- Laboratory of General Microbiology, Department of Biology of Bacteria, Institute of Microbiology, Biotechnology and Immunology, University of Łódź, Łódź, Poland
The genera Klebsiella and Proteus were independently described in 1885. These Gram-negative rods colonize the human intestinal tract regarded as the main reservoir of these opportunistic pathogens. In favorable conditions they cause infections, often hospital-acquired ones. The activity of K. pneumoniae and P. mirabilis, the leading pathogens within each genus, results in infections of the urinary (UTIs) and respiratory tracts, wounds, bacteremia, affecting mainly immunocompromised patients. P. mirabilis and K. pneumoniae cause polymicrobial UTIs, which are often persistent due to the catheter biofilm formation or increasing resistance of the bacteria to antibiotics. In this situation a need arises to find the antigens with features common to both species. Among many virulence factors produced by both pathogens urease shows some structural similarities but the biggest similarities have been observed in lipids A and the core regions of lipopolysaccharides (LPSs). Both species produce capsular polysaccharides (CPSs) but only in K. pneumoniae these antigens play a crucial role in the serological classification scheme, which in Proteus spp. is based on the structural and serological diversity of LPS O-polysaccharides (OPSs). Structural and serological similarities observed for Klebsiella spp. and Proteus spp. polysaccharides are important in the search for the cross-reacting vaccine antigens.
1 Introduction
Both Klebsiella spp. and Proteus spp. are Gram-negative rods belonging to the Enterobacterales ord. nov (Brisse et al., 2006; Adeolu et al., 2016). In contrast to Klebsiella spp., Proteus spp. rods are motile (peritrichously flagellated) and capable of swarming. Swarming growth is triggered by a contact of short rod shaped swimmer cells (normally appearing in liquid medium) with 1.5% agar medium when the swimmer cells differentiate into elongated, hyperflagellated, multinucleated swarm cells. Swarmer cells move together across a plate till the population is reduced in its density and the consolidation process starts. In consolidation, which is regarded as a resting stage, swarmer cells re-differentiate into swimmer cells. The whole process is repeated till the characteristic bull’s-eye pattern appears on agar media (Armbruster and Mobley, 2012; Różalski et al., 2012; Schaffer and Pearson, 2015). By contrast, a feature, which distinguishes encapsulated Klebsiella spp. from Proteus spp. is a mucoid character of its colonies on agar media (Brisse et al., 2006). Both genera were described and named in the same year, 1885, Klebsiella – by Trevisan to honor the German microbiologist Edwin Klebs, and Proteus – by Hauser with reference to the Greek deity and the swarming nature of the bacteria (O’Hara et al., 2000; Brisse et al., 2006).
The taxonomy of each genus has been modified, although the taxonomic history of Klebsiella spp. aroused some controversial opinions concerning: the exclusion of K. planticola from the genus Klebsiella and transferring this species, together with K. terrigena and K. ornithinolytica, to the new genus Raoultella on the basis of the rpoB phylogenies (Podschun and Ullmann, 1998; Drancourt et al., 2001; Brisse et al., 2006). Currently, in the genus Klebsiella two major species are well known as causing infections in humans: Klebsiella pneumoniae with three subspecies – pneumoniae, ozaenae and rhinoscleromatis as well as K. oxytoca. Both species have been found to be genetically heterogeneous and comprise phylogenetic groups. K. pneumonia is subdivided into seven phylogroups (Kp1-Kp7) and K. oxytoca – into six phylogroups (Ko1-Ko4, Ko6, Ko8) corresponding to the following taxa: Kp1 – K. pneumoniae, Kp2 – K. quasipneumoniae subsp. quasipneumoniae, Kp3 – K. variicola subsp. variicola, Kp4 – K. quasipneumoniae subsp. similipneumoniae, Kp5 – K. variicola subsp. tropicalensis, Kp6 – K. quasivariicola, Kp7 – K. africanensis; Ko1 – K. michiganensis, Ko2 – K. oxytoca, Ko3 – K. spallanzanii, Ko4 – K. pasteurii, Ko6 – K. grimontii and Ko8 – K. hauxiensis (Gómez et al., 2021).
Phylogenetic analysis resulted in placing Proteus within a new Morganellaceae family (Adeolu et al., 2016). The genus Proteus includes three unnamed Proteus genomospecies 4, 5 and 6, ten named species: P. mirabilis, P. vulgaris, P. penneri, P. hauseri and six newly formed species, P. cibarius, P. terrae, P. cibi, P. columbae, P. alimentorum and P. faecis (Dai et al., 2019).
2 P. mirabilis and K. pneumoniae as etiologic agents of infections in humans
The representatives of both genera colonize the lower human intestinal tract, but Klebsiella spp. were more prevalent (Drzewiecka, 2016). Klebsiella spp. also colonize the nasopharynx (Brisse et al., 2006; Paczosa and Mecsas, 2016). The human digestive tract is regarded as a reservoir of both opportunistic pathogens leading to autoinfection or person-to-person transmitted nosocomial infections (Drzewiecka, 2016; Paczosa and Mecsas, 2016; Gómez et al., 2021). Klebsiella spp. accounted for 3-9.9% of all nosocomial bacterial infections and Proteus spp. (P. mirabilis) – for 3-5% of such infections depending on the survey (Brisse et al., 2006; Magill et al., 2014; Martin and Bachman, 2018). Among Proteus species, P. mirabilis is the most important etiological factor of infections (80-90% of all Proteus infections), which has also been confirmed by the studies performed on 617 Proteus spp. strains collected in Łódź (2006–2011) from different clinical sources (86.9% of isolation frequency) (Drzewiecka et al., 2021). Among Klebsiella species, K. pneumoniae is responsible for 75-86% of Klebsiella species infections (Watanakunakorn, 1991; Hansen et al., 2004). K. pneumoniae and P. mirabilis nosocomial infections include infections of the urinary tract (UTIs), wounds and bacteremia, which affect mainly immunocompromised patients especially in neonatal intensive-care units (K. pneumoniae) (Endimiani et al., 2005; Brisse et al., 2006; Różalski et al., 2012; Martin and Bachman, 2018; Gómez et al., 2021). On the contrary, a hypervirulent variant of K. pneumoniae (hvKP) is capable of causing serious infections e.g. endophthalmitis and meningitis, which can be metastatically spread to distant sites in non-immunocompromised ambulatory younger healthy hosts (Shon et al., 2013).
Both K. pneumoniae and P. mirabilis strains are etiologic agents of bloodstream infections (BSIs), where K. pneumoniae isolates which are regarded as one of predominant species among Enterobacterales bacilli (4.4-36% depending on the surveillance) cause BSI (Endimiani et al., 2005; Musicha et al., 2017; Leal et al., 2019; Kallel et al., 2020). K. pneumoniae BSIs often associated with the MDR phenotype. In contrast to the well-proven contribution of K. pneumoniae to BSIs, P. mirabilis are isolated less often (0.3-2.9%) from blood samples (Musicha et al., 2017; Leal et al., 2019; Kallel et al., 2020). An occurrence frequency of BSIs may also depend on a world region e.g. no P. mirabilis strains out of 536 collected in Łódź (Poland) came from blood (Drzewiecka et al., 2021). P. mirabilis BSIs mostly originate from complicated UTIs in patients with community-acquired infections (Chen et al., 2012). Two cases of P. mirabilis bacteraemia originating from infected aneurysm have also been noted (Nagiah and Badbess, 2018). Klebsiella bacteraemia (both primary and secondary) arise from a primary infection in the bladder or lungs (Paczosa and Mecsas, 2016).
Although K. pneumoniae is a primary cause of hospital-acquired pneumonia -11.8% of HAPs- (often severe with characteristic brick-red sputum and often leading to local necrosis in lungs), hospital-acquired pneumonia caused by P. mirabilis has also been reported (usually mild or moderate with a good efficacy rate, often affecting elderly patients with underlying diseases) (Wu and Li, 2015; Sękowska and Gospodarek, 2007; Okimoto et al., 2010; Magill et al., 2014; Paczosa and Mecsas, 2016). Both species have been relatively rarely found among pathogens causing community-acquired pneumonia (CAP) in Europe (Ko et al., 2002; Okimoto et al., 2010; Lin et al., 2010). However, K. pneumonia has been found to be the underlying agent of CAPs in Asia and Africa, which is related to the increased prevalence of hvKP in these regions (Ko et al., 2002; Paczosa and Mecsas, 2016).
P. mirabilis and K. pneumoniae species have also been reported to play a role in diarrheal disease (Lu. et al., 2017; Zhang et al., 2018; Gong et al., 2019). In China the detection rate of K. pneumoniae in faeces of persons with diarrhea was found to range from 0.5% among outpatients to 7.8% among hospital patients. In the majority of cases hypermucoviscosity phenotypes of K. pneumoniae were detected (Lu. et al., 2017; Zhang et al., 2018). Recently a novel diarrheagenic P. mirabilis strain (C02011) has been isolated in feces specimens in a food poisoning case in China. That strain was found to be a stronger gastrointenstinal pathogen than P. mirabilis B02005 isolated from healthy people. Moreover, the type IV secretion system (T4SS), also found in K. pneumoniae, was suggested to be crucial in the pathogenesis of diarrheal P. mirabilis (Gong et al., 2019). It is worth noting that both pathogens cause diarrhea in humans less often than other infections e.g. UTIs (O’Hara et al., 2000; Brisse et al., 2006).
K. pneumoniae and P. mirabilis cause community- and hospital-acquired urinary tract infections (UTIs) (Sękowska and Gospodarek, 2007; Cuevas et al., 2010; Chen et al., 2012). K. pneumonia is a causative agent of 2 to 6% of nosocomial UTIs and 4.3 to 7% of community-acquired ones (Laupland et al., 2007; Linhares et al., 2013; Paczosa and Mecsas, 2016). Depending on the studies and the world region, P. mirabilis placed third (behind E. coli and Klebsiella spp.) or fourth as a causative agent of UTIs (Coban et al., 2014; Tajbakhsh et al., 2015; Drzewiecka and Lewandowska, 2016). The isolation frequency from UTIs cases within one study was at a similar level for both pathogens and the numbers were much lower than those for E. coli (E. coli 81.8%, K. pneumoniae 7.9%, P. mirabilis 5.2% - Spain, 2008-2009) (Cuevas et al., 2010; Drzewiecka and Lewandowska, 2016). Both K. pneumoniae and P. mirabilis cause complicated UTIs in individuals with structurally abnormal urinary tracts, those undergoing long-term catheterization or patients with type 2 diabetes mellitus, but the latter species dominates as a causative agent of complicated UTIs (Dhingra, 2008; Drzewiecka and Lewandowska, 2016).
P. mirabilis and K. pneumoniae are a common cause of catheter-associated UTIs (CAUTIs) (Schroll et al., 2010; Armbruster et al., 2018). P. mirabilis CAUTIs often affect long-term catheterized patients (28 days or longer) where they lead to pyelonephritis, urolithiasis, prostatitis, stone and biofilm formation (Fox-Moon and Shirtliff, 2015; Armbruster and Mobley, 2012; Armbruster et al., 2018). In catheter biofilms P. mirabilis was found together with K. pneumoniae, representatives of both species were isolated more often from mixed-species biofilms (34.2% and 23.7%, respectively) than from single-species ones (20.0% and 3.3%, respectively), however this correlation can be better noticed for K. pneumoniae isolates (Macleod and Stickler, 2007). After the infection of 72 h K. pneumoniae biofilm by P. mirabilis, K. pneumoniae showed temporary antagonism against P. mirabilis and extended twice its mean time to catheter blockage compared to the control (Macleod and Stickler, 2007). Conversely, co-inoculation of K. p.-P. m. did not impact the P. mirabilis ability to attach to the siliconized surface but impaired the ability of K. pneumonia to bind to that surface (Galván et al., 2016). The persistence of UTIs caused by both pathogens may be connected with their increasing resistance to antibiotics, especially to nitrofurantoin commonly used in UTIs treatment. Moreover, the persistence of P. mirabilis-associated UTIs may result from urolithiasis that may lead to the catheters and urinary tract obstruction (Jacobsen et al., 2008; Cuevas et al., 2010; Drzewiecka and Lewandowska, 2016). 65% of specimens from patients with long-term catheterization contained P. mirabilis, which was associated with the catheter obstruction (Mobley and Warren, 1987). The dual-species associations of K. p. with P. m. were found to account for 7% of the 97% polymicrobial cases of CAUTIs in a two-year survey (Galván et al., 2016).
Apart from polymicrobial UTIs, other infections may also be caused by the representatives of both species e.g. P. mirabilis and K. pneumoniae were isolated from the abdominal wound of a man with the carcinoma of the colon (Krajden et al., 1987). What is interesting, both species have been implicated in chronic inflammatory arthritis: K. pneumoniae in ankylosing spondylitis (AS) and P. mirabilis in rheumatoid arthritis (RA). In both cases the role of K. pneumoniae and P. mirabilis in the development of AS or AR, respectively, was associated with the molecular similarity between amino acid sequences of bacterial antigens and appropriate human self-antigens (Table 1) (Brisse et al., 2006; Rashid and Ebringer, 2007a; Rashid and Ebringer, 2007b; Różalski et al., 2012). The etiopathogenic role of K. pneumoniae in the AS development is connected with a molecular homology between: 1) ‘QTDRED’ amino acid sequences present in both K. pneumoniae nitrogenase reductase enzyme and HLA-B27, 2) between ‘DRDE’ amino acid sequences found in PulD secretion proteins of K. pneumoniae pullulanase enzyme and the ‘DRED’ sequence in HLA-B27, 3) between repeating sequences occurring in PulA of Klebsiella spp. pullulanase and types I, III and IV collagens (Schwimmbeck et al., 1987; Fielder et al., 1995). On the other hand, the potential role of P. mirabilis in the etiopathogenesis of RA is associated with molecular similarity between: 1) the ‘ESRRAL’ amino acid sequences found in P. mirabilis hemolysins and the ‘EQ/KRRAA, motif observed in RA-associated HLA-DR molecules, 2) between the ‘LRREI’ sequences in Proteus urease and the ‘IRRET’ motif present in type XI collagen. In both cases high titers of antibodies against K. pneumoniae or P. mirabilis were detected in the sera of the patients with active AS or RA, respectively (Ebringer et al., 1992; Wilson et al., 1995; Ebringer et al., 2003; Ebringer et al., 2006). As a consequence of the mimicry phenomenon, bacterial antigen-specific antibodies, acting as autoantibodies, recognize also human self-antigens, which results in the damage to hyaline cartilage (Wilson et al., 1995; Konieczna et al., 2012). Different body sites are regarded as the isolation sources of both pathogens in RA or AS infections – the upper urinary tract of RA patients (P. mirabilis) and the colon of AS patients (K. pneumoniae) (Rashid and Ebringer, 2007a; Rashid and Ebringer, 2007b).
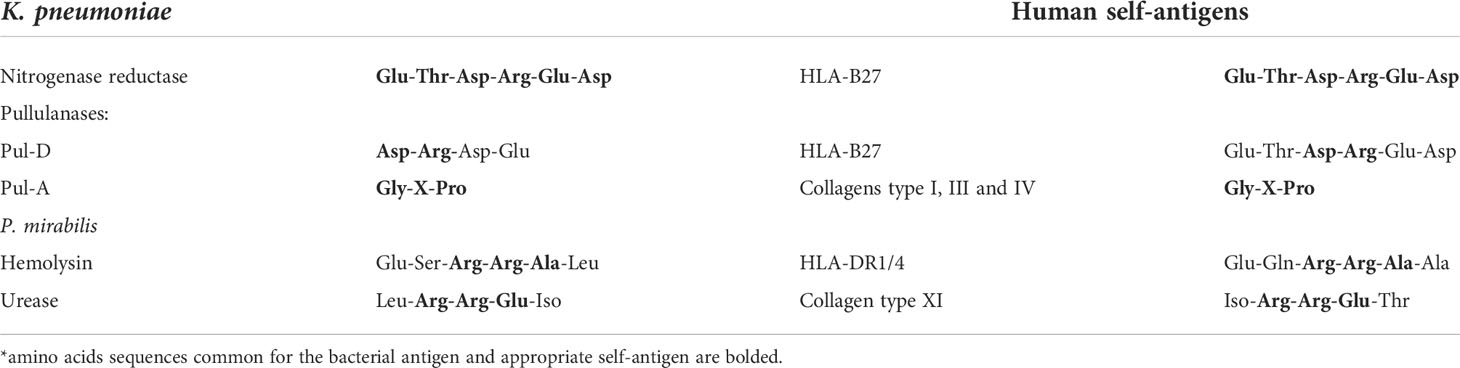
Table 1 Molecular similarities between human self-antigens and appropriate P. mirabilis and K. pneumoniae enzymes (Schwimmbeck et al., 1987; Ebringer et al., 1992; Fielder et al., 1995; Wilson et al., 1995; Ebringer et al., 2003; Ebringer et al., 2006; Rashid and Ebringer, 2007a; Rashid and Ebringer, 2007b; Konieczna et al., 2012).
3 The virulence factors with similar features observed for P. mirabilis and K. pneumoniae strains
P. mirabilis and K. pneumoniae along with Escherichia coli are the most common causes of UTI cases, both community- and hospital-acquired ones (Oloomi et al., 2020). The first two species contribute to recurrent UTIs, which derives from their multidrug-resistance (Hooton, 2001). These facts and co-isolation of both species from polymicrobial infections increase the interest in the similarities between virulence factors of K. pneumoniae and P. mirabilis (Macleod and Stickler, 2007; Galván et al., 2016). Many factors including: adhesins, siderophores, protein toxins, proteinases, ureases are implicated in the virulence of the representatives of both species, and a few have much in common in their structure and functions (Lindberg et al., 1975; Dutton et al., 1978; Joseleau et al., 1978; Joseleau and Marais, 1979; Sidorczyk et al., 1983; Joseleau and Marais, 1988; Jansson et al., 1994; Erbing et al., 1995; Mobley et al., 1995; Helander et al., 1996; Podschun and Ullmann, 1998; O’Hara et al., 2000; Vinogradov et al., 2002; Brisse et al., 2006; Holst, 2007; Knirel et al., 2011; Różalski et al., 2012; Konieczna et al., 2012; Kubler-Kielb et al., 2013). Urease, LPS and capsule polysaccharides will be discussed in detail in the next chapters as the virulence factors with the highest number of features common to both species (Lindberg et al., 1975; Dutton et al., 1978; Joseleau et al., 1978; Joseleau and Marais, 1979; Sidorczyk et al., 1983; Joseleau and Marais, 1988; Jansson et al., 1994; Erbing et al., 1995; Mobley et al., 1995; Helander et al., 1996; Vinogradov et al., 2002; Holst, 2007; Knirel et al., 2011; Konieczna et al., 2012; Kubler-Kielb et al., 2013).
3.1 Urease as the non-polysaccharide virulence factor with similar features observed for P. mirabilis and K. pneumoniae strains
Urease is the nickel containing enzyme catalyzing the hydrolysis of urea to yield ammonia and carbon dioxide. Ammonia has been found to damage the tissues and elevate urine pH resulting in crystallization of magnesium and calcium ions, which contributes to stone formation (Maroncle et al., 2006). In Klebsiella spp. urease is localized in cytoplasm and in P. mirabilis it is present in periplasm or in the outer membrane. P. mirabilis urease is induced by the presence of urea and K. pneumoniae urease – by the presence of poor nitrogen sources (Mobley et al., 1995).
Urease is a non-polysaccharide virulence factor, which shows structural similarities in both Klebsiella spp. and Proteus spp (Mobley et al., 1995; Stickler et al., 1998; Różalski et al., 2012). Similarities have been found in gene sequences in urease gene clusters (ureDABCEFG) in K. aerogenes and P. mirabilis. In contrast to K. aerogenes genome, in the P. mirabilis genome ureR gene (ureR-dependent promoter) was additionally detected - upstream of ureD (Mobley et al., 1995; Island and Mobley, 1995; Coker et al., 2000). The ureR regulates the urease expression in Proteus spp., and in Klebsiella spp. this role is played by the nitrogen regulatory system (NTR). Predicted molecular sizes of appropriate ure-encoded polypeptides are also similar in K. aerogenes [1] and in P. mirabilis [2]:
[1] UreA 11.1 kDa, UreB 11.7 kDa, UreC 60.3 kDa, UreE 17.6 kDa, UreF 25.2 kDa, UreG 21.9 kDa;
[2] UreA 11.0 kDa, UreB 12.2 kDa, UreC 61.0 kDa, UreE 17.9 kDa, UreF 23.0 kDa, UreG 22.4 kDa (Mobley et al., 1995).
The protein sequence of the UreC subunit from K. aerogenes is 72% identical to the UreC sequence from P. mirabilis (Mobley et al., 1995). Although urease is regarded as a conservative enzyme and its structure is similar for different bacteria, except Helicobacter pylori or Staphylococcus cohnii, the numbers of amino acids of particular polypeptides and the structure of the flap region in ureases of P. mirabilis and K. aerogenes show the highest homology. From the protein products of ure genes described for P. mirabilis and K. pneumoniae the highest similarities were observed for UreD [274 amino acids], UreG [205], α [567] and γ [100] (Konieczna et al., 2012).
More divergences noted for P. mirabilis and K. pneumoniae urease concerned its activity. The latter species has been reported to hydrolyze urea ten times slower than the former one (Stickler et al., 1998). Other studies using different solid media have also confirmed that correlation, i.e. the onset of urease reaction appeared after 9-10 h in the case of Klebsiella spp. strains and after 1-2 h for Proteus spp. (Vuye and Pijck, 1973). Some Klebsiella sp. strains are urease-negative, but they were found to enhance the P. mirabilis urease activity in the K. p. – P. m. coculture experiment (Armbruster et al., 2017). The underlying mechanism of enhanced urease activity of one strain by another may decrease the risk of development of complications in polymicrobial CAUTIs (Armbruster et al., 2017), thus elimination of one bacterial species may weaken the urease activity of the representatives of other species.
As was mentioned before, urease activity is connected with urinary stones occurrence. Proteus spp. is one of the most common pathogens inducing the formation of bladder and kidney stones, mainly struvite ones. Although Klebsiella spp. are mentioned as urease producing pathogens, also isolated from patients with urinary stones (mainly calculi containing oxalate and calcium phosphate) (Bichler et al., 2002; Torzewska et al., 2014), they have been found to produce none or little catheter encrustation and not to elevate urinary pH above 7.0 (Stickler et al., 1998; Broomfield et al., 2009). However, K. pneumoniae is known to produce mucoid plugs in the catheter lumen (Broomfield et al., 2009). P. mirabilis have been demonstrated to cause crystallization within the human ureter and bladder epithelial cells (Torzewska et al., 2014). It has been shown that the crystallization process may be enhanced (P. vulgaris O12) or inhibited (P. mirabilis O28 and O47) by the Proteus lipopolysaccharides (LPSs) depending on their O-polysaccharides (OPSs) structures and their affinity for Ca2+ and Mg2+ cations (Torzewska et al., 2003). Despite the role of bacterial urease in UTIs development, the metabolism of urea has also been demonstrated to be important in the gastro intestinal tract colonization by K. pneumoniae (Maroncle et al., 2006).
Urease is also produced by other Enterobacterales which are a part of human intestinal microflora but the level of the enzyme activity is species-specific and varies with the medium used (Vuye and Pijck, 1973). For example, among Escherichia coli strains, only 1% have been identified as urease-positive (Konieczna et al., 2012). Citrobacter and Enterobacter spp. are characterized as those with irregular urease activity. Among urease-positive Serratia spp. strains, urease action is delayed more than in the case of Klebsiella spp. strains (Vuye and Pijck, 1973). Despite the fact that Providencia morganii and P. rettgeri are regarded to be as effective urease producers as P. mirabilis, the urease activity, structure and genetic organization of the enzyme genes are well-known for Klebsiella and Proteus spp. frequently isolated from polymicrobial UTIs (Mobley et al., 1995; Konieczna et al., 2012; Galván et al., 2016).
3.2 Polysaccharide virulence factors with similar features observed for P. mirabilis and K. pneumoniae strains
3.2.1 Lipopolysaccharide
LPS is an essential component of the outer membrane of all Gram-negative bacteria and their major virulence factor. Due to its biological activity, LPS is regarded as an endotoxin. During infection it is released from bacterial cells to the blood where it is bound to LPS binding protein (LBP) and through CD14 and TLR4 (Toll-like receptor) induces macrophages to the pro-inflammatory cytokines secretion. Over-stimulation of the process leads to endotoxic shock development (Poxton, 1995). LPS exhibits many biological activities and its parts interact with bacterial or eukaryotic cells, e.g. O-polysaccharides chains, most outstanding from the bacterial cell, are involved in glycocalyx formation or make the bacteria resistant to the active membrane attack complex (Poxton, 1995; Różalski et al., 2012). It is worth mentioning that LPS is highly immunogenic and its parts induce the production of antibodies of different specificity, which is important in searching for broadly cross-reacting immunoglobulins (Poxton, 1995).
Smooth (S) forms of Proteus spp. and Klebsiella spp. bacteria produce LPS consisting of three parts differing in their chemical structures and biological functions: lipid A, a core region and an OPS (Vinogradov et al., 2002; Palusiak, 2013).
3.2.1.1 Lipid A
Lipid A anchors LPS in the outer leaflet of the bacterial outer membrane (Poxton, 1995). Lipid A is structurally the most conserved region of LPS among Gram-negative bacteria and in P. mirabilis and K. pneumoniae bacilli the lipid A structures are almost identical (Sidorczyk et al., 1983; Helander et al., 1996).
The lipid A structure of Proteus spp. LPS has been established for the deep rough R45 mutant of the P. mirabilis S1959 strain (Sidorczyk et al., 1983). The lipid A structure of Klebsiella spp. LPS was characterized in 1996 for the polymyxin-resistant K. pneumoniae O3 mutant OM-5 and its polymyxin-sensitive parent strain (LEN-1) (Helander et al., 1996). P. mirabilis R45, K. pneumoniae LEN-1 and OM-5 lipids A are similar in the fatty acids arrangement and composition. All contain seven fatty acids residues (heptaacylated lipid A) including four 3-hydroxytetradecanoic acids (3-OH-C14) attached directly to the backbone (bisphosporylated β(1→6) linked GlcN disaccharide), two tetradecanoic acids ester-linked to 3-OH-C14 linked to the nonreducing GlcN residue and one hexadecanoic acid that partially substitutes the hydroxyl group of 3-OH-C14 acid present at position 2 of reducing GlcN (Kotełko, 1986; Helander et al., 1996). For K. pneumoniae and P. mirabilis the hexaacylated species of lipids A (without hexadecanoic acid) have also been observed. In lipid A of LPSs from both species the ester-bound phosphate residue is substituted by 4-amino-4-deoxy-L-arabinopyranose (L-Arap4N). The feature, which probably distinguishes P. mirabilis R45 lipid A of LPS from lipids A of K. pneumoniae LEN-1 and OM-5 LPSs is a free glycosidically bound phosphate group, which in K. pneumoniae lipid A is substituted by L-Arap4N (Kotełko, 1986; Helander et al., 1996). However, L-Arap4N may be bound to the glycosidic phosphate group in a labile diester linkage, which could be cleaved under strong alkaline conditions. Thus, the structural analysis of P. mirabilis R45 LPS indicates only the putative substitution of a glycosidic phosphate residue by a pentosamine residue (Vinogradov et al., 1994). In K. pneumoniae LEN-1 and OM-5, the L-Arap4N is linked to both phosphates of the lipid A backbone. However, the degree of substitution of phosphates by L-Arap4N in both LPSs is remarkably different (Helander et al., 1996).
Lipid A of Proteus spp. as well as of other Enterobacterales ord. nov. is linked to the core region by a hydroxyl group at C6’ of a nonreducing GlcN residue (Kotełko, 1986).
3.1.1.2 The core oligosaccharide of LPS
Comparison of the structures of the LPS core regions in all tested Proteus spp. and K. pneumoniae strains shows that they share a common heptasaccharide fragment, which includes:
➢ a disaccharide of 3-deoxy-α-D-manno-oct-2-ulosonic acid (α-Kdo; A, B);
➢ a trisaccharide of L-glycero-α-D-manno-heptose (α-Hep; C, D, E);
➢ β-glucose (β-Glc; F) residue linked to α-Hep C at O (4);
➢ an α-galacturonic acid (α-GalA; G) residue linked to α-Hep D at O (3) (Figure 1) (Vinogradov et al., 2000; Vinogradov and Perry, 2001; Vinogradov et al., 2002; Regué et al., 2005; Holst, 2007; Vinogradov, 2011).
This heptasaccharide fragment is also a part of the LPS core region of two Serratia marcescens strains (Holst, 2007; Palusiak, 2013).
Other common residues which can be found in all tested LPS core regions of K. pneumoniae and some P. mirabilis strains, are: α-GlcN (H) attached to the α-GalA (G) residue at O4 and β-GalA (I/R3) linked to Hep (E) at O (7) (Figure 1) (Vinogradov et al., 2000; Vinogradov and Perry, 2001; Vinogradov et al., 2002; Regué et al., 2005; Holst, 2007; Vinogradov, 2011). A component common for P. mirabilis (O27) (Figure 1B) and K. pneumoniae (O1, O2a, O2a,c, O3-O5, O8, O12) LPS core regions (Figure 1C) is GalA (J) substituting β-Glc (F) at O (6) (non-stoichiometric substitution) (Vinogradov and Perry, 2001; Vinogradov et al., 2002; Holst, 2007). Major structural differences between LPS core regions of P. mirabilis and K. pneumoniae strains concern the outer core region (Figure 1) (Vinogradov et al., 2002; Regué et al., 2005).
It is worth mentioning that over 21 different structures of the outer core region have been determined in Proteus spp., among which eight have been characterized for P. mirabilis strains. These structures are mainly linear or branched (P. mirabilis O48) and contain from two to four sugar residues, some of which are acylated (Vinogradov et al., 2002; Vinogradov, 2011). In K. pneumoniae only two structures of the LPS core region differing in the substituents of α-GlcN (H) have been described (Figures 1C, D) (Vinogradov and Perry, 2001; Regué et al., 2005). Neither LD-Hep nor α-Kdo, present in the outer core region of K. pneumoniae LPSs (type 1 core) (Figure 1C), has ever been found in this part of Proteus spp. LPSs (Vinogradov et al., 2002; Vinogradov, 2011). Also, the P. mirabilis LPS outer core region may contain components which are not found in the K. pneumoniae LPS core region e.g. cyclic acetal formed by an 2-acetamido-2-deoxy-D-galactose residue in the open-chain form (GaloNAc) linked to O (4) and O (6) of the neighboring GalN residue (P. mirabilis O27) (Figure 1B) (Vinogradov et al., 2002).
The great structural heterogeneity of Proteus spp. LPS core regions does not result from the differences only in the outer core region but also in the inner core region, which is in general more structurally conserved in other Enterobacterales ord. nov. LPSs (Vinogradov et al., 2002; Palusiak, 2013).
In contrast to K. pneumoniae LPSs, three glycoforms differing in two substituents R2 (α-DD-Hep-(1→2)-α-DD-Hep, α-DD-Hep or H) and R3 (β-GalA or H) have been determined for the inner core region of Proteus spp. LPSs. The majority of P. mirabilis LPSs tested so far have presented glycoform II of their inner core region (Figures 1A, B) (Vinogradov et al., 2002; Kondakova et al., 2005). What is more interesting, multiple structural variants of the P. mirabilis core region may appear even within the LPS of one strain e.g. two types of the core region in P. mirabilis O27 (Figure 1B) and four different R1 (PEtn), R2, R3 combinations in the P. mirabilis O3 LPS core region (Vinogradov et al., 2002), which does not occur in the case of K. pneumonia (Holst, 2007).
The core region of the P. mirabilis O27 LPS is similar to the core region of K. pneumoniae LPS due to the lack of L-Arap4N linked to the Kdo (A) residue at O (8) (Figures 1B–D) (Regué et al., 2005; Holst, 2007). L-Arap4N occurs at this position in the other tested Proteus spp. LPSs (Figure 1A) (Vinogradov et al., 2002) and has been found to play a crucial role in the decreased binding of polymyxin B (Boll et al., 1994).
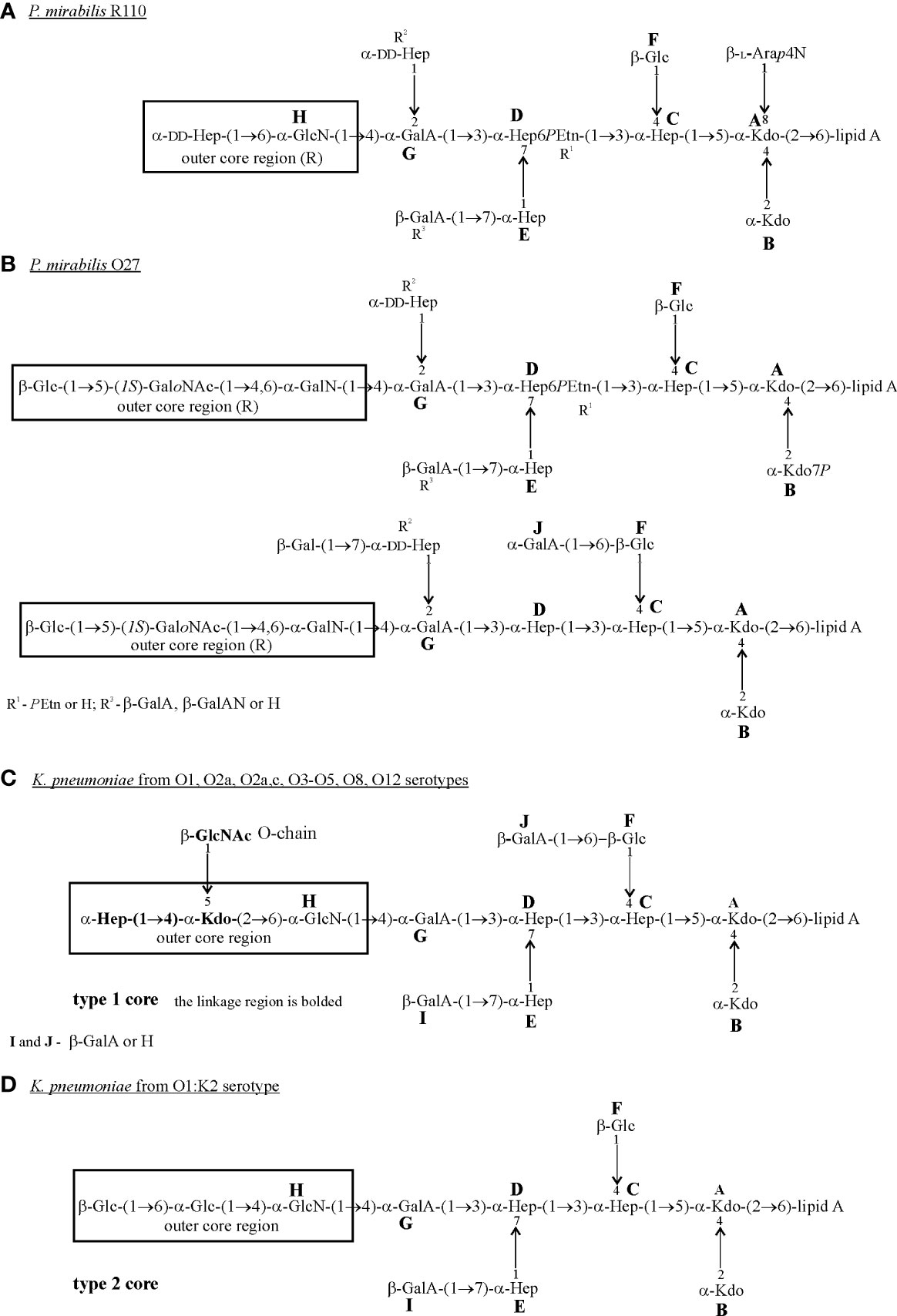
Figure 1 Structures of the core regions of: (A) P. mirabilis R110; (B) P. mirabilis O27; (C) K. pneumoniae from O1, O2a, O2a,c, O3-O5, O8 and O12 serotypes; (D) K. pneumoniae from the O1:K2 serotype (Vinogradov et al., 2000; Vinogradov and Perry, 2001; Vinogradov et al., 2002; Regué et al., 2005; Holst, 2007). The outer core region (R) is indicated by the frame. Arap4N, 4-amino-4-deoxy-L-arabinopyranose; GalA, galacturonic acid; GalAN, GalA amidated by aliphatic polyamines; GalN, galctosamine; GaloNAc, open-chain of GalNAc; Glc, glucose; GlcN, glucosamine; GlcNAc, 2-acetamido-2-deoxy-D-galactose; LD-Hep, L-glycero-D-manno-heptose; DD-Hep, D-glycero-D-manno-heptose; Kdo, 3-deoxy-D-manno-oct-2-ulosonic acid; PEtn,2-aminoethyl phosphate.
3.1.1.3 O-polysaccharide of LPS
To date, the O-antigen linkage in Proteus spp. LPS has not been precisely determined. However, the R substituent of the core region (Figure 1) is indicated as a possible linkage site of OPS (Vinogradov et al., 2002). The K. pneumoniae type 1 core oligosaccharide is linked to OPS via O-5 of the Kdo residue from the outer core region (Figure 1C) (Vinogradov et al., 2002; Holst, 2007). This Kdo residue is a part of the linkage region, which is common to all tested K. pneumoniae O antigens (Figure 1C), the bolded fragment. The primer β-GlcNAc residue forming the linkage region is derived from the O-chain biosynthesis pathway and is substituted by a repeating unit of the majority of K. pneumoniae O antigens or by the bridging disaccharide 3)-(α-Man-(1→3)-α-Man) in K. pneumoniae O3 and O5 OPSs (Figures 2D, E) (Vinogradov et al., 2002; Knirel, 2011). In K. pneumoniae strain 52145 from serotype O1:K2 (type 2 OS) (Figure 1D) the last Glc outer-core residue has been suggested to be a linkage site of OPS (Regué et al., 2005).
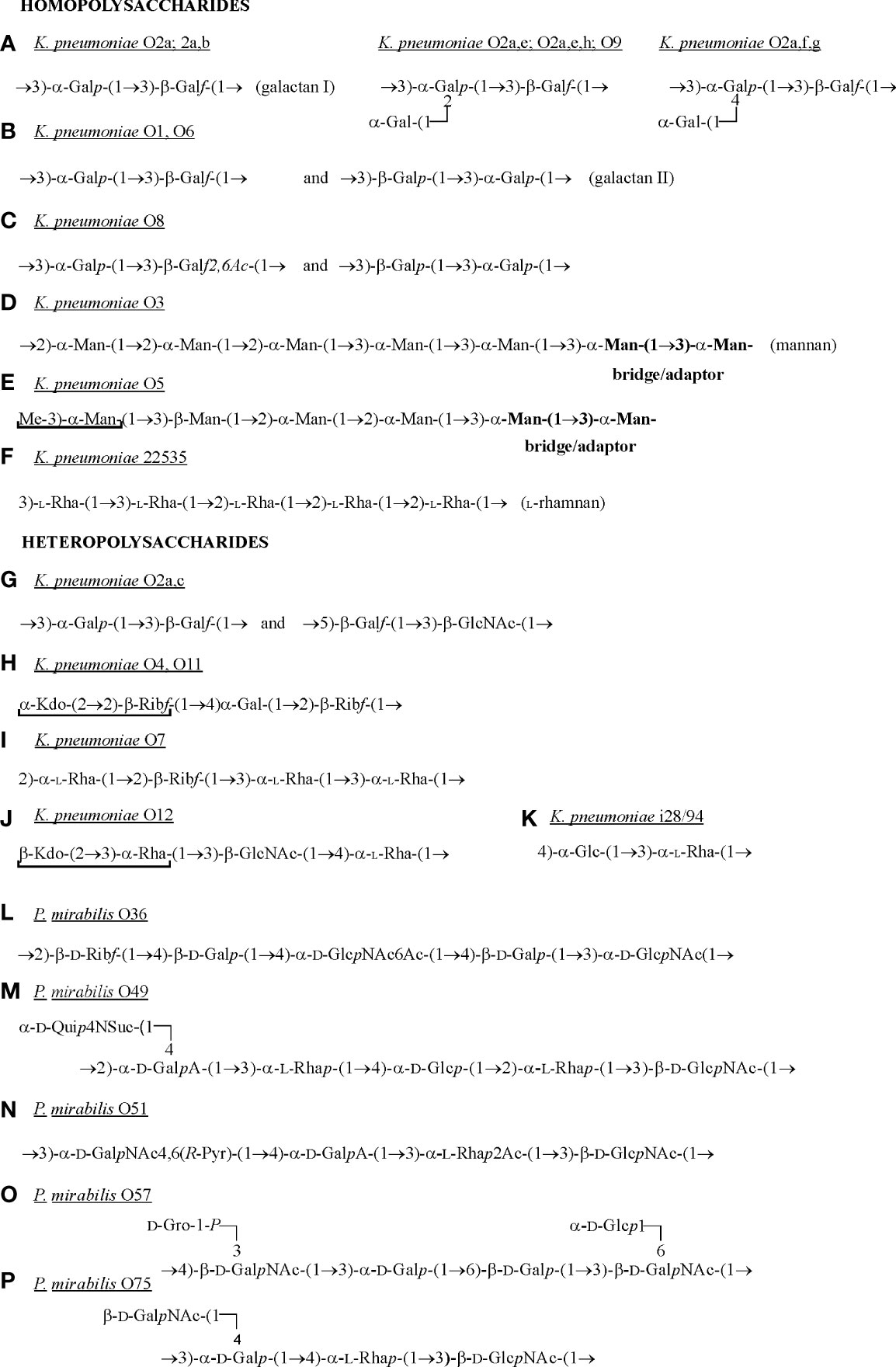
Figure 2 Structures of K. pneumoniae O-polysaccharides (A–K) and of those P. mirabilis OPSs (L-P), which have the components common with the selected K. pneumoniae OPSs (Knirel, 2011; Knirel et al., 2011). The residues which do not belong to the repeating units are underlined. Galf, galactofuranose; Galp, galactopyranose; Glc, glucose; GlcNAc 2-acetamido-2-deoxy-D-galactose; GalA, galacturonic acid; D-GalNAc, 2-acetamido-2-deoxy-D-galactose; D-Gro-1-P, D-glycerol 1-phosphate; Kdo, 3-deoxy-D-manno-oct-2-ulosonic acid; Man, mannose; Pyr – pyruvic acid; Rha, ramnose; Ribf, ribofuranose; Suc, succinic acid; Qui4n, 4-amino-4-deoxy-D-quinovose.
The OPS of P. mirabilis is definitely more structurally heterogenous than that of K. pneumoniae. For P. mirabilis strains, 49 different OPS structures have been determined and some of them (e.g. P. mirabilis O69 OPS) are common to other representatives of the genus (Knirel et al., 2011; Drzewiecka et al., 2016a; Drzewiecka et al., 2016b). The OPS structures of the most Proteus strains tested so far have been already gathered and presented in a review by Knirel et al. (2011) (Knirel et al., 2011). Therefore, in this review only structures of OPSs, which share common fragments with Klebsiella CPSs are presented (Figure 3).
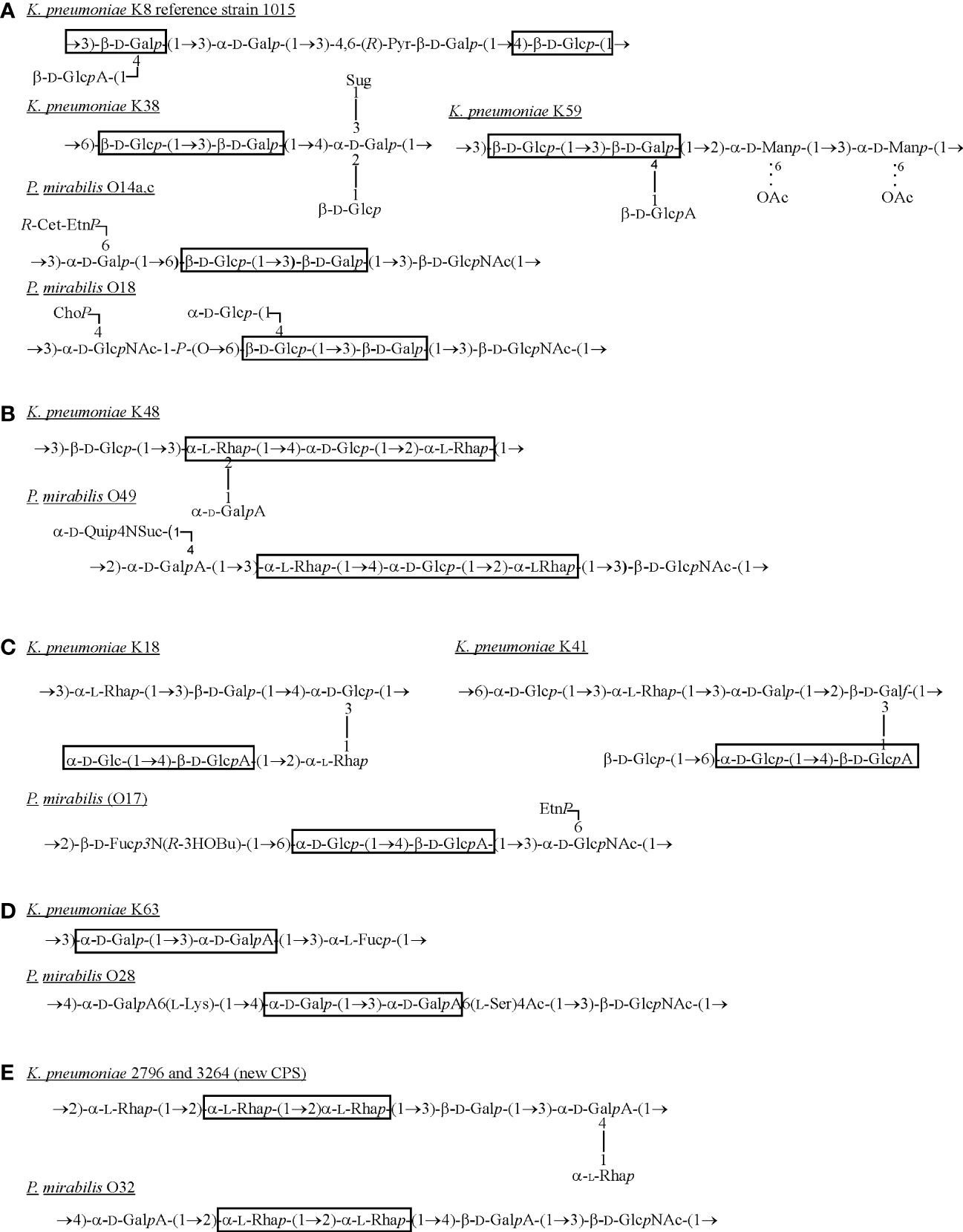
Figure 3 Structures of K. pneumoniae capsular polysaccharides and P. mirabilis OPSs (A–E) sharing the common fragments (in frames) (Lindberg et al., 1975; Dutton et al., 1978; Joseleau et al., 1978; Joseleau and Marais, 1979; Joseleau and Marais, 1988; Jansson et al., 1994; Erbing et al., 1995; Knirel et al., 2011; Kubler-Kielb et al., 2013). Dotted lines indicate that some of the residues are O-acetylated. ChoP, choline phosphate; EtnP, ethanolamine phosphate; Fuc, fucose; D-Fuc3N, 3-amino-3-deoxy-D-fucose; Galf, galactofuranose; Galp, galactopyranose; GalA, galacturonic acid; Glc, glucose; GlcA, glucuronic acid; D-GlcNAc, 2-acetamido-2-deoxy-D-glucose; L-Lys, L-lysine; Man, mannose; Pyr – pyruvic acid; R-3HOBu, (R)-3-hydroxybutanoic acid; R-Cet-EtnP, N-[(R)-1-carboxyethyl]ethanolamine phosphate; Rha, ramnose; L-Ser, L-serine; Suc, succinic acid; Sug, 4-deoxy-threo-hex-4-enopyranosyluronic acid group; Qui4N, 4-amino-4-deoxy-D-quinovose.
In K. pneumoniae only 13 OPS structures have been determined (Knirel, 2011). Most OPS structures presented by P. mirabilis strains are branched, and in K. pneumoniae LPSs the lateral substituent appears only in two OPS structures (Figure 2A) (Knirel, 2011; Knirel et al., 2011). P. mirabilis OPSs consist of repeating units comprising from three to seven sugar and non-sugar components and K. pneumoniae repeating units include from two to five sugar components. Proteus spp. OPSs are heteropolysaccharides, whereas, among K. pneumoniae O-antigens, homopolysaccharides also occur (e.g. O3, O5, 22535) (Figures 2D–F). Homopolymers are also present in K. pneumoniae OPSs in a form of galactan I: 3)-α-Galp-(1→3)-β-Galf(1-, which forms the repeating units alone (e.g. O2a, O2a,b OPSs (Figure 2A) or together with galactan II: 3)-β-Galp-(1→3)-α-Galp(1- (O1, O6 and O8) (Figures 2B, C) (Knirel, 2011). The latter is placed distally toward galactan I and defines the O1 serotype (Vinogradov et al., 2002).
The β-Gal or α-Gal residues are found in P. mirabilis OPSs quite frequently but contrary to the β-Gal residues in Klebsiella OPS, they always occur in a pyranose form. These residues are present in P. mirabilis OPSs as single Gal residues (P. mirabilis O75) (Figure 2P) or as Gal disaccharides but in a different sequence than in galactan II in K. pneumoniae OPS e.g. 3)-α-Galp-(1→6)-β-Galp(1- (P. mirabilis O57) (Figure 2O) (Vinogradov et al., 2002; Knirel et al., 2011).
Unlike P. mirabilis OPSs, the OPS chains of K. pneumoniae O4, O5, O11 and O12 antigens possess at their non-reducing ends residues that are not composed of the repeating units e.g. α-Kdo-(2→2)-β-Ribf (O4), Me-3)α-Man (O5) or β-Kdo-(2→3)-α-Rha (O12) (Figures 2E, H, J) - underlined residues (Vinogradov et al., 2002). Kdo or Man residues have not been found in P. mirabilis OPSs so far (Knirel et al., 2011). Other repeating units structures of K. pneumoniae OPSs are heteropolymers (Figures 2G–K) (Vinogradov et al., 2002; Knirel, 2011). The single components of the K. pneumoniae O12 OPS (Figure 2J) are also found in P. mirabilis OPSs: α-Rha-(1→3)-β-D-GlcNAc(1- (P. mirabilis O49 and O75 or in O51, where α-Rha is 2-O-acetylated) (Figures 2M, N, P). Components similar to those found in the repeating units of the K. pneumoniae O4 and O11 antigens [4)-α-Gal-(1→2)-β-Ribf(1-] (Figure 2H) have also been detected in the P. mirabilis O36 antigen: 2)-β-Ribf-(1→4)-β-Gal(1- (Figure 2L) (Knirel et al., 2011; Knirel, 2011).
3.1.1.3.1 Klebsiella spp. and Proteus spp. O-typing schemes
The O-antigen determines the serological specificity of the S and SR forms of bacteria (Knirel et al., 2011). The classification scheme for Proteus spp. was founded by Kauffmann (1966) and included 49 different P. mirabilis and/or P. vulgaris O serogroups (Kauffmann, 1966). This scheme was completed by Larsson et al. (1978) (six P. mirabilis O-serogroups) (Larsson et al., 1978), by Penner and Hennessy (1980) (11 O-serogroups containing P. mirabilis or P. vulgaris strains) (Penner and Hennessy, 1980) and by other authors (O-serogroups consisting of different Proteus species or of one species) (Knirel et al., 2011; Kaca et al., 2011; Palusiak, 2013; Palusiak et al., 2013). Currently, the Proteus O-antigen classification scheme includes 83 O-serogroups, among which 43 contain P. mirabilis strains (the majority of them contain P. mirabilis strains only and in eight O-serogroups there are also other representatives of the genus – O8, O11, O13, O17, O23, O34, O54, O69 and O71) (Knirel et al., 2011; Drzewiecka et al., 2016a; Drzewiecka et al., 2016b). O77-O82 serogroups were created from Proteus clinical isolates from the Łódź area (Central Poland) (Drzewiecka et al., 2021).
The serological classification of Klebsiella spp. strains was reported in 1940 by Kauffmann and Ørskov, who separated three O groups: 1, 2 (with 2A and 2B subgroups) and 3, among which the O1 group was the most numerous (Ørskov, 1954). Initially, 12 O-antigen serogroups were described but structural similarities and the cross-reactivities between some of them resulted in slight differences in the authors’ opinions on the Klebsiella O typing scheme (Trautmann et al., 1997; Hansen et al., 1999; Vinogradov et al., 2002). For example, Trautmann et al. (1997) described the Klebsiella O9 serogroup as a separate one but the investigation by Hansen et al. (1999) revealed that the LPS from the strain prototype for serogroup O9 was structurally and serologically identical to the LPS from the strain belonging to serogroup O2 (Trautmann et al., 1997; Hansen et al., 1999). Thus serogroup O9 was proposed to be treated as a part of serogroup O2 (Hansen et al., 1999). Trautmann suggested excluding serogroup O8 (together with O6) from the scheme since O8 antigens had been found to be serologically indistinguishable from O1 LPSs (Trautmann et al., 1997; Trautman et al., 2004). On the contrary, Hansen et al. (1999) presented serogroup O8 as a separate one, mentioning that genetic methods would help in the distinction between O1 and O8 representatives (Hansen et al., 1999).
Among Klebsiella spp. O serotypes, O1 (the most often), O2 and O5 dominated among clinical isolates from Japan, Germany, Denmark, Spain and the United States with the exception of blood isolates from the USA where O2 representatives dominated over O1 (Trautmann et al., 1997; Hansen et al., 1999; Brisse et al., 2006). As for Proteus spp., the O3 serogroup dominated among P. mirabilis isolates according to the studies described by Larsson. In most of the studies Proteus O10 and O30 serogroups were also frequently found (Larsson, 1984). The O78 serogroup was found to be the most numerous in the Proteus classification scheme and seemed to be prevalent among patients from the Łódź area (Drzewiecka et al., 2021). K. pneumoniae O2 is the most heterogenous serotype characterized by the presence of many partial antigens (Figures 2A, G), among which O2ab was detected in the majority of O1 and O2ab clinical isolates tested (Whitfield et al., 1992; Trautmann et al., 1997; Trautman et al., 2004). In the Proteus spp. classification scheme the most heterogenous serogroup containing P. mirabilis strains is O23 with two subgroups (O23a,b,c; O23a,b,d) and with two OPSs structural variants among O23a,b,c representatives (Knirel et al., 2011).
The Proteus spp. classification scheme is based on the structural and serological diversity of LPS OPSs (Knirel et al., 2011). In the Klebsiella spp. classification scheme the crucial role is played by the heat-stable K-antigens, which are more diverse (77 serotypes) than the OPS parts (Podschun and Ullmann, 1998). However, the huge number of K serotypes and serological cross-reactions among them limit the K serotyping (Podschun and Ullmann, 1998; Brisse et al., 2006).
3.2.1.2 Capsular polysaccharide
Hydrophilic polysaccharide capsules (K antigens) are the first virulence factors described for the genus Klebsiella and they are the most thoroughly studied ones (Brisse et al., 2006). The capsule surrounds the entire cell protecting it from drying out, phagocytosis and killing by serum and is associated with the development of the later stages of complex biofilm. Capsules are acidic structures, built of repeating units with four to six sugars and produced by the majority of uropathogenic K. pneumoniae strains (Schembri et al., 2005; Brisse et al., 2006). The size of Klebsiella CPSs influences the bacterial virulence. In contrast to highly encapsulated K. pneumoniae KP1-O which has been found to be extremely virulent in the murine burn wound sepsis model, the strain KP1-T with a smaller capsule (approximately one-third of that KP1-O) appeared to be comparatively nonvirulent (Cryz et al., 1984). The virulence of K. pneumoniae strains is also associated with a CPS structure. In less virulent K7 and K21a capsular antigens, the repetitive sequence of mannose-α-2/3-mannose is recognized by mannose-α-2/3-mannose-specific lectin of macrophages, which leads to lectinophagocytosis. On the other hand, the lack of mannose-2/3-mannose structures in more virulent K2 strains protects them against this process (Brisse et al., 2006). The capsule is so important in K. pneumoniae virulence because it may also influence the other virulence factors activity. It has been shown on K. pneumoniae strain C105 and its non-capsulated derivative that capsule expression results in impeding biofilm formation on the abiotic surface and agglutination of yeast cells, both processes mediated by type 1 fimbriae. This phenomenon has been suggested to result from the direct physical interference between type 1 fimbriae and the capsule (Schembri et al., 2005). CPS has been found to be a dominating (63%) component of the extracellular toxic complex (ETC), which is also composed of LPS (30%) and protein (7%). It has been shown that pure ETC of K. pneumoniae K8 strain exerts the cytotoxic effect on the rat embryo fibroblast cell line and changes the cell morphology. Although LPS accounts for ECT toxicity, the ETC effectiveness depends on the presence of a sufficient cell-associated capsule protecting the cell from phagocytosis (Al-Jumaily et al., 2012).
Among 78 K serotypes, 25 are mainly found in Klebsiella spp. clinical isolates causing bacteremia and the K2 serotype is predominant, followed by K1, in all Klebsiella spp. clinical isolates causing UTI, pneumonia and bacteremia (Podschun and Ullmann, 1998; Paczosa and Mecsas, 2016). Also, the K2 serotype, together with K1, is common among hvKP strains causing CA-PLA (community-acquired pyogenic liver abscesses). However, hypervirulence of K2 hvKP strains is believed to be connected with an increased expression of capsular material (hypermucoviscous phenotype) associated with the rmpA gene (a regulator of the mucoid phenotype) rather than the capsule serotype (Shon et al., 2013). Hypercapsulation increases resistance to complement killing or human neutrophil protein 1 and lactoferrin. The prevalence of more virulent K1 and K2 phenotypes among clinical K. pneumonia isolates results from their better capacity of survival in tissues and a higher resistance to lectinophagocytosis (Paczosa and Mecsas, 2016). In contrast to clinical isolates, the K33 and K69 serotypes dominated among environmental strains (e.g. from surface waters) (Podschun et al., 2001). Recently, on the basis of CPS locus and K-locus (KL) arrangement, series KL101-KL149, KL151, KL153-KL155 and KL157-159 have been additionally discovered, however their sugar composition remains unknown (Patro and Rathinavelan, 2019).
In Proteus spp. only four CPS structures have been described (two for P. mirabilis and two for P. vulgaris strains) (Beynon et al., 1992; Perry and MacLean, 1994; Rahman et al., 1997; Rahman et al., 1999). Proteus spp. CPSs consist of three to four saccharide residues and, similarly to Klebsiella CPSs, are acidic (due to the presence of uronosyl residues e.g. β-D-GalA in P. mirabilis WT19 or α-D-GlcA in P. mirabilis ATCC 49565). The acidic CPS is believed to act as a lubricant facilitating the migration of Proteus spp. swarmer cells (Rahman et al., 1997). However, in the Proteus spp. serological classification scheme, CPS is not taken into account due to the discovery that the CPSs of the two tested P. mirabilis 49565 and P. vulgaris ATCC 49990 strains had the same structures as their LPSs O chains (Beynon et al., 1992; Perry and MacLean, 1994).
What is interesting, while analyzing the selected Klebsiella spp. CPSs and Proteus spp. OPSs structures, common fragments could be observed as shown in the frames (Figure 3) (Lindberg et al., 1975; Dutton et al., 1978; Joseleau et al., 1978; Joseleau and Marais, 1979; Joseleau and Marais, 1988; Jansson et al., 1994; Erbing et al., 1995; Knirel et al., 2011; Kubler-Kielb et al., 2013). The first fragment (Figure 3A) is rather a common component of K. pneumoniae CPSs and it may be substituted by the lateral residue of β-D-GalA like in K8 (reference strain 1015) or K59 serotypes (Lindberg et al., 1975; Erbing et al., 1995). The mentioned K serotypes share the fragment, 3)- β-D-Glcp-(1→3)-β-D-Galp(1-, with two P. mirabilis O serotypes, however this structure was not found in OPS of other known O serotypes of the Enterobacterales LPSs (Knirel, 2011). The components of fragment 3a are also present in the LPS OPS of Providencia alcalifaciens O9, O23, Hafnia alvei or Edwardsiella ictaluri MT 104 but in a different sequence and conformation, which makes the fragment unique for P. mirabilis OPS and K. pneumoniae CPS. The more common fragments of polysaccharides from many different bacterial species share more cross-reactions between specific antibodies and common epitopes can be observed in serological studies (Palusiak, 2021). The α-L-Rha residues are common components of K. pneumoniae CPSs (Figures 3B, C, E) which are rarely found in Proteus OPSs (Proteus spp. O22, O32 or O75) (Figure 2P, Figure 3E) (Joseleau and Marais, 1988; Athamna et al., 1991; Knirel et al., 2011; Kubler-Kielb et al., 2013). The different rhamnose disaccharides are also commonly found in OPSs of other Enterobacterales LPSs like: Hafnia alvei, Citrobacter youngae, C. braakii, Escherichia hermannii (Knirel, 2011). The fragment 2)-α-L-Rha-(1→2)-α-L-Rha(1-, depicted in Figure 3E as common for appropriate K. pneumoniae and Proteus mirabilis serotypes, is located in H. alvei 1222 OPS, where it is phosphorylated, in many Shigella flexneri O serotypes (Knirel, 2011).
The knowledge on polysaccharide fragments common to such a wide range of Enterobacterales would be helpful in obtaining broadly cross-reactive antibodies that would be also protective and, when given to patients, would neutralize LPS and prevent from septic shock development (Poxton, 1995). Another example of applying common polysaccharide fragments is ELISA construction where the fragments would be used as coated antigens recognized by specific antibodies from the patients sera. Such an assay, EndoCAB™, was applied for screening blood donors for high levels of cross-reactive antibodies specific to LPS core regions of K. pneumoniae, E. coli, S. minnesota and Pseudomonas aeruginosa R-mutants used as antigens (Poxton, 1995).
Sharing common fragments of polysaccharides by representatives of different bacterial genera is meaningful in the development of a multivalent vaccine with a broad spectrum of cross-protection in vivo. Such a vaccine may contain whole bacterial cells (inactivated, attenuated or cell lysates), the whole antigens or their fragments (epitopes) (Oloomi et al., 2020).
4 Vaccine formula based on K. pneumoniae and P. mirabilis antigens
There are three commercially available vaccines composed of inactivated enterobacterial strains, including K. pneumoniae and Proteus spp.: Uromune® (sublingual spray), Urovac® (administered via vaginal suppositories) and Urostim® (administered orally), whose administration to volunteers led to a reduction of recurrent UTIs with slight potential adverse reactions (Nenkov, 2000; Uehling et al., 2003; Hopkins et al., 2007; Yang and Foley, 2018). However, applying a whole cell vaccine may be associated with some disadvantages like the non-specific immune responses and toxicity of such formulations (Oloomi et al., 2020; Assoni et al., 2021). The subunit of K. pneumoniae vaccines containing CPSs as antigens was tested in clinical trials, which showed encouraging response to the vaccine formula (Campbell et al., 1996). It is worth remembering that immune responses elicited by an isolated antigen are not long-lasting (lack of immunological memory) and are not T cell-dependent, thus antigen conjugation to carrier protein could enhance its immunogenicity (Lin et al., 2022). Lin et al. (2022) developed a new method of obtaining conjugated vaccine antigens by using K1 and K2 CPS depolymerases to receive depolymerized CPS without losing their modifications (related to CPS immunogenicity), which typically appear after applying standard chemical reagents. Vaccination of mice with K1 and K2 oligosaccharides conjugated with CRM197 carrier protein induced anti-CPS antibodies and protected mice from subsequent infection by the respective K. pneumoniae K-serogroup (Lin et al., 2022). The last type of the above-mentioned vaccines is the epitope-driven formula. It would be ideal if such a vaccine included many epitopes common to antigens of different bacterial species. The fragments (marked with frames in Figure 3) common for K. pneumoniae CPS and P. mirabilis OPS may be potential candidates for the multi-epitope formula development. Importantly, some of the fragments also occur in OPS of other Enterobacterales LPSs (Knirel, 2011), which gives a chance to obtain a broad spectrum of immune system inducers. It should be remembered that the construction of a vaccine of that type requires applying complex modern methodology e.g. bioinformatics tools for searching databases, multi-epitopes synthesis, cloning and protein expression methods. A novel multiepitope candidate vaccine based on the epitopes of nine common E. coli protein antigens, which were conserved for the epitopes of K. pneumoniae and P. mirabilis antigens and were MHC I and MHC II inducers, provided a significant protection in the bladder and kidneys in the UTI mice model (Oloomi et al., 2020).
Apart from the studies on the multiepitope vaccine including epitopes/fragments shared by both K. pneumoniae and P. mirabilis antigens, other investigations also concerned developing a vaccine formula based on one kind of antigen e.g. Klebsiella spp. capsule polysaccharide (mentioned above) or MR/K or P. mirabilis MrpH or Pta (Campbell et al., 1996; Lavender et al., 2005; Wang et al., 2016; Armbruster et al., 2018; Assoni et al., 2021).
MR/K-HA (type-3) - mannose-resistant Klebsiella-like hemagglutinins (19.5-21.5 kDa) agglutinate tannin treated ox erythrocytes and mediate binding of bacteria to human endothelial and uroepithelial cells, tubular basement membranes and Bowman’s capsules of the kidneys and urinary catheters (Podschun and Ullmann, 1998; Di Martino et al., 2003; Brisse et al., 2006; Sękowska and Gospodarek, 2007; Różalski et al., 2012). It has been showed that type 3 fimbrial shaft (MrkA) facilitates bacterial interactions and biofilm formation on abiotic surfaces including catheters, which may lead to CAUTIs development (Wu and Li, 2015; Langstraat et al., 2001; Schroll et al., 2010). MR/K-HA were first discovered in Klebsiella spp. strains and they are the main adhesive factors of K. pneumoniae, occurring more frequently in clinical than in sewage isolates (Di Martino et al., 2003; Brisse et al., 2006). Mice immunization with recombinant MrkA or purified type III fimbriae induced protection in a pneumonia model (Lavender et al., 2005; Wang et al., 2016; Assoni et al., 2021).
MR/P fimbriae - mannose resistant Proteus like fimbriae (agglutinating untreated erythrocytes in the presence of mannose) are the most important fimbriae for enhancing the formation of P. mirabilis initial biofilm, involved in the upper urinary tract colonization (especially the bladder), pyelonephritis development and showing autoaggregative properties (Jansen et al., 2004). Administration of the purified P. mirabilis HI4320 MR/P fimbriae to mice by different routes provided protection against reinfection with a homologous strain in 63% of animals in the case of intranasal or transurethral routes (Li et al., 2004; Armbruster et al., 2018). When purified recombinant MrpA (the main structural subunit of MR/P) was tested as a vaccine antigen in an ascending and a hematogenous model of UTI in mice, the subcutaneously immunized animals appeared to be protected against P. mirabilis UTI (Pellegrino et al., 2003).
Proteus toxic agglutinin (Pta) is a surface associated calcium-dependent subtilisin-like alkaline serine protease. Pta exhibits bifunctional action by mediating the autoaggregation of bacterial cells or eliciting cytopathic effects on cultured kidney and bladder epithelial cells. Pta seems to be pathogen specific and its activity during infection is probably urease dependent. The passenger domain containing catalytic residues (Ser366, His147, Asp533) accounts for Pta cytotoxic activity (Alamuri and Mobley, 2008). Pta is a promising vaccine antigen - mice immunization with purified intact Pta or the passenger domain (Pta-α), each conjugated with cholera toxin (CT) protects significantly against P. mirabilis UTI, mainly in the upper urinary tract (Alamuri et al., 2009).
5 Klebsiella spp. and Proteus spp. LPSs in the serological studies
Structural similarities between polysaccharides of Klebsiella spp. and Proteus spp. strains were revealed in numerous cross reactions of Klebsiella LPSs with polyclonal rabbit sera specific to 11 Proteus spp. strains (Palusiak, 2015; Palusiak, 2021). Using the Western blotting technique allowed showing the reactions which mainly concerned the high-molecular-species containing LPS moieties with O-polysaccharide chains. The patterns typical for LPS low-molecular-mass-species corresponding to core-lipid A fractions were also noticed in the case of four Klebsiella LPSs and P. penneri 2 antiserum. Considering the fact that the LPS core regions of the representatives of both genera are very similar to each other, mainly in their inner parts, the cross reactions concerning this region should be more common (Palusiak, 2021). However, it ought to be remembered that the polysaccharide part of LPS may restrict an access to the core region and thus the reactions with core-specific-antibodies may not have been detected (Palusiak, 2013).
The reactions differed in their intensity depending on the used serum and they were noticed not only for the representatives of different Proteus O serogroups but also for different species of the genus e.g. P. mirabilis, P. vulgaris or P. penneri. This observation might be crucial for the future selection of vaccine antigens which should be characterized by having common fragments with as many LPSs as it is possible to induce the broad spectrum of protection. It should be remembered that LPS exhibits high toxicity which limits the possibilities of using this antigen in the vaccine formula (Assoni et al., 2021). However polysaccharides only induced T-cell-independent response, thus a conjugated vaccine would be more recommended as promoting strong, long lasting responses in all age groups of individuals (Assoni et al., 2021; Lin et al., 2022).
An important question which should be addressed is whether the cross-reactions appear in opposite systems e.g. Proteus LPSs and Klebsiella antisera and Klebsiella LPSs and Proteus antisera. In the mentioned studies such cross-reactions appeared in the case of four LPSs, P. penneri 19, 22 and 60 and K. oxytoca 0.062, which may indicate them as potential vaccine antigens. However, the research should be further completed with the data from detailed structural studies to show which O antigen fragments contribute to cross-reactions (Palusiak, 2021). So far, antigen O of Shigella sonnei has been successfully used in vaccines administered to humans in clinical studies (Launay et al., 2017). Long-termed catheterized patients and those prone to the recurrent UTIs of Klebsiella spp. and P. mirabilis etiology may be predestined to receive the LPS vaccine via the intranasal route providing the highest protection (Armbruster et al., 2018).
Summarizing, both K. pneumoniae and P. mirabilis are important etiological factors of nosocomial infections, especially those affecting the urinary tract, which may be infected by both pathogens simultaneously (Wu and Li, 2015; O’Hara et al., 2000; Brisse et al., 2006; Macleod and Stickler, 2007; Różalski et al., 2012). Such polymicrobial infections are often difficult to treat due to the development of biofilm, in which the cells of both species may cooperate in reaching the upper parts of the tract, and to frequent multidrug resistance of the bacteria (Macleod and Stickler, 2007; Jacobsen et al., 2008; Cuevas et al., 2010; Drzewiecka and Lewandowska, 2016). Thus, there is a need to acquire better knowledge on the virulence factors of both pathogens, especially those exhibiting common features. For instance, the examination of common fragments in highly immunogenic polysaccharides i.e. K. pneumoniae CPSs and P. mirabilis OPSs (Figure 3) and detection of various cross-reactions (Palusiak, 2015; Palusiak, 2021) are of high importance for the creation of a vaccine protecting against both pathogens.
Author contributions
AP conceived and designed of the review and prepared the whole manuscript.
Conflict of interest
The author declares that the research was conducted in the absence of any commercial or financial relationships that could be construed as a potential conflict of interest.
Publisher’s note
All claims expressed in this article are solely those of the authors and do not necessarily represent those of their affiliated organizations, or those of the publisher, the editors and the reviewers. Any product that may be evaluated in this article, or claim that may be made by its manufacturer, is not guaranteed or endorsed by the publisher.
Glossary
References
Adeolu, M., Alnajar, S., Naushad, S., Gupta, R. S. (2016). Genome-based phylogeny and taxonomy of the’enterobacteriales’: Proposal for Enterobacterales ord. nov. divided into the families Enterobacteriaceae, Erwiniaceae fam. nov., Pectobacteriaceae fam. nov., Yersiniaceae fam. nov., Hafniaceae fam. nov., Morganellaceae fam. nov., and Budviciaceae fam. Nov. Int. J. Syst. Evol. Microbiol. 66, 5575–5599. doi: 10.1099/ijsem.0.001485
Alamuri, P., Eaton, K. A., Himpsl, S. D., Smith, S. N., Mobley, H. L. T. (2009). Vaccination with Proteus toxic agglutinin, a hemolysin-independent cytotoxin In vivo, protects against Proteus mirabilis urinary tract infection. Infect. Immun. 77, 632–641. doi: 10.1128/IAI.01050-08
Alamuri, P., Mobley, H. L. T. (2008). A novel autotransporter of uropathogenic Proteus mirabilis is both a cytotoxin and an agglutinin. Mol. Microbiol. 68, 997–1017. doi: 10.1111/j.1365-2958.2008.06199.x
Al-Jumaily, E. F., Mohammed, M. S., Yaseen, N. Y. (2012). In vitro cytotoxic study for pure extracellular toxin complex from Klebsiella pneumoniae K8. IOSR J. Pharm. 2, 08–13. doi: 10.9790/3013-2650813
Armbruster, C. E., Mobley, H. L. (2012). Merging mythology and morphology: The multifaceted lifestyle of Proteus mirabilis. Nat. Rev. Microbiol. 10, 743–754. doi: 10.1038/nrmicro2890
Armbruster, C. E., Mobley, H. L. T., Pearson, M. M. (2018). Pathogenesis of Proteus mirabilis infection. EcoSal Plus 8, 1. doi: 10.1128/ecosalplus.ESP-0009-2017
Armbruster, C. E., Smith, S. N., Johnson, A. O., DeOrnellas, V., Eaton, K. A., Yep, A., et al. (2017). The pathogenic potential of Proteus mirabilis is enhanced by other uropathogens during polymicrobial urinary tract infection. Infect. Immun. 85, e00808–e00816. doi: 10.1128/IAI.00808-16
Assoni, L., Girardello, R., Converso, T. R., Darrieux, M. (2021). Current stage in the development of Klebsiella pneumoniae vaccines. Infect. Dis. Ther. 10 (4), 2157–2175. doi: 10.1007/s40121-021-00533-4
Athamna, A., Ofek, I., Keisari, Y., Markowitz, S., Dutton, G. G., Sharon, N. (1991). Lectinophagocytosis of encapsulated Klebsiella pneumonia mediated by surface lectins of Guinea pig alveolar macrophages and human monocyte-derived macrophages. Infect. Immun. 59, 1673–1682. doi: 10.1128/iai.59.5.1673-1682.1991
Beynon, L. M., Dumanski, A. J., McLean, R. J. C., MacLean, L. L., Richards, J. C., Perry, M. B. (1992). Capsule structure of Proteus mirabilis (ATCC 49565). J. Bacteriol. 174, 2172–2177. doi: 10.1128/jb.174.7.2172-2177.1992
Bichler, K. H., Eipper, E., Naber, K., Braun, V., Zimmermann, R., Lahme, S. (2002). Urinary infection stones. Int. J. Antimicrob. Agents. 19, 488–498. doi: 10.1016/s0924-8579(02)00088-2
Boll, M., Radziejewska-Lebrecht, J., Warth, C., Krajewska-Pietrasik, D., Mayer, H. (1994). 4-Amino-4-Deoxy-L-Arabinose in LPS of enterobacterial r mutants and its possible role for their polymyxin reactivity. FEMS Immunol. Med. Microbiol. 8, 329–341. doi: 10.1111/j.1574-695X.1994.tb00460.x
Brisse, S., Grimont, F., Grimont, P. A. D. (2006). “The genus Klebsiella,” in The prokaryotes. Eds. Dworkin, M., Falkow, S., Rosenberg, E., Schleifer, K.-H., Stackebrandt, E. (New York: Springer), 159–196.
Broomfield, R. J., Morgan, S. D., Khan, A., Stickler, D. J. (2009). Crystalline bacterial biofilm formation on urinary catheters by urease-producing urinary tract pathogens: A simple method of control. J. Med. Microbiol. 58, 1367–1375. doi: 10.1099/jmm.0.012419-0
Campbell, W. N., Hendrix, E., Cryz, S., Jr, Cross, A. S. (1996). Immunogenicity of a 24-valent Klebsiella capsular polysaccharide vaccine and an eight-valent Pseudomonas O-polysaccharide conjugate vaccine administered to victims of acute trauma. Clin. Infect. Dis. 23 (1), 179–181. doi: 10.1093/clinids/23.1.179
Chen, C. Y., Chen, Y. H., Lu, P. L., Lin, W. R., Chen, T. C., Lin, C. Y. (2012). Proteus mirabilis urinary tract infection and bacteremia: Risk factors, clinical presentation, and outcomes. J. Microbiol. Immunol. Infect. 45, 228–236. doi: 10.1016/j.jmii.2011.11.007
Coban, B., Ülkü, N., Kaplan, H., Topal, B., Erdogan, H., Baskin, E. (2014). Five-year assessment of causative agents and antibiotic resistances in urinary tract infections. Turk. Pediatri. Ars. 49, 124–129. doi: 10.5152/tpa.2014.1505
Coker, C., Poore, C. A., Li, X., Mobley, H. L. T. (2000). Pathogenesis of Proteus mirabilis urinary tract infection. Microbes Infection 2, 1497–1505. doi: 10.1016/s1286-4579(00)01304-6
Cryz, S. J., Jr, Fürer, E., Germanier, R. (1984). Experimental Klebsiella pneumoniae burn wound sepsis: Role of capsular polysaccharide. Infect. Immun. 43, 440–441. doi: 10.1128/iai.43.1.440-441
Cuevas, O., Cercenado, E., Gimeno, M., Marín, M., Coronel, P., Bouza, E. (2010). Comparative In vitro activity of cefditoren and other antimicrobials against Enterobacteriaceae causing community-acquired uncomplicated urinary tract infections in women: A Spanish nationwide multicenter study. Diagnostic. Microbiol. Infect. Dis. 67, 251–260. doi: 10.1016/j.diagmicrobio.2010.02.013
Dai, H., Chen, A., Wang, Y., Lu, B., Wang, Y., Chen, J., et al. (2019). Proteus faecis sp. nov., and Proteus cibi sp. nov., two newspecies isolated from food and clinical samples in China. Int. J. Syst. Evol. Microbiol. 69, 852–858. doi: 10.1099/ijsem.0.003248
Dhingra, K. R. (2008). A case of complicated urinary tract infection: Klebsiella pneumoniae emphysematous cystitis presenting as abdominal pain in the emergency departament. West. J. Emerg. Med. 9, 171–173.
Di Martino, P., Cafferini, N., Joly, B., Darfeuille-Michaud, A. (2003). Klebsiella pneumoniae type 3 pili facilitate adherence and biofilm formation on abiotic surfaces. Res. Microbiol. 154, 9–16. doi: 10.1016/s0923-2508(02)00004-9
Drancourt, M., Bollet, C., Carta, A., Rousselier, P. (2001). Phylogenetic analyses of Klebsiella species delineate Klebsiella and Raoultella gen. nov., with description of Raoultella ornithinolytica comb. nov., Raoultella terrigena comb. nov. and Raoultella planticola comb. Nov. Int. J. Syst. Evol. Microbiol. 51 (3), 925–932. doi: 10.1099/00207713-51-3-925
Drzewiecka, D. (2016). Significance and roles of Proteus spp. bacteria in natural environments. Microb. Ecol. 72 (4), 741–758. doi: 10.1007/s00248-015-0720-6
Drzewiecka, D., Arbatsky, N. P., Kondakova, A. N., Shashkov, A. S., Knirel, Y. A. (2016). Structures and serospecificity of threonine-containing O polysaccharides of two clinical isolates belonging to the genus Proteus and their classification into O11 subserogroups. J. Med. Microbiol. 65, 1260–1266. doi: 10.1099/jmm.0.000360
Drzewiecka, D., Lewandowska, G. (2016a). Vaccines in prophylaxis of urinary tract infections caused by the bacteria from the genus Proteus. Postepy. Hig. Med. Dosw. 70, 1032–1043. doi: 10.5604/01.3001.0009.6882
Drzewiecka, D., Palusiak, A., Siwińska, M., Zabłotni, A. (2021). The prevailing O serogroups among the serologically differentiated clinical Proteus spp. strains in central Poland. Sci. Rep. 11, 18982. doi: 10.1038/s41598-021-98228-w
Drzewiecka, D., Shashkov, A. S., Arbatsky, N. P., Knirel, Y. A. (2016b). Immunochemical characterization of the O antigens of two Proteus strains, O8-related antigen of Proteus mirabilis 12 b-r and O2-related antigen of Proteus genomospecies 5/6 12 b-K, infecting a hospitalized patient in Poland. Microbiol. 162, 789–797. doi: 10.1099/mic.0.000274
Dutton, G. G. S., Mackie, K. L., Mo-Tai, Y. (1978). Structural investigation of Klebsiella serotype K18 polyssacharide. Carbohydr. Res. 65, 251–263. doi: 10.1016/S0008-6215(00)84317-7
Ebringer, A., Cunningham, P., Ahmadi, K., Wrigglesworth, J., Hosseini, R., Wilson, C. (1992). Sequence similarity between HLA-DR1 and DR4 subtypes associated with rheumatoid arthritis and Proteus/Serratia membrane haemolysins. Ann. Rheumatol. Dis. 51, 1245–1246. doi: 10.1136/ard.51.11.1245
Ebringer, A., Rashid, T., Wilson, C. (2003). Rheumatoid arthritis: Proposal for the use of anti-microbial therapy in early cases. Scand. J. Rheumatol. 32, 2–11. doi: 10.1080/03009740310000337
Ebringer, A., Rashid, T., Wilson, C., Ptaszynska, T., Fielder, M. (2006). Ankylosing spondylitis as an auto-immune disease linked to intestinal Klebsiella infection: Prospects for a new therapeutic approach. Curr. Rheumatol. Rev. 2, 55–68. doi: 10.2174/157339706775697044
Endimiani, A., Luzzaro, F., Brigante, G., Perilli, M., Lombardi, G., Amicosante, G., et al. (2005). Proteus mirabilis bloodstream infections: Risk factors and treatment outcome related to the expression of extended-spectrum β-lactamases. Antimicrob. Agents. Chemother. 49, 2598–2605. doi: 10.1128/AAC.49.7.2598-2605.2005
Erbing, B., Jansson, P. E., Widmalm, G., Nimmich, W. (1995). Structure of the capsular polysaccharide from the Klebsiella K8 reference strain 1015. Carbohydr. Res. 273, 197–205. doi: 10.1016/0008-6215(95)00099-F
Fielder, M., Pirt, S. J., Tarpey, I., Wilson, C., Cunningham, P., Ettelaie, C., et al. (1995). Molecular mimicry and ankylosing spondylitis: Possible role of a novel sequence in pullulanase of Klebsiella pneumoniae. FEBS Lett. 369, 243–248. doi: 10.1016/0014-5793(95)00760-7
Fox-Moon, S. M., Shirtliff, M. E. (2015) “Urinary tract infections caused by Proteus mirabilis,” in Mol med microbiol, 2nd ed. (US: Academic Press). doi: 10.1016/B978-0-12-397169-2.00077-9
Galván, E. M., Mateyca, C., Ielpi, L. (2016). Role of interspecies interactions in dual-species biofilms developed In vitro by uropathogens isolated from polymicrobial urinary catheter-associated bacteriuria. Biofouling 32, 1067–1077. doi: 10.1080/08927014.2016.1231300
Gómez, M., Valverde, A., del Campo, R., Rodríguez, J. M., Maldonado-Barragán, A. (2021). Phenotypic and molecular characterization of commensal, community-acquired and nosocomial Klebsiella spp. Microorganisms. 9, 2344. doi: 10.3390/microorganisms9112344
Gong, Z., Shi, X., Bai, F., He, X., Zhang, H., Li, Y., et al. (2019). Characterization of a novel diarrheagenic strain of Proteus mirabilis associated with food poisoning in China. Front. Microbiol. 10. doi: 10.3389/fmicb.2019.02810
Hansen, D. S., Aucken, H. M., Abiola, T., Podschun, R. (2004). Recommended test panel for differentiation of Klebsiella species on the basis of a trilateral interlaboratory evaluation of 18 biochemical tests. J. Clin. Microbiol. 42, 3665–3669. doi: 10.1128/JCM.42.8.3665-3669.2004
Hansen, D. S., Mestre, F., Albertí, S., Hernández-Allés, S., Álvarez, D., Doménech-Sánchez, A., et al. (1999). Klebsiella pneumoniae lipopolysaccharide O typing: Revision of prototype strains and O-group distribution among clinical isolates from different sources and countries. J. Clin. Microbiol. 37, 56–62. doi: 10.1128/JCM.37.1.56-62.1999
Helander, I. M., Kato, Y., Kilpeläinen, I., Kostiainen, R., Lindner, B., Nummila, K., et al. (1996). Characterization of lipopolysaccharides of polymyxin-resistant and polymyxin-sensitive Klebsiella pneumoniae O3. Eur. J. Biochem. 237, 272–278. doi: 10.1111/j.1432-1033.1996.0272n.x
Holst, O. (2007). The structures of core regions from enterobacterial lipopolysaccharides-an update. FEMS Microbiol. Lett. 271, 3–11. doi: 10.1111/j.1574-6968.2007.00708.x
Hooton, T. M. (2001). Recurrent urinary tract infection in women. Int. J. Antimicrob. Agents 17, 259–268. doi: 10.1016/s0924-8579(00)00350-2
Hopkins, W. J., Elkahwaji, J., Beierle, L. M., Leverson, G. E., Uehling, D. T. (2007). Vaginal mucosal vaccine for recurrent urinary tract infections in women: Results of a phase 2 clinical trial. J. Urol. 177 (4), 1349–1353. doi: 10.1016/j.juro.2006.11.093
Island, M. D., Mobley, H. L. T. (1995). Proteus mirabilis urease: Operon fusion and linker insertion analysis of ure gene organization, regulation, and function. J. Bacteriol. 177 (9), 5653–5660. doi: 10.1128/jb.177.19.5653-5660.1995
Jacobsen, S. M., Stickler, D. J., Mobley, H. L. T., Shirtliff, M. E. (2008). Complicated catheter-associated urinary tract infections due to Escherichia coli and Proteus mirabilis. Clin. Microbiol. Rev. 21, 26–59:5653–60. doi: 10.1128/CMR.00019-07
Jansen, A. M., Lockatell, V., Johnson, D. E., Mobley, H. L. T. (2004). Mannose-resistant Proteus-Like fimbriae are produced by most Proteus mirabilis strains infecting the urinary tract, dictate the In vivo localization of bacteria, and contribute to biofilm formation. Infect. Immun. 72, 7294–7305. doi: 10.1128/IAI.72.12.7294–7305.2004
Jansson, P. E., Lindberg, B., Manca, M. C., Nimmich, W., Widmalm, G. (1994). Structural studies of the capsular polysaccharide from Klebsiella type 38: A reinvestigation. Carbohydr. Res. 261, 111–118. doi: 10.1016/0008-6215(94)80010-3
Joseleau, J. P., Lapeyre, M., Vignon, M. (1978). Chemical and N.M.R.-spectroscopic investigation of the capsular polysaccharide of Klebsiella serotype K41. Carbohydr. Res. 67, 197–212. doi: 10.1016/S0008-6215(00)83742-8
Joseleau, J. P., Marais, M. F. (1979). Structure of the capsular polysaccharide of Klebsiella K-type 63. Carbohydr. Res. 77, 183–190. doi: 10.1016/s0008-6215(00)83804-5
Joseleau, J. P., Marais, M. F. (1988). The structural repeating-unit of the capsular polysaccharide from klebsiella serotype K48. Carbohydr. Res. 179, 321–326. doi: 10.1016/0008-6215(88)84128-4
Kaca, W., Glenska, J., Lechowicz, L., Grabowski, S., Brauner, A., Kwinkowski, M. (2011). Serotyping of Proteus mirabilis clinical strains based on lipopolysaccharide O-polysaccharide and core oligosaccharide structures. Biochem. (Moscow) 76, 851–861. doi: 10.1134/S0006297911070169
Kallel, H., Houcke, S., Resiere, D., Roy, M., Mayence, C., Mathien, C., et al. (2020). Epidemiology and prognosis of intensive care unit–acquired bloodstream infection am. J. Trop. Med. Hyg. 103 (1), 508–514. doi: 10.4269/ajtmh.19-0877
Kauffmann, F. (1966). The bacteriology of enterobacteriaceae (Baltimore: The Williams & Wilkins Co), 333–360.
Knirel, Y. A. (2011). “Structure of O-antigens,” in Bacterial lipopolysaccharides. Eds. Knirel, Y. A., Valvano, M. A. (Vienna: Springer), 41–115.
Knirel, Y. A., Perepelov, A. V., Kondakova, A. N., Senchenkova, S. N., Sidorczyk, Z., Rozalski, A., et al. (2011). Structure and serology of O-antigens as the basis for classification of Proteus strains. Innate. Immun. 17, 70–96. doi: 10.1177/1753425909360668
Kondakova, A. N., Vinogradov, E. V., Knirel, Y. A., Lindner, B. (2005). Application of electrospray ionization with Fourier transform ion cyclotron resonance mass spectrometry for structural screening of core oligosaccharides from lipopolysaccharides of the bacteria Proteus. Rapid. Commun. Mass. Spectrom 19, 2343–2349. doi: 10.1002/rcm.2064
Konieczna, I., Żarnowiec, P., Kwinkowski, M., Kolesińska, B., Frączyk., J., Kamiński, Z., et al. (2012). Bacterial urease and its role in long-lasting human diseases. Curr. Protein Pept. Sci. 13, 789–806. doi: 10.2174/138920312804871094
Ko, W. C., Paterson, D. L., Sagnimeni, A. J., Hansen, D. S., Von Gottberg, A., Mohapatra, S., et al. (2002). Community-acquired Klebsiella pneumoniae bacteremia: Global differences in clinical patterns. Emerg. Infect. Dis. 8, 160–166. doi: 10.3201/eid0802.010025
Kotełko, K. (1986). Proteus mirabilis: Taxonomic position, peculiarities of growth, components of the cell envelope. Curr. Top. Microbiol. Immunol. 129, 181–215. doi: 10.1007/978-3-642-71399-6_3
Krajden, S., Fuksa, M., Petrea, C., Crisp, L. J., Penner, J. L. (1987). Expanded clinical spectrum of infections caused by Proteus penneri. J. Clin. Microbiol. 25, 578–579. doi: 10.1128/jcm.25.3.578-579.1987
Kubler-Kielb, J., Vinogradov, E., Ng, W. I., Maczynska, B., Junka, A., Bartoszewicz, M., et al. (2013). The capsular polysaccharide and lipopolysaccharide structures of two carbapenem resistant Klebsiella pneumoniae outbreak isolates. Carbohydr. Res. 369, 6–9. doi: 10.1016/j.carres.2012.12.018
Langstraat, J., Bohse, M., Clegg, S. (2001). Type 3 fimbrial shaft (MrkA) of Klebsiella pneumoniae, but not the fimbrial adhesin (MrkD), facilitates biofilm formation. Infect. Immun. 69, 5805–5812. doi: 10.1128/IAI.69.9.5805–5812.2001
Larsson, P. (1984). “Serology of Proteus mirabilis and Proteus vulgaris,” in Methods microbiol, vol. 14 . Ed. Bergan, T. (London: Academic Press), 187–214. doi: 10.1016/S0580-9517(08)70451-3
Larsson, P., Andersson, H. E., Norlen, B. (1978). Serotyping in epidemiological tracing of nosocomially acquired Proteus mirabilis in a geriatric ward. Infection 6, 105–110. doi: 10.1007/BF01642257
Launay, O., Lewis, D. J. M., Anemona, A., Loulergue, P., Leahy, J., Sciré, A. S., et al. (2017). Safety profile and immunologic responses of a novel vaccine against Shigella sonnei administered intramuscularly, intradermally and intranasally: Results from two parallel randomized phase 1 clinical studies in healthy adult volunteers in Europe. EBioMedicine 22, 164–172. doi: 10.1016/j.ebiom.2017.07.013
Laupland, K. B., Ross, T., Pitout, J. D., Church, D. L., Gregson, D. B. (2007). Community-onset urinary tract infections: A population-based assessment. Infection 35, 150–1153. doi: 10.1007/s15010-007-6180-2
Lavender, H., Jagnow, J. J., Clegg, S. (2005). Klebsiella pneumoniae type 3 fimbria-mediated immunity to infection in the murine model of respiratory disease. Int. J. Med. Microbiol. 295 (3), 153–159. doi: 10.1016/j.ijmm.2005.04.001
Leal, H. L., Azevedo, J., Silva, G. E. O., Amorim, A. M. L., de Roma, L. R. C., Arraes, A. C. P., et al. (2019). Bloodstream infections caused by multidrug-resistant gram-negative bacteria: Epidemiological, clinical and microbiological features. BMC Infect. Dis. 19, 609. doi: 10.1186/s12879-019-4265-z
Li, X., Lockatell, C. V., Johnson, D. E., Lane, M. C., Warren, J. W., Mobley, H. L. T. (2004). Development of an intranasal vaccine to prevent urinary tract infection by Proteus mirabilis. Infect. Immun. 72, 66–75. doi: 10.1128/IAI.72.1.66-75.2004
Lindberg, B., Lönngren, J., Rudén, U. (1975). Structural studies of the capsular polysaccharide of Klebsiella type 59. Carbohydr. Res. 42, 83–93. doi: 10.1016/s0008-6215(00)84102-6
Linhares, I., Raposo, T., Rodrigues, A., Almeida, A. (2013). Frequency and antimicrobial resistance patterns of bacteria implicated in community urinary tract infections: A ten-year surveillance study (2000-2009). BMC Infect. Dis. 13, 19. doi: 10.1186/1471-2334-13-19
Lin, Y. T., Jeng, Y. Y., Chen, T. L., Fung, C. P. (2010). Bacteremic community-acquired pneumonia due to Klebsiella pneumoniae: Clinical and microbiological characteristics in Taiwan, 2001-2008. BMC Infect. Dis. 10, 307. doi: 10.1186/1471-2334-10-307
Lin, T.-L., Yang, F.-L., Ren, C.-T., Pan, Y.-J., Liao, K.-S., Tu, I.-F., et al. (2022). Development of Klebsiella pneumoniae capsule polysaccharide-conjugated vaccine candidates using phage depolymerases. Front. Immunol. 13. doi: 10.3389/fimmu.2022.843183
Lu., B., Zhou, H., Zhang, X., Qu, M., Huang, Y., Lu, Q. W. (2017). Molecular characterization of Klebsiella pneumoniae isolates from stool specimens of outpatients in sentinel hospitals Beijing, China, 2010–2015. Gut Pathog. 9, 39. doi: 10.1186/s13099-017-0188-7
Macleod, S. M., Stickler, D. J. (2007). Species interactions in mixed-community crystalline biofilms on urinary catheters. J. Med. Microbiol. 56, 1549–1557. doi: 10.1099/jmm.0.47395-0
Magill, S. S., Edwards, J. R., Bamberg, W., Beldavs, Z. G., Dumyati, G., Kainer, M. A., et al. (2014). Multistate point-prevalence survey of health care–associated infections. N. Engl. J. Med. 370, 1198–1208. doi: 10.1056/NEJMoa1306801
Maroncle, N., Rich, C., Forestier, C. (2006). The role of Klebsiella pneumoniae urease in intestinal colonization and resistance to gastrointestinal stress. Res. Microbiol. 157, 184–193. doi: 10.1016/j.resmic.2005.06.006
Martin, R. M., Bachman, M. A. (2018). Colonization, infection, and the accessory genome of Klebsiella pneumoniae. Front. Cell. Infect. Microbiol. 8. doi: 10.3389/fcimb.2018.00004
Mobley, H. L. T., Island, M. D., Hausinger, R. P. (1995). Molecular biology of microbial ureases. Microbiol. Rev. 59, 451–480. doi: 10.1128/mr.59.3.451-480.1995
Mobley, H. L. T., Warren, J. W. (1987). Urease-positive bacteriuria and obstruction of long-term urinary catheters. J. Clin. Microbiol. 25 (11), 2216–2217. doi: 10.1128/jcm.25.11.2216-2217.1987
Musicha, P., Cornick, J. E., Bar-Zeev, N., French, N., Masesa, C., Denis, B., et al. (2017). Trends in antimicrobial resistance in bloodstream infection isolates at a Large urban hospital in Malawi (1998–2016): A surveillance study. Lancet Infect. Dis. 17, 1042–1052. doi: 10.1016/S1473-3099(17)30394-8
Nagiah, S., Badbess, R. (2018). Unexpected source of Proteus mirabilis bacteraemia. BMJ Case Rep. 11, e226744. doi: 10.1136/bcr-2018-226744
Nenkov, P. (2000). Overview on the clinical studies with urostim immunostimulator against urogenital infections. Adv. Exp. Med. Biol. 485, 325–329. doi: 10.1007/0-306-46840-9_44
O’Hara, C. M., Brenner, F. W., Miller, J. M. (2000). Classification, identification, and clinical significance of Proteus, Providencia, and Morganella. Clin. Microbiol. Rev. 13, 534–546. doi: 10.1128/CMR.13.4.534
Okimoto, N., Hayashi, T., Ishiga, M., Nanba, F., Kishimoto, M., Yagi, S., et al. (2010). Clinical features of Proteus mirabilis pneumonia. J. Infect. Chemother. 16, 364–366. doi: 10.1007/s10156-010-0059-3
Oloomi, M., Javadi, M., Karam, M. R. A., Khezerloo, J. K., Haghri, Z., Bouzari, S. (2020). Protective multi-epitope candidate vaccine for urinary tract infection. Biotechnol. Rep. 28, e00564. doi: 10.1016/j.btre.2020.e00564
Ørskov, I. (1954). O Antigens in the Klebsiella group. Acta Pathol. Microbiol. Scand. 34, 145–156. doi: 10.1111/j.1699-0463.1954.tb00811.x
Paczosa, M. K., Mecsas, J. (2016). Klebsiella pneumoniae: Going on the offense with a strong defense. Microbiol. Mol. Biol. Rev. 80, 629–661. doi: 10.1128/MMBR.00078-15
Palusiak, A. (2013). Immunochemical properties of Proteus penneri lipopolysaccharides – one of the major Proteus sp. virulence factors. Carbohydr. Res. 380, 16–22. doi: 10.1016/j.carres.2013.06.025
Palusiak, A. (2015). The antigens contributing to the serological cross-reactions of Proteus antisera with Klebsiella representatives. Mol. Immunol. 64, 228–234. doi: 10.1016/j.molimm.2014.11.016
Palusiak, A. (2021). The contribution of polysaccharide antigens from clinical Proteus spp. and Klebsiella spp. isolates to the serological cross-reactions. Front. Cell. Infect. Microbiol. 11. doi: 10.3389/fcimb.2021.707578
Palusiak, A., Siwińska, M., Sidorczyk, Z. (2013). Serological studies of Proteus penneri determining qualification to appropriate O-serogroup. Polish. J. Microbiol. 62, 211–216. doi: 10.33073/pjm-2013-028
Patro, L. P. P., Rathinavelan, T. (2019). Targeting the sugary armor of Klebsiella species. Front. Cell. Infect. Microbiol. 9. doi: 10.3389/fcimb.2019.00367
Pellegrino, R., Galvalisi, U., Scavone, P., Sosa, V., Zunino, P. (2003). Evaluation of Proteus mirabilis structural fimbrial proteins as antigens against urinary tract infections. FEMS Immunol. Med. Microbiol. 36, 103–110. doi: 10.1016/S0928-8244(03)00103-2
Penner, J. L., Hennessy, J. N. (1980). Separate O-grouping schemes for serotyping clinical isolates of Proteus vulgaris and Proteus mirabilis. J. Clin. Microbiol. 12, 304–309. doi: 10.1128/jcm.12.3.304-309.1980
Perry, M. B., MacLean, L. L. (1994). The structure of the polysaccharide produced by Proteus vulgaris (ATCC 49990). Carbohydr. Res. 253, 257–263. doi: 10.1016/0008-6215(94)80070-7
Podschun, R., Pietsch, S., Höller, C., Ullmann, U. (2001). Incidence of Klebsiella species in surface waters and their expression of virulence factors. Appl. Environ. Microbiol. 67, 3325–3327. doi: 10.1128/AEM.67.7.3325-3327.2001
Podschun, R., Ullmann, U. (1998). Klebsiella spp. as nosocomial pathogens: Epidemiology, taxonomy, typing methods, and pathogenicity factors. Clin. Microbiol. Rev. 11, 589–603. doi: 10.1128/CMR.11.4.589
Poxton, I. R. (1995). Antibodies to lipopolysaccharide. J. Immunol. Methods 186, 1–15. doi: 10.1016/0022-1759(95)00123-R
Rahman, M. M., Guard-Petter, J., Asokan, K., Carlson, R. W. (1997). The structure of the capsular polysaccharide from a swarming strain of pathogenic Proteus vulgaris. Carbohydr. Res. 301, 213–220. doi: 10.1016/S0008-6215(97)00093-1
Rahman, M. M., Guard-Petter, J., Asokan, K., Hughes, C., Carlson, R. W. (1999). The structure of the colony migration factor from pathogenic Proteus mirabilis. a capsular polysaccharide that facilitates swarming. J. Biol. Chem. 274, 22993–22998. doi: 10.1074/jbc.274.33.22993
Rashid, T., Ebringer, A. (2007a). Rheumatoid arthritis is linked to Proteus - the evidence. Clin. Rheumatol. 26, 1036–1043. doi: 10.1007/s10067-006-0491-z
Rashid, T., Ebringer, A. (2007b). Ankylosing spondylitis is linked to Klebsiella - the evidence. Clin. Rheumatol. 26, 858–864. doi: 10.1007/s10067-006-0488-7
Regué, M., Izquierdo, L., Fresno, S., Piqué, N., Corsaro, M. M., Naldi, T., et al. (2005). A second outer-core region in Klebsiella pneumoniae lipopolysaccharide. J. Bacteriol. 187, 4198–4206. doi: 10.1128/JB.187.12.4198-4206.2005
Różalski, A., Torzewska, A., Moryl, M., Kwil, I., Maszewska, A., Ostrowska, K., et al. (2012). Proteus sp. – an opportunistic bacterial pathogen – classification, swarming growth, clinical significance and virulence factors. Folia Biologica Oecologica. 8, 1–17. doi: 10.2478/fobio-2013-0001
Sękowska, A., Gospodarek, E. (2007). Czynniki wirulencji I zakażenia wywoływane przez pałeczki Klebsiella spp. Med. Biol. Sci. 21, 23–29.
Schaffer, J. N., Pearson, M. M. (2015). And urinary tract infections. Microbiol. Spectr. 3, 5. doi: 10.1128/microbiolspec.UTI-0017-2013
Schembri, M. A., Blom, J., Krogfelt, K. A., Klemm, P. (2005). Capsule and fimbria interaction in Klebsiella pneumoniae. Infect. Immune. 73, 4626–4633. doi: 10.1128/IAI.73.8.4626–4633
Schroll, C., Barken, K. B., Krogfelt, K. A., Struve, C. (2010). Role of type 1 and type 3 fimbriae in Klebsiella pneumoniae biofilm formation. BMC Microbiol. 10, 179. doi: 10.1186/1471-2180-10-17
Schwimmbeck, P. L., Yu, D. T. Y., Oldstone, M. B. A. (1987). Autoantibodies to HLA-B27 in the sera of HLA-B27 patients with ankylosing spondylitis and reiter’s syndrome: Molecular mimicry with Klebsiella pneumoniae as potential mechanism of autoimmune disease. J. Exp. Med. 166, 173–181. doi: 10.1084/jem.166.1.173
Shon, A. S., Bajwa, R. P. S., Russo, T. A. (2013). Hypervirulent (Hypermucoviscous) Klebsiella pneumonia. a new and dangerous breed. Virulence 4, 107–118. doi: 10.4161/viru.2271810.4161/viru.22718
Sidorczyk, Z., Zähringer, U., Rietschel, E. T. (1983). Chemical structure of the lipid a component of the lipopolysaccharide from a Proteus mirabilis re mutant. Eur. J. Biochem. 137, 15–22. doi: 10.1111/j.1432-1033.1983.tb07789.x
Stickler, D., Morris, N., Moreno, M.-C., Sabbuba, N. (1998). Studies on the formation of crystalline bacterial biofilms on urethral catheters. Eur. J. Clin. Microbiol. Infect. Dis. 17, 649–652. doi: 10.1007/BF01708349
Tajbakhsh, E., Tajbakhsh, S., Khamesipour, F. (2015). Isolation and molecular detection of gram-negative bacteria causing urinary tract infection in patients referred to shahrekord hospitals, Iran. Iran. Red. Crescent Med. J. 17, 24779. doi: 10.5812/ircmj.17(5)2015.24779
Torzewska, A., Budzyńska, A., Białczak-Kokot, M., Różalski, A. (2014). In vitro studies of epithelium-associated crystallization caused by uropathogens during urinary calculi development. Microb. Pathog. 71-72, 25–31. doi: 10.1016/j.micpath.2014.04.007
Torzewska, A., Stączek, P., Różalski, A. (2003). Crystallization of urine mineral components may depend on the chemical nature of Proteus endotoxin polysaccharides. J. Med. Microbiol. 52, 471–477. doi: 10.1099/jmm.0.05161-0
Trautman, M., Held, T. K., Cross, A. S. (2004). O Antigen seroepidemiology of Klebsiella clinical isolates and implications for immunoprophylaxis of Klebsiella infections. Vaccine 22, 818–821. doi: 10.1128/cdli.4.5.550-555.1997
Trautmann, M., Ruhnke, M., Rukavina, T., Held, T. K., Cross, A. S., Marre, R., et al. (1997). O-Antigen seroepidemiology of Klebsiella clinical isolates and implications for immunoprophylaxis of Klebsiella infections. Clin. Diagn. Lab. Immunol. 4, 550–555. doi: 10.1128/cdli.4.5.550-555.1997
Uehling, D. T., Hopkins, W. J., Elkahwaji, J. E., Schmidt, D. M., Leverson, G. E. (2003). Phase 2 clinical trial of a vaginal mucosal vaccine for urinary tract infections. J. Urol. 170 (3), 867–869. doi: 10.1097/01.ju.0000075094.54767.6e
Vinogradov, E. (2011). Structure of the core part of the lipopolysaccharide from Proteus mirabilis genomic strain HI4320. Biochem. (Moscow) 76, 803–807. doi: 10.1134/S000629791107011X``3
Vinogradov, E., Frirdich, E., MacLean, L. L., Perry, M. B., Petersen, B. O., Duus, J.Ø., et al. (2002). Structures of lipopolysaccharides from Klebsiella pneumoniae. elucidation of the structure of the linkage region between core and polysaccharide O chain and identification of the residues at the non-reducing termini of the O chains. J. Biol. Chem. 277, 25070–25081. doi: 10.1074/jbc.M202683200
Vinogradov, E. V., Perry, M. B. (2001). Structural analysis of the core region of the lipopolysaccharides from eight serotypes of Klebsiella pneumoniae. Carbohydr. Res. 335, 291–296. doi: 10.1016/S0008-6215(01)00216-6
Vinogradov, E., Radziejewska-Lebrecht, J., Kaca, W. (2000). The structure of the carbohydrate backbone of core-lipid a region of the lipopolysaccharides from Proteus mirabilis wild-type strain S1959 (Serotype O3) and its Ra mutant R110/1959. Eur. J. Biochem. 267, 262–268. doi: 10.1046/j.1432-1327.2000.01001.x
Vinogradov, E., Sidorczyk, Z., Knirel, Y. A. (2002). Structure of the lipopolysaccharide core region of the bacteria of the genus Proteus. Aust. J. Chem. 55, 61–67. doi: 10.1071/CH01184
Vinogradov, E. V., Thomas-Oates, J. E., Brade, H., Holst., O. (1994). Structural investigation of the lipopolysaccharide from Proteus mirabilis R45 (Re-chemotype). J. Endotoxin. Res. 1, 199–206. doi: 10.1177/096805199400100401
Vuye, A., Pijck, J. (1973). Urease activity of Enterobacteriaceae: Which medium to choose. Appl. Microbiol. 26 (6), 850–854. doi: 10.1128/am.26.6.850-854.1973
Wang, Q., Chang, C. S., Pennini, M., Pelletier, M., Rajan, S., Zha, J., et al. (2016). Target-agnostic identification of functional monoclonal antibodies against Klebsiella pneumoniae multimeric MrkA fimbrial subunit. J. Infect. Dis. 213 (11), 1800–1808. doi: 10.1093/infdis/jiw0.21
Watanakunakorn, C. (1991). Klebsiella bacteremia: A review of 196 episodes during a decade (1980–1989). Scand. J. Infect. Dis. 23, 399–405. doi: 10.3109/00365549109075086
Whitfield, C., Perry, M. B., MacLean, L. L., Yu, S. H. (1992). Structural analysis of the O-antigen side chain polysaccharides in the lipopolysaccharides of Klebsiella serotypes O2(2a), O2(2a,2b), and O2(2a,2c). J. Bacteriol. 174, 4913–4919. doi: 10.1128/jb.174.15.4913-4919.1992
Wilson, C., Ebringer, A., Ahmadi, K., Wrigglesworth, J., Tiwana, H., Fielder, M., et al. (1995). Shared amino acid sequences between major histocompatibility complex class II glycoproteins, type XI collagen and Proteus mirabilis in rheumatoid arthritis. Ann. Rheumatol. Dis. 54, 216–220. doi: 10.1136/ard.54.3.216
Wu, M., Li, X. (2015) “Klebsiella pneumoniae and pseudomonas aeruginosa,” in Mol med microbiol. Eds. Tang, Y. W., Sussman, M., Liu, D., Poxton, I., Schwartzman, J.(USA), 1547–1564. doi: 10.1016/B978-0-12-397169-2.00087-1
Yang, B., Foley, S. (2018). First experience in the UK of treating women with recurrent urinary tract infections with the bacterial vaccine Uromune(R). BJU Int. 121 (2), 289–292. doi: 10.1111/bju.14067
Keywords: core oligosaccharide, Klebsiella pneumoniae, lipopolysaccharide, Proteus mirabilis, virulence factors
Citation: Palusiak A (2022) Proteus mirabilis and Klebsiella pneumoniae as pathogens capable of causing co-infections and exhibiting similarities in their virulence factors. Front. Cell. Infect. Microbiol. 12:991657. doi: 10.3389/fcimb.2022.991657
Received: 11 July 2022; Accepted: 03 October 2022;
Published: 20 October 2022.
Edited by:
Charles Martin Dozois, Université du Québec, CanadaReviewed by:
Jorge Alberto Giron, University of Puebla, MexicoMark T. Anderson, Michigan Medicine, University of Michigan, United States
Copyright © 2022 Palusiak. This is an open-access article distributed under the terms of the Creative Commons Attribution License (CC BY). The use, distribution or reproduction in other forums is permitted, provided the original author(s) and the copyright owner(s) are credited and that the original publication in this journal is cited, in accordance with accepted academic practice. No use, distribution or reproduction is permitted which does not comply with these terms.
*Correspondence: Agata Palusiak, YWdhdGEucGFsdXNpYWtAYmlvbC51bmkubG9kei5wbA==