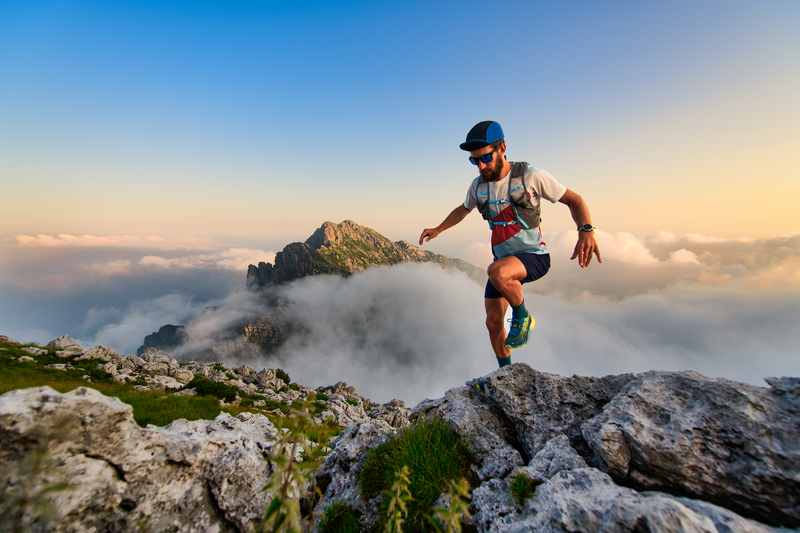
95% of researchers rate our articles as excellent or good
Learn more about the work of our research integrity team to safeguard the quality of each article we publish.
Find out more
REVIEW article
Front. Cell. Infect. Microbiol. , 21 June 2022
Sec. Biofilms
Volume 12 - 2022 | https://doi.org/10.3389/fcimb.2022.926363
This article is part of the Research Topic Novel approaches in the prevention of bacterial biofilm formation View all 8 articles
The biofilm community of microorganisms has been identified as the dominant mode of microbial growth in nature and a common characteristic of different microorganisms such as Pseudomonas aeruginosa, Staphylococcus aureus, and Staphylococcus epidermidis. The biofilm structure helps in the protection from environmental threats including host immune system and antimicrobial agents. Thus, the biofilm community has led to a higher prevalence of multidrug-resistant (MDR) strains in recent years. In this regard, the use of a new class of antibiotics, natural compounds, and anti-biofilm enzymes has been considered for the destruction of the microbial biofilm. However, different drawbacks such as low penetration, high susceptibility to degradation, instability, and poor solubility in aqueous solutions limit the use of anti-biofilm agents (ABAs) in a clinical setting. As such, recent studies have been using poly lactic-co-glycolic acid (PLGA)-based nanoplatforms (PLGA NPFs) for delivery of ABAs that have reported promising results. These particles, due to proper drug loading and release kinetics, could suppress microbial attachment, colonization, and biofilm formation for a long time. Additionally, PLGA NPFs, because of the high drug-loading efficiencies, hydrophilic surface, negative charge, and electrostatic interaction, lead to effective penetration of antibiotics to the deeper layer of the biofilm, thereby eliminating the microbial biofilm. Thus, PLGA NPFs could be considered as a potential candidate for coating catheters and other medical material surfaces for inhibition and destruction of the microbial biofilm. However, the exact interaction of PLGA NPFs and the microbial biofilm should be evaluated in animal studies. Additionally, a future goal will be to develop PLGA formulations as systems that can be used for the treatment of the MDR microbial biofilm, since the exact interactions of PLGA NPFs and these biofilm structures are not elucidated. In the present review article, we have discussed various aspects of PLGA usage for inhibition and destruction of the microbial biofilm along with different methods and procedures that have been used for improving PLGA NPF efficacy against the microbial biofilm.
Antibiotic resistance and biofilm formation by pathogenic microorganism have become major global health and economic problems for which many researchers worldwide are looking for new and more effective approaches. Biofilms are a community of microbial cells embedded in an extracellular matrix containing exopolysaccharides (EPSs), proteins, DNA, antibiotic-inactivating enzymes, and effluent pumps (Iannitelli et al., 2011b). Many diseases, including chronic rhinosinusitis and endocarditis, are examples associated with the growth of bacterial biofilms (Sanderson et al., 2006; Iannitelli et al., 2011b). Biofilm formation increases the resistance of a microorganism to many antimicrobial agents, with the biofilm-forming bacteria being several times (100–1,000-fold) resistant to antibacterial compounds, immune system, and environmental stresses than their planktonic counterparts. Higher biofilm communities resistant to various antimicrobial agents could be related to their limited penetration to the deeper layers of the biofilm, slowing the growth of bacteria and the molecular interactions between them (Stewart and Costerton, 2001; Kang et al., 2019). Many microorganisms have been shown to have the ability to form biofilms, specifically, Staphylococcus aureus, Staphylococcus epidermidis, and Pseudomonas aeruginosa are considered bacterial pathogens with the ability to form biofilm communities on various environmental and body surfaces (Deepika et al., 2019; Francisconi et al., 2020).
To combat the bacterial biofilm, various methods such as inhibiting the biofilm formation and improving the penetration of antimicrobial agents into the extracellular matrix have been proposed (Guo et al., 2021). Hence, new anti-biofilm agents including bacteriophages, natural compounds, biomolecules that target the biofilm structure, and various nanoparticles (NPs) have been used to destroy the biofilm and prevent its formation by inhibiting bacterial adhesion (Moghadam et al., 2021).
Studies have shown that the integration of nanotechnology and pharmaceutical sciences greatly helps improve the treatment of biofilm-related infections (Iannitelli et al., 2011b). Drug delivery platforms, photothermal therapy, and photodynamic therapy that are nanotechnology-based strategies have shown great success in the treatment of biofilm infections (Xiu et al., 2021). Various drug delivery systems, such as biodegradable NPs, have proven their superior ability to release drug shipments in a controlled and stable manner (Kamaly et al., 2016). Additionally, nanocarriers have several advantages, including the ability to administer therapeutic compounds directly, the ability to adapt multiple drugs, and the effective delivery of drug-related synergistic effects (Deepika et al., 2019). Furthermore, drug carriers can enhance the stability and release of the compound over time and boost its effectiveness (Wolfmeier et al., 2018).
Biocompatible polymers such as polyethylene glycol (PEG) and poly (lactide co-glycolide) (PLGA) are good carriers in drug delivery. Due to its biocompatibility and biodegradability, PLGA is commonly used in the development of drug delivery platforms (Xiu et al., 2021). In this regard, various drug delivery platforms are made of PLGA. According to the results of various studies, it has been determined that PLGA nanoplatforms (NPFs) can deliver and disperse therapeutic agents in a controlled and stable way (Mundargi et al., 2008; Han et al., 2017). In general, PLGA NPFs have shown sustained release in vitro by providing a robust drug delivery system for most encapsulated antibiotics and natural compounds and in many studies have had high biofilm penetration (Han et al., 2017).
Given the importance and applicability of PLGA NPFs for inhibiting and destroying the microbial biofilm in recent studies, in the present review article, we comprehensively investigated the effect of PLGA on the microbial biofilm to promote its wider usage in clinical practice.
NPs are solid particles with a spherical shape ranging in size from 10 to 1,000 nm and are manufactured from natural or synthetic polymers (Ding and Zhu, 2018). A wide range of drugs can be delivered through NPs such as small hydrophilic and hydrophobic drugs, vaccines, and biological macromolecules. These particles also allow targeted administration to specific organs or cells or controlled drug delivery. Recent developments in treatment have increased the demand for a new intelligent drug delivery design with more advanced power, efficiency, and properties to ensure the effectiveness of treatment with sufficient availability and ability of encapsulation (Hosseini et al., 2022).
One of the most important challenges in pharmacology is controlled drug delivery to the human body (Patra et al., 2018). Over recent decades, nanotechnology has arrived in many fields and actuated the whole world to phenomenal developments. Meanwhile, the pharmaceutical industry has not been an exception, and new nanopharmaceutical products have entered different markets (Wang et al., 2021; Mohamed et al., 2022). In the past two decades, the pattern of using nanocarriers in the human body to enhance the efficacy and effectiveness of many drugs, especially anticancer drugs, has been well confirmed in both pharmaceutical and clinical experiments. Nowadays, there are various NP-based systems for targeting drug delivery that is either well-developed or underdeveloped. The primary purpose of employing nanocarriers is to reduce drug breakdown, prevent their side effects, increase drug availability, and drug accumulation at the site of the lesion (Nasrollahzadeh et al., 2019; Said et al., 2022).
In the common methods of taking drugs, whether oral, injectable, or others, the medication is distributed throughout the body, and the entire body is affected by the effects of the drugs as well as their side effects (Hosseini et al., 2019a). Here, large amounts of medication are required to achieve a specific effect (Singh et al., 2019). So far, many researchers have been addressing this problem, and with the arrival of nanotechnology into the field of pharmaceutical science, the use of this technology in achieving targeted drug delivery has also been considered by many researchers (Hosseini et al., 2019b; Alphandéry, 2020). The use of drug delivery has its advantages over routine medications that can be referred to as follows: preventing the destruction of drugs, increasing the storage time of drugs, storing high amounts of drugs, and having high biocompatibility (Barclay et al., 2019). One of the most important features that makes this technology unique is targeting drug delivery to the specific cell or tissue of the body in cases such as the need to apply low-solubility or insoluble drugs, low-absorption drugs, and gastrointestinal or chemically unstable drugs. Generally, drug nanocarriers can be classified into three main groups: vesicular, particulate, and micelle (Soussan et al., 2009; Banerjee and Pillai, 2019). Each of these groups can be subdivided into smaller subcategories (Figure 1).
PLGA is a co-polymer with Food and Drug Administration (FDA) approval (Duskey et al., 2020). These particles are generally synthesized through ring-opening copolymerization of glycolic acid (GA) cyclic dimers and lactic acid (LA) in the presence of catalysts such as tin (II) 2-ethylhexanoate or tin (II) alkoxides, as well as aluminum isopropoxidem. Furthermore, different solvents such as acetone, chlorinated solvents, tetrahydrofuran, and ethyl acetate could be used for PLGA copolymers. To this end, various PLGA copolymers are synthesized using diverse GA : LA ratios where the PLGA crystallinity could range from fully crystalline to fully amorphous depending on the molar ratio and block structure (Gentile et al., 2014; Zare et al., 2020).
Parameters such as the degree of crystallinity, molecular weight porosity of the polymeric network, and LA : GA ratio could affect the biodegradation characteristics of PLGA copolymers. In this regard, the degradation rate rises upon enhancing the GA units in the main chain structure; however, there is an exception, the 50:50 LA : GA ratio, which has the fastest rate of degradation. Furthermore, a higher content of the polyglycolic acid (below 50%) leads to improved degradation (Swider et al., 2018; Zare et al., 2020). Indeed, a higher monomer unit number leads to a shortened degradation time, while a lower glycol content prolongs degradation. Due to the mentioned characteristics, it is possible to design and fabricate PLGA-based NPs with a time-controlled and programmable drug release (Lagreca et al., 2020).
In this regard, fine-tuning the degradation and release rate is gaining increasing attention as researchers keep pushing the boundaries of novel delivery carriers. Notably, different approaches and procedures have been used such as physical blending as well as surface and chemical modification and have been found to be an effective means of release modulation in delivery systems such as oral, parenteral, and tissue- and topical engineering scaffolds (Singh and Singha, 2021).
PLGA is widely employed in the design and formulation of drug delivery systems for biomedical applications due to its biocompatibility, biodegradability, and adaptability in formulation and function. PLGA NPs are used extensively in various applications, including the treatment of neurological/brain disorders and cancer, anti-inflammation, treatment of infectious diseases, and cardiovascular therapy (Won et al., 2021). It can also be encapsulated in a wide range of biologically active molecules including various drugs, proteins, vaccines, and nucleic acids (Ding and Zhu, 2018). Since these nanocarriers can be formulated for systemic injection, oral use, and inhalation, any method of administration can be chosen. While the effectiveness of PLGA NPFs in drug delivery applications has been well confirmed and reported in many research studies, this development makes these micro/nanoformulations undergo clinical trial or currently in use in the pharmaceutical markets, which also has FDA approval (Allavena et al., 2020; Shen et al., 2020; Cunha et al., 2021). Today, of the total number of nano-available pharmaceutical products in the market, PLGA NPFs have received massive consideration due to their strong therapeutic delivery systems, applicability in the treatment of various disorders such as cancer and infectious disease, and commercial impact on micro/nano formed products that have been approved by the FDA. Annual revenues from the sale of these pharmaceutical products are estimated to exceed a total of US $100 billion (Anselmo and Mitragotri, 2014; Zhong et al., 2018; Molavi et al., 2020).
PLGA NPFs can enter cells through endocytosis mediated by clathrin and partly liquid phase pinocytosis. They are rapidly eliminated from the endolysosomes and enter the cytoplasm after 10 min of incubation (Durán-Lobato et al., 2022). In addition to the attractive properties of PLGA NPFs such as small size, high structural integrity, stability, adaptability, and adjustable surface, their physical and chemical properties can be altered. These mentioned properties are obtained from molecular weight, distribution of molecular weight, and composition (GA : LA ratios); as a result, controlling the size, shape, and biodegradability of the prepared nanocarriers is possible, which in turn affects the pharmacokinetics of the encapsulated drug (Rizzo and Kehr, 2021; Rocha et al., 2022). On the other hand, some limitations include their weak drug loading, especially for lipophilic drugs, high explosive release, phagocytic absorption, short half-life, immune response, and uncontrolled distribution, making these drugs not always reliable as a multifaceted and undeniable agent in biomedicine (Kalhapure et al., 2015).
One of the most important biological barriers against controlled drug delivery based on NPs is when the body perceives and recognizes hydrophobic particles as foreign objects. As a result, the reticuloendothelial system (RES) removes these particles from the bloodstream and leads them to the liver or spleen. Additionally, serum opsonin proteins bind to injected NPs and lead them to phagocytosis by attaching to macrophages (Sah et al., 2013; Rocha et al., 2022). To solve this, a hydrophilic layer coats the surface of NPs to cover hydrophobicity. Another advantage of surface modification is to target tumors or organs to enhance selective cell attachment and internalization through receptor-mediated endocytosis. Target ligands are often bonded at the NP surface by bonding to PEG chains; PEG is a hydrophilic and non-ionic polymer that is most common for surface modification. The ligands must be optimally conjugated to the NPs to maintain their tendency to bind to the receptors. Since adequate PEG coating is necessary to prevent detection by RES, ligands must be removed from NP surfaces to avoid protection by PEG chains (Danhier et al., 2012; Ding and Zhu, 2018) (Figure 2).
Figure 2 Representation of several methods of surface modifications of PLGA, (A) nanosphere and (B) PEGylated PLGA. Polyethylene glycol is a non-ionic and hydrophilic polymer; binding to the PLGA surface increases its half-life in the blood circulation. (C) Targeted PLGA, increased cell binding and selective internalization through receptor-mediated endocytosis. Target ligands often bind to PEG chains at the nanoparticle surface. The ligands must be optimally conjugated to the nanoparticles to maintain their combined affinity for receptor binding. (D) Theragnostics PLGA, load the drug with a fluorescent traceable substance that can detect PLGA. PEG, Polyethylene glycol; PLGA, poly (lactide co-glycolide).
To prepare PLGA NPFs, there are several methods that vary depending on the purpose of the preparation and structure. The drug is either loaded inside the nucleus of a “nanocapsule” or trapped at the surface of the “nanosphere” matrix. In this part, we will review various methods that have been used for the preparation of PLGA NPFs.
The most prevalent method used to prepare PLGA NPFs is the solvent-emulsion evaporation method that prepares the encapsulation of hydrophobic and hydrophilic drugs. This process involves dissolving a polymer in an organic solvent (such as dichloromethane). The oil emulsion in water (O/W) is prepared by adding water and a surfactant (such as polysorbate-80, poloxamer-188) to the polymer solution. Next, NP droplets are induced by homogenization or sonication. The solvent is then evaporated or extracted, and after centrifugation, the NPs are collected. A modification of this method, W/O/W double-emulsion, was used to enclose hydrophilic drugs, such as peptides, proteins, and nucleic acids. Emulsion evaporation methods are advantageous, as they allow for relative control of particle size and rate of release. The W/O/W method is generally appropriate to encapsulate water-soluble drugs such as peptides, proteins, and vaccines, while the O/W method is suitable for water-insoluble drugs such as steroids (Jain, 2000; Luo et al., 2009). Nevertheless, these mentioned methods are also associated with inherent flaws and problems, especially bio-macromolecule instability. NP size can be controlled by adjusting the stirring speed, type, and amount of dispersing agent, organic and aqueous phase viscosity, and temperature (Zhang et al., 2021) (Figure 3).
Figure 3 Representation of two preparation techniques of PLGA particles. (A) Single-emulsion solvent evaporation. (B) Encapsulation of a drug into PLGA nanoparticles using double-emulsion solvent evaporation.
Spray-drying is a quick and comfortable method to prepare PLGA in smooth conditions with very slow processing parameters, thus making it usable in industrial scaling. In this process, drug-loaded microspheres are sprayed with a solid dispersion in oil or an emulsion of water in oil in a stream of heated air. The type of drug (hydrophilic or hydrophobic) determines the appropriate solvent for the process. The nature of the solvent used, the solvent evaporation temperature, and the feed rate affect the morphology of the final product. This is the most common method to encapsulate drugs and proteins in microparticles without significant loss of biological activity. However, two main drawbacks of this method are microparticle attachments to interior walls of the spray-dryer and difficulty to control particle size, while the yield is average for small batches (Guo et al., 2014; Wan et al., 2014). There are different spray-drying techniques to prepare micro-PLGA particles for proteins, peptides, and DNA delivery (Takada et al., 1995) (Figure 4).
It is a single-phase process known as the solvent transfer method and performed using systems containing three basic components: polymer, polymer-solvent, and non-solvent polymer. This technique is usually used to trap hydrophobic and hydrophobic drugs but is also applicable for hydrophilic drugs. Polymer and drugs dissolve in a polar solvent and are water-miscible such as acetone, acetonitrile, ethanol, or methanol. The solution is poured in a controlled manner (add dropwise) in an aqueous solution with surfactant. NPs are formed immediately with the rapid release of the solvent. Finally, the solvent is removed under reduced pressure (Takada et al., 1995; Astete and Sabliov, 2006). There are also other techniques to synthesize PLGA including the emulsification reverse salting-out method (Naskar et al., 2021), phase separation (Mckenna et al., 2019), simple mechanical stirring (Operti et al., 2021), high shear mixing (HSM), and high-pressure homogenization (HPH) (Operti et al., 2018).
P. aeruginosa is known as one of the most important opportunistic bacterial pathogens found in many environments and leads to a high rate of deadly hospital-acquired infections (Bahramian et al., 2019). The results of recent investigations have shown a high mortality rate for P. aeruginosa-associated infections mainly in patients with predisposing conditions such as cancer, cystic fibrosis, urinary tract infection, and burn wound infections (Bahramian et al., 2019; Glen and Lamont, 2021). Different adhesion factors such as flagella, type IV pili, and biofilms cause adhesion and survivor of this bacterium on various surfaces, medical devices, and water (Remold et al., 2011).
It is now almost accepted that most bacterial species could form a biofilm in a cyclic process, although the first biofilm developmental life cycle has been reported for P. aeruginosa (Park and Sauer, 2021). The biofilm community of this bacterium could lead to chronic infections such as chronic pneumonia in patients with cystic fibrosis because of the higher resistance to environmental challenges such as diverse disinfectants, irradiation treatments, antibacterial agents, and phagocytosis by neutrophils. Additionally, biofilms can contain dormant antibiotic-insensitive persister cells (Glen and Lamont, 2021; Reynolds and Kollef, 2021).
Recent studies have reported that a higher dosage of antibiotics is needed for the destruction of the P. aeruginosa biofilm community compared to the planktonic cells due to the limited antibiotic penetration into the complex polysaccharide matrix (glycocalyx) of biofilms (Spoering and Lewis, 2001; Ma et al., 2009). Thus, the biofilm is implicated in the increasing prevalence of multidrug resistant (MDR) P. aeruginosa strains in recent years, with scientists searching hard for new anti-biofilm agents to control it. In this regard, the delivery of various antibacterial agents such as antibiotics, natural compounds, and enzymes by PLGA platform has also been considered for eliminating P. aeruginosa biofilm, and in this section, we will review these studies.
Hua et al., 2014 successfully encapsulated ciprofloxacin (CIP) in PLGA magnetic micro/nanoparticles using W/O/W method. Scanning electron microscopy (SEM) revealed spherical shapes and narrow size distributions for PLGA magnetic particle-encapsulated CIP. The total drug release was 95% and 80.3% from NPs and microparticles, respectively. Additionally, an external oscillating magnetic field triggered the release of CIP from PLGA magnetic particles that then continuously released for 2 weeks after triggered release. Notably, the sustained release of CIP from the PLGA microparticles reduced 20.4% of bacterial activity in the P. aeruginosa biofilm, while this rate was 25.8% for PLGA NPs. Thus, the encapsulation of CIP in PLGA NPs could lead to better antibacterial activity in comparison to the PLGA microparticles. Altogether, CIP maintained its antimicrobial activity after encapsulation and triggered release; thus, the authors suggested that the application of PLGA magnetic particles should be considered as facilitators of drug delivery and drug release switches in future studies (Hua et al., 2014).
In another investigation, NPs composed of a PLGA core and D-α-tocopheryl polyethylene glycol 1000 succinate (TPGS) were again used for the site-specific delivery of azithromycin to the P. aeruginosa biofilms via aerosol direction. The synthesized NPs were self-assembled via a nanoprecipitation process using a facile microfluidic method. The enzymatically cleavable shell of TPGS led to the acceptable penetration of TPGS-PLGA NPs to the mucus and deeper layers of the biofilm, thereby boosting the azithromycin killing efficacy against the biofilm community of P. aeruginosa. Notably, confocal laser scanning microscopy (CLSM) showed that PLGA NPs were fundamentally localized at the superficial layer of the biofilm, while TPGS-PLGA hybrid NPs penetrated to the deeper layer of the biofilm. Thus, it seemed that the hydrophilic moiety of TPGS successfully reduced the binding and interaction of the biofilm EPS and NPs, thereby causing enough penetration of the drug to the biofilm. In this regard, quantitative analysis revealed that azithromycin-loaded TPGS-PLGA hybrid NPs presented approximately 2.5 times greater bactericidal effects in comparison to the azithromycin alone at 6 h. These data suggested that the synthesized NPs could boost the azithromycin site-specific delivery and enhance the drug concentration in the P. aeruginosa biofilm. Hence, the authors introduced the TPGS-PLGA hybrid NPs as a suitable drug delivery system for releasing antibiotics in a sustained manner and for overcoming the various barriers in the treatment of microbial biofilm-associated infection (Wan et al., 2019).
These data support the findings by Sylvia et al., who used TPGS-PLGA hybrid NPs and octenyl succinic anhydride-modified low-molecular weight hyaluronic acid (OSA-HA) nanogels to enhance the efficacy of azithromycin for destroying the P. aeruginosa biofilm. The results indicated that both delivery systems could penetrate the bacterial biofilm and enhance the antivirulence and antibacterial activity of azithromycin. Furthermore, TPGS-PLGA hybrid NPs and OSA-HA nanogels prevented biofilm formation of P. aeruginosa and removed the established biofilm of this bacterium at considerably lower doses of azithromycin in comparison to the non-formulated form of this antibiotic. Note that smaller TPGS-PLGA hybrid NPs revealed longer retention in the biofilm in comparison to the hydrophilic OSA-HA nanogels (Kłodzińska et al., 2019).
In 2019, in another study, the authors used PLGA-methyl ether-block-PEG micelle to boost the performance of piperacillin/tazobactam against the biofilm of clinical isolates of P. aeruginosa with resistance to various antibiotics. Different analyses showed a semi-spherical morphology, a surface charge of 2.98 mV, and a mean diameter of less than 30 nm for piperacillin/tazobactam PLGA-PEG micelle. As compared to the free drug form, this micelle suppressed bacterial movement more effectively, eradicated biofilm communities, and lowered the minimum inhibitory concentration (MIC) (Morteza et al., 2019). In this regard, another examination for degrading the P. aeruginosa biofilm also encapsulated tobramycin on the PLGA-PEG with negative zeta potentials, spherical shape, and particle sizes of 896–902 nm (microparticles) and 225–231 nm (NPs). These NPs significantly enhanced the anti-biofilm activity under fluidic and static experimental conditions in artificial mucus. Interestingly, 0.77 mg/l of encapsulated tobramycin killed bacteria in the biofilm community, while 1,000 mg/l of mixtures of particles and tobramycin or free tobramycin were not capable enough to suppress the P. aeruginosa biofilm. Notably, tobramycin-PEG-PLGA NPs did not show a cytotoxic effect against human lung epithelial cells. Thus, this drug delivery platform could boost the efficacy of tobramycin against the bacterial biofilm, even aminoglycoside-resistant isolates (Ernst et al., 2018).
Amikacin and gentamycin were other members of the aminoglycoside family that have been loaded in PLGA NPs to eliminate the P. aeruginosa biofilm. Amikacin-PLGA NPs, with a zeta potential of 29.8 ± 1.5 mV and a mean diameter of 447 ± 7 nm, were distributed uniformly all along the biofilm thickness and penetrated through the entire biofilm thickness of P. aeruginosa. Additionally, CLSM analysis indicated that these NPs dramatically reduced the bacterial biofilm when compared to the free medication. The higher penetration of the amikacin-PLGA NPs than the free drug could be related to the NP negative charge and the resulting electrostatic interaction (Sabaeifard et al., 2017).
Additionally, researchers claimed that their synthesized PLGA particle-encapsulated gentamicin demonstrated regulated gentamicin release for up to 16 days. These particles significantly enhanced the anti-biofilm effect of gentamycin against P. aeruginosa. Notably, in this study, the authors used the 96-h peritoneal murine infection model for the in vivo assessment of synthesized particles. Compared to the free gentamycin, PLGA particle-encapsulated gentamicin effectively reduced bacterial count (from peritoneal lavage) and inflammatory cytokines. In this regard, the authors suggested that the controlled release of gentamicin NPs could have enhanced the efficacy over free gentamicin (Abdelghany et al., 2012).
Finally, Huang et al. (2020) reported that combining carbon quantum dots (CQDs) with the conventional PLGA NPs could reduce some drawbacks of these NPs such as premature burst release and incapability of enough drug loading as well as their on-demand release at the site of action. Hence, the authors used the microfluidic method and incorporated CQDs into the PLGA NPs (CQD-PLGA hybrid NPs). These NPs showed proper loading capability of tobramycin and azithromycin, stimulus-responsive release of the drugs upon laser irradiation, and good biocompatibility with the eukaryotic cells. Additionally, azithromycin-loaded CQD-PLGA hybrid NPs killed more bacterial cells in the P. aeruginosa biofilm community in comparison to the free azithromycin. This increased killing efficacy could be related to the delivery of antibiotics in the specific site of action by NPs, thereby elevating the drug concentration in deeper layers of the biofilm. These results suggested that CQD-PLGA hybrid NPs could be a promising approach for the inhibition of the bacterial biofilm due to the high antibiotic loading capacity and reduced premature burst release (Huang et al., 2020).
The use of the various natural compounds for inhibition of the microbial biofilm has been considered in recent years, and some promising results have also been reported. Nevertheless, poor solubility in aqueous solutions, instability, and volatility are some disadvantages of natural compounds limiting their major use in clinical settings. In this regard, researchers are interested in loading natural compounds on various drug delivery platforms such as PLGA. For example, Kim et al. (2015) reported that incorporation of PLGA with Cinnamomum bark oil and its main constituent, cinnamaldehyde, effectively inhibited the biofilm formation of P. aeruginosa and Escherichia coli. As a result, the scientists recommended that polymer coatings containing natural chemicals might be utilized to limit the formation of the bacterial biofilms on various surfaces such as food processing and biomedical surfaces (Kim et al., 2015). In a recently published study, authors used the emulsification solvent diffusion method and encapsulated Hydroethanolic Extract of Red Propolis (HERP) in PLGA for the destruction of the P. aeruginosa biofilm. These NPs were spherical with a size range of 42.4–69.2 nm, with encapsulation efficiencies of 96.99%. HERP-PLGA NPs showed a promising inhibitory effect against the bacterial biofilm. The authors suggested that the penetration of hydrophobic HERP through bacterial cells increased not only due to the small size of PLGA NPs but also because of their hydrophilic surface. The continuous release of HERP from PLGA NPs makes the HERP-PLGA NP a suitable alternative for local antibiotic therapy to inhibit the bacterial biofilm that reduces systemic side effects and patient complications (De Mélo Silva et al., 2020).
In line with the mentioned studies, another investigation also functionalized PLGA and dextran micelles with curcumin, one of the main phytochemical ingredients of Curcuma longa. The findings indicated that the synthesized NPs (hydrodynamic diameters 498.7 ± 35.4 nm) have a good penetration to the Pseudomonas species biofilm, leading to enhanced antibacterial activity of the conjugated micelles when compared to free curcumin. Additionally, curcumin micelles showed an antibacterial function toward biofilm-embedded cells and effectively disrupted the preformed biofilm. Thus, curcumin conjugation on the micelle structure could enhance curcumin solubility and its transport in the bacterial suspension, which in turn accentuated its antimicrobial capacity. Furthermore, the high biocompatibility of polysaccharides present within the biofilm EPS with dextran presence in the NPs could lead to greater incorporation of micelles into the biofilm architecture (Barros et al., 2021).
In a recently published study, the authors considered that by applying pyruvate dehydrogenase and eliminating pyruvate supply in the biofilm community, it is possible to trigger biofilm bacteria to disperse from the surface-associated mode of growth into the surrounding environment. Thus, it is conceivable that using pyruvate dehydrogenase, it is possible to revert biofilm bacteria to the planktonic mode of growth. However, in clinical settings, direct use of enzymes is not practical, as enzymes are susceptible to denaturation under different storage conditions. In this regard, the authors immobilized pyruvate dehydrogenase in PLGA and prepared NPs with an average size of 266.7 ± 1.8 nm. After 6 days of incubation at 37°C, these NPs remained active and successfully destroyed the P. aeruginosa mature biofilms by depleting pyruvate supply. These data suggested that pyruvate dehydrogenase-PLGA NPs lead to biofilm dispersion and could render bacteria susceptible to common antibiotics; accordingly, these NPs should be considered for the treatment of P. aeruginosa biofilm-associated infections (Han et al., 2019). Baelo et al. (2015) also functionalized CIP-loaded PLGA NPs with DNase I using a green solvent-based method. The authors assumed that the synthesized NPs can disrupt the bacterial biofilm in two distinct ways: releasing CIP in a controlled fashion and degrading the extracellular DNA that stabilizes the biofilm matrix. The results were in line with their theory as DNase I-PLGA NPs not only reduced 95% of the P. aeruginosa biofilm production but also eradicated more than 99.8% of the mature biofilm. The described anti-biofilm effect, on the other hand, was not seen for free drug or a combination of DNase I and free drug. Additionally, the synthesized NPs showed low cytotoxicity against 774 macrophages (Baelo et al., 2015).
As such, as mentioned in the above sections, different characteristics such as proper size, drug loading, drug release kinetics, and low cytotoxicity against eukaryotic cells make PLGA NPFs an applicable candidate for the destruction of P. aeruginosa biofilm-associated infections such as cystic fibrosis. This is because encapsulation of various anti-biofilm agents with PLGA could enhance inhaled cystic fibrosis therapy. However, the effectiveness of various PLGA formulations should be evaluated in inhaled antimicrobial therapy in in vivo models to completely explore the potential of these platforms.
MDR S. aureus, especially methicillin- and vancomycin-resistant strains, has led to huge financial and social burdens in clinical settings that have been increasing in recent years (Wu et al., 2021). In addition to the frequent occurrence of antimicrobial-resistant strains, S. aureus often resides within biofilms at the site of infection (Van Den Driessche et al., 2017). The ability of this bacterium to form a biofilm is the main virulence factor related to chronic infections such as the chronicity of wounds (Simonetti et al., 2021).
Additionally, the formation of biofilm by S. aureus on different medical surfaces, such as catheters, is an important problem in healthcare-associated infections (Ceylan and Ugur, 2015). Accordingly, researchers are trying to enhance the efficacy of antibiotics against the S. aureus biofilm; meanwhile, other possibilities to the existing antibiotics against S. aureus biofilm-associated infections are still of interest (Taubes, 2008). In this regard, recent studies have used PLGA NPFs for this purpose.
Ficai et al. (2018) hypothesized that magnetite NPs could enhance the therapeutic efficacy of single antibiotics (even at low drug concentrations) due to the specific nanosize-related properties. Additionally, these NPs could be considered active carriers of the antibiotic, as their eventual exposure to external magnetic fields could lead to the complete release of antibiotics and enhance their efficacy. Furthermore, the mentioned effects could be boosted by applying PLGA matrix, since by increasing local temperature due to the infection and inflammatory processes, the PLGA matrix will enable the local release of antibiotic-coated NPs, thus providing enhanced antibacterial activity (Grumezescu et al., 2014a; Ficai et al., 2018).
In this regard, the authors firstly functionalized iron oxide particles with cefepime (Fe3O4/CEF), and afterward, using the matrix-assisted pulsed laser evaporation method, they coated these particles with PLGA (PLGA-Fe3O4/CEF). Covering the glass slides with PLGA-Fe3O4/CEF significantly suppressed S. aureus biofilm formation for at least 72 h. Thus, this nanosystem releases the antibiotic continuously and in a controlled manner and enhances the potential of the antibiotic for the destruction of the bacterial biofilm. Accordingly, PLGA-Fe3O4/CEF could be considered a promising anti-biofilm coating for implantable devices (Ficai et al., 2018).
Note that various etiological factors such as bone necrosis and compressed vascular channels limit antibiotic penetration to the active site of infection, thus making osteomyelitis a persistent infection. As such, local antibiotic therapy could be a better therapeutic approach in comparison to the systemic administration of antibiotics. In this regard, recent studies due to the various features such as sustained drug release capabilities and acceptable biocompatibility have used PLGA-based drug delivery platforms for local delivery of antibiotics for the destruction of the bacterial biofilm and treatment of osteomyelitis (Bastari et al., 2014).
In one of these studies, mesoporous bioactive glass (MBG) was loaded with vancomycin, after which freeze-drying fabrication was used to prepare a bone tissue-engineering scaffold combining PLGA and MBG/vancomycin. Different analyses revealed that this compound could provide sustained release of antibiotics lasting for more than 8 weeks. In addition, coating the scaffold with vancomycin promoted antibacterial and anti-biofilm activity against S. aureus without detrimental activity on cytocompatibility. Indeed, the PLGA scaffold with vancomycin-loaded MBG yielded an initial fast release of drug (beneficial for the acute phase of infections) followed by a relatively slow release, which successfully suppressed the adhesion and biofilm formation of S. aureus. Hence, the authors suggested this scaffold as a suitable candidate for the treatment of S. aureus-associated osteomyelitis due to its anti-biofilm properties (Cheng et al., 2018).
In another investigation, W/O/W double-emulsion solvent evaporation technique was again used for preparing moxifloxacin-PLGA and rifampicin/moxifloxacin-PLGA microspheres for local delivery treatment of S. aureus-associated osteomyelitis. Notably, both of these antibiotics were used for the treatment of osteomyelitis. The results indicated that compared to the moxifloxacin-PLGA microsphere, rifampicin/moxifloxacin-PLGA microspheres showed better loading rate, stability, and slow drug release as well as anti-biofilm effects. Collectively, rifampicin/moxifloxacin-PLGA microspheres have a potential for the treatment of osteomyelitis because of their different characteristics such as local drug delivery, suppression of biofilm formation, and biodegradability (Qiao et al., 2019).
In another drug delivery platform for inhibition of S. aureus biofilm-associated osteomyelitis, Bastari et al. (2014) loaded levofloxacin into PLGA particles coated with calcium phosphate (CaP). The CaP coating lowered burst release, however, providing continuous release of the antibiotics for up to 4 weeks. Furthermore, the synthesized particles suppressed S. aureus biofilm formation and disrupted the established biofilm community of this bacterium. Thus, inhibition of the biofilm formation and destruction of the mature biofilm by levofloxacin-PLGA/CaP particles make these particles a novel therapeutic agent for the prevention of osteomyelitis recurrence after surgery and treatment of this infection mainly when it has reached the stage of attachment and maturation of the biofilm on the bone surface (Bastari et al., 2014).
In addition, Ashbaugh et al. (2016) used the mouse model of methicillin-resistant S. aureus (MRSA) biofilm-associated orthopedic implant infection for evaluating PLGA-based drug delivery platform efficacy in the treatment of osteomyelitis. To this end, PLGA nanofibers embedded in a poly (Ɛ-caprolactone) (PCL) film were developed to locally codeliver vancomycin/rifampicin (VAN/RIF) from the implant surface. Based on the animal model results, antibiotic-loaded coatings effectively prevented bone/joint tissue infection and MRSA biofilm formation on the implant; additionally, they were biocompatible with enhanced osseointegration. These data suggested that these antibiotic-loaded coatings should be considered as an applicable tailor for the delivery of conventional antibiotics from different prostheses or metallic implantable devices to effectively reduce biofilm-associated osteomyelitis in patients (Ashbaugh et al., 2016).
Based on the mentioned studies, PLGA NPFs could be considered an applicable approach for the local delivery of various antibiotics for the treatment of osteomyelitis. Since the bacterial biofilm makes this infection more resistant to the treatment, antibiotic-PLGA platforms could be used for the inhibition and destruction of the biofilm community, thereby facilitating the treatment of osteomyelitis. However, the reported data have been collected from in vitro evaluations, and for assessing the exact function of antibiotic-PLGA platforms in the treatment of osteomyelitis, this compound should be tested in further animal studies and clinical trials (Figure 5).
Figure 5 Different interactions of PLGA-based nanoplatforms with the microbial biofilm structure. (A) Anti-biofilm agents have limited activity against the biofilm community of microorganisms. (B) PLGA platforms release antibiotics in a sustained manner and boost the anti-biofilm agent’s site-specific delivery, as well as enhance their concentration in the microbial biofilm community. (C) PLGA increased the penetration of various anti-biofilm agents to the mucus and deeper layers of the biofilm. (D) PLGA has low cytotoxic effects on various human cells.
In addition to antibiotics, PLGAs have been used for the delivery of various natural compounds for the inhibition and destruction of the S. aureus biofilm community. Totarol, an ingredient of Podocarpus totara, is one of these natural compounds that in combination with PLGA was used for coating sutures (totarol/PLGA-coated sutures) to inhibit S. aureus growth and colonization. The results showed totarol/PLGA-coated sutures inhibited the growth of S. aureus (over a period of 15 days); additionally, this bacterium failed to form a biofilm on the coated sutures in comparison to uncoated sutures. In comparison to sutures coated only with totarol, sutures coated with PLGA and totarol had a better antibacterial activity. Thus, these findings suggested that totarol/PLGA-coated sutures have the potential to reduce the risk of postoperative biofilm formation on suture material and hence might decrease surgical site infection (Reinbold et al., 2017).
These data support the findings of Reinbold et al. who used the single-emulsion evaporation method and encapsulated totarol with PLGA microspheres. Totarol release was evaluated in a 90-day in vitro drug release assay, with the results showing a release of 53.76%. Notably, S. aureus could not form a biofilm on the surface of totarol-PLGA microspheres, while the biofilm of this bacterium was detected on unloaded PLGA microspheres. Furthermore, the authors did not detect a significant cytotoxic effect against human embryonic kidney cells. As such, these data suggested that the PLGA microspheres due to the great entrapment efficiency, low cytotoxicity, and slow release of totarol could be considered a new anti-biofilm agent that could be highly useful for the long-term therapy of bacterial infections (Reinbold et al., 2016).
In addition to totarol, in another examination, usnic acid (UA), a secondary metabolite of lichen was loaded on the PLGA-polyvinyl alcohol microspheres, after which matrix-assisted pulsed laser evaporation was performed to obtain thin coatings. UA-loaded microspheres not only efficiently suppressed S. aureus attachment, colonization, and biofilm formation for a period of up to 3 days under static conditions but also showed great biocompatibility (Grumezescu et al., 2014b). Hence, it seems that some drawbacks of natural compounds, such as low solubility in water that has been limited to their use in cosmetic formulations, topical ointments, and oral care, could be overcome by applying a PLGA-based drug delivery platform; nevertheless, confirmatory studies are required.
Nitric oxide (NO) has been considered a potential alternative for antimicrobial therapy due to the killing of bacteria in the planktonic and biofilm community. NO is an endogenously produced gas with considerable antimicrobial, vasorelaxation, and antitumor characteristics. Nonetheless, the clinical use of NO has been restricted because of some reasons such as instability during storage, short half-life, poor dose control, and lack of efficient localized and systemic delivery (Saraiva et al., 2011; Hasan et al., 2017). As a result, current research has employed various platforms to deliver therapeutic doses of NO in biological systems.
In this regard, a recently published study encapsulated NO precursor isosorbide mononitrate (ISMN) into the PLGA micro/nanoparticles (w/o/w emulsification/solvent evaporation technique). Both of the synthesized particles continuously released ISMN in physiological media over 3–5 days. Notably, ISMN-PLGA microparticles, with diameters of 3 µm and ISMN loading of 2.2% (w/w), were recognized as the best delivery system and indicated a remarkable anti-biofilm effect against S. aureus biofilms. The initial burst release followed by a continuous release of ISMN from PLGA microparticles boosted the diffusion of ISMN into the biofilm. Thus, the authors proposed that PLGA microparticles (but not NPs) have an acceptable potential to deliver sufficient levels of ISMN for inhibiting the S. aureus biofilm (Hasan et al., 2017).
In another investigation, the authors also prepared an NO-releasing film by fabricating doping dibutyhexyldiamine diazeniumdiolate (DBHD/N2O2) in PLGA. Next, this base layer was encapsulated with a silicone rubber top coating. PLGA in this compound acted as an NO release promoter and controller from the coating by providing protons through its hydrolysis products and intrinsic acid residues. DBHD/N2O2-doped PLGA-based NO-releasing coatings prevented the S. aureus and E. coli biofilm formation (over more than a week’s period). Accordingly, this NO-releasing platform could be considered as an anti-biofilm coating for the preparation of indwelling devices (Cai et al., 2012).
Finally, Hasan et al. prepared doped PLGA-polyethyleneimine/diazeniumdiolate NPs (PLGA-PEI/NO NPs) to enhance the NO penetration to the MRSA biofilm-infected wound. Notably, in the synthesized NPs, PLGA and polyethyleneimine/diazeniumdiolate were used as NP-forming polymer and NO donors, respectively. The synthesized NPs had an acceptable NO loading along with a prolonged NO release over 4 days. In vitro analysis revealed that PLGA-PEI/NO NPs were potently bound to the MRSA biofilm, leading to biofilm destruction and killing the planktonic bacteria upon biofilm dispersal. Additionally, the authors successfully formed MRSA biofilms on wound beds of diabetic mice and used the synthesized NPs for treating the wound infection. Treatment with PLGA-PEI/NO NPs removed the MRSA biofilm from the wound completely and accelerated wound healing in the non-diabetic and diabetic mice. Thus, this novel NO-releasing platform could be used for the treatment of biofilm-infected chronic wounds (Hasan et al., 2019).
In addition to S. aureus, other types of coagulase-negative staphylococci such as S. epidermidis may also produce biofilms and be responsible for chronic wound maintenance. This bacterium is the most common microbe in device-associated infections and could colonize the surface of an implant and develop a biofilm (Rogers et al., 2009). In this regard, previous studies have used various approaches to suppress the adhesion and biofilm formation of S. epidermidis. PLGA NPFs are one of these approaches that have been used in recent studies for the delivery of antibiotics and natural compounds for the inhibition and destruction of the S. epidermidis biofilm, with this section reviewing these studies.
Niemelä et al. (2006) evaluated anti-biofilm characteristics of self-reinforced (SR) CIP-PLGA-releasing implant (the length of the implants was 30 mm and diameter was 3 mm) against S. epidermidis. SR-CIP/PLGA not only inhibited bacterial growth but also destructed the biofilm community by up to 99%. Notably, inhibition effects were not reported around implants without CIP (Niemelä et al., 2006b). These authors also compared this implant function with titanium in preventing S. epidermidis attachment plus biofilm formation in culture media. PLGA/CIP-releasing material significantly suppressed the S. epidermidis attachment and biofilm formation in comparison to the titanium and pure PLGA (Niemelä et al., 2006a). In another formulation, the authors also added CIP and bioactive glass to the PLGA 80/20 and polylactide (P (L/DL) LA 70/30; afterward, the mixture was extruded and self-reinforced. This compound, in line with the two mentioned CIP-release implants, also suppressed the bacterial attachment and biofilm formation (Ashammakhi et al., 2006). As a result, the scientists hypothesized that this CIP-containing biomaterial might play a role in the prevention and treatment of S. epidermidis biofilm-related infections such as trauma surgery and chronic osteomyelitis. Nonetheless, in the clinical infection, there may be several pathogenic microbes involved that may lead to the complicated interactions between the implant and the host; thus, in vitro findings should be confirmed by animal models and clinical trials.
In line with these results, in another examination, rifampicin- and calcium-eluting micropatterns were again used for the destruction of the S. epidermidis biofilm on orthopedic implant surfaces. Rifampicin-containing micropatterns consisted of a periodic array of 50-µm circular dots arranged 150 µm apart. For this purpose, PLGA and rifampicin were dissolved in an organic solvent with 100-nm biphasic calcium phosphate NPs suspended in the solution. Note that the rifampicin release rate was mainly influenced by the antibiotic loading in the micropattern, especially on the first day. The synthesized micropattern killed S. epidermidis before forming colonies, thus suppressing the biofilm community of this bacterium. Additionally, the presence of biphasic calcium phosphate in this nanostructure accelerated osteoblast cell differentiation without compromising cell proliferation (Gu et al., 2012).
These results support the findings of Chen et al. (2012) who deposed rifampicin-containing PLGA micropatterns onto the polycaprolactone/chitosan nanofiber meshes via ink-jet printing. This compound not only prevented the S. epidermidis biofilm formation but also boosted the osteogenic differentiation of preosteoblasts by upregulating the gene expression of bone markers. Hence, these data suggested that this PLGA-based nanostructure could be considered for covering orthopedic implant surfaces—thanks to proper anti-biofilm and healing effects.
A recently published study also used PLGA NPFs for the destruction of the S. epidermidis biofilm. In this regard, the emulsion solvent diffusion method was performed to synthesize clarithromycin-loaded + chitosan-modified PLGA (416.5 ± 4.6 nm size and 19.9 ± 1.7). CLSM analysis revealed that this compound showed high antibacterial and anti-biofilm effects against S. epidermidis. Microscopic study revealed that the produced NPs destroyed bacteria in the biofilm community’s deeper layer and eradicated the outer layer of the biofilm. Notably, the attachment and penetration capacity of NPs were enhanced by the modification of the NP surface with chitosan (Takahashi et al., 2015).
In addition to the mentioned antibiotics, Iannitelli et al. (2011a) used the solvent displacement method for the synthesis of carvacrol/PLGA NPs. Notably, carvacrol is an active compound of various natural products such as thyme and oregano. The synthesized NPs were 209.8 ± 7.2 nm in size and had -18.99 ± 3.01 zeta potential. Carvacrol/PLGA NPs showed good drug loading and encapsulation efficiency and were used for the destruction of S. epidermidis. The results revealed that these NPs would alter the properties of preformed S. epidermidis biofilms and significantly reduced the mechanical stability and elasticity of matured biofilms (Iannitelli et al., 2011a). Thus, as mentioned in the above studies, PLGA NPFs could lead to the acceptable penetration of anti-biofilm agents such as antibiotics and natural compounds to the deeper layer of the bacterial biofilm. However, more in vitro and animal model studies are required for better understanding of the PLGA-based platform interaction and S. epidermidis biofilm community.
Notably, other studies that used PLGA for the destruction of other microbial biofilm and bacterial biofilm-associated oral disorders are presented in Tables 1 and 2, respectively.
Table 1 Previous studies that used PLGA NPFs for inhibition of the microbial biofilm. PLGA: poly (lactide co-glycolide)
Table 2 Different PLGA NPFs that have been used for inhibition of bacterial biofilm-associated oral disorders.
Bacterial infections caused by MDR pathogens can put public health and human lives at risk. Additionally, due to the low penetration of antibiotics, biofilm community of microorganisms extend antibiotics resistance. In this case, nanotechnology and anti-biofilm agents could be used together to fight MDR pathogen biofilms. Today, PLGA NPFs are the basis for medical applications in various fields used by chemists, biologists, and physicians, as well as academic and industrial groups. In the future, we expect new design techniques to further expand the use of PLGA and create efficient delivery systems, which will play an important role in the inhibition and destruction of the microbial biofilm, especially in the MDR biofilm community. For instance, it could be possible to enhance the efficacy of PLGA NPFs by modifying particle surfaces with a cationic polymer such as chitosan. This procedure potentially enhances the interaction of PLGA NPs with anionic mucopolysaccharide in the biofilm and maximizes biofilm exposure to various anti-biofilm agents. Notably, the relative nontoxic nature of PLGA degradation products and its degradability under physiological conditions make it an applicable candidate for creating tissue-engineering scaffolds. However, some PLGA disadvantages such as suboptimal bioactivity and low hydrophilicity limit its applications in a clinical setting and should be considered in future investigations.
This review shows that PLGA could be used for the delivery of various anti-biofilm agents such as antibiotics, natural compounds, NO, and enzymes. However, the data about exact interactions of PLGA NPFs and biofilm structure of microorganisms, product composition, manufacturing procedure for NPs, final particle size, and route of administration, as well as drug release rate requirements are limited. Furthermore, there are no proper standards and guidelines for testing the efficacy, safety, and performance of PLGA NPFs against the microbial biofilm. Thus, more investigations are needed to evaluate the mechanisms by which PLGA NPs suppressed the biofilm formation and eliminated the mature biofilm.
AS and SH conceived and designed the study. AS, ZC, and SH contributed to the comprehensive research and wrote the paper. EG-R and ENZ participated in article editing. Notably, all authors have read and approved the article.
The authors declare that the research was conducted in the absence of any commercial or financial relationships that could be construed as a potential conflict of interest.
All claims expressed in this article are solely those of the authors and do not necessarily represent those of their affiliated organizations, or those of the publisher, the editors and the reviewers. Any product that may be evaluated in this article, or claim that may be made by its manufacturer, is not guaranteed or endorsed by the publisher.
EPS, extracellular matrix; NPs, nanoparticles; PEG, polyethylene glycol; PLGA, poly (lactide co-glycolide); CIP, ciprofloxacin; SEM, scanning electron microscope; W/O/W, water-in-oil-in-water; OMF, oscillating magnetic field; CLSM, confocal laser scanning microscopy; OSA-HA, octenyl succinic anhydride-modified low-molecular weight hyaluronic acid; MBG, mesoporous bioactive glass; PVA, polyvinyl alcohol; UA, usnic acid; NO, nitric oxide; ISMN, isosorbide mononitrate; DBHD, dibutyhexyldiamine diazeniumdiolate; MRSA, methicillin-resistant S. aureus; MDR, multidrug resistant.
Abdelghany, S. M., Quinn, D. J., Ingram, R. J., Gilmore, B. F., Donnelly, R. F., Taggart, C. C., et al. (2012). Gentamicin-Loaded Nanoparticles Show Improved Antimicrobial Effects Towards Pseudomonas Aeruginosa Infection. Int. J. Nanomed. 7, 4053–4063. doi: 10.2147/IJN.S34341
Akram, Z., Daood, U., Aati, S., Ngo, H., Fawzy, A. S. (2021). Formulation of pH-Sensitive Chlorhexidine-Loaded/Mesoporous Silica Nanoparticles Modified Experimental Dentin Adhesive. Mater Sci. Eng. C Mater Biol. Appl. 122, 111894. doi: 10.1016/j.msec.2021.111894
Allavena, P., Palmioli, A., Avigni, R., Sironi, M., La Ferla, B., Maeda, A. (2020). PLGA Based Nanoparticles for the Monocyte-Mediated Anti-Tumor Drug Delivery System. J. Biomed. Nanotech. 16, 212–223. doi: 10.1166/jbn.2020.2881
Alphandéry, E. (2020). Nano-Therapies for Glioblastoma Treatment. Cancers 12, 242. doi: 10.3390/cancers12010242
Anselmo, A. C., Mitragotri, S. (2014). An Overview of Clinical and Commercial Impact of Drug Delivery Systems. J. Controlled Release 190, 15–28. doi: 10.1016/j.jconrel.2014.03.053
Arafa, M. G., Mousa, H. A., Afifi, N. N. (2020). Preparation of PLGA-Chitosan Based Nanocarriers for Enhancing Antibacterial Effect of Ciprofloxacin in Root Canal Infection. Drug Delivery 27, 26–39. doi: 10.1080/10717544.2019.1701140
Ashammakhi, N., Veiranto, M., Suokas, E., Tiainen, J., Niemelä, S. M., Törmälä, P. (2006). Innovation in Multifunctional Bioabsorbable Osteoconductive Drug-Releasing Hard Tissue Fixation Devices. J. Mater Sci. Mater Med. 17, 1275–1282. doi: 10.1007/s10856-006-0602-z
Ashbaugh, A. G., Jiang, X., Zheng, J., Tsai, A. S., Kim, W. S., Thompson, J. M., et al. (2016). Polymeric Nanofiber Coating With Tunable Combinatorial Antibiotic Delivery Prevents Biofilm-Associated Infection In Vivo. Proc. Natl. Acad. Sci. U.S.A. 113, E6919–e6928. doi: 10.1073/pnas.1613722113
Astete, C. E., Sabliov, C. M. (2006). Synthesis and Characterization of PLGA Nanoparticles. J. biomat. science polymer edition 17, 247–289. doi: 10.1163/156856206775997322
Baelo, A., Levato, R., Julián, E., Crespo, A., Astola, J., Gavaldà, J., et al. (2015). Disassembling Bacterial Extracellular Matrix With DNase-Coated Nanoparticles to Enhance Antibiotic Delivery in Biofilm Infections. J. Controlled Release 209, 150–158. doi: 10.1016/j.jconrel.2015.04.028
Bahramian, A., Khoshnood, S., Shariati, A., Doustdar, F., Chirani, A. S., Heidary, M. (2019). Molecular Characterization of the Pils2 Gene and Its Association With the Frequency of Pseudomonas Aeruginosa Plasmid Pklc102 and PAPI-1 Pathogenicity Island. Infection Drug resistance 12, 221. doi: 10.2147/IDR.S188527
Banerjee, S., Pillai, J. (2019). Solid Lipid Matrix Mediated Nanoarchitectonics for Improved Oral Bioavailability of Drugs. Expert Opin. Drug Metab. Toxicol. 15, 499–515. doi: 10.1080/17425255.2019.1621289
Barclay, T. G., Day, C. M., Petrovsky, N., Garg, S. (2019). Review of Polysaccharide Particle-Based Functional Drug Delivery. Carbohydr. polymers 221, 94–112. doi: 10.1016/j.carbpol.2019.05.067
Barros, C. H., Hiebner, D. W., Fulaz, S., Vitale, S., Quinn, L., Casey, E. (2021). Synthesis and Self-Assembly of Curcumin-Modified Amphiphilic Polymeric Micelles With Antibacterial Activity. J. nanobiotech. 19, 1–15. doi: 10.1186/s12951-021-00851-2
Bastari, K., Arshath, M., Ng, Z. H., Chia, J. H., Yow, Z. X., Sana, B., et al. (2014). A Controlled Release of Antibiotics From Calcium Phosphate-Coated Poly(Lactic-Co-Glycolic Acid) Particles and Their In Vitro Efficacy Against Staphylococcus Aureus Biofilm. J. Mater Sci. Mater Med. 25, 747–757. doi: 10.1007/s10856-013-5125-9
Cai, W., Wu, J., Xi, C., Meyerhoff, M. E. (2012). Diazeniumdiolate-Doped Poly(Lactic-Co-Glycolic Acid)-Based Nitric Oxide Releasing Films as Antibiofilm Coatings. Biomaterials 33, 7933–7944. doi: 10.1016/j.biomaterials.2012.07.027
Ceylan, O., Ugur, A. (2015). Chemical Composition and Anti-Biofilm Activity of Thymus Sipyleus BOISS. Subsp. Sipyleus BOISS. Var. Davisianus RONNIGER Essential Oil. Arch. pharmacal. Res. 38, 957–965. doi: 10.1007/s12272-014-0516-0
Cheng, T., Qu, H., Zhang, G., Zhang, X. (2018). Osteogenic and Antibacterial Properties of Vancomycin-Laden Mesoporous Bioglass/PLGA Composite Scaffolds for Bone Regeneration in Infected Bone Defects. Artif. Cells Nanomed. Biotechnol. 46, 1935–1947. doi: 10.1080/21691401.2017.1396997
Chen, X. N., Gu, Y. X., Lee, J. H., Lee, W. Y., Wang, H. J. (2012). Multifunctional Surfaces With Biomimetic Nanofibres and Drug-Eluting Micro-Patterns for Infection Control and Bone Tissue Formation. Eur. Cell Mater 24, 237–248. doi: 10.22203/eCM.v024a17
Cheow, W. S., Chang, M. W., Hadinoto, K. (2010a). Antibacterial Efficacy of Inhalable Antibiotic-Encapsulated Biodegradable Polymeric Nanoparticles Against E. Coli Biofilm Cells. J. BioMed. Nanotechnol 6, 391–403. doi: 10.1166/jbn.2010.1116
Cheow, W. S., Chang, M. W., Hadinoto, K. (2010b). Antibacterial Efficacy of Inhalable Levofloxacin-Loaded Polymeric Nanoparticles Against E. Coli Biofilm Cells: The Effect of Antibiotic Release Profile. Pharm. Res. 27, 1597–1609. doi: 10.1007/s11095-010-0142-6
Cunha, A., Gaubert, A., Latxague, L., Dehay, B. (2021). PLGA-Based Nanoparticles for Neuroprotective Drug Delivery in Neurodegenerative Diseases. Pharmaceutics 13, 1042. doi: 10.3390/pharmaceutics13071042
D'angelo, I., Casciaro, B., Miro, A., Quaglia, F., Mangoni, M. L., Ungaro, F. (2015). Overcoming Barriers in Pseudomonas Aeruginosa Lung Infections: Engineered Nanoparticles for Local Delivery of a Cationic Antimicrobial Peptide. Colloids Surf B Biointerfaces 135, 717–725. doi: 10.1016/j.colsurfb.2015.08.027
Danhier, F., Ansorena, E., Silva, J. M., Coco, R., Le Breton, A., Préat, V. (2012). PLGA-Based Nanoparticles: An Overview of Biomedical Applications. J. Controlled release 161, 505–522. doi: 10.1016/j.jconrel.2012.01.043
Deepika, M. S., Thangam, R., Sundarraj, S., Sheena, T. S., Sivasubramanian, S., Kulandaivel, J., et al. (2019). Co-Delivery of Diverse Therapeutic Compounds Using PEG–PLGA Nanoparticle Cargo Against Drug-Resistant Bacteria: An Improved Anti-Biofilm Strategy. ACS Appl. Bio Materials 3, 385–399. doi: 10.1021/acsabm.9b00850
Deepika, M. S., Thangam, R., Sundarraj, S., Sheena, T. S., Sivasubramanian, S., Kulandaivel, J., et al. (2020). Co-Delivery of Diverse Therapeutic Compounds Using PEG-PLGA Nanoparticle Cargo Against Drug-Resistant Bacteria: An Improved Anti-Biofilm Strategy. ACS Appl. Bio Mater 3, 385–399. doi: 10.1021/acsabm.9b00850
De Mélo Silva, I. S., Do Amorim Costa Gaspar, ,. L. M., Rocha, ,. A. M. O., Da Costa, ,. L. P., Tada, D. B., Franceschi, E., et al. (2020). Encapsulation of Red Propolis in Polymer Nanoparticles for the Destruction of Pathogenic Biofilms. AAPS Pharmscitech 21, 1–9. doi: 10.1208/s12249-019-1576-8
Ding, D., Zhu, Q. (2018). Recent Advances of PLGA Micro/Nanoparticles for the Delivery of Biomacromolecular Therapeutics. Materials Sci. Engineering: C 92, 1041–1060. doi: 10.1016/j.msec.2017.12.036
Durán-Lobato, M., Álvarez-Fuentes, J., Fernández-Arévalo, M., Martín-Banderas, L. (2022). Receptor-Targeted Nanoparticles Modulate Cannabinoid Anticancer Activity Through Delayed Cell Internalization. Sci. Rep. 12, 1–17. doi: 10.1038/s41598-022-05301-z
Duskey, J. T., Baraldi, C., Gamberini, M. C., Ottonelli, I., Da Ros, F., Tosi, G., et al. (2020). Investigating Novel Syntheses of a Series of Unique Hybrid PLGA-Chitosan Polymers for Potential Therapeutic Delivery Applications. Polymers 12, 823. doi: 10.3390/polym12040823
Ernst, J., Klinger-Strobel, M., Arnold, K., Thamm, J., Hartung, A., Pletz, M. W., et al. (2018). Polyester-Based Particles to Overcome the Obstacles of Mucus and Biofilms in the Lung for Tobramycin Application Under Static and Dynamic Fluidic Conditions. Eur. J. Pharm. Biopharm 131, 120–129. doi: 10.1016/j.ejpb.2018.07.025
Fan, W., Liu, D., Li, Y., Sun, Q., Fan, B. (2018). AgCa-PLGA Submicron Particles Inhibit the Growth and Colonization of E. Faecalis and P. Gingivalis on Dentin Through Infiltration Into Dentinal Tubules. Int. J. Pharm. 552, 206–216. doi: 10.1016/j.ijpharm.2018.09.066
Ficai, D., Grumezescu, V., Fufă, O. M., Popescu, R. C., Holban, A. M., Ficai, A., et al. (2018). Antibiofilm Coatings Based on PLGA and Nanostructured Cefepime-Functionalized Magnetite. Nanomat. (Basel) 8 (9), 633. doi: 10.3390/nano8090633
Francisconi, R. S., Huacho, P. M. M., Tonon, C. C., Bordini, ,.E., Correia, M. F., Sardi, ,. J. C. O., et al. (2020). Antibiofilm Efficacy of Tea Tree Oil and of its Main Component Terpinen-4-Ol Against Candida Albicans. Braz. Oral. Res. 34, e050. doi: 10.1590/1807-3107bor-2020.vol34.0050
Freire, M. O., Devaraj, A., Young, A., Navarro, J. B., Downey, J. S., Chen, C., et al. (2017). A Bacterial-Biofilm-Induced Oral Osteolytic Infection can be Successfully Treated by Immuno-Targeting an Extracellular Nucleoid-Associated Protein. Mol. Oral. Microbiol. 32, 74–88. doi: 10.1111/omi.12155
Gentile, P., Chiono, V., Carmagnola, I., Hatton, P. V. (2014). An Overview of Poly(Lactic-Co-Glycolic) Acid (PLGA)-Based Biomaterials for Bone Tissue Engineering. Int. J. Mol. Sci. 15, 3640–3659. doi: 10.3390/ijms15033640
Geremias, T. C., Sartoretto, S. C., Batistella, M. A., Souza, ,.A., Alves, A., Uzeda, M. J. P., et al. (2021). In Vivo Biological Evaluation of Biodegradable Nanofibrous Membranes Incorporated With Antibiofilm Compounds. Polymers (Basel) 13 (15), 2457. doi: 10.3390/polym13152457
Glen, K. A., Lamont, I. L. (2021). β-Lactam Resistance in Pseudomonas Aeruginosa: Current Status, Future Prospects. Pathogens 10 (12), 1638. doi: 10.3390/pathogens10121638
Grumezescu, A. M., Gestal, M. C., Holban, A. M., Grumezescu, V., Vasile, B. S., Mogoantă, L., et al. (2014a). Biocompatible Fe3O4 Increases the Efficacy of Amoxicillin Delivery Against Gram-Positive and Gram-Negative Bacteria. Molecules 19, 5013–5027. doi: 10.3390/molecules19045013
Grumezescu, V., Holban, A. M., Grumezescu, A. M., Socol, G., Ficai, A., Vasile, B. S., et al. (2014b). Usnic Acid-Loaded Biocompatible Magnetic PLGA-PVA Microsphere Thin Films Fabricated by MAPLE With Increased Resistance to Staphylococcal Colonization. Biofabrication 6, 035002. doi: 10.1088/1758-5082/6/3/035002
Gu, Y., Chen, X., Lee, J. H., Monteiro, D. A., Wang, H., Lee, W. Y. (2012). Inkjet Printed Antibiotic- and Calcium-Eluting Bioresorbable Nanocomposite Micropatterns for Orthopedic Implants. Acta Biomater 8, 424–431. doi: 10.1016/j.actbio.2011.08.006
Gumus, B., Acar, T., Atabey, T., Derman, S., Sahin, F., Arasoglu, T. (2020). The Battle Against Biofilm Infections: Juglone Loaded Nanoparticles as an Anticandidal Agent. J. Biotechnol. 316, 17–26. doi: 10.1016/j.jbiotec.2020.04.009
Guo, R., Li, K., Tian, B., Wang, C., Chen, X., Jiang, X., et al. (2021). Elaboration on the Architecture of pH-Sensitive Surface Charge-Adaptive Micelles With Enhanced Penetration and Bactericidal Activity in Biofilms. J. nanobiotech. 19, 1–18. doi: 10.1186/s12951-021-00980-8
Guo, X., Zhang, X., Ye, L., Zhang, Y., Ding, R., Hao, Y., et al. (2014). Inhalable Microspheres Embedding Chitosan-Coated PLGA Nanoparticles for 2-Methoxyestradiol. J. Drug Targeting 22, 421–427. doi: 10.3109/1061186X.2013.878944
Han, C., Goodwine, J., Romero, N., Steck, K. S., Sauer, K., Doiron, A. (2019). Enzyme-Encapsulating Polymeric Nanoparticles: A Potential Adjunctive Therapy in Pseudomonas Aeruginosa Biofilm-Associated Infection Treatment. Colloids Surfaces B: Biointerfaces 184, 110512. doi: 10.1016/j.colsurfb.2019.110512
Han, C., Romero, N., Fischer, S., Dookran, J., Berger, A., Doiron, A. L. (2017). Recent Developments in the Use of Nanoparticles for Treatment of Biofilms. Nanotech. Rev. 6, 383–404. doi: 10.1515/ntrev-2016-0054
Hasan, N., Cao, J., Lee, J., Naeem, M., Hlaing, S. P., Kim, J., et al. (2019). PEI/NONOates-Doped PLGA Nanoparticles for Eradicating Methicillin-Resistant Staphylococcus Aureus Biofilm in Diabetic Wounds via Binding to the Biofilm Matrix. Mater Sci. Eng. C Mater Biol. Appl. 103, 109741. doi: 10.1016/j.msec.2019.109741
Hasan, S., Thomas, N., Thierry, B., Prestidge, C. A. (2017). Biodegradable Nitric Oxide Precursor-Loaded Micro- and Nanoparticles for the Treatment of Staphylococcus Aureus Biofilms. J. Mater Chem. B 5, 1005–1014. doi: 10.1039/C6TB03290G
Hosseini, S. M., Abbasalipourkabir, R., Jalilian, F. A., Asl, S. S., Farmany, A., Roshanaei, G., et al. (2019a). Doxycycline-Encapsulated Solid Lipid Nanoparticles as Promising Tool Against Brucella Melitensis Enclosed in Macrophage: A Pharmacodynamics Study on J774A. 1 Cell Line. Antimicrobial Resistance Infection Control 8, 1–12. doi: 10.1186/s13756-019-0504-8
Hosseini, S. M., Farmany, A., Abbasalipourkabir, R., Soleimani Asl, S., Nourian, A., Arabestani, M. R. (2019b). Doxycycline-Encapsulated Solid Lipid Nanoparticles for the Enhanced Antibacterial Potential to Treat the Chronic Brucellosis and Preventing its Relapse: In Vivo Study. Ann. Clin. Microbiol. Antimicrobials 18, 1–10. doi: 10.1186/s12941-019-0333-x
Hosseini, S. M., Taheri, M., Nouri, F., Farmani, A., Moez, N. M., Arabestani, M. R. (2022). Nano Drug Delivery in Intracellular Bacterial Infection Treatments. Biomed. Pharmacother. 146, 112609. doi: 10.1016/j.biopha.2021.112609
Huang, Z., Zhou, T., Yuan, Y., Kłodzińska, S. N., Zheng, T., Sternberg, C., et al. (2020). Synthesis of Carbon Quantum Dot-Poly Lactic-Co-Glycolic Acid Hybrid Nanoparticles for Chemo-Photothermal Therapy Against Bacterial Biofilms. J. Colloid Interface Sci. 577, 66–74. doi: 10.1016/j.jcis.2020.05.067
Hua, X., Tan, S., Bandara, H. M., Fu, Y., Liu, S., Smyth, H. D. (2014). Externally Controlled Triggered-Release of Drug From PLGA Micro and Nanoparticles. PLoS One 9, e114271. doi: 10.1371/journal.pone.0114271
Iadnut, A., Mamoon, K., Thammasit, P., Pawichai, S., Tima, S., Preechasuth, K., et al. (2019). In Vitro Antifungal and Antivirulence Activities of Biologically Synthesized Ethanolic Extract of Propolis-Loaded PLGA Nanoparticles Against Candida Albicans. Evid Based Complement Alternat Med. 2019, 3715481. doi: 10.1155/2019/3715481
Iannitelli, A., Grande, R., Di Stefano, A., Di Giulio, M., Sozio, P., Bessa, L. J., et al. (2011a). Potential Antibacterial Activity of Carvacrol-Loaded Poly(DL-Lactide-Co-Glycolide) (PLGA) Nanoparticles Against Microbial Biofilm. Int. J. Mol. Sci. 12, 5039–5051. doi: 10.3390/ijms12085039
Iannitelli, A., Grande, R., Stefano, A. D., Giulio, M. D., Sozio, P., Bessa, L. J., et al. (2011b). Potential Antibacterial Activity of Carvacrol-Loaded Poly (DL-Lactide-Co-Glycolide)(PLGA) Nanoparticles Against Microbial Biofilm. Int. J. Mol. Sci. 12, 5039–5051. doi: 10.3390/ijms12085039
Jain, R. A. (2000). The Manufacturing Techniques of Various Drug Loaded Biodegradable Poly (Lactide-Co-Glycolide)(PLGA) Devices. Biomaterials 21, 2475–2490. doi: 10.1016/S0142-9612(00)00115-0
Kalhapure, R. S., Suleman, N., Mocktar, C., Seedat, N., Govender, T. (2015). Nanoengineered Drug Delivery Systems for Enhancing Antibiotic Therapy. J. Pharm. Sci. 104, 872–905. doi: 10.1002/jps.24298
Kalia, P., Jain, A., Radha Krishnan, R., Demuth, D. R., Steinbach-Rankins, J. M. (2017). Peptide-Modified Nanoparticles Inhibit Formation of Porphyromonas Gingivalis Biofilms With Streptococcus Gordonii. Int. J. Nanomed. 12, 4553–4562. doi: 10.2147/IJN.S139178
Kamaly, N., Yameen, B., Wu, J., Farokhzad, O. C. (2016). Degradable Controlled-Release Polymers and Polymeric Nanoparticles: Mechanisms of Controlling Drug Release. Chem. Rev. 116, 2602–2663. doi: 10.1021/acs.chemrev.5b00346
Kang, M., Kim, S., Kim, H., Song, Y., Jung, D., Kang, S., et al. (2019). Calcium-Binding Polymer-Coated Poly (Lactide-Co-Glycolide) Microparticles for Sustained Release of Quorum Sensing Inhibitors to Prevent Biofilm Formation on Hydroxyapatite Surfaces. ACS Appl. materials interfaces 11, 7686–7694. doi: 10.1021/acsami.8b18301
Kim, Y. G., Lee, J. H., Gwon, G., Kim, S. I., Park, J. G., Lee, J. (2016). Essential Oils and Eugenols Inhibit Biofilm Formation and the Virulence of Escherichia Coli O157:H7. Sci. Rep. 6, 36377. doi: 10.1038/srep36377
Kim, Y.-G., Lee, J.-H., Kim, S.-I., Baek, K.-H., Lee, J. (2015). Cinnamon Bark Oil and its Components Inhibit Biofilm Formation and Toxin Production. Int. J. Food Microbiol. 195, 30–39. doi: 10.1016/j.ijfoodmicro.2014.11.028
Kłodzińska, S. N., Wan, F., Jumaa, H., Sternberg, C., Rades, T., Nielsen, H. M. (2019). Utilizing Nanoparticles for Improving Anti-Biofilm Effects of Azithromycin: A Head-to-Head Comparison of Modified Hyaluronic Acid Nanogels and Coated Poly (Lactic-Co-Glycolic Acid) Nanoparticles. J. colloid Interface Sci. 555, 595–606. doi: 10.1016/j.jcis.2019.08.006
Lagreca, E., Onesto, V., Di Natale, C., La Manna, S., Netti, P. A., Vecchione, R. (2020). Recent Advances in the Formulation of PLGA Microparticles for Controlled Drug Delivery. Prog. Biomater 9, 153–174. doi: 10.1007/s40204-020-00139-y
Li, Y., Na, R., Wang, X., Liu, H., Zhao, L., Sun, X., et al. (2017). Fabrication of Antimicrobial Peptide-Loaded PLGA/Chitosan Composite Microspheres for Long-Acting Bacterial Resistance. Molecules 22 (10), 1637. doi: 10.3390/molecules22101637
Liu, X., Wang, Z., Feng, X., Bai, E., Xiong, Y., Zhu, X., et al. (2020). Platensimycin-Encapsulated Poly(lactic-Co-Glycolic Acid) and Poly(amidoamine) Dendrimers Nanoparticles With Enhanced Anti-Staphylococcal Activity in Vivo. Bioconjug Chem. 31, 1425–1437. doi: 10.1021/acs.bioconjchem.0c00121
Luo, G., Jin, C., Long, J., Fu, D., Yang, F., Xu, J., et al. (2009). RNA Interference of MBD1 in BxPC-3 Human Pancreatic Cancer Cells Delivered by PLGA-Poloxamer Nanoparticles. Cancer Biol. Ther. 8, 594–598. doi: 10.4161/cbt.8.7.7790
Ma, L., Conover, M., Lu, H., Parsek, M. R., Bayles, K., Wozniak, D. J. (2009). Assembly and Development of the Pseudomonas Aeruginosa Biofilm Matrix. PLoS Pathog. 5 (3), e1000354. doi: 10.1371/journal.ppat.1000354
Mahmoud, M. Y., Demuth, D. R., Steinbach-Rankins, J. M. (2018). BAR-Encapsulated Nanoparticles for the Inhibition and Disruption of Porphyromonas Gingivalis-Streptococcus Gordonii Biofilms. J. Nanobiotech. 16, 69. doi: 10.1186/s12951-018-0396-4
Mahmoud, M. Y., Sapare, S., Curry, K. C., Demuth, D. R., Steinbach-Rankins, J. M. (2019a). Rapid Release Polymeric Fibers for Inhibition of Porphyromonas Gingivalis Adherence to Streptococcus Gordonii. Front. Chem. 7, 926. doi: 10.3389/fchem.2019.00926
Mahmoud, M. Y., Steinbach-Rankins, J. M., Demuth, D. R. (2019b). Functional Assessment of Peptide-Modified PLGA Nanoparticles Against Oral Biofilms in a Murine Model of Periodontitis. J. Control Release 297, 3–13. doi: 10.1016/j.jconrel.2019.01.036
Ma, R., Lai, Y. X., Li, L., Tan, H. L., Wang, J. L., Li, Y., et al. (2015). Bacterial Inhibition Potential of 3D Rapid-Prototyped Magnesium-Based Porous Composite Scaffolds–an In Vitro Efficacy Study. Sci. Rep. 5, 13775. doi: 10.1038/srep13775
Mao, Z., Li, Y., Yang, Y., Fang, Z., Chen, X., Wang, Y., et al. (2018). Osteoinductivity and Antibacterial Properties of Strontium Ranelate-Loaded Poly(Lactic-Co-Glycolic Acid) Microspheres With Assembled Silver and Hydroxyapatite Nanoparticles. Front. Pharmacol. 9, 368. doi: 10.3389/fphar.2018.00368
Ma, R., Wang, W., Yang, P., Wang, C., Guo, D., Wang, K. (2020). In Vitro Antibacterial Activity and Cytocompatibility of Magnesium-Incorporated Poly(Lactide-Co-Glycolic Acid) Scaffolds. BioMed. Eng. Online 19, 12. doi: 10.1186/s12938-020-0755-x
Mckenna, E., Klein, T. J., Doran, M. R., Futrega, K. (2019). Integration of an Ultra-Strong Poly (Lactic-Co-Glycolic Acid)(PLGA) Knitted Mesh Into a Thermally Induced Phase Separation (TIPS) PLGA Porous Structure to Yield a Thin Biphasic Scaffold Suitable for Dermal Tissue Engineering. Biofabrication 12, 015015. doi: 10.1088/1758-5090/ab4053
Moghadam, M. T., Chegini, Z., Khoshbayan, A., Farahani, I., Shariati, A. (2021). Helicobacter Pylori Biofilm and New Strategies to Combat it. Curr. Mol. Med. 21, 549–561. doi: 10.2174/1566524020666201203165649
Mohamed, N. A., Marei, I., Crovella, S., Abou-Saleh, H. (2022). Recent Developments in Nanomaterials-Based Drug Delivery and Upgrading Treatment of Cardiovascular Diseases. Int. J. Mol. Sci. 23, 1404. doi: 10.3390/ijms23031404
Molavi, F., Barzegar-Jalali, M., Hamishehkar, H. (2020). Polyester Based Polymeric Nano and Microparticles for Pharmaceutical Purposes: A Review on Formulation Approaches. J. Controlled Release 320, 265–282. doi: 10.1016/j.jconrel.2020.01.028
Morteza, M., Roya, S., Hamed, H., Amir, Z., Abolfazl, A. (2019). Synthesis and Evaluation of Polymeric Micelle Containing Piperacillin/Tazobactam for Enhanced Antibacterial Activity. Drug delivery 26, 1292–1299. doi: 10.1080/10717544.2019.1693708
Mundargi, R. C., Babu, V. R., Rangaswamy, V., Patel, P., Aminabhavi, T. M. (2008). Nano/micro Technologies for Delivering Macromolecular Therapeutics Using Poly (D, L-Lactide-Co-Glycolide) and its Derivatives. J. Controlled Release 125, 193–209. doi: 10.1016/j.jconrel.2007.09.013
Naskar, S., Das, S. K., Sharma, S., Kuotsu, K. (2021). A Review on Designing Poly (Lactic-Co-Glycolic Acid) Nanoparticles as Drug Delivery Systems. Pharm. Nanotech. 9, 36–50. doi: 10.2174/2211738508666201214103010
Nasrollahzadeh, M., Sajadi, S. M., Sajjadi, M., Issaabadi, Z. (2019). “An Introduction to Nanotechnology,” in Interface Science and Technology (Elsevier), 1–27.
Niemelä, S. M., Ikäheimo, I., Koskela, M., Veiranto, M., Suokas, E., Törmälä, P., et al. (2006a). Ciprofloxacin-Releasing Bioabsorbable Polymer is Superior to Titanium in Preventing Staphylococcus Epidermidis Attachment and Biofilm Formation In Vitro. J. BioMed. Mater Res. B Appl. Biomater 76, 8–14. doi: 10.1002/jbm.b.30414
Niemelä, S. M., Länsman, S., Ikäheimo, I., Koskela, M., Veiranto, M., Suokas, E., et al. (2006b). Self-Reinforced Ciprofloxacin-Releasing Polylactide-Co-Glycolide 80/20 Inhibits Attachment and Biofilm Formation by Staphylococcus Epidermidis: An In Vitro Study. J. Craniofac Surg. 17, 950–956. doi: 10.1097/01.scs.0000231621.02888.46
Operti, M. C., Bernhardt, A., Grimm, S., Engel, A., Figdor, C. G., Tagit, O. (2021). PLGA-Based Nanomedicines Manufacturing: Technologies Overview and Challenges in Industrial Scale-Up. Int. J. Pharmaceutics 605, 120807. doi: 10.1016/j.ijpharm.2021.120807
Operti, M. C., Fecher, D., Van Dinther, E. A., Grimm, S., Jaber, R., Figdor, C. G., et al. (2018). A Comparative Assessment of Continuous Production Techniques to Generate Sub-Micron Size PLGA Particles. Int. J. pharmaceutics 550, 140–148. doi: 10.1016/j.ijpharm.2018.08.044
Park, S., Sauer, K. (2021). SagS and its Unorthodox Contributions to Pseudomonas Aeruginosa Biofilm Development. Biofilm 3, 100059. doi: 10.1016/j.bioflm.2021.100059
Patra, J. K., Das, G., Fraceto, L. F., Campos, E. V. R., Rodriguez-Torres, M. D. P., Acosta-Torres, L. S., et al. (2018). Nano Based Drug Delivery Systems: Recent Developments and Future Prospects. J. nanobiotech. 16, 1–33. doi: 10.1186/s12951-018-0392-8
Permana, A. D., Mir, M., Utomo, E., Donnelly, R. F. (2020). Bacterially Sensitive Nanoparticle-Based Dissolving Microneedles of Doxycycline for Enhanced Treatment of Bacterial Biofilm Skin Infection: A Proof of Concept Study. Int. J. Pharm. X 2, 100047. doi: 10.1016/j.ijpx.2020.100047
Qiao, J., Purro, M., Liu, Z., Xiong, M. P. (2018). Terpyridine-Micelles for Inhibiting Bacterial Biofilm Development. ACS Infect. Dis. 4, 1346–1354. doi: 10.1021/acsinfecdis.8b00091
Qiao, Z., Yuan, Z., Zhang, W., Wei, D., Hu, N. (2019). Preparation, In Vitro Release and Antibacterial Activity Evaluation of Rifampicin and Moxifloxacin-Loaded Poly(D,L-Lactide-Co-Glycolide) Microspheres. Artif. Cells Nanomed. Biotechnol. 47, 790–798. doi: 10.1080/21691401.2019.1581792
Reinbold, J., Hierlemann, T., Hinkel, H., Müller, I., Maier, M. E., Weindl, T., et al. (2016). Development and In Vitro Characterization of Poly(Lactide-Co-Glycolide) Microspheres Loaded With an Antibacterial Natural Drug for the Treatment of Long-Term Bacterial Infections. Drug Des. Devel Ther. 10, 2823–2832. doi: 10.2147/DDDT.S105367
Reinbold, J., Uhde, A. K., Müller, I., Weindl, T., Geis-Gerstorfer, J., Schlensak, C., et al. (2017). Preventing Surgical Site Infections Using a Natural, Biodegradable, Antibacterial Coating on Surgical Sutures. Molecules 22 (9), 1570. doi: 10.3390/molecules22091570
Remold, S. K., Brown, C. K., Farris, J. E., Hundley, T. C., Perpich, J. A., Purdy, M. E. (2011). Differential Habitat Use and Niche Partitioning by Pseudomonas Species in Human Homes. Microbial Ecol. 62, 505. doi: 10.1007/s00248-011-9844-5
Reynolds, D., Kollef, M. (2021). The Epidemiology and Pathogenesis and Treatment of Pseudomonas Aeruginosa Infections: An Update. Drugs 81, 2117–2131. doi: 10.1007/s40265-021-01635-6
Rizzo, F., Kehr, N. S. (2021). Recent Advances in Injectable Hydrogels for Controlled and Local Drug Delivery. Advanced Healthcare Materials 10, 2001341. doi: 10.1002/adhm.202001341
Rocha, C. V., Gonçalves, V., Da Silva, M. C., Bañobre-López, M., Gallo, J. (2022). PLGA-Based Composites for Various Biomedical Applications. Int. J. Mol. Sci. 23, 2034. doi: 10.3390/ijms23042034
Rogers, K. L., Fey, P. D., Rupp, M. E. (2009). Coagulase-Negative Staphylococcal Infections. Infect. Dis. Clin. North Am. 23, 73–98. doi: 10.1016/j.idc.2008.10.001
Sabaeifard, P., Abdi-Ali, A., Gamazo, C., Irache, J. M., Soudi, M. R. (2017). Improved Effect of Amikacin-Loaded Poly (D, L-Lactide-Co-Glycolide) Nanoparticles Against Planktonic and Biofilm Cells of Pseudomonas Aeruginosa. J. Med. Microbiol. 66, 137–148. doi: 10.1099/jmm.0.000430
Sah, H., Thoma, L. A., Desu, H. R., Sah, E., Wood, G. C. (2013). Concepts and Practices Used to Develop Functional PLGA-Based Nanoparticulate Systems. Int. J. nanomedicine 8, 747. doi: 10.2147/IJN.S40579
Said, S. S., Aloufy, A. K., El-Halfawy, O. M., Boraei, N. A., El-Khordagui, L. K. (2011). Antimicrobial PLGA Ultrafine Fibers: Interaction With Wound Bacteria. Eur. J. Pharm. Biopharm 79, 108–118. doi: 10.1016/j.ejpb.2011.03.002
Said, Z., Sundar, L. S., Tiwari, A. K., Ali, H. M., Sheikholeslami, M., Bellos, E., et al. (2022). Recent Advances on the Fundamental Physical Phenomena Behind Stability, Dynamic Motion, Thermophysical Properties, Heat Transport, Applications, and Challenges of Nanofluids. Phys. Rep. 946, 1–94. doi: 10.1016/j.physrep.2021.07.002
Sanderson, A. R., Leid, J. G., Hunsaker, D. (2006). Bacterial Biofilms on the Sinus Mucosa of Human Subjects With Chronic Rhinosinusitis. Laryngoscope 116, 1121–1126. doi: 10.1097/01.mlg.0000221954.05467.54
Saraiva, J., Marotta-Oliveira, S. S., Cicillini, S. A., Eloy, J. D. O., Marchetti, J. M. (2011). Nanocarriers for Nitric Oxide Delivery. J. Drug delivery 2011 (2011), 936438. doi: 10.1155/2011/936438
Sebelemetja, M., Moeno, S., Patel, M. (2020). Anti-Acidogenic, Anti-Biofilm and Slow Release Properties of Dodonaea Viscosa Var. Angustifolia Flavone Stabilized Polymeric Nanoparticles. Arch. Oral. Biol. 109, 104586. doi: 10.1016/j.archoralbio.2019.104586
Shen, X., Li, T., Xie, X., Feng, Y., Chen, Z., Yang, H., et al. (2020). PLGA-Based Drug Delivery Systems for Remotely Triggered Cancer Therapeutic and Diagnostic Applications. Front. Bioengineering Biotechnol. 8, 381. doi: 10.3389/fbioe.2020.00381
Simonetti, G., Palocci, C., Valletta, A., Kolesova, O., Chronopoulou, L., Donati, L., et al. (2019). Anti-Candida Biofilm Activity of Pterostilbene or Crude Extract From Non-Fermented Grape Pomace Entrapped in Biopolymeric Nanoparticles. Molecules 24 (11), 2070. doi: 10.3390/molecules24112070
Simonetti, O., Rizzetto, G., Radi, G., Molinelli, E., Cirioni, O., Giacometti, A., et al. (2021). New Perspectives on Old and New Therapies of Staphylococcal Skin Infections: The Role of Biofilm Targeting in Wound Healing. Antibiotics (Basel) 10 (11), 1377. doi: 10.3390/antibiotics10111377
Singh, A. P., Biswas, A., Shukla, A., Maiti, P. (2019). Targeted Therapy in Chronic Diseases Using Nanomaterial-Based Drug Delivery Vehicles. Signal transduction targeted Ther. 4, 1–21. doi: 10.1038/s41392-019-0068-3
Singh, S., Singha, P. (2021). Effect of Modifications in Poly (Lactide-Co-Glycolide) (PLGA) on Drug Release and Degradation Characteristics: A Mini Review. Curr. Drug Delivery 18, 1378–1390. doi: 10.2174/1567201818666210510165938
Soussan, E., Cassel, S., Blanzat, M., Rico-Lattes, I. (2009). Drug Delivery by Soft Matter: Matrix and Vesicular Carriers. Angewandte Chemie Int. Edition 48, 274–288. doi: 10.1002/anie.200802453
Spoering, A. L., Lewis, K. (2001). Biofilms and Planktonic Cells of Pseudomonas Aeruginosa Have Similar Resistance to Killing by Antimicrobials. J. bacteriol. 183, 6746–6751. doi: 10.1128/JB.183.23.6746-6751.2001
Stewart, P. S., Costerton, J. W. (2001). Antibiotic Resistance of Bacteria in Biofilms. Lancet 358, 135–138. doi: 10.1016/S0140-6736(01)05321-1
Swider, E., Koshkina, O., Tel, J., Cruz, L. J., De Vries, I. J. M., Srinivas, M. (2018). Customizing Poly(Lactic-Co-Glycolic Acid) Particles for Biomedical Applications. Acta Biomater 73, 38–51. doi: 10.1016/j.actbio.2018.04.006
Takada, S., Uda, Y., Toguchi, H., Ogawa, Y. (1995). Application of a Spray Drying Technique in the Production of TRH-Containing Injectable Sustained-Release Microparticles of Biodegradable Polymers. PDA J. Pharm. Sci. Technol. 49, 180–184.
Takahashi, C., Hattori, Y., Yagi, S., Murai, T., Takai, C., Ogawa, N., et al. (2019). Optimization of Ionic Liquid-Incorporated PLGA Nanoparticles for Treatment of Biofilm Infections. Mater Sci. Eng. C Mater Biol. Appl. 97, 78–83. doi: 10.1016/j.msec.2018.11.079
Takahashi, C., Matsubara, N., Akachi, Y., Ogawa, N., Kalita, G., Asaka, T., et al. (2017). Visualization of Silver-Decorated Poly (DL-Lactide-Co-Glycolide) Nanoparticles and Their Efficacy Against Staphylococcus Epidermidis. Mater Sci. Eng. C Mater Biol. Appl. 72, 143–149. doi: 10.1016/j.msec.2016.11.051
Takahashi, C., Ogawa, N., Kawashima, Y., Yamamoto, H. (2015). Observation of Antibacterial Effect of Biodegradable Polymeric Nanoparticles on Staphylococcus Epidermidis Biofilm Using FE-SEM With an Ionic Liquid. Microscopy (Oxf) 64, 169–180. doi: 10.1093/jmicro/dfv010
Tan, C. H., Jiang, L., Li, W., Chan, S. H., Baek, J. S., Ng, N. K. J., et al. (2021). Lipid-Polymer Hybrid Nanoparticles Enhance the Potency of Ampicillin Against Enterococcus Faecalis in a Protozoa Infection Model. ACS Infect. Dis. 7, 1607–1618. doi: 10.1021/acsinfecdis.0c00774
Taubes, G. (2008). The Bacteria Fight Back. Science 321 (5887), 356–361 doi: 10.1126/science.321.5887.356
Van Den Driessche, F., Brackman, G., Swimberghe, R., Rigole, P., Coenye, T. (2017). Screening a Repurposing Library for Potentiators of Antibiotics Against Staphylococcus Aureus Biofilms. Int. J. antimicrobial Agents 49, 315–320. doi: 10.1016/j.ijantimicag.2016.11.023
Wan, F., Bohr, S. S.-R., KłodziníSka, ,., Jumaa, H., Huang, Z., Nylander, T., et al. (2019). Ultrasmall TPGS–PLGA Hybrid Nanoparticles for Site-Specific Delivery of Antibiotics Into Pseudomonas Aeruginosa Biofilms in Lungs. ACS Appl. materials interfaces 12, 380–389. doi: 10.1021/acsami.9b19644
Wang, B., Kostarelos, K., Nelson, B. J., Zhang, L. (2021). Trends in Micro-/Nanorobotics: Materials Development, Actuation, Localization, and System Integration for Biomedical Applications. Advanced Materials 33, 2002047. doi: 10.1002/adma.202002047
Wan, F., Maltesen, M. J., Andersen, S. K., Bjerregaard, S., Foged, C., Rantanen, J., et al. (2014). One-Step Production of Protein-Loaded PLGA Microparticles via Spray Drying Using 3-Fluid Nozzle. Pharm. Res. 31, 1967–1977. doi: 10.1007/s11095-014-1299-1
Wolfmeier, H., Pletzer, D., Mansour, S. C., Hancock, R. E. (2018). New Perspectives in Biofilm Eradication. ACS Infect. Dis. 4, 93–106. doi: 10.1021/acsinfecdis.7b00170
Won, C., Kwon, C., Park, K., Seo, J., Lee, T. (2021). Electronic Drugs: Spatial and Temporal Medical Treatment of Human Diseases. Advanced Materials 33, 2005930. doi: 10.1002/adma.202005930
Wu, S., Zhang, J., Peng, Q., Liu, Y., Lei, L., Zhang, H. (2021). The Role of Staphylococcus Aureus YycFG in Gene Regulation, Biofilm Organization and Drug Resistance. Antibiotics (Basel) 10 (12), 1555. doi: 10.3390/antibiotics10121555
Xiu, W., Shan, J., Yang, K., Xiao, H., Yuwen, L., Wang, L. (2021). Recent Development of Nanomedicine for the Treatment of Bacterial Biofilm Infections. View 2, 20200065. doi: 10.1002/VIW.20200065
Yang, M., Du, K., Hou, Y., Xie, S., Dong, Y., Li, D., et al. (2019). Synergistic Antifungal Effect of Amphotericin B-Loaded Poly (Lactic-Co-Glycolic Acid) Nanoparticles and Ultrasound Against Candida Albicans Biofilms. Antimicrobial Agents chemother. 63, e02022–e02018. doi: 10.1128/AAC.02022-18
Zare, E. N., Jamaledin, R., Naserzadeh, P., Afjeh-Dana, E., Ashtari, B., Hosseinzadeh, M., et al. (2020). Metal-Based Nanostructures/PLGA Nanocomposites: Antimicrobial Activity, Cytotoxicity, and Their Biomedical Applications. ACS Appl. Mater Interfaces 12, 3279–3300. doi: 10.1021/acsami.9b19435
Zhang, J., Wang, J., Qiao, F., Liu, Y., Zhou, Y., Li, M., et al. (2021). Polymeric non-Spherical Coarse Microparticles Fabricated by Double Emulsion-Solvent Evaporation for Simvastatin Delivery. Colloids Surfaces B: Biointerfaces 199, 111560. doi: 10.1016/j.colsurfb.2021.111560
Zhong, H., Chan, G., Hu, Y., Hu, H., Ouyang, D. (2018). A Comprehensive Map of FDA-Approved Pharmaceutical Products. Pharmaceutics 10, 263. doi: 10.3390/pharmaceutics10040263
Keywords: biofilm, PLGA, poly lactic-co-glycolic acid, Pseudomonas aeruginosa, Staphylococcus aeruginosa, Staphylococcus epidermidis
Citation: Shariati A, Chegini Z, Ghaznavi-Rad E, Zare EN and Hosseini SM (2022) PLGA-Based Nanoplatforms in Drug Delivery for Inhibition and Destruction of Microbial Biofilm. Front. Cell. Infect. Microbiol. 12:926363. doi: 10.3389/fcimb.2022.926363
Received: 22 April 2022; Accepted: 23 May 2022;
Published: 21 June 2022.
Edited by:
Jayendrakumar Patel, Ganpat University, IndiaReviewed by:
Rakesh Patel, Shree S. K. Patel College of Pharmaceutical Education and Research Ganpat University, IndiaCopyright © 2022 Shariati, Chegini, Ghaznavi-Rad, Zare and Hosseini. This is an open-access article distributed under the terms of the Creative Commons Attribution License (CC BY). The use, distribution or reproduction in other forums is permitted, provided the original author(s) and the copyright owner(s) are credited and that the original publication in this journal is cited, in accordance with accepted academic practice. No use, distribution or reproduction is permitted which does not comply with these terms.
*Correspondence: Seyed Mostafa Hosseini, c21ob3NlaW55ODhAeWFob28uY29t
Disclaimer: All claims expressed in this article are solely those of the authors and do not necessarily represent those of their affiliated organizations, or those of the publisher, the editors and the reviewers. Any product that may be evaluated in this article or claim that may be made by its manufacturer is not guaranteed or endorsed by the publisher.
Research integrity at Frontiers
Learn more about the work of our research integrity team to safeguard the quality of each article we publish.