopczynska, M., Konopelski, P., Aleksandrowicz, M., Sawicka, A., Koźniewska, E., et al. (2019). Butyric acid, a gut bacteria metabolite, lowers arterial blood pressure via colon-vagus nerve signaling and GPR41/43 receptors. Pflugers Arch. 471 (11-12), 1441–1453. doi: 10.1007/s00424-019-02322-y
Parada Venegas, D., de la Fuente, M. K., Landskron, G., González, M. J., Quera, R., Dijkstra, G., et al. (2019). Short chain fatty acids (SCFAs)-mediated gut epithelial and immune regulation and its relevance for inflammatory bowel diseases. Front. Immunol. 10, 277. doi: 10.3389/fimmu.2019.00277
Paul, H. A., Bomhof, M. R., Vogel, H. J., Reimer, R. A. (2016). Diet-induced changes in maternal gut microbiota and metabolomic profiles influence programming of offspring obesity risk in rats. Sci. Rep. 6, 20683. doi: 10.1038/srep20683
Phoswa, W. N., Khaliq, O. P. (2021). The role of oxidative stress in hypertensive disorders of pregnancy (Preeclampsia, gestational hypertension) and metabolic disorder of pregnancy (Gestational diabetes mellitus). Oxid. Med. Cell Longev 2021, 5581570. doi: 10.1155/2021/5581570
Pickard, J. M., Zeng, M. Y., Caruso, R., Núñez, G. (2017). Gut microbiota: role in pathogen colonization, immune responses, and inflammatory disease. Immunol. Rev. 279 (1), 70–89. doi: 10.1111/imr.12567
Pinheiro, R. L., Areia, A. L., Mota Pinto, A., Donato, H. (2019). Advanced maternal age: adverse outcomes of pregnancy, a meta-analysis. Acta Med. Port 32 (3), 219–226. doi: 10.20344/amp.11057
Pluznick, J. (2014). A novel SCFA receptor, the microbiota, and blood pressure regulation. Gut Microbes 5 (2), 202–207. doi: 10.4161/gmic.27492
Pluznick, J. L., Protzko, R. J., Gevorgyan, H., Peterlin, Z., Sipos, A., Han, J., et al. (2013). Olfactory receptor responding to gut microbiota-derived signals plays a role in renin secretion and blood pressure regulation. Proc. Natl. Acad. Sci. U.S.A. 110 (11), 4410–4415. doi: 10.1073/pnas.1215927110
Poon, L. C., Shennan, A., Hyett, J. A., Kapur, A., Hadar, E., Divakar, H., et al. (2019). The international federation of gynecology and obstetrics (FIGO) initiative on pre-eclampsia: a pragmatic guide for first-trimester screening and prevention. Int. J. Gynaecol Obstet 145 (Suppl 1), 1–33. doi: 10.1002/ijgo.12802
Pouteau, E., Nguyen, P., Ballèvre, O., Krempf, M. (2003). Production rates and metabolism of short-chain fatty acids in the colon and whole body using stable isotopes. Proc. Nutr. Soc. 62 (1), 87–93. doi: 10.1079/PNS2003208
Qi, Y., Kim, S., Richards, E. M., Raizada, M. K., Pepine, C. J. (2017). Gut microbiota: potential for a unifying hypothesis for prevention and treatment of hypertension. Circ. Res. 120 (11), 1724–1726. doi: 10.1161/CIRCRESAHA.117.310734
Ragsdale, S. W., Pierce, E. (2008). Acetogenesis and the wood-ljungdahl pathway of CO(2) fixation. Biochim. Biophys. Acta 1784 (12), 1873–1898. doi: 10.1016/j.bbapap.2008.08.012
Rana, S., Lemoine, E., Granger, J. P., Karumanchi, S. A. (2019). Preeclampsia: pathophysiology, challenges, and perspectives. Circ. Res. 124 (7), 1094–1112. doi: 10.1161/CIRCRESAHA.118.313276
Raqib, R., Sarker, P., Mily, A., Alam, N. H., Arifuzzaman, A. S., Rekha, R. S., et al. (2012). Efficacy of sodium butyrate adjunct therapy in shigellosis: a randomized, double-blind, placebo-controlled clinical trial. BMC Infect. Dis. 12, 111. doi: 10.1186/1471-2334-12-111
Reuschel, E., Toelge, M., Haeusler, S., Deml, L., Seelbach-Goebel, B., Solano, M. E., et al. (2020). Perinatal gram-positive bacteria exposure elicits distinct cytokine responses In vitro. Int. J. Mol. Sci. 22 (1), 332. doi: 10.3390/ijms22010332
Robles-Vera, I., Toral, M., de la Visitación, N., Sánchez, M., Romero, M., Olivares, M., et al. (2018). The probiotic lactobacillus fermentum prevents dysbiosis and vascular oxidative stress in rats with hypertension induced by chronic nitric oxide blockade. Mol. Nutr. Food Res. 62 (19), e1800298. doi: 10.1002/mnfr.201800298
Robles-Vera, I., Toral, M., de la Visitación, N., Sánchez, M., Gómez-Guzmán, M., Romero, M., et al. (2020). Probiotics prevent dysbiosis and the rise in blood pressure in genetic hypertension: role of short-chain fatty acids. Mol. Nutr. Food Res. 64 (6), e1900616. doi: 10.1002/mnfr.201900616
Roy, R., Nguyen-Ngo, C., Lappas, M. (2020). Short-chain fatty acids as novel therapeutics for gestational diabetes. J. Mol. Endocrinol. 65 (2), 21–34. doi: 10.1530/JME-20-0094
Ruppin, H., Bar-Meir, S., Soergel, K. H., Wood, C. M., Schmitt, M. G., Jr. (1980). Absorption of short-chain fatty acids by the colon. Gastroenterology 78 (6), 1500–1507. doi: 10.1016/S0016-5085(19)30508-6
Saji, N., Hisada, T., Tsuduki, T., Niida, S., Toba, K., Sakurai, T. (2019). Proportional changes in the gut microbiome: a risk factor for cardiovascular disease and dementia? Hypertens. Res. 42 (7), 1090–1091. doi: 10.1038/s41440-019-0218-6
Sakakibara, S., Yamauchi, T., Oshima, Y., Tsukamoto, Y., Kadowaki, T. (2006). Acetic acid activates hepatic AMPK and reduces hyperglycemia in diabetic KK-a(y) mice. Biochem. Biophys. Res. Commun. 344 (2), 597–604. doi: 10.1016/j.bbrc.2006.03.176
Smith, P. M., Howitt, M. R., Panikov, N., Michaud, M., Gallini, C. A., Bohlooly, Y. M. (2013). The microbial metabolites, short-chain fatty acids, regulate colonic treg cell homeostasis. Science 341 (6145), 569–573.
Soderborg, T. K., Carpenter, C. M., Janssen, R. C., Weir, T. L., Robertson, C. E., Ir, D., et al. (2020). Gestational diabetes is uniquely associated with altered early seeding of the infant gut microbiota. Front. Endocrinol. (Lausanne) 11, 603021. doi: 10.3389/fendo.2020.603021
Sun, M., Wu, W., Chen, L., Yang, W., Huang, X., Ma, C., et al. (2018). Microbiota-derived short-chain fatty acids promote Th1 cell IL-10 production to maintain intestinal homeostasis. Nat. Commun. 9 (1), 3555. doi: 10.1038/s41467-018-05901-2
Tang, W. H. W., Li, D. Y., Hazen, S. L. (2019). Dietary metabolism, the gut microbiome, and heart failure. Nat. Rev. Cardiol. 16 (3), 137–154. doi: 10.1038/s41569-018-0108-7
Tang, R., Xiao, G., Jian, Y., Yuan, Q., Jiang, C., Wang, W., et al. (2022). The gut microbiota dysbiosis in preeclampsia contributed to trophoblast cell proliferation, invasion, and migration via lncRNA BC030099/NF-κB pathway. Mediators Inflammation 2022, 6367264. doi: 10.1155/2022/6367264
Toral, M., Robles-Vera, I., de la Visitación, N., Romero, M., Sánchez, M., Gómez-Guzmán, M., et al. (2019a). Role of the immune system in vascular function and blood pressure control induced by faecal microbiota transplantation in rats. Acta Physiol. (Oxf) 227 (1), e13285. doi: 10.1111/apha.13285
Toral, M., Robles-Vera, I., de la Visitación, N., Romero, M., Yang, T., Sánchez, M., et al. (2019b). Critical role of the interaction gut microbiota - sympathetic nervous system in the regulation of blood pressure. Front. Physiol. 10, 231. doi: 10.3389/fphys.2019.00231
Torres, J., Hu, J., Seki, A., Eisele, C., Nair, N., Huang, R., et al. (2020). Infants born to mothers with IBD present with altered gut microbiome that transfers abnormalities of the adaptive immune system to germ-free mice. Gut 69 (1), 42–51. doi: 10.1136/gutjnl-2018-317855
Turnbaugh, P. J., Ley, R. E., Mahowald, M. A., Magrini, V., Mardis, E. R., Gordon, J. I., et al. (2006). An obesity-associated gut microbiome with increased capacity for energy harvest. Nature 444 (7122), 1027–1031. doi: 10.1038/nature05414
Van Den Hoek, A. M., Heijboer, A. C., Corssmit, E. P., Voshol, P. J., Romijn, J. A., Havekes, L. M., et al., , et al. (2004). PYY3-36 reinforces insulin action on glucose disposal in mice fed a high-fat diet. Diabetes 53 (8), 1949–1952. doi: 10.2337/diabetes.53.8.1949
Vinolo, M. A., Rodrigues, H. G., Hatanaka, E., Hebeda, C. B., Farsky, S. H., Curi, R., et al. (2009). Short-chain fatty acids stimulate the migration of neutrophils to inflammatory sites. Clin. Sci. (Lond) 117 (9), 331–338. doi: 10.1042/CS20080642
Vinolo, M. A., Rodrigues, H. G., Nachbar, R. T., Curi, R. (2011). Regulation of inflammation by short chain fatty acids. Nutrients 3 (10), 858–876. doi: 10.3390/nu3100858
Wang, J., Gu, X., Yang, J., Wei, Y., Zhao, Y. (2019). Gut microbiota dysbiosis and increased plasma LPS and TMAO levels in patients with preeclampsia. Front. Cell Infect. Microbiol. 9, 409. doi: 10.3389/fcimb.2019.00409
Wang, J., Shi, Z. H., Yang, J., Wei, Y., Wang, X. Y., Zhao, Y. Y., et al. (2020). Gut microbiota dysbiosis in preeclampsia patients in the second and third trimesters. Chin. Med. J. (Engl) 133 (9), 1057–1065. doi: 10.1097/CM9.0000000000000734
Wang, S. Z., Yu, Y. J., Adeli, K. (2020). Role of gut microbiota in neuroendocrine regulation of carbohydrate and lipid metabolism via the microbiota-Gut-Brain-Liver axis. Microorganisms 8 (4), 527. doi: 10.3390/microorganisms8040527
Wu, Y., Xu, H., Tu, X., Gao, Z. (2021). The role of short-chain fatty acids of gut microbiota origin in hypertension. Front. Microbiol. 12, 730809. doi: 10.3389/fmicb.2021.730809
Yan, H., Ajuwon, K. M. (2015). Mechanism of butyrate stimulation of triglyceride storage and adipokine expression during adipogenic differentiation of porcine stromovascular cells. PloS One 10 (12), e0145940. doi: 10.1371/journal.pone.0145940
Yan, Q., Gu, Y., Li, X., Yang, W., Jia, L., Chen, C., et al. (2017). Alterations of the gut microbiome in hypertension. Front. Cell Infect. Microbiol. 7, 381. doi: 10.3389/fcimb.2017.00381
Yang, T., Richards, E. M., Pepine, C. J., Raizada, M. K. (2018). The gut microbiota and the brain-gut-kidney axis in hypertension and chronic kidney disease. Nat. Rev. Nephrol. 14 (7), 442–456. doi: 10.1038/s41581-018-0018-2
Yang, T., Santisteban, M. M., Rodriguez, V., Li, E., Ahmari, N., Carvajal, J. M., et al. (2015). Gut dysbiosis is linked to hypertension. Hypertension 65 (6), 1331–1340. doi: 10.1161/HYPERTENSIONAHA.115.05315
Yang, Y., Wu, N. (2022). Gestational diabetes mellitus and preeclampsia: correlation and influencing factors. Front. Cardiovasc. Med. 9, 831297. doi: 10.3389/fcvm.2022.831297
Yong, W., Zhao, Y., Jiang, X., Li, P. (2022). Sodium butyrate alleviates pre-eclampsia in pregnant rats by improving the gut microbiota and short-chain fatty acid metabolites production. J. Appl. Microbiol. 132 (2), 1370–1383. doi: 10.1111/jam.15279
Yu, Y., Mao, G., Wang, J., Zhu, L., Lv, X., Tong, Q., et al. (2018). Gut dysbiosis is associated with the reduced exercise capacity of elderly patients with hypertension. Hypertens. Res. 41 (12), 1036–1044. doi: 10.1038/s41440-018-0110-9
Zasloff, M. (2002). Antimicrobial peptides of multicellular organisms. Nature 415 (6870), 389–395. doi: 10.1038/415389a
Zhao, S., Liu, W., Wang, J., Shi, J., Sun, Y., Wang, W., et al. (2017). Akkermansia muciniphila improves metabolic profiles by reducing inflammation in chow diet-fed mice. J. Mol. Endocrinol. 58 (1), 1–14. doi: 10.1530/JME-16-0054
Zhao, D., Su, H., Cheng, J., Wang, X., Xie, M., Li, K., et al. (2015). Prenatal antibiotic use and risk of childhood wheeze/asthma: a meta-analysis. Pediatr. Allergy Immunol. 26 (8), 756–764. doi: 10.1111/pai.12436
Zhao, Y., Wang, B., Zhao, X., Guo, Y., Sun, L., Zhang, C., et al. (2022). The effect of gut microbiota dysbiosis on patients with preeclampsia. Front. Cell Infect. Microbiol. 12, 1022857. doi: 10.3389/fcimb.2022.1022857
Keywords: short-chain fatty acid, preeclampsia, gut microbiota, gestational diseases, pathogenesis of preeclampsia, immunocyte, inflammatory response
Citation: Cui J, Wang J and Wang Y (2023) The role of short-chain fatty acids produced by gut microbiota in the regulation of pre-eclampsia onset. Front. Cell. Infect. Microbiol. 13:1177768. doi: 10.3389/fcimb.2023.1177768
Received: 04 March 2023; Accepted: 21 June 2023;
Published: 12 July 2023.
Edited by:
Zhengwei Huang, Shanghai Jiao Tong University, ChinaReviewed by:
Naser Alsharairi, Griffith University, AustraliaXiangfeng Zeng, Chinese Academy of Sciences (CAS), China
Lijuan Lv, Guangdong Province Women and Children Hospital, China
Copyright © 2023 Cui, Wang and Wang. This is an open-access article distributed under the terms of the Creative Commons Attribution License (CC BY). The use, distribution or reproduction in other forums is permitted, provided the original author(s) and the copyright owner(s) are credited and that the original publication in this journal is cited, in accordance with accepted academic practice. No use, distribution or reproduction is permitted which does not comply with these terms.
*Correspondence: Ying Wang, d2FuZ3lpbmdfc2hlbmdqaW5nQDE2My5jb20=; Jun Wang, Y2hlcnJ5X2pfd0AxMjYuY29t