- Beijing Key Laboratory of Antimicrobial Agents, Institute of Medicinal Biotechnology, Chinese Academy of Medical Sciences & Peking Union Medical College, Beijing, China
The increasing incidence of tigecycline resistance undoubtedly constitutes a serious threat to global public health. The combination therapies had become the indispensable strategy against this threat. Herein, 11 clinical tigecycline-resistant Klebsiella pneumoniae which mainly has mutations in ramR, acrR, or macB were collected for tigecycline adjuvant screening. Interestingly, ML-7 hydrochloride (ML-7) dramatically potentiated tigecycline activity. We further picked up five analogs of ML-7 and evaluated their synergistic activities with tigecycline by using checkerboard assay. The results revealed that ML-7 showed certain synergy with tigecycline, while other analogs exerted attenuated synergistic effects among tigecycline-resistant isolates. Thus, ML-7 was selected for further investigation. The results from growth curves showed that ML-7 combined with tigecycline could completely inhibit the growth of bacteria, and the time-kill analysis revealed that the combination exhibited synergistic bactericidal activities for tigecycline-resistant isolates during 24 h. The ethidium bromide (EtBr) efflux assay demonstrated that ML-7 could inhibit the functions of efflux pump. Besides, ML-7 disrupted the proton motive force (PMF) via increasing ΔpH, which in turn lead to the inhibition of the functions of efflux pump, reduction of intracellular ATP levels, as well as accumulation of ROS. All of which promoted the death of bacteria. And further transcriptomic analysis revealed that genes related to the mechanism of ML-7 mainly enriched in ABC transporters. Taken together, these results revealed the potential of ML-7 as a novel tigecycline adjuvant to circumvent tigecycline-resistant Klebsiella pneumoniae.
Introduction
Antimicrobial resistance has become one of the greatest global public health challenges. In 2019, Centers for Disease Control and Prevention (CDC) listed carbapenem-resistant Enterobacteriaceae as an urgent threat for the public (Centers for Disease Control and Prevention, 2019). Particularly, infections caused by carbapenem-resistant Klebsiella pneumoniae (CRKP) have been an urgent concern because it is associated with high mortality and morbidity (Jin et al., 2021).
Tigecycline, which belongs to a class of glycylcyclines antibiotics, possesses broad-spectrum antibacterial activity and it is regarded as the last resort antibiotic for treating serious infections caused by multidrug-resistant (MDR) Gram-negative bacteria, especially extensively drug-resistant (XDR) Enterobacteriaceae (Doi, 2019). Unfortunately, upon increasing clinical usage, tigecycline-resistant K. pneumoniae has been discovered in many countries, including China (Xu et al., 2017), Japan (Hayashi et al., 2021), Vietnam (Hirabayashi et al., 2021) and Austria (Hladicz et al., 2017). The resistance mechanism of tigecycline in K. pneumoniae is extensively related to the upregulated transcription of acrAB, oqxAB, and macAB efflux pumps, which results from mutations in transcriptional regulator ramR and acrR (Sheng et al., 2014; Zheng et al., 2018). Mutations in rpsJ, which encodes the ribosome S10 protective protein targeted by tetracyclines, are also associated with tigecycline resistance (He et al., 2018). Besides, mutations in tet(A), which was another tetracycline efflux pump gene, have also been reported to cause resistance to tigecycline (Yao et al., 2018). What’s worse, the emergence and rapid spread of tet(X3/X4), plasmid-mediated high level tigecycline resistant genes (He et al., 2019; Sun et al., 2019), constitutes a more serious threat to the role of tigecycline as the “last-resort” antibiotic. Therefore, there is an urgent need to explore alternative strategies against tigecycline-resistant pathogens.
Tigecycline-based combination therapies with other antibiotics have been widely used over the past years, but the therapeutic efficiency was unsatisfactory (Bassetti et al., 2015). Different from antibiotics, the non-antibiotic adjuvant strategy could maximize the antimicrobial efficacy of existing antibiotics through enhancing the killing effect of antibiotics, which is a promising approach against antimicrobial resistance. Furthermore, the strategy is able to theoretically slow down the process of antibiotic resistance. Up to now, a few studies have been reported on non-antibiotic adjuvants of tigecycline (Liu et al., 2020; Tong et al., 2021). For example, azidothymidine, as an adjuvant of tigecycline, dramatically potentiates tigecycline activity against Tet(X)-mediated resistance of tigecycline to E.coli both in vitro and in vivo through inhibiting DNA synthesis and suppressing Tet(X) resistance enzyme activity (Liu et al., 2020). Recently, Tong et al. showed that the non-steroidal anti-inflammatory drug benzydamine in combination with tigecycline could reverse temxCD-toprJ-positive K. pneumoniae (Tong et al., 2021).
In this study, we collected 11 clinical tigecycline-resistant K. pneumoniae for tigecycline adjuvants screening. Before screening, the mutations of relevant resistance genes and regulators were elucidated. Based on these pathogens, we screened a collection of compounds and fortunately found that ML-7 hydrochloride (ML-7) displayed the most potent synergistic activity with tigecycline. The in vitro synergistic activity of ML-7 with tigecycline was evaluated and the mechanisms of ML-7 were also explored. This work provides a promising combination strategy to treat infections caused by tigecycline-resistant K. pneumoniae.
Materials and Methods
Bacterial and Chemical Agents
A total of 11 clinical strains used in this study were listed in Table 1. Tigecycline resistant K. pneumoniae clinical isolates were obtained from the CAMS Collection Center of Pathogen Microorganisms (CAMS-CCPM-A) in China. Tigecycline standard powder and N-Phenyl-1-naphthylamine (NPN) were purchased from TCI (Shanghai, China). Propidium iodide (PI) and ethidium bromide (EtBr) were purchased from Sangon Biotech (Shanghai) Co., Ltd. (Shanghai, China). Carbonyl cyanide m-chlorophenyl hydrazone (CCCP), 3,3’-dipropylthiadicarbocyanine iodide [DiSC3(5)] and BCECF-AM were purchased from Sigma-Aldrich (St Louis, USA). An enhanced ATP Assay Kit and ROS Assay Kit were purchased from Beyotime Biotechnology (Shanghai, China). ML-7 hydrochloride, ML-9, HA-100, Ripasudil hydrochloride dihydrate, Fasudil hydrochloride, Hydroxyfasudil hydrochloride, tavaborole, and tegaserod maleate were purchased from Target Molecule Company (Boston, USA).
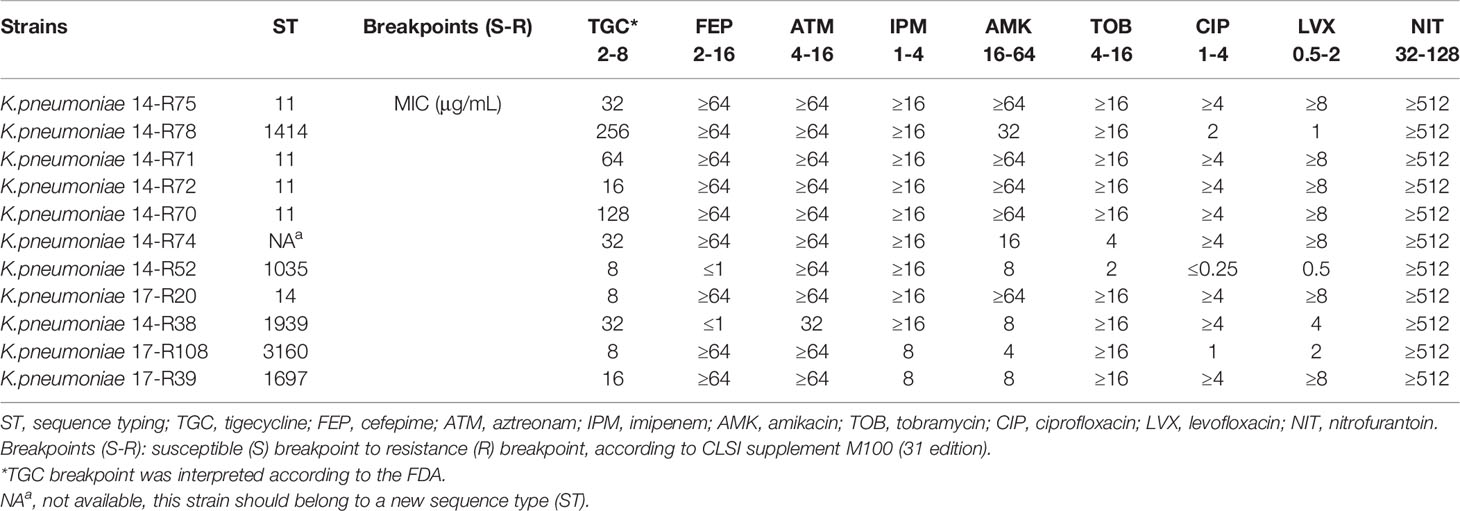
Table 1 Multilocus sequence typing (MLST) and MICs of representative antibiotics for the study strains.
Antimicrobial Susceptibility Testing
MICs of tigecycline were determined by broth microdilution as recommended by the CLSI (Clinical and Laboratory Standards Institute, 2021). Briefly, bacterial cultures were grown overnight and then diluted to a final concentration of approximately 5 × 105 CFU/mL. The experiment was performed on a 96-well plate where the tested agents were 2-fold serially diluted in CAMHB and incubated with equal volumes of bacterial inoculum at 37°C for 18 h. The breakpoint of tigecycline for Enterobacteriaceae was interpreted according to the U.S. Food and Drug Administration (FDA) criteria (susceptible, ≤ 2 μg/mL; intermediate, 4 μg/mL; resistant, ≥ 8 μg/mL) (Sun et al., 2019). The experiment was performed in triplicate on different days. The susceptibility to other antibiotics was evaluated using VITEK 2 Compact System (bioMerieux, France).
Multilocus Sequence Typing
MLST was performed using seven housekeeping genes (rpoB, gapA, mdh, pgi, phoE, infB and tonB) of K. pneumoniae (Table S1). The experiment was conducted following the protocol as the reference described (Diancourt et al., 2005). The sequences of PCR products were determined by Sangon Biotech Company (Shanghai, China). Allele numbers and sequence types were analyzed on the website https://bigsdb.pasteur.fr/klebsiella/klebsiella.html.
Characterization of Tigecycline Resistance Determinant Genes
Tigecycline resistant K. pneumoniae isolates were screened for the tigecycline resistance genes by performing PCR with specific primers (see Table S3 in the Supplemental Material), as previously described (Chiu et al., 2017). The sequences of PCR products were determined by Sangon Biotech Company (Shanghai, China). Mutations were characterized by comparing the sequences with reference strains [K. pneumoniae ATCC 700721 for acrR, rpsJ, tet(A), macB (NCBI Reference Sequence NC_009648 and ATCC Genome Portal: 700721) and K. pneumoniae ATCC 13883 for ramR (GeneBank accession number KY465996.1]. The experiment was repeated in triplicate independently.
Checkerboard Assays
Synergistic activity between compounds and tigecycline, and the fractional inhibitory concentrations (FIC) indices were measured by checkerboard assays (Zhang et al., 2019). Two drugs were mixed in a 96-well plate to create an 8 × 8 matrix followed by the addition of a standard bacterial suspension at 5 x 105 CFU/mL. The experiment was repeated in triplicate independently. After incubated at 37℃ for 18 h, the results were recorded by naked eyes. The FIC index (FICI) was calculated according to the formula as follows:
The antimicrobial combination was defined as synergistic when the FICI was ≤ 0.5; indifferent when 0.5 < FICI ≤ 4, and antagonistic when the FICI was > 4.
Growth Curves
Three single colonies of bacteria were picked and inoculated into 3 mL CAMH broth and grown overnight at 37°C with shaking at 200 rpm. The overnight cultures were standardized to match a 0.5 McFarland and followed by 1:100 dilution in CAMH broth medium. Different concentrations of ML-7, 2 μg/mL tigecycline alone and combination were added to a 100-well microplate. The growth curves were recorded for 24 h under the wavelength of 600 nm with an interval of 1 h at 37°C. The experiment was repeated in triplicate independently.
Time-Kill Curves
Time-kill curves were performed according to the method described with minor modifications (Cebrero-Cangueiro et al., 2018). Experiments were carried out with a starting inoculum of 1×106 CFU/mL. Tubes were incubated at 37°C without shaking, and samples were taken at 0, 2, 4, 6, 8, and 24 h. The experiment was repeated in triplicate independently. Bactericidal activities of single drugs or combination were defined as a decrease ≥ 3 log10 CFU/mL from the starting inoculum, bacteriostatic effect was defined as no change respect to the initial bacterial concentration during the 24 h. Synergy was defined as a decrease ≥ 2 log10 CFU/mL for the drugs combination compared with the most active single agent.
Outer Membrane Permeability Assay
Fluorescent probe NPN was used to evaluated outer membrane permeability. Briefly, bacteria were grown to the mid-log phase (OD600 = 0.5). The cultures were washed three times and resuspended with 5 mM HEPES containing 20 mM of glucose (pH=7.2). NPN was added with a final concentration of 10 μM to 100 μL the same buffer containing various concentrations of ML-7, tigecycline alone or combination. Then, 100 μL bacterial solution was added. Fluorescence intensity was measured on a microplate reader with the excitation wavelength at 355 nm and the emission wavelength at 420 nm and the absorbance of different groups at 600 nm. The normalized fluorescence intensity is the ratio of fluorescence intensity to OD600. The experiment was repeated in triplicate independently.
Membrane Integrity Assay
Inner membrane integrity was measured by staining cells with PI. Cells were washed and resuspended with 1 × PBS buffer to obtain an OD600 = 0.5. The cells were treated with different concentrations of ML-7, tigecycline or combination. After 30 min, PI was added at a final concentration of 5 μg/mL and kept in the dark at 4°C for 30 min. The fluorescence was measured with the excitation wavelength at 515 nm and emission wavelength at 615 nm. The experiment was repeated in triplicate independently.
Reactive Oxygen Species Assay
The levels of ROS were measured with 2′,7′-dichlorodihydrofluorescein diacetate (DCFH-DA, 10 μM) according to the manufacturer’s instruction (Beyotime, Shanghai, China). Briefly, bacteria were grown overnight to obtain an OD600 of 0.5. DCFH-DA was added to a final concentration of 10 μM and the mixture was incubated at 37°C for 30 min. After that, 180 μL of probe-labeled bacterial cells were added to a 96-well plate containing 20 μL drug liquids. After incubation for another 30 min, fluorescence intensity was immediately measured with the excitation wavelength at 488 nm and the emission wavelength at 525 nm. The experiment was repeated in triplicate independently.
Ethidium Bromide Efflux Assay
To assess the effect of ML-7 on the inhibition of multidrug efflux pump, an ethidium bromide (EtBr) efflux assay was performed based on a previous study (Maisuria et al., 2015). Briefly, bacterial culture was grown to an OD600 ≈ 0.5. Cells were co-incubated with 5 μM EtBr and ML-7 (32-128 μg/mL) or tigecycline (2 μg/mL) alone or combination, or known efflux pump inhibitor CCCP (100 μM) at 37°C for 1 h. After being centrifuged at 5000 g at 4°C g for 10 min, the pellets were collected and resuspended in fresh MH broth. Subsequently, EtBr efflux from the cells was monitored with the excitation wavelength at 530 nm and emission wavelength at 600 nm. The experiment was repeated in triplicate independently.
ΔpH Measurement
Intracellular pH measurement was conducted as previously described (Song et al., 2021). Briefly, K. pneumoniae 14-R75 was grown to an OD600 of 0.5 and then washed with 5 mM HEPES containing 5 mM glucose. Cells were treated with 10 μM BCECF-AM for 30 minutes at 37°C. Then tested solution was added and incubated for 30 minutes and the fluorescence value was measured by a Microplate reader (Perkin Elmer), with the excitation wavelength at 488 nm and emission wavelength at 535 nm. The experiment was repeated in triplicate independently.
Transmembrane Potential Assay
The transmembrane potential was determined as previously with the fluorescent probe DiSC3(5) (Ejim et al., 2011). In this assay, mid-log phase cells were pelleted at 5000 × g for 10 min and resuspended in 50 mM HEPES with 20 mM glucose, pH 7.2. Cells loaded with 1 μM DiSC3(5) dye were monitored for 30 min for fluorescence to stabilize. After adding tigecycline and/or ML-7, a time-course acquisition was performed immediately at 620 nm excitation and 670 nm emission wavelengths for 30 min.
ATP Determination
ATP luminescence assay was performed as previously described with slight modifications (Song et al., 2020). Intracellular ATP levels of K. pneumoniae 14-R75 were determined using an Enhanced ATP Assay Kit according to the instructions. Luminescence was measured using a Microplate reader (PerkinElmer). The experiment was repeated in triplicate independently.
Transcriptomic Analysis
Cells from an overnight culture were diluted and incubated to OD600 of 0.5. Tigecycline and ML-7 alone or in combination were added and incubated for 4 h. Total RNA was extracted using RNAprep pure Bacteria Kit (TIANGEN, Beijing, China) according to the manufacturer’s instructions. RNA was quantified using NanoDrop spectrophotometers (Thermo Fisher Scientific). Qualified RNA was sequenced by the Illumina HiSeq platform at GENEWIZ Company (Suzhou, China), with a paired-end mode of 2 × 150 bp. The RNA-Seq raw data was filtrated and employed to assemble the bacterial transcriptome using Cutadapt software and RNA-Seq reads were mapped using the bowtie2 software against the genome of K. pneumoniae 14-R75. The experiment was designed with three biological replicates.
Statistical Analysis
All data were presented as the means ± SEM and transcriptomic analysis was presented as means. Experiment results were statistically analyzed with one-way ANOVA and unpaired Student’s t-test by GraphPad Prism version 8.0 software. For sequence alignment of tigecycline-resistant genes, the data was analyzed using SnapGene software.
Results
The Clinical Tigecycline-Resistant K. pneumoniae Were Multidrug-Resistant and Multisequence Type
We collected 11 clinical tigecycline-resistant K. pneumoniae and assessed the susceptibility of tigecycline and eight other antibiotics including aztreonam, imipenem, nitrofurantoin, cefepime, amikacin, tobramycin, ciprofloxacin, and levofloxacin via the determination of minimal inhibitory concentrations (MICs). The results were shown in Table 1. The tigecycline MIC of the strains ranges from 8 µg/mL to 256 µg/mL. All isolates displayed multidrug resistant phenotypes, and 6 out of 11 isolates were resistant to all of the antibiotics. Among the 11 isolates, all of them were resistant to aztreonam, imipenem, and nitrofurantoin. Meanwhile, cefepime, amikacin, tobramycin, ciprofloxacin, and levofloxacin resistance were existed as well, in most of them.
Further characterization of the isolates was carried out by using MLST. Results showed that seven different sequence types ST 11, 14, 1035, 1414, 1697, 1939 and 3160 were identified among 11 isolates (Table 1 and Table S2).
Identification of Tigecycline Resistance Mutated Genes in Tigecycline-Resistant K. pneumoniae Clinical Isolates
In K. pneumoniae, tigecycline resistance is extensively associated with the overexpression of chromosomal resistance nodulation-division type (RND-type) efflux pumps, such as AcrAB and OqxAB (Yaghoubi et al., 2021), which is commonly caused by the mutation of ramR and acrR. In addition, tet(X), rpsJ, and tet(A) have also been reported to be associated with tigecycline resistance (Yang et al., 2004; Chiu et al., 2017). To determine tigecycline-resistant genes of the 11 isolates, PCR and sequencing of ramR, acrR, rpsJ, tet(A) and macB using designed full-length primers (Table S3) were conducted. The results were presented in Table 2. 44% isolates showed base deletion at 368 in ramR. For acrR and macB, there were many nucleotide changes in comparison to the sequence of the reference strain. Among all of the isolates, no mutations of rpsJ gene were detected. Besides, the tet(X) gene was not found in all isolates (data not shown). Therefore, mutations in ramR, acrR and macB exhibited high prevalence among these isolates.
ML-7 Is a Potent Adjuvant of Tigecycline
To find potential tigecycline adjuvants, we performed initial screening among 2711 compounds at the concentration of 64 μg/mL combined with tigecycline at the concentration of 2 μg/mL using K. pneumoniae 17-R20. As a result, we found that three compounds, which were ML-7, tavaborole and tegaserod maleate (Figure 1), can significantly enhance the susceptibility of K. pneumoniae 17-R20 to tigecycline. Checkerboard assay was used to further evaluate the synergistic activity between tigecycline and three compounds against all tigecycline-resistant clinical isolates. As shown in Table 3, the combination of ML-7 with tigecycline showed synergistic activity with FICI ≤ 0.5; while both tavaborole and tegaserod maleate displayed selective synergy with tigecycline against 73% tigecycline-resistant isolates. Of particular, we observed that ML-7 exerts the best synergistic effect with tigecycline. Accordingly, we picked up five analogs of ML-7 from the commercial compound library and investigated their synergy effects combined with tigecycline by using checkerboard assay (Figure 2). As shown in Figure 2 and Table S4, all analogs exhibited selective synergistic activity with tigecycline and none of them were as good as ML-7. Therefore, the combination of ML-7 with tigecycline was selected for further investigation.
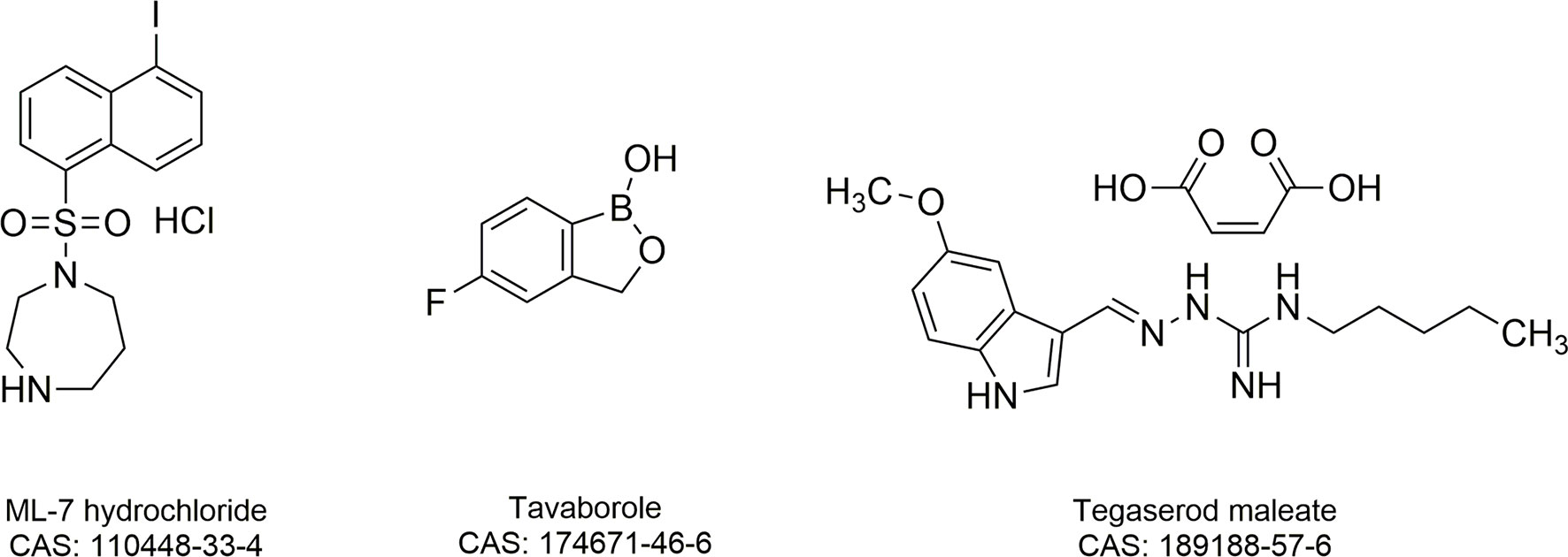
Figure 1 Chemical structures and Chemical Abstracts Service (CAS) registry numbers of ML-7 hydrochloride, tavaborole and tegaserod maleate.
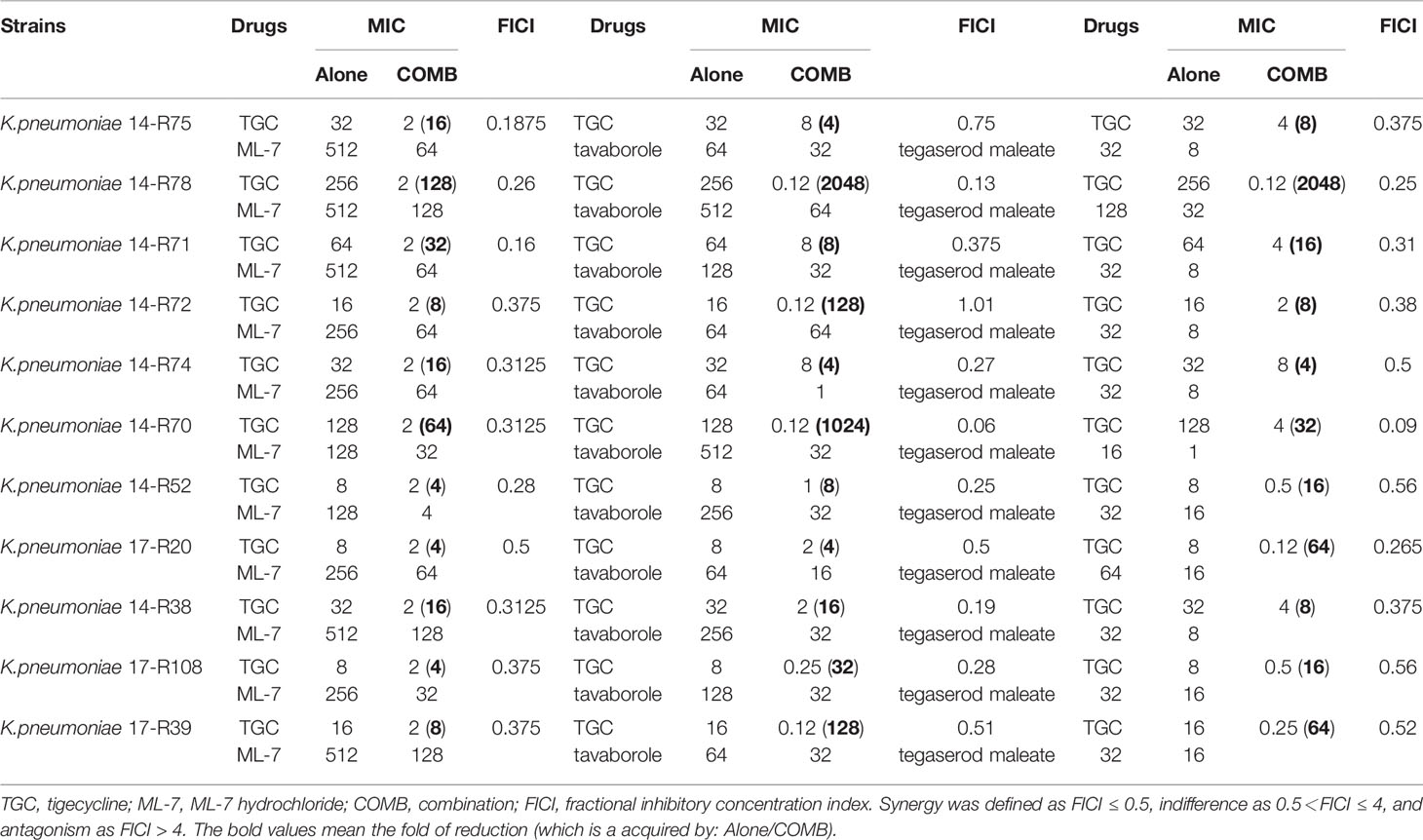
Table 3 MICs of tigecycline combination with ML-7 hydrochloride, tavaborole and tegaserod maleate for all tested isolates.
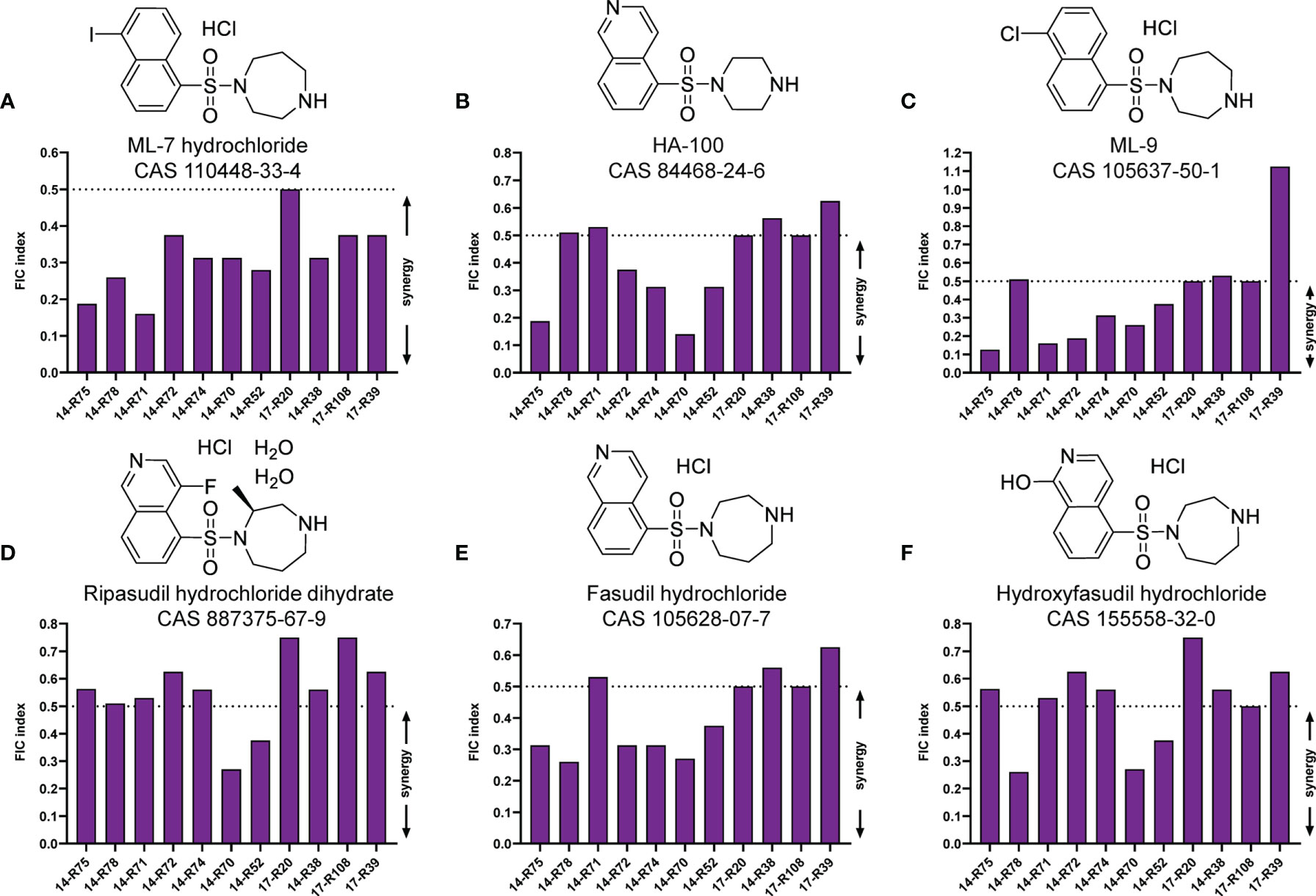
Figure 2 FIC indices of the combination between tigecycline and each of ML-7 analogs against 11 tigecycline-resistant K. pneumoniae isolates. (A) ML-7 hydrochloride. (B) HA-100. (C) ML-9. (D) Ripasudil hydrochloride dihydrate. (E) Fasudil hydrochloride. (F) Hydroxyfasudil hydrochloride. Synergy is defined as a FIC index ≤ 0.5.
Growth curves and time-kill curves with ML-7 and tigecycline alone or in combination were presented in Figure 3. Data from representative strains was shown to display the synergistic activities. The results from growth curves showed that 64 μg/mL ML-7 and 2 μg/mL tigecycline alone could inhibit the growth of bacteria to a certain extent; whereas the combined incubation could completely inhibit the growth of bacteria (Figure 3A). Time-kill curves of three tigecycline-resistant K. pneumoniae strains 14-R71, 14-R74, and 14-R75 were revealed in Figure 3B. There was no obvious influence on strains treated with tigecycline or ML-7 alone during 24 h incubation. The combination of ML-7 and tigecycline exhibited both synergistic and constant bactericidal activities for 14-R71 and 14-R75 with a ~5-6 log10 CFU/mL reduction. As for 14-R74, synergistic bacteriostatic activity was observed over 24 h for the combination of ML-7 and tigecycline, compared with ML-7 or tigecycline alone. These findings suggested that ML-7 could enhance the killing activity of tigecycline.
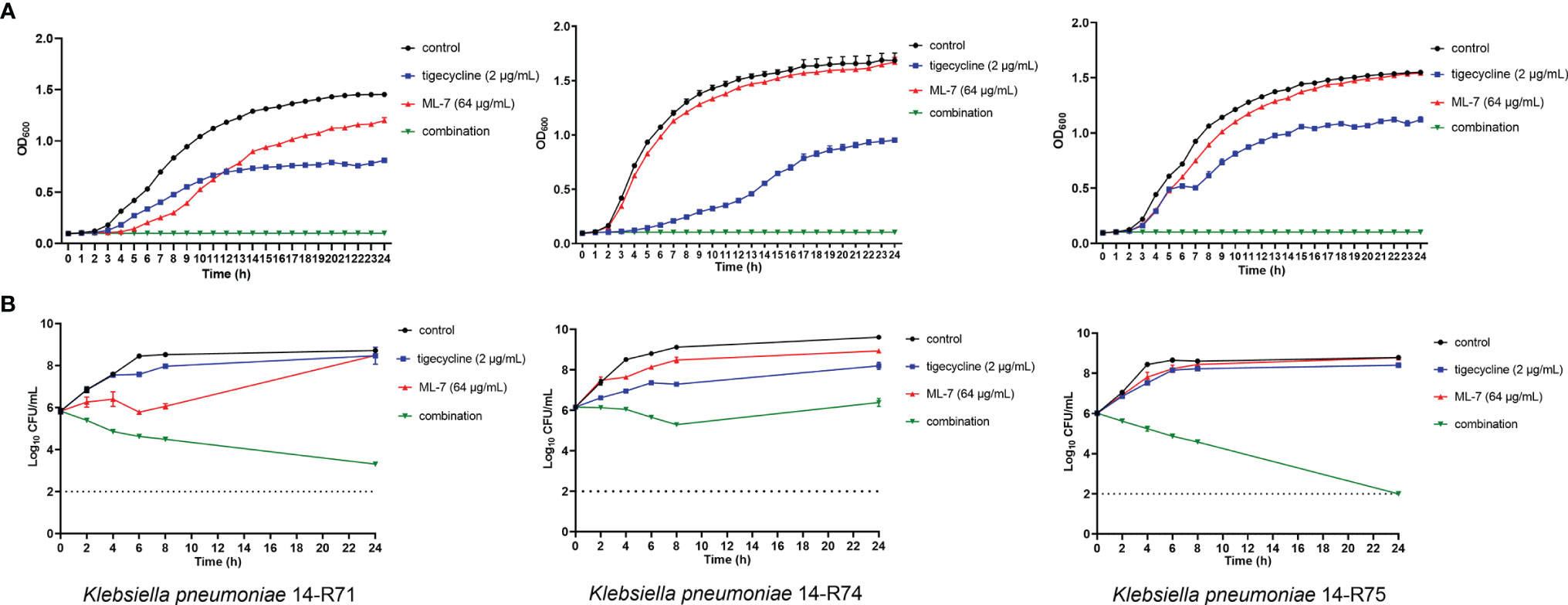
Figure 3 Growth curves (A) and time-kill curves (B) of ML-7 and tigecycline alone or in combination against K. pneumoniae 14-R71, 14-R74 and 14-R75. ML-7, ML-7 hydrochloride.
Synergistic Mechanism of ML-7 in Combination With Tigecycline
Previous studies have been shown that overexpression of RND-efflux pumps plays an important role in tigecycline resistance in K. pneumoniae (Lv et al., 2020). To assess if ML-7 was capable of inhibiting the efflux pump, we explored the effect of ML-7 on the functions of efflux pump by using ethidium bromide (EtBr) as a fluorescent probe. As shown in Figure 4A, the application of ML-7 alone caused significant increase of fluorescence intensity compared with the control group. When ML-7 was combined with tigecycline, the fluorescence intensity also exerted a dose-dependent increase compared with tigecycline alone. These results indicated that ML-7 showed the synergistic effect with tigecycline by inhibiting drug resistance efflux pump. Furthermore, we evaluated the ΔpH, a key component of the proton motive force (PMF) (Farha et al., 2013), by using a pH indicator BCECF-AM. Compared with control and tigecycline alone, ML-7 significantly increased the level of ΔpH, implying the disruption of PMF in bacteria (Figure 4B). Transmembrane potential (Δψ) was another important parameter of PMF. Membrane potential-sensitive dye DiSC3(5) was used to measure transmembrane potential of bacteria. If the bacterial membrane is in its native hyperpolarized state, the cationic dye accumulates in the membrane and its fluorescence emission is self-quenched. However, if the membrane is disrupted, the membrane potential will be dissipated and the dye will be released into the medium, causing an increase in fluorescence (Farha et al., 2013). Upon dissipation in ΔpH, bacterial cells will compensate by a counteracting increase in Δψ in order to maintain a constant PMF. So DiSC3(5) can also inform on disruption in ΔpH through observed decreases in fluorescence. As expected, we observed that ML-7 led to a significant decrease in fluorescence in the presence or absence of tigecycline, which further indicating ML-7 selectively disrupt ΔpH (Figure S1).
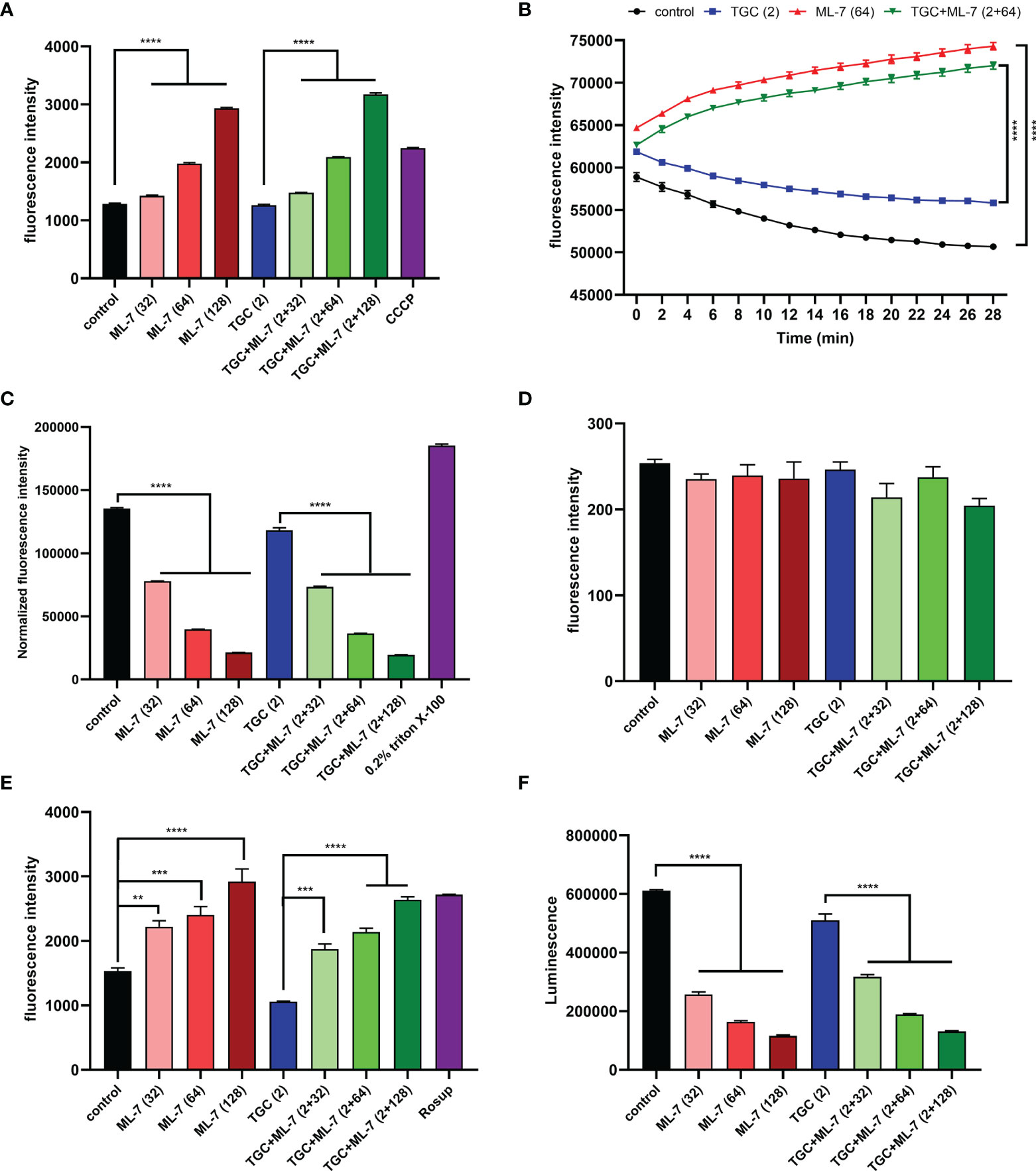
Figure 4 Mechanism of ML-7 in combination with tigecycline. (A) Inhibition of efflux pump by ML-7. Efflux pump inhibitor, CCCP, was recognized as the positive control. (B) Increased ΔpH by ML-7 in treated cells. (C) Permeability of the outer membrane probed with NPN. 0.2% Triton X-100 was recognized as the positive control. The normalized fluorescence intensity is the ratio of fluorescence intensity to OD600. (D) Permeability of the inner membrane probed with PI. (E) Total ROS accumulation in K. pneumoniae 14-R75 treated with ML-7 alone or in combination with tigecycline. Rosup was recognized as the positive control. (F) Decreased intracellular ATP levels after the treatment of ML-7. All experiments were performed as three biologically independent experiments, data presented as mean ± SEM, n = 3 (for F, two biologically independent experiments, n = 2). P-values in (A, C–F) were calculated using non-parametric one-way ANOVA. P-values in (B) were calculated using unpaired Student’s t-test. **P < 0.01, ***P < 0.001, ****P < 0.0001. TGC, tigecycline; ML-7, ML-7 hydrochloride; COMB, combination of tigecycline with tigecycline. Units, μg/mL.
Next, we characterized the impact of ML-7 on the permeability of outer membrane by using hydrophobic fluorophore 1-N-phenylnapthylamine (NPN). Interestingly, we observed that the fluorescence intensity markedly decreased in a dose-dependent manner after the addition of ML-7 (Figure 4C). We further investigated the effect of ML-7 on the integrity of inner membrane by using a fluorescent probe propidium iodide (PI). However, no significant increase of fluorescence was observed when addition of ML-7 in the presence or absence of tigecycline (Figure 4D). These results demonstrated that ML-7 decreased the outer membrane permeability; while had no impact on the permeability of inner membrane.
Subsequently, we measured the sequential responses to decipher the synergy between ML-7 and tigecycline. Previous studies had reported that the PMF is also related to the overproduction of reactive oxygen species (ROS) (Dupuy et al., 2009). To examine whether ML-7 treatment could result in an increased production of ROS, we tested levels of ROS in cells treated with ML-7 and/or tigecycline. As expected, we found that ML-7 could trigger the accumulation of intracellular ROS no matter tigecycline presence or not (Figure 4E). Besides, we observed that the intracellular levels of ATP significantly decreased in a dose-dependent manner in cells treated with ML-7 (Figure 4F).
To gain insight into the mechanisms of ML-7, we performed transcription analysis of K. pneumoniae 14-R75 exposure to tigecycline and ML-7 alone or in combination for 4 h. Compared with tigecycline alone, following treatment with the combination of tigecycline with ML-7, the transcription of 1000 genes were significantly up-regulated and 899 genes were significantly down-regulated (Figure 5A). As shown in Figure 5B, Venn diagram analysis of four different treated groups was performed. In order to further identify the targeted genes associated with the mechanism of ML-7, we firstly narrowed it down to the overlapping regions between TGC VS COMB and Control VS ML-7. Subsequently, we excluded the genes located in tigecycline treated groups from overlapping regions and finally targeted 358 genes, which were the overlapping regions among TGC VS COMB, Control VS ML-7 and Control VS COMB. GO annotation analysis showed that these genes were mainly correlated with the plasma membrane and their molecular function was mainly transmembrane transporter activity (Figure 5C). The results of KEGG pathway analysis demonstrated that these genes were markedly enriched in ABC transporters (Figure 5D). ABC transporters were a series of transmembrane transport systems aimed at importing or exporting a wide range of molecules across membranes, including antibiotics, sugars and lipids and so on, such as efflux pumps (Kolich et al., 2020). We selected 15 genes involved in ABC transporters from 358 genes. As shown in Figure 5E, we found that 6 genes were down-regulated and 9 genes were up-regulated. The results further verified that ML-7 showed synergistic activity with tigecycline through efflux pumps.
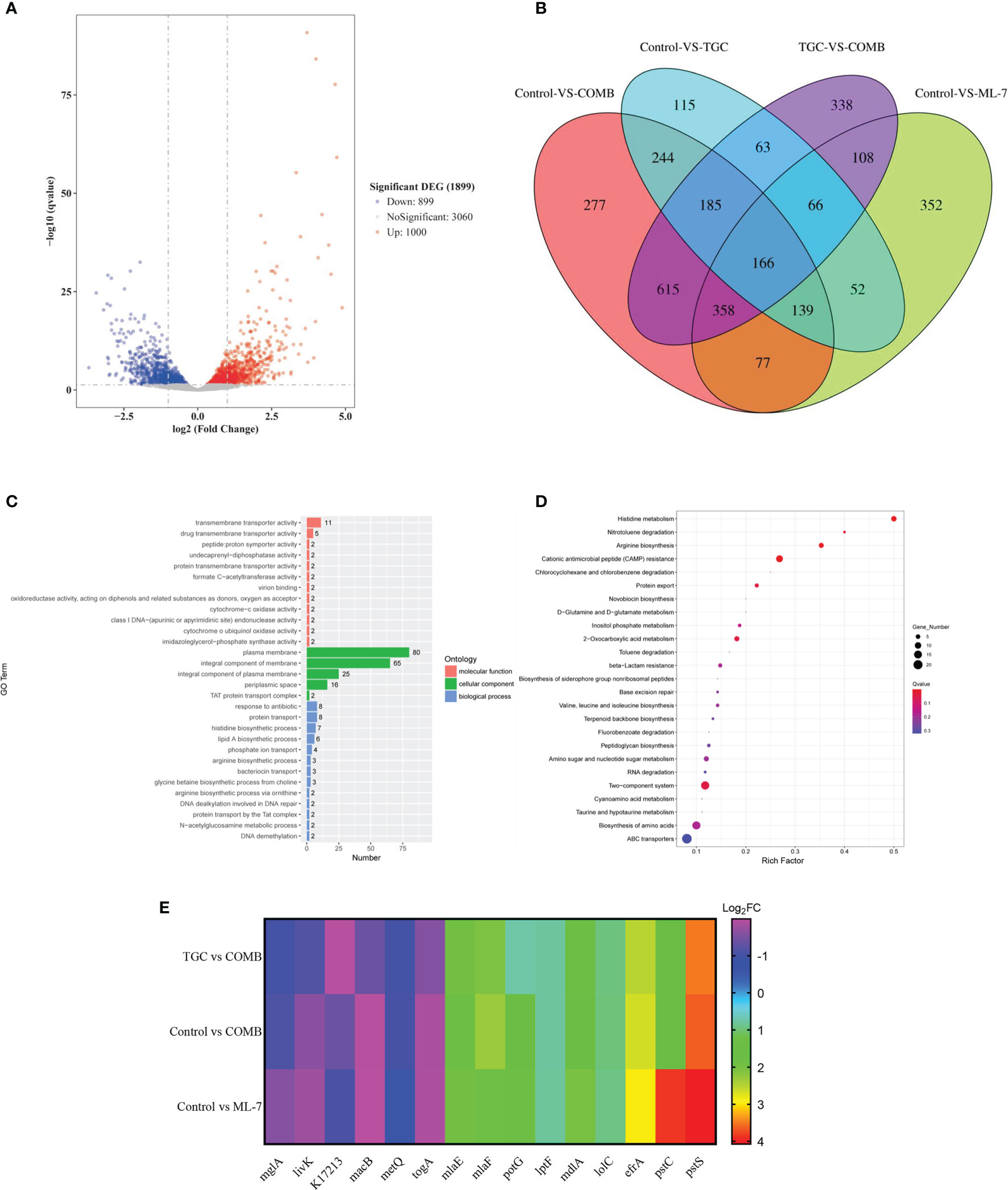
Figure 5 Transcriptome analysis of K. pneumoniae 14-R75 after exposure to tigecycline or ML-7 alone and in combination for 4 h. (A) Volcano plots of genes after treatment by tigecycline plus ML-7 compared to tigecycline treated cells. (B) Venngram of genes identified in four groups. (C, D) Functional analysis of 358 targeted genes. (E) Heatmap of selected genes related to ABC transporters. Data were presented as means of three biological replicates. TGC, tigecycline alone; COMB, the combination of tigecycline and ML-7; ML-7, ML-7 hydrochloride; Log2FC, Log2 Fold Change.
Collectively, our findings indicated that ML-7 restored the susceptibility of tigecycline-resistant K. pneumoniae through two pathways: (1) ML-7 could inhibit the functions of efflux pump and lead to the accumulation of tigecycline in bacteria; (2) ML-7 increased ΔpH and resulted in perturbing the PMF, which subsequently inhibited the functions of efflux pump, decreased the levels of intracellular ATP and resulted in the accumulation of ROS (Figure 6).
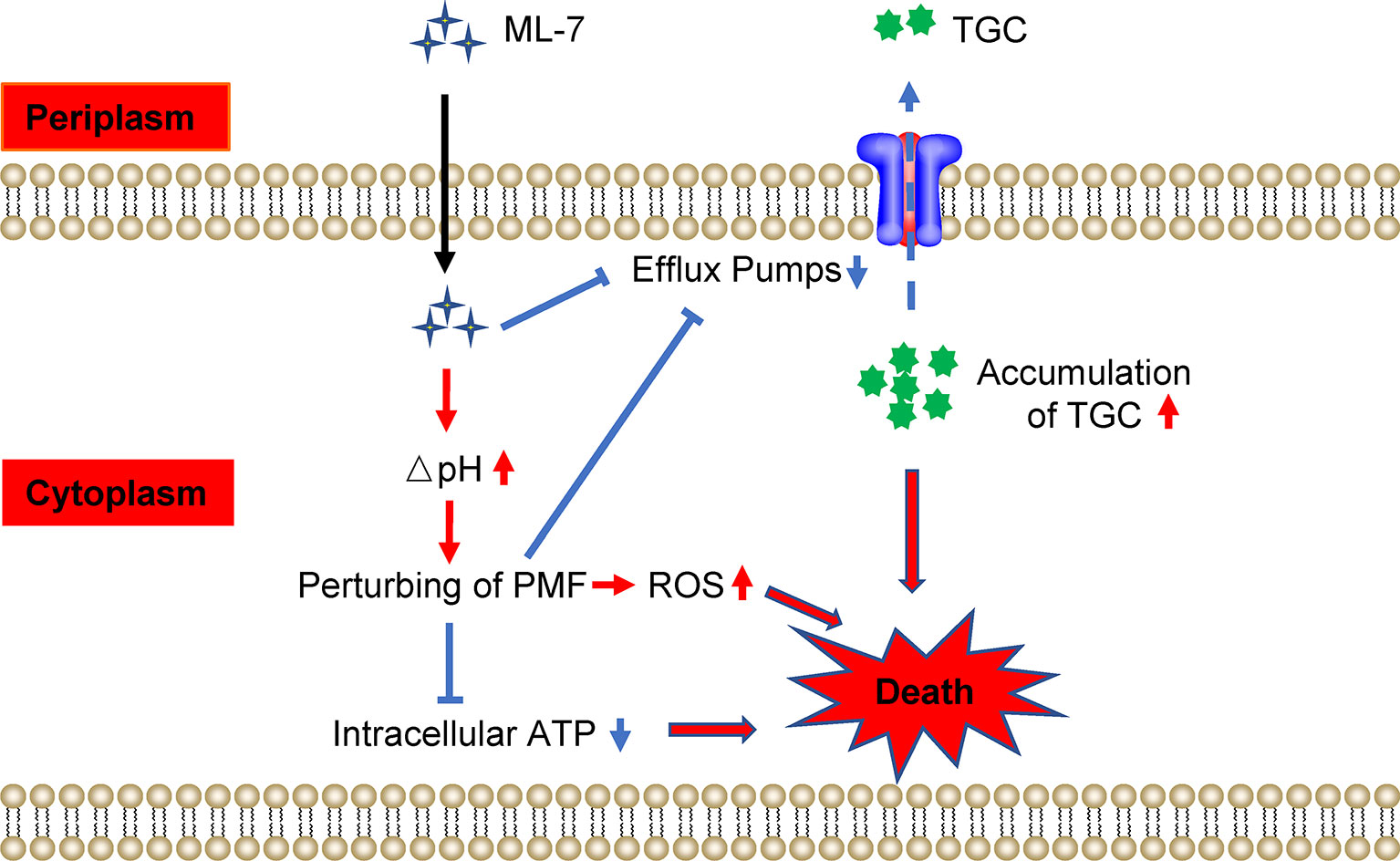
Figure 6 Scheme of the synergy of ML-7 in combination with tigecycline against tigecycline-resistant K. pneumoniae. ML-7 enhances tigecycline activity through two pathways: (1) ML-7 promoted intracellular accumulation of tigecycline via inhibiting the functions of multidrug efflux pump; (2) ML-7 increased ΔpH and lead to perturb the proton motive force (PMF), which subsequently inhibited the functions of efflux pump, decreased the levels of intracellular ATP and resulted in the accumulation of ROS. All of which promoted the death of bacteria. TGC, tigecycline; ML-7, ML-7 hydrochloride. The red arrows mean promotion; blue arrows mean inhibition.
Discussion
In recent years, the development and rapid spread of antimicrobial resistance represent a major threat to human health worldwide. Carbapenems have been widely used to against MDR Enterobacteriaceae. Unfortunately, with the increased use, carbapenem-resistant Enterobacteriaceae has been emerged and spread rapidly, especially in K. pneumoniae (Ni et al., 2021). Infections induced by CRKP have been considered a great challenge in clinical practices (Agyeman et al., 2020). Treatment options for CRKP are very limited, and they are generally only susceptible to polymyxins, tigecycline (Durante-Mangoni et al., 2019). However, no matter if it is monotherapy or in combination, the clinical efficacy is not satisfactory. Besides, we found that pan drug-resistant K. pneumoniae is increasingly being reported worldwide and is associated with high mortality (Karakonstantis et al., 2020). Therefore, there is an urgent need to explore effective strategies to deal with this challenge. The goal of this study was to characterize the resistance mechanism of 11 K. pneumoniae clinical isolates and screen non-antibiotic adjuvants of tigecycline against MDR K. pneumoniae.
The tigecycline resistance determinants were detected in 11 K. pneumoniae clinical isolates, which exhibited multidrug-resistant phenotype. Mutations of most strains were found in ramR, acrR and macB genes, which were the regulators of the efflux pump. Consistently, previous studies have also demonstrated that the resistance mechanisms of CRKP to tigecycline were associated with the efflux pumps and regulators (Pournaras et al., 2016).
After the screening of in-house compounds library, we discovered that several compounds could exert the synergist effect with tigecycline, which including ML-7, tavaborole, tegaserod maleate and analogs of ML-7. Of particular, ML-7 exerted the greatest synergy with tigecycline and thereby was performed for our further assessment. ML-7 is an inhibitor of myosin light chain kinase (MLCK) (Xiong et al., 2017). It has been found it is beneficial to heart ischemia/reperfusion injury (Lin et al., 2012), inflammatory bowel disease (IBD) (Xiong et al., 2017), and atherosclerosis (Cheng et al., 2015). However, the potential application of ML-7 toward bacteria diseases has not been reported so far. In the present study, we found that ML-7 effectively potentiated the antibacterial activity of tigecycline against tigecycline-resistant K. pneumoniae, which belonging to 7 different sequence types, indicating tigecycline MIC reduction induced by ML-7 might be a general rule for different sequence type K. pneumoniae isolates.
Efflux pumps play a vital role in the resistance of tigecycline in K. pneumoniae. Our results showed that ML-7 was able to undermine the functions of multidrug efflux pump. Meanwhile, tetracycline accumulation is a ΔpH-dependent process (Yamaguchi et al., 1991). The ΔpH was increased in ML-7-treated bacteria, which in turn would enhance the uptake of tigecycline. Consequently, the above together might promote intracellular accumulation of tigecycline.
The unexpected new finding of the present study was that ML-7 decreased permeability of outer membrane in a dose-dependent manner, while no effect on inner permeability. These results implied that the potentiation activity of ML-7 with tigecycline was independent of membrane disruption.
As an electrochemical proton gradient across the cytoplasmic membrane, PMF powers ATP synthesis (Maloney et al., 1974) and RND-type efflux pumps transport (Zgurskaya et al., 2018) in bacteria. ML-7 disrupted the homeostasis of PMF via increasing ΔpH, which in turn lead to the reduction of intracellular ATP levels, inhibition of the functions of efflux pump as well as accumulation of ROS, which could potentiate the bactericidal activity of tigecycline and promote the death of bacteria. ROS represents a novel antimicrobial strategy. Oxidative stress, caused by ROS, can damage DNA, RNA, proteins and lipids (Brynildsen et al., 2013), and disturb the cellular oxidative environment and induce cell death (Dharmaraja, 2017). Therapies involving ROS as a mechanism of action are already available in clinical use for wounds (Dryden, 2018). Previous study has been demonstrated that increased ROS production could potentiate killing by antibiotics, including ampicillin, kanamycin, norfloxacin and aminoglycoside (Lam et al., 2020). A common mechanism mainly involved the tricarboxylic acid cycle, a transient depletion of NADH, destabilization of iron-sulfur clusters, and stimulation of the Fenton reaction (Kohanski et al., 2007). Recently, it has been reported that a peptide called SLAP-S25 could trigger the accumulation of ROS in bacteria, and such property had made it be a broad-spectrum antibiotic adjuvant (Song et al., 2020). Consistently, our results revealed that ML-7, as an adjuvant of tigecycline, also promoted the accumulation of ROS.
Analysis of transcriptome revealed that genes associated with the mechanism of ML-7 were mainly involved in ABC transporters. It had been demonstrated that MacAB-TolC pump assembly, as a bacterial ABC drug exporter, was able to extrude antibiotics (Zheng et al., 2018). We found the transcription of macB gene, as a component of MacAB-TolC pump, was down-regulated, which lead to the inhibition of the functions of efflux pump. It was consistent with the results from ethidium bromide (EtBr) efflux assay. In order to maintain lipid asymmetry of the outer membrane, LptBFGC, LolCDE and MlaBDEF were required to transport outer membrane components, including LPS, lipoprotein and glycerophospholipids (GPL) from cytoplasm to the outer membrane (Rao et al., 2020). Our results showed that mlaE, mlaF, lptF and lolC were significantly up-regulated, which may be the reason for the decreased permeability of outer membrane by ML-7.
In summary, in this study, we characterized the mutations of resistance genes of 11 tigecycline-resistant K. pneumoniae and further assessed the in vitro synergistic activity of tigecycline combined with ML-7 against tigecycline-resistant K. pneumoniae. We conclude that ML-7 has remarkable power as a tigecycline adjuvant. More importantly, our work provides a new scaffold lead compound for tigecycline adjuvant discovery and may have broad prospects in discovering more potential antibiotic adjuvants.
Data Availability Statement
The datasets presented in this study can be found in online repositories. The names of the repository/repositories and accession number(s) can be found below: Sequence Read Archive (SRA); SRR16962309, SRR16962310, SRR16962311, and SRR16962312.
Author Contributions
YZ and LiS conceived and designed the study. LiS, XH, XW and TN performed the experiments. LiS analyzed the data and drafted the manuscript. LaS, XL, and YZ revised the manuscript. YZ and XY finalized the manuscript. All authors contributed to the article and approved the submitted version.
Funding
This research was supported by the National Natural Science Foundation of China (grant number 32141003, 82104248, 82104249 and 81803413), the CAMS Innovation Fund for Medical Sciences (CIFMS) (grant number 2021-1-12M-030, 2021-1-12M-039), the Fundamental Research Funds for the Central Universities (grant number 2021-PT350-001 and 2020-PT310-003), and the National Science and Technology Infrastructure of China (no. National Pathogen Resource Center-NPRC-32).
Conflict of Interest
The authors declare that the research was conducted in the absence of any commercial or financial relationships that could be construed as a potential conflict of interest.
Publisher’s Note
All claims expressed in this article are solely those of the authors and do not necessarily represent those of their affiliated organizations, or those of the publisher, the editors and the reviewers. Any product that may be evaluated in this article, or claim that may be made by its manufacturer, is not guaranteed or endorsed by the publisher.
Supplementary Material
The Supplementary Material for this article can be found online at: https://www.frontiersin.org/articles/10.3389/fcimb.2021.809542/full#supplementary-material
References
Agyeman, A. A., Bergen, P. J., Rao, G. G., Nation, R. L., Landersdorfer, C. B. (2020). A Systematic Review and Meta-Analysis of Treatment Outcomes Following Antibiotic Therapy Among Patients With Carbapenem-Resistant Klebsiella Pneumoniae Infections. Int. J. Antimicrob. Agents 55 (1), 105833. doi: 10.1016/j.ijantimicag.2019.10.014
Bassetti, M., De Waele, J. J., Eggimann, P., Garnacho-Montero, J., Kahlmeter, G., Menichetti, F., et al. (2015). Preventive and Therapeutic Strategies in Critically Ill Patients With Highly Resistant Bacteria. Intensive Care Med. 41 (5), 776–795. doi: 10.1007/s00134-015-3719-z
Brynildsen, M. P., Winkler, J. A., Spina, C. S., MacDonald, I. C., Collins, J. J. (2013). Potentiating Antibacterial Activity by Predictably Enhancing Endogenous Microbial ROS Production. Nat. Biotechnol. 31 (2), 160–165. doi: 10.1038/nbt.2458
Cebrero-Cangueiro, T., Alvarez-Marin, R., Labrador-Herrera, G., Smani, Y., Cordero-Matia, E., Pachon, J., et al. (2018). In Vitro Activity of Pentamidine Alone and in Combination With Aminoglycosides, Tigecycline, Rifampicin, and Doripenem Against Clinical Strains of Carbapenemase-Producing and/or Colistin-Resistant Enterobacteriaceae. Front. Cell Infect. Microbiol. 8, 363. doi: 10.3389/fcimb.2018.00363
Centers for Disease Control and Prevention (2019). Antibiotic Resistance Threats in the United States (Atlanta, GA: Centers for Disease Control and Prevention).
Cheng, X., Wang, X., Wan, Y., Zhou, Q., Zhu, H. (2015). And Wang, YMyosin Light Chain Kinase Inhibitor ML7 Improves Vascular Endothelial Dysfunction via Tight Junction Regulation in a Rabbit Model of Atherosclerosis. Mol. Med. Rep. 12 (3), 4109–4116. doi: 10.3892/mmr.2015.3973
Chiu, S. K., Huang, L. Y., Chen, H., Tsai, Y. K., Liou, C. H., Lin, J. C., et al. (2017). Roles of Ramr and Tet(a) Mutations in Conferring Tigecycline Resistance in Carbapenem-Resistant Klebsiella Pneumoniae Clinical Isolates. Antimicrob. Agents Chemother. 61 (8), e00391–17. doi: 10.1128/AAC.00391-17
Clinical and Laboratory Standards Institute (2021). Performance Standards for Antimicrobial Susceptibility Testing, 31th Ed. CLSI Supplement M100 (Wayne, PA: Clinical and Laboratory Standards Institute).
Dharmaraja, A. T. (2017). Role of Reactive Oxygen Species (ROS) in Therapeutics and Drug Resistance in Cancer and Bacteria. J. Med. Chem. 60 (8), 3221–3240. doi: 10.1021/acs.jmedchem.6b01243
Diancourt, L., Passet, V., Verhoef, J., Grimont, P. A., Brisse, S. (2005). Multilocus Sequence Typing of Klebsiella Pneumoniae Nosocomial Isolates. J. Clin. Microbiol. 43 (8), 4178–4182. doi: 10.1128/JCM.43.8.4178-4182.2005
Doi, Y. (2019). Treatment Options for Carbapenem-Resistant Gram-Negative Bacterial Infections. Clin. Infect. Dis. 69 (Suppl 7), S565–S575. doi: 10.1093/cid/ciz830
Dryden, M. (2018). Reactive Oxygen Species: A Novel Antimicrobial. Int. J. Antimicrob. Agents 51 (3), 299–303. doi: 10.1016/j.ijantimicag.2017.08.029
Dupuy, F. G., Chirou, M. V., de Arcuri, B. F., Minahk, C. J., Morero, R. D. (2009). Proton Motive Force Dissipation Precludes Interaction of Microcin J25 With RNA Polymerase, But Enhances Reactive Oxygen Species Overproduction. Biochim. Biophys. Acta 1790 (10), 1307–1313. doi: 10.1016/j.bbagen.2009.07.006
Durante-Mangoni, E., Andini, R., Zampino, R. (2019). Management of Carbapenem-Resistant Enterobacteriaceae Infections. Clin. Microbiol. Infect. 25 (8), 943–950. doi: 10.1016/j.cmi.2019.04.013
Ejim, L., Farha, M. A., Falconer, S. B., Wildenhain, J., Coombes, B. K., Tyers, M., et al. (2011). Combinations of Antibiotics and Nonantibiotic Drugs Enhance Antimicrobial Efficacy. Nat. Chem. Biol. 7 (6), 348–350. doi: 10.1038/nchembio.559
Farha, M. A., Verschoor, C. P., Bowdish, D., Brown, E. D. (2013). Collapsing the Proton Motive Force to Identify Synergistic Combinations Against Staphylococcus Aureus. Chem. Biol. 20 (9), 1168–1178. doi: 10.1016/j.chembiol.2013.07.006
Hayashi, W., Iimura, M., Soga, E., Koide, S., Izumi, K., Yoshida, S., et al. (2021). Presence of Colistin- and Tigecycline-Resistant Klebsiella Pneumoniae ST29 in Municipal Wastewater Influents in Japan. Microb. Drug Resist 27 (10), 1433–1442. doi: 10.1089/mdr.2020.0514
He, F., Shi, Q., Fu, Y., Xu, J., Yu, Y., Du, X. (2018). Tigecycline Resistance Caused by Rpsj Evolution in a 59-Year-Old Male Patient Infected With KPC-Producing Klebsiella Pneumoniae During Tigecycline Treatment. Infect. Genet. Evol. 66, 188–191. doi: 10.1016/j.meegid.2018.09.025
He, T., Wang, R., Liu, D., Walsh, T. R., Zhang, R., Lv, Y., et al. (2019). Emergence of Plasmid-Mediated High-Level Tigecycline Resistance Genes in Animals and Humans. Nat. Microbiol. 4 (9), 1450–1456. doi: 10.1038/s41564-019-0445-2
Hirabayashi, A., Ha, V. T. T., Nguyen, A. V., Nguyen, S. T., Shibayama, K., Suzuki, M. (2021). Emergence of a Plasmid-Borne Tigecycline Resistance in Klebsiella Pneumoniae in Vietnam. J. Med. Microbiol. 70 (3). doi: 10.1099/jmm.0.001320
Hladicz, A., Kittinger, C., Zarfel, G. (2017). Tigecycline Resistant Klebsiella Pneumoniae Isolated From Austrian River Water. Int. J. Environ. Res. Public Health 14 (10), 1169. doi: 10.3390/ijerph14101169
Jin, X., Chen, Q., Shen, F., Jiang, Y., Wu, X., Hua, X., et al. (2021). Resistance Evolution of Hypervirulent Carbapenem-Resistant Klebsiella Pneumoniae ST11 During Treatment With Tigecycline and Polymyxin. Emerg. Microbes Infect. 10 (1), 1129–1136. doi: 10.1080/22221751.2021.1937327
Karakonstantis, S., Kritsotakis, E. I., Gikas, A. (2020). Pandrug-Resistant Gram-Negative Bacteria: A Systematic Review of Current Epidemiology, Prognosis and Treatment Options. J. Antimicrob. Chemother. 75 (2), 271–282. doi: 10.1093/jac/dkz401
Kohanski, M. A., Dwyer, D. J., Hayete, B., Lawrence, C. A., Collins, J. J. (2007). A Common Mechanism of Cellular Death Induced by Bactericidal Antibiotics. Cell 130 (5), 797–810. doi: 10.1016/j.cell.2007.06.049
Kolich, L. R., Chang, Y. T., Coudray, N., Giacometti, S. I., MacRae, M. R., Isom, G. L., et al. (2020). Structure of Mlafb Uncovers Novel Mechanisms of ABC Transporter Regulation. Elife 9, e60030. doi: 10.7554/eLife.60030
Lam, P. L., Wong, R. S., Lam, K. H., Hung, L. K., Wong, M. M., Yung, L. H., et al. (2020). The Role of Reactive Oxygen Species in the Biological Activity of Antimicrobial Agents: An Updated Mini Review. Chem. Biol. Interact. 320, 109023. doi: 10.1016/j.cbi.2020.109023
Lin, H. B., Cadete, V. J., Sawicka, J., Wozniak, M., Sawicki, G. (2012). Effect of the Myosin Light Chain Kinase Inhibitor ML-7 on the Proteome of Hearts Subjected to Ischemia-Reperfusion Injury. J. Proteomics 75 (17), 5386–5395. doi: 10.1016/j.jprot.2012.06.016
Liu, Y., Jia, Y., Yang, K., Li, R., Xiao, X., Wang, Z. (2020). Anti-HIV Agent Azidothymidine Decreases Tet(X)-Mediated Bacterial Resistance to Tigecycline in Escherichia Coli. Commun. Biol. 3 (1), 162. doi: 10.1038/s42003-020-0877-5
Lv, L., Wan, M., Wang, C., Gao, X., Yang, Q., Partridge, S. R., et al. (2020). Emergence of a Plasmid-Encoded Resistance-Nodulation-Division Efflux Pump Conferring Resistance to Multiple Drugs, Including Tigecycline, in Klebsiella Pneumoniae. mBio 11 (2), e02930–19. doi: 10.1128/mBio.02930-19
Maisuria, V. B., Hosseinidoust, Z., Tufenkji, N. (2015). Polyphenolic Extract From Maple Syrup Potentiates Antibiotic Susceptibility and Reduces Biofilm Formation of Pathogenic Bacteria. Appl. Environ. Microbiol. 81 (11), 3782–3792. doi: 10.1128/AEM.00239-15
Maloney, P. C., Kashket, E. R., Wilson, T. H. (1974). A Protonmotive Force Drives ATP Synthesis in Bacteria. Proc. Natl. Acad. Sci. U.S.A. 71 (10), 3896–3900. doi: 10.1073/pnas.71.10.3896
Ni, W., Yang, D., Guan, J., Xi, W., Zhou, D., Zhao, L., et al. (2021). In Vitro and In Vivo Synergistic Effects of Tigecycline Combined With Aminoglycosides on Carbapenem-Resistant Klebsiella Pneumoniae. J. Antimicrob. Chemother. 76 (8), 2097–2105. doi: 10.1093/jac/dkab122
Pournaras, S., Koumaki, V., Spanakis, N., Gennimata, V., Tsakris, A. (2016). Current Perspectives on Tigecycline Resistance in Enterobacteriaceae: Susceptibility Testing Issues and Mechanisms of Resistance. Int. J. Antimicrob. Agents 48 (1), 11–18. doi: 10.1016/j.ijantimicag.2016.04.017
Rao, S., Bates, G. T., Matthews, C. R., Newport, T. D., Vickery, O. N., Stansfeld, P. J. (2020). Characterizing Membrane Association and Periplasmic Transfer of Bacterial Lipoproteins Through Molecular Dynamics Simulations. Structure 28 (4), 475–487 e473. doi: 10.1016/j.str.2020.01.012
Sheng, Z. K., Hu, F., Wang, W., Guo, Q., Chen, Z., Xu, X., et al. (2014). Mechanisms of Tigecycline Resistance Among Klebsiella Pneumoniae Clinical Isolates. Antimicrob. Agents Chemother. 58 (11), 6982–6985. doi: 10.1128/AAC.03808-14
Song, M., Liu, Y., Huang, X., Ding, S., Wang, Y., Shen, J., et al. (2020). A Broad-Spectrum Antibiotic Adjuvant Reverses Multidrug-Resistant Gram-Negative Pathogens. Nat. Microbiol. 5 (8), 1040–1050. doi: 10.1038/s41564-020-0723-z
Song, M., Liu, Y., Li, T., Liu, X., Hao, Z., Ding, S., et al. (2021). Plant Natural Flavonoids Against Multidrug Resistant Pathogens. Adv. Sci. (Weinh) 8 (15), e2100749. doi: 10.1002/advs.202100749
Sun, J., Chen, C., Cui, C. Y., Zhang, Y., Liu, X., Cui, Z. H., et al. (2019). Plasmid-Encoded Tet(X) Genes That Confer High-Level Tigecycline Resistance in Escherichia Coli. Nat. Microbiol. 4 (9), 1457–1464. doi: 10.1038/s41564-019-0496-4
Tong, Z., Xu, T., Deng, T., Shi, J., Wang, Z., Liu, Y. (2021). Benzydamine Reverses Tmexcd-Toprj-Mediated High-Level Tigecycline Resistance in Gram-Negative Bacteria. Pharmaceuticals (Basel) 14 (9), 907. doi: 10.3390/ph14090907
Xiong, Y., Wang, C., Shi, L., Wang, L., Zhou, Z., Chen, D., et al. (2017). Myosin Light Chain Kinase: A Potential Target for Treatment of Inflammatory Diseases. Front. Pharmacol. 8:292. doi: 10.3389/fphar.2017.00292
Xu, J., Gao, M., Hong, Y., He, F. (2017). Draft Genome Sequence of a Tigecycline-Resistant KPC-2-Producing Klebsiella Pneumoniae ST15 Clinical Isolate From China. J. Glob Antimicrob. Resist. 11, 156–158. doi: 10.1016/j.jgar.2017.10.017
Yaghoubi, S., Zekiy, A. O., Krutova, M., Gholami, M., Kouhsari, E., Sholeh, M., et al. (2021). Tigecycline Antibacterial Activity, Clinical Effectiveness, and Mechanisms and Epidemiology of Resistance: Narrative Review. Eur. J. Clin. Microbiol. Infect. Dis, 1–20. doi: 10.1007/s10096-020-04121-1
Yamaguchi, A., Ohmori, H., Kaneko-Ohdera, M., Nomura, T., Sawai, T. (1991). Delta Ph-Dependent Accumulation of Tetracycline in Escherichia Coli. Antimicrob. Agents Chemother. 35 (1), 53–56. doi: 10.1128/AAC.35.1.53
Yang, W., Moore, I. F., Koteva, K. P., Bareich, D. C., Hughes, D. W., Wright, G. D. (2004). Tetx Is a Flavin-Dependent Monooxygenase Conferring Resistance to Tetracycline Antibiotics. J. Biol. Chem. 279 (50), 52346–52352. doi: 10.1074/jbc.M409573200
Yao, H., Qin, S., Chen, S., Shen, J., Du, X. D. (2018). Emergence of Carbapenem-Resistant Hypervirulent Klebsiella Pneumoniae. Lancet Infect. Dis. 18 (1), 25. doi: 10.1016/S1473-3099(17)30628-X
Zgurskaya, H. I., Rybenkov, V. V., Krishnamoorthy, G., Leus, I. V. (2018). Trans-Envelope Multidrug Efflux Pumps of Gram-Negative Bacteria and Their Synergism With the Outer Membrane Barrier. Res. Microbiol. 169 (7-8), 351–356. doi: 10.1016/j.resmic.2018.02.002
Zhang, Y., Wang, X., Li, X., Dong, L., Hu, X., Nie, T., et al. (2019). Synergistic Effect of Colistin Combined With PFK-158 Against Colistin-Resistant Enterobacteriaceae. Antimicrob. Agents Chemother. 63 (7), e00271–19. doi: 10.1128/AAC.00271-19
Zheng, J. X., Lin, Z. W., Sun, X., Lin, W. H., Chen, Z., Wu, Y., et al. (2018). Overexpression of Oqxab and Macab Efflux Pumps Contributes to Eravacycline Resistance and Heteroresistance in Clinical Isolates of Klebsiella Pneumoniae. Emerg. Microbes Infect. 7 (1), 139. doi: 10.1038/s41426-018-0141-y
Keywords: Klebsiella pneumoniae, resistance genes, tigecycline, ML-7, combination therapy
Citation: Sun L, Sun L, Li X, Hu X, Wang X, Nie T, Zhang Y and You X (2022) A Novel Tigecycline Adjuvant ML-7 Reverses the Susceptibility of Tigecycline-Resistant Klebsiella pneumoniae. Front. Cell. Infect. Microbiol. 11:809542. doi: 10.3389/fcimb.2021.809542
Received: 05 November 2021; Accepted: 09 December 2021;
Published: 05 January 2022.
Edited by:
Ghassan M. Matar, American University of Beirut, LebanonReviewed by:
Luigi Principe, Azienda Sanitaria Provinciale di Crotone, ItalyWeihui Wu, Nankai University, China
Copyright © 2022 Sun, Sun, Li, Hu, Wang, Nie, Zhang and You. This is an open-access article distributed under the terms of the Creative Commons Attribution License (CC BY). The use, distribution or reproduction in other forums is permitted, provided the original author(s) and the copyright owner(s) are credited and that the original publication in this journal is cited, in accordance with accepted academic practice. No use, distribution or reproduction is permitted which does not comply with these terms.
*Correspondence: Youwen Zhang, eW91d2VuemhhbmdAaW1iLmNhbXMuY24=; Xuefu You, eHVlZnV5b3VAaW1iLnB1bWMuZWR1LmNu