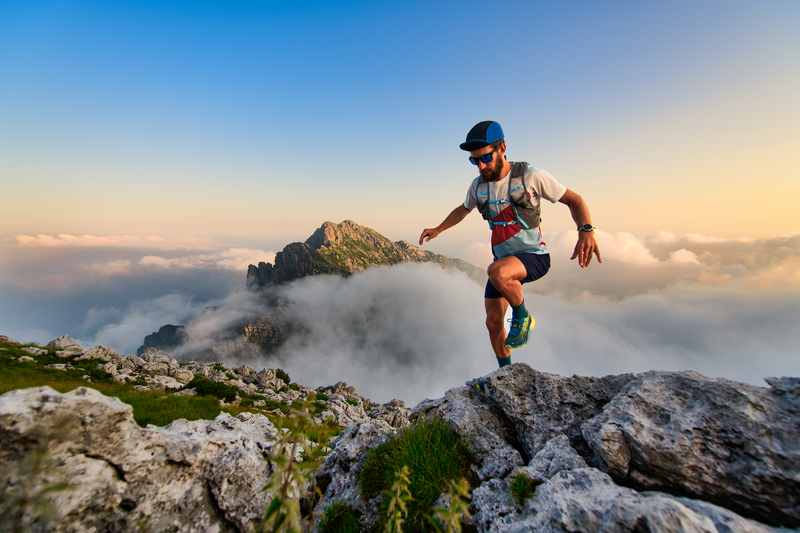
95% of researchers rate our articles as excellent or good
Learn more about the work of our research integrity team to safeguard the quality of each article we publish.
Find out more
ORIGINAL RESEARCH article
Front. Cell. Infect. Microbiol. , 27 December 2022
Sec. Intestinal Microbiome
Volume 12 - 2022 | https://doi.org/10.3389/fcimb.2022.1061444
This article is part of the Research Topic The Gut Microbiome and Sepsis View all 5 articles
Background: The mortality rate associated with sepsis in elderly individuals is higher than that in younger individuals. The intestinal microbiota has been demonstrated to play an important role in the occurrence and development of sepsis. The purpose of this study was to investigate the differences in the intestinal microbiota between aged and adult mice with sepsis.
Methods: Thirty male C57BL mice were randomly divided into two groups: 15 in the adult group (AD group) and 15 in the age group (Age group). All the mice underwent caecal ligation and puncture to induce sepsis. Mice faeces were collected, and analysed using 16S rRNA sequencing. The liver and colon tissues were collected.
Results: There were significant differences in intestinal microbiota composition between the two groups. Compared with adult sepsis mice, the diversity of intestinal microbiota in the aged group was significantly reduced and the structure of dominant intestinal microbiota was changed. In the Age group, the microbiota associated with inflammatory factors increased, and the microbiota associated with the production of SCFAs (Ruminiclostridium, Prevotellaceae_UCG-001, Rikenella, Parabacteroides, Oscillibacter, Odoribacter, Muribaculum, Lachnoclostridium, Intestinimonas, Faecalibaculum, Anaerotruncus, Alloprevotella and Absiella) decreased. The metabolic pathways related to the microbiota also changed. Moreover, the proportion of inflammatory factors in Age group was higher than that in AD group.
Conclusion: Our results showed that there were significant differences in the abundance and structure of microbiota between aged and adult sepsis mice, Aged sepsis mice have more severe intestinal microbiota destruction and liver tissue inflammation than adult sepsis mice.
Sepsis is a life-threatening organ dysfunction caused by the maladjusted response of the host to infection (Shankar-Hari et al., 2016). Failure of regulatory mechanism during sepsis may lead to uncontrolled inflammation, and excessive activation of inflammatory reaction may lead to organ damage (Rittirsch et al., 2008). Sepsis is associated with a high mortality rate, approximately one-quarter of the global mortality rate (Vincent et al., 2014; Haak and Wiersinga, 2017). Sepsis is regarded as a “typical disease of the elderly” (Brakenridge et al., 2019). Age is an independent predictor of mortality in severely sick patients with sepsis, especially those over the age of 65, and sepsis morbidity and in-hospital mortality rates have increased exponentially (Milbrandt et al., 2010). Therefore, studying the difference between elderly patients and young patients with sepsis and identifying how the mechanism of sepsis differs in elderly patients is a problem to be solved.
Destruction of intestinal microbiome predisposes to sepsis, and has a negative impact on the results of sepsis (Fay et al., 2017; Agudelo-Ochoa et al., 2020; Sun et al., 2022). There are abundant bacteria in the intestinal microbiota of healthy people. The Bacteroidetes and Firmicutes are the most abundant, accounting for more than 90% of the intestinal microbiota (Adak and Khan, 2019). It is generally believed that the ratio of Firmicutes to Bacteroidetes is strongly correlated with health and disease (Mariat et al., 2009). Bacteroidetes and Firmicutes are major producers of short chain fatty acids (SCFAs), which can promote the antibacterial activity of macrophages and regulate the immune function of T cells (Smith et al., 2013; Schulthess et al., 2019; Miller et al., 2021). Butyric acid plays an important role in maintaining the integrity of the colon epithelium, and butyric acid is the main raw material for the utilization of the colon epithelium (Nava et al., 2011; Leonel and Alvarez-Leite, 2012; Morrison and Preston, 2016; Tanca et al., 2017). Propionate and its receptor GPR41 regulate Ang II levels and myocardial I/R injury (Deng et al., 2022). The intestinal microbiota and their metabolites are closely related to immunity, endocrinology and inflammation (Belkaid and Hand, 2014; Lin and Zhang, 2017; Deng et al., 2022). The imbalance in gut microbiota will cause a series of diseases, such as obesity, fatty liver and inflammatory diseases (Turnbaugh et al., 2006; Frank et al., 2007; Liu et al., 2018). The intestinal microbiota is an important part of the intestinal barrier. The breakdown of intestinal barrier function and microbiota translocation hasten the progression of sepsis or lead to sepsis (Sun et al., 2022). Patients with sepsis have less fecal SCFAs, which may worsen intestinal epithelial integrity and immune dysfunction in sepsis (Shimizu et al., 2006; Hotchkiss et al., 2013; Yamada et al., 2015). Furthermore, some studies have shown that patients with decreased microbial diversity and increased abundance of pathogenic bacteria are more susceptible to sepsis (Shimizu et al., 2006; Hotchkiss et al., 2013; Yamada et al., 2015; Adhi et al., 2019; Adelman et al., 2020). In this study, we studied the differences in intestinal microbiota between adult and aged mice with sepsis.
Thirty male C57BL mice (6–8 weeks) obtained from Beijing Vital River Laboratory Animal Technology (Beijing, China) were kept in a specific-pathogen-free (SPF) animal laboratory with unlimited access to food and water in a temperature-controlled and light-regulated environment (20-25°C, 1:1 light dark cycle). The mice were randomly categorized into two groups: the adult (AD) group (n = 15) and the aged group (n = 15). The mice in the aged group were fed until 20-21 months. Sepsis was induced in both groups by caecal ligation and puncture (CLP). The establishment of CLP model was based on our previous research (Cui et al., 2020; Liang et al., 2022). The mice were anaesthetized with an intraperitoneal dose of pentobarbital (30 mg/kg). Half of the caecum was ligated, and the caecum was punctured twice with a No. 21 needle. The contents of the caecum were extruded, treated and put back, and two layers of sutures were used to close the incision (muscle layer and skin). In addition, normal saline (37°C; 1 ml/100 g) was injected subcutaneously into the mice. The mice were rewarmed for 1 h and then returned to the cage. The mice were watered, fed and maintained on a light/dark (12/12 hours) cycle. At 24 hours after CLP treatment, 5 mice in the aged group and 8 mice in the AD group were alive. We chose to collect the feces of surviving rats of the two groups, 24 hours after operation. All the experiments in this study were approved by the Life Science Ethics Review Committee of Zhengzhou University.
16s rRNA gene sequencing is a high-throughput sequencing method for all bacteria in specific environmental (or specific habitat) samples and is used to study the composition of microbial populations in those samples, interpret the diversity, richness and population structure of microbial populations, and to explore the relationship between microorganisms and the environment or host. The faeces from both groups of mice were collected and kept at -80°C. DNA was extracted from various samples using the E.Z.N.A. ®Stool DNA Kit (D4015, Omega, Inc., USA). Total DNA was eluted in 50 L of elution buffer and stored at -80°C until PCR assessment. To eliminate the possibility of false-positive PCR results from the negative control, ultrapure water, instead of sample solution, was used throughout the DNA extraction process. AMPure XT beads (Beckman Coulter Genomics, Danvers, MA, USA) were used to purify the PCR products, and Qubit was used to quantify them (Invitrogen, USA). The amplicon pools were prepared for sequencing, and the size and quantity of the amplicon library were determined using an Agilent 2100 Bioanalyzer (Agilent, USA) and the Library Quantification Kit for Illumina (Kapa Biosciences, Woburn, MA, USA). The libraries were sequenced on the NovaSeq PE250 platform. After computer sequencing, the dual terminated data were patched by overlap, and a quality check and chimaera filtering were conducted to obtain high-quality clean data. The Divisive Amplicon Denoising Algorithm 2 (DADA2) was used to obtain representative sequences with single base accuracy, calculated using the QIIME2 microbiome bioinformatics platform. We used the SILVA (Release 132, https://www.arb-silva.de/documentation/release-132/) and NT-16S databases for species classification and subsequent analysis.
We extracted total RNA from liver tissue using TRIzol reagent (Takara, Tokyo, Japan) and determined the RNA concentration and purity by ultraviolet spectrophotometry. A TaqMan reverse transcription kit (UE, Suzhou, China) was used to reverse transcribed mRNA and synthesize corresponding cDNA. Gene expression was normalized using reduced glyceraldehyde-phosphate dehydrogenase (GAPDH) expression. The gene primers were TNF-α forward primer, CCACCACGCTCTTCTGTCTAC, reverse primer, AGGGTCTGGGCCATAGAACT;
IL-6 forward primer, TGATGCACTTGCAGAAAACA, reverse primer, ACCAGAGGAAATTTTCAATAGGC; ccl2 forward primer, CCTGCTGTTCACAGTTGCC, reverse primer, ATTGGGATCATCTTGCTGGT; ccl3 forward primer, ACCATGACACTCTGCAACCA, reverse primer, GTGGAATCTTCCGGCTGTAG; ccl4 forward primer, CATGAAGCTCTGCGTGTCTG, reverse primer, GAAACAGCAGGAAGTGGGAG; ccl5 forward primer, CCACTTCTTCTCTGGGTTGG, reverse primer, GTGCCCACGTCAAGGAGTAT; ccl7 forward primer, CTGCTTTCAGCATCCAAGTG, reverse primer, TTGCCTCTTGGGGATCTTTTG; ccl8 forward primer, TCTTTGCCTGCTGCTCATAG, reverse primer, GAAGGGGGATCTTCAGCTTT; CXCL1 forward primer, ACCCAAACCGAAGTCATAGC, reverse primer, TCTCCGTTACTTGGGGACAC; CXCL10 forward primer, CTCATCCTGCTGGGTCTGAG, reverse primer, CCTATGGCCCTCATTCTCAC; and GAPDH forward primer, TGACCTCAACTACATGGTCTACA, reverse primer, CTTCCCATTCTCGGCCTTG.
Species difference tests were performed using Fisher’s exact test, the Mann–Whitney U test, or the Kruskal–Wallis test. We defined P < 0.05 as significantly different species. Alpha and beta diversity indexes were calculated using QIIME2 and plotted using the R package. Linear discriminant analysis (LDA) effect size (LEfSe) was carried out; the purpose of which is to compare two or more groups and uncover species with significant abundance differences. All distinctive species were detected using the Kruskal–Wallis rank sum test. To acquire significantly distinct species, the difference in species abundance between various groups was detected. The Wilcoxon rank sum test was used to determine whether all subspecies of the significantly distinct species from the previous stage converged to the same categorization level. Finally, LDA was used to obtain the final differential species (biomarker). PICRUSt2 was used for functional prediction (https://github.com/picrust/picrust2). According to the t test, the threshold value of P value < 0.05 was considered a significant difference. The results showed that there were statistically significant differences in the abundance data in the functional database (confidence interval 95%). Statistical analysis was performed using R-package and GraphPad Prism version 5.0 (GraphPad Software, La Jolla, CA, USA).T-tests and Mann-Whitney test were used for two-group comparisons. P < 0.05 was considered statistically significant.
To explore the difference in mortality between aged mice and adult mice, we recorded the mortality of the two groups 24 hours after modelling. In this experiment, we raised thirty mice and generated a CLP model. After 24 hours of modelling, 8 mice survived in the AD group, and 5 mice survived in the Age group. The 24-hour mortality rates of the two groups were 47% and 67%, respectively(Supplement Table 1).
To study the changes of inflammatory factor levels between two groups, The mRNA expression of inflammatory factors in liver and colon tissue were measured. In colon tissue, the mRNA expression of inflammatory factors in the aged mice were higher than those in the adult mice. The levels of TNF-α, IL-6, ccl3, ccl5, ccl8, CXCL10, and ccl4 increased in Age group (Figure 1). We also analysed inflammatory factors in liver tissue. The mRNA expression of inflammatory factors in the age group was higher (Supplementary Figure 1). The results showed that the Age group had higher levels of inflammatory factors, which may be associated with the poor prognosis and high mortality rate in elderly patients with sepsis.
Figure 1 Inflammatory factors. RT–PCR showed that in colon tissue, the Age group exhibited increased levels of inflammatory factors (TNF-α, IL-6, ccl3, ccl5, ccl8, cxcl10 and ccl4) when compared with the AD group *P< 0.05, **P< 0.01, ***P< 0.001.
To study the differences in diversity of the intestinal microbiota between the two groups, we first used a Venn diagram to visually describe microbiota abundance differences between two groups. According to obtained eigenvalue abundance table, the number of common features of each group was calculated, and the number of common and unique features of each group was intuitively presented through a Venn diagram (Supplement Figure 2A). The abundance curve was also plotted. It assess the richness and variety from each sample, the number of features in various samples were compared at the same sequencing depth. If the curve steeply increases, the amount of sequencing data is insufficient. If the curve is flat, the amount of sequencing data has reached its limit. The Shannon curve was flat, indicating that the amount of sample sequencing data was sufficient (Supplement Figure 2B). The rank abundance curve was used to explain both the abundance and uniformity of species in a sample. The results showed that the abundance and uniformity of species were reliable (Supplement Figure 2C).
The similarity of operational taxonomic units (OTUs) was 97%. Each OTU is usually considered a microbial specie. The two groups of observed OTUs were compared and analysed by using the Wilcoxon method. The results showed that compared to the AD group, the Age group showed a decreasing trend but the difference was not statistically significant (Figure 2A). Alpha diversity is used to reflect species richness, homogeneity, and sequencing depth within a certain area or ecosystem. The species diversity between distinct environmental communities is referred to as beta diversity. Beta diversity and alpha diversity describe an environmental community’s overall variety or biological heterogeneity. We analysed alpha diversity, and the findings revealed that, when compared to the AD group (Figures 2B–D), the diversity of the microbiota in the Age group showed a decreasing trend and the Shannon and Simpson index were statistically significant. However, chao1 index was not statistically significant. (Shannon P=0.0016, Simpson P=0.0016, Chao1P=0.17). We used Non-metric multidimensional scaling (NMDS) for beta diversity, unweighted unifrac and weighted unifrac were used to evaluate the analysis. (Figures 2E, F). There were considerable microbial differences between two groups and structure of the intestinal microorganisms in the two groups were significantly different.
Figure 2 Microbial diversity. (A–D) The main purpose of alpha diversity is to reflect species richness, evenness, and sequencing depth. To reflect richness and uniformity, we used the Chao1, observed species, Shannon, and Simpson indexes. (E, F) β diversity reflects the microbial richness within and between groups. We used NMDS to observe the differences between samples. As shown in the figure, the two samples are far apart. The two groups of microbiota differ significantly, and the difference is statistically significant. *P< 0.05, **P< 0.01, ***P< 0.001.
We analysed the microbiota structure at the phylum and genus levels to evaluate the differences in the microbiota structure between the adult mice and the aged mice. The results showed that the microbiota structure of the adult mice was significantly different from that of the aged mice. At the phylum level (Figures 3A, B), Bacteroidetes, verrucomicrobia, Firmicutes and Proteobacteria account for the main part. Compared with AD group, Bacteroides in the Age group decreased and verrucomicrobia increased (Supplement Figure 3). At the Genus level (Figures 3C, D), the microbiota associated with inflammation, such as Robinsoniella, Eubacterium Coriobacteriaceae-Ucg-002, Clostridioides, Akkermansia and other microbiota increased significantly when compared with the AD group, while the microbiota related to the production of short chain fatty acids(SCFAs), such as Ruminiclostridium, Rikenella, Prevotellaceae Ucg-001, Parabacteroides, Oscillibacter, Odoribacter, Muribaculum, Lachnoclostridium, Intestinimonas, Faecalibaculum, Anaerotruncus, Alloprevotella and Absiella decreased significantly. We performed relative abundance analysis in Supplementary Figure 3. The findings suggested substantial differences in dominant microbiota between two groups. In addition, we analyzed the correlation between intestinal microbiota and inflammatory factors. In liver and colon tissue, the correlation analysis between inflammatory factors and microbiota showed that all inflammatory factors were positively correlated with Verrucomicrobia, Robinsonella, Firmicutes, Eubacterium, Coriobateriaceae_ UCG-002, Clostridioides and Akkermania (Supplementary Figure 4)
Figure 3 Relative abundance. Relative microbiota distribution between the two groups. (A, B) phylum level. (C, D) genus level.
In addition, we explored the correlation between the microbiota and calculated the correlation between species through the abundance and changes in the different species in each sample. The results showed that Akkermansia was positively correlated with the Lachnospiraceae-NK4A136-group, Bilophil group, and Eubacterium coprostanoligenes group and negatively correlated with Bacteroides, Helicobacter, and Muribaculaceae_ Unclassified (Figure 4A). Figure 4B describes the taxonomic information of the two groups, and the results showed that the Age group was significantly correlated with the Akkermansia, while the AD group was significantly correlated with the Muribaculaceae-Unclassified.
Figure 4 (A) Correlation network diagram: Different nodes in the network diagram represent different dominant genera. The connection between nodes indicates that there is a correlation between the two genera. By default, we show the relationship pair of correlation coefficientsRho> 0.4. (B) Bubble plot: The species annotation information and relative abundance (circle size) at the genus level in various sample groups as well as the species annotation information (circle colour) of the species-corresponding gate.
To investigate the microbial changes between the AD group and the Age group, we used LEfSe (LDA Effect Size) to analysis. The cladogram showed that the taxa of the Age group differed the most from the AD groups. We set the linear discriminant analysis (LDA) threshold to 4 and to explore microbial species with significant differences between the AD and Age groups. A total of 13 enriched species were identified. Among them, 7 species were enriched in the AD group and 6 species were enriched in the aged group (Figure 5). The results showed that at the species level, Lachnospiraceae NK4A136 group unclassified and Akkermania muciniphila were significantly enriched in Age group, and Desulfovibrio sp and Muribaculeae unclassified were significantly enriched in AD group.
Figure 5 Linear discriminant analysis (LDA) integrated with effect size (LEfSe). (A) The circle radiating from inside to outside represents the taxonomic level from phylum to genus, and the species with no significant difference are yellow. (B) LDA value distribution histogram.
We used PICRUSt to link the microbiota to different functions. The differences of functional annotation results among the groups were compared by PICRUSt analysis (Figure 6). The picture shows the threshold value of P value <0.05 according to t-test difference test. The functions with statistically significant differences in abundance data in the functional database are shown in the results (95% confidence interval) (Table 1). As illustrated in the figure, compared with the Age group, the microbiota function of the AD group was primarily related to PWY-6147, PWY-7539, PWY-6519, PWY0-1241, P124-PWY, BIOTIN-BIOSYNTHESIS-PWY, GLUCONEO-PWY, P122-PWY, PWY-5154, HISDEG-PWY, HOMOSER-METSYN-PWY, PENTOSE-P-PWY, PWY-7199, PWY-7323, GLYCOLYSIS-E-D, PWY-5347, PWY-6263 and MET-SAM-PWY pathways. They Participate in carbohydrate, amino acid, protein and other metabolic pathways of the body. In conclusion, the aged mice exhibited not only changes in the dominant microbiota and reduced relative abundance of beneficial microbiota but also changes in the metabolic pathways of the microbiota.
Figure 6 PICRUSt analysis. Functional prediction pathways in microbial differences among the groups.
This article discusses the microbiome differences and the changes in inflammatory indexes between aged and adult mice during sepsis. It provides a theoretical basis for studying the effect of aging on the intestinal flora of sepsis
Our results show the following: 1. The mortality of old mice is higher than that of adult mice. 2. We analysed the levels of inflammatory factors in the liver and colon of the AD group and Age group. The results suggested substantial changes in the levels of inflammatory factors in the two groups. 3. Compared with the AD group, the intestinal microbiota of the aged group showed a downward trend, the structure of the dominant microbiota changed, and the microbiota related to inflammation increased. 4. Due to the change in the dominant microbiota structure, the functional pathways of the microbiota of the aged mice with sepsis also changed.
The intestine is a complex microbial ecosystem, and its microbiome is strongly linked to human health. The gastrointestinal tract is crucial in the pathophysiology of sepsis. Studies have found that intestinal microbiota and its metabolites play an important role in a variety of diseases, and the occurrence and development of sepsis has also been proved to be related to intestinal microbiota (Deng et al., 2021; Hu et al., 2022). The imbalance of intestinal flora will breakdown intestinal barrier function and induce mucosal immune dysfunction (Wang et al., 2019). Sepsis leads to the destruction of intestinal barrier function and flora displacement, which aggravate the level of tissue inflammation and lead to organ damage (Sun et al., 2022). We compared the levels of inflammatory factors in the liver and colon between the AD and Age groups in this study. The results revealed that inflammatory factor levels were higher in the Age group. The explanation for the high acute inflammation in elderly patients includes that the low clearance efficiency of pathogens leads to the prolongation of the stimulation time of immune response, the increased susceptibility to inflammation during immune aging, and the limited physiological reserves, leading to greater feedback on the release of pro-inflammatory cytokines (Kale and Yende, 2011; Leentjens et al., 2013; Ginde et al., 2014). This may be related to the weakening of intestinal barrier function caused by ageing (Fransen et al., 2017; Fulop et al., 2017; Lin et al., 2019). The weakening of the intestinal barrier caused by ageing may lead to systemic invasion of inflammatory microbiota components. This may be connected to the poor prognosis and high mortality among elderly sepsis patients.
Comparing the intestinal microbiota of the AD group and the Age group, we found that the diversity of the intestinal microbiota decreased significantly in the Age group. Research shows that the microbial diversity of the elderly usually decreases (Woodmansey, 2007). On the other hand, the decrease in intestinal microbiota abundance may be related to sepsis. According to certain research, sepsis can induce an imbalance in the intestinal, decrease microbiota abundance and increase opportunistic pathogenic bacteria (Alverdy and Krezalek, 2017; Fay et al., 2017). In this study, the changes of intestinal microbiota in sepsis mice caused by age were mainly compared. Moreover, there were significant differences in the intestinal microbial composition between the AD group and Age group. We found that Bacteroidetes decreased significantly in the Age group, while Verrucomicrobiota had a greater advantage in the Age group. Woodmansey EJ’s study also showed that the diversity and number of Bacteroidetes decreased significantly in the intestinal flora of elderly individuals, (Woodmansey, 2007). Verrucomicrobiota was increased in elderly patients with Parkinson’s disease, and the abundance of Verrucomicrobiota was correlated with plasma IFN γ with a moderate correlation between concentrations (Lin et al., 2019). At the genus level, Akkermansia and Clostridioides increased significantly in the Age group when compared with the AD group, while Ruminiclostridium, Prevotellaceae_UCG-001, Rikenella, Parabacteroides, Oscillibacter, Odoribacter, Muribaculum, Lachnoclostridium, Intestinimonas, Faecalibaculum, Anaerotruncus and Alloprevotella decreased significantly in the Age group. Akkermansia is the only genus of Verrucomicrobiota found in gastrointestinal samples, and in many studies, Akkermansia bacteria have been shown to improve host function, regulate immunity, reduce inflammation and improve diseases (Geerlings et al., 2018; Zhai et al., 2019; Zhang et al., 2019). Interestingly, our results showed that Akkermansia increased significantly in the Age group and was the dominant flora in the Age mice with sepsis. Mucin is the energy source of mucin-degrading bacteria such as Akkermansia (Crouch et al., 2020). The main barrier against intestinal pathogens is the mucous barrier of the colon. The intestinal microbiota relies on mucus glycoproteins secreted by the host as a source of nutrition, resulting in the erosion of the colonic mucus barrier (McGuckin et al., 2011; Johansson et al., 2013; Crouch et al., 2020). Akkermansia is a beneficial bacterium, but too much Akkermansia may be associated with depleted mucus protein, resulting in the destruction of the intestinal mucosal barrier. The destruction of the mucus barrier may be related to the high inflammation of sepsis in elderly individuals. Clostridioides difficile can cause severe diarrhoea (Sandhu and McBride, 2018), and increase susceptibility to sepsis and lead to the loss of normal intestinal microbiome structure and function (Shreiner et al., 2015).
Studies have shown that the intestinal flora and its metabolites can decompose and metabolize polysaccharides to produce SCFAs. SCFAs could maintain intestinal barrier function, which is important for intestinal homeostasis. SCFAs not only maintain the cell barrier but also prevent the translocation of LPS from the intestinal barrier (Kelly et al., 2015; Zhou et al., 2017). Butyrate could improve the barrier function of intestinal epithelial cells by regulating IL-10, occludin, zonulin and claudins (Wang et al., 2012; Zheng et al., 2017). Ruminiclostridium, Prevotellaceae_UCG-001, Rikenella, Parabacteroides, Oscillibacter, Odoribacter, Muribaculum, Lachnoclostridium, Intestinimonas, Faecalibaculum, Anaerotruncus and Alloprevotella have been proven to be related to the formation of SCFAs (Bui et al., 2016; Koh et al., 2016; Shi et al., 2017; Van den Abbeele et al., 2020; Yao et al., 2020; Nogal et al., 2021). Ruminiclostridium degrades polysaccharides by producing multienzyme complexes (including xylan endonuclease and acetylxylan esterase) to produce SCFAs, such as acetate and butyrate, that can inhibit the inflammatory response (Wang et al., 2018; Xiao et al., 2020). Furthermore, Odoribacter and Rikenella are effective anti-diarrhoea probiotics that are negatively correlated with the diarrhoea index (Xu et al., 2020). Prevotella and Muribaculaceae have positive effects on the intestine via immune regulation and intestinal homeostasis regulation (Zhang et al., 2015; de la Cuesta-Zuluaga et al., 2017). Parabacteroides can reduce tumour occurrence and reduce the level of inflammatory markers in mice (Koh et al., 2020). The above conclusions show that the flora related to the formation of SCFAs in elderly individuals with sepsis is significantly reduced.
In terms of functional prediction, the metabolic pathways of AD group and the Age group were significantly different. The functional pathways related to energy generation, antioxidation and amino acid production were relatively reduced in the aged group. For example, AD group promoted L-arginine biosynthesis. L-arginine is catabolized by various enzymes to finally produce urea, proline, glutamate, polyamines, nitric oxide, creatine or agmatine (Wu and Morris, 1998). Agmatine has anti diabetes effect on diabetic animals. Agmatine can not only increase β-Insulin secretion of pancreatic cells but can also inhibit hyperglycemia and reduce insulin resistance in rats (Zhang et al., 2021). In addition, a systematic review and meta-analysis showed that L-arginine could significantly reduce triglyceride (TG) levels (Sepandi et al., 2019). Bifidobacterium shunt is a special pathway involving phosphoketolase activity. Hexose, such as glucose or fructose, is metabolized into acetate and lactate through this pathway and used for energy production (Lee and O'Sullivan, 2010; De Vuyst et al., 2014). The pentose phosphate pathway (PPP) is required for ribonucleotide synthesis and is a major source of NADPH. NADPH plays a key role in the redox state of cells and is also required for fatty acid synthesis (Patra and Hay, 2014). PPP generates NADPH, which is critical for the reduction of oxidized glutathione to GSH, thereby maintaining the redox homeostasis of cells (Blacker and Duchen, 2016; Xiao et al., 2018). In addition, PPP metabolic pathway can regulate the phenotype, function and survival of inflammatory macrophages (Ma et al., 2020).
Our study has some limitations. First, the sample size of the experimental groups was very small, and the results of this study need to be confirmed by other similar studies. Second, we only counted mortality after 24 hours and did not count longer-term mortality. Third, our study only explored the difference of intestinal microbiota between adult sepsis mice and aged sepsis mice, and we did not include a control group before modeling, which weakens the rigor of our study. In addition, the analysis of fecal transplantation experiments are important for future studies exploring the impact of the intestinal microbiota in the host. Regarding Akkermansia, other studies have reported that the abundance of Akkermansia decreases in elderly individuals (Collado et al., 2007; Biagi et al., 2010), so the relationship between Akkermansia and elderly individuals needs to be studied further and discussed.
In conclusion, this study explored the differences in intestinal microbiota diversity and dominant flora structure between aged and adult mice with sepsis and it provided theoretical basis for studying the effect of aging on the intestinal flora of sepsis.
The data presented in the study are deposited in the NCBI repository, accession number PRJNA912904.
The animal study was reviewed and approved by the Life Science Ethics Review Committee of Zhengzhou University.
All authors contributed to the article and approved the submitted version.
This study was supported by the United Fund of National Natural Science Foundation of China (grant number U2004110), the National Natural Science Foundation of China (grant number 82172129, 82202370), the 2021 Youth Talent Promotion Project in Henan Province (grant number 2021HYTP053), the 2021 joint construction project of Henan Medical Science and Technology Breakthrough Plan (grant number LHGJ20210299), the central government guides local science and technology development funds (grant number Z20221343037), Medical Science and Technology Tackling Plan Provincial and Ministerial Major Projects of Henan Province (Grant No. SBGJ202101015).
The authors declare that the research was conducted in the absence of any commercial or financial relationships that could be construed as a potential conflict of interest.
All claims expressed in this article are solely those of the authors and do not necessarily represent those of their affiliated organizations, or those of the publisher, the editors and the reviewers. Any product that may be evaluated in this article, or claim that may be made by its manufacturer, is not guaranteed or endorsed by the publisher.
The Supplementary Material for this article can be found online at: https://www.frontiersin.org/articles/10.3389/fcimb.2022.1061444/full#supplementary-material
Supplementary Figure 1 | Expression of inflammatory factors in liver tissue. (*P< 0.05, **P< 0.01, ***P< 0.001)
Supplementary Figure 2 | Species richness. (A) Venn diagram between the two groups. (B) Shannon–Wiener index: The abscissa is the number of randomly selected sequences, and the ordinate is the Shannon index of species diversity. The saturation of the curve proves that the sequencing results were sufficient. The results show that the curve tends to be flat, indicating that the amount of sequencing data is sufficient. (C) The smoother the decline of the curve, the more uniform the distribution of species.
Supplementary Figure 3 | Relative abundance of intestinal flora. Abundance of specific intestinal microbiota in two groups. *P<0.05.
Supplementary Figure 4 | The correlation between microbiota and inflammatory factors. (A) colon tissue (B) liver tissue.
SCFA, short-chain fatty acid; PICRUSt, Phylogenetic Investigation of Communities by Reconstruction of Unobserved States.
Adak, A., Khan, M. R. (2019). An insight into gut microbiota and its functionalities. Cell Mol. Life Sci. 76 (3), 473–493. doi: 10.1007/s00018-018-2943-4
Adelman, M. W., Woodworth, M. H., Langelier, C., Busch, L. M., Kempker, J. A., Kraft, C. S., et al. (2020). The gut microbiome's role in the development, maintenance, and outcomes of sepsis. Crit. Care 24 (1), 278. doi: 10.1186/s13054-020-02989-1
Agudelo-Ochoa, G. M., Valdes-Duque, B. E., Giraldo-Giraldo, N. A., Jaillier-Ramirez, A. M., Giraldo-Villa, A., Acevedo-Castano, I., et al. (2020). Gut microbiota profiles in critically ill patients, potential biomarkers and risk variables for sepsis. Gut. Microbes 12 (1), 1707610.
Alverdy, J. C., Krezalek, M. A. (2017). Collapse of the microbiome, emergence of the pathobiome, and the immunopathology of sepsis. Crit. Care Med. 45 (2), 337–347. doi: 10.1097/CCM.0000000000002172
Belkaid, Y., Hand, T. W. (2014). Role of the microbiota in immunity and inflammation. Cell 157 (1), 121–141. doi: 10.1016/j.cell.2014.03.011
Biagi, E., Nylund, L., Candela, M., Ostan, R., Bucci, L., Pini, E., et al. (2010). Through ageing, and beyond: gut microbiota and inflammatory status in seniors and centenarians. PloS One 5 (5), e10667. doi: 10.1371/annotation/df45912f-d15c-44ab-8312-e7ec0607604d
Blacker, T. S., Duchen, M. R. (2016). Investigating mitochondrial redox state using NADH and NADPH autofluorescence. Free Radic. Biol. Med. 100, 53–65. doi: 10.1016/j.freeradbiomed.2016.08.010
Brakenridge, S. C., Efron, P. A., Cox, M. C., Stortz, J. A., Hawkins, R. B., Ghita, G., et al. (2019). Current epidemiology of surgical sepsis: Discordance between inpatient mortality and 1-year outcomes. Ann. Surg. 270 (3), 502–510. doi: 10.1097/SLA.0000000000003458
Bui, T. P., Shetty, S. A., Lagkouvardos, I., Ritari, J., Chamlagain, B., Douillard, F. P., et al. (2016). Comparative genomics and physiology of the butyrate-producing bacterium intestinimonas butyriciproducens. Environ. Microbiol. Rep. 8 (6), 1024–1037. doi: 10.1111/1758-2229.12483
Collado, M. C., Derrien, M., Isolauri, E., de Vos, W. M., Salminen, S. (2007). Intestinal integrity and akkermansia muciniphila, a mucin-degrading member of the intestinal microbiota present in infants, adults, and the elderly. Appl. Environ. Microbiol. 73 (23), 7767–7770. doi: 10.1128/AEM.01477-07
Crouch, L. I., Liberato, M. V., Urbanowicz, P. A., Basle, A., Lamb, C. A., Stewart, C. J., et al. (2020). Prominent members of the human gut microbiota express endo-acting O-glycanases to initiate mucin breakdown. Nat. Commun. 11 (1), 4017. doi: 10.1038/s41467-020-17847-5
Cui, Y., Liu, S., Zhang, X., Ding, X., Duan, X., Zhu, Z., et al. (2020). Metabolomic Analysis of the Effects of Adipose-Derived Mesenchymal Stem Cell Treatment on Rats With Sepsis-Induced Acute Lung Injury. Front Pharmacol. 11, 902. doi: 10.3389/fphar.2020.00902
de la Cuesta-Zuluaga, J., Mueller, N. T., Corrales-Agudelo, V., Velasquez-Mejia, E. P., Carmona, J. A., Abad, J. M., et al. (2017). Metformin is associated with higher relative abundance of mucin-degrading akkermansia muciniphila and several short-chain fatty acid-producing microbiota in the gut. Diabetes Care 40 (1), 54–62. doi: 10.2337/dc16-1324
Deng, F., Zhao, B. C., Yang, X., Lin, Z. B., Sun, Q. S., Wang, Y. F., et al. (2021). The gut microbiota metabolite capsiate promotes Gpx4 expression by activating TRPV1 to inhibit intestinal ischemia reperfusion-induced ferroptosis. Gut. Microbes 13 (1), 1–21. doi: 10.1080/19490976.2021.1902719
Deng, F., Chen, Y., Sun, Q. S., Lin, Z. B., Min, Y., Zhao, B. C., et al. (2022). Gut microbiota dysbiosis is associated with sepsis-induced cardiomyopathy in patients: A case-control study. J. Med. Virol., 95(1):e28267.
Deng, F., Zhang, L. Q., Wu, H., Chen, Y., Yu, W. Q., Han, R. H., et al. (2022). Propionate alleviates myocardial ischemia-reperfusion injury aggravated by angiotensin II dependent on caveolin-1/ACE2 axis through GPR41. Int. J. Biol. Sci. 18 (2), 858–872. doi: 10.7150/ijbs.67724
De Vuyst, L., Moens, F., Selak, M., Riviere, A., Leroy, F. (2014). Summer meeting 2013: growth and physiology of bifidobacteria. J. Appl. Microbiol. 116 (3), 477–491. doi: 10.1111/jam.12415
Fay, K. T., Ford, M. L., Coopersmith, C. M. (2017). The intestinal microenvironment in sepsis. Biochim. Biophys. Acta Mol. Basis Dis. 1863 (10 Pt B), 2574–2583. doi: 10.1016/j.bbadis.2017.03.005
Frank, D. N., St Amand, A. L., Feldman, R. A., Boedeker, E. C., Harpaz, N., Pace, N. R. (2007). Molecular-phylogenetic characterization of microbial community imbalances in human inflammatory bowel diseases. Proc. Natl. Acad. Sci. U.S.A. 104 (34), 13780–13785. doi: 10.1073/pnas.0706625104
Fransen, F., van Beek, A. A., Borghuis, T., Aidy, S. E., Hugenholtz, F., van der Gaast-de Jongh, C., et al. (2017). Aged gut microbiota contributes to systemical inflammaging after transfer to germ-free mice. Front. Immunol. 8, 1385. doi: 10.3389/fimmu.2017.01385
Fulop, T., Larbi, A., Dupuis, G., Le Page, A., Frost, E. H., Cohen, A. A., et al. (2017). Immunosenescence and inflamm-aging as two sides of the same coin: Friends or foes? Front. Immunol. 8, 1960. doi: 10.3389/fimmu.2017.01960
Geerlings, S. Y., Kostopoulos, I., de Vos, W. M., Belzer, C. (2018). Akkermansia muciniphila in the human gastrointestinal tract: When, where, and how? Microorganisms 6 (3). doi: 10.3390/microorganisms6030075
Ginde, A. A., Blatchford, P. J., Trzeciak, S., Hollander, J. E., Birkhahn, R., Otero, R., et al. (2014). Age-related differences in biomarkers of acute inflammation during hospitalization for sepsis. Shock 42 (2), 99–107. doi: 10.1097/SHK.0000000000000182
Haak, B. W., Wiersinga, W. J. (2017). The role of the gut microbiota in sepsis. Lancet Gastroenterol. Hepatol. 2 (2), 135–143. doi: 10.1016/S2468-1253(16)30119-4
Hotchkiss, R. S., Monneret, G., Payen, D. (2013). Immunosuppression in sepsis: a novel understanding of the disorder and a new therapeutic approach. Lancet Infect. Dis. 13 (3), 260–268. doi: 10.1016/S1473-3099(13)70001-X
Hu, J., Deng, F., Zhao, B., Lin, Z., Sun, Q., Yang, X., et al. (2022). Lactobacillus murinus alleviate intestinal ischemia/reperfusion injury through promoting the release of interleukin-10 from M2 macrophages via toll-like receptor 2 signaling. Microbiome 10 (1), 38. doi: 10.1186/s40168-022-01227-w
Johansson, M. E., Sjovall, H., Hansson, G. C. (2013). The gastrointestinal mucus system in health and disease. Nat. Rev. Gastroenterol. Hepatol. 10 (6), 352–361. doi: 10.1038/nrgastro.2013.35
Kale, S. S., Yende, S. (2011). Effects of aging on inflammation and hemostasis through the continuum of critical illness. Aging Dis. 2 (6), 501–511.
Kelly, C. J., Zheng, L., Campbell, E. L., Saeedi, B., Scholz, C. C., Bayless, A. J., et al. (2015). Crosstalk between microbiota-derived short-chain fatty acids and intestinal epithelial HIF augments tissue barrier function. Cell Host Microbe 17 (5), 662–671. doi: 10.1016/j.chom.2015.03.005
Koh, A., De Vadder, F., Kovatcheva-Datchary, P., Backhed, F. (2016). From dietary fiber to host physiology: Short-chain fatty acids as key bacterial metabolites. Cell 165 (6), 1332–1345. doi: 10.1016/j.cell.2016.05.041
Koh, G. Y., Kane, A. V., Wu, X., Crott, J. W. (2020). Parabacteroides distasonis attenuates tumorigenesis, modulates inflammatory markers and promotes intestinal barrier integrity in azoxymethane-treated A/J mice. Carcinogenesis 41 (7), 909–917. doi: 10.1093/carcin/bgaa018
Leentjens, J., Kox, M., van der Hoeven, J. G., Netea, M. G., Pickkers, P. (2013). Immunotherapy for the adjunctive treatment of sepsis: from immunosuppression to immunostimulation. time for a paradigm change? Am. J. Respir. Crit. Care Med. 187 (12), 1287–1293. doi: 10.1164/rccm.201301-0036CP
Lee, J. H., O'Sullivan, D. J. (2010). Genomic insights into bifidobacteria. Microbiol. Mol. Biol. Rev. 74 (3), 378–416. doi: 10.1128/MMBR.00004-10
Leonel, A. J., Alvarez-Leite, J. I. (2012). Butyrate: implications for intestinal function. Curr. Opin. Clin. Nutr. Metab. Care 15 (5), 474–479. doi: 10.1097/MCO.0b013e32835665fa
Liang, H., Song, H., Zhang, X., Song, G., Wang, Y., Ding, X., et al. (2022). Metformin attenuated sepsis-related liver injury by modulating gut microbiota. Emerg Microbes Infect. 11 (1), 815–828. doi: 10.1080/22221751.2022.2045876
Lin, C. H., Chen, C. C., Chiang, H. L., Liou, J. M., Chang, C. M., Lu, T. P., et al. (2019). Altered gut microbiota and inflammatory cytokine responses in patients with parkinson's disease. J. Neuroinflamm. 16 (1), 129. doi: 10.1186/s12974-019-1528-y
Lin, L., Zhang, J. (2017). Role of intestinal microbiota and metabolites on gut homeostasis and human diseases. BMC Immunol. 18 (1), 2. doi: 10.1186/s12865-016-0187-3
Liu, J., Yue, S., Yang, Z., Feng, W., Meng, X., Wang, A., et al. (2018). Oral hydroxysafflor yellow a reduces obesity in mice by modulating the gut microbiota and serum metabolism. Pharmacol. Res. 134, 40–50. doi: 10.1016/j.phrs.2018.05.012
Mariat, D., Firmesse, O., Levenez, F., Guimaraes, V., Sokol, H., Dore, J., et al. (2009). The Firmicutes/Bacteroidetes ratio of the human microbiota changes with age. BMC Microbiol. 9, 123. doi: 10.1186/1471-2180-9-123
Ma, J., Wei, K., Liu, J., Tang, K., Zhang, H., Zhu, L., et al. (2020). Glycogen metabolism regulates macrophage-mediated acute inflammatory responses. Nat. Commun. 11 (1), 1769. doi: 10.1038/s41467-020-15636-8
McGuckin, M. A., Linden, S. K., Sutton, P., Florin, T. H. (2011). Mucin dynamics and enteric pathogens. Nat. Rev. Microbiol. 9 (4), 265–278. doi: 10.1038/nrmicro2538
Milbrandt, E. B., Eldadah, B., Nayfield, S., Hadley, E., Angus, D. C. (2010). Toward an integrated research agenda for critical illness in aging. Am. J. Respir. Crit. Care Med. 182 (8), 995–1003. doi: 10.1164/rccm.200904-0630CP
Miller, W. D., Keskey, R., Alverdy, J. C. (2021). Sepsis and the microbiome: A vicious cycle. J. Infect. Dis. 223 (12 Suppl 2), S264–S2S9. doi: 10.1093/infdis/jiaa682
Morrison, D. J., Preston, T. (2016). Formation of short chain fatty acids by the gut microbiota and their impact on human metabolism. Gut. Microbes 7 (3), 189–200. doi: 10.1080/19490976.2015.1134082
Nava, G. M., Friedrichsen, H. J., Stappenbeck, T. S. (2011). Spatial organization of intestinal microbiota in the mouse ascending colon. ISME J. 5 (4), 627–638. doi: 10.1038/ismej.2010.161
Nogal, A., Louca, P., Zhang, X., Wells, P. M., Steves, C. J., Spector, T. D., et al. (2021). Circulating levels of the short-chain fatty acid acetate mediate the effect of the gut microbiome on visceral fat. Front. Microbiol. 12, 711359. doi: 10.3389/fmicb.2021.711359
Patra, K. C., Hay, N. (2014). The pentose phosphate pathway and cancer. Trends Biochem. Sci. 39 (8), 347–354. doi: 10.1016/j.tibs.2014.06.005
Rittirsch, D., Flierl, M. A., Ward, P. A. (2008). Harmful molecular mechanisms in sepsis. Nat. Rev. Immunol. 8 (10), 776–787. doi: 10.1038/nri2402
Sandhu, B. K., McBride, S. M. (2018). Clostridioides difficile. Trends Microbiol. 26 (12), 1049–1050. doi: 10.1016/j.tim.2018.09.004
Schulthess, J., Pandey, S., Capitani, M., Rue-Albrecht, K. C., Arnold, I., Franchini, F., et al. (2019). The short chain fatty acid butyrate imprints an antimicrobial program in macrophages. Immunity 50 (2), 432–45.e7. doi: 10.1016/j.immuni.2018.12.018
Sepandi, M., Abbaszadeh, S., Qobady, S., Taghdir, M. (2019). Effect of l-arginine supplementation on lipid profiles and inflammatory markers: A systematic review and meta-analysis of randomized controlled trials. Pharmacol. Res. 148, 104407. doi: 10.1016/j.phrs.2019.104407
Shankar-Hari, M., Phillips, G. S., Levy, M. L., Seymour, C. W., Liu, V. X., Deutschman, C. S., et al. (2016). Developing a new definition and assessing new clinical criteria for septic shock: For the third international consensus definitions for sepsis and septic shock (Sepsis-3). JAMA 315 (8), 775–787. doi: 10.1001/jama.2016.0289
Shi, H., Chang, Y., Gao, Y., Wang, X., Chen, X., Wang, Y., et al. (2017). Dietary fucoidan of acaudina molpadioides alters gut microbiota and mitigates intestinal mucosal injury induced by cyclophosphamide. Food Funct. 8 (9), 3383–3393. doi: 10.1039/C7FO00932A
Shimizu, K., Ogura, H., Goto, M., Asahara, T., Nomoto, K., Morotomi, M., et al. (2006). Altered gut flora and environment in patients with severe SIRS. J. Trauma 60 (1), 126–133. doi: 10.1097/01.ta.0000197374.99755.fe
Shreiner, A. B., Kao, J. Y., Young, V. B. (2015). The gut microbiome in health and in disease. Curr. Opin. Gastroenterol. 31 (1), 69–75. doi: 10.1097/MOG.0000000000000139
Smith, P. M., Howitt, M. R., Panikov, N., Michaud, M., Gallini, C. A., Bohlooly, Y. M., et al. (2013). The microbial metabolites, short-chain fatty acids, regulate colonic treg cell homeostasis. Science 341 (6145), 569–573. doi: 10.1126/science.1241165
Sun, T., Wang, L., Zhang, H. (2022). Intestinal microbiota in sepsis. Intensive Care Res 2, 1–7. https://doi.org/10.1007/s44231-022-00001-8
Tanca, A., Abbondio, M., Palomba, A., Fraumene, C., Manghina, V., Cucca, F., et al. (2017). Potential and active functions in the gut microbiota of a healthy human cohort. Microbiome 5 (1), 79. doi: 10.1186/s40168-017-0293-3
Turnbaugh, P. J., Ley, R. E., Mahowald, M. A., Magrini, V., Mardis, E. R., Gordon, J. I. (2006). An obesity-associated gut microbiome with increased capacity for energy harvest. Nature 444 (7122), 1027–1031. doi: 10.1038/nature05414
Van den Abbeele, P., Moens, F., Pignataro, G., Schnurr, J., Ribecco, C., Gramenzi, A., et al. (2020). Yeast-derived formulations are differentially fermented by the canine and feline microbiome as assessed in a novel In vitro colonic fermentation model. J. Agric. Food Chem. 68 (46), 13102–13110. doi: 10.1021/acs.jafc.9b05085
Vincent, J. L., Marshall, J. C., Namendys-Silva, S. A., Francois, B., Martin-Loeches, I., Lipman, J., et al. (2014). Assessment of the worldwide burden of critical illness: the intensive care over nations (ICON) audit. Lancet Respir. Med. 2 (5), 380–386. doi: 10.1016/S2213-2600(14)70061-X
Wang, C., Li, Q., Ren, J. (2019). Microbiota-immune interaction in the pathogenesis of gut-derived infection. Front. Immunol. 10, 1873. doi: 10.3389/fimmu.2019.01873
Wang, Y., Sakka, M., Yagi, H., Kaneko, S., Katsuzaki, H., Kunitake, E., et al. (2018). Ruminiclostridium josui Abf62A-Axe6A: A tri-functional xylanolytic enzyme exhibiting alpha-l-arabinofuranosidase, endoxylanase, and acetylxylan esterase activities. Enzyme Microb. Technol. 117, 1–8. doi: 10.1016/j.enzmictec.2018.05.016
Wang, H. B., Wang, P. Y., Wang, X., Wan, Y. L., Liu, Y. C. (2012). Butyrate enhances intestinal epithelial barrier function via up-regulation of tight junction protein claudin-1 transcription. Dig. Dis. Sci. 57 (12), 3126–3135. doi: 10.1007/s10620-012-2259-4
Woodmansey, E. J. (2007). Intestinal bacteria and ageing. J. Appl. Microbiol. 102 (5), 1178–1186. doi: 10.1111/j.1365-2672.2007.03400.x
Wu, G., Morris, S. M., Jr. (1998). Arginine metabolism: nitric oxide and beyond. Biochem. J. 336 (Pt 1), 1–17. doi: 10.1042/bj3360001
Xiao, S., Liu, C., Chen, M., Zou, J., Zhang, Z., Cui, X., et al. (2020). Scutellariae radix and coptidis rhizoma ameliorate glycolipid metabolism of type 2 diabetic rats by modulating gut microbiota and its metabolites. Appl. Microbiol. Biotechnol. 104 (1), 303–317. doi: 10.1007/s00253-019-10174-w
Xiao, W., Wang, R. S., Handy, D. E., Loscalzo, J. (2018). NAD(H) and NADP(H) redox couples and cellular energy metabolism. Antioxid. Redox Signal 28 (3), 251–272. doi: 10.1089/ars.2017.7216
Xu, B., Yan, Y., Huang, J., Yin, B., Pan, Y., Ma, L. (2020). Cortex phellodendri extract's anti-diarrhea effect in mice related to its modification of gut microbiota. BioMed. Pharmacother. 123, 109720. doi: 10.1016/j.biopha.2019.109720
Yamada, T., Shimizu, K., Ogura, H., Asahara, T., Nomoto, K., Yamakawa, K., et al. (2015). Rapid and sustained long-term decrease of fecal short-chain fatty acids in critically ill patients with systemic inflammatory response syndrome. JPEN J. Parenter Enteral Nutr. 39 (5), 569–577. doi: 10.1177/0148607114529596
Yao, Y., Yan, L., Chen, H., Wu, N., Wang, W., Wang, D. (2020). Cyclocarya paliurus polysaccharides alleviate type 2 diabetic symptoms by modulating gut microbiota and short-chain fatty acids. Phytomedicine 77, 153268. doi: 10.1016/j.phymed.2020.153268
Zhai, Q., Feng, S., Arjan, N., Chen, W. (2019). A next generation probiotic, akkermansia muciniphila. Crit. Rev. Food Sci. Nutr. 59 (19), 3227–3236. doi: 10.1080/10408398.2018.1517725
Zhang, T., Li, Q., Cheng, L., Buch, H., Zhang, F. (2019). Akkermansia muciniphila is a promising probiotic. Microb. Biotechnol. 12 (6), 1109–1125. doi: 10.1111/1751-7915.13410
Zhang, Y., Yuan, S., Che, T., He, J. (2021). Agmatine and glycolipid metabolism. Zhong Nan Da Xue Xue Bao Yi Xue Ban 46 (8), 889–893.
Zhang, X., Zhao, Y., Xu, J., Xue, Z., Zhang, M., Pang, X., et al. (2015). Modulation of gut microbiota by berberine and metformin during the treatment of high-fat diet-induced obesity in rats. Sci. Rep. 5, 14405. doi: 10.1038/srep14405
Zheng, L., Kelly, C. J., Battista, K. D., Schaefer, R., Lanis, J. M., Alexeev, E. E., et al. (2017). Microbial-derived butyrate promotes epithelial barrier function through IL-10 receptor-dependent repression of claudin-2. J. Immunol. 199 (8), 2976–2984. doi: 10.4049/jimmunol.1700105
Keywords: sepsis, intestinal microbiota, aged mice, adult mice, 16S
Citation: Yuan Y, Liu S, Ding X, Li Y, Zhang X, Song H, Qi X, Zhang Z, Guo K and Sun T (2022) Early intestinal microbiota changes in aged and adult mice with sepsis. Front. Cell. Infect. Microbiol. 12:1061444. doi: 10.3389/fcimb.2022.1061444
Received: 04 October 2022; Accepted: 29 November 2022;
Published: 27 December 2022.
Edited by:
Fan Deng, Southern Medical University, ChinaReviewed by:
You Shang, Huazhong University of Science and Technology, ChinaCopyright © 2022 Yuan, Liu, Ding, Li, Zhang, Song, Qi, Zhang, Guo and Sun. This is an open-access article distributed under the terms of the Creative Commons Attribution License (CC BY). The use, distribution or reproduction in other forums is permitted, provided the original author(s) and the copyright owner(s) are credited and that the original publication in this journal is cited, in accordance with accepted academic practice. No use, distribution or reproduction is permitted which does not comply with these terms.
*Correspondence: Tongwen Sun, c3VudG9uZ3dlbkAxNjMuY29t
†These authors have contributed equally to this work
Disclaimer: All claims expressed in this article are solely those of the authors and do not necessarily represent those of their affiliated organizations, or those of the publisher, the editors and the reviewers. Any product that may be evaluated in this article or claim that may be made by its manufacturer is not guaranteed or endorsed by the publisher.
Research integrity at Frontiers
Learn more about the work of our research integrity team to safeguard the quality of each article we publish.