- 1Guangdong Provincial Key Laboratory of Infectious Diseases and Molecular Immunopathology, Shantou University Medical College, Shantou, China
- 2School of Biomedical Sciences and Pharmacy, College of Health, Medicine and Wellbeing, University of Newcastle, Callaghan, NSW, Australia
Blood microorganisms were once thought to indicate infection. Blood in healthy people appears to be devoid of growing bacteria; nonetheless, intracellular dormant forms of bacteria have been reported previously. With breakthroughs in sequencing and bioinformatics, the presence of bacterial DNA in healthy human blood initiated the controversy of human blood microbiota (HBM). Recently, bacteria-specific DNA and culturable bacteria were found in healthy human blood. Researchers wanted to study the phenomena of a “healthy blood microbiota” by providing a thorough description of bacterially produced nucleic acids using many complementing molecular and traditional microbiological approaches. Because blood is a relatively limited and particular environment, culturability and plate count issues can be overcome using enhanced cultured procedures. However, more evidence is required to confirm that healthy human blood contains normal microbiota. Cavities, mouth and intestinal microbiota, trauma, surgery, and animal/insect bites can introduce bacteria into human blood. All these factors strengthen the concept of transient blood bacteria too. The presence of blood bacteria may be caused by temporary immunological clearance and absorption by dendritic or M cells. This review provides an extensive and comprehensive analysis that suggests that healthy blood bacteria may not be typical microbiota but transient circulatory microorganisms. In this study, we look at how contaminants (Escherichia, Shigella, Pseudomonads, etc.) from the skin, laboratory environments, and reagents can affect the interpretation of blood-derived microbial information and the relationship between the circulating bacteria and non-communicable diseases. Circulating transient bacteria may play a role in the pathogenesis of non-infectious diseases such as diabetes and CVD. Contamination-free hematological studies can aid in understanding the disease mechanisms, therapy, and biomarkers.
Introduction
Over the last two decades, studies on the human microbiome have been getting huge consideration. Enormous numbers (10–100 trillion) of microorganisms colonize significant parts of the human body, such as the skin, oral cavity, gastrointestinal tract (GIT), respiratory tract, and urogenital tract. Microbiome research received much attention due to the long-lasting effects on significant health issues, including metabolism, depression, blood pressure, brain health, and chronic infectious diseases. Through the evolutionary process, many microbial species have successfully adapted to the normal microbiome of humans. Most of these microbes could not be cultured in in vitro environments. Inside the human body, these microbes multiply and participate in essential biological processes and set the bases for several communicable and non-communicable human diseases. At the same time, the colonization of microbes in the body compartments continuously in contact with external microorganisms (such as mouth, respiratory tract, GIT, skin, urethra, and vagina) is well studied and widely accepted (Markova, 2017).
The human gut harbors the most complex human microbiota niche, most of which belong to the Gram-positive Firmicutes and Actinobacteria, while among the Gram-negative phyla Bacteroidetes and Proteobacteria, Fusobacteria are most abundant and Euryarchaeota are found in minor quantities. However, it varies among individuals due to certain factors such as diet, age, geographical location, use of antibiotics, and polluted drinking water (Arumugam et al., 2011; Segata et al., 2012; Brown et al., 2013). The gut microbiota, which might be changeable, performs different functions in the human body, including digestion and metabolite production. Human microbiota helps establish the early immune system, modulation, and protection against pathogens (Clemente et al., 2012; Brown et al., 2013). Similarly, oral microbiota comprises more than 300 genera from different parts of the buccal cavity (Zhou et al., 2013). It is expected that disease conditions such as periodontitis and caries construct complex microbiota consisting of Gram-negative anaerobic bacteria, including Porphyromonas gingivalis, Treponema denticola, Prevotella intermedia, Tannerella forsythia, and Agregatibacter actinomycetemcomitans coexisting with Gram-positive anaerobic bacteria (Mason et al., 2013; Jiang et al., 2014). The skin microbiota is composed of over 100 phylotypes, most of which are non-pathogenic. According to geographical location, environmental conditions, and vocations, the colonization of skin microbiota might differ significantly among individuals. Among the skin microbiota, Actinobacteria are the most prevalent phylum of bacteria. Likewise, vaginal microbiota comprises more than 200 phyla and is dominated by Firmicutes, Bacteroidetes, Actinobacteria, and Fusobacteria (Romero et al., 2014), while there is less information available about the microbiota of circulatory fluids of confined human body compartments, such as spinal fluid, blood, and other minor secretions of the human body. Recently, it is suggested that blood uptakes healthy bacteria and their metabolites while it circulates throughout the human body.
Microbial populations (including pathogens) in circulatory fluids of confined compartments (classically considered as sterile), including the spinal fluid and blood, are a relatively new and barely studied concept (Ghose et al., 2019; Lathe and St Clair, 2020; Kang et al., 2021; Liao et al., 2021). In the past, blood microbes were unanimously considered an indication of infection. Conversely, with advances in sequencing and bioinformatics tools, Schierwagen and coworkers analyzed the portal venous blood microbiota via the liver, central venous blood, and peripheral blood (PB) in seven patients with decompensated liver cirrhosis. Buffy coat (BC) 16S rRNA gene sequencing discovered 65 genera across four phyla (predominantly Proteobacteria) (Schierwagen et al., 2019). However, postscript response emphasized that essential controls are required for blood microbiota research (Schierwagen et al., 2020). Human blood runs throughout the body without interacting with the external environment, except for the uptake of nutrients, microbial metabolites, and microbes from the epithelial cells of GIT and other body tissues. Previously, studies showed traces of bacterial DNA in healthy individuals (Nikkari et al., 2001; Païssé et al., 2016), but due to the concept of sterile blood, and high chances of contamination, blood microbiota has been criticized widely. However, the blood microbiota in various domesticated animals and birds has been observed and reported (Mandal et al., 2016; Vientós-Plotts et al., 2017; Scarsella et al., 2020). The evidence of foreign cells in healthy human blood was reported in the late 1960s, and the presence of a metabolically active form of mycoplasma or bacterial L-forms in the blood of clinically healthy humans was detected (Tedeschi et al., 1969). Subsequently, in 1977, researchers found bacterial traces in the blood of healthy subjects (Domingue and Schlegel, 1977). However, the concept was criticized by some researchers. For instance, Mitchell et al. (2016) argue that pleomorphic bacterial-like structures were erythrocyte-derived micro-particles and 16S rDNA sequences were the laboratory contaminants (Mitchell et al., 2016). In another corresponding study, Martel et al. (2017) suggested that the observed bacteria-like structures in human blood are the artifacts of non-living membrane vesicles and aggregates of blood-derived proteins (Martel et al., 2017).
In the 21st century, findings based on new technologies further strengthen the notion that bacterial DNA is present in healthy individuals. In 2001, Nikkari and colleagues found bacterial DNA in the blood of healthy individuals by qPCR, consisting of rRNA-specific fluorescent probes and primers to target the conserved regions of 16S ribosomal DNA of bacteria (Nikkari et al., 2001). Sterile water was filled in vacutainer tubes used as a negative control. It was unclear if the bacterial DNA in blood came from skin, blood, or both. They also suggested to avoid PCR reagent’s background bacterial DNA contamination and to evaluate the most abundant DNA present in PCR reagents. The fractionation of whole blood of healthy individuals identified that of all bacterial DNA, 93.74%, 6.23%, and 0.03% were found in the BC, red blood cells, and plasma (Païssé et al., 2016), respectively. Proteobacteria DNA was the most abundant (more than 80%), including Actinobacteria, Firmicutes, and Bacteroidetes. Using 16S rRNA (rDNA)-specific primers, Moriyama et al. (2008) found a diversified clone of the bacterial population, including Aquabacterium, Stenotrophomonas, Budvicia, Serratia, Bacillus, and Flavobacteria, while no traces of GIT endogenous (bacteroides, clostridium, and lactobacillus) cluster were found (Moriyama et al., 2008). These shreds of evidence initiated the concept of bacterial DNA existence in healthy human instead of conventional thoughts of considering blood as a sterile fluid. Although the presence of blood microbiota requires visual and viable confirmation, studies providing the evidence of bacterial genetic material in healthy human blood and its role in non-communicable diseases are increasing (Païssé et al., 2016; Panaiotov et al., 2018; Whittle et al., 2019; Velmurugan et al., 2020). However, it is unclear whether the bacteria found are the blood microbiota or transient bacteremia as blood cells regularly uptake the bacteria from gut, mucosa, or local infections. Application of robust, innovative technologies, including 16S rRNA sequencing and metagenomics, can improve the blood bacteria detection in detail. Parallel to this, considerable attention must be paid to experimental controls to minimize the effect of unknown factors when studying the microbiome, as the samples are prone to contamination from environmental sources.
How bacteria get into sterile blood
The blood is considered sterile and lacks culturable bacteria. It serves as the best-quality growth medium for in vitro culture of bacteria, since as few as 1–10 ml−1 bacterial cells in blood could be life-threatening during any infection (Bennett et al., 2019). However, few recent studies questioned the sterility of blood in healthy individuals, as it does not imply the lack of latent or non-cultural organisms. In a study, 71% of blood samples obtained from sick individuals and 7% from allegedly healthy people were reported to have a new bacteriological niche (Domingue and Schlegel, 1977). In 2001, samples from healthy individuals were shown to contain 16S bacterial ribosomal DNA following the detection of corynebacteria-like microorganisms in RBC hemocultures (Nikkari et al., 2001). These studies debated whether blood-borne bacteria are valid biological niches or just transitory blood-borne inhabitants. Some experts believe that bacteria in the blood result from translocation from other bodily locations, notably the gastrointestinal system.
Primarily, the presence of bacteria in the blood could be the outcome of microbial translocation from the microbiota enriched locations of the human body, or they can enter during clinical procedures (physical or surgical). Besides these, humans can also acquire a variety of microbes from the environment directly into the human blood (physical injuries, insect bites, scratches, or animal bites and brushing of teeth) (Figure 1). The first-ever entry of the microbes into infants’ blood can occur during gestation. There is evidence that the fetal surroundings, such as amniotic fluid, placenta, fetal membranes, and umbilical cord blood, may have microorganisms (Funkhouser and Bordenstein, 2013). Blood from the maternal side cannot be mixed with fetal blood during gestation. Still, there is evidence that maternal bacteria can be found in fetal blood during pregnancy. The uterus has long been considered sterile, while bacteria have been cultured from the umbilical cord blood samples (Jiménez et al., 2005). This study also favors the concept of healthy circulatory transient bacteria transferred from mother to fetus. In another study, to check the transfer of maternal bacteria to fetus, pregnant mice were fed with genetically tagged E. fecium strain from women’s breast milk. PCR validated the tagged strain in pups’ meconium (Jiménez et al., 2008). In contrast, some believe that the fetal microbiome is acquired through the uterus and enriched after birth (Romano-Keeler and Weitkamp, 2015).
The oral microbiota can enter the blood when the tight junctions of cells are compromised (Iwai, 2009) or the gums are damaged during the brushing of teeth. Skin bacteria can also escape into the blood in case of any damage to the skin barrier (Cogen et al., 2008). Comparing human microbiome project (HMP) data and healthy human blood microbiota mostly shares microbial DNA of skin and oral microbiota (Whittle et al., 2019). However, previous studies claim that DNA analysis showed significant variation from the gut microbiota. We believe that it can be due to the diffusion of bacteria into blood circulation as an unusual incidence, which may frequently occur in healthy people. Usually, it is considered that bacteria can enter blood circulation only when the epithelial barriers are compromised. However, even if the intestinal membranes are intact, bacteria can enter the circulatory system through different intestinal and circulatory cells (Wiest et al., 2014). Dendritic cells, for instance, can uptake microbial products via crossing processes between epithelial cells without affecting tight junction function (Niess et al., 2005). These are the primary routes that challenge blood sterility. Intestinal mucus-secreting goblet cells and mucosal lymph cells (specialized epithelial cells of the mucosa-associated lymphoid tissues) lying over Peyer’s patches also act as the microbial carrier from the intestine to blood circulation (Castillo et al., 2019). To date, we have mere information regarding the transfer of bacteria to blood via general injuries, surgical and non-surgical procedures at hospitals, and animal or insect bites. Extensive studies are required to unveil the acquisition of healthy blood microflora from different possible sources. Major pilot studies from different countries across the continents can solve this myth to some extent. Recently, a pilot study reported the presence of blood microbiome in a generally healthy population recruited under the MARK-AGE project of European countries (D’aquila et al., 2021). Additionally, researchers have to investigate the escape of lung microbiota from lung tissues to the blood. As blood continues to circulate, it is likely to contain transitory bacteria or bacterial DNA.
Human presumptive blood microbiota profile
Knowing if and how bacteria may survive in the blood is critical because blood is a hostile environment for germs due to bacteriostatic and bactericidal components (Markova, 2017). It is considered that microbiota can be taken up from the intestine while the composition of healthy blood bacteria is different from the intestinal microbiota. Unlike the intestinal microbiota, where Firmicutes and Bacteroidetes are the most common bacterial phyla, the reported blood microbiota is dominated by the Proteobacteria, followed by Actinobacteria, Firmicutes, and Bacteroidetes (Velmurugan et al., 2020). When it comes to blood microbiota compositions, there are some commonalities among them: Proteobacteria predominates (with relative abundance values typically ranging from 85% to 90%), while Firmicutes, Actinobacteria, and Bacteroidetes show up in much smaller numbers (Figure 2A) (Amar et al., 2013; Dinakaran et al., 2014; Qiu et al., 2019). There are reservations that blood samples may be contaminated by the laboratory environment, DNA extraction kits, or equipment. However, the studies published to date have similar results at the phyla level, suggesting that a core blood microbiota profile may exist and is independent of the research environment or analysis approach. However, several considerations need to be taken into account, including transient bacteremia, immune clearance via macrophages, and contamination from skin, equipment, or reagents while studying blood microbiota. Early studies only detected L-form bacteria (Tedeschi et al., 1969), and growing phases of bacteria and pleomorphic antibiotics-susceptible bacteria (Domingue and Schlegel, 1977; Mclaughlin et al., 2002) in the blood with old-fashioned technologies. The new and innovative molecular techniques are being used to discover non-culturable bacteria from human microbiota, showing the wide range of individual differences among the microorganisms. The revised bacteria-human cell ratio (B/H= 1.3), indicate that bacterial cells are slightly more than the human cells (Sender et al., 2016). Though microbial cells are more numerous and smaller in size than human cells, they account for only 0.3% of human body weight and over 99% genes (Figure 2B) present in human body (Qin et al., 2010; Sender et al., 2016).
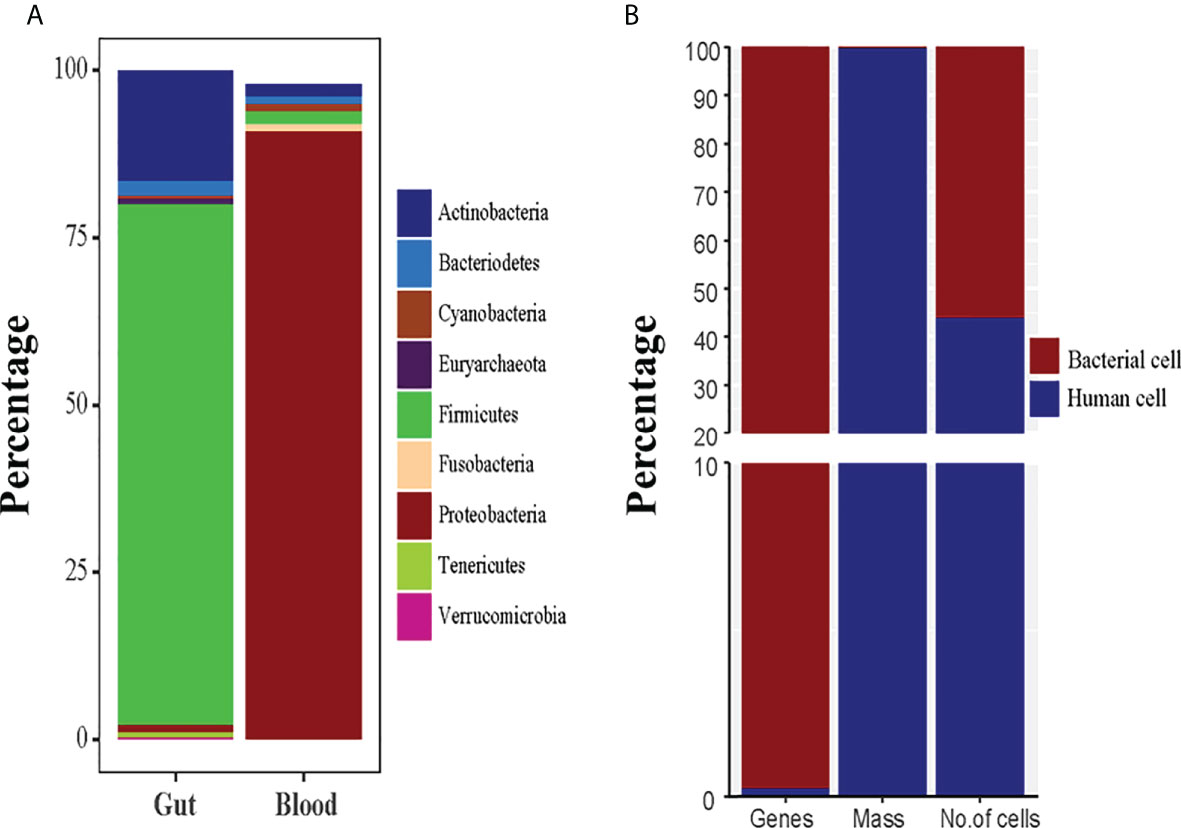
Figure 2 (A) The normal healthy microbiota of the human gut and blood are compared in terms of microbial diversity. (B) The human body system and the human microbiota are contrasted in terms of weight, cells, and genes.
Recent studies have comprehensively characterized bacterial diversity among healthy human blood. In a culture-based study by Damgaard et al. (2015), Propionibacterium acnes were the most prominent taxa found in the blood of 62% of healthy individuals, followed by Staphylococcus epidermis and Bacilli and Micrococcus species (Damgaard et al., 2015), which might be due to contamination of skin microbiota as these bacteria are common skin microbiota. However, Bacillus, Flavobacteria, Stenotrophomas, and Serratia were the most prevalent taxa found in an earlier study of two healthy individuals’ blood (Moriyama et al., 2008). A study was designed to characterize the bacterial diversity in blood fraction (RBCs, BC, and plasma) of 32 healthy individuals at the French national blood collection center (Etablissement Français du sang). The blood bacteria were characterized by 16S rDNA qPCR and 16S metagenomics. The majority of the blood bacterial DNA (93.74%) was found in the BC as compared to RBCs (6.23%) and plasma (0.03%). Blood fractions include bacterial DNA primarily from the Proteobacteria phylum (more than 80%) and Actinobacteria, Firmicutes, and Bacteroidetes. At a higher taxonomic level, the bacterial profiles of the different blood fractions differ dramatically. Fusobacteria and Flavobacteria were abundant in RBCs, while Actinobacteria, Bacilli, and Clostridia members were more prevalent in plasma and erythrocyte fractions than BC (Païssé et al., 2016).
The presence of bacterial DNA can be the outcome of immune or intestinal cell-related bacteria or free bacterial DNA due to transient event of immune clearance. The Sphingobacteria class is predominantly found in BC and plasma. Similarly, a study was designed to analyze the blood bacteria in elderly subjects; interestingly, the results were in line with previous studies. Most of the bacterial DNA found in blood samples of elderly subjects belonged to the phyla Proteobacteria and Actinobacteria, while Firmicutes and Bacteroidetes were underrepresented and were not found in all subjects (Gargari et al., 2021). Bacterial DNA-based results of all these studies are almost similar, which urges scientists to study more to establish the details of blood microbiota. However, bacterial DNA contamination from extraction kits and reagents has been found in bacteriology laboratories and often comprises Bacillus, Flavobacteria, Fusobacteria, Propionibacterium, and Serratia (Glassing et al., 2016). These studies have tested the reagents and designed particular protocols to minimize contamination. Blood microbiota affects host physiology and health, regardless of origin (skin, stomach, oral cavity, placenta, or lung) or form of bacterial DNA (free DNA, free bacterial cells, or bacteria internalized in blood cells). The blood bacterial populations reported in several studies are stable regardless of age or gender, with minor alterations due to past disease, surgery, and non-infectious and metabolic diseases. Non-communicable and metabolic disorders have altered the blood microbiome, according to studies (Qiu et al., 2019; Shah et al., 2019). These findings may support the presence of bacterial DNA in healthy humans’ blood and lack the evidence for live bacteria due to culture limitations. Still, its source must be determined (transient bacteria, accidental uptake, or contaminations). Blood microbiota is only significant and scientifically intriguing if it represents an undisturbed condition, not background contamination, WBC- or RBC-related bacteria, or immune clearance.
Is blood microbiota consistent with transient bacteremia
The study of blood microbiota is even more challenging because of the low concentration of bacterial DNA, higher chances of contamination during phlebotomy, and background contamination. Therefore it requires highly sterile laboratory equipment and advanced molecular techniques. The use of microbial blood culture, quantitative PCR, next-generation 16S rRNA gene sequencing (Moriyama et al., 2008; Amar et al., 2013; Païssé et al., 2016), shotgun metagenome sequencing (Kowarsky et al., 2017), and 16 rRNA gene targeted sequencing Illumina MiSeq (Panaiotov et al., 2018; Qiu et al., 2019) has provided the supporting evidence of the existence of diverse bacterial population in healthy human blood. There are considerable differences between the blood bacteria found in healthy and diseased individuals although samples were being processed equally. Certain diseases appear to be linked to an organism’s morphology (for example, coccus versus bacillus). Pleomorphic forms of different pathogenic bacteria such as coccus or bacilli are present in diseased individuals, while no such variation is found in healthy individuals. Various studies of healthy blood bacteria in different laboratories have reported a similar composition of microbial phyla and slight variations at the class level. Collectively, these results minimize the possibilities of contamination. In a separate study, blood and neutrophil-associated microbiota were characterized by Qiurong Li and colleagues in patients with severe acute pancreatitis (SAP). Bacteroidetes and Firmicutes were more prevalent in patients’ blood and neutrophil-associated microbiota than in healthy controls, though Actinobacteria were less prevalent. While there was no statistically significant difference in bacterial composition between patient subgroups, these findings suggest that changes in the blood bacteria may not be associated with the presence or absence of infectious complications in SAP (Li et al., 2018).
In clinical microbiology studies, molecular techniques are being used extensively to detect blood bacteria in diseased or healthy individuals. Culture- and molecular method-based studies found that the BC samples of patients with liver cirrhosis were dominated by proteobacteria, consistent with the previously reported blood microbiota of healthy individuals (Païssé et al., 2016) and patients with liver fibrosis (Lelouvier et al., 2016). Different comparison studies of diseases and healthy individuals reported the same blood microbiota of healthy individuals (Table 1). In a commentary, Hornung et al. (2020) proposed that those culturable strains of Staphylococcus and Acinetobacter are known contaminants of skin and water, respectively (Hornung et al., 2020). In response, Schierwagen and colleagues explained that the bacterial genera listed by Hornung et al. (2020) as possible contaminants were not the result of any contamination in their study. These bacteria were absent or significantly lower in negative controls than in blood samples (Schierwagen et al., 2020).
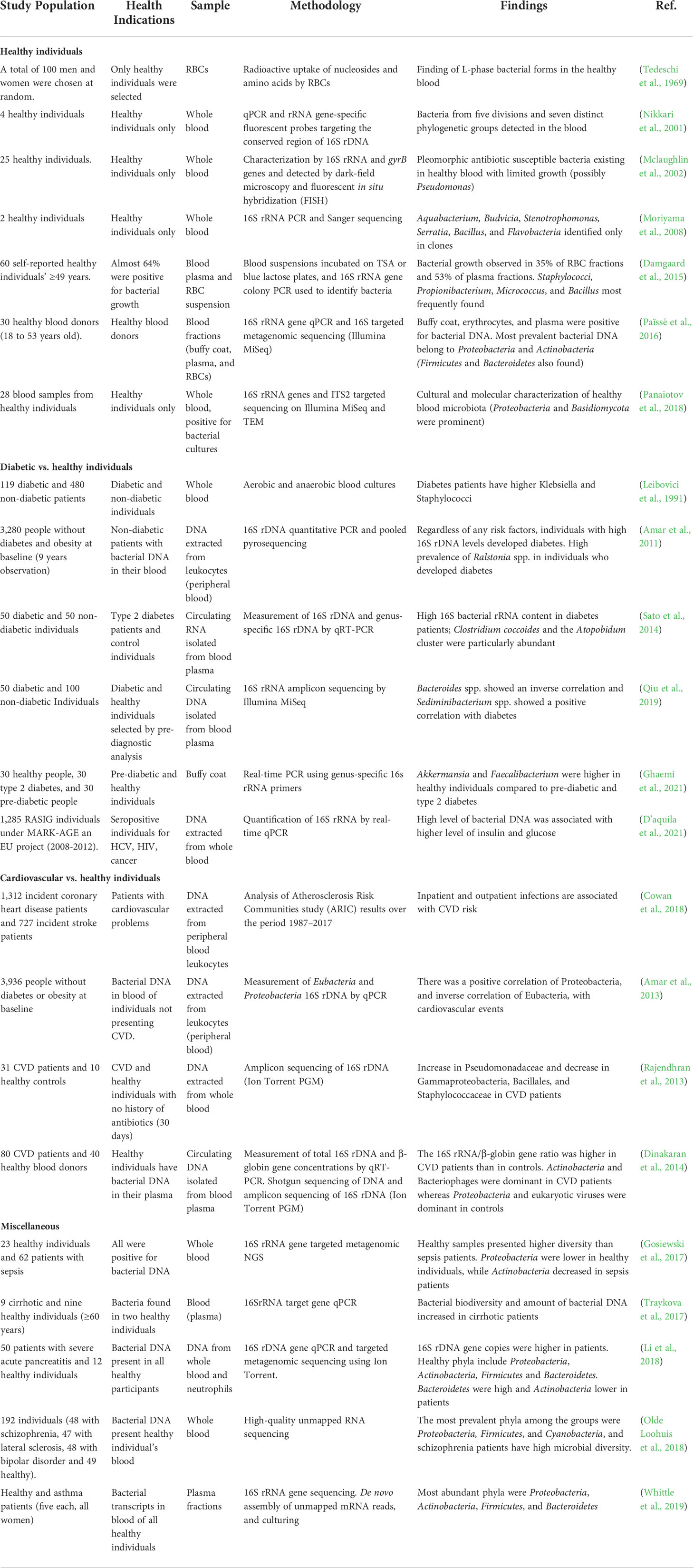
Table 1 Summary of different human blood microbiota studies conducted on healthy or diseased individuals.
In many cases, relevant organisms are found during intracellular or transient episodes of bacteremia, which is difficult to explain through contamination. Though the skin or laboratory contamination will remain a serious concern, many studies have proposed the blood microbiota with the help of advanced molecular techniques in blood and serum, all of which use proper and cautious controls. The same methods used to detect the bacteria in blood cultures during sepsis is also considered the standard diagnostic tool for bloodstream infections (Muñoz et al., 2008; Varani et al., 2009); this supports the fact that the careful sample collection and appropriate negative controls can be helpful to study the healthy blood microbiota.
Healthy blood bacteria dysbiosis in chronic diseases
Since blood microbiota does not induce complications like inflammation and sepsis, it may play an essential role in normal physiology and immunity. Previously, the majority of non-culturable forms of bacteria found in wounds and certain diseases such as cystic fibrosis or tuberculosis are “normally culturable” now (Potgieter et al., 2015). Dormancy is well-known in microbiological research and is critical when studying microbial ecology. Blood microbiota may not cause infections or other complications because it is dormant (Potgieter et al., 2015). However, it might be related to dysbiosis in some conditions. The term “dysbiosis” refers to a change in symbiotic or commensal microbial communities (Petersen and Round, 2014; Levy et al., 2017). Yet, it is unclear whether dysbiosis is a cause of a disease or simply a reflection of it (Bäckhed et al., 2012; Yoo et al., 2020).
To date, dysbiosis of intestinal microbiota in diabetes (Xie et al., 2021), CVD (Zhu et al., 2020), asthma (Lu and Zou, 2020), and complex inflammatory diseases, including Alzheimer’s disease (Shabbir et al., 2021; Zhang et al., 2021) and Parkinson’s disease (Peng et al., 2021), has been discussed extensively. Non-communicable and metabolic diseases that impact bacterial translocation and dysbiosis in the blood are listed in Table 1. In contrast, little research has been conducted to uncover the role of blood microbiota dysbiosis in metabolic or cognitive disorders. In most cases, blood is protected from intestinal microbes and microbial products by gut immunological barriers and intestinal cells. The reticuloendothelial system attempts to clear microbes, microbial metabolites, or toxins passed through the gut barrier into the bloodstream. If this is unsuccessful, dysbiosis of blood bacteria may lead to chronic inflammation, which leads to metabolic disorders including diabetes, pancreatitis, liver cirrhosis, and CVD. Patients with Alzheimer’s disease have periodontitis-causing bacterium P. gingivalis in their brain tissue, indicating that the bacteria had moved from the oral cavity to the brain through the bloodstream (Dominy et al., 2019). A number of studies have been conducted to investigate the link between blood microbial dysbiosis and chronic illnesses. Blood bacteria and bacterial lipopolysaccharides (LPS) are associated with diabetes. Patients with type 2 diabetes mellitus have significantly higher levels of the LPS-binding protein (LBP), and LBP was positively correlated with the glycated hemoglobin, body mass index, and inflammatory markers of study participants (Donath and Shoelson, 2011).
In a longitudinal study of 3,280 non-diabetic individuals, higher baseline levels of blood bacterial 16S rDNA were found as an independent risk factor for the onset of type 2 diabetes. However, the same study reported no significant difference between the HBM of healthy and diabetic individuals (Amar et al., 2011). The pyrosequencing analysis revealed a higher abundance of Proteobacteria in both type 2 diabetes mellitus and non-diabetic individuals, with diabatic individuals having a higher abundance of Ralstonia spp. (Amar et al., 2011), while subsequent studies have found detailed variations at the genus level in gut microbiota and blood plasma and cellular levels. Patients with pre-diabetes or type 2 diabetes have fewer Faecalibacterium, Akkermansia, and Bifidobacterium bacteria in their leukocytes than healthy people, according to an epidemiological study on Iranian diabetes patients. The bacterial loads of E. coli and Bacteroides fragilis were higher in pre-diabetic individuals than healthy individuals, while type 2 diabetes groups had higher Lactobacillus, E. coli, and Bacteroides fragilis (Ghaemi et al., 2021). However, according to a case study in the Chinese population, no differences were reported in the baseline microbiota diversity of diabetes and control participants. The same study reported significant variations in diversity at the genus level. For example, the relative abundance of Aquabacterium, Xanthomonas, and Pseudonocardia was low and that of Actinotalea, Alishewanella, Sediminibacterium, and Pseudoclavibacter was highly prevalent in T2DM patients compared to healthy individuals. The genus Bacteroides was inversely correlated and Sediminibacterium was positively correlated with the risk of diabetes (Qiu et al., 2019). IM bacteria have been found in 28% of diabetes patients in a study, but healthy participants had only 4% of the IM-derived bacterial load.
Similarly, individuals with type 2 diabetes had higher blood plasma, and gut microbiota levels of Gram-positive bacteria (specifically Clostridium coccoides and the Atopobium cluster) were significantly higher in type 2 diabetes patients than in healthy controls (Sato et al., 2014). Recently, a European study of 1,285 individuals showed that higher levels of blood bacterial DNA were linked to higher blood glucose and insulin levels in a randomly selected age-stratified general population (D’aquila et al., 2021) (Table 1). These studies suggest a causal link between the onset of diabetes and HBM dysbiosis. The findings suggest that dysbiosis of blood bacteria can be used as a biomarker for the early diagnosis and control of diabetes.
In an epidemiological study, gut bacterial DNA was examined in blood using 16S rRNA and IM Microbial qPCR microarray;, 90% of patients with liver cirrhosis had higher gut microbiota diversity than healthy individuals. The diseased cohort’s blood had a higher total bacterial DNA concentration than healthy controls (Traykova et al., 2017). Additionally, researchers used the LDA effect size (LEfSe) method to examine the circulating blood bacteria of patients with liver cirrhosis. Enterobacteriaceae abundance in PB patients with liver cirrhosis was higher than healthy individuals. At the same time, the levels of Akkermansia, Rikenellaceae, and Erysipelotrichales were significantly higher in the PB of healthy individuals compared to cirrhotics (Kajihara et al., 2019). An increase in Bacteroidetes and Firmicutes was observed, while an overall decrease in Actinobacteria was observed in pancreatitis patients compared to the healthy human cohort. Bacteroidia and Clostridia numbers increased in the diseased group while Actinobacteria, Flavobacteria, and Bacilli numbers decreased. However, no significant composition differences were found between patient subgroups (Li et al., 2018). This suggests that changes in the microbiota are not linked to the presence or absence of infectious complications in SAP, and discrepancies in dominant taxa in patients with pancreatitis have a dysbiotic blood microbiota. Researchers have also hypothesized that blood microbiota could risk developing nonalcoholic fatty liver disease (NAFLD) (Lelouvier et al., 2016; Yun et al., 2019). It was found that patients with liver fibrosis (LF) had higher levels of the 16S rDNA gene in their blood than healthy individuals. Analysis of the LEfSe algorithm showed that the patients with LF had lower proportions of Actinobacteria than healthy controls, whereas Proteobacteria was higher in the same group (Lelouvier et al., 2016).
Blood bacterial dysbiosis may cause cardiovascular diseases. Microbes’ role in CVD was previously limited to pathogen-infected complications like rheumatic carditis, pericarditis, myocarditis, and endocarditis. A recent study found that intestinal tight junction protein disruption causes microbial translocation from the gut to the blood in ST-segment elevation myocardial infarction patients and mouse models. Intestinal bacteria were found in the blood of STEMI patients (Lactobacillus, Bacteroides, and Streptococcus) (Zhou et al., 2018). In a long-term study, Amar et al. (2013) discovered that the blood of patients with an acute cardiovascular event significantly decreased total bacterial DNA compared to a healthy cohort and increased taxa assigned to the Proteobacteria (Amar et al., 2013). 16S rRNA sequencing was used to characterize the microbial communities in the whole blood of CVD patients and healthy controls (barcoded ion sequencing). Despite no change in bacterial diversity at the phylum level, Proteobacteria increased while Firmicutes decreased. Gammaproteobacteria, Bacillales, and Staphylococcaceae saw significant increases at lower taxonomic levels, but Pseudomonadaceae was the only one to show a significant increase. The study also showed that bacterial DNA and circulating virome were abundant in CVD patients. There are significant variations at the species level in different studies as the subjects of the studies have different selection criteria. Furthermore, the different origins of sample processing (cellular blood DNA, RNA from whole blood, or DNA from blood plasma) and methodologies used to characterize blood microbiota (qPCR, pyrosequencing, amplicon, and metagenome sequencing) can affect the results of blood microbiota.
Blood bacteria as potential biomarkers
Molecular technologies such as next-generation sequencing, metagenomics, and targeted detection methods have dispelled the myth that blood is a sterile environment. Circulating bacterial populations can be a considerable biomarker for diagnosing infectious and non-infectious diseases. Recently, several fascinating studies proved that the gut microbiome acts as a diagnostic biomarker for numerous diseases, including type 2 diabetes (Qiu et al., 2019), CVD, heart failure (Kazemian et al., 2020), liver cirrhosis (Qin et al., 2014), colorectal cancer, and pancreatic carcinoma (Veziant et al., 2021). Similarly, altered blood bacteria in type 2 diabetes and cardiovascular disease could serve as a potential biomarker. Sequencing methods can be helpful to reveal the association of circulating bacteria dysbiosis and liver diseases. Variations in blood bacteria have been observed, and therefore, changes in circulating bacteria at early stages of CVD (Dinakaran et al., 2014), atherosclerosis (Sato et al., 2014), type 2 diabetes (Amar et al., 2013), and other non-congenital diseases can be used as biomarkers. A separate study recommended that variation in blood bacteria could be used as a biomarker to diagnose nonalcoholic fatty liver diseases (Lelouvier et al., 2016) in obese individuals. Similarly, the concentration of 16S rDNA in the blood can be used as an early predictor of diabetes in a healthy population (Amar et al., 2011). Although the patient with type 2 diabetes mellitus has no obvious difference at phyla and class level from healthy individuals, the genera Sediminibacterium was abundant in diabetes patients while Bacteroides were higher in non-diabetic patients (Qiu et al., 2019; Anhê et al., 2020). Cardiovascular disease is associated with an increase in Proteobacteria phylum in blood, while an elevated Actinobacteria : Proteobacteria ratio is a characteristic of individuals with cardiovascular disease (Amar et al., 2013; Dinakaran et al., 2014).
Recently, circulating bacterial DNA was used to characterize rheumatoid arthritis (RA) and results showed that the taxonomic ecology of RA patients’ blood microbiota was different from ankylosing spondylitis (AS), psoriatic arthritis (PA), and healthy cases (Hammad et al., 2020). In Parkinson’s disease, Alzheimer’s disease, and type 2 diabetes mellitus patients, correlative light-electron microscopy has revealed an accumulation of LPS secreted by reactivated dormant blood bacteria in the blood, which may alter blood coagulation (Pretorius et al., 2016), systemic inflammation, and blood–brain barrier permeability, and affect the hematological system (Kell and Pretorius, 2018). Recently, a study related to the biomarkers of the human aging project explained that microbial blood DNA could be positively associated with the level of FFA (free fatty acids). FFA plays a crucial role in different immunological and physiological processes. Furthermore, blood bacterial DNA was positively correlated with increased leukocytes, blood insulin, and glucose (D’aquila et al., 2021). The remarkable agreement of data across separate investigations demonstrated the presence of a core blood bacterial population dominated by Proteobacteria, with Actinobacteria, Firmicutes, and Bacteroidetes present in a lower amount. Thus, changes in the microbial blood composition have been linked to pathological states, suggesting that the microbiota’s composition can serve as an early biomarker of disease risk.
Concluding remarks and future perspectives
Years of ongoing research and advancements in molecular techniques enabled the study of unculturable bacteria from various settings such as soil, water, gut, and blood samples. All this has been done to refute the idea that blood is a sterile environment. Using these techniques, studies have reported the presence of blood bacteria in healthy and diseased conditions. However, at this stage, it is very confusing to state it as blood microbiota research has been marred by fundamental faults such as skin, reagent, and laboratory equipment contamination, casting doubt on the findings’ validity. Moreover, transient immune clearance and uptake of bacterial cells by dendritic or M cells are considered one reason for the presence of blood bacteria. Considering these entire issues, still, researchers use healthy human blood as negative controls to compare the dysbiosis of blood bacteria of diseased individuals. Despite the shortcomings of the studies, a consistent image of blood microbiota will emerge by adopting sophisticated advanced techniques, including microscopy and DNA and RNA sequencing analysis. As it turns out, the blood bacteria are dominated by Proteobacteria, with smaller proportions of Actinobacteria, Firmicutes, and Bacteroidetes present (Moriyama et al., 2008; Amar et al., 2013; Damgaard et al., 2015; Lelouvier et al., 2016; Païssé et al., 2016; Panaiotov et al., 2018). A healthy (non-diseased) human blood microbiota is contentious. The evidence presented here and the tendency toward comparing blood microbiota as analytical (positive and negative) controls in diseased and healthy individuals cannot rule out background contamination, immunological clearance of bacteria by blood cells, or temporary bacteremia events. The concept of a healthy (disease-free) human blood microbiota is relatively novel and disputed. This knowledge and the tendency toward comparing blood bacteria as analytical (positive and negative) controls in diseased and healthy individuals are insufficient to exclude the possibility of background contamination, immunological clearance of bacteria by blood cells, and temporary bacteremia episodes. Although some bacterial phyla are beneficial or non-pathogenic, they cannot be considered microbiota because they are not alive or capable of multiplying in blood. In reality, these bacterial populations can result from physiological health problems or the factors mentioned before.
More detailed and thorough studies will help to understand the role of blood bacteria dysbiosis in disease mechanisms and as a biomarker for the early diagnosis of different diseases. Circulating bacteria can also be used as a therapeutic tool to restore the altered blood bacteria and bacterial metabolites in different metabolic and infectious diseases. However, it is still crucial to assume that reported blood microbiota is a static or dynamic population of microbes as there are serious concerns to be addressed first, such as background contamination of reagents, phlebotomy, immune clearance, or temporary bacterial load due to transient bacteremia. We encourage future research that considers the addition of external bacteria with the “time” component. Geographic translocation, animal and insect bites, surgical histories, oral irrigation devices, and wounds can add additional bacteria to healthy human blood. All these factors transfer infectious and non-infectious bacteria to healthy humans. Circulating bacterial composition can be affected by age, geography, and socioeconomic level (e.g., access to nutritious food and healthcare services). What is most important is to design solid experimental designs that require minimum handling and avoid cross-contamination of skin, reagents, and equipment. Scientists must improve the sample collection, processing, and data production process. Microbial DNA from needles, vacutainers, chemicals, and other consumables can be analyzed to see if any possible contamination is present. Finally, blood microbiota research is essential for establishing bacteria and other microorganisms’ potential roles and functions in human blood. The accumulating data on hypothetical blood microorganisms in healthy individuals support the development of novel, therapeutically significant research avenues.
Author contributions
MG and RL: Conceptualization, investigation, writing the original draft, and preparation. AM: Collection of data, reviewing, and editing. LG and HD: Writing the original draft and reviewing the manuscript. GW: Conceptualization, critical review and formatting of the final manuscript, and supervision. All authors contributed to the article and approved the submitted version.
Funding
This study was supported by grants from 2020 Li Ka Shing Foundation Cross-Disciplinary Research Grant (2020LKSFG01E), the Natural Science Foundation of Guangdong Province (2021A1515012470), the Department of Education of Guangdong Province (2020KZDZX1083), and Shantou Science and Technology Bureau (Shanfuke[2020]88-STKJ2021197, Shanfuke[2020]53-51 and [2020]16-2).
Conflict of interest
The authors declare that the research was conducted in the absence of any commercial or financial relationships that could be construed as a potential conflict of interest.
Publisher’s note
All claims expressed in this article are solely those of the authors and do not necessarily represent those of their affiliated organizations, or those of the publisher, the editors and the reviewers. Any product that may be evaluated in this article, or claim that may be made by its manufacturer, is not guaranteed or endorsed by the publisher.
References
Amar, J., Lange, C., Payros, G., Garret, C., Chabo, C., Lantieri, O., et al. (2013). Blood microbiota dysbiosis is associated with the onset of cardiovascular events in a large general population: the DESIR study. PloS One 8, e54461. doi: 10.1371/journal.pone.0054461
Amar, J., Serino, M., Lange, C., Chabo, C., Iacovoni, J., Mondot, S., et al. (2011). Involvement of tissue bacteria in the onset of diabetes in humans: evidence for a concept. Diabetologia 54, 3055–3061. doi: 10.1007/s00125-011-2329-8
Anhê, F. F., Jensen, B., Varin, T. V., Servant, F., Van Blerk, S., Richard, D., et al. (2020). Type 2 diabetes influences bacterial tissue compartmentalisation in human obesity. Nat. Metab. 2, 233–242. doi: 10.1038/s42255-020-0178-9
Arumugam, M., Raes, J., Pelletier, E., Le Paslier, D., Yamada, T., Mende, D. R., et al. (2011). Enterotypes of the human gut microbiome. Nature 473, 174–180. doi: 10.1038/nature09944
Bäckhed, F., Fraser, C. M., Ringel, Y., Sanders, M. E., Sartor, R. B., Sherman, P. M., et al. (2012). Defining a healthy human gut microbiome: current concepts, future directions, and clinical applications. Cell Host µbe 12, 611–622. doi: 10.1016/j.chom.2012.10.012
Bennett, J. E., Dolin, R., Blaser, M. J. (2019). Principles and Practice of Infectious Diseases. (USA: Elsevier Inc.).
Brown, C. T., Sharon, I., Thomas, B. C., Castelle, C. J., Morowitz, M. J., Banfield, J. F. (2013). Genome resolved analysis of a premature infant gut microbial community reveals a varibaculum cambriense genome and a shift towards fermentation-based metabolism during the third week of life. Microbiome 1, 1–19. doi: 10.1186/2049-2618-1-30
Castillo, D. J., Rifkin, R. F., Cowan, D. A., Potgieter, M. (2019). The healthy human blood microbiome: Fact or fiction? Front. Cell. Infection Microbiol. 9. doi: 10.3389/fcimb.2019.00148
Clemente, J. C., Ursell, L. K., Parfrey, L. W., Knight, R. (2012). The impact of the gut microbiota on human health: an integrative view. Cell 148, 1258–1270. doi: 10.1016/j.cell.2012.01.035
Cogen, A. L., Nizet, V., Gallo, R. L. (2008). Skin microbiota: a source of disease or defence? Br. J. Dermatol. 158, 442–455. doi: 10.1111/j.1365-2133.2008.08437.x
Cowan, L. T., Lutsey, P. L., Pankow, J. S., Matsushita, K., Ishigami, J., Lakshminarayan, K. (2018). Inpatient and outpatient infection as a trigger of cardiovascular disease: The ARIC study. J. Am. Heart Assoc. 7, e009683. doi: 10.1161/JAHA.118.009683
Damgaard, C., Magnussen, K., Enevold, C., Nilsson, M., Tolker-Nielsen, T., Holmstrup, P., et al. (2015). Viable bacteria associated with red blood cells and plasma in freshly drawn blood donations. PloS One 10, e0120826. doi: 10.1371/journal.pone.0120826
D’aquila, P., Giacconi, R., Malavolta, M., Piacenza, F., Bürkle, A., Villanueva, M. M., et al. (2021). Microbiome in blood samples from the general population recruited in the MARK-AGE project: A pilot study. Front. Cell. Infection Microbiol. 12, 707515. doi: 10.3389/fmicb.2021.707515
Dinakaran, V., Rathinavel, A., Pushpanathan, M., Sivakumar, R., Gunasekaran, P., Rajendhran, J. (2014). Elevated levels of circulating DNA in cardiovascular disease patients: Metagenomic profiling of microbiome in the circulation. PloS One 9, e105221. doi: 10.1371/journal.pone.0105221
Domingue, G. J., Schlegel, J. U. (1977). ). novel bacterial structures in human blood: cultural isolation. Infection Immun. 15, 621–627. doi: 10.1128/iai.15.2.621-627.1977
Dominy, S. S., Lynch, C., Ermini, F., Benedyk, M., Marczyk, A., Konradi, A., et al. (2019). Porphyromonas gingivalis in alzheimer’s disease brains: Evidence for disease causation and treatment with small-molecule inhibitors. Sci. Adv. 5, eaau3333. doi: 10.1126/sciadv.aau3333
Donath, M. Y., Shoelson, S. E. (2011). Type 2 diabetes as an inflammatory disease. Nat. Rev. Immunol. 11, 98–107. doi: 10.1038/nri2925
Funkhouser, L. J., Bordenstein, S. R. (2013). Mom knows best: The universality of maternal microbial transmission. PloS Biol. 11, e1001631. doi: 10.1371/journal.pbio.1001631
Gargari, G., Mantegazza, G., Taverniti, V., Del Bo, C., Bernardi, S., Andres-Lacueva, C., et al. (2021). Bacterial DNAemia is associated with serum zonulin levels in older subjects. Sci. Rep. 11, 1–13. doi: 10.1038/s41598-021-90476-0
Ghaemi, F., Fateh, A., Sepahy, A. A., Zangeneh, M., Ghanei, M., Siadat, S. D. (2021). Blood microbiota composition in Iranian pre-diabetic and type 2 diabetic patients. Hum. Antibodies 29 (4), 243–248. doi: 10.3233/HAB-210450
Ghose, C., Ly, M., Schwanemann, L. K., Shin, J. H., Atab, K., Barr, J. J., et al. (2019). The virome of cerebrospinal fluid: Viruses where we once thought there were none. Front. Microbiol. 10, 2061. doi: 10.3389/fmicb.2019.02061
Glassing, A., Dowd, S. E., Galandiuk, S., Davis, B., Chiodini, R. J. (2016). Inherent bacterial DNA contamination of extraction and sequencing reagents may affect interpretation of microbiota in low bacterial biomass samples. Gut Pathog. 8, 24. doi: 10.1186/s13099-016-0103-7
Gosiewski, T., Ludwig-Galezowska, A. H., Huminska, K., Sroka-Oleksiak, A., Radkowski, P., Salamon, D., et al. (2017). Comprehensive detection and identification of bacterial DNA in the blood of patients with sepsis and healthy volunteers using next-generation sequencing method - the observation of DNAemia. Eur. J. Clin. Microbiol. Infect. Dis. 36, 329–336. doi: 10.1007/s10096-016-2805-7
Hammad, D. B. M., Hider, S. L., Liyanapathirana, V. C., Tonge, D. P. (2020). Molecular characterization of circulating microbiome signatures in rheumatoid arthritis. Front. Cell. Infection Microbiol. 9. doi: 10.3389/fcimb.2019.00440
Hornung, B. V. H., Zwittink, R. D., Ducarmon, Q. R., Kuijper, E. J. (2020). Response to: ‘Circulating microbiome in blood of different circulatory compartments’ by schierwagen et al. Gut 69, 789–790. doi: 10.1136/gutjnl-2019-318601
Iwai, T. (2009). Periodontal bacteremia and various vascular diseases. J. periodontal Res. 44, 689–694. doi: 10.1111/j.1600-0765.2008.01165.x
Jiang, W., Ling, Z., Lin, X., Chen, Y., Zhang, J., Yu, J., et al. (2014). Pyrosequencing analysis of oral microbiota shifting in various caries states in childhood. Microbial Ecol. 67, 962–969. doi: 10.1007/s00248-014-0372-y
Jiménez, E., Fernández, L., Marín, M. L., Martín, R., Odriozola, J. M., Nueno-Palop, C., et al. (2005). Isolation of commensal bacteria from umbilical cord blood of healthy neonates born by cesarean section. Curr. Microbiol. 51, 270–274. doi: 10.1007/s00284-005-0020-3
Jiménez, E., Marín, M. L., Martín, R., Odriozola, J. M., Olivares, M., Xaus, J., et al. (2008). Is meconium from healthy newborns actually sterile? Res. Microbiol. 159, 187–193. doi: 10.1016/j.resmic.2007.12.007
Kajihara, M., Koido, S., Kanai, T., Ito, Z., Matsumoto, Y., Takakura, K., et al. (2019). Characterisation of blood microbiota in patients with liver cirrhosis. Eur. J. Gastroenterol. Hepatol. 31, 1577–1583. doi: 10.1097/MEG.0000000000001494
Kang, Y., Ji, X., Guo, L., Xia, H., Yang, X., Xie, Z., et al. (2021).Cerebrospinal fluid from healthy pregnant women does not harbor a detectable microbial community. Microbiology Spectrum 9, e00769–e00721. doi: 10.1128/Spectrum.00769-21
Kazemian, N., Mahmoudi, M., Halperin, F., Wu, J. C., Pakpour, S. (2020). Gut microbiota and cardiovascular disease: opportunities and challenges. Microbiome 8, 36. doi: 10.1186/s40168-020-00821-0
Kell, D. B., Pretorius, E. (2018). No effects without causes: the iron dysregulation and dormant microbes hypothesis for chronic, inflammatory diseases. Biol. Rev. Camb Philos. Soc. 93, 1518–1557. doi: 10.1111/brv.12407
Kowarsky, M., Camunas-Soler, J., Kertesz, M., De Vlaminck, I., Koh, W., Pan, W., et al. (2017). Numerous uncharacterized and highly divergent microbes which colonize humans are revealed by circulating cell-free DNA. Proc. Natl. Acad. Sci. 114, 9623–9628. doi: 10.1073/pnas.1707009114
Lathe, R., St Clair, D. (2020). From conifers to cognition: Microbes, brain and behavior. Genes Brain Behav. 19, e12680. doi: 10.1111/gbb.12680
Leibovici, L., Samra, Z., Konisberger, H., Kalter-Leibovici, O., Pitlik, S. D., Drucker, M. (1991). Bacteremia in adult diabetic patients. Diabetes Care 14, 89–94. doi: 10.2337/diacare.14.2.89
Lelouvier, B., Servant, F., Païssé, S., Brunet, A. C., Benyahya, S., Serino, M., et al. (2016). Changes in blood microbiota profiles associated with liver fibrosis in obese patients: A pilot analysis. Hepatology 64, 2015–2027. doi: 10.1002/hep.28829
Levy, M., Kolodziejczyk, A. A., Thaiss, C. A., Elinav, E. (2017). Dysbiosis and the immune system. Nature Reviews Immunolog 17, 219–232. doi: 10.1038/nri.2017.7
Liao, H., Zhang, Y., Guo, W., Wang, X., Wang, H., Ye, H., et al. (2021). Characterization of the blood and cerebrospinal fluid microbiome in children with bacterial meningitis and its potential correlation with inflammation. mSystems 6, e0004921. doi: 10.1128/mSystems.00049-21
Li, Q., Wang, C., Tang, C., Zhao, X., He, Q., Li, J. (2018). Identification and characterization of blood and neutrophil-associated microbiomes in patients with severe acute pancreatitis using next-generation sequencing. Front. Cell. Infection Microbiol. 8. doi: 10.3389/fcimb.2018.00005
Lu, T., Zou, Y. (2020). Progress on the relationship between intestinal flora and asthma in children. Int. J. Pediatr. 6, 389–393. doi: 10.1051/e3sconf/20197801008
Mandal, R. K., Jiang, T., Al-Rubaye, A. A., Rhoads, D. D., Wideman, R. F., Zhao, J., et al. (2016). An investigation into blood microbiota and its potential association with bacterial chondronecrosis with osteomyelitis (BCO) in broilers. Sci. Rep. 6, 25882. doi: 10.1038/srep25882
Markova, N. D. (2017). L-form bacteria cohabitants in human blood: significance for health and diseases. Discovery Med. 23, 305–313.
Martel, J., Wu, C.-Y., Huang, P.-R., Cheng, W.-Y., Young, J. D. (2017). Pleomorphic bacteria-like structures in human blood represent non-living membrane vesicles and protein particles. Sci. Rep. 7, 10650. doi: 10.1038/s41598-017-10479-8
Mason, M. R., Nagaraja, H. N., Camerlengo, T., Joshi, V., Kumar, P. S. (2013). Deep sequencing identifies ethnicity-specific bacterial signatures in the oral microbiome. PloS One 8, e77287. doi: 10.1371/journal.pone.0077287
Mclaughlin, R. W., Vali, H., Lau, P. C. K., Palfree, R. G. E., De Ciccio, A., Sirois, M., et al. (2002). Are there naturally occurring pleomorphic bacteria in the blood of healthy humans? J. Clin. Microbiol. 40, 4771–4775. doi: 10.1128/JCM.40.12.4771-4775.2002
Mitchell, A. J., Gray, W. D., Schroeder, M., Yi, H., Taylor, J. V., Dillard, R. S., et al. (2016). Pleomorphic structures in human blood are red blood cell-derived microparticles, not bacteria. PloS One 11, e0163582. doi: 10.1371/journal.pone.0163582
Moriyama, K., Ando, C., Tashiro, K., Kuhara, S., Okamura, S., Nakano, S., et al. (2008). Polymerase chain reaction detection of bacterial 16S rRNA gene in human blood. Microbiol. Immunol. 52, 375–382. doi: 10.1111/j.1348-0421.2008.00048.x
Muñoz, P., Cruz, A. F., Rodríguez-Créixems, M., Bouza, E. (2008). Gram-negative bloodstream infections. Int. J. antimicrobial Agents 32, S10–S14. doi: 10.1016/j.ijantimicag.2008.06.015
Niess, J. H., Brand, S., Gu, X., Landsman, L., Jung, S., Mccormick, B. A., et al. (2005). CX3CR1-mediated dendritic cell access to the intestinal lumen and bacterial clearance. Science 307, 254–258. doi: 10.1126/science.1102901
Nikkari, S., Mclaughlin, I. J., Bi, W., Dodge, D. E., Relman, D. A. (2001). Does blood of healthy subjects contain bacterial ribosomal DNA? J. Clin. Microbiol. 39, 1956–1959. doi: 10.1128/JCM.39.5.1956-1959.2001
Olde Loohuis, L. M., Mangul, S., Ori, A. P. S., Jospin, G., Koslicki, D., Yang, H. T., et al. (2018). Transcriptome analysis in whole blood reveals increased microbial diversity in schizophrenia. Trans. Psychiatry 8, 96. doi: 10.1038/s41398-018-0107-9
Païssé, S., Valle, C., Servant, F., Courtney, M., Burcelin, R., Amar, J., et al. (2016). Comprehensive description of blood microbiome from healthy donors assessed by 16S targeted metagenomic sequencing. Transfusion 56, 1138–1147. doi: 10.1111/trf.13477
Panaiotov, S., Filevski, G., Equestre, M., Nikolova, E., Kalfin, R. (2018). Cultural isolation and characteristics of the blood microbiome of healthy individuals. Adv. Microbiol. 8, 406–421. doi: 10.4236/aim.2018.85027
Peng, Y., He, Y., Wang, L., Wang, B. (2021). Changes of intestinal flora and serum amino acid levels in elderly patients with parkinson’s disease at different stages. Chin. J. Geriatrics 12 (12), 153–157. doi: 10.3760/cma.j.issn.0254-9026.2021.02.004
Petersen, C., Round, J. L. (2014). Defining dysbiosis and its influence on host immunity and disease. Cell. Microbiol. 16, 1024–1033. doi: 10.1111/cmi.12308
Potgieter, M., Bester, J., Kell, D. B., Pretorius, E. (2015). The dormant blood microbiome in chronic, inflammatory diseases. FEMS Microbiol. Rev. 39, 567–591. doi: 10.1093/femsre/fuv013
Pretorius, E., Mbotwe, S., Bester, J., Robinson, C. J., Kell, D. B. (2016). Acute induction of anomalous and amyloidogenic blood clotting by molecular amplification of highly substoichiometric levels of bacterial lipopolysaccharide. J. R. Soc. Interface 13, 20160539. doi: 10.1098/rsif.2016.0539
Qin, J., Li, R., Raes, J., Arumugam, M., Burgdorf, K. S., Manichanh, L., et al. (2020). A human gut microbial gene catalogue established by metagenomic sequencing. Nature 464, 59–65. doi: 10.1038/nature13568
Qin, N., Yang, F., Li, A., Prifti, E., Chen, Y., Shao, L., et al. (2014). Alterations of the human gut microbiome in liver cirrhosis. Nature 513, 59–64. doi: 10.1038/nature13568
Qiu, J., Zhou, H., Jing, Y., Dong, C. (2019). Association between blood microbiome and type 2 diabetes mellitus: A nested case-control study. J. Clin. Lab. Anal. 33, e22842. doi: 10.1002/jcla.22842
Rajendhran, J., Shankar, M., Dinakaran, V., Rathinavel, A., Gunasekaran, P. (2013). Contrasting circulating microbiome in cardiovascular disease patients and healthy individuals. Int. J. Cardiol. 168, 5118–5120. doi: 10.1016/j.ijcard.2013.07.232
Romano-Keeler, J., Weitkamp, J.-H. (2015). Maternal influences on fetal microbial colonization and immune development. Pediatr. Res. 77, 189–195. doi: 10.1038/pr.2014.163
Romero, R., Hassan, S. S., Gajer, P., Tarca, A. L., Fadrosh, D. W., Nikita, L., et al. (2014). The composition and stability of the vaginal microbiota of normal pregnant women is different from that of non-pregnant women. Microbiome 2, 1–19. doi: 10.1186/2049-2618-2-4
Sato, J., Kanazawa, A., Ikeda, F., Yoshihara, T., Goto, H., Abe, H., et al. (2014). Gut dysbiosis and detection of “live gut bacteria” in blood of Japanese patients with type 2 diabetes. Diabetes Care 37, 2343–2350. doi: 10.2337/dc13-2817
Sender, R., Fuchs, S., Milo, R. (2016). Revised estimates for the number of human and bacteria cells in the body. PLOS Biology 14, e1002533.
Scarsella, E., Sandri, M., Monego, S. D., Licastro, D., Stefanon, B. (2020). Blood microbiome: A new marker of gut microbial population in dogs? Veterinary Sci. 7, 198. doi: 10.3390/vetsci7040198
Schierwagen, R., Alvarez-Silva, C., Madsen, M. S. A., Kolbe, C. C., Meyer, C., Thomas, D., et al. (2019). Circulating microbiome in blood of different circulatory compartments. Gut 68, 578–580. doi: 10.1136/gutjnl-2018-316227
Schierwagen, R., Alvarez-Silva, C., Servant, F., Trebicka, J., Lelouvier, B., Arumugam, M. (2020). Trust is good, control is better: technical considerations in blood microbiome analysis. Gut 69, 1362–1363. doi: 10.1136/gutjnl-2019-319123
Segata, N., Haake, S. K., Mannon, P., Lemon, K. P., Waldron, L., Gevers, D., et al. (2012). Composition of the adult digestive tract bacterial microbiome based on seven mouth surfaces, tonsils, throat and stool samples. Genome Biol. 13, 1–18. doi: 10.1186/gb-2012-13-6-r42
Shabbir, U., Arshad, M. S., Sameen, A., Oh, D.-H. (2021). Crosstalk between gut and brain in alzheimer’s disease: the role of gut microbiota modulation strategies. Nutrients 13, 690. doi: 10.3390/nu13020690
Shah, N. B., Allegretti, A. S., Nigwekar, S. U., Kalim, S., Zhao, S., Lelouvier, B., et al. (2019). Blood microbiome profile in CKD: a pilot study. Clin. J. Am. Soc. Nephrol. 14, 692–701. doi: 10.2215/CJN.12161018
Tedeschi, G. G., Amici, D., Paparelli, M. (1969). Incorporation of nucleosides and amino-acids in human erythrocyte suspensions: Possible relation with a diffuse infection of mycoplasms or bacteria in the l form. Nature 222, 1285–1286. doi: 10.1038/2221285a0
Traykova, D., Schneider, B., Chojkier, M., Buck, M. (2017). Blood microbiome quantity and the hyperdynamic circulation in decompensated cirrhotic patients. PloS One 12, e0169310. doi: 10.1371/journal.pone.0169310
Varani, S., Stanzani, M., Paolucci, M., Melchionda, F., Castellani, G., Nardi, L., et al. (2009). Diagnosis of bloodstream infections in immunocompromised patients by real-time PCR. J. Infection 58, 346–351. doi: 10.1016/j.jinf.2009.03.001
Velmurugan, G., Dinakaran, V., Rajendhran, J., Swaminathan, K. (2020). Blood microbiota and circulating microbial metabolites in diabetes and cardiovascular disease. Trends Endocrinol. Metab. 31, 835–847. doi: 10.1016/j.tem.2020.01.013
Veziant, J., Villéger, R., Barnich, N., Bonnet, M. (2021). Gut microbiota as potential biomarker and/or therapeutic target to improve the management of cancer: Focus on colibactin-producing escherichia coli in colorectal cancer. Cancers 13, 2215. doi: 10.3390/cancers13092215
Vientós-Plotts, A. I., Ericsson, A. C., Rindt, H., Grobman, M. E., Graham, A., Bishop, K., et al. (2017). Dynamic changes of the respiratory microbiota and its relationship to fecal and blood microbiota in healthy young cats. PloS One 12, e0173818. doi: 10.1371/journal.pone.0173818
Whittle, E., Leonard, M. O., Harrison, R., Gant, T. W., Tonge, D. P. (2019). Multi-method characterization of the human circulating microbiome. Front. Microbiol. 9. doi: 10.3389/fmicb.2018.03266
Wiest, R., Lawson, M., Geuking, M. (2014). Pathological bacterial translocation in liver cirrhosis. J. Hepatol. 60, 197–209. doi: 10.1016/j.jhep.2013.07.044
Xie, D., Zhao, X., Chen, M. (2021). Prevention and treatment strategies for type 2 diabetes based on regulating intestinal flora. BioScience Trends 15 (5), 313–320. doi: 10.5582/bst.2021.01275
Yoo, J. Y., Groer, M., Dutra, S. V. O., Sarkar, A., Mcskimming, D. I. (2020). Gut microbiota and immune system interactions. Microorganisms 8, 1587. doi: 10.3390/microorganisms8101587
Yun, Y., Kim, H. N., Lee, E. J., Ryu, S., Chang, Y., Shin, H., et al. (2019). Fecal and blood microbiota profiles and presence of nonalcoholic fatty liver disease in obese versus lean subjects. PloS One 14, e0213692. doi: 10.1371/journal.pone.0213692
Zhang, W., Zhang, X., Zhang, Y., Wu, H., Liu, Q., Zhou, X., et al. (2021). Analysis of changes of intestinal flora in elderly patients with alzheimer’s disease and liver cancer and its correlation with abnormal gastrointestinal motility. J. Oncol 2021. doi: 10.1155/2021/7517379
Zhou, Y., Gao, H., Mihindukulasuriya, K. A., La Rosa, P. S., Wylie, K. M., Vishnivetskaya, T., et al. (2013). Biogeography of the ecosystems of the healthy human body. Genome Biol. 14, 1–18. doi: 10.1186/gb-2013-14-1-r1
Zhou, X., Li, J., Guo, J., Geng, B., Ji, W., Zhao, Q., et al. (2018). Gut-dependent microbial translocation induces inflammation and cardiovascular events after ST-elevation myocardial infarction. Microbiome 6, 66. doi: 10.1186/s40168-018-0441-4
Keywords: blood microbiota, transient bacteremia, blood bacteria and dysbiosis, 16S rDNA, diabetes, cardiovascular diseases
Citation: Goraya MU, Li R, Mannan A, Gu L, Deng H and Wang G (2022) Human circulating bacteria and dysbiosis in non-infectious diseases. Front. Cell. Infect. Microbiol. 12:932702. doi: 10.3389/fcimb.2022.932702
Received: 30 April 2022; Accepted: 01 August 2022;
Published: 24 August 2022.
Edited by:
Yijie Dong, Chinese Academy of Agricultural Sciences (CAAS), ChinaReviewed by:
Md. Ashik Ullah, QIMR Berghofer Medical Research Institute, The University of Queensland, AustraliaKristina Marie Feye, National Center for Toxicological Research (FDA), United States
Copyright © 2022 Goraya, Li, Mannan, Gu, Deng and Wang. This is an open-access article distributed under the terms of the Creative Commons Attribution License (CC BY). The use, distribution or reproduction in other forums is permitted, provided the original author(s) and the copyright owner(s) are credited and that the original publication in this journal is cited, in accordance with accepted academic practice. No use, distribution or reproduction is permitted which does not comply with these terms.
*Correspondence: Gefei Wang, Z2VmZWl3YW5nQHN0dS5lZHUuY24=