- 1Southern Plains Agricultural Research Center, United States Department of Agriculture-Agricultural Research Service (USDA ARS), College Station, TX, United States
- 2Department of Poultry Science, Texas A&M University, College Station, TX, United States
- 3Department of Animal and Food Sciences, University of Delaware, Newark, DE, United States
Poultry is a major source of human foodborne illness caused by broad host range Salmonella serovars (paratyphoid), and developing cost-effective, pre-harvest interventions to reduce these pathogens would be valuable to the industry and consumer. Host responses to infectious agents are often regulated through phosphorylation. However, proteomic mechanisms of Salmonella acute infection biology and host responses to the bacteria have been limited concentrating predominately on the genomic responses of the host to infection. Our recent development of chicken-specific peptide arrays for kinome analysis of host phosphorylation-based cellular signaling responses provided us with the opportunity to develop a more detailed understanding of the early (4-24 h post-infection) host-pathogen interactions during the initial colonization of the cecum by Salmonella. Using the chicken-specific kinomic immune peptide array, biological pathway analysis showed infection with S. Enteritidis increased signaling related to the innate immune response, relative to the non-infected control ceca. Notably, the acute innate immune signaling pathways were characterized by increased peptide phosphorylation (activation) of the Toll-like receptor and NOD-like receptor signaling pathways, the activation of the chemokine signaling pathway, and the activation of the apoptosis signaling pathways. In addition, Salmonella infection induced a dramatic alteration in the phosphorylation events of the JAK-STAT signaling pathway. Lastly, there is also significant activation of the T cell receptor signaling pathway demonstrating the initiation of the acquired immune response to Salmonella infection. Based on the individual phosphorylation events altered by the early Salmonella infection of the cecum, certain conclusions can be drawn: (1) Salmonella was recognized by both TLR and NOD receptors that initiated the innate immune response; (2) activation of the PPRs induced the production of chemokines CXCLi2 (IL-8) and cytokines IL-2, IL-6, IFN-α, and IFN-γ; (3) Salmonella infection targeted the JAK-STAT pathway as a means of evading the host response by targeting the dephosphorylation of JAK1 and TYK2 and STAT1,2,3,4, and 6; (4) apoptosis appears to be a host defense mechanism where the infection with Salmonella induced both the intrinsic and extrinsic apoptotic pathways; and (5) the T cell receptor signaling pathway activates the AP-1 and NF-κB transcription factor cascades, but not NFAT.
Introduction
Salmonella invasion of the chicken cecum induces a short-lived inflammation mediated by the increased expression of pro-inflammatory cytokine and chemokine genes in the intestinal tissue (Withanage et al., 2005; Setta et al., 2012; Matulova et al., 2013; Rychlik et al., 2014). The activation of the innate immune response induces an influx of heterophils (granulocytes) to the intestine that limits bacterial invasion (Kogut et al., 1994; Kogut et al., 2012) but does not lead to a pathological inflammation that is seen in mammals (Foster et al., 2003; Patel and McCormick, 2014). However, this heterophil infiltration of the intestine does not have a significant protective response against the salmonellae bacteria that remain in the lumenal side of the ceca. Interestingly, this inflammatory response is largely resolved by 2-3 days post-infection (Kogut et al., 2012; Kogut et al., 2016; Kogut et al., 2017) and is characterized by the reduction of pro-inflammatory cytokines mRNA transcription in the cecum to non-infected control levels yet Salmonella can persist in the intestine and be shed in the feces for several weeks (Withanage et al., 2005).
We have previously used a chicken-specific kinome peptide array analysis to define changes in cecal tissue phenotype during the establishment of a Salmonella persistent infection in chickens. Using the kinome array, we described a Salmonella-mediated reprogramming of host signaling pathways around 3 days post-infection to be the principal mechanism developed by the bacteria to establish a successful persistent long-term colonization in chicken cecum (Kogut et al., 2017). A wide range of host immune and metabolic pathways acted as Salmonella targets, including the T cell receptor signaling pathway, JAK-STAT signaling pathway, the Wnt-Ca2+ signaling pathway, NF-κB signaling, the mTOR and AMPK metabolic signaling pathways (Kogut and Arsenault, 2015; Kogut et al., 2017; Kogut and Arsenault, 2017). These pathways control cellular functions such as immunity and metabolism and are an indication of the complexity and scale with which Salmonella infection interferes and thwarts host defense mechanisms to establish and maintain long-term cecal colonization.
However, until now, there has been no characterization of the acute host signaling interactome in the chicken intestine during the initial (first 24 h) infection with Salmonella. In the murine Salmonella infection model, Salmonella enterica serovars use self-induced pathologic inflammation which provides a growth advantage in the host gut that favors intestinal colonization (Winter et al., 2010; Winter and Baumler, 2011; Thiennimitr et al., 2012; Patel and McCormick, 2014). An inflammatory response is generated in chickens, but the signaling pathways that are activated or altered are unknown. In this study, we used a chicken-specific global immunologic peptide array to study the changes in cecal immune signaling pathways during the first 24 h following Salmonella Enteritidis infection.
Materials and Methods
Ethics Statement
These studies were approved by the Animal Care and Use Committee (ACUC) at the Southern Plains Agricultural Research Center, Agricultural Research Service, United States Department of Agriculture (ACUC #2012007), which meets all federal requirements as defined in the Animal Welfare Act, the Public Health Service Policy, and the Humane Care and Use of Laboratory Animals.
Experimental Animals
Experiments were conducted according to the regulations established by the United States Department of Agriculture Animal Care and Use Committee. Broiler chickens used in this study were obtained from a commercial breeder and were all the same genetic background and were not vaccinated at any time. Chicks were placed in floor pens containing wood shavings, provided supplemental heat, water, and a balanced, unmedicated corn and soybean meal-based chick starter diet ad libitum that met or exceeded the levels of critical nutrients recommended by the National Research Council (1994). Salmonella was not detected in the feed or from the paper tray liners.
S. Enteritidis Challenge
A poultry isolate of Salmonella enterica serovar Enteritidis (S. Enteritidis; (ID 9711771, part 24) was obtained from the National Veterinary Services Laboratory (Ames, IA, USA), and was selected for resistance to nalidixic acid and novobiocin and maintained in tryptic soy broth (Difco Laboratories, Sparks, MD, USA) containing antibiotics (20 μg/mL nalidixic acid and 25 μg/mL novobiocin; Sigma Chemical Co.; St. Louis, MO, USA). A stock culture was prepared in sterile PBS and adjusted to a concentration of 1 x 109 colony forming units (CFU/mL). The viable cell concentration of the challenge dose for each experiment was determined by colony counts on XLT4 agar base plates with XLT4 supplement (Difco) and nalidixic acid and novobiocin (XLT-NN).
Experimental Design
One-day-old broiler chickens were randomly distributed into two experimental groups: non-infected control and infected. Each group contained 15 birds fed a balanced, unmedicated corn and soybean meal-based diet. Two days post-hatch, all chickens were orally challenged with either 1 mL of 5 x 106 CFU/mL S. Enteritidis (infected group) or mock challenged with 1 mL sterile PBS (non-infected group). At 4, 8 and 24 hours after challenge, 4 chickens from each group were humanly euthanized; all euthanasia procedures followed the guidelines set down in the American Veterinary Medical Association (AVMA) Guidelines for the Euthanasia of Animals. Cecal contents were analyzed for S. Enteritidis colonization and cecal tissue was flash frozen in liquid nitrogen and stored at -80°C for use in the peptide arrays. The experiment was conducted three times. Therefore, the ceca from a total of 15 chickens for each of the 2 groups (5 chickens each in 3 experiments) were used.
Sample Collection to Confirm Bacteria Presence
The ceca from each chicken were removed aseptically, and the contents (0.25 g) were added to tetrathionate broth for enrichment, as well as serially diluted to 1:10, 1:100, 1:1,000, or 1:10,000, and spread onto selection plates. Diluent was produced by adding tissue to 1 mL Butterfield’s Buffered Phosphate Diluent [Sigma Aldrich (St. Louis, MO)]. Selection antibiotics for SE included 20 μg/mL naladixic acid and 25 μg/mL novobiocin. The plates and enrichment tubes were incubated at 37°C for 24 h. The presence of resistant SE per gram of cecal contents was determined by colony counts on the plates. Contents of enrichment tubes were plated and incubated for an additional 24 h.
Statistical Analysis of SE Cecal Colonization
Statistical differences between groups were determined by analysis of variance (P ≤ 0.05). Means were further separated for significance with a pair-wise multiple comparison procedure (Tukey test, P ≤ 0.05). Chi square analysis was used to determine significant differences between groups in Salmonella cecal colonization rates. Differences were significant based on the 0.05 level of probability. The enrichment data were expressed as positive/total chickens (%) and the percent recovery of S. Enteritidis was compared using the chi-squared test of independence to determine the significance (P ≤ 0.05) for these studies.
Chicken-Specific Kinome (Peptide) Array
For the phenotype readout, a peptide array was used to provide tissue immunometabolism information from the host. At 3 time points (4, 8, and 24 h), 3 whole ceca from 3 randomly selected birds were defrosted for analysis (27 samples total for all 3 d). Each sample was weighed to obtain a consistent 40-mg sample for the array. The samples were homogenized with the Omni International Bead Ruptor Elite (Kennesaw, GA) in 100 mL of lysis buffer (20 mmol Tris-HCl pH 7.5, 150 mmol NaCl, 1 mmol EDTA, 1 mmol ethylene glycol tetraacetic acid, 1% Triton X-100, 2.5 mmol sodium pyrophosphate, 1 mmol Na3VO4, 1 mmol NaF, 1 mg/mL leupeptin, 1 g/mL aprotinin, and 1 mmol phenylmethylsulfonyl fluoride). All products were obtained from Sigma Aldrich (St. Louis, MO), unless indicated. After homogenization, the peptide array protocol was carried out according to Jalal et al. (2009) with alterations described in the study by Arsenault et al. (2017). The resulting tissue lysates were applied onto the PepStar peptide microarrays customized by JPT Peptide Technologies GmbH (Berlin, Germany) and incubated in a sealed container placed in a 5% CO2 incubator at 37°C for 2 h. After incubation, sample residues were washed off the arrays and the arrays were stained in phospho-specific fluorescent ProQ Diamond Phosphoprotein Stain (Life Technologies, Carlsbad, CA, USA) for 1 h. The arrays were submerged in a destain solution containing 20% acetonitrile (EMD Millipore Chemicals, Billerica, MA, USA) and 50 mM sodium acetate (Sigma-Aldrich, St. Louis, MO, USA) to remove non-phospho-specific binding. The arrays were scanned in a Tecan PowerScanner microarray scanner (Tecan Systems, San Jose, CA, USA) at 532 to 560 nm with a 580-nm filter to detect dye fluorescence. The images of the scanned array were gridded manually to fit the phospho-specific spots and extract signal intensity using GenePix Pro software (version 7.2.29 1, Molecular Devices, CA, USA). Microsoft Excel 2016 (Redmond, WA).
Data Analysis: Kinome Array
Data normalization was performed for the kinome array, based on the study by Li et al. (2012) using the PIIKA2 online platform (http://saphire.usask.ca/saphire/piika/index.html), a tool designed for in silico analysis of phosphorylation sites (Trost et al., 2013). The array data were analyzed by conducting variance stabilization normalization and then performing t test, clustering and pathway analysis for statistical data. Gene ontology and Kyoto Encyclopedia of Genes and Genomes (KEGG) pathway analysis were performed by uploading the statistically significant peptide lists to the Search Tool for the Retrieval of Interacting Genes (STRING) (Szklarczyk et al., 2017).
Results
Salmonella Infection
At 4-, 8-, and 24-hours post-infection, animals were sacrificed, and samples collected. Infection status was confirmed by Salmonella Enteritidis culturing of cecal contents and feces from each animal with and without enrichment. Cultures confirmed that the infected group displayed Salmonella infection throughout the 24 h experiment, while Salmonella was not isolated from animals in the control group (Table 1).
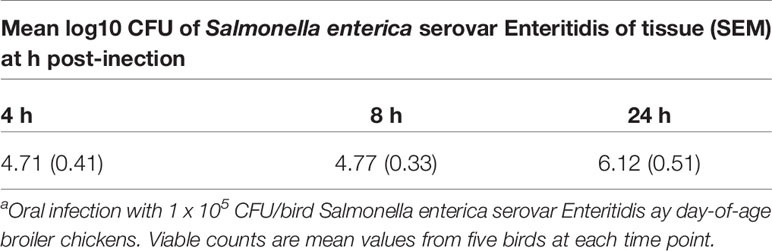
Table 1 Number of Salmonella organisms in cecal contents of chickens after oral administration on day 1 of agea.
Kinome Arrays
Kinome analysis was carried out on the cecal samples from non-infected and infected chickens. The results from three animals from each group (S. Enteritidis-infected and non-infected) and time point were combined to provide a representative result. To remove any non-specific or baseline phosphorylation signal from the analysis data from each time point was corrected using the matched uninfected controls. The kinome data were subjected to pathway overrepresentation analysis to determine which cellular pathways/processes are altered under the infected and non-infected conditions. To ensure that the identified pathways represent conserved and consistent biological responses, input data were limited to peptides with a consistent pattern of differential phosphorylation across the three biological replicates in at least one of the treatment sets as well as significant changes (p ≤ 0.05) in phosphorylation level relative to the non-infected control treatment. These select data from the three animals were merged to generate a representative data set for each treatment condition. All peptides that showed significant phosphorylation changes relative to control (p ≤ 0.05) for each time point were input into the Search Tool for the Retrieval of Interacting Genes/Proteins (STRING) database (Szklarczyk et al., 2017). Using STRING functionality, KEGG pathway results were generated for each dataset.
Biological Process Analysis
We next considered the individual peptide phosphorylation results generated by the PIIKA2 online analysis platform (Trost et al., 2013). These data are a series of fold changes and significance values for each peptide on the array from each sample analyzed. These values are generated by comparing treatment/challenge tissue array outputs to non-challenged control array outputs. The STRING protein-protein interaction database (Szklarczyk et al., 2017) generates GO Biological Process terms and a false discovery rate (FDR) which indicates the likelihood of a biological process being truly represented in the data and not generated by random chance. The top 10 biological processes for each group were compared to find the differences between the lists generated for Salmonella-challenged group and the non-infected control group (Table 2). The results provided convincing evidence for the significant involvement of the local cecal immune response, specifically the innate response, during the first 24 h post-infection with S. Enteritidis.
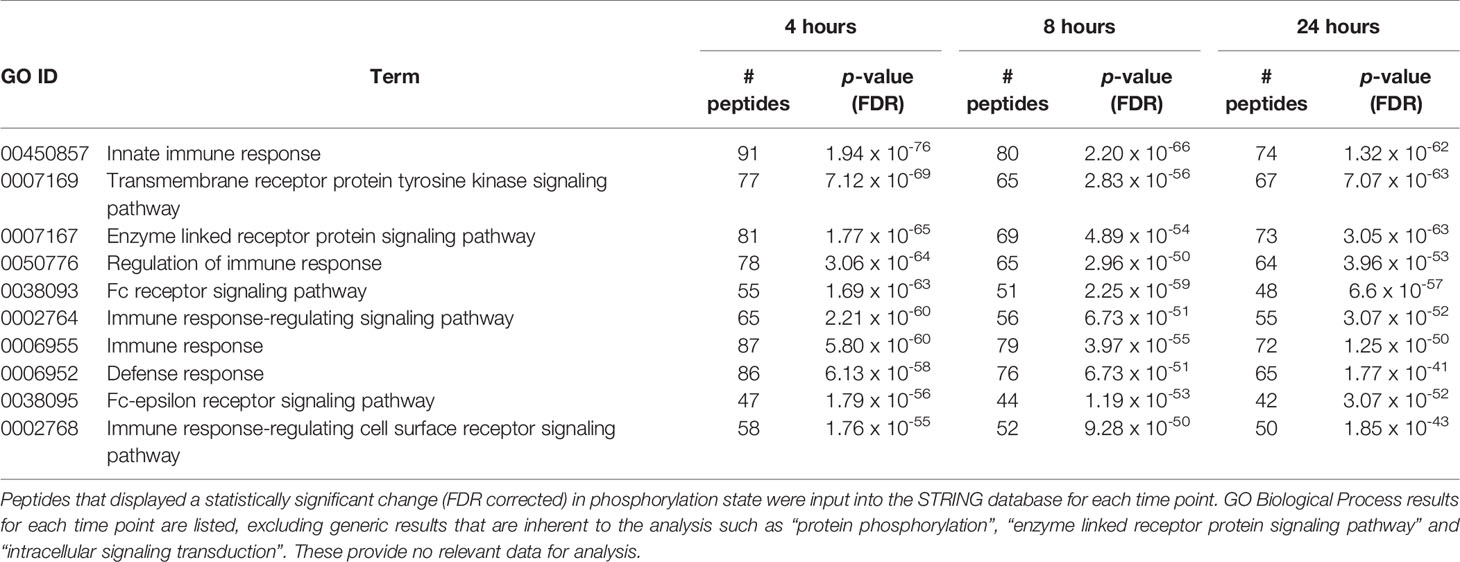
Table 2 Top 10 GO Biological processes altered between the Salmonella-infected and noninfected groups at 4, 8 and 24 h post-infection from STRING analysis.
The STRING generated KEGG pathway results showed several pathways altered by the S. Enteritidis infection at a statistically significant level (p ≤ 0.05 false discovery rate (FDR) corrected). Of particular interest were the immune pathways that contained substantial numbers of peptides that were significantly differentially phosphorylated at multiple times over the course of the study. A subset of these pathways is shown in Table 3. Specifically, there were a total of 139 different significantly altered phosphorylation events within these 6 immune signaling pathways at the 4-hour post-infection time point, 120 at the 8-hour time point and another 124 after 24 h post-infection.
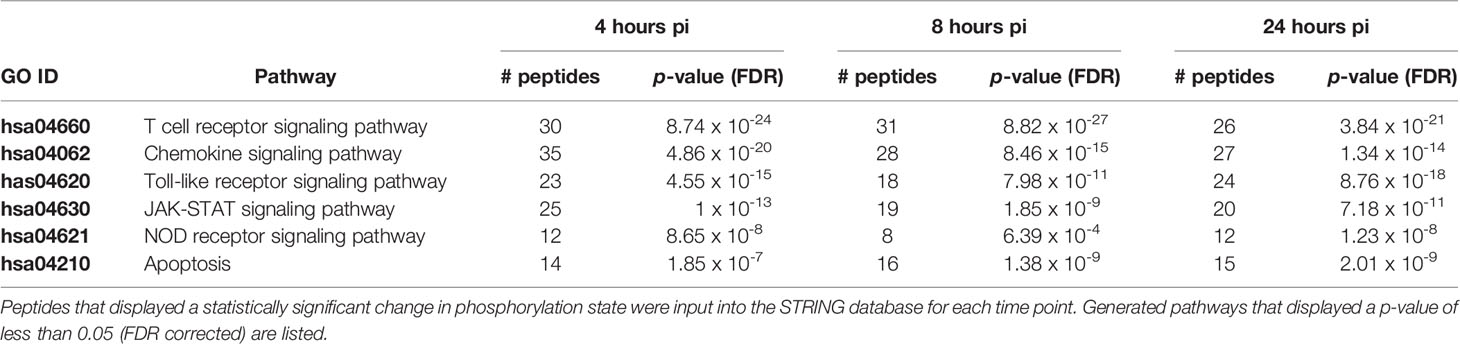
Table 3 Kegg pathways generated by Search Tool for the Retrieval of Interacting Genes/Proteins (STRING) with consistent altered phosphorylation across all time points.
Phosphorylation Events Within Specific Pathways
Toll-Like Receptor Pathway
Toll-like receptors (TLRs) initiate a rapid activation of innate immunity by inducing production of pro-inflammatory cytokines and chemokines. As shown in Table 4 and Supplemental Figure 1, TLR signaling pathways in the cecum phosphorylated by S. Enteritidis infection appears to be MyD88-dependent with: (1) the phosphorylation (activation) of NF-κB and MAPK signaling pathways and (2) associated with the phosphorylation of and IRF7 induction of interferon-α/β and the activation of the JAK-STAT pathway through the phosphorylation of IFNAR1 receptor.
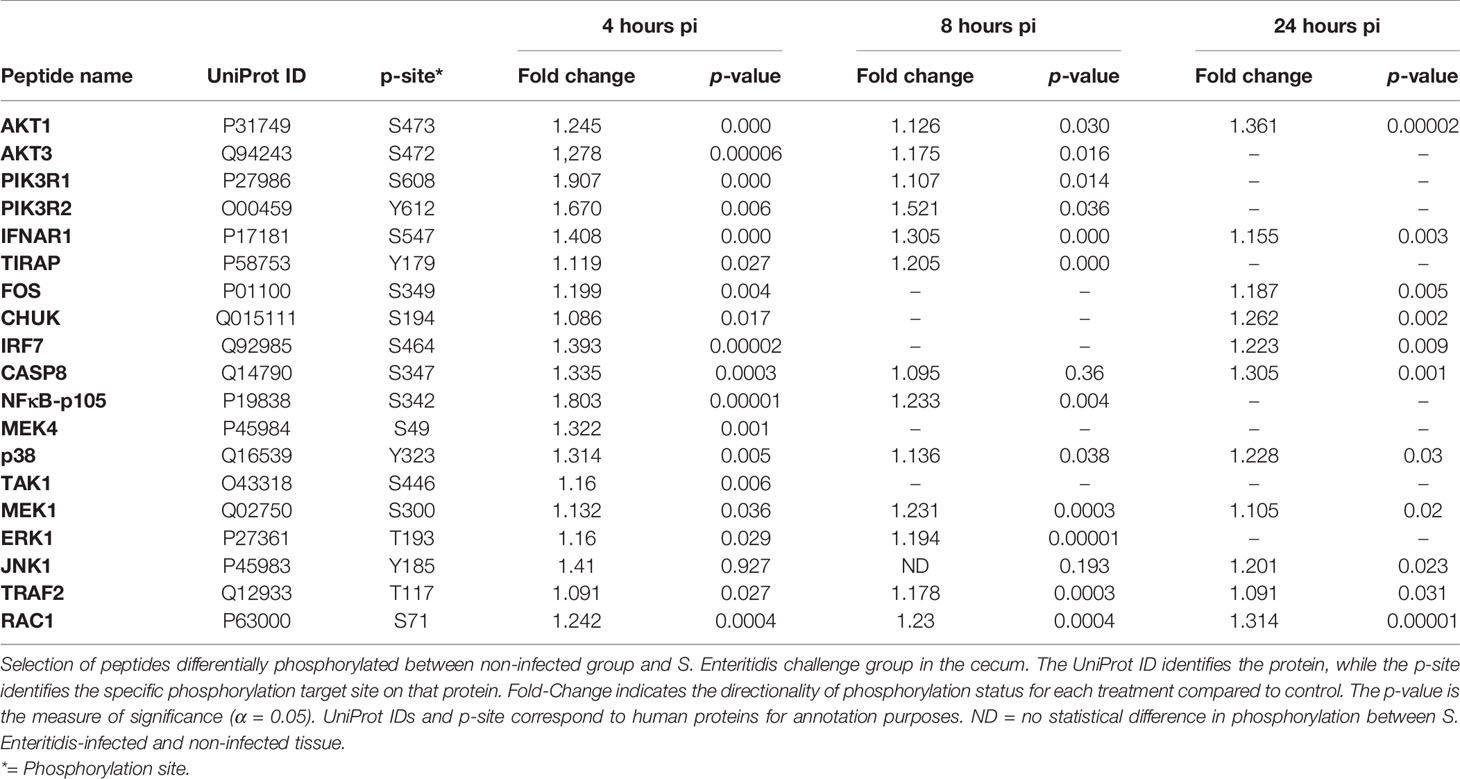
Table 4 Members of the TLR signaling pathway showing differential phosphorylation between Salmonella enterica serovar Enteritidis-infected cecal tissue and non-infected cecal tissue.
NOD-Like Receptor Pathway
Cecal infection by S. Enteritidis induced the activation of the NOD-like receptor (NLR) signaling pathway within 4-hours post-infection probably via the prototypic NOD-1 pathway which sense the cytosolic presence of bacterial peptidoglycan fragments that escaped from endosomal compartments, driving the activation of both the NF-κB and MAPK signaling pathways. Based on the phosphorylation events shown in Table 5 and Supplemental Figure 2, the NOD-1 pathway is characterized by the phosphorylation of RIP2, CARD9, TRAF2, TAK1, TAB that leads to the activation of IκB that activates the NF-κB pathway that leads to pro-inflammatory cytokine and chemokine production and the phosphorylation (activation) of the MAPK (ERK1/3, JNK1, and p38 proteins) and AP-1 (FOS and JUN) signaling pathways that results in pro-inflammatory cytokine and chemokine production (Table 5; Supplemental Figure 3).
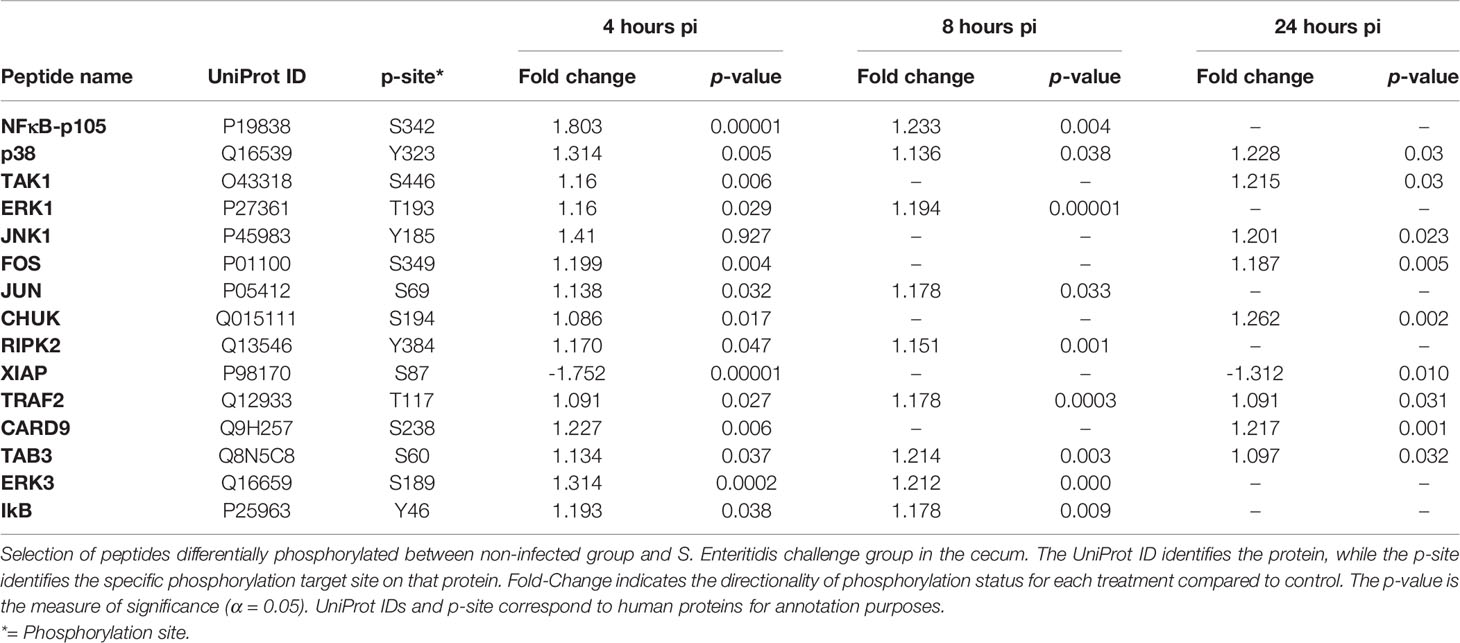
Table 5 Members of the NOD-like receptor signaling pathway showing differential phosphorylation between Salmonella enterica serovar Enteritidis-infected cecal tissue and non-infected cecal tissue.
Chemokine Signaling Pathways
Following infection with S. Enteritidis, a number of chemokine signaling-associated pathways were found to be altered when compared to the non-infected control tissues: MAPK-JAK-STAT signaling pathways (Table 6A), the PI3K signaling pathway (Table 6B), and the CCR5P-PLC-PKC signaling pathway (Table 6C) (Supplemental Figure 3). The most consistent observation in all three downstream pathways regardless of ultimate functional activity is that there was a change in the phosphorylation state of a significant number of proteins within the pathway induced within 4 hours of infection, maintained through 8 hours post-infection, but essentially returned to control levels by 24 hours post-infection. These data provide evidence that the NF-κB-mediated activation of pro-inflammatory functions induced by the MAPK-JAK-STAT signaling pathways (Table 6A), the actin cytoskeleton regulation and leukocyte migration activity mediated by the PI3K signaling pathway (Table 6B), and the migration, NO induction, and ROS production mediated by the CCR5P-PLC-PKC signaling pathway (Table 6C) are rapidly induced by the host in response to the bacterial colonization. Chemokine-mediated signaling leading to cytoskeletal rearrangements allow cell polarization towards the chemokine gradient that will lead to acquisition of a migratory phenotype (Wang et al., 2013).
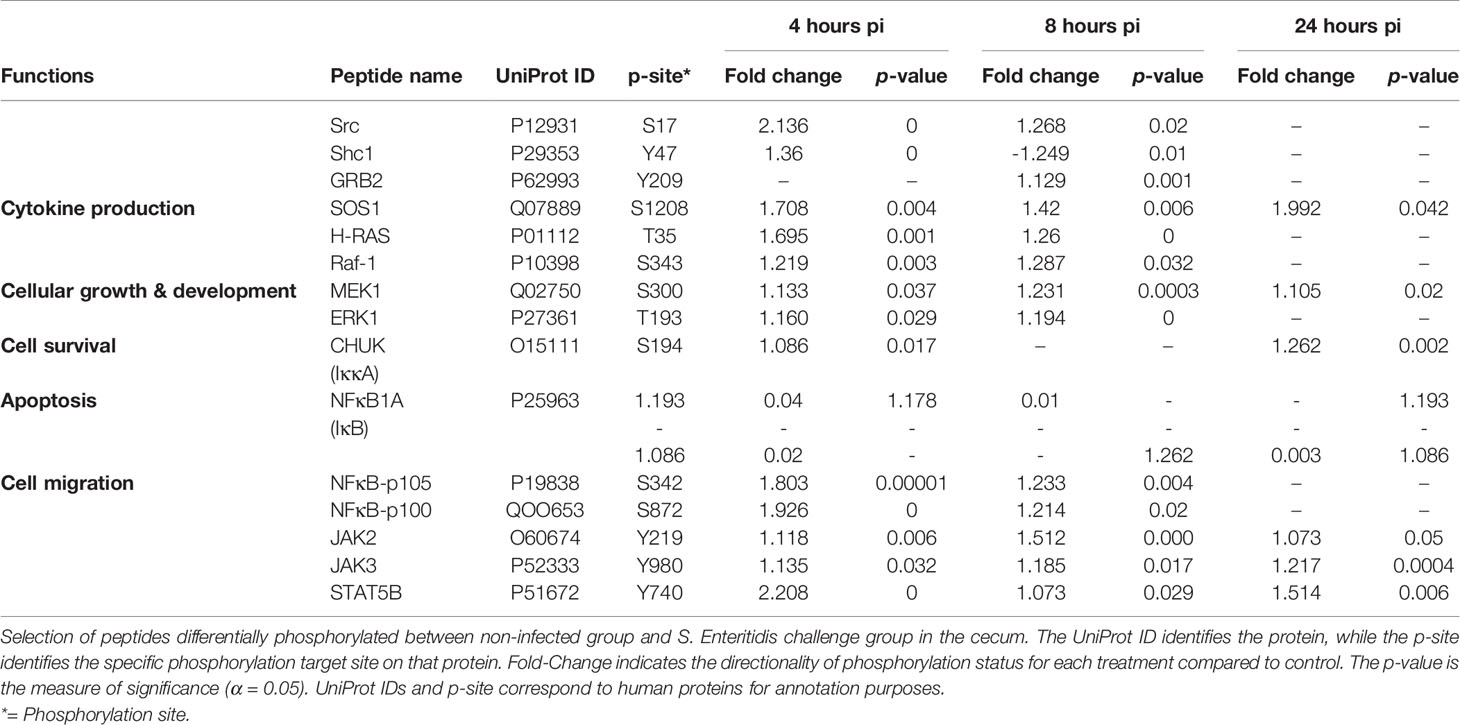
Table 6A Members of the chemokine signaling pathway showing differential phosphorylation between Salmonella enterica serovar Enteritidis-infected cecal tissue and non-infected cecal tissue: MAPK and JAK-STAT signaling pathways.
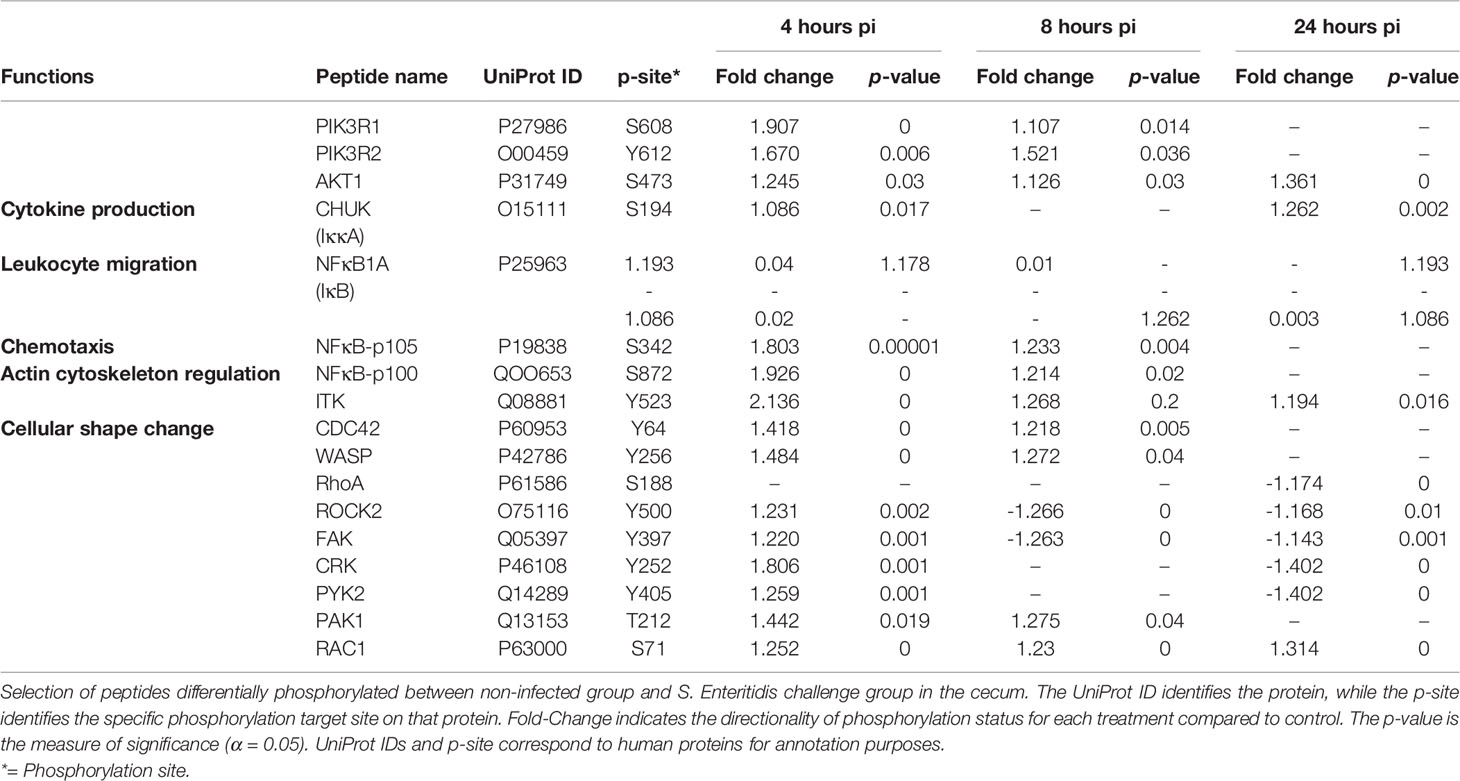
Table 6B Members of the chemokine signaling pathway showing differential phosphorylation between Salmonella enterica serovar Enteritidis-infected cecal tissue and non-infected cecal tissue: PI3K signaling pathway.
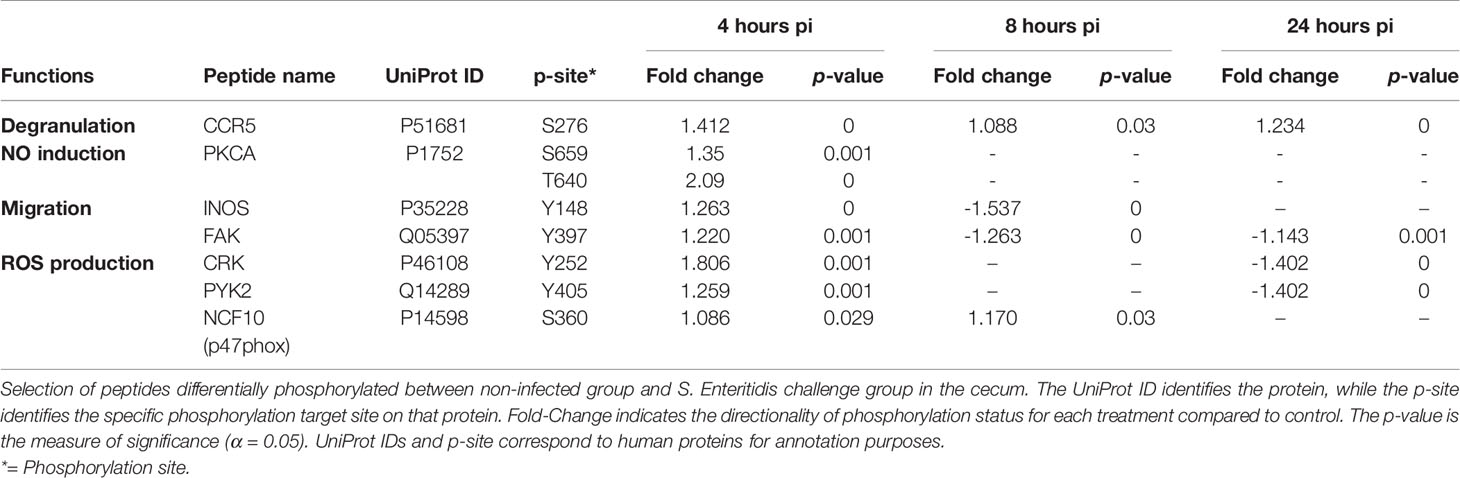
Table 6C Members of the chemokine signaling pathway showing differential phosphorylation between Salmonella enterica serovar Enteritidis-infected cecal tissue and non-infected cecal tissue: CCR5P-PLC-PKC signaling pathway.
Apoptosis Signaling Pathway
Early infection of the chicken cecum by S. Enteritidis appears to trigger apoptosis via both intrinsic and extrinsic pathways (Table 7; Supplemental Figure 4). Both the Fas and TNFR1 death receptors were activated by phosphorylation by 8 h post-infection. The extrinsic pathway via Fas is stimulated by the binding of the Fas ligand to the Fas receptor which the phosphorylated FADD (Fas-associated death domain) which activates (phosphorylates) the initiator caspase, caspase 8 (CASP8). CASP8 the activates (phosphorylates) caspase 3 that results in the subsequent apoptosis. Remarkably, the TNFR1 receptor appears to activate both the extrinsic and intrinsic apoptosis pathways during early infection of the cecum by S. Enteritidis. Like the Fas receptor, TNFR1 activates the extrinsic pathway by activating the FADD →CASP8→CASP3 cascade. TNFR1 also activates the intrinsic pathway by activating (phosphorylating) the RIPK1 (receptor interacting serine/threonine protein kinase 1) and TRAF2 (TNF-R associated 2) adaptor protein complex. This complex phosphorylates ASK (Apoptosis signal-regulating kinase 1) which activates c-Jun N-terminal kinase (JNK). JNK then activates the two pro-apoptotic proteins BAD (BCL2 associated agonist of cell death) and BID (BH3 interacting-domain death agonist) which initiate apoptosis by forming a pore in the mitochondrial outer membrane that allows cytochrome c to escape into the cytoplasm and activate the pro-apoptotic caspase cascade. Lastly, the X-linked inhibitor of apoptosis protein (XIAP), whose function when phosphorylated, is to inhibit apoptosis was, in fact, dephosphorylated in the S. Enteritidis-infected tissue. The dephosphorylation of XIAP blocks the activation of an apoptosis inhibitory pathway during Salmonella infection.
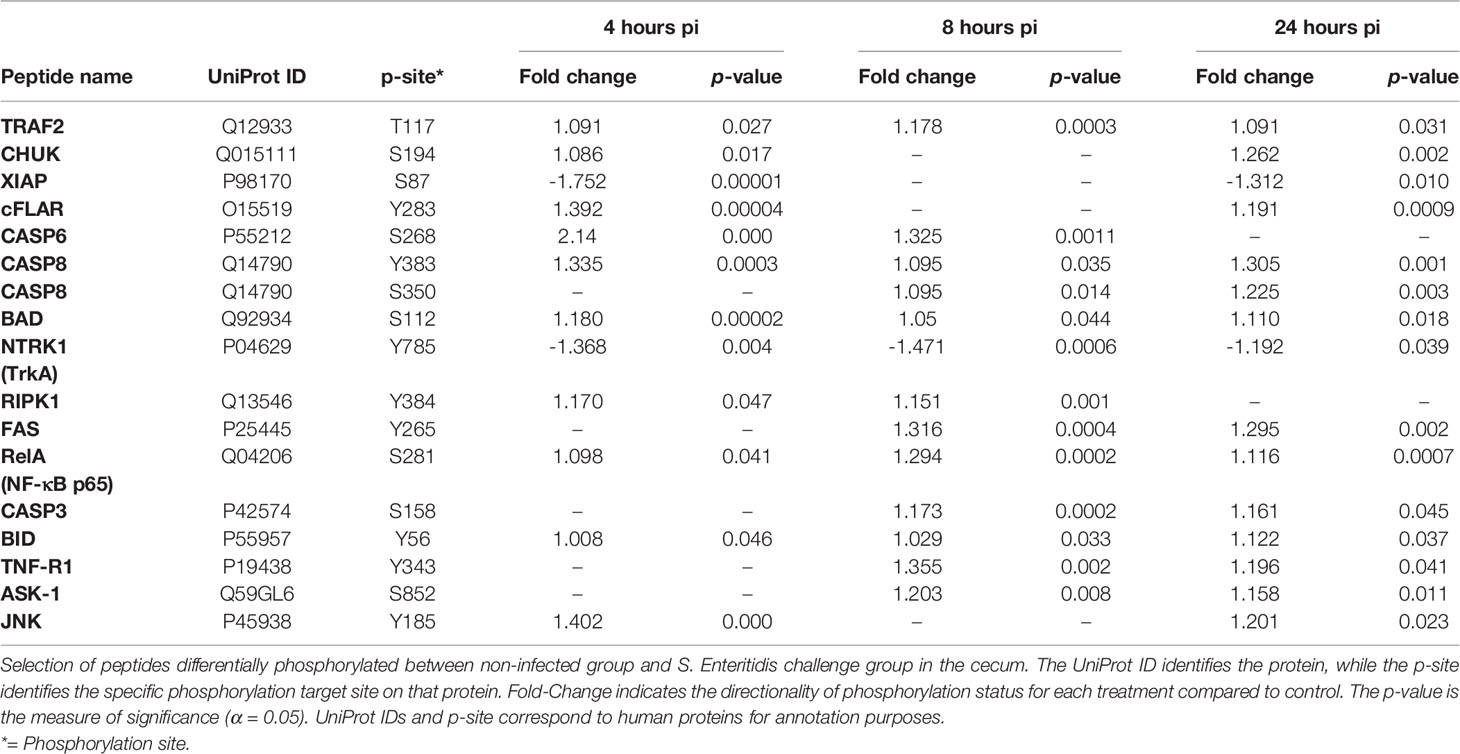
Table 7 Members of the apoptosis signaling pathway showing differential phosphorylation between Salmonella enterica serovar Enteritidis-infected cecal tissue and non-infected cecal tissue.
JAK-STAT Signaling Pathway
The Janus kinase (JAK)/signal transducer and activator of transcription (STAT) signaling pathway is a mutual conduit of many cytokine signal transductions involved in cell proliferation, apoptosis, differentiation, and inflammatory response. The JAK-STAT pathway is fundamental for inhibiting the inflammatory response, initiating innate immunity, and coordinating adaptive immune mechanisms (O’Shea et al., 2015). Within the first 24 hours post-infection, a differentiated series of phosphorylation events occurred at the cytokine receptor level in the S. Enteritidis-infected birds when compared to the non-infected control birds (Table 8). First, a significant increase in phosphorylation of the interferon-alpha receptor (IFNAR1), interferon-gamma receptor (IFNGR1), interleukin-2 receptor (IL2RB), and IL6ST (gp130) (Table 8). Simultaneously, there is a significant decrease on the phosphorylation of the IL-4 receptor (IL-4R) at all time points. Interestingly, there was no change in the phosphorylation of the IL-7 receptor (IL-7R) from the non-infected controls at 4 and 8 h pi, but was significantly dephosphorylated at 24 h p.i. The IL10R was only significantly dephosphorylated at 4 h pi with no change from the control at 8 and 24 h p.i. (Table 8).
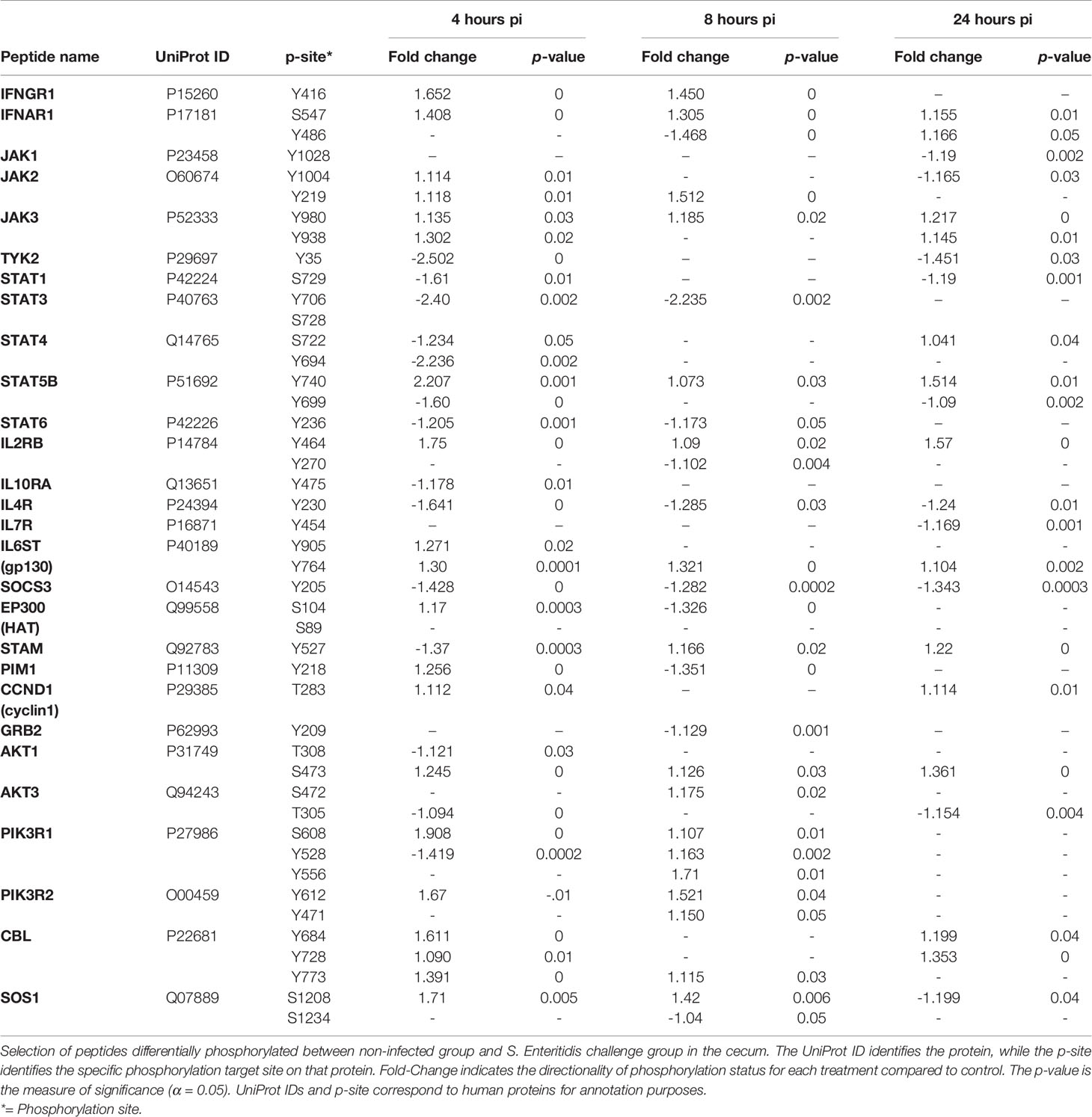
Table 8 Members of the JAK-STAT signaling pathway showing differential phosphorylation between Salmonella enterica serovar Enteritidis-infected cecal tissue and non-infected cecal tissue.
Early infection of the cecum by S. Enteritidis appears have differential effects in the JAK kinases. JAK1 and TYK2 are targets for dephosphorylation at 4 h and 4-24 h p.i., respectively; whereas JAK2 and JAK3 were significantly phosphorylated by S. Enteritidis infection when compared to the non-infected controls (Table 8).
Additionally, early infection of the cecum by S. Enteritidis appears to target most STAT proteins (STAT1, STAT3, STAT4, STAT6) which were all significantly dephosphorylated during the first 24 h p.i. The lone exception was STAT5B which was significantly phosphorylated at Tyr749 at all three early post-infection time points following infection with S. Enteritidis.
It should also be noted that the suppressor of cytokine signaling 3 (SOCS3) was dephosphorylated during the entire initial 24 hours of S. Enteritidis infection.
T Cell Receptor Signaling Pathway
T cells need two signals to become fully activated. Signal 1 is provided by the T-cell receptor when recognizing a specific antigen associated with major histocompatibility complex molecules. Signal 2 comes from co-stimulatory receptors such as CD28, presented on the surface of other immune cells. It is expressed only when an infection was detected by the innate immune system. Engagement of the T cell receptor through these 2 signals results in a series of signaling cascades that lead to T-cell proliferation, cytokine production and differentiation into effector cells (Smith and Gobel, 2022). These protein cascades lead to the activation of transcription factors. Transcription factors involved in T cell signaling pathway are the NFAT, NF-κB and AP1 (Smith and Gobel, 2022). Specifically, TCR activation results in the phosphorylation and activation of the lymphocyte-specific protein tyrosine kinase. Phosphorylation of Lck can lead to the activation of two transcription factors, NFAT via phospholipase C and AP-1 through MAPK signaling pathway (Salmond et al., 2009; Smith-Garvin et al., 2009; Nika et al., 2010; Van der Merwe and Dushek, 2011). The third transcription factor, NF-kB can be activated through the stimulation of the co-stimulatory molecule CD28 via the phosphoinositide 3-kinase (PI-3K) pathway (Smith and Gobel, 2022).
Analysis of the T cell receptor signaling pathway revealed several significant changes in phosphorylation events (Table 9):
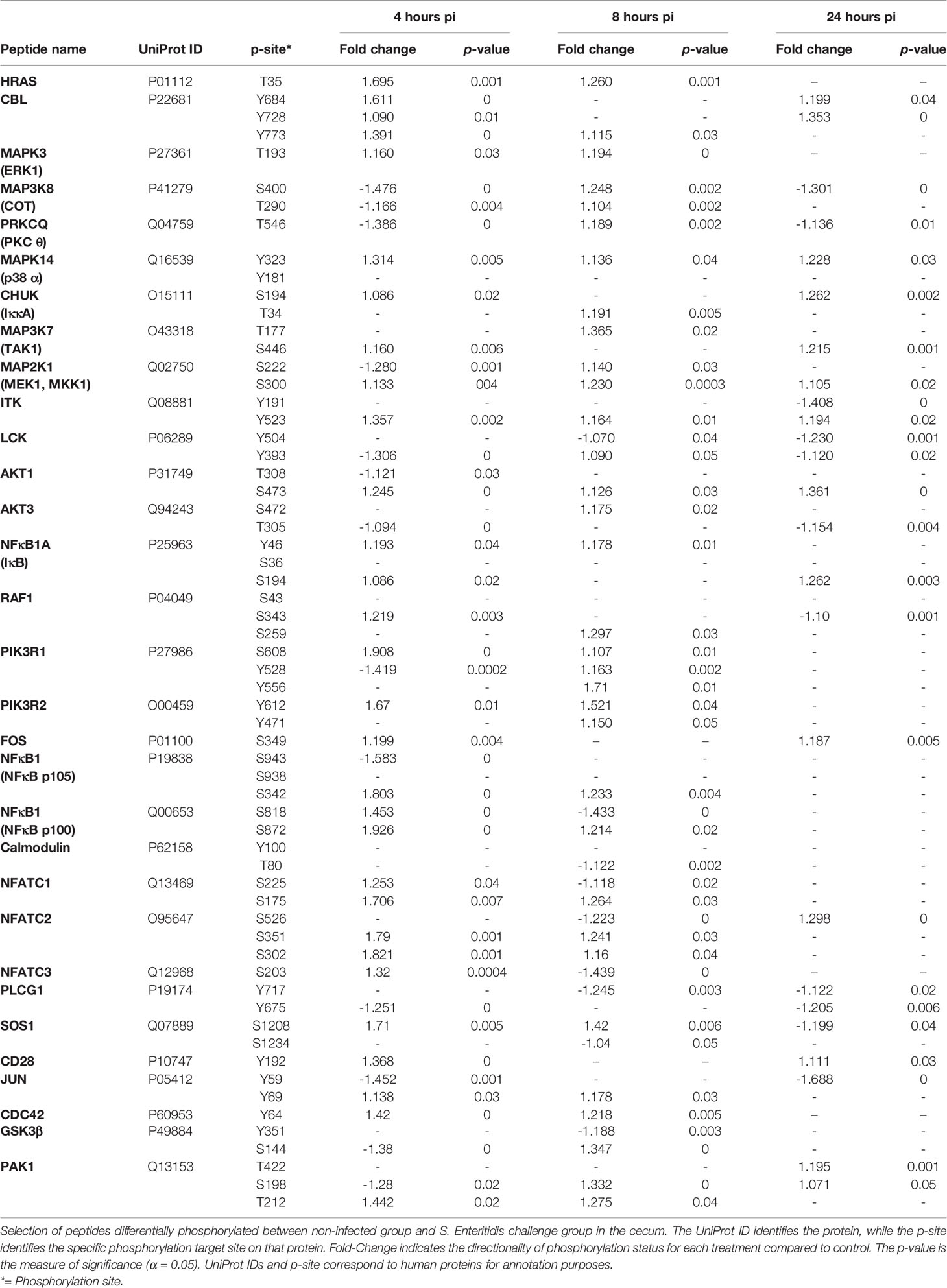
Table 9 Members of the T cell receptor signaling pathway showing differential phosphorylation between Salmonella enterica serovar Enteritidis-infected cecal tissue and non-infected cecal tissue.
First, the three transcription factors (NFAT, AP-1, and NF-κB) exhibited differential phosphorylation activity within 4-8 h post infection with S. Enteritidis with a return to control levels by 24 h (Table 9). The significant phosphorylation of the members of the NFAT family n the ceca of S. Enteritidis-infected chickens (Table 9) is a meaningful finding because inactivated NFAT proteins in the cytoplasm of a cell are in their phosphorylated form. Following T cell receptor (TCR) stimulation, cytoplasmic NFAT proteins are dephosphorylated and translocate from the cytoplasm to the nucleus where they regulate transcription of key cytokine genes. Thus, based on the findings here the increased phosphorylation of NFAT prevents the protein from translocating to the nucleus. The significant phosphorylation of the adapter protein GRB2, SOS, and the MAPKs (MEK1, ERK1, and p38) and the JUN and FOS proteins demonstrates the activation of the transcription factor, AP-1. AP-1 controls a number of cellular processes including differentiation, proliferation, and apoptosis (Karin et al., 1997). Lastly, The Ser/Thr kinase COT, CHUK (Iκκ-A), NF-κB1A (IκB), and NF-κB (p105 and p100), all significantly phosphorylated within 4-8 h of infection with S. Enteritidis (Table 9), but all were found to be no different than non-infected control levels by 24 h (Table 4). Both NFAT and NF-κB are transcription factor that when phosphorylated can regulate genes responsible for both the innate and adaptive immune responses when activated by various intra- and extra-cellular stimuli then translocate into the nucleus and stimulates the expression of genes involved in a variety of immune functions (Zanoni and Granucci, 2012; Banoth et al., 2015). Secondly, phospholipase C-1 (PLCG1) and calmodulin were significantly dephosphorylated in the S. Enteritidis-infected cecal tissue by 4 hours post-infection when compared to the non-infected control cecal tissue. The importance of this is twofold: PLCG1 generates second messenger molecule inositol 1,4,5-trisphosphate (IP3) (Essen et al., 1997). IP3 binds to calcium channel receptors on the endoplasmic reticulum (ER) induces the release of calcium (Ca2+) into the cytosol of the T cell.
Discussion
Understanding both sides of the host –Salmonella interaction is essential in discovering alternative strategies to manage infections in poultry. The host’s antimicrobial immunity and the bacteria’s evasion of that immunity both rely on appropriate regulation of transcriptional and signaling networks. To date, the majority of the studies in the chicken-Salmonella interaction has been microbe-centric, concentrating on the pathogen’s infection of the bird intestine by using the Salmonella pathogenicity islands 1 and 2 (SPI-1 and SPI-2) that encode a specialized type III secretion system (T3SS) to secrete multiple protein effectors into the intestinal epithelium which manipulate the host cell biology to aid in bacteria invasion, intracellular survival, and to modulate host immune responses (Barrow et al., 2012; Figueira and Holden, 2012; Wigley, 2014; Eng et al., 2015). On the host side of the interaction, the transcriptional and signaling descriptions of the interaction has concentrated on the post-infection period starting 2 days after the initial infection by the pathogen. This breach results in a directed innate immune response to purge the bacterium and includes the release of antimicrobial peptides (Crhanova et al., 2011; Garcia et al., 2020), activation of pattern-recognition receptors (Keestra et al., 2013, Kogut, 2022a; Kogut, 2022b), release of chemokines and cytokines (Withanage et al., 2005; Bernd et al., 2007; Rychlik et al., 2014; Meijerink et al., 2021), and recruitment of a variety of immune cells (Van Immerseel et al., 2003; Bernd et al., 2007; Pieper et al., 2011; Meijerink et al., 2021).
Therefore, what is needed is more insight into the immediate response of the chicken to the Salmonella infection process (first 24 hours). For example, chickens infected with Salmonella do not develop clinical disease and the acute inflammatory response is short-lived (Wigley, 2014). Thus, it is vital to understand how the chicken responds to a Salmonella breach of the mucosal barrier system and what is involved in the regulation of the intestinal response that prevents excessive intestinal inflammation and damage yet does not eliminate pathogen colonization. Our recent development of chicken-specific peptide arrays for kinome analysis of host phosphorylation-based cellular signaling responses provided us with the opportunity to develop a more detailed understanding of the chicken host-pathogen interactions with Salmonella (Arsenault and Kogut, 2015; Perry et al., 2020). We have used this technology to describe the immunometabolic phenotypic changes in the avian cecum of Salmonella-infected chickens that decreased the host responsiveness resulting in the establishment of persistent colonization starting around 4 days after the initial infection (Kogut et al., 2016).
Overall, in all the pathways described here, the majority of the differential phosphorylation events between the infected cecal tissue compared to the non-infected control tissue occurred with the first 4-8 hours post-infection. In general, from 8-24 hours post-infection, there was a decrease in phosphorylation alterations in the infected tissue with a return to control levels of phosphorylation. Based on the signaling pathways that were differentially activated (TLR, NOD, chemokine, apoptosis, JAK-STAT), the chicken responds very rapidly with an orchestrated innate response: recognition (TLR and NOD signaling pathway), attraction of activated immune cells (chemokine and JAK-STAT signaling pathways), and the prevention/reduction in pathogen numbers (apoptosis).
Based on the findings described here, the chicken pattern recognition receptors, Toll-like receptors (TLR) and nucleotide-binding oligomerization domains receptors (NOD), recognized molecular associated microbial patterns (MAMPs) associated with S. Enteritidis (Tables 4, 5; Supplemental Figures 1 and 2). The activity of both pathways was activated (phosphorylated) 4-8 hours post-infection and their activity decreased by 24 post-infection. Pathways analysis suggests that NOD1 and NOD2 are specifically involved in the immediate recognition of a Salmonella in chickens. NOD1 and NOD2, two prototypic NLRs, sense the cytosolic presence of bacterial peptidoglycan fragments that escaped from endosomal compartments, driving the activation of NF-κB and MAPK, cytokine production and apoptosis (Li et al., 2017; Tao et al., 2017). However, NOD2 appears to be absent in birds (Boyle et al., 2013). There is no evidence, based on the pathway analysis, that inflammasome-mediated pathways were involved the chicken recognition of S. Enteritidis.
Although kinome analysis of the S. Enteritidis-infected tissue does not provide the specific TLR that initiated the TLR signaling pathway in the chicken, previous studies have convincingly shown that recognition of flagellin by TLR5 is the primary recognition receptor that stimulates the bird’s innate response (Iqbal et al., 2003). Is also possible that TLR4 that recognizes LPS on the Salmonella cell wall and TLR21 which recognizes CpG (bacterial unmethylated DNA) could be involved in initiating the TLR signaling pathway as both have been found in chickens (Higgs et al., 2006). However, chickens are very refractory to LPS except at high levels not found in bacterial cell walls (Adler and DaMassa, 1979; Keestra and Van Putten, 2008).
Chemokines are small chemoattractant peptides that provide directional cues for the cell trafficking and thus are vital for protective host response. In addition, chemokines regulate several biological processes of hematopoietic cells that lead to cellular activation, differentiation and survival. The chemokine signal is transduced by chemokine receptors expressed on the hematopoietic immune cells. After receptor activation, several diverse downstream pathways are activated which encompass a number of biological activities of hematopoietic cells that lead to cellular activation, differentiation and survival. Based on the findings here, the kinomic analysis of the chemokine signaling pathway demonstrates the activation of three downstream pathways: (1) the MAPK/JAK-STAT cascades involved in cell migration, cytokine production, and cell survival, (2) the PI3K → AKT→ Itk cascade that regulates leukocyte transmembrane migration and actin reorganization, and (3) the CCL5 → PLC → PKC cascade that causes the induction of nitric oxide, production of reactive oxygen species, and degranulation (Hughes et al., 2001; Wang et al., 2005; Sick et al., 2006; Hughes et al., 2007). All three cascades were highly phosphorylated in the 4 h post-infection cecal tissue when compared to the non-infected control tissue. From 8-24 h post-infection, there is a dramatic increase in either dephosphorylation events or no differences from the control inn the S. Enteritidis-infected tissues. The kinome analysis of the timing of the chemokine signaling pathway coincides with our previous studies where we found that paratyphoid Salmonella serovars induced a rapid (within 4 h) inflammatory response characterized by an influx of heterophils (the primary granulocytic cell the gastrointestinal tract of chickens (Kogut et al., 1993; Kogut et al., 1994; Genovese et al., 1999; Swaggerty et al., 2006).
Apoptosis is a genetically regulated process of caspase-dependent form of cell death of damaged or infected cells in response to intrinsic or extrinsic signaling cascades (Galluzzi et al., 2018; Wemyss and Pearson, 2019). Typically, in the intestine apoptosis of epithelial cells from the mucosa occurs with little, if any, inflammation and disruption of the epithelial barrier integrity (Schwerk and Schultze-Osthoff, 2005). However, Salmonella-induced cell death (apoptosis, necroptosis, pyroptosis) are crucial components of bacterial-mediated gastroenteritis in mammals (Kim et al., 1998; Fink and Cookson, 2007; Hefele et al., 2018; Wemyss and Pearson, 2019). Alternatively, the major paratyphoid Salmonella serovar infections of poultry leads to little or no clinical signs of disease (Barrow et al., 2012) which may coincide with the fact that there have been no reports of Salmonella-induced cell death in the chicken intestine during colonization and infection. Based on the findings here, S. Enteritidis infection of the cecum induces the apoptosis signaling pathway when compared to the non-infected control cecal tissue. More specifically, in fact, based on the alterations in phosphorylation, S Enteritidis infection stimulated both intrinsic and extrinsic apoptotic signaling cascades (Table 7; Supplemental Figure 4). The lack of clinical signs of disease in chickens during paratyphoid Salmonella infection, the importance of maintaining both intestinal homeostasis and the intestinal barrier during the initial phase of infection, strongly suggests that the apoptotic pathway outlined here is a host defense strategy to limit bacterial replication and survival (Sellin et al., 2014). Limiting the initial infection and replication of the pathogen may be an important component of the ‘disease tolerance’ mechanism of host defense that is unique to the chicken-Salmonella interaction that we have described previously (Kogut and Arsenault, 2017; Lee et al., 2020). Finally, we found no evidence, based on phosphorylation events, of either of the pro-inflammatory forms of cell death, necroptosis nor pyroptosis in the Salmonella-infected cecal tissues. Specifically, necroptosis is an inflammatory-mediated, caspase-independent cell death mechanism. We have shown clearly (Table 7; Supplemental Figure 4) that caspases 3, 6, and 8 are all phosphorylated; thus activated as part of the extrinsic apoptosis pathway. Further, pyroptosis is a highly inflammatory cell death pathway that requires the development of an inflammasome which mediates the event. We found no evident of an inflammasome development in our studies.
Further kinome pathway analysis of the S. Enteritidis-infected cecal tissue revealed the differential phosphorylation of individual peptides in the JAK-STAT pathway (Table 8). The JAK-STAT pathway is a signaling cascade that provides a direct mechanism to translate an extracellular signal into a transcriptional response, in S. Enteritidis-infected cecal tissue. The JAK-STAT system consists of three main components: (1) a receptor; (2) Janus kinase (JAK); and (3) Signal Transducers and Activator of Transcription (STAT) (Hu et al., 2021). Based on the results from the present experiment (Table 8), the IFN-α, IFN-γ, IL-2, and IL-6 cytokine family (gp130) receptors were phosphorylated; whereas, IL-4, IL-10 were dephosphorylated, and IL-7 receptor showed no change from the non-infected control cecal tissue, at 4 and 8 hours after infection, but was dephosphorylated at 24 h post-infection. IFN-γ is characteristic of a Th1 response whereas IL-2 is normally produced by T cells during an immune response and involved in growth, proliferation, and differentiation of T cells to become “effector” T cells (Cantrell and Smith, 1984; Smith, 1988). Traditionally, IL-6 is a pro-inflammatory cytokine involved in stimulating an immune response during infection which may very well be the case with these findings. However, we also consider strong evidence that IL-6 is involved in metabolic function, rather than just immune response. IL-6 induction has been found to stimulate a metabolic reprogramming [Pavlov and Tracey (2012); Flint et al., 2016 and Ghanemi and St Amand (2018)] which could be happening here with the initiation of an innate response to Salmonella infection. Salmonella infection activates the innate immune system as described above but activating the innate immune system places considerable energy and resource demands on the chicken that requires amplified metabolic requirements and nutrient consumption (Klasing, 2007; Klasing, 2017). The increased phosphorylation of the IL6ST (gp130) receptor throughout the initial 24 hours of infection with Salmonella may be an immunometabolic signature of the instigation of the innate immune response.
Neither the IL-4R nor the IL7R were activated (phosphorylated) during the first 24 h after S. Enteritidis infection in the cecum when compared to the non-infected control tissues. Since IL-4 normally induces differentiation of naïve T helper cells to Th2 cells and IL-7 is involved in early T cell development, it is undoubtedly too early in the immune process for responses, the results are suggestive of premature involvement of T cell-mediated immune responsiveness so early in the infection process. Interestingly, the IL2RB was phosphorylated throughout the 24 hr initial infection period. Classically, IL-2 was described as a T cell growth factor and to also stimulate growth promoting activity in B cells and NK cells (Henney et al., 1981; Robb et al., 1981; Waldmann et al., 1984). However, we showed 20 years ago that IL-2 can directly activate chicken heterophils to exert effector functions and induce heterophil activation (Kogut et al., 2002). It has subsequently been proven that IL-2 has functional activity that connects innate and acquired immunity (reviewed Bendickova and Fric, 2020).
Both the type I interferon (IFNAR1, IFN-α) and type II interferon receptors (IFNGR1, IFN-γ) were phosphorylated 4-8 hours after cecal infection with S. Enteritidis. Traditionally, IFN-α is an antiviral cytokine, but recent evidence suggests that it is involved further in the development of innate immunity by playing a role in restricting bacterial infections, including Salmonella (Alphonse et al., 2021). In fact, further evidence is that IFN-α signaling can be regulated by the NOD-1 receptor (Kienes et al., 2021). We can speculate that the sensing of the S. Enteritidis infection by NOD-1, as described above, can lead to the production of IFN-α that controls the level of colonization and infection by the pathogenic bacteria in the chicken cecum. We have further shown that IFN-γ can activate in vitro heterophil functional activity against Salmonella (Kogut et al., 2001; Kogut et al., 2005) and the presence of IFN-γ and IL-2 in a S. Enteritidis immune cytokine cocktail protects day-old chicks against Salmonella infections mediated by activated heterophils (Kogut et al., 1996; Verduzzo et al., 2009).
The dephosphorylation of SOCS3 suggests that Salmonella virulence factors are not directly involved in the inhibition of cytokine signaling during the early infection period in the cecum. Alternatively, the host is initiating the innate response necessary to fight infection through the release of cytokines and that the activation of the negative regulator of cytokine signaling is not required at this time.
Cytokine receptor proteins lack enzymatic activity, thus are dependent upon JAKs to initiate signaling upon binding of their ligands. The JAK family has four members: JAK1, JAK2, JAK3 and tyrosine kinase 2 (TYK2) (Hu et al., 2021). The STAT family consist of seven members: STAT1, STAT2, STAT3, STAT4, STAT5a, STAT5b, and STAT6 (Hu et al., 2021; Nobel et al., 2021). We observed a selective activation of the JAK proteins with JAK2 and JAK3 the only JAK family members that were phosphorylated in the ceca from the S. Enteritidis-infected chickens (Table 8). JAK2 signaling is activated by interferon receptors and the gp130 receptor family (IL-6R) and is an essential tyrosine kinase for initiating the innate immune response (Pena et al., 2010). JAK3 is predominantly expressed in hematopoietic lineage and intestinal epithelial cells, but its role in cytokine signaling is more restricted than other JAKs with IL-2R, IL-4R, and IL-7R being the most common receptors (Kumar et al., 2007; Mishra et al., 2012). In the intestinal epithelium, IL-2 induces activation of Jak3 to facilitate/maintain mucosal homeostasis including mucin secretion and tight junction proteins (Kumar et al., 2021).
STAT5b is the sole member of the STAT family that is phosphorylated in the cecum during the initial infection process (4-24 hours) by S. Enteritidis. JAK-STAT5B signaling in the intestine is involved in the host response to inflammation and infection (Nobel et al., 2021). In the intestine, STAT5B activation is regulated by specific members of IL-2 family of cytokines (Awasthi et al., 2021) and IL-6 (Jiang et al., 2009; Rani and Murphy, 2016) is involved in multiple intestinal physiological responses including mucosal barrier function through tight junction permeability, mucin production, and regeneration and proliferation of intestinal epithelial stem cells (Han et al., 2009; Gilbert et al., 2015; Karin and Clevers, 2016; Degirmenci et al., 2018). However, activated STAT5 is short-lived and undergo rapid deactivation (Grimley et al., 1999). Combined with the cytokine receptor phosphorylation data discusses previously (IL2R and IL6R), it is reasonable to assume that STAT5B phosphorylation found here is involved in maintaining the cecal function during the initial Salmonella infection stage.
Stimulation of the T cell receptor results in the activation of the TCR signal transduction pathway. This pathway can activate the transcription factors nuclear factor κB (NF-κB), nuclear factor of activated T-cells (NFAT), and activator protein 1 (AP-1), that induce expression of cytokine genes (Smith-Garvin et al., 2009). However, based on up-stream regulators, neither NFAT nor NF-κB are activated through the T cell receptor pathway. First, the NFAT transcription factor family are regulated by calcium signaling which is critical to activation of NFAT because calmodulin activates the serine/threonine phosphatase calcineurin. Nuclear import of NFAT is dependent on the calcium level inside of a cell. If the calcium level drops, the exporting kinases in a nucleus rephosphorylate NFAT causing the transcription factor to revert into its inactive state and is exported back to the cytosol where maintenance kinases finish the rephosphorylation to keep it in the inactivated state (Baba and Kuroski, 2016; Park et al., 2020). Here we found a significant dephosphorylation in calmodulin, meaning that there is a dramatic reduction in calcium levels in the S. Enteritidis-infected tissue thus inhibiting NFAT activity. Second, NFAT requires the activity of PLC-1, which generates the second messengers, diacylglycerol (DAG) and inositol 1,4,5-trisphosphate (IP3). IP3 induces an increase in the concentration of cytoplasmic calcium (Ca2+) and activation of the Ca2+-dependent phosphatase calcineurin, which results in the rapid activation of NFAT, which is followed by its translocation to the nucleus (Baine et al., 2009). Thus, the dephosphorylation of PLCG1 prevents NFAT activation due to the decrease in intracellular calcium transport into the immune cells. Likewise, the dephosphorylation of PLCG1 inhibits the generation of DAG which is a vital second messenger in NF-κB activation via the T cell receptor. However, activation of NF-κB by PRRs in the innate response does not require PLCG1 generation of the DAG molecule to activate downstream proteins. PRRs use MyD88-dependent pathways to activate NF-κB activity (Akira et al., 2006, Kumar et al., 2011).
One intriguing finding from the current studies that links with our previous report on Salmonella persistence is the consistent dephosphorylation of phospholipase cγ1 (PLCG1) enzyme in the S. Enteritidis -infect cecal tissue (Kogut et al., 2017). This ‘targeting’ of PLCG1 by Salmonella seems to be a feature of infection between 24-96 hours post-infection. Even though we are not aware of what bacterial factors may be involved in dephosphorylating PLCG1, it is reasonable to assume that this action is part of the asymptomatic inflammatory response of chickens that leads to the development of a persistent Salmonella infection in the chicken. Further it appears possible that Salmonella inhibiting PLCG1 phosphorylation is part of the unique survival strategy of paratyphoid Salmonella in poultry that minimizes host defenses mechanisms (disease resistance) during the initial infection and then further uses the inhibition of PLCG1 activity as part of the immunometabolic reprogramming that converts host defense strategy to disease tolerance. Disease tolerance is the ability of the host to limit the damage caused by both the pathogen and the host immune response, i.e., immunopathology (Ayres and Schneider, 2012). PLCG1 would be a logical focal point of the initial Salmonella colonization/infection repertoire since it is involved in regulating innate immune functions including phagocytosis, the oxidative burst, cell migration and TLR-mediated signaling (Bae et al., 2017; Zhu et al., 2018; Jing et al., 2021).
Data Availability Statement
The raw data supporting the conclusions of this article will be made available by the authors, without undue reservation.
Ethics Statement
These studies were approved by the Animal Care and Use Committee (ACUC) at the Southern Plains Agricultural Research Center, Agricultural Research Service, United States Department of Agriculture (ACUC #2012007), which meets all federal requirements as defined in the Animal Welfare Act, the Public Health Service Policy, and the Humane Care and Use of Laboratory Animals.
Author Contributions
All authors contributed to the conception and design of the study. MK, KG, JB, HH, and CS conducted the infection studies and samples collections. RA conducted the kinome array and MK and RA conducted the bioinformatic analysis of the kinome data. YF and RA conducted the statistical analyses of all the data. MK wrote the first draft of the manuscript. All of the authors contributed to the manuscript revision and read and approved the submitted version.
Funding
The research was conducted as part of the ARS CRIS Project: ‘Immunological and Practical Approaches to Manipulate the Ecological Niches and Reduce Foodborne Pathogens in Poultry’ Project #3091-32000-037-00D).
Conflict of Interest
The authors declare that the research was conducted in the absence of any commercial or financial relationships that could be construed as a potential conflict of interest.
Publisher’s Note
All claims expressed in this article are solely those of the authors and do not necessarily represent those of their affiliated organizations, or those of the publisher, the editors and the reviewers. Any product that may be evaluated in this article, or claim that may be made by its manufacturer, is not guaranteed or endorsed by the publisher.
Supplementary Material
The Supplementary Material for this article can be found online at: https://www.frontiersin.org/articles/10.3389/fcimb.2022.899395/full#supplementary-material
References
Adler, H. E., DaMassa, A. J. (1979). Toxicity of Endotoxin in Chicks. Avian Dis. 23, 174–178. doi: 10.2307/1589684
Akira, S., Uematsu, S., Takeguchi, O. (2006). Pathogen Recognition in Innate Immunity. Cell 124, 783–801. doi: 10.1016/j.cell.2006.02.015
Alphonse, N., Dickensen, R. E., Dendall, C. (2021). Interferons: Tug of War Between Bacteria and Their. Front. Cell. Infect. Microbiol. 11. doi: 10.3389/fcimb.2021.624094
Arsenault, R. J., Kogut, M. H. (2015). Immunometabolism and the Kinome Peptide Array: A New Perspective and Tool for the Study of Gut Health. Front. Vet. Sci. 2. doi: 10.3389/fvets.2015.00044
Arsenault, R. J., Lee, J. T., Latham, R., Carter, B., Kogut, M. H. (2017). Changes in Immune and Metabolic Gut Response in Broilers Fed β-Mannase in β-Mannan Containing Diets. Poult. Sci. 96, 4307–4316. doi: 10.3382/ps/pex246
Awasthi, N., Liongue, C., Ward, A. C. (2021). STAT Proteins: A Kaleidoscope of Canonical and non-Canonical Functions in Immunity and Cancer. J. Hematol. Oncol. 14, 198. doi: 10.1186/s13045-021-01214-y
Ayres, J., Schneider, D. S. (2012). Tolerance of Infection. Annu. Rev. Immunol. 30, 271–294. doi: 10.1146/aaurev-immunol-020711-075030
Baba, Y., Kuroski, T. (2016). Role of Calcium Signaling in B Cell Activation and Biology. Curr. Topics Microbiol. Immunol. 393, 143–174. doi: 10.1807/82_2015_477
Bae, Y.-S., Lee, H. Y., Jung, Y. S., Lee, M., Suh, P.-G. (2017). Phospholipase Cγ in Toll-Like Receptor-Mediated Inflammation and Innate Immunity. Adv. Biol. Regul. 63, 92–97. doi: 10.1016/j.jbior.2016.10.003
Baine, I., Abe, B. I., Mecian, F. (2009). Regulation of T-Cell Tolerance by Calcium/NFAT Signaling. Immunol. Rev. 231, 225–240. doi: 10.1111/j-1600.065X.2009.0087X
Banoth, B., Chatterjee, B., Vijayaragavan, B., Prasad, M. V. R., Roy, P., Basak, S. (2015). Stimulus-Selective Crosstalk via the NF-κb Signaling System Reinforces Innate Immune Response to Alleviate Gut Infection. eLife 2015, e05648. doi: 10.7554/eLife.05648
Barrow, P. A., Jones, M. A., Smith, A. L., Wigley, P. (2012). The Long View: Salmonella – the Last Forty Years. Avian Pathol. 41, 413–420. doi: 10.1080/03079457.2012.718071
Bendickova, K., Fric, J. (2020). Roles of IL-2 in Bridging Adaptive and Innate Immunity, and as a Tool for Cellular Immunotherapy. J. Leuk. Biol. 108, 427–437. doi: 10.1002/JLB.5MIR0420-055R
Bernd, A., Wilhelm, A., Jugeri, C., Pieper, J., Sachse, K., Methner, U. (2007). Chicken Cecum Immune Response to Salmonella Enterica Serovars of Different Levels of Invasiveness. Infect. Immun. 75, 5993–6007. doi: 10.1128/IAI.00695-07
Boyle, J. P., Mayle, S., Parkhouse, R., Monie, T. P. (2013). Comparative Genomic and Sequence Analysis Provides Insight Into the Molecular Functionality of NOD1 and NOD2. Front. Immunol. 4. doi: 10.3389/fimmu.2013.00317
Cantrell, D. A., Smith, K. A. (1984). The Interleukin-2 T-Cell System: A New Cell Growth Model. Science 224, 1312–1316. doi: 10.1126/science.6427923
Crhanova, M., Hradecka, H., Faldynova, M., Mutulova, M., Havlickova, H., Sisak, et al. (2011). Immune Response of Chicken Gut to Natural Colonization by Gut Microflora and to Salmonella Enterica Serovar Enteritidis Infection. Infect. Immun. 79, 2755–2763. doi: 10.1128/IAI.01375-10
Degirmenci, B., Valenta, T., Dimitieva, S., Hausmann, G., Basler, K. (2018). GLI1-Expressing Mesenchymal Cells Form the Essential Wnt-Secreting Niche for Colon Stem Cells. Nature 558, 449–453. doi: 10.1038/s41586-018-0190-3
Eng, S.-K., Pusparajah, P., Mutalib, N.-S. A., Ser, H.-L., Chan, K.-G., Lee, L.-H. (2015). Salmonella: A Review on Pathogenesis, Epidemiology and Antibiotic Resistance. Front. Life Sci. 8, 284–293. doi: 10.1080/21553769.2015.1051243
Essen, L.-O., Perisic, O., Katan, M., Wu, Y., Roberts, M. F., Williams, R. L. (1997). Structural Mapping of the Catalylic Mechanism for a Mammalian Phosphoinositide-Specific Phospholipase C. Biochemistry 36, 1704–1718. doi: 10.1021/bi962512p
Figueira, R., Holden, D. W. (2012). Functions of the Salmonella Pathogenicity Island 2 (SPI-2) Type III Secretion System Effectors. Microbiology 158, 1147–1161. doi: 10.1099/mic.0.058115-0
Fink, S. L., Cookson, B. T. (2007). Pyroptosis and Host Cell Death Responses During Salmonella Infection. Cell. Microbiol. 9, 2562–2570. doi: 10.1111/j.1462-5822.2007.01036.x
Flint, T. R., Janowitz, T., Connell, C. M., Coll, A. P., Jodrell, D. I., Fearon, D. T. (2016). Tumor-Induced IL-6 Reprograms Host Metabolism to Suppress Anti-Tumor Immunity. Cell Metab. 24, 672–684. doi: 10.1016/j.cmet.2016.10.010
Foster, N., Lovell, M. A., Marston, K. L., Hulme, S. D., Frost, A. J., Bland, P., et al. (2003). Rapid Protection of Gnotobiotic Pigs Against Experimental Salmonellosis Following Induction of Polymorphonuclear Leukocytes by Avirulent. Infect. Immun. 71, 2182–2191. doi: 10.1128/IAI.71.4.2182-2191.2003
Galluzzi, L., Vitale, I., Aaronson, S. A., Abrams, J. M., Adam, D., Agostinis, P., et al. (2018). Molecular Mechanisms of Cell Death: Recommendations of the Nomenclature Committee on Cell Death 2018. Cell Death Differ. 25, 486–541. doi: 10.1038/s41418-017-0012-4
Garcia, J. S., Byrd, J. A., Wong, E. A. (2020). Tissue-, Age- and Dose-Dependent Changes in Avian β-Defensin and LEAP2 mRNA Abundance in the Intestines of Salmonella Typhimurium Challenged Broilers. Anim. Biotechnol. 32, 637–645. doi: 10.1080/10495398.2020.1738449
Genovese, L., Lowry, V. K., Genovese, K. J., Kogut, M. H. (1999). Longevity of Augmented Phagocytic Activity of Heterophils in Neonatal Chickens Following Administration of Salmonella enteritidis –Immune Lymphokines. Avian Pathol. 29, 117–122. doi: 10.1080/03079450094144
Ghanemi, A., St Amand, J. (2018). Interleukin-6 as a “Metabolic Hormone”. Cytokine 112, 132–136. doi: 10.1016/j.cyto.2018.06.034
Gilbert, S., Nivarthi, H., Mayhew, C. N., Lo, Y.-H., Noah, T. K., Vallance, J., et al. (2015). Activated STAT5 Confers Resistance to Intestinal Injury by Increasing Intestinal Stem Cell Proliferation and Regeneration. Stem Cell Rep. 4, 209. doi: 10.016/j.stemcr.2014.12.004
Grimley, P. M., Dong, F., Rui, H. (1999). Stat5a and Stat5b: Fraternal Twins of Signal Transduction and Transcriptional Activation. Cytokine Growth Fact. Rev. 10, 131–137. doi: 10.1016/S1359-6101(99)00011-8
Han, X., Ren, X., Jurickova, I., Groschwitz, K., Pasternak, B. A., Xu, H., et al. (2009). Regulation of Intestinal Barrier Function by Signal Transducer and Activator of Transcription 5b. Gut 58, 49–58. doi: 10.1136/gut.2007.145094
Hefele, M., Stolzer, I., Ruder, B., He, G.-W., Mahapatro, M., Wirtz, S., et al. (2018). Intestinal Epithelial Caspase-8 Signaling is Essential to Prevent Necroptosis During Salmonella Typhimurium Induced Enteritis. Mucosal Immunol. 11, 1191–1201. doi: 10.1038/s41385-018-0011-x
Henney, C., Kuribayashi, K., Kern, D., Gillis, S. (1981). Interleukin-2 Augments Natural Killer Cell Activity. Nature 291, 335–338. doi: 10.1038/291335a0
Higgs, R., Cormican, P., Cahalane, S., Allan, B., Lloyd, A. T., Meade, K., et al. (2006). Induction of a Novel Chicken Toll-Like Receptor Following Salmonella Enterica Serovar Typhimurium Infection. Infect. Immun. 74, 1692–1698. doi: 10.1128/IAI.74.3.1692-1698.2006
Hughes, S., Haynes, A., O’Regan, Bumstead, B. (2001). Identification, Mapping, and Phylogenetic Analysis of Three Novel Chicken CC Chemokines. Immunogenetics 53, 674–683. doi: 10.1007/s002510100368
Hughes, S., Poh, T.-Y., Bumstead, N., Kaiser, P. (2007). Re-Evaluation of the Chicken MIP Family of Chemokines and Their Receptors Suggests That CCL5 Is the Prototypic MIP Family Chemokine, and That Different Species Have Developed Different Repertoires of Both the CC Chemokines and Their Receptors. Dev. Comp. Immunol. 31, 72–86. doi: 10.1016/j.dci.2006.04.003
Hu, X., Li, J., Fu, M., Zhao, X., Wang, W. (2021). The JAK/STAT Signaling Pathway: From Bench to Clinic. Signal Trans. Targeted Ther. 6, 402. doi: 10.1038/s41392-021-00791-1
Iqbal, M., Philbin, V. J., Withanage, G. S. K., Wigley, P., Beal, R. K., Goodchild, M. J., et al. (2003). Identification and Functional Characterization of Chicken Toll-Like Receptor 5 Reveals a Fundamental Role in the Biology of Infection With Salmonella Enterica Serovar Typhimurium. Infect. Immun. 73, 2344–2350. doi: 10.1128/IAI.73.4.2344-2350.2005
Jalal, S., Arsenault, R., Potter, A. A., Babiuk, L. A., Griebel, P. J., Napper, S. (2009). Genome to Kinome: Species-Specific Peptide Arrays for Kinome Analysis. Sci. Signal 2, pl1 doi: 10.1126/scisignal.254pl1.
Jiang, H., Patel, P. H., Kohlmaier, A., Grenley, M. O., McEwen, D. G., Edgar, B. A. (2009). Cytokine/Jak/Stat Signaling Mediates Regeneration and Drosophila Homeostasis in the Midgut. Cell 137, 1343–1455. doi: 10.1016/j.cell.2009.05.014
Jing, H., Reed, A., Ulnaovskaya, O. A., Grigoleit, J.-S., Henry, C. L., Li, H., et al. (2021). Phospholipase Cγ2 Regulates Endocannabinoid and Eicosanoid Networks in Innate Immune Cells. Proc. Natl. Acad. Sci. 118, e2112971118. doi: 10.1073/pnas.2112971118
Kanehisa, M. (2019). Toward Understanding the Origin and Evolution of Cellular Organisms. Protein Sci. 28, 1947–1951. doi: 10.1002/pro.3715
Karin, M., Clevers, H. (2016). Reparative Inflammation Takes Charge of Tissue Regeneration. Nature 529, 307–315. doi: 10.1038/nature17039
Karin, M., Liu, Z.-G., Zandi, E. (1997). AP-1 Function and Regulation. Curr. Opin. Cell Biol. 9, 240–246. doi: 10.1016/S0955-0674(97)80068-3
Keestra, A. M., De Zoete, M. R., Bouwman, M. M., Vaezirad, M. M., Van Putten, J. P. M. (2013). Unique Features of Chicken Toll-Like Receptors. Dev. Comp. Immunol. 41, 316–323. doi: 10.1016/j.dci.2013.04.009
Keestra, A. M., Van Putten, J. P. M. (2008). Unique Properties of the Chicken TLR4/MD-2 Complex: Selective Lipopolysaccharide Activation of the MyD88-Dependent Pathway. J. Immunol. 181, 4354–4362. doi: 10.4049/jimmunol.181.6.4354
Kienes, I., Weidl, T., Mirza, N., Chamaillard, M., Kufer, T. A. (2021). Role of NLRs in the Regulation of Type I Interferon Signaling, Host Defense and Tolerance to Inflammation. Int. J. Mol. Sci. 22, 1301. doi: 10.3390/ijms22031301
Kim, J. M., Eckmann, L., Savidge, T. C., Lowe, D., Witthöft, T., Kagnoff, M. F. (1998). Apoptosis of Human Intestinal Epithelial Cells After Bacterial Invasion. J. Cin. Invest. 102, 1815–1823. doi: 10.1172/JCI2466
Klasing, K. C. (2007). Nutrition and the Immune System. Br. Poult. Sci. 48, 525–537. doi: 10.1080/00071660701671336
Klasing, K. C. (2017). Inflammation: Costs and Control. Proc. 17th Annual Midwest Swine Nutrition Conference (Indianapolis, IN, USA: Organizing Committee, Midwest Swine Nutrition Conference).
Kogut, M. H. (2022a). “Avian Granulocytes” in Avian Immunology, 3rd Edition. Eds. Kaspers, B., Schat, K. A., Gobel, T. (London, UK: Academic Press), 197–204.
Kogut, M. H. (2022b). “Immunophysiology of the Avian Immune System” in Sturkie’s Avian Physiology, 7th Edition. Eds. Scanes, C. G., Dridi, S. (London, UK: Academic Press), 571–590.
Kogut, M. H., Arsenault, R. J. (2015). A Role for the non-Canonical Wnt-β-Catenin and TGF-β Signaling Pathways in the Induction of Tolerance During the Establishment of Salmonella Enterica Serovar Enteritidis Persistent Cecal Infection in Chickens. Front. Vet. Sci. 2, 33. doi: 10.3389/fvets.2015.00033
Kogut, M. H., Tellez, G. I., McGruder, E. D., Wong, R. A., Isibasi, A., Ortiz, V. N., et al. (1996). Evaluation of Salmonella enteritidis-immune lymphokines on host resistance to Salmonella gallinarum infection in broiler chicks. Avian Pathol. 25, 737–749. doi: 10.1080/03079459608419178
Kogut, M., Arsenault, R. J. (2017). Immunometabolic Phenotype Alterations Associated With the Induction of Disease Tolerance and Persistent Asymptomatic Infection of Salmonella in the Chicken Intestine. Front. Immunol. 8. doi: 10.3389/fimmu.2017.00372
Kogut, M. H., Chiang, H. I., Swaggerty, C. L., Zhou, H. (2012). Gene Expression Analysis of Toll-Like Receptor Pathways in Heterophils From Genetic Chicken Lines That Differ in Their Susceptibility to Salmonella Enteritidis. Front. Genet. 3. doi: 10.3389/fgene.2012.00121
Kogut, M. H., Genovese, K. J., He, H., Arsenault, R. J. (2016). AMPK and mTOR: Sensors and Regulators of Immunometabolic Changes During Salmonella Infection in the Chicken. Poult. Sci. 95, 345–353. doi: 10.3382/ps/pev349
Kogut, M. H., Pishko, E. J., Kaspers, B., Weining, K. C. (2001). Modulation of Functional Activities of Chicken Heterophils by Recombinant Chicken IFN-γ. J. IFN Cyto. Res. 21, 85–92. doi: 10.1089/107999001750069944
Kogut, M. H., Rothwell, L., Kaiser, P. (2002). Differential Effects of Age on Chicken Heterophil Functional Activation by Recombinant Chicken Interleukin-2. Dev. Comp. Immunol. 26, 817–830. doi: 10.1016/S0145-305X(02)00040-X
Kogut, M. H., Rothwell, L., Kaiser, P. (2005). Interferon-γ Upregulates the Expression of Proinflammatory and Th1 Cytokine mRNA in Chicken Heterophils During Receptor-Mediated Phagocytosis of Opsonized and Nonopsonized Salmonella Enterica Serovar Enteritidis. J. IFN Cyto. Res. 25, 73–81. doi: 10.1089/jir.2005.25.73
Kogut, M. H., Swaggerty, C. L., Byrd, J. A., Salvaraj, R., Arsenault, R. J. (2017). Chicken-Specific Kinome Array Reveals That Salmonella Enterica Serovars Enteritidis Modulates Host Immune Signaling Pathways in the Cecum to Establish a Persistent Infection. Int. J. Med. Sci. 17, 1207. doi: 10.3390/ijms17081207
Kogut, M. H., Tellez, G. I., Hargis, B. M., Corrier, D. E., DeLoach, J. R. (1993). The Effect of 5-Fluorouracil Treatment of Chicks: A Cell Depletion Model for the Study of Avian Polymorphonuclear Leukocytes and Natural Host Defenses. Poult. Sci. 72, 1873–1880. doi: 10.3382/ps.0721873
Kogut, M. H., Tellez, G. I., McGruder, E. D., Hargis, B. M., Williams, J. D., Corrier, D. E., et al. (1994). Heterophils are Decisive Components in the Early Responses of Chickens to Salmonella Enteritidis Infections. Microb. Path. 16, 141–151. doi: 10.1006/mpat.1994.1015
Kogut, M. H., Tellez, G. I., McGruder, E. D., Wong, , R. A, Isibasi, , A., Ortiz, V. N., Hargis, B. M., DeLoach, J. R.. (1996).Evaluation of Salmonella enteritidis-immune lymphokines on host resistance to Salmonella gallinarum infection in broiler chicks. Avian Pathology 25, 737–749 doi: 10.1080/03079459608419178
Kumar, H., Kawai, T., Akira, S. (2011). Pathogen Recognition by the Innate Immune System. Int. Rev. Immunol. 30, 16–37. doi: 10.3109/088
Kumar, N., Kuang, L., Villa, R., Kumar, P., Mishra, J. (2021). Mucosal Epithelial JAK Kinases in Health and Disease. Mediators Inflammation 2021, 6618924. doi: 10.1155/2021/6618924
Kumar, N., Mishra, J., Narang, V. S., Waters, C. N. (2007). Janus Kinase 3 Regulates Interleukin-2-Induced Mucosal Repair Through Tyrosine Phosphorylation of Villin. J. Biol. Chem. 282, 30341–30351. doi: 10.1074/jbc.C600319200
Lee, A., Bortoluzzi, C., Pilla, R., Kogut, M. H. (2020). A Role for the Microbiota in the Immune Phenotype Alteration Associated With the Induction of Disease Tolerance and Persistent Asymptomatic Infection of Salmonella in the Chicken. Microorganisms 8, 1879. doi: 10.3390/microorganisms8121879
Li, Y., Arsenault, R. J., Trost, B., Slind, J., Griebel, P. J., Napper, S., et al. (2012). A Systematic Approach for Analysis of Peptide Array Kinome Data. Sci. Signal. 5, pl2. doi: 10.1126/scisignal.2002429
Li, H., Jin, H., Li, Y., Liu, D., Foda, M. F., Jiang, Y., et al. (2017). Molecular Cloning and Functional Characterization of Duck Nucleotide-Binding Oligomerization Domain 1 (NOD1). Dev. Comp. Immunol. 74, 82–89. doi: 10.1016/j.dci.2017.04.012
Matulova, M., Varmuzova, K., Sisak, F., Havlickova, H., Babak, V., Stejskal, K., et al. (2013). Chicken Innate Response to Oral Infection With Salmonella Enterica Serovar Enteritidis. Vet. Res. 44, 37. doi: 10.1186/1297-9716-44-37
Meijerink, N., van den Biggelaar, R. H. H. A., van Haarlem, C. A., Stegeman, J. A., Rutten, V. P. M. G., Jansen, C. A. (2021). A Detailed Analysis of Innate and Adaptive Immune Responsiveness Upon Infection With Salmonella Enterica Serotype Enteritidis in Young Broiler Chickens. Vet. Res. 52, 109. doi: 10.1186/s13567-021-00978-y
Mishra, J., Karanski, S. S., Kumar, N. (2012). Identification of Molecular Switch Regulating Interactions of Janus Kinase 3 With Cytoskeletal Proteins. J. Biol. Chem. 287, 41386–41391. doi: 10.1074/jbc.C1121.363507
National Research Council (1994). Nutritional Requirements of Poultry. 9th ed (Washington, DC, USA: National Academy Press).
Nika, K., Soldani, C., Salek, M., Paster, W., Gray, A., Etzensperger, R., et al. (2010). Constitutively Active Lck Kinase in T Cells Drives Antigen Receptor Signal Transduction. Immunity 32, 766–777. doi: 10.1016/j.immuni.2010.05.011
Nobel, Y. R., Stier, K., Krishnareddy, S. (2021). STAT Signaling in the Intestine. Int. Rev. Cell Mol. Biol. 361, 1–20. doi: 10.1016/bs.ircmb.2021.02.001
O’Shea, J. J., Schwartz, D. M., Villarino, A. V., Gadina, M., McInnes, I. B., Laurence, A. (2015). The JAK-STAT Pathway: Impact on Human Disease and Therapeutic Intervention. Annu Rev. Med. 66, 311–328. doi: 10.1146/annurev-med-05113-024537
Park, U.-J., Yoo, S. A., Kim, M. (2020). The Role of Calcium-Calcineurin-NFAT Signaling Pathway Inn Health and Autoimmune Disease. Front. Immunol. 1. doi: 10.3389/fimmu.2020.00195
Patel, S., McCormick, B. A. (2014). Mucosal Inflammatory Response to Salmonella typhimurium Infection. Front. Immunol. 5. doi: 10.3389/fimmu.2014.00311
Pavlov, V. A., Tracey, K. J. (2012). The Vagus Nerve and the Inflammatory Reflex-Linking Immunity and Metabolism. Nat. Rev. Endocrinol. 8, 743–754. doi: 10.1038/nrendo.2012.189
Pena, G., Cai, B., Deitch, E. A., Ulloa, L. (2010). JAK2 Inhibition Prevents Innate Immune Responses and Rescues Animals From Sepsis. J. Mol. Med. 88, 851–859. doi: 10.1007/s00109-010-0628-z
Perry, F., Johnson, C., Aylward, B., Arsenault, R. J. (2020). The Differential Phosphorylation-Dependent Signaling and Glucose Immunometabolic Responses Induced During Infection by Salmonella Enteritidis and Salmonella Heidelberg in Chicken Macrophage-Like Cells. Microorganisms 8, 1041. doi: 10.3390/microorganisms8071041
Pieper, J., Methner, U., Berndt, A. (2011). Characterization of Avian γδ T-Cell Subsets After Salmonella Enterica Serovar Typhimurium Infection of Chicks. Infect. Immun. 79, 822–829. doi: 10.1128/IAI.00788-10
Rani, A., Murphy, J. J. (2016). STAT5 in Cancer and Immunity. J. IFN Cyto. Res. 36, 226–237. doi: 10.1089/jir.2015.0054
Robb, R. J., Munck, A., Smith, K. A. (1981). T Cell Growth Factor Receptors. Quantification, Specificity, and Relevance. J. Exp. Med. 154, 1455–1484. doi: 10.1084/jem_154.5.1455
Rychlik, I., Elsheimer-Matuloya, M., Kyrova, K. (2014). Gene Expression in the Chicken Caecum in Response to Infections With Non-Typhoid Salmonell. A. Vet. Res. 45, 119. doi: 10.1186/s13567-014-0119-2
Salmond, R. J., Filby, A., Qureshi, I., Caserta, S., Zamoyska, R. (2009). T-Cell Receptor Proximal Signaling via the Src-Family Kinases, Lck and Fyn, Influences T-Cell Activation, Differentiation, and Tolerance. Immunol. Rev. 228, 9–22. doi: 10.1111/j.1600-065X.2008.00745.x
Schwerk, C., Schultze-Osthoff, K. (2005). Regulation of Apoptosis by Alternative pre-mRNA Splicing. Mol. Cell 19, 1–13. doi: 10.1016/j.molcel.2005.05.026
Sellin, M. E., Muller, A. A., Felmy, B., Dolowschiak, T., Diard, M., Tardivel, A., et al. (2014). Epithelium-Intrinsic NAIP/NLRC4 Inflammasome Drives Infected Enterocyte Expulsion to Restrict Salmonella Replication in the Intestinal Mucosa. Cell Host Microbe 16, 237–248. doi: 10.1016/jchom.2014.07.001
Setta, A., Barrow, P. A., Kaiser, P., Jones, M. A. (2012). Immune Dynamics Following Infection of Avian Macrophages and Epithelial Cells With Typhoidal and Non-Typhoidal Salmonella Enterica Serovars: Bacterial Invasion and Persistence, Nitric Oxide and Oxygen Production, Differential Host Gene Expression, NF-κb Signaling and Cell Cytotoxicity. Vet. Immunol. Immunopathol. 146, 212–224. doi: 10.1016/j.vetimm.2012.03.008
Sick, C., Schneidwer, K., Staheli, P., Weining, K. C. (2006). Novel Chicken CXC and CC Chemokines. Cytokine 12, 181–186. doi: 10.1006/cyto.1999.0543
Smith, K. A. (1988). Interleukin-2: Inception, Impact, and Implications. Science 240, 1169–1176. doi: 10.1126/science.313876
Smith-Garvin, J. E., Koretzky, G. A., Jordan, M. S. (2009). T Cell Activation. Ann. Rev. Immunol. 27, 591–619. doi: 10.1146/annurev.immunol.021908.132706
Smith, A. L., Gobel, T. W. (2022). “Avian T Cells: Antigen Recognition and Lineages,” in Avian Immunology, 3rd Edition. Eds. Kaspers, B., Schat, K. A., Gobel, T., Vervelde, L. (London, UK: Academic Press), 121–133.
Swaggerty, C. L., Kaiser, P., Roth well, L., Pevzner, I., Kogut, M. H. (2006). Heterophil Cytokine mRNA Profiles From Genetically Distinct Lines of Chickens With Differential Heterophil-Mediated Innate Immune Responses. Avian Pathol. 35, 102–108. doi: 10.1080/030794506000597535
Szklarczyk, D., Morris, J. H., Cook, H., Kuhn, M., Wyder, S., Simonovic, M., et al. (2017). The STRING Database in 2017: Quality-Controlled Protein-Protein Association Networks, Made Broadly Accessible. Nucleic Acid. Res. 45, D362–D368. doi: 10.1093/nar/gwk937
Tao, Z., Zhu, C., Song, W., Xu, W., Zhang, S., Liu, H., et al. (2017). Inducive Expression of the NOD1 Signaling Pathway in Chickens Infected With Salmonella pullorum. Br. Poult. Sci. 58, 242–250. doi: 10.1080/00071688.2017.1280771
Thiennimitr, P., Winter, S. E., Baumler, A. J. (2012). Salmonella, the Host and its Microbiota. Curr. Opin. Microbiol. 15, 108–114. doi: 10.1016/j.mib.2011.10.002
Trost, B., Kindrachuk, J., Maatanen, P., Naper, S., Kusalik, A. (2013). PIIKA 2: An Expanded, Web-Based Platform for Analysis of Kinome Microarray Data. PLoS One 8, e80837. doi: 10.1371/journal.pone.0080837
Van der Merwe, P. A., Dushek, O. (2011). Mechanisms for T Cell Recpeotr Triggering. Nat. Rev. Immunol. 11, 47–55. doi: 10.1038/nri2887
Van Immerseel, F., de Buck, J., De Smet, I., Mast, J., Haesebrouck, F., Ducatelle, R. (2003). Dynamics of Immune Cell Infiltration in the Cecal Lamina Propria of Chickens After Neonatal Infection With a Salmonella enteritidis Strain. Dev. Comp. Immunol. 26, 355–364. doi: 10.1016/s0145-305x(01)00084-2
Verduzzo, G. V., Tellez, G., Kogut, M. H., Isibasi, A., Ortiz-Navarrete, V. (2009). Presence of Interferon-Gamma and IL-2 in Supernatants of Salmonella Enteritidis-Immune Lymphokines. Int. J. Poult. Sci. 8, 820–823. doi: 10.3923/ijps.2009.820.823
Waldmann, T. A., Goldman, C. K., Robb, R. J., Depper, J. M., Leonard, W. J., Sharrow, S. O., et al. (1984). Expression of Interleukin 2 Receptors on Activated B Cells. J. Exp. Med. 160, 1450–1466. doi: 10.1084/jem.160.5.1450
Wang, J., Adelson, D. L., Yilmaz, A., Sze, S.-H., Jin, Y., Zhu, J. J. (2005). Genomic Organization, Annotation, and Ligand-Receptor Inferences of Chicken Chemokines and Chemokine Receptor Genes Based on Comparative Genomics. BMC Geniomics 6:45. doi: 10.1186/1471-2164-6-45
Wang, X., Sharp, J. S., Handel, T. M., Prestegard, J. H. (2013). Chemokine Oligomerization in Cell Signaling and Migration. Prog. Mol. Biol. Tansl. Sci. 117, 531–578. doi: 10.1016/B978-0-12-386931-9.000020.9
Wemyss, M. A., Pearson, J. S. (2019). Host Cell Death Responses to non-Typhoid Salmonella Infection. Front. Immunol. 10. doi: 10.3389/fimmu.2019.01.01758
Wigley, P. (2014). Salmonella Enterica in the Chicken: How it has Helped Our Understanding of Immunology in a non-Biomedical Model Species. Front. Immunol. 5. doi: 10.3389/fimmu.2014.00482
Winter, S. E., Baumler, A. J. (2011). A Breathtaking Feat: To Compete With the Gut Microbiota, Salmonella Drives its Host to Provide a Respiratory Electron Acceptor. Gut Microbes 2, 58–60. doi: 10.4161/gmic.2.1.14911
Winter, S. E., Keestra, A. M., Tsolis, R. M., Baumler, A. J. (2010). The Blessings and Curses of Intestinal Inflammation. Cell Host Microbe 22, 36–43. doi: 10.1016/j.chom.2010.06.003
Withanage, G. S., Wigley, P., Kaiser, P., Mastroeni, P., Brooks, H., Powers, C., et al. (2005). Cytokine and Chemokine Responses Associated With Clearance of a Primary Salmonella Enterica Serovar Typhimurium Infection in the Chicken and in Protective Immunity to Rechallenge. Infect. Immun. 73, 5173–7183. doi: 10.1128/IAI.73.8.5173-5182.2005
Zanoni, I., Granucci, F. (2012). Regulation and Dysregulation of Innate Immunity by NFAT Signaling Downstream of Pattern Recognition Receptors (PRRs). Eur. J. Immunol. 42, 1924–1931. doi: 10.1002/eji.201242580
Keywords: Salmonella, chicken, kinome, phosphorylation, innate immunity, immune phenotype
Citation: Kogut MH, Genovese KJ, Byrd JA, Swaggerty CL, He H, Farnell Y and Arsenault RJ (2022) Chicken-Specific Kinome Analysis of Early Host Immune Signaling Pathways in the Cecum of Newly Hatched Chickens Infected With Salmonella enterica Serovar Enteritidis. Front. Cell. Infect. Microbiol. 12:899395. doi: 10.3389/fcimb.2022.899395
Received: 18 March 2022; Accepted: 30 May 2022;
Published: 30 June 2022.
Edited by:
Xin Zhang, Ningbo University, ChinaReviewed by:
Vincent Tam, Temple University, United StatesMarijke Keestra-Gounder, University of Colorado Denver, United States
Copyright © 2022 Kogut, Genovese, Byrd, Swaggerty, He, Farnell and Arsenault. This is an open-access article distributed under the terms of the Creative Commons Attribution License (CC BY). The use, distribution or reproduction in other forums is permitted, provided the original author(s) and the copyright owner(s) are credited and that the original publication in this journal is cited, in accordance with accepted academic practice. No use, distribution or reproduction is permitted which does not comply with these terms.
*Correspondence: Michael H. Kogut, bWlrZS5rb2d1dEBhcnMudXNkYS5nb3Y=