- 1Department of Pediatric Dentistry, Okayama University Graduate School of Medicine, Dentistry and Pharmaceutical Sciences, Okayama, Japan
- 2Division of Nephrology, Seirei Hamamatsu General Hospital, Hamamatsu, Japan
- 3Department of Nursing, Faculty of Nursing, Seirei Christopher University, Hamamatsu, Japan
- 4Department of General Internal Medicine, Hyogo College of Medicine, Nishinomiya, Japan
- 5Department of Internal Medicine, Japan Self-Defense Iruma Hospital, Iruma, Japan
- 6Department of Pediatric Dentistry, Division of Oral infection and Disease Control, Osaka University Graduate School of Dentistry, Suita, Japan
Streptococcus mutans, a Gram-positive facultative anaerobic bacterium, is a major pathogen of dental caries. The protein Cnm of S. mutans is involved in collagen binding, but its other biological functions are unknown. In this study, a Cnm-deficient isogenic mutant and a complementation strain were generated from a Cnm-positive S. mutans strain to help determine the properties of Cnm. Initially, comparison of the cell surface structure was performed by electron microscopy, which demonstrated that Cnm appears to be localized on the cell surface and associated with a protruding cell surface structure. Deep RNA sequencing of the strains revealed that the defect in Cnm caused upregulated expression of many genes related to ABC transporters and cell-surface proteins, while a few genes were downregulated. The amount of biofilm formed by the Cnm-defective strain increased compared with the parental and complemented strains, but the biofilm structure was thinner because of elevated expression of genes encoding glucan synthesis enzymes, leading to increased production of extracellular polysaccharides. Particular antibiotics, including bacitracin and chloramphenicol, had a lower minimum inhibitory concentration for the Cnm-defective strain than particular antibiotics, including bacitracin and chloramphenicol, compared with the parental and complemented strains. Our results suggest that S. mutans Cnm is located on the cell surface, gives rise to the observed protruding cell surface, and is associated with several biological properties related to membrane permeability.
Introduction
Streptococcus mutans, a Gram-positive facultative anaerobic bacterium, is a major pathogen related to dental caries (Bowen, 2016). The major surface proteins of S. mutans involved in the pathogenicity of dental caries include the 190-kDa protein antigen (PA), glucosyltransferases (GTFB/C/D), and glucan binding proteins (GbpA/B/C/D), all of which can be detected with high frequency in clinical isolates of S. mutans (Matsumoto-Nakano, 2018). Biofilm formation by S. mutans is established via two distinct adhesion mechanisms, the sucrose-dependent and sucrose-independent mechanisms. GTFs and Gbps are major pathogenic proteins in the sucrose-dependent mechanisms, while PA functions in the sucrose-independent mechanism. The importance of the GTFs in cariogenicity, particularly the products of the gtfB and gtfC genes, has been established by many researchers (Bowen and Koo, 2011). Simultaneous synthesis of glucans by GTFB and GTFC is essential for establishment of a matrix that enhances the coherence of bacterial cells and adherence to tooth surfaces, allowing for formation of high-density biofilms (Tamesada et al., 2004; Xiao and Koo, 2010). However, GTFD, which synthesizes water-soluble glucans rich in α-1, 6-glucosidic linkages, has been detected in culture supernatant (Hanada and Kuramitsu, 1989). Binding of S. mutans to glucans formed in situ is mediated by the presence of GTF enzymes and Gbps (Banas and Vickerman, 2003). GbpC appears to be anchored to the cell wall, is somewhat similar to members of the Spa family of oral streptococcal proteins, and is involved in rapid dextran-dependent aggregation in stressful growth conditions (Sato et al., 2004). These proteins participate in bacterial adherence to tooth surface and coordinate the biofilm formation.
Cnm, a cell-wall anchored protein related to binding to type I collagen, is also identified in approximately 10% of clinical isolates of S. mutans (Sato et al., 2004). Cnm has been found to function in the sucrose-independent adhesion mechanism in the biofilm formation involved in colonization of oral tissues, and in adhesion in extraoral infections. Cnm is an important virulence factor in the onset or aggravation of systemic disease (Nakano et al., 2011; Nomura et al., 2013; Naka et al., 2014; Naka et al., 2016; Naka et al., 2018).
Aside from these proteins, there are many membrane transporters in the plasma membrane of S. mutans, which are involved in the absorption of substances for the growth of the bacteria (Ajdic et al., 2002), and export and import of various molecules (Ajdic et al., 2007). Upregulation of and/or the presence of proteins associated with several types of ABC transporter are associated with the efflux of antibiotics in several multidrug-resistant species (Nikaido, 2009). Alteration of the cell-wall architecture affects resistance to antimicrobial compounds (Nikaido, 2009; Fischer et al., 2011). Therefore, the lack of cell surface proteins and/or cell-wall associated proteins may lead to multiple alterations of pathogenic factors of S. mutans, in addition to impaired adherence and biofilm formation.
The purpose of this study was to analyze the localization and function of Cnm, to identify its contribution to the virulence of S. mutans.
Materials and methods
S. mutans strains and culture conditions
S. mutans strain SN74 (serotype e, Cnm-positive) was isolated from the oral cavity of a patient with a severe IgA nephropathy (Naka et al., 2020). The strain was cultured on Mitis-Salivarius (MS; Becton Dickinson, Franklin Lakes, NJ, USA) agar plates containing bacitracin (0.2 U/ml; Sigma Chemical Co., St. Louis, MO, USA) or in brain heart infusion (BHI; Becton Dickinson) broth or Todd Hewitt (TH; Becton Dickinson) broth.
Preparation and purification of Recombinant Cnm protein
The DNA fragment encoding the entire Cnm protein in S. mutans TW871, a strain isolated from blood of a patient with infective endocarditis-complicated subarachnoid hemorrhage, were amplified using specific primer set cnm (pGEX6p-1) (Table 1) and PrimeSTAR® MAX DNA Polymerase (TaKaRa Bio, Shiga, Japan), and ligated into vector pGEX6p-1 (Cytiva, Tokyo, Japan). The PCR amplification reaction was performed in a thermal cycler (iCycler; Bio-Rad, Hercules, CA, USA) with the following cycling parameters: initial denaturation at 95°C for 4 min; 30 cycles of 95°C for 30 s, 60°C for 30 s, and 72°C for 2 min; and a final extension at 72°C for 7 min. The DNA fragments were assembled using the GeneArt® Seamless Cloning and Assembly Kit (Thermo Fisher Scientific, Waltham, MA). rCnm protein was obtained by transforming the plasmid into Escherichia coli BL21 (DE3) (Nippon Gene, Tokyo, Japan), as described previously (Takashima et al., 2015). The cells were grown in Luria-Bertani broth to the mid-exponential phase at 37°C, then recombinant protein expression was induced with 1.0 mM isopropyl-1-thio-β-D-galactopyranoside (Wako Pure Chemical Corporation, Osaka, Japan) at 16°C for 24 h. Cells were harvested by centrifugation and pelleted cells were resuspended in phosphate-buffered saline (PBS). Lysozyme (0.25 mg/ml; Roche Diagnostics, Basel, Switzerland) was added and the mixture was incubated at 4°C for 30 min, followed by intermittent sonication on ice for 3 min with a 1 min rest period; and this process was repeated three times. The supernatant was obtained by centrifugation, and applied to a glutathione-Sepharose 4B column (particle size: 90 µm; Cytiva) and eluted with 10 mM glutathione buffer (50 mM Tris-HCl, 10 mM glutathione, pH8.0) at 4°C. Finally, the purified glutathione S-transferase (GST)-fusion protein was freeze‐dried and used in this study.
Generation of antiserum against rCnm
Cnm antiserum was generated by intramuscular administration of rCnm in rabbits, as described previously (Matsumoto-Nakano et al., 2007; Nomura et al., 2012). Briefly, antiserum against Cnm was generated by injecting rabbits (New Zealand White; Oriental Yeast Co. Ltd., Tokyo, Japan) with four intramuscular injections of purified rCnm (1200 μg) emulsified with a block copolymer adjuvant (Titer-Max Gold; CytRx Co., Atlanta, GA, USA) every 2 weeks for a total of 8 weeks. The antibody titer of each antiserum sample was confirmed by an enzyme-linked immunosorbent assay (ELISA) using rCnm.
Construction of Cnm-defective isogenic mutant and complemented strains
A Cnm-defective mutant strain of S. mutans was generated according to the method of Nakano et al., 2004. Initially, the full length of the cnm gene was amplified by PCR using the template of DNA from S. mutans TW871, AmpliTaq® (Life Technologies, Grand Island, NY, USA) and primers cnm-1F and cnm-1R (Table 1) designed based on the cnm sequence of S. mutans TW295 (GenBank accession number AB469913). The amplified DNA fragments were ligated into pGEM-T Easy Vector (Promega Co., Madison, WI, USA) to produce plasmid pTN11 carrying the cnm gene. Next, the erythromycin (EM) resistance gene derived from pVA838 (Macrina et al., 1983) was amplified by PCR using primers cnm-ermF and cnm-ermR (Table 1) and inserted into pTN11 in the middle of the cnm gene (which had been cut with BsmI) to produce pTN12. We then transformed pTN12 into S. mutans strain SN74 using the protocol of Tobian and Macrina (1982) and produced Cnm-defective mutant strain SN74CND in which the cnm gene was inactivated.
To generate a complemented strain, first, the open reading frame region of the cnm gene (GenBank accession number: AB469914) of S. mutans strain TW871 was amplified by PCR using the template of DNA from S. mutans TW871 and primers cnm-HindIII-F and cnm-BamHI-R (Table 1). The resulting DNA was digested with restriction enzymes HindIII and BamHI and ligated into pDL278 (Dunny et al., 1991) to produce a plasmid containing the cnm gene. Then, this plasmid was transduced into S. mutans strain SN74CND to produce complemented strain SN74CNDcomp.
To verify the mutant strains, chromosomal DNA was extracted and PCR was performed using primers cnm-1F and cnm-1R to confirm the full-length sequence of the cnm gene (containing the EM resistance gene where applicable). Reverse transcription PCR (RT-PCR) (Nomura et al., 2005) was used to confirm cnm gene expression. The collagen binding activity of the strains was measured by the method described below.
Bacterial growth rates
Overnight cultures of S. mutans were inoculated into TH medium with the cultures performed in duplicate. Growth curves were determined by monitoring OD550 values at 1-h intervals using a spectrophotometer (GE Healthcare, Fairfield, CT, USA).
Collagen-binding properties of strains
Collagen-binding properties were analyzed according to the protocol described by Waterhouse and Russell (2006) with some modifications. Type I collagen (0.002–2 mg collagen in 0.25 M acetic acid; Sigma) was coated onto 96-well tissue culture plates (Beckton Dickinson) and incubated overnight at 4°C, then the plates were washed and blocked with bovine serum albumin (BSA) solution. Next, the wells were washed and reacted with overnight cultures of S. mutans [1 × 108–1 × 1010 colony-forming units (CFU)] for 3 h at 37°C, then adherent cells were washed and fixed with 25% formaldehyde at room temperature for 30 min. After washing, the adherent cells were stained with 0.05% crystal violet (Wako) for 1 min and washed, then the dye was dissolved by adding 7% acetic acid (200 µl) before determining OD595 values. The results for each strain were expressed as a percentage compared with the binding of parental strain SN74, which was defined as 100%.
Scanning electron microscopy
SEM was performed according to the protocol described by Yuan et al. (2021) to observe morphologic changes of tested strains. Overnight cultures of S. mutans were harvested and pre-fixed with 2% glutaraldehyde and 2% paraformaldehyde at 4°C for 16 h then washed with 0.1 M phosphate buffer (pH 7.4), fixed with 2% osmium tetroxide for 1.5 h, and washed with 0.1 M phosphate buffer (pH 7.4). Then, samples were dehydrated using an ethanol gradient, immersed in t-butyl alcohol for 30 min, and dried with CO2 for 2 h. The prepared specimens were placed on aluminum stubs, coated with osmium (HPC-IS, Vacuum Device, Ibaraki, Japan) and observed by SEM (S-4500, Hitachi, Tokyo, Japan).
Transmission electron microscopy
TEM was performed according to the method of Naka et al. (2020). For pre-fixation, cell specimens were immersed in 0.1 M PBS, pH 7.4, containing 2% glutaraldehyde and 2% paraformaldehyde for 16–18 h. Post-fixation was performed in 2% osmium tetroxide for 1.5 h. After washing with PBS, the specimens were dehydrated in a graded ethanol series and embedded in low viscosity resin (Spurr resin, Polysciences). Then, 80-nm sections were prepared using an ultramicrotome (EM-UC7; Leica, Tokyo, Japan) and stained with uranyl acetate and lead citrate. Specimens were observed by TEM (H-7650, Hitachi, Tokyo, Japan).
Immunogold TEM
Sections (80-nm) were mounted on a 100-mesh nickel grid, and incubated with PBS containing 10% goat serum (GEMINI Bio, San Carlos, CA) and 1% BSA. Sections were incubated with anti-Cnm antibody overnight at 4°C and washed with PBS containing 0.1% BSA >5 times. Then, they were incubated with gold colloid [Anti-IgG (H+L), Rabbit, Goat-Poly, Gold 15 nm; BBI solutions, Crumlin, UK] conjugate with goat anti-rat IgG antibody (Biolegend, SanDiego, CA, USA) and washed three times with PBS containing 0.1% BSA and then washed once with distilled water. Specimens were finally fixed with 2% glutaraldehyde.
RNA extraction and deep sequencing
Total RNAs of S. mutans strains SN74, SN74CND, and SN74CNDcomp were purified as described previously (Matsumoto-Nakano and Kuramitsu, 2006). The quality of enriched mRNA samples was determined using an Agilent Bioanalyzer (Agilent Technologies, Santa Clara, CA, USA).
cDNA libraries were constructed from the enriched mRNA samples by using an NEBNext Ultra Directional RNA Library Prep Kit for Illumina and NEBNext Multiplex Oligonucleotides for Illumina (New England BioLabs, Ipswich, MA, USA), following the protocol of the supplier. Deep sequencing was performed by the NextGen DNA Sequencing Core Laboratory of ICBR at the University of Florida (Gainesville, FL, USA). Read mapping was performed on a Galaxy server hosted by the research computing center at the University of Florida, using Map with Bowtie for Illumina (version 1.1.2).
Gene expression analysis
To confirm the validity of results obtained using RNA-Seq, conventional real-time quantitative reverse transcription PCR (qRT-PCR) was employed to measure changes in the mRNA levels of open reading frames. SuperScript III® Reverse Transcriptase (Invitrogen, Carlsbad, CA, USA) and random primers (Promega) were used to obtain cDNA from DNA-free RNA from S. mutans strains SN74 and SN74CND. PCR was then performed on DNA (as a positive control), cDNA, and MilliQ water (as a negative control), with primers for either the 16S ribosomal RNA (rRNA) gene or a specific gene (Table 2). The qRT-PCR reaction was conducted using SYBR® Green (Bio-Rad Laboratories, Hercules, CA, USA) in an iCycler thermal cycler (Bio-Rad), according to the manufacturer’s protocol. The mRNA expression values were quantified by the ΔΔCT method using 16s rRNA as the internal control.
Fluorescence efflux measurement
Fluorescence measurements were performed using the method described by Ocaktan et al. (1997) with some modifications. Strains SN74, SN74CND, and SN74CNDcomp were grown to OD550 = 0.4 in TH medium and pelleted by centrifugation at 2400 × g for 10 min at 4°C. The cells were then washed with 10 mM NaCl and 50 mM sodium phosphate (pH 7.0), and resuspended in the same buffer. Before fluorescence probe labeling, cultures were adjusted to an optical density of 0.2 at 600 nm.
One milliliter of adjusted sample was labeled with N-phenyl-2-naphtylamine (NPN) reacted at a final concentration of 5 or 10 µg/ml and incubated with light shielding for 30 min. NPN is a fluorescence polarization probe that is a useful indicator of phase transitions in the dispersion of intact membranes (Trauble and Overath, 1973). Following incubation, labeled cultures were centrifuged at 2400 × g for 10 min at 4°C, and the resultant pellets were washed twice with 500 µl of 10 mM NaCl and 50 mM sodium phosphate (pH 7.0). Thereafter, 100-µl samples were plated in 96-well plates (Nunc, Roskilde, Denmark), and fluorescence was determined using a Twinkle LB970 fluorometer (Berthold Technologies GmbH & Co. KG, Bad Wildbad, Germany) with excitation at 355 nm and emission at 460 nm.
Determination of minimum inhibitory concentrations
MICs of antibiotics were determined using a microbroth dilution method previously described by the Clinical and Laboratory Standards Institute (CLSI) (de Jong et al., 2020). Briefly, tested strains in 3 ml of Muller-Hinton broth (Becton Dickinson) supplemented with 4.25 µM MgCl2 and 90 µM CaCl2 and containing two fold serial dilution of antibiotics were placed in sterile 13 mm × 100 mm test tubes. Test strains were cultured in BHI broth at 37°C for 18 h and washed, then 5×105 CFU were added to the tubes containing the antimicrobial agents and incubated at 37°C for 18 h. Breakpoints were set based on CLSI guidelines (de Jong et al., 2020).
Quantitative and structural analyses of biofilms
The ability of strains to form biofilms was evaluated by growing cells in wells of 96-well polystyrene microtiter plates according to previously described protocol (Ardin et al., 2014). TH medium (diluted 1:4) containing 0.1% sucrose was mixed with a pre-grown cell suspension (OD550 = 2.0), and then 100 µl of the samples was inoculated into the individual wells. The plates were incubated anaerobically at 37°C for 48 h. After incubation, formed biofilms were stained with 1% crystal violet (Sigma) for 15 min at room temperature. The plate was next rinsed six times with sterile distilled water to remove loosely bound bacteria, dried, then fixed with 95% ethanol. Stained biofilms were quantified by measuring the absorbance at 570 nm using an ELISA microplate reader (Thermo Fisher Scientific, Waltham, MA, USA). Three independent experiments were performed in triplicate.
Quantitative and structural analyses of biofilms were performed using confocal laser scanning microscopy (CLSM), according to the protocol described by Kuboniwa et al. (2006). The tested strains were separately cultured in 10 ml of TH medium overnight at 37°C, then centrifuged at 2400 × g for 5 min at 4°C and the cells were washed with distilled water. Next, the cells were labeled with hexidium iodide (Invitrogen) and incubated in the dark for 15 min at room temperature. Each cell suspension was adjusted to OD600 = 0.1 in chemically-defined medium (CDM) supplemented with 0.5% sucrose (van de Rijn and Kessler, 1980), and then 100 µl of each suspension was added to a Lab-Tek Chambered #1.0 Borosilicate Coverglass System with eight chambers (Nunc) that had been coated with filtered 25% human saliva to allow biofilm formation. The chambers were incubated at 37°C with light shielding in an anaerobic chamber for 24 h, after which the CDM supplemented with 0.5% sucrose was removed and 100 µl of PBS was added.
Imaging was performed using an LSM 510 confocal laser scanning microscope (version 4.2, Carl Zeiss MicroImaging Co., Ltd., Jena, Germany) at a laser wavelength of 543 nm. Biofilm images of each sample were acquired from three random positions. The obtained confocal microscopy images were analyzed using ImageJ software for Macintosh (version 10.2, National Institute of Health, Bethesda, MD, USA, USA).
Statistical Analysis
Statistical analyses were performed using GraphPad Prism 8 software (Graph Pad Software Inc., San Diego, CA). All data are presented as the mean ± standard deviation of the means. Differences in fluorescence intensity and amount of biofilm formation were assessed using analysis of variance with Bonferroni’s correction. Expression levels of genes (gtfB, gtfC, gtfD, gbpA, gbpB, and gbpC) were compared using analysis of variance with the Mann-Whitney U test. P-values <0.05 were considered statistically significant.
Results
Construction of Cnm-deficient mutant strain
Primer extension analysis was used to determine the cnm transcription sites in S. mutans strain SN74 using primers cnm-1F and cnm-1R (Table 1). Agarose gel electrophoresis of the PCR products showed an amplified band of approximately 1728 bp (Figure 1A). RT-PCR did not amplify the cnm gene from cDNA obtained from Cnm-defective mutant strain SN74CND. In contrast, the cnm gene was amplified from cDNA obtained from strain SN74CNDcomp and the band was the same size as that from the parental strain SN74 (Figure 1B). The growth rates of strains SN74, SN74CND, and SN74CNDcomp were determined. There was no difference in the growth of strain SN74 and SN74CNDcomp whereas that of strain SN74CND was slightly reduced (Supplementary Figure 1). In addition, typical rough colonies were observed on MS-agar plates for strains SN74, SN74CND, and SN74CNDcomp (Supplementary Figure 2). Strain SN74CND could scarcely bind collagen, whereas strain SN74CNDcomp bound collagen at approximately 60% of the level of the parental strain SN74 (Figure 1C).
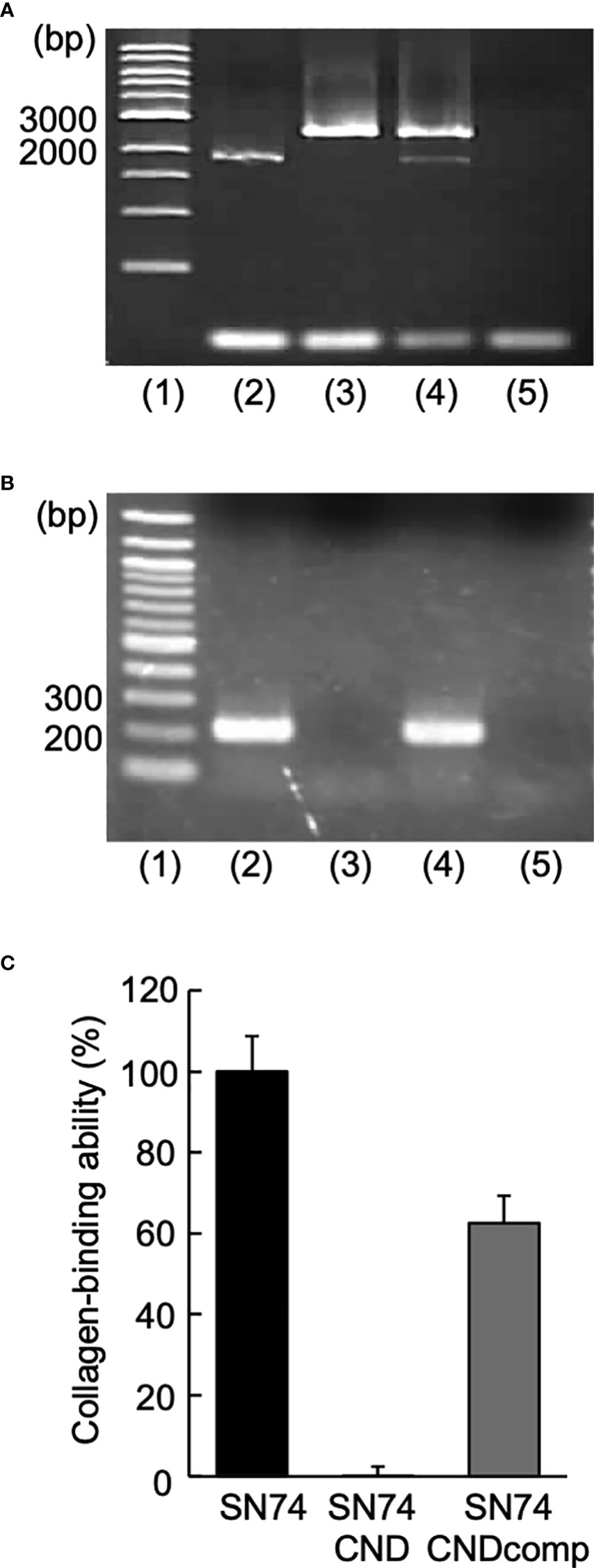
Figure 1 Construction of Streptococcus mutans strains SN74CND and SN74CNDcomp. (A) Confirmation of chromosomal DNA [lane 1: 1-kbp DNA ladder, 2: strain SN74 (the parental strain), 3: strain SN74CND (the cnm deletion strain), 4: strain SN74CNDcomp (complementation of the cnm deletion strain), and 5: MilliQ water as a negative control). (B) Confirmation of cDNA using reverse transcription-PCR (lane 1: 100-bp DNA ladder; 2: strain SN74; 3: strain SN74CND; 4: strain SN74CNDcomp; 5: ultrapure water as a negative control). (C) Collagen binding ability. The results for each strain are expressed as a percentage relative to the binding ability of parental strain SN74, which was defined as 100%. Data are presented as the mean ± SD from five independent experiments.
Generation and purification of rCnm and Cnm antiserum
Purified GST-rCnm fusion protein was subjected to sodium dodecyl sulfate-polyacrylamide gel electrophoresis stained with Coomassie blue (Figure 2A). The expected value of the molecular weight of the GST-rCnm fusion proteins was approximately 120 kDa (Figure 2A).
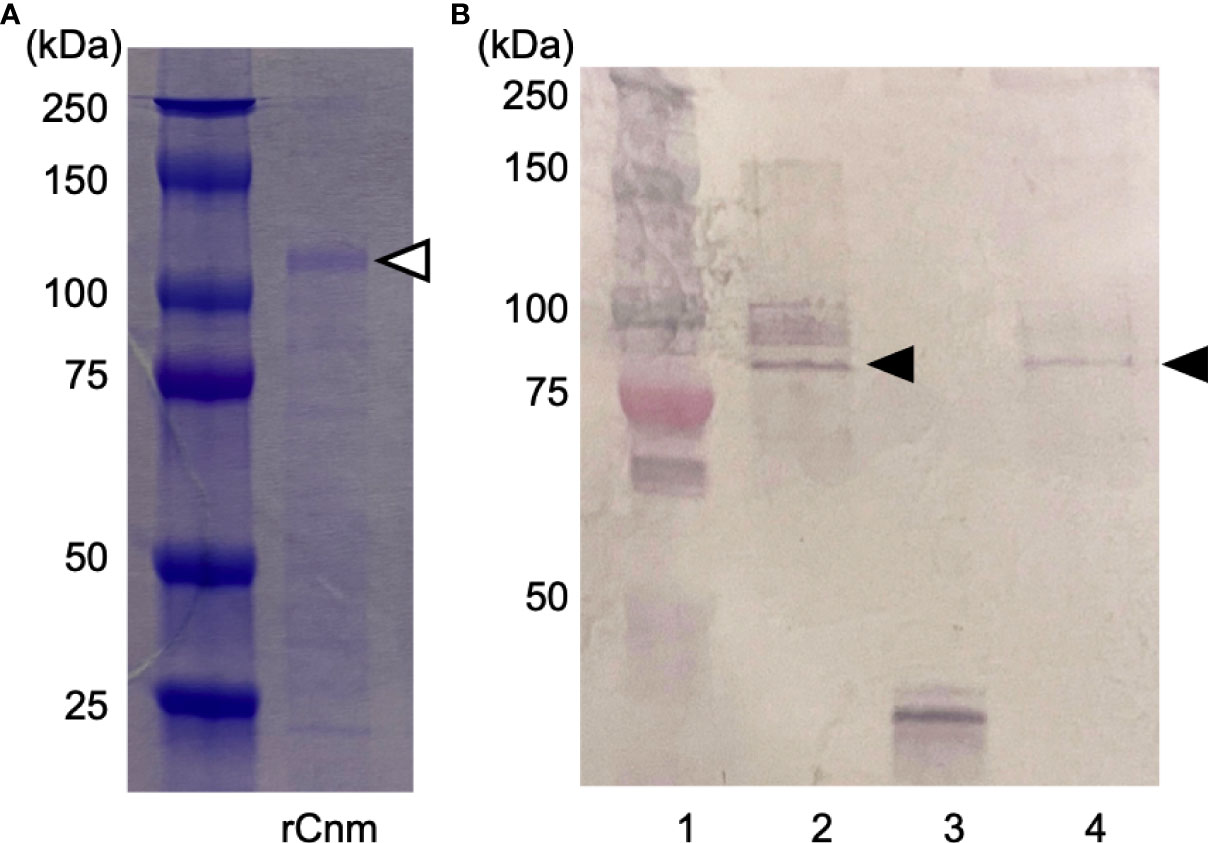
Figure 2 Generation and purification of recombinant Cnm and Cnm antiserum. (A) SDS-PAGE (Coomassie blue staining). The Cnm-glutathione S-transferase fusion protein is indicated with a white arrowhead. (B) Western blot analysis of expression of Cnm (lane 1: protein molecular weight markers; 2: strain SN74; 3: strain SN74CND; 4: strain SN74CNDcomp). Cnm is indicated with a black arrowhead.
To confirm the immunoreactive specificity of our rCnm antiserum, we used cells of strains SN74, SN74CND, and SN74CNDcomp. Western blot analysis produced a positive band in whole cell extracts of strains SN74 and SN74CNDcomp, whereas no such band was observed when using extracts of cells of strain SN74CND (Figure 2B).
Bacterial cell surface conditions and Cnm
SEM images of S. mutans cells were very different for strains SN74 and SN74CND (Figure 3). In SEM images of strain SN74, a bumpy structure of the cell surface was observed, while nothing was observed on the cell surface of strain SN74CND (i.e., it was smooth). The bumpy structure of the cell surface was recovered in strain SN74CNDcomp. Therefore, we observed SEM images of other clinical isolates of S. mutans, and found that strains TW871, TW295, and OMZ175, which have Cnm, had bumpy structures on the cell surface similar to those of strain SN74 (Supplementary Figure 3A). However, bumpy structures were not observed on the cell surfaces of strains MT8148, UA159, and GS5, each of which lacks Cnm (Supplementary Figure 3B). To confirm that the protruding structures involved Cnm, we performed immunogold TEM imaging using the anti-Cnm antibody (Figure 4). In the TEM images of strain SN74, numerous adherent gold colloidal particles were observed around the bacterial cell wall, but these were not observed in images of strain SN74CND (Figure 4). TEM images of strain SN74CNDcomp showed recovery of gold colloidal particle attachment around the bacterial cell wall (Figure 4). In addition, TEM images also showed differences in the peptidoglycan layer between strains SN74 and SN74CND (Figure 4). The peptidoglycan layer of strain SN74 appeared clear and smooth. In contrast, the peptidoglycan layer of strain SN74CND was obscure. These results indicated that the protrusion from the cell surface involved Cnm and the presence of Cnm affects the bacterial surface conditions.
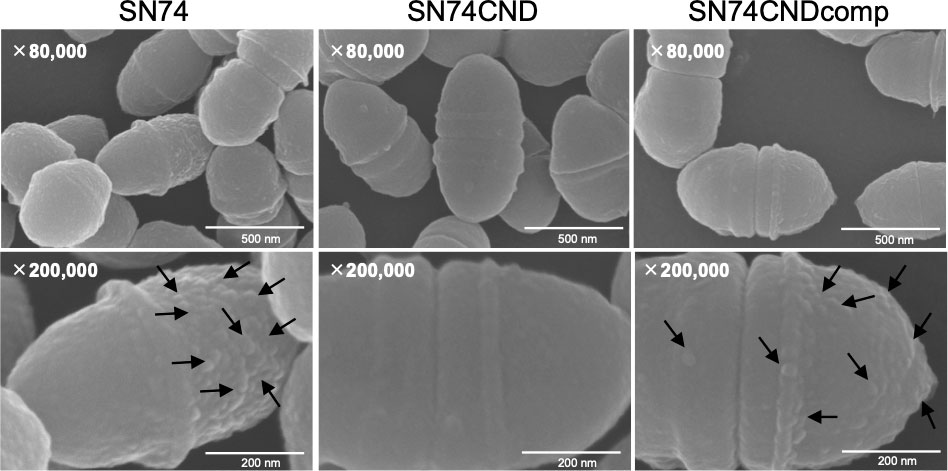
Figure 3 Scanning electron microscopic images of S. mutans strains SN74, SN74CND, and SN74CNDcomp. Scale bar of the upper image, 500 nm; scale bar of the lower image, 200 nm; magnification of the upper image, ×80,000; magnification of the lower image, ×200,000. The arrows indicate the bumpy structures.
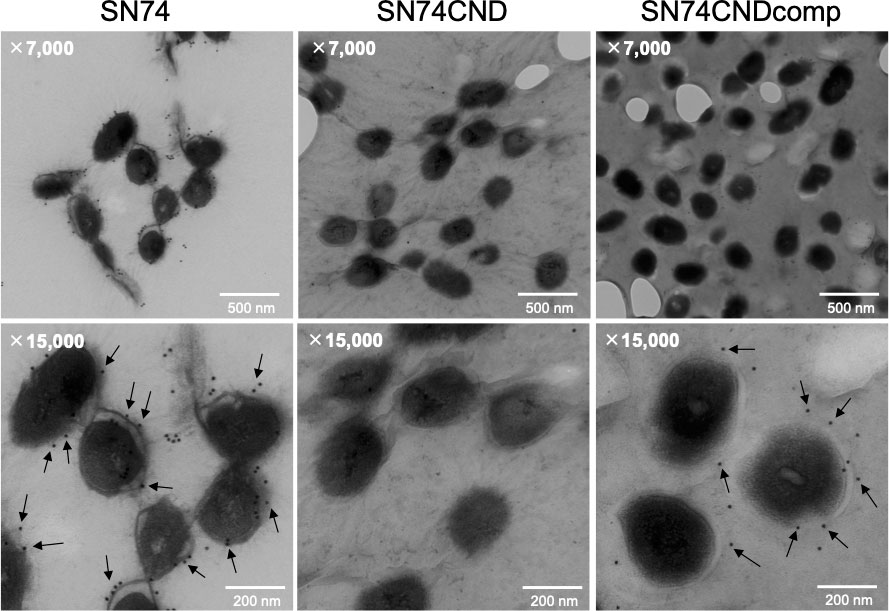
Figure 4 Immunogold transmission electron microscopic images of S. mutans strains SN74, SN74CND, and SN74CNDcomp. Scale bar of the upper image, 500 nm; scale bar of the lower image, 200 nm; magnification of the upper image, ×7,000; magnification of the lower image, ×15,000. The arrows indicate gold colloidal particles.
RNA-seq to identify genes regulated by Cnm
The cut-off for designating a gene as being differentially expressed was a change in mRNA level of at least twofold (up- or downregulated). Gene expression in strain SN74CND compared with that in strain SN74 is shown in Tables 3–5. Expression of transcripts in strain SN74CND was significantly altered compared with the parental strain SN74. Interestingly, the lack of Cnm altered the expression levels of genes related to cell surface proteins, including GTFs and Gbps, which are involved in biofilm formation. In contrast, the absence of Cnm did not influence expression of PA, which is also a cell-anchored protein. Focusing on genes showing the highest levels of differential expression that were related to biofilm formation, a subset of these results was confirmed using qRT-PCR (Figure 5). S. mutans produces three types of GTF, encoded by gtfB, gtfC, and gtfD, respectively, and four types of Gbps encoded by gbpA, gbpB, gbpC, and gbpD, respectively, which are components associated with the adhesion phase of caries development (Matsumoto-Nakano, 2018). The expression of gtfB, gtfC, and gbpC in strain SN74CND was significantly higher than that in strain SN74 (P < 0.001). In contrast, the expression levels of gtfD, gbpA, and gbpB in strain SN74CND were significantly lower than those in strain SN74 (P < 0.05, P < 0.05, and P < 0.001, respectively). The expression level of gbpD did not change. Furthermore, the absence of Cnm affected the expression of genes related to transporters, competence, and stress tolerance. Therefore, Cnm has a strong relationship with mature biofilm formation.
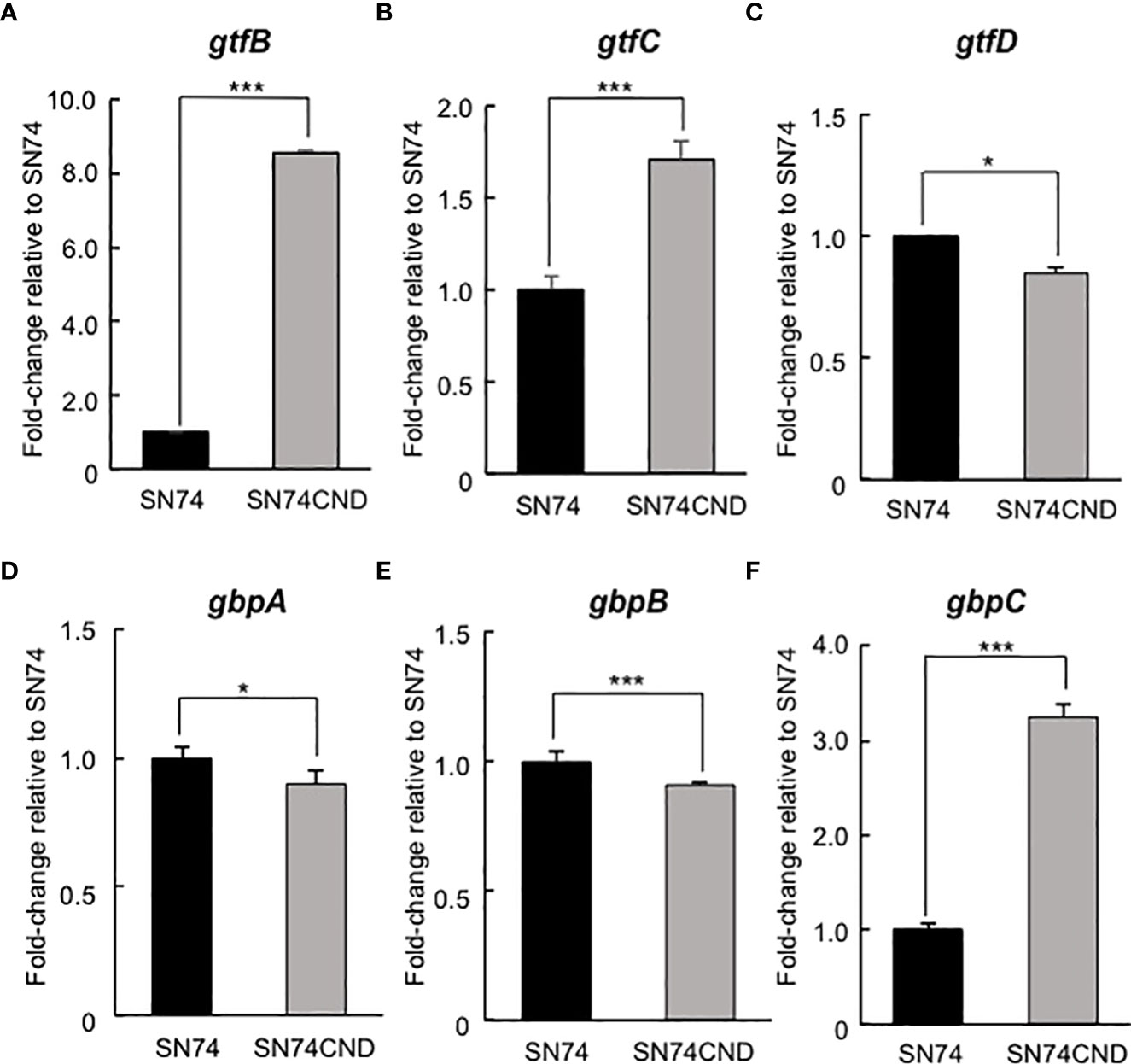
Figure 5 Comparison of gene expression levels in strains SN74 and SN74CND by real-time quantitative reverase transcription PCR. (A) gtfB, (B) gtfC, (C) gtfD, (D) gbpA, (E) gbpB, (F) gbpC. The mRNA expression values were quantified by the ΔΔCT method using 16s rRNA as the internal control. Data are presented as the mean ± SD from five independent experiments. Statistical significance was determined using analysis of variance with the Mann-Whitney U test. *P < 0.05, ***P < 0.001.
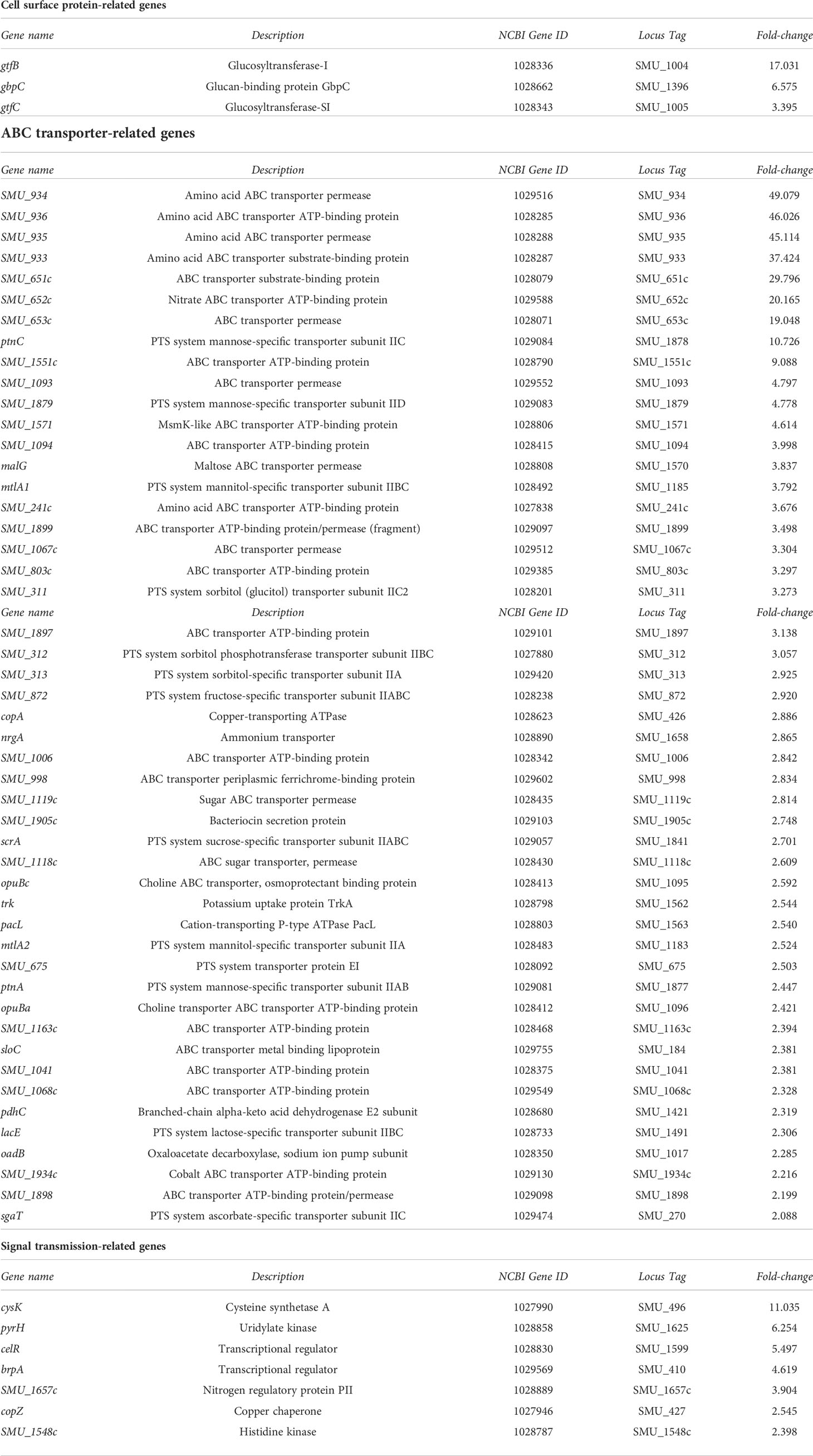
Table 3 List of genes whose expression was upregulated (>2-fold) in strain SN74CND compared with parental strain SN74 by RNA-Sequencing.
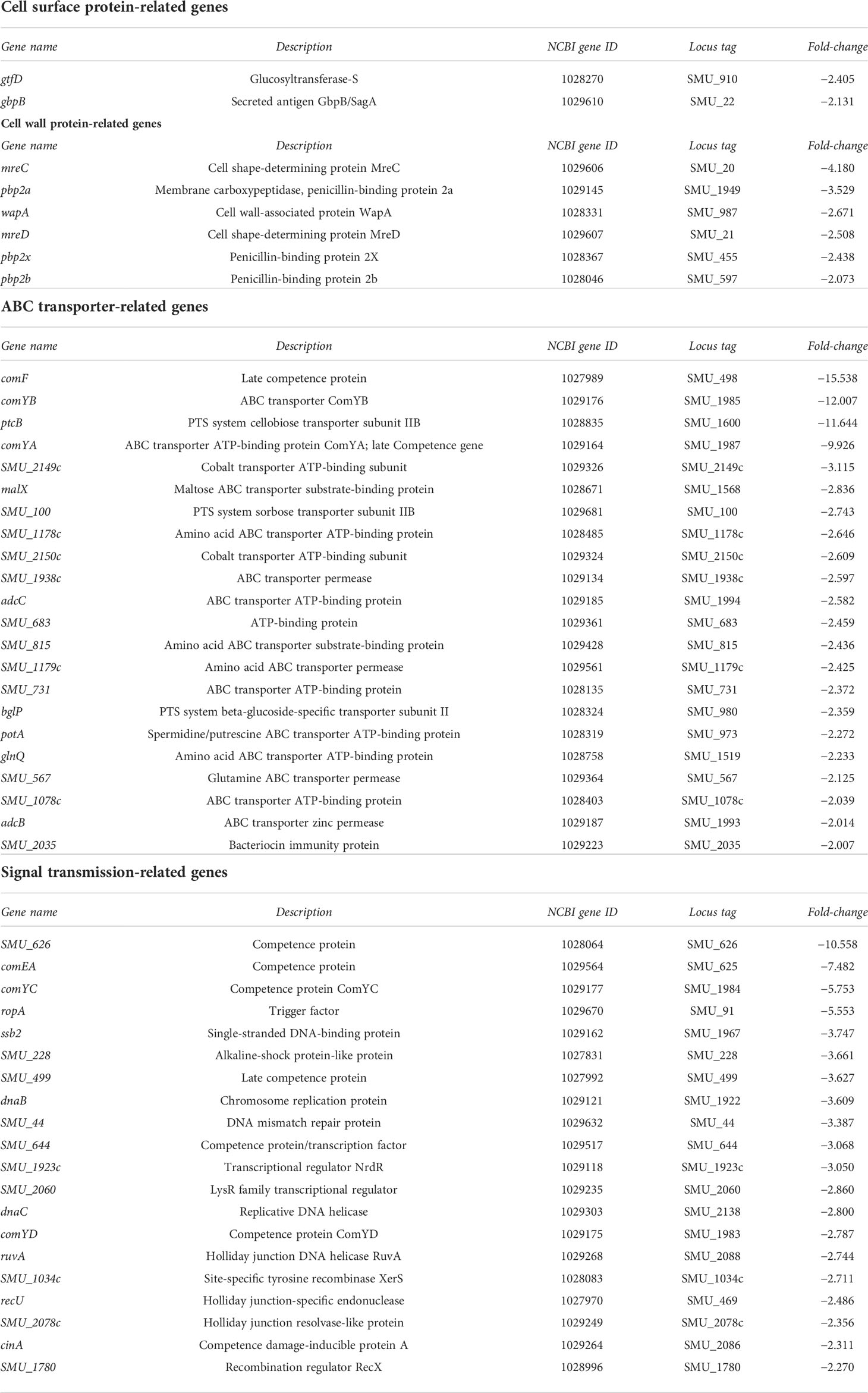
Table 4 List of genes whose expression was downregulated (>2-fold) in strain SN74CND compared with parental strain SN74 by RNA-Sequencing.
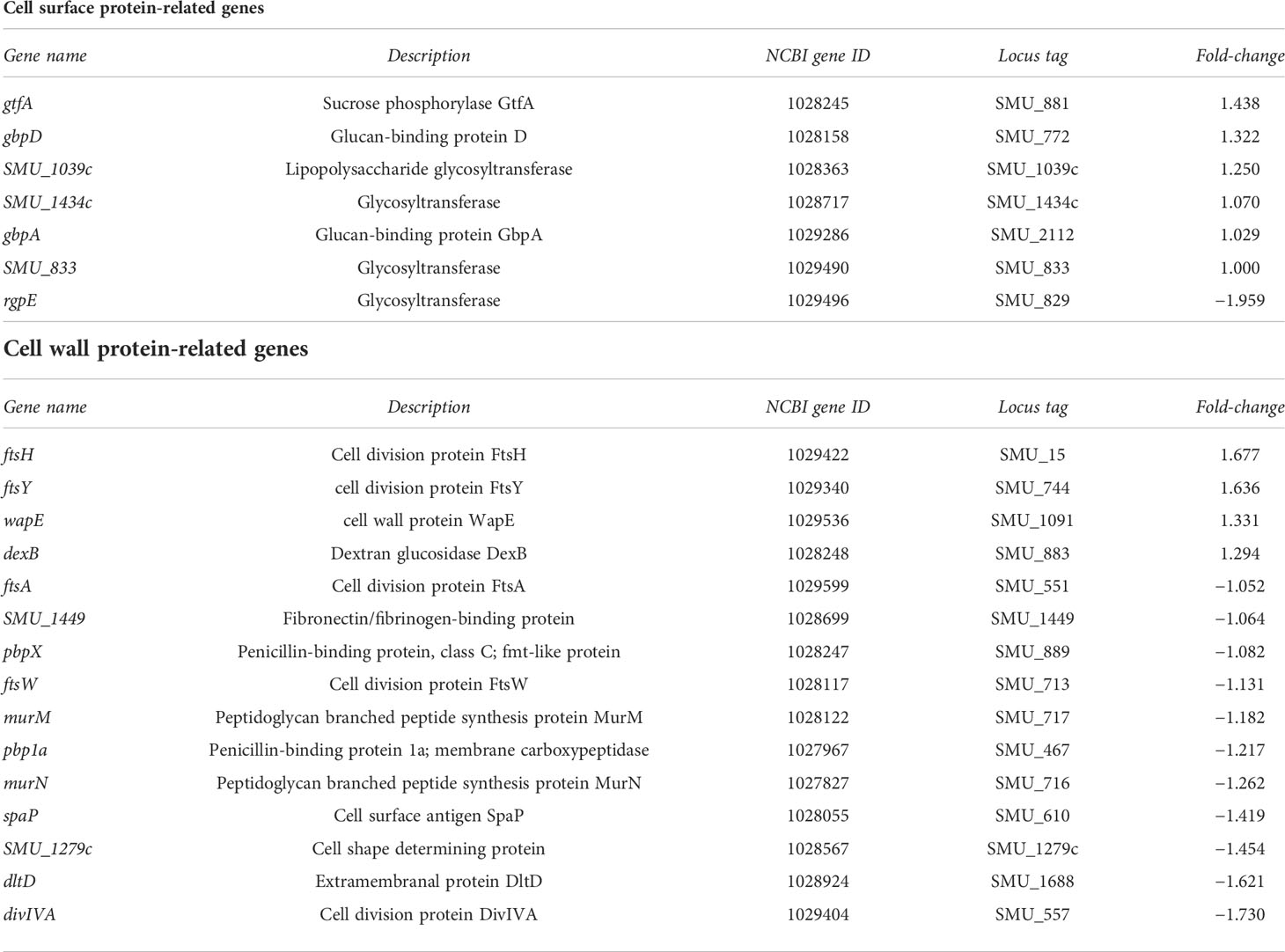
Table 5 List of genes showing no significant change in expression in strain SN74CND compared with parental strain SN74 by RNA-Sequencing.
Analysis of exocytosis
NPN is an uncharged lipophilic probe that can be used to monitor the fluidity of the lipid layer (Nieva-Gomez and Gennis, 1977). Addition of 10 or 20 μg/ml NPN led to an increase in fluorescence intensity of strains SN74, SN74CND, and SN74CNDcomp (Figure 6). However, the intensity of strain SN74CND was significantly less than that of strain SN74 (P < 0.001), suggesting a decrease to less than half of the amount of exported molecules. The wild-type phenotype was fully restored in strain SN74CNDcomp (P < 0.001). Our analysis of exocytosis with NPN showed fewer molecules being released from structures in the plasma membrane in strain SN74CND than in strains SN74 and SN74CNDcomp. These results indicate that the lack of Cnm may affect transmembrane processes involving molecular export and import.
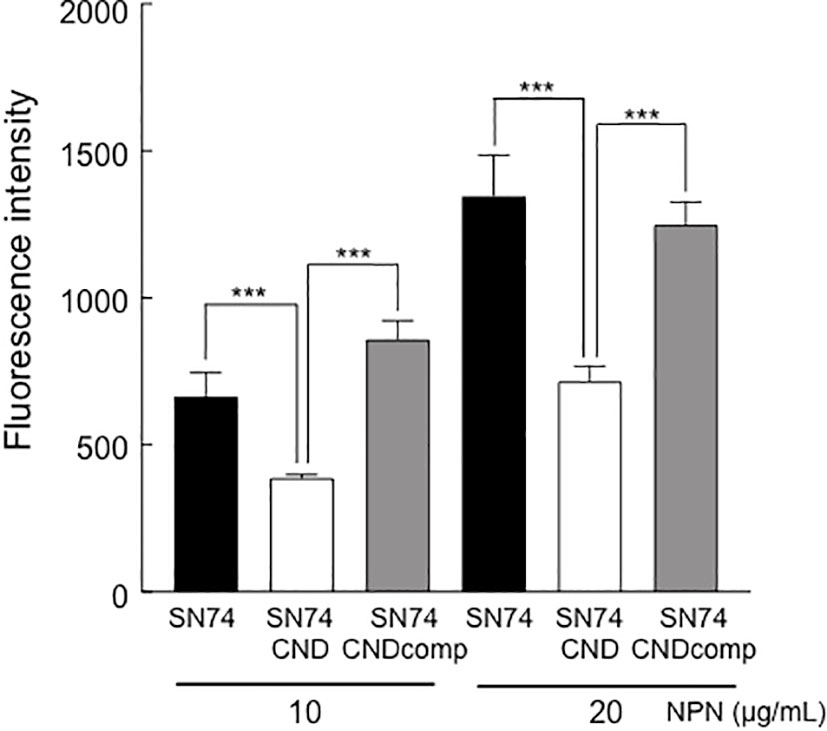
Figure 6 Analysis of exocytosis in strains SN74, SN74CND, and SN74CNDcomp. Fluorescence of the cells was examined in the presence of different concentrations of the fluorescent probe N-phenyl-2-naphtylamine. Data are presented as the mean ± SD from five independent experiments. Statistical significance was determined using analysis of variance with Bonferroni’s correction. ***P < 0.001.
Evaluation of MICs
MICs were evaluated nine antibiotics with strains SN74, SN74CND, and SN74CNDcomp. The MICs of for doripenem and ofloxacin for strain SN74CND were higher, and those of bacitracin and chloramphenicol were lower, than for the other strains (Table 6). Furthermore, strain SN74 was sensitive to clindamycin, but strain SN74CND and SN74CNDcomp were resistant to CLDM. CLSI proposed in 2004 that the erythromycin gene product methylates the ribosome leading to resistance to CLDM. An erythromycin-resistance gene was inserted in the nucleotide sequence of the strain SN74CND produced in this study, suggesting that resistance to CLDM may be acquired through resistance to erythromycin.
Biofilm formation
Cnm-deficient mutant strain SN74CND formed significantly more biofilm than strain SN74 (P < 0.001) (Figure 7). The Cnm-complemented strain SN74CNDcomp formed significantly less biofilm than strain SN74CND (P < 0.001), and a comparable amount to the parental strain SN74 (Figure 7). The biofilms formed by strain SN74CND had greater thickness than those formed by SN74. However, biofilms formed by strain SN74CND showed both small and large amorphous microcolonies (Figure 8A). The morphology of the biofilms of strain SN74CNDcomp was the same as that of the parental strain SN74 (Figure 8A). Biofilm density and thickness were quantified using ImageJ software, and the biofilms of strain SN74CND showed significantly lower values than those for the parental strain SN74 (P < 0.01). The values for strain SN74CNDcomp were significantly higher than those for strain SN74CND (P < 0.05) and comparable to those for the parental strain SN74 (Figure 8B).
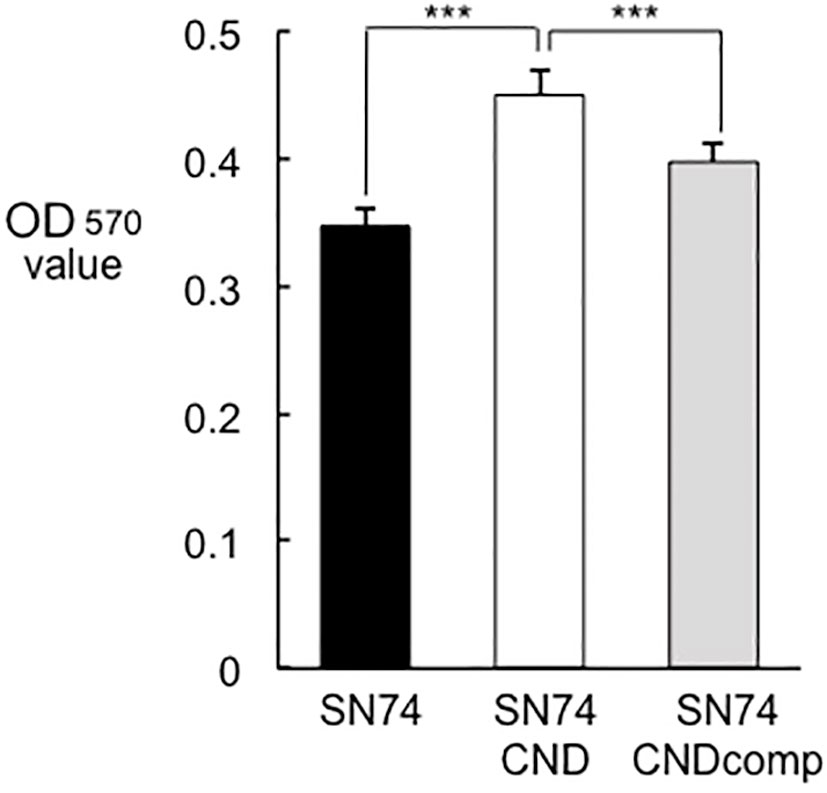
Figure 7 Amount of biofilm formation by strains SN74, SN74CND, and SN74CNDcomp. Biofilm formation by S. mutans strains SN74, SN74CND, and SN74CNDcomp cultured in 1.0% sucrose-added Todd Hewitt broth. The quantity of biofilm formation was determined from the OD570 value following crystal violet staining. Data are presented as the mean ± SD from five independent experiments. Statistical significance was determined using analysis of variance with Bonferroni’s correction. ***P < 0.001.
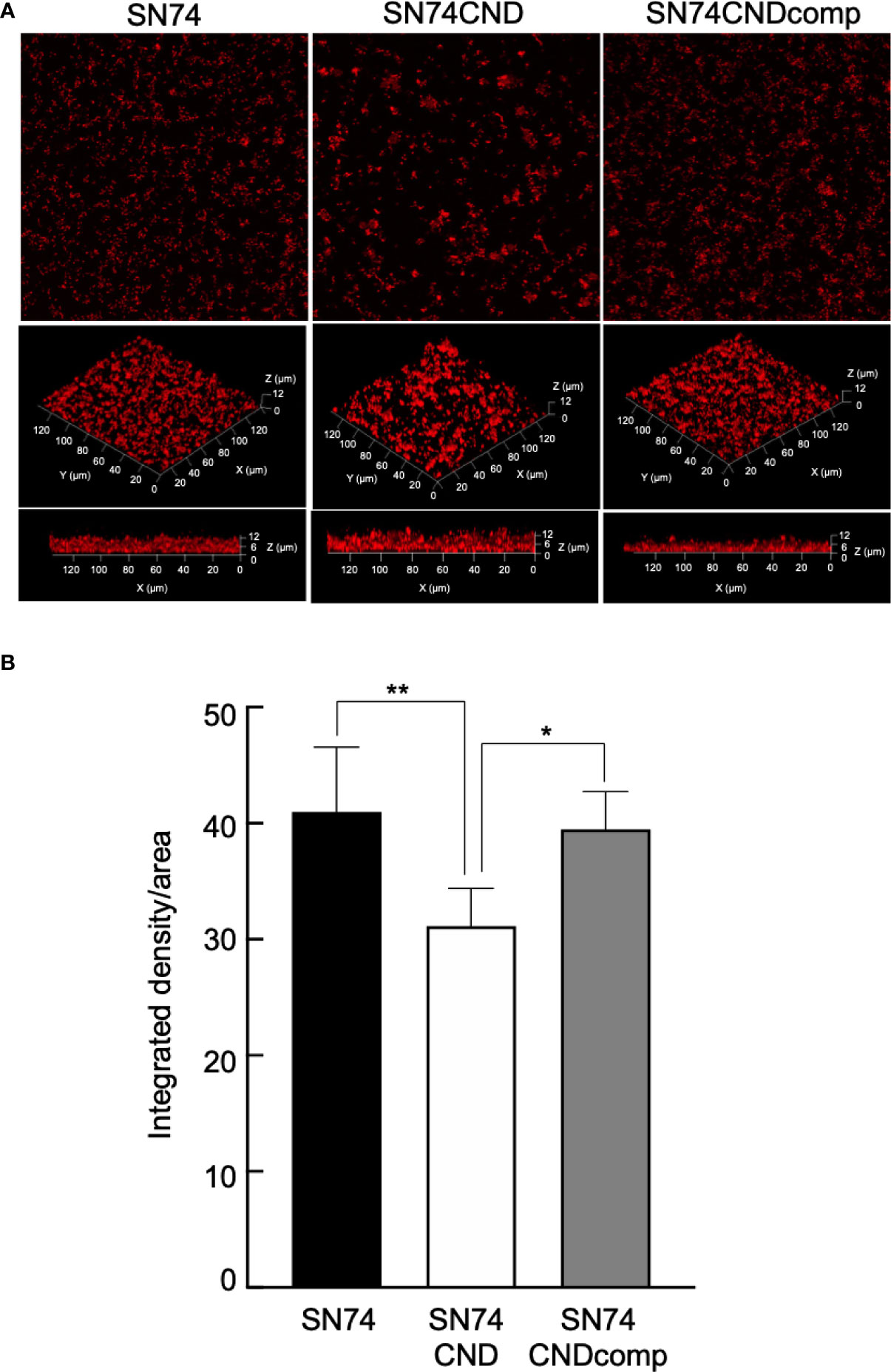
Figure 8 Observation of biofilm formation using confocal laser scanning microscopy (A). Confocal laser scanning microscopic images of S. mutans strains SN74, SN74CND and SN74CNDcomp. (B) Densitometric analysis of biofilms generated by strains SN74, SN74CND, and SN74CNDcomp. Biofilm images for each sample were acquired using three random positions and three independent experiments were performed in triplicate for each strain. Data are presented as the mean ± SD. Statistical significance was determined using analysis of variance with Bonferroni’s correction. *P < 0.05; **P < 0.01.
Discussion
Although S. mutans is a major causative pathogen of dental caries, it is not associated with binding to soft tissues. Recently, however, Cnm has been characterized as a novel LPXTG (leucine, proline, X, threonine, and glycine, where X is any amino acid) motif-anchored protein of S. mutans, which contributes to adhesion to and invasion of soft tissues, such as arterial and venous endothelial cells (Nomura et al., 2013). However, the biological properties of Cnm are yet to be fully elucidated. In this study, we investigated the role of Cnm in pathogenicity of S. mutans.
SEM images and immunogold TEM images indicated that Cnm is a cell surface protein and that the bumpy structures on the surfaces of cells possessing Cnm are possibly Cnm. Surface components for bacterial contact with environmental niches are involved in bacterial adhesion to and invasion of a host, and escape from host defense systems (Bucior et al., 2012; Chagnot et al., 2013). In Gram-negative bacteria, these virulence factors are pilus or fimbrial structures, but they are seldom observed in micrographs of Gram-positive bacteria (Proft and Baker, 2009; Bucior et al., 2012). This surface structure, Cnm, is an important virulence factor of S. mutans.
The ability of bacteria to colonize and persist within their respective environments is strongly influenced by the cell surface composition and resultant functional properties (Crowley and Brady, 2016). Protein translocation and folding pathways are pivotal for the proper insertion of integral membrane proteins, and for the secretion, maturation, and function of fully externalized molecules (Yuan et al., 2010; Forster and Marquis, 2012; Schneewind and Missiakas, 2014). S. mutans lacking the extracellular chaperone/foldase PrsA showed auto-aggregation and altered cell surface properties (Guo et al., 2013). In a mutant strain deficient in RopA, a ribosome-associated chaperone, the expression of GTFB and GTFD was lower compared with that in the parental strain (Wen et al., 2005). Furthermore, inactivation of the gbpA gene altered the expression of gtfB and gtfC (Hazlett et al., 1998). Therefore, the lack of cell surface proteins may cause a change of cell structure. In the present study, the deletion of Cnm resulted in changes in the expression levels of many genes, in particular, many membrane transporter-associated genes (Tables 3–5). Membrane transporters are common and comprise one of the largest protein families (Linton, 2007). S. mutans is subject to a number of environmental fluctuations, such as nutrient availability, aerobic-to-anaerobic transitions, and pH changes (Lemos and Burne, 2008). The transport systems are one of the methods used for surviving these fluctuations, and S. mutans contains >280 genes associated with various transport systems involved in the uptake of ions, molecules, and carbohydrates (Ajdic et al., 2002). The upregulation of membrane transporter genes could promote these functions.
In the present study, the expression of many transporter-related genes was changed by deletion of Cnm. We also examined exocytosis of our strains using a fluorescent polarization probe, NPN, which can used to monitor outer membrane permeabilization (Helander, 2000). The amounts of exocytosis by strain SN74CND decreased significantly when compared with strains SN74 and SN74CNDcomp (Figure 6). In addition, the MICs of chloramphenicol and bacitracin toward strain SN74CND were lower than those toward strains SN74 and SN74CNDcomp. These findings suggest that Cnm blocks uptake and diffusion of some molecules, which may be one strategy used by S. mutans to survive changes in its environment.
In addition, Cnm may be a negative regulator of gtf and gbp genes because the expression levels of gtfB, gtfC, and gbpC increased in strain SN74CND relative to those in the parental strain. In the present study, the quantity of biofilm formation by strain SN74CND increased when compared with the amounts formed by strains SN74 and SN74CNDcomp (Figure 7). However, the structure of the biofilm formed by strain SN74CND showed both small and large amorphous microcolonies and it was not stable and firm compared with the biofilms formed by strains SN74 and SN74CNDcomp (i. e., it was tinner, and less dense) (Figure 8). Upregulation of gtfB, gtfC, and gbpC in strain SN74CND would exacerbate glucan synthesis and cell aggregation by GTFB, GTFC, and GbpC. As a result, many microcolonies formed and the density of the density of the resulting biofilm was decreased. Our results suggest that the lack of Cnm influences biofilm formation by S. mutans. In other words, Cnm is related to biofilm formation (extracellular polymeric substance synthesis and binding) in S. mutans by regulating several genes such as those encoding GTFs and Gbps.
Although Cnm is not associated with the development of dental caries, Cnm has been reported to enter the bloodstream and to be associated with various systemic diseases. Recently, exacerbation mechanisms of systemic diseases such as cerebral hemorrhage, ulcerative colitis and nonalcoholic steatohepatitis caused by S. mutans harboring Cnm have been clarified (Nakano et al., 2011; Kojima et al., 2012; Naka et al., 2014; Naka et al., 2016; Naka et al., 2018). S. mutans possessing Cnm is able to induce infective endocarditis when entering the bloodstream during invasive dental procedures such as tooth extraction (Naka et al., 2016). Cnm protein is involved in adhesion to and invasion of vascular endothelial cells, and is considered to be an important factor in the pathogenesis of their systemic disease (Abranches et al., 2011; Nomura et al., 2013). In the present study, Cnm was found to be located at the cell surface of S. mutans and showed a protrusive structure. These results suggest that Cnm may function as an antigen or like fimbrillin of Porphyromonas gingivalis.
In summary, Cnm located on the cell surface of S. mutans, appears to show a protruding structure, and is associated with several biological properties related to membrane permeability and the regulation of surface and transporter protein genes in the bacterium.
Data availability statement
The original contributions presented in the study are included in the article/Supplementary Material. Further inquiries can be directed to the corresponding author.
Author contributions
SN and DM designed the study under the supervision of MM-N and KN. YN, TM, and SI advised on MIC analysis. Statistical analyses were performed by SN, TM, and SI. Data interpretation was performed by SN, TM, KG, DM, and RN. SN, KN, and MM-N wrote the manuscript and all authors approved the final version. All authors contributed to the article and approved the submitted version.
Acknowledgments
This work was supported by funding from JSPS KAKENHI (grant numbers JP17K09721, JP18H03010, JP19K10098, JP20K10225, and JP21K08242). We thank Masumi Furutani, Urata Haruo and Moemi Tsukano (Central Research Laboratory, Okayama University Graduate School of Medicine, Dentistry and Pharmaceutical Sciences) for assistance with electron microscopic analyses. We thank Edanz Group (https://en-author-services.edanzgroup.com/ac) for editing a draft of this manuscript.
Conflict of interest
The authors declare that the research was conducted in the absence of any commercial or financial relationships that could be construed as a potential conflict of interest.
Publisher’s note
All claims expressed in this article are solely those of the authors and do not necessarily represent those of their affiliated organizations, or those of the publisher, the editors and the reviewers. Any product that may be evaluated in this article, or claim that may be made by its manufacturer, is not guaranteed or endorsed by the publisher.
Supplementary material
The Supplementary Material for this article can be found online at: https://www.frontiersin.org/articles/10.3389/fcimb.2022.994014/full#supplementary-material
Supplementary Figure 1 | Bacterial growth rates of strains SN74, SN74CND, and SN74CNDcomp. ○ SN74, Δ SN74CND, SN74CNDcomp. There were significant differences in the values between strains SN74 and SN74CND (***P < 0.001), and between strains SN74CNDcomp and SN74CND (###P < 0.001). Data are presented as the mean ± SD from five independent experiments. Statistical significance was determined using analysis of variance with Bonferroni’s correction.
Supplementary Figure 2 | Colony morphology of S. mutans strains SN74, SN74CND, and SN74CNDcomp.
Supplementary Figure 3 | Scanning electron microscopic images of S. mutans strains. A. Cnm-positive S. mutans strains TW871, TW295, and OMZ175. Scale bar of the upper image, 500 nM; scale bar of the lower image, 200 nM; magnification of the upper image, ×80,000; magnification of the lower image, ×200,000. B. Cnm-negative S. mutans strains MT8148, UA159, and GS5. Scale bar of the upper image, 500 nM; scale bar of the lower image, 200 nM; magnification of the upper image, ×80,000; magnification of the lower image, ×200,000.
References
Abranches, J., Miller, J. H., Martinez, A. R., Simpson-Haidaris, P. J., Burne, R. A., Lemos, J. A. (2011). The collagen-binding protein cnm is required for Streptococcus mutans adherence to and intracellular invasion of human coronary artery endothelial cells. Infect. Immun. 79 (6), 2277–2284. doi: 10.1128/IAI.00767-10
Ajdic, D., McShan, W. H., McLaughlin, R. E., Savic, G., Chang, J., Carson, M. B., et al. (2002). Genome sequence of Streptococcus mutans UA159, a cariogenic dental pathogen. Proc. Natl. Acad. Sci. U.S.A. 99 (22), 14434–14439. doi: 10.1073/pnas.172501299
Ajdic, D., Pham, Vi., T. T. (2007). Global transcriptional analysis of Streptococcus mutans sugar transporters using microarrays. J. Bacteriol. 189 (14), 5049–5059. doi: 10.1128/JB.00338-07
Ardin, A. C., Fujita, K., Nagayama, K., Takashima, Y., Nomura, R., Nakano, K., et al. (2014). Identification and functional analysis of an ammonium transporter in streptococcus mutans. PloS One 9 (9), e107569. doi: 10.1371/journal.pone.0107569
Banas, J. A., Vickerman, M. M. (2003). Glucan-binding proteins of the oral streptococci. Crit. Rev. Oral. Biol. Med. 14 (2), 89–99. doi: 10.1177/154411130301400203
Bowen, W. H. (2016). Dental caries – not just holes in teeth! a perspective. Mol. Oral. Microbiol. 31 (3), 228–2335. doi: 10.1111/omi.12132
Bowen, W. H., Koo, H. (2011). Biology of streptococcus mutans-derived glucosyltransferases: role in extracellular matrix formation of cariogenic biofilms. Caries. Res. 45 (1), 69–865. doi: 10.1159/000324598
Bucior, I., Pielage, J. F., Engel, J. N. (2012). Pseudomonas aeruginosa pili and flagella mediate distinct binding and signaling events at the apical and basolateral surface of airway epithelium. PloS Pathog. 8 (4), e1002616. doi: 10.1371/journal.ppat.1002616
Chagnot, C., Zorgani, M. A., Astruc, T., Desvaux, M. (2013). Proteinaceous determinants of surface colonization in bacteria: bacterial adhesion and biofilm formation from a protein secretion perspective. Front. Microbiol. 4. doi: 10.3389/fmicb.2013.00303
Crowley, P. J., Brady, L. J. (2016). Evaluation of the effects of Streptococcus mutans chaperones and protein secretion machinery components on cell surface protein biogenesis, competence, and mutacin production. Mol. Oral. Microbiol. 31 (1), 59–77. doi: 10.1111/omi.12130
de Jong, A., Youala, M., Garch, F. E., Simjee, S., Rose, M., Morrissey, I., et al. (2020). Antimicrobial susceptibility monitoring of canine and feline skin and ear pathogens isolated from European veterinary clinics: results of the ComPath surveillance programme. Vet. Dermatol. 31 (6), 431–e114. doi: 10.1111/vde.12886
Dunny, G. M., Lee, L. N., LeBlanc, D. J. (1991). Improved electroporation and cloning vector system for gram-positive bacteria. Appl. Environ. Microbiol. 57 (4), 1194–1201. doi: 10.1128/aem.57.4.1194-1201.1991
Fischer, A., Yang, S. J., Bayer, A. S., Vaezzadeh, A. R., Herzig, S., Stenz, L., et al. (2011). Daptomycin resistance mechanisms in clinically derived Staphylococcus aureus strains assessed by a combined transcriptomics and proteomics approach. J. Antimicrob. Chemother. 66 (8), 1696–1711. doi: 10.1093/jac/dkr195
Forster, B. M., Marquis, H. (2012). Protein transport across the cell wall of monoderm gram-positive bacteria. Mol. Microbiol. 84 (3), 405–413. doi: 10.1111/j.1365-2958.2012.08040.x
Guo, L., Wu, T., Hu, W., He, X., Sharma, S., Webster, P., et al. (2013). Phenotypic characterization of the foldase homologue PrsA in Streptococcus mutans. Mol. Oral. Microbiol. 28 (2), 154–165. doi: 10.1111/omi.12014
Hanada, N., Kuramitsu, H. K. (1989). Isolation and characterization of streptococcus mutans gtfD gene, coding for primer-dependent soluble glucan synthesis. Infect. Immun. 57 (7), 20792085. doi: 10.1128/iai.57.7.2079-2085.1989
Hazlett, K. R., Michalek, S. M., Banas, J. A. (1998). Inactivation of the gbpA gene of Streptococcus mutans increases virulence and promotes in vivo accumulation of recombinations between the glucosyltransferase b and c genes. Infect. Immun. 66 (5), 2180–2185. doi: 10.1128/IAI.66.5.2180-2185.199811
Helander, I. M., Mattila-Sandholm, T.. (2000). Fluorometric assessment of Gram-negative bacterial permeabilization. J App Microbiol. 88(2):213–219. doi: 10.1046/j.1365-2672.2000.00971.x
Inagaki, S., Fujita, K., Takashima, Y., Nagayama, K., Ardin, A. C., Matsumi, Y., et al. (2013). Regulation of recombination between gtfB/gtfC genes in Streptococcus mutans by recombinase A. Sci. World J. 2013, 405075. doi: 10.1155/2013/405075
Kojima, A., Nakano, K., Wada, K., Takahashi, H., Katayama, K., Yoneda, M., et al. (2012). Infection of specific strains of Streptococcus mutans, oral bacteria, confers a risk of ulcerative colitis. Sci. Rep. 2, 332. doi: 10.1038/srep00332
Kuboniwa, M., Tribble, G. D., James, C. E., Kilic, A. O., Tao, L., Herzberg, M. C., et al. (2006). Streptococcus gordonii utilizes several distinct gene functions to recruit Porphyromonas gingivalis into a mixed community. Mol. Microbiol. 60 (1), 121–139. doi: 10.1111/j.1365-2958.2006.05099.x
Lemos, J. A., Burne, R. A. (2008). A model of efficiency: stress tolerance by Streptococcus mutans. Microbiol. (Reading). 154 (Pt 11), 3247–3255. doi: 10.1099/mic.0.2008/023770-0
Linton, K. J. (2007). Structure and function of ABC transporters. Physiol. (Bethesda). 22, 122–130. doi: 10.1152/physiol.00046.2006
Macrina, F. L., Evans, R. P., Tobian, J. A., Hartley, D. L., Clewell, D. B., Jones, K. R. (1983). Novel shuttle plasmid vehicles for Escherichia-streptococcus transgeneric cloning. Gene. 25 (1), 145–150. doi: 10.1016/0378-1119(83)90176-2
Matsumoto-Nakano, M. (2018). Role of Streptococcus mutans surface proteins for biofilm formation. Jpn. Dent. Sci. Rev. 54 (1), 22–29. doi: 10.1016/j.jdsr.2017.08.002
Matsumoto-Nakano, M., Fujita, K., Ooshima, T. (2007). Comparison of glucan-binding proteins in cariogenicity of Streptococcus mutans. Oral. Microbiol. Immunol. 22 (1), 30–35. doi: 10.1111/j.1399-302x.2007.00318.x
Matsumoto-Nakano, M., Kuramitsu, H. K. (2006). Role of bacteriocin immunity proteins in the antimicrobial sensitivity of Streptococcus mutans. J. Bacteriol. 188 (23), 8095–8102. doi: 10.1128/JB.00908-06
Naka, S., Hatakeyama, R., Takashima, Y., Matsumoto-Nakano, M., Nomura, R., Nakano, K. (2016). Contributions of Streptococcus mutans cnm and PA antigens to aggravation of non-alcoholic steatohepatitis in mice. Sci. Rep. 6, 36886. doi: 10.1038/srep36886
Nakano, K., Hokamura, K., Taniguchi, N., Wada, K., Kudo, C., Nomura, R., et al. (2011). The collagen-binding protein of Streptococcus mutans is involved in haemorrhagic stroke. Nat. Commun. 2, 485. doi: 10.1038/ncomms1491
Naka, S., Nomura, R., Takashima, Y., Okawa, R., Ooshima, T., Nakano, K. (2014). A specific Streptococcus mutans strain aggravates non-alcoholic fatty liver disease. Oral. Dis. 20 (7), 700–706. doi: 10.1111/odi.12191
Nakano, K., Nomura, R., Shimizu, N., Nakagawa, I., Hamada, S., Ooshima, T. (2004). Development of a PCR method for rapid identification of new Streptococcus mutans serotype k strains. J. Clin. Microbiol. 42 (11), 4925–4930. doi: 10.1128/JCM.42.11.4925-4930.2004
Naka, S., Wato, K., Hatakeyama, R., Okawa, R., Nomura, R., Nakano, K. (2018). Longitudinal comparison of Streptococcus mutans-induced aggravation of non-alcoholic steatohepatitis in mice. J. Oral. Microbiol. 10 (1), 1428005. doi: 10.1080/20002297.2018.1428005
Naka, S., Wato, K., Misaki, T., Ito, S., Nagasawa, Y., Nomura, R., et al. (2020). Intravenous administration of Streptococcus mutans induces IgA nephropathy-like lesions. Clin. Exp. Nephrol. 24 (12), 1122–1131. doi: 10.1007/s10157-020-01961-1
Nieva-Gomez, D., Gennis, R. B. (1977). Affinity of intact Escherichia coli for hydrophobic membrane probes is a function of the physiological state of the cells. Proc. Natl. Acad. Sci. 74 (5), 1811–1815. doi: 10.1073/pnas.74.5.1811
Nikaido, H. (2009). Multidrug resistance in bacteria. Annu. Rev. Biochem. 78, 119–146. doi: 10.1146/annurev.biochem.78.082907.145923
Nomura, R., Naka, S., Nemoto, H., Inagaki, S., Taniguchi, K., Ooshima, T., et al. (2013). Potential involvement of collagen-binding proteins of Streptococcus mutans in infective endocarditis. Oral. Dis. 19 (4), 387–393. doi: 10.1111/odi.12016
Nomura, R., Nakano, K., Naka, S., Nemoto, H., Masuda, K., Lapirattanakul, J., et al. (2012). Identification and characterization of a collagen-binding protein, cbm, in Streptococcus mutans. Mol. Oral. Microbiol. 27 (4), 308–323. doi: 10.1111/j.2041-1014.2012.00649.x
Nomura, R., Nakano, K., Ooshima, T. (2005). Molecular analysis of the genes involved in the biosynthesis of serotype specific polysaccharide in the novel serotype k strains of Streptococcus mutans. Oral. Microbiol. Immunol. 20 (5), 303–309. doi: 10.1111/j.1399-302X.2005.00231.x
Ocaktan, A., Yoneyama, H., Nakae, T. (1997). Use of fluorescence probes to monitor function of the subunit proteins of the MexA-MexB-oprM drug extrusion machinery in Pseudomonas aeruginosa. J. Biol. Chem. 272 (35), 21964–21969. doi: 10.1074/jbc.272.35.21964
Proft, T., Baker, E. N. (2009). Pili in gram-negative and gram-positive bacteria-structure, assembly and their role in disease. Cell Mol. Life Sci. 66 (4), 613–635. doi: 10.1007/s00018-008-8477-4
Sato, Y., Okamato, K., Kagami, A., Yamamoto, Y., Igarashi, T., Kizaki, H. (2004). Streptococcus mutans strains harboring collagen-binding adhesin. J. Dent. Res. 83 (7), 534–539. doi: 10.1177/154405910408300705
Schneewind, O., Missiakas, D. (20141843). Sec-secretion and sortase-mediated anchoring of proteins in gram-positive bacteria. Biochim. Biophys. Acta 8), 1687–1697. doi: 10.1016/j.bbamcr.2013.11.009
Takashima, Y., Fujita, K., Ardin, A. C., Nagayama, K., Nomura, R., Nakano, K., et al. (2015). Characterization of the dextran-binding domain in the glucan-binding protein c of streptococcus mutans. J. Appl. Microbiol. 119 (4), 1148–1157. doi: 10.1111/jam.12895
Tamesada, M., Kawabata, S., Fujiwara, T., Hamada, S. (2004). Synergistic effects of streptococcal glucosyltransferases on adhesive biofilm formation. J. Dent. Res. 83 (11), 874–879. doi: 10.1177/154405910408301110
Tobian, J. A., Macrina, F. L. (1982). Helper plasmid cloning in Streptococcus sanguis: cloning of a tetracycline resistance determinant from the Streptococcus mutans chromosome. J. Bacteriol. 152 (1), 215–222. doi: 10.1128/jb.152.1.215-222.1982
Trauble, H., Overath, P. (1973). The structure of Escherichia coli membranes studied by fluorescence measurements of lipid phase transitions. Biochim. Biophys. Acta 307 (3), 491–512. doi: 10.1016/0005-2736(73)90296-4
van de Rijn, I., Kessler, R. E. (1980). Growth characteristics of group a streptococci in a new chemically defined medium. Infect. Immun. 27 (2), 444–448. doi: 10.1128/iai.27.2.444-448.1980
Waterhouse, J. C., Russell, R. R. B. (2006). Dispensable genes and foreign DNA in Streptococcus mutans. Microbiol. (Reading). 152 (Pt 6), p. doi: 10.1099/mic.0.28647-0
Wen, Z. T., Suntharaligham, P., Cvitkovitch, D. G., Burne, R. A. (2005). Trigger factor in Streptococcus mutans is involved in stress tolerance, competence development, and biofilm formation. Infect. Immun. 73 (1), 219–225. doi: 10.1128/IAI.73.1.219-225.2005
Xiao, J., Koo, H. (2010). Structural organization and dynamics of exopolysaccharide matrix and microcolonies formation by streptococcus mutans in biofilms. J. Appl. Microbiol. 108 (6), 21032113. doi: 10.1111/j.1365-2672.2009.04616.x
Yuan, K., Hou, L., Jin, Q., Niu, C., Mao, M., Wang, R., et al. (2021). Comparative transcriptomics analysis of Streptococcus mutans with disruption of LuxS/AI-2 quorum sensing and recovery of methy cycle. Arch. Oral. Biol. 127, 105137. doi: 10.1016/j.archoralbio.2021.105137
Keywords: Streptococcus mutans, collagen-binding protein, membrane permeability, cell structure, RNA-seq
Citation: Naka S, Matsuoka D, Goto K, Misaki T, Nagasawa Y, Ito S, Nomura R, Nakano K and Matsumoto-Nakano M (2022) Cnm of Streptococcus mutans is important for cell surface structure and membrane permeability. Front. Cell. Infect. Microbiol. 12:994014. doi: 10.3389/fcimb.2022.994014
Received: 14 July 2022; Accepted: 18 August 2022;
Published: 13 September 2022.
Edited by:
Harapriya Mohapatra, National Institute of Science Education and Research (NISER), IndiaReviewed by:
Karthik Subramanian, Rajiv Gandhi Centre for Biotechnology, IndiaMuhsin Jamal, Abdul Wali Khan University, Pakistan
Copyright © 2022 Naka, Matsuoka, Goto, Misaki, Nagasawa, Ito, Nomura, Nakano and Matsumoto-Nakano. This is an open-access article distributed under the terms of the Creative Commons Attribution License (CC BY). The use, distribution or reproduction in other forums is permitted, provided the original author(s) and the copyright owner(s) are credited and that the original publication in this journal is cited, in accordance with accepted academic practice. No use, distribution or reproduction is permitted which does not comply with these terms.
*Correspondence: Michiyo Matsumoto-Nakano, bW5ha2Fub0Bva2F5YW1hLXUuYWMuanA=
†These authors have contributed equally to this work