- 1Division of Infectious Diseases, Rhode Island Hospital, The Miriam Hospital, Alpert Medical School and Brown University, Providence, RI, United States
- 2Center for Biomedical Engineering, School of Engineering, Institute for Molecular and Nanoscale Innovation, Brown University, Providence, RI, United States
Microbe entry through catheter ports can lead to biofilm accumulation and complications from catheter-related bloodstream infection and ultimately require antimicrobial treatment and catheter replacement. Although strides have been made with microbial prevention by applying standardized antiseptic techniques during catheter implantation, both bacterial and fungal microbes can present health risks to already sick individuals. To reduce microbial adhesion, murine and human catheters were coated with polyurethane and auranofin using a dip coating method and compared to non-coated materials. Upon passage of fluid through the coated material in vitro, flow dynamics were not impacted. The unique antimicrobial properties of the coating material auranofin has shown inhibitory activity against bacteria such as Staphylococcus aureus and fungi such as Candida albicans. Auranofin coating on catheters at 10mg/mL reduced C. albicans accumulation in vitro from 2.0 x 108 to 7.8 x 105 CFU for mouse catheters and from 1.6 x 107 to 2.8 x 106 for human catheters, showing an impact to mature biofilms. Assessment of a dual microbe biofilm on auranofin-coated catheters resulted in a 2-log reduction in S. aureus and a 3-log reduction in C. albicans compared to uncoated catheters. In vivo assessment in a murine subcutaneous model demonstrated that catheters coated with 10 mg/mL auranofin reduced independent S. aureus and C. albicans accumulation by 4-log and 1-log, respectively, compared to non-coated catheters. In conclusion, the auranofin-coated catheters demonstrate proficiency at inhibiting multiple pathogens by decreasing S. aureus and C. albicans biofilm accumulation.
Introduction
Medical devices provide significant advances to improve human health but present an opportunity for infection by creating a portal for microbes to enter the patient’s bloodstream and potentially compromise recovery and health (Feneley et al., 2015). In the United States, about 150 million intravascular catheters are implanted yearly to support patients with renal failure, monitor hemodynamics, administer medications, and provide nutritional support (Shah et al., 2013). However, 250,000 catheter-related bloodstream infections (CRBIs) have been associated with intravascular catheters (Marik et al., 2012; Mccoy et al., 2017). In emergency rooms and intensive care units, central venous catheter insertion is associated with 5.3 bloodstream infections per thousand days (Gahlot et al., 2014). In addition to bloodstream infections, catheters present urinary tract infection vulnerability, for which catheter-associated urinary tract infections (CAUTI) account for approximately 40% of device-related infections in hospitalized patients (Feneley et al., 2015). Among urinary tract infections acquired in the hospital, 75% are catheter-related (CDC, 2015). During antineoplastic chemotherapy infusion, catheters provide a portal to bacteria and fungi by subverting the natural skin that serves as a barrier and essential defense mechanism, creating a niche for microbe adhesion and colonization of microbes derived from the skin microbiome or the external environment (Douglas, 2003; Neugent et al., 2020).
In most cases, CRBI and CAUTI are caused by pathogens that form biofilms (Lynch and Robertson, 2008), which are the complex structure of microorganisms that contains proteins, nucleic acids, and polysaccharides. Compared to planktonic cells, biofilms are more resistant to conventional antimicrobials through their recalcitrant nature and employment of efflux pumps (Gilbert et al., 2002). As a result of biofilm formation, bacteria (e.g., Staphylococcus aureus, Staphylococcus epidermidis, and Pseudomonas aeruginosa) and fungi (e.g., Candida albicans) can cause infections, such as bloodstream infections that are challenging to treat (Chandra et al., 2001; Donlan, 2001; Mah and O’toole, 2001; Yousif et al., 2015; Brindhadevi et al., 2020; Wolcott, 2021).
Among catheter-related microbial infections, S. aureus and C. albicans are the most common catheter-dwelling, biofilm-forming bacterial and fungal pathogens, respectively (Esposito et al., 2013; Laverty et al., 2015; Kohse et al., 2019). Strenuous efforts have been made to reduce the number of catheter-related infections by employing stringent local sterilization and catheter implant procedures (O’grady et al., 2002; Panepinto et al., 2021; Selby et al., 2021) however, infections still occur. Central venous catheters are risk factors for acquiring C. albicans catheter-related bloodstream infections (CRBI), especially among immunocompromised patients. Catheter-related fungemia can be associated with parenteral nutrition, central lines, and other implanted catheters. Infection rates can vary between the global region and even hospitals. Overall, C. albicans as well as other Candida species, pose a risk of infection. Despite best practices, the skin microbiome often serves as the inoculum reservoir. In the UK, CRBI accounts for 10 to 20% of hospital-acquired infections (Gahlot et al., 2014). A review by Gahlot and colleagues finds Candida species as the infecting agents in 11.7 to 16% of central venous catheters (Gahlot et al., 2014). In a single-site study from a pediatric intensive care unit in Turkey, C. albicans accounted for 16% of the central line Candida infections (236 in total) over a 11-year span (2009-2019) (Devrim et al., 2022).
Surface modification and/or coating catheters with bioactive molecules (peptides, small antimicrobial molecules) provide means to prevent the colonization of S. aureus and C. albicans under in vitro and in vivo conditions (Li et al., 2014; Francolini et al., 2017; Liu et al., 2019b; Pathak et al., 2019; Balaure and Grumezescu, 2020). Application of bioactive molecules like antimicrobial agents, small molecules, metallic nanoparticles, enzymes, or antiseptic agents helps to slow the release of the bioactive molecule causing bacterial death and preventing biofilm formation (Stenger et al., 2016; Vaterrodt et al., 2016; Lewisoscar et al., 2018; She et al., 2020; Lewisoscar et al., 2021).
In the present study, auranofin, a Food and Drug Administration (FDA)-approved antirheumatic drug that exhibits antibacterial activity against Gram-positive bacteria and some fungi, was selected to coat catheters (Fuchs et al., 2016; Liu et al., 2019a; She et al., 2020; Felix et al., 2021). Auranofin shows antibacterial activity against Gram-positive bacteria (Bacillus subtilis, Enterococcus faecalis, Enterococcus faecium, Mycobacterium tuberculosis, and S. aureus) and fungi like C. albicans, Candida glabrata, Candida tropicalis, Candida neoformans, Cryptococcus gattii (Siles et al., 2013; Fuchs et al., 2016; Torres et al., 2016; Thangamani et al., 2017; Wiederhold et al., 2017; She et al., 2020; Felix et al., 2021). Auranofin exhibits antibacterial activity by inhibiting thioredoxin reductase, which protects against reactive oxygen species (May and Yu, 2018; Liu et al., 2019b). Disruption of the bacterial thioredoxin reductase and redox balance causes bacterial cell death.
In our previous study, we coated catheters with polyurethane (PU) and auranofin and tested the efficacy and stability of the coating for antibacterial and antibiofilm activity against S. aureus in vitro (Liu et al., 2019b). Expanding on this previous study, we analyzed the flow dynamics of auranofin-coated catheters. Additionally, since auranofin has a unique capacity to inhibit bacterial and fungal pathogens (She et al., 2020), auranofin-coated catheter resistance potential was evaluated for both S. aureus and C. albicans using a murine subcutaneous model.
Methods
PU catheter coating
Using the methods previously published by (Liu et al., 2019a), catheters were coated with antimicrobial agents. In brief, mouse and human catheters were coated with auranofin (3 mg/mL and 10 mg/mL), fluconazole (10 mg/mL), and vancomycin (15 mg/mL). Negative control catheters were coated with THF + PU alone, absent any antimicrobial agents. Human (14G x 2”, Exel Safelite Catheters, 26726, Radiopaque teflon material) and mouse (14G x 2”, Surflo I.V. Catheters, SR-OX1451CA, Polyurethane material) catheters were cut into 1 cm segments using a sharp scalpel and submerged in a 20 mL scintillation vial containing 3 mL of THF + PU + auranofin/fluconazole/vancomycin. The setup was placed on a shaker overnight at room temperature. Post incubation, catheters were air-dried in a fume hood overnight and then stored at 4°C until further use.
Fluid flow dynamics with antimicrobial coated catheters
The fluid flow rate of auranofin-coated and uncoated human and mouse catheters was evaluated using a syringe pump (Harvard Apparatus, Holliston, MA). Assessment entailed providing a constant flow of liquid (phosphate buffered saline (PBS)) and measuring the capture volume.
For both human and mouse catheters, assessment groups included: untreated catheters, THF-coated catheters, PU dissolved in THF catheters, catheters coated with auranofin (3 and 10 mg) suspended in THF + PU coating, vancomycin (15 mg) suspended in THF + PU coating, and fluconazole (10 mg) suspended in THF + PU coating. The flow of PBS through human and mouse catheters was determined by keeping the volume of liquid constant. A 20 mL syringe was filled with 20 mL (the syringe was filled 3 times) of PBS and connected to a catheter adapter that married the catheters to the syringe tip, connecting the devices to the syringe pump (Supplemental figure 1), which was then set to run at a flow rate of 1 mL/min for 10 min at a 50% force level to assess the final volume captured during a fixed period.
By contrast, to quantify the time needed to collect a fixed volume of liquid, a syringe was filled with PBS, locked into the syringe pump, and run for 10 minutes. The flow rate was set at 1 mL/min with 50% force. The volume of PBS collected was measured via an analytical scale and confirmed by measuring volume in a graduated tube.
Murine subcutaneous implant model to measure Staphylococcus aureus biofilm accumulation
Female BALB/c mice (n=5/group) were used according to the protocol approved by the Institutional Animal Care and Use Committee. The 1 cm drug-coated catheters were put inside culture tubes containing luminescent S. aureus USA300 (Dai et al., 2013) logarithmic culture and incubated for 2 h with agitation. To implant, the catheters, BALB/c female mice (Charles River, Wilmington, MA.) were anesthetized via intraperitoneal (IP) injection with ketamine (100 mg/kg) and xylazine (10 mg/kg). Mouse flanks were shaved, and the skin was sterilized topically using betadine and isopropyl alcohol. A 5 mm skin incision was made using sterile scissors to create a subcutaneous tunnel for the 1 cm catheter previously seeded with bacteria and the incision site was then closed with surgical staples. Two procedures were performed per animal with placement of two catheters per animal, one on each flank.
Bacterial colonization was analyzed using the live animal imaging system (IVIS Lumina, Series III; Perkin Elmer, Waltham, MA, USA) at various time points following inoculation: 24, 48, and 72 h post insertion. Total photon emission from defined regions of interest within the images of each mouse was quantified using Living Image software (Perkin Elmer, Waltham, MA). After the final imaging time, mice were sacrificed, the catheters were surgically removed, sonicated in 1 mL tryptic soy broth (TSB), and the bacterial load was enumerated after serial dilution and plating on TSB agar plates. Bacteria burden from the experimental catheters was compared using One-way ANOVA using GraphPad Prism Version 6.04 (GraphPad Software, La Jolla, CA).
Candida albicans biofilm accumulation on coated catheters
Both mouse and human catheters were assessed for fungal biofilm formation and accumulation of microbial cells after placing uncoated and coated 1 cm segments in 12-well plates and adding 2 mL of bovine serum (BS) (ThermoFisher Scientific, Waltham, MA) that were then incubated overnight at 37 °C with agitation (150 rpm) in an orbital shaker. Post incubation, the BS was removed through aspiration, and the catheters were washed with 2 mL of PBS. Catheters were then transferred to a fresh plate with 2 mL of spider medium (10 g of Difco nutrient broth; 10 g of Mannitol; 4g of K2HPO4; pH to 7.2; autoclave in 1 L of water) and inoculated with 40 µL (OD600 = 0.5) of an overnight culture of constitutively expressed green fluorescent protein (GFP) C. albicans MLR62 (Nobile and Mitchell, 2005). Seeded cultures were incubated for 90 min at 150 rpm on an orbital shaker, allowing fungal cells to adhere to catheter surfaces, then washed with PBS before being transferred to a fresh 12-well plate containing 2 mL of Spider broth. Catheters were allowed to incubate with agitation for 60 hours, allowing the formation of mature biofilms before being gently washed with 2 mL of PBS. While suspended in PBS, the catheters were incubated for 10 min in a sonicating water bath (Model: Branson 2800, SonicsOnline, Richmond, VA, USA) (with an interval of 5 sec after every 30 sec of sonication). A 100 µL aliquot for each sample was transferred to a fresh 96-well plate (Corning, ThermoFisher Scientific, Waltham, MA). The released fungal cells were quantified at 488 nm excitation and 535 nm emission using SpectraMax i3X (Molecular Devices, San Jose, CA). The remaining sonicated slurry was serially diluted in PBS and plated on a YPD agar, then incubated for 18 h before enumerating the colony forming units (CFU).
Staphylococcus aureus - Candida albicans dual biofilm formation on catheters
Both mouse and human catheters were assessed for dual biofilms (S. aureus - C. albicans biofilm) by placing segments in 12-well plates and adding 2 mL of bovine serum (BS) (ThermoFisher Scientific, Waltham, MA). Catheters were incubated overnight at 37°C with agitation (150 rpm) in an orbital shaker. Post incubation, the BS was removed through aspiration, and the catheters were washed with 2 mL of PBS. The catheters were then transferred to a fresh plate 2 mL plate with 40 µL of C. albicans MLR62 (1 × 106 cells/mL) (Nobile and Mitchell, 2005) and S. aureus USA 300 (1 × 107 cells/mL) in 1:1 v/v YPD/BHI (2 mL) media. Seeded cultures were incubated for 90 min at 37 °C at 150 rpm on an orbital shaker, allowing fungal/bacteria cells to adhere to catheter surfaces, then washed with PBS before being transferred to a fresh 12-well plate containing 2 mL of Spider broth. Catheters were allowed to incubate with agitation for 60 hours, allowing the formation of mature biofilms, before being gently washed with 2 mL of PBS. While suspended in 2 mL of PBS, the catheters were sonicated for 10 min (with an interval of 5 sec after every 30 sec of sonication) in a water bath sonicator (Model: Branson 2800, SonicsOnline, Richmond, VA, USA). A 100 µL aliquot for each sample was serially diluted. Fungal cell growth was selected on a YPD plate containing vancomycin (15 mg/mL), and bacterial cells were set on TSB media containing fluconazole (25 mg/mL). The plate was incubated at 37°C for 18 h, and the CFU was enumerated.
Murine subcutaneous catheter model infected with Candida albicans biofilm
Female BALB/c mice (n=5/group) were used according to the protocol approved by the Institutional Animal Care and Use Committee. Mouse catheters (uncoated, THF+PU, auranofin 3 mg + PU 50 mg, auranofin 10 mg + PU 50 mg, and fluconazole 10 mg + PU 50 mg) were treated overnight in 2 mL of FBS and inoculated with 1x104 CFU/mL of C. albicans MLR62 and incubated at 37°C for 90 min (Nobile and Mitchell, 2005). On day 1, the BALB/c female mice (Charles River, Wilmington, MA.) were anesthetized via IP injection with ketamine (100 mg/kg) and xylazine (10 mg/kg). The mouse flanks were shaved, and the skin was sterilized topically using betadine and isopropyl alcohol. A 5 mm skin incision was made using sterile scissors to create subcutaneous tunnels on both animal flanks for 1 cm catheters that were previously seeded with C. albicans MLR62. Upon completion, the incisions were closed with steel wound clips. Control animals were implanted with two drug-free catheters. Post-procedure, the animals were revived with atipamezole (1 mg/10 mg of xylazine used in a 100 PL volume) via IP injection. After catheter implantation and upon animal revival, the mice were given buprenorphine SR at 0.5 to 1 mg/kg via SQ injection.
After the 4-day evaluation period, animals were anesthetized with ketamine-xylazine and euthanized via exsanguination by cardiac puncture to collect blood (~1 mL). Subsequently, the catheters were removed from the euthanized mice and the blood was plated on YPD media to enumerate C. albicans MLR62 CFU. The retrieved catheters were sonicated in 1 mL YPD broth, and the fungal load was enumerated after serial dilution and plating on YPD agar plates (Supplemental figure 2).
Results
Fluid flow dynamics through human and mouse catheters are not altered by the drug-coating process
PBS was passed through coated catheters and analyzed using various antimicrobial coating agents to determine if the coating process impedes fluid flow. Flow dynamics were assessed for mouse and human catheters coated with auranofin (3 and 10 mg/mL), vancomycin (15 mg/mL), and fluconazole (10 mg/mL). Coated catheters were studied in three aspects, namely: time, volume, and mass.
Fluid flow volume
Fluid flow was assessed by passing PBS fluid through catheters at a constant rate of 1 mL/min using a syringe pump (Figure 1). The total collected volume was measured to see if the THF, PU, or drug coatings impeded fluid. The fluid that passed through the dip-coated catheters (mouse and human) remained consistent compared to the uncoated control catheters, achieving a 60 mL collection volume after 1 h as expected with the 1 mL/min flow rate (Figures 1A, B).
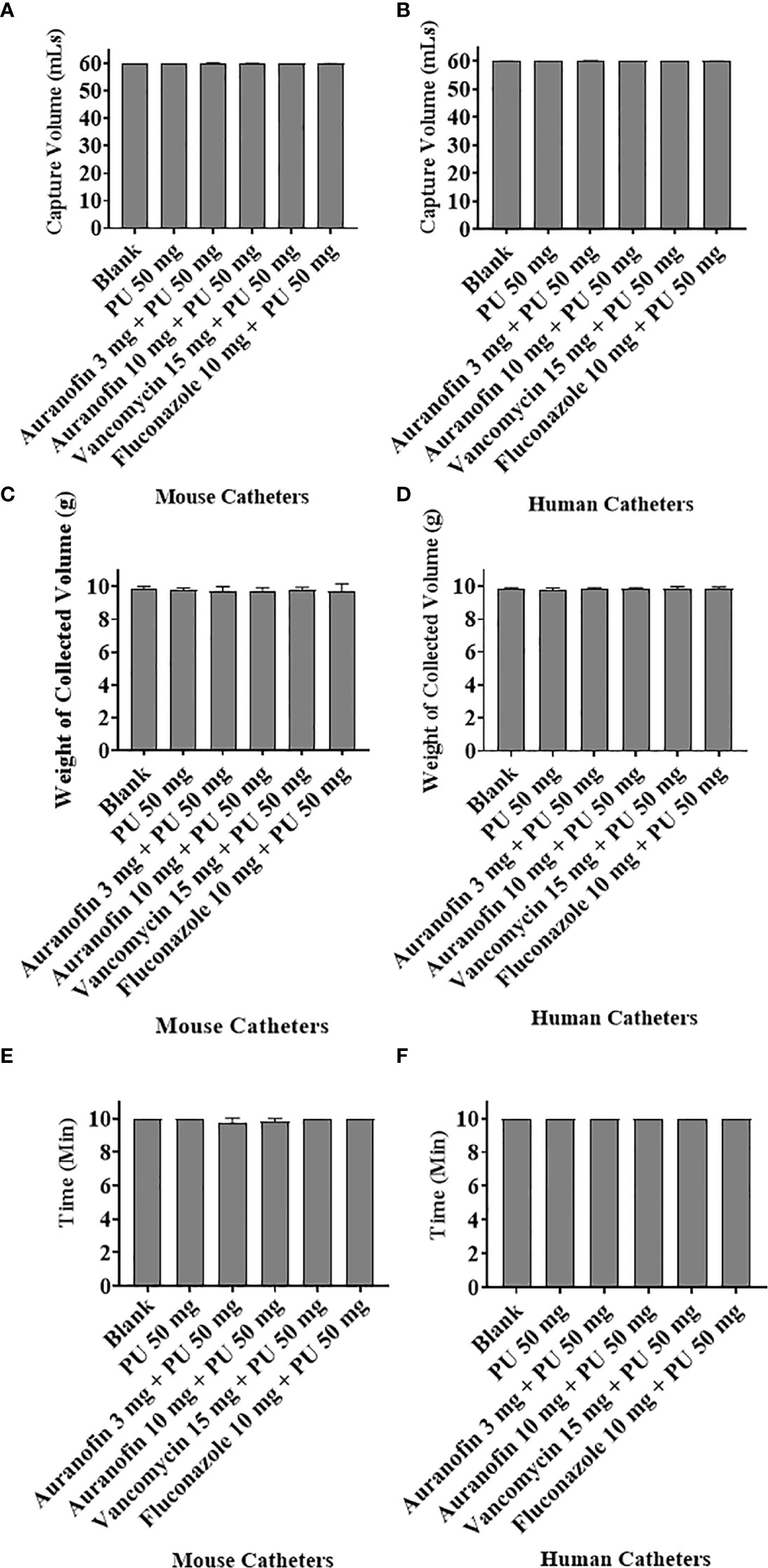
Figure 1 The flow dynamics of drug-coated mouse and human catheters were tested using a Harvard Apparatus syringe pump. (A, B) Qualitative analysis evaluated the time for 60 mL of PBS to flow through coated and uncoated mouse and human catheters, keeping the volume constant. (C, D) Quantitative analysis weighted total PBS flowing through catheters in 10 min. (E, F) The time required for 10 mL of PBS to flow through coated catheters was assessed.
Analytical measurement of the total weight of PBS passing through the catheters at a fixed time of 10 min and at a flow rate set at 1 mL/min found that 9.8 and 9.9 g of PBS passed through the mouse and human catheters, respectively (Figures 1C, D) within the designated time frame. Again, indicating that the coating process does not impede fluid compared to the non-coated catheters (no statistical difference compared to the control) and that the total volume flowed through the catheters unimpeded. The average blood flow rate under normal conditions can vary for different parts of the body. The arterial flow rate ranges from 3 to 26 mL/min, and the venous flow rate can be 1.2-4.8 mL/min (Klarhöfer et al., 2001). The tested flow rate of 1 mL/min provides proof of concept. We have provided additional evidence that higher flow rates of 5 mL/min and 10 mL/min do not impact fluid flowing through the coated catheters (5 mL/min and 10 mL/min) (Supplemental Figure 3). The catheters were incubated with 3 mL of PEG400 overnight, and the next day they were removed and dried in a sterile chamber for 24 h. Further, the flow rate of 60 mL PBS was analyzed using a Harvard apparatus. The PEG400 coating was also on human and mouse catheters and used as a comparison with PU coated catheters (Supplemental Figure 4). There was no significant change in the flow rate in both catheters.
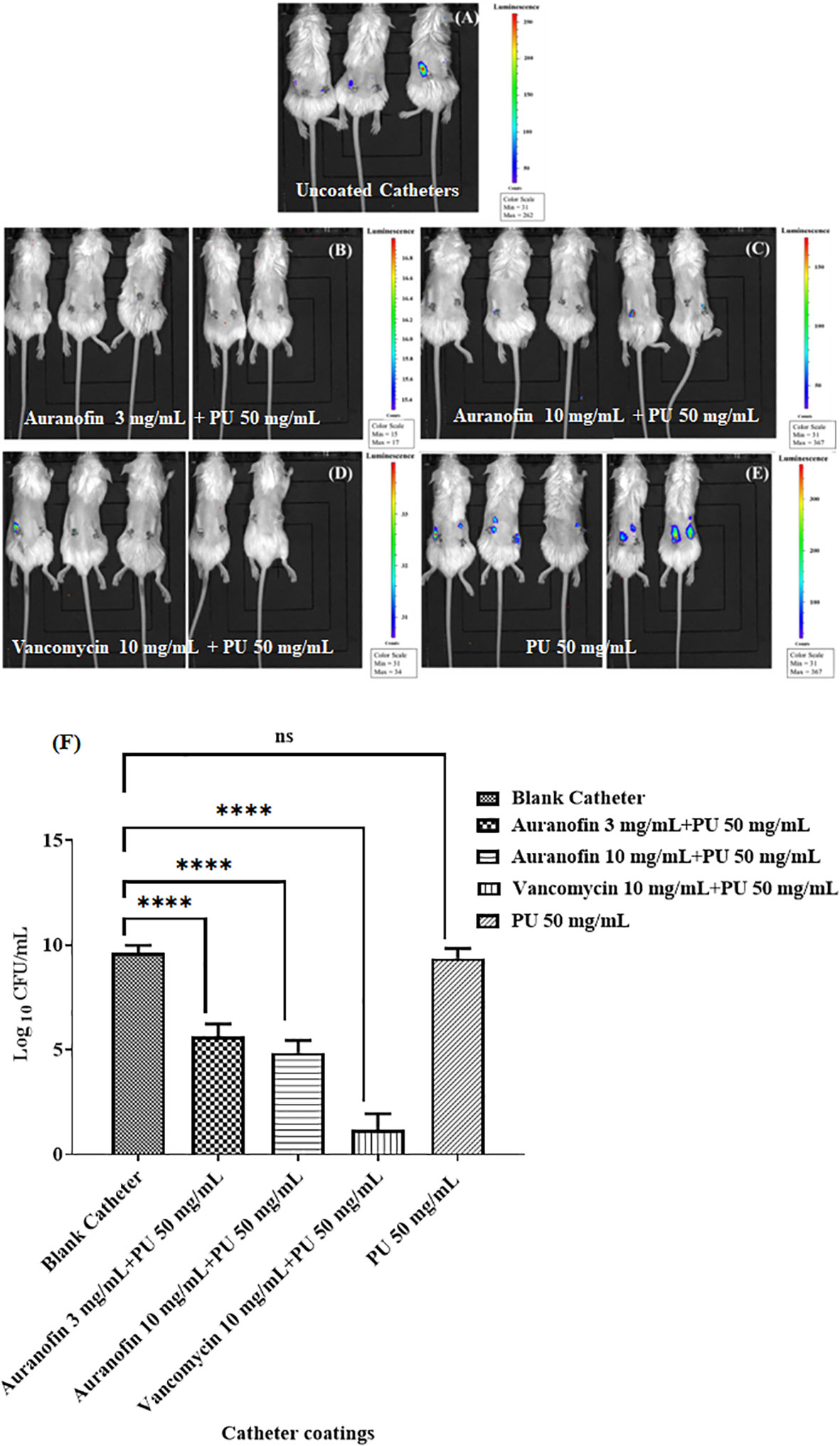
Figure 2 Post implantation, IVIS imaging displayed biofilm accumulation differences in auranofin coated and uncoated catheters in BALB/c mice inoculated with S. aureus USA300. (A) Uncoated catheter (B) Auranofin 3 mg/mL + PU 50 mg/mL (C) Auranofin 10 mg/mL + PU 50 mg/mL (D) Vancomycin 10 mg/ml + PU 50 mg/mL (E) PU 50 mg/mL + THF (F) Enumeration of S. aureus CFU/mL from recovered catheters. Experiments were performed using 5 animals per group with 2 catheters per animal. Significant differences (P ≤ 0.05) are shown with an asterisk. Color scale bar indicates S. aureus density.
Fluid flow time
The time for a fixed volume of PBS to flow through the catheters was tested to determine if the added coating altered the fluid dynamics. A total volume of 10 mL of PBS was passed through the catheters, with a flow rate set at 1 mL/min, and at optimal flow, the total volume would be collected in 10 min. For both the coated and uncoated catheters, the expected 10 mL volume was recovered in the 10 min period (Figures 1E, F), indicating that the coating process did not alter the flow rate. Importantly, with the cumulative assessment of volume and time, it appears that neither the carrier PU in THF nor the added antimicrobial drugs altered the flow dynamics compared to uncoated catheters. Although the tested antimicrobial compounds differ in chemistry, size, and hydrophobicity, none adjusted fluid flow based on the tested parameters.
Auranofin coated catheters inhibit Staphylococcus aureus biofilm formation in a murine subcutaneous implant model
Previous in vitro investigation of the auranofin and vancomycin-coated catheters demonstrated a significant reduction in S. aureus biofilm accumulation on catheters, suggesting this specific antimicrobial drug coating impedes bacterial biofilm formation and accumulation on the devices (Liu et al., 2019a). To advance this early finding, coated catheters were implanted subcutaneously, and the bacterial load was measured at the implant site in live animals, visualized via luminescence imaging. Captured images showed bacterial accumulation when non-coated catheters were implanted (Figures 2A-E). Bacteria were also visualized accumulating at the implant site when catheters were coated with PU dissolved in THF. However, bacterial accumulation was reduced when auranofin or vancomycin was included as part of the catheter coatings.
Post implantation, catheters were recovered from the subcutaneous pockets and S. aureus associated with the catheters was enumerated by sonicating the catheter and plating on TSA plates. The uncoated and PU-alone coated catheters accumulated bacteria, reaching 4 x 109 and 1.7 x 109 CFU/mL, respectively. However, a 3 mg/mL auranofin-coated catheter was reduced to 7.4 x 105 CFU/mL, and a 10 mg/mL auranofin-coated catheter reduced bacteria accumulation further to 1.2 x 105 CFU/mL, a 4-log reduction from the controls (Figure 2F). The positive control vancomycin demonstrated the most significant reduction of bacteria, with no bacteria recovered from the catheters. While auranofin efficacy was surpassed by vancomycin (a standard-of-care antibiotic) at the tested concentrations, it provides evidence that auranofin can exert potent bacterial inhibition. Although catheters are coated with mg/mL amounts of compounds, previously published data indicate that µg amounts are released. For example, catheters coated with 10 mg/mL auranofin were found to release approximately 37 µg (Liu et al., 2019a).
Inhibition of Candida albicans biofilm formation on auranofin-coated catheters
In prior work, we determined that auranofin coated catheters could reduce the accumulation of S. aureus biofilm in vitro (Liu et al., 2019a). The remarkable nature of auranofin is imparted by further testing the impact on fungal accumulation. Indeed, the antimicrobial nature of auranofin has been shown to inhibit both bacterial and fungal pathogens (Fuchs et al., 2016), making it uniquely suited as a catheter coating that can be exposed to numerous types of microbes. A constitutively expressing GFP C. albicans strain (MLR62) was used to monitor biofilm accumulation on the catheters. The absorbance measurement for C. albicans GFP expression showed a decrease in biofilm formation on auranofin (3 mg and 10 mg) coated catheters compared to the uncoated catheters (Figures 3A, B). No GFP was recorded with the 3 mg auranofin-coated mouse catheter, suggesting no detectable accumulation of C. albicans cells.
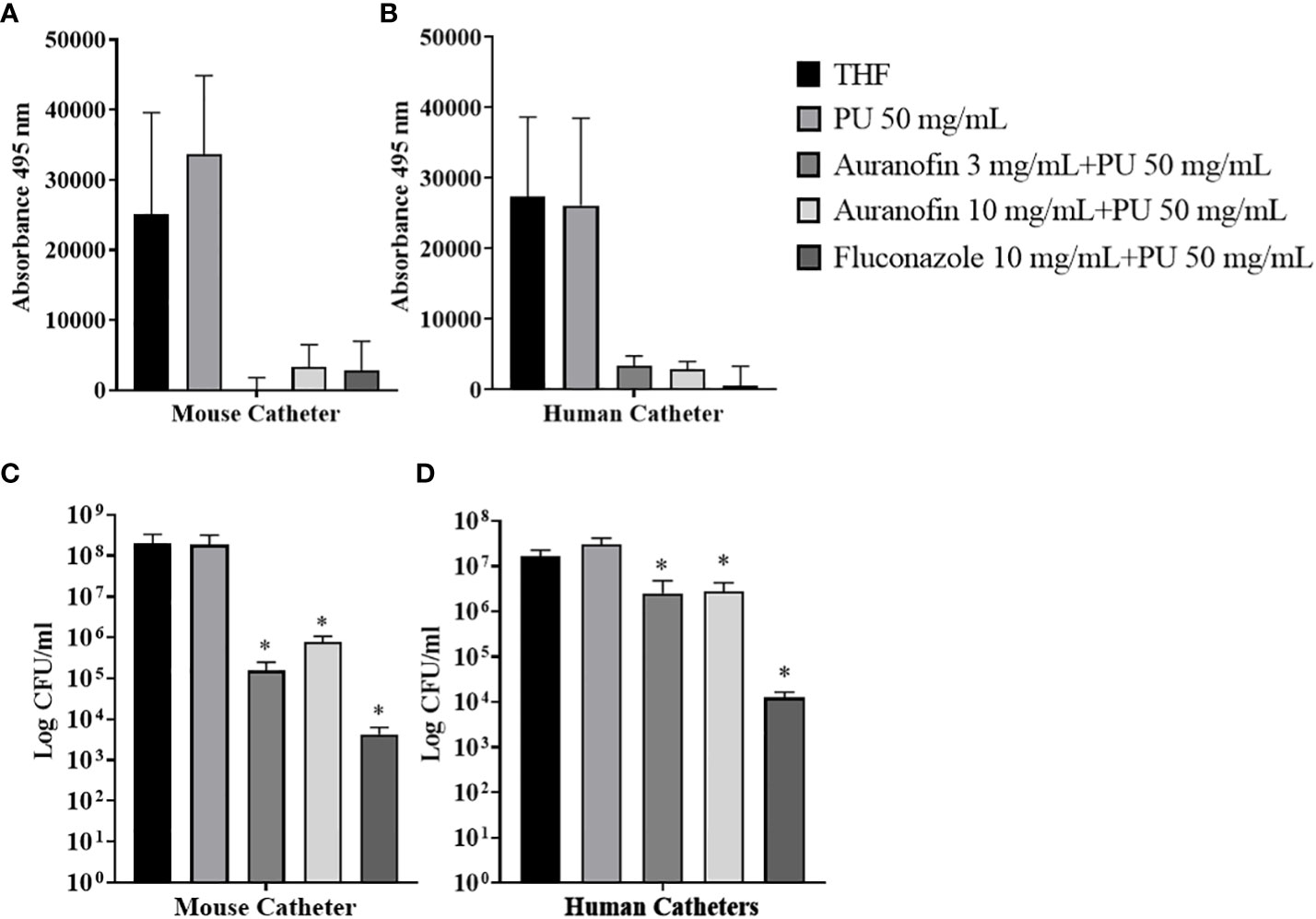
Figure 3 Antimicrobial coatings reduce (C) albicans biofilm accumulation on catheters. (A, B) Quantification of (C) albicans biofilm formed on catheters coated with 3 mg/mL auranofin, 10 mg/mL auranofin, or 10 mg/mL fluconazole. Biofilms formed on catheters were measured by reading fluorescence from GFP expression. (B, C) Catheters were sonicated to dislodge the (C) albicans biofilm and the material was plated to determine CFU/mL. Significance was determined using Student’s t-test. * Indicates P<0.05.
Enumeration of C. albicans cells on auranofin-coated and uncoated catheters (mouse and human) found a reduction attributed to auranofin coating. When coated with 3 mg and 10 mg auranofin, mouse catheters accumulated 1.6 x 105 and 7.8 x 105 CFU compared to 2.0 x 108 CFU associated with catheters exposed to THF solvent alone (Figure 3C). Thus, a 3-log reduction in C. albicans cells was achieved with the auranofin coating with P=0.0229 and P=0.023, respectively. When the dip coating method was applied to human catheters, associated C. albicans cells were also reduced from accumulating on the material. Catheters exposed to THF solvent were found to have 1.6 x 107 CFU compared to 2.5 x 106 (P=0.0052) and 2.8 x 106 (P=0.0049) CFU recovered from catheters coated with 3 and 10 mg/mL auranofin, respectively (Figure 3D). Fluconazole-coated catheters (1x104 CFU/mL) also demonstrated a reduction in C. albicans accumulation. Measuring C. albicans biofilm biomass can be challenging because this requires weighing the catheters prior to introducing the C. albicans post-biofilm formation. In this case, there can be variations in the coating volume added to the individual catheters due to slight differences in release volumes during the incubation period that can impact weight. Thus, mass cannot be accurately measured. Therefore, we used C. albicans strain MLR62 which constitutively expresses GFP as a means of measuring live C. albicans cells associated with the catheters. To provide insights into the impact of auranofin on C. albicans biofilm accumulations, C. albicans biofilm biomass changes on silicone pads were demonstrated in the presence of auranofin (Supplemental Figure 5). Treating wells with auranofin demonstrate that C. albicans biofilm accumulation is reduced compared to untreated control wells based on dry weight. Therefore, the result demonstrated that mouse and human catheters coated with auranofin exhibited a significant reduction in C. albicans biofilm formation and accumulation over the 60-hour test period, enough time for mature biofilms to form.
Inhibition of mixed Staphylococcus aureus - Candida albicans biofilm formation on auranofin-coated catheters
A unique feature of auranofin is its ability to inhibit both bacterial and fungal pathogens (Harbut et al., 2015; Fuchs et al., 2016). To evaluate the ability of auranofin to impact a mixed microbe biofilm, catheters were exposed to a mixed pathogen culture of S. aureus and C. albicans biofilm allowed to mature before being disrupted by sonication and plated on TSA and YPA selection media to enumerate the individual microbes. Applying 10 mg auranofin on human catheters reduced S. aureus accumulation from 5.1 x105 CFU/mL found on non-coated catheters to 6 x103 CFU/mL but failed to reach significance. However, C. albicans biofilm on the human catheter was reduced from 1.2 x 108 CFU/mL on non-coated catheters to 1.3 x 105 CFU/mL on the 10 mg auranofin coated catheters, an efficient reduction (P=0.0163) as demonstrated with a Student’s t-test (Figure 4A). Although both vancomycin and fluconazole impacted microbe accumulation on human catheters, they failed to have cross-species efficacy as seen with high concentrations of auranofin. Fluconazole reduced S. aureus accumulation from 5.1 x105 CFU/mL found on non-coated catheters to 2.5 x104 CFU/mL (P=0.1262) and did not reduce C. albicans accumulation (2.8 x 108 CFU/mL; P=0.4312). Vancomycin coating at 15 mg caused an increase in S. aureus associated with the catheters, reaching 3.4 x 106 CFU/mL. C. albicans accumulation was also not reduced by vancomycin, reaching 2.43 x 107 CFU/mL with the 10 mg coating concentration.
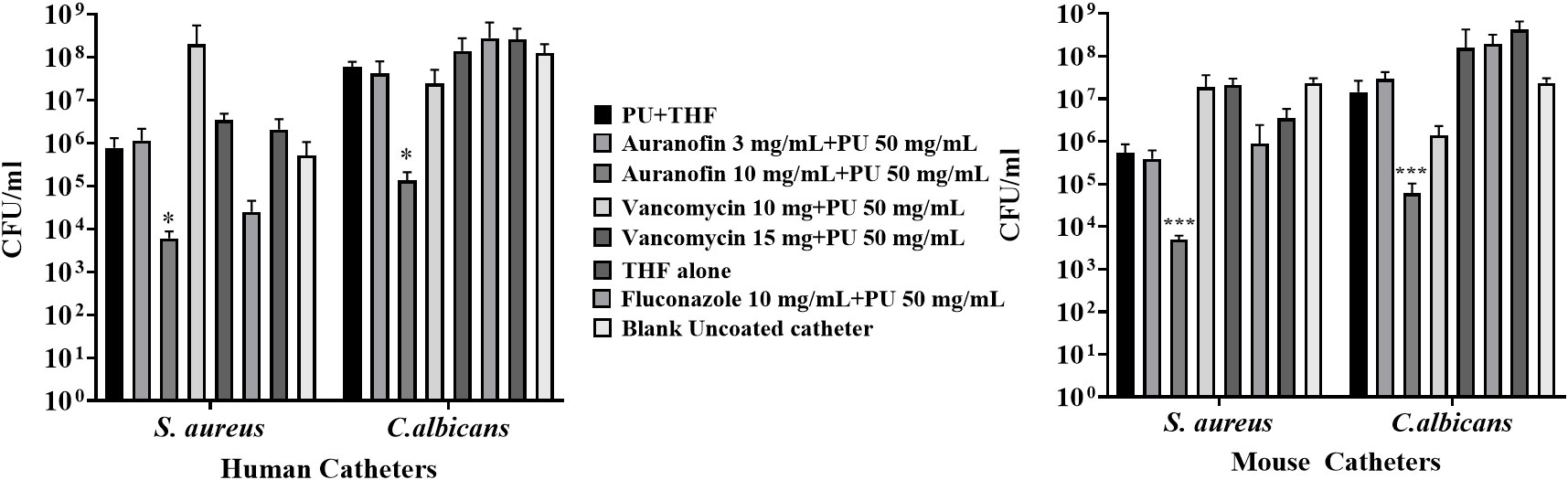
Figure 4 Enumeration of bacterial and fungal biofilm formed on auranofin coated and uncoated mouse and human catheters. Biofilms were allowed to form, and after 60 hours, the catheters were washed and sonicated to collect and enumerate attached cells. Statistical analysis was carried out using Student’s t-test. Significant differences (P ≤ 0.05) are shown with an asterisk.
Murine catheters were also evaluated in the same manner. Catheters coated in 10 mg auranofin exhibited S. aureus reduction from 2.3 x 107 CFU/mL to 5 x 103 CFU/mL (P=0.0005) (Figure 4B). C. albicans biofilm was reduced from 2.3 x 107 CFU/mL on non-coated catheters to 6 x 104 CFU/mL when coated with 10 mg of auranofin (P=0.0005) (Figure 4B). Thus, both catheters coated with auranofin 10 mg + PU significantly reduced microbe accumulation on the catheters under in vitro conditions that usually promote microbial attachment and mature biofilm formation.
Auranofin coated catheters inhibit Candida albicans biofilm formation in a murine subcutaneous implant model
Finding that auranofin-coated catheters demonstrated in vitro reduction of C. albicans biofilm accumulation prompted further evaluation using a subcutaneous murine model. C. albicans cells were allowed to attach to coated catheters before implantation. At 4 days post-implantation, catheters were removed, sonicated to detach C. albicans, and the CFU was enumerated on YPD plates. Both fluconazole (10 mg/mL) and auranofin-coated catheters (10 mg/mL) exhibited a reduction in the C. albicans biofilm, recovering 1.6 x 104 CFU/mL (P=0.0003) and 6.9 x 104 CFU/mL (P=0.0019), respectively, compared to the non-coated catheters (3.9 x105 CFU/mL) (Figure 5). Biofilm reductions depict the efficacy of the coating method and material. The study mainly focuses on testing the efficacy of polyurethane coating with auranofin on catheters. Also, increasing the concentration of auranofin will significantly decrease the biofilm at higher folds in comparison to uncoated catheters. We noted that there was no significant inflammation on the site of catheter insertion in the mouse model. We also collected blood by heart puncture, but no microbe growth was recorded.
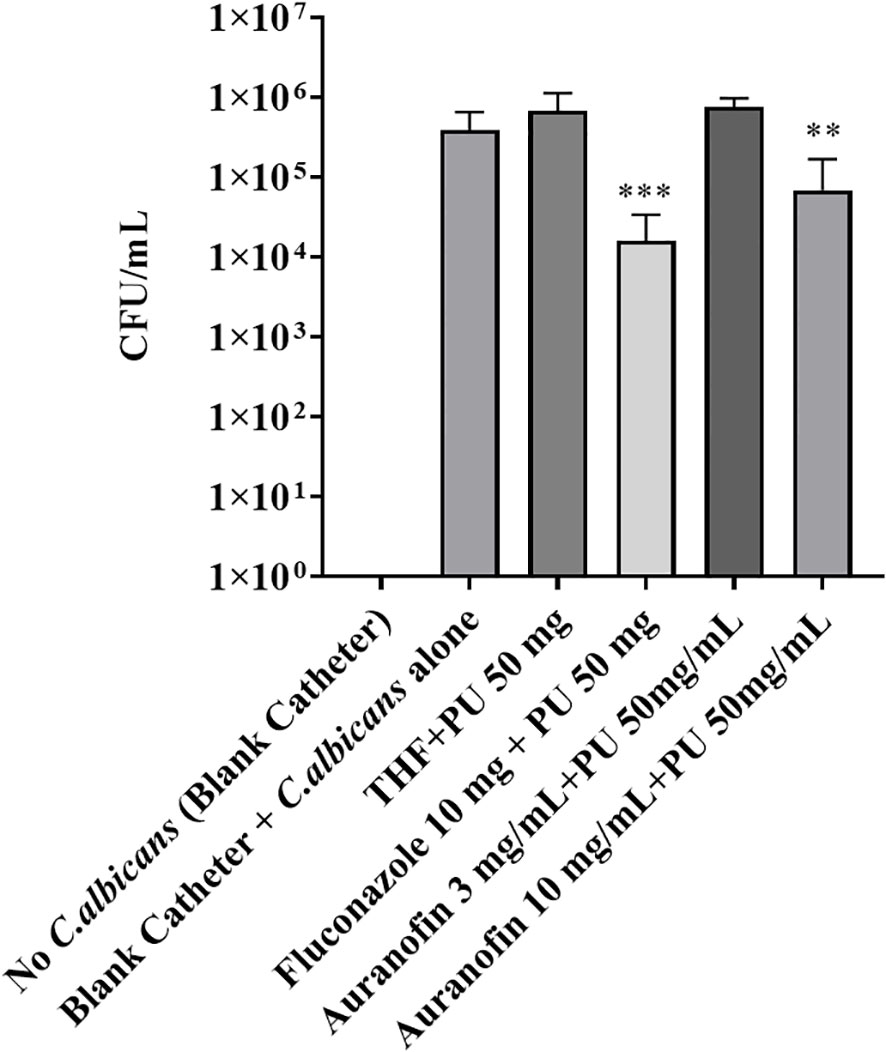
Figure 5 Auranofin coated catheters significantly reduced C. albicans biofilm on catheters in a subcutaneous murine model. Catheters were recovered from mice (day 4), and the materials were sonicated to release attached cells. The collected cells were enumerated for C. albicans CFU/mL on YPD agar plates. C. albicans biofilm reduction showed 6.9 x 104 CFU/mL (P=0.0019) on auranofin coated catheters (10 mg/mL). The uncoated (blank) catheters had 3.9 x105 CFU/mL of C. albicans biofilm. Statistical analysis was carried out using Student’s t-test. Experiments included 5 animals per group with 2 catheters per animal. Significant differences (P ≤ 0.05) are shown with an asterisk.
Discussion
Infections involving implant devices impact patient health, recovery time, and overall mortality rates. Prevention practices are essential and reduce widespread nosocomial infection, but more elements can be addressed to improve patient outcomes. Coating catheters have been a means to prevent bacterial attachment and biofilm formation (Suresh et al., 2019). While numerous drugs can be incorporated into the coating process, auranofin presents a particularly intriguing option as an agent that impacts both S. aureus and C. albicans, representing efficacy against Gram-positive bacteria and fungi (Fuchs et al., 2016; Liu et al., 2019a).
C. albicans and S. aureus are responsible for most opportunistic nosocomial infections (Harriott and Noverr, 2011), and can be co-isolated from the same host (Krüger et al., 2019). It is common for these polymicrobial infections to be associated with mixed biofilms formed in catheters or other indwelling devices, where C. albicans and S. aureus display symbiotic relationships (Peters et al., 2010; Todd and Peters, 2019). The use of antimicrobial-coated catheters has been suggested to decrease the risk of acquiring a catheter-related bloodstream infection, and biofilm-inhibiting antimicrobial compounds have been proposed to prevent colonization and biofilm formation (Wang et al., 2018; Zhu et al., 2019).
Auranofin has demonstrated antimicrobial efficacy against bacteria and fungi (Siles et al., 2013; Harbut et al., 2015; Fuchs et al., 2016), suggesting that it could inhibit both types of pathogens which cause catheter-related infections. Further, auranofin impacts bacterial and fungal planktonic cells and biofilms (Siles et al., 2013; Torres et al., 2016; She et al., 2020). In their study, Siles and colleagues demonstrated that auranofin caused 94% C. albicans biofilm inhibition (Siles et al., 2013). In our previous study, we used auranofin coating on intravenous catheters to prevent the biofilm of S. aureus under in vitro conditions (Liu et al., 2019a). As a continuation of the previous work, this report shows the efficacy of auranofin-coated catheters against S. aureus and C. albicans biofilm under in vitro and in vivo conditions. This is the first report of auranofin-coated catheters inhibiting mixed biofilm of S. aureus- C. albicans.
Despite coating mouse and human catheters with auranofin, there was no change in the flow, volume, and weight of liquid passing through the coated catheter. This suggested that PU and auranofin coating on the catheter does not influence the flow rate concerning time or volume. But in a study by (Gefter Shenderovich et al., 2018), chlorhexidine coated using polyethylene glycol on a catheter affected the release rate due to thicker coating and less stability. Thus, the present study demonstrates PU as an efficient coating material with better flow dynamics.
Previous reports suggested that coating catheters with silver nanoparticles (Lara and Lopez-Ribot, 2020) and antibiotics (Vila et al., 2021) provided efficient inhibition of mixed biofilm of S. aureus and C. albicans. Antimicrobial catheters should offer long-term antimicrobial surface effects without side effects or toxicity and a low application cost (Singha et al., 2017). In the present study, catheter coated with PU + auranofin offers an attractive alternative to prevent the colonization and biofilm formation of S. aureus and C. albicans while not taxing current standard of care antibiotic or antifungal therapeutic agents, thus respecting antimicrobial stewardship. The auranofin-coated catheter showed efficient anti-biofilm activity against the mixed microbe biofilm composed of S. aureus and C. albicans. In addition to auranofin, both the control antimicrobial agents (fluconazole and vancomycin) were able to act as effective coating agents against C. albicans and S. aureus, but the standard of care controls failed to inhibit microbial accumulation from both S. aureus and C. albicans showing the inability to affect dual biofilms. The difference in biofilm formation of C. albicans on the mouse and human catheter is due to the different materials used for manufacturing them. The mouse catheters are made with polyurethane, while human catheters are made using radiopaque teflon.
In conclusion, in vitro assays demonstrated that auranofin-coated catheters inhibit S. aureus and C. albicans biofilm formation. Qualitative and quantitative analysis of auranofin-coated catheter in a murine subcutaneous implant model showed reduced biofilm formation. Both S. aureus and C. albicans subcutaneous murine models demonstrated that catheters coated with 10 mg of auranofin experienced reduced microbe accumulation. Although vancomycin is an effective antimicrobial that inhibits S. aureus planktonic cells, it is not as impactful against biofilm. Further, although coated at 10 mg/mL, a previous study by (Liu et al., 2019a) demonstrated that coating concentration greatly exceeded release concentration (Liu et al., 2019a) and in this case, it’s likely much vancomycin release concentration is much lower. Thus, it fails to be impactful in reducing S. aureus biofilm accumulation on catheters. Successful reduction in biofilm formation indicated that auranofin is an excellent candidate for catheter coating against bacterial and fungal biofilm without altering catheter flow dynamics. The cumulative results indicate that the dip-coating method successfully provides a protective coating on the catheters and S. aureus and C. albicans biofilm formation is reduced by auranofin application.
Data availability statement
The original contributions presented in the study are included in the article/Supplementary material. Further inquiries can be directed to the corresponding author.
Ethics statement
The animal study was reviewed and approved by Institutional Animal Care and Use Committee.
Author contributions
LF and BF designed and carried out experiments. CW, NV-G, and AS helped with the coating of catheters. NT and BM assisted with animal experiments. BF, EM, and AS directed research, provided insightful discussion, and contributed to the writing. All authors contributed to the article and approved the submitted version.
Funding
This work was supported by P20GM121344 from the National Institute of General Medical Sciences, which funds the Center for Antimicrobial Resistance and Therapeutic Discovery and BF. Additional support was provided by National Institutes of Health Grant P01AI083214 to EM.
Acknowledgments
We thank Alec McCall (Brown University) for assistance with coated catheter preparation. We also thank Alexis Jacober and Dr. Gerard Nau for their assistance with animal experiments.
Conflict of interest
The authors declare that the research was conducted in the absence of any commercial or financial relationships that could be construed as a potential conflict of interest.
Publisher’s note
All claims expressed in this article are solely those of the authors and do not necessarily represent those of their affiliated organizations, or those of the publisher, the editors and the reviewers. Any product that may be evaluated in this article, or claim that may be made by its manufacturer, is not guaranteed or endorsed by the publisher.
Supplementary material
The Supplementary Material for this article can be found online at: https://www.frontiersin.org/articles/10.3389/fcimb.2023.1135942/full#supplementary-material
Supplementary Figure 1 | Schematic of flow rate assessment for coated and uncoated mouse and human catheters. 1. Syringe pump (Harvard apparatus). 2. Fluid-filled syringe. 3. Connecting syringe adapter. 4. Catheter. 5. Collection tube.
Supplementary Figure 2 | In vivo assessment of S. aureus biofilm accumulation. Catheters incubated with S. aureus USA300 were implanted into subcutaneous pockets on either side of the mouse flanks. Post incubation, the catheters were recovered and sonicated to dislodge attached cells, and the slurry was plated on selection media to enumerate the CFU on each catheter.
Supplementary Figure 3 | The flow kinetic of the drug-coated mouse and the human catheter was tested using a Harvard Apparatus syringe pump. The analysis evaluated the time for 60 mLs of PBS to flow through coated and uncoated mouse and human catheters, keeping the volume constant at 5 mL/min and 10 mL/min.
Supplementary Figure 4 | PEG400 coating was applied to the mouse and human catheters to determine the flow rate of 60 mL PBS. The flow rate was fixed at 1 mL/min for a total time of 60 min. No significant changes were observed in the flow rate compared to PU-coated catheters.
Supplementary Figure 5 | The different concentration of auranofin and fluconazole was tested on C. albicans MLR62 biofilm. The C. albicans biofilm was formed on a silicone pad in the presence of auranofin and fluconazole, and the biomass was weighed after the incubation period. The experiments were performed in triplicate.
References
Balaure, P. C., Grumezescu, A. M. (2020). Recent advances in surface nanoengineering for biofilm prevention and control. part II: active, combined active and passive, and smart bacteria-responsive antibiofilm nanocoatings. Nanomaterials 10, 1527.
Brindhadevi, K., Lewisoscar, F., Mylonakis, E., Shanmugam, S., Verma, T. N., Pugazhendhi, A. (2020). Biofilm and quorum sensing mediated pathogenicity in pseudomonas aeruginosa. Process Biochem. 96, 49–57. doi: 10.1016/j.procbio.2020.06.001
Chandra, J., Mukherjee, P., Leidich, S., Faddoul, F., Hoyer, L., Douglas, L., et al. (2001). Antifungal resistance of candidal biofilms formed on denture acrylic in vitro. J. Dental Res. 80, 903–908. doi: 10.1177/00220345010800031101
Dai, T., Gupta, A., Huang, Y. Y., Sherwood, M. E., Murray, C. K., Vrahas, M. S., et al. (2013). Blue light eliminates community-acquired methicillin-resistant staphylococcus aureus in infected mouse skin abrasions. Photomed. Laser Surg. 31, 531–538. doi: 10.1089/pho.2012.3365
Devrim, İ., Topal, S., Ceylan, G., Oruç, Y., Gülfidan, G., Ayhan, Y., et al. (2022). Changes in the incidence of candida-related central line-associated bloodstream infections in pediatric intensive care unit: could central line bundle have a role? J. Med. Mycology 32, 101277.
Donlan, R. M. (2001). Biofilms and device-associated infections. Emerging Infect. Dis. 7, 277. doi: 10.3201/eid0702.010226
Douglas, L. J. (2003). Candida biofilms and their role in infection. Trends Microbiol. 11, 30–36. doi: 10.1016/S0966-842X(02)00002-1
Esposito, S., Purrello, S., Bonnet, E., Novelli, A., Tripodi, F., Pascale, R., et al. (2013). Central venous catheter-related biofilm infections: an up-to-date focus on meticillin-resistant staphylococcus aureus. J. Global Antimicrobial Resistance 1, 71–78. doi: 10.1016/j.jgar.2013.03.002
Felix, L., Mylonakis, E., Fuchs, B. B. (2021). Thioredoxin reductase is a valid target for antimicrobial therapeutic development against gram-positive bacteria. Front. Microbiol. 12, 663481. doi: 10.3389/fmicb.2021.663481
Feneley, R. C., Hopley, I. B., Wells, P. N. (2015). Urinary catheters: history, current status, adverse events and research agenda. J. Med. Eng. Technol. 39, 459–470. doi: 10.3109/03091902.2015.1085600
Francolini, I., Vuotto, C., Piozzi, A., Donelli, G. (2017). Antifouling and antimicrobial biomaterials: an overview. Apmis 125, 392–417. doi: 10.1111/apm.12675
Fuchs, B. B., Rajamuthiah, R., Souza, A. C. R., Eatemadpour, S., Rossoni, R. D., Santos, D. A., et al. (2016). Inhibition of bacterial and fungal pathogens by the orphaned drug auranofin. Future medicinal Chem. 8, 117–132. doi: 10.4155/fmc.15.182
Gahlot, R., Nigam, C., Kumar, V., Yadav, G., Anupurba, S. (2014). Catheter-related bloodstream infections. Int. J. Crit. Illn Inj Sci. 4, 162–167. doi: 10.4103/2229-5151.134184
Gefter Shenderovich, J., Zaks, B., Kirmayer, D., Lavy, E., Steinberg, D., Friedman, M. (2018). Chlorhexidine sustained-release varnishes for catheter coating - dissolution kinetics and antibiofilm properties. Eur. J. Pharm. Sci. 112, 1–7. doi: 10.1016/j.ejps.2017.10.041
Gilbert, P., Maira-Litran, T., Mcbain, A. J., Rickard, A. H., Whyte, F. W. (2002). The physiology and collective recalcitrance of microbial biofilm communities. Adv. Microbial Physiol. 46, 203–256. doi: 10.1016/S0065-2911(02)46005-5
Harbut, M. B., Vilchèze, C., Luo, X., Hensler, M. E., Guo, H., Yang, B., et al. (2015). Auranofin exerts broad-spectrum bactericidal activities by targeting thiol-redox homeostasis. Proc. Natl. Acad. Sci. 112, 4453–4458. doi: 10.1073/pnas.1504022112
Harriott, M. M., Noverr, M. C. (2011). Importance of candida-bacterial polymicrobial biofilms in disease. Trends Microbiol. 19, 557–563. doi: 10.1016/j.tim.2011.07.004
Klarhöfer, M., Csapo, B., Balassy, C., Szeles, J. C., Moser, E. (2001). High-resolution blood flow velocity measurements in the human finger. Magnet. Resonance Med. 45, 716–719. doi: 10.1002/mrm.1096
Kohse, S., Wulf, K., Pauker, V., Arbeiter, D., Fiedler, N., Bajer, D., et al. (2019). Session 2: Biofilms/Implant associated infections. Biomed. Engineering/Biomedizinische Technik 64, 19–24. doi: 10.1515/bmt-2019-7002
Krüger, W., Vielreicher, S., Kapitan, M., Jacobsen, I. D., Niemiec, M. J. (2019). Fungal-bacterial interactions in health and disease. Pathogens 8. doi: 10.3390/pathogens8020070
Lara, H. H., Lopez-Ribot, J. L. (2020). Inhibition of mixed biofilms of candida albicans and methicillin-resistant staphylococcus aureus by positively charged silver nanoparticles and functionalized silicone elastomers. Pathogens 9. doi: 10.3390/pathogens9100784
Laverty, G., Gorman, S. P., Gilmore, B. F. (2015). Biofilms and implant-associated infections. Biomat. Med. Device-Associated Infections, 19–46. doi: 10.1533/9780857097224.1.19
Lewisoscar, F., Nithya, C., Alharbi, S. A., Alharbi, N. S., Thajuddin, N. (2018). In vitro and in silico attenuation of quorum sensing mediated pathogenicity in pseudomonas aeruginosa using spirulina platensis. Microbial pathogenesis 116, 246–256. doi: 10.1016/j.micpath.2018.01.046
Lewisoscar, F., Nithya, C., Vismaya, S., Arunkumar, M., Pugazhendhi, A., Nguyen-Tri, P., et al. (2021). In vitro analysis of green fabricated silver nanoparticles (AgNPs) against pseudomonas aeruginosa PA14 biofilm formation, their application on urinary catheter. Prog. Org. Coatings 151, 106058. doi: 10.1016/j.porgcoat.2020.106058
Li, X., Li, P., Saravanan, R., Basu, A., Mishra, B., Lim, S. H., et al. (2014). Antimicrobial functionalization of silicone surfaces with engineered short peptides having broad spectrum antimicrobial and salt-resistant properties. Acta biomaterialia 10, 258–266. doi: 10.1016/j.actbio.2013.09.009
Liu, L., Shi, H., Yu, H., Zhou, R., Yin, J., Luan, S. (2019b). One-step hydrophobization of tannic acid for antibacterial coating on catheters to prevent catheter-associated infections. Biomat. Sci. 7, 5035–5043. doi: 10.1039/C9BM01223K
Liu, H., Shukla, S., Vera-González, N., Tharmalingam, N., Mylonakis, E., Fuchs, B. B., et al. (2019a). Auranofin releasing antibacterial and antibiofilm polyurethane intravascular catheter coatings. Front. Cell. Infect. Microbiol. 9, 37. doi: 10.3389/fcimb.2019.00037
Lynch, A. S., Robertson, G. T. (2008). Bacterial and fungal biofilm infections. Annu. Rev. Med. 59, 415–428. doi: 10.1146/annurev.med.59.110106.132000
Mah, T.-F. C., O’toole, G. A. (2001). Mechanisms of biofilm resistance to antimicrobial agents. Trends Microbiol. 9, 34–39. doi: 10.1016/S0966-842X(00)01913-2
Marik, P. E., Flemmer, M., Harrison, W. (2012). The risk of catheter-related bloodstream infection with femoral venous catheters as compared to subclavian and internal jugular venous catheters: a systematic review of the literature and meta-analysis. Crit. Care Med. 40, 2479–2485. doi: 10.1097/CCM.0b013e318255d9bc
May, H., Yu, J. (2018). Repurposing auranofin, ebselen, and PX-12 as antimicrobial agents targeting the thioredoxin system. Front. Microbiol. 9, 1–10. doi: 10.3389/fmicb.2018.00336
Mccoy, C., Paredes, M., Allen, S., Blackey, J., Nielsen, C., Paluzzi, A., et al. (2017). Catheter-associated urinary tract infections: implementing a protocol to decrease incidence in oncology populations. Clin. J. Oncol. Nurs. 21, 460–465.
Neugent, M. L., Hulyalkar, N. V., Nguyen, V. H., Zimmern, P. E., De Nisco, N. J. (2020). Advances in understanding the human urinary microbiome and its potential role in urinary tract infection. mBio 11. doi: 10.1128/mBio.00218-20
Nobile, C. J., Mitchell, A. P. (2005). Regulation of cell-surface genes and biofilm formation by the c. albicans transcription factor Bcr1p. Curr. Biol. 15, 1150–1155. doi: 10.1016/j.cub.2005.05.047
O’grady, N. P., Alexander, M., Dellinger, E. P., Gerberding, J. L., Heard, S. O., Maki, D. G., et al. (2002). Guidelines for the prevention of intravascular catheter–related infections. Clin. Infect. Dis. 35, 1281–1307. doi: 10.1086/344188
Panepinto, R., Harris, J., Wellette, J. (2021). A review of best practices related to intravenous line management for nurses. Nurs. Clinics 56, 389–399. doi: 10.1016/j.cnur.2021.05.001
Pathak, R. K., Ariyarathna, N., Garcia, F. C., Sanders, P., Marchlinski, F. E. (2019). Catheter ablation of idiopathic ventricular arrhythmias. Heart Lung Circ. 28, 102–109. doi: 10.1016/j.hlc.2018.10.012
Peters, B. M., Jabra-Rizk, M. A., Scheper, M. A., Leid, J. G., Costerton, J. W., Shirtliff, M. E. (2010). Microbial interactions and differential protein expression in staphylococcus aureus -candida albicans dual-species biofilms. FEMS Immunol. Med. Microbiol. 59, 493–503. doi: 10.1111/j.1574-695X.2010.00710.x
Selby, L. M., Rupp, M. E., Cawcutt, K. A. (2021). Prevention of central-line associated bloodstream infections: 2021 update. Infect. Dis. Clinics 35, 841–856. doi: 10.1016/j.idc.2021.07.004
Shah, H., Bosch, W., Thompson, K. M., Hellinger, W. C. (2013). Intravascular catheter-related bloodstream infection. Neurohospitalist 3, 144–151. doi: 10.1177/1941874413476043
She, P., Liu, Y., Wang, Y., Tan, F., Luo, Z., Wu, Y. (2020). Antibiofilm efficacy of the gold compound auranofin on dual species biofilms of staphylococcus aureus and candida sp. J. Appl. Microbiol. 128, 88–101. doi: 10.1111/jam.14443
Siles, S. A., Srinivasan, A., Pierce, C. G., Lopez-Ribot, J. L., Ramasubramanian, A. K. (2013). High-throughput screening of a collection of known pharmacologically active small compounds for identification of candida albicans biofilm inhibitors. Antimicrobial Agents Chemother. 57, 3681–3687. doi: 10.1128/AAC.00680-13
Singha, P., Locklin, J., Handa, H. (2017). A review of the recent advances in antimicrobial coatings for urinary catheters. Acta Biomaterialia 50, 20–40. doi: 10.1016/j.actbio.2016.11.070
Stenger, M., Klein, K., Grønnemose, R. B., Klitgaard, J. K., Kolmos, H. J., Lindholt, J. S., et al. (2016). Co-Release of dicloxacillin and thioridazine from catheter material containing an interpenetrating polymer network for inhibiting device-associated staphylococcus aureus infection. J. Controlled Release 241, 125–134. doi: 10.1016/j.jconrel.2016.09.018
Suresh, M. K., Biswas, R., Biswas, L. (2019). An update on recent developments in the prevention and treatment of staphylococcus aureus biofilms. Int. J. Med. Microbiol. 309, 1–12. doi: 10.1016/j.ijmm.2018.11.002
Thangamani, S., Maland, M., Mohammad, H., Pascuzzi, P. E., Avramova, L., Koehler, C. M., et al. (2017). Repurposing approach identifies auranofin with broad spectrum antifungal activity that targets Mia40-Erv1 pathway. Front. Cell. Infect. Microbiol. 7, 4. doi: 10.3389/fcimb.2017.00004
Todd, O. A., Peters, B. M. (2019). Candida albicans and staphylococcus aureus pathogenicity and polymicrobial interactions: lessons beyond koch’s postulates. J. Fungi 5. doi: 10.3390/jof5030081
Torres, N. S., Abercrombie, J. J., Srinivasan, A., Lopez-Ribot, J. L., Ramasubramanian, A. K., Leung, K. P. (2016). Screening a commercial library of pharmacologically active small molecules against staphylococcus aureus biofilms. Antimicrobial Agents Chemother. 60, 5663–5672. doi: 10.1128/AAC.00377-16
Vaterrodt, A., Thallinger, B., Daumann, K., Koch, D., Guebitz, G. M., Ulbricht, M. (2016). Antifouling and antibacterial multifunctional polyzwitterion/enzyme coating on silicone catheter material prepared by electrostatic layer-by-layer assembly. Langmuir 32, 1347–1359. doi: 10.1021/acs.langmuir.5b04303
Vila, T., Kong, E. F., Montelongo-Jauregui, D., Van Dijck, P., Shetty, A. C., Mccracken, C., et al. (2021). Therapeutic implications of c. albicans-s. aureus mixed biofilm in a murine subcutaneous catheter model of polymicrobial infection. Virulence 12, 835–851. doi: 10.1080/21505594.2021.1894834
Wang, H., Tong, H., Liu, H., Wang, Y., Wang, R., Gao, H., et al. (2018). Effectiveness of antimicrobial-coated central venous catheters for preventing catheter-related blood-stream infections with the implementation of bundles: a systematic review and network meta-analysis. Ann. Intensive Care 8, 71. doi: 10.1186/s13613-018-0416-4
Wiederhold, N. P., Patterson, T. F., Srinivasan, A., Chaturvedi, A. K., Fothergill, A. W., Wormley, F. L., et al. (2017). Repurposing auranofin as an antifungal: In vitro activity against a variety of medically important fungi. Virulence 8, 138–142. doi: 10.1080/21505594.2016.1196301
Wolcott, R. (2021). Biofilm and catheter-related bloodstream infections. Br. J. Nurs. 30, S4–S9. doi: 10.12968/bjon.2021.30.8.S4
Yousif, A., Jamal, M. A., Raad, I. (2015). “Biofilm-based central line-associated bloodstream infections,” in Biofilm-based healthcare-associated infections: volume I, 157–179.
Keywords: antimicrobial catheter, auranofin, biofilm, Candida albicans, drug-coated catheter, Staphylococcus aureus
Citation: Felix L, Whitely C, Tharmalingam N, Mishra B, Vera-Gonzalez N, Mylonakis E, Shukla A and Fuchs BB (2023) Auranofin coated catheters inhibit bacterial and fungal biofilms in a murine subcutaneous model. Front. Cell. Infect. Microbiol. 13:1135942. doi: 10.3389/fcimb.2023.1135942
Received: 02 January 2023; Accepted: 24 April 2023;
Published: 29 May 2023.
Edited by:
Ashwini Chauhan, Tripura University, IndiaReviewed by:
Neha Jain, Indian Institute of Technology Jodhpur, IndiaMegan R. Kiedrowski, University of Alabama at Birmingham, United States
Copyright © 2023 Felix, Whitely, Tharmalingam, Mishra, Vera-Gonzalez, Mylonakis, Shukla and Fuchs. This is an open-access article distributed under the terms of the Creative Commons Attribution License (CC BY). The use, distribution or reproduction in other forums is permitted, provided the original author(s) and the copyright owner(s) are credited and that the original publication in this journal is cited, in accordance with accepted academic practice. No use, distribution or reproduction is permitted which does not comply with these terms.
*Correspondence: Beth Burgwyn Fuchs, aGVsZW5fZnVjaHNAYnJvd24uZWR1