- 1Faculty of Medicine and University Hospital of Cologne, Institute for Medical Microbiology, Immunology and Hygiene, Cologne, Germany
- 2Cologne Cluster of Excellence in Cellular Stress Responses in Aging-Associated Diseases (CECAD), University of Cologne, Cologne, Germany
- 3German Center for Infection Research, Bonn-Cologne, Germany
Macrophages remove bacteria from the extracellular milieu via phagocytosis. While most of the engulfed bacteria are degraded in the antimicrobial environment of the phagolysosome, several bacterial pathogens have evolved virulence factors, which evade degradation or allow escape into the cytosol. To counter this situation, macrophages activate LC3-associated phagocytosis (LAP), a highly bactericidal non-canonical autophagy pathway, which destroys the bacterial pathogens in so called LAPosomes. Moreover, macrophages can also target intracellular bacteria by pore-forming toxin-induced non-canonical autophagy (PINCA), a recently described non-canonical autophagy pathway, which is activated by phagosomal damage induced by bacteria-derived pore-forming toxins. Similar to LAP, PINCA involves LC3 recruitment to the bacteria-containing phagosome independently of the ULK complex, but in contrast to LAP, this process does not require ROS production by Nox2. As last resort of autophagic targeting, macrophages activate xenophagy, a selective form of macroautophagy, to recapture bacteria, which evaded successful targeting by LAP or PINCA through rupture of the phagosome. However, xenophagy can also be hijacked by bacterial pathogens for their benefit or can be completely inhibited resulting in intracellular growth of the bacterial pathogen. In this perspective, we discuss the molecular differences and similarities between LAP, PINCA and xenophagy in macrophages during bacterial infections.
Phagocytosis: Main Weapon of Macrophages
The most prominent, characteristic feature of macrophages is their ability to phagocytose extracellular material ranging from cellular debris to whole cells (Fadok et al., 1998; Erwig and Henson, 2008; Kono and Rock, 2008; Suzanne and Steller, 2013; Kourtzelis et al., 2020), but also invading pathogens (Djaldetti et al., 2002; Haas, 2007; Lemke, 2019). With this ability to separate foreign invaders like bacteria, fungi or parasites from the rest of the organism, they represent one of the first lines of defense against invading pathogens (Rosales and Uribe-Querol, 2017). After induction by various cell surface receptors, such as the mannose receptor, Fc‐receptors and scavenger receptors (Freeman and Grinstein, 2014; Uribe-Querol and Rosales, 2020), the cargo is enclosed in a single-membrane structure called phagosome. Several factors, like vacuolar-type H+-ATPase (V-ATPase)-mediated acidification (Sun-Wada et al., 2009; Dragotakes et al., 2020; Westman and Grinstein, 2021), production of reactive oxygen species (ROS) (Craig and Slauch, 2009; Slauch, 2011; Wink et al., 2011; Herb and Schramm, 2021) and exposure to hydrolases after fusion with lysosomes (del Cerro-Vadillo et al., 2006; Schramm et al., 2014; Weiss and Schaible, 2015) lead to the formation of a highly antimicrobial environment for engulfed pathogens and, in most cases, result in their degradation (Haas, 2007). However, several bacterial pathogens have established strategies to evade this degradative fate in the phagosome (Mitchell et al., 2016; Grijmans et al., 2022), e.g. Staphylococcus aureus (S. aureus) (Fraunholz and Sinha, 2012; Moldovan and Fraunholz, 2019; Rao et al., 2020), Salmonella typhimurium (S. typhimurium) (Eriksson et al., 2003; Fenlon and Slauch, 2014; Burton et al., 2014; Rhen, 2019; Rao et al., 2020) or Mycobacterium tuberculosis (Queval et al., 2017; Koster et al., 2017), which can alter the phagosomal composition and structure for their benefit and do not only remain unharmed, but also replicate inside the phagosome.
LC3-Associated Phagocytosis
Since several bacterial pathogens can evade the degradative fate of the phagosome, macrophages activate a non-canonical autophagy pathway called LC3-associated phagocytosis (LAP), which can enhance phagolysosomal fusion. For example, phagosomes containing Toll-like receptor ligand-coated latex beads (Sanjuan et al., 2007), dead cells (Martinez et al., 2011) or pathogens, such as Legionella dumoffii (Hubber et al., 2017), Listeria monocytogenes (L. monocytogenes) (Gluschko et al., 2018) and Aspergillus fumigatus (Martinez et al., 2015) show increased fusion with lysosomes during LAP, resulting in enhanced degradation of the cargo. Notably, LAP can also delay phagolysosomal fusion, leading to prolonged antigen presentation by major histocompatibility complex (MHC) class II (Romao et al., 2013; Ma et al., 2014; Fletcher et al., 2018). LAP is induced by various surface receptors found on macrophages (Sanjuan et al., 2007; Ma et al., 2012; Tam et al., 2014; Gluschko et al., 2018; Hayashi et al., 2018) and results in the decoration of phagosomes with microtubule-associated protein 1 light chain-3 (LC3) family proteins, resulting in so called LAPosomes. (Sanjuan et al., 2007; Martinez et al., 2011; Durgan et al., 2021). LAP and macroautophagy share some but not all components of the autophagic machinery, e.g. parts of the class III phosphatidylinositol 3-kinase (PI3KC3) complex or the two ubiquitin-like conjugation systems, i.e. the autophagy-related protein (ATG) 12 conjugation system and the LC3 lipidation system (Florey et al., 2011; Lystad et al., 2019) (Figure 1). Generation of LAPosomes requires the production of the membrane lipid phosphatidylinositol 3-phosphate (PI3P) (by components of the PI3KC3 complex) and ATG16L1 recruitment to the PI3P-containing target membrane (Kim et al., 2013; Martinez et al., 2015). Comparable to macroautophagy, the PI3KC3 complex, which is involved in LAPosome formation, also contains Beclin-1 (BECN1) (Sanjuan et al., 2007; Martinez et al., 2015; Backer, 2016), vacuolar protein sorting-associated proteins (VPS) 15 and 34, as well as UV radiation resistance-associated gene protein (UVRAG), but lacks ATG14 and activating molecule in BECN1-regulated autophagy protein 1 (AMBRA1) (Martinez et al., 2015; Backer, 2016). In contrast to macroautophagy, LAP requires a specific component of the PI3KC3 complex called Rubicon, which facilitates VPS34 activity and sustains PI3P presence on the LAPosome (Martinez et al., 2015). Another difference is the dispensability of WD repeat domain phosphoinositide-interacting proteins (WIPI) and ATG2, which are required for macroautophagy but not for LAP (Martinez et al., 2015; Fischer et al., 2020). Recently, it was shown that the WD40 domain of ATG16L1 is required for its recruitment to the PI3P-containing target membrane during LAP. Mice lacking the WD40 domain of ATG16L1 are deficient for LAP but not for macroautophagy (Rai et al., 2019). This implicates that a complete different factor than WIPI is required for recruitment of ATG16L1 to the PI3P-containing membrane on the LAPosome. Some studies have shown that the V-ATPase can recruit ATG16L1 onto single-membrane vesicles via its WD40 domain (Florey et al., 2015; Fletcher et al., 2018; Xu et al., 2019; Fischer et al., 2020). Moreover, activity of V-ATPase can be induced by osmotic imbalances caused by pore-forming toxins e.g. by the Helicobacter pylori (H. pylori) pore-forming toxin vacuolating cytotoxin A (VacA) (Florey et al., 2015). V-ATPase activation and ATG16L1 recruitment lead to LC3 lipidation onto single-membrane vacuoles, a mechanism which is independent of the upstream macroautophagy machinery, e.g. the unc-51-like kinase (ULK) complex (Fletcher et al., 2018; Xu et al., 2019), which resembles LAP. A recent preprint study by Hooper et al. indicates that also during LAP, V-ATPase is responsible for ATG16L1 recruitment and subsequent conjugation of LC3 onto the phagosomal membrane (Hooper et al., 2021) (Figure 1). LC3 lipidation resembles the conjugation of ubiquitin to proteins (Slobodkin and Elazar, 2013), therefore similar terms were used for the enzymes of the two ubiquitin like conjugation systems, which carry out the process (Ichimura et al., 2000). For both complexes E1-like ATG7 and E2-like ATG3 catalyze the reactions during LC3 lipidation. The ATG12–ATG5–ATG16L1 complex carries out an analogous function as the ubiquitin E3 ligase and mediates transfer of LC3 from ATG3 to phosphatidylethanolamine (PE) in the phagosomal membrane (Hanada et al., 2007; Sakoh-Nakatogawa et al., 2013).
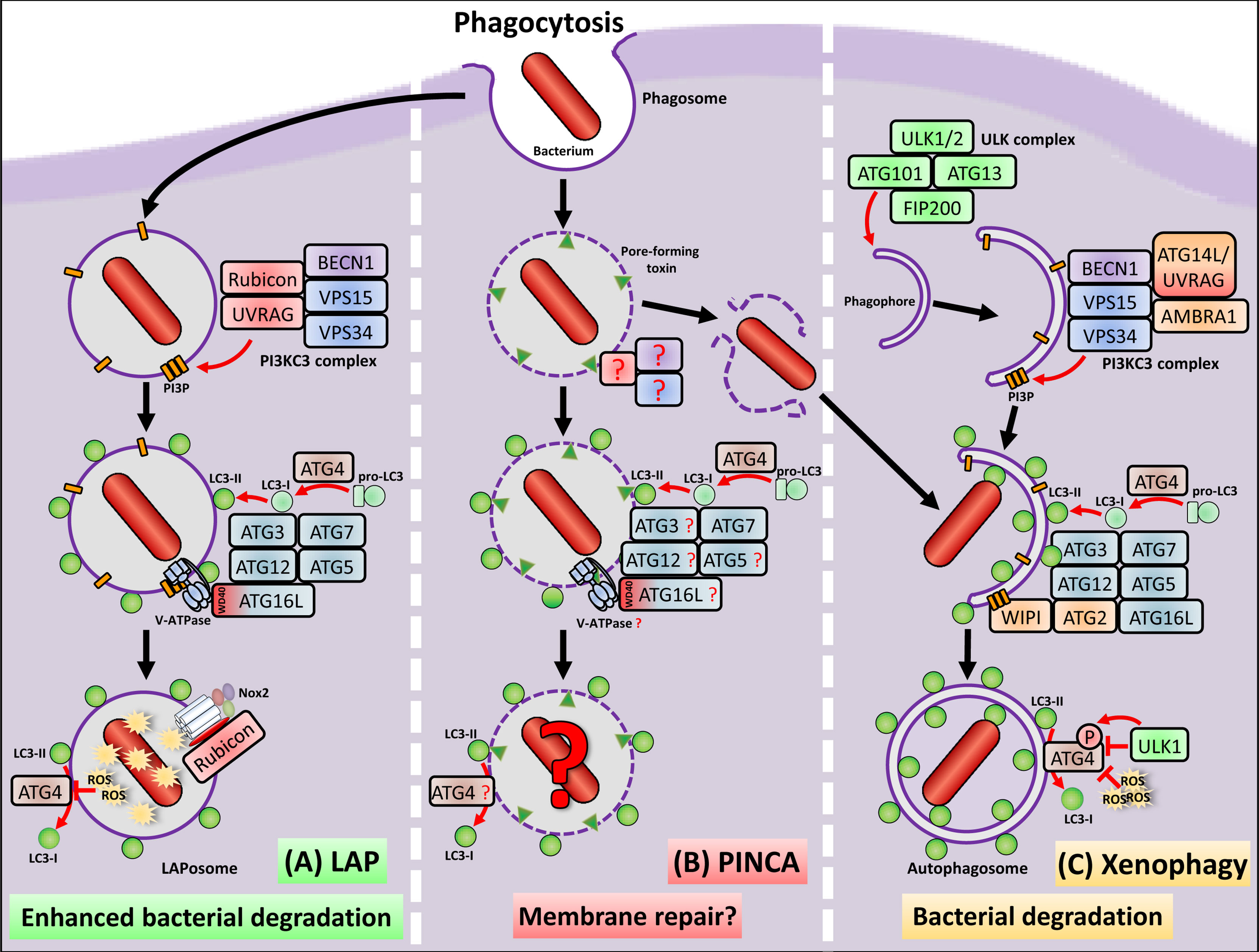
Figure 1 Macrophages remove bacteria from the extracellular milieu via phagocytosis, which is the engulfment and enclosure in a single membrane vesicle, called phagosome. Most of the engulfed bacteria are degraded due to the highly antimicrobial environment of the phagosome. However, several bacterial pathogens have evolved virulence factors, which allow escape from the phagosome into the cytosol. (A) Macrophages activate LAP to inactivate and destroy the bacterial pathogen before phagosomal escape. LAP shares some, but not all components of the autophagy machinery to conjugate LC3 to the phagosomal membrane. Importantly, LAP is completely independent from the ULK complex, defining it as non-canonical autophagy pathway. The PI3KC3 complex, which is activated during LAP, shares the core components BECN1, VSP15 and VSP34 but exclusively contains UVRAG and Rubicon, which is not only important for recruitment of the PI3KC3 complex, but also stabilizes the p22phox subunit of Nox2. To the PI3P platforms formed by the PI3KC3 complex, a so far unknown factor binds to which ATG16L1 is recruited via its WD40 domain together with the LC3 conjugation machinery consisting of ATG3, ATG7, ATG5 and ATG12. A preprint study (Hooper et al., 2021) marks the V-ATPase as possible candidate, which recruits ATG16L1 and the LC3 conjugation machinery to the phagosome during LAP. ROS production by Nox2 leads to oxidative inactivation of the protease ATG4, which prevents ATG4-mediated deconjugation of LC3 and stabilization of the LAPosome. LAPosomes show enhanced fusion with lysosomes and enhanced degradation of the bacterial pathogen. (B) During PINCA, damage induced by bacteria-derived pore-forming toxins induces LC3 recruitment to the phagosome independent of the ULK complex components FIP200 and ULK1/2. With the exception of ATG7, the factors for induction and execution, which are necessary for LC3 recruitment during PINCA, as well as its precise functions remain to be resolved. Since pore-forming toxins lead to V-ATPase and ATG16L recruitment during other non-canonical pathways, this might also be the case during PINCA. (C) To recapture bacterial pathogens that had managed to escape from phagosomes, either during conventional phagocytosis, LAP or PINCA, macrophages activate xenophagy, a selective form of macroautophagy. Initiation of xenophagy depends on the ULK complex, i.e. ULK1/2, FIP200, ATG13 and ATG101, which generates a double-membrane structure called phagophore. Recruitment of the proteins BECN1, VSP15, VSP34, AMBRA1 and either UVRAG or ATG14L to the phagophore leads to formation of the PI3KC3 complex, which then generates PI3P in the growing phagophore membrane. These PI3P platforms serve as docking station for the WIPI-ATG2 complex, which subsequently recruits the machinery for LC3 conjugation, facilitating degradation of the recaptured bacterial pathogen in autophagolysosomes. During xenophagy, inactivation of ATG4 can be mediated by cytotosolic ROS production or phosphorylation of ATG4 by ULK1 both preventing deconjugation of LC3 from the autophagosome.
Notably, Durgan and colleagues have shown that single-membrane structures during non-canonical autophagy, e.g. LAP, show a different LC3 lipidation pattern. In addition to PE-conjugated LC3, the study demonstrated that LC3 is also conjugated to phosphatidylserine (PS). This is in contrast to autophagosomes during macroautophagy, which exclusively display PE-conjugated LC3 (Durgan et al., 2021). However, the major difference beyond LC3 conjugation onto single- vs. double-membrane vesicles is that LAP, in contrast to macroautophagy, is completely independent of the ULK complex (Martinez et al., 2011; Martinez et al., 2015). Most importantly, LC3 recruitment to phagosomes by LAP requires production of ROS by the NADPH oxidase 2 (Nox2) (Huang et al., 2009; Yang et al., 2012; Martinez et al., 2015; Gluschko et al., 2018; Gluschko et al., 2021; Herb and Schramm, 2021; Ligeon et al., 2021). The Nox2 complex consists of two integral membrane subunits, gp91phox, and p22phox, and the cytosolic subunits p67phox, p47phox, p40phox and Rac1/2 (Herb and Schramm, 2021). For induction of ROS production by Nox2, the cytosolic subunits are recruited to the integral membrane subunits. Thereby, Rubicon plays an important role beyond its involvement in generation of PI3P by the PI3KC3 complex, by stabilizing p22phox via direct binding (Yang et al., 2012; Martinez et al., 2015). However, why Nox2-derived ROS are crucial for LAP induction is not understood in detail. Notably, it was recently shown that ROS production by Nox2 mediates oxidative inactivation of the protease ATG4 thereby preventing deconjugation of LC3 from the phagosome (Ligeon et al., 2021).
PINCA – a New Non-Canonical Autophagy Pathway
We recently described a new variant of non-canonical autophagy in macrophages, which we termed pore-forming toxin-induced non-canonical autophagy (PINCA) (Gluschko et al., 2021). Similar to other forms of non-canonical autophagy, e.g. LAP, PINCA also is independent of the ULK complex components focal adhesion kinase family interacting protein of 200 kD (FIP200) and ULK1/2. Importantly, in contrast to LAP, PINCA did also not require Nox2-derived ROS production, which is crucial for LC3 decoration of phagosomes during LAP (Sanjuan et al., 2007; Martinez et al., 2015; Gluschko et al., 2018; Herb et al., 2020; Ligeon et al., 2021). Thus, we observed induction of PINCA in Nox2-deficient peritoneal macrophages (PM) and in wildtype bone marrow-derived macrophages (BMDM), which fail to produce sufficient levels of ROS for induction of LAP due to low expression of Nox2 (Gluschko et al., 2021). Instead, damage induced by the pore-forming toxin listeriolysin O (LLO) of L. monocytogenes or by the several pore-forming toxins of S. aureus were necessary to induce LC3 recruitment to the damaged phagosomes, i.e. PINCA (Figure 1).
Notably, damage induced by the needle-like Type three secretion system (T3SS) of Shigella flexneri (S. flexneri) or S. typhimurium did not induce PINCA in macrophages. A possible reason for this is the expression of bacterial virulence factors such as Salmonella outer protein F (SopF) of S. typhimurium, which inhibits the vacuolar V-ATPase and thereby prevents ATG16L recruitment and LC3 lipidation onto S. typhimurium-containing vacuoles (Xu et al., 2019). Interestingly, vacuolar damage caused by SopF-deficient S. typhimurium triggered ATG16L recruitment and LC3 lipidation onto S. typhimurium-containing vacuoles in epithelial cells (Xu et al., 2019), which was independent of FIP200 and resembled PINCA. Thus, it is likely that also in macrophages T3SS-induced damage can trigger PINCA, when this process is not actively inhibited by bacterial virulence factors such as SopF. Notably, this T3SS-induced LC3 lipidation on damaged, but still intact vacuoles/phagosomes should not be mistaken with xenophagy, a selective form of macroautophagy, which targets ruptured vacuoles, membrane remnants or cytosolic bacteria and involves exposure of glycans and the recruitment of galectins and several other factors such as TANK-binding kinase 1 (TBK1) (Thurston et al., 2012; Ravenhill et al., 2019; Bell et al., 2021). As already mentioned, in mouse embryonic fibroblasts (MEFs) pore-forming toxins can induce osmotic imbalances within endolysosomal compartments, which are sensed by V-ATPase and result in ATG16L recruitment and LC3 conjugation (Florey et al., 2015). Due to this, it is plausible that bacterial toxin-induced pore formation during PINCA can also induce osmotic imbalances within phagosomes in macrophages. Wether these osmotic imbalances can trigger V-ATPase-coupled ATG16L recruitment and LC3 lipidation during PINCA, as observed during other non-canonical autophagy pathways (Florey et al., 2015; Fletcher et al., 2018; Xu et al., 2019; Hooper et al., 2021), are interesting topics for future studies.
The functional purpose of PINCA seems to be related to LAP during L. monocytogenes infection of wildytpe PM. We observed that during PINCA, LC3-positive phagosomes fused more often with lysosomes than conventional, LC3-negative phagosomes indicating that also PINCA promotes phagolysosomal fusion (Gluschko et al., 2021). However, in sharp contrast to LAP, which clearly promotes the anti-listerial activity of tissue macrophages, e.g. PM (Gluschko et al., 2018), LC3 recruitment to phagosomal membranes by PINCA and subsequently increased phagolysosomal fusion did not substantially contribute to anti-listerial activity of BMDM (Gluschko et al., 2021). Both LAP and PINCA require ATG7 for LC3 conjugation (Herb et al., 2020). Therefore, the question whether PINCA contributes to anti-listerial activity could unfortunately not be answered by the sole use of ATG7-deficient macrophages (Gluschko et al., 2021), since these cells can induce neither LAP nor PINCA. In addition, Nox2-deficient PM are not well suited to investigate the functional purpose of PINCA, since Nox2-derived ROS are not only necessary for LAP induction but also fulfill a plethora of other antimicrobial functions (Canton et al., 2021; Herb and Schramm, 2021). Moreover, ROS have been shown to inactivate ATG4, thereby preventing deconjugation of LC3 from the phagosome (Ligeon et al., 2021). It is conceivable that during PINCA, LC3 is continuously deconjugated from the phagosome in the absence of ROS production, except there is a, yet unknown, ROS-independent mechanism, which inactivates ATG4 during PINCA. Due to this, a possible antimicrobial function of PINCA could be easily overlooked, when PINCA is induced in the absence of ROS, which not only prevent LC3-deconjugation (Ligeon et al., 2021), but also substantially contribute to other antimicrobial functions independent of autophagic targeting of any kind (Canton et al., 2021; Herb and Schramm, 2021). Otherwise, when PINCA is induced in the presence of ROS, it is likely that also LAP is induced in parallel to PINCA, making it difficult to distinguish between these two pathways. The identification of a mechanistic component, which exclusively activates PINCA in the presence of functional ROS production, but without activating LAP, will be necessary to address this unanswered question.
Notably, in addition to enhanced phagolysosomal fusion, we found that LC3-positive phagosomes formed by PINCA were damaged less often than conventional, LC3-negative phagosomes (Gluschko et al., 2021). This indicates that either targeting by PINCA impedes the damage to the phagosomal membrane, or that LC3-decorated phagosomes are pre-assigned for membrane damage repair, as observed during autophagy induced in S. typhimurium-infected epithelial cells (Thurston et al., 2012; Kreibich et al., 2015). Thus, PINCA might represent an attempt of macrophages to repair damaged phagosomal membranes as last resort against the bacteria, which have not yet escaped from the phagosome.
Antibacterial Xenophagy in Macrophages
Several bacteria manage to escape form the phagosome via rupture of the phagosomal membrane prior to degradation or targeting by LAP or PINCA (Fernandez-Prada et al., 2000; Hamon et al., 2012; Jamwal et al., 2016; Bell et al., 2021). Escape into the cytosol not only means the evasion from degradation, but provides also a rich pool of nutrients for the escaped pathogen, which enables cytosolic replication within the cell without being detected by other phagocytes. However, macrophages have established a counter measure to recapture cytosolic bacteria, namley xenophagy (Sharma et al., 2018) (Figure 1). During xenophagy, the cargo for the autophagic machinery is a bacterial pathogen that is escaping or has already escaped from the phagosome/vacuole (Pao and Rape, 2019). The cytosolic bacterium can be tagged with ubiquitin and recognized by various autophagy receptors, e.g. Sequestosome-1 (SQSTM1)/p62 or calcium-binding and coiled-coil domain-containing protein 2 (CALCOCO2)/NDP52 (Thurston et al., 2009; Johansen and Lamark, 2011), which recruit the autophagic components to the target. Thereby, the cargo is enclosed and isolated from the rest of the cell by formation of a double-membrane structure, called phagophore (Yla-Anttila et al., 2009; Lamb et al., 2013; Chang et al., 2021). Initiation of phagophore formation is, in contrast to LAP and PINCA, dependent on activation of the ULK complex (Suzuki et al., 2007; Jung et al., 2009; Koyama-Honda et al., 2013; Fujioka et al., 2014; Shi et al., 2020; Mercer et al., 2021), which is composed of ULK1 or ULK2 (ULK1/2), FIP200, ATG13, as well as ATG101 (Chang and Neufeld, 2009; Ganley et al., 2009; Hara and Mizushima, 2009; Hosokawa et al., 2009; Mercer et al., 2009; Lin and Hurley, 2016; Hurley and Young, 2017; Chang et al., 2021). ULK complex activity, which is not required for LAP (Martinez et al., 2011; Martinez et al., 2015) or PINCA (Gluschko et al., 2021), leads to the generation of PI3P at the membrane of the forming phagophore via one of the two PI3KC3 complexes (Russell et al., 2013; Backer, 2016). The two PI3KC3 complexes activated during macroautophagy contain the same core components, namely VPS34 and VPS15, BECN1 and AMBRA1 (Yu et al., 2015; Young et al., 2019), but can either recruit ATG14 (found in the PI3KC3 complex 1) or UVRAG (found in the PI3KC3 complex 2) (Itakura et al., 2008), (Figure 1). The PI3P generated at the membrane serves as a platform for recruitment of a complex consisting of WIPI proteins and ATG2, which are dispensable for LAP (Martinez et al., 2015; Fischer et al., 2020). After recruitment to the forming membrane of the phagophore, the WIPI-ATG2 complex itself recruits the LC3 conjugation machinery (Kabeya et al., 2000; Martens, 2016; Schaaf et al., 2016). Notably, ULK1 inhibits the catalytic activity of ATG4 by phosphorylation, thereby preventing the deconjugation of LC3 from the autophagosome (Pengo et al., 2017). In addition, cytosolic ROS can also inhibit ATG4 deconjugation activity (Scherz-Shouval et al., 2007), similar to Nox2-mediated oxidative inactivation of ATG4 during LAP (Ligeon et al., 2021). Finally, the closed autophagosome subsequently fuses with lysosomes, which leads to degradation of the recaptured bacterial pathogen in an autophagolysosome (Sharma et al., 2018). Xenophagy therefore plays a crucial role in the cellular defense against invading bacteria (Levine et al., 2011), not only in macrophages (Niu et al., 2008; English et al., 2009; Moreau et al., 2010; Travassos et al., 2010; Starr et al., 2012; Park et al., 2016; Ganesan et al., 2017; Zhou et al., 2017), but also in non-immune cells (Gutierrez et al., 2004; Birmingham et al., 2006; Thurston et al., 2009; Zheng et al., 2009; Wild et al., 2011; Thurston et al., 2012; von Muhlinen et al., 2012; Xu et al., 2019).
However, bacteria have also evolved mechanism to avoid degradation by xenophagy (Huang and Brumell, 2014). Some bacteria can reside in autophagosomes or autophagosome-like structures for replication, such as H. pylori (Wang et al., 2009; Hu et al., 2019), Legionella pneumophila (Amer and Swanson, 2005; Joshi and Swanson, 2011) or Yersinia pseudotubercolosis (Moreau et al., 2010), while others completely inhibit xenophagy and freely replicate in the cytosol. L. monocytogenes, for example, inhibits xenophagy via the virulence factors actin assembly-inducing protein (ActA) (Yoshikawa et al., 2009) and the two phosphatidylinositol-specific phospholipases C PlcA and PlcB (Mitchell et al., 2015), S. flexneri inhibits binding of ATG5 during xenophagy via the virulence factor IscB (Ogawa et al., 2005) and S. typhimurium secrets several virulence factors, such as SseL (Mesquita et al., 2012), SseF and SseG (Feng et al., 2018) to counter xenophagic targeting (Casanova, 2017).
Conclusions
Macrophages are among the first line of defense against invading pathogens. Due to distinct virulence factors, some bacterial pathogens can evade the destruction in the phagosome, either by re-modulation of the phagosomal milieu or via escape into the cytosol. Activation of LAP, a highly microbicidal non-canonical autophagy pathway (Herb et al., 2020; Grijmans et al., 2022), enhances the degradative capacity of macrophages. We recently described another non-canonical autophagy pathway termed PINCA (Gluschko et al., 2021), which is triggered by perforation of bacteria-containing phagosomes, independent of the ULK complex components ULK1/2 and FIP200 and also independent of Nox2-derived ROS, therefore representing a non-canonical autophagy pathway distinct from LAP. Pore-forming toxins can induce osmotic imbalances, which are sensed by V-ATPase and result in ATG16L recruitment and LC3 conjugation (Florey et al., 2015). It is very likely that LC3 recruitment to perforated phagosomes during PINCA is also activated by the V-ATPase-ATG16L1-axis, which might represent a general pathway to recruit LC3 to damaged, yet not ruptured compartments. Furthermore, it is reasonable that also during LAP, V-ATPase is responsible for ATG16L1 recruitment and subsequent LC3 conjugation, since ROS production by Nox2 is not sufficient to induce LAP, when V-ATPase is inhibited (Hooper et al., 2021). It is tempting to speculate that LC3 conjugation during LAP is not triggered by Nox2-generated ROS but by V-ATPase-induced ATG16L1 recruitment. Instead, ROS production during LAP only prevents deconjugation of LC3 through oxidative inactivation of ATG4 as shown by Ligeon et al. (2021), which resembles redox-dependent inactivation of ATG4 during autophagy (Scherz-Shouval et al., 2007; Pérez-Pérez et al., 2016). While LAP and xenophagy have clear degradative functions, despite some bacterial pathogens exploiting autophagosomes as a replicative niche (Huang and Brumell, 2014; Siqueira et al., 2018; Riebisch et al., 2021), the functional purpose of PINCA remains unclear. It is possible that PINCA might represent an emergency repair mechanism for damaged phagosomes, similar to membrane repair mechanisms in S. typhimurium-infected epithelial cells (Thurston et al., 2012; Kreibich et al., 2015). Alternatively, LC3 on the perforated phagosome may recruit an entire spectrum of proteins containing a LC3-interacting region (Johansen and Lamark, 2020), which in turn may accelerate phagolysosomal fusion, or exert another, yet unknown function of PINCA.
Data Availability Statement
The original contributions presented in the study are included in the article/supplementary material, further inquiries can be directed to the corresponding author.
Author Contributions
Conceptualization, MH. writing—original draft preparation, MH, AG and MK. writing—review and editing, MH, AG, AF and MK. visualization, MH. supervision, MK. project administration, MK. funding acquisition, MH, AG and MK. All authors have read and agreed to the published version of the manuscript.
Funding
This work was supported by the Deutsche Forschungsgemeinschaft (DFG) grant SCHR 1627/2-1 and intramural grants to A.G. and M.H and by the Federal state grant (NRW) 323-8.04.10.02-141905 to MK.
Conflict of Interest
The authors declare that the research was conducted in the absence of any commercial or financial relationships that could be construed as a potential conflict of interest.
Publisher’s Note
All claims expressed in this article are solely those of the authors and do not necessarily represent those of their affiliated organizations, or those of the publisher, the editors and the reviewers. Any product that may be evaluated in this article, or claim that may be made by its manufacturer, is not guaranteed or endorsed by the publisher.
References
Amer, A. O., Swanson, M. S. (2005). Autophagy Is an Immediate Macrophage Response to Legionella Pneumophila. Cell Microbiol. 76, 765–778. doi: 10.1111/j.1462-5822.2005.00509.x
Backer, J. M. (2016). The Intricate Regulation and Complex Functions of the Class III Phosphoinositide 3-Kinase Vps34. Biochem. J. 47315, 2251–2271. doi: 10.1042/BCJ20160170
Bell, S. L., Lopez, K. L., Cox, J. S., Patrick, K. L., Watson, R. O. (2021). Galectin-8 Senses Phagosomal Damage and Recruits Selective Autophagy Adapter TAX1BP1 To Control Mycobacterium Tuberculosis Infection in Macrophages. mBio 124, e0187120. doi: 10.1128/mBio.01871-20
Birmingham, C. L., Smith, A. C., Bakowski, M. A., Yoshimori, T., Brumell, J. H. (2006). Autophagy Controls Salmonella Infection in Response to Damage to the Salmonella-Containing Vacuole. J. Biol. Chem. 28116, 11374–11383. doi: 10.1074/jbc.M509157200
Burton, N. A., Schurmann, N., Casse, O., Steeb, A. K., Claudi, B., Zankl, J., et al. (2014). Disparate Impact of Oxidative Host Defenses Determines the Fate of Salmonella During Systemic Infection in Mice. Cell Host Microbe 151, 72–83. doi: 10.1016/j.chom.2013.12.006
Canton, M., Sanchez-Rodriguez, R., Spera, I., Venegas, F. C., Favia, M., Viola, A., et al. (2021). Reactive Oxygen Species in Macrophages: Sources and Targets. Front. Immunol. 124077, 734229. doi: 10.3389/fimmu.2021.734229
Casanova, J. E. (2017). Bacterial Autophagy: Offense and Defense at the Host-Pathogen Interface. Cell Mol. Gastroenterol. Hepatol. 42, 237–243. doi: 10.1016/j.jcmgh.2017.05.002
Chang, C., Jensen, L. E., Hurley, J. H. (2021). Autophagosome Biogenesis Comes Out of the Black Box. Nat. Cell Biol. 235, 450–456. doi: 10.1038/s41556-021-00669-y
Chang, Y. Y., Neufeld, T. P. (2009). An Atg1/Atg13 Complex With Multiple Roles in TOR-Mediated Autophagy Regulation. Mol. Biol. Cell 207, 2004–2014. doi: 10.1091/mbc.e08-12-1250
Craig, M., Slauch, J. M. (2009). Phagocytic Superoxide Specifically Damages an Extracytoplasmic Target to Inhibit or Kill Salmonella. PloS One 43, e4975. doi: 10.1371/journal.pone.0004975
del Cerro-Vadillo, E., Madrazo-Toca, F., Carrasco-Marin, E., Fernandez-Prieto, L., Beck, C., Leyva-Cobian, F., et al. (2006). Cutting Edge: A Novel Nonoxidative Phagosomal Mechanism Exerted by Cathepsin-D Controls Listeria Monocytogenes Intracellular Growth. J. Immunol. 1763, 1321–1325. doi: 10.4049/jimmunol.176.3.1321
Djaldetti, M., Salman, H., Bergman, M., Djaldetti, R., Bessler, H. (2002). Phagocytosis–the Mighty Weapon of the Silent Warriors. Microsc Res. Tech 576, 421–431. doi: 10.1002/jemt.10096
Dragotakes, Q., Stouffer, K. M., Fu, M. S., Sella, Y., Youn, C., Yoon, O. I., et al. (2020). Macrophages Use a Bet-Hedging Strategy for Antimicrobial Activity in Phagolysosomal Acidification. J. Clin. Invest. 1307, 3805–3819. doi: 10.1172/JCI133938
Durgan, J., Lystad, A. H., Sloan, K., Carlsson, S. R., Wilson, M. I., Marcassa, E., et al. (2021). Non-Canonical Autophagy Drives Alternative ATG8 Conjugation to Phosphatidylserine. Mol. Cell 819, 2031–40 e8. doi: 10.1016/j.molcel.2021.03.020
English, L., Chemali, M., Duron, J., Rondeau, C., Laplante, A., Gingras, D., et al. (2009). Autophagy Enhances the Presentation of Endogenous Viral Antigens on MHC Class I Molecules During HSV-1 Infection. Nat. Immunol. 105, 480–487. doi: 10.1038/ni.1720
Eriksson, S., Lucchini, S., Thompson, A., Rhen, M., Hinton, J. C. (2003). Unravelling the Biology of Macrophage Infection by Gene Expression Profiling of Intracellular Salmonella Enterica. Mol. Microbiol. 471, 103–118. doi: 10.1046/j.1365-2958.2003.03313.x
Erwig, L. P., Henson, P. M. (2008). Clearance of Apoptotic Cells by Phagocytes. Cell Death differentiation 152, 243–250. doi: 10.1038/sj.cdd.4402184
Fadok, V. A., Bratton, D. L., Konowal, A., Freed, P. W., Westcott, J. Y., Henson, P. M. (1998). Macrophages That Have Ingested Apoptotic Cells In Vitro Inhibit Proinflammatory Cytokine Production Through Autocrine/Paracrine Mechanisms Involving TGF-Beta, PGE2, and PAF. J. Clin. Invest. 1014, 890–898. doi: 10.1172/JCI1112
Feng, Z. Z., Jiang, A. J., Mao, A. W., Feng, Y., Wang, W., Li, J., et al. (2018). The Salmonella Effectors SseF and SseG Inhibit Rab1A-Mediated Autophagy to Facilitate Intracellular Bacterial Survival and Replication. J. Biol. Chem. 29325, 9662–9673. doi: 10.1074/jbc.M117.811737
Fenlon, L. A., Slauch, J. M. (2014). Phagocyte Roulette in Salmonella Killing. Cell Host Microbe 151, 7–8. doi: 10.1016/j.chom.2014.01.001
Fernandez-Prada, C. M., Hoover, D. L., Tall, B. D., Hartman, A. B., Kopelowitz, J., Venkatesan, M. M. (2000). Shigella Flexneri IpaH(7.8) Facilitates Escape of Virulent Bacteria From the Endocytic Vacuoles of Mouse and Human Macrophages. Infect. Immun. 686, 3608–3619. doi: 10.1128/IAI.68.6.3608-3619.2000
Fischer, T. D., Wang, C., Padman, B. S., Lazarou, M., Youle, R. J. (2020). STING Induces LC3B Lipidation Onto Single-Membrane Vesicles via the V-ATPase and ATG16L1-WD40 Domain. J. Cell Biol. 21912. doi: 10.1083/jcb.202009128
Fletcher, K., Ulferts, R., Jacquin, E., Veith, T., Gammoh, N., Arasteh, J. M., et al. (2018). The WD40 Domain of ATG16L1 Is Required for Its Non-Canonical Role in Lipidation of LC3 at Single Membranes. EMBO J. 374, e97840. doi: 10.15252/embj.201797840
Florey, O., Gammoh, N., Kim, S. E., Jiang, X., Overholtzer, M. (2015). V-ATPase and Osmotic Imbalances Activate Endolysosomal LC3 Lipidation. Autophagy 111, 88–99. doi: 10.4161/15548627.2014.984277
Florey, O., Kim, S. E., Sandoval, C. P., Haynes, C. M., Overholtzer, M. (2011). Autophagy Machinery Mediates Macroendocytic Processing and Entotic Cell Death by Targeting Single Membranes. Nat. Cell Biol. 1311, 1335–1343. doi: 10.1038/ncb2363
Fraunholz, M., Sinha, B. (2012). Intracellular Staphylococcus Aureus: Live-In and Let Die. Front. Cell Infect. Microbiol. 2, 43. doi: 10.3389/fcimb.2012.00043
Freeman, S. A., Grinstein, S. (2014). Phagocytosis: Receptors, Signal Integration, and the Cytoskeleton. Immunol. Rev. 2621, 193–215. doi: 10.1111/imr.12212
Fujioka, Y., Suzuki, S. W., Yamamoto, H., Kondo-Kakuta, C., Kimura, Y., Hirano, H., et al. (2014). Structural Basis of Starvation-Induced Assembly of the Autophagy Initiation Complex. Nat. Struct. Mol. Biol. 216, 513–521. doi: 10.1038/nsmb.2822
Ganesan, R., Hos, N. J., Gutierrez, S., Fischer, J., Stepek, J. M., Daglidu, E., et al. (2017). Salmonella Typhimurium Disrupts Sirt1/AMPK Checkpoint Control of mTOR to Impair Autophagy. PloS Pathog. 132, e1006227. doi: 10.1371/journal.ppat.1006227
Ganley, I. G., Lam du, H., Wang, J., Ding, X., Chen, S., Jiang, X. (2009). ULK1.ATG13.FIP200 Complex Mediates mTOR Signaling and Is Essential for Autophagy. J. Biol. Chem. 28418, 12297–12305. doi: 10.1074/jbc.M900573200
Gluschko, A., Farid, A., Herb, M., Grumme, D., Kronke, M., Schramm, M. (2021). Macrophages Target Listeria Monocytogenes by Two Discrete Non-Canonical Autophagy Pathways. Autophagy, 1–18. doi: 10.1080/15548627.2021.1969765
Gluschko, A., Herb, M., Wiegmann, K., Krut, O., Neiss, W. F., Utermohlen, O., et al. (2018). The Beta2 Integrin Mac-1 Induces Protective LC3-Associated Phagocytosis of Listeria Monocytogenes. Cell Host Microbe 233, 324–37 e5. doi: 10.1016/j.chom.2018.01.018
Grijmans, B. J. M., van der Kooij, S. B., Varela, M., Meijer, A. H. (2022). LAPped in Proof: LC3-Associated Phagocytosis and the Arms Race Against Bacterial Pathogens. Front. Cell. Infection Microbiol. 11. doi: 10.3389/fcimb.2021.809121
Gutierrez, M. G., Master, S. S., Singh, S. B., Taylor, G. A., Colombo, M. I., Deretic, V. (2004). Autophagy Is a Defense Mechanism Inhibiting BCG and Mycobacterium Tuberculosis Survival in Infected Macrophages. Cell 1196, 753–766. doi: 10.1016/j.cell.2004.11.038
Haas, A. (2007). The Phagosome: Compartment With a License to Kill. Traffic (Copenhagen Denmark) 84, 311–330. doi: 10.1111/j.1600-0854.2006.00531.x
Hamon, M. A., Ribet, D., Stavru, F., Cossart, P. (2012). Listeriolysin O: The Swiss Army Knife of Listeria. Trends Microbiol. 208, 360–368. doi: 10.1016/j.tim.2012.04.006
Hanada, T., Noda, N. N., Satomi, Y., Ichimura, Y., Fujioka, Y., Takao, T., et al. (2007). The Atg12-Atg5 Conjugate Has a Novel E3-Like Activity for Protein Lipidation in Autophagy. J. Biol. Chem. 28252, 37298–37302. doi: 10.1074/jbc.C700195200
Hara, T., Mizushima, N. (2009). Role of ULK-FIP200 Complex in Mammalian Autophagy: FIP200, a Counterpart of Yeast Atg17? Autophagy 51, 85–87. doi: 10.4161/auto.5.1.7180
Hayashi, K., Taura, M., Iwasaki, A. (2018). The Interaction Between IKKalpha and LC3 Promotes Type I Interferon Production Through the TLR9-Containing LAPosome. Sci. Signal. 11528, eaan4144. doi: 10.1126/scisignal.aan4144
Herb, M., Gluschko, A., Schramm, M. (2020). LC3-Associated Phagocytosis - The Highway to Hell for Phagocytosed Microbes. Semin. Cell Dev. Biol. 101, 68–76. doi: 10.1016/j.semcdb.2019.04.016
Herb, M., Schramm, M. (2021). Functions of ROS in Macrophages and Antimicrobial Immunity. Antioxidants (Basel Switzerland) 102, 313. doi: 10.3390/antiox10020313
Hooper, K. M., Jacquin, E., Li, T., Goodwin, J. M., Brumell, J. H., Durgan, J., et al. V-ATPase Is a Universal Regulator of LC3 Associated Phagocytosis and Non-Canonical Autophagy. bioRxiv (2021). doi: 10.1101/2021.05.20.444917
Hosokawa, N., Sasaki, T., Iemura, S., Natsume, T., Hara, T., Mizushima, N. (2009). Atg101, a Novel Mammalian Autophagy Protein Interacting With Atg13. Autophagy 57, 973–979. doi: 10.4161/auto.5.7.9296
Huang, J., Brumell, J. H. (2014). Bacteria-Autophagy Interplay: A Battle for Survival. Nat. Rev. Microbiol. 122, 101–114. doi: 10.1038/nrmicro3160
Huang, J., Canadien, V., Lam, G. Y., Steinberg, B. E., Dinauer, M. C., Magalhaes, M. A., et al. (2009). Activation of Antibacterial Autophagy by NADPH Oxidases. Proc. Natl. Acad. Sci. U.S.A. 10615, 6226–6231. doi: 10.1073/pnas.0811045106
Hubber, A., Kubori, T., Coban, C., Matsuzawa, T., Ogawa, M., Kawabata, T., et al. (2017). Bacterial Secretion System Skews the Fate of Legionella-Containing Vacuoles Towards LC3-Associated Phagocytosis. Sci. Rep. 71, 44795. doi: 10.1038/srep44795
Hurley, J. H., Young, L. N. (2017). Mechanisms of Autophagy Initiation. Annu. Rev. Biochem. 86, 225–244. doi: 10.1146/annurev-biochem-061516-044820
Hu, W., Zhang, L., Li, M. X., Shen, J., Liu, X. D., Xiao, Z. G., et al. (2019). Vitamin D3 Activates the Autolysosomal Degradation Function Against Helicobacter Pylori Through the PDIA3 Receptor in Gastric Epithelial Cells. Autophagy 154, 707–725. doi: 10.1080/15548627.2018.1557835
Ichimura, Y., Kirisako, T., Takao, T., Satomi, Y., Shimonishi, Y., Ishihara, N., et al. (2000). A Ubiquitin-Like System Mediates Protein Lipidation. Nature 4086811, 488–492. doi: 10.1038/35044114
Itakura, E., Kishi, C., Inoue, K., Mizushima, N. (2008). Beclin 1 Forms Two Distinct Phosphatidylinositol 3-Kinase Complexes With Mammalian Atg14 and UVRAG. Mol. Biol. Cell 1912, 5360–5372. doi: 10.1091/mbc.e08-01-0080
Jamwal, S. V., Mehrotra, P., Singh, A., Siddiqui, Z., Basu, A., Rao, K. V. (2016). Mycobacterial Escape From Macrophage Phagosomes to the Cytoplasm Represents an Alternate Adaptation Mechanism. Sci. Rep. 61, 23089. doi: 10.1038/srep23089
Johansen, T., Lamark, T. (2011). Selective Autophagy Mediated by Autophagic Adapter Proteins. Autophagy 73, 279–296. doi: 10.4161/auto.7.3.14487
Johansen, T., Lamark, T. (2020). Selective Autophagy: ATG8 Family Proteins, LIR Motifs and Cargo Receptors. J. Mol. Biol. 4321, 80–103. doi: 10.1016/j.jmb.2019.07.016
Joshi, A. D., Swanson, M. S. (2011). Secrets of a Successful Pathogen: Legionella Resistance to Progression Along the Autophagic Pathway. Front. Microbiol. 2, 138. doi: 10.3389/fmicb.2011.00138
Jung, C. H., Jun, C. B., Ro, S. H., Kim, Y. M., Otto, N. M., Cao, J., et al. (2009). ULK-Atg13-FIP200 Complexes Mediate mTOR Signaling to the Autophagy Machinery. Mol. Biol. Cell 207, 1992–2003. doi: 10.1091/mbc.e08-12-1249
Kabeya, Y., Mizushima, N., Ueno, T., Yamamoto, A., Kirisako, T., Noda, T., et al. (2000). LC3, a Mammalian Homologue of Yeast Apg8p, Is Localized in Autophagosome Membranes After Processing. EMBO J. 1921, 5720–5728. doi: 10.1093/emboj/19.21.5720
Kim, J. Y., Zhao, H., Martinez, J., Doggett, T. A., Kolesnikov, A. V., Tang, P. H., et al. (2013). Noncanonical Autophagy Promotes the Visual Cycle. Cell 1542, 365–376. doi: 10.1016/j.cell.2013.06.012
Kono, H., Rock, K. L. (2008). How Dying Cells Alert the Immune System to Danger. Nat. Rev. Immunol. 84, 279–289. doi: 10.1038/nri2215
Koster, S., Upadhyay, S., Chandra, P., Papavinasasundaram, K., Yang, G., Hassan, A., et al. (2017). Mycobacterium Tuberculosis Is Protected From NADPH Oxidase and LC3-Associated Phagocytosis by the LCP Protein CpsA. Proc. Natl. Acad. Sci. U.S.A. 11441, E8711–E8E20. doi: 10.1073/pnas.1707792114
Kourtzelis, I., Hajishengallis, G., Chavakis, T. (2020). Phagocytosis of Apoptotic Cells in Resolution of Inflammation. Front. Immunol. 11, 553. doi: 10.3389/fimmu.2020.00553
Koyama-Honda, I., Itakura, E., Fujiwara, T. K., Mizushima, N. (2013). Temporal Analysis of Recruitment of Mammalian ATG Proteins to the Autophagosome Formation Site. Autophagy 910, 1491–1499. doi: 10.4161/auto.25529
Kreibich, S., Emmenlauer, M., Fredlund, J., Ramo, P., Munz, C., Dehio, C., et al. (2015). Autophagy Proteins Promote Repair of Endosomal Membranes Damaged by the Salmonella Type Three Secretion System 1. Cell Host Microbe 185, 527–537. doi: 10.1016/j.chom.2015.10.015
Lamb, C. A., Yoshimori, T., Tooze, S. A. (2013). The Autophagosome: Origins Unknown, Biogenesis Complex. Nat. Rev. Mol. Cell Biol. 1412, 759–774. doi: 10.1038/nrm3696
Lemke, G. (2019). How Macrophages Deal With Death. Nat. Rev. Immunol. 199, 539–549. doi: 10.1038/s41577-019-0167-y
Levine, B., Mizushima, N., Virgin, H. W. (2011). Autophagy in Immunity and Inflammation. Nature 4697330, 323–335. doi: 10.1038/nature09782
Ligeon, L. A., Pena-Francesch, M., Vanoaica, L. D., Nunez, N. G., Talwar, D., Dick, T. P., et al. (2021). Oxidation Inhibits Autophagy Protein Deconjugation From Phagosomes to Sustain MHC Class II Restricted Antigen Presentation. Nat. Commun. 121, 1508. doi: 10.1038/s41467-021-21829-6
Lin, M. G., Hurley, J. H. (2016). Structure and Function of the ULK1 Complex in Autophagy. Curr. Opin. Cell Biol. 39, 61–68. doi: 10.1016/j.ceb.2016.02.010
Lystad, A. H., Carlsson, S. R., Simonsen, A. (2019). Toward the Function of Mammalian ATG12-ATG5-ATG16L1 Complex in Autophagy and Related Processes. Autophagy 158, 1485–1486. doi: 10.1080/15548627.2019.1618100
Ma, J., Becker, C., Lowell, C. A., Underhill, D. M. (2012). Dectin-1-Triggered Recruitment of Light Chain 3 Protein to Phagosomes Facilitates Major Histocompatibility Complex Class II Presentation of Fungal-Derived Antigens. J. Biol. Chem. 28741, 34149–34156. doi: 10.1074/jbc.M112.382812
Ma, J., Becker, C., Reyes, C., Underhill, D. M. (2014). Cutting Edge: FYCO1 Recruitment to Dectin-1 Phagosomes Is Accelerated by Light Chain 3 Protein and Regulates Phagosome Maturation and Reactive Oxygen Production. J. Immunol. 1924, 1356–1360. doi: 10.4049/jimmunol.1302835
Martens, S. (2016). No ATG8s, No Problem? How LC3/GABARAP Proteins Contribute to Autophagy. J. Cell Biol. 2156, 761–763. doi: 10.1083/jcb.201611116
Martinez, J., Almendinger, J., Oberst, A., Ness, R., Dillon, C. P., Fitzgerald, P., et al. (2011). Microtubule-Associated Protein 1 Light Chain 3 Alpha (LC3)-Associated Phagocytosis Is Required for the Efficient Clearance of Dead Cells. Proc. Natl. Acad. Sci. U.S.A. 10842, 17396–17401. doi: 10.1073/pnas.1113421108
Martinez, J., Malireddi, R. K., Lu, Q., Cunha, L. D., Pelletier, S., Gingras, S., et al. (2015). Molecular Characterization of LC3-Associated Phagocytosis Reveals Distinct Roles for Rubicon, NOX2 and Autophagy Proteins. Nat. Cell Biol. 177, 893–906. doi: 10.1038/ncb3192
Mercer, C. A., Kaliappan, A., Dennis, P. B. (2009). A Novel, Human Atg13 Binding Protein, Atg101, Interacts With ULK1 and Is Essential for Macroautophagy. Autophagy 55, 649–662. doi: 10.4161/auto.5.5.8249
Mercer, T. J., Ohashi, Y., Boeing, S., Jefferies, H. B. J., De Tito, S., Flynn, H., et al. (2021). Phosphoproteomic Identification of ULK Substrates Reveals VPS15-Dependent ULK/VPS34 Interplay in the Regulation of Autophagy. EMBO J. 4014, e105985. doi: 10.15252/embj.2020105985
Mesquita, F. S., Thomas, M., Sachse, M., Santos, A. J., Figueira, R., Holden, D. W. (2012). The Salmonella Deubiquitinase SseL Inhibits Selective Autophagy of Cytosolic Aggregates. PloS Pathog. 86, e1002743. doi: 10.1371/journal.ppat.1002743
Mitchell, G., Chen, C., Portnoy, D. A. (2016). Strategies Used by Bacteria to Grow in Macrophages. Microbiol. Spectr. 43. doi: 10.1128/microbiolspec.MCHD-0012-2015
Mitchell, G., Ge, L., Huang, Q., Chen, C., Kianian, S., Roberts, M. F., et al. (2015). Avoidance of Autophagy Mediated by PlcA or ActA Is Required for Listeria Monocytogenes Growth in Macrophages. Infect. Immun. 835, 2175–2184. doi: 10.1128/IAI.00110-15
Moldovan, A., Fraunholz, M. J. (2019). In or Out: Phagosomal Escape of Staphylococcus Aureus. Cell Microbiol. 213, e12997. doi: 10.1111/cmi.12997
Moreau, K., Lacas-Gervais, S., Fujita, N., Sebbane, F., Yoshimori, T., Simonet, M., et al. (2010). Autophagosomes can Support Yersinia Pseudotuberculosis Replication in Macrophages. Cell Microbiol. 128, 1108–1123. doi: 10.1111/j.1462-5822.2010.01456.x
Niu, H., Yamaguchi, M., Rikihisa, Y. (2008). Subversion of Cellular Autophagy by Anaplasma Phagocytophilum. Cell Microbiol. 103, 593–605. doi: 10.1111/j.1462-5822.2007.01068.x
Ogawa, M., Yoshimori, T., Suzuki, T., Sagara, H., Mizushima, N., Sasakawa, C. (2005). Escape of Intracellular Shigella From Autophagy. Sci. (New York NY) 3075710, 727–731. doi: 10.1126/science.1106036
Pao, K. C., Rape, M. (2019). Tug of War in the Xenophagy World. Trends Cell Biol. 2910, 767–769. doi: 10.1016/j.tcb.2019.08.001
Park, S., Buck, M. D., Desai, C., Zhang, X., Loginicheva, E., Martinez, J., et al. (2016). Autophagy Genes Enhance Murine Gammaherpesvirus 68 Reactivation From Latency by Preventing Virus-Induced Systemic Inflammation. Cell Host Microbe 191, 91–101. doi: 10.1016/j.chom.2015.12.010
Pengo, N., Agrotis, A., Prak, K., Jones, J., Ketteler, R. (2017). A Reversible Phospho-Switch Mediated by ULK1 Regulates the Activity of Autophagy Protease ATG4B. Nat. Commun. 81, 294. doi: 10.1038/s41467-017-00303-2
Pérez-Pérez, M. E., Lemaire, S. D., Crespo, J. L. (2016). Control of Autophagy in Chlamydomonas Is Mediated Through Redox-Dependent Inactivation of the ATG4 Protease. Plant Physiol. 1724, 2219–2234. doi: 10.1104/pp.16.01582
Queval, C. J., Brosch, R., Simeone, R. (2017). The Macrophage: A Disputed Fortress in the Battle Against Mycobacterium Tuberculosis. Front. Microbiol. 8, 2284. doi: 10.3389/fmicb.2017.02284
Rai, S., Arasteh, M., Jefferson, M., Pearson, T., Wang, Y., Zhang, W., et al. (2019). The ATG5-Binding and Coiled Coil Domains of ATG16L1 Maintain Autophagy and Tissue Homeostasis in Mice Independently of the WD Domain Required for LC3-Associated Phagocytosis. Autophagy 154, 599–612. doi: 10.1080/15548627.2018.1534507
Rao, S., Xu, T., Xia, Y., Zhang, H. (2020). Salmonella and S. Aureus Escape From the Clearance of Macrophages via Controlling TFEB. Front. Microbiol. 11, 573844. doi: 10.3389/fmicb.2020.573844
Ravenhill, B. J., Boyle, K. B., von Muhlinen, N., Ellison, C. J., Masson, G. R., Otten, E. G., et al. (2019). The Cargo Receptor NDP52 Initiates Selective Autophagy by Recruiting the ULK Complex to Cytosol-Invading Bacteria. Mol. Cell 742, 320–9 e6. doi: 10.1016/j.molcel.2019.01.041
Rhen, M. (2019). Salmonella and Reactive Oxygen Species: A Love-Hate Relationship. J. innate Immun. 113, 216–226. doi: 10.1159/000496370
Riebisch, A. K., Mühlen, S., Beer, Y. Y., Schmitz, I. (2021). Autophagy—A Story of Bacteria Interfering With the Host Cell Degradation Machinery. Pathogens 102, 110. doi: 10.3390/pathogens10020110
Romao, S., Gasser, N., Becker, A. C., Guhl, B., Bajagic, M., Vanoaica, D., et al. (2013). Autophagy Proteins Stabilize Pathogen-Containing Phagosomes for Prolonged MHC II Antigen Processing. J. Cell Biol. 2035, 757–766. doi: 10.1083/jcb.201308173
Rosales, C., Uribe-Querol, E. (2017). Phagocytosis: A Fundamental Process in Immunity. BioMed. Res. Int. 2017, 9042851. doi: 10.1155/2017/9042851
Russell, R. C., Tian, Y., Yuan, H., Park, H. W., Chang, Y. Y., Kim, J., et al. (2013). ULK1 Induces Autophagy by Phosphorylating Beclin-1 and Activating VPS34 Lipid Kinase. Nat. Cell Biol. 157, 741–750. doi: 10.1038/ncb2757
Sakoh-Nakatogawa, M., Matoba, K., Asai, E., Kirisako, H., Ishii, J., Noda, N. N., et al. (2013). Atg12-Atg5 Conjugate Enhances E2 Activity of Atg3 by Rearranging Its Catalytic Site. Nat. Struct. Mol. Biol. 204, 433–439. doi: 10.1038/nsmb.2527
Sanjuan, M. A., Dillon, C. P., Tait, S. W., Moshiach, S., Dorsey, F., Connell, S., et al. (2007). Toll-Like Receptor Signalling in Macrophages Links the Autophagy Pathway to Phagocytosis. Nature 4507173, 1253–1257. doi: 10.1038/nature06421
Schaaf, M. B., Keulers, T. G., Vooijs, M. A., Rouschop, K. M. (2016). LC3/GABARAP Family Proteins: Autophagy-(Un)Related Functions. FASEB J. 3012, 3961–3978. doi: 10.1096/fj.201600698R
Scherz-Shouval, R., Shvets, E., Fass, E., Shorer, H., Gil, L., Elazar, Z. (2007). Reactive Oxygen Species are Essential for Autophagy and Specifically Regulate the Activity of Atg4. EMBO J. 267, 1749–1760. doi: 10.1038/sj.emboj.7601623
Schramm, M., Wiegmann, K., Schramm, S., Gluschko, A., Herb, M., Utermohlen, O., et al. (2014). Riboflavin (Vitamin B2) Deficiency Impairs NADPH Oxidase 2 (Nox2) Priming and Defense Against Listeria Monocytogenes. Eur. J. Immunol. 443, 728–741. doi: 10.1002/eji.201343940
Sharma, V., Verma, S., Seranova, E., Sarkar, S., Kumar, D. (2018). Selective Autophagy and Xenophagy in Infection and Disease. Front. Cell Dev. Biol. 6, 147. doi: 10.3389/fcell.2018.00147
Shi, X., Yokom, A. L., Wang, C., Young, L. N., Youle, R. J., Hurley, J. H. (2020). ULK Complex Organization in Autophagy by a C-Shaped FIP200 N-Terminal Domain Dimer. J. Cell Biol. 2197. doi: 10.1083/jcb.201911047
Siqueira, M. D. S., Ribeiro, R. M., Travassos, L. H. (2018). Autophagy and Its Interaction With Intracellular Bacterial Pathogens. Front. Immunol. 9, 935. doi: 10.3389/fimmu.2018.00935
Slauch, J. M. (2011). How Does the Oxidative Burst of Macrophages Kill Bacteria? Still an Open Question. Mol. Microbiol. 803, 580–583. doi: 10.1111/j.1365-2958.2011.07612.x
Slobodkin, M. R., Elazar, Z. (2013). The Atg8 Family: Multifunctional Ubiquitin-Like Key Regulators of Autophagy. Essays Biochem. 55, 51–64. doi: 10.1042/bse0550051
Starr, T., Child, R., Wehrly, T. D., Hansen, B., Hwang, S., Lopez-Otin, C., et al. (2012). Selective Subversion of Autophagy Complexes Facilitates Completion of the Brucella Intracellular Cycle. Cell Host Microbe 111, 33–45. doi: 10.1016/j.chom.2011.12.002
Sun-Wada, G. H., Tabata, H., Kawamura, N., Aoyama, M., Wada, Y. (2009). Direct Recruitment of H+-ATPase From Lysosomes for Phagosomal Acidification. J. Cell Sci. 122 (Pt 14), 2504–2513. doi: 10.1242/jcs.050443
Suzanne, M., Steller, H. (2013). Shaping Organisms With Apoptosis. Cell Death differentiation 205, 669–675. doi: 10.1038/cdd.2013.11
Suzuki, K., Kubota, Y., Sekito, T., Ohsumi, Y. (2007). Hierarchy of Atg Proteins in Pre-Autophagosomal Structure Organization. Genes to cells: devoted to Mol. Cell. Mech. 122, 209–218. doi: 10.1111/j.1365-2443.2007.01050.x
Tam, J. M., Mansour, M. K., Khan, N. S., Seward, M., Puranam, S., Tanne, A., et al. (2014). Dectin-1-Dependent LC3 Recruitment to Phagosomes Enhances Fungicidal Activity in Macrophages. J. Infect. Dis. 21011, 1844–1854. doi: 10.1093/infdis/jiu290
Thurston, T. L., Ryzhakov, G., Bloor, S., von Muhlinen, N., Randow, F. (2009). The TBK1 Adaptor and Autophagy Receptor NDP52 Restricts the Proliferation of Ubiquitin-Coated Bacteria. Nat. Immunol. 1011, 1215–1221. doi: 10.1038/ni.1800
Thurston, T. L., Wandel, M. P., von Muhlinen, N., Foeglein, A., Randow, F. (2012). Galectin 8 Targets Damaged Vesicles for Autophagy to Defend Cells Against Bacterial Invasion. Nature 4827385, 414–418. doi: 10.1038/nature10744
Travassos, L. H., Carneiro, L. A., Ramjeet, M., Hussey, S., Kim, Y. G., Magalhaes, J. G., et al. (2010). Nod1 and Nod2 Direct Autophagy by Recruiting ATG16L1 to the Plasma Membrane at the Site of Bacterial Entry. Nat. Immunol. 111, 55–62. doi: 10.1038/ni.1823
Uribe-Querol, E., Rosales, C. (2020). Phagocytosis: Our Current Understanding of a Universal Biological Process. Front. Immunol. 111066, 1066. doi: 10.3389/fimmu.2020.01066
von Muhlinen, N., Akutsu, M., Ravenhill, B. J., Foeglein, A., Bloor, S., Rutherford, T. J., et al. (2012). LC3C, Bound Selectively by a Noncanonical LIR Motif in NDP52, Is Required for Antibacterial Autophagy. Mol. Cell 483, 329–342. doi: 10.1016/j.molcel.2012.08.024
Wang, Y. H., Wu, J. J., Lei, H. Y. (2009). The Autophagic Induction in Helicobacter Pylori-Infected Macrophage. Exp. Biol. Med. (Maywood NJ) 2342, 171–180. doi: 10.3181/0808-RM-252
Weiss, G., Schaible, U. E. (2015). Macrophage Defense Mechanisms Against Intracellular Bacteria. Immunol. Rev. 2641, 182–203. doi: 10.1111/imr.12266
Westman, J., Grinstein, S. (2021). Determinants of Phagosomal pH During Host-Pathogen Interactions. Front. Cell Dev. Biol. 8. doi: 10.3389/fcell.2020.624958
Wild, P., Farhan, H., McEwan, D. G., Wagner, S., Rogov, V. V., Brady, N. R., et al. (2011). Phosphorylation of the Autophagy Receptor Optineurin Restricts Salmonella Growth. Sci. (New York NY) 3336039, 228–233. doi: 10.1126/science.1205405
Wink, D. A., Hines, H. B., Cheng, R. Y., Switzer, C. H., Flores-Santana, W., Vitek, M. P., et al. (2011). Nitric Oxide and Redox Mechanisms in the Immune Response. J. Leukoc. Biol. 896, 873–891. doi: 10.1189/jlb.1010550
Xu, Y., Zhou, P., Cheng, S., Lu, Q., Nowak, K., Hopp, A. K., et al. (2019). A Bacterial Effector Reveals the V-ATPase-ATG16L1 Axis That Initiates Xenophagy. Cell 1783, 552–66 e20. doi: 10.1016/j.cell.2019.06.007
Yang, C. S., Lee, J. S., Rodgers, M., Min, C. K., Lee, J. Y., Kim, H. J., et al. (2012). Autophagy Protein Rubicon Mediates Phagocytic NADPH Oxidase Activation in Response to Microbial Infection or TLR Stimulation. Cell Host Microbe 113, 264–276. doi: 10.1016/j.chom.2012.01.018
Yla-Anttila, P., Vihinen, H., Jokitalo, E., Eskelinen, E. L. (2009). 3D Tomography Reveals Connections Between the Phagophore and Endoplasmic Reticulum. Autophagy 58, 1180–1185. doi: 10.4161/auto.5.8.10274
Yoshikawa, Y., Ogawa, M., Hain, T., Yoshida, M., Fukumatsu, M., Kim, M., et al. (2009). Listeria Monocytogenes ActA-Mediated Escape From Autophagic Recognition. Nat. Cell Biol. 1110, 1233–1240. doi: 10.1038/ncb1967
Young, L. N., Goerdeler, F., Hurley, J. H. (2019). Structural Pathway for Allosteric Activation of the Autophagic PI 3-Kinase Complex I. Proc. Natl. Acad. Sci. U.S.A. 11643, 21508–21513. doi: 10.1073/pnas.1911612116
Yu, X., Long, Y. C., Shen, H. M. (2015). Differential Regulatory Functions of Three Classes of Phosphatidylinositol and Phosphoinositide 3-Kinases in Autophagy. Autophagy 1110, 1711–1728. doi: 10.1080/15548627.2015.1043076
Zheng, Y. T., Shahnazari, S., Brech, A., Lamark, T., Johansen, T., Brumell, J. H. (2009). The Adaptor Protein P62/SQSTM1 Targets Invading Bacteria to the Autophagy Pathway. J. Immunol. 1839, 5909–5916. doi: 10.4049/jimmunol.0900441
Keywords: non-canonical autophagy, macrophages, ULK complex, pore-forming toxins, macroautophagy, xenophagy, LC3-associated phagocytosis, PINCA
Citation: Herb M, Gluschko A, Farid A and Krönke M (2022) When the Phagosome Gets Leaky: Pore-Forming Toxin-Induced Non-Canonical Autophagy (PINCA). Front. Cell. Infect. Microbiol. 12:834321. doi: 10.3389/fcimb.2022.834321
Received: 13 December 2021; Accepted: 14 February 2022;
Published: 17 March 2022.
Edited by:
Hua Niu, Affiliated Hospital of Guilin Medical University, ChinaReviewed by:
Ann M. Wehman, University of Denver, United StatesCopyright © 2022 Herb, Gluschko, Farid and Krönke. This is an open-access article distributed under the terms of the Creative Commons Attribution License (CC BY). The use, distribution or reproduction in other forums is permitted, provided the original author(s) and the copyright owner(s) are credited and that the original publication in this journal is cited, in accordance with accepted academic practice. No use, distribution or reproduction is permitted which does not comply with these terms.
*Correspondence: Marc Herb, bWFyYy5oZXJiQHVrLWtvZWxuLmRl
†These authors have contributed equally to this work