- 1Department of Parasitology, Xiangya School of Medicine, Central South University, Changsha, China
- 2Department of Immunology, Xiangya School of Medicine, Central South University, Changsha, China
- 3China-Africa Research Center of Infectious Diseases, Xiangya School of Medicine, Central South University, Changsha, China
Toxoplasma gondii infection is a severe health threat that endangers billions of people worldwide. T. gondii utilizes the host cell membrane to form a parasitophorous vacuole (PV), thereby fully isolating itself from the host cell cytoplasm and making intracellular clearance difficult. PV can be targeted and destroyed by autophagy. Autophagic targeting results in T. gondii killing via the fusion of autophagosomes and lysosomes. However, T. gondii has developed many strategies to suppress autophagic targeting. Accordingly, the interplay between host cell autophagy and T. gondii is an emerging area with important practical implications. By promoting the canonical autophagy pathway or attenuating the suppression of autophagic targeting, autophagy can be effectively utilized in the development of novel therapeutic strategies against T gondii. Here, we have illustrated the complex interplay between host cell mediated autophagy and T. gondii. Different strategies to promote autophagy in order to target the parasite have been elucidated. Besides, we have analyzed some potential new drug molecules from the DrugBank database using bioinformatics tools, which can modulate autophagy. Various challenges and opportunities focusing autophagy mediated T. gondii clearance have been discussed, which will provide new insights for the development of novel drugs against the parasite.
1. Introduction
Toxoplasma gondii (T. gondii), an intracellular protozoan parasite infectious to warm-blooded animals, is estimated to infect billions of people worldwide. As the causal pathogen of toxoplasmosis, T. gondii can lead to encephalitis and ocular diseases and may cause severe systemic infections in immunocompromised individuals, such as patients with cancer and AIDS (Halonen and Weiss, 2013). Owing to the high prevalence of T. gondii infection and the difficulty of specific elimination, the identification of suitable drugs is an urgent research goal. T. gondii resides and proliferates in the nucleated cells of the host. Structures like micronemes and rhoptries enable the parasites to bind to the host cell membrane, invade, and form the parasitophorous vacuole (PV), a niche encapsulating the parasite and separating from host cell cytoplasm by parasitophorous vacuole membrane (PVM). The Th1 type cellular immune response mediated by interferon-γ (IFN-γ) and interleukin-12 (IL-12) is the primary immune response against T. gondii (Suzuki et al., 1988; Sher et al., 2003), in which dendritic cells, CD8+ T cells, monocytes, and natural killer cells function as the major effectors in the different stages of T. gondii infection (Parker et al., 1991; Dunay et al., 2010; López-Yglesias et al., 2021). Immunological mechanisms to eliminate intracellular T. gondii include phagocytosis, cytotoxicity, the release of nitric oxide (NO) and cytokine-dependent pathways. Among these, autophagy induced by T. gondii has drawn substantial attention because of its parasiticidal effects.
Autophagy is a highly conserved process for the maintenance of cellular homeostasis and the elimination of damaged or harmful components in eukaryotic cells. As a self-digestive lysosomal degradation system, autophagy is also regarded as a part of the innate immune response defending against intracellular microbes, such as viruses, bacteria, and protozoa (Deretic et al., 2013). There are generally three types of autophagy: autophagosome-mediated macro-autophagy, micro-autophagy, and chaperone-mediated autophagy. Macro-autophagy inhibits T. gondii infection depending on the formation of a parasite-containing autophagosome and its fusion with lysosomes (Figure 1). The basic steps of macro-autophagy include (I) initiation: in which the assembly of the Unc-51-like kinase 1 (ULK1) complex initiates the pre-autophagosome structure formation; (II) nucleation-elongation-maturation: in which phosphatidylinositol 3-kinase catalytic subunit type 3 (PI3KC3) interacts with ULK1 to form phagophores, with the coupling of phosphatidylethanolamine (PE) and microtubule-associated protein light chain 3(LC3) facilitated by the autophagy protein (ATG) complex (ATG12-ATG5-ATG16), the phagophore membrane elongates and closes to form mature autophagosome; (III) fusion of the mature autophagosome and lysosome; (IV) degradation of engulfed contents for recycling uses or discarding (Cao et al., 2021). It is reported that CD40 is the key initiator of canonical autophagy against T. gondii infection (Andrade et al., 2006; Van Grol et al., 2013) in both hematopoietic cells (such as macrophages) and non-hematopoietic cells (such as fibroblasts and epithelial cells). Signaling molecules such as mammalian target of rapamycin (mTOR) and 5′ AMP-activated protein kinase (AMPK) function as important regulators during this process (Ling et al., 2006; Liu et al., 2016). Besides, IFN-γ mediated signaling pathways and the oxidative stress in host cells have synergistic effects with autophagy to eliminate T. gondii (Ohshima et al., 2014).
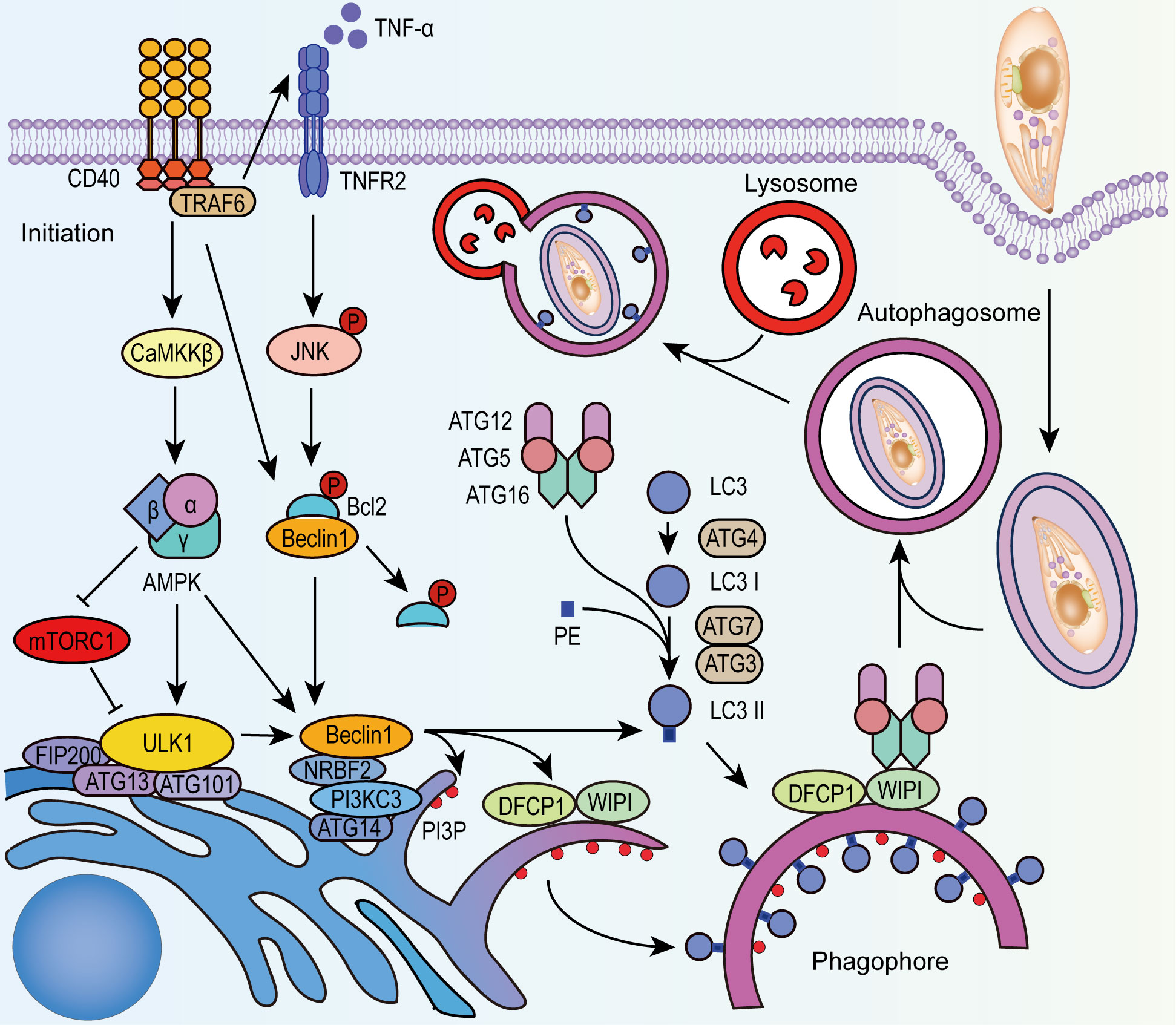
Figure 1 CD40 stimulates autophagy in T. gondii infection. After the invasion of T. gondii, CD40 activates ULK1 through CaMKKβ-AMPK signaling and releases Beclin1 from phosphorylated Bcl-2. AMPK also alleviates the negative regulation of mTORC1 to ULK1. CD40 promotes the activation of TNFR-JNK signaling through phosphorylation. Activated ULK1 complex and Beclin 1-PI3KC3 complex recruit PI3P, DFCP1, and WIPI to form phagophore. With the recruitment of LC3-II that is lipidated from LC3 by ATG4, ATG7, ATG3, and ATG12-ATG5-ATG16 complex, the phagophore expands and closes to form autophagosome that encapsulates PV of T. gondii, and fuses with lysosomes to degrade the parasites. PI3P, phosphatidylinositol-3-phosphate; NRBF2, nuclear receptor binding factor 2; AMBRA1, BECN1-regulated autophagy protein 1; DFCP1, double FYVE domain-containing protein 1; WIPI, WD-repeat protein interacting with phosphoinositides.
To avoid autophagic killing from the host, T. gondii has evolved strategies to manipulate host autophagy signalings. By excluding host proteins and inserting its own to the PVM, the extensively modified PV not only allows the transport of nutrients, but also protects T. gondii from host targeting and degradation (Lee et al., 2013). Meanwhile, rhoptry proteins (ROP), such as ROP5, ROP16 and ROP18, are released from the apical structure rhoptry during the invasion and located on the cytosolic side of the PVM, which inhibit the accumulation of host immunity related GTPases (IRGs) on PVM to protect PVM integrity (Hermanns et al., 2016). ROP16, released and translocated into the nucleus, affects host gene expression by intersecting host STAT pathway (Saeij et al., 2007). However, there is little evidence indicating ROPs could directly regulate autophagy, and the correlation between various ROPs and autophagy pathways needs further exploration. By phosphorylating the epidermal growth factor receptor (EGFR), T. gondii could alter autophagic targeting and suppress autophagy-mediated clearance (Muniz-Feliciano et al., 2013; Portillo et al., 2017). T. gondii could also exploit the endo-lysosomal system and recruit autophagosomes to provide nutrition, especially fatty acids, for its proliferation. Such interactions between T. gondii and host autophagy can be targeted for future drug development. A clear understanding of the parasiticidal mechanisms mediated by CD40 and IFN-γ and the interplay between host cells and T. gondii is expected to provide a basis for infection control. In this review, we present the mechanisms of autophagy during T. gondii infection. Targets to enhance host cell autophagy or prevent T. gondii from blocking autophagy process with potential therapeutic value are highlighted. With bioinformatics tools, candidate drugs targeting CD40/IFN-γ-related pathways for anti-toxoplasmosis therapy are also summarized from DrugBank database, which are a subject for further exploration.
2. T. gondii infection and current treatments
Being firstly identified in 1908, T. gondii is estimated to infect about one-third of the world’s population (Flegr et al., 2014). T. gondii is a zoonotic protozoan parasite belonging to genus Toxoplasma of Phylum Apicomplexa (Khan et al., 2005). In early studies, 106 T. gondii strains isolated from North America and Europe were initially classified into three classical clonal lineages, type I, II, and III, based on six marker genes (Howe et al., 1997). Further analyses using PCR-RFLP (polymerase chain reaction-restricted fragment length polymorphism) based on 10 marker genes have identified hundreds of atypical genotypes with differences in virulence and prevalence reported in Toxoplasma genome database. Type I shows greater lethality in mice and is associated with acute outbreaks. Type II is the dominant strain in Europe and North America and the most common strain isolated from clinical cases of toxoplasmosis (Howe et al., 1997).
Most patients infected by T. gondii are asymptomatic or present mild symptoms, but some are as severe as lethal toxoplasmosis, depending on host immune response (Montoya and Liesenfeld, 2004). In immunocompromised hosts, such as patients with AIDS/HIV, the newly acquired or the reactivated chronic infection can lead to severe encephalitis, chorioretinitis, or myocarditis (Rodgers and Harris, 1996; Eza and Lucas, 2006). Primary infection of T. gondii during pregnancy may result in abortion and congenital toxoplasmosis. It is noteworthy that T. gondii infection is related to psychiatric disorders in some subclinical cases, as numerous studies have shown the correlation between schizophrenia and T. gondii infection (Xiao et al., 2018). Chronic toxoplasmosis is an significant risk factor for schizophrenia, which might be attributed to the dysregulation of neuroendocrine and the neurotransmitter imbalance caused by chronic neuroinflammation, while psychiatric disorders also increase the exposure risk of T. gondii (Yin et al., 2022).
Since Sabin and Warren (1942) discovered the anti-toxoplasmosis effect of sulfonamides as the antagonist of folic acid, Eyles and Coleman subsequently reported the synergistic effects of sulfonamides and pyrimethamine, which have become the standard therapy for toxoplasmosis (Dubey, 2008). However, increasing drug resistance and serious adverse reactions of sulfonamides were reported (Shammaa et al., 2021), leading to an urgent need to develop new treatment methods. Spiramycin is used to reduce vertical transmission in pregnant women due to its low toxicity and impermeability to the blood-placenta barrier (Foulon et al., 1999). Atovaquone showed therapeutic potential in the salvage therapy of Toxoplasma encephalitis or in patients who could not tolerate the sulfonamides treatment (Torres et al., 1997). Clindamycin, clarithromycin, and azithromycin also serve as alternatives to sulfonamides therapy, but the efficacy of these alternative antibiotics remains controversial (Dunay et al., 2018). Furthermore, several vaccines have been reported to increase the immune response and survival rate in mice (Chu and Quan, 2021). However, substantial work is needed for clinical translation. To reduce the adverse reactions and drug resistance of anti-T. gondii therapies, it is essential to further explore the anti-T. gondii mechanisms and identify novel targets for T. gondii treatment.
3. The interplay of T. gondii and host cell autophagy
Autophagy is an important cellular mechanism to eliminate T. gondii and can be mediated by different signaling pathways. In T. gondii-infected host cells, autophagy is mainly stimulated by CD40. In addition, IFN-γ can regulate the growth or elimination of T. gondii by inducing an ATG-dependent signaling cascade (Sasai et al., 2018).
3.1 CD40 stimulates autophagy pathways in host cells infected by T. gondii
CD40 is a member of the tumor necrosis factor receptor (TNFR) superfamily that is expressed on both antigen-presenting cells and various non-hematopoietic cells. It contributes to cell-mediated immunity against T. gondii following the interaction with the ligand CD154 (Reichmann et al., 2000). In 2006, Andrade et al. found that CD40 could activate macrophages and alter the nonfusogenic nature of PVs, thereby inducing the autophagic degradation of T. gondii both in human and mouse cells (Andrade et al., 2006).
CD40 regulates autophagy in the infected cells via several pathways (Figure 1): (I)CD40 activates AMPK depending on Calcium/calmodulin-depend kinase kinase-β (CaMKKβ) resulting in the phosphorylation of ULK1 (Liu et al., 2016), and this process can be inhibited by mTOR complex1 (mTORC1). (II) CD40 stimulates the production of TNF-α, activates the MAPK/JNK signaling pathway and phosphorylates Bcl-2, contributing to the dissociation of Bcl-2 from Beclin 1-Bcl-2 complex (Liu et al., 2016). Similarly, CD40 downregulates the Beclin-degrading protein p21, which reduces Beclin 1 consumption (Portillo et al., 2010). (III)By binding to TNFR-associated factor, CD40 activates double-stranded RNA-dependent protein kinase (PKR) which phosphorylates eukaryotic initiation factor 2 (eIF2α) and finally stimulates autophagy in T. gondii-infected cells via several mechanisms (B’Chir et al., 2013). Exploring key molecules in these pathways and mechanisms of action will provide a basis for autophagy-targeting strategies.
3.2 IFN-γ restricts T. gondii growth through autophagy-independent effects of autophagy proteins
IFN-γ eliminates T. gondii by autophagy-independent mechanisms in cells infected by different strains. IFN-γ causes PV vesiculation and rupture rather than lysosomal degradation in mouse cells infected by type II and type III T. gondii strains (Selleck et al., 2015). Alternatively, IFN-γ has no significant effects on cells infected by type I strains, whose specific virulence factors, (e.g. ROP5, ROP17, and ROP18), can hinder the recruitment of IRGs induced by IFN-γ (Etheridge et al., 2014). In infected mouse macrophages and fibroblasts, selective autophagy proteins, such as ATG3, ATG7, and the ATG5-ATG12-ATG16L1 complex, are necessary to recruit guanylate binding proteins (GBPs) and IRGs (Zhao et al., 2009; Khetarpal and Rader, 2014; Sasai et al., 2017). Subsequently, IRG stimulates ubiquitin deposition around the PV and results in the destruction of the PVM. IRG also recruits GBPs in a ubiquitinated protein 62 (p62)-dependent manner and promotes the killing of T. gondii in the cytoplasm by GBPs (Figure 2A) (Ling et al., 2006).
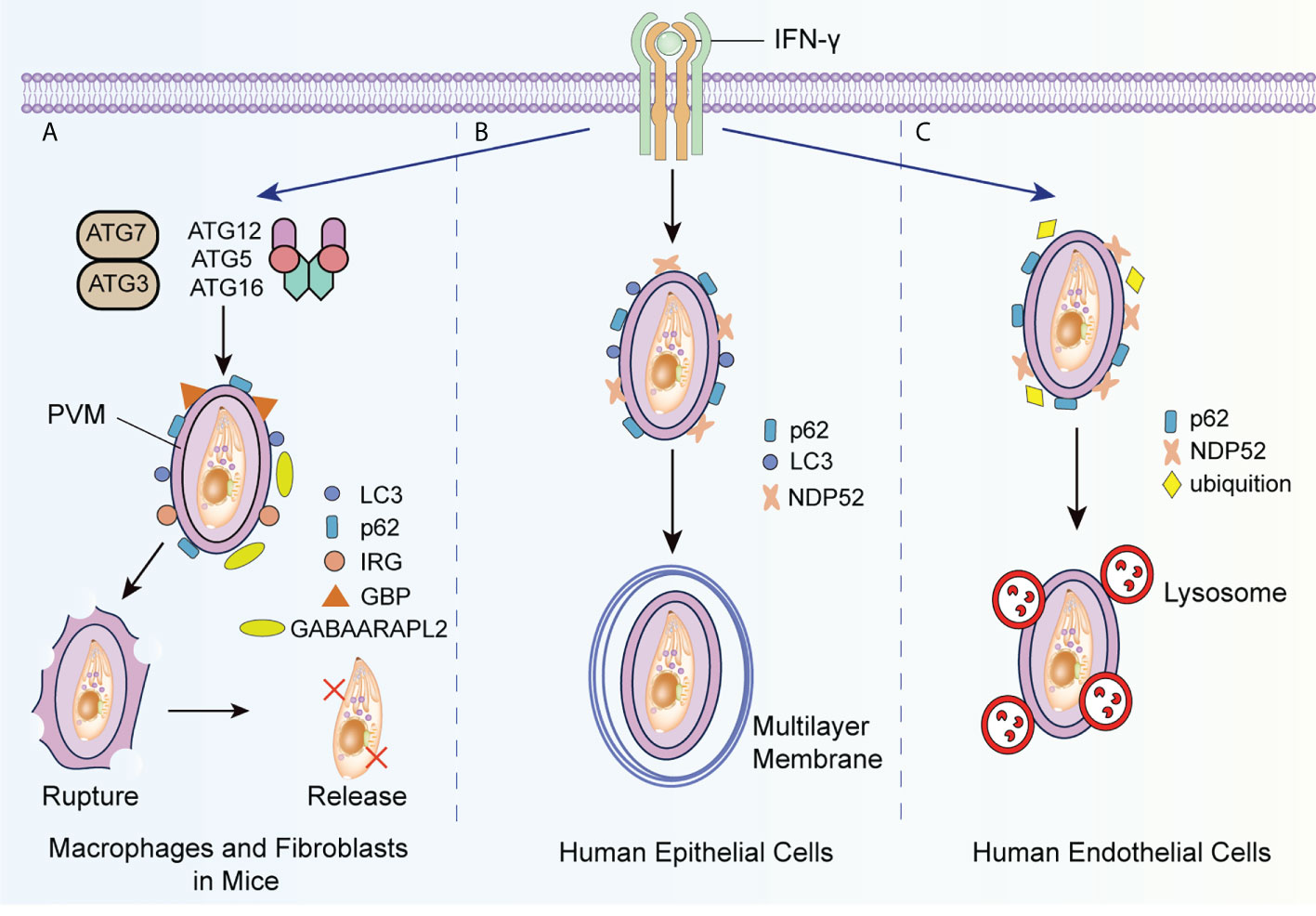
Figure 2 IFN-γ induced autophagic killing to T. gondii in different host cells. (A) IFN-γ promotes the recruitment of ATG3, ATG7 and ATG5-ATG12-ATG16L1 complex-dependent LC3, ATG8 homologs and ubiquitinated p62 to the PVM, and that of GBP and IRG to the PMV surface with the assistance of ATG8 homologs such as GABAARAPL2, causing the collapse of the PVM bubble and the release of T. gondii into the cytoplasm. (B) When type II and III T. gondii strains infect epithelial cells, IFN-γ recruits LC3, ubiquitinated p62, and ubiquitinated NDP52 to the PVM, and generates the multilayer membrane structure around the PVM to suppress the growth of T. gondii. (C) In human endothelial cells infected with T. gondii, IFN-γ promotes the recruitment of ubiquitinated p62 and NDP52 to the PVM and indues the fusion of lysosome with PV. GABAARAPL2, gamma-aminobutyric-A receptor-associated protein like 2.
Interestingly, in human cells, IFN-γ regulates the growth and elimination of T. gondii by other molecular pathways. In human epithelial cells infected with type II or type III strains, IFN-γ induces the deposition of p62, nuclear dot protein 52 (NDP52), and LC3 with ATG7 and ATG16L, and forms an encapsulated PV multilayer structure to suppress the growth of T. gondii (Figure 2B) (Selleck et al., 2015). Recently, Bhushan et al. argued that this process occurs under the regulation of IFN-stimulated genes (Bhushan et al., 2020). Nevertheless, in human endothelial cells infected by type II strains, ubiquitinated K63 is recruited by IFN-γ to induce the fusion of lysosomes and PVM to T. gondii (Clough et al., 2016; Subauste, 2021). NDP52 and p62 are subsequently recruited around PV in the absence of LC3 and ATG16L (Figure 2C).
3.3 T. gondii alters autophagy targeting via EGFR signaling
To avoid lysosomal degradation, T. gondii has developed strategies to change autophagy targeting via EGFR signaling. As shown in Figure 3, EGFR, expressed in various cells, is an important receptor to suppress cell autophagy (Purba et al., 2017). T. gondii leads to the phosphorylation of EGFR via parasitic proteins microneme (MIC) 3 and MIC6, followed by PI3K activation and PI3P accumulation (Muniz-Feliciano et al., 2013). PI3K activates the serine/threonine kinases Akt, which negatively regulates autophagy via the mTORC1 signaling pathway (Menon et al., 2014). Additionally, the MIC3 and MIC6 inhibit CD40-stimulated autophagy to protect parasites from autophagic degradation. Muniz-Feliciano et al. reported that parasites defective in MIC3 and MIC6 (MIC1 ko, deficient in MIC1 and secretion of MIC6; MIC3 ko, deficient in MIC3; and MIC1-3 ko, deficient in MIC1, MIC3 and secretion of MIC6) show impaired EGFR-Akt activation, indicating the dominant role of MIC3 and MIC6 in the autophagy-mediated suppression of T. gondii (Muniz-Feliciano et al., 2013).
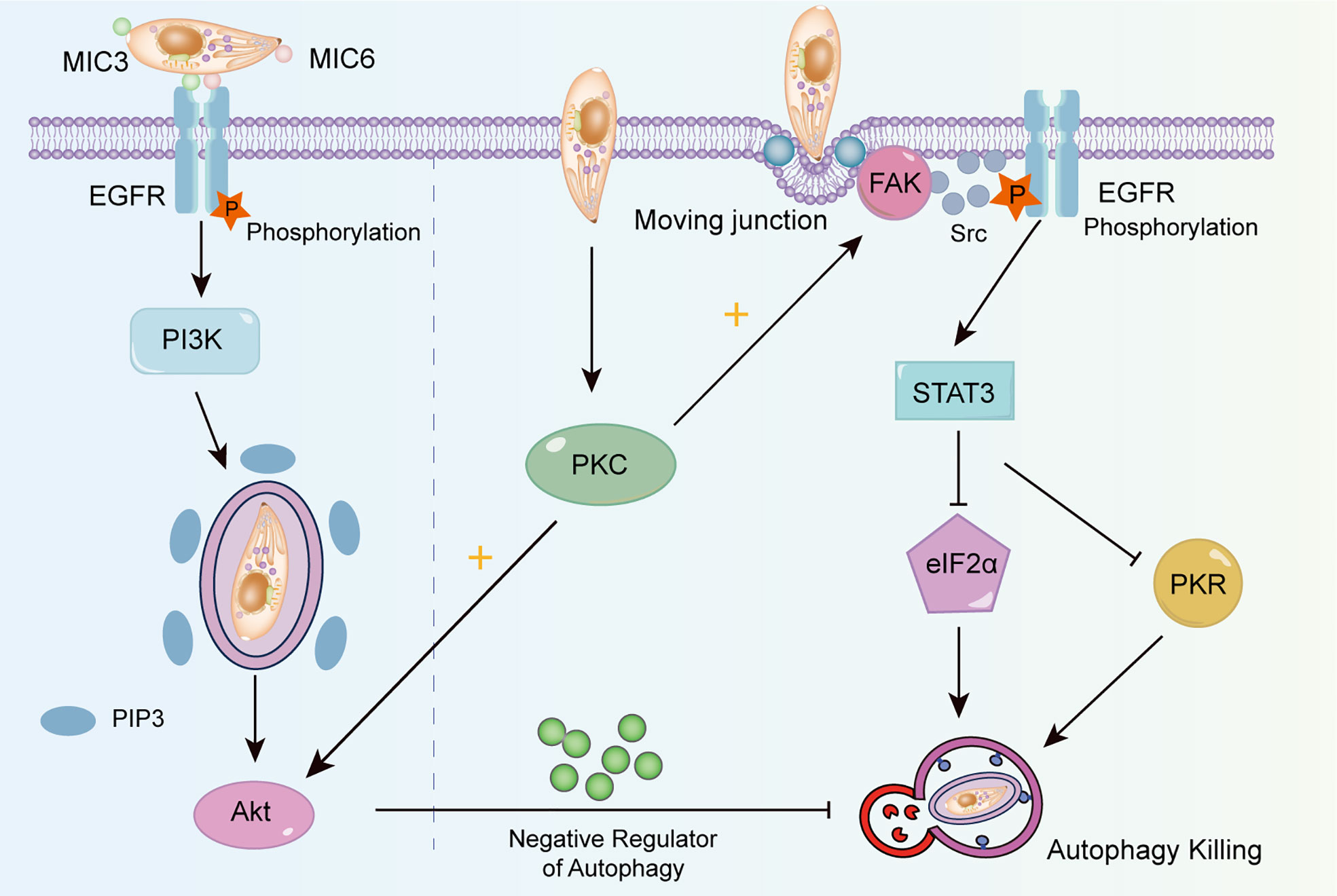
Figure 3 The mechanism of T. gondii changing autophagy targeting. T. gondii induces EGFR autophosphorylation through MIC3 and MIC6, triggers PI3K to produce PIP3, and then activates Akt to inhibit autophagy by producing negative regulators. The activation of FAK depends on the mobile connection formed when T. gondii invades. Activated FAK induces the transactivation of Src-dependent EGFR, and activates STAT3, thus suppressing PKR and eIF2α signaling. T. gondii can also maintain the blockade of intracellular autophagy targets by activating PKC, which helps to maintain Src signaling, EGFR autophosphorylation and Akt activation.
Another signaling cascade activated by T. gondii can be utilized to avoid autophagic degradation depending on the focal adhesion kinase (FAK)/Src signal (Figure 3). When T. gondii invades cells and forms the moving junctions (Biscardi et al., 1999), FAK is phosphorylated, which in turn activates Src. This process induces the transactivation of EGFR (phospho Y845) independent of MICs (Portillo et al., 2017). Lastly, signal transducer and activator of transcription 3 (STAT3) is activated and suppresses downstream pro-autophagic PKR/eIF2α signaling (Hunter, 2011; Ullmann et al., 2013). A study has revealed that T. gondii activates protein kinase C (PKC) α/β to maintain the long-term activation of Src-EGFR signaling, thus effectively suppressing autophagy (Lopez Corcino et al., 2019).
4. Promising targets in autophagy pathway for T. gondii infection
It is widely accepted that stimulating autophagy ameliorates T. gondii infection and prevents further invasion. Current autophagy-promoting strategies can be mainly categorized into three types: promoting canonical autophagy pathway, correcting autophagy targeting, and facilitating oxidative stress to induce autophagy. Key studies and relevant mechanisms related to these strategies are summarized below.
4.1 Promoting canonical autophagy
Canonical autophagy signaling plays a key role in controlling host cell survival during T. gondii infection. It is quite possible to interfere with the replication and pathogenic effects of T. gondii by regulating the canonical autophagy pathway (Besteiro, 2019), in which AMPK and mTORC1 are remarkable targets.
4.1.1 AMPK
The anti-T. gondii effect of AMPK has been widely reported. Several lines of evidence indicate that the regulation of AMPK is associated with the anti-T. gondii property of omega-3 polyunsaturated fatty acids (ω3-PUFAs), primarily docosahexaenoic acid (DHA). As early as 2014, Williams-Bey et al. reported that DHA promotes macrophage autophagy and thus suppresses inflammasome production (Shi et al., 2012; Williams-Bey et al., 2014). Given this, Choi et al. treated murine bone marrow-derived macrophages with DHA and found that DHA effectively enhanced the colocalization of Toxoplasma parasitophorous vacuoles and autophagosomes, resulting in an effective antiparasitic response (Choi et al., 2019). DHA increased the phosphorylation of AMPK, CaMKKβ, and LKB1, and specific AMPK inhibitors abrogated DHA-induce autophagy (Choi et al., 2019). Similar results were found in vitro, as endogenous ω3-PUFAs stimulated autophagy in bone marrow-derived macrophages, and even decreased the tissue cyst counts in the brain (Choi et al., 2019). Although ω3-PUFAs exhibited excellent antiparasitic effects in mice both in vitro and in vivo, the exact effects on humans need further investigation. AMPK probably contributes to the anti-T. gondii effects of statins. Although statins are well known for interfering with isoprenoid synthesis (Cortez et al., 2009; Li et al., 2013), emerging studies have demonstrated that they function as autophagy stimulators by activating AMPK and upregulating lysosomal-associated membrane protein 1 (Piplani et al., 2019). However, the role of AMPK in the effect of simvastatin has not been reported in T. gondii infection. Determining the relation between the antiparasitic efficacy of statins and autophagy regulation may provide new insights into the anti-T. gondii effects of statins (Parihar et al., 2014; Martín-Navarro et al., 2015).
4.1.2 mTOR/mTORC1
As a key negative regulator of the canonical autophagy pathway, the mTOR/mTORC1 interaction has been studied extensively. The mTOR inhibitors have been widely applied in the studies aimed at enhancing autophagy (Andrade et al., 2006). Andrade and colleagues revealed that autophagy is increased in mouse macrophages treated with rapamycin, a classic mTOR inhibitor, resulting in T. gondii elimination in mammalian cells (Andrade et al., 2006). The mTOR inhibitor rottlerin was also shown to promote autophagy in the choriocarcinoma-derived cell line BeWo, which significantly suppressed intracellular T. gondii proliferation under sub-toxic concentrations (Ietta et al., 2017).
4.2 Correcting autophagy targeting
Accumulating research has highlighted the ability of T. gondii to change autophagy targeting and avoid autophagic killing (Portillo et al., 2017; Lopez Corcino et al., 2019) indicating that host-directed treatment is a plausible strategy for T. gondii elimination. EGFR/PI3K/AKT participates in many fundamental intracellular pathways and is an important regulator in the survival of some protozoan parasites (Penas et al., 2019; Phan et al., 2020). EGFR has emerged as a critical initial molecule in altering autophagy targeting, and EGFR targeting with EGFR tyrosine kinase inhibitors (TKIs) is a widely explored strategy. For instance, gefitinib, an EGFR TKIs with antineoplastic properties, has been applied to investigate anti-T. gondii activity by correcting the suppression of autophagy targeting (Lopez Corcino et al., 2019). Gefitinib promotes autophagic killing of T. gondii by inhibiting the EGFR/PI3K/AKT pathway, and this process is Beclin1-dependent (Lopez Corcino et al., 2019). It was further shown that the antiparasitic activity of gefitinib primarily relies on direct killing, rather than the inhibition of intracellular replication. The pharmacologic efficacy of TKIs was further evaluated in vivo, revealing that a relatively low dose of gefitinib could relieve cerebral toxoplasmosis in mice and control the disease process, with similar results obtained for ocular toxoplasmosis (Lopez Corcino et al., 2019). In addition to regulating autophagy, PI3K/AKT signaling may exert therapeutic effects via the regulation of apoptosis or cellular stress to eliminate T. gondii (Xu et al., 2020; Yan et al., 2021).
4.3 Facilitating oxidative stress
Oxidative stress in the host cells is toxic to parasites, at the same time, it has deleterious consequences on the host (Zhuang et al., 2020). The immune response evoked by parasite infection induces the production of reactive oxygen species (ROS) which suppresses the activity of T. gondii in monocytes of infected animals. For instance, a high concentration of H2O2 can suppress the intracellular proliferation of tachyzoites (Szewczyk-Golec et al., 2021). In one study, resveratrol significantly limited the growth of RH tachyzoites by disrupting the redox homeostasis of parasites. Moreover, the inhibitory effects of resveratrol include releasing cellular stress, promoting apoptosis, maintaining the autophagic status of macrophages, and finally, promoting the eradication of intracellular tachyzoites by activating macrophages (Chen et al., 2019). T. gondii thioredoxin reductase, superoxide dismutases (SODs) (Ding et al., 2004), Catalase (CAT), and three peroxiredoxins have antioxidant functions (Akerman and Müller, 2005; Deponte and Becker, 2005; Xue et al., 2017), and enable persistent infection. ROS are intensively produced in response to the early stage of the acute phase of T. gondii infection, inducing oxidative stress in the tissues of the host as the defense mechanism. In the later stages of the acute phase of infection, accumulated SOD downregulate the level of ROS (Figure 4).
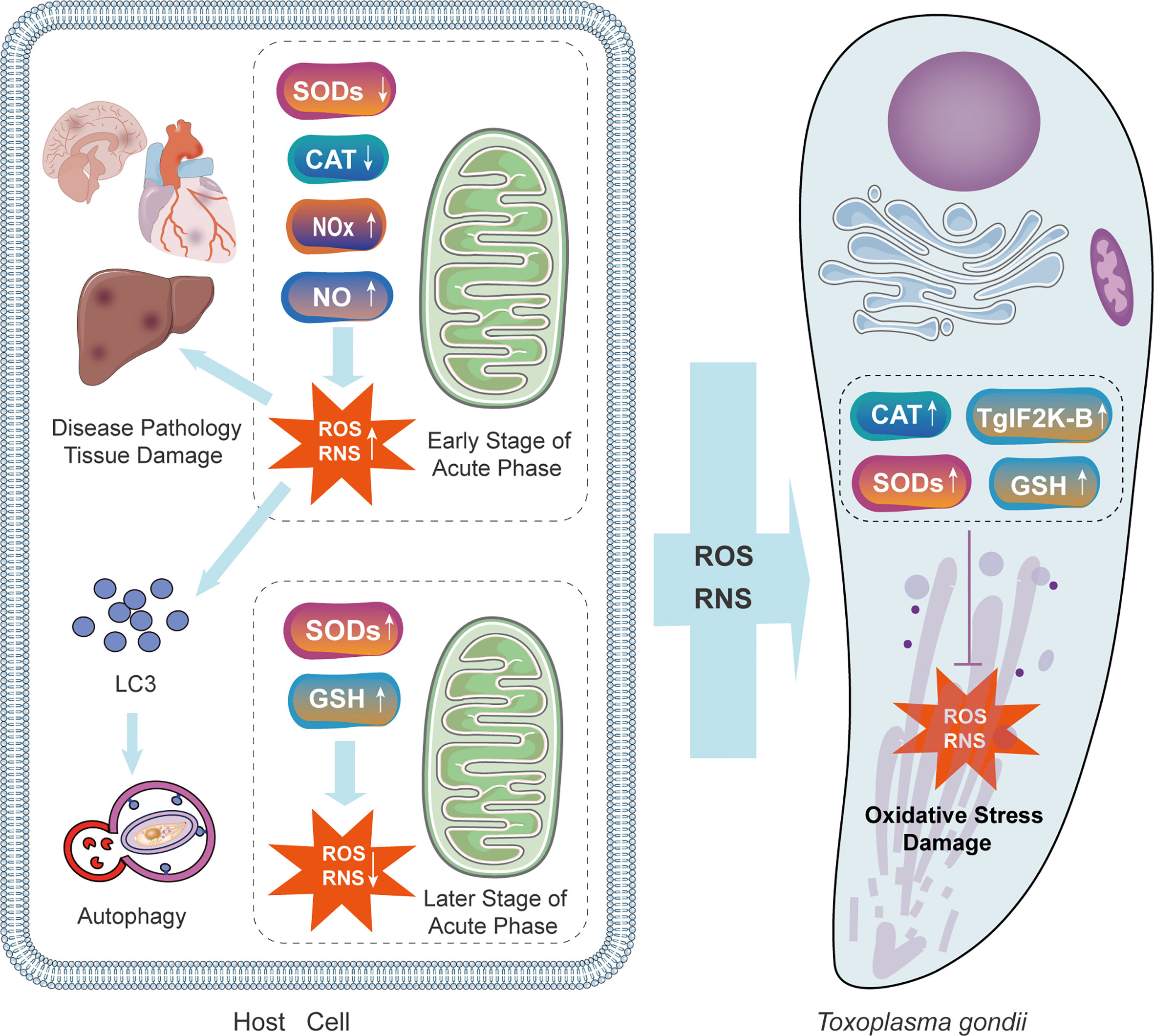
Figure 4 The mechanisms of oxidative injury and antioxidant defense during T. gondii infection in the parasite and the host. T. gondii infection causes immune system reaction of the host. In the early stage, the concentration of NOx and NO is raised, increasing the level of ROS and RNS. Oxidative stress caused by the host response is toxic both to parasites and the host itself. Increased ROS in host cells recruits LC3 and stimulates autophagy. In the later stage, levels of SOD and GSH are up-regulated due to the antioxidant defense of the host. Simultaneously, T. gondii expresses CAT, TgIF2K-B, SODs, and GSH to protect itself. GSH, glutathione; NOx, NADPH oxidase complex; TgIF2K-B, T. gondii eIF2α kinase.
Autophagy is a catabolic process in response to different stress conditions Increasing evidence has demonstrated that oxidative stress results from a wide range of stimuli, with ROS and reactive nitrogen species (RNS) acting as the dominant intracellular signal transducers initiating autophagy (Filomeni et al., 2015). Increased ROS and RNS can recruit LC3 to autophagosomes and initiate autophagy (Figure 4). A certain number of studies have indicated that by facilitating oxidative stress, autophagy is a potential therapeutic target for T. gondii. Therefore, researchers are committed to exploring the relationship between oxidative stress and autophagic killing in T. gondii infection and its practical application. Methods to facilitate oxidative stress is worthy of future research. In one study myrislignan originating from nutmeg induced a redox imbalance in T. gondii, which led to the autophagic killing of T. gondii (Zhang et al., 2021a). With respect to the underlying mechanisms, myrislignan can lower the mitochondrial membrane potential as well as ATP levels in T. gondii and disrupt mitochondrial function (Zhang et al., 2021a). In another study, Jili-Zhang et al. found that licarin-B from nutmeg exhibits excellent anti-T. gondii activity by inducing mitochondrial swelling and nuclear disintegration (Zhang et al., 2021b). Consequently, licarin-B damages mitochondria and activates autophagy to kill T. gondii (Zhang et al., 2021b). Moreover, kijimicin decreases mitochondrial membrane potential and ROS production in T. gondii, thus inhibiting T. gondii growth (Leesombun et al., 2022). However, owing to the limited number of studies, our understanding of methods targeting oxidative stress is incomplete.
Moreover, oxidative stress caused by the host immune response exerts negative effects on the host. It is believed that antioxidant defense response of T. gondii helps host cells survive the attack. Consequently, antioxidants such as tryptanthrin, melatonin, and some vitamins, have been evaluated as anti-T. gondii therapeutics. Moon et al. demonstrated that tryptanthrin pre-treatment reduces liver cell damage by effectively decreasing ROS levels and mitochondrial dysfunction (Moon et al., 2014). Majumdar et al. reported that melatonin reduces H2O2 levels produced in the host cells via tert-butyl hydroperoxide. As a result, the survival of infected cells was prolonged (Majumdar et al., 2019). These studies support the beneficial effects of antioxidants for the treatment of toxoplasmosis. Further research is of great necessity to estimate the feasibility and safety of this therapy. However, research in this field is likely to have far-reaching implications for toxoplasmosis therapy.
4.4 Other mechanisms
In addition to the targeting mechanisms mentioned above, other promising targets with similar anti-T. gondii effects by affecting autophagy have been reported. Prior studies have shown that sirtuin-1 (SIRT1), a nicotine adenine dinucleotide-dependent protein deacetylase, could regulate autophagy activation (Ng and Tang, 2013). Lee and colleagues have found that the regulation of SIRT1 protein expression may exert an anti-T. gondii effect via autophagy. This phenomenon can be reversed by selective SIRT1 inhibitors (Lee et al., 2020). In another study, autophagy was enhanced by activating MutS DNA damage repair enzyme (TgMSH-1)-mediated cell cycle arrest and affecting the energy supply of the host (Lavine and Arrizabalaga, 2012). The recruitment of LC3 and ATG8 orthologs on PVM is necessary for autophagosome formation, and ATG8 labelled with a green fluorescent protein (GFP) at its amino terminus has emerged as a useful indicator of autophagy levels (Besteiro et al., 2011; Subauste, 2021). Increasing the combination of LC3-GFP and PV limits the growth of parasites by inducing the autophagic degradation of PV (Dittmar et al., 2016). Varberg et al. found that a high concentration of Plasmodium ATG3-ATG8 interaction inhibitors can promote the lipidation of ATG8, thereby stimulating autophagy in T. gondii-infected cells. Interestingly, inhibitors of the ATG3-ATG8 interaction exert opposite effects on P. falciparum-infected human hepatocytes (Hain et al., 2014; Varberg et al., 2018). Decreased incidence rate and improved clinical course of Toxoplasma encephalitis have been obtained by introducing protease inhibitors to the HIV treatment regimen (HIV-PIs) (Abou-El-Naga et al., 2017). Heat shock proteins (HSP), involved in the maintenance of protein homeostasis, are also potential targets for suppressing T. gondii. Our earlier research has shown that the downregulation of HSP29 expression levels may contribute to T. gondii elimination (Liu et al., 2021). Inhibiting HSP70 could significantly dysregulate host cell autophagy and decrease the reproduction of T. gondii (Mitra et al., 2021). Taken together, in addition to affecting common autophagy pathways, various candidate targets able to influence host cell autophagy to suppress T. gondii infection have been identified.
5. Potential drugs or chemicals promoting autophagy
Current mechanisms of promoting autophagy for anti-T. gondii therapy have been summarized, and we have also identified new drugs or chemicals targeting CD40 and IFN-γ to enhance autophagy with the bioinformatics approaches. Using the DrugBank database (https://go.drugbank.com/), we revealed several drugs targeting IFN-γ, and the primary agents have been summarized in Table 1. Surface antigen SAG1 (P30) serves as a type of major surface protein of T. gondii tachyzoites. SAG1 is inserted in the plasma membrane of T. gondii with a glycosyl-phosphatidylinositol anchor. Two glycoforms are included in the key anchor structure of the protein: Man-alpha1,2-Man-alpha1,6-Man-[GalNAc-beta1,4-]-alpha1,4-GlcN-PI and Man-alpha1,2 -Man-alpha1,6-Man [Glc-alpha1,4 -GalNAc-beta1,4-]-alpha1,4-GlcN-PI. Also, glucosamine blocks glycosyl interactions in T. gondii infection, thereby contributing to the acute infection of T. gondii tachyzoites (Zinecker et al., 2001). This was confirmed by an in vitro attachment assay, where soluble BSA-glucosamine blocks the attachment of SAG1 to Mabin-Darby bovine kidney cells (Robinson et al., 2004). However, the immune mechanism differs between the acute infection phase and the chronic infection phase (Robinson et al., 2004). Unfortunately, the pharmacological role of monosaccharides in T. gondii infection is unclear, as the nine monosaccharides making up the cell surface carbohydrates do not show clear inhibitory effects on tachyzoites infecting bovine embryonic skin and muscle cells (Crane and Dvorak, 1982). These results indicated that glucosamine might interfere with T. gondii infection in another form, and its mechanism of action may involve IFN-γ, which is a product of Th1/Th2 polarization in response to SAG1 antigen stimulation and facilitates the cascade immune response (Khan et al., 1988; Caetano et al., 2006; Lakhrif et al., 2018; Choi and Park, 2020).
In addition, we found that olsalazine, VIR201, and fontolizumab in the DrugBank database are predicted to interact with IFN-γ. Olsalazine increases the production of arachidonic acid metabolites via the cyclooxygenase pathway (i.e., prostaglandins) and lipoxygenase pathway (i.e., leukotrienes and hydroxyeicosatetraenoic acids) in patients with chronic inflammatory bowel disease, and mesalazine may reduce inflammation by blocking cyclooxygenase and inhibiting prostaglandin production in the colon. This anti-inflammatory effect of olsalazine may be related to the function of IFN-γ. VIR201 is a DNA vaccine, the second component of which is designed to increase levels of IFN-γ and improve immune function. Fontolizumab is a humanized antibody against recombinant human IFN-γ. The antibody binds to natural human IFN-γ and inhibits the expression of IFN-γ regulatory genes known to be upregulated in Crohn’s disease. Although no clear anti-T. gondii pharmacological effects have been reported to date, these agents should be prioritized for compound screening. High-throughput compound screening studies have shown that anti-T. gondii compounds with low concentrations and low levels of IFN-γ evaluated by in vitro parasite growth inhibition assays were likely to affect autophagy-related molecules such as LC3 (Radke et al., 2018). Therefore, focusing on autophagy-related molecules is the next step in the discovery of drugs that have synergistic effects with IFN-γ.
We were unable to identify drugs targeting CD40; however, many compounds inhibit both IFN-γ and CD40 and are potential anti-T. gondii drugs (see Table S1 for a summary of specific interactions of each agent). These potential compounds that interfere with CD40 could serve as molecular manipulation tools to clarify the role of CD40 in the autophagy of T. gondii-infected cells and to identify compounds with therapeutic effects. Of note, simvastatin is among the compounds that may affect both IFN-γ and CD40. Although some reports have indicated that simvastatin inhibits T. gondii adherence, invasion, and proliferation in HeLa cells (Sanfelice et al., 2017; Sanfelice et al., 2019), the pharmacological mechanism of action has not been determined. Paclitaxel (dihydroquercetin) and a dihydrofolate inhibitor (ethacrynic acid) suppress the growth of rapidly proliferating T. gondii RH strains in vitro (Abugri et al., 2018). Curcumin is also an active ingredient in Chinese herbal medicine that inhibits the growth of tachyzoites in vitro (Saki et al., 2020). It could also reduce DNA methylation induced by T. gondii (Saki et al., 2020), and is a potentially effective agent for chronic T. gondii infection (Azami et al., 2018; El-Shafey et al., 2020). These compounds have varying degrees of efficacy against T. gondii; however, the specific mechanisms of action are not clearly defined, hindering their clinical application. Since IFN-γ and CD40 are central components in the autophagy-related mechanism underlying anti-T. gondii infection, future studies should evaluate the anti-T. gondii pharmacological effects of these plant-derived compounds targeting IFN-γ and CD40.
6. Challenges and opportunities
Autophagy singling exerts complex interactions with T. gondii survival, and accumulating studies have focused on autophagy as a target to eliminate T. gondii. However, established strategies are generally confined to activating autophagy via chemical substances, without sufficient consideration of T. gondii-mediated autophagy suppression. Thus, limited and unstable effects have been obtained.
Also, future autophagy-promoting anti-T. gondii drug research should consider strategies that incorporate highly specific key proteins. For example, AR12, which induces autophagy and reduces EGFR expression in SARS-COV-2-infected Vero cells, may have similar effects on T. gondii infection (Rayner et al., 2020). Reported drugs do not cover all inhibitors of potential targets, and ATG4, as the only protease of the core mammalian autophagy proteins, is closely associated with cancer, neurodegeneration, and microbial infection. Various in vivo and in vitro methods to detect ATG4 activity have been established in the last decade (Li et al., 2011; Fu et al., 2019). Moreover, ATGB is a potent target for suppressing T. gondii, as evidenced by the antiproliferative effects of an ATG4B agonist in MDA-MB-231 cells (Pengo et al., 2018). Anti-T. gondii therapies targeting ATG4 are promising and should be a focus of basic research and clinical trials.
Since T. gondii has a long history of coexistence and antagonistic relationships with human hosts, therapies targeting autophagy should consider the evolution of T. gondii Strategies aimed at the complete removal of T. gondii intracellularly or in vivo for a certain period using methods independent of autophagy modulation can lead to drug resistance and the development of super-virulent T. gondii. To avoid this problem, monitoring genetic changes in T. gondii and the use of multi-targeted agents are important strategies. Additionally, a shift in emphasis from killing T. gondii to the removal of T. gondii pathogenicity may provide a basis for the next generation of anti-T. gondii therapies. To this end, further elucidation of the mechanisms underlying T. gondii pathogenesis will be the key to the development of novel treatment strategies.
7. Conclusion
Given the important antiparasitic effects of autophagy, it is not surprising that modulation of autophagy serves as a therapeutic strategy for T. gondii infection. Autophagy-related molecules, including CD40, IFN-γ, EGFR, mTORC1, and AMPK, as well as oxidative stress are potential targets. A series of drugs and chemicals have been developed to promote autophagy against T. gondii infection, with clearly demonstrated pharmacological value. However, these strategies are still in their early stages, and more precise targets and better assessments of pharmacological effects are needed. In addition, the possibility of drug resistance should be evaluated in future studies.
Author contributions
AC: Conceptualization, Writing—original draft preparation, review and editing, Project administration. HZ, BC, SZ, HW, YS, and SY contributed to the paper writing and revisions. LJ and ML: Conceptualization, Writing—review and editing, Project administration, Funding acquisition. All authors have read and agreed to the published version of the manuscript.
Funding
This work was supported by the National Natural Science Foundation of China (grant number 32170510), the Natural Science Foundation of Hunan Province, China (grant number 2020JJ4765), the Open Sharing Fund for the Large-scale Instruments and Equipment of Central South University (grant number CSUZC202236), Science and Technology Program of Hunan Province (project number 2021ZK4154), Graduate Case base construction project of Central South University (project number 2020ALK91), and Innovation Training Program of Central South University (project number 20220026020009).
Acknowledgments
We would like to thank Editage (www.editage.cn) for English language editing.
Conflict of interest
The authors declare that the research was conducted in the absence of any commercial or financial relationships that could be construed as a potential conflict of interest.
Publisher’s note
All claims expressed in this article are solely those of the authors and do not necessarily represent those of their affiliated organizations, or those of the publisher, the editors and the reviewers. Any product that may be evaluated in this article, or claim that may be made by its manufacturer, is not guaranteed or endorsed by the publisher.
Supplementary material
The Supplementary Material for this article can be found online at: https://www.frontiersin.org/articles/10.3389/fcimb.2022.902428/full#supplementary-material
References
Abou-El-Naga, I. F., El Kerdany, E. D., Mady, R. F., Shalaby, T. I., Zaytoun, E. M. (2017). The effect of lopinavir/ritonavir and lopinavir/ritonavir loaded PLGA nanoparticles on experimental toxoplasmosis. Parasitol. Int. 66 (6), 735–747. doi: 10.1016/j.parint.2017.08.007
Abugri, D. A., Witola, W. H., Russell, A. E., Troy, R. M. (2018). In vitro activity of the interaction between taxifolin (dihydroquercetin) and pyrimethamine against toxoplasma gondii. Chem. Biol. Drug design 91 (1), 194–201. doi: 10.1111/cbdd.13070
Akerman, S. E., Müller, S. (2005). Peroxiredoxin-linked detoxification of hydroperoxides in toxoplasma gondii. J. Biol. Chem. 280 (1), 564–570. doi: 10.1074/jbc.M406367200
Andrade, R. M., Wessendarp, M., Gubbels, M.-J., Striepen, B., Subauste, C. S. (2006). CD40 induces macrophage anti-toxoplasma gondii activity by triggering autophagy-dependent fusion of pathogen-containing vacuoles and lysosomes. J. Clin. Invest. 116 (9), 2366–2377. doi: 10.1172/JCI28796
Azami, S. J., Teimouri, A., Keshavarz, H., Amani, A., Esmaeili, F., Hasanpour, H., et al. (2018). Curcumin nanoemulsion as a novel chemical for the treatment of acute and chronic toxoplasmosis in mice. Int. J. nanomed. 13, 7363–7374. doi: 10.2147/IJN.S181896
B’Chir, W., Maurin, A.-C., Carraro, V., Averous, J., Jousse, C., Muranishi, Y., et al. (2013). The eIF2α/ATF4 pathway is essential for stress-induced autophagy gene expression. Nucleic Acids Res. 41 (16), 7683–7699. doi: 10.1093/nar/gkt563
Besteiro, S. (2019). The role of host autophagy machinery in controlling infection. Virulence 10 (1), 438–447. doi: 10.1080/21505594.2018.1518102
Besteiro, S., Brooks, C. F., Striepen, B., Dubremetz, J.-F. (2011). Autophagy protein Atg3 is essential for maintaining mitochondrial integrity and for normal intracellular development of toxoplasma gondii tachyzoites. PloS Pathog. 7 (12), e1002416. doi: 10.1371/journal.ppat.1002416
Bhushan, J., Radke, J. B., Perng, Y.-C., McAllaster, M., Lenschow, D. J., Virgin, H. W., et al. (2020). ISG15 connects autophagy and IFN-γ-Dependent control of toxoplasma gondii infection in human cells. mBio 11 (5), e00852–20. doi: 10.1128/mBio.00852-20
Biscardi, J. S., Maa, M. C., Tice, D. A., Cox, M. E., Leu, T. H., Parsons, S. J. (1999). C-src-mediated phosphorylation of the epidermal growth factor receptor on Tyr845 and Tyr1101 is associated with modulation of receptor function. J. Biol. Chem. 274 (12), 8335–8343. doi: 10.1074/jbc.274.12.8335
Caetano, B. C., Bruña-Romero, O., Fux, B., Mendes, E. A., Penido, M. L. O., Gazzinelli, R. T. (2006). Vaccination with replication-deficient recombinant adenoviruses encoding the main surface antigens of toxoplasma gondii induces immune response and protection against infection in mice. Hum. Gene Ther. 17 (4), 415–426. doi: 10.1089/hum.2006.17.415
Cao, W., Li, J., Yang, K., Cao, D. (2021). An overview of autophagy: Mechanism, regulation and research progress. Bull. du Cancer 108 (3), 304–322. doi: 10.1016/j.bulcan.2020.11.004
Chen, Q.-W., Dong, K., Qin, H.-X., Yang, Y.-K., He, J.-L., Li, J., et al. (2019). Direct and indirect inhibition effects of resveratrol against tachyzoites. Antimicrob. Agents chemother. 63 (3), e01233–18 doi: 10.1128/AAC.01233-18
Choi, J.-W., Lee, J., Lee, J.-H., Park, B.-J., Lee, E. J., Shin, S., et al. (2019). Omega-3 polyunsaturated fatty acids prevent infection by inducing autophagy via AMPK activation. Nutrients 11 (9), 2137. doi: 10.3390/nu11092137
Choi, W. H., Park, J. S. (2020). Immunogenicity and protective effect of a virus-like particle containing the SAG1 antigen of as a potential vaccine candidate for toxoplasmosis. Biomedicines 8 (4), 91. doi: 10.3390/biomedicines8040091
Chu, K.-B., Quan, F.-S. (2021). Advances in vaccines: Current strategies and challenges for vaccine development. Vaccines 9 (5), 413. doi: 10.3390/vaccines9050413
Clough, B., Wright, J. D., Pereira, P. M., Hirst, E. M., Johnston, A. C., Henriques, R., et al. (2016). K63-linked ubiquitination targets toxoplasma gondii for endo-lysosomal destruction in IFNγ-stimulated human cells. PloS Pathog. 12 (11), e1006027. doi: 10.1371/journal.ppat.1006027
Cortez, E., Stumbo, A. C., Oliveira, M., Barbosa, H. S., Carvalho, L. (2009). Statins inhibit toxoplasma gondii multiplication in macrophages in vitro. Int. J. antimicrob. Agents 33 (2), 185–186. doi: 10.1016/j.ijantimicag.2008.07.026
Crane, M. S., Dvorak, J. A. (1982). Influence of monosaccharides on the infection of vertebrate cells by trypanosoma cruzi and toxoplasma gondii. Mol. Biochem. Parasitol. 5 (5), 333–341. doi: 10.1016/0166-6851(82)90040-8
Deponte, M., Becker, K. (2005). Biochemical characterization of toxoplasma gondii 1-cys peroxiredoxin 2 with mechanistic similarities to typical 2-cys prx. Mol. Biochem. Parasitol. 140 (1), 87–96. doi: 10.1016/j.molbiopara.2004.12.008
Deretic, V., Saitoh, T., Akira, S. (2013). Autophagy in infection, inflammation and immunity. Nat. Rev. Immunol. 13 (10), 722–737. doi: 10.1038/nri3532
Ding, M., Kwok, L. Y., Schlüter, D., Clayton, C., Soldati, D. (2004). The antioxidant systems in toxoplasma gondii and the role of cytosolic catalase in defence against oxidative injury. Mol. Microbiol. 51 (1), 47–61. doi: 10.1046/j.1365-2958.2003.03823.x
Dittmar, A. J., Drozda, A. A., Blader, I. J. (2016). Drug repurposing screening identifies novel compounds that effectively inhibit toxoplasma gondii growth. mSphere 1 (2), e00042–15. doi: 10.1128/mSphere.00042-15
Dubey, J. P. (2008). The history of toxoplasma gondii–the first 100 years. J. eukaryotic Microbiol. 55 (6), 467–475. doi: 10.1111/j.1550-7408.2008.00345.x
Dunay, I. R., Fuchs, A., Sibley, L. D. (2010). Inflammatory monocytes but not neutrophils are necessary to control infection with toxoplasma gondii in mice. Infection Immun. 78 (4), 1564–1570. doi: 10.1128/IAI.00472-09
Dunay, I. R., Gajurel, K., Dhakal, R., Liesenfeld, O., Montoya, J. G. (2018). Treatment of toxoplasmosis: Historical perspective, animal models, and current clinical practice. Clin. Microbiol. Rev. 31 (4), e00057–17. doi: 10.1128/CMR.00057-17
El-Shafey, A. A. M., Hegab, M. H. A., Seliem, M. M. E., Barakat, A. M. A., Mostafa, N. E., Abdel-Maksoud, H. A., et al. (2020). Curcumin@metal organic frameworks nano-composite for treatment of chronic toxoplasmosis. J. materials science. Materials Med. 31 (11), 90. doi: 10.1007/s10856-020-06429-y
Etheridge, R. D., Alaganan, A., Tang, K., Lou, H. J., Turk, B. E., Sibley, L. D. (2014). The toxoplasma pseudokinase ROP5 forms complexes with ROP18 and ROP17 kinases that synergize to control acute virulence in mice. Cell Host Microbe 15 (5), 537–550. doi: 10.1016/j.chom.2014.04.002
Eza, D. E., Lucas, S. B. (2006). Fulminant toxoplasmosis causing fatal pneumonitis and myocarditis. HIV Med. 7 (6), 415–420. doi: 10.1111/j.1468-1293.2006.00393.x
Filomeni, G., De Zio, D., Cecconi, F. (2015). Oxidative stress and autophagy: The clash between damage and metabolic needs. Cell Death differentiation 22 (3), 377–388. doi: 10.1038/cdd.2014.150
Flegr, J., Prandota, J., Sovičková, M., Israili, Z. H. (2014). Toxoplasmosis–a global threat. correlation of latent toxoplasmosis with specific disease burden in a set of 88 countries. PloS One 9 (3), e90203. doi: 10.1371/journal.pone.0090203
Foulon, W., Villena, I., Stray-Pedersen, B., Decoster, A., Lappalainen, M., Pinon, J. M., et al. (1999). Treatment of toxoplasmosis during pregnancy: A multicenter study of impact on fetal transmission and children’s sequelae at age 1 year. Am. J. Obstetrics Gynecology 180 (2 Pt 1), 410–415. doi: 10.1016/s0002-9378(99)70224-3
Fu, Y., Huang, Z., Hong, L., Lu, J.-H., Feng, D., Yin, X.-M., et al. (2019). Targeting ATG4 in cancer therapy. Cancers 11 (5), 649. doi: 10.3390/cancers11050649
Hain, A. U. P., Bartee, D., Sanders, N. G., Miller, A. S., Sullivan, D. J., Levitskaya, J., et al. (2014). Identification of an Atg8-Atg3 protein-protein interaction inhibitor from the medicines for malaria venture malaria box active in blood and liver stage plasmodium falciparum parasites. J. medicinal Chem. 57 (11), 4521–4531. doi: 10.1021/jm401675a
Halonen, S. K., Weiss, L. M. (2013). Toxoplasmosis. Handb. Clin. Neurol. 114, 125–145. doi: 10.1016/B978-0-444-53490-3.00008-X
Hermanns, T., Müller, U. B., Könen-Waisman, S., Howard, J. C., Steinfeldt, T. (2016). The toxoplasma gondii rhoptry protein ROP18 is an Irga6-specific kinase and regulated by the dense granule protein GRA7. Cell. Microbiol. 18 (2), 244–259. doi: 10.1111/cmi.12499
Howe, D. K., Honoré, S., Derouin, F., Sibley, L. D. (1997). Determination of genotypes of toxoplasma gondii strains isolated from patients with toxoplasmosis. J. Clin. Microbiol. 35 (6), 1411–1414. doi: 10.1128/jcm.35.6.1411-1414.1997
Hunter, P. (2011). The cost of living longer. fertility trades with immunity and life expectancy. EMBO Rep. 12 (10), 1000–1002. doi: 10.1038/embor.2011.183
Ietta, F., Maioli, E., Daveri, E., Gonzaga Oliveira, J., da Silva, R. J., Romagnoli, R., et al. (2017). Rottlerin-mediated inhibition of toxoplasma gondii growth in BeWo trophoblast-like cells. Sci. Rep. 7 (1), 1279. doi: 10.1038/s41598-017-01525-6
Khan, I. A., Eckel, M. E., Pfefferkorn, E. R., Kasper, L. H. (1988). Production of gamma interferon by cultured human lymphocytes stimulated with a purified membrane protein (P30) from toxoplasma gondii. J. Infect. Dis. 157 (5), 979–984. doi: 10.1093/infdis/157.5.979
Khan, A., Taylor, S., Su, C., Mackey, A. J., Boyle, J., Cole, R., et al. (2005). Composite genome map and recombination parameters derived from three archetypal lineages of toxoplasma gondii. Nucleic Acids Res. 33 (9), 2980–2992. doi: 10.1093/nar/gki604
Khetarpal, S. A., Rader, D. J. (2014). Genetics of lipid traits: Genome-wide approaches yield new biology and clues to causality in coronary artery disease. Biochim. Biophys. Acta 1842 (10), 2010–2020. doi: 10.1016/j.bbadis.2014.06.007
Lakhrif, Z., Moreau, A., Hérault, B., Di-Tommaso, A., Juste, M., Moiré, N., et al. (2018). Targeted delivery of antigens to dendritic cells promote immunogenicity and protective efficiency against toxoplasmosis. Front. Immunol. 9. doi: 10.3389/fimmu.2018.00317
Lavine, M. D., Arrizabalaga, G. (2012). Analysis of monensin sensitivity in toxoplasma gondii reveals autophagy as a mechanism for drug induced death. PloS One 7 (7), e42107. doi: 10.1371/journal.pone.0042107
Lee, J., Choi, J.-W., Han, H. Y., Kim, W. S., Song, H.-Y., Byun, E.-B., et al. (2020). 4-hydroxybenzaldehyde restricts the intracellular growth of toxoplasma gondii by inducing SIRT1-mediated autophagy in macrophages. Korean J. Parasitol. 58 (1), 7–14. doi: 10.3347/kjp.2020.58.1.7
Leesombun, A., Nihei, C.-I., Kondoh, D., Nishikawa, Y. (2022). Polyether ionophore kijimicin inhibits growth of toxoplasma gondii and controls acute toxoplasmosis in mice. Parasitol. Res. 121 (1), 413–422. doi: 10.1007/s00436-021-07363-w
Lee, Y.-J., Song, H.-O., Lee, Y.-H., Ryu, J.-S., Ahn, M.-H. (2013). Proliferation of toxoplasma gondii suppresses host cell autophagy. Korean J. Parasitol. 51 (3), 279–287. doi: 10.3347/kjp.2013.51.3.279
Li, M., Hou, Y., Wang, J., Chen, X., Shao, Z.-M., Yin, X.-M. (2011). Kinetics comparisons of mammalian Atg4 homologues indicate selective preferences toward diverse Atg8 substrates. J. Biol. Chem. 286 (9), 7327–7338. doi: 10.1074/jbc.M110.199059
Ling, Y. M., Shaw, M. H., Ayala, C., Coppens, I., Taylor, G. A., Ferguson, D. J. P., et al. (2006). Vacuolar and plasma membrane stripping and autophagic elimination of toxoplasma gondii in primed effector macrophages. J. Exp. Med. 203 (9), 2063–2071. doi: 10.1084/jem.20061318
Li, Z.-H., Ramakrishnan, S., Striepen, B., Moreno, S. N. J. (2013). Toxoplasma gondii relies on both host and parasite isoprenoids and can be rendered sensitive to atorvastatin. PloS Pathog. 9 (10), e1003665. doi: 10.1371/journal.ppat.1003665
Liu, E., Lopez Corcino, Y., Portillo, J.-A. C., Miao, Y., Subauste, C. S. (2016). Identification of signaling pathways by which CD40 stimulates autophagy and antimicrobial activity against toxoplasma gondii in macrophages. Infection Immun. 84 (9), 2616–2626. doi: 10.1128/IAI.00101-16
Liu, Y., Tang, Y., Tang, X., Wu, M., Hou, S., Liu, X., et al. (2021). Anti- effects of a novel spider peptide XYP1 In vitro and In vivo. Biomedicines 9 (8), 934. doi: 10.3390/biomedicines9080934
Lopez Corcino, Y., Gonzalez Ferrer, S., Mantilla, L. E., Trikeriotis, S., Yu, J.-S., Kim, S., et al. (2019). Toxoplasma gondii induces prolonged host epidermal growth factor receptor signalling to prevent parasite elimination by autophagy: Perspectives for in vivo control of the parasite. Cell. Microbiol. 21 (10), e13084. doi: 10.1111/cmi.13084
López-Yglesias, A. H., Burger, E., Camanzo, E., Martin, A. T., Araujo, A. M., Kwok, S. F., et al. (2021). T-Bet-dependent ILC1- and NK cell-derived IFN-γ mediates cDC1-dependent host resistance against toxoplasma gondii. PloS Pathog. 17 (1), e1008299. doi: 10.1371/journal.ppat.1008299
Majumdar, T., Sharma, S., Kumar, M., Hussain, M. A., Chauhan, N., Kalia, I., et al. (2019). Tryptophan-kynurenine pathway attenuates β-catenin-dependent pro-parasitic role of STING-TICAM2-IRF3-IDO1 signalosome in toxoplasma gondii infection. Cell Death Dis. 10 (3), 161. doi: 10.1038/s41419-019-1420-9
Martín-Navarro, C. M., López-Arencibia, A., Sifaoui, I., Reyes-Batlle, M., Valladares, B., Martínez-Carretero, E., et al. (2015). Statins and voriconazole induce programmed cell death in acanthamoeba castellanii. Antimicrob. Agents chemother. 59 (5), 2817–2824. doi: 10.1128/AAC.00066-15
Menon, S., Dibble, C. C., Talbott, G., Hoxhaj, G., Valvezan, A. J., Takahashi, H., et al. (2014). Spatial control of the TSC complex integrates insulin and nutrient regulation of mTORC1 at the lysosome. Cell 156 (4), 771–785. doi: 10.1016/j.cell.2013.11.049
Mitra, P., Deshmukh, A. S., Choudhury, C. (2021). Molecular chaperone function of stress inducible Hsp70 is critical for intracellular multiplication of toxoplasma gondii. biochimica et biophysica acta. Mol. Cell Res. 1868 (2), 118898. doi: 10.1016/j.bbamcr.2020.118898
Montoya, J. G., Liesenfeld, O. (2004). Toxoplasmosis. Lancet 363 (9425), 1965–1976. doi: 10.1016/S0140-6736(04)16412-X
Moon, S. Y., Lee, J.-H., Choi, H. Y., Cho, I. J., Kim, S. C., Kim, Y. W. (2014). Tryptanthrin protects hepatocytes against oxidative stress via activation of the extracellular signal-regulated kinase/NF-E2-related factor 2 pathway. Biol. Pharm. Bull. 37 (10), 1633–1640. doi: 10.1248/bpb.b14-00363
Muniz-Feliciano, L., Van Grol, J., Portillo, J.-A. C., Liew, L., Liu, B., Carlin, C. R., et al. (2013). Toxoplasma gondii-induced activation of EGFR prevents autophagy protein-mediated killing of the parasite. PloS Pathog. 9 (12), e1003809. doi: 10.1371/journal.ppat.1003809
Ng, F., Tang, B. L. (2013). Sirtuins’ modulation of autophagy. J. Cell. Physiol. 228 (12), 2262–2270. doi: 10.1002/jcp.24399
Ohshima, J., Lee, Y., Sasai, M., Saitoh, T., Su Ma, J., Kamiyama, N., et al. (2014). Role of mouse and human autophagy proteins in IFN-γ-induced cell-autonomous responses against toxoplasma gondii. J. Immunol. 192 (7), 3328–3335. doi: 10.4049/jimmunol.1302822
Parihar, S. P., Guler, R., Khutlang, R., Lang, D. M., Hurdayal, R., Mhlanga, M. M., et al. (2014). Statin therapy reduces the mycobacterium tuberculosis burden in human macrophages and in mice by enhancing autophagy and phagosome maturation. J. Infect. Dis. 209 (5), 754–763. doi: 10.1093/infdis/jit550
Parker, S. J., Roberts, C. W., Alexander, J. (1991). CD8+ T cells are the major lymphocyte subpopulation involved in the protective immune response to toxoplasma gondii in mice. Clin. Exp. Immunol. 84 (2), 207–212. doi: 10.1111/j.1365-2249.1991.tb08150.x
Penas, F. N., Carta, D., Cevey, Á.C., Rada, M. J., Pieralisi, A. V., Ferlin, M. G., et al. (2019). Pyridinecarboxylic acid derivative stimulates pro-angiogenic mediators by PI3K/AKT/mTOR and inhibits reactive nitrogen and oxygen species and NF-κB activation through a PPARγ-dependent pathway in -infected macrophages. Front. Immunol. 10. doi: 10.3389/fimmu.2019.02955
Pengo, N., Prak, K., Costa, J. R., Luft, C., Agrotis, A., Freeman, J., et al. (2018). Identification of kinases and phosphatases that regulate ATG4B activity by siRNA and small molecule screening in cells. Front. Cell Dev. Biol. 6. doi: 10.3389/fcell.2018.00148
Phan, T.-N., Baek, K.-H., Lee, N., Byun, S. Y., Shum, D., No, J. H. (2020). In vitro and in vivo activity of mTOR kinase and PI3K inhibitors against and. Molecules 25 (8), 1980. doi: 10.3390/molecules25081980
Piplani, H., Marek-Iannucci, S., Sin, J., Hou, J., Takahashi, T., Sharma, A., et al. (2019). Simvastatin induces autophagic flux to restore cerulein-impaired phagosome-lysosome fusion in acute pancreatitis. Biochim. Biophys. Acta Mol. basis Dis. 1865 (11), 165530. doi: 10.1016/j.bbadis.2019.08.006
Portillo, J.-A. C., Muniz-Feliciano, L., Lopez Corcino, Y., Lee, S. J., Van Grol, J., Parsons, S. J., et al. (2017). Toxoplasma gondii induces FAK-Src-STAT3 signaling during infection of host cells that prevents parasite targeting by autophagy. PloS Pathog. 13 (10), e1006671. doi: 10.1371/journal.ppat.1006671
Portillo, J.-A. C., Okenka, G., Reed, E., Subauste, A., Van Grol, J., Gentil, K., et al. (2010). The CD40-autophagy pathway is needed for host protection despite IFN-Γ-dependent immunity and CD40 induces autophagy via control of P21 levels. PloS One 5 (12), e14472. doi: 10.1371/journal.pone.0014472
Purba, E. R., Saita, E.-I., Maruyama, I. N. (2017). Activation of the EGF receptor by ligand binding and oncogenic mutations: The “Rotation model”. Cells 6 (2), 13. doi: 10.3390/cells6020013
Radke, J. B., Carey, K. L., Shaw, S., Metkar, S. R., Mulrooney, C., Gale, J. P., et al. (2018). High throughput screen identifies interferon γ-dependent inhibitors of toxoplasma gondii growth. ACS Infect. Dis. 4 (10), 1499–1507. doi: 10.1021/acsinfecdis.8b00135
Rayner, J. O., Roberts, R. A., Kim, J., Poklepovic, A., Roberts, J. L., Booth, L., et al. (2020). AR12 (OSU-03012) suppresses GRP78 expression and inhibits SARS-CoV-2 replication. Biochem. Pharmacol. 182, 114227. doi: 10.1016/j.bcp.2020.114227
Reichmann, G., Walker, W., Villegas, E. N., Craig, L., Cai, G., Alexander, J., et al. (2000). The CD40/CD40 ligand interaction is required for resistance to toxoplasmic encephalitis. Infection Immun. 68 (3), 1312–1318. doi: 10.1128/IAI.68.3.1312-1318.2000
Robinson, S. A., Smith, J. E., Millner, P. A. (2004). Toxoplasma gondii major surface antigen (SAG1): In vitro analysis of host cell binding. Parasitology 128 (Pt 4), 391–396. doi: 10.1017/s0031182003004736
Rodgers, C. A., Harris, J. R. (1996). Ocular toxoplasmosis in HIV infection. Int. J. STD AIDS 7 (5), 307–309. doi: 10.1258/0956462961918068
Saeij, J. P. J., Coller, S., Boyle, J. P., Jerome, M. E., White, M. W., Boothroyd, J. C. (2007). Toxoplasma co-opts host gene expression by injection of a polymorphic kinase homologue. Nature 445 (7125), 324–327. doi: 10.1038/nature05395
Saki, J., Sabaghan, M., Arjmand, R., Teimoori, A., Rashno, M., Saki, G., et al. (2020). Curcumin as an indirect methylation inhibitor modulates the effects of on genes involved in male fertility. EXCLI J. 19, 1196–1207. doi: 10.17179/excli2020-2052
Sanfelice, R., da Silva, S. S., Bosqui, L. R., Machado, L. F., Miranda-Sapla, M. M., Panagio, L. A., et al. (2019). Pravastatin and simvastatin pretreatment in combination with pyrimethamine and sulfadiazine reduces infection process of toxoplasma gondii tachyzoites (RH strain) in HeLa cells. Acta parasitol. 64 (3), 612–616. doi: 10.2478/s11686-019-00076-2
Sanfelice, R. A., da Silva, S. S., Bosqui, L. R., Miranda-Sapla, M. M., Barbosa, B. F., Silva, R. J., et al. (2017). Pravastatin and simvastatin inhibit the adhesion, replication and proliferation of toxoplasma gondii (RH strain) in HeLa cells. Acta tropica 167, 208–215. doi: 10.1016/j.actatropica.2016.12.006
Sasai, M., Pradipta, A., Yamamoto, M. (2018). Host immune responses to toxoplasma gondii. Int. Immunol. 30 (3), 113–119. doi: 10.1093/intimm/dxy004
Sasai, M., Sakaguchi, N., Ma, J. S., Nakamura, S., Kawabata, T., Bando, H., et al. (2017). Essential role for GABARAP autophagy proteins in interferon-inducible GTPase-mediated host defense. Nat. Immunol. 18 (8), 899–910. doi: 10.1038/ni.3767
Selleck, E. M., Orchard, R. C., Lassen, K. G., Beatty, W. L., Xavier, R. J., Levine, B., et al. (2015). A noncanonical autophagy pathway restricts toxoplasma gondii growth in a strain-specific manner in IFN-γ-Activated human cells. mBio 6 (5), e01157–e01115. doi: 10.1128/mBio.01157-15
Shammaa, A. M., Powell, T. G., Benmerzouga, I. (2021). Adverse outcomes associated with the treatment of toxoplasma infections. Sci. Rep. 11 (1), 1035. doi: 10.1038/s41598-020-80569-7
Sher, A., Collazzo, C., Scanga, C., Jankovic, D., Yap, G., Aliberti, J. (2003). Induction and regulation of IL-12-dependent host resistance to toxoplasma gondii. Immunol. Res. 27 (2-3), 521–528. doi: 10.1385/IR:27:2-3:521
Shi, C.-S., Shenderov, K., Huang, N.-N., Kabat, J., Abu-Asab, M., Fitzgerald, K. A., et al. (2012). Activation of autophagy by inflammatory signals limits IL-1β production by targeting ubiquitinated inflammasomes for destruction. Nat. Immunol. 13 (3), 255–263. doi: 10.1038/ni.2215
Subauste, C. S. (2021). Recent advances in the roles of autophagy and autophagy proteins in host cells during infection and potential therapeutic implications. Front. Cell Dev. Biol. 9. doi: 10.3389/fcell.2021.673813
Suzuki, Y., Orellana, M. A., Schreiber, R. D., Remington, J. S. (1988). Interferon-gamma: The major mediator of resistance against toxoplasma gondii. Science 240 (4851), 516–518. doi: 10.1126/science.3128869
Szewczyk-Golec, K., Pawłowska, M., Wesołowski, R., Wróblewski, M., Mila-Kierzenkowska, C. (2021). Oxidative stress as a possible target in the treatment of toxoplasmosis: Perspectives and ambiguities. Int. J. Mol. Sci. 22 (11), 5705. doi: 10.3390/ijms22115705
Torres, R. A., Weinberg, W., Stansell, J., Leoung, G., Kovacs, J., Rogers, M., et al. (1997). Atovaquone for salvage treatment and suppression of toxoplasmic encephalitis in patients with AIDS. Atovaquone/Toxoplasmic encephalitis study group. Clin. Infect. Diseases: An Off. Publ. Infect. Dis. Soc. America 24 (3), 422–429. doi: 10.1093/clinids/24.3.422
Ullmann, A. J., Dolan, M. C., Sackal, C. A., Fikrig, E., Piesman, J., Zeidner, N. S. (2013). Immunization with adenoviral-vectored tick salivary gland proteins (SALPs) in a murine model of Lyme borreliosis. Ticks tick-borne Dis. 4 (1-2), 160–163. doi: 10.1016/j.ttbdis.2012.08.006
Van Grol, J., Muniz-Feliciano, L., Portillo, J.-A. C., Bonilha, V. L., Subauste, C. S. (2013). CD40 induces anti-toxoplasma gondii activity in nonhematopoietic cells dependent on autophagy proteins. Infection Immun. 81 (6), 2002–2011. doi: 10.1128/IAI.01145-12
Varberg, J. M., LaFavers, K. A., Arrizabalaga, G., Sullivan, W. J. (2018). Characterization of plasmodium Atg3-Atg8 interaction inhibitors identifies novel alternative mechanisms of action in toxoplasma gondii. Antimicrob. Agents chemother. 62 (2), e01489–17. doi: 10.1128/AAC.01489-17
Williams-Bey, Y., Boularan, C., Vural, A., Huang, N.-N., Hwang, I.-Y., Shan-Shi, C., et al. (2014). Omega-3 free fatty acids suppress macrophage inflammasome activation by inhibiting NF-κB activation and enhancing autophagy. PloS One 9 (6), e97957. doi: 10.1371/journal.pone.0097957
Xiao, J., Prandovszky, E., Kannan, G., Pletnikov, M. V., Dickerson, F., Severance, E. G., et al. (2018). Toxoplasma gondii: Biological parameters of the connection to schizophrenia. Schizophr. Bull. 44 (5), 983–992. doi: 10.1093/schbul/sby082
Xue, J., Jiang, W., Chen, Y., Gong, F., Wang, M., Zeng, P., et al. (2017). Thioredoxin reductase from: An essential virulence effector with antioxidant function. FASEB journal: Off. Publ. Fed. Am. Societies Exp. Biol. 31 (10), 4447–4457. doi: 10.1096/fj.201700008R
Xu, L., Yu, Y., Sang, R., Ge, B., Wang, M., Zhou, H., et al. (2020). Inonotus obliquus polysaccharide protects against adverse pregnancy caused by toxoplasma gondii infection through regulating Th17/Treg balance via TLR4/NF-κB pathway. Int. J. Biol. macromolecules 146, 832–840. doi: 10.1016/j.ijbiomac.2019.10.051
Yan, K., Zhou, H., Wang, M., Li, H., Sang, R., Ge, B., et al. (2021). Inhibitory effects of polysaccharide on inflammatory response in infected RAW264.7 macrophages. Evidence-Based complementary Altern. med.: eCAM 2021, 2245496. doi: 10.1155/2021/2245496
Yin, K., Xu, C., Zhao, G., Xie, H. (2022). Epigenetic manipulation of psychiatric behavioral disorders induced by. Front. In Cell. Infection Microbiol. 12. doi: 10.3389/fcimb.2022.803502
Zhang, J., Chen, J., Lv, K., Li, B., Yan, B., Gai, L., et al. (2021a). Myrislignan induces redox imbalance and activates autophagy in. Front. Cell. infection Microbiol. 11. doi: 10.3389/fcimb.2021.730222
Zhang, J., Si, H., Lv, K., Qiu, Y., Sun, J., Bai, Y., et al. (2021b). Licarin-b exhibits activity against the RH strain by damaging mitochondria and activating autophagy. Front. Cell Dev. Biol. 9. doi: 10.3389/fcell.2021.684393
Zhao, Y., Ferguson, D. J. P., Wilson, D. C., Howard, J. C., Sibley, L. D., Yap, G. S. (2009). Virulent toxoplasma gondii evade immunity-related GTPase-mediated parasite vacuole disruption within primed macrophages. J. Immunol. 182 (6), 3775–3781. doi: 10.4049/jimmunol.0804190
Zhuang, H., Yao, C., Zhao, X., Chen, X., Yang, Y., Huang, S., et al. (2020). DNA Double-strand breaks in the toxoplasma gondii-infected cells by the action of reactive oxygen species. Parasites Vectors 13 (1), 490. doi: 10.1186/s13071-020-04324-7
Keywords: autophagy, Toxoplasma gondii, CD40, IFN-γ, AMPK, mTOR
Citation: Cheng A, Zhang H, Chen B, Zheng S, Wang H, Shi Y, You S, Li M and Jiang L (2022) Modulation of autophagy as a therapeutic strategy for Toxoplasma gondii infection. Front. Cell. Infect. Microbiol. 12:902428. doi: 10.3389/fcimb.2022.902428
Received: 23 March 2022; Accepted: 05 August 2022;
Published: 24 August 2022.
Edited by:
Fred David Mast, Seattle Children’s Research Institute, United StatesReviewed by:
Klaus Pfeffer, Heinrich Heine University, Düsseldorf, GermanyPraveen Kumar, Institute of Medical Sciences, Banaras Hindu University, India
Copyright © 2022 Cheng, Zhang, Chen, Zheng, Wang, Shi, You, Li and Jiang. This is an open-access article distributed under the terms of the Creative Commons Attribution License (CC BY). The use, distribution or reproduction in other forums is permitted, provided the original author(s) and the copyright owner(s) are credited and that the original publication in this journal is cited, in accordance with accepted academic practice. No use, distribution or reproduction is permitted which does not comply with these terms.
*Correspondence: Liping Jiang, amlhbmdsaXBpbmdAY3N1LmVkdS5jbg==; Ming Li, bGltaW5nQGNzdS5lZHUuY24=