- 1Department of Medicine and Epidemiology, School of Veterinary Medicine, University of California, Davis, Davis, CA, United States
- 2Fisheries, Embrapa Amazônia Ocidental, Manaus, Amazonas, Brazil
- 3Fish Health Laboratory, California Department of Fish and Wildlife, Rancho Cordova, CA, United States
- 4Department of Fish and Wildlife Sciences, College of Natural Resources, University of Idaho, Moscow, ID, United States
- 5Department of Fisheries and Wildlife, College of Agriculture and Natural Resources, Department of Pathobiology and Diagnostic Investigation, College of Veterinary Medicine, Michigan State University, East Lansing, MI, United States
Flavobacterial diseases, caused by bacteria in the order Flavobacteriales, are responsible for devastating losses in farmed and wild fish populations worldwide. The genera Flavobacterium (Family Flavobacteriaceae) and Chryseobacterium (Weeksellaceae) encompass the most well-known agents of fish disease in the order, but the full extent of piscine-pathogenic species within these diverse groups is unresolved, and likely underappreciated. To identify emerging agents of flavobacterial disease in US aquaculture, 183 presumptive Flavobacterium and Chryseobacterium isolates were collected from clinically affected fish representing 19 host types, from across six western states. Isolates were characterized by 16S rRNA gene sequencing and phylogenetic analysis using the gyrB gene. Antimicrobial susceptibility profiles were compared between representatives from each major phylogenetic clade. Of the isolates, 52 were identified as Chryseobacterium species and 131 as Flavobacterium. The majority of Chryseobacterium isolates fell into six clades (A-F) consisting of ≥ 5 fish isolates with ≥ 70% bootstrap support, and Flavobacterium into nine (A-I). Phylogenetic clades showed distinct patterns in antimicrobial susceptibility. Two Chryseobacterium clades (F & G), and four Flavobacterium clades (B, G-I) had comparably high minimal inhibitory concentrations (MICs) for 11/18 antimicrobials tested. Multiple clades in both genera exhibited MICs surpassing the established F. psychrophilum breakpoints for oxytetracycline and florfenicol, indicating potential resistance to two of the three antimicrobials approved for use in finfish aquaculture. Further work to investigate the virulence and antigenic diversity of these genetic groups will improve our understanding of flavobacterial disease, with applications for treatment and vaccination strategies.
1 Introduction
The order Flavobacteriales comprises a rapidly expanding group of gram-negative bacteria. Many species within the order are environmental bacteria and populate a wide range of aquatic and terrestrial habitats worldwide (Bernardet, 2015). However, some genera contain established or emerging pathogens of animals and humans, such as Flavobacterium, Chryseobacterium, Tenacibaculum, Elizabethkingia, and Ornithobacterium (Loch and Faisal, 2015a). Flavobacterium and Chryseobacterium hold particular significance for aquaculture and fish health, as they include bacterial species responsible for devastating losses in farmed and wild fish populations, with consequently substantial economic and ecological effects. Acute outbreaks of piscine flavobacteriosis can cause mortality rates upwards of 70%, while subacute or chronic cases result in persistent low-level mortalities (Austin and Austin, 2016). Flavobacteria may also cause lingering deficits in surviving fish, or act as spoilage organisms to further reduce fish fitness and production outputs (Kent et al., 1989; Madsen et al., 2001; Bernardet et al., 2005). Flavobacterial fish disease was first reported in 1922 (Davis, 1922), and in the hundred years since, there has been extensive scientific effort towards preventing and controlling outbreaks. These efforts, however, have been compromised by the rapid expansion and continual restructuring of the genera, species, and strains associated with the disease.
The genus Chryseobacterium was first proposed to accommodate six species excluded from Flavobacterium in 1994 (Vandamme et al., 1994). In 2006, following further revision of the genera, Chryseobacterium included 10 species (Bernardet et al., 2006), and Flavobacterium, 26 (Bernardet and Bowman, 2006). Both genera have seen extensive additions over the last decade, and at the time of writing, the number of validly published, nonsynonymous names has soared to 136 Chryseobacterium species and 269 Flavobacterium species (Parte et al., 2020). The expansion in newly described species has been matched by increasing reports of new agents associated with fish and fish disease. The most well-established flavobacterial piscine pathogens are Flavobacterium psychrophilum, the causative agent of bacterial coldwater disease, F. branchiophilum the agent of bacterial gill disease, and F. columnare, of columnaris disease (Loch and Faisal, 2015a; Wahli and Madsen, 2018). Flavobacterium columnare has been recently reclassified into four related species: F. columnare, F. covae, F. davisii and F. oreochromis (LaFrentz et al., 2022). In addition to these historical pathogens, however, almost fifty other species of Flavobacterium and Chryseobacterium have been implicated in fish disease (Tables 1, 2). For example, Flavobacterium tructae, F. oncorhynchi, F. plurextorum, Chryseobacterium piscicola, C. scopthalmum, C. chaponense, and C. indologenes have been isolated from a diverse range of both healthy and diseased, cultured or wild fish worldwide (Loch and Faisal, 2015a; Sebastião et al., 2019a). Chryseobacterium species such as C. indologenes may also be capable of causing disease in other animals, including humans (Vaneechoutte et al., 2007; Holmes et al., 2013; Lin et al., 2019). Some of these atypical flavobacterial strains have been assessed for their pathogenic potential through laboratory-controlled challenges in fish hosts (Tables 1, 2). However, the majority are linked to disease by nature of their isolation from the external or internal tissues of clinically affected fish. Infected fish often display signs mimicking those caused by established etiologic agents, including skin ulceration, fin erosion, and gill necrosis (Loch and Faisal, 2016b; Jung et al., 2022; Malick et al., 2022). Additionally, fish may be concurrently infected by more than one species, and atypical flavobacteria may not be easily distinguished by conventional diagnostic methods (Bernardet et al., 2005; Bruce et al., 2020). Current limitations in epidemiological and pathological data obfuscate the risk these potential pathogens pose to aquaculture.
To address these knowledge gaps, international efforts in surveillance and characterization of fish associated Flavobacterium and Chryseobacterium strains are gaining in momentum. Methods to identify isolates more conclusively through biochemical or molecular means continue to be improved, and the advent of next generation sequencing has aided the discovery of genetic factors related to virulence, immunogenicity, and antimicrobial resistance, together supporting development of new tools for disease treatment and prevention (García-López et al., 2019; Saticioglu et al., 2021c; Jung et al., 2022; Malick et al., 2022). Antimicrobial resistance is a particularly notorious issue for both human and animal flavobacterial infections, as high intrinsic resistance to multiple classes of antimicrobials is archetypal of some genera (Verner-Jeffreys et al., 2017). The susceptibility profiles of historic pathogens, such as F. psychrophilum and F. columnare are well-studied, but there is a lack of information on antimicrobial resistance (AMR) characteristics for more recently described species.
In the United States, such newly described Flavobacterium and Chryseobacterium species have been identified in the Great Lakes region, through several studies investigating flavobacterial community composition in salmonids at different life stages (Loch et al., 2013; Loch and Faisal, 2014a; Loch and Faisal, 2015b; Loch and Faisal, 2016a; Loch and Faisal, 2016c; Loch and Faisal, 2018). Through these studies, species previously described only in other continents were identified, indicating the presence of these flavobacteria in the US. There is a paucity of information, however, on the distribution and prevalence of atypical Flavobacterium and Chryseobacterium in other regions of the country. The Western United States has a high concentration of salmonid production, an industry perpetually plagued by flavobacterial disease (Wahli and Madsen, 2018; United States Department of Agriculture, 2019). Despite this, only one study has investigated the circulating diversity of novel fish-associated flavobacteria in the region, limited to a sampling of Chryseobacterium spp. in California (Sebastião et al., 2019b). To better understand potential emerging threats to aquaculture in Western United States, we aimed to assess the diversity of Chryseobacterium and Flavobacterium associated with fish disease in the region. Yellow-pigmented bacteria isolated from clinically affected fish in six western states were collected and screened by sequencing of the 16S rRNA gene. Isolates were then typed by gyrB analysis to identify major clades associated with diseased fish for further phenotypic and antimicrobial characterization. Sequencing of the ubiquitous 16S rRNA gene remains the most common method for bacterial classification to the genus level but lacks the phylogenetic power to resolve closely related species in genera like Flavobacterium and Chryseobacterium (Janda and Abbott, 2007; Kumru et al., 2020). The gyrB gene typically has a faster evolution rate than the 16S rRNA gene, resulting in higher genetic heterogeneity and improved discrimination at the species level for bacterial genotyping (Wang et al., 2007; Galloway-Peña et al., 2014; Reichley et al., 2017).
2 Materials and methods
2.1 Bacteria
Over a period of 5 years, diverse yellow pigmented bacterial (YPB) strains were compiled from fish exhibiting signs of flavobacterial disease in the Western United States, either submitted to the Aquatic Animal Health Laboratory at the University of California, Davis, or collected in-house from diagnostic cases. Historical untyped or unusual YPB were also accepted, with initial collection dates ranging from 1991-2022. Bacteria were isolated from external lesions or internal organs and submitted as pure cultures on solid media. Bacteria of interest were considered to be those that exhibited morphologies typical for flavobacteria (yellow to orange pigmentation, gram-negative, catalase and oxidase positive), but were not initially identified as F. columnare or F. psychrophilum by species-specific qPCR (“atypical”) or other diagnostic methods. Bacterial cultures were expanded in 5 mL of Flavobacterium columnare growth media (FCGM; Farmer, 2004), diluted Mueller-Hinton media (dMH; Sigma-Aldrich, St. Louis, MO, USA), Modified Shieh media (MS; LaFrentz and Klesius, 2009) or tryptone yeast extract salts media (TYES; University of California Davis, Biological Media Services, USA) for 24-72h at 20°C with shaking. Bacterial suspensions were saved in 1 mL aliquots with 20% glycerol at −80°C until further use.
2.2 Genomic DNA extraction
Bacterial strains were revived from freezer stocks on TYES or MS agar at 20°C for 72-96 h. A single isolated colony was then transferred into 5 mL broth media for expansion at 20°C for 24-72 h with shaking. One milliliter of the expanded bacterial suspension was centrifuged for 10 min at 5,000 x g (7500 rpm). Genomic DNA (gDNA) was extracted from the concentrated pellet using the DNeasy® Blood and Tissue kit (Qiagen, Germantown, MD, USA) following manufacturer recommendations for gram-negative bacteria. The quality and quantity of recovered DNA was assessed using a NanoDrop™ One Microvolume UV-Vis Spectrophotometer (Thermo Fisher Scientific™, Waltham, MA USA) and samples with 260/280 ratios of 1.8-2.0 were cryogenically stored (-20°C) until further analysis.
2.3 Molecular identification
Primers previously used for identification and typing of unknown Flavobacteriales were employed (Table 3, Sebastião et al., 2019b). Amplification by PCR was conducted using either Phusion™, Platinum™ Taq or DreamTaq™ DNA polymerases (Thermo Fisher Scientific™, Waltham, MA USA) following manufacturer’s instructions. Phusion reaction mixtures (20 µL) consisted of 4 µL 5X Phusion™ HF Buffer, 0.4 µL dNTPs (10 mM), 1 µL each of the forward and reverse primers (10 µM), 0.2 µL Phusion™ High-Fidelity DNA polymerase (2 U/µL), 1-2 µL of template DNA and up to 20 µL diethyl pyrocarbonate (DEPC) water. Thirty seconds of initial denaturation at 98°C were followed by 35 cycles of 98°C for 10 s, 57°C for 30 s, and 72°C for 30s, with a final extension at 72°C for 5 min. For difficult templates, 3% DMSO was included in Phusion reaction mixtures and annealing temperatures adjusted to 6°C below the suggested melting temperature for each primer set (Table 3). Platinum Taq reaction mixtures (25 µL) consisted of 2.5 µL 10X high fidelity PCR buffer, 1 µL MgSO4, 0.5 µL dNTPs (10 mM), 0.5 µL each of the forward and reverse primers (10 µM), 0.1 µL Platinum™ Taq DNA polymerase high fidelity (5 U/µL), 1-2 µL of template DNA and up to 25 µL DEPC water. Thirty seconds of initial denaturation at 94°C were followed by 35 cycles of 94°C for 15 s, 51°C for 30 s, and final extension at 68°C for 1 minute. DreamTaq™ reaction mixtures (25 µL) consisted of 12.5 µl 2X DreamTaq Green PCR Master Mix, 1µL of each forward and reverse primer (10 µM), 7 µl of DNA, and 3.5 µl of DEPC water to volume. Five seconds of initial denaturation at 95°C were followed by 35 cycles of 95°C for 30 s, 55°C for 10 s, and 72°C for 60s, with a final extension at 72°C for 5 min.
Amplification reactions were electrophoresed through 1% agarose gels supplemented with SYBR® Safe DNA gel stain (Invitrogen, Waltham, MA USA; 1 µL/mL) alongside concurrently run molecular weight standards (Quick-Load® Purple 100 bp DNA Ladder, New England BioLabs, Ipswich, MA, USA) and visualized under ultraviolet light to confirm the presence of appropriately sized bands. PCR products were purified using the QIAquick PCR Purification Kit (Qiagen, Germantown MD, USA) and their concentration and purity assessed by Nanodrop. Purified products and corresponding forward and reverse primers were diluted and submitted for Sanger sequencing at the University of California, Davis Sequencing facility (UC Davis, CA, USA) or through GENEWIZ (South San Francisco, CA, USA). Forward and reverse sequences were imported into Geneious Prime (2022.2.2), trimmed to a 0.05 error probability limit, and de novo assembled. Isolate sequences for each gene were aligned by MUSCLE using default settings and trimmed to the region of quality bases shared by all isolate sequences. The nucleotide Basic Local Alignment Search Tool in Geneious (blastn; Altschul et al., 1990) was used to compare each isolate’s 16S rRNA gene fragment to a local database populated by 136 Chryseobacterium and 269 Flavobacterium type strains. The “closest known species” was determined based on the type-strain with the highest pairwise identity (PI) to the isolate along the entire sequenced fragment. Isolates that were not within the Flavobacterium or Chryseobacterium genera were removed from further analyses.
2.4 Phylogenetic analysis
Phylogenetic analyses using the gyrB gene were performed to compare evolutionary relationships between isolates and established species, and to identify fish disease associated clusters. Analyses were performed separately for Flavobacterium and Chryseobacterium isolates. A representative isolate sequence for the gyrB gene fragment was used in BLAST searches of a localized database populated by validly published Flavobacterium and Chryseobacterium species with whole genomes available for download from GenBank. Where type-strain genomes were absent, NCBI reference genomes were used as available. The database represented 178 Flavobacterium species and 94 Chryseobacterium species isolated from animal hosts or the environment (Supplementary Table 1). The resulting sequences from isolates and published strains were exported to MEGA-X (Kumar et al., 2018) and aligned by MUSCLE using default settings. A maximum likelihood tree was generated using the General Time Reversible model (Nei and Kumar, 2000) with a gamma distribution and invariable rates (GTR+G+I), selected based on Bayesian and corrected Akaike Information Criterion in MEGA-X. The percentage bootstrap confidence levels were calculated from 1000 re-samplings of the original data. Major clades were defined as clusters containing ≥ 5 fish isolates with ≥ 70% bootstrap support. Phylogenetic trees were exported from MEGA as Newick files and formatted and annotated in iTOL (Letunic and Bork, 2019).
2.5 Phenotypic characterization
A representative isolate from each of the major clades (n=15) was used for preliminary phenotypic comparison by conventional methods. Colony morphology was compared by assessing bacterial growth on TYES after 72 h at 20°C, while hemolytic capability was investigated by growth on trypticase soy agar supplemented with 5% sheep blood (SBA; University of California Davis, Biological Media Services, USA). Gram staining was carried out using established protocols. Catalase activity was assessed by application of 3% H2O2 and cytochrome oxidase by the swab test using tetramethyl-p-phenylenediamine dihydrochloride reagent (Remel, Lenexa, Kansas, USA).
2.6 Antimicrobial susceptibility profiling
Antimicrobial susceptibility profiles were investigated for three representative isolates from each of the major clades of atypical Flavobacterium and Chryseobacterium (n=39) recovered from clinically infected fish in the Western United States using the broth microdilution method. The minimal inhibitory concentrations for 18 antimicrobials were determined using the Sensititre Avian 1F plates (Thermo Fisher Scientific™, Waltham, MA USA) following CLSI guidelines established for F. psychrophilum (CLSI, 2020). Briefly, bacteria were revived from freezer stocks on TYES or MS agar at 20°C for 72 h and used to generate a 0.5 McFarland standard (~1.5 x 108 CFU/mL) in sterile phosphate buffered saline (1X PBS). Bacterial suspensions were diluted 1:1,000 in cation-adjusted diluted Mueller Hinton media (CAT-dMH; BD Biosciences, San Jose, CA, USA) and 50 µL aliquoted into each well of the Sensititre plate. Inoculated plates were incubated at 20°C and read at 96 h. The minimal inhibitory concentration (MIC) was defined as the lowest concentration for which no bacterial growth was grossly observed.
3 Results
3.1 Bacterial identification
A total of 183 isolates presumptively ascribed to the genera Flavobacterium and Chryseobacterium were collected for analysis in this study. These isolates were collected over the span of 31 years from 19 fish types in 6 western states – California (CA), Colorado (CO), Washington (WA), Oregon (OR), Utah (UT), and Idaho (ID) (Supplementary Tables 3, 4). Of the 183 isolates, 52 were identified as Chryseobacterium and 131 as Flavobacterium by BLASTN of partial 16S rRNA gene sequences. Most isolates (125/183) shared ≥ 99% pairwise nucleotide identity to a type-strain in the sequenced fragment. However, 44 Flavobacterium isolates and 14 Chryseobacterium isolates fell below this threshold, with pairwise identities ranging from 95.8-98.9%. The 16S rRNA gene was highly conserved within the genera. Within Flavobacterium, the 269 type-strains and 131 isolates (n = 400 total) shared average 92.5% nucleotide identity within the sequenced fragment. Similarly, within the Chryseobacterium genus, the 136 type-strains and 52 isolates (n = 188 total) shared a pairwise identity of 94.8%.
3.2 Phylogenetic analyses
3.2.1 Flavobacterium
Phylogenetic analysis by the gyrase B gene placed the 131 Flavobacterium isolates into 9 major clades (A-I) consisting of at least five clinical isolates with high (≥ 70%) bootstrap support (BsS; Hillis and Bull, 1993) as well as several smaller groups of less than 5 isolates, and single lineages with, or without, published strains (Figure 1; https://itol.embl.de/tree/3420946248377721671151594). All clades contained isolates from more than one state. Clade A and F represented established fish pathogens, the columnaris causing bacteria (CCB; LaFrentz et al., 2022) and F. psychrophilum groups, respectively. Clade A (96.2% BsS) included 15 isolates from diverse fish hosts in CA, ID, and WA and F. columnare, F. covae, F. davisii and F. oreochromis. Isolate CA 211 clustered with F. davisii, and CA 59 and CA 123 with F. covae. All other isolates grouped closest to F. columnare. These groupings agreed with the 16S analysis, except for CA 59, an isolate from a chocolate cichlid (H. temporalis) that was most related to the type-strain of F. inkyongense (99.9% PI; Supplementary Table 3). Clade F (100% BsS) contained 16 salmonid isolates from OR and CA with the type-strain of F. psychrophilum from Coho salmon (Oncorhynchus kisutch). Isolates shared 99.2-100% PI with this type-strain in the 16S rRNA gene fragment.
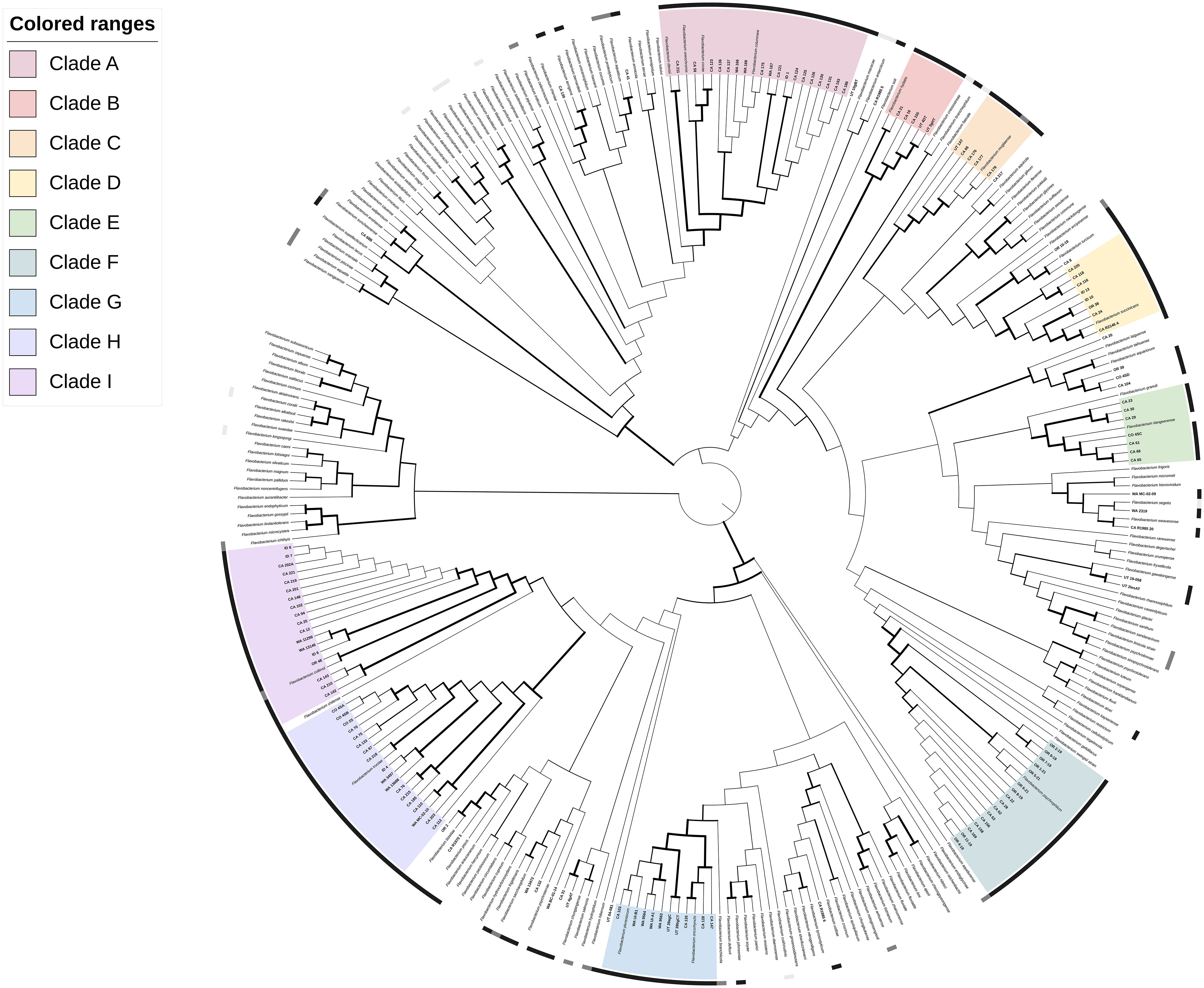
Figure 1 Phylogenetic relationships of 131 Flavobacterium spp. isolates recovered from diseased fishes in the Western US to 178 formally described Flavobacterium spp., based on partial gyrB gene sequences. The dendrogram was generated using the Maximum-Likelihood method and General Time Reversible model with a Gamma distribution allowing for evolutionarily invariable sites (GTR+G+I) and 1000 pseudoreplicates. Branch width is proportional to bootstrap support with increasing thickness from 0-1. Color strips indicate host relationship: black – fish disease associated, dark grey – fish associated, light gray – non-fish animal associated. Range color indicates clade: A, pink; B, red; C, orange; D, yellow; E, light green; F, teal; G, light blue; H, dark blue; I, purple. An interactive version of this tree with annotations, branch lengths and other features can be accessed at https://itol.embl.de/tree/3420946248377721671151594.
Of the atypical fish disease-associated clades, Clade B (97.8% BsS) contained F. hydatis, previously isolated from diseased fish, and five UT and CA salmonid isolates. There were at least two subgroupings within the clade: the F. hydatis type-strain with CA 21 (73.9% BsS), and CA 16 with CA 155, UT 4DT and UT 3gHT (97.8% BsS). Clade C (100% BsS) was a well-defined group of salmonid isolates with high similarity to the F. muglaense type-strain from rainbow trout (O. mykiss). Clade D (100% BsS) contained isolates from salmonids, sturgeon and tilapia in CA, ID, and OR, along with the type-strain of F. succinicans from Chinook salmon (Oncorhynchus tshawytscha). The phylogenetic topography and 16S rRNA analysis of isolates in this clade suggest it consists of two to three species, including F. succinicans. Clade E (100% BsS) contains the type-strain of F. tiangeerense and clinical isolates from CA and CO. Two subgroups are evident: CA 23, CA 29, and CA 30 (95.4% BsS) and F. tiangeerense, CO 4SC, CA 61, CA 65 and CA 68 (99.9% BsS). Clade G (100% BsS) similarly contains two well-supported groups, with an established species in each. Group G1 (100% BsS) contains F. oncorhynchi-like isolates from koi and salmon in CA. Group G2 (100% BsS) contains F. plurextorum-like strains from salmonids in CA, WA and UT. The remaining atypical Flavobacterium clades were large groups of 18 isolates each. Clade H (100% BsS) consisted of isolates from salmonids and Gambusia in CA, CO, ID and WA, with the type-strain of F. tructae from a fish farm in Spain (Kämpfer et al., 2020). The type-strain formed an internal subgrouping with 8 of the 18 isolates (84.4% BsS) but there were additional potential subdivisions between isolates. Clade I (99.9% BsS) contained salmonid and sturgeon isolates from CA, OR and WA with the type-strain of F. collinsii from rainbow trout (O. mykiss) in Spain (Zamora et al., 2013b). Isolates CA 142, CA 143 and CA 210 formed a separate group (I1; 100% BsS) from the rest of the isolates and the type-strain (I2; 99.9% BsS).
Twenty-six isolates did not fall into a defined “major clade.” Isolates CA 104, CO-4SD, CA 39 and CA 20 formed a small group (100% BsS) by gyrB analysis that was not closely related to any reference strain (Figure 1). Isolate CA 41 clustered with F. aquaticum (BsS 100%) and isolates OR 2 and CA R19703 clustered with F. bizetiae from diseased freshwater fish in Canada (BsS 100%). Isolates CA 8 and OR 10-19 grouped with F. erciyesense and F. turcicum (100% BsS), which have been reported from diseased fish (Table 1). CA 139 was most closely related to F. bernardetii from rainbow trout (O. mykiss) and F. terrigena from soil (100% BsS). The remaining isolates were not well-resolved in the phylogeny.
3.2.2 Chryseobacterium
Phylogenetic analysis through the gyrase B gene placed the 52 Chryseobacterium isolates into six major clades of more than five isolates with high (≥ 70%) bootstrap support (A-F), as well as several smaller lineages with, or without, related reference strains (Figure 2; https://itol.embl.de/tree/31386026428981670886889). Clade A (100% BsS) consisted of a single type-strain, C. chaponense, and seven isolates from Oncorhynchus mykiss varieties in CA and WA. Clade B (96.3% BsS) contained two species associated with fish disease, C. aahli and C. piscicola, and nine salmonid isolates from CA, WA, and ID. The C. aahli reference strain and WA NS-910-01 grouped together (91.1% BsS) and the remainder with C. piscicola (100% BsS). Clade C contained three type-strains, but only one from a species associated with fish disease – C. scopthalmum. Isolate ID 2 and WA 9757 grouped with C. schmidteae from a planarian (99.9% BsS) and WA NS-96-11 and CA R2137 loosely with C. scopthalmum and C. multrae (56.5% BsS). Isolate CA 134 branched off from the other strains at an earlier node. Clade D contained eight salmonid isolates from CA and WA and the type-strains from C. piscium and C. balustinum. The CA isolates were more closely related to the type-strains (D1; 97.5% BsS) than the WA strains, which formed their own subgroup (D2; 100% BsS). Clade E had 3 subgroups. Isolates CA R2136, WA UI-6B and WA UI-4G grouped without a type-strain (100% BsS), while CA 146 grouped with C. aurantiacum (88.5% BsS) and WA BC-05-03 with C. oncorhynchi (100% BsS). Clade F (97.1% BsS) contained five isolates and an equal number of type-strains from fish, human and environmental associated species. Within the clade, CA 46, 129 and 127 formed a subgroup with 99.9% bootstrap support. Twelve Chryseobacterium isolates did not fall into a major clade, though most branched closely (100% BsS) to a type-strain: CA 15 with C. panacisoli, CA 43 with C. aquaticum, CA 45 with C. vaccae, CA 196 with C. soli, CA 213 with C. gambrini, CA R1970 11 with C. ureilyticum, CA R2154 D with C. candidae, UT SK1 with C. antibioticum, and ID 3 with C. viscerum, The remainder of isolates were not well-resolved.
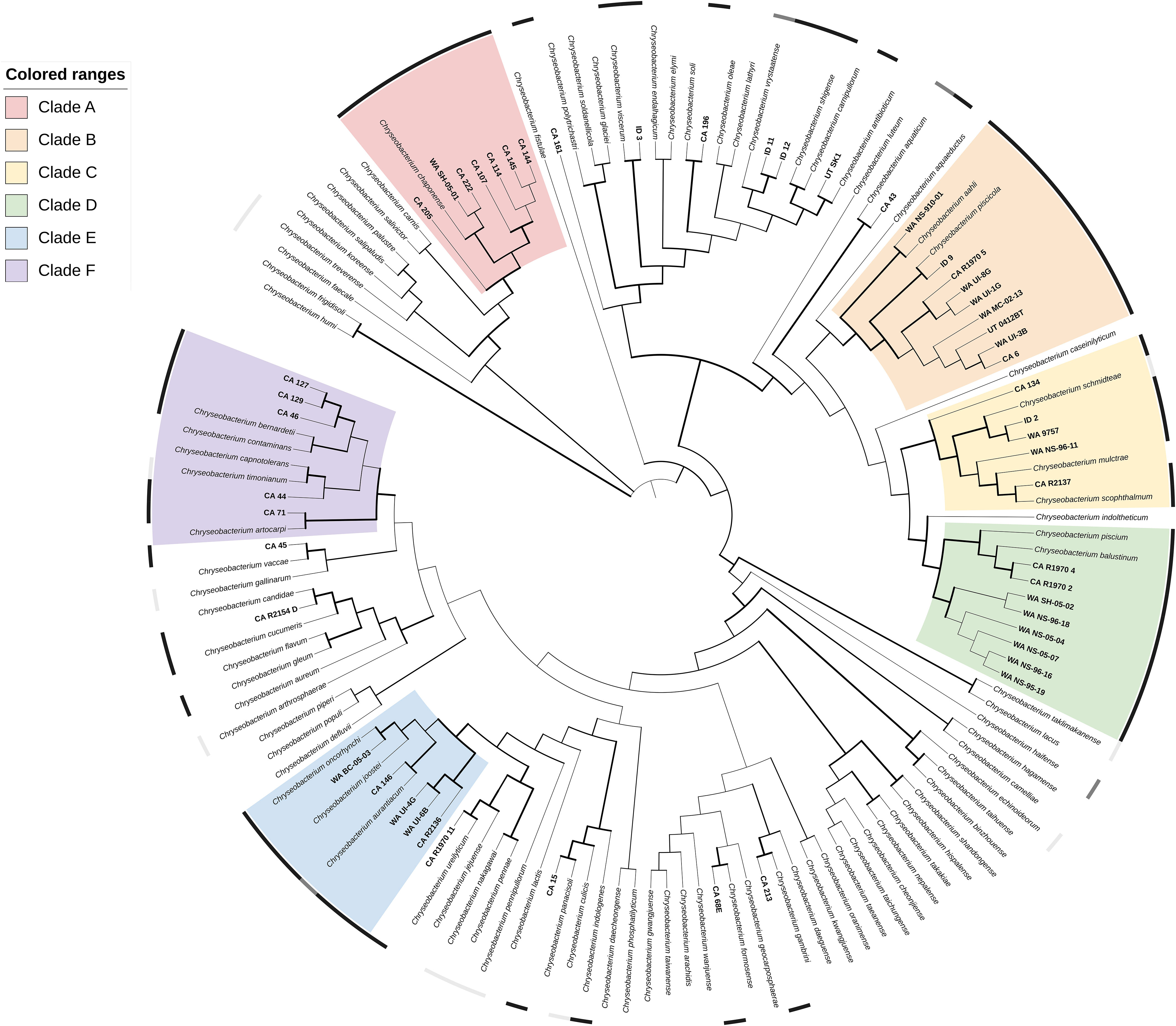
Figure 2 Phylogenetic relationships of 53 Chryseobacterium spp. isolates recovered from diseased fishes in the Western US to 94 formally described Chryseobacterium spp, based on partial gyrB gene sequences. The dendrogram was generated using the Maximum-Likelihood method and General Time Reversible model with a Gamma distribution allowing for evolutionarily invariable sites (GTR+G+I) and 1000 pseudoreplicates. Branch width is proportional to bootstrap support with increasing thickness from 0-1. Color strips indicate host relationship: black – fish disease associated, dark grey – fish associated, light gray – non-fish animal associated. Range color indicates clade: A, red; B, orange; C, yellow; D, green; E, blue; F, purple. An interactive version of this tree can be accessed at https://itol.embl.de/tree/31386026428981670886889.
3.3 Phenotypic characterization
All tested isolates were gram-negative, catalase and oxidase positive rods. The representative Chryseobacterium isolates were all small, short and rounded. Flavobacterium isolates displayed higher morphological diversity, ranging from the long thin rods characteristic of F. columnare (LaFrentz et al., 2022) to shorter rounded rods, as observed with the Clade B isolate UT 3gHT (Supplementary Figures 1, 2). While all submitted isolates demonstrated yellow pigmentation, there were observed differences in hue. Flavobacterium exhibited either bright yellow (Clade A), gold (B, C, I), or translucent gold colonies (D-H) colonies on TYES. Chryseobacterium isolates displayed pale yellow (A), green-yellow (B) or gold (C-F) colonies (Table 4 and Supplementary Figures 1, 2), although some isolates outside the major clades had orange and yellow-gray pigmentation. After 72 h of incubation at 20°C, Chryseobacterium formed smooth and rounded colonies of 0.25-1.25mm in diameter while Flavobacterium isolates either showed similar small, round morphologies or displayed spreading rhizoid colonies reaching ~4-7mm. All representative Chryseobacterium isolates tested grew on blood agar (BA), with α- or γ- hemolysis. The F. columnare and F. psychrophilum isolates tested, ID 1 and OR 1-21 respectively, did not grow on BA, nor did Clade E isolate CO 4SC. The remainder of tested isolates from the major clades were capable of growing on BA and were either α- or γ-hemolytic.
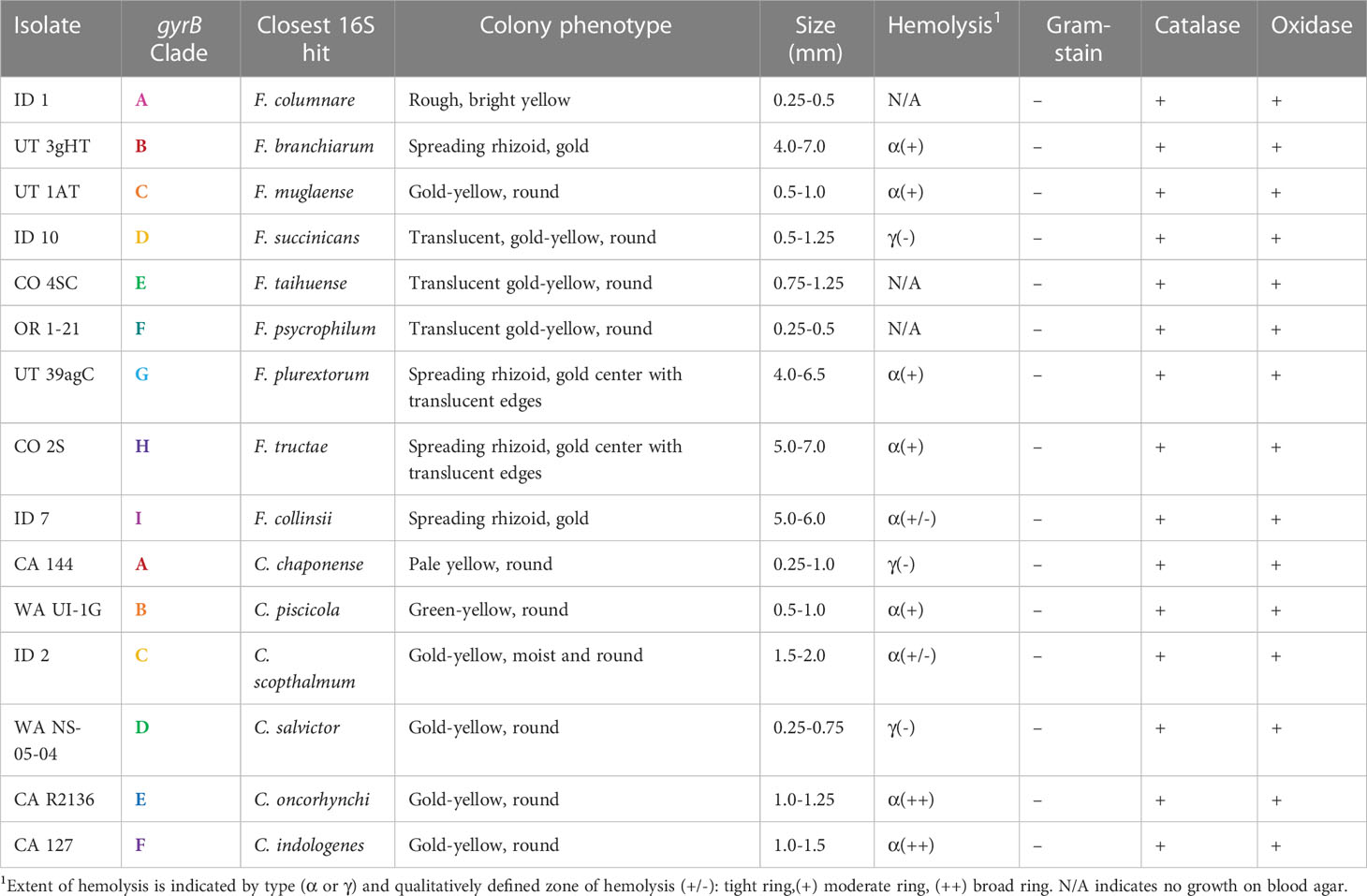
Table 4 Morphological characteristics of representative Flavobacterium and Chryseobacterium isolates from each major gyrB phylogenetic clade (Figures 1, 2).
3.4 Antimicrobial susceptibility profiling
There were trends in MIC profiles related to antimicrobial type and clade (Table 5). All clades in both the atypical Flavobacterium and Chryseobacterium genera had at least one tested isolate (total 33/39) with an MIC above the established F. psychrophilum breakpoint for oxytetracycline (CLSI, 2020). The MICs for florfenicol were also above the F. psychrophilum breakpoint for just over half of the tested isolates in each genus (24/39). A majority of isolates exhibited growth in the highest tested concentrations of cetifour (32/39), erythromycin (27/39), sulphadimethoxine (26/39) and penicillin (24/39) and MICs at the upper ranges of amoxicillin and spectinomycin. The MICs were generally, but not unanimously, lower for streptomycin. Flavobacterium isolates exhibited higher tolerance for trimethoprim/sulfamethoxazole, while Chryseobacterium isolates grew in higher concentrations of clindamycin. Within genera, Flavobacterium Clades B and G-I displayed overall comparably high MICs for florfenicol, trimethoprim/sulfamethoxazole, enrofloxacin, gentamicin, neomycin, erythromycin, amoxicillin, spectinomycin, sulphadimethoxine, sulphathiazole, and tylosin tartrate. Chryseobacterium clades E and F showed higher relative MICs for florfenicol, enrofloxacin, gentamicin, neomycin, amoxicillin, spectinomycin, sulphathiazole, streptomycin, novobiocin, tylosin tartrate and clindamycin. Chryseobacterium had overall a greater number of isolates with MICs exceeding testing concentration ranges.
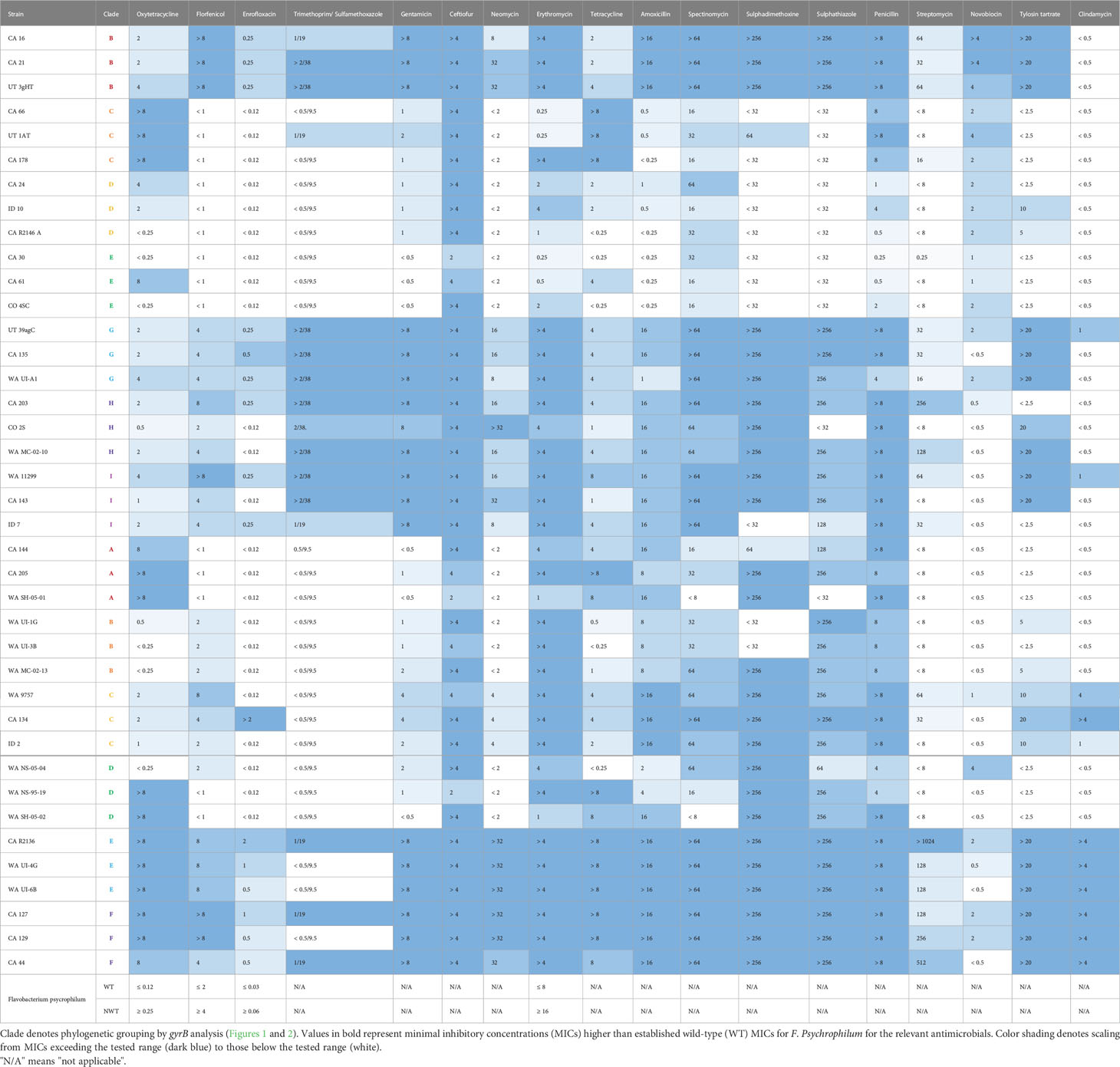
Table 5 Antimicrobial susceptibility profiles of representative atypical Flavobacterium (CA 16-ID 7) and Chryseobacterium (CA 144-CA 44) strains.
4 Discussion
Flavobacteria are widely recognized as important members of environmental and piscine microbial communities, but the nuances to their taxonomic placements and their contribution to disease remain enigmatic. With ongoing refinement in the methods available for bacterial characterization, substantial readjustments to the structure of Flavobacteriales have occurred at every scale. Following the formal description and delineation of the genus Chryseobacterium from Flavobacterium in the early 2000s, the taxonomy of the genus has been further revised and moved from Flavobacteriaceae to a new family, Weeksellaceae (Bernardet et al., 2006; García-López et al., 2019). Within both genera, new species are frequently described, and existing species either subsumed into synonymous species or split. Even the most well-studied flavobacteria are subject to changes under new scrutiny, with the recent division of Flavobacterium columnare genetic groups into four distinct species (LaFrentz et al., 2022). This constant upheaval requires constant vigilance to remain aligned with current taxonomic structures, complicating identification of new isolates by comparison to historical strains. In addition to discrepancies in strain names in databases and the literature, conclusive classification of flavobacteria remains difficult by standard diagnostic methods (Loch and Faisal, 2015a).
The most common method for bacterial molecular identification is sequencing of the 16S rRNA gene, with the locus having been successfully used in conjunction with other markers for the delineation of many novel flavobacterial taxa (Bernardet et al., 2002). However, use of this locus in flavobacterial typing is also accompanied by some pitfalls. The genomic 16S rRNA gene copy number ranges from 1-13 in flavobacterial species (Stoddard et al., 2015), and there is often high interspecies sequence similarity (Loch and Faisal, 2015a). Conventionally, the cut-off indicating speciation in the 16S rRNA gene is 97% pairwise identity between an isolate and the most closely related reference species (Edgar, 2018). However, a higher threshold of 98.6-99% has been proposed to improve discrimination of bacterial species with high genetic homogeneity in the gene, such as with Flavobacterium and Chryseobacterium (Stackebrandt and Ebers, 2006; Edgar, 2018). Even using this elevated threshold, there are abundant examples of isolates with high 16S rRNA gene similarity to a type species that may group disparately phylogenetically, or be assigned as a novel or separate species by subsequent polyphasic analysis (Zamora et al., 2012a; Loch et al., 2013; Loch and Faisal, 2015b; Loch and Faisal, 2016a; Verner-Jeffreys et al., 2017; Loch and Faisal, 2018). The gene for DNA gyrase subunit B, gyrB, has been suggested as an alternative or supplementary housekeeping gene for bacterial genotyping (Wang et al., 2007; Galloway-Peña et al., 2014; Reichley et al., 2017). As a single copy gene with a faster evolutionary rate than the 16S rRNA gene, gyrB often offers improved and more accurate discriminatory power. Reflecting this, there was high homogeneity in the 16S rRNA gene fragment between type-strains and isolates in both genera, with an average pairwise identity of 92.5% across 400 strains in Flavobacterium and 94.8% across 189 Chryseobacterium strains. While a comparable analysis cannot be run for the gyrB gene, as many type-strains do not have published gyrB sequences available, running alignments using the subset of type-strains with whole genomes available shows the average PI for the 16S rRNA gene remains similar, with an average of 93.4% pairwise identity across 309 Flavobacterium strains and 94.9% across 144 Chryseobacterium strains. Comparison of the gyrB fragment among those same strains reveals higher inter-strain heterogeneity, with 79.1% PI across the Flavobacterium strains and 80.6% across Chryseobacterium. The maximum likelihood trees generated by gyrB analysis subsequently offered higher resolution and more defined clades with higher bootstrap support than those generated by equivalent methods using the 16S rRNA gene (Figures 1, 2 and Supplementary Figures 3, 4).
We found a diverse assemblage of flavobacteria associated with aquaculture and fish disease in the Western United States. The 131 Flavobacterium spp. and 53 Chryseobacterium spp. were recovered from wild fish, ornamental fish, hatchery raised, or commercially produced species. Salmonids, in particular rainbow trout (O. mykiss) varieties, were most represented, consistent with regional aquaculture activities and historical epidemiological trends for flavobacterial disease (Wahli and Madsen, 2018; United States Department of Agriculture, 2019). Most isolates (125/183) shared high (≥ 99%) similarity to one or more reference species previously linked to fish or fish disease (Supplementary Table 1) either in the Great Lakes region of the United States, or in South America, Europe, Asia, or Africa (Loch and Faisal, 2015a). Though conclusive confirmation of isolate identities by whole genome analysis is warranted, the results of this study expand the geographic and host ranges of established species, the majority of which have never been reported in the Western United States.
Phylogenetic analysis using the gyrB gene determined the presence of multiple genetic clusters in both genera associated with fish disease (Figures 1, 2). The majority of isolates (151/184) collected from clinically affected fish in the Western United States fell into one of 15 major clades within these larger clusters. Genetic groups were not clearly based on geographic origin, and isolates from the same state were phylogenetically diverse. Flavobacterium isolates formed nine clades (A-I), including isolates closely related to the well-known flavobacterial pathogens – F. psychrophilum and the CCB. No isolates were identified as F. branchiophilum. Clade F (F. psychrophilum-like) isolates were largely homogenous and were all isolated from salmonid species, consistent with previous reports. The CCB group had diverse representation by different fish hosts, with 10 different fish types from 6 different genera. The CCB species have shown some patterns in host specificity in previous studies (LaFrentz et al., 2021). Flavobacterium oreochromis, associated with tilapia, has not been reported in the US and was not identified in this study. The cichlid isolate in the CCB group (CA 59) clustered with F. covae by gyrB analysis, a species previously tied to catfish disease, but shared 99.9% PI with related species F. inkyongense in the 16S rRNA gene fragment. Flavobacterium inkyongense did not have a reference genome available and was therefore not included in phylogenetic analysis. Isolate CA 123 from zebrafish (D. reino) also grouped with F. covae and shared high (99.9%) 16S rRNA gene similarity with the type-strain. Flavobacterium davisii has been recovered from columnaris cases in rainbow and steelhead trout in the US (Sebastião et al., 2019a), and similarly was most closely related to rainbow trout (O. mykiss) isolate CA 211 by both 16S and gyrB analyses. Flavobacterium columnare is also associated with salmonids, and the phylogenetic subgroup in this study included mostly salmonid strains, alongside two koi (C. rubrofuscus) isolates.
The atypical clusters B-E and G-I contained species of growing interest to aquaculture and fish health, along with potentially novel agents of fish disease. For example, Clade B, contained the type-strain of F. hydatis, which has been historically associated with fish disease (Strohl and Tait, 1978; Bernardet and Bowman, 2006; Mantareva et al., 2022). However, the five clinical isolates in the group did not share high 16S rRNA gene sequence similarity to F. hydatis nor any other type-strain (Supplementary Table 3). It is possible they represent up to three new species. In previous studies in other regions of the US, isolates most closely related to F. hydatis, but similarly falling below the 99% 16S rRNA gene pairwise identity threshold, have been recovered (Loch et al., 2013; Loch and Faisal, 2014a). It would be interesting to compare these isolates more closely. Clade E was also made up of isolates all below the 99% 16S rRNA gene threshold, with the type-strain F. tiangeerense (Xin et al., 2009). Similarly, to F. hydatis, other studies in the US have recovered fish isolates moderately similar to this species (Loch et al., 2013; Loch and Faisal, 2014a; Loch and Faisal, 2018), suggesting additional yet-undefined agents associated with flavobacterial disease.
Phylogenetic subgroupings observed in the other clades suggest that additional novel species or subspecies are likely. Clade D contains F. succinicans, previously implicated in fish disease (Anderson and Ordal, 1961; Good et al., 2015; Adamek et al., 2018; Loch and Faisal, 2018; Einarsdottir et al., 2020), but isolates ranged in pairwise 16S rRNA gene similarity from 98.2-99.9%. The type-strain in Clade H, F. tructae, is one of the few atypical Flavobacterium with demonstrated pathogenicity in fish (Zamora et al., 2013a; Loch and Faisal, 2016b). Flavobacterium tructae has only been reported in salmonids, but Clade H also included an isolate from Gambusia with high 16S rRNA gene similarity (99.6% PI). Six out of 18 isolates in Clade H, however, showed low pairwise identity to F. tructae, and higher similarities to F. salmonis (98.4-99.1%) or F. chungangense (98%). Three isolates from Clade I also shared more sequence identity (98.8% PI) with F. salmonis in the 16S rRNA gene. The remainder of Clade I isolates were most related (99.7-100% PI) to F. collinsii, which has not been clearly linked to fish disease nor identified in the US. The type-strain of F. collinsii was recovered from healthy rainbow trout (O. mykiss) (Zamora et al., 2013b), and a recently released pre-print recovered the species from a fish that died without clinical signs or apparent lesions (Lee et al., 2022). Attempts to fulfill Koch’s postulates were unsuccessful, although the bacteria was recovered from internal tissues of sampled, apparently healthy survivors. Nevertheless, the frequency of F. collinsii isolations from diseased fishes in this study warrants further comparisons of strains under different challenge models.
The most well-defined clades outside of Clade A (CCB) and Clade F (F. psychrophilum) were Clades C and G. Clade C contained a type-strain from a species associated only with healthy rainbow trout outside of the US – F. muglaense (Duman et al., 2021). Salmonid isolates from CA and UT shared 99-100% 16S rRNA gene similarity to the type-strain, and the phylogenetic tree topography indicates high similarity within the gyrB gene as well. Recently, an isolate sharing 100% sequence identity to F. muglaense across an ~ 1,100 bp stretch of the 16s rRNA gene was also recovered from the kidneys of diseased hatchery-raised rainbow trout (O. mykiss) in Michigan (Loch, unpublished), indicating it is a species of interest for aquaculture. Clade G included two subgroups defined by closely related species – F. oncorhynchi and F. plurextorum. Both species have been reported in association with salmonids and other species, in diseased or healthy farmed and wild fish (Zamora et al., 2012a; Loch et al., 2013; Zamora et al., 2013a; Loch and Faisal, 2014a; Loch and Faisal, 2018). Flavobacterium oncorhynchi has also been implicated in a fatal human fetal infection (Ferreira et al., 2022). Virulence in fish, however, has not been formally investigated for either species. Although these species share high genetic similarity, they have been confirmed as distinct, and 16S results also support delineation of the clade.
In addition to the major clades, there were single isolates and minor clades of interest. Isolate CA 139 was closely related to F. bernardetii, a recently described species from diseased rainbow trout (O. mykiss) that has not been reported in the United States (Saticioglu et al., 2021b). Isolate OR 10-19 and CA 8 grouped with F. erciyesense and F. turcicum, previously associated with fish disease in Turkey (Saticioglu, 2021; Saticioglu et al., 2021a), while CA 44 grouped with F. aquaticum, which has only been reported in healthy catla (Catla catla) in India (Verma and Rathore, 2015). Isolates CA R1970 3 and CA 2 branched with F. bizetiae, F. araucananum and F. piscis, which have all been linked to fish disease (Table 1). Two isolates also grouped weakly with the strains isolated from penguin feces or habitats, possibly indicating a dietary transmission from fish (Figure 1).
Chryseobacterium clades were somewhat less resolved compared to Flavobacterium, likely due to undersampling (Figure 2). In particular, Clade F was a group of five isolates and an equal number of type-strains. The C. bernardetii and C. timonianum type-strains are human clinical isolates (Supplementary Table 2), but C. bernardetii has also been recovered from diseased rainbow trout (O. mykiss; Saticioglu et al., 2021b) indicating a potential avenue for zoonotic transmission. Chryseobacterium spp. can be opportunistic human pathogens in invasive disease. Chyrseobacterium indologenes for example, has a wide host range that includes both fish and humans, though zoonotic transmission has not been reported (Hugo et al., 2019). Interestingly, while two Clade F isolates shared the highest 16S rRNA gene sequence similarity with C. indologenes, no isolates grouped closely with it in the gyrB tree. The 16S rRNA and whole genome (gyrB) gene sequences used in analysis were both from the C. indologenes type-strain, so this difference may be due to low resolution in 16S rRNA gene, or perhaps differences in published sequence assemblies.
All remaining Chryseobacterium clades (A-E) also had ties to fish disease. Clade A was a well-defined group of isolates collected from O. mykiss varieties and the type-strain of C. chaponense, also recovered from diseased rainbow trout (O. mykiss) (Kämpfer et al., 2011). A strain with high similarity to C. chaponense (> 99.6%) has been assessed for virulence in salmonids, and while it did not cause any mortalities, it did induce lesions, including melanosis, swollen congested liver, swollen enlarged spleen and mottled kidney (Loch and Faisal, 2015b). Clade B contained salmonid isolates and two species with confirmed piscine pathogenicity – C. aahli and C. piscicola. Isolate WA-NS-910-01 grouped closely with C. aahli by gyrB analysis but was not highly similar to any of the type-strains by 16S analysis (98.4% PI to C. aquaticum). The remaining isolates, however, shared high sequence similarity with the C. piscicola type-strain (99.6-100%). This species has been reported in outbreaks of disease in salmonids in Chile and recovered in the Great Lakes region (Ilardi et al., 2009; Ilardi et al., 2010; Loch et al., 2013; Loch and Faisal, 2015b). Clade C consisted of koi and salmonid isolates, with type-strains related to animals and animal products. Chryseobacterium schmidteae was collected from a planarian, and C. mulctrae from raw cow milk. Chryseobacterium scophthalmum is an established fish pathogen with demonstrated pathogenicity in more than one fish host (Mudarris and Austin, 1989; Mudarris et al., 1994; Shahi et al., 2018). Interestingly, while CA R2137 clustered closest to this species by gyrB analysis, ID 2 showed higher similarity by 16S rRNA gene alignment. The type-strains in Clade D - C. balustinum and C. piscium – represent species with experimental evidence suggesting pathogenic potential (Table 2). Chinook salmon (O. tshawytscha) isolates from California, CA R1970 2 and CA R1970 4 grouped closely to the type strains and were most related to C. piscium by 16S analysis (98.9% PI), but the WA isolates from rainbow trout (O. mykiss) formed a distinct subgroup. Finally, Clade E contained three piscine pathogenic species – C. oncorhynchi, C. joostei, and C. aurantiacum – but only the relationships to C. oncorhynchi and C. joostei were supported by the 16S results. Previously, C. oncorhynchi has been reported in rainbow trout (O. mykiss) and Siberian sturgeon (Acipenser baerii) (Zamora et al., 2012a; SuXu et al., 2019), but the clade included CA R2136 from tilapia (Oreochromis spp.) and CA 146 from koi (C. rubrofuscus). Outside of the major clades, several isolates still branched closely with fish associated species, such as C. vrystaatense, which has been recovered previously from healthy wild fish in the Great Lakes (Loch et al., 2013) and C. aquaticum from diseased fish cases (Bernardet et al., 2005; Loveland-Curtze et al., 2010; Hugo et al., 2019; Sebastião et al., 2019b; Saticioglu et al., 2021c)
The tangled taxonomy of Flavobacteriales and the lack of consistently effective challenge models have limited our understanding of the risk these atypical species pose to aquaculture. As such, the full characterization of species of interest and their virulence to fish hosts remain largely unexplored and are the subject of ongoing investigations. Preliminary results demonstrate notable variations in colony and cell morphology between study isolates, as well as in hemolytic profiles (Table 4 and Supplementary Figures 1, 2), suggesting that the observed genetic diversity underlies important phenotypic and virulence differences. Additionally, there were 58 isolates below the 99% 16S rRNA gene sequence similarity threshold to any available type-strain, and a number of isolates that formed phylogenetic subgroupings distinct from their closest type species. These potentially represent new species or subspecies in the genera. Different strains within highly represented clades like F. collinsii, F. tructae or C. chaponense should also be assessed for any strain-specific trends in virulence using biologically relevant challenge models.
The high association of these flavobacterial groups with recent and historical outbreaks of disease remain suggestive of their pathogenic potential. It is likely that some of these atypical piscine flavobacteria represent opportunistic pathogens, but this does not diminish their potential importance to aquaculture. High intensity rearing systems favor conditions for opportunistic infections, and flavobacterial diseases are notoriously difficult to treat and control (Verner-Jeffreys et al., 2017; Kumru et al., 2020; Saticioglu et al., 2021c). Both Chryseobacterium and Flavobacterium display a propensity for resistance across antimicrobial classes. Though there is some evidence for horizontal transfer of resistance genes from other gram-negative bacteria, this elevated level of resistance is thought to be primarily intrinsic rather than anthropogenically driven (Verner-Jeffreys et al., 2017). Mechanisms may include non-specific efflux pumps, chromosomally linked extended-spectrum beta-lactamases, and alterations in DNA gyrase and topoisomerase genes (Michel et al., 2005; Chen et al., 2017; Verner-Jeffreys et al., 2017; Kumru et al., 2020; Saticioglu et al., 2021b; Saticioglu et al., 2021c). The representative isolates tested from each major clade reflected established trends, with generally high MICs across multiple antimicrobial types. Clades F and G in Chryseobacterium and B and G-I in Flavobacterium showed comparably higher MICs for at least 11/18 antimicrobials. Flavobacterium hydatis from clade B, F. plurextorum from clade G, and F. chilense, which branched closely with Clades H and I, were also reported in a previous study to have the highest number of antimicrobial resistance (AMR) genes out of 86 different Flavobacterium genomes investigated (Kumru et al., 2020). Clinical CLSI breakpoints are not established for any of the atypical flavobacteria, but at least one isolate in every clade demonstrated an MIC at or above the concentration required for resistance in F. psychrophilum for oxytetracycline and florfenicol, two of the three antimicrobials approved for use in aquaculture in the United States. Isolates from Flavobacterium Clade B/G-I also exceeded the testing range for trimethoprim/sulfamethoxazole, a potentiated sulfonamide, while almost all Chryseobacterium tested were inhibited by this antimicrobial at the lowest concentration. The elevated susceptibility of Chryseobacterium to trimethoprim/sulfamethoxazole is consistent with the literature but is not universal. A survey of 70 C. aquaticum isolates from diseased rainbow trout in Turkey found the average MIC for trimethoprim/sulfamethoxazole to be 2/38, the highest concentration tested in our study format (Saticioglu et al., 2021c).
High MICs indicate that treatment of emerging flavobacterial pathogens with approved antimicrobials may be unsuccessful. Preventing outbreaks is also difficult, as some Flavobacterium and Chryseobacterium spp. are ubiquitous in the environment and disinfection of fish eggs or tanks with common antimicrobials can be insufficient to eradicate the bacteria. Many flavobacteria form biofilms, and there is evidence that iodophor disinfection of salmon eggs may not eliminate some of the bacterial species identified in this study (Loch and Faisal, 2018). Successful vaccination against flavobacterial disease is another challenge complicated by the high diversity of piscine pathogenic species and the propensity for mixed species outbreaks. Recent studies with a live-attenuated vaccine against F. psychrophilum have shown some promising preliminary results for cross-protection and conserved antigens between F. psychrophilum and atypical Flavobacterium and Chryseobacterium isolates (Bruce et al., 2020), but there remains much work to be completed to reach targets for widespread protection.
Assembling the depth and breadth of information required to effectively control this complex group of diseases is a tremendous undertaking. The results of this study add another piece to our understanding of the flavobacteria contributing to fish disease, in an understudied, but crucial region for aquaculture. Isolates similar to fish pathogenic species identified in the Great Lakes region of the US and in South America, Europe, Africa and Asia were recovered in the Western United States, expanding the geographic, and in some cases, host range, of these atypical agents of flavobacterial disease. Continuing investigations stemming from this study, addressing isolate pathogenesis, ecology, and genetic and antigenic diversity, will create a more comprehensive picture of piscine flavobacterial disease in the United States, with improved recommendations for treatment and control.
Data availability statement
Partial 16S rRNA and gyrase B gene sequences generated as part of this study were uploaded to GenBank, Accession numbers OQ546252-OQ546434 and OQ561006-OQ561188. All phylogenetic trees generated in this project can be accessed at https://itol.embl.de/shared/tiheckman under project “Atypical Flavobacteria in Aquaculture.
Author contributions
KC, TH, TL, ES, FS, BV and ZY contributed to the conceptualization and design of the study. All authors contributed to the acquisition and characterization of bacterial isolates. TH, KM, EP, FS and ZY contributed to the sequencing of bacterial isolates. ZY and ES preformed MIC analyses. TH preformed genetic, phylogenetic, and phenotypic analyses and wrote the first draft of the manuscript. All authors contributed to the article and approved the submitted version.
Funding
This work was funded by the Western Regional Aquaculture Center grant number UWSC12733.
Acknowledgments
We would like to thank the many individuals and organizations who contributed yellow pigmented bacterial isolates over the years, including Tresa Veek, Mark Adkison, Kevin Kwak, and Christine Richey from the California Department of Fish and Wildlife (CDFW); Aimee Reed, Amelia Thornhill and Gordon Gibbs from the Oregon Department of Fish and Wildlife (ODFW), Richard Holt from Oregon State University (OSU); Danielle Van Vliet, Christine Swan, and Wade Cavender from the Utah Division of Wildlife (UDW); Joe Rockefeller and Jim Thompson from the Washington Aquatic Disease Diagnostic Laboratory (WADDL); Victoria Vincent from the Colorado Division of Natural Resources (CDNR); Renee Yamamoto from the Montana Fish and Wildlife Services (MFWS); and Stephen Reichley from Clear Springs Foods and Mississippi State University (MSU).
Conflict of interest
The authors declare that the research was conducted in the absence of any commercial or financial relationships that could be construed as a potential conflict of interest.
Publisher’s note
All claims expressed in this article are solely those of the authors and do not necessarily represent those of their affiliated organizations, or those of the publisher, the editors and the reviewers. Any product that may be evaluated in this article, or claim that may be made by its manufacturer, is not guaranteed or endorsed by the publisher.
Supplementary material
The Supplementary Material for this article can be found online at: https://www.frontiersin.org/articles/10.3389/fcimb.2023.1149032/full#supplementary-material
References
Adamek, M., Teitge, F., Jung-Schroers, V., Heling, M., Gela, D., Piackova, V., et al. (2018). Flavobacteria as secondary pathogens in carp suffering from koi sleepy disease. J. Fish Dis. 41, 1631–1642. doi: 10.1111/jfd.12872
Altschul, S. F., Gish, W., Miller, W., Myers, E. W., Lipman, D. J. (1990). Basic local alignment search tool. J. Mol. Biol. 215, 403–410. doi: 10.1016/S0022-2836(05)80360-2
Anderson, R. L., Ordal, E. J. (1961). Cytophaga succinicans sp. n., a facultatively anaerobic, aquatic myxobacterium. J. Bacteriology 81, 130–138. doi: 10.1128/jb.81.1.130-138.1961
Austin, B., Austin, D. A. (2016). “Flavobacteria and cytophagas,” in Bacterial fish pathogens: Disease of farmed and wild fish. Eds. Austin, B., Austin, D. A. (Cham: Springer International Publishing), 397–465. doi: 10.1007/978-3-319-32674-0_7
Avendaño-Herrera, R., Irgang, R., Poblete-Morales, M., Vásquez-Martínez, Y., Robles, C., Toro-Ascuy, D., et al. (2016). Predominant bacteria associated with black rockcod (Notothenia coriiceps, Richardson 1844) from king George island, Antarctica. Bull. Eur. Ass. Fish Pathol. 36 (6), 246–255.
Ben-Dov, E., Shapiro, O. H., Siboni, N., Kushmaro, A. (2006). Advantage of using inosine at the 3′ termini of 16S rRNA gene universal primers for the study of microbial diversity. Appl. Environ. Microbiol. 72, 6902–6906. doi: 10.1128/AEM.00849-06
Bernardet, J.-F. (2015). “Flavobacteriales ord. nov,” in Bergey’s manual of systematics of archaea and bacteria. Eds. Trujillo, M. E., Dedysh, S., DeVos, P., Hedlund, B., Kämpfer, P., Rainey, F. A. (New York, NY: Springer), 1–2. doi: 10.1002/9781118960608.obm00033
Bernardet, J.-F., Bowman, J. P. (2006). “The genus flavobacterium,” in The prokaryotes: Volume 7: Proteobacteria: Delta, epsilon subclass. Eds. Dworkin, M., Falkow, S., Rosenberg, E., Schleifer, K.-H., Stackebrandt, E. (New York, NY: Springer), 481–531. doi: 10.1007/0-387-30747-8_17
Bernardet, J.-F., Hugo, C., Bruun, B. (2006). “The genera chryseobacterium and elizabethkingia,” in The prokaryotes: Volume 7: Proteobacteria: Delta, epsilon subclass. Eds. Dworkin, M., Falkow, S., Rosenberg, E., Schleifer, K.-H., Stackebrandt, E. (New York, NY: Springer), 638–676. doi: 10.1007/0-387-30747-8_25
Bernardet, J. F., Nakagawa, Y., Holmes, B., Subcommittee On The Taxonomy Of Flavobacterium And Cytophaga-Like Bacteria Of The International Committee On Systematics Of Prokaryotes (2002). Proposed minimal standards for describing new taxa of the family flavobacteriaceae and emended description of the family. Int. J. Syst. Evol. Microbiol. 52 (Pt 3), 1049–1070. doi: 10.1099/00207713-52-3-1049
Bernardet, J.-F., Vancanneyt, M., Matte-Tailliez, O., Grisez, L., Tailliez, P., Bizet, C., et al. (2005). Polyphasic study of Chryseobacterium strains isolated from diseased aquatic animals. Systematic Appl. Microbiol. 28, 640–660. doi: 10.1016/j.syapm.2005.03.016
Brisou, J. (1959). Etude de trois souches microbiennes de la famille des Pseudomonadaceae dont la synergie provoque une maladie de caractere septicemique chez les poissons blancs de la dordogne, du lot et de leurs affluents. Ann. Inst. Pasteur 96, 689–696.
Bruce, T. J., Ma, J., Knupp, C., Loch, T. P., Faisal, M., Cain, K. D. (2020). Cross-protection of a live-attenuated Flavobacterium psychrophilum immersion vaccine against novel Flavobacterium spp. and Chryseobacterium spp. strains. J. Fish Dis. 43, 915–928. doi: 10.1111/jfd.13201
Burešová, V., Franta, Z., Kopáček, P. (2006). A comparison of Chryseobacterium indologenes pathogenicity to the soft tick Ornithodoros moubata and hard tick Ixodes ricinus. J. Invertebrate Pathol. 93, 96–104. doi: 10.1016/j.jip.2006.05.006
Campbell, S., Harada, R. M., Li, Q. X. (2008). Chryseobacterium arothri sp. nov., isolated from the kidneys of a pufferfish. Int. J. Systematic Evolutionary Microbiol. 58, 290–293. doi: 10.1099/ijs.0.65276-0
Carson, J., Schmidtke, L. M., Munday, B. L. (1993). Cytophaga johnsonae: A putative skin pathogen of juvenile farmed barramundi, Lates calcarifer Bloch. J. Fish Dis. 16, 209–218. doi: 10.1111/j.1365-2761.1993.tb01250.x
Chalupnicki, M., Dittman, D., Starliper, C. E., Iwanowicz, D. D. (2015). Efficacy of iodine for disinfection of lake sturgeon eggs from the st. Lawrence river, new York. North Am. J. Aquaculture 77, 82–89. doi: 10.1080/15222055.2014.963768
Chen, S., Blom, J., Loch, T. P., Faisal, M., Walker, E. D. (2017). The emerging fish pathogen Flavobacterium spartansii isolated from Chinook salmon: Comparative genome analysis and molecular manipulation. Front. Microbiol. 8. doi: 10.3389/fmicb.2017.02339
Chen, W.-M., Huang, W.-C., Young, C.-C., Sheu, S.-Y. (2013). Flavobacterium tilapiae sp. nov., isolated from a freshwater pond, and emended descriptions of Flavobacterium defluvii and Flavobacterium johnsoniae. Int. J. Systematic Evolutionary Microbiol. 63, 827–834. doi: 10.1099/ijs.0.041178-0
Chen, W.-M., Yang, C.-C., Sheu, C., Kwon, S.-W., Sheu, S.-Y. (2020). Flavobacterium ichthyis sp. nov., isolated from a fish pond. Int. J. Systematic Evolutionary Microbiol. 70, 5075–5086. doi: 10.1099/ijsem.0.004384
Chong, S. M., Douay, G., Heng, Y., Chng, Y. R., Tay, Y. H., Wong, W. K. (2022). Fatal Chryseobacterium indologenes infection in a captive red-shanked douc langur (Pygathrix nemaeus). J. Med. Primatology 51, 256–258. doi: 10.1111/jmp.12582
Christensen, P. J. (1977). The history, biology, and taxonomy of the Cytophaga group. Can. J. Microbiol. 23, 1599–1653. doi: 10.1139/m77-236
LSI (2020). Performance Standards for Antimicrobial Susceptibility Testing of bacteria isolated from aquatic animals. 3rd ed. CLSI supplement VET04 (Wayne, PA: Clinical and Laboratory Standards Institute).
de Beer, H., Hugo, C. J., Jooste, P. J., Vancanneyt, M., Coenye, T., Vandamme, P. (2006). Chryseobacterium piscium sp. nov., isolated from fish of the south Atlantic ocean off south Africa. Int. J. Systematic Evolutionary Microbiol. 56, 1317–1322. doi: 10.1099/ijs.0.64014-0
Duman, M., Ay, H., Altun, S., Sahin, N., Saticioglu, I. B. (2021). Flavobacterium muglaense sp. nov. isolated from internal organs of apparently healthy rainbow trout. Int. J. Syst. Evol. Microbiol. 71, e004903. doi: 10.1099/ijsem.0.004903
Edgar, R. C. (2018). Updating the 97% identity threshold for 16S ribosomal RNA OTUs. Bioinformatics 34, 2371–2375. doi: 10.1093/bioinformatics/bty113
Einarsdottir, T., Guttormsdottir, G., Connaghan, D., Hjartardottir, S. (2020). Longitudinal survey of Flavobacterium species in icelandic salmonid fish farms. Dis. Aquat. Organisms 141, 15–24. doi: 10.3354/dao03508
Farmer, B. (2004). Improved methods for the isolation and characterization of flavobacterium columnare (Baton Rouge (LA: Louisiana State University).
Felske, A., Rheims, H., Wolterink, A., Stackebrandt, E., Akkermans, A. D. L. (1997). Ribosome analysis reveals prominent activity of an uncultured member of the class actinobacteria in grassland soils. Microbiol. (Reading) 143 (Pt 9), 2983–2989. doi: 10.1099/00221287-143-9-2983
Ferreira, E. O., Schutt, V., Stein, M., Burdz, T., Bernard, K., Stefanovici, C. (2022). First case of intrauterine fetal demise by Flavobacterium oncorhynchi diagnosed with placental microscopy. J. Obstetrics Gynaecology Canada. doi: 10.1016/j.jogc.2022.04.020
Flemming, L., Rawlings, D., Chenia, H. (2007). Phenotypic and molecular characterisation of fish-borne Flavobacterium johnsoniae-like isolates from aquaculture systems in south Africa. Res. Microbiol. 158, 18–30. doi: 10.1016/j.resmic.2006.09.001
Galloway-Peña, J., Sahasrabhojane, P., Tarrand, J., Han, X. Y., Shelburne, S. A. (2014). GyrB polymorphisms accurately assign invasive viridans group streptococcal species. J. Clin. Microbiol. 52, 2905–2912. doi: 10.1128/JCM.01068-14
García-López, M., Meier-Kolthoff, J. P., Tindall, B. J., Gronow, S., Woyke, T., Kyrpides, N. C., et al. (2019). Analysis of 1,000 type-strain genomes improves taxonomic classification of bacteroidetes. Front. Microbiol. 10. doi: 10.3389/fmicb.2019.02083
Gholamhosseini, A., Taghadosi, V., Shiry, N., Akhlaghi, M., Sharifiyazdi, H., Soltanian, S., et al. (2018). First isolation and identification of Aeromonas veronii and Chryseobacterium joostei from reared sturgeons in fars province, Iran. Vet. Res. Forum 9, 113–119. doi: 10.30466/VRF.2018.30826
Good, C., Davidson, J., Wiens, G. D., Welch, T. J., Summerfelt, S. (2015). Flavobacterium branchiophilum and F. succinicans associated with bacterial gill disease in rainbow trout Oncorhynchus mykiss (Walbaum) in water recirculation aquaculture systems. J. Fish Dis. 38, 409–413. doi: 10.1111/jfd.12249
Harrison, F. C. (1929). The discoloration of halibut. Can. J. Res. 1, 214–239. doi: 10.1139/cjr29-013
Hillis, D. M., Bull, J. J. (1993). An empirical test of bootstrapping as a method for assessing confidence in phylogenetic analysis. Systematic Biol. 42, 182–192. doi: 10.1093/sysbio/42.2.182
Holmes, B., Steigerwalt, A. G., Nicholson, A. C. (2013). DNA-DNA Hybridization study of strains of Chryseobacterium, Elizabethkingia and Empedobacter and of other usually indole-producing non-fermenters of CDC groups IIc, IIe, IIh and IIi, mostly from human clinical sources, and proposals of Chryseobacterium bernardetii sp. nov., Chryseobacterium carnis sp. nov., Chryseobacterium lactis sp. nov., Chryseobacterium nakagawai sp. nov. and Chryseobacterium taklimakanense comb. nov. Int. J. Syst. Evol. Microbiol. 63, 4639–4662. doi: 10.1099/ijs.0.054353-0
Hugo, C., Bernardet, J.-F., Nicholson, A., Kämpfer, P. (2019). “Chryseobacterium,” in Bergey’s manual of systematics of archaea and bacteria (John Wiley & Sons, Ltd), 1–107. doi: 10.1002/9781118960608.gbm00301.pub2
Ilardi, P., Abad, J., Rintamäki, P., Bernardet, J.-F., Avendaño-Herrera, R. (2010). Phenotypic, serological and molecular evidence of Chryseobacterium piscicola in farmed Atlantic salmon, Salmo salar l., in Finland. J. Fish Dis. 33, 179–181. doi: 10.1111/j.1365-2761.2009.01091.x
Ilardi, P., Fernández, J., Avendaño-Herrera, R. (2009). Chryseobacterium piscicola sp. nov., isolated from diseased salmonid fish. Int. J. Systematic Evolutionary Microbiol. 59, 3001–3005. doi: 10.1099/ijs.0.007021-0
Janda, J. M., Abbott, S. L. (2007). 16S rRNA gene sequencing for bacterial identification in the diagnostic laboratory: Pluses, perils, and pitfalls. J. Clin. Microbiol. 45, 2761–2764. doi: 10.1128/JCM.01228-07
Jung, W. J., Kim, S. G., Giri, S. S., Kim, S. W., Kang, J. W., Kwon, J., et al. (2022). The opportunistic pathogen Chryseobacterium balustinum WLT: Pathogenicity and antibiotic resistance. Fishes 7, 26. doi: 10.3390/fishes7010026
Kämpfer, P., Fallschissel, K., Avendaño-Herrera, R. (2011). Chryseobacterium chaponense sp. nov., isolated from farmed Atlantic salmon (Salmo salar). Int. J. Systematic Evolutionary Microbiol. 61, 497–501. doi: 10.1099/ijs.0.022004-0
Kämpfer, P., Irgang, R., Glaeser, S. P., Busse, H.-J., Criscuolo, A., Clermont, D., et al. (2020). Flavobacterium salmonis sp. nov. isolated from Atlantic salmon (Salmo salar) and formal proposal to reclassify Flavobacterium spartansii as a later heterotypic synonym of Flavobacterium tructae. Int. J. Systematic Evolutionary Microbiol. 70, 6147–6154. doi: 10.1099/ijsem.0.004510
Kämpfer, P., Lodders, N., Martin, K., Avendaño-Herrera, R. Y. (2012). Flavobacterium chilense sp. nov. and Flavobacterium araucananum sp. nov., isolated from farmed salmonid fish. Int. J. Systematic Evolutionary Microbiol. 62, 1402–1408. doi: 10.1099/ijs.0.033431-0
Kämpfer, P., Vaneechoutte, M., Wauters, G. (2009). Chryseobacterium arothri Campbell et al. 2008 is a later heterotypic synonym of Chryseobacterium hominis vaneechoutte et al. 2007. Int. J. Systematic Evolutionary Microbiol. 59, 695–697. doi: 10.1099/ijs.0.004093-0
Kent, M. L., Groff, J. M., Morrison, J. K., Yasutake, W. T., Holt, R. A. (1989). Spiral swimming behavior due to cranial and vertebral lesions associated with Cytophaga psychrophila infection in salmonid fishes. Dis. Aquat Organ 6, 11–16. doi: 10.3354/dao006011
Kim, S. G., Giri, S. S., Kim, S. W., Kwon, J., Lee, S. B., Park, S. C. (2020). First isolation and characterization of Chryseobacterium cucumeris SKNUCL01, isolated from diseased pond loach (Misgurnus anguillicaudatus) in Korea. Pathogens 9, E397. doi: 10.3390/pathogens9050397
Kumar, S., Stecher, G., Li, M., Knyaz, C., Tamura, K. (2018). MEGA X: Molecular evolutionary genetics analysis across computing platforms. Mol. Biol. Evol. 35, 1547–1549. doi: 10.1093/molbev/msy096
Kumru, S., Tekedar, H. C., Blom, J., Lawrence, M. L., Karsi, A. (2020). Genomic diversity in flavobacterial pathogens of aquatic origin. Microbial Pathogenesis 142, 104053. doi: 10.1016/j.micpath.2020.104053
LaFrentz, B. R., Klesius, P. H. (2009). Development of a culture independent method to characterize the chemotactic response of Flavobacterium columnare to fish mucus. J. Microbiological Methods 77, 37–40. doi: 10.1016/j.mimet.2008.12.011
LaFrentz, B. R., Králová, S., Burbick, C. R., Alexander, T. L., Phillips, C. W., Griffin, M. J., et al. (2022). The fish pathogen Flavobacterium columnare represents four distinct species: Flavobacterium columnare, flavobacterium covae sp. nov., Flavobacterium davisii sp. nov. and Flavobacterium oreochromis sp. nov., and emended description of Flavobacterium columnare. Systematic Appl. Microbiol. 45, 126293. doi: 10.1016/j.syapm.2021.126293
Lane, D. J., Pace, B., Olsen, G. J., Stahl, D. A., Sogin, M. L., Pace, N. R. (1985). Rapid determination of 16S ribosomal RNA sequences for phylogenetic analyses. Proc. Natl. Acad. Sci. U.S.A. 82, 6955–6959. doi: 10.1073/pnas.82.20.6955
Lee, B.-H., Nicolas, P., Saticioglu, I. B., Fradet, B., Bernardet, J.-F., Rigaudeau, D., et al. (2022). Investigation of the genus Flavobacterium as a reservoir for fish-pathogenic bacterial species: the case of Flavobacterium collinsii. Microbiology. doi: 10.1101/2022.09.27.509832
Letunic, I., Bork, P. (2019). Interactive tree of life (iTOL) v4: recent updates and new developments. Nucleic Acids Res. 47, W256–W259. doi: 10.1093/nar/gkz239
Lin, J.-N., Lai, C.-H., Yang, C.-H., Huang, Y.-H. (2019). Differences in clinical manifestations, antimicrobial susceptibility patterns, and mutations of fluoroquinolone target genes between Chryseobacterium gleum and Chryseobacterium indologenes. Antimicrobial Agents Chemotherapy 63, e02256–e02218. doi: 10.1128/AAC.02256-18
Loch, T. P. (2012). Identification of novel flavobacteria from Michigan and assessment of their impacts on fish health (East Lansing (MI: Michigan State University).
Loch, T. P., Faisal, M. (2014a). Deciphering the biodiversity of fish-pathogenic Flavobacterium spp. recovered from the great lakes basin. Dis. Aquat. Organisms 112, 45–57. doi: 10.3354/dao02791
Loch, T. P., Faisal, M. (2014b). Chryseobacterium aahli sp. nov., isolated from lake trout (Salvelinus namaycush) and brown trout (Salmo trutta), and emended descriptions of Chryseobacterium ginsenosidimutans and Chryseobacterium gregarium. Int. J. Systematic Evolutionary Microbiol. 64, 1573–1579. doi: 10.1099/ijs.0.052373-0
Loch, T. P., Faisal, M. (2014c). Flavobacterium spartansii sp. nov., a pathogen of fishes, and emended descriptions of Flavobacterium aquidurense and Flavobacterium araucananum. Int. J. Systematic Evolutionary Microbiol. 64, 406–412. doi: 10.1099/ijs.0.051433-0
Loch, T. P., Faisal, M. (2015a). Emerging flavobacterial infections in fish: A review. J. Advanced Res. 6, 283–300. doi: 10.1016/j.jare.2014.10.009
Loch, T. P., Faisal, M. (2015b). Polyphasic characterization reveals the presence of novel fish-associated Chryseobacterium spp. in the great lakes of north America. Dis. Aquat. Organisms 113, 113–125. doi: 10.3354/dao02819
Loch, T. P., Faisal, M. (2016a). Flavobacteria isolated from the milt of feral Chinook salmon of the great lakes. North Am. J. Aquaculture 78, 25–33. doi: 10.1080/15222055.2015.1090506
Loch, T. P., Faisal, M. (2016b). Flavobacterium spartansii induces pathological changes and mortality in experimentally challenged Chinook salmon Oncorhynchus tshawytscha (Walbaum). J. Fish Dis. 39, 483–488. doi: 10.1111/jfd.12363
Loch, T. P., Faisal, M. (2016c). Gamete-associated flavobacteria of the oviparous Chinook salmon (Oncorhynchus tshawytscha) in lakes Michigan and Huron, north America. J. Microbiol. 54, 477–486. doi: 10.1007/s12275-016-5629-3
Loch, T. P., Faisal, M. (2018). Flavobacteria colonizing the early life stages of hatchery-incubated Chinook salmon Oncorhynchus tshawytscha (Walbaum 1792) are markedly diverse. J. Fish Dis. 41, 829–845. doi: 10.1111/jfd.12795
Loch, T. P., Fujimoto, M., Woodiga, S. A., Walker, E. D., Marsh, T. L., Faisal, M. (2013). Diversity of fish-associated Flavobacteria of Michigan. J. Aquat. Anim. Health 25, 149–164. doi: 10.1080/08997659.2012.758189
Loveland-Curtze, J., Miteva, V., Brenchley, J. (2010). Novel ultramicrobacterial isolates from a deep Greenland ice core represent a proposed new species, Chryseobacterium greenlandense sp. nov. Extremophiles 14, 61–69. doi: 10.1007/s00792-009-0287-6
Madsen, L., Arnbjerg, J., Dalsgaard, I. (2001). Radiological examination of the spinal column in farmed rainbow trout Oncorhynchus mykiss (Walbaum): Experiments with Flavobacterium psychrophilum and oxytetracycline. Aquaculture Res. 32, 235–241. doi: 10.1046/j.1365-2109.2001.00552.x
Malick, R. C., Das, B. K., Pal, B. B., Bera, A. K., Maurye, P., Sahoo, A. K. (2022). Molecular identification, whole-cell protein profiling, antibiotics sensitivity and pathogenicity of Chryseobacterium gleum recovered from diseased fishes of freshwater wetlands in India. Aquaculture 559, 738411. doi: 10.1016/j.aquaculture.2022.738411
Malka, S., Goller, M., Dennison-Gibby, S. (2020). Septic arthritis and osteomyelitis caused by Chryseobacterium indologenes in a mute swan (Cygnus olor) and a ring-billed gull (Larus delawarensis). avms 34, 289–294. doi: 10.1647/1082-6742-34.3.289
Mantareva, V., Kussovski, V., Orozova, P., Angelov, I., Durmuş, M., Najdenski, H. (2022). Palladium phthalocyanines varying in substituents position for photodynamic inactivation of Flavobacterium hydatis as sensitive and resistant species. Curr. Issues Mol. Biol. 44, 1950–1959. doi: 10.3390/cimb44050133
Maravić, A., Skočibušić, M., Šamanić, I., Puizina, J. (2013). Profile and multidrug resistance determinants of Chryseobacterium indologenes from seawater and marine fauna. World J. Microbiol. Biotechnol. 29, 515–522. doi: 10.1007/s11274-012-1205-0
Michel, C., Matte-Tailliez, O., Kerouault, B., Bernardet, J.-F. (2005). Resistance pattern and assessment of phenicol agents’ minimum inhibitory concentration in multiple drug resistant Chryseobacterium isolates from fish and aquatic habitats. J. Appl. Microbiol. 99, 323–332. doi: 10.1111/j.1365-2672.2005.02592.x
Mudarris, M., Austin, B. (1989). Systemic disease in turbot Scophthalmus maximus caused by a previously unrecognised Cytophaga like bacterium. Dis. Aquat. Org. 6, 161–166. doi: 10.3354/dao006161
Mudarris, M., Austin, B., Segers, P., Vancanneyt, M., Hoste, B., Bernardet, J. F. (1994). Flavobacterium scophthalmum sp. nov., a pathogen of turbot (Scophthalmus maximus l.). Int. J. Systematic Evolutionary Microbiol. 44, 447–453. doi: 10.1099/00207713-44-3-447
Mühle, E., Abry, C., Leclerc, P., Goly, G.-M., Criscuolo, A., Busse, H.-J., et al. (2020). Flavobacterium bizetiae sp. nov., isolated from diseased freshwater fish in Canada at the end of the 1970s. Int. J. Systematic Evolutionary Microbiol. 71, 4576. doi: 10.1099/ijsem.0.004576
Mwega, E., Colquhoun, D. J., Tuntufye, H., Mdegela, R., Mutoloki, S., Evensen, Ø., et al. (2019). Isolation and characterization of flavobacteriaceae from farmed and wild Nile tilapia in Tanzania. J. Aquat. Anim. Health 31, 23–30. doi: 10.1002/aah.10048
Nei, M., Kumar, S. (2000). Molecular evolution and phylogenetics (Oxford, New York: Oxford University Press).
Oh, W. T., Jun, J. W., Giri, S. S., Yun, S., Kim, H. J., Kim, S. G., et al. (2020). Isolation of chryseobacterium siluri sp. nov., from liver of diseased catfish (Silurus asotus). Heliyon 6, e03454. doi: 10.1016/j.heliyon.2020.e03454
Parte, A. C., Sardà Carbasse, J., Meier-Kolthoff, J. P., Reimer, L. C., Göker, M. (2020). List of prokaryotic names with standing in nomenclature (LPSN) moves to the DSMZ. Int. J. Systematic Evolutionary Microbiol. 70, 5607–5612. doi: 10.1099/ijsem.0.004332
Pridgeon, J. W., Klesius, P. H., Garcia, J. C. (2013). Identification and virulence of chryseobacterium indologenes isolated from diseased yellow perch (Perca flavescens). J. Appl. Microbiol. 114, 636–643. doi: 10.1111/jam.12070
Reichley, S. R., Ware, C., Steadman, J., Gaunt, P. S., García, J. C., LaFrentz, B. R., et al. (2017). Comparative phenotypic and genotypic analysis of Edwardsiella isolates from different hosts and geographic origins, with emphasis on isolates formerly classified as E. tarda, and evaluation of diagnostic methods. J. Clin. Microbiol. 55, 3466–3491. doi: 10.1128/JCM.00970-17
Rintamäki-Kinnunen, P., Bernardet, J.-F., Bloigu, A. (1997). Yellow pigmented filamentous bacteria connected with farmed salmonid fish mortality. Aquaculture 149, 1–14. doi: 10.1016/S0044-8486(96)01431-7
Saticioglu, I. B. (2021). Flavobacterium erciyesense sp. nov., a putative non-pathogenic fish symbiont. Arch. Microbiol. 203, 5783–5792. doi: 10.1007/s00203-021-02566-2
Saticioglu, I. B., Ay, H., Altun, S., Duman, M., Sahin, N. (2021a). Flavobacterium turcicum sp. nov. and Flavobacterium kayseriense sp. nov. isolated from farmed rainbow trout in Turkey. Systematic Appl. Microbiol. 44, 126186. doi: 10.1016/j.syapm.2021.126186
Saticioglu, I. B., Ay, H., Altun, S., Sahin, N., Duman, M. (2021b). Flavobacterium bernardetii sp. nov., a possible emerging pathogen of farmed rainbow trout (Oncorhynchus mykiss) in cold water. Aquaculture 540, 736717. doi: 10.1016/j.aquaculture.2021.736717
Saticioglu, I. B., Duman, M., Altun, S. (2021c). Genome analysis and antimicrobial resistance characteristics of Chryseobacterium aquaticum isolated from farmed salmonids. Aquaculture 535, 736364. doi: 10.1016/j.aquaculture.2021.736364
Sebastião, F., de, A., LaFrentz, B. R., Shelley, J. P., Stevens, B., Marancik, D., et al. (2019a). Flavobacterium inkyongense isolated from ornamental cichlids. J. Fish Dis. 42, 1309–1313. doi: 10.1111/jfd.13043
Sebastião, F., de, A., Loch, T. P., Marancik, D. P., Griffin, M. J., Maret, J., et al. (2019b). Identification of chryseobacterium spp. isolated from clinically affected fish in California, USA. Dis. Aquat. Organisms 136, 227–234. doi: 10.3354/dao03409
Shahi, N., Sharma, P., Pandey, J., Bisht, I., Mallik, S. K. (2018). Characterization and pathogenicity study of Chryseobacterium scophthalmum recovered from gill lesions of diseased golden mahseer, Tor putitora (Hamilton 1822) in India. Aquaculture 485, 81–92. doi: 10.1016/j.aquaculture.2017.11.018
Soltani, M., Munday, B. L., Carson, J. (1994). Susceptibility of some freshwater species of fish to infection by Cytophaga johnsonae. Bull. Eur. Ass. Fish. 14 (4), 133–135.
Stackebrandt, E., Ebers, J. (2006). Taxonomic parameters revisited: Tarnished gold standards. Microbiol. Today 8, 6–9.
Stoddard, S. F., Smith, B. J., Hein, R., Roller, B. R. K., Schmidt, T. M. (2015). rrnDB: improved tools for interpreting rRNA gene abundance in bacteria and archaea and a new foundation for future development. Nucleic Acids Res. 43, D593–D598. doi: 10.1093/nar/gku1201
Strohl, W. R., Tait, L. R. (1978). Cytophaga aquatilis sp. nov., a facultative anaerobe isolated from the gills of freshwater fish. Int. J. Systematic Evolutionary Microbiol. 28, 293–303. doi: 10.1099/00207713-28-2-293
SuXu, H., GuoQing, M., WenJing, N., YouShen, L., ZhiGang, Z. (2019). Pathogenicity study of Chryseobacterium oncorhynchi B8 isolated from sturgeon (Acipenser baerii) in north China. J. Agric. Sci. Technol. (Beijing) 21, 96–103.
Tamai, I. A., Pakbin, B., Kafi, Z. Z., Brück, W. M. (2021). Oral abscess caused by Chryseobacterium indologenes in ball Python (Python regius); A case report. Antibiotics 10, 686. doi: 10.3390/antibiotics10060686
United States Department of Agriculture (2019) 2018 census of aquaculture. Available at: https://www.nass.usda.gov/Publications/AgCensus/2017/Online_Resources/Aquaculture/Aqua.pdf.
Vandamme, P., Bernardet, J.-F., Segers, P., Kersters, K., Holmes, B. Y. (1994). NOTES: New perspectives in the classification of the flavobacteria: Description of Chryseobacterium gen. nov., Bergeyella gen. nov., and Empedobacter nom. rev. Int. J. Systematic Evolutionary Microbiol. 44, 827–831. doi: 10.1099/00207713-44-4-827
Vaneechoutte, M., Kämpfer, P., De Baere, T., Avesani, V., Janssens, M., Wauters, G. (2007). Chryseobacterium hominis sp. nov., to accommodate clinical isolates biochemically similar to CDC groups II-h and II-c. Int. J. Systematic Evolutionary Microbiol. 57, 2623–2628. doi: 10.1099/ijs.0.65158-0
Verma, D. K., Rathore, G. (2015). New host record of five Flavobacterium species associated with tropical fresh water farmed fishes from north India. Braz. J. Microbiol. 46, 969–976. doi: 10.1590/S1517-838246420131081
Verner-Jeffreys, D. W., Brazier, T., Perez, R. Y., Ryder, D., Card, R. M., Welch, T. J., et al. (2017). Detection of the florfenicol resistance gene floR in Chryseobacterium isolates from rainbow trout. exception to the general rule? FEMS Microbiol. Ecol. 93. doi: 10.1093/femsec/fix015
Wahli, T., Madsen, L. (2018). Flavobacteria, a never ending threat for fish: a review. Curr. Clin. Micro Rpt 5, 26–37. doi: 10.1007/s40588-018-0086-x
Wang, L.-T., Lee, F.-L., Tai, C.-J., Kasai, H. (2007). Comparison of gyrB gene sequences, 16S rRNA gene sequences and DNA–DNA hybridization in the Bacillus subtilis group. Int. J. Systematic Evolutionary Microbiol. 57, 1846–1850. doi: 10.1099/ijs.0.64685-0
Xin, Y.-H., Liang, Z.-H., Zhang, D.-C., Liu, H.-C., Zhang, J.-L., Yu, Y., et al. (2009). Flavobacterium tiangeerense sp. nov., a cold-living bacterium isolated from a glacier. Int. J. Syst. Evol. Microbiol. 59, 2773–2777. doi: 10.1099/ijs.0.007906-0
YiBin, Y., XiaoHui, A., Yi, S., HaiPeng, C., XianLe, Y., JiaYun, Y., et al. (2018). Isolation, identification and antibiotic sensitivity of Chryseobacterium indologenes from Trionyx sinensis. Acta Hydrobiologica Sin. 42, 786–793.
Zamora, L., Fernández-Garayzábal, J. F., Palacios, M. A., Sánchez-Porro, C., Svensson-Stadler, L. A., Domínguez, L., et al. (2012a). Chryseobacterium oncorhynchi sp. nov., isolated from rainbow trout (Oncorhynchus mykiss). Systematic Appl. Microbiol. 35, 24–29. doi: 10.1016/j.syapm.2011.10.002
Zamora, L., Fernández-Garayzábal, J. F., Sánchez-Porro, C., Palacios, M. A., Moore, E. R. B., Domínguez, L., et al. (2013a). Flavobacterium plurextorum sp. nov. isolated from farmed rainbow trout (Oncorhynchus mykiss). PloS One 8, e67741. doi: 10.1371/journal.pone.0067741
Zamora, L., Vela, A. I., Palacios, M. A., Domínguez, L., Fernández-Garayzábal, J. F. (2012b). First isolation and characterization of chryseobacterium shigense from rainbow trout. BMC Veterinary Res. 8, 77. doi: 10.1186/1746-6148-8-77
Zamora, L., Vela, A. I., Palacios, M. A., Sánchez-Porro, C., Moore, E. R. B., Domínguez, L., et al. (2012c). Chryseobacterium tructae sp. nov., isolated from rainbow trout (Oncorhynchus mykiss). Syst. Appl. Microbiol. 35, 315–319. doi: 10.1016/j.syapm.2012.06.003
Zamora, L., Vela, A. I., Palacios, M. A., Sánchez-Porro, C., Svensson-Stadler, L. A., Domínguez, L., et al. (2012d). Chryseobacterium viscerum sp. nov., isolated from diseased fish. Int. J. Systematic Evolutionary Microbiol. 62, 2934–2940. doi: 10.1099/ijs.0.036699-0
Zamora, L., Vela, A. I., Sánchez-Porro, C., Palacios, M. A., Domínguez, L., Moore, E. R. B., et al. (2013b). Characterization of flavobacteria possibly associated with fish and fish farm environment. description of three novel Flavobacterium species: Flavobacterium collinsii sp. nov., Flavobacterium branchiarum sp. nov., and Flavobacterium branchiicola sp. nov. Aquaculture 416–417, 346–353. doi: 10.1016/j.aquaculture.2013.09.019
Zamora, L., Vela, A. I., Sánchez-Porro, C., Palacios, M. A., Moore, E. R. B., Domínguez, L., et al. (2014). Flavobacterium tructae sp. nov. and flavobacterium piscis sp. nov., isolated from farmed rainbow trout (Oncorhynchus mykiss). Int. J. Systematic Evolutionary Microbiol. 64, 392–399. doi: 10.1099/ijs.0.056341-0
Keywords: Flavobacteriales, Flavobacterium, Chryseobacterium, 16S rRNA, gyrB, flavobacteria
Citation: Heckman TI, Yazdi Z, Pomaranski EK, Sebastião FdA, Mukkatira K, Vuglar BM, Cain KD, Loch TP and Soto E (2023) Atypical flavobacteria recovered from diseased fish in the Western United States. Front. Cell. Infect. Microbiol. 13:1149032. doi: 10.3389/fcimb.2023.1149032
Received: 20 January 2023; Accepted: 07 March 2023;
Published: 19 April 2023.
Edited by:
Erina Nagata, Kindai University, JapanCopyright © 2023 Heckman, Yazdi, Pomaranski, Sebastião, Mukkatira, Vuglar, Cain, Loch and Soto. This is an open-access article distributed under the terms of the Creative Commons Attribution License (CC BY). The use, distribution or reproduction in other forums is permitted, provided the original author(s) and the copyright owner(s) are credited and that the original publication in this journal is cited, in accordance with accepted academic practice. No use, distribution or reproduction is permitted which does not comply with these terms.
*Correspondence: Esteban Soto, c290b21hcnRpbmV6QHVjZGF2aXMuZWR1
†Present address: Kenneth D. Cain, NOAA – Fisheries, Manchester Research Station, Northwest Fisheries Science Center, Port Orchard, WA, United States