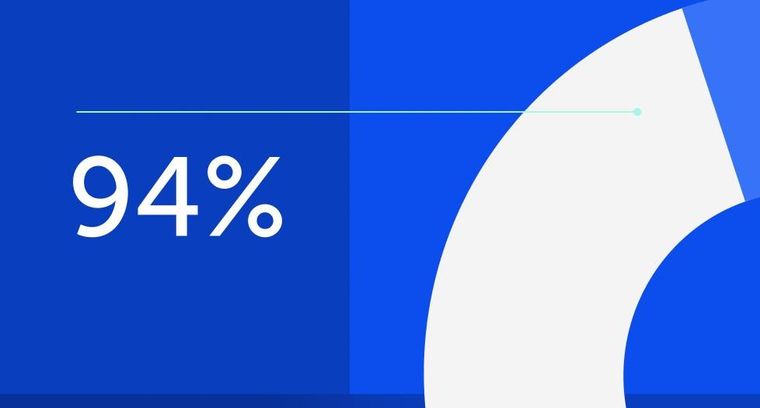
94% of researchers rate our articles as excellent or good
Learn more about the work of our research integrity team to safeguard the quality of each article we publish.
Find out more
ORIGINAL RESEARCH article
Front. Cell. Infect. Microbiol., 16 June 2022
Sec. Parasite and Host
Volume 12 - 2022 | https://doi.org/10.3389/fcimb.2022.901253
This article is part of the Research TopicWomen in Parasite and Host 2021View all 22 articles
Malaria, an infection caused by apicomplexan parasites of the genus Plasmodium, continues to exact a significant toll on public health with over 200 million cases world-wide, and annual deaths in excess of 600,000. Considerable progress has been made to reduce malaria burden in endemic countries in the last two decades. However, parasite and mosquito resistance to frontline chemotherapies and insecticides, respectively, highlights the continuing need for the development of safe and effective vaccines. Here we describe the development of recombinant human antibodies to three target proteins from Plasmodium falciparum: reticulocyte binding protein homologue 5 (PfRH5), cysteine-rich protective antigen (PfCyRPA), and circumsporozoite protein (PfCSP). All three proteins are key targets in the development of vaccines for blood-stage or pre-erythrocytic stage infections. We have developed potent anti-PfRH5, PfCyRPA and PfCSP monoclonal antibodies that will prove useful tools for the standardisation of assays in preclinical research and the assessment of these antigens in clinical trials. We have generated some very potent anti-PfRH5 and anti-PfCyRPA antibodies with some clones >200 times more potent than the polyclonal anti-AMA-1 antibodies used for the evaluation of blood stage antigens. While the monoclonal and polyclonal antibodies are not directly comparable, the data provide evidence that these new antibodies are very good at blocking invasion. These antibodies will therefore provide a valuable resource and have potential as biological standards to help harmonise pre-clinical malaria research.
Malaria is a vector-borne disease endemic to large parts of the world. It is estimated that nearly half of the global population is at risk with annual deaths of over 627,000 people, primarily African children under 5 years of age (WHO, 2021). The first malaria vaccine for children in areas of moderate to high transmission was recommended by the World Health Organisation (WHO) in October 2021. This recommendation is based on the results from an extended pilot programme of Mosquirix® (RTS,S/AS01) in Ghana, Malawi, and Kenya as part of routine childhood immunisations (Adepoju, 2019) and represents significant progress in the fight against malaria. In addition, a second generation subunit vaccine (R21/Matrix-M; Datoo et al., 2021) received authorisation for a phase 3 clinical trial (NCT04704830). Additionally, phase I clinical trials in controlled human malaria infections with whole attenuated sporozoite vaccines have recently been published (NCT02511054, NCT03083847) with promising results (Mwakingwe-Omari et al., 2021). Finally, a blood-stage vaccine has undergone phase I/IIa clinical trials (Minassian et al., 2021), further adding to the vaccine portfolio.
Malaria is caused by parasites of the genus Plasmodium that have a complex life cycle with development in a mosquito vector and in a vertebrate host. In humans, the first step of infection occurs when parasites enter the human host via an infected mosquito bite and begin to replicate in the liver. Parasites released from the liver enter the circulation where they replicate within erythrocytes; the intra-erythrocytic cycle is associated with malaria pathology and is the primary target of anti-malarial chemotherapies (Maier et al., 2019). While anti-malarial drugs and control strategies are vital to malaria control efforts, parasite drug resistance and mosquito insecticide resistance are on the rise and pose risks to the gains obtained over the past two decades (WHO, 2021). Furthermore, disruptions in healthcare access caused by the COVID-19 pandemic have increased deaths resulting from malaria infection in 2020 with an estimated 241 million cases and 627,000 deaths (WHO, 2021).
Bearing in mind that eradicating malaria parasites following infection requires protracted treatment regimens with serious socioeconomic consequences, the need for malaria vaccines remains as urgent as ever. Malaria vaccines targeting parasite antigens across all key stages of the parasite life cycle are being developed. These include vaccines blocking transmission to the vector (Challenger et al., 2021) and those targeting liver infection (Mwakingwe-Omari et al., 2021), and blood stage parasites (Vijayan and Chitnis, 2019). In addition, vaccines to prevent placental malaria are also in phase Ia clinical trials (Sirima et al., 2020). The vaccines closest to regulatory approval and licensure, including Mosquirix® (RTS,S/AS01) and R21/Matrix-M, target parasite infection of the liver with other vaccine candidates targeting development of the parasites in red blood cells (Sato, 2021a; Sato, 2021b).
Many assays are available for malaria research, but they are not standardised, making inter-laboratory comparison of data very challenging. Furthermore, the high genetic variability of P. falciparum presents an additional, and considerable, challenge for harmonisation of the field, especially in diagnosis and treatment of the disease. Additional challenges exist in the characterisation of immune responses, disease surveillance, and in the spectrum of assays used to understand the disease in terms of management and treatment. The first serological WHO Reference Reagent for P. falciparum (Bryan et al., 2017) and the first WHO International Standard for P. falciparum antigens (Harris et al., 2017) were major breakthroughs for standardising vaccine assays and diagnostics, respectively. However, reagents that enable standardisation throughout all aspects of preclinical development of malaria vaccine candidates are still needed for reliable quantification of malaria antigens. The evaluation of vaccine efficacy using in vitro and in vivo assays is not currently harmonised which in turn effects reproducibility, inhibits progress and squanders investment.
Preclinical evaluation of malaria vaccines prior to controlled human malaria infection studies and clinical trials (Chattopadhyay and Pratt, 2017; Good and Miller, 2018; Artaud et al., 2019; Matuschewski and Borrmann, 2019; Lyke et al., 2020) includes standard laboratory assays for malaria blood stages, such as in vitro growth inhibition activity (GIA) assay (Malkin et al., 2005) in vivo passive antibody transfer in mice as well as lesser used immunogenicity and efficacy studies in non-human primates (Douglas et al., 2015). Variability in these assays can be caused by the community using a number of different reagents, some being commercial or shared, some being used by one or few laboratories, and many being finite (e.g. the anti-AMA-1 polyclonal antibody BG98 (Faber et al., 2013), meaning again that results are not always or comparable across laboratories and over time. The lack of a defined standard makes meaningful comparisons difficult and impedes the development of novel invasion-blocking candidate antigens.
In addition to these problems for assays directed against blood stage malaria parasites, similar challenges exist for assays involved in targeting P. falciparum sporozoites, e.g. invasion, cell traversal, opsonic phagocytosis and cytotoxicity assays (Sinnis et al., 2013; Tavares et al., 2013a; Tavares et al., 2013b; Formaglio et al., 2014; Steel et al., 2017; Aliprandini et al., 2018). The ‘gold standard’ control antibody for these assays is a mouse monoclonal antibody named 2A10 that recognises NANP repeats of the P. falciparum circumsporozoite protein (PfCSP) (Zavala et al., 1983; Hollingdale et al., 1984; Anker et al., 1990; Zhang et al., 2017). However, the hybridoma cell line used for production of has 2A10 been widely shared and propagated in different laboratories likely leading to a drift in its biochemical and biophysical properties. Differences may arise due to the potential for genetic instability of the hybridomas - as such it is no longer suitable as a standard (Bradbury and Pluckthun, 2015). This potential drift is difficult to quantify in the absence of a primary standard. As such the generation of the recombinant monoclonal antibodies described here provides an opportunity for the development new standards that target PfCSP.
The European Research Infrastructures for Poverty Related Diseases (EURIPRED) was a collaborative research and infrastructure project that aimed to generate, coordinate and integrate resources to support international research on HIV/AIDS, tuberculosis, malaria and Hepatitis B & C. As part of this project we generated recombinant human monoclonal antibodies that could be used to develop and standardise assays for P. falciparum malaria vaccine research and development. These materials, intended to support the research and development community, are available from the Centre for AIDS Reagents repository at NIBSC (www.nibsc.org).
We have generated recombinant human monoclonal antibodies directed against the P. falciparum blood stage invasion proteins Reticulocyte binding protein Homologue 5 (PfRH5), Cysteine-Rich Protective Antigen (PfCyRPA) and the dominant sporozoite antigen circumsporozoite protein (PfCSP). PfRH5 is a leading blood stage malaria vaccine target and forms a complex with PfCyRPA and PfRH5-interacting protein (PfRIPR) critical for red-cell invasion by merozoites (Healer et al., 2019; Knuepfer et al., 2019; Ragotte et al., 2020; Ndwiga et al., 2021). Antibodies to both antigens are highly effective at inhibiting growth of P. falciparum (Dreyer et al., 2012; Douglas et al., 2014; Volz et al., 2016) and such antibodies may provide suitable reference reagents to standardise GIA assays and other erythrocyte invasion assays – by having a standard with defined neutralising activity, to which the neutralising activity of other materials can be compared. We also developed human monoclonal antibodies to PfCSP, the key antigen of the Mosquirix® (Wilby et al., 2012; Adepoju, 2019; Cotton, 2020) and R21 (Datoo et al., 2021) vaccines designed to prevent P. falciparum infection in the liver. PfCSP is expressed on the surface of sporozoites and is the main target of the immune response during the initial phase of infection (Zhang et al., 2017).
Here we describe the generation and characterisation of human monoclonal antibodies against key P. falciparum antigens and discuss how their use may support malaria vaccine development. We hope that these monoclonal antibodies will facilitate better cross-laboratory comparisons of novel blood and liver stage malaria vaccine candidates.
P. falciparum laboratory isolates FCR3 and NF54 were obtained from The European Malaria Reagent Repository (http://www.malariaresearch.eu/). Parasites were maintained at 4% haematocrit in A+ erythrocytes (National Health Service Blood and Transplant, UK) at 5% CO2 and atmospheric O2 as previously described (Trager and Jensen, 1976).
Recombinant monoclonal antibodies were produced using HybriFree Technology (Icosagen) as previously described (Kivi et al., 2016). Two 4-8 month old chickens (1.7-2.1 kg) and three rabbits (4-5.5 kg) of >4 months old each were immunised with recombinant PfRH5 (Jin et al., 2018) and PfCyRPA (generated in house, Jenner Institute, University of Oxford) and recombinant PfCSP (kindly provided by D. Narum, NIAID, NIH, DHHS) as previously described (Kivi et al., 2016). Rabbits and chickens were immunised in parallel as antigens elicit different responses in different species thereby increasing the likelihood of identifying antibodies of interest. Briefly, 0.2 mg of antigen per test animal per injection was used. Rabbits received four subscapular immunisations at approximately 3 week intervals. Chickens were immunised intramuscularly three times with at ~2 week intervals. The primary immunisation was performed in Freund's complete adjuvant, while all subsequent immunisations were with Freund's incomplete adjuvant (IFA). Booster doses were performed as two separate injections, where the first (0.1 mg) was administered by subscapular injection (rabbits) or intramuscularly (chickens) in Freund's incomplete adjuvant and the second (0.1 mg) was administered intravenously in phosphate buffered saline (PBS) – both injections were given in this way to all test animals and at the same time point (Kivi et al., 2016). Spleens were isolated 3 days after the final boost, homogenized in ice-cold PBS, and cryopreserved in heat inactivated foetal bovine serum with 10% DMSO prior to long-term storage in liquid nitrogen (Kivi et al., 2016). All procedures on animals were performed in compliance with European Union directive 86/609/EEC and approved by the Estonian National Board of Animal Experiments (No. 115, 07.09.2012; No. 87, 28.08.2007).
Recombinant monoclonal antibodies were generated as previously described (Kivi et al., 2016). Briefly, panning of 2 x 104 splenic cells from immunised animals was performed on antigen-coated (5 μg/ml) immune modules (ThermoFisher Scientific) for 45 min after which unbound cells were removed by washing in PBS. RNA was extracted from the bound cells, reverse transcribed in cDNA using the Superscript IV First-Strand Synthesis System (Invitrogen), which was subsequently used as a template to amplify by PCR the variable light (VL) and heavy (VH) chains.
Amplified VL and VH chains were cloned into human immunoglobulin G1 (hIgG1) expression vectors by circular polymerase extension cloning (CPEC) (Quan and Tian, 2009). Escherichia coli DH5α were transformed with hIgG1 expression vector pools and grown in liquid medium on a shaker at 37°C overnight. Plasmid DNA was extracted, purified, and transfected into a transgenic Chinese hamster ovary (CHO) cell line named CHOEBNALT85-1E9 for hIgG1 pool production as previously described (Kivi et al., 2016). Single clones were selected on Luria Broth ampicillin agar medium. 48- 72 hours after transfection, recombinant human IgG1 (rIgG1) pools were tested in enzyme linked immunosorbent assay (ELISA). Maxisorp Immuno modules (Thermo Fisher Scientific) were coated with the specific antigen at 1 µg/ml in PBS overnight. Plates were washed 4 times and blocked with PBS containing 2% bovine serum albumin (BSA) and 0.05% Tween-20. Colonies from ELISA positive pools were grown in liquid medium overnight in a shaker at 37°C and in a 96-well microtiter plate. Plasmid DNA was isolated and transfected into CHOEBNALT85-1E9 cells for transient antibody production and cell culture supernatants were collected after 48 - 72 h and analysed by ELISA to confirm antigen specific binding. Positive clones were then sequenced for VH/VL sequence verification using the international ImmunoGeneTics (IMGT) information system (Lefranc et al., 1999; Giudicelli et al., 2005; Lefranc, 2014; Lefranc et al., 2015).
Plasmid clones were transfected into CHOEBNALT85-1E9 for transient production in 2 ml of media. Ten days after transfection, cells were removed by centrifugation (300g for 5 min) and 1.5 ml of each supernatant was aliquoted into microtubes. The concentration of the antibodies produced in crude cell supernatant was measured by biolayer interferometry (Octet® K2 System; ForteBio) using Protein A sensors and appropriate human IgG1 standards. The supernatants were stored at -80°C until further testing. Cell culture supernatants containing anti-PfRH5 and anti-PfCyRPA antibodies were individually screened using the red cell invasion assay to assess functional activity. Selected PfRH5 and PfCyRPA mAbs were then expressed and purified on a small scale to confirm inhibitory activity, followed by scale up to 400 ml cultures of CHOEBNALT85-1E9 cells and purified by HiTrap MabSelect SuRe affinity chromatography with buffer exchange using HiTrap Desalting Columns to PBS pH 7.4 (GE Healthcare). Purified antibodies were adjusted to a concentration of 1 mg/ml, analysed by ELISA and non-reduced gel electrophoresis, filtered, aliquoted and stored at -75°C; no additives or stabilising reagents were added to the purified antibodies to prevent interference in the functional assays. Culture supernatants containing anti-PfCSP mAbs were used for initial screenings and concentrations determined as described above for the PfRh5 and PfCyRPA antibodies. Further work to express and purify anti-PfCSP mAbs is on-going. All mAbs described here are available from the Centre for AIDS Reagents repository at NIBSC (www.nibsc.org).
PfRH5 and PfCyRPA form a complex with PfRIPR during merozoite invasion of erythrocytes (Healer et al., 2019; Knuepfer et al., 2019; Ndwiga et al., 2021). The formation of the complex is required for erythrocyte invasion (Volz et al., 2016). Biolayer interferometry (Octet® K2 System, Pall ForteBio) (Li et al., 2017) was performed to determine the potential of the mAbs to block PfCyRPA-PfRH5 interactions. Monoclonal antibody cell culture supernatants were incubated with immobilised recombinant proteins to measure the dissociation of antigen-antibody and infer antibody blocking activity.
Recombinant PfCyRPA molecules were conjugated to biotin with a ratio of 1:5 using Thermo Scientific™ EZ-Link™ NHS-PEG4-Biotin, No-Weigh™ Format (A39259). Two streptavidin (SA) sensors were incubated in kinetic buffer (PBS containing 0.1% BSA, 0.02% Tween-20, and 0.1% Proclin™ 300) for 11 min in plates with shaking at 1000 rpm to determine the baseline. The two sensors were then used to immobilise biotinylated PfCyRPA by incubation in kinetic buffer containing PfCyRPA-biotin (2 μg/ml) for 11 min at 1000 rpm. The sensors were incubated once more in kinetic buffer alone for 11 min at 1000 rpm to reset to baseline and then incubated in cell culture supernatants diluted 1:2 with kinetic buffer for 22 min at 1000 rpm to saturate the immobilised PfCyRPA-biotin molecules with anti-PfCyRPA antibodies. The sensors were incubated again in kinetic buffer alone for 11 min at 1000 rpm. Finally, one sensor was incubated in kinetic buffer and the other in kinetic buffer containing 12 µg/ml (~0.08 nM) recombinant PfRH5 for 33 min at 1000 rpm.
Blocking activity measurements of anti-PfRH5 antibodies were performed with the following differences. Anti-PfRH5 antibody cell culture supernatants were incubated with recombinant PfRH5 (5 μg/ml) for ≥ 1h at room temperature (RT) to achieve a state of equilibrium. In addition, between sample measurements, used sensors were regenerated using glycine buffer (pH 1.7) and kinetic buffer. Finally, one sensor was incubated in negative cell culture supernatant diluted 1:2 with kinetic buffer containing recombinant PfRH5 (5 µg/ml) and the other sensor was incubated in PfRH5 antibody cell culture supernatant diluted 1:2 in kinetic buffer containing 5 μg/ml recombinant PfRH5 for 33 min at 1000 rpm. The operating temperature for the Octet was at 30°C and all other processes performed at RT unless indicated otherwise.
We generated overlapping 15-mer peptides for 18 P. falciparum and 3 P. vivax antigens. We included key vaccine antigens for both species (e.g. PfAMA-1, PfCSP, and Pfs25 or PvCSP, Pvs25, and PvDBP). To ensure we captured sequence diversity for these highly polymorphic antigens we used sequence information available from MalariaGEN (MalariaGen et al., 2021). We included all mutations found in P. vivax and only those with a frequency of >0.5% for P. falciparum proteins. The peptides were synthesised and spotted onto microarray slides (RT-HD-Plas, JPT Technologies). Peptides were printed into three individual subarrays per slide and used to screen the polyclonal sera, culture supernatants containing the mAbs, and purified mAbs. Briefly, samples were diluted 1:2000 for cell culture supernatants or used at 50 µg/ml for purified recombinant mAbs in Superblock T20 buffer (Thermo/Pierce, #37516); 300 µl was added to the slides and they were incubated for 2 h at 30°C on each of the triplicate arrays. The primary analyte was washed 5 times for 3 min with 2-4 ml of wash buffer (1X Tris buffered saline (TBS) + 0.1% Tween20). The arrays were then incubated with a Cy5-conjugated anti-human IgG1, anti-rabbit IgG, or anti-chicken IgY secondary antibody, as appropriate, diluted to 1 μg/ml in blocking buffer for 45 min at 30°C. Slides were washed again in wash buffer and dried with a gentle stream of nitrogen and stored in the dark until fluorescence scanning on a GenePix 4330A scanner using a scanning resolution of 10 µm per pixel.
Crude cell culture supernatants containing anti-PfRH5 and anti-PfCyRPA antibodies were screened by invasion assay to determine their capacity to inhibit invasion of P. falciparum FCR3 parasites in vitro. Briefly, P. falciparum FCR3 mature stage parasites (schizonts) were enriched by magnet purification, diluted into culture medium containing A+ red blood cells at 4% haematocrit and dispensed into 96-well plates. Three dilutions were tested in duplicate for each antibody; 1:5, 1:25, and 1:125. Antibodies were added to each well for a final volume of 125 µl/well. For each plate a serial dilution of BG98 antibodies (anti-PfAMA-1; NIBSC #NR0014) starting at 6 mg/ml was included to provide a standard curve as well as a positive control for the inhibition of merozoite invasion. Cell culture supernatant from non-transfected cells was used a negative control. Finally, a minimum of 8 wells containing no antibodies was included per plate as an additional control. A volume of 25 µl of culture was removed from each well, fixed with PBS containing 1% PFA and 0.05% GTA. Cells were stained with SYBR Green to determine parasitaemia by flow cytometry. A further 25 µl was removed 48 h later to measure parasitaemia and calculate invasion inhibition after one intra-erythrocytic cycle. For the selection of the recombinant mAb candidates, invasion assays were performed as described above with some modifications. Three concentrations were tested in duplicate for each antibody; 16.4 µg/ml [112.3 nM], 3.28 µg/ml [22.5 nM], and 0.656 µg/ml [4.5 nM]. These concentrations, based on the antibodies with the lowest concentrations within the crude cell supernatant, were selected to enable comparisons at set concentrations across all antibodies.
Sporozoite cell traversal assays were performed using Hepa1-6 cells (ATCC CRL-1830) and P. falciparum NF54 sporozoites (PfNF54) isolated by hand dissection of infected A. stephensi mosquitoes (provided by the Department of Medical Microbiology, University Medical Centre, St Radboud, Nijmegen, the Netherlands). Hepa1-6 cells were seeded at 3 x 104/well in 96 well plates and cultured at 37°C under 5% CO2 in DMEM medium (41966029, Gibco) supplemented with 10% (v/v) foetal calf serum (10500064, Gibco), 2 mM L-glutamine (25030024, Thermo Scientific), and 1% (v/v) of a penicillin–streptomycin solution (15140122, Gibco). The following day 5 x 104 PfNF54 sporozoites in complete culture medium were added to the cells in triplicate wells with 1 mg/ml rhodamine-conjugated Dextran (D1817, Molecular Probes) and either 2 or 20 µg/ml of the test antibodies with normal cell culture supernatant as a negative control. Cell cultures were incubated for 3 h at 37°C, then washed, trypsinised, fixed in 1% formaldehyde in PBS, and analysed on a Guava EasyCyte 6/2L bench cytometer equipped with a 532 nm laser (Millipore), for detection and quantification of dextran-positive cells.
Characterisation of antibody binding to sporozoites was performed using 2.5 x 104 P. falciparum NF54 sporozoites per sample fixed in 2% PFA in PBS and detected with 2 µg/ml of the rIgG1 diluted in 1% BSA-PBS. Sporozoites were labelled in 15 µl final solution for 1 h on ice before addition of 4 µg/ml AlexaFlour 488 goat anti-human IgG1 (H + L) (Invitrogen, A11013) + 0.5 µg/ml AlexaFluor 647-conjugated 2A10 in 1% BSA-PBS in a final volume of 30 µl. Samples were incubated for 30 min on ice and analysed by flow cytometry on a CytoFLEX S flow cytometer (Beckman Coulter). A minimum of 3000 2A10-positive PfNF54 sporozoites were acquired.
Antibody cytotoxicity was determined as previously described (Aliprandini et al., 2018) using P. berghei NK65 sporozoites expressing GFP and a hybrid CSP containing the central repeats region of P. falciparum CSP (PbPf-GFP). After incubation of 1.2 x 104 sporozoites for 45 min at 37°C with 80 µg/ml of PfCSP antibodies (except for 2G12, which was tested at 60 µg/ml) in HybriMed supernatant and 10% FCS, and with 5 µg/ml of propidium iodide (PI), sporozoites were analysed by flow cytometry. Viable sporozoites were considered as GFP positive and PI negative, and viability was normalized to HybriMed medium without PfCSP antibodies, set to 100% viability.
Invasion assays were performed as described above. Briefly, P. falciparum FCR3 or PfNF54 mature stage parasites (schizonts) were enriched by magnet purification, diluted into culture medium containing A+ red blood cells at 4% haematocrit and dispensed into 96-well plates. Three concentrations were tested in duplicate for each antibody; 16.4 µg/ml [112.3 nM], 3.28 µg/ml [22.5 nM], and 0.656 µg/ml [4.5 nM]. Antibodies were added to each well for a final volume of 125 µl/well. For each plate a serial dilution of BG98 antibodies (anti-PfAMA-1; NIBSC #NR0014) starting at 6 mg/ml was included to provide a standard curve as well as a positive control for the inhibition of merozoite invasion. Finally, a minimum of 2 wells without antibodies was included per plate as a negative control. A volume of 25 µl of culture was removed from each well, fixed with PBS containing 1% PFA and 0.05% GTA. Cells were stained with SYBR Green to determine parasitaemia by flow cytometry. A further 25 µl was removed 48 h later to measure parasitaemia and calculate invasion inhibition relative to the no antibody control after one intra-erythrocytic cycle. Statistical analyses were performed using a General Linear Model in Minitab® 21.1.1 after verifying assumptions for a parametric test were met (i.e. equality of variances and normal distribution).
A total of 64 and 32 panning reactions were performed for PfRH5 from chicken and rabbit splenocytes, respectively (Table 1), from which 1034 clones were tested by ELISA and 256 were positive. A total of 194 PfRH5 positive clones were sequenced and of those 33 unique sequences were identified; 26/33 unique sequences were obtained from chicken immunisations and 7/33 from rabbits. Cell culture supernatants from the 33 PfRH5 antibody clones were tested for antigen binding using biolayer interferometry (BLI), which identified 18 PfRH5 mAbs with blocking activity. To do this, biotinylated recombinant rPfRH5 was exposed to its recombinant binding partner rPfCyRPA in the presence or absence of the mAb. Figure 1 shows examples of two different BLI profiles observed for PfRH5 and PfCyRPA recombinant IgG1 clones. The clones shown exhibit either a reduced rPfRH5-rPfCyRPA binding (5E6#36, 3A7#22) or not (1A4#27, 3G11#15. All but one of the PfRH5 mAbs with blocking activity were isolated from chicken.
Figure 1 Biolayer interferometry traces of crude hIgG1 clones showing different blocking profiles for (A) PfRH5 clones 1A4#27 and 5E6#36; and (B) PfCyRPA clones 3A7#22 and 3G11#15. For PfRH5 (A) traces show cell culture supernatant containing only PfRH5 recombinant hIgG1 antibodies (green) and cell culture supernatant containing both recombinant PfRH5 (rPfRH5) and recombinant human IgG1 antibodies (pink). Antibody 1A4#27 does not block PfRH5 binding to PfCyRPA. Conversely, antibody 5E6#39 blocks binding of PfRH5 to PfCyRPA (step 4). The numbered steps show 1) baseline for both sensors incubated in kinetic buffer; 2) incubation of the sensors with recombinant PfCyRPA-biotin conjugate (rPfCyRPA); 3) baseline with the immobilised rPfCyRPA-biotin in kinetic buffer; and 4) association of rPfRH5 to PfCyRPA. For PfCyRPA (B) traces show incubation of one sensor in kinetic buffer (green) and incubation of the other sensor (pink) with rPfRH5 in association step (step 6). The numbered steps show 1) baseline readings for both sensors in kinetic buffer; 2) incubation with biotin-conjugated recombinant PfCyRPA in kinetic buffer; 3) baseline readings for sensors with immobilised rPfCyRPA in kinetic buffer; 4) saturation of the sensors with crude antibody cell culture supernatant containing specific anti-PfCyRPA recombinant hIgG1s (pink); 5) baseline with immobilized PfCyRPA-antibody complex in kinetic buffer; 6) One sensor incubated in kinetic buffer (green) and the second in kinetic buffer containing PfRH5 (pink). Clone 3G11#15 shows blocking activity (orange outline) while antibody clone 3A7#22 does not block binding of rPfRH5 to rPfCyRPA (blue outline). The y-axis shows binding (nm) and x-axis time (sec).
Similarly to PfRH5, 64 panning reactions were performed for PfCyRPA from chicken splenocytes and sixteen from rabbit splenocytes (Table 1). Of those, 55 cDNAs were synthesised and antibody expression of positive clones confirmed by ELISA. Of the 1040 clones tested, 139 were positive for PfCyRPA of which 91 were sequenced; 17 unique sequences were identified. Twelve of the unique PfCyRPA sequences were obtained from the chicken immunisations and 5 from rabbits. Again, using the Octet K2 system to evaluate the blocking activity, cell culture supernatants from the 17 PfCyRPA antibody clones were tested. 13 Thirteen had blocking activity (11 from chicken immunisations and 2 from rabbits). Biolayer interferometry traces for PfRH5 and PfCyRPA of rIgG1 monoclonal antibodies with and without blocking activity are shown in Supplementary Figure 1.
The ability of the recombinant antibodies to block P. falciparum invasion was next tested by screening all 33 PfRH5 clones and 17 PfCyRPA clones with the in vitro invasion assay. Invasion assays using PfFCR3 parasites revealed a range invasion inhibition activities for culture supernatant containing PfRH5 and PfCyRPA rIgG1 antibodies (Figure 2). Furthermore, invasion inhibition of antibodies during the initial screening of crude supernatants showed concentration-dependent effects for most antibodies (Figure 2). The potency of the antibodies was compared to the anti-AMA-1 polyclonal antibody BG98 by parallel line analysis (CombiStats 7.0). Relative potency estimates for each of the rIgG1 tested compared to anti-AMA-1 (BG98) ranged from 1 to 967 (median 20) for PfRH5 and from 3 to 201 (median 27) for PfCyRPA (Supplementary Table 1). All of the antibodies generated here were either as effective (relative potency of 1) or better at inhibiting invasion than BG98 (relative potency >1). Furthermore, the antibodies with the highest invasion blocking activity for PfRh5 and PfCyRPA compared to BG98, were 967-fold and 201-fold more potent than BG98, respectively (see Supplementary Table 1 for the full list of antibodies). It should be noted that the potency of these rhIgG1 clones is not directly comparable to BG98 as it is a polyclonal antibody mix and it is not possible to assess the potency of individual clones within the mixture to the mAbs generated here.
Figure 2 Initial screen of crude cell culture supernatant of expressed anti-PfRH5 (A) and anti-PfCyRPA (B) antibodies. Antibodies were tested on P. falciparum FCR3 during one intra-erythrocytic cycle. Invasion inhibition was determined by measuring parasitaemia by flow cytometry, percentage inhibition was calculated relative to the no antibody control wells. Invasion of parasite by AMA-1 polyclonal antibodies (BG98 standard) is shown for comparison. Many monoclonal antibodies show high potency at low nanomolar (nM) concentrations as compared to polyclonal anti-AMA-1 where the concentration of total IgG is used rather than the antigen-specific fraction.
Interestingly, three anti-PfRH5 clones (1D8#11, 14D5#250, 15D12#256) and one anti-PfCyRPA clone (7B7#7) showed invasion inhibition activity in the invasion assays despite having no blocking activity by BLI (Supplementary Figure 1). Of those, 1D8#11 recognised a single overlapping peptide by peptide microarray (ISEEIDDKSEETDDETEEVEDSI). Invasion inhibition data along with BLI traces (Supplementary Figure 1) and microarray data (see Supplementary Figure 2 and Supplementary Spreadsheet File) were used to select clones for a more comparable assessment of mAb activity of the crude supernatants. Thirteen PfRH5 mAbs and 10 PfCyRPA mAbs displaying dilution effects in the initial screen were further evaluated, using the invasion assay, at defined antibody concentrations (16.4 µg/ml [112 nM], 3.28 µg/ml [22.5 nM], 0.656 µg/ml [4.5 nM]; data not shown) confirming concentration-dependent effects allowing further down selection.
Four anti-PfRH5 clones and 4 anti-PfCyRPA clones were selected for up-scaled production and purification. These purified mAbs retained invasion inhibition activity (Figure 3) and generally showed greater specificity by microarray with single or overlapping epitopes binding to the target antigen in the majority of cases (Supplementary Figure 3). Recombinant anti-PfRH5 and anti-PfCyRPA antibodies inhibited invasion in both PfFCR3 and PfNF54, but as can be seen in Figure 3, PfFCR3 parasites were consistently more susceptible to rIgG1 invasion blocking activity. With the exception of clone 2A7#70 PfRH5 rhIgG1 clones were significantly more inhibitory in PfFCR3 compared to PfNF54 (GLM(1,3,2,2): P <0.001). All four purified PfCyRPA rhIgG1 clones showed greater inhibition of invasion in PfFCR3 (GLM(1,3,2,2): P <0.001). Similarly, significant differences in replication rate between PfFCR3 and PfNF4 were observed for PfRH5 rhIgG1 clones 5E6#36 (GLM(2,1,2): P = 0.001) and 15E3#259 (GLM(2,1,2): P = 0.001). No significant differences in replication rate were observed for rhIgG1 clones 2A7#70 (GLM(2,1,2): P = 0.242) and 15D12#256 (GLM(2,1,2): P = 0.068). For Pf CyRPA all rgIgG1 clones tested were significantly different between PfFCR3 and PfNF54; 3A7#22 (GLM(2,1,2): P = 0.005), 3B3#17 (GLM(2,1,2): P <0.001), 4D12#30 (GLM(2,1,2): P <0.05), 7B8#13 (GLM(2,1,2): P = 0.004), 5E6#36 (GLM(2,1,2): P = 0.001), 15D12#256 (GLM(2,1,2): P = 0.068), 15E3#259 (GLM(2,1,2): P = 0.001) (Supplementary Figure 4).
Figure 3 Potency of purified anti-PfRH5 and -PfCyRPA monoclonal antibodies on P. falciparum FCR3 (A) and NF54 parasites (B) after incubation during one intra-erythrocytic cycle. Invasion inhibition was determined by measuring parasitaemia by flow cytometry, percentage inhibition was calculated relative to the no antibody control wells. The percentage inhibition and standard error of the mean (error bars) are shown for 3 replicate experiments. Assays were performed using antibodies diluted to 112.3, 22.5, and 4.5 nM (i.e. 16.4, 3.28, and 0.656 µg/ml respectively) to facilitate comparisons with data using cell culture supernatants. Recombinant hIgG1 clones 2A7#70, 5E6#36, 15D12#256, and 15E3#259 are all directed against PfRH5. Antibodies to PfCyRPA are 3A7# 22, 3B3#17, 4D12#30, and 7B9#13.
The only available malaria vaccine, Mosquirix (RTS,S/AS01) includes the PfCSP antigen, and new PfCSP vaccines in development, so we next sought to produce anti-PfCSP antibodies. For both chicken and rabbit immunisations a total of 128 panning reactions were performed resulting in 110 cloning reactions. Heavy and light chains were amplified and cloned into human IgG1 expression vectors, with a total of 50 panning reactions from chicken immunisations, and 76 from rabbit immunisations. Following transient expression, the presence of anti-PfCSP antibodies in culture media was determined using ELISA. Eleven rIgG1 pools were positive; ~470 clones were selected of which 47 were bound to PfCSP. From these, 19 clones were selected for sequencing and 7 unique sequence were identified; 3 were derived from chicken immunisations and 4 from rabbits.
Using immunofluorescence and flow cytometry, 4/7 clones were found to bind to the surface of parasites (2G12#8, 4E11#20, 4H1#15 [rabbit] and 6F1#25 [chicken]; Figure 4A) and 3/7 displayed cytotoxicity and cell traversal inhibition profiles at 20 and 2 µg/ml (2G12#8, 4H11, 4E11#20, and 6F1#25; Figure 4B and Table 2).
Figure 4 (A) Inhibition and cytotoxicity of recombinant IgG1 (rIgG1) against PfCSP from cell culture supernatants. Cell traversal (CT) inhibition (y-axis) of PfNF54 sporozoites is shown against viability of P. berghei PfCSP transgenic parasites following incubation with the various mAbs. To note, cytotoxic activity in PbPfCSP sporozoites correlates with inhibition of Pf CT. Antibodies that do not bind to the PfCSP on the sporozoite surface (red) are non-cytotoxic. IFA, immunofluorescence assay. (B) Viability of transgenic P. berghei Pf CSP parasites following incubation with cell culture supernatant containing recombinant anti-PfCSP rIgG1. Controls included in the assay were cell culture medium alone and a recombinant anti-PfRH5 rIgG1. The recombinant antibodies generated had a limited effect on sporozoite viability with 4E11#20 showing a moderate reduction in viability.
To determine the range of epitopes present from the immunisations in rabbits and chickens the sera from the final bleeds was tested by peptide microarray. We found the major target was the PfCSP NANP repeats (Supplementary Figure 4), with some additional reactivity against two additional regions outside of the NANP repeats. Sera from two of the immunised rabbits bound strongly to a 15-mer peptide (GQGHNMPNDPNRNVD). Sera from only one rabbit bound to an overlapping peptide (RNVDENANANSAVKNNNNEEPSDKHI). However, no clones were recovered from that animal. For the chicken immunisations, only sera from one chicken bound outside the NANP repeats to peptide EPSDKHIKEYLNKIQ but with no reactivity to the polymorphic sequences (EPSDKHITEYLNKIQ and EPSDKHIEEYLNKIQ). Three clones were isolated from that immunization; 2C4#2, 6F1#25, 6F8#32).
Here we describe the generation and initial characterisation of novel reference reagents to support malaria vaccine development. Through the EURIPRED project, we have produced, characterised and made available recombinant human IgG1 for the wider malaria research community. The recombinantly produced PfRH5 and PfCyRPA antibodies described provide a characterised and sequence defined material that can be used to standardise assays. Indeed, the antibodies produced inhibited invasion at similar concentrations (~16 µg/ml [~100 nm]) to some previously reported antibodies isolated from animals and humans (Douglas et al., 2011; Alanine et al., 2019; Knudsen et al., 2021). Furthermore, the activity of the some of the recombinant human antibodies described here appears to be comparable to monoclonal antibodies isolated from humans (Alanine et al., 2019) suggesting they have potential for development as biological standards.
We evaluated the antibodies to blood stage antigens in terms of relatively potency compared to an existing reference material (BG98). BG98 represents a large, but finite, supply of antibodies to PfAMA-1 (Faber et al., 2013) and in this work also demonstrates the utility of a reference material. Indeed, the potency of the rhIgG1 clones described here at concentrations similar to previously reported PfCyRPA and PfRH5 antibodies (e.g. Douglas et al., 2011; Alanine et al., 2019; Knudsen et al., 2021) may make them more suitable as standards than BG98 which requires high concentrations to be used. In addition, independent testing to determine inter-laboratory variability of the antibodies was performed by GIA using Pf3D7. The relative antibody activity was consistent across laboratories (data not shown) highlighting that these materials can be used to harmonise laboratory tests globally. Indeed, the rIgG1 revealed similar potency of the antibodies as determined by rank order for inhibition (data not shown). Interestingly we observed increased sensitivity of PfFCR3 to blocking antibodies relative to PfNF54. The initial screening and selection steps were performed with only one laboratory isolate (PfFCR3) which highlights, again, the value of standards as these differences can be identified and quantified. Strain differences in growth inhibition assays has previously been reported for both PfRH5 (Douglas et al., 2011)and PfCyRPA (Knudsen et al., 2021) despite low levels of polymorphisms in both antigens. Our data support these observations in additional laboratory isolates. Interestingly, we identified assay-dependent variability with the blocking antibodies, with Pf3D7 showing increased sensitivity to the rIgG1 in the invasion assay compared to GIA (data not shown); this has been found with other neutralising antibodies (Coxon et al., 2021) and is another reason why reference reagents are so valuable as they highlight that reliance on a single assay or strain can cause reproducibility issues and inconsistencies.
We observed few clones for the purified anti-PfRH5 rIgG1 that recognised linear epitopes on the microarray. Conversely, most anti-PfCyRPA rIgG1 bound to multiple epitopes on their target antigen but also on the other antigens present on the array. It is likely that conformational epitopes are critical for the binding and blocking activities of the rIgG1 described here. Indeed, it has previously been reported that many PfRH5 epitopes are conformational (Alanine et al., 2019). This may explain why some of the antibodies for which we could not clearly identify linear epitopes were potent in inhibiting invasion (e.g. 3A7#22, 3B3#17, 4D12#30). Furthermore, the epitopes recognized by the purified rhIgG1 clones did not contain polymorphic sequences, suggesting that differences in antibody activity in PfFCR3 and PfNF54 are likely due to conformational epitopes. It will be interesting that given we found differences in invasion inhibition between strains, to fine tune epitope mapping to determine if these can be used to predict or confirm likely differences in antibody potency.
The materials evaluated were developed and purified as part of this work were prepared in relatively small quantity, but importantly the sequence and therefore the capability to prepare more are available. As these antibodies are recombinant they theoretically constitute an infinite resource ensuring a supply for the future. However, as a note of caution the route of production may be important as it is know that glycosylation patterns of immunoglobulins are important for functional activity (Schroeder and Cavacini, 2010; Subedi and Barb, 2015; van de Bovenkamp et al., 2016). Indeed, if we are proposing that the community uses these antibodies to harmonise and standardise assays, it is important that we understand the effects of post-translational modifications on the material and ensure we are using a defined and optimised production process. Indeed, work by our group and others (Ragotte et al., 2022) has already shown that the choice of cell expression system used in the production of the antibodies effects antibody activity and we currently are investigating this in more detail at NIBSC. Furthermore, it is known that post-translational modifications are important for the activity of biological molecules including glycosylation in the context of immunoglobulins (Schroeder and Cavacini, 2010; Subedi and Barb, 2015; van de Bovenkamp et al., 2016). Glycosylation can be influenced by many factors including the cells used for the production of antibodies and the additives used in cell culture media (Kim et al., 2018; Ehret et al., 2019). It is therefore important to consider production of recombinant monoclonal antibodies. Indeed, variations in the biological activity of antibodies may result not from their sequences but their glycosylation patterns.
All the antibodies described were tested individually. It is probable that further screening of the purified rIgG1 would enable the identification of synergistic effects with different antibody combinations as has been previously reported for PfCyRPA (Knudsen et al., 2021) and PfRH5 (Alanine et al., 2019) As PfRH5 and PfCyRPA form a complex during invasion, the presence of antibodies to both antigens could further inhibit invasion. Combining antibodies to the same target with different binding profiles could allow for the identification of more inhibitory antibody cocktails which would also enable the formulation of more robust standards. This approach would enable the formulation of polyclonal rIgG1 materials that may be more commutable and therefore comparable to the breadth of responses seen in individuals exposed to P. falciparum. In addition, many antibodies with high potency were not purified and further characterised, and these may warrant further investigation such as anti-PfRH5 clone 18D8 #291 with >900-fold increased potency compared to anti-AMA-1 (BG98), or anti-PfCyRPA clone 17H7 #73 with a potency 80-fold higher than anti-AMA-1 (BG98).
The antibodies described here also offer significant opportunities to generate new reference materials and research reagents as recombinant antibodies can be cloned into different immunoglobulin backbones. Specific subclasses of IgG are associated with P. falciparum infection (Dobano et al., 2019), namely IgG1 and IgG3. The system described here for the expression of human recombinant IgGs allows for the complementary determining regions (CDRs) to be cloned into other IgG subclasses (e.g., IgG3 framework) to determine how changing Fc functionality affects in vitro or in vivo activity at the two key stages of the parasite life-cycle (liver and blood). Altering the IgG subclass may enable the development of other reagents that more closely reflect the human immune response in assays and therefore, provide better indicators of candidate antigen performance during clinical development. These would also provide standardisation reagents more closely aligned to the biological targets of interest.
While the production of the PfCSP antibodies was challenging we have successfully generated seven new monoclonal antibodies. The reasons for the difficulties in expression and isolation of the PfCSP rIgG1 are not clear and further work is on-going with the clones generated. The microarray data showed that the polyclonal sera from one chicken and two rabbits bound to regions outside the NANP repeats. Three clones were isolated from the immunized chicken (2C4#2, 6F1#25, 6F8#32) and one clone from the rabbits (5B12#21). Determining what epitopes the purified rhIgG1 clones recognise by microarray will further allow characterisation of these mAbs. The antibodies generated did not show increased potency compared to 2A10 as crude cell supernatants. However, the data obtained from the crude supernatants is encouraging and all antibodies generated showed some activity in the various assays used (i.e. cell traversal inhibition, cell toxicity). For example, antibody 4E11#20 showed relatively high levels of activity in the cell traversal assay (75.45 ± 12.1% inhibition) and cytotoxicity (54% viability). Further work will be required on the purified rIgG1 to better understand their activity and potential as reference reagents and/or research reagents.
The materials described here are a first step in the provision of quality standards for malaria research that can support robust assay development and material characterisation for vaccines.
The recombinant human monoclonal antibodies described herein are available from CFAR-NIBSC.
The VH/VL sequence were analysed and validated using the international ImmunoGeneTics (IMGT) information system https://www.imgt.org/.
The animal study was reviewed and approved by Estonian National Board of Animal Experiments (No. 115, 07.09.2012; No. 87, 28.08.2007).
AN performed the invasion assays, blood-stage antibody selection, data analysis, project management, coordinated with partners for assays, and wrote the manuscript. GK performed antibody isolation by HybriFree technology and project management. RP performed the immunisations, Octet blocking assay, and project management. EJ did the antibody purification and antibody screening by ELISA. PH performed the microarray experiments. EA and RA performed the sporozoite cytotoxicity, cell traversal and immunofluorescence assays and corresponding figures. OS performed the sporozoite cell traversal assays. DQ performed GIA with the purified mAbs. YLD and MH provided critical logistics for the shipment and storage of the antibodies, and project management. UR led the work at JPT Technologies, designed the microarray slides, provided data insights and analysis. AT generated the monoclonal antibodies, and provided scientific insights. SJD provided reagents and input into the selection of the antigens. SG and MMH coordinated the EURIPRED grant. PWB conceived of the project, selected the antigens and provided project management. All authors reviewed the manuscript and agreed with the version submitted.
This work was the European Union’s Seventh Framework Programme [FP7-INFRA-2012] under Grant Agreement No: 312661 - European Research Infrastructures for Poverty Related Diseases (EURIPRED). SD is a Jenner Investigator, a Lister Institute Research Prize Fellow, and held a Wellcome Trust Senior Fellowship (106917/Z/15/Z).
SJD is a named inventor on patent applications relating to PfRH5 and/or other malaria vaccines, mAbs, and immunisation regimes. UR and PH are employed by JPT Peptide Technologies GmbH. GK, RP, EJ, and AT are employed by Icosagen Cell Factory.
The remaining authors declare that the research was conducted in the absence of any commercial or financial relationships that could be construed as a potential conflict of interest.
All claims expressed in this article are solely those of the authors and do not necessarily represent those of their affiliated organizations, or those of the publisher, the editors and the reviewers. Any product that may be evaluated in this article, or claim that may be made by its manufacturer, is not guaranteed or endorsed by the publisher.
BG98 was generously provided by Ed Remarque (BPRC, Netherlands) and is available from CFAR-NIBSC (cat# NR0014).
The authors thank Carmen Coxon (NIBSC, UK) for critically reviewing the manuscript and providing valuable suggestions and feedback.
The Supplementary Material for this article can be found online at: https://www.frontiersin.org/articles/10.3389/fcimb.2022.901253/full#supplementary-material
Adepoju, P. (2019). RTS,S Malaria Vaccine Pilots in Three African Countries. Lancet 393 (10182), 1685. doi: 10.1016/S0140-6736(19)30937-7
Alanine, D. G. W., Quinkert, D., Kumarasingha, R., Mehmood, S., Donnellan, F. R., Minkah, N. K., et al. (2019). Human Antibodies That Slow Erythrocyte Invasion Potentiate Malaria-Neutralizing Antibodies. Cell 178 (1), 216–228.e221. doi: 10.1016/j.cell.2019.05.025
Aliprandini, E., Tavares, J., Panatieri, R. H., Thiberge, S., Yamamoto, M. M., Silvie, O., et al. (2018). Cytotoxic Anti-Circumsporozoite Antibodies Target Malaria Sporozoites in the Host Skin. Nat. Microbiol. 3 (11), 1224–1233. doi: 10.1038/s41564-018-0254-z
Anker, R., Zavala, F., Pollok, B. A. (1990). VH and VL Region Structure of Antibodies That Recognize the (NANP)3 Dodecapeptide Sequence in the Circumsporozoite Protein of Plasmodium Falciparum. Eur. J. Immunol. 20 (12), 2757–2761. doi: 10.1002/eji.1830201233
Artaud, C., Kara, L., Launay, O. (2019). Vaccine Development: From Preclinical Studies to Phase 1/2 Clinical Trials. Methods Mol. Biol. 2013, 165–176. doi: 10.1007/978-1-4939-9550-9_12
Bradbury, A., Pluckthun, A. (2015). Reproducibility: Standardize Antibodies Used in Research. Nature 518 (7537), 27–29. doi: 10.1038/518027a
Bryan, D., Silva, N., Rigsby, P., Dougall, T., Corran, P., Bowyer, P. W., et al. (2017). The Establishment of a WHO Reference Reagent for Anti-Malaria (Plasmodium Falciparum) Human Serum. Malar. J. 16 (1), 314. doi: 10.1186/s12936-017-1958-x
Challenger, J. D., Olivera Mesa, D., Da, D. F., Yerbanga, R. S., Lefevre, T., Cohuet, A., et al. (2021). Predicting the Public Health Impact of a Malaria Transmission-Blocking Vaccine. Nat. Commun. 12 (1), 1494. doi: 10.1038/s41467-021-21775-3
Chattopadhyay, R., Pratt, D. (2017). Role of Controlled Human Malaria Infection (CHMI) in Malaria Vaccine Development: A U.S. Food & Drug Administration (FDA) Perspective. Vaccine 35 (21), 2767–2769. doi: 10.1016/j.vaccine.2017.03.072
Cotton, M. (2020). The Mosquirix (RTS.S) Malaria Vaccine. Trop. Doct. 50 (2), 107. doi: 10.1177/0049475520916978
Coxon, C. H., Yu, X., Beavis, J., Diaz-Saez, L., Riches-Duit, A., Ball, C., et al. (2021). Characterisation and Application of Recombinant FVIII-Neutralising Antibodies From Haemophilia A Inhibitor Patients. Br. J. Haematol. 193 (5), 976–987. doi: 10.1111/bjh.17227
Datoo, M. S., Natama, M. H., Some, A., Traore, O., Rouamba, T., Bellamy, D., et al. (2021). Efficacy of a Low-Dose Candidate Malaria Vaccine, R21 in Adjuvant Matrix-M, With Seasonal Administration to Children in Burkina Faso: A Randomised Controlled Trial. Lancet 397 (10287), 1809–1818. doi: 10.1016/S0140-6736(21)00943-0
Dobano, C., Santano, R., Vidal, M., Jimenez, A., Jairoce, C., Ubillos, I., et al. (2019). Differential Patterns of IgG Subclass Responses to Plasmodium Falciparum Antigens in Relation to Malaria Protection and RTS,S Vaccination. Front. Immunol. 10. doi: 10.3389/fimmu.2019.00439
Douglas, A. D., Baldeviano, G. C., Lucas, C. M., Lugo-Roman, L. A., Crosnier, C., Bartholdson, S. J., et al. (2015). A PfRH5-Based Vaccine is Efficacious Against Heterologous Strain Blood-Stage Plasmodium Falciparum Infection in Aotus Monkeys. Cell Host Microbe 17 (1), 130–139. doi: 10.1016/j.chom.2014.11.017
Douglas, A. D., Williams, A. R., Illingworth, J. J., Kamuyu, G., Biswas, S., Goodman, A. L., et al. (2011). The Blood-Stage Malaria Antigen PfRH5 is Susceptible to Vaccine-Inducible Cross-Strain Neutralizing Antibody. Nat. Commun. 2, 601. doi: 10.1038/ncomms1615
Douglas, A. D., Williams, A. R., Knuepfer, E., Illingworth, J. J., Furze, J. M., Crosnier, C., et al. (2014). Neutralization of Plasmodium Falciparum Merozoites by Antibodies Against Pfrh5. J. Immunol. 192 (1), 245–258. doi: 10.4049/jimmunol.1302045
Dreyer, A. M., Matile, H., Papastogiannidis, P., Kamber, J., Favuzza, P., Voss, T. S., et al. (2012). Passive Immunoprotection of Plasmodium Falciparum-Infected Mice Designates the CyRPA as Candidate Malaria Vaccine Antigen. J. Immunol. 188 (12), 6225–6237. doi: 10.4049/jimmunol.1103177
Ehret, J., Zimmermann, M., Eichhorn, T., Zimmer, A. (2019). Impact of Cell Culture Media Additives on IgG Glycosylation Produced in Chinese Hamster Ovary Cells. Biotechnol. Bioeng. 116 (4), 816–830. doi: 10.1002/bit.26904
Faber, B. W., Younis, S., Remarque, E. J., Rodriguez Garcia, R., Riasat, V., Walraven, V., et al. (2013). Diversity Covering AMA1-MSP119 Fusion Proteins as Malaria Vaccines. Infect. Immun. 81 (5), 1479–1490. doi: 10.1128/IAI.01267-12
Formaglio, P., Tavares, J., Menard, R., Amino, R. (2014). Loss of Host Cell Plasma Membrane Integrity Following Cell Traversal by Plasmodium Sporozoites in the Skin. Parasitol. Int. 63 (1), 237–244. doi: 10.1016/j.parint.2013.07.009
Giudicelli, V., Chaume, D., Jabado-Michaloud, J., Lefranc, M. P. (2005). Immunogenetics Sequence Annotation: The Strategy of IMGT Based on IMGT-ONTOLOGY. Stud. Health Technol. Inform 116, 3–8.
Good, M. F., Miller, L. H. (2018). Interpreting Challenge Data From Early Phase Malaria Blood Stage Vaccine Trials. Expert Rev. Vaccines 17 (3), 189–196. doi: 10.1080/14760584.2018.1435278
Harris, L. M., Campillo, A., Rigsby, P., Atkinson, E., Poonawala, R., Aidoo, M., et al. (2017). Collaborative Study to Evaluate the Proposed First World Health Organization International Standard for Plasmodium Falciparum Antigens (Geneva: World Health Organization).
Healer, J., Wong, W., Thompson, J. K., He, W., Birkinshaw, R. W., Miura, K., et al. (2019). Neutralising Antibodies Block the Function of Rh5/Ripr/CyRPA Complex During Invasion of Plasmodium Falciparum Into Human Erythrocytes. Cell Microbiol. 21 (7), e13030. doi: 10.1111/cmi.13030
Hollingdale, M. R., Nardin, E. H., Tharavanij, S., Schwartz, A. L., Nussenzweig, R. S. (1984). Inhibition of Entry of Plasmodium Falciparum and P. Vivax Sporozoites Into Cultured Cells; an In Vitro Assay of Protective Antibodies. J. Immunol. 132 (2), 909–913.
Jin, J., Tarrant, R. D., Bolam, E. J., Angell-Manning, P., Soegaard, M., Pattinson, D. J., et al. (2018). Production, Quality Control, Stability, and Potency of cGMP-Produced Plasmodium Falciparum RH5.1 Protein Vaccine Expressed in Drosophila S2 Cells. NPJ Vaccines 3, 32. doi: 10.1038/s41541-018-0071-7
Kim, S.-M., Chang, K.-H., Oh, D. J. (2018). Effect of Environmental Parameters on Glycosylation of Recombinant Immunoglobulin G Produced From Recombinant CHO Cells. Biotechnol. Bioprocess Eng. 23 (4), 456–464. doi: 10.1007/s12257-018-0109-8
Kivi, G., Teesalu, K., Parik, J., Kontkar, E., Ustav, M., Jr., Noodla, L., et al. (2016). HybriFree: A Robust and Rapid Method for the Development of Monoclonal Antibodies From Different Host Species. BMC Biotechnol. 16, 2. doi: 10.1186/s12896-016-0232-6
Knudsen, A. S., Bjornsson, K. H., Bassi, M. R., Walker, M. R., Kok, A., Cristinoi, B., et al. (2021). Strain-Dependent Inhibition of Erythrocyte Invasion by Monoclonal Antibodies Against Plasmodium Falciparum CyRPA. Front. Immunol. 12. doi: 10.3389/fimmu.2021.716305
Knuepfer, E., Wright, K. E., Kumar Prajapati, S., Rawlinson, T. A., Mohring, F., Koch, M., et al. (2019). Divergent Roles for the RH5 Complex Components, CyRPA and RIPR in Human-Infective Malaria Parasites. PloS Pathog. 15 (6), e1007809. doi: 10.1371/journal.ppat.1007809
Lefranc, M. P. (2014). Antibody Informatics: IMGT, the International ImMunoGeneTics Information System. Microbiol. Spectr. 2 (2), 2.2.01. doi: 10.1128/microbiolspec.AID-0001-2012
Lefranc, M. P., Giudicelli, V., Duroux, P., Jabado-Michaloud, J., Folch, G., Aouinti, S., et al. (2015). IMGT(R), the International ImMunoGeneTics Information System(R) 25 Years on. Nucleic Acids Res. 43 (Database issue), D413–D422. doi: 10.1093/nar/gku1056
Lefranc, M. P., Giudicelli, V., Ginestoux, C., Bodmer, J., Muller, W., Bontrop, R., et al. (1999). IMGT, the International ImMunoGeneTics Database. Nucleic Acids Res. 27 (1), 209–212. doi: 10.1093/nar/27.1.209
Li, C., Li, W., Lucio de Esesarte, E., Guo, H., van den Elzen, P., Aarts, E., et al. (2017). Cell Attachment Domains of the Porcine Epidemic Diarrhea Virus Spike Protein Are Key Targets of Neutralizing Antibodies. J. Virol. 91 (12), e00273–17. doi: 10.1128/JVI.00273-17
Lyke, K. E., Singer, A., Berry, A. A., Reyes, S., Chakravarty, S., James, E. R., et al. (2020). Multidose Priming and Delayed Boosting Improve PfSPZ Vaccine Efficacy Against Heterologous P. Falciparum Controlled Human Malaria Infection. Clin. Infect. Dis. 73 (7), e2424–e2435. doi: 10.1093/cid/ciaa1294
Maier, A. G., Matuschewski, K., Zhang, M., Rug, M. (2019). Plasmodium Falciparum. Trends Parasitol. 35 (6), 481–482. doi: 10.1016/j.pt.2018.11.010
MalariaGen, Ahouidi, A., Ali, M., Almagro-Garcia, J., Amambua-Ngwa, A., Amaratunga, C., et al. (2021). An Open Dataset of Plasmodium Falciparum Genome Variation in 7,000 Worldwide Samples. Wellcome Open Res. 6, 42. doi: 10.12688/wellcomeopenres.16168.2
Malkin, E. M., Diemert, D. J., McArthur, J. H., Perreault, J. R., Miles, A. P., Giersing, B. K., et al. (2005). Phase 1 Clinical Trial of Apical Membrane Antigen 1: An Asexual Blood-Stage Vaccine for Plasmodium Falciparum Malaria. Infect. Immun. 73 (6), 3677–3685. doi: 10.1128/IAI.73.6.3677-3685.2005
Matuschewski, K., Borrmann, S. (2019). Controlled Human Malaria Infection (CHMI) Studies: Over 100 Years of Experience With Parasite Injections. Methods Mol. Biol. 2013, 91–101. doi: 10.1007/978-1-4939-9550-9_7
Minassian, A. M., Silk, S. E., Barrett, J. R., Nielsen, C. M., Miura, K., Diouf, A., et al. (2021). Reduced Blood-Stage Malaria Growth and Immune Correlates in Humans Following RH5 Vaccination. Med. (N Y) 2 (6), 701–719.e719. doi: 10.1016/j.medj.2021.03.014
Mwakingwe-Omari, A., Healy, S. A., Lane, J., Cook, D. M., Kalhori, S., Wyatt, C., et al. (2021). Two Chemoattenuated PfSPZ Malaria Vaccines Induce Sterile Hepatic Immunity. Nature 595 (7866), 289–294. doi: 10.1038/s41586-021-03684-z
Ndwiga, L., Osoti, V., Ochwedo, K. O., Wamae, K., Bejon, P., Rayner, J. C., et al. (2021). The Plasmodium Falciparum Rh5 Invasion Protein Complex Reveals an Excess of Rare Variant Mutations. Malar. J. 20 (1), 278. doi: 10.1186/s12936-021-03815-x
Quan, J., Tian, J. (2009). Circular Polymerase Extension Cloning of Complex Gene Libraries and Pathways. PloS One 4 (7), e6441. doi: 10.1371/journal.pone.0006441
Ragotte, R. J., Higgins, M. K., Draper, S. J. (2020). The RH5-CyRPA-Ripr Complex as a Malaria Vaccine Target. Trends Parasitol. 36 (6), 545–559. doi: 10.1016/j.pt.2020.04.003
Ragotte, R. J., Pulido, D., Lias, A. M., Quinkert, D., Alanine, D. G. W., Jamwal, A., et al. (2022). Heterotypic Interactions Drive Antibody Synergy Against a Malaria Vaccine Candidate. Nat. Commun. 13 (1), 933. doi: 10.1038/s41467-022-28601-4
Sato, S. (2021a). Correction to: Plasmodium-A Brief Introduction to the Parasites Causing Human Malaria and Their Basic Biology. J. Physiol. Anthropol. 40 (1), 3. doi: 10.1186/s40101-021-00254-0
Sato, S. (2021b). Plasmodium-A Brief Introduction to the Parasites Causing Human Malaria and Their Basic Biology. J. Physiol. Anthropol. 40 (1), 1. doi: 10.1186/s40101-020-00251-9
Schroeder, H. W., Jr., Cavacini, L. (2010). Structure and Function of Immunoglobulins. J. Allergy Clin. Immunol. 125 (2 Suppl 2), S41–S52. doi: 10.1016/j.jaci.2009.09.046
Sinnis, P., de la Vega, P., Coppi, A., Krzych, U., Mota, M. M. (2013). Quantification of Sporozoite Invasion, Migration, and Development by Microscopy and Flow Cytometry. Methods Mol. Biol. 923, 385–400. doi: 10.1007/978-1-62703-026-7_27
Sirima, S. B., Richert, L., Chene, A., Konate, A. T., Campion, C., Dechavanne, S., et al. (2020). PRIMVAC Vaccine Adjuvanted With Alhydrogel or GLA-SE to Prevent Placental Malaria: A First-in-Human, Randomised, Double-Blind, Placebo-Controlled Study. Lancet Infect. Dis. 20 (5), 585–597. doi: 10.1016/S1473-3099(19)30739-X
Steel, R. W., Sack, B. K., Tsuji, M., Navarro, M. J., Betz, W., Fishbaugher, M. E., et al. (2017). An Opsonic Phagocytosis Assay for Plasmodium Falciparum Sporozoites. Clin. Vaccine Immunol. 24 (2), e00445–16. doi: 10.1128/CVI.00445-16
Subedi, G. P., Barb, A. W. (2015). The Structural Role of Antibody N-Glycosylation in Receptor Interactions. Structure 23 (9), 1573–1583. doi: 10.1016/j.str.2015.06.015
Tavares, J., Formaglio, P., Medvinsky, A., Menard, R., Amino, R. (2013a). Imaging Sporozoite Cell Traversal in the Liver of Mice. Methods Mol. Biol. 923, 401–410. doi: 10.1007/978-1-62703-026-7_28
Tavares, J., Formaglio, P., Thiberge, S., Mordelet, E., Van Rooijen, N., Medvinsky, A., et al. (2013b). Role of Host Cell Traversal by the Malaria Sporozoite During Liver Infection. J. Exp. Med. 210 (5), 905–915. doi: 10.1084/jem.20121130
Trager, W., Jensen, J. B. (1976). Human Malaria Parasites in Continuous Culture. Science 193 (4254), 673–675. doi: 10.1126/science.781840
van de Bovenkamp, F. S., Hafkenscheid, L., Rispens, T., Rombouts, Y. (2016). The Emerging Importance of IgG Fab Glycosylation in Immunity. J. Immunol. 196 (4), 1435–1441. doi: 10.4049/jimmunol.1502136
Vijayan, A., Chitnis, C. E. (2019). Development of Blood Stage Malaria Vaccines. Methods Mol. Biol. 2013, 199–218. doi: 10.1007/978-1-4939-9550-9_15
Volz, J. C., Yap, A., Sisquella, X., Thompson, J. K., Lim, N. T., Whitehead, L. W., et al. (2016). Essential Role of the PfRh5/PfRipr/CyRPA Complex During Plasmodium Falciparum Invasion of Erythrocytes. Cell Host Microbe 20 (1), 60–71. doi: 10.1016/j.chom.2016.06.004
Wilby, K. J., Lau, T. T., Gilchrist, S. E., Ensom, M. H. (2012). Mosquirix (RTS,S): A Novel Vaccine for the Prevention of Plasmodium Falciparum Malaria. Ann. Pharmacother. 46 (3), 384–393. doi: 10.1345/aph.1AQ634
Zavala, F., Cochrane, A. H., Nardin, E. H., Nussenzweig, R. S., Nussenzweig, V. (1983). Circumsporozoite Proteins of Malaria Parasites Contain a Single Immunodominant Region With Two or More Identical Epitopes. J. Exp. Med. 157 (6), 1947–1957. doi: 10.1084/jem.157.6.1947
Keywords: Plasmodium falciparum, monoclonal antibodies, standardisation, assay development, malaria, PfRH5, PfCyRPA, PfCSP
Citation: Nacer A, Kivi G, Pert R, Juronen E, Holenya P, Aliprandini E, Amino R, Silvie O, Quinkert D, Le Duff Y, Hurley M, Reimer U, Tover A, Draper SJ, Gilbert S, Ho MM and Bowyer PW (2022) Expanding the Malaria Antibody Toolkit: Development and Characterisation of Plasmodium falciparum RH5, CyRPA, and CSP Recombinant Human Monoclonal Antibodies. Front. Cell. Infect. Microbiol. 12:901253. doi: 10.3389/fcimb.2022.901253
Received: 21 March 2022; Accepted: 20 May 2022;
Published: 16 June 2022.
Edited by:
Tania F. De Koning-Ward, Deakin University, AustraliaReviewed by:
Miguel Prudêncio, Universidade de Lisboa, PortugalCopyright © 2022 Nacer, Kivi, Pert, Juronen, Holenya, Aliprandini, Amino, Silvie, Quinkert, Le Duff, Hurley, Reimer, Tover, Draper, Gilbert, Ho and Bowyer. This is an open-access article distributed under the terms of the Creative Commons Attribution License (CC BY). The use, distribution or reproduction in other forums is permitted, provided the original author(s) and the copyright owner(s) are credited and that the original publication in this journal is cited, in accordance with accepted academic practice. No use, distribution or reproduction is permitted which does not comply with these terms.
*Correspondence: Adéla Nacer, ZGVsYS5uYWNlckBnbWFpbC5jb20=; Paul W. Bowyer, UGF1bC5Cb3d5ZXJAbmlic2Mub3Jn
Disclaimer: All claims expressed in this article are solely those of the authors and do not necessarily represent those of their affiliated organizations, or those of the publisher, the editors and the reviewers. Any product that may be evaluated in this article or claim that may be made by its manufacturer is not guaranteed or endorsed by the publisher.
Research integrity at Frontiers
Learn more about the work of our research integrity team to safeguard the quality of each article we publish.