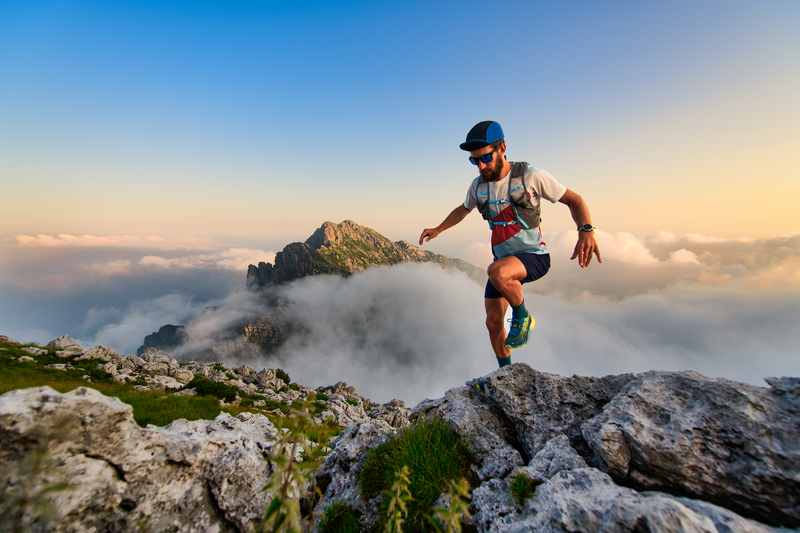
95% of researchers rate our articles as excellent or good
Learn more about the work of our research integrity team to safeguard the quality of each article we publish.
Find out more
REVIEW article
Front. Cell. Infect. Microbiol. , 13 March 2023
Sec. Intestinal Microbiome
Volume 13 - 2023 | https://doi.org/10.3389/fcimb.2023.1139800
This article is part of the Research Topic The Role of Gut Microbes and Their Metabolites in Immune-related Diseases View all 12 articles
Obesity, a chronic metabolic disorder caused by an energy imbalance, has been increasingly prevalent and poses a global health concern. The multifactorial etiology of obesity includes genetics factors, high-fat diet, gut microbiota, and other factors. Among these factors, the implication of gut microbiota in the pathogenesis of obesity has been prominently acknowledged. This study endeavors to investigate the potential contribution of gut microbiota to the development of high-fat diet induced obesity, as well as the current state of probiotic intervention therapy research, in order to provide novel insights for the prevention and management of obesity.
Obesity is a chronic and recurring condition that results from excessive or inappropriate fat accumulation (Obesity: preventing and managing the global epidemic. Report of a WHO consultation, 2000; Bray et al., 2017). The global incidence of obesity among adults has increased by 1.5-fold since 2000, with over 1.9 billion overweight adults in 2016. Children and adolescents have also experienced a rise in the prevalence of obesity, with an increase from 2.9% to 6.8% in the population aged 5 to 19 years (Abarca-Gómez et al., 2017). Obesity has serious implications for health, including an elevated risk of mortality, type 2 diabetes, and cardiovascular disease. The etiology of obesity is multifactorial, with contributing factors including genetics, a high-fat diet (HFD), and gut microbiota. The gut microbiota, which is composed mainly of anaerobic bacteria, facultative anaerobic bacteria, and aerobic bacteria, is a dynamic ecosystem that coevolves with its host (Wu et al., 2022). The gut microbiota plays a crucial role in maintaining the health of the host through vitamin production, nutrient absorption, and the secretion of small molecules involved in immune regulation, angiogenesis, and nerve function (Dominguez-Bello et al., 2019; Robertson et al., 2019). The human gut contains approximately 1014 microorganisms (Gill et al., 2006), predominantly composed of Firmicutes and Bacteroidetes species (Bolam and van den Berg, 2018). Different bacterial species occupy distinct sections of the intestine; for instance, Firmicutes often predominate at the top of the gut crypto-villous unit while Proteus predominates at the bottom (Sommer and Backhed, 2016). The functional consistency of each bacterial genus is quite high (Costea et al., 2018) and is not affected by the host’s age, sex, BMI, or nationality (Sebastian Domingo and Sanchez Sanchez, 2018).
The present study shows that the manifestation of obesity and its metabolic dysfunctions were absent in germ-free mice. Notably, the transplantation of cecal or fecal samples from obese mice into germ-free mice resulted in the development of similar symptoms, indicating that the gut microbiota plays a critical role in the pathogenesis of obesity (Ridaura et al., 2013). Furthermore, it was observed that the transfer of gut microbiota could also transmit the obesity phenotype (Kapoor et al., 2021; Romani-Perez et al., 2021). In mice fed the same HFD, some developed obesity and some were resistant to it, and differences in gut microbiota composition may be the most important factor in both outcomes. In addition, intestinal barrier function, intestinal inflammation and neurotrophic factors also play an important role in diet-induced obesity (Zhang et al., 2019b). A growing body of evidence from animal studies suggests a link between diet, gut microbiota and obesity, as well as in humans. But studies have not reached a consistent conclusion on exactly what microbial composition is at work. Moreover, an interesting study found that transfer of the whole microbiota may not reduce diabetes incidence despite a major change in gut microbiota of the non-obese diabetes (NOD) mice model. NOD mouse models can be divided into two colonies (high or low diabetes incidence), transplanting intestinal flora from low-incidence NOD mice to high-incidence NOD mice did not change the incidence of diabetes, but transplantation of A. muciniphila to high-incidence NOD mice can promote mucogenesis, increase the expression of antimicrobial peptide Reg3γ, inhibit the growth of rumen contortus, reduce the level of serum endotoxin, reduce the expression of TLR in pancreatic islets, promote regulatory immunity, and delay the development of diabetes (Hanninen et al., 2018). It shows that some single species of bacteria, rather than the entire intestinal flora, may play a major role in inducing or resisting metabolic diseases under certain conditions.
As per conventional understanding, the establishment of gut microbiota occurs after birth, while the mother’s uterus remains free of microorganisms. Various factors, such as delivery mode, feeding type, and medication administration (including antibiotics), impact the diversity of gut microbiota, as stated in the literature (Theis et al., 2019; Akagawa et al., 2021). By age 3, the gut microbiota progresses towards a complex and stable state similar to that of adults (Derrien et al., 2019), which then remains mostly consistent throughout adulthood. According to a population-based study, the obese population demonstrates significant differences in gut microbiota composition compared to the general population (Cuevas-Sierra et al., 2019). A few studies propose that the “enterotype of the fertility microbiota” is characterized by a higher abundance of Firmicutes/Bacteroidetes (Kim et al., 2021). Nevertheless, the distribution of this distinct microbiota is still subject to debate due to variation in sample size, individual clinical and anthropometric traits (age, sex, microbiota distribution, and degree of obesity), and microbiota analysis techniques (qPCR, 16S rRNA gene sequencing, and Fluorescence in situ hybridization) (Zeng et al., 2019; Assmann et al., 2020).
The human gut microbiota is highly responsive to changes in food intake and the physiological state of the digestive system (Turnbaugh et al., 2009; Qin et al., 2020), with alterations observed within a period as short as 24 hours (David et al., 2014). A HFD has been found to significantly reduce the diversity of gut microbiota (Wan et al., 2019), resulting in a decrease in the number of bacteria that are responsible for maintaining the integrity of the gut mucosal barrier and an increase in the number of bacteria that breach it (Monk et al., 2019; Zhang et al., 2019a). This alteration in gut microbiota is characterized by a reduction in the relative abundance of Bacteroides and an increase in the relative abundance of Firmicutes (An et al., 2022). Moreover, the concentration of lipopolysaccharide (LPS) has been found to increase with the number of Actinomycetes while the number of Bifidobacteria declines as Vibrio desulfonate increased. Excess sulfate is converted to hydrogen sulfide, which further compromises the gut barrier and promotes inflammation (Chen et al., 2019). Additionally, the gut barrier is disrupted by Akkermansia muciniphila (A. muciniphila), a member of phylum Verrucomicrobia that degrades mucins and has anti-inflammatory and protective effects on the intestinal mucosal barrier (Hanninen et al., 2018).
Previous research has provided evidence that a HFD can lead to obesity, inflammation, and enhance gut epithelial cell permeability (Lemons and Liu, 2022). The mechanism through which HFD induces increased gut permeability involves several processes (Figure 1).
In the HFD, intestinal epithelial cells in the lower intestine actively ingest a significant amount of fat, which leads to the simultaneous generation of reactive oxygen species (ROS), iron, copper, aldehydes, lipid peroxidation, as well as ATP by the mitochondrial respiratory chain (Spinelli and Haigis, 2018). The ROS generated under the influence of the HFD cause increased gut epithelial cell permeability (Ballard and Towarnicki, 2020), ultimately leading to the destruction of the gut barrier function and the proliferation of harmful bacteria like Salmonella and Escherichia coli in the gut cavity. Furthermore, the hydrogen sulfide generated by the HFD inhibits the mitochondrial respiratory chain, which makes it easier for pathogenic bacteria to infect more cells (Mottawea et al., 2016). The production of iron, copper, aldehydes, and lipid peroxidation during the digestion and absorption of high dietary fats leads to an increase in oxidative stress in gut tissues, destroying the microbiota’s living environment, resulting in an imbalance of gut microbiota.
The HFD contains a large amount of polyunsaturated fatty acids that are prone to oxidation of their double bonds (Mariamenatu and Abdu, 2021). The free fatty acids generated under the influence of the HFD impact the gut immune system directly (Tanaka et al., 2020) raising the levels of barrier-damaging cytokines such as TNF-α, IL-1β, IL-6, IFN-γ, while decreasing barrier-protective cytokines such as IL-10, IL-17, IL-22, ultimately leading to an increase in gut permeability (Bartoszek et al., 2020; Stoeva et al., 2021). The resulting pathological changes, including low-grade inflammation, decreased expression of antimicrobial peptides, mucus secretion, and expression of tight junction protein, impact multiple system functions and lead to obesity and its metabolic complications (insulin resistance, hyperglycemia, systemic inflammation, and dyslipidemia) (Araújo et al., 2017; Jiang et al., 2020; Kumar et al., 2021).
The gut barrier system comprises mucus layers, gut epithelial cells (IECs), tight junctions (TJS), immune cells, and gut microbiota (Rohr et al., 2020). The apical junctional complex (AJC) is composed of the membrane proteins TJS and adhere junctions (AJS) (Capaldo et al., 2017). The AJC’s integrity is critical for the selective passage of nutrients while obstructing the entry of toxins and antigens, leading to high permeability of the gut. Dietary fat has the potential to directly impact the integrity of the AJC (Netto Candido et al., 2018; Tsukita et al., 2019; Otani and Furuse, 2020). In long-term HFD, gut occlusion zone-1 (ZO-1) and occludin gene expression are decreased, which leads to an increase in gut permeability (Oliveira et al., 2019; Nascimento et al., 2021). The HFD’s abundance of docosahexaenoic acid and γ-linolenic acid triggers protein kinase activation, actin and TJ protein redistribution, and increased gut permeability (Usami et al., 2003). Additionally, part of the eicosapentaenoic acids in HFD can be converted into bioactive metabolites to increase gut permeability (Usami et al., 2001).
Dietary fat consumption and bile acid secretion exhibit a positive correlation (Ocvirk and O'Keefe, 2021), and IECs possess the ability to resist bile acid degradation under normal physiological conditions. However, HFD induces long-term and high-level secretion of bile acids, resulting in the release of numerous hydrophobic bile acids, such as cholic acid and deoxycholic acid (Iwamoto et al., 2021). These bile acids promote occludin protein dephosphorylation, leading to the dissociation of the adhesive junction complex and ultimately causing an increase in gut permeability. In addition, they can cause harm to the gut mucosal barrier and induce oxidative stress and cell apoptosis in IECs (Raimondi et al., 2008; Di Ciaula et al., 2017; Sarathy et al., 2017; Gupta et al., 2020).
Furthermore, HFD inhibits the peroxisome proliferator-activated receptor-γ (PPAR-γ) pathway in mice, disrupting the gut mucus layer, decreasing electrolyte secretion, and impairing mucosal immune defense. However, a week of treatment with a specific PPAR-γ agonist, rosiglitazone, or a return to a normal diet can reverse the increased gut epithelial permeability caused by HFD (Lee et al., 2020), resulting in the disruption of the gut mucus layer, reduced electrolyte secretion, and decreased mucosal immune defense. Following a week of therapy with rosiglitazone, a particular PPAR-agonist, or returning to the usual diet, this increase in gut epithelial permeability was reversed.
The consumption of a HFD has been observed to enhance the permeability of gut epithelial cells and disrupt the interplay between the local intestine mucosal immune system and the gut microbiota, leading to an imbalance in the microbiota composition. This imbalance is characterized by a rise in the number of gram-negative bacteria, and the resultant LPS produced by these bacteria interact with the CD14/Toll-like receptor 4 (TLR4) complexes of gut epithelial cells, leading to the activation of the innate immune system. This activation causes local and systemic persistent low-level inflammation, which leads to further destruction of the mucous layer and increased permeability of IEC. The heightened permeability of IECs facilitates the entry of gut microbiota metabolites into the bloodstream, resulting in a vicious cycle of inflammation and dysbacteriosis. The ongoing activation of the LPS/TLR4 signal pathway is believed to be a major contributor to the development of obesity and related metabolic disorders (Kasselman et al., 2018; Giordano et al., 2020; Mohammad and Thiemermann, 2020) (Figure 2).
The modulation of host gene promoters related to lipid metabolism, obesity, and inflammatory responses by the dominant Firmicutes within the gut microbiota has been reported through recent investigations (Cuevas-Sierra et al., 2019; Amabebe et al., 2020). However, the examination of gut microbiota-obesity association at a population level presents a significant challenge, given the inadequate sample sizes and inadequate representation of individual subjects in existing gut microbiota studies (Stanislawski et al., 2019). This shortcoming necessitates further research efforts towards resolving these limitations.
The gut microbiota is responsible for the production of short-chain fatty acids (SCFAs) which impact the host’s ability to absorb and store energy from the diet (Blaak et al., 2020). Production of SCFAs, including acetate, butyrate, and propionate, occurs through fermentation of soluble dietary fiber and resistant starch by gut microbiota (den Besten et al., 2013). The SCFAs bind to the G-protein-coupled receptors GPR41 and GPR43 (Kim et al., 2018; Carretta et al., 2021; Moniri and Farah, 2021), and regulate molecular signaling pathways that indirectly affect gene expression, such as increasing the expression of glucagon-like peptide 1 (GLP-1) and peptide YY (PYY) in the gut (Tanaka et al., 2020). Both GLP-1 and PYY have been found to inhibit appetite (Stubbs et al., 2018), reduce body weight, and improve insulin resistance in obese mice (McNabney and Henagan, 2017; Blanco, 2020). However, in the absence of GPR41 signaling, PYY levels in plasma decrease, causing an increase in gut motility and a decrease in the amount of energy gained from meals (Samuel et al., 2008). Moreover, acetate has been found to positively influence appetite, insulin and ghrelin release, and obesity and its associated complications by influencing the parasympathetic neural system (Hernandez et al., 2019). On the other hand, propionate has been shown to produce insulin resistance and hyperinsulinemia, increases glucagon and fatty acid-binding protein production, activates the sympathetic nervous system, and promotes obesity and metabolic abnormalities (Tirosh et al., 2019). Therefore, further research is needed to explore the relationship between changes in the types and quantity of SCFAs and obesity as it appears that SCFAs act as mediators between diet, gut microbiota, and body physiology.
Bäckhed et al. have proposed potential pathways that contribute to the development of obesity (Backhed et al., 2005). One such pathway involves the gut microbiota promoting the absorption of monosaccharide in the gut, thereby increasing triglyceride synthesis in the liver. Furthermore, gut microbiota has been identified as the primary regulator of lipid metabolism, with both promoting and inhibitory effects. Fasting-induced adipocyte factor (FIAF, also known as PPAR-Angiopoietin Related Protein, which is a cell signal glycoprotein hormone) is known to increase adipocytes’ lipoprotein lipase (LPL) activity and fatty acid accumulation (Backhed et al., 2005). Notably, FIAF is produced by various tissues, including white adipose tissue (WAT), the colon, the liver, the heart, and the skeletal muscle (Baek et al., 2021; Montaigne et al., 2021). Studies have shown that A. muciniphila fermentation products, such as SCFAs, promote FIAF expression in gut cells through PPAR-γ (Carvalho and Saad, 2013), inhibit LPL and stimulate WAT lipolysis (Thyagarajan and Foster, 2017). In contrast, Bacteroides thetaiotaomicron can stimulate lipogenesis by inhibiting FIAF expression (Backhed et al., 2007). Therefore, FIAF may serve as a gut microbiota modulator, influencing lipid metabolism and contributing to obesity. Additionally, the endogenous cannabinoid system (EC) has been implicated in regulating blood lipid and glucose metabolism, with over-activation posing a significant risk for obesity. Specific gut microbiota, such as A. muciniphila, can interfere with fat metabolism in vivo by blocking EC-driven lipogenesis, promoting adipocyte proliferation, and increasing fat accumulation in adipocytes (Geurts et al., 2011; Forte et al., 2020; Jansma et al., 2021).
Currently, clinical approaches to treating obesity involve reducing caloric intake, increasing exercise consumption, using appetite suppressants, and gastrectomy (Blundell et al., 2017; El Moussaoui et al., 2021; Fanti et al., 2021). Nevertheless, these methods exhibit certain limitations such as limited therapeutic efficacy, drug abuse, and a high incidence of complications (Sarwer et al., 2019; Paccosi et al., 2020; Bray and Ryan, 2021). As a result, innovative treatments are necessary.
Probiotics are living strains that are considered beneficial to the host’s health when consumed in adequate amounts. These microorganisms aid in nutrient digestion and absorption, maintain the digestive system, and improve key metabolic disease risk variables such body mass index, fasting blood glucose, alanine and aspartate transaminase (Jager et al., 2018; Kijmanawat et al., 2019). Utilizing probiotics to regulate gut microbiota has emerged as a promising approach for treating obesity, particularly in cases of HFD obesity (Bianchi et al., 2018; Kong et al., 2019). Numerous animal studies and clinical trials have confirmed the efficacy of probiotics, particularly those from the Bifidobacterium and Lacto bacillusstrains, as well as some members of Bacillus and Propioni bacteriumin treating obesity and overweight by controlling gut microbiota function, bile acid metabolism, and gene expression associated with calorie homeostasis and fat formation (summarized in Supplementary Tables 1, 2). Obese animals treated with multiple Lactobacillus strains alone (Figure 3) or in combination with Bifido bacteriums trains (Figure 4) exhibited lower body weight and fat mass, improved dyslipidemia and insulin resistance, and lessened liver damage and chronic low-grade inflammation. Clinical trials using probiotics to treat obesity and overweight have also successfully observed weight loss and improved metabolic markers in subjects, probiotics’s increased presence has negative associations with obesity and diabetes while positively impacting gut health (Figure 5). Although data from current human testing studies are limited and urgently need further research and detailed documentation, intestinal bacterial transplantation has emerged in the treatment of HFD obesity and related metabolic issues following successful applications in diseases such as Clostridium difficile infection, providing a new option for the prevention and treatment of human HFD obesity.
Figure 4 Treatment of HFD obese mice with Lactobacillus strains in combination with Bifidobacterium strains.
The gut microbiota of healthy adults and children typically contains 1%-4% of the probiotic A. muciniphila (Derrien et al., 2008). A. muciniphila has special survival advantages due to its ability to utilize mucin, the primary growth and metabolic substrate produced by goblet cells in the host gastrogut tissue. Its unique structure enables A. muciniphila to modulate gut barrier integrity, enhance gut permeability, and thicken the mucus layer in HFD mice (Chelakkot et al., 2018; Liu et al., 2019). Furthermore, the Type IV pili of A. muciniphilaare able to directly signal to host immune receptors, regulate the expression of genes involved in fat synthesis and inflammation in the liver, and maintain gut immune system homeostasis (Zhou and Zhang, 2019; Kim et al., 2020; Yang et al., 2020; Xiang et al., 2021). A. muciniphila is also capable of secreting oligosaccharides and SCFA, which act as growth substrates for other beneficial bacteria and promote the abundance of microbiota associated with a reduced risk of obesity (Belzer and de Vos, 2012; Clarke et al., 2014; Keshavarz Azizi Raftar et al., 2021). Long-term supplementation with A. muciniphila can increase the thickness of the mucus layer of the gut barrier and attenuate the expression of genes and pathways associated with inflammation (Dao et al., 2016; van der Lugt et al., 2019), thus making it a promising candidate for the treatment of HFD obesity and a potential new generation of probiotics.
Recent studies have shown that there is a distinct distribution of gut bacteria in obese individuals compared to those with a normal weight. This suggests that gut microbiota may play a significant role in the development of obesity and related metabolic disorders, as it is involved in energy metabolism through processes such as acquiring energy from the diet, controlling fat storage, controlling fat creation, and controlling fatty acid oxidation. In light of these findings, new therapeutic approaches such as improving high-fat diet obesity, reducing systemic inflammation, and participating in weight control through targeting gut bacteria have been explored with some success. However, human gut microbiota is a complex research area with various influencing factors, including nutrition, exercise, medications, country, and gender. Some of these variables are beyond our control. Understanding the intricate interaction between billions of distinct bacterial populations, thousands of host cell types, and chemical mediators requires developing well-designed and suitable experimental models. Probiotics have emerged as a safe and effective option for treating HFD-induced obesity in animals, with few adverse effects and good tolerance, making them ideal for long-term administration (Liu et al., 2017), and the combination of Lactobacillus and Bifidobacterium has been shown to significantly alter gut microbiota composition and improve insulin sensitivity in HFD mice. In clinical trials, the use of synbiotic bacteria (Bifidobacterium and Lactobacillus) supplements increased the number of potential probiotics[148], however, it was discovered that the species and quantity of lactic acid bacteria were much higher in obese individuals than in the control group (Armougom et al., 2009), leading to the hypothesis that obese patients may exhibit “resistance” to lactic acid bacteria, which may due to the widespread usage of Lactobacillus as a growth stimulant in agriculture. In 2011, MetaHIT team proposed the concept of enterotypes, which divided gut microbiota into three categories: B, P and F. This has potential research and clinical value, but it is controversial. According to different tests, algorithms and analysis methods, different people think that the gut microbiota should be divided into 2, 4 enterotypes or even continuous undivided types. In order to unify the understanding and guide the practice, 29 mainstream microflora scientists in the world jointly proposed a new intestinal type classifier and open comparison database. The new scheme makes full use of and verifies the database such as HMP, comprehensively considers the function, ecology and clinical needs of the flora, and can better indicate the flora types of disease and health status, however, the consensus is significant but still limited, the treatment of obesity still cannot “model” the use of probiotics according to the existing enterotypes classification, and use of personalized probiotics based on precise analysis of each patient’s gut bacteria composition is not yet feasible.
Probiotics therapy may be a novel option for treating HDF-induced obesity, and recent research has shown that using synbiotic supplements and isolating new probiotic strains could increase the potential benefits of probiotic therapy. Nevertheless, it is important to note that a brief course of probiotics may not undo the long-term effects of a physiological disorder, and more research is required to fully understand the role of probiotics in appetite control (Liang et al., 2021).
Although intestinal bacteria transplantation has shown potential for disease prevention and treatment in both animal and human experiments, there are still great controversies over enterotypes, the selection of specific transplant strains and the combination of prebiotics. Since its establishment, microbiology has been limited by axenic culture, but the emergence of mixed culture mode opens up another way for understanding microorganisms and application development, and also has a profound impact on microbial ecology, symbiosis, pathology and other fields. The transition from pure culture to hybrid culture depends on three advances: microfluidic technology, next-generation 3D bioprinting, and single-cell metabolomics. The progress of these technologies is expected to lead to systematic large-scale symbiotic culture studies involving three or more microorganisms in the future. On the basis of in-depth understanding of the correlation between specific enterotypes and metabolic diseases, mixed culture will greatly accelerate the clinical transformation of intestinal bacteria transplantation research. As microbiota science and analytical technology continue to advance, targeted gut microbiota intervention presents potential therapeutic options towards promoting host health in the future.
SF is the first author, responsible for consulting the literature and forming the first draft, and LL is the corresponding author, responsible for revising the draft. All authors contributed to the article and approved the submitted version.
This article is funded by Innovation and entrepreneurship training program for college students of Xiamen Medical 12 College, Fujian Province (202012631050).
The authors declare that the research was conducted in the absence of any commercial or financial relationships that could be construed as a potential conflict of interest.
All claims expressed in this article are solely those of the authors and do not necessarily represent those of their affiliated organizations, or those of the publisher, the editors and the reviewers. Any product that may be evaluated in this article, or claim that may be made by its manufacturer, is not guaranteed or endorsed by the publisher.
The Supplementary Material for this article can be found online at: https://www.frontiersin.org/articles/10.3389/fcimb.2023.1139800/full#supplementary-material
Abarca-Gómez, L., Abdeen, Z. A., Hamid, Z. A., Abu-Rmeileh, N. M., Acosta-Cazares, B., Acuin, C., et al. (2017). Worldwide trends in body-mass index, underweight, overweight, and obesity from 1975 to 2016: a pooled analysis of 2416 population-based measurement studies in 128· 9 million children, adolescents, and adults. Lancet 390 (10113), 2627–2642. doi: 10.1016/S0140-6736(17)32129-3
Akagawa, S., Akagawa, Y., Yamanouchi, S., Kimata, T., Tsuji, S., Kaneko, K. (2021). Development of the gut microbiota and dysbiosis in children. Biosci. Microbio. Food Health 40 (1), 12–18. doi: 10.12938/bmfh.2020-034
Amabebe, E., Robert, F. O., Agbalalah, T., Orubu, E. S. F. (2020). Microbial dysbiosis-induced obesity: role of gut microbiota in homoeostasis of energy metabolism. Br. J. Nutr. 123 (10), 1127–1137. doi: 10.1017/S0007114520000380
An, J., Wang, Q., Yi, S., Liu, X., Jin, H., Xu, J., et al. (2022). The source of the fat significantly affects the results of high-fat diet intervention. Sci. Rep. 12 (1), 1–11. doi: 10.1038/s41598-022-08249-2
Araújo, J. R., Tomas, J., Brenner, C., Sansonetti, P. J. (2017). Impact of high-fat diet on the intestinal microbiota and small intestinal physiology before and after the onset of obesity. Biochimie 141, 97–106. doi: 10.1016/j.biochi.2017.05.019
Armougom, F., Henry, M., Vialettes, B., Raccah, D., Raoult, D. (2009). Monitoring bacterial community of human gut microbiota reveals an increase in lactobacillus in obese patients and methanogens in anorexic patients. PloS One 4 (9), e7125. doi: 10.1371/journal.pone.0007125
Assmann, T. S., Cuevas-Sierra, A., Riezu-Boj, J. I., Milagro, F. I., Martinez, J. A. (2020). Comprehensive analysis reveals novel interactions between circulating MicroRNAs and gut microbiota composition in human obesity. Int. J. Mol. Sci. 21 (24), 9509. doi: 10.3390/ijms21249509
Backhed, F., Ley, R. E., Sonnenburg, J. L., Peterson, D. A., Gordon, J. I. (2005). Host-bacterial mutualism in the human intestine. Science 307 (5717), 1915–1920. doi: 10.1126/science.1104816
Backhed, F., Manchester, J. K., Semenkovich, C. F., Gordon, J. I. (2007). Mechanisms underlying the resistance to diet-induced obesity in germ-free mice. Proc. Natl. Acad. Sci. U.S.A. 104 (3), 979–984. doi: 10.1073/pnas.0605374104
Baek, J. H., Kim, D. H., Lee, J., Kim, S. J., Chun, K. H. (2021). Galectin-1 accelerates high-fat diet-induced obesity by activation of peroxisome proliferator-activated receptor gamma (PPARgamma) in mice. Cell Death Dis. 12 (1), 66. doi: 10.1038/s41419-020-03367-z
Ballard, J. W. O., Towarnicki, S. G. (2020). Mitochondria, the gut microbiome and ROS. Cell Signal 75, 109737. doi: 10.1016/j.cellsig.2020.109737
Bartoszek, A., Makaro, A., Bartoszek, A., Kordek, R., Fichna, J., Salaga, M. (2020). Walnut oil alleviates intestinal inflammation and restores intestinal barrier function in mice. Nutrients 12 (5), 1302. doi: 10.3390/nu12051302
Belzer, C., de Vos, W. M. (2012). Microbes inside–from diversity to function: the case of akkermansia. ISME. J. 6 (8), 1449–1458. doi: 10.1038/ismej.2012.6
Bianchi, F., Larsen, N., de Mello Tieghi, T., Adorno, M. A. T., Kot, W., Saad, S. M. I., et al. (2018). Modulation of gut microbiota from obese individuals by in vitro fermentation of citrus pectin in combination with bifidobacterium longum BB-46. Appl. Microbiol. Biotechnol. 102 (20), 8827–8840. doi: 10.1007/s00253-018-9234-8
Blaak, E. E., Canfora, E. E., Theis, S., Frost, G., Groen, A. K., Mithieux, G., et al. (2020). Short chain fatty acids in human gut and metabolic health. Benef. Microbes 11 (5), 411–455. doi: 10.3920/BM2020.0057
Blanco, C. (2020). The influence of the gut microbiome on obesity. J. Am. Assoc. Nurse. Pract. 32 (7), 504–510. doi: 10.1097/JXX.0000000000000480
Blundell, J., Finlayson, G., Axelsen, M., Flint, A., Gibbons, C., Kvist, T., et al. (2017). Effects of once-weekly semaglutide on appetite, energy intake, control of eating, food preference and body weight in subjects with obesity. Diabetes Obes. Metab. 19 (9), 1242–1251. doi: 10.1111/dom.12932
Bolam, D. N., van den Berg, B. (2018). TonB-dependent transport by the gut microbiota: novel aspects of an old problem. Curr. Opin. Struct. Biol. 51, 35–43. doi: 10.1016/j.sbi.2018.03.001
Bray, G. A., Kim, K. K., Wilding, J. P. H., World Obesity, F. (2017). Obesity: a chronic relapsing progressive disease process. a position statement of the world obesity federation. Obes. Rev. 18 (7), 715–723. doi: 10.1111/obr.12551
Bray, G. A., Ryan, D. H. (2021). Evidence-based weight loss interventions: Individualized treatment options to maximize patient outcomes. Diabetes Obes. Metab. 23 Suppl 1, 50–62. doi: 10.1111/dom.14200
Capaldo, C. T., Powell, D. N., Kalman, D. (2017). Layered defense: how mucus and tight junctions seal the intestinal barrier. J. Mol. Med. (Berl). 95 (9), 927–934. doi: 10.1007/s00109-017-1557-x
Carretta, M. D., Quiroga, J., Lopez, R., Hidalgo, M. A., Burgos, R. A. (2021). Participation of short-chain fatty acids and their receptors in gut inflammation and colon cancer. Front. Physiol. 12. doi: 10.3389/fphys.2021.662739
Carvalho, B. M., Saad, M. J. (2013). Influence of gut microbiota on subclinical inflammation and insulin resistance. Mediators Inflammation 2013, 986734. doi: 10.1155/2013/986734
Chelakkot, C., Choi, Y., Kim, D. K., Park, H. T., Ghim, J., Kwon, Y., et al. (2018). Akkermansia muciniphila-derived extracellular vesicles influence gut permeability through the regulation of tight junctions. Exp. Mol. Med. 50 (2), e450. doi: 10.1038/emm.2017.282
Chen, S., Zuo, S., Zhu, J., Yue, T., Bu, D., Wang, X., et al. (2019). Decreased expression of cystathionine beta-synthase exacerbates intestinal barrier injury in ulcerative colitis. J. Crohns. Colitis. 13 (8), 1067–1080. doi: 10.1093/ecco-jcc/jjz027
Clarke, S. F., Murphy, E. F., O'Sullivan, O., Lucey, A. J., Humphreys, M., Hogan, A., et al. (2014). Exercise and associated dietary extremes impact on gut microbial diversity. Gut 63 (12), 1913–1920. doi: 10.1136/gutjnl-2013-306541
Costea, P. I., Hildebrand, F., Arumugam, M., Backhed, F., Blaser, M. J., Bushman, F. D., et al. (2018). Publisher correction: Enterotypes in the landscape of gut microbial community composition. Nat. Microbiol. 3 (3), 388. doi: 10.1038/s41564-018-0114-x
Cuevas-Sierra, A., Ramos-Lopez, O., Riezu-Boj, J. I., Milagro, F. I., Martinez, J. A. (2019). Diet, gut microbiota, and obesity: Links with host genetics and epigenetics and potential applications. Adv. Nutr. 10 (suppl_1), S17–S30. doi: 10.1093/advances/nmy078
Dao, M. C., Everard, A., Aron-Wisnewsky, J., Sokolovska, N., Prifti, E., Verger, E. O., et al. (2016). Akkermansia muciniphila and improved metabolic health during a dietary intervention in obesity: relationship with gut microbiome richness and ecology. Gut 65 (3), 426–436. doi: 10.1136/gutjnl-2014-308778
David, L. A., Maurice, C. F., Carmody, R. N., Gootenberg, D. B., Button, J. E., Wolfe, B. E., et al. (2014). Diet rapidly and reproducibly alters the human gut microbiome. Nature 505 (7484), 559–563. doi: 10.1038/nature12820
den Besten, G., van Eunen, K., Groen, A. K., Venema, K., Reijngoud, D. J., Bakker, B. M. (2013). The role of short-chain fatty acids in the interplay between diet, gut microbiota, and host energy metabolism. J. Lipid Res. 54 (9), 2325–2340. doi: 10.1194/jlr.R036012
Derrien, M., Alvarez, A. S., de Vos, W. M. (2019). The gut microbiota in the first decade of life. Trends Microbiol. 27 (12), 997–1010. doi: 10.1016/j.tim.2019.08.001
Derrien, M., Collado, M. C., Ben-Amor, K., Salminen, S., de Vos, W. M. (2008). The mucin degrader akkermansia muciniphila is an abundant resident of the human intestinal tract. Appl. Environ. Microbiol. 74 (5), 1646–1648. doi: 10.1128/AEM.01226-07
Di Ciaula, A., Wang, D. Q., Molina-Molina, E., Lunardi Baccetto, R., Calamita, G., Palmieri, V. O., et al. (2017). Bile acids and cancer: Direct and environmental-dependent effects. Ann. Hepatol. 16 Suppl 1, S87–S105. doi: 10.5604/01.3001.0010.5501
Dominguez-Bello, M. G., Godoy-Vitorino, F., Knight, R., Blaser, M. J. (2019). Role of the microbiome in human development. Gut 68 (6), 1108–1114. doi: 10.1136/gutjnl-2018-317503
El Moussaoui, I., Van Vyve, E., Johanet, H., Dabrowski, A., Piquard, A., Delaunay, T., et al. (2021). Laparoscopic sleeve gastrectomy for morbid obesity in a Belgian-French prospective multicenter study: outcomes and predictors weight loss failure. Acta Chir. Belg. 121 (6), 413–419. doi: 10.1080/00015458.2020.1841485
Fanti, M., Mishra, A., Longo, V. D., Brandhorst, S. (2021). Time-restricted eating, intermittent fasting, and fasting-mimicking diets in weight loss. Curr. Obes. Rep. 10 (2), 70–80. doi: 10.1007/s13679-021-00424-2
Forte, N., Fernandez-Rilo, A. C., Palomba, L., Di Marzo, V., Cristino, L. (2020). Obesity affects the microbiota-Gut-Brain axis and the regulation thereof by endocannabinoids and related mediators. Int. J. Mol. Sci. 21 (5), 1554. doi: 10.3390/ijms21051554
Geurts, L., Lazarevic, V., Derrien, M., Everard, A., Van Roye, M., Knauf, C., et al. (2011). Altered gut microbiota and endocannabinoid system tone in obese and diabetic leptin-resistant mice: impact on apelin regulation in adipose tissue. Front. Microbiol. 2. doi: 10.3389/fmicb.2011.00149
Gill, S. R., Pop, M., Deboy, R. T., Eckburg, P. B., Turnbaugh, P. J., Samuel, B. S., et al. (2006). Metagenomic analysis of the human distal gut microbiome. Science 312 (5778), 1355–1359. doi: 10.1126/science.1124234
Giordano, N. P., Cian, M. B., Dalebroux, Z. D. (2020). Outer membrane lipid secretion and the innate immune response to gram-negative bacteria. Infect. Immun. 88 (7), e00920–19. doi: 10.1128/IAI.00920-19
Gupta, B., Liu, Y., Chopyk, D. M., Rai, R. P., Desai, C., Kumar, P., et al. (2020). Western Diet-induced increase in colonic bile acids compromises epithelial barrier in nonalcoholic steatohepatitis. FASEB J. 34 (5), 7089–7102. doi: 10.1096/fj.201902687R
Hanninen, A., Toivonen, R., Poysti, S., Belzer, C., Plovier, H., Ouwerkerk, J. P., et al. (2018). Akkermansia muciniphila induces gut microbiota remodelling and controls islet autoimmunity in NOD mice. Gut 67 (8), 1445–1453. doi: 10.1136/gutjnl-2017-314508
Hernandez, M. A. G., Canfora, E. E., Jocken, J. W. E., Blaak, E. E. (2019). The short-chain fatty acid acetate in body weight control and insulin sensitivity. Nutrients 11 (8), 1943. doi: 10.3390/nu11081943
Iwamoto, J., Honda, A., Miyazaki, T., Monma, T., Ueda, H., Morishita, Y., et al. (2021). Western Diet changes gut microbiota and ameliorates liver injury in a mouse model with human-like bile acid composition. Hepatol. Commun. 5 (12), 2052–2067. doi: 10.1002/hep4.1778
Jager, R., Purpura, M., Farmer, S., Cash, H. A., Keller, D. (2018). Probiotic bacillus coagulans GBI-30, 6086 improves protein absorption and utilization. Probio. Antimicrob. Proteins 10 (4), 611–615. doi: 10.1007/s12602-017-9354-y
Jansma, J., Brinkman, F., van Hemert, S., El Aidy, S. (2021). Targeting the endocannabinoid system with microbial interventions to improve gut integrity. Prog. Neuropsychopharmacol. Biol. Psychiatry 106, 110169. doi: 10.1016/j.pnpbp.2020.110169
Jiang, C., Zhang, S., Li, D., Chen, L., Zhao, Y., Mei, G., et al. (2020). Impaired ferritinophagy flux induced by high fat diet mediates hepatic insulin resistance via endoplasmic reticulum stress. Food Chem. Toxicol. 140, 111329. doi: 10.1016/j.fct.2020.111329
Kapoor, N., Kota, S., Kalra, S. (2021). Obesity a communicable disease - a new age paradigm. J. Pak. Med. Assoc. 71 (8), 2100–2102.
Kasselman, L. J., Vernice, N. A., DeLeon, J., Reiss, A. B. (2018). The gut microbiome and elevated cardiovascular risk in obesity and autoimmunity. Atherosclerosis 271, 203–213. doi: 10.1016/j.atherosclerosis.2018.02.036
Keshavarz Azizi Raftar, S., Ashrafian, F., Yadegar, A., Lari, A., Moradi, H. R., Shahriary, A., et al. (2021). The protective effects of live and pasteurized akkermansia muciniphila and its extracellular vesicles against HFD/CCl4-induced liver injury. Microbiol. Spectr. 9 (2), e0048421. doi: 10.1128/Spectrum.00484-21
Kijmanawat, A., Panburana, P., Reutrakul, S., Tangshewinsirikul, C. (2019). Effects of probiotic supplements on insulin resistance in gestational diabetes mellitus: A double-blind randomized controlled trial. J. Diabetes Investig. 10 (1), 163–170. doi: 10.1111/jdi.12863
Kim, S., Choi, S., Dutta, M., Asubonteng, J. O., Polunas, M., Goedken, M., et al. (2021). Pregnane X receptor exacerbates nonalcoholic fatty liver disease accompanied by obesity- and inflammation-prone gut microbiome signature. Biochem. Pharmacol. 193, 114698. doi: 10.1016/j.bcp.2021.114698
Kim, M., Friesen, L., Park, J., Kim, H. M., Kim, C. H. (2018). Microbial metabolites, short-chain fatty acids, restrain tissue bacterial load, chronic inflammation, and associated cancer in the colon of mice. Eur. J. Immunol. 48 (7), 1235–1247. doi: 10.1002/eji.201747122
Kim, S., Lee, Y., Kim, Y., Seo, Y., Lee, H., Ha, J., et al. (2020). Akkermansia muciniphila prevents fatty liver disease, decreases serum triglycerides, and maintains gut homeostasis. Appl. Environ. Microbiol. 86 (7), e03004–19. doi: 10.1128/AEM.03004-19
Kong, C., Gao, R., Yan, X., Huang, L., Qin, H. (2019). Probiotics improve gut microbiota dysbiosis in obese mice fed a high-fat or high-sucrose diet. Nutrition 60, 175–184. doi: 10.1016/j.nut.2018.10.002
Kumar, A., Sundaram, K., Mu, J., Dryden, G. W., Sriwastva, M. K., Lei, C., et al. (2021). High-fat diet-induced upregulation of exosomal phosphatidylcholine contributes to insulin resistance. Nat. Commun. 12 (1), 213. doi: 10.1038/s41467-020-20500-w
Lee, J.-Y., Cevallos, S. A., Byndloss, M. X., Tiffany, C. R., Olsan, E. E., Butler, B. P., et al. (2020). High-fat diet and antibiotics cooperatively impair mitochondrial bioenergetics to trigger dysbiosis that exacerbates pre-inflammatory bowel disease. Cell Host Microbe 28 (2), 273–284.e276. doi: 10.1016/j.chom.2020.06.001
Lemons, J. M. S., Liu, L. (2022). Chewing the fat with microbes: Lipid crosstalk in the gut. Nutrients 14 (3), 573. doi: 10.3390/nu14030573
Liang, C., Zhou, X. H., Jiao, Y. H., Guo, M. J., Meng, L., Gong, P. M., et al. (2021). Ligilactobacillus salivarius LCK11 prevents obesity by promoting PYY secretion to inhibit appetite and regulating gut microbiota in C57BL/6J mice. Mol. Nutr. Food Res. 65 (17), e2100136. doi: 10.1002/mnfr.202100136
Liu, R., Hong, J., Xu, X., Feng, Q., Zhang, D., Gu, Y., et al. (2017). Gut microbiome and serum metabolome alterations in obesity and after weight-loss intervention. Nat. Med. 23 (7), 859–868. doi: 10.1038/nm.4358
Liu, H. Y., Walden, T. B., Ahl, D., Nyman, M., Bertilsson, S., Phillipson, M., et al. (2019). High-fat diet enriched with bilberry modifies colonic mucus dynamics and restores marked alterations of gut microbiome in rats. Mol. Nutr. Food Res. 63 (20), e1900117. doi: 10.1002/mnfr.201900117
Mariamenatu, A. H., Abdu, E. M. (2021). Overconsumption of omega-6 polyunsaturated fatty acids (PUFAs) versus deficiency of omega-3 PUFAs in modern-day diets: The disturbing factor for their "Balanced antagonistic metabolic functions" in the human body. J. Lipids 2021, 8848161. doi: 10.1155/2021/8848161
McNabney, S. M., Henagan, T. M. (2017). Short chain fatty acids in the colon and peripheral tissues: A focus on butyrate, colon cancer, obesity and insulin resistance. Nutrients 9 (12), 1348. doi: 10.3390/nu9121348
Mohammad, S., Thiemermann, C. (2020). Role of metabolic endotoxemia in systemic inflammation and potential interventions. Front. Immunol. 11. doi: 10.3389/fimmu.2020.594150
Moniri, N. H., Farah, Q. (2021). Short-chain free-fatty acid G protein-coupled receptors in colon cancer. Biochem. Pharmacol. 186, 114483. doi: 10.1016/j.bcp.2021.114483
Monk, J. M., Wu, W., Lepp, D., Wellings, H. R., Hutchinson, A. L., Liddle, D. M., et al. (2019). Navy bean supplemented high-fat diet improves intestinal health, epithelial barrier integrity and critical aspects of the obese inflammatory phenotype. J. Nutr. Biochem. 70, 91–104. doi: 10.1016/j.jnutbio.2019.04.009
Montaigne, D., Butruille, L., Staels, B. (2021). PPAR control of metabolism and cardiovascular functions. Nat. Rev. Cardiol. 18 (12), 809–823. doi: 10.1038/s41569-021-00569-6
Mottawea, W., Chiang, C. K., Muhlbauer, M., Starr, A. E., Butcher, J., Abujamel, T., et al. (2016). Altered intestinal microbiota-host mitochondria crosstalk in new onset crohn's disease. Nat. Commun. 7, 13419. doi: 10.1038/ncomms13419
Nascimento, J. C., Matheus, V. A., Oliveira, R. B., Tada, S. F. S., Collares-Buzato, C. B. (2021). High-fat diet induces disruption of the tight junction-mediated paracellular barrier in the proximal small intestine before the onset of type 2 diabetes and endotoxemia. Dig. Dis. Sci. 66 (10), 3359–3374. doi: 10.1007/s10620-020-06664-x
Netto Candido, T. L., Bressan, J., Alfenas, R. C. G. (2018). Dysbiosis and metabolic endotoxemia induced by high-fat diet. Nutr. Hosp. 35 (6), 1432–1440. doi: 10.20960/nh.1792
Obesity: preventing and managing the global epidemic. (2000) Report of a WHO consultation. World Health Organization technical report series, 849, i–253.
Ocvirk, S., O'Keefe, S. J. D. (2021). Dietary fat, bile acid metabolism and colorectal cancer. Semin. Cancer Biol. 73, 347–355. doi: 10.1016/j.semcancer.2020.10.003
Oliveira, R. B., Canuto, L. P., Collares-Buzato, C. B. (2019). Intestinal luminal content from high-fat-fed prediabetic mice changes epithelial barrier function in vitro. Life Sci. 216, 10–21. doi: 10.1016/j.lfs.2018.11.012
Otani, T., Furuse, M. (2020). Tight junction structure and function revisited: (Trends in cell biology 30, 805-817, 2020). Trends Cell Biol. 30 (12), 1014. doi: 10.1016/j.tcb.2020.10.001
Paccosi, S., Cresci, B., Pala, L., Rotella, C. M., Parenti, A. (2020). Obesity therapy: How and why? Curr. Med. Chem. 27 (2), 174–186. doi: 10.2174/0929867326666190124121725
Qin, N., Song, G., Ren, X., Zhang, L., Gao, J., Xia, X., et al. (2020). Fish oil extracted from coregonus peled improves obese phenotype and changes gut microbiota in a high-fat diet-induced mouse model of recurrent obesity. Food Funct. 11 (7), 6158–6169. doi: 10.1039/d0fo00911c
Raimondi, F., Santoro, P., Barone, M. V., Pappacoda, S., Barretta, M. L., Nanayakkara, M., et al. (2008). Bile acids modulate tight junction structure and barrier function of caco-2 monolayers via EGFR activation. Am. J. Physiol. Gastroint. Liver. Physiol. 294 (4), G906–G913. doi: 10.1152/ajpgi.00043.2007
Ridaura, V. K., Faith, J. J., Rey, F. E., Cheng, J., Duncan, A. E., Kau, A. L., et al. (2013). Gut microbiota from twins discordant for obesity modulate metabolism in mice. Science 341 (6150), 1241214. doi: 10.1126/science.1241214
Robertson, R. C., Manges, A. R., Finlay, B. B., Prendergast, A. J. (2019). The human microbiome and child growth - first 1000 days and beyond. Trends Microbiol. 27 (2), 131–147. doi: 10.1016/j.tim.2018.09.008
Rohr, M. W., Narasimhulu, C. A., Rudeski-Rohr, T. A., Parthasarathy, S. (2020). Negative effects of a high-fat diet on intestinal permeability: A review. Adv. Nutr. 11 (1), 77–91. doi: 10.1093/advances/nmz061
Romani-Perez, M., Bullich-Vilarrubias, C., Lopez-Almela, I., Liebana-Garcia, R., Olivares, M., Sanz, Y. (2021). The microbiota and the gut-brain axis in controlling food intake and energy homeostasis. Int. J. Mol. Sci. 22 (11), 5830. doi: 10.3390/ijms22115830
Samuel, B. S., Shaito, A., Motoike, T., Rey, F. E., Backhed, F., Manchester, J. K., et al. (2008). Effects of the gut microbiota on host adiposity are modulated by the short-chain fatty-acid binding G protein-coupled receptor, Gpr41. Proc. Natl. Acad. Sci. U.S.A. 105 (43), 16767–16772. doi: 10.1073/pnas.0808567105
Sarathy, J., Detloff, S. J., Ao, M., Khan, N., French, S., Sirajuddin, H., et al. (2017). The yin and yang of bile acid action on tight junctions in a model colonic epithelium. Physiol. Rep. 5 (10), e13294. doi: 10.14814/phy2.13294
Sarwer, D. B., Allison, K. C., Wadden, T. A., Ashare, R., Spitzer, J. C., McCuen-Wurst, C., et al. (2019). Psychopathology, disordered eating, and impulsivity as predictors of outcomes of bariatric surgery. Surg. Obes. Relat. Dis. 15 (4), 650–655. doi: 10.1016/j.soard.2019.01.029
Sebastian Domingo, J. J., Sanchez Sanchez, C. (2018). From the intestinal flora to the microbiome. Rev. Esp. Enferm. Dig. 110 (1), 51–56. doi: 10.17235/reed.2017.4947/2017
Sommer, F., Backhed, F. (2016). Know your neighbor: Microbiota and host epithelial cells interact locally to control intestinal function and physiology. Bioessays 38 (5), 455–464. doi: 10.1002/bies.201500151
Spinelli, J. B., Haigis, M. C. (2018). The multifaceted contributions of mitochondria to cellular metabolism. Nat. Cell Biol. 20 (7), 745–754. doi: 10.1038/s41556-018-0124-1
Stanislawski, M. A., Dabelea, D., Lange, L. A., Wagner, B. D., Lozupone, C. A. (2019). Gut microbiota phenotypes of obesity. NPJ Biofilms. Microbiomes. 5 (1), 18. doi: 10.1038/s41522-019-0091-8
Stoeva, M. K., Garcia-So, J., Justice, N., Myers, J., Tyagi, S., Nemchek, M., et al. (2021). Butyrate-producing human gut symbiont, clostridium butyricum, and its role in health and disease. Gut. Microbes 13 (1), 1–28. doi: 10.1080/19490976.2021.1907272
Stubbs, B. J., Cox, P. J., Evans, R. D., Cyranka, M., Clarke, K., de Wet, H. (2018). A ketone ester drink lowers human ghrelin and appetite. Obes. (Silver. Spring). 26 (2), 269–273. doi: 10.1002/oby.22051
Tanaka, S., Nemoto, Y., Takei, Y., Morikawa, R., Oshima, S., Nagaishi, T., et al. (2020). High-fat diet-derived free fatty acids impair the intestinal immune system and increase sensitivity to intestinal epithelial damage. Biochem. Biophys. Res. Commun. 522 (4), 971–977. doi: 10.1016/j.bbrc.2019.11.158
Theis, K. R., Romero, R., Winters, A. D., Greenberg, J. M., Gomez-Lopez, N., Alhousseini, A., et al. (2019). Does the human placenta delivered at term have a microbiota? results of cultivation, quantitative real-time PCR, 16S rRNA gene sequencing, and metagenomics. Am. J. obstet. gynecol. 220(3), 267.e261–267.e239 doi: 10.1016/j.ajog.2018.10.018
Thyagarajan, B., Foster, M. T. (2017). Beiging of white adipose tissue as a therapeutic strategy for weight loss in humans. Horm. Mol. Biol. Clin. Investig. 31 (2). doi: 10.1515/hmbci-2017-0016
Tirosh, A., Calay, E. S., Tuncman, G., Claiborn, K. C., Inouye, K. E., Eguchi, K., et al. (2019). The short-chain fatty acid propionate increases glucagon and FABP4 production, impairing insulin action in mice and humans. Sci. Transl. Med. 11 (489), eaav0120. doi: 10.1126/scitranslmed.aav0120
Tsukita, K., Yano, T., Tamura, A., Tsukita, S. (2019). Reciprocal association between the apical junctional complex and AMPK: A promising therapeutic target for Epithelial/Endothelial barrier function? Int. J. Mol. Sci. 20 (23), 6012. doi: 10.3390/ijms20236012
Turnbaugh, P. J., Ridaura, V. K., Faith, J. J., Rey, F. E., Knight, R., Gordon, J. I. (2009). The effect of diet on the human gut microbiome: a metagenomic analysis in humanized gnotobiotic mice. Sci. Transl. Med. 1 (6), 6ra14. doi: 10.1126/scitranslmed.3000322
Usami, M., Komurasaki, T., Hanada, A., Kinoshita, K., Ohata, A. (2003). Effect of gamma-linolenic acid or docosahexaenoic acid on tight junction permeability in intestinal monolayer cells and their mechanism by protein kinase c activation and/or eicosanoid formation. Nutrition 19 (2), 150–156. doi: 10.1016/s0899-9007(02)00927-9
Usami, M., Muraki, K., Iwamoto, M., Ohata, A., Matsushita, E., Miki, A. (2001). Effect of eicosapentaenoic acid (EPA) on tight junction permeability in intestinal monolayer cells. Clin. Nutr. 20 (4), 351–359. doi: 10.1054/clnu.2001.0430
van der Lugt, B., van Beek, A. A., Aalvink, S., Meijer, B., Sovran, B., Vermeij, W. P., et al. (2019). Akkermansia muciniphila ameliorates the age-related decline in colonic mucus thickness and attenuates immune activation in accelerated aging Ercc1 (-/Delta7) mice. Immun. Ageing 16, 6. doi: 10.1186/s12979-019-0145-z
Wan, Y., Wang, F., Yuan, J., Li, J., Jiang, D., Zhang, J., et al. (2019). Effects of dietary fat on gut microbiota and faecal metabolites, and their relationship with cardiometabolic risk factors: a 6-month randomised controlled-feeding trial. Gut 68 (8), 1417–1429. doi: 10.1136/gutjnl-2018-317609
Wu, X., Wei, Q., Wang, X., Shang, Y., Zhang, H. (2022). Evolutionary and dietary relationships of wild mammals based on the gut microbiome. Gene 808, 145999. doi: 10.1016/j.gene.2021.145999
Xiang, R., Wang, J., Xu, W., Zhang, M., Wang, M. (2021). Amuc_1102 from akkermansia muciniphila adopts an immunoglobulin-like fold related to archaeal type IV pilus. Biochem. Biophys. Res. Commun. 547, 59–64. doi: 10.1016/j.bbrc.2021.02.022
Yang, M., Bose, S., Lim, S., Seo, J., Shin, J., Lee, D., et al. (2020). Beneficial effects of newly isolated akkermansia muciniphila strains from the human gut on obesity and metabolic dysregulation. Microorganisms 8 (9), 1413. doi: 10.3390/microorganisms8091413
Zeng, Q., Li, D., He, Y., Li, Y., Yang, Z., Zhao, X., et al. (2019). Discrepant gut microbiota markers for the classification of obesity-related metabolic abnormalities. Sci. Rep. 9 (1), 13424. doi: 10.1038/s41598-019-49462-w
Zhang, H.-l., Wu, Q.-x., Qin, X.-m. (2019a). Camellia nitidissima chi flower extracts inhibit α-amylase and α-glucosidase: In vitro by analysis of optimization of addition methods, inhibitory kinetics and mechanisms. Process. Biochem. 86, 177–185. doi: 10.1016/j.procbio.2019.07.009
Zhang, P., Yu, Y., Qin, Y., Zhou, Y., Tang, R., Wang, Q., et al. (2019b). Alterations to the microbiota-colon-brain axis in high-fat-diet-induced obese mice compared to diet-resistant mice. J. Nutr. Biochem. 65, 54–65. doi: 10.1016/j.jnutbio.2018.08.016
Keywords: gut microbiota, high-fat diet, obesity, probiotics, treatment
Citation: Fan S, Chen S and Lin L (2023) Research progress of gut microbiota and obesity caused by high-fat diet. Front. Cell. Infect. Microbiol. 13:1139800. doi: 10.3389/fcimb.2023.1139800
Received: 07 January 2023; Accepted: 28 February 2023;
Published: 13 March 2023.
Edited by:
Xiangtian Yu, Shanghai Jiao Tong University, ChinaReviewed by:
Zhangran Chen, Xiamen University, ChinaCopyright © 2023 Fan, Chen and Lin. This is an open-access article distributed under the terms of the Creative Commons Attribution License (CC BY). The use, distribution or reproduction in other forums is permitted, provided the original author(s) and the copyright owner(s) are credited and that the original publication in this journal is cited, in accordance with accepted academic practice. No use, distribution or reproduction is permitted which does not comply with these terms.
*Correspondence: Lin Lin, MTU1MDc3MDczMkBxcS5jb20=
Disclaimer: All claims expressed in this article are solely those of the authors and do not necessarily represent those of their affiliated organizations, or those of the publisher, the editors and the reviewers. Any product that may be evaluated in this article or claim that may be made by its manufacturer is not guaranteed or endorsed by the publisher.
Research integrity at Frontiers
Learn more about the work of our research integrity team to safeguard the quality of each article we publish.