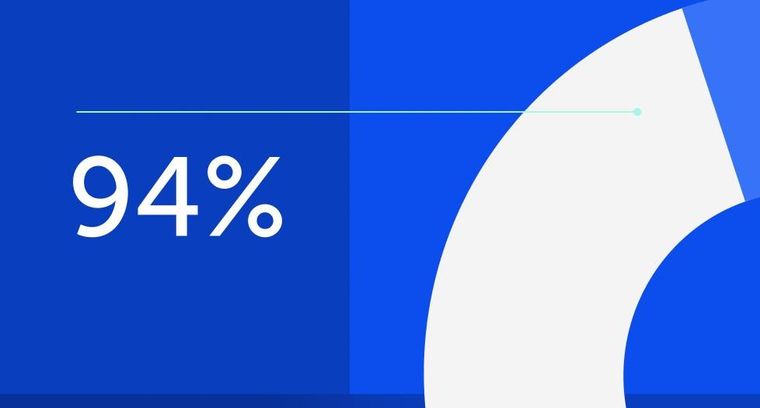
94% of researchers rate our articles as excellent or good
Learn more about the work of our research integrity team to safeguard the quality of each article we publish.
Find out more
BRIEF RESEARCH REPORT article
Front. Cell. Infect. Microbiol., 23 September 2022
Sec. Fungal Pathogenesis
Volume 12 - 2022 | https://doi.org/10.3389/fcimb.2022.967486
This article is part of the Research TopicRising Stars in Fungal Pathogenesis : 2022View all 4 articles
Understanding of how intracellular pathogens survive in their host cells is important to improve management of their diseases. This has been fruitful for intracellular bacteria, but it is an understudied area in fungal pathogens. Here we start elucidating and characterizing the strategies used by one of the commonest fungal pathogens, Cryptococcus neoformans, to survive intracellularly. The ability of the fungus to survive inside host cells is one of the main drivers of disease progression, yet it is unclear whether C. neoformans resides in a fully acidified, partially acidic, or neutral phagosome. Using a dye that only fluoresce under acidic conditions to stain C. neoformans, a hypha-defective Candida albicans mutant, and the nonpathogenic Saccharomyces cerevisiae, we characterized the fungal behaviors in infected macrophages by live microscopy. The main behavior in the C. albicans mutant strain and S. cerevisiae-phagosomes was rapid acidification after internalization, which remained for the duration of the imaging. In contrast, a significant number of C. neoformans-phagosomes exhibited alternative behaviors distinct from the normal phagosomal maturation: some phagosomes acidified with subsequent loss of acidification, and other phagosomes never acidified. Moreover, the frequency of these behaviors was affected by the immune status of the host cell. We applied the same technique to a flow cytometry analysis and found that a substantial percentage of C. neoformans-phagosomes showed impaired acidification, whereas almost 100% of the S. cerevisiae-phagosomes acidify. Lastly, using a membrane-damage reporter, we show phagosome permeabilization correlates with acidification alterations, but it is not the only strategy that C. neoformans uses to manipulate phagosomal acidification. The different behaviors described here provide an explanation to the confounding literature regarding cryptococcal-phagosome acidification and the methods can be applied to study other intracellular fungal pathogens.
Cryptococcus neoformans is a major human pathogen with worldwide distribution (Srikanta et al., 2014). Because it is found ubiquitously in the environment, we frequently inhale it, establishing a lung infection that in the immunocompetent individual is controlled, but in the immunocompromised it disseminates with tropism for the central nervous system, causing lethal meningoencephalitis. This infection is one of the original AIDS-defining illnesses and still is a leading cause of death among HIV patients, responsible for close to 200,000 deaths yearly in this population (Rajasingham et al., 2017; Charalambous et al., 2018). Since it is acquired by inhalation, the first line of defense are the alveolar macrophages and other lung phagocytes, which are able to recognize and phagocytose the fungus, but cannot efficiently kill it. The interactions between these host cells and the fungi are critical for the disease and will influence events such as clearance, latency, and dissemination (Gaylord et al., 2020). One of the most important interactions driving disease establishment is Cryptococcus’s ability to survive inside immune cells such as macrophages.
Normally, when microbes are phagocytosed by macrophages, they end up inside a membranous compartment known as a phagosome. This phagosome follows a well-studied process of maturation that results in the formation of a phagolysosome for destruction of the microbe. A property of a fully functional phagolysosome is its low pH (4.0 – 4.5), conditions that not only are inhospitable to many organisms, but also result in activation of many proteases and other antimicrobial responses. Intracellular pathogens have evolved fascinating strategies to evade this process and survive in cells designed to eradicate them, and one of the most common strategies involve inhibition of phagosomal maturation (Vaughn and Abu Kwaik, 2021). Some pathogens, such as Listeria or Mycobacteria, escape from the phagosome before it acidifies; others, such as Salmonella or Legionella, remain in a modified phagosome that does not fuse with lysosomes. C. neoformans is known to reside in a phagosome, but it is unclear if this compartment is a fully-functional phagolysosome or an altered phagosome, and if this compartment acidifies normally is controversial. Some studies suggest it is an acidified lysosome and that acidification is important for fungal replication (Levitz et al., 1997; Levitz et al., 1999; Wozniak and Levitz, 2008). Other results suggest it never, or partially, acidifies (Smith et al., 2015; De Leon-Rodriguez et al., 2018; Fu et al., 2018; Dragotakes et al., 2020). Moreover, others have seen indirect evidence that C. neoformans damages the phagosomal membrane, which would affect its acidification because contents would be neutralized by the cytoplasm (Tucker and Casadevall, 2002; Davis et al., 2015; De Leon-Rodriguez et al., 2018). It seems likely that C. neoformans actively manipulates its phagosome to create a permissive intracellular niche; however, to date, no effector proteins directly responsible for such manipulation have been reported. Furthermore, different host immune polarization states have been associated with different infection outcomes (Leopold Wager et al., 2016; Rudman et al., 2019; Gaylord et al., 2020). M1, or pro-inflammatory, macrophages are associated with clearance or control of infection, whereas M2, or anti-inflammatory, macrophages are associated with progression and dissemination of the disease. Using a ratiometric method that measures the amount of a fluorescent dye released from lysosomes, it was shown that at long time points post-infection (>24 hr) there is increased lysosomal permeabilization in naïve (M0) macrophages relative to activated macrophages, with M1 macrophages showing the least lysosomal damage (Davis et al., 2015). These were, however, static images taken 24 hr apart from each other, which preclude analysis of the dynamics of this process.
Here we use a highly-specific pH-sensitive dye to label the fungus and follow, in real time, its internalization and maturation of the resulting phagosome. This method allows us to follow the fungus by time-lapse microscopy and directly visualize the pH of its phagosome without the need for wash steps, quencher dyes, or additional sequestered fluorescent molecules. Using this method with C. neoformans, a non-filamentous mutant of C. albicans, and the non-pathogenic yeast S. cerevisiae, we were able to identify and characterize three behaviors (1): phagosomes that acidify and stay acidic (2); phagosomes that acidify with subsequent neutralization; and (3) phagosomes that never acidified during the duration of the videos. The last two behaviors were significantly more represented in C. neoformans than in the other two fungi. These behaviors represented active manipulation of phagosomal acidification, and the kinetics and frequency were influenced by the activation state of the host macrophages. More of the S. cerevisiae and C. albicans’s phagosomes acidify faster in M1 macrophages relative to M0 and M2. However, that is not seen with C. neoformans and, instead, in M2 macrophages less phagosomes acidify, and it takes longer. Using a membrane damage reporter, we correlated the abnormal behaviors with phagosomal membrane permeabilization, and we show that this can occur rapidly after internalization. These data demonstrate that C. neoformans has the ability to efficiently and rapidly manipulate its phagosome and offers a direct and rapid way to screen for potential effectors. Given the paucity of strategies used by fungal intracellular pathogens that have been reported, we believe this work will open new ways to investigate this process broadly in fungal pathogens.
Strains used were C. neoformans serotype A strain KN99α (Nielsen et al., 2003) expressing cytoplasmic mCherry (Upadhya et al., 2017), the yeast-locked C. albicans cph1/cph1 efg1/efg1 double mutant strain (Lo et al., 1997), and the S. cerevisiae strain BY4741 (Winzeler et al., 1999) expressing cytoplasmic mCherry (Santiago-Tirado et al., 2017). All fungal strains were maintained at -80°C and grown at 30°C on yeast-peptone-dextrose (YPD) plates.
The human monocytic cell line THP-1 (ATCC TIB-202) was grown in THP-1 complete media (see Text S1 for recipe) and passed 2 – 3 times per week. Every month a new low-passage stock was thawed, and the cells were never used after 12 passages. For differentiation into macrophages, the THP-1 cells were treated as described (Santiago-Tirado et al., 2015), except that we used 0.16 mM phorbol 12-myristate 12-acetate (PMA, Millipore Sigma).
Stock solutions of pHrodo IFL Green (P36012, Thermo Fisher Scientific) were prepared in DMSO according to the manufacturer’s instructions and stored at -20°C in single use aliquots. A fresh aliquot was thawed for each experiment. Fungal cultures grown overnight at 30°C with shaking (225rpm) were diluted to an OD600 of 0.2 and grown for two doublings. The log phase cultures were washed in PBS and opsonized with 40% human serum for 30 min at 37°C with rotation. Serum was obtained from healthy donors with informed consent under a protocol approved by the University of Notre Dame Institutional Review Board. 108 opsonized fungal cells were resuspended in 100 μL of sodium bicarbonate, and 5 μL of pHrodo Green stock was added to a final concentration of 0.5 mM and incubated in the dark for 30 min at 37°C with rotation. When using fungi not expressing mCherry, 10 μL of CFW was added to a final concentration of 10 μg/mL and stained at the same time as pHrodo Green. Cells were washed once with PBS and resuspended in imaging media (see Text S1) containing IFNγ (200 ng/mL) or IL-4 (50 ng/mL) when necessary. For a more detailed protocol, see Text S1.
Coincubation of opsonized, pHrodo-stained cells with differentiated THP-1 cells was done in 35mm glass-bottom dishes (MatTek Corporation) as described (Santiago-Tirado et al., 2017). Briefly, THP-1 cells were seeded at a density of 1.37x105 cells/mL and differentiated with 0.16mM PMA for 48 hr followed by a recovery step of 24 hr without PMA. If required, during the recovery step cytokines were added for polarization of the macrophages. For M1 macrophages, the media contained 200 ng/mL of recombinant human IFNγ (R&D Systems). For M2 macrophages, the media contained 50 ng/mL of recombinant human IL-4 (Shenandoah Biotechnology). Prior to imaging, culture media was replaced with prewarmed imaging media containing appropriate cytokines and opsonized and pHrodo-stained C. neoformans, C. albicans, or S. cerevisiae at a MOI of 5, 1, or 1, respectively. The dish was immediately transferred to a PeCon (Erbach, Germany) P S compact stage-top incubator preheated to 37°C with 5% CO2. A Zeiss Axio Observer 7, with an Axiocam 506 mono camera and a 40x objective was used to image 2x2 fields of view every 3 minutes for at least 180 minutes.
900,000 THP-1 cells were seeded and PMA-differentiated per well in a 6-well plate. Opsonized and pHrodo-stained C. neoformans or S. cerevisiae were added as above. An additional T25 flask was seeded, and unstained fungi grown to provide negative controls for the flow cytometry analysis. At every timepoint, the wells were washed 3X with DPBS and cells lifted with TrypLE without phenol red (Thermo Fisher Scientific). The cells were collected and resuspended in cold DPBS and immediately analyzed by flow cytometry (BD LSRFortessa).
PMA-differentiated THP-1 cells grown on #1.5 round 12mm glass cover slips (Electron Microscopy Sciences) were challenged with opsonized fungi or treated with cytokines as above. At specified timepoints, the cells were washed with DPBS, fixed in 3.7% formaldehyde in PBS for 10 minutes at room temperature (RT), washed with PBS, and permeabilized with 0.3% saponin in PBS for 10 min at RT. Cells were then washed with PBS and blocked for 60 min in 10% goat serum in PBS. Cells were immunolabeled in 1% goat serum at 4°C overnight with an antibody against Gal3 (rat; M3/38: sc23938, Santa Cruz Biotechnology), or against CD86 (PA5-88284, Invitrogen) or CD206 (PA5-101657, Invitrogen). Coverslips were then washed with PBS and a corresponding Alexa Fluor-conjugated goat secondary antibody (Invitrogen) was diluted to 1 μg/mL in 1% goat serum in PBS with 100 ng/mL of 4’6-diamidino-2-phenylindole (DAPI; Millipore Sigma) and added to cells for 60 min at RT. After washing with PBS, coverslips were mounted to imaging slides with 5 μL Prolong Diamond Antifade Mountant (Invitrogen) and allowed to cure for at least 24 hours before imaging. Images of fixed cells were acquired using a Zeiss Axio Observer 7, with an Axiocam 506 mono camera and a 100X/1.4 plan-apo oil objective.
To directly visualize pH changes in the fungal containing-phagosome, we used an amine-reactive pH-sensitive dye to decorate the surface of C. neoformans or S. cerevisiae. This dye, pHrodo Green, has a low pKa, is non-fluorescent at neutral pH but fluorescence rapidly increases as you approach pH of 4, is extremely photostable, and can be used with various platforms such as microscopes, plate readers, or flow cytometers. pHrodo staining does not affect the growth of C. neoformans or S. cerevisiae (Figure S1A), under permissive or stress conditions (Figure S1B), and it is responsive to multiple pH changes without any appreciable loss of fluorescence (Figure S1C). We incubated serum-opsonized, pHrodo-stained fungal cells with naïve (M0) THP-1 cells, a well characterized human monocytic cell line, and imaged the cells for up to 3 hr under a heated, humidified, stage-top chamber containing 5% CO2 (see Methods). C. neoformans and S. cerevisiae express soluble mCherry which facilitates the tracking of the cells before and during internalization, but we also used non-fluorescent yeasts stained with calcofluor white (CWF), including a yeast-locked C. albicans mutant strain (Figure S1D). After analyzing S. cerevisiae internalization events (n = 60), we found that 76.7% of the events showed rapid acidification that remained for at least 90 minutes (behavior 1). One event (1.6%) lost the acidification before 90 minutes (behavior 2) and the rest (21.7%) never acidified (behavior 3). Stills from the main representative behavior of S. cerevisiae are shown in Figure 1A and the behaviors are quantified in Figure 2A. In contrast, a substantial number of the C. neoformans internalization events showed behavior 2 (16.7%; n = 7) or behavior 3 (26.2%; n = 11), whereas only a bit more than half of the events exhibited behavior 1 (57.1%; n = 24). Examples of these behaviors are shown in Figures 1B–D and are quantified in Figure 2B. Notably, the C. albicans strain that is unable to form hyphae exhibits the same behaviors as S. cerevisiae (Figures 1G and 2C), suggesting that the alterations to the phagosomal maturation are specific to C. neoformans. Consistently, if we use two C. neoformans mutant strains known to be defective in intracellular growth, these exhibit mostly behavior 1, just like S. cerevisiae or the yeast-locked C. albicans (Figures 1E, F and 2D, E). These findings demonstrate that C. neoformans can alter phagosome acidification in various ways in M0 macrophages.
Figure 1 Behaviors and kinetics of phagosomal acidification in fungi-infected THP-1 cells. (A) Stills from a representative video showing the main behavior (behavior 1) in S. cerevisiae (Sc)-infected THP-1 cells. For the complete video see Video S1. (B) Stills from a representative video showing behavior 1 in C neoformans (Cn)-infected THP-1 cells. For the complete video see Video S2. (C) Stills from a representative video showing behavior 2 in Cn-infected THP-1 cells. For the complete video see Video S3. (D) Stills from a representative video showing behavior 3 in Cn-infected THP-1 cells. For the complete video see Video S4. (E) Stills from a representative video showing behavior 1 in THP-1 cells infected with Cn mutant cps1Δ. For the complete video see Video S5. (F) Stills from a representative video showing behavior 1 in in THP-1 cells infected with Cn mutant cap60Δ. For the complete video see Video S6. (G) Stills from a representative video showing behavior 1 in THP-1 cells infected with yeast-locked C albicans (Ca). For the complete video see Video S7. Numbers on lower right represent the time in minutes. The arrows point to the same fungus and are white when nonfluorescent in the pHrodo channel and green when fluorescent. Scale bar = 10 μm. (H) Violin plots showing the kinetics and distribution of behaviors in THP-1 cells infected by Sc, Cn, or Ca. For this analysis, all behavior 1 or behavior 2 events throughout all polarization states were combined. The solid line represents the median, and the dashed lines the quartiles. (I) Violin plots showing the kinetics and distribution of behaviors in THP-1 cells infected by Sc, Cn, or Ca. For this analysis, all behaviors events per polarization state were combined. The solid line represents the median, and the dashed lines the quartiles. See Table S1 for mean and standard deviation values. Violin plots were analyzed by one-way nonparametric ANOVA test, with multiple comparisons (Kruskal-Wallis test). *, P < 0.05; **, P < 0.01; ***, P < 0.001; ****, P < 0.0001.
Figure 2 C. neoformans actively manipulates phagosomal pH in an immune-dependent way. (A–C) Quantification of all behaviors under all immune conditions of THP-1 cells infected with (A) S. cerevisiae (Sc), (B) C. neoformans (Cn), and (C) C. albicans (Ca). (D, E) Quantification of the behaviors exhibited by Cn mutants (D) cap60Δ and (E) cps1Δ in M2 macrophages.
To evaluate if immune signals affected the ability of these fungi to alter acidification of their phagosomes, we repeated the assay with THP-1 cells preactivated to M1 with INFγ, or to M2 with IL-4, using published methods (Genin et al., 2015). Protein expression of M1 (CD86) and M2 (CD206) markers was confirmed by immunofluorescence (Figure S2). M1 and M2 macrophages markedly reduced the minor behaviors in S. cerevisiae (Figure 2A; n = 166 for M1 and n = 53 for M2) and yeast-locked C. albicans (Figure 2C; n = 41 for M1 and n = 80 for M2), and still most phagosomes rapidly acidified and stayed acidic. In C. neoformans, however, we found that the different polarization states had slightly opposite results (Figure 2B). In M1 macrophages (n = 31), while most phagosomes still acidify and stay acidic, there was a shift from behavior 2 to behavior 3. In contrast, in M2 macrophages (n = 162), there was a substantial decrease in behavior 1 with a concomitant increase in behavior 3. These findings indicate that C. neoformans can manipulate its phagosome in an immune-dependent manner.
To obtain a sense of the kinetics of acidification, we determined how long after phagocytosis the fungi became fluorescent (Table S1). When compared within and between fungi, none of the behaviors’ kinetics were statistically different between the different polarization states. For behavior 1, the overall time to acidification was 16.9± 12.5 min, 22.8± 12.7 min, and 21.3± 14.4 min for S. cerevisiae, C. neoformans, and C. albicans, respectively; and 22± 12.7 min, 25.3± 13.9 min, and 20± 11.2 min for behavior 2 (Table S1). Likewise, the kinetics are not statistically different between behaviors within host polarization states, however, there was an obvious difference in the pattern of C. neoformans compared to the other fungi. For both S. cerevisiae and C. albicans, M1 polarization had the faster time to acidification, while M0 and M2 had similar kinetics. For C. neoformans, on the other hand, M0 and M1 were similar, and M2 had the slowest time to acidification. These results show that the main difference between C. neoformans and the other fungi is the distribution of behaviors 2 and 3, and how frequently C. neoformans is able to avoid acidification or modulate the pH once its phagosome has acidified, which the other fungi are rarely able to do. To visualize these differences, we generated violin plots of overall times to fluorescence per behavior (Figure 1H; same behaviors between polarization states combined) or per host immune status (Figure 1I; all behaviors within the same polarization state combined). S. cerevisiae’s behavior 1 was statistically different to the others with 75% of its data points lumped together in one peak in the bottom of the plot (95% CI 12 – 15 min). In contrast, the distribution of C. neoformans’s behavior 1 shows two peaks, each with about the same amount of data points (95% CI 15 – 24 min). C. albicans’s plot also has one peak like S. cerevisiae, but the data points are spread out more than S. cerevisiae (95% CI 15 – 18 min). In terms of host polarization states, for both S. cerevisiae and C. albicans, M1 resulted in faster acidification (95% CI 11 – 13 min and 9 – 15 min, respectively). In contrast, for C. neoformans, M2 resulted in slower acidification (95% CI 21 – 27 min) whereas M1 was not statistically different from M0. This suggests that a significant percentage of the C. neoformans population is able to manipulate its phagosome, altering the resulting distribution of phagosomal kinetics.
To obtain a more general idea of how far reaching C. neoformans’s ability to modulate phagosome acidification is, we used the same setup as above but analyzed at least 10,000 events by flow cytometry. The detailed gating scheme is depicted in Figure S3. This analysis clearly showed the inability of S. cerevisiae to avoid phagosome acidification, especially in activated macrophages (Figures 3A, E). In contrast, a significant percentage of the macrophages infected with C. neoformans were unable to acidify their phagosomes (Figures 3B–E). This was evident at all timepoints under all polarization states. Notably, an increase in acidified phagosomes over time was seen only in M1 macrophages, while in M0 and M2 the percentage remained relatively constant (Figure 3E). This is in stark contrast to S. cerevisiae, which over time almost 100% of the infected macrophages had acidified phagosomes, despite more of the macrophages being infected than C. neoformans (Figure S3B). This demonstrates a clear ability in C. neoformans to modulate phagosome acidification in as short as 30 minutes post-infection, and that this ability is counteracted by host immune signals associated with M1 polarization.
Figure 3 The majority of C neoformans phagosomes exhibit impaired acidification but this is modulated by the macrophage polarization state. (A) Representative flow plots of 30, 60, and 120-minute incubation of S. cerevisiae (Sc) with M0 macrophages. The histogram to the right shows the relative number of pHrodo-positive events over time. (B–D) Representative flow plots of 30, 60, and 120-minute incubation of C neoformans (Cn) with (B) M0, (C) M1, and (D) M2 macrophages. The histogram to the right shows the relative number of pHrodo-positive events over time. See Figure S3 for more details on the gating strategy. (E) Quantification of infected macrophages with the indicated fungal species at 30, 60, and 120 minutes by flow cytometry. Shown is the average and error of the mean for 2 - 4 independent experiments. Statistics are a 2-way ANOVA with multiple comparisons. The asterisks (*) represent comparisons to S. cerevisiae-infected M0 cells. The number sign (#) represent comparisons to C neoformans-infected M0 cells. * or #, P < 0.05; ***, P < 0.001; **** or ####, P < 0.0001.
Previous studies have reported phagosomal permeabilization by indirect means (Tucker and Casadevall, 2002; Davis et al., 2015; De Leon-Rodriguez et al., 2018). They were all looking for the effects of phagosomal permeabilization at long timepoints after infection, usually 24, 48, and 72 hr. We see pH manipulation as fast as 30 min post-infection. One way C. neoformans could manipulate phagosome acidification could be by permeabilizing the phagosome membrane, causing neutralization of its contents. We can directly visualize this membrane damage using galectin-3 (Gal-3) staining. Gal-3 will recognize the sugars present inside the phagosome, in the luminal side of its membrane, but only if the membrane is damaged (Paz et al., 2010; Aits et al., 2015). Hence, when the phagosome is permeabilized, Gal-3 has access to its contents and will decorate the interior side of the phagosome (Figure 4A). In this way, Gal-3 acts as a direct membrane damage reporter. We did a timecourse with C. neoformans-infected M0 macrophages and saw little colocalization with the cryptococcal-phagosome at 1 hr, but that increased over time, peaking at 8 hr post-infection (Figure 4B). We chose this timepoint to test if host immune status also affected this membrane damage similar to how it affected pH modulation by the fungus. We found that about one-third of the cryptococcal-phagosomes were decorated with Gal-3 in M0, and that dropped to 17.9% in M1, whereas in M2 it increased to 50% (Figure 4C). In comparison, when infected with S. cerevisiae, only 10% (M0), 7% (M1), or 18% (M2) of the phagosomes are positive for Gal-3. The incidence of membrane damage found with Gal-3 staining correlates well with the percentage of pHrodo-negative macrophages found by flow cytometry or combined behaviors 2 and 3 found by live microscopy. This suggests that C. neoformans’s ability to damage its phagosome might be one of the strategies used by this fungus and it happens early on cellular infection.
Figure 4 C. neoformans damages and permeabilizes its phagosome early after infection in an immune signal-dependent way. (A) Images from a representative THP-1 cell (nuclei in blue) 24 hr-post infection with C. neoformans (red) showing membrane damage around one cryptococcal-phagosome (Gal-3 in green). The number on the lower right represent the Z-slice on a series of pictures that are reconstructed into a full projection on the last image to the right. The dark halo surrounding the cell represents its polysaccharide capsule. (B) Quantification of Gal-3 staining over time in M0 THP-1 cells infected with C. neoformans (Cn). CCP, cryptococcal-containing phagosome. (C) Quantification of Gal-3 staining at 8 hr in M0, M1, and M2 THP-1 cells infected with Cn or S. cerevisiae (Sc). Statistics are ordinary one-way ANOVA with multiple comparisons. *, P < 0.05; **, P< 0.01; ***, P < 0.001; ****, P < 0.0001.
Here we use a novel pH-sensitive staining procedure to follow and directly visualize changes in pH in fungal-containing phagosomes. We applied this method to fluorescent strains of C. neoformans and S. cerevisiae, and analyzed them by live microscopy, allowing us to identify different behaviors and measure their dynamics, as well as by flow cytometry, which allows us to capture and analyze tens of thousands of events. To demonstrate broader applicability of the method, we also used non-fluorescent strains of C. neoformans and C. albicans, highlighting the possibility of using this dye to study other intracellular fungal pathogens. This dye and similar approaches have recently been used to study phagocytosis, localization, and effects of bacterial infections on host cells (Lenzo et al., 2016; Awasthi et al., 2019; Ben Shlomo et al., 2019; Wood et al., 2020). Although it is likely that C. neoformans somehow alters its phagosome, in regard to acidification, published reports are controversial. Moreover, to date no cryptococcal effector necessary for this phagosomal manipulation has been identified. Here we show that a significant percentage of the cryptococcal phagosomes do not acidify normally, and this alteration is dependent on the immune status of the host phagocyte. This is not surprising given that different polarization states have different effects on inflammation (Atri et al., 2018). However, all macrophages, despite polarization, acidify their phagolysosomes to the same extent, albeit with different kinetics (Canton et al., 2014). This is seen, for example, with S. cerevisiae and the yeast-locked C. albicans, that regardless the polarization state, most of their phagosomes acidify. In contrast, with C. neoformans, a majority of the phagosomes do not acidify, and that observation is exacerbated in M2 macrophages.
Furthermore, for the first time, we directly visualize, and quantify, phagosomal membrane damage, which might be one of the strategies used by C. neoformans to alter phagosomal acidification, but it is not the only one. Phagosomal permeabilization is a common mechanism used by intracellular bacterial pathogens, where effectors that cause membrane damage are essential for their intracellular survival. Gal-3 staining has been used to show that Shigella and Salmonella damage their phagosomes in order to escape into the cytoplasm (Paz et al., 2010; Lisowski et al., 2022). C. neoformans, however, remains inside a phagosome, but damaging the membrane to access the host cytoplasm would represent a big advantage. Nevertheless, phagosomal membrane damage does not seems to be completely essential as in the first 2 – 3 hr post-infection, up to 34% of the phagosomes are not acidic (by flow cytometry; Figure 3E) and up to 55% of the phagosomes exhibit some alteration (by live microscopy; Figure 2B), yet only ~5% of cryptococcal-phagosomes stain with Gal-3 in that timeframe. Clearly, there are other mechanisms used by this fungus to manipulate phagosomal acidification. Nevertheless, both of these phenotypes (large percentage of pHrodo-negative phagosomes and phagosomes decorated with Gal-3) are mostly absent from S. cerevisiae, a fungus of similar morphology and size, demonstrating that C. neoformans actively cause these alterations. The fact that there are multiple behaviors, including behavior 1 which is shared with S. cerevisiae, may explain the different results that have been reported about the cryptococcal phagosome (Figure S4).
Interestingly, C. neoformans is known to escape phagocytes by non-lytic exocytosis (NLE), a phenomenon whereby the fungal cells are secreted from the host cell without any adverse effect to any cell type (Ma et al., 2006; Alvarez and Casadevall, 2006; Nicola et al., 2011). This has been reported to happen in about 10 – 30% of the infected cells, which is similar to the percentage of infected macrophages that are pHrodo negative in our flow cytometry studies (Figure 3E) and the percentage of phagosomes that are decorated with Gal-3 (Figure 4C). Phagosomal acidification has been shown to affect NLE, with NLE events happening from nonacidified phagosomes (Smith et al., 2015). Notably, we captured two NLE events where the pHrodo signal was lost and shortly thereafter the fungi underwent NLE (Video S8). The fact that we only saw two events in our live imaging is not surprising since the Casadevall group has shown that these events usually happen after several hours of intracellular residence (>6 hr) while we only imaged for up to 3 hr (Stukes et al., 2014). Nevertheless, our findings are in line and consistent with NLE reports, providing additional support that NLE is an active process triggered by C. neoformans’s manipulation of its phagosome.
M1 and M2 macrophages exhibit different abilities controlling cryptococcal intracellular growth; however, it is not known if these differing outcomes correlate with different fungal intracellular niches. Interestingly, we show that the frequency of phagosome manipulation by C. neoformans varies between the different host activation states. Although in our flow cytometry analysis only the M1 macrophages were statistically different to other conditions, we could clearly see a trend in the M2 macrophages where the population of pHrodo negative phagosomes was higher than in M0. We acknowledge that the analysis by flow cytometry is limited in the sense that not all phagosomes within the same host cell are manipulated equally; however, the fact that with S. cerevisiae the pHrodo-positive population increased to almost 100% in all conditions whereas with C. neoformans this population remains constant argues that the trend seen in M2 macrophages is relevant. Consistently, when analyzing the kinetics of acidification in the different polarization states, M2 had the higher acidification times relative to M0 or M1 (Figure 1I). Moreover, the differences in manipulation over time in M1 relative to M2 macrophages correlate with animal studies where M1 macrophages have been shown to control infection better than M2 macrophages. Hence, our studies support the hypothesis that the ability to manipulate phagosomal maturation correlates with the infection outcome. Consistently, cryptococcal cap60Δ and cps1Δ mutants, which cannot grow intracellularly and are avirulent, cannot alter acidification of their phagosomes (Figures 2D, E).
In conclusion, here we demonstrate that C. neoformans can manipulate acidification of its phagosomes in various ways, that this ability is affected by the immune status of the host, and that one way this manipulation could happen is by phagosomal membrane permeabilization. We present a versatile and easy method to screen phagosomal changes by live microscopy or by flow cytometry. We show that C. neoformans’s ability to manipulate its phagosome is robust and happens soon after internalization. Analysis of cryptococcal mutants with these methods might identify effectors necessary for this manipulation, but these methods can be broadly applicable to other intracellular yeasts as well.
The original contributions presented in the study are included in the article/Supplementary Material. Further inquiries can be directed to the corresponding author.
FS-T was responsible for the study conception and design. ES-B, PS, and FS-T performed the experiments, collected data, and analyzed results. PS and FS-T prepared the figures and wrote the manuscript with input from ES-B. All authors read the manuscript and provided edits and feedback. ES-B and PS share first authorship and the naming order was determined alphabetically. All authors contributed to the article and approved the submitted version.
This work was supported by start-up funds from the University of Notre Dame to FS-T. ES-B was partially supported by a Kinesis-Fernández Richards Family Fellowship.
We thank members of the Santiago-Tirado lab for comments and feedback on this paper.
The authors declare that the research was conducted in the absence of any commercial or financial relationships that could be construed as a potential conflict of interest.
All claims expressed in this article are solely those of the authors and do not necessarily represent those of their affiliated organizations, or those of the publisher, the editors and the reviewers. Any product that may be evaluated in this article, or claim that may be made by its manufacturer, is not guaranteed or endorsed by the publisher.
The Supplementary Material for this article can be found online at: https://www.frontiersin.org/articles/10.3389/fcimb.2022.967486/full#supplementary-material
Supplementary Figure 1 | Characteristics of pHrodo-stained fungal cells. (A) Growth curve of unstained and pHrodo-stained S. cerevisiae (Sc) and C. neoformans (Cn) in YPD media at 30°C over 48 hr. No effect of pHrodo staining is seen in either of the fungi. (B) Dot spot growth analysis in RPMI-agar plates of Cn under the indicated conditions. Images were taken at 48 and 72 hr. (C, D) Representative images of pHrodo-stained (C) Cn and (D) yeast-locked C. albicans (Ca) cells after sequential resuspension in PBS, pH 7.4, and sodium acetate buffer (AcONa), pH 5.0, showing that the pHrodo staining is responsive to changes in pH. Ca cells are not fluorescent and were counterstained with CFW. The CFW fluorescence was artificially colored red for ease of view. Scale bars represents 5 μm.
Supplementary Figure 2 | Polarization of THP-1 cells using IFNγ or IL-4 treatment. THP-1 cells were treated with IFNγ, IL-4, or vehicle (water) as described in the Methods section. Shown are representative fields of views of immunofluorescence analysis using DAPI (blue) to stain nuclei; anti-CD86 antibody (artificially colored green); and anti-CD206 antibody (artificially colored red). The percentage of cells positive for each marker is quantified on the right. Each circle represents a coverslip, 2 coverslips per biological independent experiment. Statistics are unpaired t-tests comparing the two conditions. ****, P < 0.0001.
Supplementary Figure 3 | Flow cytometry analysis. (A) Representative plots showing the flow cytometry gating strategy. First, unstained fungal and host cells are analyzed to determine their position in the size-scatter plot. These positions are used to eliminate free fungi (and cell debris) and select healthy THP-1 cells (gates 1 and 2). The healthy THP-1 cell population is used to select for single cells (gate 3). The singlets are then used to select for the population positive in mCherry (gate 4). The population negative to mCherry are uninfected THP-1 cells. Lastly, the infected population is used to analyze pHrodo signal (gate 5). (B) Uptake of fungal cells from a representative flow experiment using M0 macrophages. Despite using only an MOI of 1 (versus an MOI of 5 for C. neoformans), S. cerevisiae (Sc) is phagocytosed more avidly than C. neoformans (Cn). This data comes from Gate 4, the mCherry-positive population represents infected macrophages. The values in here were calculated by dividing gate 4 by gate 3.
Supplementary Figure 4 | Model summarizing our findings. We found three behaviors in cells infected with either S. cerevisiae (unbudded pink cell) or C. neoformans (budded pink cell surrounded by capsule). Behavior 1 (blue path) is shared between the two fungi and presumable results in formation of a fully-functional phagolysosome, where the fungal cells are destroyed. Behavior 2 (red path) deviates from the normal path and results in loss of acidification. This can happen in part by phagosomal membrane permeabilization (broken dark green outline). Behavior 3 (green path) results in cells that never acidify, hence this path represents an unknown phagosomal compartment (depicted by ‘?’). This compartment never acidifies so it could also result in phagosomal permeabilization, but we would not be able to see it in our live imaging.
Supplementary Video 1 | Representative video of the main behavior (behavior 1) of S. cerevisiae phagosomes.
Supplementary Video 2 | Representative video of behavior 1 of C. neoformans phagosomes.
Supplementary Video 3 | Representative video of behavior 2 of C. neoformans phagosomes.
Supplementary Video 4 | Representative video of behavior 3 of C. neoformans phagosomes.
Supplementary Video 5 | Representative video of behavior 1 of C. neoformans mutant cps1Δ. This mutant was stained with CFW, but the color was changed to red for ease of visualization.
Supplementary Video 6 | Representative video of behavior 1 of C. neoformans mutant cap60Δ. This mutant was stained with CFW, but the color was changed to red for ease of visualization.
Supplementary Video 7 | Representative video of behavior 1 of yeast-locked C. albicans mutant. This mutant was stained with CFW, but the color was changed to red for ease of visualization. Interestingly, the pHrodo dye (green) seems to be inherited by the growing bud, whereas CFW (red) is retained in the mother cell.
Supplementary Video 8 | Video depicting two instances of non-lytic exocytosis (NLE). Notice that immediately after losing fluorescence (pH neutralization) the yeast cells are expelled from the host.
Aits, S., Kricker, J., Liu, B., Ellegaard, A. M., Hamalisto, S., Tvingsholm, S., et al. (2015). Sensitive detection of lysosomal membrane permeabilization by lysosomal galectin puncta assay. Autophagy 11 (8), 1408–1424. doi: 10.1080/15548627.2015.1063871
Alvarez, M., Casadevall, A. (2006). Phagosome extrusion and host-cell survival after Cryptococcus neoformans phagocytosis by macrophages. Curr. Biol. 16 (21), 2161–2165. doi: 10.1016/j.cub.2006.09.061
Atri, C., Guerfali, F. Z., Laouini, D. (2018). Role of human macrophage polarization in inflammation during infectious diseases. Int. J. Mol. Sci. 19 (6), 1–15. doi: 10.3390/ijms19061801
Awasthi, S., Singh, B., Ramani, V., Xie, J., Kosanke, S. (2019). TLR4-interacting SPA4 peptide improves host defense and alleviates tissue injury in a mouse model of pseudomonas aeruginosa lung infection. PLoS One 14 (1), e0210979. doi: 10.1371/journal.pone.0210979
Ben Shlomo, S., Mouhadeb, O., Cohen, K., Varol, C., Gluck, N. (2019). COMMD10-guided phagolysosomal maturation promotes clearance of staphylococcus aureus in macrophages. iScience 14, 147–163. doi: 10.1016/j.isci.2019.03.024
Canton, J., Khezri, R., Glogauer, M., Grinstein, S. (2014). Contrasting phagosome pH regulation and maturation in human M1 and M2 macrophages. Mol. Biol. Cell 25 (21), 3330–3341. doi: 10.1091/mbc.e14-05-0967
Charalambous, L. T., Premji, A., Tybout, C., Hunt, A., Cutshaw, D., Elsamadicy, A. A., et al. (2018). Prevalence, healthcare resource utilization and overall burden of fungal meningitis in the united states. J. Med. Microbiol. 67 (2), 215–227. doi: 10.1099/jmm.0.000656
Davis, M. J., Eastman, A. J., Qiu, Y., Gregorka, B., Kozel, T. R., Osterholzer, J. J., et al. (2015). Cryptococcus neoformans-induced macrophage lysosome damage crucially contributes to fungal virulence. J. Immunol. 194 (5), 2219–2231. doi: 10.4049/jimmunol.1402376
De Leon-Rodriguez, C. M., Fu, M. S., Corbali, M. O., Cordero, R. J. B., Casadevall, A. (2018). The capsule of cryptococcus neoformans modulates phagosomal pH through its acid-base properties. mSphere 3 (5), 1–8. doi: 10.1128/mSphere.00437-18
De Leon-Rodriguez, C. M., Rossi, D. C. P., Fu, M. S., Dragotakes, Q., Coelho, C., Guerrero Ros, I., et al. (2018). The outcome of the cryptococcus neoformans-macrophage interaction depends on phagolysosomal membrane integrity. J. Immunol. 201 (2), 583–603. doi: 10.4049/jimmunol.1700958
Dragotakes, Q., Stouffer, K. M., Fu, M. S., Sella, Y., Youn, C., Yoon, O. I., et al. (2020). Macrophages use a bet-hedging strategy for antimicrobial activity in phagolysosomal acidification. J. Clin. Invest. 130 (7), 3805–3819. doi: 10.1172/JCI133938
Fu, M. S., Coelho, C., De Leon-Rodriguez, C. M., Rossi, D. C. P., Camacho, E., Jung, E. H., et al. (2018). Cryptococcus neoformans urease affects the outcome of intracellular pathogenesis by modulating phagolysosomal pH. PLoS Pathog. 14 (6), e1007144. doi: 10.1371/journal.ppat.1007144
Gaylord, E. A., Choy, H. L., Doering, T. L. (2020). Dangerous liaisons: Interactions of cryptococcus neoformans with host phagocytes. Pathogens 9 (11), 1–15. doi: 10.3390/pathogens9110891
Genin, M., Clement, F., Fattaccioli, A., Raes, M., Michiels, C. (2015). M1 and M2 macrophages derived from THP-1 cells differentially modulate the response of cancer cells to etoposide. BMC Cancer 15, 577. doi: 10.1186/s12885-015-1546-9
Lenzo, J. C., O'Brien-Simpson, N. M., Cecil, J., Holden, J. A., Reynolds, E. C. (2016). Determination of active phagocytosis of unopsonized porphyromonas gingivalis by macrophages and neutrophils using the pH-sensitive fluorescent dye pHrodo. Infect. Immun. 84 (6), 1753–1760. doi: 10.1128/IAI.01482-15
Leopold Wager, C. M., Hole, C. R., Wozniak, K. L., Wormley, F. L., Jr. (2016). Cryptococcus and phagocytes: Complex interactions that influence disease outcome. Front. Microbiol. 7, 105. doi: 10.3389/fmicb.2016.00105
Levitz, S. M., Harrison, T. S., Tabuni, A., Liu, X. (1997). Chloroquine induces human mononuclear phagocytes to inhibit and kill Cryptococcus neoformans by a mechanism independent of iron deprivation. J. Clin. Invest. 100 (6), 1640–1646. doi: 10.1172/JCI119688
Levitz, S. M., Nong, S. H., Seetoo, K. F., Harrison, T. S., Speizer, R. A., Simons, E. R. (1999). Cryptococcus neoformans resides in an acidic phagolysosome of human macrophages. Infect. Immun. 67 (2), 885–890. doi: 10.1128/IAI.67.2.885-890.1999
Lisowski, C., Dias, J., Costa, S., Silva, R. J., Mano, M., Eulalio, A. (2022). Dysregulated endolysosomal trafficking in cells arrested in the G1 phase of the host cell cycle impairs salmonella vacuolar replication. Autophagy 18 (8), 1785–1800. doi: 10.1080/15548627.2021.1999561
Lo, H. J., Kohler, J. R., DiDomenico, B., Loebenberg, D., Cacciapuoti, A., Fink, G. R. (1997). Nonfilamentous c. albicans mutants are avirulent. Cell 90 (5), 939–949. doi: 10.1016/s0092-8674(00)80358-x
Ma, H., Croudace, J. E., Lammas, D. A., May, R. C. (2006). Expulsion of live pathogenic yeast by macrophages. Curr. Biol. 16 (21), 2156–2160. doi: 10.1016/j.cub.2006.09.032
Nicola, A. M., Robertson, E. J., Albuquerque, P., Derengowski Lda, S., Casadevall, A. (2011). Nonlytic exocytosis of cryptococcus neoformans from macrophages occurs in vivo and is influenced by phagosomal pH. mBio 2 (4), 1–9. doi: 10.1128/mBio.00167-11
Nielsen, K., Cox, G. M., Wang, P., Toffaletti, D. L., Perfect, J. R., Heitman, J. (2003). Sexual cycle of Cryptococcus neoformans var. grubii and virulence of congenic a and alpha isolates. Infect. Immun. 71 (9), 4831–4841. doi: 10.1128/IAI.71.9.4831-4841.2003
Paz, I., Sachse, M., Dupont, N., Mounier, J., Cederfur, C., Enninga, J., et al. (2010). Galectin-3, a marker for vacuole lysis by invasive pathogens. Cell Microbiol. 12 (4), 530–544. doi: 10.1111/j.1462-5822.2009.01415.x
Rajasingham, R., Smith, R. M., Park, B. J., Jarvis, J. N., Govender, N. P., Chiller, T. M., et al. (2017). Global burden of disease of HIV-associated cryptococcal meningitis: an updated analysis. Lancet Infect. Dis. 17 (8), 873–881. doi: 10.1016/S1473-3099(17)30243-8
Rudman, J., Evans, R. J., Johnston, S. A. (2019). Are macrophages the heroes or villains during cryptococcosis? Fungal Genet. Biol. 132, 103261. doi: 10.1016/j.fgb.2019.103261
Santiago-Tirado, F. H., Onken, M. D., Cooper, J. A., Klein, R. S., Doering, T. L. (2017). Trojan Horse transit contributes to blood-brain barrier crossing of a eukaryotic pathogen. MBio 8 (1), 1–16. doi: 10.1128/mBio.02183-16
Santiago-Tirado, F. H., Peng, T., Yang, M., Hang, H. C., Doering, T. L. (2015). A single protein s-acyl transferase acts through diverse substrates to determine cryptococcal morphology, stress tolerance, and pathogenic outcome. PLoS Pathog. 11 (5), e1004908. doi: 10.1371/journal.ppat.1004908
Smith, L. M., Dixon, E. F., May, R. C. (2015). The fungal pathogen Cryptococcus neoformans manipulates macrophage phagosome maturation. Cell Microbiol. 17 (5), 702–713. doi: 10.1111/cmi.12394
Srikanta, D., Santiago-Tirado, F. H., Doering, T. L. (2014). Cryptococcus neoformans: historical curiosity to modern pathogen. Yeast 31 (2), 47–60. doi: 10.1002/yea.2997
Stukes, S. A., Cohen, H. W., Casadevall, A. (2014). Temporal kinetics and quantitative analysis of cryptococcus neoformans nonlytic exocytosis. Infect. Immun. 82 (5), 2059–2067. doi: 10.1128/IAI.01503-14
Tucker, S. C., Casadevall, A. (2002). Replication of Cryptococcus neoformans in macrophages is accompanied by phagosomal permeabilization and accumulation of vesicles containing polysaccharide in the cytoplasm. Proc. Natl. Acad. Sci. U.S.A. 99 (5), 3165–3170. doi: 10.1073/pnas.052702799
Upadhya, R., Lam, W. C., Maybruck, B. T., Donlin, M. J., Chang, A. L., Kayode, S., et al. (2017). A fluorogenic c. neoformans reporter strain with a robust expression of m-cherry expressed from a safe haven site in the genome. Fungal Genet. Biol. 108, 13–25. doi: 10.1016/j.fgb.2017.08.008
Vaughn, B., Abu Kwaik, Y. (2021). Idiosyncratic biogenesis of intracellular pathogens-containing vacuoles. Front. Cell Infect. Microbiol. 11, 722433. doi: 10.3389/fcimb.2021.722433
Winzeler, E. A., Shoemaker, D. D., Astromoff, A., Liang, H., Anderson, K., Andre, B., et al. (1999). Functional characterization of the s. cerevisiae genome by gene deletion and parallel analysis. Science 285 (5429), 901–906. doi: 10.1126/science.285.5429.901
Wood, A. J., Vassallo, A. M., Ruchaud-Sparagano, M. H., Scott, J., Zinnato, C., Gonzalez-Tejedo, C., et al. (2020). C5a impairs phagosomal maturation in the neutrophil through phosphoproteomic remodeling. JCI Insight 5 (15), 1–16. doi: 10.1172/jci.insight.137029
Keywords: Cryptococcus, intracellular pathogen, fungal pathogen, phagosome, pHrodo, galectin-3
Citation: Santiago-Burgos EJ, Stuckey PV and Santiago-Tirado FH (2022) Real-time visualization of phagosomal pH manipulation by Cryptococcus neoformans in an immune signal-dependent way. Front. Cell. Infect. Microbiol. 12:967486. doi: 10.3389/fcimb.2022.967486
Received: 12 June 2022; Accepted: 08 September 2022;
Published: 23 September 2022.
Edited by:
Iuliana V. Ene, Institut Pasteur, FranceReviewed by:
Slavena Vylkova, Friedrich Schiller University Jena, GermanyCopyright © 2022 Santiago-Burgos, Stuckey and Santiago-Tirado. This is an open-access article distributed under the terms of the Creative Commons Attribution License (CC BY). The use, distribution or reproduction in other forums is permitted, provided the original author(s) and the copyright owner(s) are credited and that the original publication in this journal is cited, in accordance with accepted academic practice. No use, distribution or reproduction is permitted which does not comply with these terms.
*Correspondence: Felipe H. Santiago-Tirado, ZnNhbnRpYWdvQG5kLmVkdQ==
†Present address: Emmanuel J. Santiago-Burgos, Imanis Life Sciences, LLC, Rochester, MN, United States
‡These authors have contributed equally to this work
Disclaimer: All claims expressed in this article are solely those of the authors and do not necessarily represent those of their affiliated organizations, or those of the publisher, the editors and the reviewers. Any product that may be evaluated in this article or claim that may be made by its manufacturer is not guaranteed or endorsed by the publisher.
Research integrity at Frontiers
Learn more about the work of our research integrity team to safeguard the quality of each article we publish.