- 1Biotechnology Unit, Technological Development Centre, Federal University of Pelotas, Pelotas, Rio Grande do Sul, Brazil
- 2Department of Medicine, University of Connecticut Health, Farmington, CT, United States
- 3School of Biosciences and Medicine, Faculty of Health and Medical Sciences, University of Surrey, Guildford, United Kingdom
Leptospirosis is a neglected disease of man and animals that affects nearly half a million people annually and causes considerable economic losses. Current human vaccines are inactivated whole-cell preparations (bacterins) of Leptospira spp. that provide strong homologous protection yet fail to induce a cross-protective immune response. Yearly boosters are required, and serious side-effects are frequently reported so the vaccine is licensed for use in humans in only a handful of countries. Novel universal vaccines require identification of conserved surface-exposed epitopes of leptospiral antigens. Outer membrane β-barrel proteins (βb-OMPs) meet these requirements and have been successfully used as vaccines for other diseases. We report the evaluation of 22 constructs containing protein fragments from 33 leptospiral βb-OMPs, previously identified by reverse and structural vaccinology and cell-surface immunoprecipitation. Three-dimensional structures for each leptospiral βb-OMP were predicted by I-TASSER. The surface-exposed epitopes were predicted using NetMHCII 2.2 and BepiPred 2.0. Recombinant constructs containing regions from one or more βb-OMPs were cloned and expressed in Escherichia coli. IMAC-purified recombinant proteins were adsorbed to an aluminium hydroxide adjuvant to produce the vaccine formulations. Hamsters (4-6 weeks old) were vaccinated with 2 doses containing 50 – 125 μg of recombinant protein, with a 14-day interval between doses. Immunoprotection was evaluated in the hamster model of leptospirosis against a homologous challenge (10 – 20× ED50) with L. interrogans serogroup Icterohaemorrhagiae serovar Copenhageni strain Fiocruz L1-130. Of the vaccine formulations, 20/22 were immunogenic and induced significant humoral immune responses (IgG) prior to challenge. Four constructs induced significant protection (100%, P < 0.001) and sterilizing immunity in two independent experiments, however, this was not reproducible in subsequent evaluations (0 – 33.3% protection, P > 0.05). The lack of reproducibility seen in these challenge experiments and in other reports in the literature, together with the lack of immune correlates and commercially available reagents to characterize the immune response, suggest that the hamster may not be the ideal model for evaluation of leptospirosis vaccines and highlight the need for evaluation of alternative models, such as the mouse.
Introduction
Leptospirosis is caused by pathogenic spirochetes of the genus Leptospira and has a high morbidity in tropical and subtropical countries (Costa et al., 2015; Picardeau, 2017; Baquero and Machado, 2018). The infection is one of the most widespread bacterial zoonosis in the world and is considered a serious public health problem. Humans are accidental and terminal hosts of the pathogen, exhibiting a wide variety of symptoms, ranging from non-specific fever, chills, headache and myalgia to severe leptospirosis, which can manifest as Weil’s disease or leptospirosis-associated pulmonary haemorrhage syndrome (McBride et al., 2005; Croda et al., 2010). Estimates of annual global incidence are of approximately 1 million human cases, but due to the neglected status of the disease this number is likely underestimated (Costa et al., 2015). The disease affects domestic and wild animals, causing economic losses in subsistence and industrial farming (Adler and de la Pena Moctezuma, 2010; Martins and Lilenbaum, 2017). Leptospirosis prevention is essential to reduce the rate of disease and to interrupt the transmission cycle.
Vaccination of human and animal populations in endemic regions is probably the most viable strategy to control the disease (Adler, 2015b; Costa et al., 2015; Grassmann et al., 2017b; Vernel-Pauillac and Werts, 2018). Commercially available whole-cell inactivated vaccines (bacterins) are routinely used in livestock and domestic animals throughout the world (Verma et al., 2013). Although protective against lethal infection, these vaccines elicit an immune response predominantly against leptospiral LPS, a T-independent antigen that induces a short-term immunity and requires annual booster immunizations. In addition, protection elicited by bacterins are restricted to the serovars included in the vaccine preparation. Some 64 different species of Leptospira have been described to date, 17 of them are potentially infectious and are classified into over 300 serovars (Adler and de la Pena Moctezuma, 2010; Vincent et al., 2019). Furthermore, bacterins are associated with adverse side-effects in humans, and this has limited their use to high-risk populations in only a few countries (Verma et al., 2013; Grassmann et al., 2017b; Vernel-Pauillac and Werts, 2018; Felix et al., 2020).
The high genomic and phenotypic diversity of pathogenic Leptospira spp. is a major drawback for vaccine development (Pereira et al., 2018). The development of a cost-effective vaccine with long-term protection against leptospirosis has been the goal of several research groups around the world, yet it remains elusive (Felix et al., 2020). A universal vaccine against leptospirosis will likely be multivalent, protective against most pathogenic Leptospira spp., induce long-term immunity, free of adverse effects and effective for both human and animal use. Most efforts have focused on developing a recombinant vaccine as a substitute for the bacterins (Dellagostin et al., 2017; Grassmann et al., 2017b; Silveira et al., 2017). Alternative approaches based on stimulating the innate immune system have shown interesting results (Potula et al., 2017; Vernel-Pauillac and Werts, 2018; Santecchia et al., 2019). Furthermore, live attenuated mutants have been developed that conferred cross-protective immunity to several pathogenic Leptospira spp. and serovars (Srikram et al., 2011; Murray et al., 2018; Wunder et al., 2021).
Reverse and structural vaccinology (RSV) has been successfully used in the design of more effective vaccines for several infectious diseases, reviewed in (Dormitzer et al., 2012; Liljeroos et al., 2015; Rappuoli et al., 2016; Cable et al., 2020). This approach allows a refined protein design for optimization of antigen structure. The application of structural vaccinology (SV) to leptospiral outer membrane proteins (OMPs) has proven useful towards the identification and localization of immunologically accessible epitopes which can bind to MHC-II receptors (Hsieh et al., 2017; Lata et al., 2018). Previously, we carried out a comprehensive bioinformatics analysis based on RSV that identified β-barrel transmembrane proteins (βb-OMPs) in L. interrogans (Grassmann et al., 2017a). βb-OMPs are of particular interest as they are integral components of the outer membrane (OM) in diderm bacteria (Schulz, 2002; Wilson and Bernstein, 2016), and usually play an essential role in the survival and successful infection of the host, such as nutrient acquisition and attachment (Adler, 2015a).
In addition, we used cell-surface immunoprecipitation (CSIP) to experimentally identify leptospiral proteins localized on the cell surface of host-adapted L. interrogans (Cunha et al., 2017). This immunoproteomics technique was originally developed to identify proteins in Neisseria meningitidis using intact meningococcal cells with patient immune sera and identifying precipitated proteins by mass spectrometry (Mendum et al., 2009; Newcombe et al., 2014). This method was successful in identifying protective antigens, including several components of the 4CMenB vaccine (Serruto et al., 2012). Host-adapted leptospires were subjected to CSIP with sera from convalescent leptospirosis patients and the immunoprecipitated proteins were identified by mass spectrometry to detect potentially immunoprotective seroreactive proteins.
In the current study, we report the application of RSV and CSIP for the selection and design of recombinant 22 constructs based on 33 newly described leptospiral OMPs. Structural modelling of these proteins allowed us to predict surface-exposed regions and to identify B-cell and major histocompatibility complex (MHC-II) binding epitopes. Recombinant proteins (individual or combined in chimeras) were evaluated as vaccine candidates in the hamster model of acute leptospirosis.
Results
Identification of the 33 leptospiral proteins used as vaccine candidates
Using an RSV approach, our group previously identified 165 putative leptospiral βb-OMPs, representing novel vaccine candidates (Grassmann et al., 2017a). In addition, we adapted the CSIP technique (Mendum et al., 2009; Newcombe et al., 2014) to confirm these findings in vitro (Cunha et al., 2017). Using CSIP we identified 157 immunogenic proteins expressed in host-adapted leptospires and recognized by convalescent human patient sera (unpublished data). In the present study, we selected 33 of these proteins for evaluation as 22 novel vaccine candidates against leptospirosis. Of these, six proteins were identified by both RSV and CSIP techniques, and the remaining 27 proteins were identified by RSV (Table 1). The selection criteria focused on exposure on the leptospiral cell surface, and was based on their predicted 3D structure and function (Table 1). Based on the identities predicted by RSV we selected several OM transporter families, including: eight TonB-dependent receptors (TBDR): LIC10714, LIC10881*, LIC10896*, LIC10964, LIC11268, LIC12374, LIC20151 and LIC20214; three alginate exporters (AlgE): LIC13229, LIC13417*, LIC13477 (*, see below); one orthologue for the COG4313 channel family: LIC11086; and six putative porins: LIC10544, LIC11271, LIC11366, LIC11506 (OmpG), LIC11975, LIC20019. Seven proteins were also predicted to be orthologues of OM efflux proteins (OEPs) and components of the type I secretion system: LIC10496 (TolC), LIC11941, LIC12307, LIC12575, LIC12693, LIC12990, LIC13135; and the leptospiral GspD orthologue LIC11570, a secretin in the type II secretion system. Proteins from other families with essential roles in OM biosynthesis such as the leptospiral LptD orthologue (LIC11458); a protein that is part of the subfamily of the FadL fatty acid transporter family (LIC11211); and five proteins from the Omp85 family: LIC10539, LIC11623 (BamA), LIC12252, LIC12254 and LIC12258 were also included.
Multiple sequence alignments of LIC13417 and its orthologues strongly suggested that it was likely to be a truncated protein, similar to our previous observations for LIC10881* and LIC10896* (Grassmann et al., 2017a). An analysis of the LIC13417 locus in the L. interrogans Fiocruz L1-130 genome, revealed the presence of a potentially erroneous stop codon in the last nucleotide of LIC13417. When the point mutation was altered to a serine codon (TAG → TCG), we reassembled LIC13417 and LIC13418 as a single CDS, named hereafter as LIC13417*. A multiple sequence alignment of LIC13417* and its orthologues showed an alignment with 80-97% amino acid identity (AAI) over the full-length of the modified protein (Figure S1 in Supplementary Material).
Conservation of the vaccine candidates among the pathogenic Leptospira spp.
The level of conservation of the vaccine candidates among nine pathogenic Leptospira spp. was evaluated by multiple sequence alignment. The alignments were performed using the full-length amino acid sequences of the proteins from the L. interrogans Fiocruz L1-130 genome and their orthologues in nine additional pathogenic species (Figure S2 in Supplementary Material). Overall, the proteins showed high levels of conservation; the AAIs ranged from 60.4 – 99.0% among the pathogenic species. Of the selected proteins, 32/33 had orthologues among the eight species that belong to node 1 in the P1 subclade of pathogenic Leptospira spp. (Vincent et al., 2019). While LIC10496 was the least conserved protein, it was only found in L. interrogans, L. kirschneri and L. noguchii, these species are generally recognised as the most virulent species of the P1 subclade, and most often associated with severe leptospirosis in humans. The βb-OMPs in these three species were highly conserved, with AAIs > 90% in 31/33 of the vaccine targets. LIC10496 (TolC) and LIC11506 (OmpG) were the least conserved, while those with essential roles in metabolism, such as LIC11623 (BamA), LIC11570 (GspD) and LIC11458 (LptD), demonstrated high AAI (88.6 – 99.0%) among the genomes analysed.
Epitope prediction in the surface exposed regions of the vaccine candidates
As opsonophagocytosis likely plays an important role in the clearance of leptospires during an infection, we predicted the immunogenic potential of the selected βb-OMPs to induce T- and B-cell responses. NetMHCII 2.2 server and BepiPred 2.0 were used to predict epitopes that could bind to MHC class II molecules encoded by 51 HLA-DRB alleles and linear B-cell epitopes, respectively. The 3D models of each βb-OMPs were aligned with their corresponding analogues, as indicated by I-TASSER (Table 1). All the βb-OMPs were predicted to contain surface-exposed linear B-cell epitopes. T-cell epitopes to all 51 of the HLA-DRB alleles were identified in surface-exposed regions in all the βb-OMPs apart from LIC13229, which did not contain epitopes for the DRB1_1502 allele. The list of the T- and B-cell epitopes identified for each βb-OMP is provided (Table S1 in Supplementary Material).
Construction of leptospiral βb-OMP vaccine candidates
Using the data from the structure and function analysis together with the epitope-mapping results, we constructed 22 recombinant proteins containing surface-exposed regions from the 33 leptospiral βb-OMPs for evaluation as vaccine candidates. Three different cloning strategies were used: 1) surface-exposed regions, n = 10 (Figure 1A); 2) full-length proteins, n = 3 (Figure 1B); 3) chimeras containing combinations of surface-exposed regions from 31 βb-OMPs, n = 9 (Figure 2). Details of the 22 recombinant proteins and the 33 βb-OMPs used in their construction are provided (Table S2 in Supplementary Material). The recombinant proteins were characterised by immunoblotting with an anti-His antibody, see (Figure S3 in Supplementary Material).
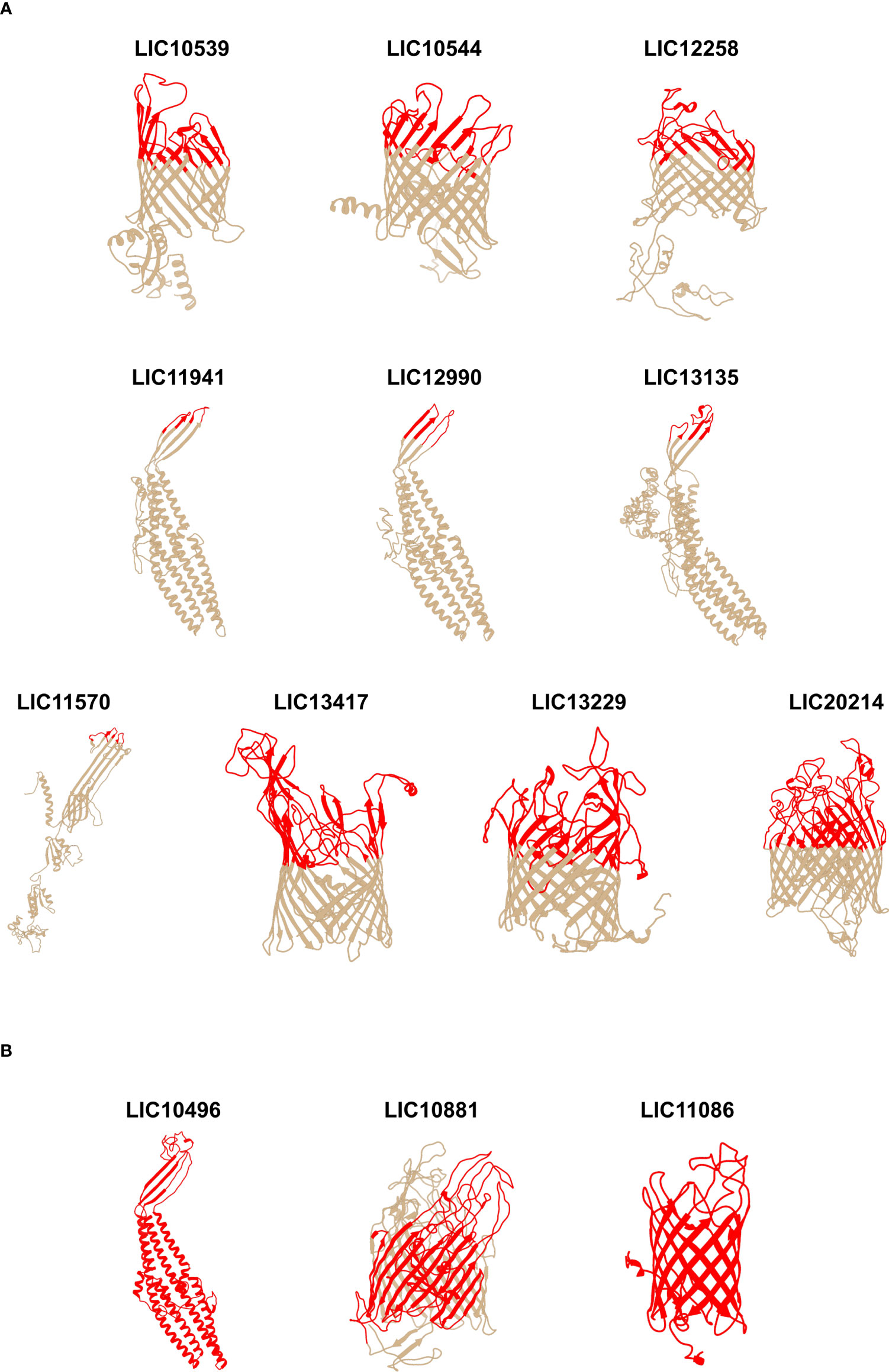
Figure 1 Predicted 3D models of the individual leptospiral β-barrel OMPs and mapping of the surface-exposed regions. The 3D models were determined using I-TASSER and the images were generated using UCSF Chimera software. (A) surface-exposed regions of 10 βb-OMPs (constructs do not contain transmembrane or periplasmic regions); (B) full-length protein constructs. The orientation of the βb-OMPs in the OM was derived from an interpretation of the best matching PDB structure. The upper, surface-exposed regions are shown in colour on the 3D models and these regions were used in the design of the recombinant proteins. The horizontal bars under the 3D models are a graphical representation of the composition of the chimeras.
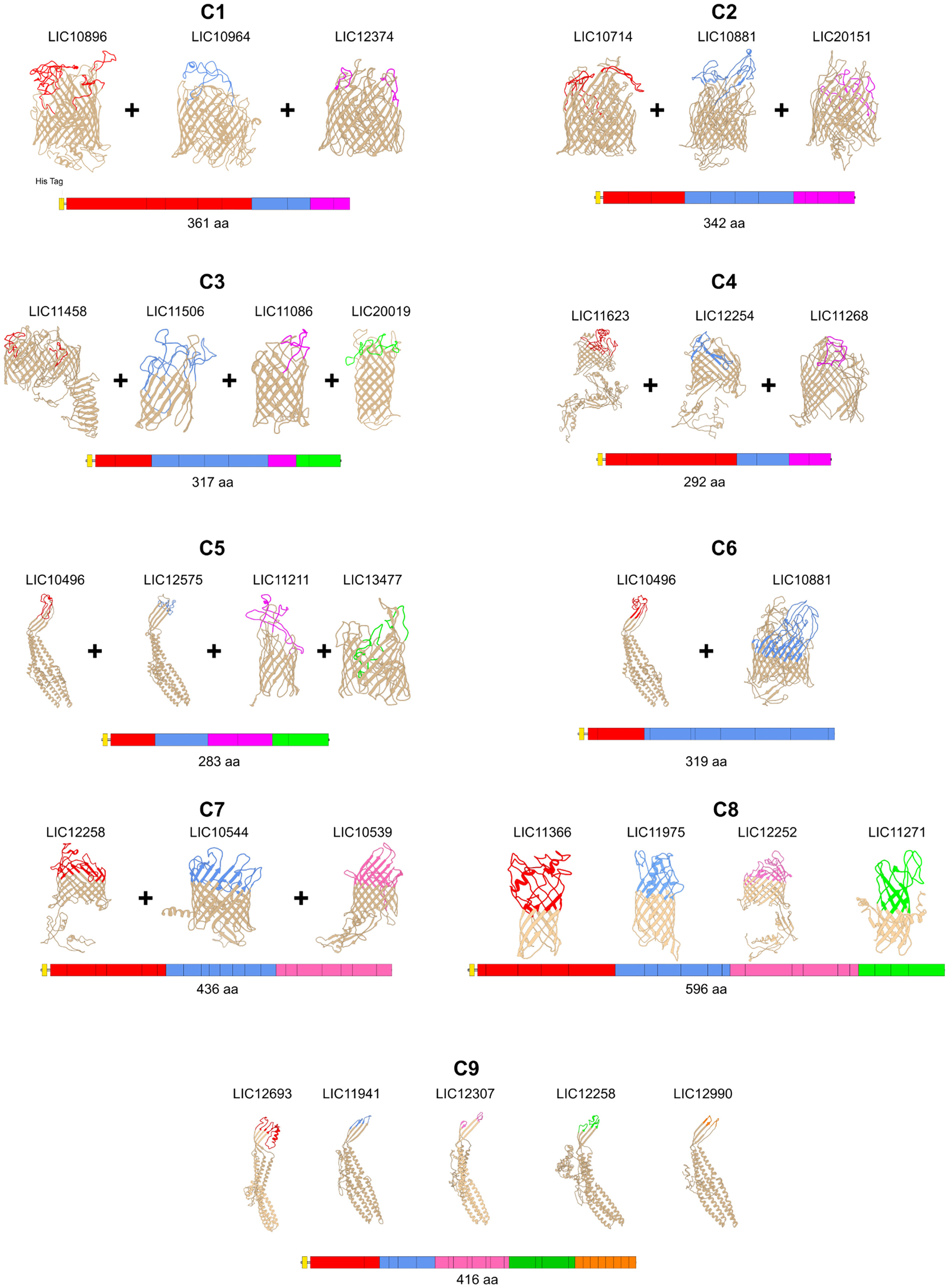
Figure 2 Predicted 3D models of the chimeric leptospiral β-barrel OMPs and mapping of the surface-exposed regions. Chimeras containing combinations of surface-exposed regions are shown. The 3D models were determined using I-TASSER and the images were generated using UCSF Chimera software. The orientation of the βb-OMPs in the OM was derived from an interpretation of the best matching PDB structure. The upper, surface-exposed regions are shown in colour on the 3D models and these regions were used in the design of the recombinant proteins. The horizontal bars under the 3D models are a graphical representation of the composition of the chimeras.
Evaluation of βb-OMP vaccine candidates against lethal leptospirosis
In total, thirteen experiments were performed to determine the efficacy of 22 vaccine candidates that were based on 33 βb-OMPs identified by RSV and CSIP, (Figure 3 and Table 2). The vaccination scheme used two doses that ranged from 50 – 125 μg total recombinant protein, adsorbed to an aluminium hydroxide adjuvant and administered intramuscularly. The endpoint for 50% (ED50) of infected hamsters was calculated as described previously (Conrad et al., 2017) and was approximately 5 leptospires for the L. interrogans Fiocruz L1-130 challenge strain. In two independent experiments, rLIC11570 (GspD), rLIC13229 (AlgE), rLIC13417* (AlgE) and rLIC20214 (TBDR) conferred significant protection in 100% of vaccinated animals (P < 0.0001), see (Figure 3A and Table 2). However, when we re-evaluated these vaccine candidates in an additional three independent experiments, they failed to induce a significant protective immune response; protection ranged from 0 – 30% (Figures 2B–D and Table 2). Endpoint criteria were observed in the control and vaccinated groups from days 9 – 13 post-challenge (PC), these animals were immediately euthanized, and all survivors were euthanized on day 28. Among the OEPs, only rLIC13135 significantly increased survival (Log-rank, P < 0.05) among vaccinated hamsters, (Figure 3E; Table 2). For LIC11941 and LIC12990, while the level of protection was the same as LIC13135 (44.4%), these results were not significant in terms of mortality or survival. An additional experiment failed to improve on these initial findings (Figure 3F; Table 2). Hamsters exhibited endpoint criteria on days 10 – 15 PC. LIC10539 (Omp85), LIC10544 (porin) and LIC12258 (Omp85) failed to induce significant protection in vaccinated animals, which ranged from 0 – 22.2% (Figures 3G–H; Table 2). Most endpoint criteria were observed on days 9 – 14 PC. For the remaining βb-OMPs, LIC10496 (TolC), LIC10881* (TBDR) and LIC11086 (AlgE), protection was not significant; ranging from 0 – 20%, see (Figure 3I; Table 2). Hamsters exhibited endpoint criteria on days 10 – 14 PC. Vaccine preparations based on the chimera constructs (C1 – C9) contained 25 μg for each protein in the chimera; each dose ranged from 50 – 125 μg total recombinant protein. In three independent experiments, most of the chimeras failed to induce significant protective responses, which ranged from 0 – 22.2%, see (Figures 3J–L; Table 2). However, vaccination with chimeras C1 (TBDRs: LIC10896* + LIC10964 + LIC12374) and C3 (LIC11458 (LptD) + LIC11506 (OmpG) + LIC11086 (OM channel) + LIC20019 (porin)) resulted in increased survival compared to the control group (Log-rank, P < 0.05), see (Figure 3I; Table 2). Hamsters exhibiting endpoint criteria were observed on days 7 – 20 PC.
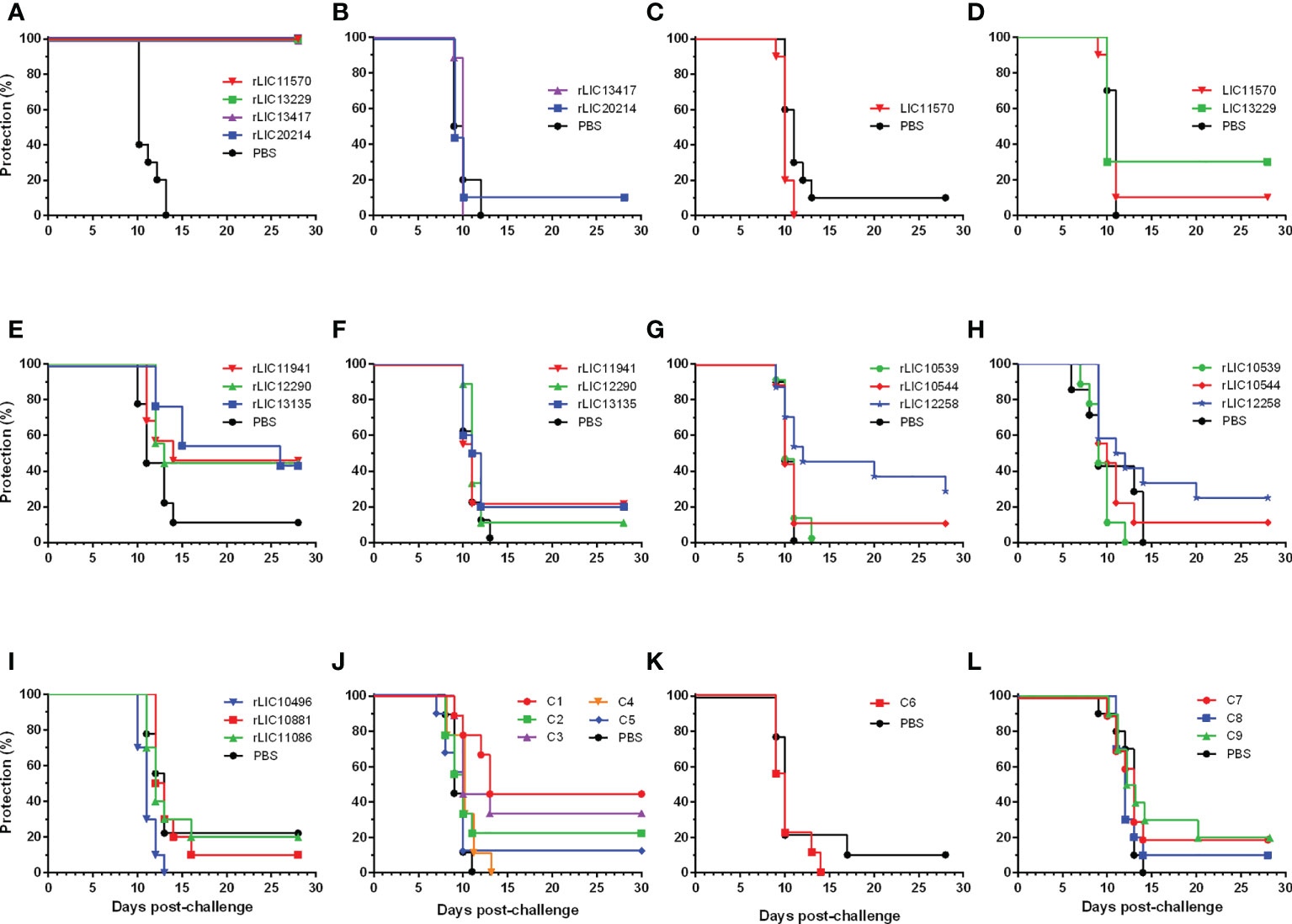
Figure 3 Protection against lethal challenge in the hamster model of acute leptospirosis. Representative experiments showing survival of hamsters vaccinated (days -28 and -14) with two doses of the rβb-OMPs vaccine candidates or injected with a PBS control, followed by challenge (day 0) with a lethal dose of L. interrogans serovar Copenhageni strain Fiocruz L1-130, see (Table 2). Groups of hamsters were vaccinated with: (A) rLIC11570 (GspD), rLIC13229 (AlgE), rLIC13417* (AlgE), rLIC20214 (TBDR) or the PBS control. All the rβb-OMPs induced 100% protection (P < 0.0001) and sterilizing immunity; (B–D) repeat experiments failed to reproduce the protective immune response seen previously; (E) vaccinated with the OEPs rLIC11941, rLIC12290, rLIC13135 or the PBS control. While the level of protection was the same (44.4%) for all proteins, only rLIC13135 significantly increased survival (P < 0.05) among vaccinated hamsters compared to the PBS control group; (F) a repeat experiment using the OEPs failed to reproduce these results; (G, H) vaccinated with rLIC10539 (Omp85), rLIC10544 (porin), rLIC12258 (Omp85) or the PBS control. None of the vaccine preparations protected against the challenge dose; (I), vaccinated with rLIC10496 (TolC), rLIC10881* (TBDR), rLIC11086 (AlgE) or the PBS control. None of the vaccine preparations protected against the challenge dose; (J), hamsters were vaccinated with chimeras C1-C5 or the PBS control. While none of the chimeras protected against the lethal challenge, chimeras C1 (TBDRs: LIC10896* + LIC10964 + LIC12374) and C3 (LIC11458 (LptD) + LIC11506 (OmpG) + LIC11086 (OM channel) + LIC20019 (porin)) significantly increased survival compared to the PBS control group; (K, L), hamsters vaccinated with chimeras C6-C9 or the PBS control group. None of the chimeras induced a significant protective immune response.
Humoral immune response in vaccinated hamsters
The specific humoral immune response was evaluated by ELISA using serum samples collected on day 0 (pre-immune) and day 28 post-immunization and an anti-hamster IgG secondary antibody (Figure 4). Of the recombinant constructs, 20/22 induced significant (P < 0.05) levels of IgG antibodies in vaccinated hamsters. Only the chimeras C2 (LIC10714 + LIC10881* + LIC20151) and C4 (LIC11623 + LIC12254 + LIC11268) failed to induce significant levels of circulating anti-βb-OMPs antibodies compared to the pre-immune sera (Figure 4). An analysis of IgG subclasses was performed on serum samples collected from animals that survived challenge (Figure S5). Compared to the PBS/Alhydrogel control group, immunization with rLIC11570, stimulated significant levels of IgG1 and IgG2 (P < 0.05). rLIC13229, and rLIC13417* induced a predominantly IgG2 response (P < 0.05), while rLIC20214 induced significant production of IgG2 and IgG3 (P < 0.05) compared to the control group. No detectable levels of antibodies were found in the pre-immune samples (data not shown).
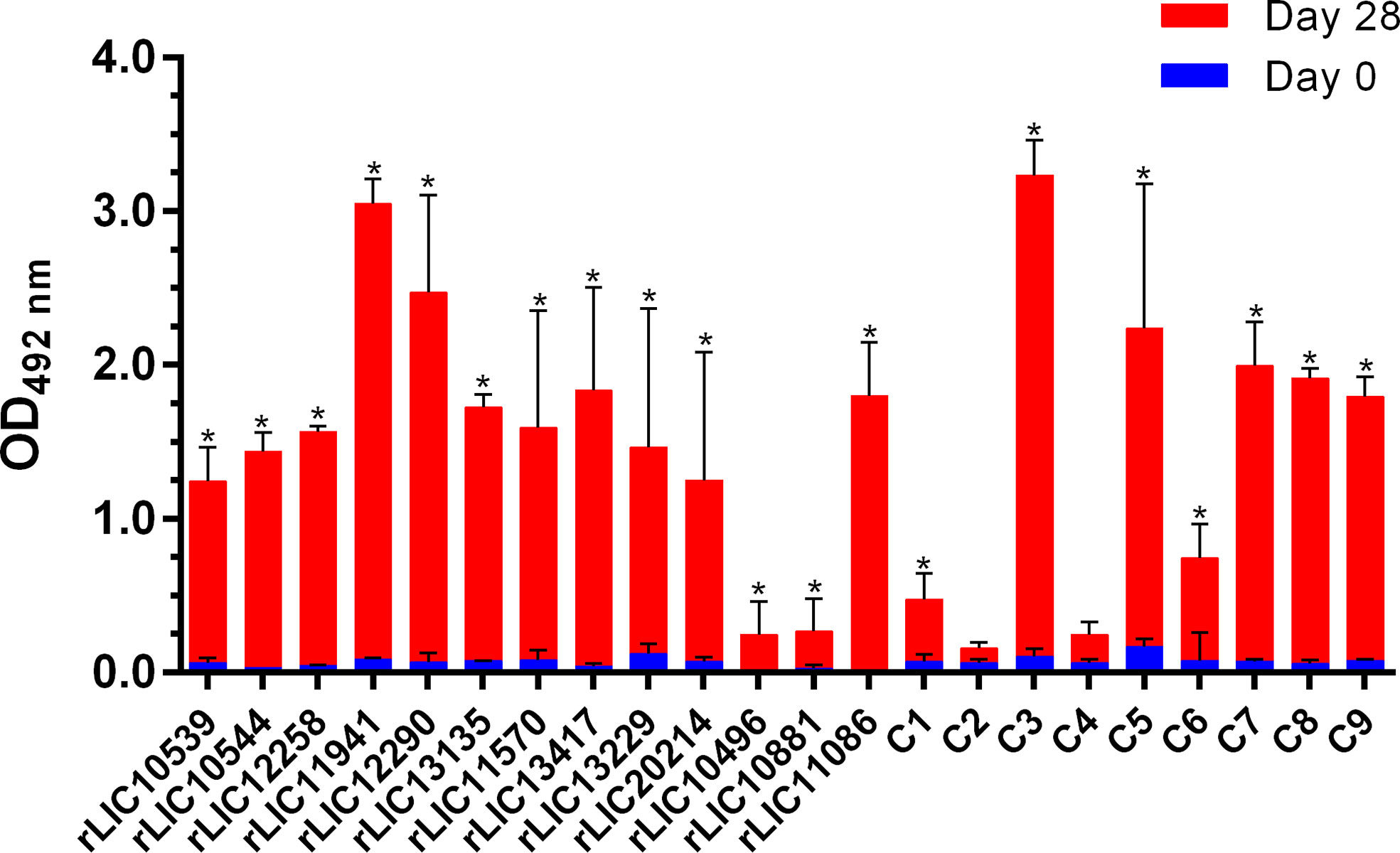
Figure 4 Specific humoral immune response (IgG) in vaccinated hamsters. ELISAs were carried out to determine the antibody levels in hamsters vaccinated with the recombinant proteins or the PBS control (Table 2). Pre-immune and pre-challenge serum samples were characterized using an anti-hamster IgG secondary antibody. The serum samples were diluted at 1:100 except for rLIC10881 and C6 (1:50), rLIC11570, rLIC13229, rLIC13417*, rLIC20214 (1:200) and LIC10496 and LIC11086 (1:400). The results represent the mean optical density (OD492) ± standard deviation (bars) calculated from individual serum samples assayed in triplicate. Significance differences (P < 0.05) were determined by one-way ANOVA (Tukey multiple comparison). *, indicates a significant difference between pre-immune sera and day 28 post immunization.
Discussion
The development of a universal vaccine against leptospirosis is a challenge due to the wide antigenic diversity of pathogenic Leptospira spp. (Picardeau, 2017; Vincent et al., 2019). RSV are in silico, high-throughput, approaches that identify all proteins, in a given genome, that are localised to the OM and that are surface-exposed (Rappuoli et al., 2016). RSV has been applied to a wide range of microorganisms and has achieved critical success in the discovery of novel vaccine candidates, reviewed in (Dormitzer et al., 2012). We applied this approach to the L. interrogans Fiocruz L1-130 genome, initially identifying 165 βb-OMPs that were refined to 18 surface-exposed, highly conserved βb-OMPs among the pathogenic Leptospira spp. (Grassmann et al., 2017a). For this study we focused on the βb-OMPs, often regarded as low-hanging-fruit, as they are among the easiest transmembrane proteins to predict in Gram-negative bacteria due to their 3D structure and that they are only present in the OM (Schulz, 2002). We updated the original βb-OMP list and selected 33 novel surface-exposed proteins for evaluation in the hamster model of leptospirosis. To further validate our in silico findings, we cross-checked them with the latest data from our CSIP experiment and found experimental evidence that six of the proteins were recognised by patient sera (Table 1). Immunoinformatics analysis and 3D structural modelling of the βb-OMPs allowed us to design 22 recombinant constructs such that each βb-OMP was evaluated in one or more challenge experiments. The recombinant constructs were based on either: full-length proteins; surface-exposed regions; or chimeras containing surface-related immunogenic epitopes (SRIEs) from 2 – 5 βb-OMPs. When considering a universal vaccine, one of the main criteria is that vaccine candidates should be well conserved among the pathogenic species of the target microorganism. Of the vaccine candidates selected in this study, 31/33 contained orthologues in the pathogenic Leptospira spp. most often associated with human disease (Vincent et al., 2019), and with AAIs > 60%, (Figure S2 in Supplementary Material). Although LIC10496 was only present in L. interrogans, L. kirschneri and L. noguchii, we included this vaccine candidate as these three species are most likely to cause severe human leptospirosis.
In two experiments, we observed 100% immunoprotection and sterilizing immunity in hamsters vaccinated with either rLIC13229, rLIC13417, rLIC11570 or rLIC20214, see (Figure 3A and Table 2). In addition, rLIC13135, C1 (LIC10896* + LIC10964 + LIC12374) and C3 (LIC11458 + LIC11506 + LIC11086 + LIC20019) significantly increased survival in vaccinated animals compared to the control groups. Moreover, four rβb-OMPs elicited significant levels of IgG antibodies, characterized by several IgG subclasses, indicative of both Th1 and Th2 profiles (Mosmann & Coffman, 1989). These observations are in broad agreement with previous studies on recombinant subunit vaccines against leptospirosis (Lin et al., 2016; Conrad et al., 2017; Fernandes et al., 2017; Oliveira et al., 2018). However, the protective results were not reproducible in subsequent experiments, see (Table 2). There is little information as to the function of these proteins in Leptospira spp. and most are annotated as conserved hypothetical proteins. LIC13229 was reported to be excreted in the urine of infected hamsters (Segawa et al., 2014) and its gene expression was downregulated by temperature shift from 37°C to 28°C (Qin et al., 2006), suggesting that LIC13229 is involved in infection. LIC13417* gene expression was significantly downregulated in DMCs, with a possible role in the early stage of infection (Caimano et al., 2014). In agreement with our RSV analysis, several reports identified LIC20214 as an OMP and potential vaccine candidate by genome comparative and reverse vaccinology studies (Louvel et al., 2006; Viratyosin et al., 2008; Zeng et al., 2017). Over the course of 13 independent experiments, we evaluated different lots of recombinant proteins, alhydrogel adjuvant and batches of hamsters, without the success seen in the initial experiments. These findings and our previous experience together with those reported by other research groups has led us to question the suitability of the hamster model for the evaluation of vaccine candidates against leptospirosis, we discuss this further below.
OMPs are considered important cellular structures of the OM of Gram-negative bacteria and therefore represent good vaccine candidates (Silhavy et al., 2010; Sperandeo et al., 2019; Walker and Black, 2021). They are frequent targets for antibody-based therapies and vaccines for several reasons: OMPs contain surface-exposed epitopes, making them potentially accessible to antibodies or T-cell receptors; they are involved in essential cellular functions such as adhesion, biofilm formation, regulation of quorum sensing, and the export of toxic substances; these proteins tend to be conserved and highly expressed, thereby increasing their bioavailability (Maiti et al., 2020). RV uses a genomics-based approach to identify all potentially surface-exposed proteins (Rappuoli et al., 2016). SV takes RV a step further and identifies SRIEs based on the structural characteristics (3D models) of the OMPs (Cozzi et al., 2013). The ultimate goal is to identify and select protein targets that can elicit robust immune and memory responses, leading to specific and lasting protection (De Temmerman et al., 2011).
Antigen presentation other than linear epitopes has been largely overlooked during vaccine development, with a few exceptions (Faisal et al., 2009a; Faisal et al., 2009b). The use of subunit vaccine formulations could play a role in the failures observed, despite the promising results from the immunoinformatics analyses. In the current study, we evaluated three TBDRs in a recombinant chimera construct (C1). While C1 failed to induce significant protection, it did significantly increase survival (P < 0.05, Log-rank) compared to the control group (Figure 3J, Table 2). This can be an indication that the TBDRs were promising vaccine candidates and if they were presented to the immune system using an alternative approach, it could result in an improvement. As seen when a Mycobacterium bovis BCG vector vaccine expressing a chimera of these TBDRs was used to vaccinate hamsters, 100% protection and sterilizing immunity was observed (Bettin et al., 2022).
All 33 of the βb-OMPs in the current study contained putative antigenic B and T-cell epitopes on the surface-exposed regions used for construction of the chimeras. These SRIEs were predicted to induce humoral as well as cellular immunity. Despite the observation that 20/22 of the recombinant constructs were immunogenic, the use of linear epitopes may have limited their impact. The practical challenges associated with the insoluble expression of recombinant βb-OMPs resulted in their exclusion from the Neisseria meningitidis serogroup B vaccine (Pizza et al., 2000). However, an OM vesicle vaccine preserved the conformational epitopes of the native proteins and improved protection in clinical trials (Awanye et al., 2019). The use of OM vesicles in vaccines for the delivery of βb-OMPs is an interesting approach for the maintenance of conformational epitopes (van de Waterbeemd et al., 2010). The importance of conformational epitopes was demonstrated using a Chlamydial OM complex (COMC) from Chlamydia muridarum that contained several OMPs (Yu et al., 2020). The COMC vaccine was highly immunogenic and protected against infection. However, when the COMC vaccine was denatured protection was significantly reduced, suggesting conformational epitopes were required for protection. Furthermore, the first report of protection against leptospirosis reported synergy between OmpL1 and LipL41 when expressed as E. coli membrane-associated proteins (Haake et al., 1999).
Another potential problem is the accessibility of antibodies to βb-OMPs in Gram-negative bacteria, with several reports of a shielding effect by lipopolysaccharides (LPS), resulting in the lack of a protective immune response (van der Ley et al., 1986; Bentley and Klebba, 1988; Murphy et al., 1990; Michaelsen et al., 2001; Patel et al., 2016; Dominguez-Medina et al., 2020). This shielding effect was proposed as an evolutionary advantage for Gram-negative pathogens. Patel and colleagues modelled the ability of LPS to interact with the E. coli OmpF polypeptide, effectively camouflaging its epitopes from host immune recognition during infection (Patel et al., 2016). Antibody accessibility to OM proteins in Leptospira spp. is another issue that should be considered, especially given its unusual LPS composition (Vinh et al., 1986; Que-Gewirth et al., 2004).
Antibodies have several biological effects against extracellular pathogens, such as neutralization, phagocytosis, antibody-dependent cellular cytotoxicity and complement-mediated lysis (Heesterbeek et al., 2018; Siegrist, 2018). In the current study, the induction of significant levels of total IgG were observed for 20/22 of the recombinant constructs evaluated (Figure 4). However, the immunoglobulin levels did not correlate with survival, as previously observed with other vaccine candidates (Coutinho et al., 2011; Monaris et al., 2015; Conrad et al., 2017; Raja et al., 2018). In contrast to the bacterin vaccines, the available data for recombinant vaccines suggests that a humoral response is not sufficient to clear the bacteria from the host. These findings suggest that a more complex immune response is required to control the infection, perhaps one involving cellular immunity. Furthermore, positive correlations were observed between TNF-α, IL-10, IL-4, IL-12p40 and IFN-γ mRNA levels and heterologous protection in animals vaccinated with RecA and FliD using a prime-boost protocol; a RecA/FliD recombinant vaccine only induced partial protection (Raja et al., 2018). Again, there was no observable association between antibody levels and protection. While it is only possible infer cytokine levels in the hamster model by quantitative real-time RT-PCR, the potential lack of correlation between mRNA levels and protein abundance means that data interpretation requires caution (Koussounadis et al., 2015; Liu et al., 2016).
Recombinant proteins produced in E. coli are often contaminated with LPS, this endotoxin is a known stimulator of the immune system and is the main cause of septic shock during a bacterial infection. The presence of endotoxins in vaccine preparations is strictly controlled during clinical trials as even trace amounts can have a major effect (Petsch and Anspach, 2000; Wakelin et al., 2006). Given the ability of endotoxins to stimulate the immune system, their presence in recombinant vaccines requires further study. Of note, the C3H/HeJ mouse model is resistant to LPS shock, further supporting its use as an alternative model for the evaluation of vaccine candidates (Gomes-Solecki et al., 2017; Shetty et al., 2021; Kundu et al., 2022).
The absence of correlation between antibody levels and protection induced by recombinant vaccines, as well as a potential role for cellular immunity in a protective immune response, represent key challenges for the discovery of vaccine candidates. The lack of immune correlates is a major limitation to screening the hundreds of novel targets identified by RSV and CSIP (Grassmann et al., 2017b; Felix et al., 2020; Vernel-Pauillac et al., 2021). It is only possible to screen significantly reduced numbers of vaccine candidates using animal models, with the possibility that promising targets may be missed. The discovery of an immune correlate would substantially reduce the use of animals and allow the screening of hundreds of targets. However, despite the evaluation of opsonophagocytosis assays developed in other spirochaetes such as Treponema and Borrelia spp. (Cruz et al., 2008; Hawley et al., 2017), this remains elusive for Leptospira spp.
Syrian hamsters (Mesocricetus auratus) have long been used as a model to isolate Leptospira spp., recover virulence in laboratory-attenuated strains, investigate aspects of pathogenesis, screen for the virulence of mutant strains and to evaluate vaccine candidates in research laboratories (Haake, 2006; Zuerner, 2015). Additionally, hamsters are used to evaluate the efficacy of commercial vaccines against leptospirosis (Srinivas et al., 2013). They are the preferred model as hamsters are susceptible to the disease, recapitulate the symptoms of severe human leptospirosis and are easily bred in animal units, while mice and rats are naturally resistant to leptospirosis, reviewed in (Gomes-Solecki et al., 2017). Nevertheless, the absence of immune correlates and the lack of commercially available reagents to study the immune response in hamsters has hampered further advances in the field (Adler, 2015b; Felix et al., 2020). Hamsters are notoriously susceptible to leptospirosis and can succumb to infection with as little as one leptospire (Haake, 2006). The hamster model is therefore of limited use as a model of sublethal or chronic infection.
In contrast, most natural hosts of leptospires develop chronic infection with kidney colonization, urinary shedding and little or no clinical signs of disease (Athanazio et al., 2008; Richer et al., 2015; Zuerner, 2015; Gomes-Solecki et al., 2017). The mouse model has been used for the evaluation of vaccine candidates; it was used in the first evaluation of a LigA vaccine (Koizumi and Watanabe, 2004). Importantly, there is an abundance of commercially available reagents for the characterisation of the immune response. In addition, there are mouse mutants e.g., the C3H/HeJ TLR4 mutant, SCID and Rag1 KO mice, which are susceptible to leptospiral infection (Bandeira et al., 2011; Shetty et al., 2021; Grassmann et al., 2021; Kundu et al., 2022). The use of a mouse model would allow the study of both lethal and sublethal forms of leptospirosis, potentially allowing the discovery of immune correlates that can be used to screen vaccine candidates (Pereira et al., 1998; Santos et al., 2010; Gomes-Solecki et al., 2017; Felix et al., 2020).
The limitations of the current study include the following: the influence of endotoxins on the immune response induced by the recombinant vaccine preparations remains unknown and requires further study; due to a lack of commercially available reagents, the cellular response cannot be evaluated in the hamster model; and the lack of immune correlates for leptospirosis is a major limitation for the screening of vaccine candidates in vitro. The use of the C3H/HeJ mouse model could resolve the first two limitations; it is susceptible to leptospirosis, does not recognise E. coli LPS, and there are a wide range of commercially available reagents available to study the humoral and cellular immune response. Furthermore, these reagents could assist in the development of an immune correlate for leptospirosis.
Conclusion
We report the use of RSV and CSIP for the identification of vaccine candidates in the L. interrogans Fiocruz L1-130 genome and the evaluation of 22 vaccine candidates (based on 33 βb-OMPs) against leptospirosis, four of which induced significant protection in two independent experiments. Furthermore, these recombinant proteins stimulated significant humoral immune responses and sterilizing immunity in immunized hamsters. In addition, two chimera constructs significantly increased survival in vaccinated animals, suggesting they have potential as vaccine candidates. However, when we tested the reproducibility of these vaccine candidates in additional experiments, they failed to induce protective immune responses. These results, together with other reports in the literature have led us to question the suitability of the hamster model of leptospirosis for the evaluation of vaccine candidates. We further propose that alternatives such as the mouse acute and chronic models should be re-evaluated.
Material and methods
Bacterial strains and cultivation
E. coli strains were grown in liquid Luria-Bertani (LB) medium (180 rpm) or on solid LB medium at 37°C. Ampicillin (100 µg/ml) and chloramphenicol (34 µg/ml) were used for selection when necessary. L. interrogans serogroup Icterohaemorrhagiae serovar Copenhageni strain Fiocruz L1-130 was maintained at 28°C in liquid Ellinghausen-McCullough-Johnson-Harris (EMJH) (Difco, BD, Brazil) supplemented with Leptospira enrichment EMJH commercial supplement (Difco, BD, Brazil).
Functional annotation and sequence conservation among orthologs
The list of 165 putative βb-OMPs previously identified by our research group RSV was updated as described (Grassmann et al., 2017a). Functional annotation was performed using UniProt and InterProScan (Zdobnov and Apweiler, 2001). Orthologues were identified in genome sequences from 20 additional Leptospira spp., using the reciprocal best hit (RBH) method based on protein BLAST (BLASTp) searches (Altschul et al., 1997) as previously described (Grassmann et al., 2017a). Protein sequences with >70% similarity and >40% coverage were considered orthologous. A multiple sequence alignment was performed with the orthologues from the available pathogenic Leptospira spp. using the Clustal Omega tool (Sievers et al., 2011). When this analysis was carried out there were 10 pathogenic Leptospira spp. genomes available, see (Figure S2 in Supplementary Material), corresponding to node 1 (9 species) in the P1 subclade of pathogenic Leptospira spp. based on the most recent genome diversity study and L. alsontii (Vincent et al., 2019).
Structural modelling and functional annotation
Given the likely importance of phagocytosis and clearance of leptospires during the infection, we confirmed the presence of SRIEs in the βb-OMPs. This was dependent on the ability to predict the orientation of the βb-OMPs in the OM, and this was achieved by analysing the closest PDB models with the orientation of proteins in membranes database. These epitopes are therefore likely to be exposed on the leptospiral surface and be capable of binding to MHC class II receptors, thereby stimulating the host immune response. The closest structural analogues in PDB of each 3D model generated by I-TASSER were used as references to determine the orientation of the leptospiral βb-OMPs in the OM, as well the probable surface exposed regions. The presence of MHCII epitopes (HLA-DRB alleles) and linear B cell epitopes in the fragments exposed on the OM was predicted using NetMHCII software and BepiPred-2.0, respectively (Lundegaard et al., 2008).
βb-OMP structural membrane allocation and epitope predictions
To identify proteins containing β-barrel transmembrane domains, conserved sequences among pathogenic species were subjected to 3D modelling by protein threading using I-TASSER server (Zhang, 2008). I-TASSER results from the top-ranking models with barrel structures were used to identify the PDB entries with the closest structures to the target protein models. For protein 3D models with secondary or tertiary I-TASSER rankings, a 3D structure-based functional annotation was performed using COFACTOR (Roy et al., 2012), as previously described (Grassmann et al., 2017a). The 3D structures were visualized using UCSF Chimera software V. 1.11.2 (Pettersen et al., 2004).
Identification of vaccine candidates by cell-surface immunoprecipitation (CSIP)
This technique was used to experimentally identify leptospiral proteins localized on the cell surface of host-adapted L. interrogans, as described previously (Cunha et al., 2017). Briefly, we produced host-adapted leptospires (L. interrogans Fiocruz L1-130) by cultivating them within dialysis membrane chambers that were surgically implanted in the peritoneal cavity of Wistar rats and cultivated for 9 – 12 days (Caimano et al., 2014; Grassmann et al., 2015). Intact, host-adapted leptospires were recovered and surface-exposed proteins were immunoprecipitated using pooled human sera from convalescent leptospirosis patients. Serum samples were kindly donated by the Public Health Central Laboratories (LACEN) in Rio de Janeiro (RJ) and Porto Alegre (RS) in Brazil. Leptospirosis was confirmed by the microscopic agglutination test (MAT) and ELISA (WHO and ILS, 2003). Following recovery, the immunoprecipitated proteins were identified using mass spectrometry (Newcombe et al., 2014).
Design and cloning of the β-barrel OMPs
The predicted 3D models of the βb-OMPs were used to design the recombinant proteins in this study. We used three strategies to clone the vaccine candidates: 1) Cell-surface exposed regions of 10 βb-OMPs (without transmembrane or periplasmic regions) for the following proteins: LIC10539, LIC10544, LIC11570, LIC11941, LIC12258, LIC12990, LIC13135, LIC13229, LIC13417* and LIC20214; 2) Three full-length proteins: LIC10496, LIC10881* and LIC11086; and 3) Cell-surface exposed regions from 29 proteins in nine multi-chimeric constructions as follows: chimera 1 (C1): LIC10896* + LIC10964 + LIC12374; chimera 2 (C2): LIC10714 + LIC10881* + LIC20151; chimera 3 (C3): LIC11458 + LIC11506 + LIC11086 + LIC20019; chimera 4 (C4): LIC11623 + LIC12254 + LIC11268; chimera 5 (C5): LIC10496 + LIC12575 + LIC11211 + LIC13417*; chimera 6 (C6): LIC10496 + LIC10881*; chimera 7 (C7): LIC10539 + LIC10544 + LIC12258; chimera 8 (C8): LIC11271 + LIC11366 + LIC11975 + LIC12252; chimera 9 (C9): LIC11941 + LIC12290 + LIC13135 + LIC12693 + LIC12307. The chimera constructs were designed to include a range of protein functions in each construct. For design strategies 1 and 3, the surface-related regions were synthesised in series without linkers, see (Table S2 in Supplementary Material). The regions from each protein were assembled using Vector NTI v.11 and commercially produced, including codon optimization for E. coli expression, and cloned into the expression vector pAE (Ramos et al., 2004), using BamHI, KpnI or HindIII restriction sites.
Recombinant protein expression and purification
Engineered recombinant proteins were expressed in E. coli BL21 (DE3) Star or pLysS cells as described previously (Bettin et al., 2022). Briefly, the product of each E. coli heat shock transformation was cultivated in LB medium containing 100 μg/ml of ampicillin and chloramphenicol (34 µg/ml for E. coli pLysS), at 37°C. When cultures reached mid-log phase (OD600 0.6 – 0.8), protein expression was induced with the addition of IPTG for 3-4 h. Pellets were suspended in lysis buffer (0.2 M NaH2PO4, 0.5 M NaCl and 20 mM imidazole, pH 8.0), sonicated by 6× 30s cycles on ice and centrifuged (11.000 x g, 40 min at 4°C). After lysis, soluble recombinant proteins were purified directly from the supernatant. For the recovery of insoluble proteins (contained in inclusion bodies) the pellets resulting from the post-lysis centrifugation were solubilized in a denaturing buffer (lysis buffer, 8 M urea). The recombinant proteins were purified by immobilized metal ion affinity chromatography (IMAC) using HisTrap FF columns (GE Healthcare, Brazil) using an AKTA Start chromatography system (GE Healthcare, Brazil), as described previously (Conrad et al., 2017). The fractions containing the soluble proteins were pooled and dialyzed against PBS pH 7.5, or 50 mM Tris pH 8.5 buffer at 4°C for up to 24 h. The fractions containing insoluble proteins were dialyzed against PBS pH 7.5 or 50 mM Tris pH 8.5 containing 0.05% Triton X-100 at 4°C. Protein concentrations were determined using a BCA protein assay kit (Thermo Fisher Scientific, Brazil) and the proteins were stored at –20°C.
Determining the challenge dose for the hamster model
Male and female Syrian hamsters (Mesocricetus auratus) were used as the animal model for acute leptospirosis. The challenge dose for the pathogenic species L. interrogans serogroup Icterohaemorrhagiae serovar Copenhageni strain Fiocruz L1-130 was determined using 8-week-old hamsters, as described previously (Silva et al., 2007). Briefly, groups of three hamsters were infected by intraperitoneal injection with 100–103 leptospires in 1 ml EMJH medium. Leptospires were quantified using a Petroff-Hauser counting chamber and darkfield microscopy and only motile leptospires were counted. Hamsters were monitored daily for clinical signs of leptospirosis over a period of 28 days. Endpoint criteria included: 10% weight loss (see Figure S4), nasal bleeding, prostration and failure to respond to stimulation (Coutinho et al., 2011). Animals that fulfilled one or more of the endpoint criteria were immediately euthanized by CO2 narcosis. The endpoint dose that caused endpoint criteria in 50% of infected animals (ED50) was calculated as previously described (Reed and Muench, 1938).
Evaluation of immunoprotection in hamster model of lethal leptospirosis
The vaccine preparations for the cell-surface exposed regions and the full-length proteins contained 50 µg recombinant protein, while the chimeric constructs contained 25 µg of each protein in the construct (Table 2); this was based on previously published data, see e.g., (Silva et al., 2007; Conrad et al., 2017). For vaccine formulations, recombinant proteins were prepared to a 15% (v/v) final concentration of aluminium hydroxide adjuvant (2% Alhydrogel, In vivoGen, USA) and gently mixed for 16 h at 4°C. For immunizations, male and female Golden Syrian hamsters aged 4-6 weeks, were randomly allocated into groups of 9-10 animals each. Animals were immunized by intramuscular injection with two doses at 14-day intervals. To evaluate vaccine-induced protection, hamsters were challenged intraperitoneally 28 days after the first immunization with 10 – 20× ED50 of L. interrogans Fiocruz L1-130. The animals were monitored 3× daily for up to 28 days PC. Animals that developed endpoint criteria or that survived to day 28 PC were euthanized by CO2 narcosis. Pre-immune blood samples were collected on day 0, prior to vaccination, and day 28, prior to challenge, and stored at –20°C. Thirteen independent experiments were performed to evaluate the 22 recombinant protein constructs.
Leptospiral renal burden
Kidney samples were collected, macerated and inoculated into EMJH medium as previously described (WHO and ILS, 2003). Cultures were periodically examined by dark-field microscopy for up to twelve weeks before being considered negative. The leptospiral renal burden was evaluated by quantitative real-time PCR (qPCR) as previously described (Conrad et al., 2017), with the following differences. Genomic DNA was extracted from the kidneys of infected animals using the SV Genomic DNA Purification kit (Promega, Brazil). DNA from L. interrogans Fiocruz L1-130 was extracted using the Illustra Bacterium Genomic Prep Mini Spin kit (GE Healthcare, Brazil) and quantified using Quant-iT dsDNA Assay Kit and the Qubit fluorometer (Thermo Fisher Scientific Inc., USA), following the manufacturer’s instructions. The purified genomic DNA was diluted to generate a standard curve ranging from 2×101 to 2×106 copies/reaction. Reactions were performed in triplicate using LipL32-f 5’-CTGAGCGAGGACACAATC and LipL32-r 5’-ATTACGGCAGGAATCCAA primers.
Evaluation of the humoral immune response
The induction of the antibody-based immune response was evaluated by indirect ELISA using purified recombinant proteins as previously described (Conrad et al., 2017), with the following modifications: polystyrene 96 well microtitration plates were coated with 50-200 ng/well of each individual recombinant protein. The plates were blocked with 5% non-fat milk solution in PBS-T, and hamster sera were added at 1:50 – 1:400 dilution. Peroxidase-conjugated anti-Syrian hamster IgG antibody (Jackson ImmunoResearch, USA) or anti-IgG subclasses (anti-IgG1, IgG2/3 and IgG3) (Southern Biotech, USA) were used as the secondary antibodies. Reactions were developed by adding o-phenylenediamine dihydrochloride (Sigma-Aldrich, Brazil) and hydrogen peroxide and stopped with addition of 3N H2SO4. Optical density was read at 492 nm and mean values were obtained from serum samples assayed in triplicate.
Statistical analysis
Protection against lethal leptospirosis and survival rates were evaluated using the two-tailed Fisher’s exact test (GraphPad QuickCalcs) and the Log-rank test (GraphPad Prism), respectively. Antibody levels were analysed with ANOVA to compare differences between the groups (Tukey’s multiple comparisons). GraphPad Prism v.8. was used to perform statistical analysis, and P values < 0.05 were considered significant.
Data availability statement
The original contributions presented in the study are included in the article/Supplementary Material. Further inquiries can be directed to the corresponding author.
Ethics statement
All animal experimentation was conducted following the Brazilian Guide for the Production, Maintenance and Use of Animals for Teaching Activities and Scientific Research, adhering to international guidelines. All protocols were reviewed and approved by the ethics committee on the use of animals (CEUA Nos: 8230-2017, 59050-2018 and 19193-2018) at the Federal University of Pelotas (UFPel). The CEUA at UFPel is accredited by the Brazilian National Council for Animal Experimentation Control (CONCEA). The hamsters used in the current study were provided by the animal unit at UFPel.
Author contributions
OD, JM, and AM contributed to conception and design of the study. MM, EB, LB, and NO performed the bioinformatics analysis, carried out the statistical analyses and wrote the first draft of the manuscript. MM, EB, LB, NO, TB, AP, GR, ER, and AS performed the laboratory experiments. EB, NO, and AM created the figures and tables. All authors contributed to manuscript revision, read, and approved the submitted version.
Funding
This work was supported by grant IC170171 from The Royal Society - UK, grants 311212/2021-2 and 315550/2020-1 from the Brazilian National Council for Scientific and Technological Development (CNPq) and grant 001 from the Coordenação de Aperfeiçoamento de Pessoal de Nível Superior - Brazil (CAPES).
Conflict of interest
The authors declare that the research was conducted in the absence of any commercial or financial relationships that could be construed as a potential conflict of interest.
Publisher’s note
All claims expressed in this article are solely those of the authors and do not necessarily represent those of their affiliated organizations, or those of the publisher, the editors and the reviewers. Any product that may be evaluated in this article, or claim that may be made by its manufacturer, is not guaranteed or endorsed by the publisher.
Supplementary material
The Supplementary Material for this article can be found online at: https://www.frontiersin.org/articles/10.3389/fcimb.2022.940966/full#supplementary-material
References
Adler, B. (2015b). Vaccines against leptospirosis. Curr. Top. Microbiol. Immunol. 387, 251–272. doi: 10.1007/978-3-662-45059-8_10
Adler, B., de la Pena Moctezuma, A. (2010). Leptospira and leptospirosis. Vet. Microbiol. 140 (3-4), 287–296. doi: 10.1016/j.vetmic.2009.03.012
Altschul, S. F., Madden, T. L., Schaffer, A. A., Zhang, J., Zhang, Z., Miller, W., et al. (1997). Gapped BLAST and PSI-BLAST: a new generation of protein database search programs. Nucleic Acids Res. 25 (17), 3389–3402. doi: 10.1093/nar/25.17.3389
Athanazio, D. A., Silva, E. F., Santos, C. S., Rocha, G. M., Vannier-Santos, M. A., McBride, A. J., et al. (2008). Rattus norvegicus as a model for persistent renal colonization by pathogenic leptospira interrogans. Acta Trop. 105 (2), 176–180. doi: 10.1016/j.actatropica.2007.10.012
Awanye, A. M., Chang, C. M., Wheeler, J. X., Chan, H., Marsay, L., Dold, C., et al. (2019). Immunogenicity profiling of protein antigens from capsular group b neisseria meningitidis. Sci. Rep. 9 (1), 6843. doi: 10.1038/s41598-019-43139-0
Bandeira, M., Santos, C. S., de Azevedo, E. C., Soares, L. M., Macedo, J. O., Marchi, S., et al. (2011). Attenuated nephritis in inducible nitric oxide synthase knockout C57BL/6 mice and pulmonary hemorrhage in CB17 SCID and recombination activating gene 1 knockout C57BL/6 mice infected with leptospira interrogans. Infect. Immun. 79 (7), 2936–2940. doi: 10.1128/IAI.05099-11
Baquero, O. S., Machado, G. (2018). Spatiotemporal dynamics and risk factors for human leptospirosis in Brazil. Sci. Rep. 8 (1), 15170. doi: 10.1038/s41598-018-33381-3
Bentley, A. T., Klebba, P. E. (1988). Effect of lipopolysaccharide structure on reactivity of antiporin monoclonal antibodies with the bacterial cell surface. J. Bacteriol 170 (3), 1063–1068. doi: 10.1128/jb.170.3.1063-1068.1988
Bettin, E. B., Dorneles, J., Hecktheuer, A. S., Madruga, A. B., Seixas Neto, A. C. P., McBride, A. J. A., et al. (2022). TonB-dependent receptor epitopes expressed in m. bovis BCG induced significant protection in the hamster model of leptospirosis. Appl. Microbiol. Biotechnol. 106 (1), 173–184. doi: 10.1007/s00253-021-11726-9
Cable, J., Srikantiah, P., Crowe, J. E., Jr., Pulendran, B., Hill, A., Ginsberg, A., et al. (2020). Vaccine innovations for emerging infectious diseases-a symposium report. Ann. N Y Acad. Sci. 1462 (1), 14–26. doi: 10.1111/nyas.14235
Caimano, M. J., Sivasankaran, S. K., Allard, A., Hurley, D., Hokamp, K., Grassmann, A. A., et al. (2014). A model system for studying the transcriptomic and physiological changes associated with mammalian host-adaptation by leptospira interrogans serovar copenhageni. PloS Pathog. 10 (3), e1004004. doi: 10.1371/journal.ppat.1004004
Conrad, N. L., Cruz McBride, F. W., Souza, J. D., Silveira, M. M., Felix, S., Mendonca, K. S., et al. (2017). LigB subunit vaccine confers sterile immunity against challenge in the hamster model of leptospirosis. PloS Negl. Trop. Dis. 11 (3), e0005441. doi: 10.1371/journal.pntd.0005441
Costa, F., Hagan, J. E., Calcagno, J., Kane, M., Torgerson, P., Martinez-Silveira, M. S., et al. (2015). Global morbidity and mortality of leptospirosis: A systematic review. PloS Negl. Trop. Dis. 9 (9), e0003898. doi: 10.1371/journal.pntd.0003898
Coutinho, M. L., Choy, H. A., Kelley, M. M., Matsunaga, J., Babbitt, J. T., Lewis, M. S., et al. (2011). A LigA three-domain region protects hamsters from lethal infection by leptospira interrogans. PloS Negl. Trop. Dis. 5 (12), e1422. doi: 10.1371/journal.pntd.0001422
Cozzi, R., Scarselli, M., Ferlenghi, I. (2013). Structural vaccinology: a three-dimensional view for vaccine development. Curr. Top. Med. Chem. 13 (20), 2629–2637. doi: 10.2174/15680266113136660187
Croda, J., Neto, A. N., Brasil, R. A., Pagliari, C., Nicodemo, A. C., Duarte, M. I. (2010). Leptospirosis pulmonary haemorrhage syndrome is associated with linear deposition of immunoglobulin and complement on the alveolar surface. Clin. Microbiol. Infect. 16 (6), 593–599. doi: 10.1111/j.1469-0691.2009.02916.x
Cruz, A. R., Moore, M. W., La Vake, C. J., Eggers, C. H., Salazar, J. C., Radolf, J. D. (2008). Phagocytosis of borrelia burgdorferi, the Lyme disease spirochete, potentiates innate immune activation and induces apoptosis in human monocytes. Infect. Immun. 76 (1), 56–70. doi: 10.1128/IAI.01039-07
Cunha, C. E. P., Newcombe, J., Dellagostin, O. A., McFadden, J. (2017). Immunoprecipitation of cell surface proteins from gram-negative bacteria. Bio Protoc. 7 (9), e2250. doi: 10.21769/BioProtoc.2250
Dellagostin, O. A., Grassmann, A. A., Rizzi, C., Schuch, R. A., Jorge, S., Oliveira, T. L., et al. (2017). Reverse vaccinology: An approach for identifying leptospiral vaccine candidates. Int. J. Mol. Sci. 18 (1), 158. doi: 10.3390/ijms18010158
De Temmerman, M. L., Rejman, J., Demeester, J., Irvine, D. J., Gander, B., De Smedt, S. C. (2011). Particulate vaccines: on the quest for optimal delivery and immune response. Drug Discovery Today 16 (13-14), 569–582. doi: 10.1016/j.drudis.2011.04.006
Dominguez-Medina, C. C., Perez-Toledo, M., Schager, A. E., Marshall, J. L., Cook, C. N., Bobat, S., et al. (2020). Outer membrane protein size and LPS O-antigen define protective antibody targeting to the salmonella surface. Nat. Commun. 11 (1), 851. doi: 10.1038/s41467-020-14655-9
Dormitzer, P. R., Grandi, G., Rappuoli, R. (2012). Structural vaccinology starts to deliver. Nat. Rev. Microbiol. 10 (12), 807–813. doi: 10.1038/nrmicro2893
Faisal, S. M., Yan, W., McDonough, S. P., Chang, C. F., Pan, M. J., Chang, Y. F. (2009a). Leptosome-entrapped leptospiral antigens conferred significant higher levels of protection than those entrapped with PC-liposomes in a hamster model. Vaccine 27 (47), 6537–6545. doi: 10.1016/j.vaccine.2009.08.051
Faisal, S. M., Yan, W., McDonough, S. P., Mohammed, H. O., Divers, T. J., Chang, Y. F. (2009b). Immune response and prophylactic efficacy of smegmosomes in a hamster model of leptospirosis. Vaccine 27 (44), 6129–6136. doi: 10.1016/j.vaccine.2009.08.029
Felix, C. R., Siedler, B. S., Barbosa, L. N., Timm, G. R., McFadden, J., McBride, A. J. A. (2020). An overview of human leptospirosis vaccine design and future perspectives. Expert Opin. Drug Discovery 15 (2), 179–188. doi: 10.1080/17460441.2020.1694508
Fernandes, L. G., Teixeira, A. F., Filho, A. F., Souza, G. O., Vasconcellos, S. A., Heinemann, M. B., et al. (2017). Immune response and protective profile elicited by a multi-epitope chimeric protein derived from leptospira interrogans. Int. J. Infect. Dis. 57, 61–69. doi: 10.1016/j.ijid.2017.01.032
Gomes-Solecki, M., Santecchia, I., Werts, C. (2017). Animal models of leptospirosis: Of mice and hamsters. Front. Immunol. 8 (58). doi: 10.3389/fimmu.2017.00058
Grassmann, A. A., Kremer, F. S., Dos Santos, J. C., Souza, J. D., Pinto, L. D. S., McBride, A. J. A. (2017a). Discovery of novel leptospirosis vaccine candidates using reverse and structural vaccinology. Front. Immunol. 8. doi: 10.3389/fimmu.2017.00463
Grassmann, A. A., McBride, A. J., Nally, J. E., Caimano, M. J. (2015). Generation of mammalian host-adapted leptospira interrogans by cultivation in peritoneal dialysis membrane chamber implantation in rats. Bio Protoc. 5 (14), e1536. doi: 10.21769/BioProtoc.1536
Grassmann, A. A., Souza, J. D., McBride, A. J. (2017b). A universal vaccine against leptospirosis: Are we going in the right direction? Front. Immunol. 8. doi: 10.3389/fimmu.2017.00256
Grassmann, A. A., Zavala-Alvarado, C., Bettin, E. B., Picardeau, M., Benaroudj, N., Caimano, M. J. (2021). The FUR-like regulators PerRA and PerRB integrate a complex regulatory network that promotes mammalian host-adaptation and virulence of leptospira interrogans. PloS Pathog. 17 (12), e1009078. doi: 10.1371/journal.ppat.1009078
Haake, D. A. (2006). Hamster model of leptospirosis. Curr. Protoc. Microbiol Chapter 12, Unit 12E 12. doi: 10.1002/9780471729259.mc12e02s02
Haake, D. A., Mazel, M. K., McCoy, A. M., Milward, F., Chao, G., Matsunaga, J., et al. (1999). Leptospiral outer membrane proteins OmpL1 and LipL41 exhibit synergistic immunoprotection. Infect. Immun. 67 (12), 6572–6582. doi: 10.1128/IAI.67.12.6572-6582.1999
Hawley, K. L., Cruz, A. R., Benjamin, S. J., La Vake, C. J., Cervantes, J. L., LeDoyt, M., et al. (2017). IFNgamma enhances CD64-potentiated phagocytosis of treponema pallidum opsonized with human syphilitic serum by human macrophages. Front. Immunol. 8. doi: 10.3389/fimmu.2017.01227
Heesterbeek, D. A. C., Angelier, M. L., Harrison, R. A., Rooijakkers, S. H. M. (2018). Complement and bacterial infections: From molecular mechanisms to therapeutic applications. J. Innate Immun. 10 (5-6), 455–464. doi: 10.1159/000491439
Hsieh, C. L., Ptak, C. P., Tseng, A., Suguiura, I. M. S., McDonough, S. P., Sritrakul, T., et al. (2017). Extended low-resolution structure of a leptospira antigen offers high bactericidal antibody accessibility amenable to vaccine design. Elife 6, e30051. doi: 10.7554/eLife.30051
Koizumi, N., Watanabe, H. (2004). Leptospiral immunoglobulin-like proteins elicit protective immunity. Vaccine 22 (11-12), 1545–1552. doi: 10.1016/j.vaccine.2003.10.007
Koussounadis, A., Langdon, S. P., Um, I. H., Harrison, D. J., Smith, V. A. (2015). Relationship between differentially expressed mRNA and mRNA-protein correlations in a xenograft model system. Sci. Rep. 5, 10775. doi: 10.1038/srep10775
Kundu, S., Shetty, A., Gomes-Solecki, M. (2022). Necroptosis contributes to persistent inflammation during acute leptospirosis. Front. Immunol. 13. doi: 10.3389/fimmu.2022.810834
Lata, K. S., Kumar, S., Vaghasia, V., Sharma, P., Bhairappanvar, S. B., Soni, S., et al. (2018). Exploring leptospiral proteomes to identify potential candidates for vaccine design against leptospirosis using an immunoinformatics approach. Sci. Rep. 8 (1), 6935. doi: 10.1038/s41598-018-25281-3
Liljeroos, L., Malito, E., Ferlenghi, I., Bottomley, M. J. (2015). Structural and computational biology in the design of immunogenic vaccine antigens. J. Immunol. Res. 2015, 156241. doi: 10.1155/2015/156241
Lin, X., Xiao, G., Luo, D., Kong, L., Chen, X., Sun, D., et al. (2016). Chimeric epitope vaccine against leptospira interrogans infection and induced specific immunity in guinea pigs. BMC Microbiol. 16 (1), 241. doi: 10.1186/s12866-016-0852-y
Liu, Y., Beyer, A., Aebersold, R. (2016). On the dependency of cellular protein levels on mRNA abundance. Cell 165 (3), 535–550. doi: 10.1016/j.cell.2016.03.014
Louvel, H., Bommezzadri, S., Zidane, N., Boursaux-Eude, C., Creno, S., Magnier, A., et al. (2006). Comparative and functional genomic analyses of iron transport and regulation in leptospira spp. J. Bacteriol 188 (22), 7893–7904. doi: 10.1128/JB.00711-06
Lundegaard, C., Lamberth, K., Harndahl, M., Buus, S., Lund, O., Nielsen, M. (2008). NetMHC-3.0: accurate web accessible predictions of human, mouse and monkey MHC class I affinities for peptides of length 8-11. Nucleic Acids Res. 36 (Web Server issue), W509–W512. doi: 10.1093/nar/gkn202
Maiti, B., Dubey, S., Munang'andu, H. M., Karunasagar, I., Karunasagar, I., Evensen, O. (2020). Application of outer membrane protein-based vaccines against major bacterial fish pathogens in India. Front. Immunol. 11. doi: 10.3389/fimmu.2020.01362
Martins, G., Lilenbaum, W. (2017). Control of bovine leptospirosis: Aspects for consideration in a tropical environment. Res. Vet. Sci. 112, 156–160. doi: 10.1016/j.rvsc.2017.03.021
McBride, A. J., Athanazio, D. A., Reis, M. G., Ko, A. I. (2005). Leptospirosis. Curr. Opin. Infect. Dis. 18 (5), 376–386. doi: 10.1097/01.qco.0000178824.05715.2c
Mendum, T. A., Newcombe, J., McNeilly, C. L., McFadden, J. (2009). Towards the immunoproteome of neisseria meningitidis. PloS One 4 (6), e5940. doi: 10.1371/journal.pone.0005940
Michaelsen, T. E., Aase, A., Kolberg, J., Wedge, E., Rosenqvist, E. (2001). PorB3 outer membrane protein on neisseria meningitidis is poorly accessible for antibody binding on live bacteria. Vaccine 19 (11-12), 1526–1533. doi: 10.1016/s0264-410x(00)00324-8
Monaris, D., Sbrogio-Almeida, M. E., Dib, C. C., Canhamero, T. A., Souza, G. O., Vasconcellos, S. A., et al. (2015). Protective immunity and reduced renal colonization induced by vaccines containing recombinant leptospira interrogans outer membrane proteins and flagellin adjuvant. Clin. Vaccine Immunol. 22 (8), 965–973. doi: 10.1128/CVI.00285-15
Mosmann, T. R., Coffman, R. L. (1989). Heterogeneity of cytokine secretion patterns and functions of helper T cells. Adv. Immunol. 46, 111–147. doi: 10.1016/s0065-2776(08)60652-5
Murphy, C. K., Kalve, V. I., Klebba, P. E. (1990). Surface topology of the escherichia coli K-12 ferric enterobactin receptor. J. Bacteriol 172 (5), 2736–2746. doi: 10.1128/jb.172.5.2736-2746.1990
Murray, G. L., Simawaranon, T., Kaewraemruaen, C., Adler, B., Sermswan, R. W. (2018). Heterologous protection elicited by a live, attenuated, leptospira vaccine. Vet. Microbiol. 223, 47–50. doi: 10.1016/j.vetmic.2018.07.018
Newcombe, J., Mendum, T. A., Ren, C. P., McFadden, J. (2014). Identification of the immunoproteome of the meningococcus by cell surface immunoprecipitation and MS. Microbiology 160 (Pt 2), 429–438. doi: 10.1099/mic.0.071829-0
Oliveira, T. L., Schuch, R. A., Inda, G. R., Roloff, B. C., Neto, A., Amaral, M., et al. (2018). LemA and erp y-like recombinant proteins from leptospira interrogans protect hamsters from challenge using AddaVax as adjuvant. Vaccine 36 (19), 2574–2580. doi: 10.1016/j.vaccine.2018.03.078
Patel, D. S., Re, S., Wu, E. L., Qi, Y., Klebba, P. E., Widmalm, G., et al. (2016). Dynamics and interactions of OmpF and LPS: Influence on pore accessibility and ion permeability. Biophys. J. 110 (4), 930–938. doi: 10.1016/j.bpj.2016.01.002
Pereira, M. M., Andrade, J., Marchevsky, R. S., Ribeiro dos Santos, R. (1998). Morphological characterization of lung and kidney lesions in C3H/HeJ mice infected with leptospira interrogans serovar icterohaemorrhagiae: defect of CD4+ and CD8+ T-cells are prognosticators of the disease progression. Exp. Toxicol. Pathol. 50 (3), 191–198. doi: 10.1016/S0940-2993(98)80083-3
Pereira, M. M., Schneider, M. C., Munoz-Zanzi, C., Costa, F., Benschop, J., Hartskeerl, R., et al. (2018). A road map for leptospirosis research and health policies based on country needs in Latin America. Rev. Panam Salud Publica 41, e131. doi: 10.26633/RPSP.2017.131
Petsch, D., Anspach, F. B. (2000). Endotoxin removal from protein solutions. J. Biotechnol. 76 (2-3), 97–119. doi: 10.1016/s0168-1656(99)00185-6
Pettersen, E. F., Goddard, T. D., Huang, C. C., Couch, G. S., Greenblatt, D. M., Meng, E. C., et al. (2004). UCSF chimera–a visualization system for exploratory research and analysis. J. Comput. Chem. 25 (13), 1605–1612. doi: 10.1002/jcc.20084
Picardeau, M. (2017). Virulence of the zoonotic agent of leptospirosis: still terra incognita? Nat. Rev. Microbiol. 15 (5), 297–307. doi: 10.1038/nrmicro.2017.5
Pizza, M., Scarlato, V., Masignani, V., Giuliani, M. M., Arico, B., Comanducci, M., et al. (2000). Identification of vaccine candidates against serogroup b meningococcus by whole-genome sequencing. Science 287 (5459), 1816–1820. doi: 10.1126/science.287.5459.1816
Potula, H. H., Richer, L., Werts, C., Gomes-Solecki, M. (2017). Pre-treatment with lactobacillus plantarum prevents severe pathogenesis in mice infected with leptospira interrogans and may be associated with recruitment of myeloid cells. PloS Negl. Trop. Dis. 11 (8), e0005870. doi: 10.1371/journal.pntd.0005870
Qin, J. H., Sheng, Y. Y., Zhang, Z. M., Shi, Y. Z., He, P., Hu, B. Y., et al. (2006). Genome-wide transcriptional analysis of temperature shift in l. interrogans serovar lai strain 56601. BMC Microbiol. 6, 51. doi: 10.1186/1471-2180-6-51
Que-Gewirth, N. L., Ribeiro, A. A., Kalb, S. R., Cotter, R. J., Bulach, D. M., Adler, B., et al. (2004). A methylated phosphate group and four amide-linked acyl chains in leptospira interrogans lipid a. the membrane anchor of an unusual lipopolysaccharide that activates TLR2. J. Biol. Chem. 279 (24), 25420–25429. doi: 10.1074/jbc.M400598200
Raja, V., Sobana, S., Mercy, C. S. A., Cotto, B., Bora, D. P., Natarajaseenivasan, K. (2018). Heterologous DNA prime-protein boost immunization with RecA and FliD offers cross-clade protection against leptospiral infection. Sci. Rep. 8 (1), 6447. doi: 10.1038/s41598-018-24674-8
Ramos, C. R., Abreu, P. A., Nascimento, A. L., Ho, P. L. (2004). A high-copy T7 escherichia coli expression vector for the production of recombinant proteins with a minimal n-terminal his-tagged fusion peptide. Braz. J. Med. Biol. Res. 37 (8), 1103–1109. doi: 10.1590/S0100-879X2004000800001
Rappuoli, R., Bottomley, M. J., D'Oro, U., Finco, O., De Gregorio, E. (2016). Reverse vaccinology 2.0: Human immunology instructs vaccine antigen design. J. Exp. Med. 213 (4), 469–481. doi: 10.1084/jem.20151960
Reed, L. J., Muench, H. (1938). A simple method of estimating fifty percent endpoints. Am. J. Hyg 27, 493–497. doi: 10.1093/oxfordjournals.aje.a118408
Richer, L., Potula, H. H., Melo, R., Vieira, A., Gomes-Solecki, M. (2015). Mouse model for sublethal leptospira interrogans infection. Infect. Immun. 83 (12), 4693–4700. doi: 10.1128/IAI.01115-15
Roy, A., Yang, J., Zhang, Y. (2012). COFACTOR: an accurate comparative algorithm for structure-based protein function annotation. Nucleic Acids Res. 40 (Web Server issue), W471–W477. doi: 10.1093/nar/gks372
Santecchia, I., Vernel-Pauillac, F., Rasid, O., Quintin, J., Gomes-Solecki, M., Boneca, I. G., et al. (2019). Innate immune memory through TLR2 and NOD2 contributes to the control of leptospira interrogans infection. PloS Pathog. 15 (5), e1007811. doi: 10.1371/journal.ppat.1007811
Santos, C. S., Macedo, J. O., Bandeira, M., Chagas-Junior, A. D., McBride, A. J. A., McBride, F. W. C., et al. (2010). Different outcomes of experimental leptospiral infection in mouse strains with distinct genotypes. J. Med. Microbiol. 59 (Pt 9), 1101–1106. doi: 10.1099/jmm.0.021089-0
Schulz, G. E. (2002). The structure of bacterial outer membrane proteins. Biochim. Biophys. Acta 1565 (2), 308–317. doi: 10.1016/s0005-2736(02)00577-1
Segawa, T., Nomura, K. H., Villanueva, S. Y., Saito, M., Nomura, K., Gloriani, N. G., et al. (2014). Identification of leptospiral 3-hydroxyacyl-CoA dehydrogenase released in the urine of infected hamsters. BMC Microbiol. 14, 132. doi: 10.1186/1471-2180-14-132
Serruto, D., Bottomley, M. J., Ram, S., Giuliani, M. M., Rappuoli, R. (2012). The new multicomponent vaccine against meningococcal serogroup b, 4CMenB: immunological, functional and structural characterization of the antigens. Vaccine 30 Suppl 2, B87–B97. doi: 10.1016/j.vaccine.2012.01.033
Shetty, A., Kundu, S., Gomes-Solecki, M. (2021). Inflammatory signatures of pathogenic and non-pathogenic leptospira infection in susceptible C3H-HeJ mice. Front. Cell Infect. Microbiol. 11. doi: 10.3389/fcimb.2021.677999
Siegrist, C.-A. (2018). “Vaccine immunology,” in Plotkin's vaccines. Eds. Plotkin, S. A., Orenstein, W. A., Offit, P. A., Edwards, K. M. (Amsterdam, Netherlands: Elsevier), 16–34.e17.
Sievers, F., Wilm, A., Dineen, D., Gibson, T. J., Karplus, K., Li, W., et al. (2011). Fast, scalable generation of high-quality protein multiple sequence alignments using clustal omega. Mol. Syst. Biol. 7, 539. doi: 10.1038/msb.2011.75
Silhavy, T. J., Kahne, D., Walker, S. (2010). The bacterial cell envelope. Cold Spring Harb. Perspect. Biol. 2 (5), a000414. doi: 10.1101/cshperspect.a000414
Silva, E. F., Medeiros, M. A., McBride, A. J., Matsunaga, J., Esteves, G. S., Ramos, J. G., et al. (2007). The terminal portion of leptospiral immunoglobulin-like protein LigA confers protective immunity against lethal infection in the hamster model of leptospirosis. Vaccine 25 (33), 6277–6286. doi: 10.1016/j.vaccine.2007.05.053
Silveira, M. M., Oliveira, T. L., Schuch, R. A., McBride, A. J. A., Dellagostin, O. A., Hartwig, D. D. (2017). DNA Vaccines against leptospirosis: A literature review. Vaccine 35 (42), 5559–5567. doi: 10.1016/j.vaccine.2017.08.067
Sperandeo, P., Martorana, A. M., Polissi, A. (2019). Lipopolysaccharide biosynthesis and transport to the outer membrane of gram-negative bacteria. Subcell Biochem. 92, 9–37. doi: 10.1007/978-3-030-18768-2_2
Srikram, A., Zhang, K., Bartpho, T., Lo, M., Hoke, D. E., Sermswan, R. W., et al. (2011). Cross-protective immunity against leptospirosis elicited by a live, attenuated lipopolysaccharide mutant. J. Infect. Dis. 203 (6), 870–879. doi: 10.1093/infdis/jiq127
Srinivas, G. B., Walker, A., Rippke, B. (2013). USDA Regulatory guidelines and practices for veterinary leptospira vaccine potency testing. Biologicals 41 (5), 298–302. doi: 10.1016/j.biologicals.2013.06.005
van der Ley, P., Kuipers, O., Tommassen, J., Lugtenberg, B. (1986). O-Antigenic chains of lipopolysaccharide prevent binding of antibody molecules to an outer membrane pore protein in enterobacteriaceae. Microb. Pathog. 1 (1), 43–49. doi: 10.1016/0882-4010(86)90030-6
van de Waterbeemd, B., Streefland, M., van der Ley, P., Zomer, B., van Dijken, H., Martens, D., et al. (2010). Improved OMV vaccine against neisseria meningitidis using genetically engineered strains and a detergent-free purification process. Vaccine 28 (30), 4810–4816. doi: 10.1016/j.vaccine.2010.04.082
Verma, R., Khanna, P., Chawla, S. (2013). Whole-cell inactivated leptospirosis vaccine: future prospects. Hum. Vaccin Immunother. 9 (4), 763–765. doi: 10.4161/hv.23059
Vernel-Pauillac, F., Murray, G. L., Adler, B., Boneca, I. G., Werts, C. (2021). Anti-leptospira immunoglobulin profiling in mice reveals strain specific IgG and persistent IgM responses associated with virulence and renal colonization. PloS Negl. Trop. Dis. 15 (3), e0008970. doi: 10.1371/journal.pntd.0008970
Vernel-Pauillac, F., Werts, C. (2018). Recent findings related to immune responses against leptospirosis and novel strategies to prevent infection. Microbes Infect. 20 (9-10), 578–588. doi: 10.1016/j.micinf.2018.02.001
Vincent, A. T., Schiettekatte, O., Goarant, C., Neela, V. K., Bernet, E., Thibeaux, R., et al. (2019). Revisiting the taxonomy and evolution of pathogenicity of the genus leptospira through the prism of genomics. PloS Negl. Trop. Dis. 13 (5), e0007270. doi: 10.1371/journal.pntd.0007270
Vinh, T., Adler, B., Faine, S. (1986). Ultrastructure and chemical composition of lipopolysaccharide extracted from leptospira interrogans serovar copenhageni. J. Gen. Microbiol. 132 (1), 103–109. doi: 10.1099/00221287-132-1-103
Viratyosin, W., Ingsriswang, S., Pacharawongsakda, E., Palittapongarnpim, P. (2008). Genome-wide subcellular localization of putative outer membrane and extracellular proteins in leptospira interrogans serovar lai genome using bioinformatics approaches. BMC Genomics 9, 181. doi: 10.1186/1471-2164-9-181
Wakelin, S. J., Sabroe, I., Gregory, C. D., Poxton, I. R., Forsythe, J. L., Garden, O. J., et al. (2006). "Dirty little secrets"–endotoxin contamination of recombinant proteins. Immunol. Lett. 106 (1), 1–7. doi: 10.1016/j.imlet.2006.04.007
Walker, S. S., Black, T. A. (2021). Are outer-membrane targets the solution for MDR gram-negative bacteria? Drug Discovery Today 26 (9), 2152–2158. doi: 10.1016/j.drudis.2021.03.027
WHO and ILS (2003). Human leptospirosis: guidance for diagnosis, surveillance and control (Malta: World Health Organization).
Wilson, M. M., Bernstein, H. D. (2016). Surface-exposed lipoproteins: An emerging secretion phenomenon in gram-negative bacteria. Trends Microbiol. 24 (3), 198–208. doi: 10.1016/j.tim.2015.11.006
Wunder, E. A., Adhikarla, H., Hamond, C., Owers Bonner, K. A., Liang, L., Rodrigues, C. B., et al. (2021). A live attenuated-vaccine model confers cross-protective immunity against different species of the leptospira genus. Elife 10, e64166. doi: 10.7554/eLife.64166
Yu, H., Karunakaran, K. P., Jiang, X., Chan, Q., Rose, C., Foster, L. J., et al. (2020). Comparison of chlamydia outer membrane complex to recombinant outer membrane proteins as vaccine. Vaccine 38 (16), 3280–3291. doi: 10.1016/j.vaccine.2020.02.059
Zdobnov, E. M., Apweiler, R. (2001). InterProScan–an integration platform for the signature-recognition methods in InterPro. Bioinformatics 17 (9), 847–848. doi: 10.1093/bioinformatics/17.9.847
Zeng, L., Wang, D., Hu, N., Zhu, Q., Chen, K., Dong, K., et al. (2017). A novel pan-genome reverse vaccinology approach employing a negative-selection strategy for screening surface-exposed antigens against leptospirosis. Front. Microbiol. 8. doi: 10.3389/fmicb.2017.00396
Zhang, Y. (2008). I-TASSER server for protein 3D structure prediction. BMC Bioinf. 9, 40. doi: 10.1186/1471-2105-9-40
Keywords: Leptospira interrogans, outer membrane proteins, reverse and structural vaccinology, cell-surface immunoprecipitation, animal model
Citation: Maia MAC, Bettin EB, Barbosa LN, de Oliveira NR, Bunde TT, Pedra ACK, Rosa GA, da Rosa EEB, Seixas Neto ACP, Grassmann AA, McFadden J, Dellagostin OA and McBride AJA (2022) Challenges for the development of a universal vaccine against leptospirosis revealed by the evaluation of 22 vaccine candidates. Front. Cell. Infect. Microbiol. 12:940966. doi: 10.3389/fcimb.2022.940966
Received: 10 May 2022; Accepted: 20 September 2022;
Published: 07 October 2022.
Edited by:
Lourdes Isaac, University of São Paulo, BrazilReviewed by:
Shakti Singh, Lundquist Institute for Biomedical Innovation, United StatesSuman Kundu, University of Tennessee Health Science Center (UTHSC), United States
Copyright © 2022 Maia, Bettin, Barbosa, de Oliveira, Bunde, Pedra, Rosa, da Rosa, Seixas Neto, Grassmann, McFadden, Dellagostin and McBride. This is an open-access article distributed under the terms of the Creative Commons Attribution License (CC BY). The use, distribution or reproduction in other forums is permitted, provided the original author(s) and the copyright owner(s) are credited and that the original publication in this journal is cited, in accordance with accepted academic practice. No use, distribution or reproduction is permitted which does not comply with these terms.
*Correspondence: Alan J. A. McBride, YWxhbi5tY2JyaWRlQHVmcGVsLmVkdS5icg==
†Present address: Everton B. Bettin, Department of Medicine, University of Connecticut Health, Farmington, CT, United States
Liana N. Barbosa, Department of Biomedical and Diagnostic Sciences, University of Tennessee, Knoxville, TN, United States
‡These authors have contributed equally to this work and share first authorship
§These authors have contributed equally to this work and share last authorship