- 1School of Sport, Shenzhen University, Shenzhen, China
- 2Key Laboratory of Adolescent Health Assessment and Exercise Intervention of Ministry of Education, East China Normal University, Shanghai, China
Innate immunity is the first line of defense against invading external pathogens, and pattern recognition receptors (PRRs) are the key receptors that mediate the innate immune response. Nowadays, there are various PRRs in cells that can activate the innate immune response by recognizing pathogen-related molecular patterns (PAMPs). The DNA sensor cGAS, which belongs to the PRRs, plays a crucial role in innate immunity. cGAS detects both foreign and host DNA and generates a second-messenger cGAMP to mediate stimulator of interferon gene (STING)-dependent antiviral responses, thereby exerting an antiviral immune response. However, the process of cGAS/STING signaling is regulated by a wide range of factors. Multiple studies have shown that viruses directly target signal transduction proteins in the cGAS/STING signaling through viral surface proteins to impede innate immunity. It is noteworthy that the virus utilizes these cGAS/STING signaling regulators to evade immune surveillance. Thus, this paper mainly summarized the regulatory mechanism of the cGAS/STING signaling pathway and the immune escape mechanism of the corresponding virus, intending to provide targeted immunotherapy ideas for dealing with specific viral infections in the future.
1 Introduction
Human beings have been fighting fiercely with viruses since ancient times. Today’s severe acute respiratory syndrome coronavirus 2 (SARS-CoV-2) seriously threatens human health and public safety. Innate immunity is the host’s first line of defense against pathogen invasion. Pattern recognition receptors (PRRs) are essential for mediating innate immune defense. The PRRs in the cell, including toll-like receptors (TLRs), nod-like receptors (NLRs), retinoic acid-inducible gene-I-like receptors (RLRs), C-type lectin receptors (CLRs), and DNA sensors, recognize the corresponding pathogen-related molecular pattern (PAMPs) to activate the relevant signal pathway to trigger type I Interferons (IFN-I), pro-inflammatory cytokines, and chemokines to eliminate invading pathogens (Carty et al., 2021). PAMPs are relatively conserved molecules in the evolution of pathogens. The main PAMPs are the nucleic acids of pathogens, including DNA (unmethylated CpG sequences), double-stranded RNA, single-stranded RNA, 5-triphosphate RNA (5’-ppp RNA), as well as lipoproteins, cell surface glycoproteins, membrane components, and viral capsids (Tang et al., 2012; Yoh et al., 2022). DNA is one of the vital genetic materials of the virus, which can release its DNA into the cytoplasm once it has infected the host cell. The cyclic GMP-AMP synthase (cGAS) can recognize DNA to trigger activation of stimulator of interferon gene (STING)-mediated innate immunity. STING, first reported by Hiroki Ishikawa and Glen Barber in 2008, is an endoplasmic reticulum adaptor protein critical for innate immune signaling processes (Ishikawa and Barber, 2008). Subsequently, STING was found to mediate IFN-I signaling through IRF3 (Zhong et al., 2008). Then, dimerization of STING was found to be critical for the activation of subsequent downstream signaling (Sun et al., 2009). Finally, it was reported that cGAS is a cytoplasmic DNA sensor that activates STING-mediated antiviral immunity by producing the second messenger cGAMP (Sun et al., 2013; Wu et al., 2013).
As a dangerous stressor, the released DNA is captured by numerous DNA receptors that belong to PRRs in the cell to trigger the innate immune response. For example, interferon-inducible protein 16 (IFI16), as an intracellular DNA sensor and interferon-inducible gene (ISG) induced by IFN-I, mediate STING-dependent antiviral responses (Unterholzner et al., 2010). However, STING can directly interact with IFI16 and facilitate IFI16 degradation via the ubiquitin-proteasome pathway by recruiting the E3 ligase triple structural domain protein 21 (TRIM21) (Li et al., 2019), which implies that STING can negatively regulate IFI16 to avoid overactivation of STING. In addition, Sox2 recognizes microbial DNA to activate the complex of kinase TGF-β-activated kinase 1 (TAK1) and its binding partner TAB2, leading to the activation of nuclear transcription factor-κB (NF-κB) and activating protein-1 (AP-1) (Xia et al., 2015). DDX41, a member of the DEXDc family of helicases, also triggers STING-mediated IFN-I production by sensing DNA in the cytoplasm (Zhang et al., 2011). Moreover, TLR9 and absent in melanoma 2 (AIM2) also sense DNA in the cytoplasm to induce innate immune responses (Hornung et al., 2009; West and Shadel, 2017). In addition to the above-mentioned DNA sensors, RNA polymerase III synthesizes 5’-ppp RNA from the poly(dA-dT) template to induce IFN-β through the retinoic acid-induced gene I (RIG-I) pathway (Chiu et al., 2009). However, the DNA sensor cGAS plays a crucial role in the process of the innate immune response. It has been demonstrated that the knockout of cGAS in immune cells fails to produce IFN-I and other inflammatory cytokines in response to DNA virus infection (Li et al., 2013). Thus, activation of the cGAS/STING signaling pathway is critical to counteract the virus compared to other DNA receptor signals. However, viruses have evolved multiple strategies to evade elimination by the cGAS/STING signaling. For example, viruses can suppress innate immunity through direct viral targeting of cGAS and STING (Ma and Damania, 2016). Furthermore, the activation of cGAS and STING is regulated by numerous factors (Wan et al., 2020). Notably, whether the virus suppresses cGAS/STING signaling through related regulatory factors deserves further consideration. Understanding the relationship between the virus and the cGAS/STING signaling is crucial for humans to defeat the virus in the future.
2 cGAS/STING signaling
cGAS is a cytoplasmic DNA sensor that synthesizes 2′3′-cyclic-GMP-AMP (cGAMP) in a DNA-dependent manner. Double-stranded DNA (dsDNA) can induce an innate immune response through the cGAS/STING signaling pathway (Sun et al., 2013; Wu et al., 2013). Surprisingly, it has been found that in the resting state, cGAS localizes to the plasma membrane via the actions of an N-terminal phosphoinositide-binding domain. In contrast, cGAS translocates to the cytoplasm upon DNA stimulation (Barnett et al., 2019). Moreover, cGAS was also found to localize in the nucleus. However, nucleosomes interact with cGAS to form steric hindrance to inhibit cGAS activation by genomic DNA (Pathare et al., 2020). BAF (barrier-to-autointegration factor 1), a chromatin-binding protein, can compete with cGAS for DNA binding to inhibit the formation of DNA-cGAS complexes (Guey et al., 2020). Cia-cGAS, a circular RNA highly expressed in the nucleus, can bind to the DNA sensor cGAS in the nucleus, blocking its synthetase activity. Mechanistically, cia-cGAS harbors a stronger binding affinity to cGAS than DNA (Xia et al., 2018). These studies suggest that multiple mechanisms exist in cells to avoid potentially damaging autoinflammatory responses caused by cGAS sensing of genomic DNA. Notably, in response to DNA stimulation, cGAS in the nucleus is exported into the cytoplasm by its nuclear export signal to sense DNA (Sun et al., 2021). After cGAS captures DNA, it catalyzes the synthesis of cGAMP from ATP and GTP to activate STING. STING contains a transmembrane domain formed by four transmembrane helices, a cytoplasmic ligand-binding domain (LBD), and a C-terminal tail. In the absence of binding to cGAMP, STING forms butterfly-shaped dimers through the ligand-binding domain. cGAMP binds to the cleft at the center of the butterfly-shaped LBD dimer, which induces a conformational change that promotes the STING oligomerization and translocation of Golgi and perinuclear microsomes (Zhang et al., 2013; Luo et al., 2016; Shang et al., 2019). Subsequently, STING recruits and activates TBK1 through its C-terminal tail containing the conserved PLPLRT/SD motif. Activated TBK1 phosphorylates STING at the PLPLRT/SD motif, promoting further recruitment and activation of TBK1, which subsequently allows STING to recruit IRF-3 and promote IRF3 phosphorylation, ultimately leading to the expression of IFN-I (Zhang et al., 2019; Zhao et al., 2019). IFN-I can further exert antiviral functions by stimulating the production of numerous interferon-stimulated genes (ISGs) products, such as IRF1, IFITM3, and HSPE (Schoggins et al., 2015).
In the process of STING activation, the E3 ubiquitin ligase TRIM32 and TRIM56 interact with STING for K63-linked ubiquitination, promoting STING oligomerization and its recruitment of TBK1 (Tsuchida et al., 2010; Zhang et al., 2012). Notably, IRF8 is essential for DNA but not RNA virus-triggered induction of downstream antiviral genes in monocytes. Upon triggering the DNA sensing pathway, IRF8 interacts with STING after phosphorylation at S151, which promotes oligomerization of STING and its recruitment to IRF3 (Luo et al., 2022). Moreover, a recent study found that a small-molecule agonist, compound 53 (C53), binds to a cryptic pocket of the STING transmembrane domain located between the two subunits of the STING dimer, promoting the oligomerization of STING. Furthermore, the coexistence of cGAMP and C53 in cells more stably activates STING than cGAMP alone (Lu et al., 2022). Thus, C53 analogs are likely to be involved in the process of cellular STING activation, which is worthy of further exploration in the future. In addition, activated STING activates NF-κB signaling through the inhibitor of NF-κB kinase (IKK) complex. Mechanistically, STING activates TRIM32 and TRIM56 to mediate the K63-linked ubiquitination of NF-κB-essential modulator (NEMO), which subsequently activates IKKβ to trigger the phosphorylation of inhibitor of NF-κB (IκBα), thereby activating NF-κB signaling. IKKβ can also activate TBK1, and in turn, TBK1 can also activate IKKβ, which forms a positive feedback loop pathway to strengthen cytokine production during cGAS-STING activation. Notably, activation of NF-κB signaling is essential for the production of IFN-I (Cai et al., 2014; Fang et al., 2017). Recent studies have found that STING is transferred to the Golgi apparatus to bind to sulfated glycosaminoglycans (sGAGs), which promotes STING polymerization and activation of the kinase TBK1. Notably, sGAGs in the Golgi apparatus are necessary and sufficient to drive STING polymerization (Fang et al., 2021). Moreover, palmitoylation at Cys88/91 of STING transported to the Golgi apparatus is required for the IFN-I response (Mukai et al., 2016). Furthermore, upon dsDNA stimulation, the autophagy-related gene 9a (Atg9a), located in the Golgi apparatus, interacts with STING to suppress the assembly of STING and TBK1, interfering with the innate immune response (Saitoh et al., 2009). These results suggest that the activity of STING after Golgi translocation is also regulated by numerous factors.
3 Regulation of cGAS/STING signaling
3.1 Ubiquitination and SUMOylation of cGAS/STING signaling
Numerous studies have found that the activation of key factors in cGAS/STING signaling is regulated by ubiquitination and SUMOylation. For example, the E3 ubiquitin ligase ring finger protein 5 (RNF5) targets STING for degradation by promoting the ubiquitination of the K48 linkage of STING (Zhong et al., 2009). Of note, RNF26 catalyzes K11-linked polyubiquitination of STING at lysine 150, a residue targeted by RNF5 for K48-linked polyubiquitination (Qin et al., 2014). Thus, RNF26 prevents RNF5 from mediating the k48-linked polyubiquitination and degradation of STING, positively regulating the STING signaling. However, further studies found that RNF26 promotes the autophagy degradation of IRF3, and knockdown of RNF26 can potentiate virus-triggered induction of IFN-I (Qin et al., 2014). These results suggest that RNF26 plays a dual role in the cGAS/STING signaling, and its role may be to limit the excessive activation of IFN-I signaling. Besides, it was found that RNF115 catalyzes the K63-linked ubiquitination of STING, whereas it catalyzes the K48-linked ubiquitination of MAVS. RNF115 knockout mice exhibit hyposensitivity to encephalomyocarditis virus (EMCV) infection and hypersensitivity to herpes simplex virus type 1 (HSV-1) infection (Zhang et al., 2020). Thus, RNF115 positively regulates the cGAS/STING signaling triggered by the DNA virus and negatively regulates the RLR signaling induced by the RNA virus. Furthermore, RNF128 interacts with TBK1 and catalyzes the K63-linked polyubiquitination of TBK1 to promote TBK1-mediated antiviral immunity (Song et al., 2016). However, receptor tyrosine kinase human epidermal growth factor receptor 2 (HER2) strongly interacts with STING and recruits protein kinase B1 (AKT1) to phosphorylate TBK1 at Ser511, a crucial step for disrupting the TBK1-STING interaction and K63-linked ubiquitination of TBK1, attenuating STING signaling (Wu et al., 2019). Finally, activation of cGAS/STING signaling is regulated by numerous other ubiquitin-related enzymes (Table 1).
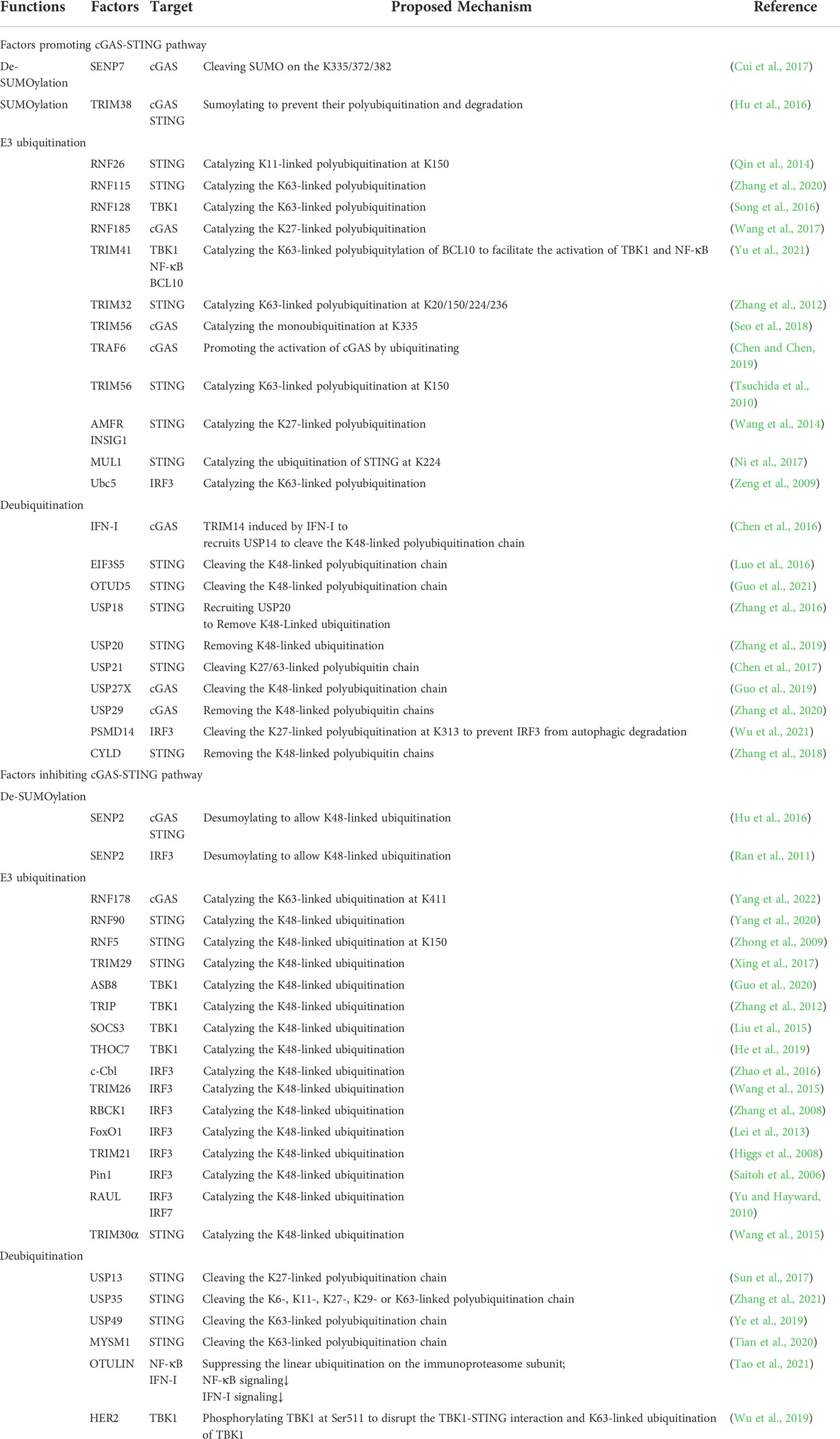
Table 1 The regulation of ubiquitination, SUMOylation and expression of key molecules in the cGAS-STING pathway.
3.2 Other regulation of cGAS/STING signaling
3.2.1 cGAS
In addition to the ubiquitination and sumoylation of cGAS, it has been found that β-arrestin 2 can strengthen the binding of dsDNA to cGAS to enhance cGAMP production by interacting with cGAS, which enhances STING-mediated innate immune responses (Zhang et al., 2020). The binding of DNA to cGAS can robustly induce the phase transition to liquidlike droplets, which function as microreactors in which the enzyme and reactants are highly concentrated to enhance the production of cGAMP. Furthermore, Zn2+ enhances the activity of cGAS by promoting cGAS-DNA phase separation (Du and Chen, 2018). Notably, liquid-liquid separation (LLPS) is a physicochemical process that governs the formation of membraneless condensates, allowing macromolecules, such as proteins and nucleic acids, to condense into dense phases (Peng et al., 2021). The LLPS formed between DNA and cGAS is beneficial to the combination of cGAS and DNA, in which this process is positively regulated by GTPase-activating protein-(SH3 domain)-binding protein 1 (G3BP1). It has been found that G3BP1 engages cGAS in a condensed state, which promotes DNA-induced LLPS and activation of cGAS (Zhao et al., 2021). In addition, recent studies have found that the nuclear-localized heterogeneous nuclear ribonucleoprotein A2B1 (hnRNPA2B1) senses viral DNA and is then translocated into the cytoplasm to activate the TBK1-mediated antiviral signaling. Moreover, hnRNPA2B1 promotes N6-methyladenosine (m6A) modification and nucleocytoplasmic trafficking of cGAS, IFI16, and STING mRNAs, which further potentiate cytoplasmic TBK1 and IRF3 activation mediated by these factors (Wang et al., 2019). Notably, cGAS is also negatively regulated by other DNA sensors. For example, DNA-dependent protein kinase (DNA-PK), a PRR located in the cytoplasm, is a heterotrimeric complex composed of Ku70/Ku80 heterodimers and the catalytic subunit DNA- pkcs, which is important in the innate immune response to intracellular DNA and DNA virus infection. It has been found that human DNA-PK activates the STING-independent DNA sensing pathway (SIDSP) to trigger heat shock protein A8 (HSPA8) and IRF3 phosphorylation, which drives a robust and broad antiviral response. However, this process is not present in mouse cells (Burleigh et al., 2020), which also suggests a large difference between innate immune signaling between species. Notably, DNA-PK negatively regulates cGAS/STING signaling in both humans and mice. It has been found that DNA-PK phosphorylates cGAS and suppresses its enzymatic activity to decrease cGAMP synthesis. Moreover, DNA-PK deficiency in mice potentiates cGAS-mediated antiviral innate immunity and restricts viral replication (Sun et al., 2020). Similarly, the activation of DNA-PK in human cells can also inhibit the activation of cGAS/STING signaling. Poly(ADP-ribose) polymerase 1 (PARP1), a critical nuclear sensor of DNA damage, maintains genomic integrity. Notably, PARP1 is also engaged in the regulation of host innate immunity. More recently, studies have revealed that activated DNA-PK in HeLa cells phosphorylates PARP1 at Thr594, thus triggering the cytoplasmic translocation of PARP1. Then, cytosolic PARP1 directly interacts with and PARylates cGAS at Asp191, which impairs its DNA-binding ability and inhibits antiviral immunity (Wang et al., 2022). The palmitoyltransferase ZDHHC18 catalyzes the palmitoylation of cGAS at C474 to restrict the interaction between cGAS and DNA (Shi et al., 2022). The glutamylation and deglutamylation of cGAS are also intimately related to its activity. Tubulin tyrosine ligase-like 6 (TTLL6)-mediated polyglutamylation of cGAS also impedes its DNA-binding ability, and TTLL4-mediated monoglutamylation of cGAS blocks its synthase activity. Conversely, cytosolic carboxypeptidase CCP6 and CCP5 can remove cGAS polyglutamylation and cGAS monoglutamylation, respectively, restoring cGAS activation (Xia et al., 2016).
Except for the previously mentioned DNA-PK negative regulation of cGAS/STING signaling by targeting cGAS, the DNA sensor AIM2 also curbs cGAS-driven innate immunity. K+ efflux was found to be sufficient to inhibit cGAS-dependent IFN-β responses, but gasdermin D activated by AIM2 inflammasomes restrains cGAS-driven IFN-β production by promoting cellular K+ efflux (Banerjee et al., 2018). These findings imply that activation of other DNA receptors can inhibit cGAS-mediated antiviral immunity, and its significance may lie in avoiding the excessive activation of its innate immune signals. Additionally, miR-23a/b dampens cGAS-mediated innate immunity and autoimmunity by targeting cGAS (Yu et al., 2021). Furthermore, immune-related GTPase M (IRGM) interacts with cGAS and RIG-I to mediate their p62-dependent autophagy degradation. Moreover, it also promotes mitophagy to decrease the accumulation of mitochondrial DAMPs, which restrains interferon signaling (Jena et al., 2020).
3.2.2 STING
Tri-methylation on histone H3 lysine 4 (H3K4me3) strongly correlates with active transcription. However, histone H3K4 lysine demethylases KDM5 suppresses the expression of STING by binding to the promoter of STING and maintaining a low level of H3K4me3, thereby inhibiting cGAS/STING-mediated innate immune response (Wu et al., 2018). cGAMP binds to and activates the adaptor protein STING, leading to IFN-I production and antiviral response. However, the activation of STING is derived not only from the production of intracellular cGAMP but also from the import of extracellular cGAMP. Firstly, cGAMP produced upon HSV-1 infection can be transported to bystander cells via the LRRC8A/LRRC8E-containing volume-regulated anion channels (VRACs) to contribute to antiviral IFN response (Zhou et al., 2020). Secondly, the transport of extracellular cGAMP into cells is also dependent on the reduced folate carrier solute carrier family 19 member 1(SLC19A1) (Luteijn et al., 2020). Thirdly, recent studies have found that SLC46A2 is the dominant cGAMP importer in human monocyte-derived macrophages (Cordova et al., 2021). However, the ectonucleotidase ENPP1 can selectively hydrolyze extracellular cGAMP (Li et al., 2021). Therefore, antiviral immune responses can be significantly enhanced by targeting extracellular ENPP1 expression or enhancing the levels of these cGAMP transporters upon viral infection. Surprisingly, recent studies have found that plasmatic membrane STING (pmSTING), an alternatively spliced STING isoform, directly senses extracellular cGAMP to mediate TBK1-mediated antiviral immunity (Li et al., 2022). Furthermore, STING restricts HSV-1 infection independently of IFN signaling (Wu et al., 2020), although the exact mechanism remains unclear. These findings delineate that STING plays a central role in the antiviral immune response. Promoting STING expression and its activation may be the key to defense against viral infection.
The transport of STING from the ER to the Golgi apparatus and perinuclear microsome is critical for its activation of downstream signaling. This process is also positively regulated by many factors. Studies have found that YIPF5, a member of the Yip family (YIPF), recruits STING to coat protein complex II (COPII)-coated vesicles, which facilitates STING trafficking from the ER to the Golgi apparatus (Ran et al., 2019). In addition, the transmembrane emp24 protein transport domain containing 2 (TMED2) interacts with STING to potentiate its activation by reinforcing its dimerization and facilitating its trafficking from the ER to the perinuclear microsome (Sun et al., 2018). Moreover, iRhom2 recruits the translocon-associated protein TRAP-β into the STING complex to facilitate the trafficking of STING from the ER to the perinuclear microsome (Luo et al., 2016). Notably, glutathione peroxidase 4 (GPX4), which maintains redox homeostasis of lipids, is critical for promoting the Golgi translocation of STING and activation of STING signaling. It was found that GPX4 deficiency increases the production of lipid peroxidation, leading to the STING carbonylation at C88 and inhibiting its transport from the ER to the Golgi complex (Jia et al., 2020). Furthermore, transmembrane protein 120A (TMEM120A), a newly discovered antiviral factor, interacts with STING to promote the translocation of STING from the ER to the Golgi apparatus (Li et al., 2022). There are likewise negative regulators in this process. It has been found that mytubin-related protein (MTMR) 3 and MTMR4, members of protein tyrosine phosphatases, disrupt the transport of STING from ER to Golgi apparatus by the production of phosphatidylinositol 3-phosphate (PtdIns3P) (Dewi et al., 2019). In addition, Ca2+ sensor stromal interaction molecule 1 (STIM1) can interact with STING to retain it in the ER membrane (Srikanth et al., 2019). Notably, excessive intracellular accumulation of cGAMP in cells triggers phase separation of ER-associated STING to form the condensate with organized membranous structures, which insulates STING-TBK1 from IRF3, thus preventing overactivation of innate immunity (Yu et al., 2021).
More recently, it was also found that the epidermal growth factor receptor (EGFR) binds STING and mediates its Tyr245 phosphorylation, which is required for IRF3 activation (Wang et al., 2020), suggesting that the phosphorylation of Tyr245 of STING is the key to its activation of downstream antiviral signals. However, many other factors negatively regulate the activity of STING. For example, the protein phosphatase 6 catalytic subunit (PPP6C) negatively regulates the cGAS-STING pathway by removing STING phosphorylation, which reduces IRF3 activation but not NF-κB activation (Ni et al., 2020). Furthermore, PPM1G, a protein phosphatase, can also dephosphorylate STING and MAVS to inhibit STING- and MAVS-mediated IFNβ production (Yu et al., 2020). Current studies have found multiple autophagy-lysosomal degradation pathways of STING, whose significance may be in avoiding excessive activation of STING signaling. For example, the lysosomal membrane protein Niemann-Pick type C1 (NPC1) interacts with STING and recruits it to the lysosome for degradation (Chu et al., 2021). Phosphorylation of S366 of STING favors the activation of downstream signaling. However, cyclic dinucleotides (CDNs) can initially activate the STING function. They subsequently trigger the phosphorylation of STING at S366 to inhibit the activity of IRF3 and subsequent lysosomal degradation of STING, which forms a negative-feedback control of STING activity to avoid the inflammatory disorder (Konno et al., 2013). Thus, phosphorylation of STING S366 is a hallmark of cGAS/STING signaling activation and subsequent degradation. In addition to inducing phosphorylation of STING to activate downstream signaling, TBK1 activated by DNA sensing also phosphorylates the selective autophagy receptor p62, which promotes autophagic degradation of STING and exerts negative control of the cGAS/STING pathway (Prabakaran et al., 2018). Thus, P62 exerts negative control of innate responses to DNA virus infection. These studies suggest that targeting these factors that negatively regulate STING are the potential targets for antipathogen treatment.
3.2.3 TBK1
Activation of TBK1 can further strengthen the antiviral immune response. TBK1 can activate methyltransferase-like 3 (METTL3), the core m6A methyltransferase, via two pathways to enhance antiviral signaling. On the one hand, TBK1 phosphorylates METTL3 at serine 67 to facilitate the interaction of METTL3 with the translational complex, which is required for enhancing protein translation, thus strengthening antiviral responses. On the other hand, TBK1 can promote the METTL3-mediated m6A modification to protect IRF3 mRNA from degradation. Furthermore, induction of IFN-I is severely impaired in METTL3-deficient cells (Chen et al., 2022), suggesting that TBK1-mediated METTL3 activation is vital in antiviral immunity. The protein arginine methyl transferase 1 (PRMT1), a type I protein arginine methyltransferase, catalyzes asymmetric methylation of TBK1 to enhance TBK1 oligomerization and phosphorylation, strengthening IFN-I production (Yan et al., 2021). However, the phosphatase Cdc25A interacts with TBK1 and decreases the phosphorylation of TBK1, which reduces the IRF3-mediated generation of IFN-I (Qi et al., 2018). In addition, the neuropeptide hormone kisspeptin secreted by the hypothalamus and pituitary gland binds to G-protein coupled receptor 54 (GPR54) to recruit calcineurin and increase its phosphatase activity, which dephosphorylates TBK1 to restrict antiviral innate immune response (Huang et al., 2018). It has been observed that lncRNA-GM can inhibit the S-glutathionylation of TBK1 to enhance the activity of TBK1 and the downstream production of antiviral mediators (Wang et al., 2020). Furthermore, S-nitrosoglutathione reductase (GSNOR) enhances the TBK1 activity and promotes innate immunity by restricting TBK1 cysteine S-nitrosation at the Cys423 (Liu et al., 2021). Therefore, enhancing the expression of TBK1-related activators and targeting related repressors may be the key to the future treatment of viral infections.
3.2.4 IRF3 and IFN-I
The downstream signaling of the cGAS/STING pathway can, in turn, enhance cGAS/STING signaling and antiviral immune response. For example, the IFN-I can further activate IRF3. Mechanistically, in resting cells, IRF3 is bound to suppressor protein Flightless-1 (Fli-1), which keeps its inactive state. Upon infection, lncRNA-ISIR, an IRF3-binding lncRNA, is induced by IFN-I, which associates with IRF3 to prevent Fli-1 binding with IRF3, thus facilitating IRF3 activation (Xu et al., 2021). Notably, the activation of cGAS can activate IRF3 by non-canonical pathways. It was found that nuclear cGAS also recruits protein arginine methyltransferase 5 (PRMT5) to catalyze the symmetric dimethylation of histone H3 arginine 2 at Ifnb and Ifna4 promoters, which facilitates IRF3 to perform its transcription functions, enhancing the innate antiviral immunity (Cui et al., 2020). Furthermore, CREB-binding protein (CBP) is a histone acetyltransferase, which plays a crucial role in transcriptional regulation. It interacts with IRF3 to enhance cGAS/STING-mediated IFN-β production (Lu et al., 2021). However, IRF3 acetylation can reduce its transcriptional activity. It has been found that lysine acetyltransferase 8 (KAT8) interacts with IRF3 and mediates IRF3 acetylation at K359 to suppress the transcriptional activity of IRF3 (Huai et al., 2019). Moreover, the activated caspase-3 can cleave cGAS, mitochondrial antiviral signaling protein (MAVS), and IRF3 to prevent the production of IFN-I during virus infection-caused apoptosis (Ning et al., 2019).
Numerous factors promote downstream IFN-I signaling (Figure 1). Firstly, TRIM6 and the E2 conjugase UbE2K synthesize unanchored K48-linked ubiquitin chains, stimulating IKKϵ for subsequent signal transducer and activator of transcription 1 (STAT1) phosphorylation and IFN-I signaling (Rajsbaum et al., 2014). Meanwhile, VAMP8, a vesicle-associated-membrane protein, is a novel IFN-I signaling regulatory protein whose expression and function depend on the activity of TRIM6 (van Tol et al., 2020). Hence, TRIM6 is critical for the activation of IFN-I signaling. Secondly, miR-101 significantly strengthens IFN-I production and IFN-I-mediated antiviral innate defense to suppress the replication of feline herpesvirus 1 (FHV-1) by targeting the suppressor of cytokine signaling factor 5 (SOCS5), a negative regulator of the JAK-STAT pathway (Zhang et al., 2020). Thirdly, β-catenin facilitates activation of the cGAS/STING signaling pathway by translocating to the nucleus and then up-regulating the IFN-β promoter activity. However, HSV-1 US3 promotes the hyperphosphorylation of β-catenin at Thr556 to block its nuclear translocation, which subverts innate antiviral immunity (You et al., 2020). Finally, the RNA binding protein RBM47, an ISG, can be up-regulated by IFN-I stimulation. It has been found that RBM47 binds to the 3’UTR of IFNAR1 mRNA to increase mRNA stability, which retards the degradation of IFNAR1 and potentiates innate immunity (Wang et al., 2021).
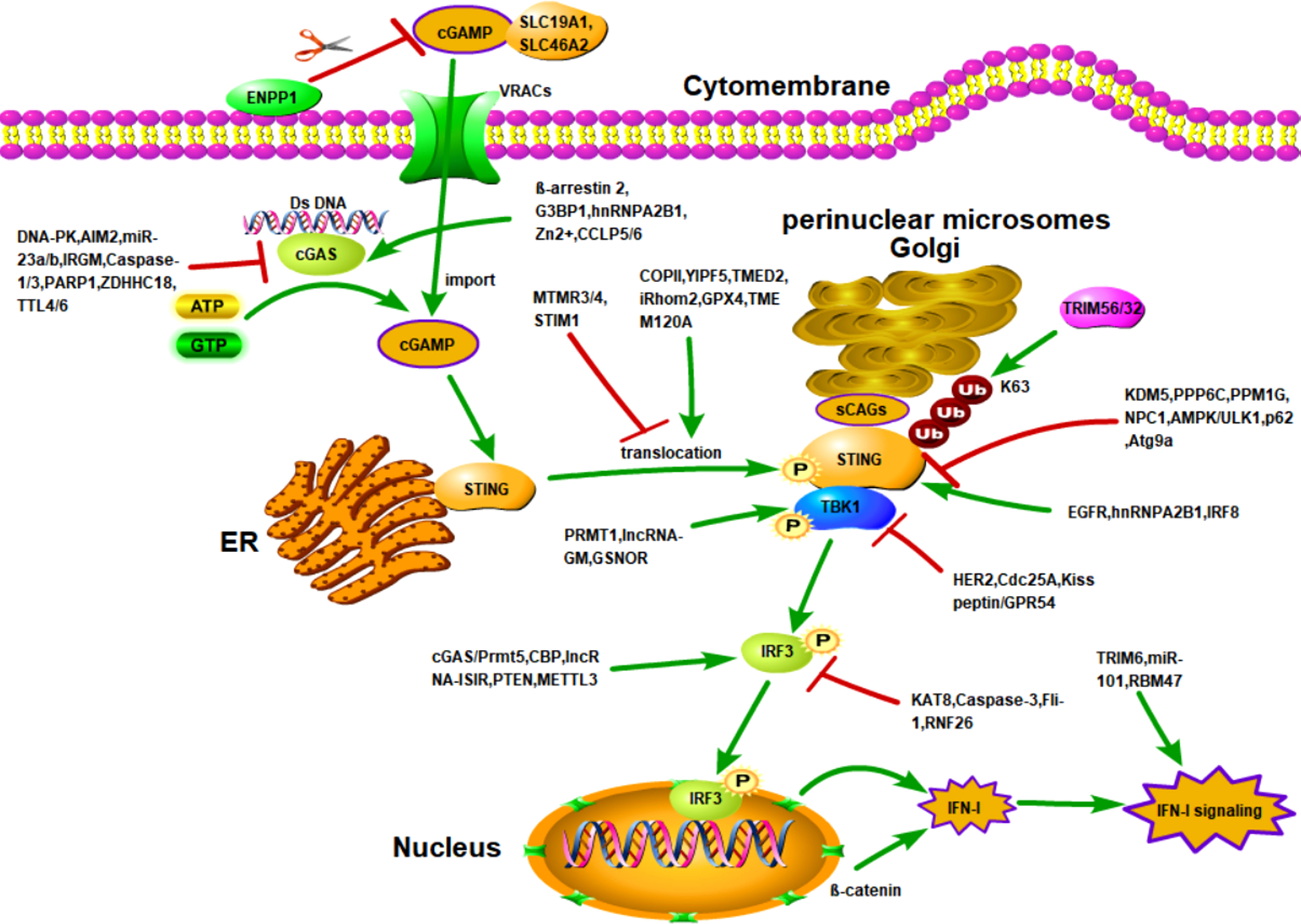
Figure 1 Activation of cGAS/STING signaling and regulation of cGAS/STING signaling by other molecules. Activation of STING is dependent on the presence of intracellular cGAMP. There are two sources of intracellular cGAMP. First, upon binding to DNA, cGAS is activated to catalyze the synthesis of intracellular cGAMP. Second, extracellular cGAMP is transported into the cell through importers. After cGAMP binds to STING, STING is translocated to the Golgi apparatus to bind sCAGs and is linked to the K63-linked ubiquitination by TRIM32 and TIRM56, which induces STING to recruit TBK1 and activate IRF3-induced IFN-I production. The cGAS/STING cascade is controlled by various factors. For example, DNA-PK, AIM2, miR-23a/b, etc. reduce the activity and expression of cGAS through different mechanisms. However, G3BP1, β-arrestin 2, and hnRNPA2B1 enhance the activity and expression of cGAS through different mechanisms. In addition, the entry of extracellular cGAMP into cells is affected by the trafficking of importers SLC19A1 and SLC46A2, as well as by VRACs and ENPP1. Numerous molecules also regulate the translocation of STING. For example, COPII, YIPF5i, Rhom2, etc., enhance the transport of STING from the ER to the Golgi and perinuclear microsomes. However, MTMR3/4 and STIM1 inhibited the translocation of STING. In addition, the activation of STING are also positively and negatively regulated by various factors, such as KDM5, PPP6C, and EGFR.
4 The hijacking of cGAS/STING signaling by viruses
Based on the above results, the cGAS-STING signal transduction is very complicated, in which the process is based on various factors. However, the virus has also evolved numerous strategies to suppress the cGAS/STING signaling (Table 2). cGAS can induce the activation of downstream signals by recognizing DNA. Therefore, DNA virus infection will cause its DNA fragments to be recognized by cGAS, which promotes activation of the cGAS/STING signaling pathway. Unsurprisingly, DNA viruses have also evolved various mechanisms to counteract the host cGAS/STING pathway. However, surprisingly, RNA virus infection can also trigger cGAS-mediated innate immunity. For example, dengue virus (DENV) and west nile virus (WNV) also initiate cGAS-mediated innate immunity in mitochondrial DNA (mtDNA)-dependent manner (Schoggins et al., 2014; Aguirre et al., 2017). Recent studies have also found that mitochondrial stress caused by measles virus (MeV) infection induces increased cytosolic mtDNA into the cytosol, where mtDNA is captured by cGAS and causes consequent priming of the DNA sensing pathway (Sato et al., 2021). Moreover, activation of cGAS relies on its recognition of cytoplasmic chromatin DNA shuttled from the nucleus as a result of cell-to-cell fusion upon SARS-CoV-2 infection (Zhou et al., 2021). Thus, the activation of cGAS triggered by RNA viruses not only depends on the release of mtDNA but also on chromosomal DNA. Similarly, RNA viruses also utilize multiple strategies to escape cGAS/STING signaling. For example, although the influenza A virus M2 protein triggers mtDNA translocation into the cytosol and mediates the antiviral immune response, the nonstructural protein 1 (NS1) of influenza A virus associates with mtDNA to evade STING-dependent antiviral immunity (Moriyama et al., 2019).
4.1 DNA viruses
4.1.1 Herpesviridae
Since cGAS activates the STING-dependent antiviral immunity by capturing DNA, various DNA viruses trigger cGAS/STING signaling, including herpes virus, vaccinia virus, and adenovirus (Lam et al., 2014; Dai et al., 2014; Wu et al., 2015). Notably, Herpesviridae family viruses can directly inhibit the activation of cGAS/STING signaling through their tegument proteins (Table 2). For example, the HSV-1 UL37 tegument protein deamidates cGAS, which decreases the ability of cGAS to catalyze cGAMP synthesis, contributing to virus replication (Zhang et al., 2018). It was recently found that the LLPS formed by cGAS-DNA facilitates the interaction between cGAS and STING and activation of innate immune signaling. However, γ-herpesvirus ORF52 and α-herpesvirus VP22 disrupt the LLPS to alter the innate immune response in favor of viral replication (Xu et al., 2021). Similarly, human cytomegalovirus (HCMV) protein UL31 also directly interacts with cGAS to disassociate DNA from cGAS, suppressing the cGAS enzymatic functions and cGAMP production (Huang et al., 2018). In addition, HSV-1 tegument protein UL41 abrogates the cGAS/STING-mediated DNA sensing pathway by selectively degrading cGAS mRNA to downregulate the expression of cGAS through its RNase activity (Su and Zheng, 2017). Furthermore, the HCMV tegument protein pp65 (pUL83) selectively binds to cGAS and inhibits its enzymatic activity (Biolatti et al., 2018). It has been mentioned above that the functional activity of cGAS is regulated by numerous factors. Therefore, viruses likely affect the function of cGAS through these pathways. Indeed, HSV-1 infection induces host cell DNA damage that promotes DNA-PK-dependent inhibition of cGAS activity (Wang et al., 2022). However, whether Herpesviridae inhibits cGAS activity through other related regulatory factors remains unclear.
Of note, many studies have proved that viruses of the herpesvirus family can inhibit the activation of STING through the above approach. Firstly, the expression of MPN domains 1 (MYSM1) is induced upon DNA virus infection. MYSM1 subsequently interacts with STING and cleaves STING K63-linked ubiquitination to suppress cGAS-STING signaling (Tian et al., 2020). Secondly, Kaposi’s sarcoma-associated herpesvirus (KSHV) ORF48 interacts with PPP6C to inhibit STING-dependent IFN-I production by removing the phosphorylation of STING (Ni et al., 2020). In addition, KSHV ORF33 interacts with STING/MAVS and enhances the recruitment of PPM1G to dephosphorylate STING/MAVS, restricting innate immune signaling (Yu et al., 2020). Furthermore, Epstein-Barr virus (EBV), a γ herpes virus, induces TRIM29 to mediate K48-linked ubiquitination of STING for its rapid degradation (Xing et al., 2017). Finally, HSV-1 reduces the expression of TRIM32 and TRIM41 (Yu et al., 2021), suggesting that HSV-1 can inhibit the activation of STING and TBK1 and negatively regulate innate immunity.
Viruses of the herpesvirus family can suppress the activation of TBK1 and IRF3 through the above approach. For example, HSV-1 infection promotes TBK1 S-nitrosation to suppress the innate immune response by inducing reactive nitrogen species (RNS) (Liu et al., 2021), which means that the virus directly utilizes the S-nitrosation of TBK1 to inactivate TBK1. Given that GSNOR inhibits s-nitrosation of TBK1 to enhance innate immunity, it is still unclear whether viral infections can hijack GSNOR to antagonize antiviral immunity. Moreover, pseudorabies virus (PRV) glycoprotein E (gE) degrades CBP to dampen cGAS/STING-mediated IFN-β production (Lu et al., 2021). In addition, HSV-1 VP16 and porcine reproductive and respiratory syndrome virus (PRRSV) nonstructural protein 1 α (nsp1α) can inhibit IFN-I production by interfering with the recruitment of CBP to IRF3 and degrading CBP, respectively (Xing et al., 2013; Han and Yoo, 2014). Thus, CBP may be a common target of herpes viruses, and how to prevent the activity of CBP from being suppressed by the virus may be an essential strategy to fight against herpes virus infections.
Notably, KSHV ORF50 mediates RAUL deubiquitination via USP7, thus decreasing the expression of IRF3 and IRF7 (Yu and Hayward, 2010). In addition, the herpes virus can directly inhibit the activation of TBK1 and IRF3 to suppress innate antiviral immunity. For example, EBV lytic infection suppresses IFN-β production. Mechanistically, EBV lytic transactivator Zta decreases the phosphorylation of TBK1 and IRF3 to block the nuclear translocation of IRF3 (Wong et al., 2017). Notably, IRF7 also plays a vital role in the innate immunity mediated by cGAS/STING. However, it has been observed that Marek’s disease virus (MDV) VP23 interacts with IRF7 to block its binding to TBK1, which inhibits phosphorylation and nuclear translocation of IRF7 and cGAS/STING-induced IFN-β production (Gao et al., 2019). Importantly, activation of NF-κB signaling is likewise essential for IFN-I production. However, MDV RLORF4 interacts with NF-κB subunits p65 and p50 to inhibit cGAS-STING-mediated NF-κB activation, thereby reducing IFN-β production (Liu et al., 2019).
In addition, HSV-1 protein ICP0, an E3 ubiquitin ligase, blocks the innate immune response induced by DNA-PK (Burleigh et al., 2020). However, the viral protein ICP0 does not appear to suppress innate immunity. It was found that STING is not degraded in wild-type HSV-1-infected cancer-derived HEp-2 or HeLa cells, but it is degraded in cells infected with ICP0 deletion mutant of HSV-1 (Kalamvoki and Roizman, 2014). However, in human embryonic lung cells, STING is not degraded after infection with either wild-type or ICP0 mutant viruses (Kalamvoki and Roizman, 2014). Hence, whether HSV-1 causes the degradation of STING may depend on cell type and context. Furthermore, HSV-1 infection results in a marked decrease in IFI16 protein levels in normal human foreskin fibroblasts (HFFs), normal oral keratinocytes (NOKs), and HeLa cells, but not in U2OS cells (Orzalli et al., 2016), suggesting that whether HSV-1 causes the degradation of IFI16 is also intimately related to cell type.
4.1.2 Hepadnaviridae
Hepatitis B virus (HBV) infection significantly reduces host cell IRF3 expression (Shravanthi et al., 2015). Moreover, HBV polymerase interacts with STING and disrupts the k63-linked ubiquitination of STING through its reverse transcriptase (RT) domains, suppressing IRF3 activation and IFN-β induction (Liu et al., 2014). Furthermore, as a tumor suppressor, phosphatase and tensin homolog (PTEN) is also involved in regulating innate immunity. It dephosphorylates IRF-3 at the Ser97 site to strengthen IRF-3 nuclear translocation, which enhances the IFN signaling pathway. However, HBV significantly increases m6A modification of PTEN mRNA, which contributes to its instability with a corresponding decrease in PTEN protein levels, thus blocking the IRF3 nuclear import and IFN signaling pathway (Kim et al., 2021).
4.1.3 Papillomaviridae and adenoviridae
Both human papillomavirus (HPV) and adenovirus can directly target STING to suppress activation of the cGAS/STING pathway. It has been demonstrated that HPV E7 and adenovirus E1A antagonize the cGAS-STING DNA-sensing pathway by interacting with STING. Further, the LXCXE motif of E7 and E1A is crucial for the blockade of the cGAS/STING signaling (Lau et al., 2015), which suggests that targeting the LXCXE motif is one of the strategies against HPV and adenovirus. Moreover, HPV E7 decreases the IFI16 expression by recruiting TRIM21, evading immune surveillance (Song et al., 2020). Furthermore, deletion of HPV E7 restores the cGAS-STING response (Bortnik et al., 2021). Thus, targeting the HPV viral E7 protein is among the critical approaches to treating HPV infection. Notably, adenovirus E1A can directly engage with STAT1 to block STAT1-dependent gene activation (Look et al., 1998). Therefore, adenovirus E1A can inhibit not only cGAS/STING signaling but also IFN-I-mediated antiviral signaling to escape innate immunity. In addition, human adenovirus E1A blocks the antiviral signaling pathway triggered by DNA-PK (Burleigh et al., 2020). However, one study found that knockdown of either cGAS or STING in Hela cells did not affect adenoviral DNA replication (Lam and Falck-Pedersen, 2014), suggesting that adenovirus replication may be independent of activating cGAS/STING signaling, and the mechanism remains unclear.
4.1.4 Poxviridae
The activated caspase-3 cleaves cGAS and IRF3, a process used by vaccinia virus (VACV) to suppress innate immunity (Ning et al., 2019). Notably, DNA-PK also binds to DNA to induce innate immunity independent of STING. However, VACV C16 directly binds to the Ku heterodimer to block DNA-PK binding to DNA, hampering DNA-PK-dependent DNA sensing (Peters et al., 2013). Moreover, VACV C4 has also been shown to antagonize DNA-PK by binding to Ku and blocking the binding of Ku to DNA, which induces the diminished production of cytokines and chemokines (Scutts et al., 2018). In addition, VACV F17 suppresses ISG responses by dysregulating the host kinase mammalian target of rapamycin (mTOR) (Meade et al., 2019), suggesting that in addition to NF-κB signaling, mTOR signaling is also critical in innate immune responses. These results also show that VACV can suppress innate immunity in various ways. Blocking these pathways is also an essential strategy in treating VACV infection. Furthermore, cowpox virus (CPXV) and ectromelia virus (ECTV) infection prevent STING phosphorylation and dimerization (Georgana et al., 2018), but the specific mechanism remains unclear.
4.2 RNA viruses
4.2.1 Flaviviridae
Many viruses in the Flaviviridae family directly target critical factors in the cGAS/STING pathway to suppress innate immunity (Table 2). Firstly, the DENV NS2B protein targets cGAS for degradation to avoid the detection of mtDNA upon infection (Aguirre et al., 2017). Secondly, Zika virus (ZIKV) infection triggers NLRP3 inflammasome activation, in which the process is further enhanced by ZIKV NS1 to facilitate its replication. Further, NS1 recruits the deubiquitinase USP8 to cleave K11-linked poly-ubiquitin chains from caspase-1, preventing the proteasomal degradation of caspase-1. Thus, the caspase-1 stabilized by NS1 strengthens the cleavage of cGAS, which attenuates cGAS-mediated IFN-I production and facilitates the immune escape of ZIKV (Zheng et al., 2018). Based on the above results, these suggest that NLRP3 inflammasome activation suppresses cGAS-mediated type I IFN signaling by stabilizing caspase-1, but this process is utilized by ZIKV NS1.
4.2.2 Coronaviridae
SARS-CoV-2 ORF9b interacts with STING and TBK1 to impede the IRF3 phosphorylation and nuclear translocation, thus negatively regulating antiviral immunity and facilitating viral replication (Han et al., 2021). Moreover, both ORF3a and 3CL of SARS-CoV-2 can inhibit the activation of STING signaling. Mechanistically, ORF3a uniquely binds STING, blocking the nuclear accumulation of p65 and inhibiting the NF-κB signaling pathway. SARS-CoV-2 3CL disrupts k63-linked ubiquitination of STING and blocks the STING-dependent downstream signaling (Rui et al., 2021). Human coronavirus (HCoV) NL63 (the membrane-anchored PLP domain, PLP2-TM) has been shown to interact with STING to inhibit STING dimer formation, which is not dependent on PLP2-TM’s deubiquitinase (DUB) activity (Sun et al., 2012). Moreover, PLP2-TM attenuates the K63-linked ubiquitination of STING, which is not dependent on PLP2-TM’s DUB activity (Clementz et al., 2010), suggesting that the interaction of PLP2-TM with STING may be the key to decreasing the K63-linked ubiquitination of STING. However, porcine epidemic diarrhea virus (PEDV) papain-like protease 2 (PLP2) has been reported to repress the K63-linked ubiquitination of STING through its DUB activity (Xing et al., 2013). Therefore, the differences between human and porcine viruses deserve further clarification. PEDV PLP1 interacts with host cell poly(C)-binding protein 2 (PCBP2) to decrease the production of IFN-I and promote the replication of PEDV (Zhang et al., 2020). However, whether this process is associated with inhibition of cGAS/STING signaling remains elusive. In addition, the SARS-CoV membrane-anchored PLpro domain (PLpro-TM) has been reported to inhibit the K63-linked ubiquitination of STING and STING-mediated activation of downstream signaling by associating with STING (Chen et al., 2014). These findings indicate that the papain-like protease domain may be the key to the suppression of innate immune responses by the Coronaviridae family. Notably, it appears that both the viral nucleocapsid (N) and membrane (M) proteins of the Coronaviridae target TBK1. PEDV-encoded nucleocapsid (N) protein has also been shown to interact with TBK1 and sequester the association between TBK1 and IRF3 (Ding et al., 2014). SARS-CoV-2 M protein interacts with TBK1 and induces TBK1 degradation via K48-linked ubiquitination (Sui et al., 2021). Furthermore, the M proteins of Middle East respiratory syndrome coronavirus (MERS-CoV) and SARS-CoV have three highly similar conserved N-terminal transmembrane domains and a C-terminal region. Further studies found that the N-terminal transmembrane domain of the MERS-CoV M protein is sufficient to inhibit IFN-I expression, but not the C-terminal domain (Lui et al., 2016), suggesting that the N-terminal transmembrane domain of M protein may primarily involve in the interaction with TBK1 to induce its degradation process. Notably, although IFN-I limits viral reproduction in the early stage of viral infection, a sustained increase in the levels of IFN-I in the late stage of infection is associated with aberrant inflammation and poor clinical outcome. It has been demonstrated that SARS-CoV-2 infection activates cGAS/STING signaling to promote the IFN-I production in endothelial cells through mtDNA release. Inhibition of STING attenuates severe SARS-CoV-2-induced lung inflammation and improves disease outcomes (Domizio et al., 2022). Thus, the activation of cGAS/STING signaling plays a more detrimental role in the severe inflammatory response at the late stage of a viral infection than the beneficial effect of limiting viral replication.
4.2.3 Picornaviridae
The lead protease (Lpro) of foot-and-mouth disease virus (FMDV) can interfere with cGAS/STING signaling through various strategies. Firstly, the Lpro of FMDV cleaves TBK1 to decrease the IFN-I production (Visser et al., 2020). Secondly, the FMDV Lpro also directly reduces the protein expression of IRF-3/7 (Wang et al., 2010). Thirdly, the Lpro of FMDV translocates to the nucleus to stimulate the degradation of nuclear p65, antagonizing the innate immune and inflammatory responses (De Los Santos et al., 2007). Therefore, targeting the Lpro of FMDV may be one of the strategies for treating FMDV infection. In addition, the L protein of mengovirus, a strain of encephalomyocarditis virus, suppresses the antiviral response by inhibiting the transcription of the IFN-I gene (Hato et al., 2010).
4.2.4 Rhabdoviridae
It has been demonstrated that vesicular stomatitis virus (VSV) utilizes a variety of cGAS/STING signal-related regulatory factors to suppress cGAS/STING signaling. First, VSV negatively regulates cGAS-mediated innate immunity by significantly reducing the expression of E3 ubiquitin ligases TRIM32 and TRIM41 (Yu et al., 2021). Secondly, the increased kisspeptin secreted by the hypothalamus and pituitary gland during VSV infection restricts the innate immune response by GPR54/calcineurin axis (Huang et al., 2018). Moreover, VSV infection promotes the degradation of β-arrestin 2 to facilitate immune evasion (Zhang et al., 2020). Furthermore, VSV infection also down-regulates the expression of lncRNA-GM to facilitate VSV escape by disrupting TBK1 activity (Wang et al., 2020). Finally, VSV infection promotes the nuclear translocation of TRIM26 to degrade IRF3 (Wang et al., 2015). It is worth noting that the viral protein on the surface of VSV has not been found to inhibit the cGAS/STING signaling directly, and further exploration is necessary.
4.2.5 Retroviridae
Sensing of HIV and other retroviruses relies on viral reverse transcription and the cellular DNA sensor GAS (Gao et al., 2013). However, studies have shown that THP-1 cells do not lead to significant innate immune induction to HIV-1 infection (Cingoz and Goff, 2019). Mechanistically, the HIV-1 capsid protects viral DNA from the cGAS DNA sensing (Sumner et al., 2020). In addition, NLRX1 interacts with STING to prevent STING-TBK1 interaction and suppress innate immunity (Guo et al., 2016). This function seems to be fully exploited by HIV-1 and simian immunodeficiency virus (SIV) (Guo et al., 2016; Barouch et al., 2016). HIV-2/SIV Vpx can also target STING to suppress cGAS-STING-mediated NF-kB signaling, impeding the induction of innate immune genes (Su et al., 2019). These also suggest that retroviruses can inhibit cGAS/STING signaling through multiple pathways.
4.2.6 Others
The leucine-rich repeat-containing protein 10B(LRRC10B) up-regulated upon Sendai virus (SeV) infection participates in the formation and interaction with ankyrin repeat and SOCS box-containing 8 (ASB8)-TBK1/IKKϵ complex and negatively regulates IFN-I production by the degradation of TBK1 (Guo et al., 2020). In addition, ASB8 also interacts with PRRSV Nsp1α to promote K63-linked ubiquitination and increase the stability of Nsp1α, which facilitates the degradation of CBP to inhibit the production of IFN-I. Moreover, ASB8 also facilitates K48-linked ubiquitination and degradation of IKKβ, consequently suppressing NF-κB signaling (Han and Yoo, 2014) (Li et al., 2019). Thus, targeting ASB8 is a new strategy to enhance innate immunity. In addition, SeV infection promotes TBK1 s-nitrosation to suppress the innate immune response by inducing RNS (Liu et al., 2021). Of note, SeV can indirectly mediate IRF3 degradation by regulating the ubiquitination of IRF3. For example, SeV infection promotes 26S proteasome non-ATPase regulatory subunit 14 (PSMD14) to disassociate with IRF3. Then IRF3 is conjugated with K27-linked ubiquitin chains, which mediates the IRF3 autophagic degradation in a nuclear dot protein 52 (NDP52)-dependent manner (Wu et al., 2021). Furthermore, SeV infection promotes the nuclear translocation of TRIM26 and up-regulates the RBCK1 expression to degrade IRF3 (Zhang et al., 2008; Wang et al., 2015).
In addition, Guertu virus (GTV) is a potentially highly pathogenic bunyavirus. It has been reported that GTV nonstructural protein (NSs) induces the formation of compact inclusion bodies (IBs) and extended filamentous structures (FSs). Then, GTV NSs interacts with TBK1 and STAT2 to sequester them into the viral IBs and FSs, which counteracts the host’s innate immunity (Min et al., 2020). Furthermore, Heartland virus (HRTV) is a pathogenic phlebovirus, whose NSs protein inhibits innate immunity by interacting with TBK1 to hinder the association of TBK1 with IRF3 (Ning et al., 2017). HRTV also antagonizes IFN antiviral signaling by diminishing both STAT2 and STAT1 phosphorylation. Moreover, decreased STAT2 activity is involved in HRTV NSs (Feng et al., 2019). However, the underlying mechanism of reduced STAT1 phosphorylation remains unclear. TRIM6 can activate the IKKϵ kinase to induce IFN-I responses (Rajsbaum et al., 2014). However, it has been found that Nipah Virus matrix protein (NiV-M) interacts with TRIM6 and suppresses TRIM6-mediated IFN-I signaling (Bharaj et al., 2016). Also, Ebola virus (EBOV) VP35 interacts with host TRIM6 to inhibit the TRIM6-mediated IFN-I induction (Bharaj et al., 2017). Thus, the virus can escape immune response by hijacking the antiviral effect of TRIM6.
5 Summary
cGAS/STING signaling plays an essential role in the process of innate antiviral immunity. Not only DNA virus infection but also RNA virus infection can initiate the activation of cGAS/STING signaling. RNA viruses can activate cGAS/STING signaling by increasing mtDNA and chromatin DNA in the cytoplasm. Notably, activation of DNA sensors DNA-PK and AIM2 can also suppress the activation of cGAS, which may be a negative feedback regulation mechanism formed by the immune system to avoid the excessive activation of its innate immune signals and prevent the occurrence of autoimmune diseases. In addition, cGAS and its mediated downstream antiviral signals are regulated by numerous factors. Numerous studies have revealed that most viral proteins directly interfere with cGAS/STING signal transduction by directly targeting essential proteins such as cGAS, STING, and TBK1. However, viruses can also indirectly inhibit the activation of cGAS/STING signaling through some related regulatory factors. For example, viral infection can cleave K63-linked ubiquitin chains from STING by increasing MYSM1 levels or enhancing the recruitment of PPM1G to dephosphorylate STING. Viral infection can also reduce PTEN expression to inhibit nuclear translocation of IRF-3. However, whether the virus can inhibit cGAS/STING signaling through various other factors remains unclear. The modulation of cGAS/STING signaling has been applied not only to the treatment of DNA virus infections but also to RNA virus infections. However, current therapeutic research for viral infections has concentrated on the use of broad-spectrum therapeutic approaches that activate cGAS/STING signaling. For example, topoisomerase II inhibitors trigger the DNA-sensing cGAS-STING pathway that restricts EBOV replication (Luthra et al., 2017). The STING agonist 5,6-dimethylxanthenone-4-acetic acid (DMXAA) can inhibit HBV replication in a chronic HBV mouse model (Li et al., 2022). In addition, diABZI, the potent non-CDN STING agonist, can restrict SARS-CoV-2 replication in human bronchial epithelial cells and mice, in which the process is IFN-I signaling dependent (Li et al., 2021). These studies suggest that the strategy of employing STING agonists during viral infection can, to some extent, combat viral infection. Moreover, PLpro is a tempting target for broad-spectrum anti-coronavirus drugs. F0213, a broad-spectrum anti-coronavirus PLpro inhibitor, can interact with SARS2-PLpro and MERS-PLpro to antagonize PLpro-mediated IFN suppression. F0213 effectively protects hamsters infected with SARS-CoV-2 and human DPP4-knockin mice infected with MERS-CoV (Yuan et al., 2022). Thus, targeting critical factors according to the strategy of viral inhibition of cGAS/STING may be able to restore hijacked cGAS/STING signaling better than directly activating STING signaling with STING agonists. Therefore, targeting these factors to reactivate cGAS/STING signaling for specific viral infections is a novel viral therapy strategy.
Author contributions
ZG is responsible for the retrieval of relevant documents and the writing of article content, and SD is responsible for the design of the overall framework of the article. All authors contributed to the article and approved the submitted version.
Funding
The present study was supported by the National Natural Science Foundation of China (grant no. 31671241) and the Scientific Research Start-up Fund for Young Teachers of Shenzhen University.
Conflict of interest
The authors declare that the research was conducted in the absence of any commercial or financial relationships that could be construed as a potential conflict of interest.
Publisher’s note
All claims expressed in this article are solely those of the authors and do not necessarily represent those of their affiliated organizations, or those of the publisher, the editors and the reviewers. Any product that may be evaluated in this article, or claim that may be made by its manufacturer, is not guaranteed or endorsed by the publisher.
References
Aguirre, S., Luthra, P., Sanchez-Aparicio, M. T., Maestre, AM., Patel, J., Lamothe, F., et al. (2017). Dengue virus Ns2B protein targets cgas for degradation and prevents mitochondrial dna sensing during infection. Nat. Microbiol. 2, 17037. doi: 10.1038/nmicrobiol.2017.37
Banerjee, I., Behl, B., Mendonca, M., Shrivastava, G., Russo, AJ., Menoret, A., et al. (2018). Gasdermin d restrains type I interferon response to cytosolic dna by disrupting ionic homeostasis. Immunity 49 (3), 413–426.e5. doi: 10.1016/j.immuni.2018.07.006
Barnett, K. C., Coronas-Serna, J. M., Zhou, W., Ernandes, MJ., Cao, A., Kranzusch, PJ., et al. (2019). Phosphoinositide interactions position cgas at the plasma membrane to ensure efficient distinction between self- and viral dna. Cell 176 (6), 1432–1446.e11. doi: 10.1016/j.cell.2019.01.049
Barouch, D. H., Ghneim, K., Bosche, W. J., Li, Y., Berkemeier, B., Hull, M., et al. (2016). Rapid inflammasome activation following mucosal siv infection of rhesus monkeys. Cell 165 (3), 656–667. doi: 10.1016/j.cell.2016.03.021
Bharaj, P., Atkins, C., Luthra, P., Giraldo, MI., Dawes, BE., Miorin, L., et al. (2017). The host E3-ubiquitin ligase Trim6 ubiquitinates the Ebola virus Vp35 protein and promotes virus replication. J. Virol. 91 (18), e00833-17. doi: 10.1128/JVI.00833-17
Bharaj, P., Wang, Y. E., Dawes, B. E., Yun, TE., Park, A., Yen, B., et al. (2016). The matrix protein of nipah virus targets the E3-ubiquitin ligase Trim6 to inhibit the ikkepsilon kinase-mediated type-I ifn antiviral response. PloS Pathog. 12 (9), e1005880. doi: 10.1371/journal.ppat.1005880
Biolatti, M., Dell’Oste, V., Pautasso, S., Gugliesi, F., von Einem, J., Krapp, C., et al. (2018). Human cytomegalovirus tegument protein Pp65 (Pul83) dampens type I interferon production by inactivating the dna sensor cgas without affecting sting. J. Virol. 92 (6), e01774-17. doi: 10.1128/JVI.01774-17
Bodda, C., Reinert, L. S., Fruhwurth, S., Richardo, T., Sun, C., Zhang, BC., et al. (2020). Hsv1 Vp1-2 deubiquitinates sting to block type I interferon expression and promote brain infection. J. Exp. Med. 217 (7), e20191422. doi: 10.1084/jem.20191422
Bo, Z., Miao, Y., Xi, R., Zhong, Q., Bao, C., Chen, H., et al. (2020). Prv Ul13 inhibits cgas-Sting-Mediated ifn-beta production by phosphorylating Irf3. Vet. Res. 51 (1), 118. doi: 10.1186/s13567-020-00843-4
Bortnik, V., Wu, M., Julcher, B., Salinas, A., Nikolic, I., Simpson, KJ., et al. (2021). Loss of hpv type 16 E7 restores cgas-sting responses in human papilloma virus-positive oropharyngeal squamous cell carcinomas cells. J. Microbiol. Immunol. Infect. 54 (4), 733–739. doi: 10.1016/j.jmii.2020.07.010
Burleigh, K., Maltbaek, J. H., Cambier, S., Green, R., Gale, MJ., James, RC., et al. (2020). Human dna-Pk activates a sting-independent dna sensing pathway. Sci. Immunol. 5 (43), eaba4219. doi: 10.1126/sciimmunol.aba4219
Cai, X., Chiu, Y. H., Chen, Z. J. (2014). The cgas-Cgamp-Sting pathway of cytosolic dna sensing and signaling. Mol. Cell. 54 (2), 289–296. doi: 10.1016/j.molcel.2014.03.040
Carty, M., Guy, C., Bowie, A. G. (2021). Detection of viral infections by innate immunity. Biochem. Pharmacol. 183, 114316. doi: 10.1016/j.bcp.2020.114316
Chen, X., Chen, Y. (2019). Ubiquitination of cgas by Traf6 regulates anti-dna viral innate immune responses. Biochem. Biophys. Res. Commun. 514 (3), 659–664. doi: 10.1016/j.bbrc.2019.05.022
Chen, M., Meng, Q., Qin, Y., Liang, P., Tan, P., He, L., et al. (2016). Trim14 inhibits cgas degradation mediated by selective autophagy receptor P62 to promote innate immune responses. Mol. Cell. 64 (1), 105–119. doi: 10.1016/j.molcel.2016.08.025
Chen, Y., Wang, L., Jin, J., Luan, Y., Chen, C., Li, Y., et al. (2017). P38 inhibition provides anti-dna virus immunity by regulation of Usp21 phosphorylation and sting activation. J. Exp. Med. 214 (4), 991–1010. doi: 10.1084/jem.20161387
Chen, J., Wei, X., Wang, X., Liu, T., Zhao, Y., Chen, L., et al. (2022). Tbk1-Mettl3 axis facilitates antiviral immunity. Cell Rep. 38 (7), 110373. doi: 10.1016/j.celrep.2022.110373
Chen, X., Yang, X., Zheng, Y., Yang, Y., Xing, Y., Chen, Z., et al. (2014). SARS coronavirus papain-like protease inhibits the type I interferon signaling pathway through interaction with the sting-Traf3-Tbk1 complex. Protein Cell. 5 (5), 369–381. doi: 10.1007/s13238-014-0026-3
Chiu, Y. H., Macmillan, J. B., Chen, Z. J. (2009). Rna polymerase iii detects cytosolic dna and induces type I interferons through the rig-I pathway. Cell 138 (3), 576–591. doi: 10.1016/j.cell.2009.06.015
Chu, T. T., Tu, X., Yang, K., Wu, J., Repa, JJ., Yan, N., et al. (2021). Tonic prime-boost of sting signalling mediates niemann-pick disease type c. Nature 596 (7873), 570–575. doi: 10.1038/s41586-021-03762-2
Cingoz, O., Goff, S. P. (2019). Hiv-1 is a poor inducer of innate immune responses. Mbio 10 (1), e02834-18. doi: 10.1128/mBio.02834-18
Clementz, M. A., Chen, Z., Banach, B. S., Wang, Y., Sun, L., Ratia, K., et al. (2010). Deubiquitinating and interferon antagonism activities of coronavirus papain-like proteases. J. Virol. 84 (9), 4619–4629. doi: 10.1128/JVI.02406-09
Cordova, A. F., Ritchie, C., Bohnert, V., Li, L. (2021). Human Slc46a2 is the dominant cgamp importer in extracellular cgamp-sensing macrophages and monocytes. ACS Cent Sci. 7 (6), 1073–1088. doi: 10.1021/acscentsci.1c00440
Cui, S., Yu, Q., Chu, L., Cui, Y., Ding, M., Wang, Q., et al. (2020). Nuclear cgas functions non-canonically to enhance antiviral immunity Via recruiting methyltransferase Prmt5. Cell Rep. 33 (10), 108490. doi: 10.1016/j.celrep.2020.108490
Cui, Y., Yu, H., Zheng, X., Peng, R., Wang, Q., Zhou, Y., et al. (2017). Senp7 potentiates cgas activation by relieving sumo-mediated inhibition of cytosolic dna sensing. PloS Pathog. 13 (1), e1006156. doi: 10.1371/journal.ppat.1006156
Dai, P., Wang, W., Cao, H., Avogadri, F., Dai, L., Drexler, I., et al. (2014). Modified vaccinia virus Ankara triggers type I ifn production in murine conventional dendritic cells Via a Cgas/Sting-mediated cytosolic dna-sensing pathway. PloS Pathog. 10 (4), e1003989. doi: 10.1371/journal.ppat.1003989
De Los Santos, T., Diaz-San Segundo, F., Grubman, M. J. (2007). Degradation of nuclear factor kappa b during foot-and-Mouth disease virus infection. J. Virol. 81 (23), 12803–12815. doi: 10.1128/JVI.01467-07
Deschamps, T., Kalamvoki, M. (2017). Evasion of the sting dna-sensing pathway by Vp11/12 of herpes simplex virus 1. J. Virol. 91 (16), e00535-17. doi: 10.1128/JVI.00535-17
Dewi, P. P. D., Kawasaki, T., Murase, M., Sueyoshi, T., Deguchi, T., Ori, D., et al. (2019). Ptdins3P phosphatases Mtmr3 and Mtmr4 negatively regulate innate immune responses to dna through modulating sting trafficking. J. Biol. Chem. 294 (21), 8412–8423. doi: 10.1074/jbc.RA118.005731
Ding, Z., Fang, L., Jing, H., Zeng, S., Wang, D., Liu, L., et al. (2014). Porcine epidemic diarrhea virus nucleocapsid protein antagonizes beta interferon production by sequestering the interaction between Irf3 and Tbk1. J. Virol. 88 (16), 8936–8945. doi: 10.1128/JVI.00700-14
Ding, Q., Gaska, J. M., Douam, F., Wei, L., Kim, D., Balev, M., et al. (2018). Species-specific disruption of sting-dependent antiviral cellular defenses by the zika virus Ns2B3 protease. Proc. Natl. Acad. Sci. 115 (27), E6310–E6318. doi: 10.1073/pnas.1803406115
Domizio, J. D., Gulen, M. F., Saidoune, F., Thacker, VV., Yatim, A., Sharma, K., et al. (2022). The cgas-sting pathway drives type I ifn immunopathology in covid-19. Nature 603 (7899), 145–151. doi: 10.1038/s41586-022-04421-w
Du, M., Chen, Z. J. (2018). Dna-induced liquid phase condensation of cgas activates innate immune signaling. Science 361 (6403), 704–709. doi: 10.1126/science.aat1022
Fang, R., Jiang, Q., Guan, Y., Gao, P., Zhang, R., Zhao, Z., et al. (2021). Golgi apparatus-synthesized sulfated glycosaminoglycans mediate polymerization and activation of the cgamp sensor sting. Immunity 54 (5), 962–975. doi: 10.1016/j.immuni.2021.03.011
Fang, R., Wang, C., Jiang, Q., Lv, M., Gao, P., Yu, X., et al. (2017). Nemo-ikkβ are essential for Irf3 and nf-κb activation in the cgas-sting pathway. J. Immunol. 199 (9), 3222. doi: 10.4049/jimmunol.1700699
Feng, K., Deng, F., Hu, Z., Wang, H., Ning, Y.. (2019). Heartland virus antagonizes type I and iii interferon antiviral signaling by inhibiting phosphorylation and nuclear translocation of Stat2 and Stat1. J. Biol. Chem. 294 (24), 9503–9517. doi: 10.1074/jbc.RA118.006563
Fu, Y. Z., Su, S., Gao, Y. Q., Wang, P. P., Huang, Z. F., Hu, M. M., et al. (2019). Human cytomegalovirus protein Ul42 antagonizes Cgas/Mita-mediated innate antiviral response. PloS Pathog. 15 (5), e1007691. doi: 10.1371/journal.ppat.1007691
Fung, S. Y., Siu, K. L., Lin, H., Wang, PP., Huang, ZF., Hu, MM., et al. (2021). SARS-Cov-2 main protease suppresses type I interferon production by preventing nuclear translocation of phosphorylated Irf3. Int. J. Biol. Sci. 17 (6), 1547–1554. doi: 10.7150/ijbs.59943
Fu, Y. Z., Su, S., Gao, Y. Q., Wang, PP., Huang, ZF., Hu, MM., et al. (2017). Human cytomegalovirus tegument protein Ul82 inhibits sting-mediated signaling to evade antiviral immunity. Cell Host Microbe 21 (2), 231–243. doi: 10.1016/j.chom.2017.01.001
Gao, L., Li, K., Zhang, Y., Liu, Y., Liu, C., Zhang, Y., et al. (2019). Inhibition of dna-sensing pathway by marek’s disease virus Vp23 protein through suppression of interferon regulatory factor 7 activation. J. Virol. 93 (4), e01934-18. doi: 10.1128/JVI.01934-18
Gao, D., Wu, J., Wu, Y. T., Du, F., Aroh, C., Yan, N., et al. (2013). Cyclic gmp-amp synthase is an innate immune sensor of hiv and other retroviruses. Science 341 (6148), 903–906. doi: 10.1126/science.1240933
García-Belmonte, R., Pérez-Núñez, D., Pittau, M., Richt, JA., Revilla, Y. (2019). African Swine fever virus Armenia/07 virulent strain controls interferon beta production through the cgas-sting pathway. J. Virol. 93 (12), e2218–e2298. doi: 10.1128/JVI.02298-18
Georgana, I., Sumner, R. P., Towers, G. J., Maluquer De Motes, C.. (2018). Virulent poxviruses inhibit dna sensing by preventing sting activation. J. Virol. 92 (10), e2117–e2145. doi: 10.1128/JVI.02145-17
Guan, X., Zhang, M., Fu, M., Luo, S., Hu, Q.. (2019). Herpes simplex virus type 2 immediate early protein Icp27 inhibits ifn-β production in mucosal epithelial cells by antagonizing Irf3 activation. Front. Immunol. 10, 290. doi: 10.3389/fimmu.2019.00290
Guey, B., Wischnewski, M., Decout, A., Makasheva, K., Kaynak, M., Sakar, MS., et al. (2020). Baf restricts cgas on nuclear dna to prevent innate immune activation. Science 369 (6505), 823–828. doi: 10.1126/science.aaw6421
Guo, Y., Jiang, F., Kong, L., Li, B., Yang, Y., Zhang, L., et al. (2019). Cutting edge: Usp27X deubiquitinates and stabilizes the dna sensor cgas to regulate cytosolic dna-mediated signaling. J. Immunol. 203 (8), 2049–2054. doi: 10.4049/jimmunol.1900514
Guo, Y., Jiang, F., Kong, L., Wu, H., Zhang, H., Chen, X., et al. (2021). Otud5 promotes innate antiviral and antitumor immunity through deubiquitinating and stabilizing sting. Cell Mol. Immunol. 18 (8), 1945–1955. doi: 10.1038/s41423-020-00531-5
Guo, H., Konig, R., Deng, M., Riess, M., Mo, J., Zhang, L., et al. (2016). Nlrx1 sequesters sting to negatively regulate the interferon response, thereby facilitating the replication of hiv-1 and dna viruses. Cell Host Microbe 19 (4), 515–528. doi: 10.1016/j.chom.2016.03.001
Guo, Y., Li, R., Tan, Z., Shi, J., Fu, Y., Song, Y., et al. (2020). E3 ubiquitin ligase Asb8 negatively regulates interferon Via regulating Tbk1/Ikki homeostasis. Mol. Immunol. 121, 195–203. doi: 10.1016/j.molimm.2020.03.011
Han, M., Yoo, D. (2014). Modulation of innate immune signaling by nonstructural protein 1 (Nsp1) in the family arteriviridae. Virus Res. 194, 100–109. doi: 10.1016/j.virusres.2014.09.007
Han, L., Zhuang, M. W., Deng, J., Zheng, Y., Zhang, J., Nan, ML., et al. (2021). SARS-Cov-2 Orf9B antagonizes type I and iii interferons by targeting multiple components of the Rig-I/Mda-5–mavs, Tlr3–trif, and cgas–sting signaling pathways. J. Med. Virol. 93 (9), 5376–5389. doi: 10.1002/jmv.27050
Hato, S. V., Sorgeloos, F., Ricour, C., Zoll, J., Melchers, WJ., Michiels, T., et al. (2010). Differential ifn-α/β production suppressing capacities of the leader proteins of mengovirus and foot-and-Mouth disease virus. Cell Microbiol. 12 (3), 310–317. doi: 10.1111/j.1462-5822.2009.01395.x
He, T. S., Xie, T., Li, J., Yang, YX., Li, C., Wang, W., et al. (2019). Tho complex subunit 7 homolog negatively regulates cellular antiviral response against rna viruses by targeting Tbk1. Viruses 11 (2), 158. doi: 10.3390/v11020158
Higgs, R., Ni, G. J., Ben, L. N., Breen, EP., Fitzgerald, KA., Jefferies, CA., et al. (2008). The E3 ubiquitin ligase Ro52 negatively regulates ifn-beta production post-pathogen recognition by polyubiquitin-mediated degradation of Irf3. J. Immunol. 181 (3), 1780–1786. doi: 10.4049/jimmunol.181.3.1780
Hornung, V., Ablasser, A., Charrel-Dennis, M., Bauernfeind, F., Horvath, G., Caffrey, DR., et al. (2009). Aim2 recognizes cytosolic dsdna and forms a caspase-1-Activating inflammasome with asc. Nature 458 (7237), 514–518. doi: 10.1038/nature07725
Huai, W., Liu, X., Wang, C., Zhang, Y., Chen, X., Chen, X., et al. (2019). Kat8 selectively inhibits antiviral immunity by acetylating Irf3. J. Exp. Med. 216 (4), 772–785. doi: 10.1084/jem.20181773
Huang, H., Xiong, Q., Wang, N., Chen, R., Ren, H., Siwko, S., et al. (2018). Kisspeptin/Gpr54 signaling restricts antiviral innate immune response through regulating calcineurin phosphatase activity. Sci. Adv. 4 (8), eaas9784. doi: 10.1126/sciadv.aas9784
Huang, Z. F., Zou, H. M., Liao, B. W., Zhang, HY., Yang, Y., Fu, YZ., et al. (2018). Human cytomegalovirus protein Ul31 inhibits dna sensing of cgas to mediate immune evasion. Cell Host Microbe 24 (1), 69–80.e4. doi: 10.1016/j.chom.2018.05.007
Hu, M. M., Yang, Q., Xie, X. Q., Liao, CY., Lin, H., Liu, TT., et al. (2016). Sumoylation promotes the stability of the dna sensor cgas and the adaptor sting to regulate the kinetics of response to dna virus. Immunity 45 (3), 555–569. doi: 10.1016/j.immuni.2016.08.014
Ishikawa, H., Barber, G. N. (2008). Sting is an endoplasmic reticulum adaptor that facilitates innate immune signalling. Nature 455 (7213), 674–678. doi: 10.1038/nature07317
Jena, K. K., Mehto, S., Nath, P., Chauhan, NR., Sahu, R., Dhar, K., et al. (2020). Autoimmunity gene irgm suppresses cgas-sting and rig-I-Mavs signaling to control interferon response. EMBO Rep. 21 (9), e50051. doi: 10.15252/embr.202050051
Jia, M., Qin, D., Zhao, C., Chai, L., Yu, Z., Wang, W., et al. (2020). Redox homeostasis maintained by Gpx4 facilitates sting activation. Nat. Immunol. 21 (7), 727–735. doi: 10.1038/s41590-020-0699-0
Kalamvoki, M., Roizman, B. (2014). Hsv-1 degrades, stabilizes, requires, or is stung by sting depending on Icp0, the Us3 protein kinase, and cell derivation. Proc. Natl. Acad. Sci. U S A. 111 (5), E611–E617. doi: 10.1073/pnas.1323414111
Kang, H., Liu, D., Tian, J., Hu, X., Zhang, X., Yin, H., et al. (2017). Feline panleucopenia virus Ns2 suppresses the host ifn-beta induction by disrupting the interaction between Tbk1 and sting. Viruses 9 (1), 23. doi: 10.3390/v9010023
Kim, G. W., Imam, H., Khan, M., Mir, SA., Kim, SJ., Yoon, SK., et al. (2021). Hbv-induced increased N6 methyladenosine modification of pten rna affects innate immunity and contributes to hcc. Hepatology 73 (2), 533–547. doi: 10.1002/hep.31313
Konno, H., Konno, K., Barber, G. N. (2013). Cyclic dinucleotides trigger Ulk1 (Atg1) phosphorylation of sting to prevent sustained innate immune signaling. Cell 155 (3), 688–698. doi: 10.1016/j.cell.2013.09.049
Lam, E., Falck-Pedersen, E. (2014). Unabated adenovirus replication following activation of the Cgas/Sting-dependent antiviral response in human cells. J. Virol. 88 (24), 14426–14439. doi: 10.1128/JVI.02608-14
Lam, E., Stein, S., Falck-Pedersen, E. (2014). Adenovirus detection by the Cgas/Sting/Tbk1 dna sensing cascade. J. Virol. 88 (2), 974–981. doi: 10.1128/JVI.02702-13
Lau, L., Gray, E. E., Brunette, R. L., Stetson, DB.. (2015). Dna tumor virus oncogenes antagonize the cgas-sting dna-sensing pathway. Science 350 (6260), 568–571. doi: 10.1126/science.aab3291
Lei, C. Q., Zhang, Y., Xia, T., Jiang, LQ., Zhong, B., Shu, HB., et al. (2013). Foxo1 negatively regulates cellular antiviral response by promoting degradation of Irf3. J. Biol. Chem. 288 (18), 12596–12604. doi: 10.1074/jbc.M112.444794
Li, R., Chen, C., He, J., Zhang, L., Zhang, L., Guo, Y., et al. (2019). E3 ligase Asb8 promotes porcine reproductive and respiratory syndrome virus proliferation by stabilizing the viral Nsp1α protein and degrading host ikkβ kinase. Virology 532, 55–68. doi: 10.1016/j.virol.2019.04.004
Li, J., Duran, M. A., Dhanota, N., Chatila, WK., Bettigole, SE., Kwon, J., et al. (2021). Metastasis and immune evasion from extracellular cgamp hydrolysis. Cancer Discovery 11 (5), 1212–1227. doi: 10.1158/2159-8290.CD-20-0387
Li, M., Ferretti, M., Ying, B., Descamps, H., Lee, E., Dittmar, M., et al. (2021). Pharmacological activation of sting blocks SARS-Cov-2 infection. Sci. Immunol. 6 (59). doi: 10.1126/sciimmunol.abi9007
Li, Y., He, M., Wang, Z., Duan, Z., Guo, Z., Wang, Z., et al. (2022). Sting signaling activation inhibits hbv replication and attenuates the severity of liver injury and hbv-induced fibrosis. Cell Mol. Immunol. 19 (1), 92–107. doi: 10.1038/s41423-021-00801-w
Li, S., Qian, N., Jiang, C., Zu, W., Liang, A., Li, M., et al. (2022). Gain-of-Function genetic screening identifies the antiviral function of Tmem120a Via sting activation. Nat. Commun. 13 (1), 105. doi: 10.1038/s41467-021-27670-1
Liu, Y., Gao, L., Xu, Z., Luo, D., Zhang, Y., Gao, Y., et al. (2019). Marek’s disease virus Rlorf4 inhibits type I interferon production by antagonizing nf-kappab activation. J. Virol. 93 (18), e01037-19. doi: 10.1128/JVI.01037-19
Liu, Q., Gu, T., Su, L. Y., Jiao, L., Qiao, X., Xu, M., et al. (2021). Gsnor facilitates antiviral innate immunity by restricting Tbk1 cysteine s-nitrosation. Redox Biol. 47, 102172. doi: 10.1016/j.redox.2021.102172
Liu, Y., Li, J., Chen, J.. (2014). Hepatitis b virus polymerase disrupts K63-linked ubiquitination of sting to block innate cytosolic dna-sensing pathways. J. Virol. 89 (4), 2287–2300. doi: 10.1128/JVI.02760-14
Liu, D., Sheng, C., Gao, S., Yao, C., Li, J., Jiang, W., et al. (2015). Socs3 drives proteasomal degradation of Tbk1 and negatively regulates antiviral innate immunity. Mol. Cell Biol. 35 (14), 2400–2413. doi: 10.1128/MCB.00090-15
Li, X. D., Wu, J., Gao, D., Wang, H., Sun, L., Chen, ZJ. (2013). Pivotal roles of cgas-cgamp signaling in antiviral defense and immune adjuvant effects. Science 341 (6152), 1390–1394. doi: 10.1126/science.1244040
Li, D., Wu, R., Guo, W., Xie, L., Qiao, Z., Chen, S., et al. (2019). Sting-mediated Ifi16 degradation negatively controls type I interferon production. Cell Rep. 29 (5), 1249–1260. doi: 10.1016/j.celrep.2019.09.069
Li, X., Zhu, Y., Zhang, X., An, X., Weng, M., Shi, J., et al. (2022). An alternatively spliced sting isoform localizes in the cytoplasmic membrane and directly senses extracellular cgamp. J. Clin. Invest. 132 (3), e144339. doi: 10.1172/JCI144339
Look, D. C., Roswit, W. T., Frick, A. G., Gris-Alevy, Y., Dickhaus, DM., Walter, MJ., et al. (1998). Direct suppression of Stat1 function during adenoviral infection. Immunity 9 (6), 871–880. doi: 10.1016/S1074-7613(00)80652-4
Lui, P. Y., Wong, L. Y., Fung, C. L., Siu, KL., Yeung, ML., Yuen, KS., et al. (2016). Middle East respiratory syndrome coronavirus m protein suppresses type I interferon expression through the inhibition of Tbk1-dependent phosphorylation of Irf3. Emerg. Microbes Infect. 5, e39. doi: 10.1038/emi.2016.33
Luo, W. W., Li, S., Li, C., Lian, H., Yang, Q., Zhong, B., et al. (2016). Irhom2 is essential for innate immunity to dna viruses by mediating trafficking and stability of the adaptor sting. Nat. Immunol. 17 (9), 1057–1066. doi: 10.1038/ni.3510
Luo, W. W., Tong, Z., Cao, P., Wang, FB., Liu, Y., Zheng, ZQ., et al. (2022). Transcription-independent regulation of sting activation and innate immune responses by Irf8 in monocytes. Nat. Commun. 13 (1), 4822. doi: 10.1038/s41467-022-32401-1
Lu, M., Qiu, S., Zhang, L., Sun, Y., Bao, E., Lv, Y. (2021). Pseudorabies virus glycoprotein Ge suppresses interferon-β production Via creb-binding protein degradation. Virus Res. 291, 198220. doi: 10.1016/j.virusres.2020.198220
Lu, D., Shang, G., Li, J., Lu, Y., Bai, XC., Zhang, X. (2022). Activation of sting by targeting a pocket in the transmembrane domain. Nature 604 (7906), 557–562. doi: 10.1038/s41586-022-04559-7
Luteijn, R. D., Zaver, S. A., Gowen, B. G., Wyman, SK., Garelis, NE., Onia, L., et al. (2020). Author correction: Slc19a1 transports immunoreactive cyclic dinucleotides. Nature 579 (7800), E12. doi: 10.1038/s41586-020-2064-8
Luthra, P., Aguirre, S., Yen, B. C., Pietzsch, CA., Sanchez-Aparicio, MT., Tigabu, B., et al. (2017). Topoisomerase ii inhibitors induce DNA damage-dependent interferon responses circumventing Ebola virus immune evasion. Mbio 8 (2), e00368-17. doi: 10.1128/mBio.00368-17
Ma, Z., Damania, B. (2016). The cgas-sting defense pathway and its counteraction by viruses. Cell Host Microbe 19 (2), 150–158. doi: 10.1016/j.chom.2016.01.010
Ma, Z., Jacobs, S. R., West, J. A., Stopford, C., Zhang, Z., Davis, Z., et al. (2015). Modulation of the cgas-sting dna sensing pathway by gammaherpesviruses. Proc. Natl. Acad. Sci. U. S. A. 112 (31), E4306–E4315. doi: 10.1073/pnas.1503831112
Mariggio, G., Koch, S., Zhang, G., Weidner-Glunde, M., Ruckert, J., Kati, S., et al. (2017). Kaposi sarcoma herpesvirus (Kshv) latency-associated nuclear antigen (Lana) recruits components of the mrn (Mre11-Rad50-Nbs1) repair complex to modulate an innate immune signaling pathway and viral latency. PloS Pathog. 13 (4), e1006335. doi: 10.1371/journal.ppat.1006335
Meade, N., King, M., Munger, J., et al. (2019). Mtor dysregulation by vaccinia virus F17 controls multiple processes with varying roles in infection. J. Virol. 93 (15), e719–e784. doi: 10.1128/JVI.00784-19
Medina, G. N., Azzinaro, P., Ramirez-Medina, E., Fang, Y., Diaz-San Segundo, F., et al. (2020). Impairment of the deisgylation activity of foot-and-Mouth disease virus lpro causes attenuation in vitro and in vivo. J. Virol. 94 (13), e320–e341. doi: 10.1128/JVI.00341-20
Min, YQ., Shi, C., Yao, T., Feng, K., Mo, Q., Deng, F., et al. (2020). The nonstructural protein of guertu virus disrupts host defenses by blocking antiviral interferon induction and action. ACS Infect. Dis. 6 (5), 857–870. doi: 10.1021/acsinfecdis.9b00492
Moriyama, M., Koshiba, T., Ichinohe, T. (2019). Influenza a virus M2 protein triggers mitochondrial dna-mediated antiviral immune responses. Nat. Commun. 10 (1), 4624. doi: 10.1038/s41467-019-12632-5
Mukai, K., Konno, H., Akiba, T., Uemura, T., Waguri, S., Kobayashi, T., et al. (2016). Activation of sting requires palmitoylation at the golgi. Nat. Commun. 7, 11932. doi: 10.1038/ncomms11932
Ni, G., Konno, H., Barber, G. N. (2017). Ubiquitination of sting at lysine 224 controls Irf3 activation. Sci. Immunol. 2 (11), eaah7119. doi: 10.1126/sciimmunol.aah7119
Ni, G., Ma, Z., Wong, J. P., Zhang, Z., Cousins, E., Major, MB., et al. (2020). Ppp6C negatively regulates sting-dependent innate immune responses. Mbio 11 (4), e01728-20. doi: 10.1128/mBio.01728-20
Ning, Y., Feng, K., Min, Y., Deng, F., Hu, Z., Wang, H., et al. (2017). Heartland virus nss protein disrupts host defenses by blocking the Tbk1 kinase–Irf3 transcription factor interaction and signaling required for interferon induction. J. Biol. Chem. 292 (40), 16722–16733. doi: 10.1074/jbc.M117.805127
Ning, X., Wang, Y., Jing, M., Sha, M., Lv, M., Gao, P., et al. (2019). Apoptotic caspases suppress type I interferon production Via the cleavage of cgas, mavs, and Irf3. Mol. Cell. 74 (1), 19–31.e7. doi: 10.1016/j.molcel.2019.02.013
Nitta, S., Sakamoto, N., Nakagawa, M., Kakinuma, S., Mishima, K., Kusano Kitazume, A., et al. (2013). Hepatitis c virus Ns4B protein targets sting and abrogates Rig-I–mediated type I interferon-dependent innate immunity. Hepatology 57 (1), 46–58. doi: 10.1002/hep.26017
Orzalli, M. H., Broekema, N. M., Knipe, D. M. (2016). Relative contributions of herpes simplex virus 1 Icp0 and vhs to loss of cellular Ifi16 vary in different human cell types. J. Virol. 90 (18), 8351–8359. doi: 10.1128/JVI.00939-16
Pathare, G. R., Decout, A., Gluck, S., Cavadini, S., Makasheva, K., Hovius, R., et al. (2020). Structural mechanism of cgas inhibition by the nucleosome. Nature 587 (7835), 668–672. doi: 10.1038/s41586-020-2750-6
Peng, P. H., Hsu, K. W., Wu, K. J. (2021). Liquid-liquid phase separation (Llps) in cellular physiology and tumor biology. Am. J. Cancer Res. 11 (8), 3766–3776.
Peters, N. E., Ferguson, B. J., Mazzon, M., Fahy, AS., Krysztofinska, E., Arribas-Bosacoma, R., et al. (2013). A mechanism for the inhibition of dna-Pk-Mediated dna sensing by a virus. PloS Pathog. 9 (10), e1003649. doi: 10.1371/journal.ppat.1003649
Prabakaran, T., Bodda, C., Krapp, C., Zhang, BC., Christensen, MH., Sun, C., et al. (2018). Attenuation of cgas-sting signaling is mediated by a P62/Sqstm1-dependent autophagy pathway activated by Tbk1. EMBO J. 37 (8), e97858. doi: 10.15252/embj.201797858
Qi, D., Hu, L., Jiao, T., Zhang, T., Tong, X., Ye, X., et al. (2018). Phosphatase Cdc25a negatively regulates the antiviral immune response by inhibiting Tbk1 activity. J. Virol. 92 (19), e01118-18. doi: 10.1128/JVI.01118-18
Qin, Y., Zhou, M. T., Hu, M. M., Hu, YH., Zhang, J., Guo, L., et al. (2014). Rnf26 temporally regulates virus-triggered type I interferon induction by two distinct mechanisms. PloS Pathog. 10 (9), e1004358. doi: 10.1371/journal.ppat.1004358
Rajsbaum, R., Versteeg, G. A., Schmid, S., Maestre, AM., Belicha-Villanueva, A., Martinez-Romero, C., et al. (2014). Unanchored K48-linked polyubiquitin synthesized by the E3-ubiquitin ligase Trim6 stimulates the interferon-ikkepsilon kinase-mediated antiviral response. Immunity 40 (6), 880–895. doi: 10.1016/j.immuni.2014.04.018
Ran, Y., Liu, T. T., Zhou, Q., Li, S., Mao, AP., Li, Y., et al. (2011). Senp2 negatively regulates cellular antiviral response by desumoylating Irf3 and conditioning it for ubiquitination and degradation. J. Mol. Cell Biol. 3 (5), 283–292. doi: 10.1093/jmcb/mjr020
Ran, Y., Xiong, M. G., Xu, Z. S., Luo, WW., Wang, YY.. (2019). Yipf5 is essential for innate immunity to dna virus and facilitates copii-dependent sting trafficking. J. Immunol. 203 (6), 1560–1570. doi: 10.4049/jimmunol.1900387
Rui, Y., Su, J., Shen, S., Hu, Y., Huang, D., Zheng, W., et al. (2021). Unique and complementary suppression of cgas-sting and rna sensing-triggered innate immune responses by SARS-Cov-2 proteins. Signal Transduction Targeted Ther. 6 (1), 1–11. doi: 10.1038/s41392-021-00515-5
Saitoh, T., Fujita, N., Hayashi, T., Takahara, K., Satoh, T., Lee, H., et al. (2009). Atg9a controls dsdna-driven dynamic translocation of sting and the innate immune response. Proc. Natl. Acad. Sci. U S A. 106 (49), 20842–20846. doi: 10.1073/pnas.0911267106
Saitoh, T., Tun-Kyi, A., Ryo, A., Yamamoto, M., Finn, G., Fujita, T., et al. (2006). Negative regulation of interferon-regulatory factor 3-dependent innate antiviral response by the prolyl isomerase Pin1. Nat. Immunol. 7 (6), 598–605. doi: 10.1038/ni1347
Sato, H., Hoshi, M., Ikeda, F., Fujiyuki, T., Yoneda, M., Kai, C., et al. (2021). Downregulation of mitochondrial biogenesis by virus infection triggers antiviral responses by cyclic gmp-amp synthase. PloS Pathog. 17 (10), e1009841. doi: 10.1371/journal.ppat.1009841
Schoggins, J. W., MacDuff, D. A., Imanaka, N., Gainey, MD., Shrestha, B., Eitson, JL., et al. (2014). Pan-viral specificity of ifn-induced genes reveals new roles for cgas in innate immunity. Nature 505 (7485), 691–695. doi: 10.1038/nature12862
Schoggins, J. W., Wilson, S. J., Panis, M., Murphy, MY., Jones, CT., Bieniasz, P., et al. (2015). Corrigendum: A diverse range of gene products are effectors of the type I interferon antiviral response. Nature 525 (7567), 144. doi: 10.1038/nature14554
Scutts, S. R., Ember, S. W., Ren, H., Ye, C., Lovejoy, CA., Mazzon, M., et al. (2018). Dna-Pk is targeted by multiple vaccinia virus proteins to inhibit dna sensing. Cell Rep. 25 (7), 1953–1965. doi: 10.1016/j.celrep.2018.10.034
Seo, G. J., Kim, C., Shin, W. J., Sklan, EH., Eoh, H., Jung, JU., et al. (2018). Trim56-mediated monoubiquitination of cgas for cytosolic dna sensing. Nat. Commun. 9 (1), 613. doi: 10.1038/s41467-018-02936-3
Shang, G., Zhang, C., Chen, Z. J., Bai, XC., Zhang, X.. (2019). Cryo-em structures of sting reveal its mechanism of activation by cyclic gmp-amp. Nature 567 (7748), 389–393. doi: 10.1038/s41586-019-0998-5
Shi, C., Yang, X., Liu, Y., Li, H., Chu, H., Li, G., et al. (2022). Zdhhc18 negatively regulates cgas-mediated innate immunity through palmitoylation. EMBO J. 41 (11), e109272. doi: 10.15252/embj.2021109272
Shravanthi, G. V., Mukherjee, RM., Reddy, RB., Rao, PN., Rao, DN. (2015). Hbv induce cytosolic dna sensor cgas but suppress Irf3 to prevent generation of antiviral defense mechanism in infected cell. J. Clin. Exp. Hepatol 5, S4. doi: 10.1016/j.jceh.2015.07.008
Song, G., Liu, B., Li, Z., Wu, H., Wang, P., Zhao, K., et al. (2016). E3 ubiquitin ligase Rnf128 promotes innate antiviral immunity through K63-linked ubiquitination of Tbk1. Nat. Immunol. 17 (12), 1342–1351. doi: 10.1038/ni.3588
Song, Y., Wu, X., Xu, Y., Zhu, J., Cheng, H.. (2020). Hpv E7 inhibits cell pyroptosis by promoting Trim21-mediated degradation and ubiquitination of the Ifi16 inflammasome. Int. J. Biol. Sci. 16 (15), 2924–2937. doi: 10.7150/ijbs.50074
Srikanth, S., Woo, J. S., Wu, B., El-Sherbiny, YM., Leung, J., Chupradit, K., et al. (2019). The Ca(2+) sensor Stim1 regulates the type I interferon response by retaining the signaling adaptor sting at the endoplasmic reticulum. Nat. Immunol. 20 (2), 152–162. doi: 10.1038/s41590-018-0287-8
Sui, L., Zhao, Y., Wang, W., Wu, P., Wang, Z., Yu, Y., et al. (2021). SARS-Cov-2 membrane protein inhibits type I interferon production through ubiquitin-mediated degradation of Tbk1. Front. Immunol. 12, 662989. doi: 10.3389/fimmu.2021.662989
Sumner, R. P., Harrison, L., Touizer, E., Peacock, TP., Spencer, M., Zuliani-Alvarez, L., et al. (2020). Disrupting hiv-1 capsid formation causes cgas sensing of viral dna. EMBO J. 39 (20), e103958. doi: 10.15252/embj.2019103958
Sun, H., Huang, Y., Mei, S., Xu, F., Liu, X., Zhao, F., et al. (2021). A nuclear export signal is required for cgas to sense cytosolic dna. Cell Rep. 34 (1), 108586. doi: 10.1016/j.celrep.2020.108586
Sun, W., Li, Y., Chen, L., Chen, H., You, F., Zhou, X., et al. (2009). Eris, an endoplasmic reticulum ifn stimulator, activates innate immune signaling through dimerization. Proc. Natl. Acad. Sci. U.S.A. 106 (21), 8653–8658. doi: 10.1073/pnas.0900850106
Sun, X., Liu, T., Zhao, J., Xia, H., Xie, J., Guo, Y., et al. (2020). Dna-Pk deficiency potentiates cgas-mediated antiviral innate immunity. Nat. Commun. 11 (1), 6182. doi: 10.1038/s41467-020-19941-0
Sun, L., Wu, J., Du, F., Chen, X., Chen, Z. J. (2013). Cyclic gmp-amp synthase is a cytosolic dna sensor that activates the type I interferon pathway. Science 339 (6121), 786–791. doi: 10.1126/science.1232458
Sun, L., Xing, Y., Chen, X., Zheng, Y., Yang, Y., Nichols, D. B., et al. (2012). Coronavirus papain-like proteases negatively regulate antiviral innate immune response through disruption of sting-mediated signaling. PloS One 7 (2), e30802. doi: 10.1371/journal.pone.0030802
Sun, M. S., Zhang, J., Jiang, L. Q., Pan, Y. X., Tan, J. Y., Yu, F., et al. (2018). Tmed2 potentiates cellular ifn responses to dna viruses by reinforcing mita dimerization and facilitating its trafficking. Cell Rep. 25 (11), 3086–3098.e3. doi: 10.1016/j.celrep.2018.11.048
Sun, H., Zhang, Q., Jing, Y. Y., Man, Z., Bo, Z. (2017). Usp13 negatively regulates antiviral responses by deubiquitinating sting. Nat. Commun. 8 (1), 15534. doi: 10.1038/ncomms15534
Su, J., Rui, Y., Lou, M., Yin, L., Xiong, H., Zhou, Z., et al. (2019). Hiv-2/Siv vpx targets a novel functional domain of sting to selectively inhibit cgas–Sting-Mediated nf-κb signalling. Nat. Microbiol. 4 (12), 2552–2564. doi: 10.1038/s41564-019-0585-4
Su, C., Zheng, C. (2017). Herpes simplex virus 1 abrogates the Cgas/Sting-mediated cytosolic dna-sensing pathway via its virion host shutoff protein, Ul41. J. Virol. 91 (6), e02414-16. doi: 10.1128/JVI.02414-16
Tang, D., Kang, R., Coyne, C. B., Zeh, HJ., Lotze, MT.. (2012). Pamps and damps: Signal 0S that spur autophagy and immunity. Immunol. Rev. 249 (1), 158–175. doi: 10.1111/j.1600-065X.2012.01146.x
Tao, P., Wang, S., Ozen, S., Lee, PY., Zhang, J., Wang, J., et al. (2021). Deubiquitination of proteasome subunits by otulin regulates type I ifn production. Sci. Adv. 7 (47), i6794. doi: 10.1126/sciadv.abi6794
Tian, M., Liu, W., Zhang, Q., Huang, Y., Li, W., Wang, W., et al. (2020). Mysm1 represses innate immunity and autoimmunity through suppressing the cgas-sting pathway. Cell Rep. 33 (3), 108297. doi: 10.1016/j.celrep.2020.108297
Tsuchida, T., Zou, J., Saitoh, T., Kumar, H., Abe, T., Matsuura, Y., et al. (2010). The ubiquitin ligase Trim56 regulates innate immune responses to intracellular double-stranded dna. Immunity 33 (5), 765–776. doi: 10.1016/j.immuni.2010.10.013
Unterholzner, L., Keating, S. E., Baran, M., Horan, KA., Jensen, SB., Sharma, S., et al. (2010). Ifi16 is an innate immune sensor for intracellular dna. Nat. Immunol. 11 (11), 997–1004. doi: 10.1038/ni.1932
van Tol, S., Atkins, C., Bharaj, P., Johnson, KN., Hage, A., Freiberg, AN., et al. (2020). Vamp8 contributes to the Trim6-mediated type I interferon antiviral response during West Nile virus infection. J. Virol. 94 (2), e01454-19. doi: 10.1128/JVI.01454-19
Visser, L. J., Aloise, C., Swatek, K. N., Medina, GN., Olek, KM., Rabouw, HH., et al. (2020). Dissecting distinct proteolytic activities of fmdv lpro implicates cleavage and degradation of rlr signaling proteins, not its Deisgylase/Dub activity, in type I interferon suppression. PloS Pathog. 16 (7), e1008702. doi: 10.1371/journal.ppat.1008702
Wang, Z., Chen, J., Wu, X., Ma, D., Zhang, X., Li, R., et al. (2021). Pcv2 targets cgas to inhibit type I interferon induction to promote other dna virus infection. PloS Pathog. 17 (9), e1009940. doi: 10.1371/journal.ppat.1009940
Wang, D., Fang, L., Luo, R., Ye, R., Fang, Y., Xie, L., et al. (2010). Foot-and-Mouth disease virus leader proteinase inhibits dsrna-induced type I interferon transcription by decreasing interferon regulatory factor 3/7 in protein levels. Biochem. Bioph Res. Co. 399 (1), 72–78. doi: 10.1016/j.bbrc.2010.07.044
Wang, Q., Huang, L., Hong, Z., Lv, Z., Mao, Z., Tang, Y., et al. (2017). The E3 ubiquitin ligase Rnf185 facilitates the cgas-mediated innate immune response. PloS Pathog. 13 (3), e1006264. doi: 10.1371/journal.ppat.1006264
Wang, K., Huang, C., Jiang, T., Chen, Z., Xue, M., Zhang, Q., et al. (2021). Rna-binding protein Rbm47 stabilizes Ifnar1 mrna to potentiate host antiviral activity. EMBO Rep. 22 (8), e52205. doi: 10.15252/embr.202052205
Wang, Y., Lian, Q., Yang, B., Yan, S., Zhou, H., He, L., et al. (2015). Trim30Alpha is a negative-feedback regulator of the intracellular dna and dna virus-triggered response by targeting sting. PloS Pathog. 11 (6), e1005012. doi: 10.1371/journal.ppat.1005012
Wang, Q., Liu, X., Cui, Y., Tang, Y., Chen, W., Li, S., et al. (2014). The E3 ubiquitin ligase amfr and Insig1 bridge the activation of Tbk1 kinase by modifying the adaptor sting. Immunity 41 (6), 919–933. doi: 10.1016/j.immuni.2014.11.011
Wang, S., Wang, K., Lin, R., Zheng, C.. (2013). Herpes simplex virus 1 Serine/Threonine kinase Us3 hyperphosphorylates Irf3 and inhibits beta interferon production. J. Virol. 87 (23), 12814–12827. doi: 10.1128/JVI.02355-13
Wang, C., Wang, X., Veleeparambil, M., Kessler, PM., Willard, B., Chattopadhyay, S., et al. (2020). Egfr-mediated tyrosine phosphorylation of sting determines its trafficking route and cellular innate immunity functions. EMBO J. 39 (22), e104106. doi: 10.15252/embj.2019104106
Wang, Y., Wang, P., Zhang, Y., Xu, J., Li, Z., Li, Z., et al. (2020). Decreased expression of the host long-noncoding rna-gm facilitates viral escape by inhibiting the kinase activity Tbk1 Via s-glutathionylation. Immunity 53 (6), 1168–1181.e7. doi: 10.1016/j.immuni.2020.11.010
Wang, L., Wen, M., Cao, X. (2019). Nuclear Hnrnpa2B1 initiates and amplifies the innate immune response to dna viruses. Science 365 (6454), eaav0758. doi: 10.1126/science.aav0758
Wang, J., Yang, S., Liu, L., Wang, H., Yang, B. (2017). Htlv-1 tax impairs K63-linked ubiquitination of sting to evade host innate immunity. Virus Res. 232, 13–21. doi: 10.1016/j.virusres.2017.01.016
Wang, F., Zhao, M., Chang, B., Zhou, Y., Wu, X., Ma, M., et al. (2022). Cytoplasmic Parp1 links the genome instability to the inhibition of antiviral immunity through parylating cgas. Mol. Cell 214–215. doi: 10.1016/j.molcel.2022.03.034
Wang, P., Zhao, W., Zhao, K., Zhang, L., Gao, C.. (2015). Trim26 negatively regulates interferon-β production and antiviral response through polyubiquitination and degradation of nuclear Irf3. PloS Pathog. 11 (3), e1004726. doi: 10.1371/journal.ppat.1004726
Wang, W., Zhou, Z., Xiao, X., Tian, Z., Dong, X., Wang, C., et al. (2021). SARS-Cov-2 Nsp12 attenuates type I interferon production by inhibiting Irf3 nuclear translocation. Cell Mol. Immunol. 18 (4), 945–953. doi: 10.1038/s41423-020-00619-y
Wan, D., Jiang, W., Hao, J. (2020). Research advances in how the cgas-sting pathway controls the cellular inflammatory response. Front. Immunol. 11, 615. doi: 10.3389/fimmu.2020.00615
West, A. P., Shadel, G. S. (2017). Mitochondrial dna in innate immune responses and inflammatory pathology. Nat. Rev. Immunol. 17 (6), 363–375. doi: 10.1038/nri.2017.21
Wong, N. H. M., Yuen, K., Chan, C., Jin, D.. (2017). Suppression of ifn-β production by Epstein-Barr virus lytic transactivator zta. Am. Assoc. Immnol. 214–15.
Wu, L., Cao, J., Cai, W. L., Lang, SM., Horton, JR., Jansen, DJ., et al. (2018). Kdm5 histone demethylases repress immune response via suppression of sting. PloS Biol. 16 (8), e2006134. doi: 10.1371/journal.pbio.2006134
Wu, J., Dobbs, N., Yang, K., Yan, N.. (2020). Interferon-independent activities of mammalian sting mediate antiviral response and tumor immune evasion. Immunity 53 (1), 115–126.e5. doi: 10.1016/j.immuni.2020.06.009
Wu, Y., Jin, S., Liu, Q., Zhang, Y., Ma, L., Zhao, Z., et al. (2021). Selective autophagy controls the stability of transcription factor Irf3 to balance type I interferon production and immune suppression. Autophagy 17 (6), 1379–1392. doi: 10.1080/15548627.2020.1761653
Wu, J. J., Li, W., Shao, Y., Avey, D., Fu, B., Gillen, J., et al. (2015). Inhibition of cgas dna sensing by a herpesvirus virion protein. Cell Host Microbe 18 (3), 333–344. doi: 10.1016/j.chom.2015.07.015
Wu, J., Sun, L., Chen, X., Du, F., Shi, H., Chen, C., et al. (2013). Cyclic gmp-amp is an endogenous second messenger in innate immune signaling by cytosolic dna. Science 339 (6121), 826–830. doi: 10.1126/science.1229963
Wu, Y., Zhang, H., Shi, Z., Chen, J., Li, M., Shi, H., et al. (2020). Porcine epidemic diarrhea virus Nsp15 antagonizes interferon signaling by rna degradation of Tbk1 and Irf3. Viruses 12 (6), 599. doi: 10.3390/v12060599
Wu, Z., Zhang, W., Wu, Y., Wang, T., Wu, S., Wang, M., et al. (2019). Binding of the duck tembusu virus protease to sting is mediated by Ns2B and is crucial for sting cleavage and for impaired induction of ifn-β. J. Immunol. 203 (12), 3374–3385. doi: 10.4049/jimmunol.1900956
Wu, S., Zhang, Q., Zhang, F., Meng, F., Liu, S., Zhou, R., et al. (2019). Her2 recruits Akt1 to disrupt sting signalling and suppress antiviral defence and antitumour immunity. Nat. Cell Biol. 21 (8), 1027–1040. doi: 10.1038/s41556-019-0352-z
Xia, P., Wang, S., Ye, B., Du, Y., Huang, G., Zhu, P., et al. (2015). Sox2 functions as a sequence-specific dna sensor in neutrophils to initiate innate immunity against microbial infection. Nat. Immunol. 16 (4), 366–375. doi: 10.1038/ni.3117
Xia, P., Wang, S., Ye, B., Du, Y., Li, C., Xiong, Z., et al. (2018). A circular rna protects dormant hematopoietic stem cells from dna sensor cgas-mediated exhaustion. Immunity 48 (4), 688–701. doi: 10.1016/j.immuni.2018.03.016
Xia, P., Ye, B., Wang, S., Zhu, X., Du, Y., Xiong, Z., et al. (2016). Glutamylation of the dna sensor cgas regulates its binding and synthase activity in antiviral immunity. Nat. Immunol. 17 (4), 369–378. doi: 10.1038/ni.3356
Xing, Y., Chen, J., Tu, J., Zhang, B., Chen, X., Shi, H., et al. (2013). The papain-like protease of porcine epidemic diarrhea virus negatively regulates type I interferon pathway by acting as a viral deubiquitinase. J. Gen. Virol. 94 (Pt 7), 1554–1567. doi: 10.1099/vir.0.051169-0
Xing, J., Ni, L., Wang, S., Wang, K., Lin, R., Zheng, C., et al. (2013). Herpes simplex virus 1-encoded tegument protein Vp16 abrogates the production of beta interferon (Ifn) by inhibiting nf-κb activation and blocking ifn regulatory factor 3 to recruit its coactivator cbp. J. Virol. 87 (17), 9788–9801. doi: 10.1128/JVI.01440-13
Xing, J., Zhang, A., Zhang, H., Wang, J., Li, XC., Zeng, MS., et al. (2017). Trim29 promotes dna virus infections by inhibiting innate immune response. Nat. Commun. 8 (1), 945. doi: 10.1038/s41467-017-00101-w
Xu, G., Liu, C., Zhou, S., Li, Q., Feng, Y., Sun, P., et al. (2021). Viral tegument proteins restrict cgas-dna phase separation to mediate immune evasion. Mol. Cell. 81 (13), 2823–2837. doi: 10.1016/j.molcel.2021.05.002
Xu, H., Su, C., Pearson, A., Mody, CH., Zheng, C.. (2017). Herpes simplex virus 1 Ul24 abrogates the dna sensing signal pathway by inhibiting nf-κb activation. J. Virol. 91 (7), e17–e25. doi: 10.1128/JVI.00025-17
Xu, J., Wang, P., Li, Z., Li, Z., Han, D., Wen, M., et al. (2021). Irf3-binding lncrna-isir strengthens interferon production in viral infection and autoinflammation. Cell Rep. 37 (5), 109926. doi: 10.1016/j.celrep.2021.109926
Yang, B., Liu, Y., Cui, Y., Song, D., Zhang, G., Ma, S., et al. (2020). Rnf90 negatively regulates cellular antiviral responses by targeting mita for degradation. PloS Pathog. 16 (3), e1008387. doi: 10.1371/journal.ppat.1008387
Yang, X., Shi, C., Li, H., Shen, S., Su, C., Yin, H., et al. (2022). March8 attenuates cgas-mediated innate immune responses through ubiquitylation. Sci. Signal 15 (732), k3067. doi: 10.1126/scisignal.abk3067
Yang, K., Xue, Y., Niu, H., Shi, C., Cheng, M., Wang, J., et al. (2022). African Swine fever virus Mgf360-11L negatively regulates cgas-Sting-Mediated inhibition of type I interferon production. Vet. Res. 53 (1), 7. doi: 10.1186/s13567-022-01025-0
Yang, Y., Ye, F., Zhu, N., Wang, W., Deng, Y., Zhao, Z., et al. (2015). Middle East respiratory syndrome coronavirus Orf4B protein inhibits type I interferon production through both cytoplasmic and nuclear targets. Sci. Rep. 5, 17554. doi: 10.1038/srep17554
Yan, Z., Wu, H., Liu, H., Zhao, G., Zhang, H., Zhuang, W., et al. (2021). The protein arginine methyltransferase Prmt1 promotes Tbk1 activation through asymmetric arginine methylation. Cell Rep. 36 (12), 109731. doi: 10.1016/j.celrep.2021.109731
Ye, R., Su, C., Xu, H., Zheng, C. (2017). Herpes simplex virus 1 ubiquitin-specific protease Ul36 abrogates nf-κb activation in dna sensing signal pathway. J. Virol. 91 (5), e2416–e2417. doi: 10.1128/JVI.02417-16
Ye, L., Zhang, Q., Liuyu, T., Xu, Z., Zhang, MX., Luo, MH., et al. (2019). Usp49 negatively regulates cellular antiviral responses via deconjugating K63-linked ubiquitination of mita. PloS Pathog. 15 (4), e1007680. doi: 10.1371/journal.ppat.1007680
Yi, G., Wen, Y., Shu, C., Han, Q., Konan, KV., Li, P., et al. (2016). Hepatitis c virus Ns4B can suppress sting accumulation to evade innate immune responses. J. Virol. 90 (1), 254–265. doi: 10.1128/JVI.01720-15
Yoh, S. M., Mamede, J. I., Lau, D., Ahn, N., Sanchez-Aparicio, MT., Temple, J., et al. (2022). Recognition of hiv-1 capsid by Pqbp1 licenses an innate immune sensing of nascent hiv-1 dna. Mol. Cell. 82 (15), 2871–2884. doi: 10.1016/j.molcel.2022.06.010
You, H., Lin, Y., Lin, F., Yang, M., Li, J., Zhang, R., et al. (2020). β-catenin is required for the Cgas/Sting signaling pathway but antagonized by the herpes simplex virus 1 Us3 protein. J. Virol. 94 (5), e1819–e1847. doi: 10.1128/JVI.01847-19
Yuan, S., Gao, X., Tang, K., Cai, JP., Hu, M., Luo, P., et al. (2022). Targeting papain-like protease for broad-spectrum coronavirus inhibition. Protein Cell 13 (12), 940–953. doi: 10.1007/s13238-022-00909-3
Yu, Q., Chu, L., Li, Y., Wang, Q., Zhu, J., Wang, C., et al. (2021). Mir-23a/B suppress cgas-mediated innate and autoimmunity. Cell Mol. Immunol. 18 (5), 1235–1248. doi: 10.1038/s41423-021-00668-x
Yuen, C., Chan, C., Fung, S., Wang, P., Wong, W., Tang, HV., et al. (2016). Suppression of type I interferon production by human T-cell leukemia virus type 1 oncoprotein tax through inhibition of Irf3 phosphorylation. J. Virol. 90 (8), 3902–3912. doi: 10.1128/JVI.00129-16
Yu, Y., Hayward, G. S. (2010). The ubiquitin E3 ligase raul negatively regulates type I interferon through ubiquitination of the transcription factors Irf7 and Irf3. Immunity 33 (6), 863–877. doi: 10.1016/j.immuni.2010.11.027
Yu, Z., Li, X., Yang, M., Huang, J., Fang, Q., Jia, J., et al. (2021). Trim41 is required to innate antiviral response by polyubiquitinating Bcl10 and recruiting nemo. Signal Transduct Target Ther. 6 (1), 90. doi: 10.1038/s41392-021-00477-8
Yu, K., Tian, H., Deng, H. (2020). Ppm1G restricts innate immune signaling mediated by sting and mavs and is hijacked by kshv for immune evasion. Sci. Adv. 6 (47), eabd0276. doi: 10.1126/sciadv.abd0276
Yu, X., Zhang, L., Shen, J., Zhai, Y., Jiang, Q., Yi, M., et al. (2021). The sting phase-separator suppresses innate immune signalling. Nat. Cell Biol. 23 (4), 330–340. doi: 10.1038/s41556-021-00659-0
Zeng, W., Xu, M., Liu, S., Chen, ZJ.. (2009). Key role of Ubc5 and lysine-63 polyubiquitination in viral activation of Irf3. Mol. Cell. 36 (2), 315–325. doi: 10.1016/j.molcel.2009.09.037
Zhang, M. X., Cai, Z., Zhang, M., Wang, XM., Wang, Y., Zhao, F., et al. (2019). Usp20 promotes cellular antiviral responses Via deconjugating K48-linked ubiquitination of mita. J. Immunol. 202 (8), 2397–2406. doi: 10.4049/jimmunol.1801447
Zhang, G., Chan, B., Samarina, N., Abere, B., Weidner-Glunde, M., Buch, A., et al. (2016). Cytoplasmic isoforms of kaposi sarcoma herpesvirus Lana recruit and antagonize the innate immune DNA sensor cgas. Proc. Natl. Acad. Sci. U S A. 113 (8), E1034–E1043. doi: 10.1073/pnas.1516812113
Zhang, J., Chen, Y., Chen, X., Zhang, W., Zhao, L., Weng, L., et al. (2021). Deubiquitinase Usp35 restrains sting-mediated interferon signaling in ovarian cancer. Cell Death Differ. 28 (1), 139–155. doi: 10.1038/s41418-020-0588-y
Zhang, M., Fu, M., Li, M., Hu, H., Gong, S., Hu, Q., et al. (2020). Herpes simplex virus type 2 inhibits type I ifn signaling mediated by the novel E3 ubiquitin protein ligase activity of viral protein Icp22. J. Immunol. 205 (5), 1281–1292. doi: 10.4049/jimmunol.2000418
Zhang, J., Hu, M. M., Wang, Y. Y., Shu, HB.. (2012). Trim32 protein modulates type I interferon induction and cellular antiviral response by targeting Mita/Sting protein for K63-linked ubiquitination. J. Biol. Chem. 287 (34), 28646–28655. doi: 10.1074/jbc.M112.362608
Zhang, W., Jiang, B., Zeng, M., Duan, Y., Wu, Z., Wu, Y., et al. (2020). Binding of duck tembusu virus nonstructural protein 2a to duck sting disrupts induction of its signal transduction cascade to inhibit beta interferon induction. J. Virol. 94 (9), e1819–e1850. doi: 10.1128/JVI.01850-19
Zhang, J., Li, Z., Huang, J., Chen, S., Yin, H., Tian, J., et al. (2020). Mir-101 inhibits feline herpesvirus 1 replication by targeting cellular suppressor of cytokine signaling 5 (Socs5). Vet. Microbiol. 245, 108707. doi: 10.1016/j.vetmic.2020.108707
Zhang, Y., Li, M., Li, L., Qian, G., Wang, Y., Chen, Z., et al. (2020). β-arrestin 2 as an activator of cgas-sting signaling and target of viral immune evasion. Nat. Commun. 11 (1), 1–15. doi: 10.1038/s41467-020-19849-9
Zhang, M., Liu, Y., Wang, P., Guan, X., He, S., Luo, S., et al. (2015). Hsv-2 immediate-early protein Us1 inhibits ifn-β production by suppressing association of irf-3 with ifn-β promoter. J. Immunol. 194 (7), 3102–3115. doi: 10.4049/jimmunol.1401538
Zhang, C., Shang, G., Gui, X., Zhang, X., Bai, XC., Chen, ZJ., et al. (2019). Structural basis of sting binding with and phosphorylation by Tbk1. Nature 567 (7748), 394–398. doi: 10.1038/s41586-019-1000-2
Zhang, X., Shi, H., Wu, J., Zhang, X., Sun, L., Chen, C., et al. (2013). Cyclic gmp-amp containing mixed phosphodiester linkages is an endogenous high-affinity ligand for sting. Mol. Cell. 51 (2), 226–235. doi: 10.1016/j.molcel.2013.05.022
Zhang, Q., Shi, K., Yoo, D. (2016). Suppression of type I interferon production by porcine epidemic diarrhea virus and degradation of creb-binding protein by Nsp1. Virology 489, 252–268. doi: 10.1016/j.virol.2015.12.010
Zhang, D., Su, C., Zheng, C. (2016). Herpes simplex virus 1 serine protease Vp24 blocks the dna-sensing signal pathway by abrogating activation of interferon regulatory factor 3. J. Virol. 90 (12), 5824–5829. doi: 10.1128/JVI.00186-16
Zhang, Q., Tang, Z., An, R., Ye, L., Zhong, B., et al. (2020). Usp29 maintains the stability of cgas and promotes cellular antiviral responses and autoimmunity. Cell Res. 30 (10), 914–927. doi: 10.1038/s41422-020-0341-6
Zhang, M., Tian, Y., Wang, R. P., Gao, D., Zhang, Y., Diao, FC., et al. (2008). Negative feedback regulation of cellular antiviral signaling by Rbck1-mediated degradation of Irf3. Cell Res. 18 (11), 1096–1104. doi: 10.1038/cr.2008.277
Zhang, M., Wang, L., Zhao, X., Zhao, K., Meng, H., Zhao, W., et al. (2012). Traf-interacting protein (Trip) negatively regulates ifn-beta production and antiviral response by promoting proteasomal degradation of tank-binding kinase 1. J. Exp. Med. 209 (10), 1703–1711. doi: 10.1084/jem.20120024
Zhang, L., Wei, N., Cui, Y., Hong, Z., Liu, X., Wang, Q., et al. (2018). The deubiquitinase cyld is a specific checkpoint of the sting antiviral signaling pathway. PloS Pathog. 14 (11), e1007435. doi: 10.1371/journal.ppat.1007435
Zhang, Z. D., Xiong, T. C., Yao, S. Q., Wei, MC., Chen, M., Lin, D., et al. (2020). Rnf115 plays dual roles in innate antiviral responses by catalyzing distinct ubiquitination of mavs and mita. Nat. Commun. 11 (1), 5536. doi: 10.1038/s41467-020-19318-3
Zhang, Z., Yuan, B., Bao, M., Lu, N., Kim, T., Liu, YJ., et al. (2011). The helicase Ddx41 senses intracellular dna mediated by the adaptor sting in dendritic cells. Nat. Immunol. 12 (10), 959–965. doi: 10.1038/ni.2091
Zhang, P., Yu, L., Dong, J., Liu, Y., Zhang, L., Liang, P., et al. (2020). Cellular Poly(C) binding protein 2 interacts with porcine epidemic diarrhea virus papain-like protease 1 and supports viral replication. Vet. Microbiol. 247, 108793. doi: 10.1016/j.vetmic.2020.108793
Zhang, M., Zhang, M. X., Zhang, Q., Zhu, GF., Yuan, L., Zhang, DE., et al. (2016). Usp18 recruits Usp20 to promote innate antiviral response through deubiquitinating Sting/Mita. Cell Res. 26 (12), 1302–1319. doi: 10.1038/cr.2016.125
Zhang, J., Zhao, J., Xu, S., Li, J., He, S., Zeng, Y., et al. (2018). Species-specific deamidation of cgas by herpes simplex virus Ul37 protein facilitates viral replication. Cell Host Microbe 24 (2), 234–248. doi: 10.1016/j.chom.2018.07.004
Zhang, Z., Zheng, Z., Luo, H., Meng, J., Li, H., Li, Q., et al. (2012). Human bocavirus Np1 inhibits ifn-β production by blocking association of ifn regulatory factor 3 with ifnb promoter. J. Immunol. 189 (3), 1144–1153. doi: 10.4049/jimmunol.1200096
Zhao, B., Du, F., Xu, P., Shu, C., Sankaran, B., Bell, SL., et al. (2019). A conserved Plplrt/Sd motif of sting mediates the recruitment and activation of Tbk1. Nature 569 (7758), 718–722. doi: 10.1038/s41586-019-1228-x
Zhao, M., Xia, T., Xing, J. Q., Yin, LH., Li, XW., Pan, J., et al. (2021). The stress granule protein G3Bp1 promotes pre-condensation of cgas to allow rapid responses to dna. EMBO Rep. 23 (1), e53166. doi: 10.15252/embr.202153166
Zhao, X., Zhu, H., Yu, J., Li, H., Ge, J., Chen, W., et al. (2016). C-Cbl-Mediated ubiquitination of Irf3 negatively regulates ifn-beta production and cellular antiviral response. Cell Signal. 28 (11), 1683–1693. doi: 10.1016/j.cellsig.2016.08.002
Zheng, Y., Liu, Q., Wu, Y., Ma, L., Zhang, Z., Liu, T., et al. (2018). Zika virus elicits inflammation to evade antiviral response by cleaving cgas Via ns 1-Caspase-1 axis. EMBO J. 37 (18), e99347. doi: 10.15252/embj.201899347
Zhong, B., Yang, Y., Li, S., Wang, YY., Li, Y., Diao, F., et al. (2008). The adaptor protein mita links virus-sensing receptors to Irf3 transcription factor activation. Immunity 29 (4), 538–550. doi: 10.1016/j.immuni.2008.09.003
Zhong, B., Zhang, L., Lei, C., Li, Y., Mao, AP., Yang, Y., et al. (2009). The ubiquitin ligase Rnf5 regulates antiviral responses by mediating degradation of the adaptor protein mita. Immunity 30 (3), 397–407. doi: 10.1016/j.immuni.2009.01.008
Zhou, C., Chen, X., Planells-Cases, R., Chu, J., Wang, L., Cao, L., et al. (2020). Transfer of cgamp into bystander cells Via Lrrc8 volume-regulated anion channels augments sting-mediated interferon responses and anti-viral immunity. Immunity 52 (5):767–781.e6. doi: 10.1016/j.immuni.2020.03.016
Zhou, Z., Zhang, X., Lei, X., Xiao, X., Jiao, T., Ma, R., et al. (2021). Sensing of cytoplasmic chromatin by cgas activates innate immune response in SARS-Cov-2 infection. Signal Transduct Target Ther. 6 (1), 382. doi: 10.1038/s41392-021-00800-3
Keywords: cGAS/STING signaling, innate immunity, virus, immune escape, PRRs
Citation: Ge Z and Ding S (2022) Regulation of cGAS/STING signaling and corresponding immune escape strategies of viruses. Front. Cell. Infect. Microbiol. 12:954581. doi: 10.3389/fcimb.2022.954581
Received: 27 May 2022; Accepted: 25 August 2022;
Published: 14 September 2022.
Edited by:
Annemarie H. Meijer, Leiden University, NetherlandsReviewed by:
Renate König, Paul-Ehrlich-Institut (PEI), GermanyMaria Teresa Sanchez-Aparicio, Icahn School of Medicine at Mount Sinai, United States
Copyright © 2022 Ge and Ding. This is an open-access article distributed under the terms of the Creative Commons Attribution License (CC BY). The use, distribution or reproduction in other forums is permitted, provided the original author(s) and the copyright owner(s) are credited and that the original publication in this journal is cited, in accordance with accepted academic practice. No use, distribution or reproduction is permitted which does not comply with these terms.
*Correspondence: Shuzhe Ding, c3pkaW5nQHR5eHguZWNudS5lZHUuY24=