- 1Medical Research Center, Kantonspital St. Gallen, St. Gallen, Switzerland
- 2Division of Infectious Diseases and Hospital Epidemiology, Kantonsspital St. Gallen, St. Gallen, Switzerland
- 3Clinic of Infectious Diseases and Hospital Hygiene, Kantonsspital Aarau, Aarau, Switzerland
- 4Department of Microbiology, Institute for Laboratory Medicine, Kantonsspital Aarau, Aarau, Switzerland
- 5Institute of Medical Microbiology, University of Zurich, Zurich, Switzerland
- 6Division of Human Microbiology, Centre for Laboratory Medicine, St. Gallen, Switzerland
- 7Medical and Molecular Microbiology, Faculty of Science and Medicine, University of Fribourg, Fribourg, Switzerland
Background: Increasing reports of multidrug resistance (MDR) in clinical Pseudomonas aeruginosa have led to a necessity for new antimicrobials. Ceftazidime-avibactam (CZA) is indicated for use against MDR P. aeruginosa across a broad range of infection types and particularly those that are carbapenem resistant. This study sought to determine the molecular mechanisms of CZA and imipenem (IPM)-resistance in clinical P. aeruginosa isolates obtained from Swiss hospitals.
Methods: Clinical P. aeruginosa isolates were obtained from inpatients in three hospitals in Switzerland. Susceptibility was determined by either antibiotic disc testing or broth microdilution according to EUCAST methodology. AmpC activity was determined using cloxacillin and efflux activity was determined using phenylalanine-arginine β-naphthylamide, in agar plates. Whole Genome Sequencing was performed on 18 clinical isolates. Sequence types (STs) and resistance genes were ascertained using the Centre for Genomic Epidemiology platform. Genes of interest were extracted from sequenced isolates and compared to reference strain P. aeruginosa PAO1.
Results: Sixteen different STs were identified amongst the 18 isolates in this study indicating a high degree of genomic diversity. No carbapenemases were detected but one isolate did harbor the ESBL blaPER-1. Eight isolates were CZA-resistant with MICs ranging from 16-64 mg/L, and the remaining ten isolates had either low/wildtype MICs (n=6; 1-2 mg/L) or elevated, but still susceptible, MICs (n=4; 4-8 mg/L). Ten isolates were IPM-resistant, seven of which had mutations resulting in truncations of OprD, and the remaining nine IPM-susceptible isolates had intact oprD genes. Within CZA-R isolates, and those with reduced susceptibility, mutations resulting in ampC derepression, OprD loss, mexAB overexpression and ESBL (blaPER-1) carriage were observed in various combinations and one harbored a truncation of the PBP4 dacB gene. Within the six isolates with wildtype-resistance levels, five had no mutations that would affect any antimicrobial resistance (AMR) genes of interest when compared to PAO1.
Conclusion: This preliminary study highlights that CZA-resistance in P. aeruginosa is multifactorial and could be caused by the interplay between different resistance mechanisms including ESBL carriage, increased efflux, loss of permeability and derepression of its intrinsic ampC.
1 Introduction
Pseudomonas aeruginosa is a leading cause of hospital acquired infections, belonging to the ESKAPE pathogens list, and since 2017 has been indicated as “critical” on the WHO’s priority pathogens list for the research and development of new antibiotics. (World Health Organization, 2017; Horcajada et al., 2019). Ceftazidime-avibactam is one of the last resort antimicrobial agents for the treatment of carbapenem-resistant, Gram-negative bacteria (Simon et al., 2022). Reports on carbapenem-resistant P. aeruginosa (CRPA) have increased significantly in recent years and caused much concern, particularly since CRPA infections have been shown to be a significant risk factor for patient mortality (Buehrle et al., 2017). Avibactam (AVI), is a non-β-lactam-based diazabicyclooctane molecule with activity against class A, C and some class D β-lactamases. It is marketed for use in combination with the broad-spectrum cephalosporin ceftazidime, for the treatment of complicated and/or MDR P. aeruginosa infections including CRPA (Pfizer, 2019). AVI functions by forming a covalent and reversible bond to the nucleophilic serine of the serine β-lactamases following ring opening, inhibiting the activity of those β-lactamases. Previous studies on the molecular basis of resistance to CZA in P. aeruginosa, both on clinical isolates and in vitro obtained mutants, have shown that CZA resistance has been associated with the production of metallo-β-lactamases (MBLs)-against which AVI has no activity- altered permeability, increased efflux, and the production of other β-lactamases, including blaPER and some class D enzymes (Ortiz de la Rosa et al., 2019; Wang et al., 2020).The overexpression of efflux pump MexAB, in combination with AmpC derepression, has been shown to result in reduced susceptibility to CZA, suggesting that MexAB may play a role in the efflux of AVI (Chalhoub et al., 2018).
This exploratory study sought to examine the mechanisms of ceftazidime-avibactam (CZA) and imipenem (IPM) resistance in a collection of clinical P. aeruginosa isolates obtained from Swiss hospitals using a combination of phenotypic testing and whole genome sequencing (WGS).
2 Methods
Over a twelve-month period, laboratories from three Swiss Hospitals (Cantonal Hospital of Aarau, University Hospital of Basel, and Cantonal Hospital of St. Gallen) were asked to randomly provide P. aeruginosa isolates, which were tested either resistant to CZA or harbored elevated MICs (≥ 4-8 mg/L). In addition, five wild-type P. aeruginosa were included. Antibiotic susceptibility testing was performed by either disk diffusion or by broth micro-dilution, according to CLSI methodology (Clinical and Laboratory Standards Institute, 2018), and interpreted according to EUCAST guidelines (EUCAST. Breakpoint Tables for Interpretation of MICs and Zone Diameters, Version 12.0). AmpC and efflux activity were determined by disk testing in the presence/absence of either 2000 mg/L cloxacillin (inhibitor of AmpC activity) or 25 mg/L phenylalanine-arginine β-naphthylamide (PaβN-efflux pump inhibitor) in Muller Hinton agar. Zone diameter differences ≥ 5 mm were considered significant and indicative of AmpC or efflux activity. All susceptibility testing and disk testing experiments were performed in duplicate. Whole genome sequencing (WGS) on 18 clinical isolates was performed as previously described (Findlay et al., 2020). Briefly, WGS was performed by MicrobesNG (https://microbesng.uk/) on a HiSeq 2500 instrument (Illumina, San Diego, CA, USA) using 2x250 bp paired end reads. Reads were trimmed using Trimmomatic (Bolger et al., 2014), assembled into contigs using SPAdes 3.13.0 (http://cab.spbu.ru/software/spades/) and contigs were annotated using Prokka (Seemann, 2014). Resistance genes and sequence types were assigned using the ResFinder and MLST 2.0 on the Centre for Genomic Epidemiology (http://www.genomicepidemiology.org/) platform (Curran et al., 2004; Zankari et al., 2012). Chromosomally encoded genes of interest related to antimicrobial resistance (e.g., efflux genes, porins, as listed in Supplementary table 1), as identified in previous studies, were manually extracted from the SPAdes assemblies and Prokka annotation files of sequenced isolates and compared to reference strain P. aeruginosa PAO1 (NC_002516.2) (Supplementary Table 1).
Sequence data from this study was submitted to the National Center for Biotechnology Information’s Sequence Read Archive (BioProject no. PRJNA914238).
3 Results
Eighteen isolates with varying CZA susceptibilities were examined in this study. Eight isolates were CZA resistant with MICs ranging from 16-64 mg/L (Table 1). The remaining ten isolates had either MICs that were considered wild-type (1-2 mg/L; n=6) or exhibited elevated but still susceptible MICs (4-8 mg/L; n=4) (EUCAST. Breakpoint Tables for Interpretation of MICs and Zone Diameters, Version 12.0).
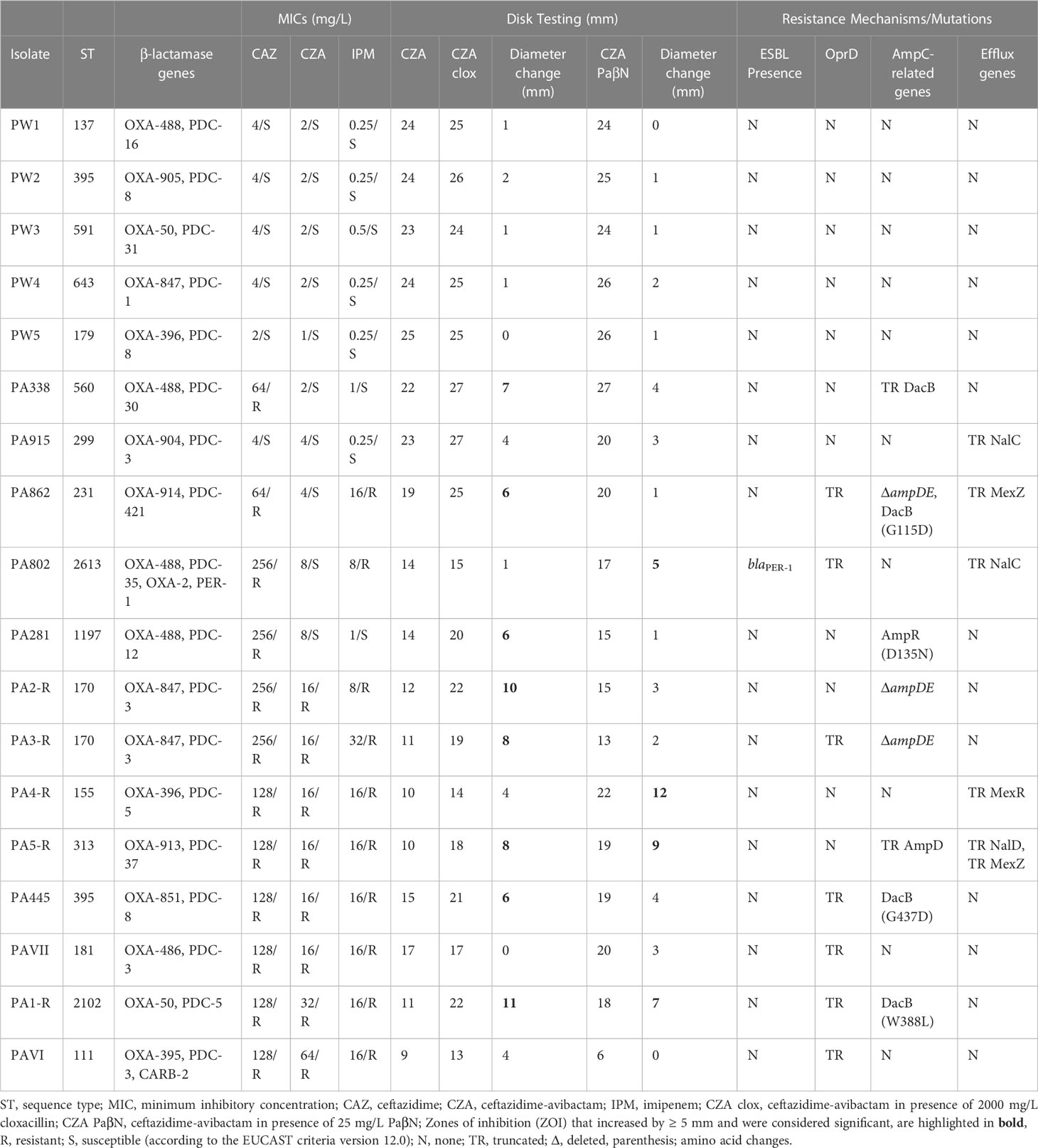
Table 1 Characteristics of the isolates in this study as identified by susceptibility testing and whole genome sequencing.
Sixteen different STs were identified amongst the 18 isolates in this study, all represented by single isolates with the exception of ST170 and ST395, for which there were two representatives each.
Ten allelic variants of the intrinsic, chromosomally encoded P. aeruginosa narrow spectrum β-lactamases blaPDC (AmpC enzyme), were identified amongst the 18 isolates.
Of β-lactamases, a blaOXA-50-like element was found in all isolates comprising of 9 allelic variants respectively. In addition, PA802 (exhibiting a CZA MIC of 8 mg/L) harbored both the narrow spectrum β-lactamase blaOXA-2 and ESBL blaPER-1, and PAVI (exhibiting a CZA MIC of 64 mg/L) the narrow spectrum β-lactamase blaCARB-2. One novel blaPDC and four novel blaOXA-50-like variants were identified and submitted to the GenBank nucleotide database under accession numbers MT195536 (for blaPDC-421), and MT210603, MT210600, MT210601, MT210602 (for blaOXA-904, -905, -913, and -914 respectively). No known carbapenemases were detected in any of the isolates.
Resistance genes of interest (according to Supplementary Table 1) were analyzed and mutations or deletions associated with porin deficiency (OprD loss), ampC derepression, and increased efflux by the MexAB or MexXY pumps, were found. Notably five isolates exhibiting CZA resistant or elevated MICs, were found to harbor the following mutations, leading to truncated proteins, and linked to efflux upregulation (see Table 1): truncations in NalC (PA915 and PA802), NalD (PA5-R) and MexR (PA4-R) which would lead to mexAB overexpression. Addition of the efflux pump inhibitor PAβN exhibited a significant effect on isolates PA802, PA5-R and PA4-R. Two isolates (PA862 and PA5-R), harbored a deleterious mutation in mexZ, which would lead to mexXY overexpression. No significant effect was observed with PAβN in PA862, whereas a zone diameter difference of 9 mm was seen in PA5-R (which in addition harbored a NalD truncation), suggesting that efflux pump MexXY does not play a significant role in CZA resistance.
Concomitant IPM resistance was detected in all isolates that were CZA resistant, including in two isolates with elevated but still susceptible MICs for CZA (Table 1). Within the 10 IPM-resistant isolates, seven were found to have mutations in oprD resulting in truncations, and 2/3 remaining IPM-resistant isolates with an intact oprD harbored deletions or truncations of ampD and/or ampE.
All eight isolates with mutations related to AmpC hyperproduction, including deletions of ampD (PA5-R), ampDE (PA2-R, PA3-R, PA862), ampR (PA281) mutations and dacB mutations or truncations (PA1-R, PA338, PA445, PA862), exhibited significantly increased CZA zones of inhibition in the presence of cloxacillin.
Within the five wild-type isolates (PW 1-5, that were susceptible to ceftazidime, CZA and IPM), no mutations were found that would affect any AMR genes of interest for this study. Overall, within the CZA resistant isolates or those with elevated MICs to CZA, mutations associated with AmpC derepression, OprD loss, mexAB overexpression, mexXY overexpression and the aforementioned ESBL (blaPER-1) carriage were observed in various combinations.
4 Discussion
We observed a high degree of genomic diversity and ten different allelic variants of the PDC enzyme in the 18 clinical P. aeruginosa from three Swiss hospitals. Notably, none of the PDC enzyme variants harbored any of the amino acid changes that have previously been associated with reduced susceptibility to CZA (Slater et al., 2020). Amongst the alleles, one novel blaPDC and four novel blaOXA-50-like variants were identified.
The increase in CZA zone of inhibition in the presence of cloxacillin in isolates with dacB mutations or truncations might be explained by the role of dacB- which encodes for penicillin-binding protein PBP4- in triggering derepression of ampC relying on a functional AmpR (Moya et al., 2009; Sanz-García et al., 2018). In a previous in-vitro study, a G115S mutation could be selected within dacB following challenge by ceftazidime, and was linked to ampC derepression (Sanz-García et al, 2018). We detected G115D in a ceftazidime resistant isolate that had elevated, but still susceptible MIC to CZA. Further mutations within dacB were detected in two isolates (G437D and W388L), both of which showed high-level resistance to ceftazidime and were resistant to CZA. However, the role of dacB in CZA-resistance needs further investigation.
The role of ampC derepression in CZA-resistance has been evaluated in 26 AmpC mutant P. aeruginosa strains in vitro by Mushtaq et al. (Mushtaq et al., 2010); Avibactam reversed AmpC-mediated – high-level- ceftazidime resistance in P. aeruginosa, reducing MICs for fully derepressed mutants, except in one isolate, that in addition had high-level efflux. On the other hand, Slater et al. could show that AmpC mutants, bearing mutations E247K G183D, T96I, and ΔG229–E247 enhanced the catalytic efficiency of AmpC toward ceftolozane and ceftazidime while simultaneously reducing susceptibility to inhibition by avibactam (Slater et al., 2020). More biochemical studies are needed to elucidate the role of AmpC and CZA-resistance in P. aeruginosa.
We did not detect carbapenemases in any of the isolates. In a two-years surveillance of more than 2000 P. aeruginosa isolates from the United States, carbapenemases were detected only in 5.9% and 1.0% of 781 IPM- and 119 CZA-resistant isolates respectively (Karlowsky et al., 2022b). The rate of susceptibility for CZA amongst multidrug-resistant (MDR) isolates was 63.3% in P. aeruginosa. However, a raise of MIC for CZA amongst all isolates, exceeding the susceptibility-breakpoint, was observed from 2018 to 2020, whereas only a minority of the isolates carried an MBL (Karlowsky et al., 2022b), suggesting adaptive resistance mechanisms as causative for the CZA-resistance. In a 5-year survey, from 2014 to 2019, of more than 7000 P. aeruginosa isolates, the annual percent susceptibility rates from North America were higher for CZA (96-99.6%) than for isolates from Europe (90.3-93.1%), 25% of the distinct carbapenem-resistant isolates were also CZA-resistant (Karlowsky et al., 2022a), however, no data was acquired on prevalence of carbapenemases in these specific isolates. Data from more than 4000 P. aeruginosa isolates collected from six Latin American countries between 2015 and 2020, revealed a CZA susceptibility rate of approximately 86% amongst all isolates (Wise et al., 2023). Although CZA was the most active antimicrobial agent tested against the subset of multidrug-resistant (MDR) P. aeruginosa, their susceptibility was substantially lower with 53.9% in isolates from 2015-2017, dropping to 45.3% in those from 2018-2020, suggesting increased presence of MBLs, against which CZA is inactive (Wise et al., 2023).
One or our isolates exhibiting an CZA MIC at the breakpoint according to EUCAST criteria (EUCAST. Breakpoint Tables for Interpretation of MICs and Zone Diameters, Version 12.0., n.d.), harbored both the narrow spectrum β-lactamase blaOXA-2 and ESBL blaPER-1. A recent study has shown that blaPER-1 alone is capable of conferring CZA-resistance when expressed in a high copy number recombinant plasmid in PAO1 (Ortiz de la Rosa et al., 2019), suggesting that this gene is at least in part responsible for raised CZA MIC observed in our isolate.
We found mutations or deletions associated with porin deficiency (OprD loss), ampC derepression, and increased efflux by the MexAB or MexXY pumps. A significant association between CZA-resistance and MexZ disruption was observed in P. aeruginosa in a recent study (Castanheira et al., 2019), the authors however concluded, that the role of mexZ disruption in CZA-resistance is yet unclear. Despite deleterious mutations in mexZ- a transcriptional repressor of the mexXY operon (Yamamoto et al., 2009)- no significant effect was observed with addition of the efflux pump inhibitor PAβN –the latter suggesting that the MexXY efflux pump does not play a major role in CZA-resistance. However, efflux pump inhibition exhibited a significant effect in isolates that harbored loss of function mutations in genes that act as transcriptional repressors for the mexAB-oprM multidrug efflux operon, namely mexR (Cabot et al., 2016) and nalD (Braz et al., 2016), indicating a major role of the MexAB-OprM efflux pump in CZA-resistance. The mexAB-oprM operon is regulated directly by the transcriptional repressors MexR and NalD and indirectly by NalC (Aguilar-Rodea et al., 2022). This might explain the fact, that efflux pump inhibition revealed significant differences in zone-diameters in the isolates with loss of function of mexR and nalD, but less so in the isolates with NalC truncation only. These observations are in line with the recent study on 46 CZA-resistant P. aeruginosa (Castanheira et al., 2019), in which increased expression of mexE and mexX, in contrast to MexAB-OprM overexpression, were not significantly associated with CZA-resistance. In addition, MexR disruption and NalD were more frequently observed with CZA-resistant isolates when compared to susceptible counterparts (Castanheira et al., 2019).
4.1 Limitations
Our exploratory study has several limitations; the sample size is small and clinical information, such as type of infection and mainly data on antimicrobial pre-treatment were unavailable. Thus we were not analyze these risk factors associated with CZA resistance. Furthermore, complementation assays are needed to prove the role of mutations on resistance. Nevertheless, our data adds to a growing body of evidence on the multifactorial CZA-resistance in P. aeruginosa.
5 Conclusion
This preliminary study highlights that CZA-resistance in P. aeruginosa is multifactorial and can be caused by the interplay between different resistance mechanisms including ESBL carriage, increased efflux, loss of permeability, and derepression of the intrinsic ampC whilst IPM-resistance appears to be mainly due to OprD loss as has been extensively reported previously (Trias and Nikaido, 1990; Shu et al., 2017). It is clear that CZA-R in P. aeruginosa is multi-faceted and complex, and that we do not yet know all of the mechanisms involved in conferring this phenotype. It is essential that further studies be undertaken to understand how resistance develops to CZA and to identify antibiotics that may promote or induce resistance to CZA, so that steps can be taken to limit the development, thereby extending the life of this increasingly important antibiotic/inhibitor combination.
Data availability statement
The data presented in the study are deposited in the NCBI GenBank repository, accession numbers MT195536 (forblaPDC-421), and MT210603, MT210600, MT210601, MT210602 (for blaOXA-904, -905, -913, and -914 respectively), and WGS data is deposited in the Sequence Read Archive(BioProject no. PRJNA914238).
Author contributions
Writing – Original Draft: JF and BB. Writing – Review and Editing: all authors; Conceptualization: JF and BB; Investigation; BB, VG, AE, SS, and ON; Analysis: AB and JF. Funding acquisition: BB. All authors contributed to the article and approved the submitted version.
Funding
BB has been funded by the research grant of Kantonsspital St. Gallen.
Acknowledgments
Genome sequencing was provided by MicrobesNG (http://www.microbesng.uk). We thank the Division of Clinical Bacteriology and Mycology for the provided bacterial isolates. We would like to thank the Research Committee of Kantonsspital St. Gallen for providing the research grant. This study was presented at the 30th European Congress of Clinical Microbiology & Infectious Diseases, in Paris, France (Abstract No 6401).
Conflict of interest
The authors declare that the research was conducted in the absence of any commercial or financial relationships that could be construed as a potential conflict of interest.
Publisher’s note
All claims expressed in this article are solely those of the authors and do not necessarily represent those of their affiliated organizations, or those of the publisher, the editors and the reviewers. Any product that may be evaluated in this article, or claim that may be made by its manufacturer, is not guaranteed or endorsed by the publisher.
Supplementary material
The Supplementary Material for this article can be found online at: https://www.frontiersin.org/articles/10.3389/fcimb.2023.1098944/full#supplementary-material
References
Aguilar-Rodea, P., Zúñiga, G., Cerritos, R., Rodríguez-Espino, B. A., Gomez-Ramirez, U., Nolasco-Romero, C. G., et al. (2022). Nucleotide substitutions in the mexR, nalC and nalD regulator genes of the MexAB-OprM efflux pump are maintained in pseudomonas aeruginosa genetic lineages. PloS One 17, e0266742. doi: 10.1371/journal.pone.0266742
Bolger, A. M., Lohse, M., Usadel, B. (2014). Trimmomatic: a flexible trimmer for illumina sequence data. Bioinformatics 30, 2114–2120. doi: 10.1093/bioinformatics/btu170
Braz, V. S., Furlan, J. P. R., Fernandes, A. F. T., Stehling, E. G. (2016). Mutations in NalC induce MexAB-OprM overexpression resulting in high level of aztreonam resistance in environmental isolates of Pseudomonas aeruginosa. FEMS Microbiol. Lett. 363, fnw166. doi: 10.1093/femsle/fnw166
Buehrle, D. J., Shields, R. K., Clarke, L. G., Potoski, B. A., Clancy, C. J., Nguyen, M. H. (2017). Carbapenem-resistant pseudomonas aeruginosa bacteremia: risk factors for mortality and microbiologic treatment failure. Antimicrob. Agents Chemother. 61, e01243–e01216. doi: 10.1128/AAC.01243-16
Cabot, G., Zamorano, L., Moyà, B., Juan, C., Navas, A., Blázquez, J., et al. (2016). Evolution of pseudomonas aeruginosa antimicrobial resistance and fitness under low and high mutation rates. Antimicrob. Agents Chemother. 60, 1767–1778. doi: 10.1128/AAC.02676-15
Castanheira, M., Doyle, T. B., Smith, C. J., Mendes, R. E., Sader, H. S. (2019). Combination of MexAB-OprM overexpression and mutations in efflux regulators, PBPs and chaperone proteins is responsible for ceftazidime/avibactam resistance in pseudomonas aeruginosa clinical isolates from US hospitals. J. Antimicrob. Chemother. 74, 2588–2595. doi: 10.1093/jac/dkz243
Chalhoub, H., Sáenz, Y., Nichols, W. W., Tulkens, P. M., Van Bambeke, F. (2018). Loss of activity of ceftazidime-avibactam due to MexAB-OprM efflux and overproduction of AmpC cephalosporinase in Pseudomonas aeruginosa isolated from patients suffering from cystic fibrosis. Int J Antimicrob Agents 52 (5), 697–701. doi: 10.1016/j.ijantimicag.2018.07.027
Clinical and Laboratory Standards Institute (2018). Methods for dilution antimicrobial susceptibility tests for bacteria that grow aerobically, 11th ed. Approved standard M07-A10. Clinical and Laboratory Standards Institute, Wayne, PA.
Curran, B., Jonas, D., Grundmann, H., Pitt, T., Dowson, C. G. (2004). Development of a multilocus sequence typing scheme for the opportunistic pathogen pseudomonas aeruginosa. J. Clin. Microbiol. 42, 5644–5649. doi: 10.1128/JCM.42.12.5644-5649.2004
EUCAST Breakpoint tables for interpretation of MICs and zone diameters, version 12.0. (n.d.). Available at: https://www.eucast.org/fileadmin/src/media/PDFs/EUCAST_files/Breakpoint_tables/v_12.0_Breakpoint_Tables.pdf.
Findlay, J., Gould, V. C., North, P., Bowker, K. E., Williams, M. O., MacGowan, A. P., et al. (2020). Characterization of cefotaxime-resistant urinary escherichia coli from primary care in south-West England 2017–18. J. Antimicrob. Chemother. 75, 65–71. doi: 10.1093/jac/dkz397
Horcajada, J. P., Montero, M., Oliver, A., Sorlí, L., Luque, S., Gómez-Zorrilla, S., et al. (2019). Epidemiology and treatment of multidrug-resistant and extensively drug-resistant pseudomonas aeruginosa infections. Clin. Microbiol. Rev. 32, e00031–e00019. doi: 10.1128/CMR.00031-19
Karlowsky, J. A., Hackel, M. A., Takemura, M., Yamano, Y., Echols, R., Sahm, D. F. (2022a). In vitro susceptibility of gram-negative pathogens to cefiderocol in five consecutive annual multinational SIDERO-WT surveillance studies 2014 to 2019. Antimicrob. Agents Chemother. 66, e01990–e01921. doi: 10.1128/AAC.01990-21
Karlowsky, J. A., Lob, S. H., DeRyke, C. A., Hilbert, D. W., Wong, M. T., Young, K., et al. (2022b). In vitro activity of ceftolozane-tazobactam, imipenem-relebactam, ceftazidime-avibactam, and comparators against pseudomonas aeruginosa isolates collected in united states hospitals according to results from the SMART surveillance program 2018 to 2020. Antimicrob. Agents Chemother. 66, e00189–e00122. doi: 10.1128/aac.00189-22
Moya, B., Dötsch, A., Juan, C., Blázquez, J., Zamorano, L., Haussler, S., et al. (2009). β-lactam resistance response triggered by inactivation of a nonessential penicillin-binding protein. PloS Pathog. 5, e1000353. doi: 10.1371/journal.ppat.1000353
Mushtaq, S., Warner, M., Livermore, D. M. (2010). In vitro activity of ceftazidime+NXL104 against pseudomonas aeruginosa and other non-fermenters. J. Antimicrob. Chemother. 65, 2376–2381. doi: 10.1093/jac/dkq306
Ortiz de la Rosa, J.-M., Nordmann, P., Poirel, L. (2019). ESBLs and resistance to ceftazidime/avibactam and ceftolozane/tazobactam combinations in escherichia coli and pseudomonas aeruginosa. J. Antimicrob. Chemother. 74, 1934–1939. doi: 10.1093/jac/dkz149
Pfizer (2019) Zavicefta: summary of product characteristics. Available at: https://www.medicines.org.uk/emc/product/2465.
Sanz-García, F., Hernando-Amado, S., Martínez, J. L. (2018). Mutation-driven evolution of pseudomonas aeruginosa in the presence of either ceftazidime or ceftazidime-avibactam. Antimicrob. Agents Chemother. 62 (10), e01379–18. doi: 10.1128/AAC.01379-18
Seemann, T. (2014). Prokka: rapid prokaryotic genome annotation. Bioinforma. Oxf. Engl. 30, 2068–2069. doi: 10.1093/bioinformatics/btu153
Shu, J.-C., Kuo, A.-J., Su, L.-H., Liu, T.-P., Lee, M.-H., Su, I.-N., et al. (2017). Development of carbapenem resistance in pseudomonas aeruginosa is associated with OprD polymorphisms, particularly the amino acid substitution at codon 170. J. Antimicrob. Chemother. 72, 2489–2495. doi: 10.1093/jac/dkx158
Simon, M., Gerlach, R. G., Pfeifer, Y., Pfennigwerth, N., Gatermann, S. G., Schröder, A., et al. (2022). Increased zinc levels facilitate phenotypic detection of ceftazidime-avibactam resistance in metallo-β-lactamase-producing gram-negative bacteria. Front. Microbiol. 13. doi: 10.3389/fmicb.2022.977330
Slater, C. L., Winogrodzki, J., Fraile-Ribot, P. A., Oliver, A., Khajehpour, M., Mark, B. L. (2020). Adding insult to injury: mechanistic basis for how AmpC mutations allow pseudomonas aeruginosa to accelerate cephalosporin hydrolysis and evade avibactam. Antimicrob. Agents Chemother. 64, e00894–e00820. doi: 10.1128/AAC.00894-20
Trias, J., Nikaido, H. (1990). Outer membrane protein D2 catalyzes facilitated diffusion of carbapenems and penems through the outer membrane of pseudomonas aeruginosa. Antimicrob. Agents Chemother. 34, 52–57. doi: 10.1128/AAC.34.1.52
Wang, Y., Wang, J., Wang, R., Cai, Y. (2020). Resistance to ceftazidime–avibactam and underlying mechanisms. J. Glob. Antimicrob. Resist. 22, 18–27. doi: 10.1016/j.jgar.2019.12.009
Wise, M. G., Karlowsky, J. A., Luengas, E. L., Valdez, R. R., Sahm, D. F. (2023). Epidemiology and in vitro activity of ceftazidime-avibactam and comparator agents against multidrug-resistant isolates of enterobacterales and pseudomonas aeruginosa collected in Latin America as part of the ATLAS surveillance program in 2015-2020. Braz. J. Infect. Dis. 27 (3), 102759. doi: 10.1016/j.bjid.2023.102759
World Health Organization (2017) Global priority list of antibiotic-resistant bacteria to guide research, discovery, and development of new antibiotics. Available at: https://www.who.int/news/item/27-02-2017-who-publishes-list-of-bacteria-for-which-new-antibiotics-are-urgently-needed.
Yamamoto, M., Ueda, A., Kudo, M., Matsuo, Y., Fukushima, J., Nakae, T., et al. (2009). Role of MexZ and PA5471 in transcriptional regulation of mexXY in pseudomonas aeruginosa. Microbiology 155, 3312–3321. doi: 10.1099/mic.0.028993-0
Keywords: Pseudomonas aeruginosa, ceftazidime-avibactam (CZA), molecular resistance mechanisms, imipenem, whole genome sequencing (WGS)
Citation: Babouee Flury B, Bösch A, Gisler V, Egli A, Seiffert SN, Nolte O and Findlay J (2023) Multifactorial resistance mechanisms associated with resistance to ceftazidime-avibactam in clinical Pseudomonas aeruginosa isolates from Switzerland. Front. Cell. Infect. Microbiol. 13:1098944. doi: 10.3389/fcimb.2023.1098944
Received: 15 November 2022; Accepted: 05 April 2023;
Published: 25 April 2023.
Edited by:
Piotr Majewski, Medical University of Bialystok, PolandReviewed by:
Pablo Laborda, National Center for Biotechnology (CSIC), SpainRoger M. Echols, Infectious Disease Drug Development Consulting, LLC, United States
Copyright © 2023 Babouee Flury, Bösch, Gisler, Egli, Seiffert, Nolte and Findlay. This is an open-access article distributed under the terms of the Creative Commons Attribution License (CC BY). The use, distribution or reproduction in other forums is permitted, provided the original author(s) and the copyright owner(s) are credited and that the original publication in this journal is cited, in accordance with accepted academic practice. No use, distribution or reproduction is permitted which does not comply with these terms.
*Correspondence: Baharak Babouee Flury, Baharak.baboueeflury@kssg.ch