- 1Department of Biological Sciences, Mississippi State University, Starkville, MS, United States
- 2Department of Comparative Biomedical Sciences, College of Veterinary Medicine, Mississippi State University, Starkville, MS, United States
- 3Department of Population and Pathobiology, College of Veterinary Medicine, Mississippi State University, Starkville, MS, United States
- 4Department of Infectious Diseases, St. Jude Children’s Research Hospital, Memphis, TN, United States
Apoptosis of cells at the site of infection is a requirement for shutdown of inflammatory signaling, avoiding tissue damage, and preventing progression of sepsis. Puma+/+ and Puma-/- mice were challenged with TIGR4 strain pneumococcus and cytokines were quantitated from lungs and blood using a magnetic bead panel analysis. Puma-/- mice exhibited higher lung and blood cytokine levels of several major inflammatory cytokines, including IL-6, G-CSF, RANTES, IL-12, IFN-ϒ, and IP-10. Puma-/- mice were more susceptible to bacterial dissemination and exhibited more weight loss than their wild-type counterparts. RNA sequencing analysis of whole pulmonary tissue revealed Puma-dependent regulation of Nrxn2, Adam19, and Eln. Enrichment of gene ontology groups differentially expressed in Puma-/- tissues were strongly correlated to IFN-β and -ϒ signaling. Here, we demonstrate for the first time the role of Puma in prohibition of the cytokine storm during bacterial pneumonia. These findings further suggest a role for targeting immunomodulation of IFN signaling during pulmonary inflammation. Additionally, our findings suggest previously undemonstrated roles for genes encoding regulatory and binding proteins during the early phase of the innate immune response of pneumococcal pneumonia.
Introduction
During pneumococcal infection, interaction of bacteria with the host epithelium initiates recruitment of neutrophils (Yamamoto et al., 2014). Following recruitment, activated neutrophils are capable of inducing programmed cell death in epithelial cells (Jia et al., 2014). Pneumococcus can induce both direct and indirect apoptosis in neutrophils (Zysk et al., 2000; Engelich et al., 2001), epithelial cells (Schmeck et al., 2004), and macrophages (Marriott et al., 2004), observations that have been mechanistically tied to PLY, cell wall components, and H2O2 (Marriott and Dockrell, 2006; Rai et al., 2015). In murine pneumococcal pneumonia, macrophage apoptosis has been shown to be crucial for resolution of pulmonary inflammation (Marriott et al., 2006). Induction of apoptosis, depending on cell type, can have detrimental or beneficial results, but coordinated and organized cell death is essential during infection resolution. Apoptosis modulates innate immune signaling, cell fate of responding phagocytes, promotes resolution of inflammation, and limits tissue damage (Haslett, 1999; Kennedy and Deleo, 2009; Esmann et al., 2010; Fox et al., 2010; Bordon et al., 2013; Greenlee-Wacker, 2016). The resultant effects of pneumococcal injury and apoptosis within the tissues strongly correlates with wound healing and repair (Matute-bello and Martin, 2003; Geiser et al., 2004; Wu and Chen, 2014; Domon et al., 2016; Holzmann et al., 2016). Numerous experimental in vitro, in vivo, and clinical studies have reported several pro-inflammatory cytokines up-regulated during the early phase of pneumococcal pneumonia, including IL-6, G-CSF, TNF-α, IL-1β, IL-12, IFN-ϒ, and IP-10 (Van Der Poll et al., 1997; Ethuin et al., 2004; Yamamoto et al., 2004; Palaniappan et al., 2006; Sun et al., 2007; Endeman et al., 2011; Yamada et al., 2011; Gomez et al., 2015; Skovbjerg et al., 2017; Loughran et al., 2018). However, in the last two decades, over-expression of IL-12, IFN-ϒ, and IP-10 has emerged as a highly potent pro-inflammatory, neutrophil-mediated signaling axis in murine models of pneumococcal pneumonia (Yamamoto et al., 2004; Sun et al., 2007; Seyoum and Yano, 2011; Yamada et al., 2011; Gomez et al., 2015).
Neutrophils are the primary effector cells of the host innate response to pneumococcal pneumonia (Craig et al., 2009). In pneumococcal pneumonia patients, the activation of neutrophils after extravasation into the lungs delays the rate of spontaneous apoptosis (Droemann et al., 2000). However, apoptosis of neutrophils is an essential feature of pneumococcal infection, as apoptotic neutrophils must be cleared from the site upon performing their effector functions (Koedel et al., 2009). Pneumococcus can induce apoptosis in neutrophils in an H2O2-dependent fashion and accelerate apoptosis by PLY membrane perforation at relatively lower multiplicities of infection (MOI) (Zysk et al., 2000).
PUMA (p53-up-regulated modulator of apoptosis), a pro-apoptotic member of the BH3-only family of the BCL-2 superfamily of eukaryotic proteins, is one of the primary effector proteins that promotes apoptosis (Nakano and Vousden, 2001; Vousden, 2005). In a murine model of pneumococcal infection, neutrophil-mediated protection against lethal dissemination of pneumococci was shown to be Puma-dependent apoptosis (Garrison et al., 2010). However, it is unclear which steps of apoptosis signaling pathways are involved in the early phase interactions between neutrophils and pneumococcus. Subsequently, the effects of Puma-dependent apoptosis on global gene expression and innate immune signaling in the lung during the early phase of pneumococcal pneumonia have not yet been investigated.
In this study, we attempted to characterize Puma-dependent responses in the lung during the early phase of pneumococcal pneumonia. Given the clinical relevance of targeting the cytokine response during the early phase of the innate immune response during acute community acquired pneumonia (Woods and José, 2017), we sought to quantitate differences in inflammatory cytokines in the lungs of Puma+/+ and Puma-/- mice. Finally, to further understand what Puma-dependent transcriptional responses may be induced during early pneumococcal infection, we attempted to characterize changes in the lung transcriptome.
Materials and Methods
Bacterial Culture
S. pneumoniae strain TIGR4 (Tettelin et al., 2001) was cultured overnight on tryptic soy agar (TSA) plates supplemented with 5% sheep’s blood (EMD Millipore) and subsequently inoculated into 10 mL pre-warmed C+Y medium (Lacks and Hotchkiss, 1960). TIGR4 was cultured to mid-log, centrifuged for 10 minutes at 15,871 x g and 4°C, and resuspended in 1 mL of C+Y with 20% glycerol (Thermo Fisher Scientific). Resuspended TIGR4 was aliquoted by volumes of 100 μL and stored at -80°C until used in animal challenges. Before challenging animals, one aliquot TIGR4 was thawed on ice, serially diluted in 1X PBS (Thermo Fisher Scientific), cultured on blood agar overnight, and quantitated.
Statement of Ethics, Animal Handling, and Challenges
All experiments involving animals were planned and conducted in accordance with guidelines of the MSU Institutional Animal Care and Use Committee (Protocol number 19-537). The committee-approved procedures performed in the study presented here. Puma heterozygote mice were crossed and resulting C57BL/6 or Puma-/- (The Jackson Laboratory, C57BL/6-Bbc3tm1Ast/J, RRID: IMSR_JAX: 011067) mice from generations F5 and F6 were used in challenges. Animals were maintained at Biosafety Level 1 and 2 facilities in the Mississippi State University Animal Facility at Harned Hall. Animals were housed among litter mates until isolated overnight for challenge. On the days of challenges, TIGR4 aliquots were removed from -80°C, thawed on ice, and resuspended in 900 μL 1X PBS, then further diluted to a working dilution of 2 x 106 CFU/mL. Fifty microliters of working dilution TIGR4 or 1X PBS were administered intranasally. Twenty-four hours post-infection (p.i.), signs of disease and body weight were recorded immediately before euthanasia. To determine CFU within tissues, nasopharyngeal tissues were aseptically flushed with filter-sterilized 1X PBS and collected in-tube, pulmonary tissues mechanically homogenized in 1 mL 1X PBS using an electric tissue homogenizer, and blood was drawn retroorbitally. Crude samples (bood or homogenized respiratory tissues) were appropriately diluted to determine CFU titres onto TSA supplemented with 5% sheep’s blood and incubated overnight at 37°C under CO2. Tracheae were surgically transversely severed and whole pulmonary tissues were prepared as follows: transferred to 1X PBS to be homogenized for cytokine analysis; perfused with and homogenized in Trizol (Thermo Fisher Scientific) reagent for RNA extraction and library preparation; perfused with and transferred to 10% neutral buffered formalin (Thermo Fisher Scientific) for paraffin embedding.
Histopathological Analysis
Tissues were collected, fixed in 10% neutral buffered formalin, routinely processed, embedded in paraffin, sectioned at 5 μm and stained with hematoxylin and eosin (Cardiff et al., 2014). Lung sections were scored for inflammation based on the following parameters: patchy interstitial hypercellularity due to increased mononuclear cells (0-absent; 1-present), perivascular suppurative inflammation with edema (0-absent; 1-present but focal (3 or fewer vessels affected); 2-present – extensive (more than 3 vessels affected), vasculitis (0-absent; 1-present) and increased alveolar macrophages (0-absent; 1-present). All scoring was done blinded to genotype (Supplementary Table S1).
Cytokine Analysis
To characterize the pneumococcal-induced cytokine profile 24 hours p.i., pulmonary tissues were harvested as described above and blood was retroorbitally drawn. Crude pulmonary tissue homogenates prepared as described above were centrifuged for 10 minutes at 9,400 x g and 4°C. Supernatants were transferred to clean tubes and stored at -80°C for analysis. Tissue homogenate pellets were washed once with 1X PBS and centrifuged for 10 minutes at 21,130 x g and 4°C. Whole tissue lysates were centrifuged for 4 minutes at 690 x g. Whole tissue lysates were transferred to clean tubes and stored at -80°C for analysis. Blood samples were centrifuged for 3 minutes at 1,550 x g. Sera were transferred to clean tubes and store at -80°C for analysis. Concentrations of granulocyte-colony stimulating factor (G-CSF), granulocyte macrophage-colony stimulating factor (GM-CSF), interleukin-1 alpha (IL-1α), IL-1β, IL-2, IL-4, IL-5, IL-6, IL-9, IL-10, IL-12p40, IL-12p70, IL-13, IL-15, IL-17, interferon-inducible protein-10 (IP-10), keratinocyte-derived chemokine (KC), monocyte chemotactic protein-1 alpha (MCP-1α), macrophage inflammatory protein-1alpha (MIP-1α), MIP-1β, MIP-2, regulated on activation normal T cells expressed and secreted (RANTES), tumor necrosis factor-alpha (TNF-α), and interferon-gamma (IFN-ϒ) were determined using the MILLIPLEX MAP Mouse Cytokine/Chemokine Magnetic Bead Panel (EMD Millipore # MCYTOMAG-70K) according to the manufacturer’s instructions.
RNA Isolation and Library Preparation
To characterize the post-pharyngeal pulmonary transcriptome 24 hour p.i., tissues were mechanically homogenized using an electrical tissue homogenizer in 5 mL Trizol reagent. From crude pulmonary homogenates, 1mL was transferred to a clean tube and incubated at room temperature for 5 minutes. The remaining crude Trizol homogenate was stored at -80°C for curation and further analysis. Two-hundred microliters chloroform (Thermo Fisher Scientific) were added, shaken, and incubated at room temperature for 2 minutes. Samples were centrifuged 20 minutes at 15,900 x g and 4°C. RNA was purified using the RNeasy kit (Qiagen) according to the vendor’s instructions. RNA purity was determined using a Qubit 2.0 fluorometer and Qubit RNA BR and dsDNA HS assay kits (Thermo Fisher Scientific). Ten micrograms total RNA served as starting material with the NEBNext Poly(A) mRNA Magnetic Isolation Module (New England Biolabs), and cDNA libraries were generated using the NEBNext Ultra RNA Library Prep Kit for Illumina (New England Biolabs). Samples of cDNA from all mice were normalized for concentration, pooled, and sequenced on their own lane of a NOVASeq 6000 sequencer at 100 base-pair paired-end reads and yielded at least 10 million reads.
Demultiplexing, Alignment, Normalization, & Bioinformatics Analysis
RNAseq reads were trimmed with BBDuk (part of BBMap version 38.5) for both quality (minimum quality set to 20 with: “qtrim=rl trimq=20”) and adapters trimming. Transcript abundances for each sample were computed with Kallisto version 0.45.0 using default parameters (kallisto quant –bias) for pseudoalignment against the Mus musculus annotated transcripts from the Ensembl database (GRCm39, release 103) (Bray et al., 2016; Yates et al., 2020). Differentially expressed genes were detected using the DESeq2 package (Love et al., 2014). Clustering of samples based on gene expression patterns was performed with the vsn and pheatmap packages and revealed that 3 samples of infected mice clustered with mock-infected mice. Blood CFU data confirmed that the infection status of these three mice was questionable, and they were removed from the analysis (Table 1). Gene Ontology enrichment was performed using the topGO package in R, using the ks/classic statistic/algorithm combination.
Results
PUMA Limits Pneumococcal Inflammation & Sepsis
To investigate the role of Puma during early pneumococcal infection, Puma+/+ (WT) and Puma-/- (KO) mice were intranasally infected with 2 x 105 total CFU TIGR4. At 24 hours p.i., TIGR4 CFU were quantitated from nasal lavage fluid (NLF), pulmonary homogenates, and blood. While numbers of TIGR4 recovered from NLF were similar among all mice, Puma-/- mice demonstrated significantly higher bacterial counts in the lungs and bloodstream at 24 hours p.i. compared to Puma+/+ mice (Figure 1A). In the same timeframe, only 30% of Puma+/+ mice exhibited TIGR4 disseminated to blood, compared to 100% of Puma-/- mice. Puma-/- mice lost significantly more weight (mean of 1.42 g) than Puma+/+ mice (mean of 0.2 g) over the 24-hour course of infection (Figure 1B) indicating excessive disease burden. These data suggest that pneumococcus is more invasive in Puma-/- mice.
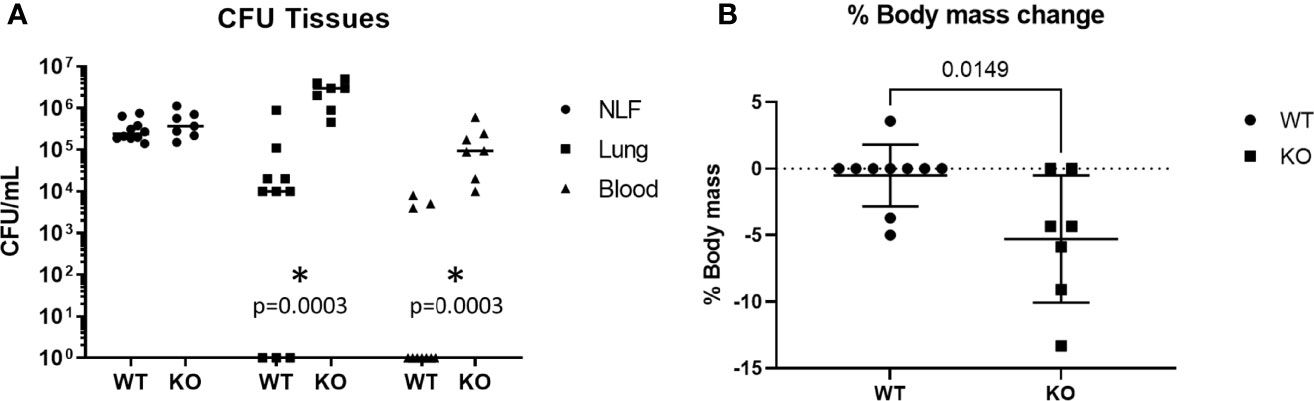
Figure 1 Puma attenuates sepsis and morbidity. (A) Mice were intranasally challenged with 2 x 105 total CFU. Pneumonia was allowed to develop for 24 h, at which time mice were euthanized and tissues were sampled. For each group, WT (n = 10), KO (n = 7). Horizontal lines represent means. A Mann-Whitney U test was performed, α = 0.05. (B) Mice were weighed immediately before challenge and before sacrifice. A two-tailed, unpaired T-test was performed, α = 0.05. *p = 0.0003.
Histological analysis showed that peribronchiolar and perivascular inflammation was noted in both Puma+/+ and Puma-/- lungs (Figure 2). In the Puma+/+ group, one of five mice had no histologic changes (Figure 2A), while four of five had patchy areas of increased cellularity of the interstitium with three of five classified as mild (Figure 2B). No evidence of vasculitis was detected in Puma+/+ lungs. In the Puma-/- group, one of five mice had increased alveolar macrophages as the only histologic change (Figure 2D), while four of five had patchy areas of increased cellularity of the interstitium with two of five lungs scored moderate (Figure 2C). One Puma-/- mouse exhibited evidence of vasculitis (Figure 2E).
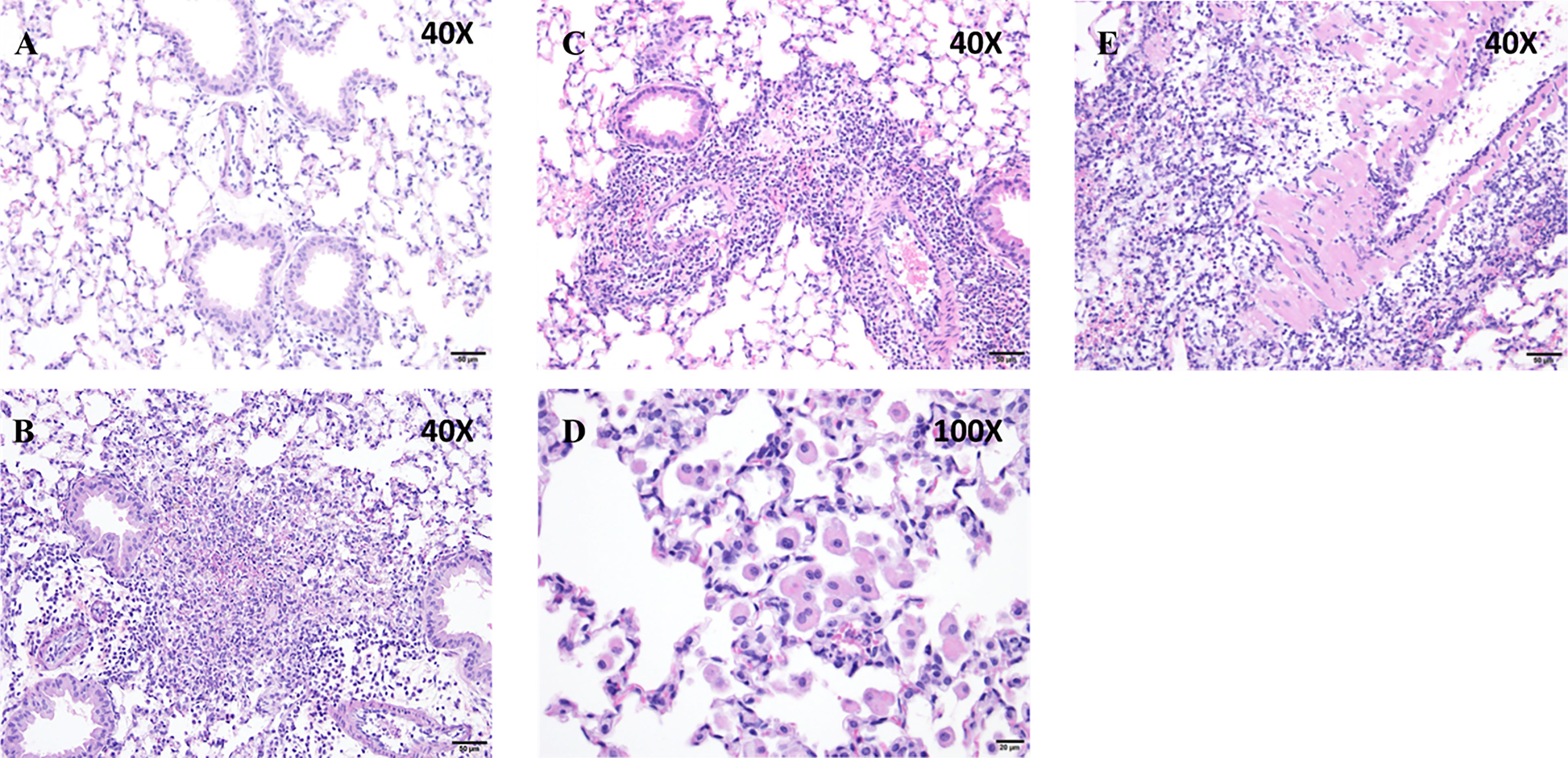
Figure 2 Puma protects against tissue inflammation. (A, B) Puma+/+ mice exhibited mild peribronchioloar inflammation with edema and neutrophils and macrophages. (C–E) Puma-/- mice exhibited moderate peribronchiolar/perivascular inflammation, abundant neutrophils, macrophages, vasculitis (eosinophilic material, vascular thickening). Scale bars: A–C, E, 50 μm; D, 20 μm. Images shown are representative of the pathology described in the results from 3 sections each from 5 mice.
Puma Dampens Cytokine Signaling and Prevents Onset of the Cytokine Storm
To characterize the effect of Puma on the cytokine response elicited by pneumococcus, lung and blood cytokine levels from Puma+/+ and Puma-/- mice were quantitated 24 hours p.i. (Table 2). The cytokines IL-1β, -6, -12, -13, IP-10, MCP-1, MIP-1α, -1β, TNF-α, and IFN-ϒ were significantly over-expressed in Puma-/- lungs and blood compared to those of Puma+/+. Similarly, in Puma-/-, differences in G-CSF, GM-CSF, IL-1α, -17, KC, MIP-2, and RANTES were significant only in lung, while IL-9, -10, and -12(p40) were only significantly different in blood. Differences in IL-2, -4, -5, and -15 were observed in Puma+/+ and Puma-/- mouse lungs and blood, all concentrations being higher in Puma-/- tissues, but none were statistically significant (Figure 3).
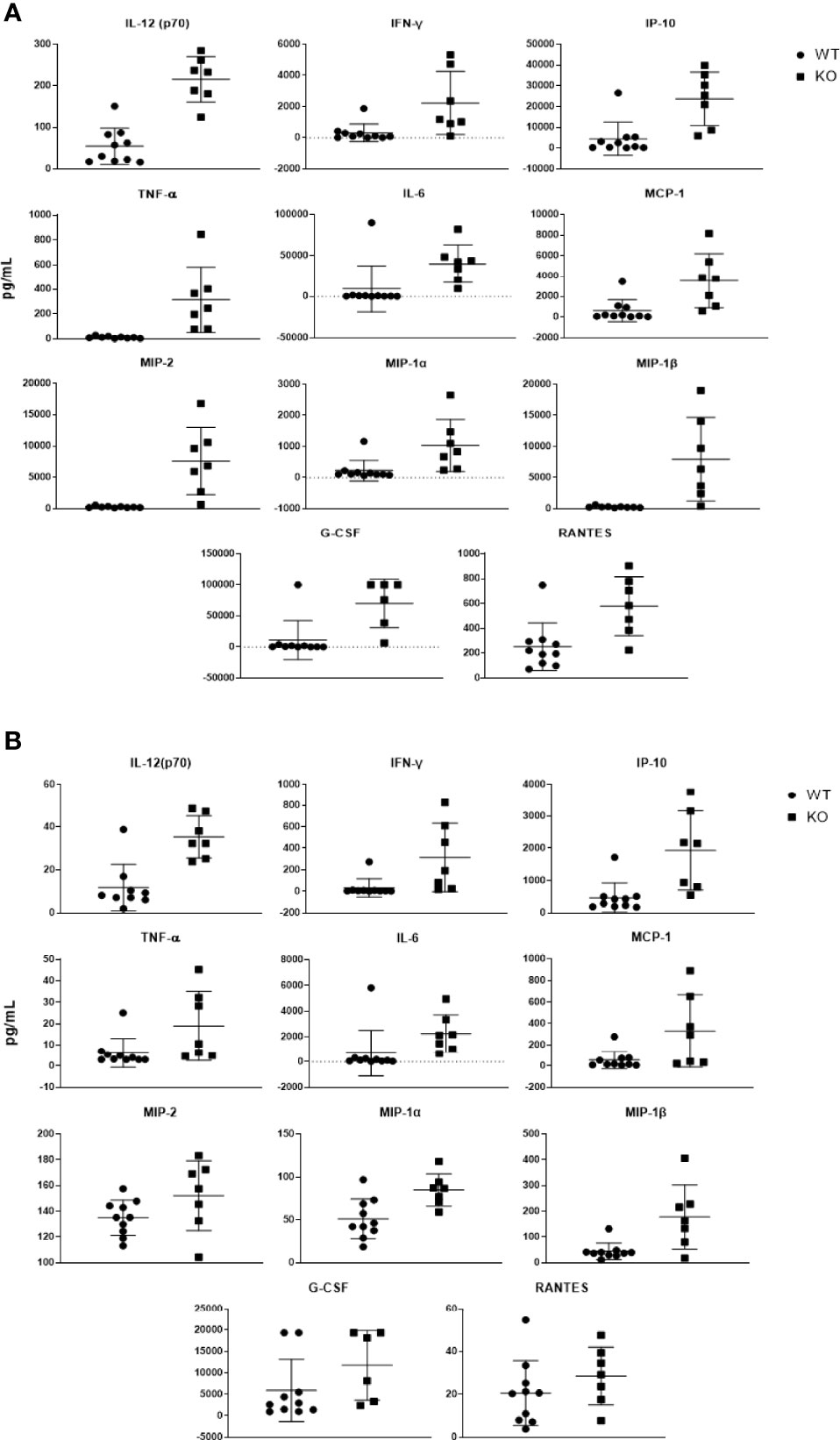
Figure 3 Puma-dependent host responses during early pneumococcal pneumonia suppress production of cytokines in the lung. Cytokines expressed more than 3-fold in Puma-/- mouse lungs (A) and sera (B) are shown here. Cytokines were detected via magnetic bead panel using flow cytometry analysis. Values are reported as pg/mL. Mann-Whitney U tests were performed to determine significance, α = 0.05.
Loss of Puma Induces Changes in the Pulmonary Transcriptome
To further investigate Puma-dependent transcriptional responses during the early phase of pneumonia, total RNA was extracted from lungs of five mice per challenge group 24 hours p.i., purified, and pooled for RNA-seq analysis. Using DEseq2, 21 differentially expressed genes, 15 of which had annotations, were detected with an adjusted p-value < 0.05 (Table 3). Of note, the genes Nrxn2, Adam19, and Eln were each up-regulated in Puma+/+ lungs and down-regulated in those of Puma-/- mice. We performed a gene ontology (GO) analysis on all three sub-ontologies: Biological Process (BP), Molecular Function (MF), and Cellular Component (CC). We found 28, 4, and 7 GO categories significantly enriched for genes differentially regulated in Puma-/- mice in BP, MF, and CC, respectively (Supplementary Table S2). To focus on the categories most likely to have a biologically significant contribution to the infection response, we added a fold-enrichment (FE) criteria and retained only GO sub-categories with FE > 2.5. This resulted in 16, 4, and 3 categories being retained, respectively, in the BP, MF, and CC sub-ontologies. In accordance with detection of IL-12 cytokine, the GO group ‘positive regulation of IL-12 production’ (GO:0032735) was enriched 3.6 fold (Figure 4). Notably, GO groups ‘cellular response to IFN-β’ (GO:0035458) and ‘cellular response to IFN-ϒ’ (GO:0071346) were enriched 5.46 and 3.45, respectively. Individual lists of gene expression from each of these GO groups are shown in Supplementary Table S3.
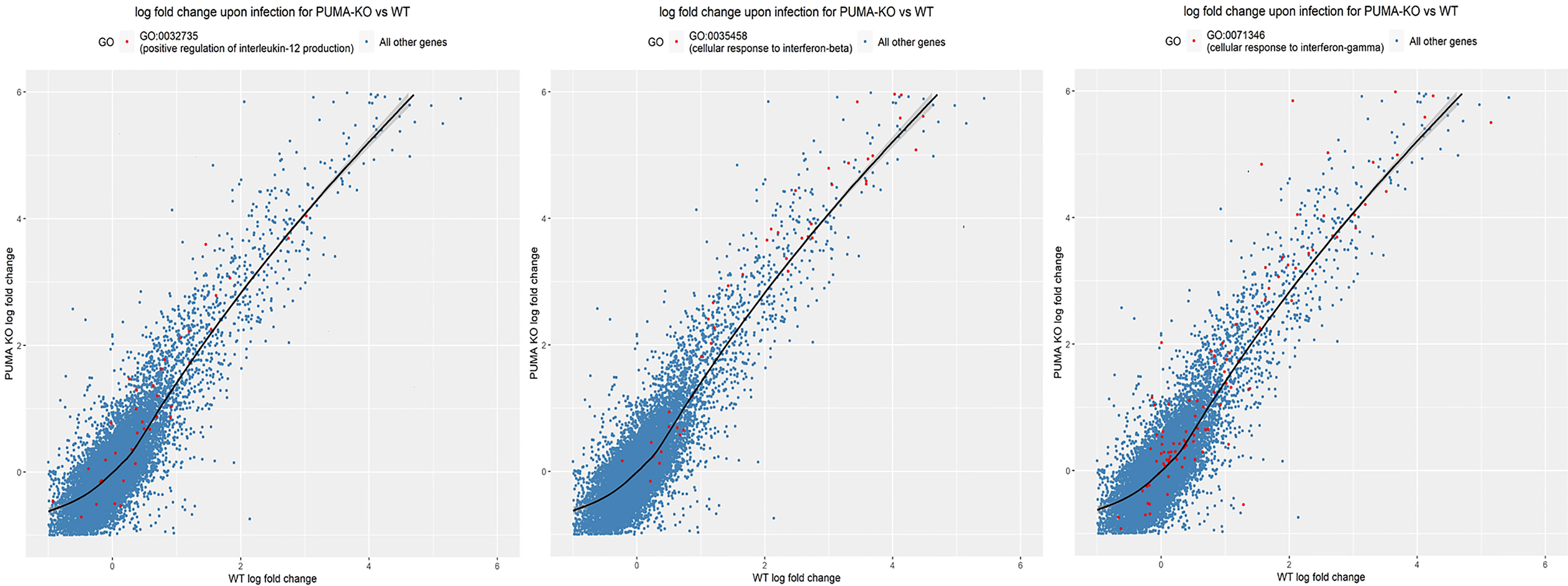
Figure 4 Gene ontology analysis reveals Puma limits IL-12 and Type I and II IFN signaling. Genes significantly expressed (red dots) in their respective GO groups are plotted against all other genes (blue dots). Significance was determined using the Kolmogorov-Smirnov test and p-values were adjusted for multiple-testing. The area above the black line indicates higher expression in Puma-/- mice, while the area under the black line indicates higher expression in Puma+/+ mice.
Discussion/Conclusion
Apoptosis is integral in the host defense response against pneumococcal infection. Neutrophils are the primary cell type responsible for direct clearance of pneumococcus from the lung (Garrison et al., 2010). Phagocytosis of pneumococcus induces apoptosis in neutrophils via phagocytosis-induced cell death (PICD) (Kobayashi et al., 2017). As neutrophils become apoptotic, they are rapidly cleared by macrophages to avoid the detrimental effects of secondary necrosis and prolonged inflammation within the lung (Savill, 1997; Henson and Bratton, 2013). Neutrophil apoptosis directly and indirectly signals surrounding cells to aid in resolution (Henson and Bratton, 2013; Jia et al., 2014). Apoptosis of other cell types also contributes to pneumococcal infection resolution, including macrophages (Marriott et al., 2004; Rai et al., 2015), epithelial cells (Schmeck et al., 2004; Marriott and Dockrell, 2006), and T-cells (Kemp et al., 2002). During the early innate immune response in the lung, all of these cell types may contribute to apoptosis-induced signaling. Multiple cell types have been documented undergoing PUMA-induced apoptosis, including epithelial cells (Dang et al., 2020), neutrophils (Garrison et al., 2010), and T-cells (Fischer et al., 2008).
PUMA distinctly contributes to protective infectious disease responses during acute viral infection by herpes simplex virus (HSV-1) in T-cells (Fischer et al., 2008), and degradation of PUMA induced by Chlamydia trachomatis contributes to persistent infection (Dong et al., 2005). However, PUMA-induced apoptosis contributes to Helicobacter pylori pathogenesis during gastritis (Dang et al., 2020). These opposing roles of PUMA during infection may lie in the pathophysiology of the respective pathogen and the cell types involved. Puma-deficient mice have been intensively studied for their resistance to cancer-causing stimuli (Garrison et al., 2010). HSV-1 can induce oral cancer in mice and is a suspected causative cancer agent in humans (Fischer et al., 2008), and H. pylori is now recognized as a causative agent of gastric cancer. C. trachomatis is an obligate intracellular pathogen and epidemiologically correlated with cancers of the female urogenital epithelium, and almost exclusively infects epithelial cells (Zhu et al., 2016). HSV-1 can invade most cell types but primarily infects epithelial cells and resides in neurons as long-term reservoirs within the host. H. pylori and pneumococcus are both generally acknowledged as extracellular pathogens and each induces strong neutrophil recruitment, which exacerbates local tissue damage. The up- and down-stream inducers of puma in the context of the immune response to infection are currently poorly understood. The contradictory observations on the Puma-/- mouse model in cancer studies versus our pneumococcal pneumonia model continue to make the Puma phenotype and its up- and down-stream regulators molecules of interest. Our findings support the notion that Puma’s role in infectious disease immunobiology is multifactorial and further demonstrates that loss of Puma may enhance risk of negative health outcomes during infection. The precise pathways involved in the protective phenotype of Puma remain to be thoroughly investigated in the context of infection.
While many cytokines have been associated with inflammation modulation during pneumococcal infection, the pro-inflammatory IL-12/IFN-ϒ/IP-10 signaling axis has emerged as a potent arm of the innate immune response. Infection-induced IL-12 secretion was first identified from dendritic cells and neutrophils and can be induced by pneumococcus via TLR3 activation (Rijneveld et al., 2002; Groom and Luster, 2011). The effects of increased IL-12 during pneumococcal pneumonia also include stimulating IFN-ϒ-dependent neutrophil recruitment through increased abundance of MIP-2 and TNF-α (Ethuin et al., 2004; Sun et al., 2007). At 24 hours p.i. Puma+/+ mice expressed less IL-12 in their lungs than Puma-/-. This could be due to the anti-inflammatory effects of apoptotic neutrophils, as pro-inflammatory signaling is dampened once apoptosis is initiated in neutrophils (Haslett, 1999). In accordance with this notion, we previously demonstrated that Puma-/- lungs contained more neutrophils than their Puma+/+ counterparts (Garrison et al., 2010). However, pro-inflammatory efferocytosis of neutrophils also induces an IL-12low phenotype in macrophages (Filardy et al., 2010). This likely contributes to the low levels of IL-12 in Puma+/+ lungs following infection, but the precise mechanisms of temporal IL-12 production and its effect on cell death in specific cellular subsets within the lung during early pneumococcal pneumonia need to be further investigated.
The importance of IFN-ϒ signaling is well demonstrated during the early host response to pneumococcal infection. A previous study showed that pneumococcal infection induced significant increases of IFN-ϒ from greater than 98% of neutrophil in the lungs at 24 hours p.i (Yamada et al., 2011). Neutrophilic production of IFN-ϒ during early pneumococcal pneumonia is likely mediated through NADPH oxidase activity, suggesting IFN-ϒ production in neutrophils may be ROS-dependent, the generation of which is also a result of induction of apoptosis (Gomez et al., 2015). Production of IFN-ϒ in pneumococcal pneumonia models has been linked to strain-dependent effects, and suppresses pneumococcal outgrowth during the early phase by recruiting neutrophils (Rubins and Pomeroy, 1997; Groom and Luster, 2011). As a ligand for the CXCR3 receptor, IP-10 can recruit and activate effector CD4+ Th1, CD8+ CTL, natural killer (NK), and the hybrid NK-T cells (Christaki et al., 2015). In human neutrophils, the combination of IFN-ϒ and TNF-α is the most potent inducer of IP-10 secretion (Cassatella et al., 1997; Gasperini et al., 2019). In accordance with this observation, IFN-ϒ and TNF-α were significantly higher in Puma-/- mice. IP-10 expression is also strain-dependent and associated with acute lung injury during early pneumococcal pneumonia (Seyoum and Yano, 2011). Interestingly, secretion of IL-12 and IP-10 are increased by human peripheral blood monocytes upon exposure to intact pneumococci, but inhibited when exposed to autolyzed pneumococci (Skovbjerg et al., 2017). In pneumococcal pneumonia, IFN-ϒ signaling is thought to exert beneficial effects by activating NK-T cells but detrimental effects upon activating NK cells (Christaki et al., 2015). However, a role for Puma in these cell types has not yet been characterized. It should be noted, though, that in an experimental pneumonia murine model at 24 hours p.i., T cells, NK cells, and macrophages did not produce IFN-ϒ (Gomez et al., 2015). The increase of IFN-ϒ and subsequent IP-10 production in Puma-/- mice 24 hours p.i. underscores the importance of apoptosis in resolving inflammation. While both groups displayed increased interstitial inflammation, overall, Puma-/- mice exhibited a greater degree of inflammation with multiple foci and vasculitis. The excessive inflammation observed in the Puma-/- mice is likely a result of accumulation of neutrophils that remain active and continue to propagate the IL-12/IFN-ϒ/IP-10 axis. Previous works of others using murine models and data presented here strongly suggest that PUMA-dependent neutrophil apoptosis suppresses the IL-12/IFN-ϒ/IP-10 signaling axis, thereby limiting inflammation and consequent pneumococcal dissemination. However, it is currently unknown if the reported IL-12/IFN-ϒ/IP-10 signaling in mice recapitulates the acute-phase cytokine signaling in humans. Previously, IFN-ϒ had been ruled out as an acute-phase cytokine in humans (Endeman et al., 2011). Although, another clinical study found significant differences in bronchoalveolar lavage fluid IFN-ϒ between healthy control subjects and those with CAP and in serum between severe and non-severe CAP patients upon admission (Paats et al., 2013). Beyond the intrinsic differences in murine and human physiology, a likely explanation for these differences may be the temporal differences in surveillance and detection of infection in humans, with testing only occurring within about 72 hours of the first onset of symptoms (Mandell et al., 2007). Consequently, the mechanistic details of apoptotic suppression of the IL-12/IFN-ϒ/IP-10 pro-inflammatory axis, temporospatial regulation of the intercellular signaling, and whether the cascade results in feedback in humans during development of bacterial pneumonia remain unknown.
Puma-dependent responses also regulate the pulmonary transcriptome. In the lung, ADAM19 is expressed in a variety of cell and tissue types. ADAM19 is expressed higher in bronchiolar than alveolar epithelial cells but expressed higher in smooth muscle cells than in bronchiolar epithelial cells (Dijkstra et al., 2009). However, ADAM19 is only weakly expressed in vascular endothelial cells. The detection of ADAM19 on the apical surfaces of airway epithelial cells may suggest a role in the positive regulation in the early innate immune response to infection since ADAM19 can induce inflammation via shedding of TNF-α (Becherer et al., 2003). ADAM19 also contributes to angiogenesis, neurogenesis, synaptogenesis, and adhesion of and invasion by leukocytes (Shirakabe et al., 2001; Dreymueller et al., 2012; Fukazawa et al., 2013). Several of the other ADAM family members have reportedly been observed modulating the immune response to multiple infectious agents, including pneumococcus (Aljohmani and Yildiz, 2020). Down-regulation of Adam19 in Puma-/- lungs suggests an immunomodulatory role in the early innate immune response that is short-lived due to yet unidentified Puma-dependent responses to pneumococcal infection. Nrxn2, a member of the neurexin gene family, encodes a transmembrane protein on pre-synaptic neurons that forms a Ca2+-dependent complex with the protein neuroligin on post-synaptic neurons (Bottos et al., 2009). In addition to regulation of synaptic transmission in the nervous system, neurexin-neuroligin complexation has been observed in vascular tissue. Each neurexin gene encodes a long (α) and short (β) isoform. While the sequence mapping could not discern Nrxn2 α or β transcripts in the analysis reported here, β isoforms have been detected in complexes with neuroligin in the vascular system while positively modulating angiogenesis in conjunction with vascular endothelial growth factor and increasing vascular tone (Berk et al., 2005; Graziano et al., 2013). Eln, the gene encoding elastin, was also up-regulated in a Puma-dependent manner. One explanation for this observation could be that the accumulation of neutrophils in the lung and the hypoxic nature of pneumonia suppress elastin fiber deposition and repair (Berk et al., 2005; Benjamin et al., 2021). Together, these data suggest a Puma-dependent integrated systems physiologic host response to pneumococcal pneumonia. However, the details of these intermolecular and intercellular interactions within the lung have not been thoroughly interrogated during infection.
For the first time, we have demonstrated the immunomodulatory effects of Puma during pneumococcal pneumonia. Puma-dependent responses suppressed pro-inflammatory cytokine signaling, including the highly inflammatory, neutrophil-mediated IL-12/IFN-ϒ/IP-10 signaling axis. Dissemination of pneumococcus and erosion of the pulmonary vasculature was attenuated by Puma-dependent responses. The precise, temporal expression and regulation of IFN-inducible genes remains to be elucidated in the context of Puma-dependent resolution of inflammation. Finally, the activity of ADAM19 during pneumococcal and other bacterial infections also requires further, more intensive study, as well as to what degree, if any, NRXN2 is playing in pulmonary blood pressure regulation during the early phase of acute pneumonia.
Data Availability Statement
The sequencing raw reads have been deposited in the NCBI Short Read Archive under Bioproject accession number: PRJNA748408.
Ethics Statement
The animal study was reviewed and approved by Mississippi State University Institutional Animal Care and Use Committee.
Author Contributions
DK, PM, WT, and JR obtained the data. DK, J-FG, AO, JR, KS, and JT, performed the data analysis. DK, JT, and KS wrote the manuscript. JT was responsible for study concept and design. JT was a supervisor of laboratory analysis of this study. JT is the guarantor of this study and, as such, had full access to all the data in the study and takes responsibility for the integrity of the data and the accuracy of the data analysis. All authors participated in data interpretation and review of the manuscript and approved the final manuscript.
Funding
Research reported in this publication was supported by the Center for Biomedical Research Excellence (COBRE) in Pathogen–Host Interactions, National Institute of General Medical Sciences, National Institutes of Health (P20 472 GM103646-06). The content is solely the responsibility of the authors and does not necessarily represent the official views of the National Institutes of Health.
Conflict of Interest
The authors declare that the research was conducted in the absence of any commercial or financial relationships that could be construed as a potential conflict of interest.
Publisher’s Note
All claims expressed in this article are solely those of the authors and do not necessarily represent those of their affiliated organizations, or those of the publisher, the editors and the reviewers. Any product that may be evaluated in this article, or claim that may be made by its manufacturer, is not guaranteed or endorsed by the publisher.
Supplementary Material
The Supplementary Material for this article can be found online at: https://www.frontiersin.org/articles/10.3389/fcimb.2022.886901/full#supplementary-material
Supplementary Table 1 | Histopathology report from lung sections of WT and KO mice challenged with S. pneumoniae. Lung sections were prepared as described in the main text and scored for inflammation. Histology scores from inflamed WT and KO lungs are plotted with mean (horizontal line) and standard deviation (error bars).
Supplementary Table 2 | GO enrichment analysis in KO pulmonary tissues. BP, MF, and CC GO categories and their respective significant differentially expressed genes are listed. Adjusted p-values and fold-enrichment scores are shown.
Supplementary Table 3 | From Figure 4 in the main text, individual gene identities represented by red dots plotted for each of the three significantly different GO groups between WT and KO mice are provided. Gene expression differences are shown as log-fold change relative to PBS mock-infected WT or KO cohorts.
References
Aljohmani, A., Yildiz, D. (2020). A Disintegrin and Metalloproteinase—Control Elements in Infectious Diseases. Front. Cardiovasc. Med. 7, 608281. doi: 10.3389/fcvm.2020.608281
Becherer, J. D., Zheng, Y., Erdjument-bromage, H., Tempst, P., Blobel, C. P. (2003). Catalytic Properties of ADAM19 Catalytic Properties of ADAM19. J. Biol. Chem. 278 (25), 22331–22340. doi: 10.1074/jbc.M302781200
Benjamin, J. T., Plosa, E. J., Sucre, J. M. S., van der Meer, R., Dave, S., Gutor, S., et al. (2021). Neutrophilic inflammation during lung development disrupts elastin assembly and predisposes adult mice to COPD. J. Clin. Invest. 131 (1), e139481. doi: 10.1172/JCI139481
Berk, J. L., Hatch, C. A., Morris, S. M., Stone, P. J., Goldstein, R. H. (2005). Hypoxia Suppresses Elastin Repair by Rat Lung Fibroblasts. Am. J. Physiol. - Lung Cell Mol. Physiol. 289 (931–936), 931–936. doi: 10.1152/ajplung.00037.2005
Bordon, J., Aliberti, S., Fernandez-Botran, R., Uriarte, S. M., Rane, M. J., Duvvuri, P., et al. (2013). Understanding the Roles of Cytokines and Neutrophil Activity and Neutrophil Apoptosis in the Protective Versus Deleterious Inflammatory Response in Pneumonia. Int. J. Infect. Dis. 17 (2), e76–e83. doi: 10.1016/j.ijid.2012.06.006
Bottos, A., Destro, E., Rissone, A., Graziano, S., Cordara, G., Assenzio, B., et al. (2009). The Synaptic Proteins Neurexins and Neuroligins Are Widely Expressed in the Vascular System and Contribute to Its Functions. Proc. Natl. Acad. Sci. USA 106 (49), 20782–20787. doi: 10.1073/pnas.0809510106
Bray, N. L., Pimentel, H., Melsted, P., Pachter, L. (2016). Near-Optimal Probabilistic RNA-seq Quantification. Nat. Biotechnol. 34 (5), 525–527. doi: 10.1038/nbt.3519
Bruce, K. E., Rued, B. E., Tsui, H. C. T., Winkler, M. E. (2018). The Opp (AmiACDEF) Oligopeptide Transporter Mediates Resistance of Serotype 2 Streptococcus pneumoniae D39 to Killing by Chemokine CXCL10 and Other Antimicrobial Peptides. J Bacteriol. 200, e00745-17. doi: 10.1128/JB.00745-17
Cardiff, R. D., Miller, C. H., Munn, R. J. (2014). Manual Hematoxylin and Eosin Staining of Mouse Tissue Sections. Cold Spring Harb. Protoc. 2014 (6), 655–658. doi: 10.1101/pdb.prot073411
Cassatella, M. A., Gasperini, S., Calzetti, F., Bertagnin, A., Luste, A. D., Mcdonald, P. P. (1997). Regulated Production of the Interferon-Y-Inducible protein40 (Ip-10) Chemokine by Human Neutrophils Eur. J. Immunol. 27(1), 111–1115. doi: 10.1002/eji.1830270117
Christaki, E., Diza, E., Giamarellos-Bourboulis, E. J., Papadopoulou, N., Pistiki, A., Droggiti, D. I., et al. (2015). NK and NKT Cell Depletion Alters the Outcome of Experimental Pneumococcal Pneumonia: Relationship With Regulation of Interferon- γ Production. J. Immunol. Res. 2015, 532717. doi: 10.1155/2015/532717
Craig, A., Mai, J., Cai, S., Jeyaseelan, S. (2009). Neutrophil Recruitment to the Lungs During Bacterial Pneumonia. Infect. Immun. 77 (2), 568–575. doi: 10.1128/IAI.00832-08
Dallaire, F., Ouellet, N., Bergeron, Y., Turmel, V., Gauthier, M. C., Simard, M., et al. (2001). Microbiological and Inflammatory Factors Associated with the Development of Pneumococcal Pneumonia. JID. 184, 292–300.
Dang, Y., Zhang, Y., Xu, L., Zhou, X., Gu, Y., Yu, J. (2020). PUMA-Mediated Epithelial Cell Apoptosis Promotes Helicobacter Pylori Infection-Mediated Gastritis. Cell Death Dis. 11, 139. doi: 10.1038/s41419-020-2339-x
Dijkstra, A., Postma, D. S., Noordhoek, J. A., Lodewijk, M. E., Kauffman, H. F., Ten Hacken, N. H. T., et al. (2009). Expression of ADAMs (“A Disintegrin and Metalloprotease”) in the Human Lung. Virchows Arch. 454 (4), 441–449. doi: 10.1007/s00428-009-0748-4
Dockrell, D. H., Whyte, M. K. B., Mitchell, T. J. (2012). Pneumococcal Pneumonia: Mechanisms of Infection and Resolution. Chest. 142(2), 482–491. doi: 10.1378/chest.12-0210
Domon, H., Oda, M., Maekawa, T., Nagai, K., Takeda, W. (2016). Streptococcus Pneumoniae Disrupts Pulmonary Immune Defence Via Elastase Release Following. Nat. Publ. Gr. 6, 38013. doi: 10.1038/srep38013
Dong, F., Pirbhai, M., Xiao, Y., Zhong, Y., Wu, Y., Zhong, G. (2005). Degradation of the Proapoptotic Proteins Bik, Puma, and Bim With Bcl-2 Domain 3 Homology in Chlamydia Trachomatis-Infected Cells. Inf. Imm. 73 (3), 1861–1864. doi: 10.1128/IAI.73.3.1861-1864.2005
Dreymueller, D., Pruessmeyer, J., Groth, E., Ludwig, A. (2012). The Role of ADAM-mediated Shedding in Vascular Biology. Eur. J. Cell Biol. 91, 472–485. doi: 10.1016/j.ejcb.2011.09.003
Droemann, D., Aries, S. P., Hansen, F. (2000). Decreased Apoptosis and Increased Activation of Alveolar Neutrophils in Bacterial Pneumonia. Chest 117 (6), 1679–1684. doi: 10.1378/chest.117.6.1679
Endeman, H., Meijvis, S. C. A., Rijkers, G. T., Van Velzen-Blad, H., Van Moorsel, C. H. M., Grutters, J. C., et al. (2011). Systemic Cytokine Response in Patients With Community-Acquired Pneumonia. Eur. Respir. J. 37 (6), 1431–1438. doi: 10.1183/09031936.00074410
Engelich, G., White, M., Hartshorn, K. L. (2001). Neutrophil Survival iIs Markedly Reduced by Incubation With Influenza Virus and Streptococcus Pneumoniae: Role of Respiratory Burst. J. Leukoc. Biol. 69 (January), 50–56. doi: 10.1189/jlb.69.1.50
Esmann, L., Idel, C., Sarkar, A., Hellberg, L., Behnen, M., Möller, S., et al. (2010). Phagocytosis of Apoptotic Cells by Neutrophil Granulocytes: Diminished Proinflammatory Neutrophil Functions in the Presence of Apoptotic Cells. J. Immunol. 184, 391–400. doi: 10.4049/jimmunol.0900564
Ethuin, F., Gérard, B., Benna, J. E., Boutten, A., Gougereot-Pocidalo, M. A., Jacob, L., et al. (2004). Human Neutrophils Produce Interferon Gamma Upon Stimulation by Interleukin-12. Lab. Investig. 84 (10), 1363–1371. doi: 10.1038/labinvest.3700148
Filardy, A. A., Pires, D. R., Nunes, M. P., Takiya, C. M., Freire-de-lima, C. G., Flavia, L., et al. (2010). Proinflammatory Clearance of Apoptotic Neutrophils Induces an il-12 Low il-10 High Regulatory Phenotype in Macrophages. J. Immunol. 185, 2044–2050. doi: 10.4049/jimmunol.1000017
Fischer, S. F., Belz, G. T., Strasser, A. (2008). BH3-Only Protein Puma Contributes to Death of Antigen-Specific T Cells During Shutdown of an Immune Response to Acute Viral Infection. Proc. Natl. Acad. Sci. U. S. A. 105 (8), 3035–3040. doi: 10.1073/pnas.0706913105
Fox, S., Leitch, A. E., Duffin, R., Haslett, C., Rossi, A. G. (2010). Neutrophil Apoptosis: Relevance to the Innate Immune Response and Inflammatory Disease. J. Innate Immun. 2 (February), 216–227. doi: 10.1159/000284367
Fukazawa, T., Matsumoto, M., Imura, T., Khalesi, E., Kajiume, T., Kawahara, Y., et al. (2013). Electrical Stimulation Accelerates Neuromuscular Junction Formation Through ADAM19/neuregulin/ErbB Signaling In Vitro. Neurosci. Lett. 545, 29–34. doi: 10.1016/j.neulet.2013.04.006
Garrison, S. P., Thornton, J. A., Häcker, H., Webby, R., Rehg, J. E., Parganas, E., et al (2010). The p53-Target Gene Puma Drives Neutrophil-Mediated Protection Against Lethal Bacterial Sepsis. PLoS Pathog. 6 (12), e1001240. doi: 10.1371/journal.ppat.1001240
Gasperini, S., Marchi, M., Calzetti, F., Vicentini, L., Olsen, H., Murphy, M., et al. (2019). Gene Expression and Production of the Monokine Induced by IFN-gamma (MIG), IFN-Inducible T Cell Alpha Chemoattractant (I-TAC), and IFN-gamma-inducible Protein 10 (IP-10) Chemokines by Human Neutrophils. J. Immunol. 162, 4928–4937.
Geiser, T., Ishigaki, M., Van Leer, C., Matthay, M. A., Broaddus, V. C., Ishigaki, M., et al. (2004). H2O2 Inhibits Alveolar Epithelial Wound Repair In Vitro by Induction of Apoptosis. Am. J. Physiol. - Lung Cell Mol. Physiol. 287 (April), L448–L453. doi: 10.1152/ajplung.00177.2003
Gomez, J. C., Yamada, M., Martin, J. R., Dang, H., Brickey, W. J., Bergmeier, W., et al. (2015). Mechanisms of Interferon-γ Production by Neutrophils and Its Function During Streptococcus Pneumoniae Pneumonia. Am. J. Respir. Cell Mol. Biol. 52 (3), 349–364. doi: 10.1165/rcmb.2013-0316OC
Gomez, J. C., Dang, H., Kanke, M., Hagan, R. S., Mock, J. R., Kelada, S. N. P., et al. (2017). Predicted Effects of Observed Changes in the mRNA and microRNA Transcriptome of Lung Neutrophils During S. Pneumoniae Pneumonia in Mice. Sci Rep. 7, 11258. doi: 10.1038/s41598-017-11638-7
Graziano, S., Marchiò, S., Bussolino, F., Arese, M. (2013). A Peptide From the Extracellular Region of the Synaptic Protein α Neurexin Stimulates Angiogenesis and the Vascular Specific Tyrosine Kinase Tie2. Biochem. Biophys. Res. Commun. 432 (4), 574–579. doi: 10.1016/j.bbrc.2013.02.045
Greenlee-Wacker, M. C. (2016). Clearance of Apoptotic Neutrophils and Resolution of Inflammation. Immunol. Rev. 273, 357–370. doi: 10.1111/imr.12453
Groom, J. R., Luster, A. D. (2011). CXCR3 Ligands: Redundant, Collaborative, and Antagonistic Functions. Immunol. Cell Biol. 89 (2), 207–215. doi: 10.1038/icb.2010.158.CXCR3
Haslett, C. (1999). Granulocyte Apoptosis and Its Role in the Resolution and Control of Lung Inflammation. Am. J. Respir. Crit. Care Med 160 (5 Pt 2), S5–S11. doi: 10.1164/ajrccm.160.supplement_1.4
Henson, P. M., Bratton, D. L. (2013). Antiinflammatory Effects of Apoptotic Cells. J. Clin. Invest. 123 (7), 2773–2774. doi: 10.1172/JCI69344
Hocke, A.C., Hartmann, I.K., Eitel, J., Optiz, B., Scharf, S., Suttorp, N., et al. (2008). Subcellular Expression Pattern and Role of IL-15 in Pneumococci Induced Lung Epithelial Apoptosis. Histochem Cell Biol. 130, 165–176. doi: 10.1007/s00418-008-0414-y
Holzmann, B., Dudek, M., Puttur, F., Ku, A. A., Berod, L., Sparwasser, T. (2016). Lung Epithelium and Myeloid Cells Cooperate to Clear Acute Pneumococcal Infection. Mucosal Immunol. 9 (5), 1298–1302. doi: 10.1038/mi.2015.128
Jeong, D. G., Seo, J. H., Heo, S. H., Choi, Y. K., Jeong, E. S. (2015). Tumor Necrosis Factor-Alpha Deficiency Impairs Host Defense against Streptococcus pneumoniae. Lab. Anim. Res. 31 (2), 78–85. doi: 10.5625/lar.2015.31.2.78
Jia, S. H., Parodo, J., Charbonney, E., Tsang, J. L. Y., Jia, Y., Rotstein, O. D., et al. (2014). Activated Neutrophils Induce Epithelial Cell Apoptosis Through Oxidant-Dependent Tyrosine Dephosphorylation of Caspase-8. Am. J. Pathol. 184 (4), 1030–1040. doi: 10.1016/j.ajpath.2013.12.031
Kemp, K., Bruunsgaard, H., Skinhøj, P., Pedersen, B. K. (2002). Pneumococcal Infections in Humans are Associated With Increased Apoptosis and Trafficking of Type 1 Cytokine-Producing T Cells. Infect. Immun. 70 (9), 5019–5025. doi: 10.1128/IAI.70.9.5019-5025.2002
Kennedy, A. D., Deleo, F. R. (2009). Neutrophil Apoptosis and the Resolution of Infection. Immunol. Res. 43 (December 2008), 25–61. doi: 10.1007/s12026-008-8049-6
Knapp, S., Hareng, L., Rijneveld, A. W., Bresser, P., Van DerZee, J. S., Florquin, S., et al. (2004). Activation of Neutrophils and inhibition of the Proinflammatory Cytokine Response by Endogenous Granulocyte Colony-Stimulating Factor in Murine Pneumococcal Pneumonia. JID. 189, 1506–1514. doi: 0022-1899/2004/18908-0022
Kobayashi, S. D., Malachowa, N., Deleo, F. R. (2017). Influence of Microbes on Neutrophil Life and Death. Front. Cell Infect. Microbiol. 7, 159. doi: 10.3389/fcimb.2017.00159
Koedel, U., Frankenberg, T., Kirschnek, S., Obermaier, B., Ha, H. (2009). Apoptosis Is Essential for Neutrophil Functional Shutdown and Determines Tissue Damage in Experimental Pneumococcal Meningitis. PloS Pathog. 5 (5), e1000461. doi: 10.1371/journal.ppat.1000461
Koppe, U., Hogner, K., Doehn, J. M., Muller, H. C., Witzenrath, M., Gutbier, B., et al. (2012). Streptococcus pneumoniae Stimulates a STING- and IFN Regulatory Factor 3-Dependent Type I IFN Production in Macrophages, Which Regulates RANTES Production in Macrophages, Cocultured Alveolar Epithelial Cells, and Mouse Lungs. J. Immunol. 188, 811–817. doi: 10.4049/jimmunol.1004143
Kuipers, K., Lokken, K. L., Zangari, T., Boyer, M. A., Shin, S., Weiser, J. N., et al. (2018). Age-Related Differences in IL-1 Signaling and Capsule Serotype Affect Persistence of Streptococcus pneumoniae Colonization. PLoS Pthog. 14(10):e1007396. doi: 10.1371/journal.ppat.1007396
Lacks, S., Hotchkiss, R. (1960). A Study of the Genetic Material Determining an Enzyme Activity in Pneumococcus. Biochim. Biophys. Acta 39 (July), 508–517. doi: 10.1016/0006-3002(60)90205-5
Lemon, J. K., Miller, M. R., Weiser, J. N. (2015). Sensing of Interleukin-1 Cytokines During Streptococcus pneumoniae Colonization Contributes to Macrophage Recruitment and Bacterial Clearance. Infect. Immun. 83, 3204–3212. doi: 10.1128/IAI.00224-15
Loose, M., Hudel, M., Zimmer, K.P., Garcia, E., Hammerschmidt, S., Lucas, R., et al. (2014) Pneumococcal Hydrogen Peroxide-Induced Stress signaling Regulates Inflammatory Genes. JID. doi: 10.1093/infdis/jiu428
Loughran, S. T., Power, P. A., Maguire, P. T., McQuaid, S. L., Buchanan, P. J., Jonsdottir, I., et al. (2018). Influenza Infection Directly Alters Innate IL-23 and IL-12p70 and Subsequent IL-17A and IFN-ϒ Responses to Pneumococcus In Vitro in Human Monocytes. PloS One 13 (9), e0203521. doi: 10.1371/journal.pone.0203521
Love, M. I., Huber, W., Anders, S. (2014). Moderated Estimation of Fold Change and Dispersion for RNA-seq Data With Deseq2. Genome Biol. 15 (550). doi: 10.1186/s13059-014-0550-8
Mandell, L. A., Wunderink, R. G., Anzueto, A., Bartlett, J. G., Campbell, G. D., Dean, N. C., et al. (2007). Infectious Diseases Society of America/American Thoracic Society Consensus Guidelines on the Management of Community-Acquired Pneumonia in Adults. Clin. Infect. Dis. 44 (SUPPL. 2), S27–S72. doi: 10.1086/511159
Marriott, H. M., Ali, F., Read, R. C., Mitchell, T. J., Whyte, M. K. B., Dockrell, D. H. (2004). Nitric Oxide Levels Regulate Macrophage Commitment to Apoptosis or Necrosis During Pneumococcal Infection. FASEB J. 18 (10), 1126–1128. doi: 10.1096/fj.03-1450fje
Marriott, H. M., Dockrell, D. H. (2006). Streptococcus Pneumoniae: The Role of Apoptosis in Host Defense and Pathogenesis. Int. J. Biochem. Cell Biol. 38 (11), 1848–1854. doi: 10.1016/j.biocel.2006.06.001
Marriott, H. M., Hellewell, P. G., Cross, S. S., Ince, P. G., Whyte, M. K. B., Dockrell, D. H. (2006). Decreased Alveolar Macrophage Apoptosis Is Associated With Increased Pulmonary Inflammation in a Murine Model of Pneumococcal Pneumonia. J. Immunol. 177 (9), 6480–6488. doi: 10.4049/jimmunol.177.9.6480
Matute-bello, G., Martin, T. R. (2003). Science Review: Apoptosis in Acute Lung Injury. Crit. Care 7 (October), 355–358. doi: 10.1186/cc1861
Nakano, K., Vousden, K. H. (2001). PUMA, a Novel Proapoptotic Gene, Is Induced by p53 National Cancer Institute at Frederick. Mol. Cell 7 (March), 683–694. doi: 10.1016/S1097-2765(01)00214-3
Paats, M. S., Bergen, I. M., Hanselaar, W. E. J. J., Van Zoelen, E. C. G., Hoogsteden, H. C., Hendriks, R. W., et al. (2013). Local and Systemic Cytokine Profiles in Nonsevere and Severe Community-Acquired Pneumonia. Eur. Respir. J. 41 (6), 1378–1385. doi: 10.1183/09031936.00060112
Palaniappan, R., Singh, S., Singh, U. P., Singh, R., Ades, E. W., Briles, D. E., et al. (2006). CCL5 Modulates Pneumococcal Immunity and Carriage. J. Immunol. 176, 2346–2356. doi: 10.4049/jimmunol.176.4.2346
Penaloza, H. F., Nieto, P. A., Munoz-Durango, N., Salazar-Echegarai, F. J., Torres, J., Parga, M. J., et al. (2015). Interleukin-10 Plays a Key Role in the Modulation of Neutrophils Recruitment and Lung Inflammation During Infection by Streptococcus pneumoniae. Immunology. 146, 100–112. doi: 10.1111/imm.12486
Penaloza, H. F., Salazar-Echegarai, F. J., Bueno, S. M. (2018). Interleukin-10 Modulation of Neutrophil Subsets Infiltrating Lungs During Streptococcus pneumoniae Infection. Biochem Biophys Rep. 13, 12–16. doi: 10.1016/j.bbrep.2017.11.004
Rai, P., Parrish, M., Jun, I., Tay, J., Li, N., Ackerman, S., et al. (2015). Streptococcus Pneumoniae Secretes Hydrogen Peroxide Leading to DNA Damage and Apoptosis in Lung Cells. Proc. Natl. Acad. Sci. U. S. A., 112 (26), 3421–3430. doi: 10.1073/pnas.1424144112
Rijneveld, A. W., Lauw, F. N., Schultz, M. J., Florquin, S., Te Velde, A. A., Speelman, P., et al. (2002). The Role of Interferon-γ in Murine Pneumococcal Pneumonia. J. Infect. Dis. 185 (1), 91–97. doi: 10.1086/338122
Ritchie, N. D., Ritchie, R., Bayes, H. K., Micthell, T. J., Evan, T. J. (2018). IL-17 Can Be Protective or Deleterious in Murine Pneumococcal Pneumonia. PLoS Pathog. 14 (5), e1007099. doi: 10.1371/journal.ppat.1007099
Rubins, J. B., Pomeroy, C. (1997). Role of Gamma Interferon in the Pathogenesis of Bacteremic Pneumococcal Pneumonia. Infect. Immun. 65 (7), 2975–2977. doi: 10.1128/iai.65.7.2975-2977.1997
Savill, J. (1997). Apoptosis in Resolution of Inflammation. J. Leukoc. Biol. 61 (April), 375–380. doi: 10.1002/jlb.61.4.375
Schmeck, B., Gross, R., Guessan, PDNЈ, Hocke, A. C., Hammerschmidt, S., Mitchell, T. J., et al. (2004). Streptococcus Pneumoniae- Induced Caspase 6-Dependent Apoptosis in Lung Epithelium. Infect. Immun. 72 (9), 4940–4947. doi: 10.1128/IAI.72.9.4940-4947.2004
Seyoum, B., Yano, M. (2011). Pirofski L Anne. The Innate Immune Response to Streptococcus Pneumoniae in the Lung Depends on Serotype and Host Response. Vaccine 29 (45), 8002–8011. doi: 10.1016/j.vaccine.2011.08.064
Shirakabe, K., Wakatsuki, S., Kurisaki, T., Fujisawa-Sehara, A. (2001). Roles of MELTRIN β/ADAM19 in the Processing of Neuregulin. J. Biol. Chem. 276 (12), 9352–9358. doi: 10.1074/jbc.M007913200
Skovbjerg, S., Nordén, R., Martner, A., Samuelsson, E., Hynsjö, L., Wold, A. E. (2017). Intact Pneumococci Trigger Transcription of Interferon-Related Genes in Human Monocytes, While Fragmented, Autolyzed Bacteria Subvert This Response. Infect. Immun. 85 (5), e00960–e00916. doi: 10.1128/IAI.00960-16
Steinwede, K., Tempelhof, O., Bolte, K., Maus, R., Bohling, J., Ueberberg, B., et al. (2011). Local Delivery of GM-CSF Protects Mice from Lethal Pneumococcal Pneumonia. J. Immunol. 187, 5346–5356. doi: 10.4049/jimmunol.1101413
Sun, K., Salmon, S. L., Lotz, S. A., Metzger, D. W. (2007). Interleukin-12 Promotes Gamma Interferon-Dependent Neutrophil Recruitment in the Lung and Improves Protection Against Respiratory Streptococcus pneumoniae Infection. Infect. Immun. 75 (3), 1196–1202. doi: 10.1128/IAI.01403-06
Tettelin, H., Nelson, K. E., Paulsen, I. T., Eisen, J. A., Read, T. D., Peterson, S., et al. (2001). Complete Genome Sequence of a Virulent Isolate of Streptococcus Pneumoniae. Science 293 (July), 498–506. doi: 10.1126/science.1061217
Van Der Poll, T., Keogh, C. V., Guirao, X., Buurman, W. A., Kopf, M., Lowry, S. F. (1997). Interleukin-6 Gene-Deficient Mice Show Impaired Defense Against Pneumococcal Pneumonia. J. Infect. Dis. 176 (2), 439–444. doi: 10.1086/514062
Vousden, K. H. (2005). p53 and PUMA: A Deadly Duo. Science 309 (September), 1685–1686. doi: 10.1126/science.1118232
Wang, J., Barke, R. A., Charboneau, R., Roy, S. (2005). Morphine Imparis Host Innate Immune Response and Increases Susceptibility to Streptococcus pneumoniae Lung Infection. J. Immunol. 174, 426–434. doi: 10.4049/jimmunol.174.1.426
Williams, A. E., Jose, R. J., Brown, J. S., Chambers, R. C. (2015). Enhanced Inflammation in Aged Mice Following Infection With Streptococcus pneumoniae Is Associated with Decreased IL-10 and Augmented Chemokine Production. Am. J. Physiol. Lung. Cell. Mol. Physiol. 308, L539–L549. doi: 10.1152/ajplung.00141.2014
Winter, C., Taut, K., Srivastava, M., Langer, F., Mack, M., Briles, D. E., et al. (2007). Lung-Specific Overexpression of CC Chemokine Ligand (CCL) 2 Enhances the Host Defense to Streptococcus pneumoniae Infection in Mice: Role of the CCL2-CCR2 Axis. J. Immunol. 178, 5828–5838.
Woods, D. R., José, R. J. (2017). Current and Emerging Evidence for Immunomodulatory Therapy in Community-Acquired Pneumonia. Ann. Res. Hosp. 1 (33). doi: 10.21037/arh.2017.08.01
Wu, Y., Chen, S. (2014). Apoptotic Cell: Linkage of Inflammation and Wound Healing. Front. Pharmacol. 5 (January), 1–6. doi: 10.3389/fphar.2014.00001
Yamada, M., Gomez, J. C., Chugh, P. E., Lowell, C. A., Dinauer, M. C., Dittmer, D. P., et al. (2011). Interferon-ϒ Production by Neutrophils During Bacterial Pneumonia in Mice. Am. J. Respir. Crit. Care Med. 183, 1391–1401. doi: 10.1164/rccm.201004-0592OC
Yamamoto, K., Ahyi, A. N. N., Pepper-Cunningham, Z. A., Ferrari, J. D., Wilson, A. A., Jones, M. R., et al. (2014). Roles of Lung Epithelium in Neutrophil Recruitment During Pneumococcal Pneumonia. Am. J. Respir. Cell Mol. Biol. 50 (2), 253–262. doi: 10.1165/rcmb.2013-0114OC
Yamamoto, N., Kawakami, K., Kinjo, Y., Miyagi, K., Kinjo, T., Uezu, K., et al. (2004). Essential Role for the p40 Subunit of interleukin-12 in Neutrophil-Mediated Early Host Defense Against Pulmonary Infection with Streptococcus pneumoniae: Involvement of Interferon-ϒ. Microbes Infect. 6 (14), 1241–1249. doi: 10.1016/j.micinf.2004.08.007
Yates, A. D., Achuthan, P., Akanni, W., Allen, J., Allen, J., Alvarez-Jarreta, J., et al. (2020). Ensembl 2020. Nucleic Acids Res. 48 (D1), D682–D688. doi: 10.1093/nar/gkz966
Zhu, H., Shen, Z., Luo, H., Zhang, W., Zhu, X. (2016). Chlamydia Trachomatis Infection-Associated Risk of Cervical Cancer. Medicine 95 (13), e3077. doi: 10.1097/MD.0000000000003077
Keywords: PUMA (p53 upregulated modulator of apoptosis), inflammation, streptococcus pneumoniae (pneumococcus), apoptosis, innate immunity
Citation: Kennedy II DE, Mody P, Gout J-F, Tan W, Seo KS, Olivier AK, Rosch JW and Thornton JA (2022) Contribution of Puma to Inflammatory Resolution During Early Pneumococcal Pneumonia. Front. Cell. Infect. Microbiol. 12:886901. doi: 10.3389/fcimb.2022.886901
Received: 01 March 2022; Accepted: 25 April 2022;
Published: 26 May 2022.
Edited by:
Stephanie Rochelle Shames, Kansas State University, United StatesReviewed by:
Federico Iovino, Karolinska Institutet (KI), SwedenBrandon Kim, University of Alabama, United States
Jeffrey Lee Bose, University of Kansas Medical Center, United States
Copyright © 2022 Kennedy II, Mody, Gout, Tan, Seo, Olivier, Rosch and Thornton. This is an open-access article distributed under the terms of the Creative Commons Attribution License (CC BY). The use, distribution or reproduction in other forums is permitted, provided the original author(s) and the copyright owner(s) are credited and that the original publication in this journal is cited, in accordance with accepted academic practice. No use, distribution or reproduction is permitted which does not comply with these terms.
*Correspondence: Justin A. Thornton, dGhvcm50b25AYmlvbG9neS5tc3N0YXRlLmVkdQ==