- 1Armauer Hansen Research Institute, Malaria and Neglected Tropical Disease Directorate, Addis Ababa, Ethiopia
- 2Department of Medical Microbiology, Radboud University Medical Center, Nijmegen, Netherlands
- 3Department of Biology, College of Natural and Computational Sciences, Wollo University, Dessie, Ethiopia
- 4Division of Malaria Research, Proteo-Science Center, Ehime University, Matsuyama, Ehime, Japan
- 5Laboratory of Malaria Immunology and Vaccinology, Division of Intramural Research, National Institute of Allergy and Infectious Disease, National Institutes of Health, Bethesda, MD, United States
- 6London School of Hygiene & Tropical Medicine, London, United Kingdom
- 7Malaria Molecular Epidemiology Unit, Pasteur Institute of Cambodia, Phnom Penh, Cambodia
Naturally acquired antibodies may reduce the transmission of Plasmodium gametocytes to mosquitoes. Here, we investigated associations between antibody prevalence and P. vivax infectivity to mosquitoes. A total of 368 microscopy confirmed P. vivax symptomatic patients were passively recruited from health centers in Ethiopia and supplemented with 56 observations from asymptomatic P. vivax parasite carriers. Direct membrane feeding assays (DMFA) were performed to assess mosquito infectivity; for selected feeds these experiments were also performed after replacing autologous plasma with malaria naïve control serum (n=61). The prevalence of antibodies against 6 sexual stage antigens (Pvs47, Pvs48/45, Pvs230, PvsHAP2, Pvs25 and PvCelTOS) and an array of asexual antigens was determined by ELISA and multiplexed bead-based assays. Gametocyte (ρ< 0.42; p = 0.0001) and parasite (ρ = 0.21; p = 0.0001) densities were positively associated with mosquito infection rates. Antibodies against Pvs47, Pvs230 and Pvs25 were associated with 23 and 34% reductions in mosquito infection rates (p<0.0001), respectively. Individuals who showed evidence of transmission blockade in serum-replacement DMFAs (n=8) were significantly more likely to have PvsHAP2 or Pvs47 antibodies. Further studies may demonstrate causality for the observed associations, improve our understanding of the natural transmission of P. vivax and support vaccine development.
1 Introduction
The significant reduction in malaria burden in the first decade of this millennium has plateaued since 2015 and even reverted in some settings in recent years (WHO, 2015). Current intervention strategies appear insufficient to achieve malaria elimination in the majority of endemic countries (Griffin et al., 2010). Whilst most malaria-attributed deaths are due to Plasmodium falciparum, Plasmodium vivax also has a high clinical burden with an estimated 4.5 million cases in 2020 (WHO, 2015). P. vivax appears particularly hard to eliminate (Price et al., 2020), principally due to its ability to form dormant liver stages that can result in relapsing infections unless treated with an effective radical cure. Another challenge in controlling and eliminating P. vivax infections is the rapid formation of gametocytes, the parasite life stage responsible for onward transmission to mosquitoes. Once taken up by blood-feeding Anopheles mosquitoes, gametocytes activate to become male and female gametes that fuse to form a motile ookinete. This ookinete penetrates the mosquito gut to develop into an oocyst that releases sporozoites that ultimately render the mosquito infectious upon its next bite. Whilst gametocyte maturation is a long process in P. falciparum, infectious P. vivax gametocytes appear in the bloodstream within 48 hours of blood stage infection (Bousema and Drakeley, 2011). The strong association between asexual parasite and gametocyte density (Koepfli et al., 2015) make clinical P. vivax malaria cases highly infectious to mosquitoes at the moment of clinical presentation (Martins-Campos et al., 2018; Tadesse et al., 2018). Given that P. vivax parasites are capable of infecting a wide variety of Anopheles mosquitoes (Rios-Velásquez et al., 2013; Araújo et al., 2020), it is unsurprising that P. vivax transmission is highly efficient in many settings.
Naturally acquired human immune responses can reduce or fully prevent the transmission of Plasmodium parasites from humans to mosquitoes (recently reviewed in (de Jong et al., 2020)). For P. vivax, the first empirical evidence for naturally acquired transmission blocking activity (TBA) (Koepfli et al., 2015) came from studies conducted in the 1980s and 1990s in Sri Lanka (Mendis et al., 1987; Peiris et al., 1988; Gamage-Mendis et al., 1992). In these studies, investigators fed mosquitoes with blood from acutely infected Sri Lankan individuals. In paired feeding experiments, gametocyte infectivity was compared between a condition where the patient’s autologous plasma was present in the bloodmeal versus a condition where this plasma was replaced with malaria naïve control serum. In most of these paired experiments, transmission was reduced in the presence of autologous plasma (indicative of plasma derived TBA) although in some experiments the autologous plasma had the opposite effect and thus enhanced transmission (Peiris et al., 1988; Gamage-Mendis et al., 1992). In supporting experiments, indirect immunofluorescence tests showed that sera with TBA were more reactive to gamete surface antigens compared to sera without this functional activity. Moreover, purified immunoglobulins from these suppressive sera blocked transmission when added to a gametocytaemic bloodmeal, confirming this TBA to be antibody-mediated (Peiris et al., 1988). Subsequent studies in Mexico (Ramsey et al., 1994), and Colombia (Arévalo-Herrera et al., 2005) similarly demonstrated the existence of naturally acquired P. vivax TBA. However, the antibody specificity or strength of the associations remain largely undescribed. Several P. vivax gametocyte antigens have been developed as transmission blocking vaccine candidates. Antibody responses that are elicited by these proteins can reduce P. vivax transmission, as demonstrated by ookinete culture experiments or by mosquito feeding experiments where serum from vaccinated animals was added to gametocytes from naturally infected malaria-positive blood donors and offered to mosquitoes. These experiments have confirmed the potency of several pre-fertilization antigens including Pvs48/45 (Tachibana et al., 2015; Arévalo-Herrera et al., 2022), Pvs230 (Tachibana et al., 2012), Pvs47 (Tachibana et al., 2015) and PvHAP2 (Qiu et al., 2020) and post-fertilization antigens Pvs25 and Pvs28 (Hisaeda et al., 2000). Lastly, P. vivax Cell-traversal protein for ookinetes and sporozoites (PvCelTOS) (Kariu et al., 2006; Jimah et al., 2016; Kumar et al., 2022) was identified as potential inducer of TBA (Mehrizi et al., 2017). Recognition of these recombinant proteins has so far not been systematically studied in malaria-endemic populations or related to P. vivax transmission efficiency.
Here, we therefore investigated the relationship between the infectivity of P. vivax infections to locally reared An. arabiensis mosquitoes and naturally acquired antibodies against P. vivax gametocyte antigens in symptomatic and asymptomatic infections in Ethiopia.
2 Methods
2.1 Study design and population
This study was conducted in Adama district and Metehara town of Oromia Regional State and Arba Minch in Southern Region, Ethiopia. Adama district is characterized by low and seasonal malaria transmission that primarily occurs during the long (July to September) and short (May to June) rainy seasons (Golassa and White, 2017). Metehara town has low perennial malaria transmission with peaks in incidence from September to November and March to May. Arba Minch is characterized by moderately intense perennial malaria transmission. P. falciparum and P. vivax are co-endemic in all study sites where Anopheles arabiensis is a primary malaria vector (Tekeste et al., 2013; Tadesse et al., 2018; Abossie et al., 2020).
Across sites, microscopy confirmed symptomatic P. vivax patients were recruited at health centers from December 2017 to March 2022 (Table 1). Demographic data was collected from patients or their guardians (if minors) at the moment of presentation. Patients were included in the study if they had microscopy-confirmed P. vivax infections, had symptoms suggestive of malaria (defined as axillary temperature ≥37.5°C or history of fever, alone or in combination with nausea, chills and headaches) and were ≥2 years of age. Patients were excluded if they had a chronic and/or an acute illness that required immediate clinical care or bleeding disorders. Hemoglobin concentration was measured by HemoCue photometer (HemoCue 201+, Angelholm, Sweden).
Observations from clinical malaria cases were complemented with observations from asymptomatic infections. These comprised samples from qPCR confirmed P. vivax infections collected during the same study period from asymptomatic individuals who were enrolled in a longitudinal observational study on the dynamics of asymptomatic infections in Adama district (Hailemeskel, Tebeje et al. in preparation). Samples from these individuals were included in the current analysis to ensure coverage of a broad range of transmissible and non-transmissible gametocyte densities to allow more precise fitting of the associations between parasite and gametocyte densities and mosquito infection rates. For asymptomatic parasite carriers, afebrile community members were eligible if they were above the age of 2 years and positive for P. vivax parasites by qPCR, without symptoms suggestive of malaria in the past 48 hours and with no evidence of chronic and/or acute illness. Venous blood samples were collected every 14 days for 2-months and used in mosquito feeding assays and for plasma collection. For the current study, we randomly selected samples from this cohort without the intention to describe (longitudinal) patterns in parasite carriage but to obtain an informative population of low parasite and gametocyte densities for curve-fitting.
2.2 Ethics statement
All participants or their guardians (if minor) provided written informed consents to participate in the study. The study protocol was approved by the Ethics Review Committees of the Armauer Hansen Research Institute (AHRI) (protocol number: PO32/18 and PO35/17), the Ethics committee of the London School of Hygiene and Tropical Medicine (LSHTM Ethics ref: 15811), and the National Research Ethics Review Committee (SHE/S.M./14.4/708/19) at the Ministry of Science and Higher Education of The Federal Democratic Republic of Ethiopia.
2.3 Study procedures
2.3.1 Blood sampling
Venous blood was collected from each participant for mosquito feeding, molecular assays and serology. Three mL of blood was collected in either EDTA (BD K2E EDTA Vacutainers) or serum tubes (BD Vacutainer Plus Serum) for nucleic acid collection and plasma/serum collection. An additional 2mL was collected in Heparin tubes (BD Lithium Heparin Vacutainers) for mosquito feeding experiments (to measure mosquito infectivity) since other anticoagulants interfere with transmission efficiency (Graumans et al., 2022). Plasma (1:1 in 0.05% NaAz) and serum were stored at -70°C for serological investigations.
2.3.2 Parasite quantification
Asexual parasites and gametocytes were detected and quantified by microscopy (WHO, 2010). For quantification of gametocytes with reverse transcriptase quantitative PCR (RT-qPCR), total nucleic acids were extracted from 100µL whole blood stored in 500µL RNAProtect (Qiagen) and extracted by MagNAPure LC automatic extractor (Roche applied Sciences). RT-qPCR targeted Pvs25 mRNA, an established marker of female gametocytes (Wampfler et al., 2013; Tadesse et al., 2018). All primer and probe sequences and combinations are included in supplementary file (Table S3).
2.3.3 Infectivity assessment
Mosquito membrane feeding assays were performed as described previously (Ouédraogo et al., 2013; Tadesse et al., 2018) to assess infectivity to locally reared An. arabiensis mosquitoes. Briefly, a heparinized whole blood sample was fed to ~ 40 (4 - 7 days old) female An. arabiensis mosquitoes per cup (three cups with a total of 120 mosquitoes per experiment) using water jacketed glass feeders (0.3mL capacity) maintained on a water bath to keep the temperature in the feeders at 37°C. Mosquitoes were starved for ~12 hours prior to feeding. For the serum replacement mosquito feedings, heparinized whole blood (~833µL) was centrifuged at 1800 × g for 5 minutes in a pre-warmed (37°C) centrifuge to collect autologous plasma (~500µL). Pre-warmed (37°C) ~500µL naïve AB European serum (Sanquin, Nijmegen, the Netherlands) was used to replace the autologous plasma. Fully fed mosquitoes were maintained on 10% sucrose solution (g/mL) at 26℃ ± 2 room temperature and 60% ± 10 humidity post feeding. Mosquitoes (30 per experiment) were dissected on day 7 post feeding, and midguts were stained with 1% mercurochrome for microscopic detection and quantification of oocysts.
2.3.4 Serological analyses
Antibody levels against P. vivax antigens, Pvs25, Pvs48/45, Pvs47, Pvs230 and PvCelTOS were assessed by Enzyme Linked Immunosorbent Assay (ELISA). Pvs25, Pvs48/45, Pvs47 and Pvs230 were expressed using a wheat germ cell-free (WGCF) system (CellFree Sciences, Matsuyama, Japan) (Liu et al., 2022); an additional Pvs230 was expressed in Pichia pastoris (Pvs230D1M (Tentokam et al., 2019)); and the sporozoite/ookinete antigen PvCelTOS was expressed in E. coli (Jimah et al., 2016; Jimah et al., 2017a; Jimah et al., 2017b; Kumar et al., 2022). ELISA plates were coated with 1µg/mL (in PBS) of antigen overnight at 4°C. After thorough washing with home-made PBS-Tween (0.05%), plates were blocked with 5% skimmed milk in 0.05% PBS-Tween for 1 hour at room temperature (RT). Plates were then washed. Diluted test samples/controls in 1% skimmed milk (in 0.05% PBS-Tween) were added to the plates and incubated for 2 hours at RT. After a washing step, Goat anti-human IgG-HRP (1:50,000) (Pierce 31412) in PBS-Tween (0.05%) was added and plates were incubated at RT for 2 hours. Following a final washing step, a colorizing substrate TMB (K-Blue substrate, Neogen, Sigma), was added and incubated for ~20 minutes. After ~20 minutes of incubation, a stopping solution of 0.2M Sulphuric acid (H2SO4,Merck KGaA cat 100731) was added to stop the reaction. Plates were immediately read by plate reader (Bio-Rad, iMark microplate reader) at 450 nm. OD values were used as measure of antibody density; antibody prevalence was assessed by a mixture model, taking the mean plus two standard deviations from the negative population as cut-off for positivity (Wu et al., 2020). A more conservative approach with three standard deviations is sometimes used (Stewart et al., 2009) but more appropriate for larger sample sizes. The availability of WGCF proteins was a limiting factor and not all samples could be processed by ELISA; samples with serum replacement observations were prioritized for a complete dataset.
Alongside ELISAs, we tested a panel of 7 P. vivax antigens from different life stages in a bead based multiplex immunoassay for comparison; one pre-erythrocytic stage (Circumsporozoite protein [VK210 CSP]), 5 asexual blood stage (Merozoite surface protein 1-19 [MSP1-19], Apical membrane antigen 1 [AMA1], Duffy binding protein [DBP RII], Reticulocyte binding protein [RBP 2b], and Erythrocyte binding protein [EBPII]), and one sexual stage (HAP2). Antibody responses were quantified using a Luminex MAGPIX© suspension bead array, as described previously (Wu et al., 2019). Briefly, plasma/serum samples were assayed at a dilution of 1:400. Secondary antibody was an R-phycoerythrin conjugated goat anti-human IgG (Jackson Immuno Research, PA, USA; 109-116-098) diluted to 1:200. Data are presented as background adjusted median fluorescence intensities (MFI).
2.4 Data analyses
Statistical analyses were performed using STATA (version 14.2, StataCorp., TX, USA) and R (version 4.1.1). Proportions were compared using McNemar’s test for paired observations, and Pearson χ2 test or Fisher exact test for independent observations. Spearman rank correlation coefficient (ρ) was used to assess associations between continuous variables. Continuous variables were presented as medians and interquartile ranges (IQRs). TBA was calculated as percent inhibition in oocyst density in naïve sera feeds compared to whole blood feeds (Lensen et al., 1996). A cut-off for antibody positivity was determined by first using a mixture model to distinguish two Gaussian distributions. The cut-off was determined as the estimated 97.5th percentile of the underlying Gaussian distribution for the negative antibodies (i.e. 2 standard deviations above the mean of the negative population). Logistic and Linear mixed effects regression models were used for multivariate analyses. Analysis with p<0.05 were considered statistically significant.
3 Results
3.1 Participant characteristics
In total, 368 symptomatic patients with a median age of 19 years (interquartile range (IQR), 13 – 28 years) were enrolled in the study between December 2017 and March 2022 (Table 1). Of these participants 47.8% (174/364) had an active fever and 0.6% (2/332) had hemoglobin levels <8g/dL. In these patients, the median asexual parasite density by microscopy was 4752.0 parasites/µL (IQR, 1547.0 - 10706.0) and 36.4% (134/368) had microscopically detected gametocytes. By Pvs25 RT-qPCR, P. vivax gametocytes were detected in 76.1% (280/368) of samples with median Pvs25 transcript numbers of 22245.9 copies/µL (IQR, 3770.4 - 70980.8). These data from clinical malaria patients, who typically harbor high parasite and gametocyte densities (McKenzie et al., 2006; Pukrittayakamee et al., 2008), were supplemented with a total of 56 follow-up observations from 24 asymptomatic parasite carriers who were for the most part microscopy negative for malaria parasites but qPCR positive for P. vivax and thus carried very low density infections (Nguyen et al., 2018) (Table 1). Asymptomatic parasite carriers had a median age of 14 years (IQR, 9 - 21.5). Microscopically quantified asexual parasites and molecularly quantified gametocyte densities differed markedly between symptomatic and asymptomatic populations (Figure 1A). When combining observations from both symptomatic and asymptomatic populations, gametocyte density was positively associated with parasite density (Figure 1B, ρ = 0.43; p < 0.0001).
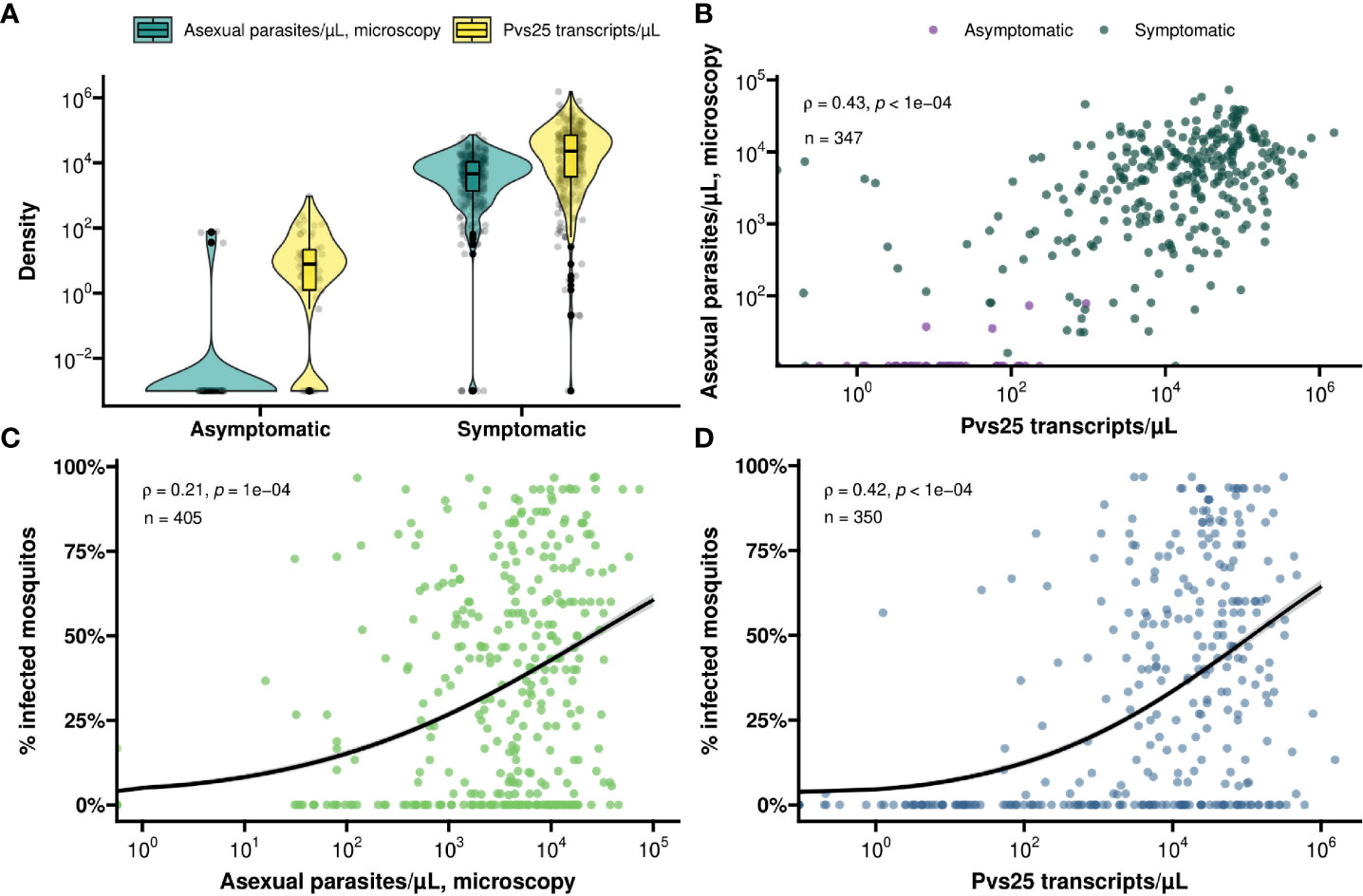
Figure 1 (A) Violin and box plot for the distribution of molecularly determined gametocyte density (yellow) and microscopically detected asexual parasite density (turquoise) by symptom status. (B) A scatter plot for the association between asexual parasite density and gametocyte density by symptom status (asymptomatic – violet, symptomatic – green). Spearman’s ρ = 0.43 indicating a moderate positive monotonic association (p<0.0001). (C) Scatter plot showing the association between asexual parasite density and mosquito infectivity. Spearman’s ρ = 0.21 indicating a weak positive monotonic association (p<0.0001). The black line shows the fit from a logistic regression model and the grey shading shows the 95% confidence interval. (D) Scatter plot showing the association between gametocyte density and mosquito infectivity. Spearman’s ρ = 0.42 indicating a moderative positive monotonic association (p<0.0001). The black line shows the fit from a logistic regression model and the grey shading shows the 95% confidence interval.
3.2 Mosquito infection rates are associated with parasite and gametocyte density.
For all clinical patients and asymptomatic parasite carriers, whole blood was offered to locally reared An. arabiensis mosquitoes that were examined 7 days later for infection status. For 61 clinical patients recruited between March 2020 and March 2022, these mosquito feeding experiments were also conducted after replacing autologous plasma with malaria-naïve serum. Asexual parasite density was positively associated with the proportion of mosquitoes that became infected when feeding on whole blood of parasite carriers (Figure 1C); this association was stronger for molecularly quantified gametocyte density (Figure 1D). Oocyst prevalence, or the proportion of infected mosquitoes, was strongly positively associated with mean oocyst density (ρ = 0.63; p < 0.0001) (Figure 2A).
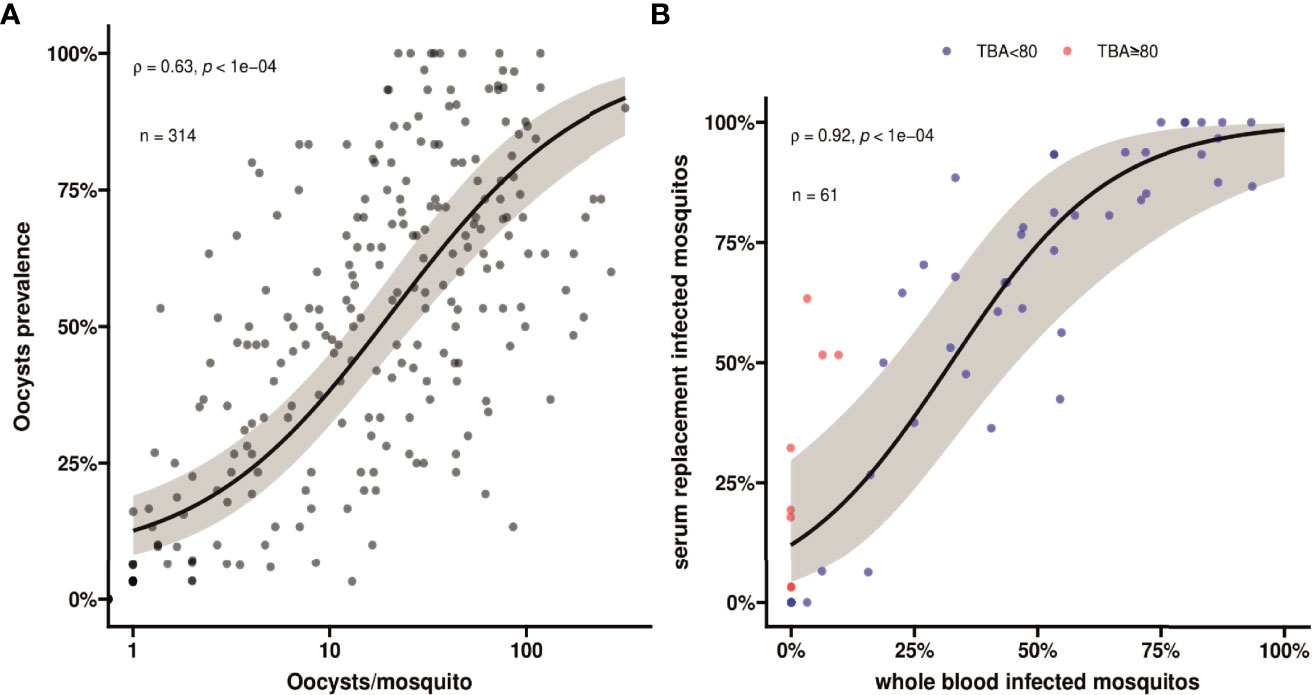
Figure 2 (A) A scatter plot showing association between oocyst prevalence, or mosquito infection rates, and mean oocyst per mosquito. Spearman’s ρ =0.63 indicating a moderate positive monotonic association p<0.0001. The black line shows the fit from a logistic regression model and the grey shading shows the 95% confidence interval. (B) A scatter plot indicating the association in mosquito infection rates between whole blood and serum replacement feeds. Observations with TBA<80% are indicated in blue and TBA≥80% are indicated in red. Spearman’s ρ = 0.92 indicates a strong positive monotonic association (p<0.0001). The black line shows the fit from a logistic regression model and the grey shading shows the 95% confidence interval. The plot also indicates that infectivity in the serum replacement condition (median 60.6%, IQR 6.3% - 85.2%) was consistently higher than in the whole blood condition (median 33.3%; IQR 0% - 57.6%) (p<0.0001). This value is different, but not contradicting, the comparison in the main text where we calculated the pairwise differences and report the median and IQR for the pairwise differences.
Despite these statistically significant associations, many individuals with relatively high gametocyte densities did not infect mosquitoes. For the subset of participants whose blood was used in serum replacement experiments (n=61), we observed a strong positive association in mosquito infection rates between the whole blood and serum replacement feeds (Figure 2B; ρ = 0.92, p<0.0001). We observed higher mosquito infection rates for the control serum condition with a median increase of 13.2% (IQR, 0% - 25.0%) infected mosquitoes. We expressed the lower mosquito infection rates in the autologous plasma condition relative to that in the naïve serum condition as transmission blocking activity (TBA). This TBA ranged from -80% (enhancement) to 100% (complete blockade). For those showing higher infection rates in whole blood conditions (n=5), this ‘transmission enhancement’ was mostly very weak (3.2 - 12.1%). Only 1 individual showed more than 80% enhancement with the proportion of infected mosquitoes being 15.6% for whole blood and 6.3% for serum replacement conditions. In contrast, 13.1% (8/61) of experiments showed greatly increased infection rates following serum replacement (>80% higher infection rates and thus TBA >80%). TBA estimates for five experiments were not interpretable since infection rates were zero in the whole blood and serum replacement conditions.
3.3 Transmission efficiency is associated with gametocyte immune responses
To test a possible impact of anti-gametocyte immune responses on transmission efficiency, we determined antibody responses to sexual stage antigens Pvs47, Pvs48/45, Pvs230 (two variants), PvCelTOS and Pvs25 by ELISA. These assessments were done for 224 individuals, including all those participating in serum replacement mosquito feeding experiments. Due to antigen scarcity, not more samples were completed by ELISA. Additional antigens, including sexual-stage antigen PvHAP2, were measured by bead-based multiplex assay. Antibody positivity was defined based on a mixture model, assuming an antibody positive and antibody negative population for each antigen within the study population. The optical density values in ELISA, reflective of antibody densities, were strongly correlated between most of the antigens (Figure 3. For instance, responses to the two Pvs230 variants were strongly correlated (ρ = 0.68, p<0.0001), as were responses to Pvs230 and Pvs25 (ρ = 0.63, p<0.0001). Among individuals with microscopy-positive infections, antibody prevalence was weakly associated with a lower parasite density at the time of sampling for PvDPB-RII (p = 0.06) but not for any of the other antigens (Table S1)
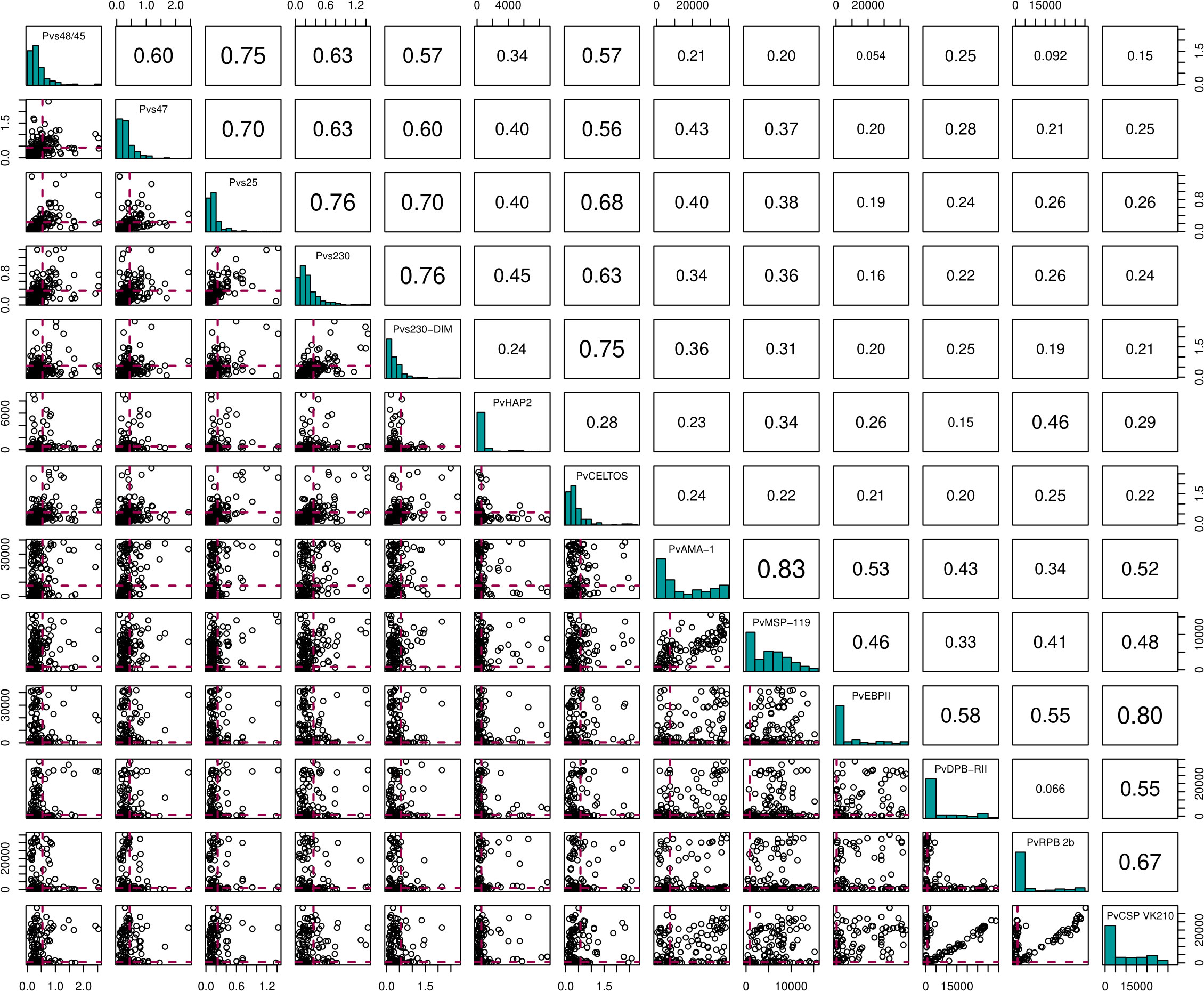
Figure 3 Correlation matrix plot for the 13 different antigens. Here the diagonal shows the histogram distribution for each of the antigens. The triangle above the diagonal shows the Spearman's Correlation Coefficient for every pair of antigens on the diagonal. The bottom triangle shows the scatter plots for every pair of antigens on the diagonal. Here, the red dashed lines indicate the cut-offs for antibody positivity.
Individuals with >80% TBA were more likely to be antibody positive for most gametocyte antigens (Figure 4), although this difference only reached statistical significance for Pvs47 and PvHAP2 (Table S2). We did not observe any evidence of associations with >80% TBA for any of the asexual antigens (Figure 4, Table S2). We next examined whether antibody prevalence was also associated with transmission efficiency in the larger dataset where whole blood samples were offered to mosquitoes and gametocytes were quantified by molecular methods. We thus determined whether the proportion infected mosquitoes for a given gametocyte density was lower in the presence of gametocyte antibodies. The presence of antibodies against Pvs47 was associated with a relative reduction in mosquito infection rates of 34% (p<0.0001). Similarly, Pvs230 and Pvs25 antibody prevalence were associated with statistically significant relative reductions of 23 and 34%, respectively; for Pvs48/45 antibodies a smaller and non-significant reduction was observed (Figure 5). For PvCelTOS and asexual stage antigens, highly heterogeneous effects were observed. The presence of antibodies against CSP and RBP-2b was associated with 61% and 20% increases in mosquito infection rates, respectively; while antibodies against AMA-1 were associated with a 26% decrease in mosquito infection rates (p<0.0001) (Figure S1).
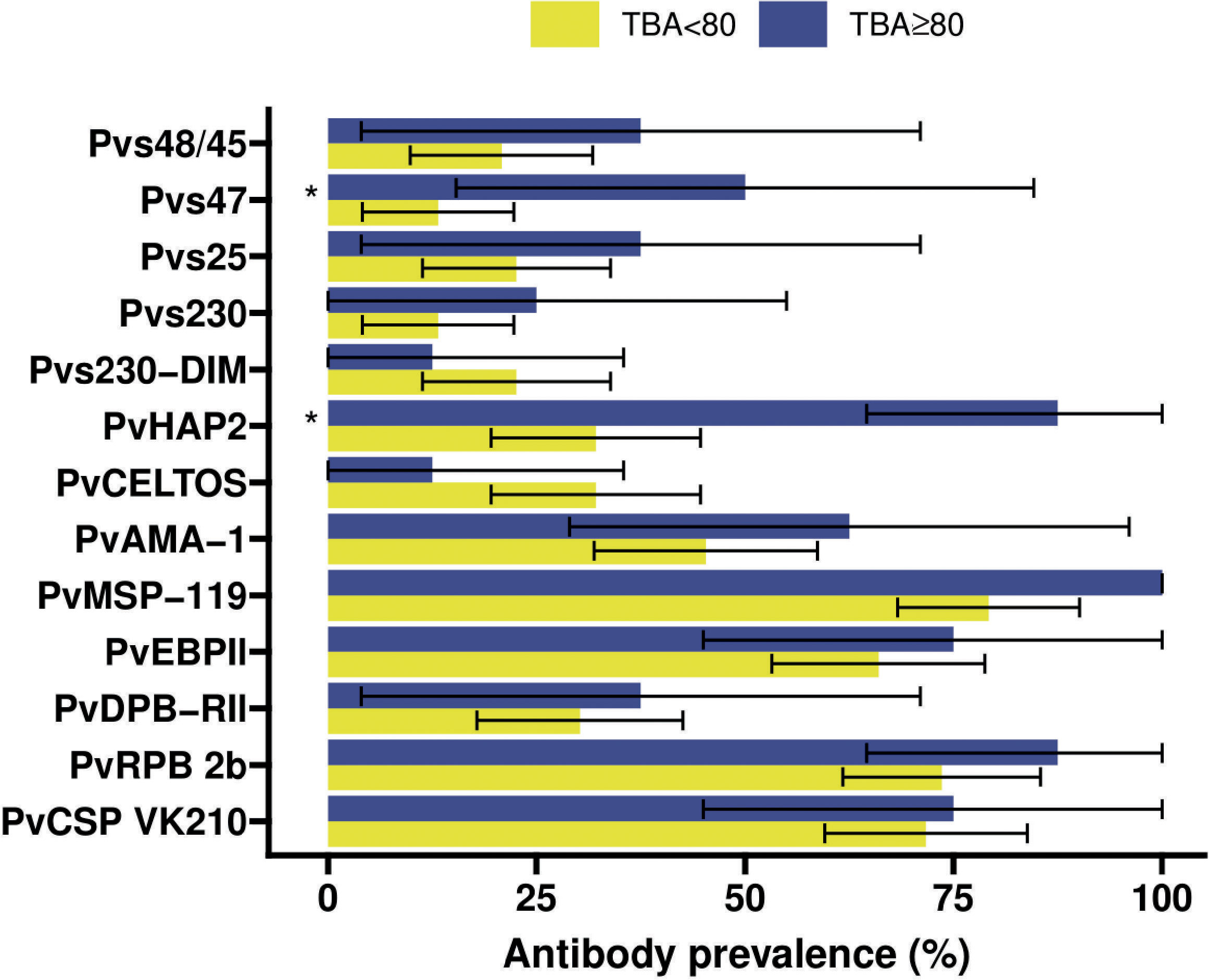
Figure 4 Antibody prevalence (Kim et al., 2011) for the 13 different antigens stratified for whether the transmission blocking activity (TBA) was at least 80% (blue) or less (yellow). 95% confidence intervals are shown by the black bars. Significant (p<0.05) differences in prevalence between TBA<80% and TBA≥80% are indicated by * where p-values were calculated from Fisher’s exact tests.
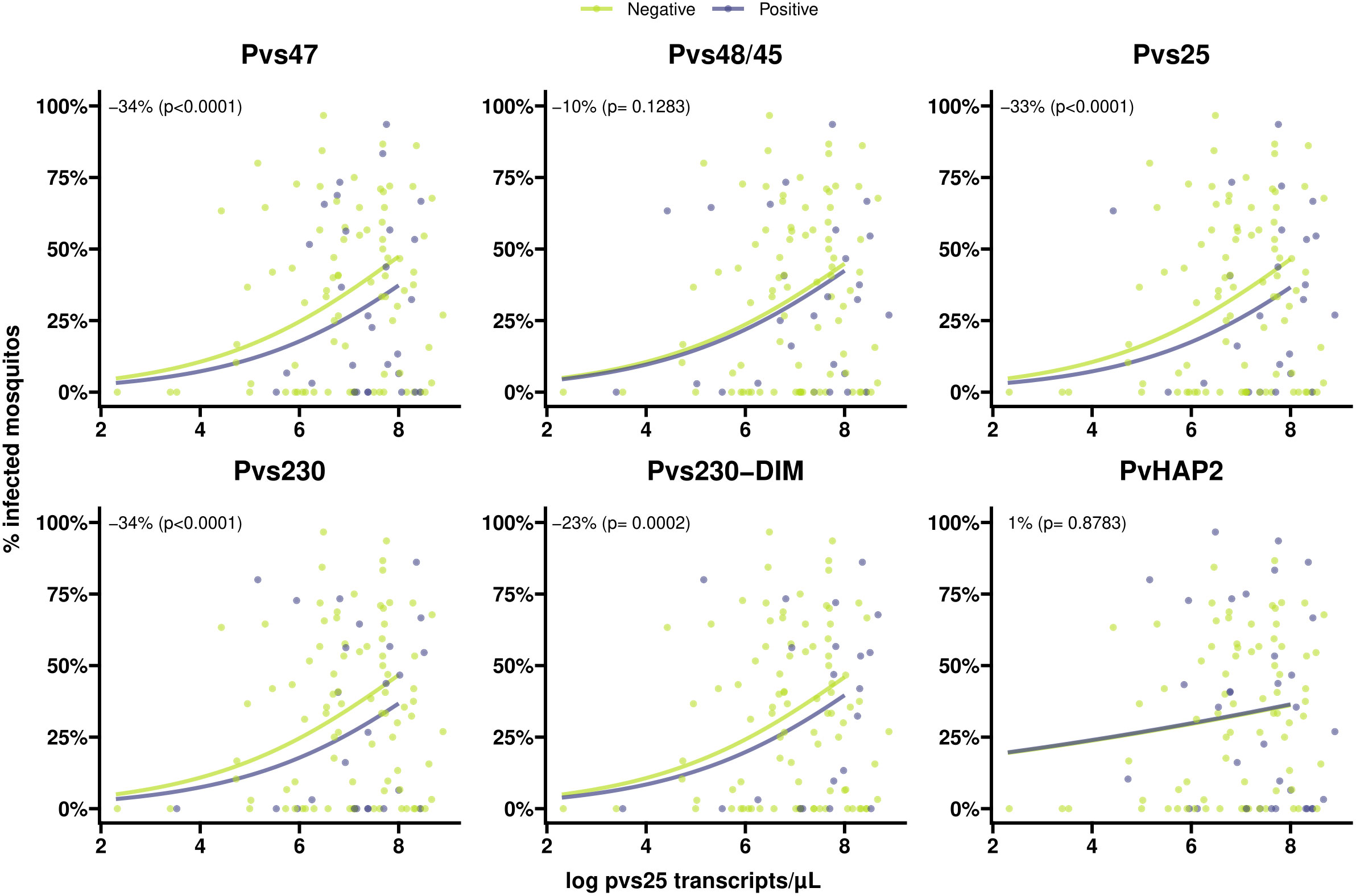
Figure 5 Scatter plots indicating the association between gametocyte density and antibody prevalence on mosquito infectivity for six different antigens. Positive antibodies are indicated in light blue and negative antibodies are indicated in green. The light blue and green lines shows the fit from logistic regression models for the association between gametocyte density and mosquito infectivity for positive and negative antibodies respectively. The numbers in the plot (estimated from logistic regression models) indicate the average difference in mosquito infectivity between positive and negative antibodies while accounting for gametocyte density, and the p-values indicate whether or not these differences are significantly different from 0%.
4 Discussion
Here, we described the association of antibody responses to a portfolio of gametocyte and non-gametocyte antigens with P. vivax transmission efficiency. We observed higher prevalences of antibodies against gametocyte and ookinete antigens Pvs47, Pvs48/45, Pvs230, PvHAP2 and Pvs25 among individuals with high levels of transmission blocking activity with statistically significant differences for Pvs47 and PvHAP2. No such association was found for antibodies against PvCelTOS and to a panel of asexual and sporozoite antigens. Antibody responses against Pv47 and Pvs25 were further associated with a lower per-gametocyte infectivity when whole blood samples were offered to mosquitoes.
The transmission of P. vivax parasites to mosquitoes is strongly associated with gametocyte density (Kiattibutr et al., 2017; Tadesse et al., 2018; Vantaux et al., 2018) that is in turn associated with asexual parasite density (Wampfler et al., 2013; Tadesse et al., 2018; Vantaux et al., 2018). Whilst the positive association between mosquito infection rates and gametocyte density is also observed for P. falciparum (Da et al., 2015; Bradley et al., 2018), for P. vivax this association is particularly strong and very high mosquito infection rates and infection intensities are commonly observed (Kiattibutr et al., 2017; Tadesse et al., 2018; Vantaux et al., 2018). At present, Pvs25 is the most widely used mRNA target to quantify circulating gametocytes although it may not accurately reflect gametocyte maturity or infectivity. These high mosquito infection rates and limited inter-person variation in per-gametocyte infectivity, suggest that host immune factors may play a relatively minor role in dictating transmission efficiency. Nevertheless, anti-gametocyte antibody responses have been observed for P. vivax and some of the most compelling evidence for immune-modulatory properties of serum factors comes from P. vivax infections (Mendis et al., 1987; Peiris et al., 1988; Gamage-Mendis et al., 1992) where antibodies may both reduce and enhance transmission (reviewed in (Stone et al., 2019)). In our cohort of patients with clinical P. vivax infections, 13.1% (8/61) individuals reduced transmission by more than 80%. We classified these individuals as individuals having high TBA. This arbitrary cut-off is commonly used in malaria transmission research although it is acknowledged that also lower levels of TBA may have a meaningful impact on transmission (Blagborough et al., 2013). Too few individuals (n=5) enhanced transmission and the magnitude of transmission enhancement was very modest for most individuals. We concluded that this did not allow for meaningful analyses of immune responses associated with enhancement in our cohort. Also our number of transmission-blockers (n=8) was very modest but the effect was much larger with 80-100% reduction in the proportion of infected mosquitoes in whole blood compared to serum replacement conditions.We observed that the prevalence of antibodies against gametocyte antigens Pvs47 (OR=6.57; 95% CI: 1.33 – 32.48, p=0.0209) and PvHAP2 (OR=14.82; 95% CI: 1.69 – 130.25, p=0.015) were significantly higher among individuals with this strong TBA (>80%) compared to those with lower levels of TBA. Whilst the small number of observations warn that these findings have to be interpreted with caution, it is striking that none of the asexual antigens was associated with TBA>80%, suggesting that cumulative prior exposure to P. vivax may not be predictive of functional transmission-reducing immunity.
We detected antibodies against the ookinete protein Pvs25 in ~25% of individuals which is in line with a previous study (Kim et al., 2011) where 19.2% of naturally infected patients had detectable Pvs25 antibodies. P. falciparum Pfs25 mRNA is translationally repressed (Lasonder et al., 2016) and no or very low levels of antibodies are detected in naturally exposed individuals (Miura et al., 2013; Stone et al., 2018; Essangui et al., 2019). In rodent models, Pys25, an ortholog of Pvs25 and Pfs25 in P. yoelii, protein expression was detected in gametocytes (Tachibana et al., 2018). Our findings suggest that translational repression could be incomplete (leaky expression) in P. vivax similar as in P. yoelii (Tachibana et al., 2018). Strikingly, the presence of Pvs25 antibodies was associated with lower transmission-efficiency in our broader dataset. Similar associations were observed for Pvs47, where antibody prevalence was associated with an estimated 34% reduction in mosquito infection rates, and Pvs230. PvCelTOS can be expressed on surfaces of both sporozoites and ookinetes (Mehrizi et al., 2017), and we speculated that antibodies against PvCelTOS expressed on sporozoites could influence ookinete development, and thus transmission. However, in our cohort, we observed no association between PvCelTOS antibodies and either TBA or general transmission efficiency.
Our study has several limitations. First of all, our findings were primarily based on clinical malaria cases who are highly infectious in P. vivax (Kiattibutr et al., 2017; Vantaux et al., 2018) and were therefore considered an informative population to examine changes in transmission efficiency. However, asymptomatic infections may also be relevant for the human infectious reservoir in some P. vivax endemic settings (Tadesse et al., 2018) and will have a different infection history and therefore plausibly a different antibody profile. Our findings can thus not be extrapolated to asymptomatic populations. We also used two platforms to quantify antibody responses. After initial attempts to couple antigens to beads for Luminex assays which were unsuccessful for all antigens except for HAP2, we used ELISA for all antigens other than HAP2. Most importantly, we describe epidemiological associations and our findings do not provide conclusive evidence for a causal role of anti-gametocyte antibodies in dictating P. vivax gametocyte infectivity. Whilst our findings for several antigens with an established role in gametocyte fertilization are biologically plausible and supported by pre-clinical studies where vaccine-induced antibodies were causally associated with TRA and also broadly corroborate findings in P. falciparum, causality would require a different set of experiments. For P. falciparum, antibodies specific for Pfs48/45 and Pfs230 were purified from large-volume plasma samples and offered to mosquitoes in the presence of cultured gametocytes (Stone et al., 2018). Similar experiments would allow demonstrating causality for antibodies against P. vivax gametocyte antigens but would have to rely on natural gametocyte donors since continuous culture of P. vivax has not been established. In addition, we did not collect the volumes of plasma (up to 9mL) that was used to demonstrate the functionality of antibodies against Pfs48/45 and Pfs230 in P. falciparum (Stone et al., 2018). Furthermore, our study did not test these associations for different concentrations of antibodies or dilutions of autologous plasma. We also did not examine TBA after depleting plasma of antibodies using the recombinant proteins; this would have allowed us to explore TBA associated with antibodies to antigens other than the panel examined here or antibody-independent TBA. It is therefore important to interpret the described associations with caution, also demonstrated by positive and negative associations of mosquito infection rates with antibodies against several asexual antigens.
In conclusion, our mosquito feeding experiments on naturally infected Ethiopians provide evidence for a plausible role of antibodies against P. vivax sexual stage antigens in determining the transmission efficiency of P. vivax gametocytes to locally relevant An. arabiensis mosquitoes. These findings, that require confirmation in other populations, can help understand the natural transmission of P. vivax and support the development of transmission-blocking vaccine candidates.
Data availability statement
The raw data supporting the conclusions of this article will be made available by the authors, without undue reservation.
Author contributions
Conceived, designed and supervised the study: TB, FGT. Wrote manuscript: SKT, TB, FGT. Edited manuscript: CD, BW, AV, MMJ, WJRS, ISH, WC, EH, NS, NT, DN. Performed experiments: SKT, WC, EH, AG, TA, NDS, EE, TE, TseT, KT, KL, ISH, WJRS. Analysed data: JR, TB, SKT. Provided validated antigens: ET, TsuT, NS, NT. All authors contributed to the article and approved the submitted version.
Funding
This work was funded by an AMMODO Science Award to TB and the Gates Foundation (INV-002098; TB is further supported by a European Research Council Consolidator Grant (ERC-CoG 864180; QUANTUM). The work resulting in the production of PvCelTOS and Pvs230 antigens was funded by the Intramural Research Program of the National Institute of Allergy and Infectious Diseases (NIAID), National Institutes of Health. WJRS is supported by a Wellcome trust fellowship (218676/Z/19/Z).
Conflict of interest
NT is listed as an inventor on patent US20190276506A1 related to CelTOS.
The remaining authors declare that the research was conducted in the absence of any commercial or financial relationships that could be construed as a potential conflict of interest.
Publisher’s note
All claims expressed in this article are solely those of the authors and do not necessarily represent those of their affiliated organizations, or those of the publisher, the editors and the reviewers. Any product that may be evaluated in this article, or claim that may be made by its manufacturer, is not guaranteed or endorsed by the publisher.
Supplementary material
The Supplementary Material for this article can be found online at: https://www.frontiersin.org/articles/10.3389/fcimb.2022.1106369/full#supplementary-material
References
Abossie, A., Yohanes, T., Nedu, A., Tafesse, W., Damitie, M. (2020). Prevalence of malaria and associated risk factors among febrile children under five years: A cross-sectional study in arba minch zuria district, south Ethiopia. Infection Drug resistance 13, 363–372. doi: 10.2147/IDR.S223873
Araújo, M. D. S., Andrade, A. O., Dos Santos, N. A. C., Castro, R. B., Pereira, D. B., Rodrigues, M. M. S., et al. (2020). First observation of experimental plasmodium vivax infection of three malaria vectors from the Brazilian Amazon. Vector borne zoonotic Dis. (Larchmont N.Y.) 20, 517–523. doi: 10.1089/vbz.2019.2527
Arévalo-Herrera, M., Miura, K., Solano, E., Sebastián Ramírez, J., Long, C. A., Corradin, G., et al. (2022). Immunogenicity of full-length p. vivax rPvs48/45 protein formulations in BALB/c mice. Vaccine 40, 133–140. doi: 10.1016/j.vaccine.2021.11.036
Arévalo-Herrera, M., Solarte, Y., Zamora, F., Mendez, F., Yasnot, M. F., Rocha, L., et al. (2005). Plasmodium vivax: Transmission-blocking immunity in a malaria-endemic area of Colombia. Am. J. Trop. Med. hygiene 73, 38–43. doi: 10.4269/ajtmh.2005.73.5_suppl.0730038
Blagborough, A. M., Churcher, T. S., Upton, L. M., Ghani, A. C., Gething, P. W., Sinden, R. E. (2013). Transmission-blocking interventions eliminate malaria from laboratory populations. Nat. Commun. 4, 1812. doi: 10.1038/ncomms2840
Bousema, T., Drakeley, C. (2011). Epidemiology and infectivity of plasmodium falciparum and plasmodium vivax gametocytes in relation to malaria control and elimination. Clin. Microbiol. Rev. 24, 377–410. doi: 10.1128/CMR.00051-10
Bradley, J., Stone, W., Da, D. F., Morlais, I., Dicko, A., Cohuet, A., et al. (2018). Predicting the likelihood and intensity of mosquito infection from sex specific plasmodium falciparum gametocyte density. eLife 7. doi: 10.7554/eLife.34463.020
Da, D. F., Churcher, T. S., Yerbanga, R. S., Yameogo, B., Sangare, I., Ouedraogo, J. B., et al. (2015). Experimental study of the relationship between plasmodium gametocyte density and infection success in mosquitoes; implications for the evaluation of malaria transmission-reducing interventions. Exp. Parasitol. 149, 74–83. doi: 10.1016/j.exppara.2014.12.010
de Jong, R. M., Tebeje, S. K., Meerstein-Kessel, L., Tadesse, F. G., Jore, M. M., Stone, W., et al. (2020). Immunity against sexual stage plasmodium falciparum and plasmodium vivax parasites. Immunol. Rev. 293, 190–215. doi: 10.1111/imr.12828
Essangui, E., Eboumbou Moukoko, C. E., Nguedia, N., Tchokwansi, M., Banlanjo, U., Maloba, F., et al. (2019). Demographical, hematological and serological risk factors for plasmodium falciparum gametocyte carriage in a high stable transmission zone in Cameroon. PloS One 14, e0216133. doi: 10.1371/journal.pone.0216133
Gamage-Mendis, A. C., Rajakaruna, J., Carter, R., Mendis, K. N. (1992). Transmission blocking immunity to human plasmodium vivax malaria in an endemic population in kataragama, Sri Lanka. Parasite Immunol. 14, 385–396. doi: 10.1111/j.1365-3024.1992.tb00013.x
Golassa, L., White, M. T. (2017). Population-level estimates of the proportion of plasmodium vivax blood-stage infections attributable to relapses among febrile patients attending adama malaria diagnostic centre, East shoa zone, oromia, Ethiopia. Malaria J. 16, 301. doi: 10.1186/s12936-017-1944-3
Graumans, W., Lanke, K., van Gemert, G. J., Alkema, M., van de Vegte-Bolmer, M., Bousema, T., et al. (2022). The effect of anticoagulants in blood collection tubes on plasmodium falciparum transmission in direct membrane feeding assays. Trans. R Soc. Trop. Med. Hyg 116, 187–189. doi: 10.1093/trstmh/trab095
Griffin, J. T., Hollingsworth, T. D., Okell, L. C., Churcher, T. S., White, M., Hinsley, W., et al. (2010). Reducing plasmodium falciparum malaria transmission in Africa: A model-based evaluation of intervention strategies. PloS Med. 7. doi: 10.1371/journal.pmed.1000324
Hisaeda, H., Stowers, A. W., Tsuboi, T., Collins, W. E., Sattabongkot, J. S., Suwanabun, N., et al. (2000). Antibodies to malaria vaccine candidates Pvs25 and Pvs28 completely block the ability of plasmodium vivax to infect mosquitoes. Infection Immun. 68, 6618–6623. doi: 10.1128/IAI.68.12.6618-6623.2000
Jimah, J. R., Salinas, N. D., Sala-Rabanal, M., Jones, N. G., Sibley, L. D., Nichols, C. G., et al. (2016). Malaria parasite CelTOS targets the inner leaflet of cell membranes for pore-dependent disruption. eLife 5. doi: 10.7554/eLife.20621.019
Jimah, J. R., Schlesinger, P. H., Tolia, N. H. (2017a). Liposome disruption assay to examine lytic properties of biomolecules. Bio-protocol 7. doi: 10.21769/BioProtoc.2433
Jimah, J. R., Schlesinger, P. H., Tolia, N. H. (2017b). Membrane lipid screen to identify molecular targets of biomolecules. Bio-protocol 7. doi: 10.21769/BioProtoc.2427
Kariu, T., Ishino, T., Yano, K., Chinzei, Y., Yuda, M. (2006). CelTOS, a novel malarial protein that mediates transmission to mosquito and vertebrate hosts. Mol. Microbiol. 59, 1369–1379. doi: 10.1111/j.1365-2958.2005.05024.x
Kiattibutr, K., Roobsoong, W., Sriwichai, P., Saeseu, T., Rachaphaew, N., Suansomjit, C., et al. (2017). Infectivity of symptomatic and asymptomatic plasmodium vivax infections to a southeast Asian vector, anopheles dirus. Int. J. Parasitol. 47, 163–170. doi: 10.1016/j.ijpara.2016.10.006
Kim, T. S., Kim, H. H., Kim, J. Y., Kong, Y., Na, B. K., Lin, K., et al. (2011). Comparison of the antibody responses to plasmodium vivax and plasmodium falciparum antigens in residents of Mandalay, Myanmar. Malaria J. 10, 228. doi: 10.1186/1475-2875-10-228
Koepfli, C., Robinson, L. J., Rarau, P., Salib, M., Sambale, N., Wampfler, R., et al. (2015). Blood-stage parasitaemia and age determine plasmodium falciparum and p. vivax gametocytaemia in Papua new Guinea. PloS One 10, e0126747. doi: 10.1371/journal.pone.0126747
Kumar, H., Jimah, J. R., Misal, S. A., Salinas, N. D., Fried, M., Schlesinger, P. H., et al. (2022). Implications of conformational flexibility, lipid binding, and regulatory domains in cell-traversal protein CelTOS for apicomplexan migration. J. Biol. Chem. 298, 102241. doi: 10.1016/j.jbc.2022.102241
Lasonder, E., Rijpma, S. R., van Schaijk, B. C., Hoeijmakers, W. A., Kensche, P. R., Gresnigt, M. S., et al. (2016). Integrated transcriptomic and proteomic analyses of p. falciparum gametocytes: Molecular insight into sex-specific processes and translational repression. Nucleic Acids Res. 44, 6087–6101. doi: 10.1093/nar/gkw536
Lensen, A., van Druten, J., Bolmer, M., van Gemert, G., Eling, W., Sauerwein, R. (1996). Measurement by membrane feeding of reduction in plasmodium falciparum transmission induced by endemic sera. Trans. R. Soc. Trop. Med. Hygiene 90, 20–22. doi: 10.1016/S0035-9203(96)90464-2
Liu, Z. S., Sattabongkot, J., White, M., Chotirat, S., Kumpitak, C., Takashima, E., et al. (2022). Naturally acquired antibody kinetics against plasmodium vivax antigens in people from a low malaria transmission region in western Thailand. BMC Med. 20, 89. doi: 10.1186/s12916-022-02281-9
Martins-Campos, K. M., Kuehn, A., Almeida, A., Duarte, A. P. M., Sampaio, V. S., Rodriguez Í, C., et al. (2018). Infection of anopheles aquasalis from symptomatic and asymptomatic plasmodium vivax infections in manaus, western Brazilian Amazon. Parasites Vectors 11, 288. doi: 10.1186/s13071-018-2749-0
McKenzie, F. E., Wongsrichanalai, C., Magill, A. J., Forney, J. R., Permpanich, B., Lucas, C., et al. (2006). Gametocytemia in Plasmodium vivax and Plasmodium falciparum. 92, 1281–1285. doi: 10.1645/GE-911R.1
Mehrizi, A. A., Torabi, F., Zakeri, S., Djadid, N. D. (2017). Limited genetic diversity in the global plasmodium vivax cell traversal protein of ookinetes and sporozoites (CelTOS) sequences; implications for PvCelTOS-based vaccine development. Infection Genet. Evol. J. Mol. Epidemiol. evolutionary Genet. Infect. Dis. 53, 239–247. doi: 10.1016/j.meegid.2017.06.005
Mendis, K. N., Munesinghe, Y. D., de Silva, Y. N., Keragalla, I., Carter, R. (1987). Malaria transmission-blocking immunity induced by natural infections of plasmodium vivax in humans. Infection Immun. 55, 369–372. doi: 10.1128/iai.55.2.369-372.1987
Miura, K., Takashima, E., Deng, B., Tullo, G., Diouf, A., Moretz, S. E., et al. (2013). Functional comparison of plasmodium falciparum transmission-blocking vaccine candidates by the standard membrane-feeding assay. Infection Immun. 81, 4377–4382. doi: 10.1128/IAI.01056-13
Nguyen, T. N., von Seidlein, L., Nguyen, T. V., Truong, P. N., Hung, S. D., Pham, H. T., et al. (2018). The persistence and oscillations of submicroscopic plasmodium falciparum and plasmodium vivax infections over time in Vietnam: An open cohort study. Lancet Infect. Dis. 18, 565–572. doi: 10.1016/S1473-3099(18)30046-X
Ouédraogo, A. L., Guelbéogo, W. M., Cohuet, A., Morlais, I., King, J. G., Gonçalves, B. P., et al. (2013). A protocol for membrane feeding assays to determine the infectiousness of p. falciparum naturally infected individuals to anopheles gambiae. MWJ 4, 1–4.
Peiris, J. S., Premawansa, S., Ranawaka, M. B., Udagama, P. V., Munasinghe, Y. D., Nanayakkara, M. V., et al. (1988). Monoclonal and polyclonal antibodies both block and enhance transmission of human plasmodium vivax malaria. Am. J. Trop. Med. hygiene 39, 26–32. doi: 10.4269/ajtmh.1988.39.26
Price, R. N., Commons, R. J., Battle, K. E., Thriemer, K., Mendis, K. (2020). Plasmodium vivax in the era of the shrinking p. falciparum map. Trends Parasitol. 36, 560–570. doi: 10.1016/j.pt.2020.03.009
Pukrittayakamee, S., Imwong, M., Singhasivanon, P., Stepniewska, K., Day, N. J., White, N. J. (2008). Effects of different antimalarial drugs on gametocyte carriage in p. vivax malaria. Am. J. Trop. Med. hygiene 79, 378–384. doi: 10.4269/ajtmh.2008.79.378
Qiu, Y., Zhao, Y., Liu, F., Ye, B., Zhao, Z., Thongpoon, S., et al. (2020). Evaluation of plasmodium vivax HAP2 as a transmission-blocking vaccine candidate. Vaccine 38, 2841–2848. doi: 10.1016/j.vaccine.2020.02.011
Ramsey, J. M., Salinas, E., Rodriguez, M. H., Beaudoin, R. L. (1994). Effects of transmission-blocking immunity on plasmodium vivax infections in anopheles albimanus populations. J. Parasitol. 80, 88–92. doi: 10.2307/3283350
Rios-Velásquez, C. M., Martins-Campos, K. M., Simões, R. C., Izzo, T., dos Santos, E. V., Pessoa, F. A., et al. (2013). Experimental plasmodium vivax infection of key anopheles species from the Brazilian Amazon. Malaria J. 12, 460. doi: 10.1186/1475-2875-12-460
Stewart, L., Gosling, R., Griffin, J., Gesase, S., Campo, J., Hashim, R., et al. (2009). Rapid assessment of malaria transmission using age-specific sero-conversion rates. PloS One 4, e6083. doi: 10.1371/journal.pone.0006083
Stone, W., Bousema, T., Sauerwein, R., Drakeley, C. (2019). Two-faced immunity? the evidence for antibody enhancement of malaria transmission. Trends Parasitol. 35, 140–153. doi: 10.1016/j.pt.2018.11.003
Stone, W. J. R., Campo, J. J., Ouédraogo, A. L., Meerstein-Kessel, L., Morlais, I., Da, D., et al. (2018). Unravelling the immune signature of plasmodium falciparum transmission-reducing immunity. Nat. Commun. 9, 558. doi: 10.1038/s41467-017-02646-2
Tachibana, M., Ishino, T., Takashima, E., Tsuboi, T., Torii, M. (2018). A male gametocyte osmiophilic body and microgamete surface protein of the rodent malaria parasite plasmodium yoelii (PyMiGS) plays a critical role in male osmiophilic body formation and exflagellation. Cell. Microbiol. 20, e12821. doi: 10.1111/cmi.12821
Tachibana, M., Sato, C., Otsuki, H., Sattabongkot, J., Kaneko, O., Torii, M., et al. (2012). Plasmodium vivax gametocyte protein Pvs230 is a transmission-blocking vaccine candidate. Vaccine 30, 1807–1812. doi: 10.1016/j.vaccine.2012.01.003
Tachibana, M., Suwanabun, N., Kaneko, O., Iriko, H., Otsuki, H., Sattabongkot, J., et al. (2015). Plasmodium vivax gametocyte proteins, Pvs48/45 and Pvs47, induce transmission-reducing antibodies by DNA immunization. Vaccine 33, 1901–1908. doi: 10.1016/j.vaccine.2015.03.008
Tadesse, F. G., Slater, H. C., Chali, W., Teelen, K., Lanke, K., Belachew, M., et al. (2018). The relative contribution of symptomatic and asymptomatic plasmodium vivax and plasmodium falciparum infections to the infectious reservoir in a low-endemic setting in Ethiopia. Clin. Infect. Dis. an Off. Publ. Infect. Dis. Soc. America 66, 1883–1891. doi: 10.1093/cid/cix1123
Tekeste, Z., Workineh, M., Petros, B. (2013). Determining the severity of plasmodium falciparum malaria in Ethiopia. J. infection Public Health 6, 10–15. doi: 10.1016/j.jiph.2012.09.016
Tentokam, B. C. N., Amaratunga, C., Alani, N. A. H., MacDonald, N. J., Narum, D. L., Salinas, N. D., et al. (2019). Naturally acquired antibody response to malaria transmission blocking vaccine candidate Pvs230 domain 1. Front. Immunol. 10, 2295. doi: 10.3389/fimmu.2019.02295
Vantaux, A., Samreth, R., Piv, E., Khim, N., Kim, S., Berne, L., et al. (2018). Contribution to malaria transmission of symptomatic and asymptomatic parasite carriers in Cambodia. J. Infect. Dis. 217, 1561–1568. doi: 10.1093/infdis/jiy060
Wampfler, R., Mwingira, F., Javati, S., Robinson, L., Betuela, I., Siba, P., et al. (2013). Strategies for detection of plasmodium species gametocytes. PloS One 8, e76316. doi: 10.1371/journal.pone.0076316
Wu, L., Hall, T., Ssewanyana, I., Oulton, T., Patterson, C., Vasileva, H., et al. (2019). Optimisation and standardisation of a multiplex immunoassay of diverse plasmodium falciparum antigens to assess changes in malaria transmission using sero-epidemiology. Wellcome Open Res. 4, 26. doi: 10.12688/wellcomeopenres.14950.1
Wu, L., Mwesigwa, J., Affara, M., Bah, M., Correa, S., Hall, T., et al. (2020). Antibody responses to a suite of novel serological markers for malaria surveillance demonstrate strong correlation with clinical and parasitological infection across seasons and transmission settings in the Gambia. BMC Med. 18, 304. doi: 10.1186/s12916-020-01724-5
Keywords: Plasmodium vivax, gametocyte, transmission, immunity, anopheles, malaria, transmission-blocking, vaccine
Citation: Tebeje SK, Chali W, Hailemeskel E, Ramjith J, Gashaw A, Ashine T, Nebret D, Esayas E, Emiru T, Tsegaye T, Teelen K, Lanke K, Takashima E, Tsuboi T, Salinas ND, Tolia NH, Narum D, Drakeley C, Witkowski B, Vantaux A, Jore MM, Stone WJR, Hansen IS, Tadesse FG and Bousema T (2023) Naturally acquired antibodies to gametocyte antigens are associated with reduced transmission of Plasmodium vivax gametocytes to Anopheles arabiensis mosquitoes. Front. Cell. Infect. Microbiol. 12:1106369. doi: 10.3389/fcimb.2022.1106369
Received: 23 November 2022; Accepted: 28 December 2022;
Published: 16 January 2023.
Edited by:
Surendra Kumar Prajapati, Henry M. Jackson Foundation for the Advancement of Military Medicine (HJF), United StatesReviewed by:
Joseph M. Vinetz, Yale University, United StatesSanjai Kumar - Kumar, Kumar, United States Food and Drug Administration, United States
Yaw Aniweh, University of Ghana, Ghana
Copyright © 2023 Tebeje, Chali, Hailemeskel, Ramjith, Gashaw, Ashine, Nebret, Esayas, Emiru, Tsegaye, Teelen, Lanke, Takashima, Tsuboi, Salinas, Tolia, Narum, Drakeley, Witkowski, Vantaux, Jore, Stone, Hansen, Tadesse and Bousema. This is an open-access article distributed under the terms of the Creative Commons Attribution License (CC BY). The use, distribution or reproduction in other forums is permitted, provided the original author(s) and the copyright owner(s) are credited and that the original publication in this journal is cited, in accordance with accepted academic practice. No use, distribution or reproduction is permitted which does not comply with these terms.
*Correspondence: Teun Bousema, dGV1bi5ib3VzZW1hQHJhZGJvdWR1bWMubmw=
†These authors share first authorship
‡These authors share senior authorship