- Department of Biochemistry, Biotechnology and Bioinformatics, Avinashilingam Institute for Home Science and Higher Education for Women, Tamil Nadu, India
Occurrence and intensity of systemic invasive fungal infections have significantly risen in recent decades with large amount of mortality and morbidity rates at global level. Treatment therapy lies on the current antifungal interventions and are often limited due to the emergence of resistance to antifungal agents. Chemosensitization of fungal strains to the conventional antimycotic drugs are of growing concern. Current antifungal drugs often have been reported with poor activity and side effects to the host and have a few number of targets to manifest their efficacy on the pathogens. Indiscriminately, the aforementioned issues have been easily resolved by the development of new intervention strategies. One such approach is to employ combinational therapy that has exhibited a great level of inhibitions than that of a single compound. Chemosensitization of pathogenic mycoses to commercial antifungal drugs could be drastically enhanced by co-application of chemosensitizers along with the conventional drugs. Chemosensitizers could address the resistance mechanisms evolved in the pathogenic fungi and targeting the system to make the organism susceptible to commercially and clinically proven antifungal drugs. However, this strategy has not been overreached to the greater level, but it needs much attention to fight against not only with the pathogen but combat the resistance mechanisms of pathogens to drugs. Natural compounds including plant compounds and microbial proteins act as potential chemosensitizers to break the resistance in mycoses. Aspergillus giganteus, a filamentous fungus, is known to produce a cysteine rich extracellular protein called as antifungal protein (AFP). AFP has shown enhanced efficacy against several filamentous and non-filamentous fungal pathogens. On the basis of the reported studies on its targeted potential against pathogenic mycoses, AFP would be fabricated as a good chemosensitizer to augment the fungicidal efficacy of commercial antimycotic drugs. This paper reviews on breakthrough in the discovery of antifungal drugs along with the resistance patterns of mycoses to commercial drugs followed by the current intervention strategies applied to augment the fungicidal potential of drugs.
Background
Fungal infections, predominantly aspergillosis, candidiasis, and cryptococcosis, are of main global concern because of their causative agents and resistance toward commercial antifungal drugs. Development of antimycotic drugs have lagged behind by the fact that fungi are eukaryotes, known to have a similar and closer evolutionary relationship to humans. Unlike other pathogens, they own a similar attributes including biochemical, genetic, and cellular biology common to humans (Campbell et al., 2012). Major antifungal drugs fall under three categories: azoles, polyenes, and echinocandins, whereas azoles and polyenes were clinically approved and applied for the treatment before 1980s. In 20th century, third-category drugs, namely, echinocandins (Caspofungin) was introduced and used in routine clinical purposes to treat fungal infections (Roemer and Krysan, 2014). Although these drugs are effectively applied in clinical area on systemic and invasive fungal infections, the emergence of antifungal resistance make fungal diseases a global human threat, especially in immunocompromised individuals (McCarthy et al., 2017). The increased incidence of fungal diseases may require an adequate level of antimycotic chemotherapy, ultimately leading to the overreaching problem of antifungal resistance. The development of resistance involves a vicious cycle of events that results in emergence of more resistant strains and thus, in turn, promotes the reinforcement of therapeutically intractable fungal diseases.
Aspergillus species are frequent causative agents of invasive fungal infections, with aspergillosis mainly affecting persons with hematological malignancies and after solid-organ or hematopoietic stem cell transplantations. Frontline antimycotic drugs therapy for the treatment of aspergillosis are voriconazole (VOR), liposomal amphotericin B lipid complex (AmB), posaconazole (POS), micafungin (MICA), itraconazole (ITR), anidulafungin (ANI), and caspofungin (CAS) (Kim et al., 2010). Since the expansion of resistance to the conventional antimycotic drugs is of global concern, there is a pressing effort to make the pathogens susceptible for antifungal drugs. Indeed, further issue is the stagnation in the development of effective and novel antifungal drugs for the treatment (Kim et al., 2016). Chemosensitization by natural compounds is a new strategy to deal with the aforementioned issue will enhance the efficacy of conventional antimycotics. Over three decades, natural compounds with no toxicity are currently tested to augment the antifungal efficacy. Chemosensitizing agents cannot be applied as alone for the effective treatment, when co-applied with the commercial antibiotics, might countermands the antifungal resistance and augment the efficacy to treat the infamy fungal infections (Kim et al., 2019).
Here, we comment on the recent chemosensitizing efforts as alternative strategy for the control and treatment of fungal infections by conventional antimycotic drugs. The review primarily encapsulated with the antifungal-resistant mechanism evolved by fungal pathogens followed by the role of chemosensitizers in the control of fungal infections.
Breakthrough in the Discovery of Antimycotic Drugs
In the early 1950s, no fungal-specific antimicrobials were developed but were possible after two decades of penicillin discovery. Figure 1 shows the evolution of antimycotic drugs for the control of fungal infections. Griseofulvin (polyketide) from Penicillium griseofulvum and nystatin (polyene) from Streptomyces bacterium were the initial antimycotic drugs isolated. Grieseofulvin follows the mechanism of disrupting the mitotic spindle microtubules, thus impeding the cellular division in fungal pathogens. Nystatin has the ability to form a hydrogen bonds with ergosterol and creating a pore on the plasma membrane for the leakage of cellular materials in fungi. Ergosterol is the fungal specific sterol responsible for the maintenance of structural integrity of fungi. Nystatin is advantageous over griseofulvin in clinical treatment. Griseofulvin has nephrotoxicity limiting to internal applications and used for dermatophytic and oropharyngeal infections (Panda et al., 2005; Campbell et al., 2012).
Importance of Polyenes in Intractable Fungal Infections
Wide spectrum of antimycotic drugs have emerged since, with nystatin able to disrupt the cell membrane integrity of the pathogen. After that, two kinds of polyene from Streptomyces nodosus were isolated, namely amphotericin A (AmA) and Amphotericin B (AmB), based on their amphoteric properties. A tetraene amphotericin A has the antifungal spectrum similar to nystatin. A heptaene AmB was found to have a greater extent of antimycotic activity than nystatin, which is considered as the milestone in the antifungal therapy. More than one and a half decades of research has been expended to unravel the chemical nature and structure of AmB. In total, six number of polyene antifungals have been exploited in antifungal treatment, namely, amphotericin B, nystatin, natamycin, candicidin, methyl partricin, and trichomycin. Among which, only three polyenes have been used widely in current antifungal therapies such as AmB for systemic invasive fungal infections, nystatin for mucosal (oral infections or vulvovaginal candidiasis), and natamycin for ophthalmic infections. Since AmB is not soluble in water due to its amphipathic properties, researchers have developed various forms of AMB (lipid, liposomal form, and complex) to minimize their side effects and make it effective in treating fungal infections with less toxic and side effects to the human (Roemer and Krysan, 2014).
There are four models of mode of polyene actions: pore forming model, surface adsorption model, sterol sponge model, and oxidative damage model. In all the proposed models, targeting the ergosterol is the key to exert their antifungal activity. Ergosterol is the important cellular components in the fungal cell actively participating in several cellular functions including regulation of membrane proteins, cell division, endocytosis, cell signaling, and membrane fluidity. AmB binds to ergosterol, apart from binding to other sterols including cholesterol, lanosterol, and others. There are three kinds of non-covalent interactions that have been involved in the interaction between AmB and ergosterol: hydrogen bonds, van der Waals interactions, and π-π electronic interactions. In the pore forming model, the drug and ergosterol interaction forms an ion-like complex that releases ions and small molecules out of the cells to cell death. In the AmB oriented on the plasma membrane based on their amphipathic nature, the hydrophobic tails are interacting with the ergosterol and eventually enter into the lipid environment, whereas the hydrophilic heads might form an aqueous channel. On the basis of AmB function, it is possible to make two types of pores on the membrane: complete pore on the membrane consists of two polyenes and a half-pore containing only one polyene ring. Both the pores have the ability to change the conformational changes on the plasma membrane (Carolus et al., 2020).
The next models, namely, surface adsorption model and sterol sponge model, destabilize the plasma membrane, leading to disturbances in the cellular process such as endocytosis and regulation of membrane protein. Several studies have claimed that AmB follows an additional model of action toward oxidative damage. In Cryptococcus neoformans, addition of AmB causes cell becomes metabolically inactive and produces a strong oxidative damage to contribute cell death via lipid peroxidation processes. However, the exact mechanism of oxidative stress in the fungal cells is not clear. The process is assumed that binding of polyenes to the plasma membrane triggers oxidative stress, eventually leading to the apoptotic events in the cells including ROS production. AmB is known to auto-oxidize and generates free radicals; thus, the antifungals itself produces strong oxidative stress inside the cell (Carolus et al., 2020).
Essential Development of Azoles as Potential Antifungal Drugs
Azoles, the next category of approved antimycotic drugs, have been developed in the year 1969. It interferes in the ergosterol biosynthesis and inhibits the enzyme 14α-sterol demethylase. Various azoles have been bifurcated into imidazoles and triazoles. Major imidazoles are miconazole, bifonazole, clotrimazole, and ketoconazole but are largely limited to systemic use because of their hepatotoxicity. In 1981, ketoconazole was the first clinically approved azole drug for the systemic use, but it has less effective against various fungal pathogens (Campbell et al., 2012).
The second group of azoles, triazoles, have much affinity toward the enzyme 14α-sterol demethylase and interfere with ergosterol synthesis, which, in turn, inhibit the fungal growth effectively than imidazoles. The first approved triazole is itraconazole followed by fluconazole. The three triazoles—voriconazole, posaconazole, and ravuconazole—have been accepted and used for systemic infections. During early 2000, the third-generation drug, echinocandins, had evolved. Caspofungin is the first approved antifungal echinocandin drug for the treatment of fungal infections and was well tolerated by the patients. Micafungin and anidulafungin have also been accepted, and echinocandins have been reported to have minimal side effects than polyenes and azoles (Lass-Flörl, 2011).
Early 2000 Revolution in Antimycotic Drug Discovery
5-fluorocytosine (5-FC) resistance can be very intrinsic and nearly 7% to 8% Candida species and filamentous fungi (Aspergillus and dermatophytes) have developed a resistance toward 5-FC. Nearly, a large number of fungi are known to be susceptible to polyenes drugs. Few of them, namely, Candida glabrata, Scedosporium prolificans, and Aspergillus terreus, have evolved resistance to polyenes (Revie et al., 2018). In the early 1990s, the azole drug resistance has been an alarming threat, and increased incidence of fungal infections was noted. However, the development in the field of antimycotic drug therapy has stabilized the issues. Echinocandins have been introduced in the year 2000, and the resistance to these drugs was rare events. Several Candida and Aspergillus species are known to be susceptible to echinocandins, but Cryptococcus neoformans shows strong resistant to these drugs. The mechanism of echinocandins is to disrupt the cell wall synthesis by inhibiting the enzyme β(1–3)-glucan synthase (Sanglard and White, 2006). The resistance mechanism has been developed due to the alteration in the composition of cell wall components in Cryptococcus neoformans. The antifungal drugs, their mode of action, and their targets along with their resistance mechanisms are explained in Figure 2.
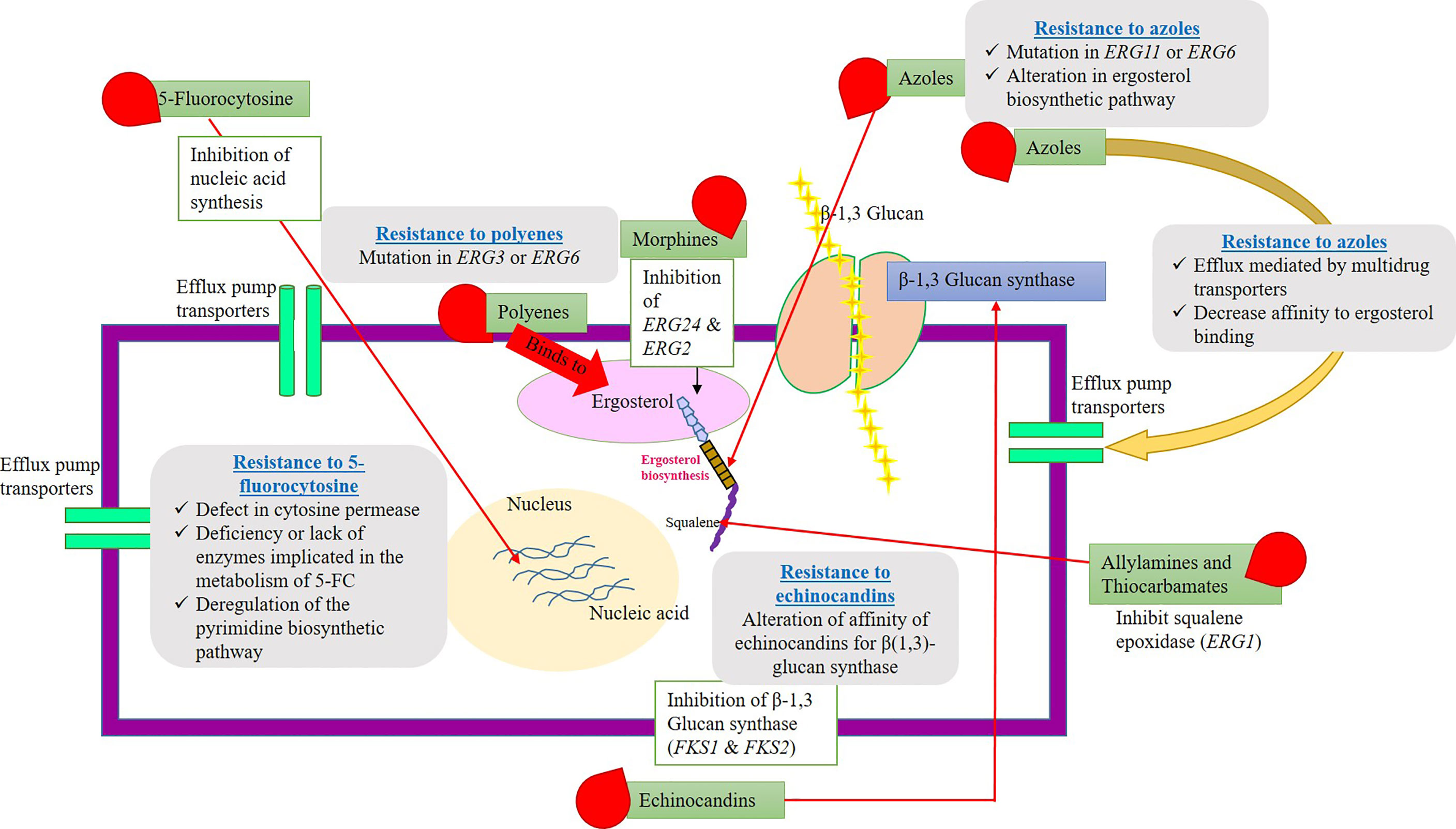
Figure 2 Schematic representation of fungal cell wall, antimycortic drugs, possible antifungal activity (target site), and resistance to commercial antifungal drugs. The picture explains various antifungal compounds and their target site on the pathogenic fungi. Along with that, the plausible resistance mechanisms evolved by the fungal species to clinically approved antimycotic drugs have clearly depicted. Resistance gene/proteins/channels might be antifungal targets for the development of novel antimycotic compounds or alternative chemosensitization/drug repurposing approaches.
Resistance Mechanism to Common Antimycotic Drugs
Resistance to antimycotic drugs is a significant threat to human. Resistant mechanisms evolved by fungal pathogens fall under different categories including (i) alterations of transporters, (ii) alteration of targets, (iii) utilization of compensatory pathways, and (iv) presence of complex multicellular structures. Figure 3 shows the antimicrobial resistance in fungal pathogens.
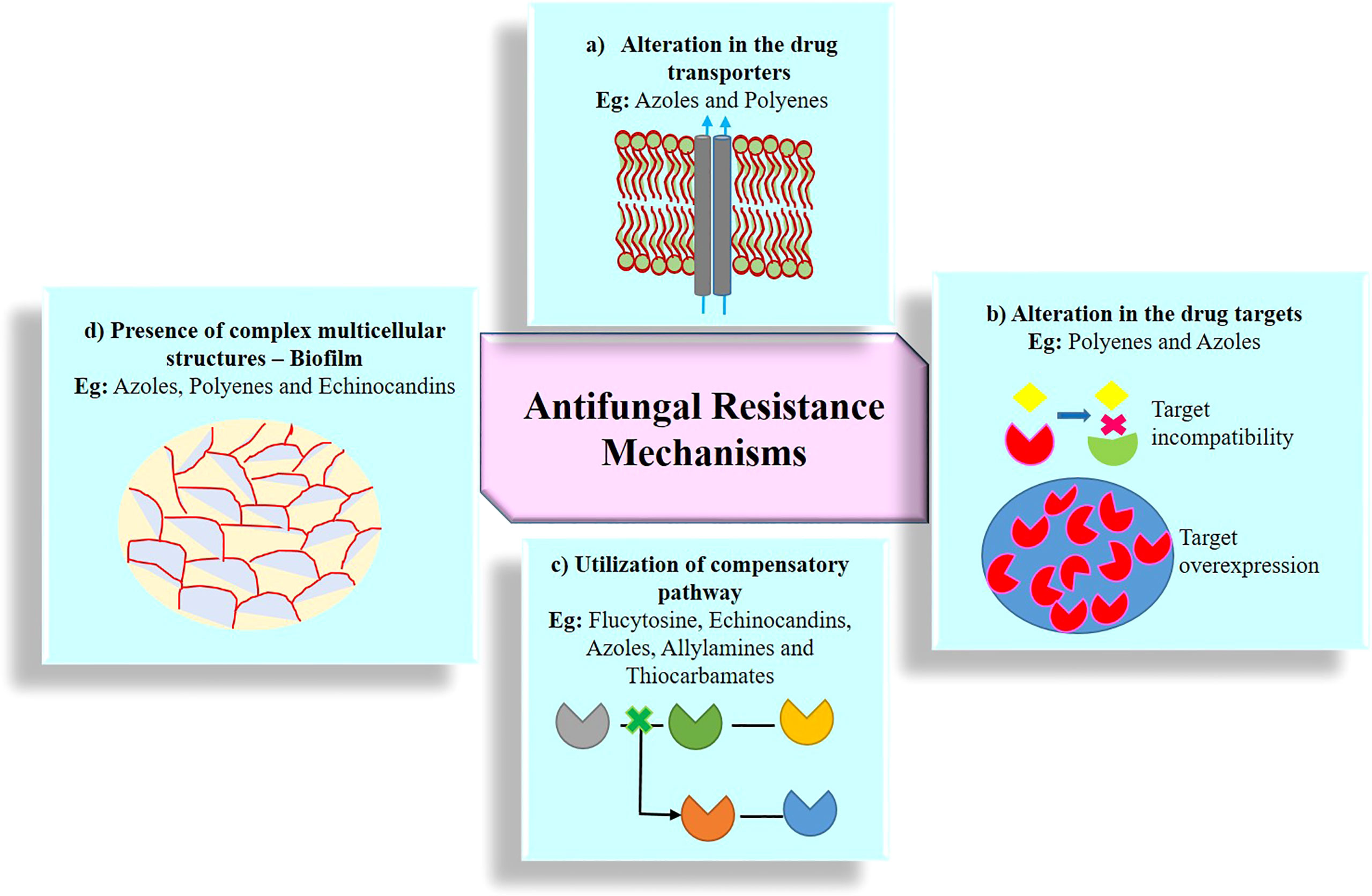
Figure 3 Emergence of antifungal resistance through various mechanisms. (A) Alteration in the drug transporters reduce the accumulation of drug molecules in the cell and expel the drugs out of the cell via efflux protein transporter; two kind of major efflux pump transporters are ABC (ATP-binding cassette) protein transporters and MFS (major facilitator superfamily) protein transporters; (B) alteration in the drug targets substantially reduces the affinity of the drug for its targets by structural modification, mutation, upregulation, downregulation, and overexpression of drug targets; (C) utilization of compensatory pathways, ergosterol biosynthesis pathway alteration, and plasma membrane composition variation; (D) presence of complex multicellular structures, formation of biofilm, and polymicrobial biofilms (bacterial species often associated with one or more fungal strains).
Alterations of Transporters
Two different efflux pumps exists in fungal pathogens, namely, ABC (ATP-binding cassette) protein transporters and MFS (major facilitator superfamily) protein transporters. Nucleotide-binding domains (NBD) and transmembrane domains (TMD) are the two main domains found in ABC proteins. NBD involves in binding and hydrolysis of ATP providing energy for the translocation of substrates, whereas TMD contributes to a substrate channel via membrane. Two NBD and two TMD domains have been reported in several fungal ABC transporters. MFS protein transporters are generally referred to as proton antiporters. They can utilize the electrochemical potential and proton motive force across the cell membrane for the translocation of substrates. They do not contain NBD but has TMD.
MFS transporter is the second class of transporters involved in the development of resistance in several fungi. It is found in almost all kingdom and involved in symport, antiport, and uniport of several substrates in and out of the cell. It exerts antifungal resistance by proton antiport and has classified into two major groups: a) the transporter: H+ antiporter-1 family, it has 12 transmembrane α-helix (TMS); b) the transporter: H+ antiporter-2 family, it has 14 transmembrane α-helix (Prasad and Rawal, 2014; Esquivel et al., 2020).
Aspergillus fumigatus is responsible for severe invasive aspergillosis in host especially, in immunocompromised individuals. Azole drugs (ITR, VOR, and POS) and echinocandins are the frontline antifungals employed in the treatment of invasive aspergillosis. Azoles directly interfere in the ergosterol pathway by inhibiting the enzyme 14-α demethylase, a key enzyme in ergosterol biosynthesis (Pfaller, 2012; Cowen et al., 2015). Important resistant mechanism in various pathogens is the ability of several membrane protein families to mediate efflux of drug compounds. This kind of resistant involves ABC transporters and MFS transporters that are known to upregulate in the azole-resistant fungal pathogens. Novel genes in Aspergillus fumigatus involved in azole resistance have been identified by developing a functional complementation system, and their cDNAs are expressed in S. cerevisiae model. Among the several genes including atrF and atrl of ABC transporters and mdrA of MFS transporters, atrl gene is responsible for the resistance toward itraconazole and voriconazole. However, mdrA shows resistance only to voriconazole in S. cerevisiae model system. Further, targeting the genes responsible for the azole resistance by chemosensitizing approach might make the pathogens susceptible to azole drugs (Meneau et al., 2016). ASP2397, a natural compound tested on in vivo Aspergillus fumigatus mouse infection model, exerted maximum fungicidal activity compared to that of commercial antimycotic drugs, namely, amphotericin B, voriconazole, posaconazole, itraconazole, and fluconazole (Nakamura et al., 2019).
Randomly, 50% of the fungal incidence were reported with Candida spp. among which Candida parapsilosis is of major global concern. Azoles are the common antimycotic drugs prescribed for the control and treatment of candidiasis but the organisms have developed resistance to azoles to sustain in the host (Silva et al., 2011; Ding et al., 2018). Berkow et al. have studied about the genes involved in the azole-resistant mechanisms including drug transporters (CDR1 and MDR1) and ergosterol biosynthesis (ERG3 and ERG11) (Berkow et al., 2015). Conclusively, the studies suggested that genes encoded for cdr1, mdr 1, and erg11 are directly involved in the formation of azole resistance in Candida parapsilosis. Aforementioned studies have recommended that these putative genes are the suitable targets for the chemosensitization strategies.
Nascimento et al. have analyzed the itraconazole-resistant mechanisms in Aspergillus fumigatus involving two major efflux protein (MDR) AfuMDR3 and AfuMDR4. They showed prominent changes in their expression level in itraconazole resistant. Among them, AfuMDR3 belongs to MFS transporters, whereas AfuMDR4 was a typical member of the ABC superfamily. Overexpression of either one or both the efflux transporter genes is responsible for the enhanced itraconazole resistance in Aspergillus fumigatus isolates, and, thus, in turn, they are the suitable drug targets to chemosensitize or finding novel drugs for the treatment of aspergillosis. Ferreira et al. have also found that overexpression of drug efflux pump transporters is the main reason for the development of drug resistance in Aspergillus fumigatus, where five ABC transporters genes and MFS transporters genes contribute to voriconazole-resistant development. cdr1B, ABC transporter efflux pump genes, led to the emergence of azole resistance in Aspergillus fumigatus (Paul et al., 2013). Overexpression of drug efflux pump protein MFS genes, AflMDR1 and AflMDR2, is responsible for cilofungin resistance in A. flavus (Tobin et al., 1997), and overexpression of ABC transporters genes, atrA, atrB, atrC, and atrD, leads to azole resistance in Aspergillus nidulans (Semighini et al., 2002).
Alteration of Drug Targets and Utilization of Compensatory Pathways
In addition to the overexpression of efflux pump transporters, the mutation of drug targets including enzymes responsible for ergosterol or β-1,3 glucan synthesis might induce potential resistance in fungal pathogens to common antifungals. Generally, 25 known enzymes are involved in the regulation of ergosterol biosynthesis in fungal cell. It is denoted by ERG enzymes where any mutation, alteration, and gene deletion of these enzymes lead to the resistance of fungi to various antimycotic drugs (Bhattacharya et al., 2018). ERG1, ERG2, ERG6, ERG11 alterations lead to the azole resistance and amphotericin B resistance in Candida albicans (Hull et al., 2012). Point mutations at the target site (drug binding site) have reduced the affinity and thus, in turn, showed the inability of the drug to bind with targets. CYP51A encodes the enzyme, namely, lanosterol 14α-demethylase, and mutations at this gene have been recorded for the development of azole resistance in Aspergillus fumigatus (Fuentefria et al., 2018). Y136F substitution on this gene has also been noted with the decreased susceptibility to fluconazole and voriconazole (Scorzoni et al., 2017). These point mutations have particularly constraint-specific amino acid substitutions on the target site (enzyme). Erg11 has altered by point mutation in Candida albicans, causing severe resistance toward triazole drugs. Same kind of resistant patterns has been observed in Aspergillus fumigatus and Cryptococcus neoformans. Mutation on glucan synthase enzyme (FKS1) has significantly enhanced the resistance to echinocandins antifungal drugs. A single–amino acid substitution in FKS1 gene largely contributes the drastic reduction in the susceptibility of Candida parapsilosis, Candida metapsilosis, and Candida orthopsilosis to echinocandins (Garcia-Effron et al., 2008). 5-flucytosine is generally imported by the enzyme, cytosine permease, and encoded by the gene Fcy2p followed by the deamination process, utilizing the cytosine deaminase enzyme (Fcy1p) and finally converted to 5-fluorouridine (5FU). With the action of phosphoribosyltransferase (Fur1p), 5-fluorouridine is converted to 5FU monophosphate. In activation or mutation of any of the abovementioned genes confer a strong resistance to 5-flucytosine (Chapeland-Leclerc et al., 2005; Papon et al., 2007). Mutation in the ERG3 gene is responsible for the formation of 14α-methyl-3,6-diol from 14α-methyl-fecosterol and eventually, accumulating the precursors, can replace the ergosterol and utilize the different pathway. Ultimately, it leads to the strong resistance toward commercial antibiotics in Candida sp. (Vandeputte et al., 2012).
Higher activity of enzymes such as catalase and superoxide dismutatse (antioxidant system) along with the intense stress response via heat-shock proteins (Hsp70 and Hsp90) in fungal cell resulted in the increased drug resistance in Aspergillus terreus (Posch et al., 2018). The key enzyme in ergosterol biosysnthesis is 14-α-demethylase (CYP51B), and their mutation and other ERG alterations contribute to the greater resistance to 5-flucytosine drugs in Candida sp. (Costa et al., 2015). Other important features are overexpression of ABC pump transporter genes cdr1, cdr2, and mdr1 that efflux azole drugs out of the fungal cell and create a greater resistance mechanism, whereas AtrF, MDR3, and MDR4 and their overexpression lead to azole resistance in Aspergillus fumigatus (Cowen et al., 2015). Often, the biofilm formation leads to triazole resistance in Candida albicans. Alterations in β-glucan synthase gene (amino acid substitutions) FKS1 and FKS2 contribute for echinocandins drug resistance in several fungal pathogens (Rodrigues et al., 2018). Thickened cell wall by increased synthesis of 1,3,β-D-glucans shows relatively greater drug resistance in Candida tropicalis (Mesa-Arango et al., 2016).
Presence of Complex Multicellular Structures
In general, biofilms are referred as surface attached microbial communities surrounded by self-produced polymer matrix. Infectious organisms might develop a biofilm on abiotic surfaces including implanted medical devices, catheters, lenses, and pacemakers that contribute greater resistance to most of the antifungals and pose a serious threat to control and treat the fungal infections (Sardi et al., 2014). For example, 80% of all infections were associated with the biofilm-mediated fungal infections in patients. One of the important resistant mechanism in Candida sp. is forming the biofilm-mediated infections that strongly confer the antifungal resistance to several commercial antibiotics. Both Candida and non-Candida species including Candida glabrata, Candida tropicalis, and Candida krusei have the ability to form a clinically relevant biofilm in patients (Benakanakere and Kinane, 2012). In molecular aspects, formation of biofilm associated with the transcriptional regulatory genes, TEC1, BCR1, and EFG1, are the important genes responsible for the strong growth of biofilms in Candida albicans. Agglutinin-like sequence (ALS) has eight members from ALS1 to ALS8 that code for glycophosphatidylinositol-anchored cell surface glycoproteins. Among them, ALS3 has been actively associated with the biofilm formation and contributes for drastic reduction in susceptibility to antifungals (Murciano et al., 2012). Moreover, ALS, other enzymes, namely, proteases (SAPs), lipase (LIP), and phospholipases (PLB), are contributing to the biofilm development in C. albicans and, thus, increased resistance evolved to various antimycotic drugs (Mayer et al., 2013).
With the aforementioned clinical data, targeting the resistance mechanism of pathogenic fungi has deserved much attention by chemosensitization approaches utilizing natural compounds.
Sensitization of Fungal Pathogens to Natural Chemosensitizers
Development of resistance of the fungal species against numerous antifungal drugs is of growing concern in the scientific community. The rate of increasing drug resistance is greater than that of novel compounds introduced for the treatment of fungal infections. Considering the dearth of current conventional antimycotic drugs, screening of molecules to enhance the fungicidal activity has been prioritized. Paradoxically, against resistant fungal strains, chemosensitization is an appealing alternative approach to make the same drug susceptible to the fungal pathogens. There are various synonymous words for chemosensitizers including enhancers, synergizers, augmenting agents, potentiators, sensitizers, and even others. We need to focus on the compounds that are also not toxic to the patients. In ancient era, herbal medicine has been widely applied for treating mycoses. Natural compounds used as chemosensitizer are initially referred as “pharmacologic circumvention of multidrug resistance”, where vinca alkaloids potentially inhibit the efflux pump overexpression for susceptibility of anticancer drugs (Ford and Hait, 1994). By considering the facts of emergence of antifungal resistance to commercial antifungal drugs, researchers have spurred on an endeavor to look for new strategies and natural compounds in antifungal chemotherapy. Wide spectrum of natural compounds acts as a chemosenitizer to augment fungicidal activity and is tabulated in Table 1.
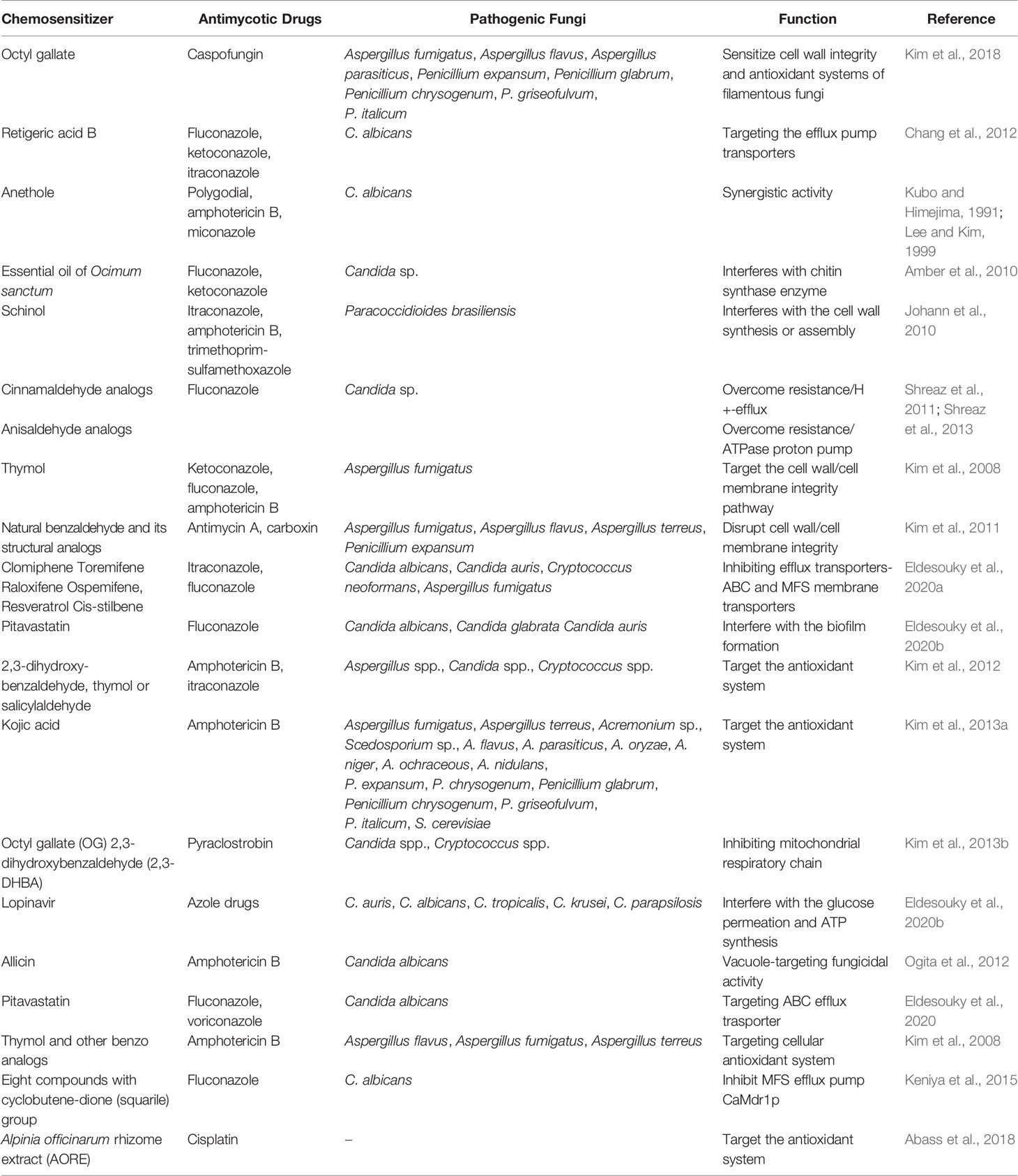
Table 1 Natural chemosensitizer along with the commercial antimycotic drugs to augment fungicidal activity against human mycoses.
Predominantly, natural phytocompounds can serve as a great therapeutic agent and precursor for the modification of structures to augment the antifungal activity of commercial antimycotic drugs. Several natural essential oils, plant extracts, and secondary metabolites have been applied as a chemosensitizer for the treatment of fungal infections along with the commercial antifungal drugs. Various plant extracts, grape seed extract, tea extracts, and essential oils have been used including carvacrol (Rao et al., 2010), anethole (Kubo and Himejima, 1991), cinnamaldehyde analogs, anisaldehyde analogs (Shreaz et al., 2013), benzol analogs (Zore et al., 2011), cinnamic acid (Faria et al., 2011), thymol, eugenol, methyeugenol (Braga et al., 2007; Ahmad et al., 2010), geraniol (Shin, 2003), oils from Origanum vulgare, Agastache rugose, Euphorbia characias, Santolina chamaecyparissus, Pelargonium graveolens, Melaleuca alternifolia, Thymus vulgaris, Cinnamomum cassia, and Ocimum sanctum. Apart from many terpenoids and phenols including farnesol, retigeric acid B, capisterone A and B, and schinol, propylgallate, octylgallate, epigallocatechin gallate greatly intensify the antifungal activity along with the commercial antimycotic drugs by targeting the resistance mechanisms such as interfering with ergosterol synthesis, β (1-3) glucan synthase enzyme, and efflux pump transporters (Shin and Kang, 2003; Giordani et al., 2004; Giordani et al., 2006; Rosato et al., 2009; Campbell et al., 2012). Application of safer chemosensitizing agent has potential efficacy against various human pathogenic mycoses.
Antifungal Pipeline for Natural Chemosensitizers
Exploring the natural compounds possessing the antifungal activity has now been reinforced at global level. A plenty of natural compounds from plants could serve as an excellent chemosensitizers and needs to be studied in detail for their properties as well. Aristolochia bracteolata applied in traditional medicine have been proved to have potent antifungal activity against Aspergillus niger, Aspergillus terreus, Penicillium notatum, and Rhizopus stolonifer (Kavitha and Nirmaladevi, 2009). Isatin is an alkaloid compound, isolated from Couroupita guianensis, having a great fungicidal efficacy against Asperillus niger, Aspergillus flavus, Aspergillus fumigatus, Candida albicans, Mucor oryzae, and Rhizopus indicus that were recorded (Kavitha et al., 2013). Trianthema portulacastrum has also proven to be an herbal medicine to combat against various fungal pathogens, namely, Aspergillus niger, Aspergillus fumigatus, Rhizopus, and Candida albicans (Kavitha et al., 2014). Khanzada et al. (2021) have studied several edible medicinal plants such as Cinnamomum zeylanicum, Cinnamomum tamala, Amomum subulatum, Trigonella foenumgraecum, Mentha piperita, Coriandrum sativum, Lactuca sativa, and Brassica oleraceae var. italic to validate their antifungal efficacy against Fusarium solani, Aspergillus niger, Aspergillus flavus, and Mucor sp.; among them, Cinnamomum zeylanicum and Cinnamomum tamala have exhibited greatest antifungal activity that might be due to the presence of rutin and kaemferol. Vitis vinifera contains phenolics and flavonoids in their extracts in sufficient amount and thus, in turn, exhibits strong antifungal activity against numerous clinical pathogens (Simonetti et al., 2020). Nearly 23 hydroethanolic extract of medicinal plants were tested on Candida albicans, Cryptococcus neoformans, and Cryptococcus gatti. Among them, Poincianella pyramidalis, A. occidentale, Anadenanthera colubrina var. cebil, Myracrodruon urundeuva, and Mimosa oftalmocentra show potential fungicidal activity against all kinds of strains (Silva et al., 2020). Aloe megalacantha is a traditional medicinal plant employed for the treatment of various symptoms and infections including wound, dandruff, malaria, diabetes, impotence, colon cleansing, amoeba, ascariasis, abdominal pain, urine retention, snake bite, and evil eye. This plant extract has exhibited highest inhibitory profile against C. albicans, C. glabrata, C. tropicalis, and C. krusei (Asmerom et al., 2020). By considering the fate of the medicinal plants and their compounds, it can be exploited as great chemosensitizers to sensitize the microbes to commercial antifungal drugs.
AFP From Aspergillus giganteus
Natural compounds from plant origin or microbial origin could serve as great platform for enhanced fungicidal activity. Further, deep exploration into antifungal protein might provide a possible breakdown of resistance mechanism counteracted by pathogenic fungi, ultimately augmenting the strong antimycotic activity. The antifungal protein AFP is a secretory protein from a filamentous fungi Aspergillus giganteus, which are small, basic, and cysteine-rich proteins that endeavor the future antimycotic strategies due to their specific inhibitory potential on several filamentous and non-filamentous fungal pathogens (Krishnamurthy et al., 2020; Ravindhiran et al., 2021). β-barrel topology in AFP is composed of five antiparallel β-strand, could be stabilized by four disulfide bridges, and contributes to the extreme resistance to heat and protease degradation. Nearly 12 lysine residues can be partially involved in the formation of cationic site such as K9, K10, and K32, due to the positive net charge of AFP. After that, the cationic site conjugates with the hydrophobic domains, namely, Y29, V30, Y45, and V50 furnish the amphipathic nature of AFP. Paradoxically, the AFP can easily traverse and perturb the plasma membrane for the suppression of growth of numerous filamentous fungal pathogens. Furthermore, wide range of functional properties make AFP as a prime candidate for the treatment of fungal infections: i) AFP is found to be resistant to heat and protease degradation; ii) it is synthesized from Aspergillus giganteus by a sustainable way; iii) microliters of AFP can be effective against several fungal pathogens (Moreno et al., 2005; Barakat et al., 2010). afp gene is encoded for the AFP from Aspergillus giganteus, and it had been widely applied to suppress resistance mechanisms in various fungal pathogens. In addition, AFP enters into the nucleic acids of host cell and causes charge neutralization and condensation of the DNA molecule and thus, in turn, produces strong antifungal activity (Narvaez et al., 2018).
Earlier studies in our laboratory have proved the efficacy of AFP protein from Aspergillus giganteus on various human pathogens, including Aspergillus fumigatus, Aspergillus flavus, Cryptococcus neoformans, and Candida albicans (Krishnamurthy et al., 2020; Ramya and Kavitha, 2021; Ravindhiran et al., 2021). Not only a human pathogen but also a phytopathogen has also been greatly governed by AFP. Aflatoxigenic Aspergillus flavus in corn at pre-harvest stage was completely eradicated by the application AFP in the field grown corn. The optimization for the carbon source, nitrogen source, pH, temperature, and water activity to enhance the AFP production and inhibition toward both phytopathogenic and zoopathogenic fungi were evaluated (Krishnamurthy et al., 2020). Virulence proteins of Aspergillus fumigatus, UDP-N-acetylglucosamine pyrophosphorylase (6TN3), N-myristoyl transferase (4CAW), and Chitinase (2A3B) are cell wall proteins, actively participated in the virulence mechanism in the host. In Cryptococcus neoformans, the target proteins are Farnesyl transferase (3SFX), Ionisine 5′-monophosphate dehydrogenase (4AF0), Ribokinase (6CW5), Serine/Threonine-protein phosphate 2B catalytic subunit A1 (6TZ8), Choline kinase (6WHP), Candida albicans Dihydrofolate reductase (1AI9), Myristoyl-CoA: protein N-Myristoyl transferases (1IYL), Secreted aspartyl proteases (1ZAP), and Lanosterol 14-α demethylase (5V5Z) are the potential virulence proteins responsible for the pathogenesis in the host. In silico prediction by molecular docking studies have proved that the antifungal proteins from Aspergillus giganteus and their derivatives has good interaction with the virulence proteins of Aspergillus fumigatus, Candida albicans, and Cryptococcus neoformans (Ravindhiran et al., 2021). Mechanism of action of afp on both plant and human fungal pathogens is depicted in Figure 4. AFP selectively inhibits the growth of fungal pathogens without affecting the host. AFP from Aspergillus giganteus has gained a much attention due to its application in medicinal panacea. AFP can act as a great chemosensitizer to make the pathogenic fungus susceptible to the commercially available antimycotic drugs. Co-application of chemosensitizing agents with AFP will alleviate the health and environmental associated risk by their unique underlying mechanisms. This could be a promising approach for the minimal amount of commercial drugs and can alleviate the burden of fungal infections at global level (Kim et al., 2008).
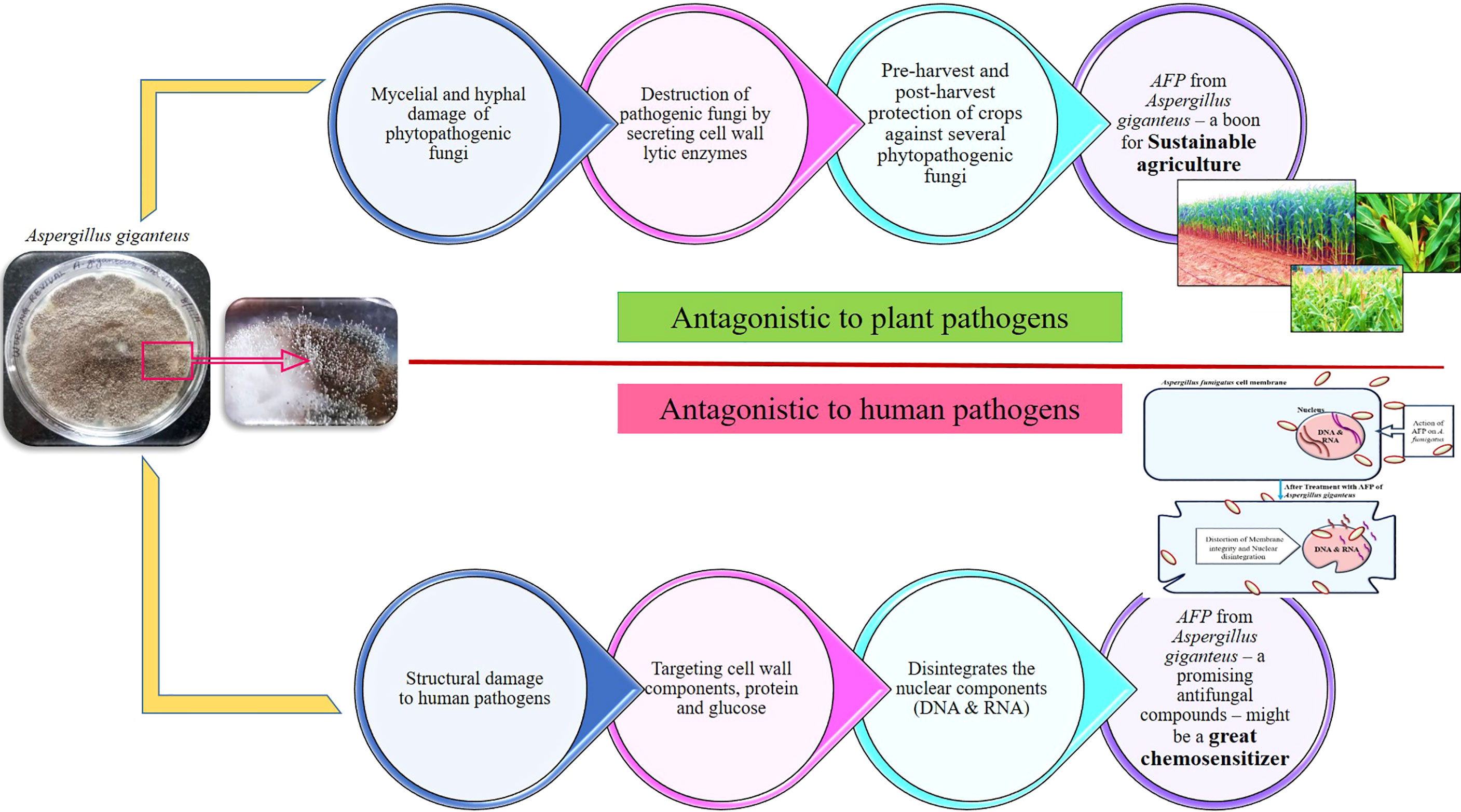
Figure 4 Antagonistic mechanism of AFP from Aspergillus giganteus on plant and human fungal pathogens. As a biocontrol agent, it selectively inhibits and kills the growth of plant fungal pathogens by secreting specific cell-wall degrading enzymes namely, amylase, protease, β-1,3 glucanase, chitinase, and cellulase. As antifungal compounds in clinical area, it could possibly perturb the membrane of human fungal pathogens by interfering with the cell wall components and nuclear components. AFP from Aspergillus giganteus might be a promising biocontrol agent for sustainable agriculture and optimistic novel compounds for the treatment of systemic fungal infections. In addition to their remarkable values, it could be a potent chemosensitizer to sensitize the plant and human fungal pathogens to commercial fungicides and antimycotic drugs.
Mode of Action of AFP From Aspergillus giganteus
Filamentous fungi are posing a petrifying threat in human; among them, Aspergillus spp. have been recorded with high mortality and morbidity rate, causing aspergillosis. Indistinguishably, Aspergillus and Fusarium spp. have been the rationality for the severe crop losses. However, the limited applications of the existing antimycotic drugs, a prerequisite for the efficient and economical antifungal agents, are highly preferable. Hence, the development of drugs with desired properties including sustainability, targeted actions, enhanced efficacy, and low toxicity is of major concern.
Deciphering the mode of action of antifungal compounds is an important aspect to improve their efficacy to fight against the pathogens and interfere in the development of antifungal resistance mechanisms and amplification of their applications in therapeutics and in agriculture. Generally, AFP from Aspergillus giganteus can act on several cellular targets to exert their potential activities to eradicate the pathogens. In this regard, here, it throws light on the mode of action of AFP and harnesses their potential efficacy on controlling the pathogenic progressions. As noted above, the AFP has abundantly produced from Aspergillus giganteus with small (51 amino acids) and cationic structures. Minimal protein concentration ranging from 1 to 20 µM is enough to inhibit the growth of Aspergillus and Fusarium spp. Moreover, it does not exhibit cytotoxic and immunogenic effects on any types of mammalian cells, which attribute to their exemplary potential to act as a drug lead compounds. The mode of action of AFP from Aspergillus giganteus is explained in Figure 5.
AFP accumulates into the cell wall of pathogenic Aspergillus niger and is localized at the plasma membrane, causing severe membrane alterations in the pathogens as observed by electron microscopy. Nevertheless, this is the first AFP localized at the cell wall and plasma membrane identified so far. Thus, it could be studied extensively for the utilization of their remarkable targeting potential. Hagen et al. have studied the interaction of AFP with chitin biosynthesis pathway in pathogens. However, they found that N-terminal part from 1 to 33 amino acid is essential for efficient target binding and have a positive result on the production of truncated protein with the introduction of C14S, C26S, and C28S substitutions. From their studies, they have come to the conclusions that AFP can able to disturb the transport of chitosomes to the hyphae, control the release of chitin synthases from chitosomes, restrict the localization and anchoring of the enzyme to the plasma membrane for the chitin synthesis, and directly inhibit the chitin synthase enzyme activity or binding to the precursor molecules or to the polymerized chitin molecules. In their study, AFP has preferentially interfered with the chitosomes and class III and V chitin synthases enzymes. Finally, the membrane localization interaction that has occurred between chitin synthases and AFP contributes to the stretching of the plasma membrane, thus, significantly reducing the membrane integrity of the pathogens (Hagen et al., 2007).
Different culture conditions and protocols might have an influence on the antagonistic properties of the AFP from Aspergillus giganteus. For example, the MIC of AFP tested on agar dilution method is 20 times higher than that of broth microdilution methods. Sensitivity of the pathogens to AFP is determined due to the interactions with a membrane-based target, and it had been confirmed by the localization experiments. The studies confirmed that the AFP was constrained with in the hyphae and spheroplasts, which has been proved by FITC labeling and immunofluorescence staining, and both the methods have exhibited that the AFP preferentially binds with the plasma membrane causing permeabilization. SYTOX green assay has detected the membrane permeabilization caused by AFP in sensitive fungi, but it can be achieved only when high concentration of AFP is treated, i.e., higher than MIC (1 µg/ml; but for membrane permeabilization 10 µg/ml is needed). Conclusively, the permeabilization of plasma membrane is achieved due to the interactions of cation with the negatively charged target site of AFP and the inhibitory potential of AFP could be attained by receptor-mediated binding mechanisms (Theis et al., 2003).
The mode of action of AFP against rice blast fungus M. grisea susceptibility was studied in plant and animal cells. The electron microscopic results have proved the AFP-induced cellular damage and pore formation on the plasma membrane was observed in treated M. grisea. The mechanism of action involves disruption of plasma membrane and permeabilization of the pathogens. Congo-red staining reveals the significant deposition of chitin at the hyphal tip and leads to no growth of hyphal elongation. Along with that, it interferes with the nucleic acids, causing neutralization and condensation, leading to cell death pertaining to their potential antifungal activity that was confirmed by Alexa-labeled SYTOX green uptake assay (Moreno et al., 2006). The antifungal properties of AFP promote the neutralization and condensation of DNA molecules, which were exhibited by electrophoretic mobility and EtBr displacement assay. Because AFP is an extracellular protein, it exerts their antagonistic function on phytopathogenic fungi on rice by binding to the membrane and nuclear targets of pathogen and also providing protections to the plants (Pozo et al., 2002). The microscopic observations revealed that the AFP has effectively reduced the hyphal elongation and swollen hyphal tip and attributes to the potential antifungal activity of Aspergillus giganteus on Botrytis cinerea. Botrytis blight is the disease caused by B. cinerea on ornamental plants and is responsible for the major crop loss. An additive effect of AFP with Cecropin A was highly measured when incubated the plants with the compounds at different time intervals. This study has claimed that the AFP is a promising biocontrol agent for sustainable agricultural practices (Moreno et al., 2003). Not only in medicine and agriculture, the prime application of AFP is also recorded in food industries for juice extraction and clarification. The polygalacturonases are pectinolytic enzymes that are used for the hydrolysis of pectin-cell wall back bone for juice preparation. The enhanced polygalacturonases activity was observed in presence of medium containing β-mercaptoethanol, Co2+, Mn2+, , Na+, and dithiotheritol; thus, it could be a good alternative for pectinase production in food industries (Pedrolli and Carmona, 2010).
AFPNN5353 is a protein secreted by Aspergillus giganteus, a defense-like protein, rich in cytosine residues. The protein is known to inhibit the growth and germination of plant and human fungal pathogens. This protein rapidly elevates the Ca++ resting level in the fungal cell, and prolonged perturbation of Ca++ homeostasis caused programmed cell death in fungal pathogens. The studies revealed the interference of AFP with the cell wall signaling pathways and inhibition of fungal pathogens (Binder et al., 2011).
Conclusion and Future directions
Global burden of fungal infections is of significant threat, and a number of treatment strategy has been emphasized to treat fungal infections. The rate of development of new antifungal drugs is unsatisfactory, and it could not fulfill the future demands. This should be taken under consideration as several patients are prone to severe fungal infections. Many fungal species have evolved resistance toward many commercial antimycotic drugs by several aforementioned mechanisms. Exploring the various aspects involved in antifungal resistance mechanisms will be a great approach of curing global incidence of fungal infections.
By virtue of the facts and factors, exploring chemosensitization strategy could be an excellent alternative approach for making the drug susceptible for fungal pathogens. Antifungal protein from Aspergillus giganteus has been proved to have an intense level of inhibition against several human and agricultural mycoses.
Because AFP has shown greater antifungal activity against both filamentous and non-filamentous fungal pathogens, combination with commercially available antibiotics could perturb the resistance mechanism to augment the fungicidal activity of antimycotic drugs. The cytotoxic profile of AFP conveys that it is found to be safe, and even a lower amount of AFP can be effective in inhibiting several pathogens.
Therefore, we find that AFP from Aspergillus giganteus recorded with promising antifungal activity against several pathogens has been proven to be a new kind of chemosensitizer. Conclusively, the AFP has the chemosensitizing attribute to enhance the sensitivity of conventional antifungal drugs. Further, the chemosensitization strategy is required to study extensively using in vitro and in vivo model system to validate their potential augmenting fungicidal capacities of existing antifungal drugs.
Author Contributions
KD conceptualized and planning the coherency of the manuscript, and all other authors contributed to the organization, review, editing, and preparation of the paper. RR and KS completed all figures and JNS provided critical data. All authors contributed to the article and approved the submitted version.
Conflict of Interest
The authors declare that the research was conducted in the absence of any commercial or financial relationships that could be construed as a potential conflict of interest.
Publisher’s Note
All claims expressed in this article are solely those of the authors and do not necessarily represent those of their affiliated organizations, or those of the publisher, the editors and the reviewers. Any product that may be evaluated in this article, or claim that may be made by its manufacturer, is not guaranteed or endorsed by the publisher.
Abbreviations
AFP, antifungal protein; VOR, voriconazole; AmB, amphotericin B; POS, posaconazole; MICA, micafungin; ITR, itraconazole; ANI, anidulafungin; CAS, caspofungin; AmA, amphotericin A; 5-FC, 5-fluorocytosine; ABC, ATP-binding cassette transporters; MFS, major facilitator superfamily transporters; NBD, nucleotide-binding domains; TMD, transmembrane domains; TMS, transmembrane α-helix; MDR, multi-drug resistance; 5FU, 5-fluorouridine; ALS, agglutinin-like sequence; SAPs, serine aspartate proteases; LIP, lipases; PLB, phospholipases; DNA, deoxyribonucleic acid; MIC, minimum inhibitory concentration; FITC, fluorescein isothiocyanate.
References
Abass, S. A., Abdel-Hamid, N. M., Abouzed, T. K., El-Shishtawy, M. M. (2018). Chemosensitizing Effect of Alpinia Officinarum Rhizome Extract in Cisplatin-Treated Rats With Hepatocellular Carcinoma. Biomed. Pharmacoth. 101, 710–718. doi: 10.1016/j.biopha.2018.02.128
Ahmad, A., Khan, A., Khan, L. A., Manzoor, N. (2010). In Vitro Synergy of Eugenol and Methyleugenol With Fluconazole Against Clinical Candida Isolates. J. Med. Microbiol. 59 (10), 1178–1184. doi: 10.1099/jmm.0.020693-0
Amber, K., Aijaz, A., Immaculata, X., Luqman, K. A., Nikhat, M. (2010). Anticandidal Effect of Ocimum Sanctum Essential Oil and its Synergy With Fluconazole and Ketoconazole. Phytomedicine 17 (12), 921–925. doi: 10.1016/j.phymed.2010.02.012
Asmerom, D., Kalay, T. H., Tafere, G. G. (2020). Antibacterial and Antifungal Activities of the Leaf Exudate of Aloe Megalacantha Baker. Int. J. Microbiol. 1–6. doi: 10.1155/2020/8840857
Barakat, H., Spielvogel, A., Hassan, M., El-Desouky, A., El-Mansy, H., Rath, F., et al. (2010). The Antifungal Protein AFP From Aspergillus Giganteus Prevents Secondary Growth of Different Fusarium Species on Barley. Appl. Microbiol. Biotechnol. 87 (2), 617–624. doi: 10.1007/s00253-010-2508-4
Benakanakere, M., Kinane, D. F. (2012). Innate Cellular Responses to the Periodontal Biofilm. Periodontal. Dis. 15, 41–55. doi: 10.1159/000329670
Berkow, E. L., Manigaba, K., Parker, J. E., Barker, K. S., Kelly, S. L., Rogers, P. D. (2015). Multidrug Transporters and Alterations in Sterol Biosynthesis Contribute to Azole Antifungal Resistance in Candida Parapsilosis. Antimicrob. Agents Chemother. 59 (10), 5942–5950. doi: 10.1128/AAC.01358-15
Bhattacharya, S., Esquivel, B. D., White, T. C. (2018). Overexpression or Deletion of Ergosterol Biosynthesis Genes Alters Doubling Time, Response to Stress Agents, and Drug Susceptibility in Saccharomyces Cerevisiae. MBio 9 (4), e01291–e01218. doi: 10.1128/mBio.01291-18
Binder, U., Bencina, M., Eigentler, A., Meyer, V., Marx, F. (2011). The Aspergillus Giganteus Antifungal Protein AFPNN5353activates the Cell Wall Integrity Pathway and Perturbs Calcium Homeostasis. BMC Microbiol. 11 (1), 1–3. doi: 10.1186/1471-2180-11-209
Braga, P. C., Dal Sasso, M., Culici, M., Alfieri, M. (2007). Eugenol and Thymol, Alone or in Combination, Induce Morphological Alterations in the Envelope of Candida Albicans. Fitoterapia 78 (6), 396–400. doi: 10.1016/j.fitote.2007.02.022
Campbell, B. C., Chan, K. L., Kim, J. H. (2012). Chemosensitization as a Means to Augment Commercial Antifungal Agents. Front. Microbiol. 3, 79. doi: 10.3389/fmicb.2012.00079
Carolus, H., Pierson, S., Lagrou, K., Van Dijck, P. (2020). Amphotericin B and Other Polyenes—Discovery, Clinical Use, Mode of Action and Drug Resistance. J. Fungi 6 (4), 321 doi: 10.3390/jof6040321.
Chang, W., Li, Y., Zhang, L., Cheng, A., Lou, H. (2012). Retigeric Acid B Attenuates the Virulence of Candida Albicans Via Inhibiting Adenylyl Cyclase Activity Targeted by Enhanced Farnesol Production. PLoS One 7 (7), e41624. doi: 10.1371/journal.pone.0041624
Chapeland-Leclerc, F., Bouchoux, J., Goumar, A., Chastin, C., Villard, J., Noël, T. (2005). Inactivation of the FCY2 Gene Encoding Purine-Cytosine Permease Promotes Cross-Resistance to Flucytosine and Fluconazole in Candida Lusitaniae. Antimicrob. Agents Chemother. 49 (8), 3101–3108. doi: 10.1128/AAC.49.8.3101-3108.2005
Costa, C., Ponte, A., Pais, P., Santos, R., Cavalheiro, M., Yaguchi, T., et al. (2015). New Mechanisms of Flucytosine Resistance in C. Glabrata Unveiled by a Chemogenomics Analysis in S. Cerevisiae. PLoS One 10 (8), e0135110. doi: 10.1371/journal.pone.0135110
Cowen, L. E., Sanglard, D., Howard, S. J., Rogers, P. D., Perlin, D. S. (2015). Mechanisms of Antifungal Drug Resistance. Cold Spring Harbor Perspect. Med. 5 (7), a019752. doi: 10.1101/cshperspect.a019752
Ding, Y. L., Shen, N., Zhou, Q. T., He, B., Zheng, J. J., Zhao, X. M. (2018). Clinical Analysis of Candidemia in Immunocompetent Patients. Beijing Da Xue Xue Bao. Yi Xue Ban= J. Peking University Health Sci. 50 (6), 1063–1069. doi: 10.19723/j.issn.1671-167X.2018.06.022
Eldesouky, H. E., Salama, E. A., Hazbun, T. R., Mayhoub, A. S., Seleem, M. N. (2020a). Ospemifene Displays Broad-Spectrum Synergistic Interactions With Itraconazole Through Potent Interference With Fungal Efflux Activities. Sci. Rep. 10 (1), 1–0. doi: 10.1038/s41598-020-62976-y
Eldesouky, H. E., Salama, E. A., Lanman, N. A., Hazbun, T. R., Seleem, M. N. (2020b). Potent Synergistic Interactions Between Lopinavir and Azole Antifungal Drugs Against Emerging Multidrug-Resistant Candida Auris. Antimicrob. Agents Chemother. 65 (1), e00684–e00620. doi: 10.1128/AAC.00684-20
Eldesouky, H. E., Salama, E. A., Li, X., Hazbun, T. R., Mayhoub, A. S., Seleem, M. N. (2020c). Repurposing Approach Identifies Pitavastatin as a Potent Azole Chemosensitizing Agent Effective Against Azole-Resistant Candida Species. Sci. Rep. 10 (1), 1–2. doi: 10.1038/s41598-020-64571-7
Esquivel, B. D., Rybak, J. M., Barker, K. S., Fortwendel, J. R., Rogers, P. D., White, T. C. (2020). Characterization of the Efflux Capability and Substrate Specificity of Aspergillus Fumigatus PDR5-Like Abc Transporters Expressed in Saccharomyces Cerevisiae. MBio 11 (2), e00338–e00320. doi: 10.1128/mBio.00338-20
Faria, N. C., Kim, J. H., Gonçalves, L. A., Martins, M. D., Chan, K. L., Campbell, B. C. (2011). Enhanced Activity of Antifungal Drugs Using Natural Phenolics Against Yeast Strains of Candida and Cryptococcus. Lett. Appl. Microbiol. 52 (5), 506–513. doi: 10.1111/j.1472-765X.2011.03032.x
Ford, J. M., Hait, W. N. (1994). Pharmacologic Circumvention of Multidrug Resistance. Multi Drug Res. Cancer 12 (1–3):171–212. doi: 10.1007/978-94-011-0826-3_9
Fuentefria, A. M., Pippi, B., Dalla Lana, D. F., Donato, K. K., de Andrade, S. F. (2018). Antifungals Discovery: An Insight Into New Strategies to Combat Antifungal Resistance. Lett. Appl. Microbiol. 66 (1), 2–13. doi: 10.1111/lam.12820
Garcia-Effron, G., Katiyar, S.K., Park, S., Edlind, T. D., Perlin, D. S. (2008). A Naturally Occurring Proline-to-Alanine Amino Acid Change in Fks1p in Candida Parapsilosis, Candida Orthopsilosis, and Candida Metapsilosis Accounts for Reduced Echinocandin Susceptibility. Antimicrob. Agents Chemother. 52, 2305–2312. doi: 10.1128/AAC.00262-08
Giordani, R., Regli, P., Kaloustian, J., Mikail, C., Abou, L., Portugal, H. (2004). Antifungal Effect of Various Essential Oils Against Candida Albicans. Potentiation of Antifungal Action of Amphotericin B by Essential Oil From Thymus Vulgaris. Phytother. Res. 18 (12), 990–995. doi: 10.1002/ptr.1594
Giordani, R., Regli, P., Kaloustian, J., Portugal, H. (2006). Potentiation of Antifungal Activity of Amphotericin B by Essential Oil From Cinnamomum Cassia. Phytother. Res. 20 (1), 58–61. doi: 10.1002/ptr.1803
Hagen, S., Marx, F., Ram, A. F., Meyer, V. (2007). The Antifungal Protein AFP From Aspergillus Giganteus Inhibits Chitin Synthesis in Sensitive Fungi. Appl. Environ. Microbiol. 73 (7), 2128–2134. doi: 10.1128/AEM.02497-06
Hull, C. M., Bader, O., Parker, J. E., Weig, M., Gross, U., Warrilow, A. G., et al. (2012). Two Clinical Isolates of Candida Glabrata Exhibiting Reduced Sensitivity to Amphotericin B Both Harbor Mutations in ERG2. Antimicrob. Agents Chemother 56 (12), 6417–6421. doi: 10.1128/AAC.01145-12
Johann, S., Sá, N. P., Lima, L. A., Cisalpino, P. S., Cota, B. B., Alves, T., et al. (2010). Antifungal Activity of Schinol and a New Biphenyl Compound Isolated From Schinus Terebinthifolius Against the Pathogenic Fungus Paracoccidioides Brasiliensis. Ann. Clin. Microbiol. Antimicrob. 9 (1), 1–6. doi: 10.1186/1476-0711-9-30
Kavitha, D., Nandhini, G., Padma, P. R. (2013). In Vitro Evaluation of Isatin From Couroupita Guianensis Aubl Against the Clinical Isolates of Bacteria and Fungi. Int. J. Pharm. Sci. Rev. Res. 23 (2), 228–230.
Kavitha, D., Nirmaladevi, R. (2009). Evaluation of Aristolochia Bracteolata Leaf Extracts for its Antibacterial and Antifungal Activity. Adv. Plant Sci. 22 (2), 407–409.
Kavitha, D., Parvatham, R., Padma, P. R. (2014). Assessment of Trianthema Portulacastrum for its Antimicrobial Potential and Investigation of Their Phytochemicals Using Hptlc, GC-MS, and IR. Int. J. Pharm. Pharm. Sci. 6 (1), 675.
Keniya, M. V., Fleischer, E., Klinger, A., Cannon, R. D., Monk, B. C. (2015). Inhibitors of the Candida Albicans Major Facilitator Superfamily Transporter Mdr1p Responsible for Fluconazole Resistance. PLoS One 10 (5), e0126350. doi: 10.1371/journal.pone.0126350
Khanzada, B., Akhtar, N., Okla, M. K., Alamri, S. A., Al-Hashimi, A., Baig, M. W., et al. (2021). Profiling of Antifungal Activities and In Silico Studies of Natural Polyphenols From Some Plants. Molecules 26 (23), 7164. doi: 10.3390/molecules26237164
Kim, J. H., Campbell, B. C., Chan, K. L., Mahoney, N., Haff, R. P. (2013a). Synergism of Antifungal Activity Between Mitochondrial Respiration Inhibitors and Kojic Acid. Molecules 18 (2), 1564–1581. doi: 10.3390/molecules18021564
Kim, J. H., Campbell, B. C., Mahoney, N., Chan, K. L., Molyneux, R. J., Balajee, A. (2010). Augmenting the Activity of Antifungal Agents Against Aspergilli Using Structural Analogues of Benzoic Acid as Chemosensitizing Agents. Fungal Biol. 114 (10), 817–824. doi: 10.1016/j.funbio.2010.07.008
Kim, J. H., Chan, K. L., Mahoney, N., Campbell, B. C. (2011). Antifungal Activity of Redox-Active Benzaldehydes That Target Cellular Antioxidation. Ann. Clin. Microbiol. Antimicrob., 10 (1), 1-16. doi: 10.1111/lam.12820
Kim, J. H., Chan, K. L., Cheng, L. W. (2016). Chemobiological Approaches for Enhancing the Efficacy of Antifungal Intervention. J. Biotechnol. Biomat. 6:8 (Suppl):24.
Kim, J. H., Chan, K. L., Cheng, L. W. (2018). Octyl Gallate as an Intervention Catalyst to Augment Antifungal Efficacy of Caspofungin. J. 1 (1), 19–28. doi: 10.3390/j1010004
Kim, J. H., Chan, K. L., Cheng, L. W., Tell, L. A., Byrne, B. A., Clothier, K., et al. (2019). High Efficiency Drug Repurposing Design for New Antifungal Agents. Methods Protoc. 2 (2), 31. doi: 10.3390/mps2020031
Kim, J. H., Chan, K. L., Faria, N. C., Martins, M. D., Campbell, B. C. (2012). Targeting the Oxidative Stress Response System of Fungi With Redox-Potent Chemosensitizing Agents. Front. Microbiol. 3, 88. doi: 10.3389/fmicb.2012.00088
Kim, J. H., Haff, R. P., Faria, N. C., Martins, M. D., Chan, K. L., Campbell, B. C. (2013b). Targeting the Mitochondrial Respiratory Chain of Cryptococcus Through Antifungal Chemosensitization: A Model for Control of non-Fermentative Pathogens. Molecules 18 (8), 8873–8894. doi: 10.3390/molecules18088873
Kim, J. H., Mahoney, N., Chan, K. L., Molyneux, R. J., May, G. S., Campbell, B. C. (2008). Chemosensitization of Fungal Pathogens to Antimicrobial Agents Using Benzo Analogs. FEMS Microbiol. Lett. 281 (1), 64–72. doi: 10.1111/j.1574-6968.2008.01072.x
Krishnamurthy, R., Padma, P., Dhandapani, K. (2020). Antagonistic Efficiency of Aspergillus Giganteus as a Biocontrol Agent Against Aflatoxigenic Aspergillus Flavus Infecting Maize. J. Pure Appl. Microbiol. 14 (1), 527–539. doi: 10.22207/JPAM.14.1.55
Kubo, I., Himejima, M. (1991). Anethole, A Synergist of Polygodial Against Filamentous Microorganisms. J. Agric. Food Chem. 39 (12), 2290–2292. doi: 10.1021/jf00012a040
Lass-Flörl, C. (2011). Triazole Antifungal Agents in Invasive Fungal Infections. Drugs 71 (18), 2405–2419. doi: 10.2165/11596540-000000000-00000
Lee, S. H., Kim, C. J. (1999). Selective Combination Effect of Anethole to the Antifungal Activities of Miconazole and Amphotericin B. Yakhak Hoeji 43 (2), 228–232.
Mayer, F. L., Wilson, D., Hube, B. (2013). Candida Albicans Pathogenicity Mechanisms. Virulence 4 (2), 119–128. doi: 10.4161/viru.22913
McCarthy, M. W., Kontoyiannis, D. P., Cornely, O. A., Perfect, J. R., Walsh, T. J. (2017). Novel Agents and Drug Targets to Meet the Challenges of Resistant Fungi. J. Infect. Dis. 216 (suppl_3), S474–S483. doi: 10.1093/infdis/jix130
Meneau, I., Coste, A. T., Sanglard, D. (2016). Identification of Aspergillus Fumigatus Multidrug Transporter Genes and Their Potential Involvement in Antifungal Resistance. Sabouraudia Chapter 14, 54 (6), 616–627. doi: 10.1093/mmy/myw005
Mesa-Arango, A. C., Rueda, C., Román, E., Quintin, J., Terrón, M. C., Luque, D., et al. (2016). Cell Wall Changes in Amphotericin B-Resistant Strains From Candida Tropicalis and Relationship With the Immune Responses Elicited by the Host. Antimicrob. Agents Chemother. 60 (4), 2326–2335. doi: 10.1128/AAC.02681-15
Moreno, A. B., Del Pozo, A. M., Borja, M., Segundo, B. S. (2003). Activity of the Antifungal Protein From Aspergillus Giganteus Against Botrytis Cinerea. Phytopathology 93 (11), 1344–1353. doi: 10.1094/PHYTO.2003.93.11.1344
Moreno, A. B., Peñas, G., Rufat, M., Bravo, J. M., Estopà, M., Messeguer, J., et al. (2005). Pathogen-Induced Production of the Antifungal Afp Protein From Aspergillus Giganteus Confers Resistance to the Blast Fungus Magnaporthe Grisea in Transgenic Rice. Mol. Plant-Microbe Interact. 18 (9), 960–972. doi: 10.1094/MPMI-18-0960
Moreno, A. B., Martínez Del Pozo, A., San Segundo, B., Martínez Del Pozo, Á., San Segundo, B.. (2006). Antifungal Mechanism of the Aspergillus Giganteus AFP Against the Rice Blast Fungus Magnaporthe Grisea. Appl. Microbiol. Biotechnol. 72, 883-895. doi: 10.1007/s00253-006-0362-1
Murciano, C., Moyes, D. L., Runglall, M., Tobouti, P., Islam, A., Hoyer, L. L., et al. (2012). Evaluation of the Role of Candida Albicans Agglutinin-Like Sequence (Als) Proteins in Human Oral Epithelial Cell Interactions. PLoS One 7 (3), e33362. doi: 10.1371/journal.pone.0033362
Nakamura, I., Ohsumi, K., Takeda, S., Katsumata, K., Matsumoto, S., Akamatsu, S., et al. (2019). ASP2397 is a Novel Natural Compound That Exhibits Rapid and Potent Fungicidal Activity Against Aspergillus Species Through a Specific Transporter. Antimicrob. Agents Chemother. 63 (10), e02689–e02618. doi: 10.1128/AAC.02689-18
Narvaez, I., Khayreddine, T., Pliego, C., Cerezo, S., Jiménez-Díaz, R. M., Trapero-Casas, J. L., et al. (2018). Usage of the Heterologous Expression of the Antimicrobial Gene Afp From Aspergillus Giganteus for Increasing Fungal Resistance in Olive. Front. Plant Sci. 9, 680. doi: 10.3389/fpls.2018.00680
Ogita, A., Fujita, K. I., Tanaka, T. (2012). Enhancing Effects on Vacuole-Targeting Fungicidal Activity of Amphotericin B. Front. Microbiol. 3, 100. doi: 10.3389/fmicb.2012.00100
Panda, D., Rathinasamy, K., Santra, M. K., Wilson, L. (2005). Kinetic Suppression of Microtubule Dynamic Instability by Griseofulvin: Implications for its Possible Use in the Treatment of Cancer. Proc. Natl. Acad. Sci. 102 (28), 9878–9883. doi: 10.1073/pnas.0501821102
Papon, N., Noël, T., Florent, M., Gibot-Leclerc, S., Jean, D., Chastin, C., et al. (2007). Molecular Mechanism of Flucytosine Resistance in Candida Lusitaniae: Contribution of the FCY2, FCY1, and FUR1 Genes to 5-Fluorouracil and Fluconazole Cross-Resistance. Antimicrob. Agents Chemother. 51 (1), 369–371. doi: 10.1128/AAC.00824-06
Paul, S., Diekema, D., Moye-Rowley, W. S. (2013). Contributions of Aspergillus Fumigatus ATP-Binding Cassette Transporter Proteins to Drug Resistance and Virulence. Eukaryot Cell 12 (12), 1619–1628. doi: 10.1128/EC.00171-13
Pedrolli, D. B., Carmona, E. C. (2010). Purification and Characterization of the Exopolygalacturonase Produced by Aspergillus Giganteus in Submerged Cultures. J. Ind. Microbiol. Biotechnol. 37 (6), 567–573. doi: 10.1007/s10295-010-0702-0
Pfaller, M. A. (2012). Antifungal Drug Resistance: Mechanisms, Epidemiology, and Consequences for Treatment. Am. J. Med. 125 (1), S3–13. doi: 10.1016/j.amjmed.2011.11.001
Posch, W., Blatzer, M., Wilflingseder, D., Lass-Flörl, C. (2018). Aspergillus Terreus: Novel Lessons Learned on Amphotericin B Resistance. Med. Mycol. 56 (suppl_1), S73–S82. doi: 10.1093/mmy/myx119
Pozo, A. M., Lacadena, V., Manchenão, J. M., Olmo, N., Onãaderra, M., Gavilanes, J. G. (2002). The Antifungal Protein AFP of Aspergillus giganteusIs an Oligonucleotide/Oligosaccharide Binding (Ob) Fold-Containing Protein That Produces Condensation of DNA. J. Biol. Chem. 277 (48), 46179–46183. doi: 10.1074/jbc.M207472200
Prasad, R., Rawal, M. K. (2014). Efflux Pump Proteins in Antifungal Resistance. Front. Pharmacol. 5, 202. doi: 10.3389/fphar.2014.00202
Ramya, R., Kavitha, D. (2021). Antagonistic Mechanism of Anti-Fungal Compounds From Aspergillus Giganteus on Human Fungal Pathogens. Int. J. Pharm. Sci. Res. 12 (12), 6393–02. doi: 10.13040/IJPSR.0975-8232
Rao, A., Zhang, Y., Muend, S., Rao, R. (2010). Mechanism of Antifungal Activity of Terpenoid Phenols Resembles Calcium Stress and Inhibition of the TOR Pathway. Antimicrob. Agents Chemother. 54 (12), 5062–5069. doi: 10.1128/AAC.01050-10
Ravindhiran, R., Krishnamurthy, R., Sivarajan, K., Sekar, J., Chidambaram, K., Dhandapani, K. (2021). Targeted Action and Molecular Interactions of Sarcin and Thionin With Aspergillosis Causing Aspergillus Fumigatus. Preprints 1–20. doi: 10.20944/preprints202111.0398.v1
Revie, N. M., Iyer, K. R., Robbins, N., Cowen, L. E. (2018). Antifungal Drug Resistance: Evolution, Mechanisms and Impact. Curr. Opin. Microbiol. 45, 70–76. doi: 10.1016/j.mib.2018.02.005
Rodrigues, C. F., Rodrigues, M. E., Henriques, M. (2018). Susceptibility of Candida Glabrata Biofilms to Echinocandins: Alterations in the Matrix Composition. Biofouling 34 (5), 569–578. doi: 10.1080/08927014.2018.1472244
Roemer, T., Krysan, D. J. (2014). Antifungal Drug Development: Challenges, Unmet Clinical Needs, and New Approaches. Cold Spring Harbor Perspect. Med. 4 (5), a019703. doi: 10.1101/cshperspect.a019703
Rosato, A., Vitali, C., Piarulli, M., Mazzotta, M., Argentieri, M. P., Mallamaci, R. (2009). In Vitro Synergic Efficacy of the Combination of Nystatin With the Essential Oils of Origanum Vulgare and Pelargonium Graveolens Against Some Candida Species. Phytomedicine 16 (10), 972–975. doi: 10.1016/j.phymed.2009.02.011
Sanglard, D., White, T. C. (2006). Molecular Principles of Antifungal Drug Resistance. Mol. Principle Fungal Pathogen. 197–212. doi: 10.1128/9781555815776.ch14
Sardi, J. D., Pitangui, N. D., Rodríguez-Arellanes, G., Taylor, M. L., Fusco-Almeida, A. M., Mendes-Giannini, M. J. (2014). Highlights in Pathogenic Fungal Biofilms. Rev. Iberoamericana Micologia 31 (1), 22–29. doi: 10.1016/j.riam.2013.09.014
Scorzoni, L., de Paula e Silva, A. C., Marcos, C. M., Assato, P. A., de Melo, W. C., de Oliveira, H. C., et al. (2017). Antifungal Therapy: New Advances in the Understanding and Treatment of Mycosis. Front. Microbiol. 36. doi: 10.3389/fmicb.2017.00036
Semighini, C. P., Marins, M., Goldman, M. H., Goldman, G. H. (2002). Quantitative Analysis of the Relative Transcript Levels of ABC Transporter Atr Genes in Aspergillus Nidulans by Real-Time Reverse Transcription-PCR Assay. Appl. Environ. Microbiol. 68 (3), 1351–1357. doi: 10.1128/AEM.68.3.1351-1357.2002
Shin, S. (2003). Anti-Aspergillus Activities of Plant Essential Oils and Their Combination Effects With Ketoconazole or Amphotericin B. Arch. Pharmacal Res. 26 (5), 389–393. doi: 10.1007/BF02976696
Shin, S., Kang, C. A. (2003). Antifungal Activity of the Essential Oil of Agastache Rugosa Kuntze and its Synergism With Ketoconazole. Lett. Appl. Microbiol. 36 (2), 111–115. doi: 10.1046/j.1472-765X.2003.01271.x
Shreaz, S., Bhatia, R., Khan, N., Ahmad, S. I., Muralidhar, S., Basir, S. F., et al. (2011). Interesting Anticandidal Effects of Anisic Aldehydes on Growth and Proton-Pumping-ATPase-Targeted Activity. Microbial. Pathogen. 51 (4), 277–284. doi: 10.1016/j.micpath.2011.05.005
Shreaz, S., Bhatia, R., Khan, N., Muralidhar, S., Manzoor, N., Khan, L. A. (2013). Influences of Cinnamic Aldehydes on H+ Extrusion Activity and Ultrastructure of Candida. J. Med. Microbiol. 62 (2), 232–240. doi: 10.1099/jmm.0.036145-0
Silva, F. D., Landell, M. F., Paulino, G. V., Coutinho, H. D., Albuquerque, U. P. (2020). Antifungal Activity of Selected Plant Extracts Based on an Ethnodirected Study. Acta Botanica Brasilica 34, 442–448. doi: 10.1590/0102-33062020abb0003
Silva, A. P., Miranda, I. M., Guida, A., Synnott, J., Rocha, R., Silva, R., et al. (2011). Transcriptional Profiling of Azole-Resistant Candida Parapsilosis Strains. Antimicrobial Agents Chemotherapy 55 (7), 3546–3556. doi: 10.1128/AAC.01127-10
Simonetti, G., Brasili, E., Pasqua, G. (2020). Antifungal Activity of Phenolic and Polyphenolic Compounds From Different Matrices of Vitis Vinifera L. Against Human Pathogens. Molecules 25 (16), 3748. doi: 10.3390/molecules25163748
Theis, T., Wedde, M., Meyer, V., Stahl, U. (2003). The Antifungal Protein From Aspergillus Giganteus Causes Membrane Permeabilization. Antimicrobial Agents Chemotherapy 47 (2), 588–593. doi: 10.1128/AAC.47.2.588-593.2003
Tobin, M. B., Peery, R. B., Skatrud, P. L. (1997). Genes Encoding Multiple Drug Resistance-Like Proteins in Aspergillus Fumigatus and Aspergillus Flavus. Gene 200 (1-2), 11–23. doi: 10.1016/S0378-1119(97)00281-3
Vandeputte, P., Ferrari, S., Coste, A. T. (2012). Antifungal Resistance and New Strategies to Control Fungal Infections. Int. J. Microbiol. 1–26. doi: 10.1155/2012/713687
Keywords: chemosensitization, antifungal drugs, natural compounds, augmentation, fungicidal activity, Aspergillus giganteus, AFP
Citation: Dhandapani K, Sivarajan K, Ravindhiran R and Sekar JN (2022) Fungal Infections as an Uprising Threat to Human Health: Chemosensitization of Fungal Pathogens With AFP From Aspergillus giganteus. Front. Cell. Infect. Microbiol. 12:887971. doi: 10.3389/fcimb.2022.887971
Received: 02 March 2022; Accepted: 19 April 2022;
Published: 25 May 2022.
Edited by:
Olihile M. Sebolai, University of the Free State, South AfricaReviewed by:
Luana P. Borba-Santos, Federal University of Rio de Janeiro, BrazilOlawunmi Adepemi Adetoro-Ogundeji, University of the Free State, South Africa
Copyright © 2022 Dhandapani, Sivarajan, Ravindhiran and Sekar. This is an open-access article distributed under the terms of the Creative Commons Attribution License (CC BY). The use, distribution or reproduction in other forums is permitted, provided the original author(s) and the copyright owner(s) are credited and that the original publication in this journal is cited, in accordance with accepted academic practice. No use, distribution or reproduction is permitted which does not comply with these terms.
*Correspondence: Kavitha Dhandapani, a2F2aXRoYV9iaW9AYXZpbnV0eS5hYy5pbg==