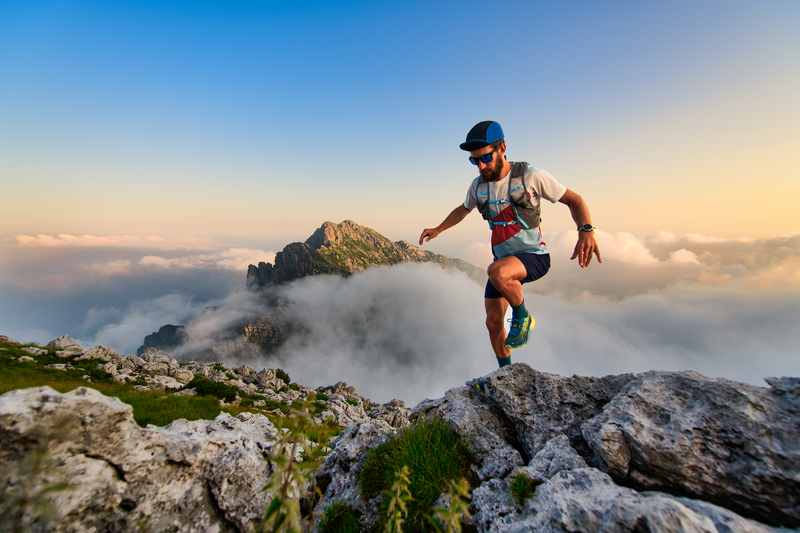
95% of researchers rate our articles as excellent or good
Learn more about the work of our research integrity team to safeguard the quality of each article we publish.
Find out more
REVIEW article
Front. Cell. Infect. Microbiol. , 21 September 2022
Sec. Biofilms
Volume 12 - 2022 | https://doi.org/10.3389/fcimb.2022.1003033
This article is part of the Research Topic Novel approaches in the prevention of bacterial biofilm formation View all 8 articles
Biofilms are colonies of bacteria embedded inside a complicated self-generating intercellular. The formation and scatter of a biofilm is an extremely complex and progressive process in constant cycles. Once formed, it can protect the inside bacteria to exist and reproduce under hostile conditions by establishing tolerance and resistance to antibiotics as well as immunological responses. In this article, we reviewed a series of innovative studies focused on inhibiting the development of biofilm and summarized a range of corresponding therapeutic methods for biological evolving stages of biofilm. Traditionally, there are four stages in the biofilm formation, while we systematize the therapeutic strategies into three main periods precisely:(i) period of preventing biofilm formation: interfering the colony effect, mass transport, chemical bonds and signaling pathway of plankton in the initial adhesion stage; (ii) period of curbing biofilm formation:targeting several pivotal molecules, for instance, polysaccharides, proteins, and extracellular DNA (eDNA) via polysaccharide hydrolases, proteases, and DNases respectively in the second stage before developing into irreversible biofilm; (iii) period of eliminating biofilm formation: applying novel multifunctional composite drugs or nanoparticle materials cooperated with ultrasonic (US), photodynamic, photothermal and even immune therapy, such as adaptive immune activated by stimulated dendritic cells (DCs), neutrophils and even immunological memory aroused by plasmocytes. The multitargeted or combinational therapies aim to prevent it from developing to the stage of maturation and dispersion and eliminate biofilms and planktonic bacteria simultaneously.
Bacteria have much longer history than human beings, paleontologists believe that bacteria arose from the collision and reaction of various inorganic and organic materials in the ancient extreme earth environment billions of years ago, and therefore have some stronger environmental adaptability than we human. These advantages in bacterial adaptability are reflected not only in their constantly gene mutations, bacterial spores, capsule and flagella, but also in getting together to counteract disturbances, known as biofilms: a complex multicellular and animated lifestyle, which is one of the most wide-spread and predominant living pattern on earth. The terminology biofilm was firstly documented in the fields of microbiology on environmental technology, and was not introduced into medicine for the first time until 1982 by Dr. Costerton, as he observed that microbial community aggregated on the surface of a cardiac pace-maker catheter and formed biofilm matrix (Marrie et al., 1982). The self-produced extracellular polymeric substances (EPS) are mainly comprised of polysaccharides, proteins, eDNA and overexpressed reductive glutathione (GSH) (Flemming et al., 2016). The all above are responsible for protecting the bacterium from deleterious environmental conditions, including antimicrobial agents and the physiological immune system (Teirlinck et al., 2018). Additionally, the constituents of EPS are greatly diversified, relying on nutritional availability, host conditions and physical stresses strength. A couple of studies have recently validated that eDNA may be an important and essential component of the biofilm matrix by adding DNase to the surface of mature biofilms in vitro experiments and detecting structure damaged.
Besides, the biofilm-induced inflammation can lead to DNA damage in host cells, thus increasing the risk of cancer (Parsonnet, 1995; Jobin, 2014; van Elsland & Neefjes, 2018). Microbial biofilm-related persistent infections and tissue damage are becoming extremely difficult to treat and eradicate permanently by conventional antibiotic therapy due to their intrinsic resistance to antibiotic agents (sensitivity only up to 1/1000 of previous) compared to plankton in the flowing milieu (Sundin et al., 2020).
The mechanisms of bacterial antibiotic resistance, such as efflux pumps, antibiotic-modifying enzymes and gene mutations, are well investigated, but they are limited to planktonic bacteria (Sun et al., 2020; Xiu et al., 2022). Novel evidence indicates that bacteria situated inside biofilms have lower oxygen and nutrient levels, which means a lower micro-metabolic level (Zhang et al., 2019). Exactly this resulted in the enhancement of tolerability to antibiotics that chiefly target metabolically active bacteria. Furthermore, biofilm can spread as a source of infection to nearby healthy sites. The switch between single planktonic and complex biofilms is a key factor for bacteria to trigger different infectious diseases, including prosthetic joint infections (PJIs), infective endocarditis and osteomyelitis (Peng et al., 2019). Bacteria are always vulnerable to antimicrobial agents once they scatter out of biofilms, which indicates that the antibiotic resistance of bacteria in biofilms isn’t acquired through mutations. The explicit mechanism has not been utterly researched and has been the topic of comprehensive investigation until now. Especially in a surgical implant, once the biofilm formed, there is very few viable treatments or managing options available (Xie et al., 2018). Although bacterial antigen can spur generation of antibodies, they are unlikely to slay bacterium inside biofilms efficiently. On contrast, they accompany with unfavorable immune complexes to nearby healthy cells expect for monoclonal antibodies (Raafat et al., 2019). The persistence of biofilms can lead to medical implants fault or biological material deterioration as well. Above all, these are exactly why bacterial biofilm related diseases, such as osteoarthritis, valvulitis, rhinitis, cystitis, vaginitis, periodontitis, specific pneumonia, otitis, etc., of which implant-associated infections are the most common, are currently the culprits of chronic infections bringing tremendous survival hazards to mankind (Luis Del Pozo, 2018). While bacterial biofilms develop over the exterior of surgical implants (for example, a prosthesis of the knee joint), they tend to aggregate inflammatory compositions and secrete metabolic substances, resulting in an acidic multi-radical microenvironment, which contributes to the failure of wound healing (Rumyantceva et al., 2021). Most secondary surgeries for implant removal in clinical practices are linked closely to biofilm development by Staphylococcus aureus (S. aureus). Especially, methicillin-resistant Staphylococcus aureus (MRSA) and Vancomycin-resistant Staphylococcus aureus (VRSA) have caused grave damages to many people (Sun et al., 2020). We argue that the treatment of biofilms should be focused on their adhesion and formation phases, since biofilms in this phase have not yet developed their distinctive resistance properties. As well, since new biofilms usually originate from the dispersion of previous existing biofilms, blocking dispersion of the previous biofilm also falls under the key aspect of preventing the formation of new biofilms. Thus, methods to interfere with mature biofilm dispersion will also be discussed in our review as a complementary part.
Methods conventionally used for biofilm treatment have their limitations:
(i) Surgical sterile precautions and techniques aim to prevent infection, but often fail to achieve sterilization in the treatment of acute contaminated wounds and the curative rate of debridement is not entirely satisfying (Gilbert-Girard et al., 2020); (ii) Eluting devices and wound by using anti-microbial or antibiotic solutions to inhibit early planktonic adhesion to infixed devices, while effective, can sometimes compromise the function of certain devices and accelerates the emergence of antibiotic-resistant bacteria; (iii) Injecting agents to disrupt established biofilms and eliminate microbes within them is not a common clinical treatment, but often causes cause secondary damage to the patient without guarantee efficacy.
As of today, removing the contaminated equipments continues to be the most effective therapeutic option, which is extremely painful and brings not only multiple surgeries, but also considerable harm and financial burden to patients. We therefore review numerous new therapeutic strategies that differ from the above conventional approaches, since these strategies are characterized by precise and even targeted therapies based on the characteristics of each period of biofilm formation. Here, we systematize the therapeutic strategies into three main periods precisely: (i) period of preventing biofilm formation: interfering the colony effect, mass transport, chemical bonds and signaling pathway of plankton in the initial adhesion stage; (ii) period of curbing biofilm formation: targeting several pivotal molecules, for instance, polysaccharides, proteins, and eDNA via polysaccharide hydrolases, proteases, and DNases respectively in the second stage before developing into irreversible biofilm; (iii) period of blocking biofilm dispersion to prevent the sprouting of new biofilms: applying novel multifunctional composite drugs or nanoparticle materials cooperated with ultrasonic, photodynamic, photothermal and even immune therapies. With all these curative strategies, the structure and function of biofilms are compromised by different mechanisms and efficiencies across different phases of biofilm formation. Herein, we review the characteristics of each period of biofilm formation and the corresponding innovative healing therapies in detail.
As a dynamic biological system, biofilm has evolved a large number of networks to coordinate biofilm formation in different outer circumstances. Formation steps of biofilms are closely modulated through multiple modulatory chains (Tolker-Nielsen, 2015). The physiological status of bacteria, subtle variations in ambient settings and the ever-changing events inside the bacterial colonies are all underlying signaling factors that impact on biofilm formation. Many works have been done towards identifying and characterizing bacterial physiological activity at the cellular level, and these technological advances will contribute to understanding the complex biofilm-forming mechanisms and developing novel specific therapeutic approaches (Qu et al., 2020). Activating biofilm generation by regulatory pathways will guarantee that bacteria do not establish biofilm under adverse circumstances. It is evident that the establishment of a steady biofilm is regulated by multiple factors, and part of these engage as two-component signaling pathways (Landry et al., 2018). The overall processes of biofilm formation are shown in Figure 1.
Figure 1 From planktonic bacteria to mature biofilms. (A) Planktonic bacteria invade the wound and swim randomly. (B) Planktonic bacteria adhere to the implant surface, which is a reversible process. From forming microcolonies (C) to developing into mature biofilms (D). (E) Biofilm breaks down and bacteria spread outside.
Reversible adhesion is usually initiated by the non-specific forces (van-der Waals, Brownian motion and electrostatic forces) haphazardly and then followed by the irreversible adhesion on the surface via a variety of different factors and mechanisms to form a complicated three-dimensional colony that is known as a biofilm (Dixit et al., 2019). Bacteria can sense contacts with surfaces and accordingly adjust gene expression to facilitate stable cell-surface interactions. Superficial adhesion of planktonic cells primarily contact substrates of the host organism during infection with various adhesins, such as fibrinogen, fibronectin and collagens such as fibronectin binding proteins (FnBPs) and fibrinogen-binding clumping factors (Clfs) (Palmqvist et al., 2005).
Environmental signals inside the biofilm allow bacteria to efficiently colonize their preferred environment. Previous studies have demonstrated that under extreme conditions of nutrient enrichment or deprivation, bacteria are more likely to be in the planktonic mode, as this provides them with greater access to nutrients and new habitats. Satisfactory meld of implants with host tissues or plankton is the cornerstone of implant success. At the moment the biomaterials implanted, the competition for surface between host cells and bacteria starts. Once planktonic bacteria preempt to adhere on the surface, the innate immune system alone can hardly prevent the biofilm formation (Dapunt et al., 2020). Thus, the architectural features of the implant itself have a critical impact on the occurrence of bacterial infections.
The bacterial flagella and pilus are the two main power sources in the planktonic state, which are motivated by ion inflow via membrane-spanning motor complexes. The flagella are generally several folds longer than the bacteria itself and is the most powerful motor to drive the plankton towards the surface of the implants. When the bacteria sense that the surrounding environment is favorable for their proliferation, the flagella will anchor to the surface, otherwise they will drive the bacteria away to find better habitats (Guttenplan & Kearns, 2013). S. aureus have no flagella and therefore may rely on bacterial pilus and other signaling agents to mediate attachment to a surface.
The shear stress, which constantly varies with positions and fluid flows, acts as another key factor affecting the initial adherence of the plankton. Increased shear stress enhances the polysaccharide intercellular adhesin (PIA) expression of the bacteria to accommodate the shear stress (Schaeffer et al., 2016). Lectins also facilitate and consolidate the surface adhesion process of planktonic bacteria, especially the effect of surfactants to reduce the surface tension of the contact surface is critical to stabilize the later formed micro-colonial structure (Shi et al., 2021). Besides, cell wall anchoring (CWA) proteins promote planktonic bacteria to bind with surface matrixes (Bannoehr et al., 2011; Milles et al., 2018). The bacterial FnBPs, Clfs, CWA proteins and serine-aspartate repeat (Sdr) proteins are collectively referred as microbial surface components recognizing adhesive matrix molecules (MSCRAMMs), which can modulate interactions between host extracellular matrix components and microbes (Flick et al., 2013). Such recognizing adhesion molecules are strain-specific, for example the adhesin involved in diffuse adherence (AIDA-I) is involved in the initial diffuse adhesion of E. coli, while P. aeruginosa is rely on the lectins (Charbonneau et al., 2007). The adhesion regulatory factors are summarized in Table 1.
In the process of biofilm formation, bacteria regulate the interconnection of adhesion factors within the matrix via a distinctive info-communication webs. Ultimately, a sophisticated three-dimensional tower-like biofilm structure is formed. As soon as the bacterial settle on the surface successfully, the bacteria begin to multiply and excrete all kinds of extracellular substances to envelop themselves, which marks the beginning of irreversible adhesion and opens the portal to biofilm formation. While numerous studies have been conducted on the composition of bacterial extracellular secretions, the exact components remain uncertain. The major known compounds consist of (i) extracellular polysaccharide, the main component of which is polysaccharide intercellular adhesin (PIA) produced by icaADBC locus with strong ability to promote adhesion; (ii) eDNA, which is presumed to be generated by bacteria either by active apoptosis or passive autophagy to facilitate the survival of bacterial community (Valle et al., 2019). And eDNA can stabilize the biofilm matrix and enhance gene exchange between bacteria, and may even be a key factor in promoting bacterial gene mutations (Lei et al., 2019). It also triggers immune responses when eDNA interacts with host immune cells, but the ultimate effect of such immunity is anti-inflammatory, which is not conducive to biofilm clearance, instead leading to refractory and chronic infections; (iii) diverse proteins that mediate adhesion and signaling communications, e.g., Bap, the surface protein of S. aureus, can build amyloid scaffolds to stabilize bacterial accumulation (Taglialegna et al., 2016). And some bacteria secrete amyloid curli, a protein that can bind to eDNA to forge firm fiber-like polymers inside biofilms and react with host cells to yield immunogenic complexes that can activate a variety of immune cells, including DCs characterized by the production of cytokines such as type I interferon (IFN-I) (Yan et al., 2020); (iv) phospholipids, which can be covalently coupled to peptidoglycan to form wall teichoic acids (WTAs) to increase bacterial attachment towards implant by binding with fibronectin or to cytoplasmic membranes to form lipophospholipids. Besides, it has also been reported that bacterial secreted virulence agents and ribosomal proteins also contribute to the stabilization of biofilms. Bacteria possess a specific surface sensing system, and the system can be activated within minutes after the bacteria adhere to the surface.
Regulation of QS is almost throughout biofilm formation. The high density of bacteria within biofilms enables a variety of signaling communications among them, which relies on bacterial density to coordinate signaling transductions (Wu et al., 2022). And QS can link diverse elements that are responsible for enabling the necessary virulence and metabolism to maintain the survival of bacteria (Carnes et al., 2010).
The accessory gene regulator (agr) is a bioregulator of Gram-positive pathogens, especially for Staphylococcus (Kavanaugh & Horswill, 2016; Jenul & Horswill, 2019; Qu et al., 2020). Agr QS can directly regulate the virulence and adhesion of S. aureus (Hardy et al., 2019). With low expression of agr, S. aureus tends to form biofilms by secreting more intercellular adhesins and reducing the secretion of toxins (Valliammai et al., 2019). Conversely, increased intracellular expression of AgrD is followed by the secretion of autoinducer peptide (AIP) (Kim et al., 2017).The detailed composition of Agr varies between strains. The extracellular AIP concentration increases proportionally with increasing cell density. Once the local AIP concentration meets a threshold level, AIP binds to AgrC to activate the AgrC-AgrA two-component system. The activated Agr system restrains the expression of AtlE (an essential adhesive protein involved in biofilm construction), thereby inhibiting biofilm formation and enhancing the expression of virulence factors such as pore-forming toxins and tissue-degrading enzymes, reinforcing the bacterial resistance to external harmful substances such as ROS.
Further tests in vitro proved that the addition of AIP can activate the agr-mediated breakage of S. aureus biofilm, and the bacteria will restore to the planktonic state, thus completing the biofilm life cycle. This may be due to the fact that Agr activation can lead to elevated levels of staphylococcal proteases that cleave bio-membrane proteins and disrupt intercellular interactions within the biofilm, and that proteases can also be applied to the biofilm. There are also matrix-degrading materials, such as dispensin B, which can cause biofilm disintegration by weakening the structural integrity of the biofilm matrix. Activation of the agr system induces the expression of phenol-soluble modulins (PSM), a low molecular weight pore-forming toxin with surfactant-like properties. S-ribosylhomocysteine lyase (LuxS) is involved in production of autoinducer 2 (AI-2).
There are certain alternative regulatory pathways that are also relevant to the formation of biofilms, which allow bacteria to micro-modulate their response to ever-changing environmental conditions and moderate biofilm formation. Intracellular second messengers, such as cyclic dinucleotides (cDN), c-di-GMP, have multiple physiological and immunomodulatory abilities in bacteria, often enhancing bacterial virulence and biofilm formation. Besides, cyclic dimeric (3′→5′) GMP (c-di-GMP) induces the production of interferon-gamma (IFNγ) to prolong the host type I interferon immune response.
A dual regulatory CpxA/CpxR signaling system relies on the outer membrane protein NlpE, acting as a direct sensor of E.coli for surface contact. The Cpx pathway becomes activated in response to the interaction of E.coli with hydrophobic surfaces, accelerating the oscillation of pilus to drive bacteria moving. EnvZ/OmpR signaling system is another dual component signaling pathway used in E. coli, can be activated under elevated osmolarity to strengthen the adhesion of bacteria to biological surfaces to counteract the adversity. If under excessive osmotic pressure, EnvZ/OmpR signaling will be overtaken by a negatively regulated system, which means the bacterial cells will remain in the planktonic mode and freely migrate to more favorable conditions.
Mature biofilms may seem to be thick and homogeneous cell cushion structures, but they are complex architectures feature with a hydraulic tunnel structure in order to keep nutrients inflowing and wastes out just as Figure 2 presented. Such a complex structure is not exclusively controlled by physical factors, such as shear. In fact, several modulatory variations have already influenced the integral deepness and structure of the mature biofilm. And c-di-GMP is another ubiquitous second messenger with potent immunomodulatory properties in biofilm, stimulating innate immunity and regulating biofilm formation, planktonic motility, and virulence. Besides, c-di-GMP can bind to a wide range of receptors, including enzymes, splice proteins, transcription factors and nucleoprotein switches, enhancing the recruitment of neutrophils, macrophages, natural killer cells and even DCs to kill bacteria. Low doses nitric oxide (NO) signaling has been shown to stimulate specific phosphodiesterases (PDEs), triggering c-di-GMP degradation and concomitant diffusion of bacterial biofilms. In vitro, low doses of NO are the primary dispersion-driven mediator, and release of NO contact with biofilms can be targeted to enhance antimicrobial efficacy while limiting potentially toxic effects on target tissues. In E. coli, high levels of c-di-GMP enhance the adhesion of planktonic bacteria and promote biofilm formation, while it drives biofilm rupture at low levels, allowing the internal bacteria to spread out. In S. aureus, this second messenger role is assumed by c-di-AMP. A number of small regulatory RNAs (sRNAs) regulate targets associated with bacterial colony behavior, including QS (Malgaonkar & Nair, 2019).
Figure 2 Three-dimensional reconstructions of the fluorescence-labeled (scale bars, 30 µm.) and Scanning electron microscope images (scale bars,1 µm) of (A) mature E. coli and MRSA biofilms; (B) damaged biofilm treated with photodynamic nanomaterials. Copyright © 2022, Advanced science.
The formation of microcolonies by planktonic bacteria is a critical step in biofilm formation. A microcolony is usually a three-to-five-layer-deep colony of bacterial cells that evolves as bacterial cells adhere to the surface. The development of stable interactions between single bacteria and the surface alone is insufficient to form the microcolony; Some destructive factors such as nucleases and PSMs break down the biofilms leading to catastrophic secondary mass release of bacteria and the spread of inflammation. Bacterial spreading during biofilm rupture is mainly driven by proteases and PSMs to degrade and disrupt the biofilm matrix synergistically (Hommes et al., 2021). Each stage of biofilm formation is influenced by different factors just as shown in Figure 3. Factors associated with biofilm maturation and diffusion are summarized in Table 2.
Traditional treatments for bacterial infections fail to clear biofilm related chronic infections in vivo, and the abuse of high dosages of antibiotics for a prolonged period is obviously inadvisable, since in many cases it induces drug resistance and further biofilm development (Yan & Bassler, 2019). Most current therapies target the acute exacerbation of infection caused by the release of planktonic bacteria but do not focus on the characteristics of biofilm growth to prevent its formation before it fully matures. Until now, surgical excision of the infected implant and wound debridement are still the principal method of biofilm elimination, but this is not always feasible because of the large physical trauma and the high risk of complications (Beebout et al., 2021). Apart from the mechanisms such as efflux pumps and genetic mutations which exist in usual drug-resistant bacteria, bacteria within biofilms have some specific tolerant mechanisms. Antibiotics may function in inhibiting the further progression of biofilms. Yet, they are unable to kill them. It has long been assumed that an essential mechanism of biofilm resistance is due to the complex matrix components such as eDNA and polysaccharides contained in biofilms to sequester drugs. However, further studies have shown that the reality is not so easy. Tetracyclines can infiltrate biofilm to cover all bacteria within the E. coli biofilm in less than 10 minutes at the cost of losing their killing capacity. Thus the biofilm does not simply rely on blocking the antibiotic to exert resistance. It may be a more important mechanism to slow the penetration of the antibiotics and giving the bacteria enough time to develop resistance (Grygorcewicz et al., 2020). In addition small colony variants (SCVs) inside biofilms are strongly associated with persistent and recurrent osteomyelitis and implant-associated infections, especially in S. aureus biofilms (Tuchscherr et al., 2015). β-lactamases that degrade antimicrobials are also present in the biofilm matrix, further preventing the drug from reaching the cells inside. Therefore, we present innovative approaches based on targeting on each period of clinical biofilm formation, such as the application of QS inhibitors (QSI) targeting the QS system, drugs that interfere with bacterial metabolic pathways, reagents that can destroy the biofilm components and even combine with activated immune cells such as DCs and macrophages to kill bacteria.
Planktonic bacteria reproduction and migration is essential for subsequent bacterial colonization in dynamic fluidic milieu. Despite planktonic bacteria possessing robust mobility, the concentration of agents required to kill them tends to be only one thousandth of the biofilm (Fan et al., 2017). Therefore, targeting planktonic bacteria is critical for early containment of biofilm formation.
Interfering the signaling transductions between bacteria. Disrupting QS-related signaling pathways to restrain the biofilm associated gene expression is considered as an advisable approach to curb biofilm formation. Previous studies have well recognized that genetic variations and bacterial transcriptome can be modulated by suppressing QS (Cole et al., 2018). QS governs the expression of bacterial virulence factors, and therefore blocking QS can largely diminish the virulence of bacteria (Piewngam et al., 2020). QSI act primarily through five interactions with QS signaling molecules: (i) inhibition of synthesis; (ii) acceleration of degradation; (iii) competition for receptor sites; (iv) inhibition of gene expression; (v) removal of AIs (Kalia et al., 2019). For example, mutations in the LasR/pqsH/cdpR gene appear to influence QS in biofilm, which have inspired the creation of gene-targeted drugs (Kuang et al., 2020). While urea in urine has been reported to suppress bacteria by interfering with the QS signaling communication (Kang et al., 2017). Due to this, some experts argue that QS is almost negligible in urinary tract infections (Feltner et al., 2016; Kang et al., 2017; Cole et al., 2018). The occurrence ascribed to downregulation or silencing of the QS system is called quorum quenching (Rajput & Kumar, 2017). Most QS rely on acyl-homoserine lactone (AHL), AIP and AI-2 for communication (Kim et al., 2017; Kim et al., 2018). Therefore, QS can be modulated by using analogs of the above signaling molecules (Billerbeck et al., 2018).
As already mentioned previously, host reactions with FnBPs and ClfA are decisive drivers of bacterial virulence, and therapies based on intervening FnBPs and Clfs may be another effective way to prevent initial adhesion (Flick et al., 2013).
Autophagy originally refers to the degradation behavior of long-lived proteins and organelles in eukaryotic cells upon binding to lysosomes. Recently, autophagy has been found to be linked to infectious conditions and plays a vital role (Huang et al., 2011). During bacterial infection, autophagy can be activated by a variety of host factors and pathways, including the formation of autophagosomes around the targeted bacteria and then transferring these pathogens to lysosomes for further degradation (Sparrer et al., 2017). However, bacteria have evolved multiple strategies to interfere with autophagic signals to avoid autophagy, and in some cases bacteria can even utilise autophagy to benefit their survival (Holla et al., 2014). Autophagosomes begin at the phagocytic assembly site (PAS), an endoplasmic reticulum (ER) sub-structural domain rich in phosphatidylinositol 3-phosphate (PtdIns3P) (Huang & Brumell, 2014). A set of autophagy-associated (ATG) proteins is a critical player in precisely regulating autophagy (Moreau et al., 2014). Studying the interactions among bacterial factors and ATG proteins will be emphasized in the future for the treatment of bacterial infections. In physiological conditions, complement protein C3 is deposited on invasive pathogens, and Matthew T Sorbara et al. utilized C3 complement to trigger antimicrobial cell autophagy intracellularly (Sorbara et al., 2018). The role of selective macroautophagy in targeting intracellular pathogens for lysosomal degradation is a relatively well-established direction as well as the immune effect of xenophagy (Kimmey & Stallings, 2016). Although autophagy mainly eliminates planktonic and intracellular bacteria, this is prospective for the prevention of biofilm formation (Wu & Li, 2019).
Modulation of the surface where bacteria adhere with the increasing popularity of surgical implants, bacterial adhesion infection is becoming more common. Therefore, it is an innovative approach to make anti-bacterial implant by modifying their surface characteristics. The application of biocides coated on the surface of implants is an effective inhibitor of biofilm formation, but has not been used on a large scale in clinical practice due to toxicity and other limitations. Natural and effective biofilm inhibitor coatings to alter the surface of implants have become the focus of new research. Multi-species biofilms formed by Escherichia coli and S. aureus can be effectively eliminated by coating with various silver coatings (Hou et al., 2020; Lee et al., 2021). Nanotopologies kill bacteria within minutes by penetrating their membranes with mechanical force. Duy H K Nguyen et al. developed a 35 nm diameter silica nanopillar to rupture P. aeruginosa and the sterilizing rate was up to 85%, but less than 10% for S. aureus, indicating the effectiveness and non-broad-spectrum limitations of nanomaterial topography for antibacterial activity (Nguyen et al., 2019). Nanostructured mechanical sterilization is assumed to be realized by piercing the phospholipid bilayers of microbes. Titanium surfaces with pocket-type nanostructures killed nearly 50% of the initially adherent bacteria and effectively prevented potential recurrence (Cao et al., 2018).
Antimicrobial peptides (AMPs), which target bacterial membranes, are generally amphiphilic cationic micromolecules consisting of 10-50 amino acids with broad-spectrum antimicrobial capacity and retaining high sensitivity to metabolically dormant bacteria in biofilms (Jiang et al., 2022). AMPs are commonly applied in combination with antibiotics and other anti-biofilm compositions to combat biofilms. As an integral member of innate immunity, the host defense peptide (HDP) directly targets planktonic cells and exhibits both anti-biofilm and host-directed immunomodulatory activity. Recently, there have been increasing reports referring to the emerging anti-biofilm properties of HDPs that are different from those previously recognized. Meanwhile, the synergistic effects with other therapeutic approaches such as antibiotics are making HDPs shine in the fight versus biofilms. Daniel T. Cohen et al. synthesized antimicrobial peptide-vancomycin complexes by using coupling chemistry and proved the broad-spectrum antimicrobial effect of the complexes exceeding vancomycin in vitro (Cohen et al., 2019).
The exploitation of antimicrobial nanomaterials as a substitute for antibiotics is currently a hot spot in medicine with promising prospects. The micro diameter of the nanomaterials is pivotal to their functions. However, a considerable number of nanoparticles tend to aggregate in solution, which may limit their application with photothermal therapy (PTT)/Photodynamic therapy (PDT). An effective approach to overcome this barrier is to use polymers (e.g., PEG, and BSA) to carry them as well as to conduct the necessary surface functionalization to enhance dispersibility and biocompatibility (Jiang et al., 2022).
In the last century PTT has entered a phase of rapid development and is widely used to fight against cancer and microbial infections (Cheng et al., 2015; Qi et al., 2018; Deng et al., 2020; Yang et al., 2020). PDT requires applying specific wavelengths of light upon photosensitizers to produce reactive oxygen species (ROS) in the specific inflammatory microenvironment, which can lead to bacterial damage and even death. However, excessive amounts of ROS may also harm normal tissues and be detrimental to wound recovery, which is one of the challenges to be overcome for novel photothermal therapies (Mitsunaga et al., 2011). As regards how PDT kills bacteria hidden within the biofilm, it may be due to the destruction of the channels engaged in the transport of nutrients to the core region and the loss of biofilm integrity (Xie et al., 2018). In recent years, many nanomaterials possess both excellent antibacterial properties and good biocompatibility, some of which have strong killing effect on drug-resistant bacteria. However, these photothermal effects cause certain damage to normal tissues while exerting antibacterial properties, which is not conducive to wound recovery. Therefore, the preparation of low-cost, rapid and effective antibacterial and tissue repair nano-composite materials has become one of the research frontiers. From a synergistic perspective, the combination of PDT and PTT maximizes efficacy and meanwhile minimizes side effects (Zou et al., 2019).
Triggered gas-releasing nanoparticles that serve as gas donors or gas carriers to apply as a substitute to conventional antibacterial drugs in medicine. Bactericidal gase s, such as hydrogen sulfide (H2S), NO and hydrogen (H2), are emerging to provide for efficient gas therapy for infectious illnesses combined with an excellent biosafety in vivo (Su et al., 2022). Gas therapy is frequently synergized with near infrared (NIR) stimulated phototherapies (e.g., PTT or PDT). NO gas exhibit powerful anti-biofilm efficacy primarily through mediating bacterial DNA damage. Besides, NO has the potential to eradicate bacterial biofilms in host by stimulating M1 polarization of macrophages. In contrast, the anti-inflammatory properties of carbon monoxide (CO) and NO can mitigate the inflammation-related response in the anti-biofilm process. H2S is another DNA-damaging mutagen, but it exerts an anti-inflammatory effect by facilitating the polarization of M2 macrophages, i.e., it has the advantage of both NO and CO, which can diminish the negative effects of PTT while improving the therapeutic effect and eliminating the biofilm. Up-to-date researches focus more on the smart responsive gas release function of nanoparticles to achieve spatio-temporal regulation, thus improving the sustainability and controllability of treatment. Nano-carrier MoS2-BNN6 can not only effectively treat Escherichia coli and S. aureus, but also provides precise control of NO release by irradiating with 808 nm laser. Then, Mos2-BNN6 destroys cell membranes through PTT/NO, which synergistically induced ROS. At the same time, MoS2 also accelerated the oxidation of GSH under 808nm irradiation, destroyed the balance of antioxidant in bacteria, shortened the treatment time, and achieved efficient bacterial inactivation within 10 minutes (>97.2%). In addition, mos2-BNN6 nanocarriers can release NO at low concentrations after infection control, promoting tissue repair (Gao et al., 2018). AI-MPDA, an integrated phototherapy nanoplatform composed of L-Arginine (L-ARG), indocyanine green (ICG) and mesoporous polydopamine (MPDA), provides PTT and PDT via generating ROS to induce the l-ARG cascade catalytic release of NO (Yuan et al., 2020). In April 2021, a Chinese scholar reported a dual-acting nanoparticles, deoxyribonuctinase I (DNase I)-CO-mesoporous polydopamine nanoparticles (MPDA NPs), featured controlled release of CO gas by NIR. DNase-CO@MPDA NPs that can effectively eliminate methicillin-resistant MRSA biofilms were made by encapsulating the photosensitive CO donor FeCO in MPDA NPs and then covalently anchoring DNase I on the surface of MPDA NPs. under NIR irradiation, the released DNase I can degrade eDNA in biofilm and disrupt the outer sphere of biofilm. Simultaneously, CO gas is released, which can fully infiltrate the damaged biofilm and thoroughly eradicate the residual bacteria. Finally, NIR-activated DNase I-CO@MPDA NPs promotes healing of infected skin wounds by locally accelerating CO release, which may be related to CO increasing mitochondrial biogenesis and driving mitochondrial increased ATP production (Yuan et al., 2020). Jun Li et al. published an exogenous antibacterial agent composed of Zinc-doped Prussian blue (ZnPB), which can accelerate the emission and infiltration of ions into microbes by local heat induced by photothermal effect, resulting in changes in cellular metabolic changes. Besides, ZnPB upregulates the expression of genes involving in cell proliferation, promoting collagen deposition and facilitates wound healing (Li et al., 2019).
Gallium (Ga)-based nanoparticles has been tested to eradicate biofilms in mice and achieved an excellent result in vivo and in vitro. The PDT effect induced by ICG-Ga NPs destroys bacterial membranes and accelerates the endocytosis of Ga3+, which substitutes ions in bacteria with Ga3+ blocks bacterial iron metabolism, exerting a synergistic effect of bacterial killing and biofilm destruction. The ultra-small size of ICG-Ga NPs can be removed rapidly by the kidney, guaranteeing the biocompatibility (Xie et al., 2021). Another recently proposed article of the exact opposite mechanism of anti-inflammatory action of classical Ga ions (Ga3+) via delivering Ga nanodroplets (GNDs) to lipopolysaccharide-induced macrophages. GNDs exerted a selective inhibition of NO generation without interfering with the accumulation of pro-inflammatory agents by disrupting the synthesis of inducible NO synthase in activated macrophages through up-regulation of eIF2α phosphorylation levels, without disturbing Fe homeostasis (Zhang et al., 2022). A lipophilic Ga complex, Ga2L3(bpy)2, has both Ga (disruption of iron metabolism) and ligand effects (production of ROS) in the fight against drug-resistant bacteria (Wang et al., 2021).
As a star material of the century, graphene and its derivatives have got a splash in the medical field due to its favorable biocompatibility and antibacterial potential (Cao et al., 2021). Compared to other nanocomposites, graphene is cheap, environmentally friendly and easy to manufacture. The graphene oxide (GO) can produce mechanical damage and oxidative stress to biofilms (Palmieri et al., 2017). An innovative protective coating based on graphene and hydrogels has been proposed as new anti-biofilm coating material to prevent microbial adhesion (Cacaci et al., 2020). GO films can be used as biocompatible sites for bacterial adhesion on their surfaces (Ming et al., 2020). Graphene-based nanomaterials (GBNMs), with their unique structures and extraordinary physicochemical properties, have been intensively investigated and widely used in many biomedical fields to improve bactericidal efficacy and reduce adverse effects in the treatment of bacterial biofilm (Wang et al., 2022).
It is known that biofilms preserve their dominance by suppressing host immunity, and thus modulating body immunity to regain a proactive status is a pressing medical issue (Heim et al., 2020). The studies of the interaction between bacteria and the host immune system to adapt and develop strategies for the treatment of bacterial infections is an extremely promising new path. Intrinsic immunity has evolved with the body over tens of thousands of years and has a well-established and elaborated system, and the skillful activation of some beneficial immune capacity can be pivotal and essential in combating biofilms (Campos & Zampieri, 2019). The most prospective is the activation of in situ immunity via advanced biomimetic nanomaterials that act as vaccines to produce a powerful and sustained antimicrobial effect with self-immune cells, such as neutrophils, macrophages, and DCs (Kimkes and Heinemann, 2020).
As antigen-presenting cells, DCs are core in stimulating and mediating the host immune system, and numerous previous works have revealed that DCs perform a key function in initiating antigen-specific immunity and immune tolerance when facing with bacterial infections (Sabado et al., 2010; Cao et al., 2013). The cGas/sting-IFN1 pathway, which is known to sense accumulation of nucleic acids and induce inflammatory responses, is crucial in the immune response of DC cells. The cGas/sting-IFN1 pathway has made significant progress in the treatment of cancer (Thomas et al., 2017; Alicea-Torres et al., 2021). With the presence of biofilm, various inflammatory mediators produced by the bacterial inflammatory response can upregulate DC-Sting by enhancing DCs to engulf bacteria via targeting C-type lectin (CTL), which is specific in the recognition and capture of pathogens by DCs and the subsequent generation of effector T cell (Ramakrishna et al., 2019). However, it has been reported that in vitro co-culture assays of biofilms, DCs maturation got stunted and CTL expression decreases significantly, which means the powerful STING pathway may be inactivated (Srikanth et al., 2019). Eventually, DCs convert from antigen-capturing cells to antigen-presenting cells, leading to adaptive immune dysregulation and persistent growth and random invasion of microorganisms in DCs (El-Awady et al., 2015). Kaya, E et al. reported that significant activation of CD56(+) CD3(-) natural killer cells was observed after co-culture of peripheral blood mononuclear cells (PBMC) and bacterial biofilms. Natural killer (NK) cells exert not only direct antibacterial effects, but also interact with other immune cells through cytokines such as perforin and interferons to generate indirect antibacterial activity. Thus, transmigration of NK cells to local infectious sites may be a promising option (Schmidt et al., 2016)
As effector cells of the innate immune system, neutrophils are involved in a variety of immune inflammatory response processes. Neutrophils exert extracellular neutrophil traps (NETs) through the cell death program of suicidal NETosis. The reticular DNA structure released by the extrusion of genomic DNA is a siege for invading pathogens and preventing dissemination (Dhanesha et al., 2020). Nuclear and granule protein (histone G and proteinase 3, etc.) will soon eliminate the trapped bacteria by binding to the reticular DNA (Dhanesha et al., 2020). S. aureus biofilms skewed neutrophil to neutrophil NETs formation via the combined activity of the leukocyte inhibitor Panton-Valentine leukocyte inhibitor and γ-hemolysin AB causing the antibacterial activity of NETs to be ineffective in eliminating biofilm and even exacerbating biofilm infection (Bhattacharya et al., 2018). Current research aims to treat refractory bacterial infections by targeting neutrophil development and proliferation to regulate the accumulation of neutrophils at the site of infection and to mitigate the deleterious effects of NETs (Nemeth et al., 2020). Augmentation of neutrophil numbers and function by adding G- CSF, inhibiting CXCR4 and blocking CD47-SIRPα interactions may be a therapeutic approach to enhance the function of neutrophils in infections (Németh et al., 2020).
Macrophages function centrally in antimicrobial immunity, with recognition, phagocytosis and bactericidal capabilities (Serezani et al., 2009). There is growing evidence that S. aureus biofilm infection in PJIs establishes an immunosuppressive environment associated with myeloid-derived suppressor cells and M2-macrophages (Peng et al., 2017). Therefore, in the battle against biofilms, it is crucial to convert macrophages from the immunosuppressive M2 type to the antimicrobial M1 type (Lei et al., 2019). Gold nanoclusters (Au NCs) coupled with mercaptopyrimidines can be used as highly effective nanoantibiotics that can target and kill bacteria. The antibacterial mechanism is disruption of biofilm structure, such as eDNA induction of ROS production and macrophage polarization (Zheng et al., 2018). He et al. forged an antimicrobial polymer polyhexamethylene biguanide (PHMB) hybridized with gold nanoparticles (Au NPs) platform (PHMB@Au NPs). PHMB@Au NPs exhibit superior synergistic effect to enhance both photothermal bactericidal effect under NIR irradiation and tissue repair by converting macrophages from M1 type to M2 type (He et al., 2022). The mechanisms and period of action of the various treatments are summarized in Table 3.
There are numerous and disparate approaches to prevent, treat, and eradicate biofilms in clinical practice, but ultimately there is a lack of comprehensive and uniform understanding of the overall physio-pathological process of biofilm-induced inflammation, resulting in a dearth of effective treatments and alternative strategies for serious bacterial infections and chronic refractory biofilm inflammation that occur after surgical operations. The necessity to reasonably tailor the treatment by understanding the characteristics of the biofilm itself. The therapeutic approaches we present above are based on three major components. Firstly, we aim to preempt to thwart the ability of planktonic bacteria to form further biofilms during the migration and initial surface adhesion phase. Secondly, we design to destroy the developing seeds through a combination of therapeutic approaches between initial adhesion and biofilm maturation stages. Thirdly, we plan to stop the spread of planktonic bacteria at the time of biofilm dispersal to contain the development of biofilms.
Conventional antibiotic therapy is often insufficient to eradicate biofilm infections. Rather than the singular therapy of the direct treatment of biofilm formation or proliferation, we recommend combination therapies that are intelligently targeted according to the characteristics of their material composition at each stage of formation. The advantages of these special therapies have been described in detail above, but there are still some shortcomings that need to be overcome.
Numerous studies on interfering QS systems and other signaling pathways have been reported, but there is still a lack of sufficient animal models to confirm the applicability of such methods in vivo. Investigations of QSI in vivo need to be further enhanced and optimized to reach the level of clinical application to better overcome severe inflammations arising from antibiotic-resistant pathogens (Seghal Kiran et al., 2016). Disruption of biofilm structure is also a viable and effective strategy, but it often requires combination of sensitive antibiotics to eradicate the remaining bacteria within the biofilm. Antimicrobial topological surface have been explored for years, but it functions mainly in the early adhesion stage and the underlying mechanisms are still unclear and the diverse effects of topographies such as micropillars, rows and concaves, especially at the nanoscale, need to be explored further (Echeverria et al., 2020).
While local drug injections and ultrasound therapy are still commonly used to combat implant infections, these alone are not sufficient to deal with recalcitrant drug-resistant bacteria, and surgical debridement is often ineluctable eventually. Hence, combining treatments depending on the period of biofilm formation described in this review will be necessary to eradicate the biofilm utterly.
The application of nanomaterials has opened a new chapter in the field of antibacteria, but the impacts of the materials themselves on the host remains a thorny issue that cannot be avoided. Admittedly, although nanotechnology and membrane-coatings enable materials to be more biocompatible and powerful, the practicality and durability of such composite materials hinder their further application. The emerging biomimetic nano vaccine technology further refines the nano antimicrobial therapy. As artificial nanocomposites wrapped by host cell membranes, biomimetic vaccines possess excellent biocompatibility and different immunophysiological characteristics based on the coating membranes. For example, nanomaterials covered with macrophage membranes can target bacterial infections autonomously through toll-like receptors (TLRs) on the coating (Lin et al., 2022). Moreover, membranes from erythrocytes, platelets, tumor cells and other cells are available for generating bionic antibacterial materials and deserve further investigation (Xiang et al., 2021).
Despite current comprehensive and significant advances in biofilm therapy, the popularity of “biofilm” has kept the term at the forefront of any literature on bacterial infections. Therefore, in the future, we need to further develop the above-mentioned biofilm treatment strategies and combine them with each other to perform a comprehensive therapeutic system from prevention to eradication and eventually to prevent recurrence, so as to completely stop any possible development of biofilm and to eliminate inflammatory infections in their cradle.
RM, XH wrote the manuscript. CZ revised the review. All authors contributed to the article and approved the submitted version.
This work was supported by the National Natural Science Foundation of China (Grant No. 81871788), the Key Research and Development Program of Anhui Province (No. 202004j07020013 and 2022e07020017), the Natural Science Foundation of Anhui Province (Grant No. 2108085QH319), the Fundamental Research Funds for the Central Universities (Grant No. WK9110000173), the National Natural Science Foundation of China (82102586); the Fundamental Research Funds for the Central Universities (WK9110000155).
The authors declare that the research was conducted in the absence of any commercial or financial relationships that could be construed as a potential conflict of interest.
All claims expressed in this article are solely those of the authors and do not necessarily represent those of their affiliated organizations, or those of the publisher, the editors and the reviewers. Any product that may be evaluated in this article, or claim that may be made by its manufacturer, is not guaranteed or endorsed by the publisher.
Akazawa, T., Ohashi, T., Wijewardana, V., Sugiura, K., Inoue, N. (2018). Development of a vaccine based on bacteria-mimicking tumor cells coated with novel engineered toll-like receptor 2 ligands. Cancer Sci. 109 (5), 1319–1329. doi: 10.1111/cas.13576
Alicea-Torres, K., Sanseviero, E., Gui, J., Chen, J., Veglia, F., Yu, Q., et al. (2021). Immune suppressive activity of myeloid-derived suppressor cells in cancer requires inactivation of the type I interferon pathway. Nat. Commun. 12 (1), 1717. doi: 10.1038/s41467-021-22033-2
Balaban, N., Cirioni, O., Giacometti, A., Ghiselli, R., Braunstein, J. B., Silvestri, C., et al. (2007). Treatment of staphylococcus aureus biofilm infection by the quorum-sensing inhibitor RIP. Antimicrob. Agents Chemother. 51 (6), 2226–2229. doi: 10.1128/aac.01097-06
Bannoehr, J., Ben Zakour, N. L., Reglinski, M., Inglis, N. F., Prabhakaran, S., Fossum, E., et al. (2011). Genomic and surface proteomic analysis of the canine pathogen staphylococcus pseudintermedius reveals proteins that mediate adherence to the extracellular matrix. Infect. Immun. 79 (8), 3074–3086. doi: 10.1128/iai.00137-11
Beebout, C. J., Sominsky, L. A., Eberly, A. R., Van Horn, G. T., Hadjifrangiskou, M. (2021). Cytochrome bd promotes escherichia coli biofilm antibiotic tolerance by regulating accumulation of noxious chemicals. NPJ Biofilms Microbiomes 7 (1), 35. doi: 10.1038/s41522-021-00210-x
Bhat, A. H., Nguyen, M. T., Das, A., Ton-That, H. (2021). Anchoring surface proteins to the bacterial cell wall by sortase enzymes: How it started and what we know now. Curr. Opin. Microbiol. 60, 73–79. doi: 10.1016/j.mib.2021.01.013
Bhattacharya, M., Berends, E. T. M., Chan, R., Schwab, E., Roy, S., Sen, C. K., et al. (2018). Staphylococcus aureus biofilms release leukocidins to elicit extracellular trap formation and evade neutrophil-mediated killing. Proc. Natl. Acad. Sci. U.S.A. 115 (28), 7416–7421. doi: 10.1073/pnas.1721949115
Billerbeck, S., Brisbois, J., Agmon, N., Jimenez, M., Temple, J., Shen, M., et al. (2018). A scalable peptide-GPCR language for engineering multicellular communication. Nat. Commun. 9 (1), 5057. doi: 10.1038/s41467-018-07610-2
Bonar, E., Wojcik, I., Jankowska, U., Kedracka-Krok, S., Bukowski, M., Polakowska, K., et al. (2016). Identification of secreted exoproteome fingerprints of highly-virulent and non-virulent staphylococcus aureus strains. Front. Cell Infect. Microbiol. 6. doi: 10.3389/fcimb.2016.00051
Cacaci, M., Martini, C., Guarino, C., Torelli, R., Bugli, F., Sanguinetti, M. (2020). Graphene oxide coatings as tools to prevent microbial biofilm formation on medical device. Adv. Exp. Med. Biol. 1282, 21–35. doi: 10.1007/5584_2019_434
Campos, A. I., Zampieri, M. (2019). Metabolomics-driven exploration of the chemical drug space to predict combination antimicrobial therapies. Mol. Cell 74 (6), 1291–1303.e1296. doi: 10.1016/j.molcel.2019.04.001
Cao, Y., Bender, I. K., Konstantinidis, A. K., Shin, S. C., Jewell, C. M., Cidlowski, J. A., et al. (2013). Glucocorticoid receptor translational isoforms underlie maturational stage-specific glucocorticoid sensitivities of dendritic cells in mice and humans. Blood 121 (9), 1553–1562. doi: 10.1182/blood-2012-05-432336
Cao, Y., Su, B., Chinnaraj, S., Jana, S., Bowen, L., Charlton, S., et al. (2018). Nanostructured titanium surfaces exhibit recalcitrance towards staphylococcus epidermidis biofilm formation. Sci. Rep. 8 (1), 1071. doi: 10.1038/s41598-018-19484-x
Cao, G., Yan, J., Ning, X., Zhang, Q., Wu, Q., Bi, L., et al. (2021). Antibacterial and antibiofilm properties of graphene and its derivatives. Colloids Surf B Biointerfaces 200, 111588. doi: 10.1016/j.colsurfb.2021.111588
Carnes, E. C., Lopez, D. M., Donegan, N. P., Cheung, A., Gresham, H., Timmins, G. S., et al. (2010). Confinement-induced quorum sensing of individual staphylococcus aureus bacteria. Nat. Chem. Biol. 6 (1), 41–45. doi: 10.1038/nchembio.264
Carniello, V., Peterson, B. W., van der Mei, H. C., Busscher, H. J. (2018). Physico-chemistry from initial bacterial adhesion to surface-programmed biofilm growth. Adv. Colloid Interface Sci. 261, 1–14. doi: 10.1016/j.cis.2018.10.005
Charbonneau, M. E., Girard, V., Nikolakakis, A., Campos, M., Berthiaume, F., Dumas, F., et al. (2007). O-Linked glycosylation ensures the normal conformation of the autotransporter adhesin involved in diffuse adherence. J. Bacteriol. 189 (24), 8880–8889. doi: 10.1128/jb.00969-07
Cheng, Y., Cheng, H., Jiang, C., Qiu, X., Wang, K., Huan, W., et al. (2015). Perfluorocarbon nanoparticles enhance reactive oxygen levels and tumour growth inhibition in photodynamic therapy. Nat. Commun. 6, 8785. doi: 10.1038/ncomms9785
Chen, Y., Harapanahalli, A. K., Busscher, H. J., Norde, W., van der Mei, H. C. (2014). Nanoscale cell wall deformation impacts long-range bacterial adhesion forces on surfaces. Appl. And Environ. Microbiol. 80 (2), 637–643. doi: 10.1128/AEM.02745-13
Claes, J., Ditkowski, B., Liesenborghs, L., Veloso, T. R., Entenza, J. M., Moreillon, P., et al. (2018). Assessment of the dual role of clumping factor a in s. aureus adhesion to endothelium in absence and presence of plasma. Thromb. And Haemostasis 118 (7), 1230–1241. doi: 10.1055/s-0038-1660435
Cohen, D. T., Zhang, C., Fadzen, C. M., Mijalis, A. J., Hie, L., Johnson, K. D., et al. (2019). A chemoselective strategy for late-stage functionalization of complex small molecules with polypeptides and proteins. Nat. Chem. 11 (1), 78–85. doi: 10.1038/s41557-018-0154-0
Cole, S. J., Hall, C. L., Schniederberend, M., Farrow Iii, J. M., Goodson, J. R., Pesci, E. C., et al. (2018). Host suppression of quorum sensing during catheter-associated urinary tract infections. Nat. Commun. 9 (1), 4436. doi: 10.1038/s41467-018-06882-y
Dane, E. L., Ballok, A. E., O'Toole, G. A., Grinstaff, M. W. (2014). Synthesis of bioinspired carbohydrate amphiphiles that promote and inhibit biofilms. Chem. Sci. 5 (2), 551–557. doi: 10.1039/c3sc52777h
Dapunt, U., Prior, B., Oelkrug, C., Kretzer, J. P. (2020). IgY targeting bacterial quorum-sensing molecules in implant-associated infections. Molecules 25 (17), 4027. doi: 10.3390/molecules25174027
Dasari Shareena, T. P., McShan, D., Dasmahapatra, A. K., Tchounwou, P. B. (2018). A review on graphene-based nanomaterials in biomedical applications and risks in environment and health. Nanomicro Lett. 10 (3), 53. doi: 10.1007/s40820-018-0206-4
Deng, W., McKelvey, K. J., Guller, A., Fayzullin, A., Campbell, J. M., Clement, S., et al. (2020). Application of mitochondrially targeted nanoconstructs to neoadjuvant X-ray-Induced photodynamic therapy for rectal cancer. ACS Cent Sci. 6 (5), 715–726. doi: 10.1021/acscentsci.9b01121
Dhanesha, N., Nayak, M. K., Doddapattar, P., Jain, M., Flora, G. D., Kon, S., et al. (2020). Targeting myeloid-cell specific integrin α9β1 inhibits arterial thrombosis in mice. Blood 135 (11), 857–861. doi: 10.1182/blood.2019002846
Dixit, D., Soppina, V., Ghoroi, C. (2019). A non-electric and affordable surface engineered particle (SEP) based point-of-Use (POU) water disinfection system. Sci. Rep. 9 (1), 18245. doi: 10.1038/s41598-019-54602-3
Dziewanowska, K., Carson, A. R., Patti, J. M., Deobald, C. F., Bayles, K. W., Bohach, G. A. (2000). Staphylococcal fibronectin binding protein interacts with heat shock protein 60 and integrins: Role in internalization by epithelial cells. Infect. And Immun. 68 (11), 6321–6328. doi: 10.1128/IAI.68.11.6321-6328.2000
Echeverria, C., Torres, M. T., Fernández-García, M., de la Fuente-Nunez, C., Muñoz-Bonilla, A. (2020). Physical methods for controlling bacterial colonization on polymer surfaces. Biotechnol. Adv. 43, 107586. doi: 10.1016/j.biotechadv.2020.107586
El-Awady, A. R., Arce, R. M., Cutler, C. W. (2015). Dendritic cells: microbial clearance via autophagy and potential immunobiological consequences for periodontal disease. Periodontology 2000 69 (1), 160–180. doi: 10.1111/prd.12096
Fan, X., Liang, M., Wang, L., Chen, R., Li, H., Liu, X. (2017). Aii810, a novel cold-adapted n-acylhomoserine lactonase discovered in a metagenome, can strongly attenuate pseudomonas aeruginosa virulence factors and biofilm formation. Front. Microbiol. 8. doi: 10.3389/fmicb.2017.01950
Feltner, J. B., Wolter, D. J., Pope, C. E., Groleau, M. C., Smalley, N. E., Greenberg, E. P., et al. (2016). LasR variant cystic fibrosis isolates reveal an adaptable quorum-sensing hierarchy in pseudomonas aeruginosa. mBio 7 (5), e01513–16. doi: 10.1128/mBio.01513-16
Feuillie, C., Formosa-Dague, C., Hays, L. M., Vervaeck, O., Derclaye, S., Brennan, M. P., et al. (2017). Molecular interactions and inhibition of the staphylococcal biofilm-forming protein SdrC. Proc. Natl. Acad. Sci. U.S.A. 114 (14), 3738–3743. doi: 10.1073/pnas.1616805114
Flemming, H.-C., Wingender, J., Szewzyk, U., Steinberg, P., Rice, S. A., Kjelleberg, S. (2016). Biofilms: An emergent form of bacterial life. Nat. Rev. Microbiol. 14 (9), 563–575. doi: 10.1038/nrmicro.2016.94
Flick, M. J., Du, X., Prasad, J. M., Raghu, H., Palumbo, J. S., Smeds, E., et al. (2013). Genetic elimination of the binding motif on fibrinogen for the s. aureus virulence factor ClfA improves host survival in septicemia. Blood 121 (10), 1783–1794. doi: 10.1182/blood-2012-09-453894
Foster, T. J. (2016). The remarkably multifunctional fibronectin binding proteins of staphylococcus aureus. Eur. J. Of Clin. Microbiol. Infect. Dis. 35 (12), 1923–1931. doi: 10.1007/s10096-016-2763-0
Foster, T. J., Geoghegan, J. A., Ganesh, V. K., Höök, M. (2014). Adhesion, invasion and evasion: the many functions of the surface proteins of staphylococcus aureus. Nat. Rev. Microbiol. 12 (1), 49–62. doi: 10.1038/nrmicro3161
Fu, J., Li, Y., Zhang, Y., Liang, Y., Zheng, Y., Li, Z., et al. (2021). An engineered pseudo-macrophage for rapid treatment of bacteria-infected osteomyelitis via microwave-excited anti-infection and immunoregulation. Adv. Mater 33 (41), e2102926. doi: 10.1002/adma.202102926
Gao, Q., Zhang, X., Yin, W., Ma, D., Xie, C., Zheng, L., et al. (2018). Functionalized MoS2 nanovehicle with near-infrared laser-mediated nitric oxide release and photothermal activities for advanced bacteria-infected wound therapy. Small 14 (45), e1802290. doi: 10.1002/smll.201802290
Geisel, S., Secchi, E., Vermant, J. (2022). The role of surface adhesion on the macroscopic wrinkling of biofilms. Elife 11:e76027. doi: 10.7554/eLife.76027
Gilbert-Girard, S., Savijoki, K., Yli-Kauhaluoma, J., Fallarero, A. (2020). Optimization of a high-throughput 384-well plate-based screening platform with staphylococcus aureus ATCC 25923 and pseudomonas aeruginosa ATCC 15442 biofilms. Int. J. Mol. Sci. 21 (9). doi: 10.3390/ijms21093034
Gogoi-Tiwari, J., Waryah, C. B., Eto, K. Y., Tau, M., Wells, K., Costantino, P., et al. (2015). Relative distribution of virulence-associated factors among Australian bovine staphylococcus aureus isolates: Potential relevance to development of an effective bovine mastitis vaccine. Virulence 6 (5), 419–423. doi: 10.1080/21505594.2015.1043508
Gonzalez, E., Pitre, F. E., Pagé, A. P., Marleau, J., Guidi Nissim, W., St-Arnaud, M., et al. (2018). Trees, fungi and bacteria: tripartite metatranscriptomics of a root microbiome responding to soil contamination. Microbiome 6 (1), 53. doi: 10.1186/s40168-018-0432-5
Grygorcewicz, B., Roszak, M., Golec, P., Śleboda-Taront, D., Łubowska, N., Górska, M., et al. (2020). Antibiotics act with vB_AbaP_AGC01 phage against acinetobacter baumannii in human heat-inactivated plasma blood and galleria mellonella models. Int. J. Mol. Sci. 21 (12), 3034. doi: 10.3390/ijms21124390
Guilhen, C., Charbonnel, N., Parisot, N., Gueguen, N., Iltis, A., Forestier, C., et al. (2016). Transcriptional profiling of klebsiella pneumoniae defines signatures for planktonic, sessile and biofilm-dispersed cells. BMC Genomics 17, 237. doi: 10.1186/s12864-016-2557-x
Guo, G., Zhang, H., Shen, H., Zhu, C., He, R., Tang, J., et al. (2020). Space-selective chemodynamic therapy of CuFe(5)O(8) nanocubes for implant-related infections. ACS Nano 14 (10), 13391–13405. doi: 10.1021/acsnano.0c05255
Guttenplan, S. B., Kearns, D. B. (2013). Regulation of flagellar motility during biofilm formation. FEMS Microbiol. Rev. 37 (6), 849–871. doi: 10.1111/1574-6976.12018
Hardiansyah, A., Yang, M. C., Liao, H. L., Cheng, Y. W., Destyorini, F., Irmawati, Y., et al. (2020). Magnetic graphene-based sheets for bacteria capture and destruction using a high-frequency magnetic field. Nanomater. (Basel) 10 (4), 674. doi: 10.3390/nano10040674
Hardy, B. L., Dickey, S. W., Plaut, R. D., Riggins, D. P., Stibitz, S., Otto, M., et al. (2019). Corynebacterium pseudodiphtheriticum exploits staphylococcus aureus virulence components in a novel polymicrobial defense strategy. mBio 10 (1), e02491–18. doi: 10.1128/mBio.02491-18
He, X., Dai, L., Ye, L., Sun, X., Enoch, O., Hu, R., et al. (2022). A vehicle-free antimicrobial polymer hybrid gold nanoparticle as synergistically therapeutic platforms for staphylococcus aureus infected wound healing. Adv. Sci. (Weinh) 9 (14), e2105223. doi: 10.1002/advs.202105223
Heim, C. E., Bosch, M. E., Yamada, K. J., Aldrich, A. L., Chaudhari, S. S., Klinkebiel, D., et al. (2020). Lactate production by staphylococcus aureus biofilm inhibits HDAC11 to reprogramme the host immune response during persistent infection. Nat. Microbiol. 5 (10), 1271–1284. doi: 10.1038/s41564-020-0756-3
Holla, S., Kurowska-Stolarska, M., Bayry, J., Balaji, K. N. (2014). Selective inhibition of IFNG-induced autophagy by Mir155- and Mir31-responsive WNT5A and SHH signaling. Autophagy 10 (2), 311–330. doi: 10.4161/auto.27225
Hommes, J. W., Kratofil, R. M., Wahlen, S., de Haas, C. J. C., Hildebrand, R. B., Hovingh, G. K., et al. (2021). High density lipoproteins mediate in vivo protection against staphylococcal phenol-soluble modulins. Sci. Rep. 11 (1), 15357. doi: 10.1038/s41598-021-94651-1
Hou, Z., Wu, Y., Xu, C., Reghu, S., Shang, Z., Chen, J., et al. (2020). Precisely structured nitric-Oxide-Releasing copolymer brush defeats broad-spectrum catheter-associated biofilm infections In vivo. ACS Cent Sci. 6 (11), 2031–2045. doi: 10.1021/acscentsci.0c00755
Huang, J., Birmingham, C. L., Shahnazari, S., Shiu, J., Zheng, Y. T., Smith, A. C., et al. (2011). Antibacterial autophagy occurs at PI(3)P-enriched domains of the endoplasmic reticulum and requires Rab1 GTPase. Autophagy 7 (1), 17–26. doi: 10.4161/auto.7.1.13840
Huang, J., Brumell, J. H. (2014). Bacteria-autophagy interplay: a battle for survival. Nat. Rev. Microbiol. 12 (2), 101–114. doi: 10.1038/nrmicro3160
Jenul, C., Horswill, A. R. (2019). Regulation of staphylococcus aureus virulence. Microbiol. Spectr. 7 (2), 10.1128. doi: 10.1128/microbiolspec.GPP3-0031-2018
Jiang, W., Bikard, D., Cox, D., Zhang, F., Marraffini, L. A. (2013). RNA-Guided editing of bacterial genomes using CRISPR-cas systems. Nat. Biotechnol. 31 (3), 233–239. doi: 10.1038/nbt.2508
Jiang, Y., Zheng, W., Tran, K., Kamilar, E., Bariwal, J., Ma, H., et al. (2022). Hydrophilic nanoparticles that kill bacteria while sparing mammalian cells reveal the antibiotic role of nanostructures. Nat. Commun. 13 (1), 197. doi: 10.1038/s41467-021-27193-9
Jobin, C. (2014). Do bugs define cancer geography? J. Exp. Med. 211 (3), 385. doi: 10.1084/jem.2113insight3
Kalia, V. C., Patel, S. K. S., Kang, Y. C., Lee, J. K. (2019). Quorum sensing inhibitors as antipathogens: Biotechnological applications. Biotechnol. Adv. 37 (1), 68–90. doi: 10.1016/j.biotechadv.2018.11.006
Kang, H., Gan, J., Zhao, J., Kong, W., Zhang, J., Zhu, M., et al. (2017). Crystal structure of pseudomonas aeruginosa RsaL bound to promoter DNA reaffirms its role as a global regulator involved in quorum-sensing. Nucleic Acids Res. 45 (2), 699–710. doi: 10.1093/nar/gkw954
Kaplan, J. B., Izano, E. A., Gopal, P., Karwacki, M. T., Kim, S., Bose, J. L., et al. (2012). Low levels of β-lactam antibiotics induce extracellular DNA release and biofilm formation in staphylococcus aureus. mBio 3 (4), e00198–e00112. doi: 10.1128/mBio.00198-12
Kavanaugh, J. S., Horswill, A. R. (2016). Impact of environmental cues on staphylococcal quorum sensing and biofilm development. J. Biol. Chem. 291 (24), 12556–12564. doi: 10.1074/jbc.R116.722710
Khalid, S., Gao, A., Wang, G., Chu, P. K., Wang, H. (2020). Tuning surface topographies on biomaterials to control bacterial infection. Biomater Sci. 8 (24), 6840–6857. doi: 10.1039/d0bm00845a
Kim, B. S., Jang, S. Y., Bang, Y. J., Hwang, J., Koo, Y., Jang, K. K., et al. (2018). QStatin, a selective inhibitor of quorum sensing in vibrio species. mBio 9 (1), e02262–17. doi: 10.1128/mBio.02262-17
Kimkes, T. E. P., Heinemann, M. (2020). How bacteria recognise and respond to surface contact. FEMS Microbiol. Rev. 44 (1), 106–122. doi: 10.1093/femsre/fuz029
Kimmey, J. M., Stallings, C. L. (2016). Bacterial pathogens versus autophagy: Implications for therapeutic interventions. Trends Mol. Med. 22 (12), 1060–1076. doi: 10.1016/j.molmed.2016.10.008
Kim, J. K., Youn, Y. J., Lee, Y. B., Kim, S. H., Song, D. K., Shin, M., et al. (2021). Extracellular vesicles from dHL-60 cells as delivery vehicles for diverse therapeutics. Sci. Rep. 11 (1), 8289. doi: 10.1038/s41598-021-87891-8
Kim, M. K., Zhao, A., Wang, A., Brown, Z. Z., Muir, T. W., Stone, H. A., et al. (2017). Surface-attached molecules control staphylococcus aureus quorum sensing and biofilm development. Nat. Microbiol. 2, 17080. doi: 10.1038/nmicrobiol.2017.80
Kolodkin-Gal, I., Romero, D., Cao, S., Clardy, J., Kolter, R., Losick, R. (2010). D-amino acids trigger biofilm disassembly. Science 328 (5978), 627–629. doi: 10.1126/science.1188628
Krsmanovic, M., Biswas, D., Ali, H., Kumar, A., Ghosh, R., Dickerson, A. K. (2021). Hydrodynamics and surface properties influence biofilm proliferation. Adv. Colloid Interface Sci. 288, 102336. doi: 10.1016/j.cis.2020.102336
Kuang, Z., Bennett, R. C., Lin, J., Hao, Y., Zhu, L., Akinbi, H. T., et al. (2020). Surfactant phospholipids act as molecular switches for premature induction of quorum sensing-dependent virulence in pseudomonas aeruginosa. Virulence 11 (1), 1090–1107. doi: 10.1080/21505594.2020.1809327
Landry, B. P., Palanki, R., Dyulgyarov, N., Hartsough, L. A., Tabor, J. J. (2018). Phosphatase activity tunes two-component system sensor detection threshold. Nat. Commun. 9 (1), 1433. doi: 10.1038/s41467-018-03929-y
Lee, S. W., Phillips, K. S., Gu, H., Kazemzadeh-Narbat, M., Ren, D. (2021). How microbes read the map: Effects of implant topography on bacterial adhesion and biofilm formation. Biomaterials 268, 120595. doi: 10.1016/j.biomaterials.2020.120595
Lei, W., Bruchmann, J., Rüping, J. L., Levkin, P. A., Schwartz, T. (2019). Biofilm bridges forming structural networks on patterned lubricant-infused surfaces. Adv. Sci. (Weinh) 6 (13), 1900519. doi: 10.1002/advs.201900519
Leiman, S. A., May, J. M., Lebar, M. D., Kahne, D., Kolter, R., Losick, R. (2013). D-amino acids indirectly inhibit biofilm formation in bacillus subtilis by interfering with protein synthesis. J. Bacteriol. 195 (23), 5391–5395. doi: 10.1128/jb.00975-13
Li, F., Liu, F., Huang, K., Yang, S. (2022). Advancement of gallium and gallium-based compounds as antimicrobial agents. Front. Bioeng Biotechnol. 10. doi: 10.3389/fbioe.2022.827960
Li, J., Liu, X., Tan, L., Cui, Z., Yang, X., Liang, Y., et al. (2019). Zinc-doped Prussian blue enhances photothermal clearance of staphylococcus aureus and promotes tissue repair in infected wounds. Nat. Commun. 10:4490. doi: 10.1038/s41467-019-12429-6
Lin, H., Yang, C., Luo, Y., Ge, M., Shen, H., Zhang, X., et al. (2022). Biomimetic nanomedicine-triggered in situ vaccination for innate and adaptive immunity activations for bacterial osteomyelitis treatment. ACS Nano 16 (4), 5943–5960. doi: 10.1021/acsnano.1c11132
Liu, J., Fu, K., Wu, C., Qin, K., Li, F., Zhou, L. (2018). "In-group" communication in marine vibrio: A review of n-acyl homoserine lactones-driven quorum sensing. Front. Cell Infect. Microbiol. 8. doi: 10.3389/fcimb.2018.00139
Luis Del Pozo, J. (2018). Biofilm-related disease. Expert Rev. Of Anti-Infective Ther. 16 (1), 51–65. doi: 10.1080/14787210.2018.1417036
Malgaonkar, A., Nair, M. (2019). Quorum sensing in pseudomonas aeruginosa mediated by RhlR is regulated by a small RNA PhrD. Sci. Rep. 9 (1), 432. doi: 10.1038/s41598-018-36488-9
Marrie, T. J., Nelligan, J., Costerton, J. W. (1982). A scanning and transmission electron microscopic study of an infected endocardial pacemaker lead. Circulation 66 (6), 1339–1341. doi: 10.1161/01.cir.66.6.1339
Mashruwala, A. A., Guchte, A. V., Boyd, J. M. (2017). Impaired respiration elicits SrrAB-dependent programmed cell lysis and biofilm formation in staphylococcus aureus. Elife 6:e23845. doi: 10.7554/eLife.23845
Matilla-Cuenca, L., Gil, C., Cuesta, S., Rapún-Araiz, B., Žiemytė, M., Mira, A., et al. (2020). Antibiofilm activity of flavonoids on staphylococcal biofilms through targeting BAP amyloids. Sci. Rep. 10 (1), 18968. doi: 10.1038/s41598-020-75929-2
Milles, L. F., Unterauer, E. M., Nicolaus, T., Gaub, H. E. (2018). Calcium stabilizes the strongest protein fold. Nat. Commun. 9 (1), 4764. doi: 10.1038/s41467-018-07145-6
Ming, J., Sun, D., Wei, J., Chen, X., Zheng, N. (2020). Adhesion of bacteria to a graphene oxide film. ACS Appl. Bio Mater 3 (1), 704–712. doi: 10.1021/acsabm.9b01028
Mi, W., Qiao, S., Zhang, X., Wu, D., Zhou, L., Lai, H. (2021). PRMT5 inhibition modulates murine dendritic cells activation by inhibiting the metabolism switch: a new therapeutic target in periodontitis. Ann. Transl. Med. 9 (9), 755. doi: 10.21037/atm-20-7362
Mitsunaga, M., Ogawa, M., Kosaka, N., Rosenblum, L. T., Choyke, P. L., Kobayashi, H. (2011). Cancer cell-selective in vivo near infrared photoimmunotherapy targeting specific membrane molecules. Nat. Med. 17 (12), 1685–1691. doi: 10.1038/nm.2554
Moreau, K., Fleming, A., Imarisio, S., Lopez Ramirez, A., Mercer, J. L., Jimenez-Sanchez, M., et al. (2014). PICALM modulates autophagy activity and tau accumulation. Nat. Commun. 5, 4998. doi: 10.1038/ncomms5998
Mottola, C., Semedo-Lemsaddek, T., Mendes, J. J., Melo-Cristino, J., Tavares, L., Cavaco-Silva, P., et al. (2016). Molecular typing, virulence traits and antimicrobial resistance of diabetic foot staphylococci. J. BioMed. Sci. 23, 33. doi: 10.1186/s12929-016-0250-7
Nakashige, T. G., Zhang, B., Krebs, C., Nolan, E. M. (2015). Human calprotectin is an iron-sequestering host-defense protein. Nat. Chem. Biol. 11 (10), 765–771. doi: 10.1038/nchembio.1891
Németh, T., Sperandio, M., Mócsai, A. (2020). Neutrophils as emerging therapeutic targets. Nat. Rev. Drug Discovery 19 (4), 253–275. doi: 10.1038/s41573-019-0054-z
Nguyen, D. H. K., Loebbe, C., Linklater, D. P., Xu, X., Vrancken, N., Katkus, T., et al. (2019). The idiosyncratic self-cleaning cycle of bacteria on regularly arrayed mechano-bactericidal nanostructures. Nanoscale 11 (35), 16455–16462. doi: 10.1039/c9nr05923g
Novotny, L. A., Jurcisek, J. A., Goodman, S. D., Bakaletz, L. O. (2016). Monoclonal antibodies against DNA-binding tips of DNABII proteins disrupt biofilms in vitro and induce bacterial clearance in vivo. EBioMedicine 10, 33–44. doi: 10.1016/j.ebiom.2016.06.022
O'Neill, E., Pozzi, C., Houston, P., Humphreys, H., Robinson, D. A., Loughman, A., et al. (2008). A novel staphylococcus aureus biofilm phenotype mediated by the fibronectin-binding proteins, FnBPA and FnBPB. J. Bacteriol. 190 (11), 3835–3850. doi: 10.1128/jb.00167-08
Palmieri, V., Carmela Lauriola, M., Ciasca, G., Conti, C., De Spirito, M., Papi, M. (2017). The graphene oxide contradictory effects against human pathogens. Nanotechnology 28 (15), 152001. doi: 10.1088/1361-6528/aa6150
Palmqvist, N., Foster, T., Fitzgerald, J. R., Josefsson, E., Tarkowski, A. (2005). Fibronectin-binding proteins and fibrinogen-binding clumping factors play distinct roles in staphylococcal arthritis and systemic inflammation. J. Infect. Dis. 191 (5), 791–798. doi: 10.1086/427663
Parsonnet, J. (1995). Bacterial infection as a cause of cancer. Environ. Health Perspect. 103 Suppl 8 (Suppl 8), 263–268. doi: 10.1289/ehp.95103s8263
Passos da Silva, D., Matwichuk, M. L., Townsend, D. O., Reichhardt, C., Lamba, D., Wozniak, D. J., et al. (2019). The pseudomonas aeruginosa lectin LecB binds to the exopolysaccharide psl and stabilizes the biofilm matrix. Nat. Commun. 10 (1), 2183. doi: 10.1038/s41467-019-10201-4
Peng, K. T., Hsieh, C. C., Huang, T. Y., Chen, P. C., Shih, H. N., Lee, M. S., et al. (2017). Staphylococcus aureus biofilm elicits the expansion, activation and polarization of myeloid-derived suppressor cells in vivo and in vitro. PLoS One 12 (8), e0183271. doi: 10.1371/journal.pone.0183271
Peng, K. T., Huang, T. Y., Chiang, Y. C., Hsu, Y. Y., Chuang, F. Y., Lee, C. W., et al. (2019). Comparison of methicillin-resistant staphylococcus aureus isolates from cellulitis and from osteomyelitis in a Taiwan hospital 2016-2018. J. Clin. Med. 8 (6), e0183271. doi: 10.3390/jcm8060816
Periasamy, S., Joo, H. S., Duong, A. C., Bach, T. H., Tan, V. Y., Chatterjee, S. S., et al. (2012). How staphylococcus aureus biofilms develop their characteristic structure. Proc. Natl. Acad. Sci. U.S.A. 109 (4), 1281–1286. doi: 10.1073/pnas.1115006109
Peterson, B. W., van der Mei, H. C., Sjollema, J., Busscher, H. J., Sharma, P. K. (2013). A distinguishable role of eDNA in the viscoelastic relaxation of biofilms. mBio 4 (5), e00497–e00413. doi: 10.1128/mBio.00497-13
Piewngam, P., Chiou, J., Chatterjee, P., Otto, M. (2020). Alternative approaches to treat bacterial infections: targeting quorum-sensing. Expert Rev. Anti Infect. Ther. 18 (6), 499–510. doi: 10.1080/14787210.2020.1750951
Qi, J., Chen, C., Zhang, X., Hu, X., Ji, S., Kwok, R. T. K., et al. (2018). Light-driven transformable optical agent with adaptive functions for boosting cancer surgery outcomes. Nat. Commun. 9 (1), 1848. doi: 10.1038/s41467-018-04222-8
Qu, D., Hou, Z., Li, J., Luo, L., Su, S., Ye, Z., et al. (2020). A new coumarin compound DCH combats methicillin-resistant staphylococcus aureus biofilm by targeting arginine repressor. Sci. Adv. 6 (30), eaay9597. doi: 10.1126/sciadv.aay9597
Raafat, D., Otto, M., Reppschlaeger, K., Iqbal, J., Holtfreter, S. (2019). Fighting staphylococcus aureus biofilms with monoclonal antibodies. Trends In Microbiol. 27 (4), 303–322. doi: 10.1016/j.tim.2018.12.009
Rajput, A., Kumar, M. (2017). In silico analyses of conservational, functional and phylogenetic distribution of the LuxI and LuxR homologs in gram-positive bacteria. Sci. Rep. 7 (1), 6969. doi: 10.1038/s41598-017-07241-5
Ramakrishna, C., Kujawski, M., Chu, H., Li, L., Mazmanian, S. K., Cantin, E. M. (2019). Bacteroides fragilis polysaccharide a induces IL-10 secreting b and T cells that prevent viral encephalitis. Nat. Commun. 10 (1), 2153. doi: 10.1038/s41467-019-09884-6
Rao, L., Zhao, S. K., Wen, C., Tian, R., Lin, L., Cai, B., et al. (2020). Activating macrophage-mediated cancer immunotherapy by genetically edited nanoparticles. Adv. Mater 32 (47), e2004853. doi: 10.1002/adma.202004853
Rosman, C. W. K., van der Mei, H. C., Sjollema, J. (2021). Influence of sub-inhibitory concentrations of antimicrobials on micrococcal nuclease and biofilm formation in staphylococcus aureus. Sci. Rep. 11 (1), 13241. doi: 10.1038/s41598-021-92619-9
Rumyantceva, V., Rumyantceva, V., Andreeva, Y., Tsvetikova, S., Radaev, A., Vishnevskaya, M., et al. (2021). Magnetically controlled carbonate nanocomposite with ciprofloxacin for biofilm eradication. Int. J. Mol. Sci. 22 (12), 6187. doi: 10.3390/ijms22126187
Sabado, R. L., O'Brien, M., Subedi, A., Qin, L., Hu, N., Taylor, E., et al. (2010). Evidence of dysregulation of dendritic cells in primary HIV infection. Blood 116 (19), 3839–3852. doi: 10.1182/blood-2010-03-273763
Schaeffer, C. R., Hoang, T. N., Sudbeck, C. M., Alawi, M., Tolo, I. E., Robinson, D. A., et al. (2016). Versatility of biofilm matrix molecules in staphylococcus epidermidis clinical isolates and importance of polysaccharide intercellular adhesin expression during high shear stress. mSphere 1 (5):e00165–16. doi: 10.1128/mSphere.00165-16
Schmidt, S., Ullrich, E., Bochennek, K., Zimmermann, S.-Y., Lehrnbecher, T. (2016). Role of natural killer cells in antibacterial immunity. Expert Rev. Of Hematol. 9 (12), 1119–1127. doi: 10.1080/17474086.2016.1254546
Seghal Kiran, G., Priyadharshini, S., Dobson, A. D. W., Gnanamani, E., Selvin, J. (2016). Degradation intermediates of polyhydroxy butyrate inhibits phenotypic expression of virulence factors and biofilm formation in luminescent vibrio sp. PUGSK8. NPJ Biofilms Microbiomes 2, 16002. doi: 10.1038/npjbiofilms.2016.2
Serezani, C. H., Aronoff, D. M., Sitrin, R. G., Peters-Golden, M. (2009). FcgammaRI ligation leads to a complex with BLT1 in lipid rafts that enhances rat lung macrophage antimicrobial functions. Blood 114 (15), 3316–3324. doi: 10.1182/blood-2009-01-199919
Sethupathy, S., Sathiyamoorthi, E., Kim, Y. G., Lee, J. H., Lee, J. (2020). Antibiofilm and antivirulence properties of indoles against serratia marcescens. Front. Microbiol. 11. doi: 10.3389/fmicb.2020.584812
Shi, T., Hou, X., Guo, S., Zhang, L., Wei, C., Peng, T., et al. (2021). Nanohole-boosted electron transport between nanomaterials and bacteria as a concept for nano-bio interactions. Nat. Commun. 12 (1), 493. doi: 10.1038/s41467-020-20547-9
Sorbara, M. T., Foerster, E. G., Tsalikis, J., Abdel-Nour, M., Mangiapane, J., Sirluck-Schroeder, I., et al. (2018). Complement C3 drives autophagy-dependent restriction of cyto-invasive bacteria. Cell Host Microbe 23 (5), 644–652.e645. doi: 10.1016/j.chom.2018.04.008
Sparrer, K. M. J., Gableske, S., Zurenski, M. A., Parker, Z. M., Full, F., Baumgart, G. J., et al. (2017). TRIM23 mediates virus-induced autophagy via activation of TBK1. Nat. Microbiol. 2 (11), 1543–1557. doi: 10.1038/s41564-017-0017-2
Srikanth, S., Woo, J. S., Wu, B., El-Sherbiny, Y. M., Leung, J., Chupradit, K., et al. (2019). The Ca(2+) sensor STIM1 regulates the type I interferon response by retaining the signaling adaptor STING at the endoplasmic reticulum. Nat. Immunol. 20 (2), 152–162. doi: 10.1038/s41590-018-0287-8
Sugimoto, A., Maeda, A., Itto, K., Arimoto, H. (2017). Deciphering the mode of action of cell wall-inhibiting antibiotics using metabolic labeling of growing peptidoglycan in streptococcus pyogenes. Sci. Rep. 7 (1), 1129. doi: 10.1038/s41598-017-01267-5
Su, Z., Kong, L., Dai, Y., Tang, J., Mei, J., Qian, Z., et al. (2022). Bioresponsive nano-antibacterials for H(2)S-sensitized hyperthermia and immunomodulation against refractory implant-related infections. Sci. Adv. 8 (14), eabn1701. doi: 10.1126/sciadv.abn1701
Sultan, A. R., Hoppenbrouwers, T., Lemmens-den Toom, N. A., Snijders, S. V., van Neck, J. W., Verbon, A., et al. (2019). During the early stages of staphylococcus aureus biofilm formation, induced neutrophil extracellular traps are degraded by autologous thermonuclease. Infect. Immun. 87 (12), e00605–19. doi: 10.1128/iai.00605-19
Sun, L., Ashcroft, P., Ackermann, M., Bonhoeffer, S. (2020). Stochastic gene expression influences the selection of antibiotic resistance mutations. Mol. Biol. Evol. 37 (1), 58–70. doi: 10.1093/molbev/msz199
Sundin, C., Zetterström, C. E., Vo, D. D., Brkljača, R., Urban, S., Elofsson, M. (2020). Exploring resveratrol dimers as virulence blocking agents - attenuation of type III secretion in yersinia pseudotuberculosis and pseudomonas aeruginosa. Sci. Rep. 10 (1), 2103. doi: 10.1038/s41598-020-58872-0
Sun, G., Hu, C., Mei, Q., Luo, M., Chen, X., Li, Z., et al. (2020). Uncovering the cytochrome P450-catalyzed methylenedioxy bridge formation in streptovaricins biosynthesis. Nat. Commun. 11 (1), 4501. doi: 10.1038/s41467-020-18336-5
Taglialegna, A., Navarro, S., Ventura, S., Garnett, J. A., Matthews, S., Penades, J. R., et al. (2016). Staphylococcal bap proteins build amyloid scaffold biofilm matrices in response to environmental signals. PLoS Pathog. 12 (6), e1005711. doi: 10.1371/journal.ppat.1005711
Teirlinck, E., Xiong, R., Brans, T., Forier, K., Fraire, J., Van Acker, H., et al. (2018). Laser-induced vapour nanobubbles improve drug diffusion and efficiency in bacterial biofilms. Nat. Commun. 9 (1), 4518. doi: 10.1038/s41467-018-06884-w
Thomas, C. A., Tejwani, L., Trujillo, C. A., Negraes, P. D., Herai, R. H., Mesci, P., et al. (2017). Modeling of TREX1-dependent autoimmune disease using human stem cells highlights L1 accumulation as a source of neuroinflammation. Cell Stem Cell 21 (3), 319–331.e318. doi: 10.1016/j.stem.2017.07.009
Tolker-Nielsen, T. (2015). Biofilm development. Microbiol. Spectr. 3 (2), Mb–0001-2014. doi: 10.1128/microbiolspec.MB-0001-2014
Towell, A. M., Feuillie, C., Vitry, P., Costa, T. M., Mathelie-Guinlet, M., Kezic, S., et al. (2021). Staphylococcus aureus binds to the n-terminal region of corneodesmosin to adhere to the stratum corneum in atopic dermatitis. Proc. Of Natl. Acad. Of Sci. Of United States Of America 118 (1), e2014444118. doi: 10.1073/pnas.2014444118
Tuchscherr, L., Bischoff, M., Lattar, S. M., Noto Llana, M., Pförtner, H., Niemann, S., et al. (2015). Sigma factor SigB is crucial to mediate staphylococcus aureus adaptation during chronic infections. PLoS Pathog. 11 (4), e1004870. doi: 10.1371/journal.ppat.1004870
Ueda, Y., Mashima, K., Miyazaki, M., Hara, S., Takata, T., Kamimura, H., et al. (2019). Inhibitory effects of polysorbate 80 on MRSA biofilm formed on different substrates including dermal tissue. Sci. Rep. 9 (1), 3128. doi: 10.1038/s41598-019-39997-3
Valle, J., Echeverz, M., Lasa, I. (2019). σ(B) inhibits poly-N-Acetylglucosamine exopolysaccharide synthesis and biofilm formation in staphylococcus aureus. J. Bacteriol. 201 (11), e00098–19. doi: 10.1128/jb.00098-19
Valliammai, A., Sethupathy, S., Priya, A., Selvaraj, A., Bhaskar, J. P., Krishnan, V., et al. (2019). 5-dodecanolide interferes with biofilm formation and reduces the virulence of methicillin-resistant staphylococcus aureus (MRSA) through up regulation of agr system. Sci. Rep. 9 (1), 13744. doi: 10.1038/s41598-019-50207-y
Van Elsland, D., Neefjes, J. (2018). Bacterial infections and cancer. EMBO Rep. 19 (11):e46632. doi: 10.15252/embr.201846632
Velic, A., Hasan, J., Li, Z., Yarlagadda, P. (2021). Mechanics of bacterial interaction and death on nanopatterned surfaces. Biophys. J. 120 (2), 217–231. doi: 10.1016/j.bpj.2020.12.003
Vinuesa, V., McConnell, M. J. (2021). Recent advances in iron chelation and gallium-based therapies for antibiotic resistant bacterial infections. Int. J. Mol. Sci. 22 (6), 2876. doi: 10.3390/ijms22062876
Wang, Z., Li, J., Benin, B. M., Yu, B., Bunge, S. D., Abeydeera, N., et al. (2021). Lipophilic Ga complex with broad-spectrum antimicrobial activity and the ability to overcome gallium resistance in both pseudomonas aeruginosa and staphylococcus aureus. J. Med. Chem. 64 (13), 9381–9388. doi: 10.1021/acs.jmedchem.1c00656
Wang, Y., Li, H., Cui, X., Zhang, X. H. (2017). A novel stress response mechanism, triggered by indole, involved in quorum quenching enzyme MomL and iron-sulfur cluster in muricauda olearia Th120. Sci. Rep. 7 (1), 4252. doi: 10.1038/s41598-017-04606-8
Wang, Y., Li, J., Li, X., Shi, J., Jiang, Z., Zhang, C. Y. (2022). Graphene-based nanomaterials for cancer therapy and anti-infections. Bioact Mater 14, 335–349. doi: 10.1016/j.bioactmat.2022.01.045
Wang, H., Zang, J., Zhao, Z., Zhang, Q., Chen, S. (2021). The advances of neutrophil-derived effective drug delivery systems: A key review of managing tumors and inflammation. Int. J. Nanomed. 16, 7663–7681. doi: 10.2147/ijn.S328705
Welp, A. L., Bomberger, J. M. (2020). Bacterial community interactions during chronic respiratory disease. Front. Cell Infect. Microbiol. 10. doi: 10.3389/fcimb.2020.00213
Wu, S., Feng, J., Liu, C., Wu, H., Qiu, Z., Ge, J., et al. (2022). Machine learning aided construction of the quorum sensing communication network for human gut microbiota. Nat. Commun. 13 (1), 3079. doi: 10.1038/s41467-022-30741-6
Wu, Y. W., Li, F. (2019). Bacterial interaction with host autophagy. Virulence 10 (1), 352–362. doi: 10.1080/21505594.2019.1602020
Wu, X., Pedersen, K., Edlund, J., Eriksson, L., Åström, M., Andersson, A. F., et al. (2017). Potential for hydrogen-oxidizing chemolithoautotrophic and diazotrophic populations to initiate biofilm formation in oligotrophic, deep terrestrial subsurface waters. Microbiome 5 (1), 37. doi: 10.1186/s40168-017-0253-y
Xiang, X., Pang, H., Ma, T., Du, F., Li, L., Huang, J., et al. (2021). Ultrasound targeted microbubble destruction combined with fe-MOF based bio-/enzyme-mimics nanoparticles for treating of cancer. J. Nanobiotech. 19 (1), 92. doi: 10.1186/s12951-021-00835-2
Xiao, P., Wang, J., Fang, L., Zhao, Z. T., Sun, X. S., Liu, X. C., et al. (2021). Nanovaccine-mediated cell selective delivery of neoantigens potentiating adoptive dendritic cell transfer for personalized immunization. Advanced Funct. Materials 31 (36), 1616–301X. doi: 10.1002/adfm.202104068
Xie, X., Mao, C., Liu, X., Tan, L., Cui, Z., Yang, X., et al. (2018). Tuning the bandgap of photo-sensitive Polydopamine/Ag(3)PO(4)/Graphene oxide coating for rapid, noninvasive disinfection of implants. ACS Cent Sci. 4 (6), 724–738. doi: 10.1021/acscentsci.8b00177
Xie, T., Qi, Y., Li, Y., Zhang, F., Li, W., Zhong, D., et al. (2021). Ultrasmall Ga-ICG nanoparticles based gallium ion/photodynamic synergistic therapy to eradicate biofilms and against drug-resistant bacterial liver abscess. Bioact Mater 6 (11), 3812–3823. doi: 10.1016/j.bioactmat.2021.03.032
Xiu, W., Wan, L., Yang, K., Li, X., Yuwen, L., Dong, H., et al. (2022). Potentiating hypoxic microenvironment for antibiotic activation by photodynamic therapy to combat bacterial biofilm infections. Nat. Commun. 13 (1), 3875. doi: 10.1038/s41467-022-31479-x
Yan, J., Bassler, B. L. (2019). Surviving as a community: Antibiotic tolerance and persistence in bacterial biofilms. Cell Host Microbe 26 (1), 15–21. doi: 10.1016/j.chom.2019.06.002
Yang, J., Hou, M., Sun, W., Wu, Q., Xu, J., Xiong, L., et al. (2020). Sequential PDT and PTT using dual-modal single-walled carbon nanohorns synergistically promote systemic immune responses against tumor metastasis and relapse. Adv. Sci. (Weinh) 7 (16), 2001088. doi: 10.1002/advs.202001088
Yan, Z., Yin, M., Chen, J., Li, X. (2020). Assembly and substrate recognition of curli biogenesis system. Nat. Commun. 11 (1), 241. doi: 10.1038/s41467-019-14145-7
Yin, Y., Yao, H., Doijad, S., Kong, S., Shen, Y., Cai, X., et al. (2019). A hybrid sub-lineage of listeria monocytogenes comprising hypervirulent isolates. Nat. Commun. 10 (1), 4283. doi: 10.1038/s41467-019-12072-1
Yi, G., Riduan, S. N., Yuan, Y., Zhang, Y. (2019). Microbicide surface nano-structures. Crit. Rev. Biotechnol. 39 (7), 964–979. doi: 10.1080/07388551.2019.1641788
Yuan, Z., Lin, C., He, Y., Tao, B., Chen, M., Zhang, J., et al. (2020). Near-infrared light-triggered nitric-Oxide-Enhanced photodynamic therapy and low-temperature photothermal therapy for biofilm elimination. ACS Nano 14 (3), 3546–3562. doi: 10.1021/acsnano.9b09871
Yu, X., Dai, Y., Zhao, Y., Qi, S., Liu, L., Lu, L., et al. (2020). Melittin-lipid nanoparticles target to lymph nodes and elicit a systemic anti-tumor immune response. Nat. Commun. 11 (1), 1110. doi: 10.1038/s41467-020-14906-9
Yu, C., Li, X., Zhang, N., Wen, D., Liu, C., Li, Q. (2016). Inhibition of biofilm formation by d-tyrosine: Effect of bacterial type and d-tyrosine concentration. Water Res. 92, 173–179. doi: 10.1016/j.watres.2016.01.037
Zhang, K., Du, Y., Si, Z., Liu, Y., Turvey, M. E., Raju, C., et al. (2019). Enantiomeric glycosylated cationic block co-beta-peptides eradicate staphylococcus aureus biofilms and antibiotic-tolerant persisters. Nat. Commun. 10 (1), 4792. doi: 10.1038/s41467-019-12702-8
Zhang, C., Liao, Q., Chen, R., Zhu, X. (2015). Locomotion of bacteria in liquid flow and the boundary layer effect on bacterial attachment. Biochem. And Biophys. Res. Commun. 461 (4), 671–676. doi: 10.1016/j.bbrc.2015.04.089
Zhang, R., Wang, C., Guan, Y., Wei, X., Sha, M., Yi, M., et al. (2021). Manganese salts function as potent adjuvants. Cell Mol. Immunol. 18 (5), 1222–1234. doi: 10.1038/s41423-021-00669-w
Zhang, C., Yang, B., Biazik, J. M., Webster, R. F., Xie, W., Tang, J., et al. (2022). Gallium nanodroplets are anti-inflammatory without interfering with iron homeostasis. ACS Nano 16 (6), 8891–8903. doi: 10.1021/acsnano.1c10981
Zhao, G., Usui, M. L., Lippman, S. I., James, G. A., Stewart, P. S., Fleckman, P., et al. (2013). Biofilms and inflammation in chronic wounds. Adv. Wound Care (New Rochelle) 2 (7), 389–399. doi: 10.1089/wound.2012.0381
Zheng, S., Bawazir, M., Dhall, A., Kim, H. E., He, L., Heo, J., et al. (2021). Implication of surface properties, bacterial motility, and hydrodynamic conditions on bacterial surface sensing and their initial adhesion. Front. Bioeng Biotechnol. 9. doi: 10.3389/fbioe.2021.643722
Zheng, Y., Liu, W., Qin, Z., Chen, Y., Jiang, H., Wang, X. (2018). Mercaptopyrimidine-conjugated gold nanoclusters as nanoantibiotics for combating multidrug-resistant superbugs. Bioconjug Chem. 29 (9), 3094–3103. doi: 10.1021/acs.bioconjchem.8b00452
Zhou, J., Ventura, C. J., Fang, R. H., Zhang, L. (2022). Nanodelivery of STING agonists against cancer and infectious diseases. Mol. Aspects Med. 83, 101007. doi: 10.1016/j.mam.2021.101007
Keywords: bacterial infection, biofilm formation, plankton adhesion, quorum sensing, nanomaterial, combined therapy, antibiotics resistant
Citation: Ma R, Hu X, Zhang X, Wang W, Sun J, Su Z and Zhu C (2022) Strategies to prevent, curb and eliminate biofilm formation based on the characteristics of various periods in one biofilm life cycle. Front. Cell. Infect. Microbiol. 12:1003033. doi: 10.3389/fcimb.2022.1003033
Received: 25 July 2022; Accepted: 12 August 2022;
Published: 21 September 2022.
Edited by:
Jayendrakumar Patel, Ganpat University, IndiaReviewed by:
Shwetaben Patel, Pyrrhic Pharma Private Limited, IndiaCopyright © 2022 Ma, Hu, Zhang, Wang, Sun, Su and Zhu. This is an open-access article distributed under the terms of the Creative Commons Attribution License (CC BY). The use, distribution or reproduction in other forums is permitted, provided the original author(s) and the copyright owner(s) are credited and that the original publication in this journal is cited, in accordance with accepted academic practice. No use, distribution or reproduction is permitted which does not comply with these terms.
*Correspondence: Chen Zhu, emh1Y2hlbmFAdXN0Yy5lZHUuY24=; Zheng Su, c3V6OTI0QG1haWwudXN0Yy5lZHUuY24=
†These authors have contributed equally to this work
Disclaimer: All claims expressed in this article are solely those of the authors and do not necessarily represent those of their affiliated organizations, or those of the publisher, the editors and the reviewers. Any product that may be evaluated in this article or claim that may be made by its manufacturer is not guaranteed or endorsed by the publisher.
Research integrity at Frontiers
Learn more about the work of our research integrity team to safeguard the quality of each article we publish.