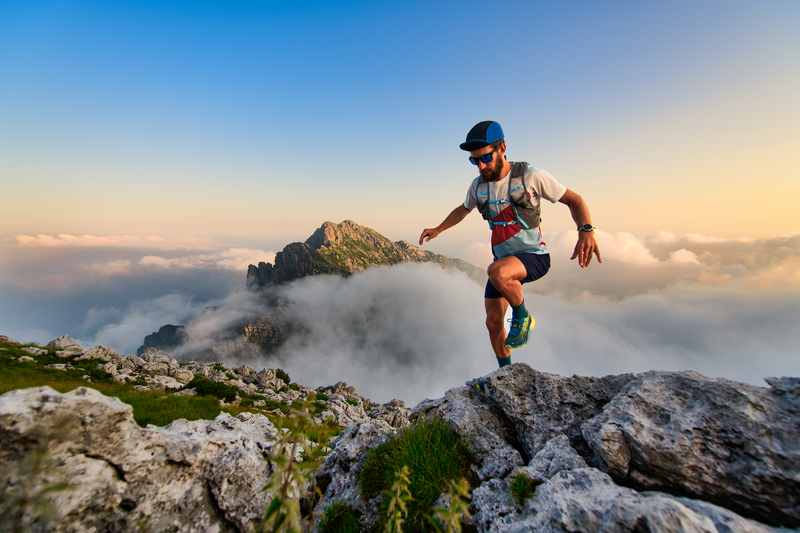
95% of researchers rate our articles as excellent or good
Learn more about the work of our research integrity team to safeguard the quality of each article we publish.
Find out more
REVIEW article
Front. Cell. Infect. Microbiol. , 17 December 2021
Sec. Microbiome in Health and Disease
Volume 11 - 2021 | https://doi.org/10.3389/fcimb.2021.790627
This article is part of the Research Topic The Female Reproductive Tract Microbiome - Gatekeeper for Sexual and Reproductive Health View all 20 articles
The microbiome, the collection of microbial species at a site or compartment, has been an underappreciated realm of human health up until the last decade. Mounting evidence suggests the microbiome has a critical role in regulating the female genital tract (FGT) mucosa’s function as a barrier against sexually transmitted infections (STIs) and pathogens. In this review, we provide the most recent experimental systems and studies for analyzing the interplay between the microbiome and host cells and soluble factors with an influence on barrier function. Key components, such as microbial diversity, soluble factors secreted by host and microbe, as well as host immune system, all contribute to both the physical and immunologic aspects of the FGT mucosal barrier. Current gaps in what is known about the effects of the microbiome on FGT mucosal barrier function are compared and contrasted with the literature of the gut and respiratory mucosa. This review article presents evidence supporting that the vaginal microbiome, directly and indirectly, contributes to how well the FGT protects against infection.
The female genital tract (FGT) can be divided into two distinct regions: lower and upper. The lower part of the FGT is composed of the vaginal canal and ectocervix, while the upper region is defined by the endocervix and uterus proper (Carias and Hope, 2018). The epithelium of the vaginal canal is organized in a stratified squamous configuration up until and including the ectocervix, which enables a barrier with multiple layers of epithelial cells to protect against extracellular pathogens (Figure 1). The stratified squamous epithelium originates from a basement membrane lined with progenitor cells, which then mature into fully senescent, and then keratinized epithelium (Chung et al., 2019; Ali et al., 2020). This allows for multiple levels of tight junction formation that keep out pathogens, while allowing the layer closest to the lumen to still be permissive to transudate from the blood and slough off to allow for mucosal shedding. This stratified squamous layer transitions to a simple columnar epithelial pattern at a point known as the ‘transformation zone’, which defines the area where the ectocervix becomes the endocervix. The simple columnar epithelial barrier continues into the uterine endometrium and beyond, and, as a result, the upper FGT has historically been thought to be more vulnerable to pathogens (Shattock and Moore, 2003). This is relevant in cases of cervical ectopy, more common among women who are young, pregnant, or on oral contraceptives, where the endocervix protrudes into the vaginal canal (Loudon et al., 1978; Wright et al., 2014). This protruding endocervix increases the vulnerability of the upper FGT to infection during sexual intercourse, and has been associated with the acquisition of sexually transmitted infections (STIs), such as in the case of chlamydia or human immunodeficiency virus (HIV) (Lee et al., 2006; Kleppa et al., 2015). In addition to the physical barrier of the epithelium, there is a layer of cervicovaginal mucus composed of mucus secreted by goblet cells of the endocervix that combines with cellular debris, vaginal transudate, and immune components to create a matrix that traps potential pathogens and prevents them from reaching or interacting with the epithelial layer and potential target cells (Lacroix et al., 2020). These immune components include secreted proteins such as immunoglobulins, which can bind to pathogens and interact with the cervicovaginal matrix to allow clearance from the FGT by mucus flow (Jensen et al., 2019), antimicrobial peptides (AMPs), and proteases. Despite being a mucosal membrane, where IgA antibodies tend to predominate, the vaginal mucosa is primarily characterized by IgG antibodies that are thought to originate from the vaginal transudate produced from the plasma that crosses from the bloodstream into the vaginal canal (Fahrbach et al., 2013; Oh et al., 2019). In addition to physical methods, there are chemical methods of pathogen protection that rely on the maintenance of an acidic environment within the lower FGT. This low pH environment feeds into, and in turn is fed by, a Lactobacillus dominant microbiome (Linhares et al., 2011).
Figure 1 Overview of FGT anatomy and components of barrier function. The FGT is divided generally into an upper (endometrium and endocervix) and a lower (vagina and ectocervix) tract. The lower FGT is considered the primary point of contact for pathogens, and harbors multiple innate immune mechanisms, such as AMPs, mucus, and immunoglobulins that prevent transmission across the epithelial barrier. The epithelium itself is composed of a stratified squamous epithelium that arises from a basement membrane and terminates in fully keratinized, senescent, cells. Microbiota, primarily Lactobacillus, can be found in high abundance within the lower FGT, but have also been shown to be resident within the upper FGT in lower numbers. As the epithelium progresses to the upper FGT it changes to a single columnar epithelium at a junction known as the ‘transformation zone’ between the ectocervix and endocervix. The upper FGT harbors unique uNK populations, as well as lymphoid aggregates consisting of B cells surrounded by CD8+ T cells and macrophage. These immune gatekeepers balance immune surveillance and response with the need to maintain a fertile environment for pregnancy. FGT, Female genital tract; IgA, Immunoglobulin A; IgG, Immunoglobulin G; DC, Dendritic cell; NK, Natural killer; uNK, Uterine natural killer; AMP, Antimicrobial peptide.
The microbiome of the FGT differs between the lower and upper portions of the tract, with the lower FGT estimated to have a bacterial load of 102-104 fold higher than that seen in the upper FGT (Chen et al., 2017; Baker et al., 2018). Microbiomes of the lower FGT, henceforth referred to as vaginal microbiomes, are divided into five separate community state types (CSTs) that capture the overall dominance of a particular bacterium within the vagina (Ravel et al., 2011; Ma and Li, 2017). While bacteria of each state are all considered commensal, or regular residents in healthy individuals, microbiomes that are non-Lactobacillus dominant tend to be associated with poor health outcomes and high degrees of inflammation (Gautam et al., 2015; Petrova et al., 2015; Ma and Li, 2017; De Seta et al., 2019). The vaginal mucosal microbiome is typically less diverse than other sites, with Lactobacillus species comprising the major microbiota of the vaginal mucosa (Ravel et al., 2011; Aldunate et al., 2015; Petrova et al., 2015; Song et al., 2020). Among the Lactobacillus species, Lactobacillus crispatus, L. gasseri, L. iners, and L. jensenii are the most common species identified at the human vaginal mucosa. A large-scale cross-sectional study showed that L. crispatus, L. gasseri, L. iners, and L. jensenii were dominant in 26.2%, 6.3%, 34.1% and 5.3% of the samples analyzed, respectively (Ma et al., 2012). The remaining 28% of samples were dominated with non-Lactobacillus species and displayed high diversity in microbial composition (Ma et al., 2012). Women are grouped into the five CSTs based primarily on the predominance of a given Lactobacillus species in the vaginal mucosa. CST-I, -II, -III, and -V are characterized by L. crispatus, L. gasseri, L. iners, and L. jensenii dominance, respectively, whereas CST-IV represents a highly diverse, or non-Lactobacillus dominant, microbiome (Ravel et al., 2011). CST-I, -II, and -V are considered optimal vaginal microbiomes, characterized by low pH (pH less than 4.0–5.0), high lactic acid concentrations, and a less inflammatory state of the vaginal microenvironment (Hedges et al., 2006; Ravel et al., 2011). CST-III women who are dominated by L. iners differ from other Lactobacillus dominated CST groups in that they display higher pH ranges and relatively higher inflammatory statuses at the vaginal mucosa (Verstraelen et al., 2009). Ongoing studies found differences in the genome size and genome structure of L. iners compared to other Lactobacillus species, however additional studies are required to explain how these differences contribute to the differences in vaginal milieu (Mendes-Soares et al., 2014). CST-IV is dominated by a varied range of facultative or strictly anaerobic bacteria in the vaginal mucosa, and is considered a non-optimal vaginal microbiome. It is characterized by higher pH levels (pH >5.0), lower levels of lactic acid, and an increased inflammatory state (Hedges et al., 2006; Ravel et al., 2011; Vagios and Mitchell, 2021). Despite the differences displayed in CST-IV, when present without any symptoms it is considered a healthy state. Bacterial genera that dominate in the CST-IV group, however, such as Atopobium, Gardenella, Mobiluncus, Prevotella, Anaerococcus, and Sneathia, are often associated with a symptomatic disorder of the vaginal microbiome known as bacterial vaginosis (BV) (Schellenberg et al., 2011; Ma et al., 2012).
The microbiome is regulated through (1) the microbial species that exert their own influence on species pressure, (2) the introduction of new species through foreign introduction, and (3) host factors such as genetics, diet, and hormones that promote the growth of certain bacterial genera. There have been a great deal of association studies performed analyzing the link between the vaginal microbiome and FGT barrier function and immunity, but studies of regulatory mechanisms have been lacking. In order to examine these relationships in depth, several models have been generated in which the conditions of both the lower and upper FGT are simulated and microbes of interest can be included to characterize their role in affecting immune response, barrier permeability, and the secretion of metabolites. These models present the best opportunity to move many of the discovered relationships past association and into causal explanation.
Most experimental models of the FGT mucosa focus primarily on the lower FGT, composed of the ectocervix and vagina, with a smaller number focusing on the upper FGT, composed of the endocervix and endometrium. Initial studies utilized monolayer cultures of cervical and vaginal epithelial cells to study barrier function, but more recently three-dimensional (3D) models using cervical and vaginal epithelial cells have been developed. Cervical and vaginal explant tissue models, and in vivo systems, have also been used to study the regulation of cervicovaginal barrier function. A brief description of these models, and their use in studying FGT mucosal barrier function, will be discussed in the following sections (Figure 2 and Table 1).
Figure 2 Different types of models to study cervicovaginal mucosal barrier function. 2D, Two dimensional; 3D, Three dimensional; ECM, Extracellular matrix; ALI, Air-liquid interface; CVL, Cervicovaginal liquid; RWV, Rotating well vessel; CE-OOC, Organ-on-chip cervical epithelial.
Immortalized vaginal (Vk2), ectocervical (Ect1), and endocervical (End1) epithelial cell lines have been generated to study FGT epithelial function by transducing the primary cells isolated from cervicovaginal biopsies with human papillomavirus 16 (HPV-16) E6 and E7 genes (Fichorova et al., 1997). These epithelial cell lines have been used in generating both 2D (monolayer) and 3D models (Figure 2). These epithelial cells are grown on tissue culture-treated plasticware in monolayer models to study the effects of exogenous factors on epithelial function and properties. Vk2, Ect1, and End1 cells have been reported to express certain toll like receptors (TLRs) in 2D culture and have been exposed to TLR agonists to induce the production of cytokines, chemokines, and antimicrobial peptides (AMPs) (Fichorova et al., 1997; Dusio et al., 2011). Study of epithelial wound-healing properties has primarily been performed using 2D culture models (Dusio et al., 2011). The FGT mucosal metabolite low-molecular-weight hyaluronic acid (LMW-HA) has been shown to regulate barrier function using 2D culture of Vk2 cells. Treatment of Vk2 monolayers with LMW-HA induces secretion of AMPs, such as β-defensin-2, and promotes the wound-healing process by increasing migration of Vk2 cells through activation of phosphatidylinositol 3-kinase and myosin light chain kinase (Dusio et al., 2011). The 2D epithelial monolayer model is often used because of its simplicity, short-time frame, cost-effectiveness, and reproducibility of the results. The 2D monolayer model of cervicovaginal epithelium, however, differs vastly from physiological cervicovaginal tissue in terms of cellular differentiation, proliferation, protein expression, multilayer formation, and cell to cell junction formation between stratified squamous epithelial cells, as well as in the interaction between epithelial cells and the extracellular matrix (ECM) which is completely absent in the 2D model (Soares et al., 2012; Chitcholtan et al., 2013) (Table 1). Hence, 3D models of the cervicovaginal epithelium have been developed to overcome some of these limitations.
In 3D models, epithelial cells are grown on ECM such as collagen, matrigel, or fibronectin. When a dual-chamber culture system is used in a 3D model, transwell inserts with semipermeable membranes coated with ECM material are used (Gali et al., 2010; Lee et al., 2016; Woods et al., 2021). Primary or immortalized epithelial cells are grown on the supporting ECM in the upper chamber, and the lower chamber can be used for culturing any tissue resident cells, such as immune cells. Using this culture system, epithelial cells can be induced to differentiate and polarize in air-liquid interface (ALI) conditions (Lee et al., 2016). In ALI conditions, liquid culture media in the upper chamber (the apical side of the epithelium) is removed after the epithelial monolayer on the ECM becomes confluent (Figure 2), allowing the nutrients to be supplied by the culture media in the bottom chamber (the basal side of the epithelium). ALI conditions, simulating a mucosal environment where nutrients are supplied by blood and epithelium is exposed to air, trigger cellular differentiation and formation of cell-cell junctions (Lee et al., 2016). This model is suitable for studying epithelial integrity, regulation of tight junction and adhesion junction formation, and transmigration of pathogens through the epithelium. Trans-epithelial electrical resistance (TEER) and fluorophore-conjugated dextran diffusion assay are commonly used to assess epithelial integrity in the dual-chamber model systems (Gali et al., 2010). Vk2 and Ect1 cells in particular have been grown in dual-chamber 3D culture model systems to study epithelial immune and barrier function (Gali et al., 2010; Lee et al., 2016) (Table 1). The ALI model of Vk2 cells exhibits both multilayer and tight junction formation, and has been used to show that stimulation of Vk2 cells with the hormone progesterone increases their susceptibility to herpes simplex virus 2 (HSV2) infection (Lee et al., 2016). Primary genital epithelial cells (GECs), particularly endocervical and endometrial cells, have been isolated from hysterectomy tissue, cultured in the dual-chamber system, and shown to form tight junctions as well (Kaushic et al., 2011; Ferreira et al., 2013). Following exposure to HIV-1, increased GEC permeability was observed in this model system. Co-culture of HIV-exposed GECs with the probiotic bacteria Lactobacillus reuteri RC-14 and Lactobacillus rhamnosus GR-1, as well as the female sex hormone estrogen, was shown to restore GEC barrier function and tight junction formation (Dizzell et al., 2019). The major limitation of this 3D model includes high technical variability, variations between cell lines and primary cells, and, most of all, its deviation from physiological conditions, for example mucus-secretion and an anaerobic environment (Gali et al., 2010; Lee et al., 2016). As a consequence, this is not an ideal system to study interactions between the microbiome and epithelium.
The organotypic vaginal-ectocervical (VEC) tissue model also uses a dual-chamber system. In the VEC tissue model, primary ectocervical cells are isolated from the VEC tissue obtained from hysterectomy and cultured on transwell inserts using ALI conditions to induce differentiation and polarization (Aldunate et al., 2015) (Figure 2). Before culturing the ectocervical epithelial cells on the transwell inserts, the inserts are seeded with a fibroblast and collagen mixture to create a basal membrane. Fibroblast cells are isolated from the same donor used to obtain ectocervical cells to avoid allogeneic immune effects. Ectocervical cells are then cultured on top of the fibroblast and collagen gel matrix, which creates a VEC tissue model containing an apical cell layer, suprabasal layer, and basal cell layer post-differentiation, along with a similar type of cytokeratin expression compared to actual VEC tissue (Ayehunie et al., 2006). This model shows more resistance against washings, lubricants, and anti-fungal creams compared to non-organotypic epithelial models, and represents a more relevant physiological scenario (Aldunate et al., 2015) (Table 1). Although the 3D and VEC tissue dual-chamber models are advantageous for cell differentiation, tight junction formation, and cytokeratin expression, they still lack many aspects of the FGT mucosa. For example, studying the barrier properties of mucus at the FGT mucosa is not possible with these models, since mucus secretion has yet to be reported using VEC tissue models.
Another 3D epithelial tissue model using a rotating wall vessel (RWV) bioreactor and immortal vaginal (V191), endocervical (A2EN), and endometrial (HEC-1A) cell lines has been developed by Dr. M. Herbst-Kralovetz’s group. The epithelial tissue in this model has been shown to secrete mucus, and exhibit proper cytokeratin expression, microvilli, and tight junction formation (Figure 2 and Table 1) (Hjelm et al., 2010; Radtke et al., 2012; Doerflinger et al., 2014; Laniewski et al., 2017; Łaniewski and Herbst-Kralovetz, 2019; Ilhan et al., 2020). To start, epithelial cells are grown on collagen-coated dextran microcarrier beads. The cell and bead suspensions are then transferred to the RWV bioreactor to be cultured for 39-42 days to generate fully differentiated 3D tissue models (Figure 2). When used in studying the interaction between epithelium and microbes, colonization of this vaginal RWV-3D tissue model with the anaerobic bacterium Atopobium vaginae resulted in increased secretion of cell-associated mucins, proinflammatory cytokines and chemokines, and AMPs as compared to colonization with Lactobacillus species (Doerflinger et al., 2014). Although the RWV-3D tissue model allows the evaluation of the role of mucus and anaerobic microbes in regulating epithelial barrier function, the challenges of reproducibility and the differences in gene expression between cell lines and primary tissue have not been resolved.
The organoid models, such as the stratified ectocervical organoid and cystic endocervical organoid models, use primary ectocervical and endocervical cells isolated from cervical tissue obtained during hysterectomy procedures (Table 1) (Lõhmussaar et al., 2021). Primary cells are embedded into a basement membrane extract and plated in 30 µL droplets on pre-warmed 24-well suspension culture plates, then grown in culture media with growth supplements to develop spherical organoids (Figure 2) (Lõhmussaar et al., 2021). Both organoid models have been used to study cellular susceptibility to HSV-2 infection, but have yet to be used to study epithelial barrier function (Lõhmussaar et al., 2021).
The most current in vitro cervical tissue model is the organ-on-chip cervical epithelial (CE-OOC) model. The CE-OOC model contains two chambers to co-culture immortalized ectocervical and endocervical epithelial cells, and the two chambers are connected by micro-channels that allow the epithelial cells to migrate (Figure 2) (Tantengco et al., 2021). The phenomena of epithelial to mesenchymal transition (EMT) and mesenchymal to epithelial transition (MET) have been studied using the CE-OOC model (Table 1). In that, ectocervical cells have been reported to form fibroblastoid morphology, and endocervical cells form pseudopods while migrating through micro-channels from one chamber to the other, in monoculture systems (Tantengco et al., 2021). This model has been used to study normal and pathogenic cellular remodeling of the cervix, and the interaction between ectocervical and endocervical cells during infection and inflammation (Tantengco et al., 2021). Although in vitro 3D models of epithelium provide a close resemblance to physiological tissue, they still present challenges, such as high technical variability, large-scale experimental requirements, high costs, and being time intensive. Moreover, these 3D models cannot be used to study epithelial barrier properties in the presence of non-epithelial cells such as stromal and sub-epithelial immune cells (Table 1).
Cervical tissue explants, either collected from cervical tissue after hysterectomy or cervical punch biopsies, have been previously used for HIV transmission studies (Phalguni et al., 2006; Grivel and Margolis, 2009; Mitchell et al., 2014; Trifonova et al., 2018). Ectocervical, endocervical, and transformation zone physiology in particular have been studied using this model (Table 1) (Goldfien et al., 2015). The cervical explant tissue model is the closest model to an in vivo model, since the explant tissue is composed of epithelial cells, subepithelial immune cells, and fibroblasts. This model allows for studying the interaction between epithelial, immune, and fibroblast cells during infection as well. Fresh and cryopreserved cervical tissue explants provide similar results in infection studies, indicating the ability to use frozen tissue explants as an alternative to fresh tissue explants. This allows researchers to circumvent one of the major potential drawbacks of using cervical tissue explants—sample preservation (Phalguni et al., 2006; Fox et al., 2017). The cervical and endometrial tissue explant model has been used to observe the effect of hormonal contraceptives in changing barrier properties and infection susceptibility as well. Explant tissue models are used to study hormonal contraceptive effects on epithelial layer thickness, cell proliferation, alteration of tight junction and secretory proteins, and gene transcription (Table 1). The explant tissue model is not without challenges. It still lacks an anaerobic environment, and can be highly variable due to biological differences between tissue donors.
Besides isolating explant tissue, cervicovaginal lavage (CVL) and vaginal swabs from women have also provided crucial insights in uncovering the regulation of cervicovaginal barrier function (Figure 2). Proteomic studies with CVL using high throughput mass spectrophotometry have reported that an increase in vaginal microbiome diversity is associated with a decrease in a protein expression profile that supports barrier function (Borgdorff et al., 2016). The limitations that come with this model are challenges achieving the proper ethics and appropriate number of clinical participants, along with longer time frames and higher expense (Table 1).
Although not perfect, the use of animal models allows the investigation of how vaginal bacterial composition, and other local factors, regulate cervicovaginal barrier function in a complete living system (Lewis et al., 2013). Various types of tissue, such as upper and lower FGT, and fluids, such as CVL and plasma, can be isolated from animal models for systematic analyses to obtain a more comprehensive, and unbiased, picture (Figure 2 and Table 1). For example, a study using C57BL/6 mice (6–8 weeks old) colonized with the mouse-adapted strain of G. vaginalis showed degradation of vaginal mucus and consequent weakening of vaginal barrier properties compared to control mice (Lewis et al., 2013). To study microbes that infect only human cells, bone marrow-liver-thymus (BLT)-transplanted humanized mouse models have been used. The BLT mouse has been used to test microbicides against HIV-1 and study changes in cervicovaginal barrier properties during HIV transmission (Paul et al., 2011; Maud and Andrew, 2013; Nirk et al., 2018; Shariq et al., 2019). These BLT mouse models, in concert with non-human primate (NHP) models, are critical in studying the interaction between cervicovaginal epithelial cells and sub-epithelial immune cells in the regulation of cervicovaginal barrier function in the context of HIV infection. The microbial composition and physiological conditions of these animal models are different from that of the human, and need to be considered when translating findings to human research or treatment.
The composition of vaginal microbial communities influences the vaginal defense system of the mucosal barrier by inhibiting the growth of disease-causing pathogenic microorganisms by either producing microbicidal compounds, or by enhancing host physical and immunological barrier function. One of the critical components of physical barrier function at the FGT mucosa is mucus. L. crispatus dominated vaginal microbiomes are associated with mucus that traps microbes, such as HIV, more efficiently as compared to the mucus associated with vaginal microbiomes dominated by L. iners or Gardenella species (Nunn et al., 2015). The knowledge of how vaginal microbes affect the role of mucus in defense remains scarce. Mucus can be compromised in the case of degradation by BV-associated bacteria. BV-associated bacteria, such as Gardnerella, Prevotella, and Bacteroides, make the sialidase enzyme that actively degrades sialic acid—a critical component of FGT mucus (Lewis et al., 2013; Vagios and Mitchell, 2021). Other mucus-degrading enzymes, including mucinases, sulfatases, galactosidases, and prolidases, damage FGT barrier integrity by contributing to the watery vaginal discharge characteristic of BV (Lewis et al., 2013; Vagios and Mitchell, 2021). Besides mucus, host proteins in vaginal secretions also play a key role in vaginal epithelial barrier function. Proteomic analysis of CVL samples collected from a cross-sectional study of 50 Rwandan female sex workers resulted in four groups with distinct compositions of dominating vaginal microbiomes. Group 1 is associated with L. crispatus dominant vaginal microbiomes, group 2 is associated with L. iners dominant vaginal microbiomes, group 3 is associated with anaerobe dominant vaginal microbiomes along with intermediate vaginal microbial diversity, and group 4 is associated with anaerobe dominated vaginal microbiomes along with high vaginal microbial diversity. The protein profiles of the CVL samples from these women further showed that increasing vaginal microbial diversity was positively associated with increased protein levels in the biological pathways of cell death, proteasome and protease activity, and pro-inflammatory cytokines. The increased vaginal microbial diversity was negatively associated with the levels of keratin protein, cornified envelop proteins, humoral immune molecules, such as IGHA1 and IGHG2, and anti-protease activity (Borgdorff et al., 2016). Together, this study showed that increasing vaginal microbial diversity is associated with increases of detrimental, and decreases of beneficial, host protein levels pertaining to epithelial vaginal barrier function. As these are associations, it remains to be shown whether changes in host barrier function permit the diversification of vaginal microbiome or whether the diversification of vaginal microbiome causes the changes in host barrier function. Moreover, the molecular mechanisms of how vaginal microbial diversity interacts with vaginal mucosal protein expression remain to be sought.
AMPs are part of the mucosal chemical barrier against the foreign environment. Vaginal levels of AMPs are also found to be closely related to vaginal microbial diversity. Increased FGT microbial diversity is associated with decreases in AMPs, such as lysozyme C (LYZ) and ubiquitin (RPS27A), and increased S100A9 (Borgdorff et al., 2016). Changes in AMP levels have also been reported during BV, with human beta defensin-2 (HBD-2), lactoferrin, and cathelicidin (LL-37) found to be higher in the CVL of women with BV (Fan et al., 2008; Gregory et al., 2011; Frew et al., 2014). In vitro experiments also found increased secretion of HBD-2 from epithelial cells co-cultured with BV associated bacteria, such as A. vaginae and L. iners (Doerflinger et al., 2014). However, how increases in vaginal microbial diversity, and the condition of BV, influence secretion of AMPs remains to be explored.
Vaginal fluid contains not only host factors, but also microbial metabolites and enzymes. This profile is largely dependent on the composition of the vaginal microbiome and the interaction between host cells and microbiota (Srinivasan et al., 2015). The D-lactic acid (D-LA) isoform is predominantly produced over L-lactic acid (L-LA) in L. crispatus, L. gasseri, and L. jensenii, as compared to L. iners, which produces only L-LA (Steven et al., 2013; Nunn et al., 2015). It is suggested that vaginal concentrations of D-LA and L-LA may be a key determinant in vaginal microbiome diversity and vaginal health. Vaginal mucus with high concentrations of D-LA exhibits enhanced trapping of HIV-1 particles, compared to mucus with low concentrations of D-LA characteristic of L. iners dominant microbiomes. (Nunn et al., 2015). Both D-LA and L-LA can reduce the pH of the vaginal mucosa and prevent the growth of yeast and other microbes, as well as reduce the release of pro-inflammatory molecules by cervicovaginal epithelial cells (Hearps et al., 2017). Hearps et al. showed that in vitro treatment of human vaginal and cervical epithelial cell lines with LA (pH 3.9) induced production of the anti-inflammatory cytokine IL-1RA. Further, when added simultaneously or prior to stimulation, LA inhibited the TLR agonist-induced production of pro-inflammatory mediators IL-6, IL-8, TNFα, RANTES, and MIP3α from epithelial cell lines, and prevented IL-6 and IL-8 production elicited by exposure to seminal plasma (Hearps et al., 2017). Similar anti-inflammatory effects of LA hold true for primary cervicovaginal cells and when organotypic epithelial tissue models are used.
In addition, Lactobacillus species, except for L. iners, also produce hydrogen peroxide and bacteriocins as byproducts that inhibit growth of pathogenic non-indigenous bacteria (Vallor et al., 2001; Mich` et al., 2015). Alternatively, L. iners secretes cholesterol-dependent cytolysin (CDC) and G. vaginalis secretes vaginolysin, both of which are known to have cytotoxic activity against vaginal and cervical epithelial cells (Cadieux et al., 2009; Macklaim et al., 2013). In an in vitro study, culture supernatant of L. iners and Gardenella increased the permeability of ectocervical and endocervical cell culture by impairing the cellular adhesion molecule E-cadherin, and increased release of soluble cadherin compared to L. crispatus culture supernatant (Anton et al., 2018). In contrast, L. crispatus secreted byproducts in culture supernatant that helped to restore ectocervical and endocervival barrier integrity, previously disrupted by Gardenella culture supernatant (Anton et al., 2018). Unfortunately, this study did not explore the byproduct profiles in these culture supernatants. Succinate and short-chain fatty acids, such as butyrate, propionate, and acetate, commonly present in the gut mucosa, are also found at low levels in the vaginal mucosa. In the condition of BV, however, much higher levels of SCFAs have been found (Mirmonsef et al., 2011). A meta-transcriptomic study also showed the up regulation of butyrate metabolizing enzymes, butyryl-CoA-dehydrogenase, and butyrate kinase during BV, associated with increased P. amnii and Megasphaera (Macklaim et al., 2013). Usually, SCFAs exhibit a beneficial role in gut mucosa by improving gut epithelial integrity, differentiation, proliferation, and reducing the secretion of pro-inflammatory molecules from gut mucosal epithelial and immune cells (Blacher et al., 2017). Knowledge of the role of SCFAs in the FGT mucosa remains limited. An in vitro study found contrary results that high levels of SCFAs can induce pro-inflammatory molecule secretion from vaginal and cervical epithelial cells (Delgado-diaz et al., 2020). More studies are required to explore the impact of higher SCFA levels in changing FGT mucosal barrier function and its association with BV conditions.
The description of the FGT immunologic milieu deserves another review or book chapter by itself. This review will only summarize key immune cells and mediators that are relevant for the discussion of the interactions between FGT host cells and the microbiome.
The lower FGT is characterized by IgG present from vaginal transudate and local mucosal IgA antibodies (Fahrbach et al., 2013; Gunn et al., 2016), antimicrobial peptide secretion (Yarbrough et al., 2015), and the presence of both adaptive and innate immune cells (Zhou et al., 2018; Mei et al., 2019). T cells exist primarily below the substratum of the epithelium, and provide cytokine and chemokine responses to address invading pathogens or barrier tissue damage. Macrophage and neutrophils are present in the tissue, maintaining the homeostasis between the microbial environment and the host epithelium. In response to invasion of the FGT, macrophage and neutrophils function to remove pathogenic, and bystander commensal, bacteria and promote wound healing (Wira et al., 2005; Jennifer et al., 2021). The FGT immunologic milieu changes as the FGT transforms to the upper compartment, where the physiological emphasis changes from focusing on homeostasis and defense to focusing on the balances of both homeostasis and the readiness to maintain a potential pregnancy (Ochiel et al., 2008; Agostinis et al., 2019). The uterine cavity is home to the unique uterine natural killer (uNK) cell population, as well as a high degree of gamma delta (γδ) T cells and macrophage (Amy et al., 2012; Zhou et al., 2018; Monin et al., 2020). At the upper FGT, the profiles of secreted antimicrobial peptides and cytokines focus on the production of anti-inflammatory cytokines alongside AMPs. The uterus is able to achieve high degrees of immune surveillance due to the presence of lymphoid aggregates, dense groupings of B and CD8+ T cells, which allow for targeted and controlled responses to foreign antigen in the localized immune environment of the uterus (Yeaman et al., 2001; Agostinis et al., 2019). Advances in uterine immune regulation are summarized in detail in the review articles by Anne et al, Abebe et al, and Liman et al. (Anne et al., 2014; Abebe et al., 2021; Liman et al., 2021).
The composition of the microbiome found in the lower and upper FGTs are quite distinct; however, there is a clear association between the species present within the lower FGT and the species found in the upper FGT (Baker et al., 2018; Agostinis et al., 2019). For example, Lactobacillus species typically dominate the lower FGT, but comprise only approximately a quarter of the upper FGT microbiome on average, while other genera such as Pseudomonas, Acinetobacter, and Sphingobium make up the remaining portion. It is unknown whether these genera arise from occult introduction, or if they travel from the gut and other sites to colonize the uterus directly (Łaniewski et al., 2020). For much of the history of FGT microbiome research, the upper FGT has been thought to be a sterile environment in healthy women, but this notion has been challenged by recent advances in sequencing and sampling technology that have uncovered a potentially omnipresent, if variable, uterine microbiome.
Microbiome composition has been associated directly with health and disease (Swain and Ewald, 2018). The interplay between the microbiota and the human immune system in the gut, and to a lesser extent in the respiratory tract, have been examined extensively during the last decade to yield translational knowledge with implications for mucosal health. However, such knowledge at the FGT remains scarce. It was thought that host immune cells and immune mediators play a direct role in shaping the composition of the FGT microbiome, which consequently impacts barrier function and the ability to protect against STIs. There still remains no direct evidence for this hypothesis, however. Based on what has been learned of the gut mucosa (Yasmine and Oliver, 2017; Zheng et al., 2020), the FGT microbiota could provide critical signals for the development and function of the host immune system, and, the host immune system, in turn, could have evolved multiple means by which to maintain its symbiotic relationship with the microbiome. The microbiome of the lower and upper FGTs interact with the immune mechanisms of the host primarily through interaction with immune components, such as immunoglobulins, where they can reduce the efficacy of these molecules via neutralization through degradation or competitive binding. General inflammation and mucus breakdown has also been observed with the CST-IV, or BV-associated, microbiome (Lewis et al., 2013; Lacroix et al., 2020). How the host immune system is ‘educated’ to accommodate the commensal microbial community is critical. At the gut mucosa, such mechanisms exist to tolerate food antigens and microbes that are essential for gut function (Chistiakov et al., 2014). If such a tolerating mechanism fails, undesirable inflammation results in disease. At the FGT, the combination of liquefaction of the mucus barrier and increased recruitment of immune cells due to local pro-inflammatory cytokines and chemokines leads to increased risk of immune cell infection by pathogens such as HIV, as well as direct epithelial infection by pathogens such as HSV-2 and chlamydia (Koumans et al., 2007; Passmore et al., 2016; Bautista et al., 2017). This also creates a self-reinforcing cycle where host pro-inflammatory immunity can have non-specific and antigen-specific removal and/or inhibition of commensal microbes, and potentially further prevent the establishment of a Lactobacillus dominant microbiome. Disrupting the homeostasis of the commensal microbial environment may potentiate further exacerbation of inflammation at the FGT mucosa. How the uterus, and pregnancy benefit from hosting commensal microbes, and how the uterine/endometrial immune system tolerates its associated microbiota to maintain a homeostatic microenvironment, urgently require further studies.
Hormones have a direct influence on both the immune system and the microbial community present within the FGT. Together, all three play crucial roles in regulating FGT barrier function. During pregnancy, and at puberty, increases in the female sex hormone estrogen promote the proliferation and differentiation of vaginal epithelial cells. Glycogen from exfoliated and lysed epithelial cells is converted by α-amylase, and the product is then metabolized to lactic acid by Lactobacillus species in the vaginal lumen. A glycogen-rich environment directly promotes the growth and dominance of Lactobacillus species (Amabebe and Anumba, 2018). This is further reinforced through the production of hydrogen peroxide, bacteriocins, and biosurfactants from Lactobacillus species. Elevated estrogen levels are also associated with a thicker epithelial lining with greater elasticity, perhaps via promoting the proliferation and differentiation of FGT epithelial cells. The synergistic effect of estrogen-high and Lactobacillus dominant environments associates most strongly with improved barrier function, vaginal health metrics, and protection from STIs and cancer.
In contrast, progesterone, the other commonly considered female sex hormone, has no significant association with any CST group. Although progesterone has been shown to play a protective role in preventing inflammation-induced preterm labor during pregnancy (Dodd and Crowther, 2009), the use of the hormonal contraceptive medroxyprogesterone acetate, a progesterone analogue that has different biological effects, is associated with increased vaginal microbiome diversity that potentially modulates vaginal inflammation and increased HIV-1 susceptibility in humanized mouse models (Wessels et al., 2019). The use of depot medroxyprogesterone acetate (DMPA) is also associated with the CST IV-type microbiome in the FGT, and a higher risk for acquiring BV (Wessels et al., 2019). The reduction in robust epithelial barrier support, with concomitant increased risk of inflammatory and dysbiotic states, is thought to be partially responsible for explaining DMPA’s association with increased HIV risk and proteome signatures of epithelial barrier disruption (Birse et al., 2017). Several studies also reported the association of elevated progesterone with a more inflammatory environment and a less robust barrier at the vaginal epithelium via unknown mechanisms (Tjernlund et al., 2015; Vitali et al., 2017; Bradley et al., 2018). While the mechanism requires further investigation, DMPA has also been reported to have neutral or even detrimental effects to the elasticity of the vaginal membrane leading to increased chances of vaginal dryness and epithelial tearing (Irvin and Herold, 2015; Birse et al., 2017; Zalenskaya et al., 2018). With the cyclic nature of the human menstrual cycle, FGT barrier function may change throughout a given cycle depending on the balance of estrogen and progesterone. Indeed, during menses there is often a complete loss of a woman’s characteristic CST, which is often restored shortly at the end of menses (Gajer et al., 2012). In general, the human FGT microbiome appears to remain stable despite the shifts in hormones throughout the menstrual cycle. While it is clear that highly elevated estrogen and DMPA levels have direct effects on vaginal microbiome composition, it is unknown if the FGT microbiome has its own impacts on hormone production, or activity, locally within the FGT.
Outside of female sex hormones, oxytocin, a hormone and neuropeptide normally produced in the hypothalamus and released by the posterior pituitary, has been explored as an alternative to estrogen-based therapies for vaginal dryness. It has been shown to be effective clinically as a vaginal gel in alleviating menopausal vaginal symptoms and in vitro in promoting proliferation of Vk2 vaginal epithelial cell lines (Kallak and Uvnäs-Moberg, 2017; Torky et al., 2018). Tremendous work remains to validate oxytocin’s role in the interplay between the immune system, the microbiome, and its effects on FGT barrier function.
Age, inextricably linked with hormone levels, is a core determinant of the FGT microbiome. Data across ages for FGT microbiomes only exists for the lower FGT, with microbiome signatures for the upper FGT only existing for women of reproductive age (Gupta et al., 2019; O’Callaghan et al., 2020). In early infancy the lower FGT is colonized by Lactobacillus species due to the prenatally inherited high estrogen levels from the mother’s blood. Approximately six weeks post-birth, vaginal microbiomes progress to a microbiome more reminiscent of skin with a complete loss of Lactobacillus dominance. This persists up until puberty, when production of estrogen increases, and menstruation begins, and Lactobacillus species dominate the lower FGT once again. This is notably true in those of Caucasian and Asian descent, but this pattern is less prevalent in those of African ancestry where vaginal microbiomes may develop into polymicrobial communities with greater frequency (Ravel et al., 2011; Fettweis et al., 2014). It remains unknown whether this is the influence of genetic or cultural and epigenetic factors. Regardless, the higher prevalence of polymicrobial vaginal microbiomes is considered a risk factor for HIV acquisition and BV development in this population (Kyongo et al., 2015; Alcendor, 2016; Dabee et al., 2019). Along with changes to the microbiome, pH levels begin to decrease from 7.0 in pre-puberty to the 4.0–5.0 range typical of normal, healthy, vaginas (Gupta et al., 2019). This is mostly due to the dominance of Lactobacillus species and the production of lactic acid and hydrogen peroxide at the vaginal mucosa. These influences persist throughout a woman’s sexual lifetime, even after the onset of menopause, where, due to a decline in estrogen levels the microbiome transitions back to a phenotype similar to the pre-pubertal state, with a coinciding increase in pH and loss of Lactobacillus dominance. This puts postmenopausal women at increased risk of barrier disruption and STIs due to the combined effects of a less robust epithelial barrier, a lack of Lactobacillus-mediated protection at the FGT, and increased pH levels (Brotman et al., 2018; Gliniewicz et al., 2019; Murphy et al., 2019).
It is not always possible to distinguish host vs microbial metabolites, and, indeed, both can be responsible for the production of the same molecule. Lactic acid, for example, is produced by both vaginal epithelial cells and by Lactobacillus species as a result of glycogen metabolism and is thought to be one of the main drivers behind the reduction in vaginal pH seen with increased Lactobacillus dominance (Amabebe and Anumba, 2018; Ratter et al., 2018; Song et al., 2020). There is evidence that in heightened states of inflammation, the metabolic profile of the FGT changes to one consistent with greater cellular leakage and cellular breakdown. In addition, variation in the metabolic profile of the FGT between the subsets of women with or without BV has been observed (Srinivasan et al., 2015; Parolin et al., 2018; Ceccarani et al., 2019). Lower levels of lactate, amino acids, and dipeptide, and higher amounts of bioactive metabolites such as signaling eicosanoid 12-hydroxyeicosatetraenoic acid (12-HETE), were found in the vaginal mucosa of women with BV (Srinivasan et al., 2015; Parolin et al., 2018). Lower amounts of sialic acid and higher levels of mannose epitopes were also found in the CVL of women with BV as compared to those without BV (Linlin et al., 2015). Here, decreased levels of vaginal sialic acid during BV may be explained by the increased activity of the sialidase enzyme produced by BV-associated bacteria (Lewis et al., 2013; Vagios and Mitchell, 2021). How changes in host metabolites impact microbial composition and host cell function, and how the microbiome, in turn, influences the metabolic expression of host cells remains to be characterized. It is still unclear to what degree host-derived metabolites originate from the blood, and enter the lower FGT via plasma transudate, and to what degree they are produced locally by resident epithelial and immune cells.
Our knowledge regarding the microbiome and host factors in regulating cervicovaginal mucosal barrier properties lags far behind what is known at other mucosal sites, such as the gut and respiratory mucosa. Although higher diversity of the vaginal microbiome is associated with reduced FGT barrier properties, the mechanism behind the feature of Lactobacillus species in preventing growth and biofilm formation of pathogenic microbes at the FGT remains a particularly interesting point of research (Parolin et al., 2021). Similarly, the interaction of host cells, including immune cells and non-immune cells, with bacterial communities in different CST groups and BV-associated microbes needs to be investigated in depth. Recently, studies showed the difference in the metabolomic profile of the vaginal mucosa in healthy and BV states (Srinivasan et al., 2015; Parolin et al., 2018; Ceccarani et al., 2019). However, the effect of specific bacteria in regulating host metabolic pathways, and the role of those differentially abundant metabolites in regulating barrier properties and disease susceptibility, has not yet been explored. Furthermore, tryptophan levels were reported to be higher in women’s vaginal mucosa during dysbiosis, or BV, as compared to healthy women. The effect of gut bacteria-derived tryptophan metabolites, such as indole-3-ethanol, indole-3-pyruvate, and the receptor of these metabolites, aryl hydrocarbon receptor (AHR), in regulating gut epithelial and skin keratinocyte barrier function implores us to investigate their role in vaginal epithelial barrier function (Scott et al., 2020; Uberoi et al., 2021). Metatranscriptomic, metaproteomic, and metabolomic approaches are necessary to identify reproductively and metabolically active bacteria, secreted bioactive metabolites from those bacteria, and metabolic proteins or enzymes involved in generating those metabolites. Altogether this can be used to determine the role of those bacteria and bioactive metabolites in regulating the ecology of the FGT mucosal microenvironment and host barrier properties (Macklaim et al., 2013). Besides having gaps in which factors affect FGT barrier function, using both in vitro and ex vivo models to test microbiome effects on FGT barrier function presents its own challenges, especially with regards to representing the physiological condition of the FGT mucosa properly. For example, studying the composition of microbes, microaerophilic and low pH conditions of the mucosa, and the shedding nature of the outer epithelial layer are all insufficient with current models. In vivo models provide alternative approaches, but the translation of the findings from animal models to human application is challenging given the discrepancy in microbial compositions.
Mucosal barriers are critical gatekeepers in allowing nutrients and beneficial molecules to, and preventing pathogenic and infectious agents from, entering the body. The health of the FGT is guarded by its mucosal barriers and the integrity of FGT mucosal barriers is governed by the interplay between host immune system, hormones, and the FGT microbiota. However, our knowledge of how FGT homeostasis is maintained is still lacking. With this knowledge, an understanding of risk factors for STI acquisition, and a broader understanding of vaginal and uterine health, can be implemented in future research questions as well as care and policy decisions for sexual health, sexual quality of life, and reproductive interventions.
AP participated in the researching and reviewing of research articles, defining the hypothesis, writing and revising the manuscript, and designing Figure 1. ABS participated in the researching and reviewing of research articles, defining the hypothesis, writing and revising the manuscript, and designing Figure 2 and Table 1. RCS participated in the researching and reviewing of research articles, defining the hypothesis, writing, reviewing, and revising the manuscript, and reviewing the figures and table. AP and ABS contributed equally to this work and share first authorship. All authors contributed to the article and approved the submitted version.
This work is primarily funded by the National Microbiology Laboratories of Public Health Agency of Canada. AP, ABS and RCS are supported by Public Health Agency of Canada. RCS also received research funding from the Canadian Institute of Health Research (CIHR/NIH-154043).
The authors declare that the research was conducted in the absence of any commercial or financial relationships that could be construed as a potential conflict of interest.
All claims expressed in this article are solely those of the authors and do not necessarily represent those of their affiliated organizations, or those of the publisher, the editors and the reviewers. Any product that may be evaluated in this article, or claim that may be made by its manufacturer, is not guaranteed or endorsed by the publisher.
All figures were created with BioRender.com.
Abebe, E. C., Asmamaw, D. T., Ayele, T. M., Baye, N. D., Teshome, A. A., Muche, Z. T. (2021). The Role of Regulatory B Cells in Health and Diseases: A Systemic Review. J. Inflammation Res. 14, 75–84. doi: 10.2147/JIR.S286426
Agostinis, C., Mangogna, A., Bossi, F., Ricci, G., Kishore, U., Bulla, R. (2019). Uterine Immunity and Microbiota: A Shifting Paradigm. Front. Immunol. 10, 2387. doi: 10.3389/fimmu.2019.02387
Alcendor, D. J. (2016). Evaluation of Health Disparity in Bacterial Vaginosis and the Implications for HIV-1 Acquisition in African American Women. Am. J. Reprod. Immunol. 76 (2), 99–107. doi: 10.1111/aji.12497
Aldunate, M., Srbinovski, D., Hearps, A. C., Latham, C. F., Ramsland, P. A., Gugasyan, R., et al. (2015). Antimicrobial and Immune Modulatory Effects of Lactic Acid and Short Chain Fatty Acids Produced by Vaginal Microbiota Associated With Eubiosis and Bacterial Vaginosis. Front. Physiol. 6, 164. doi: 10.3389/FPHYS.2015.00164/BIBTEX
Ali, A., Syed, S. M., Jamaluddin, M. F. B., Colino-Sanguino, Y., Gallego-Ortega, D., Tanwar, P. S. (2020). Cell Lineage Tracing Identifies Hormone-Regulated and Wnt-Responsive Vaginal Epithelial Stem Cells. Cell Rep. 30 (5), 1463–1477.e7. doi: 10.1016/j.celrep.2020.01.003
Amabebe, E., Anumba, D. O. C. (2018). The Vaginal Microenvironment: The Physiologic Role of Lactobacilli. Front. Med. 5, 181. doi: 10.3389/FMED.2018.00181
Amy, L.J., Jane, C., Emilie, P.S., Charles, R.W., Paul M, G., Patricia, A.P. (2012). A Subset of Human Uterine Endometrial Macrophages is Alternatively Activated. Am. J. Reprod. Immunol. (New York N.Y.: 1989) 68 (5), 374–386. doi: 10.1111/J.1600-0897.2012.01181.X
Anne, S., Serban-Dan, C., Ana Claudia, Z. (2014). Endocrine Factors Modulating Immune Responses in Pregnancy. Front. Immunol. 5, 196. doi: 10.3389/FIMMU.2014.00196
Anton, L., Sierra, L.-J., DeVine, A., Barila, G., Heiser, L., Brown, A. G., et al. (2018). Common Cervicovaginal Microbial Supernatants Alter Cervical Epithelial Function: Mechanisms by Which Lactobacillus crispatus Contributes to Cervical Health. Front. Microbiol. 0, 2181. doi: 10.3389/FMICB.2018.02181
Ayehunie, S., Cannon, C., Lamore, S., Kubilus, J., Anderson, D. J., Pudney, J., et al. (2006). Organotypic Human Vaginal-Ectocervical Tissue Model for Irritation Studies of Spermicides, Microbicides, and Feminine-Care Products. Toxicol. Vitro 20, 689–698. doi: 10.1016/j.tiv.2005.10.002
Baker, J. M., Chase, D. M., Herbst-kralovetz, M. M. (2018). Uterine Microbiota: Residents, Tourists, or Invaders? Front. Immunol. 9, 208. doi: 10.3389/fimmu.2018.00208
Bautista, C. T., Wurapa, E. K., Sateren, W. B., Morris, S. M., Hollingsworth, B. P., Sanchez, J. L. (2017). Association of Bacterial Vaginosis With Chlamydia and Gonorrhea Among Women in the U.S. Army. Am. J. Prev. Med. 52 (5), 632–639. doi: 10.1016/j.amepre.2016.09.016
Birse, K. D., Romas, L. M., Guthrie, B. L., Nilsson, P., Bosire, R., Kiarie, J., et al. (2017). Genital Injury Signatures and Microbiome Alterations Associated With Depot Medroxyprogesterone Acetate Usage and Intravaginal Drying Practices. J. Infect. Dis. 215 (4), 590–598. doi: 10.1093/infdis/jiw590
Blacher, E., Levy, M., Tatirovsky, E., Elinav, E. (2017). Microbiome-Modulated Metabolites at the Interface of Host Immunity. J. Immunol. 198 (2), 572–580. doi: 10.4049/JIMMUNOL.1601247
Borgdorff, H., Gautam, R., Armstrong, S. D., Xia, D., Ndayisaba, G. F., Van Teijlingen, N. H., et al. (2016). Cervicovaginal Microbiome Dysbiosis Is Associated With Proteome Changes Related to Alterations of the Cervicovaginal Mucosal Barrier. Mucosal Immunol. 9 (3), 621–633. doi: 10.1038/mi.2015.86
Bradley, F., Birse, K., Hasselrot, K., Noël-Romas, L., Introini, A., Wefer, H., et al. (2018). The Vaginal Microbiome Amplifies Sex Hormone-Associated Cyclic Changes in Cervicovaginal Inflammation and Epithelial Barrier Disruption. Am. J. Reprod. Immunol. 80 (1), e12863. doi: 10.1111/AJI.12863
Brotman, R. M., Shardell, M. D., Gajer, P., Fadrosh, D., Chang, K., Silver, M. I., et al. (2018). Association Between the Vaginal Microbiota, Menopause Status, and Signs of Vulvovaginal Atrophy. Menopause 25 (11), 1321–1330. doi: 10.1097/gme.0b013e3182a4690b
Cadieux, P. A., Burton, J., Devillard, E., Reid, G. (2009). Lactobacillus by-Products Inhibit the Growth and Virulence of Uropathogenic Escherichia Coli. J. Physiol. Pharmacol.: Off. J. Polish Physiol. Soc. 60 (Suppl 6), 13–18.
Carias, A. M., Hope, T. J. (2018). Barriers of Mucosal Entry of HIV/SIV. Curr. Immunol. Rev. 15 (1), 4–13. doi: 10.2174/1573395514666180604084404
Ceccarani, C., Foschi, C., Parolin, C., D’Antuono, A., Gaspari, V., Consolandi, C., et al. (2019). Diversity of Vaginal Microbiome and Metabolome During Genital Infections. Sci. Rep. 9 (1), 1–12. doi: 10.1038/s41598-019-50410-x
Chen, C., Song, X., Wei, W., Zhong, H., Dai, J., Lan, Z., et al. (2017). The Microbiota Continuum Along the Female Reproductive Tract and its Relation to Uterine-Related Diseases. Nat. Commun. 8 (875). doi: 10.1038/s41467-017-00901-0
Chistiakov, D. A., Bobryshev, Y. V., Kozarov, E., Sobenin, I. A., Orekhov, A. N. (2014). Intestinal Mucosal Tolerance and Impact of Gut Microbiota to Mucosal Tolerance. Front. Microbiol. 5, 781. doi: 10.3389/FMICB.2014.00781
Chitcholtan, K., Asselin, E., Parent, S., Sykes, P. H., Evans, J. J. (2013). Differences in Growth Properties of Endometrial Cancer in Three Dimensional (3D) Culture and 2D Cell Monolayer. Exp. Cell Res. 319 (1), 75–87. doi: 10.1016/J.YEXCR.2012.09.012
Chung, H. S., Lee, H. S., Kim, M. E., Lee, J. S., Park, K. (2019). Identification and Localization of Epithelial Progenitor Cells in the Vagina. Int. J. Impotence Res. 31 (1), 46–49. doi: 10.1038/s41443-018-0079-6
Dabee, S., Barnabas, S. L., Lennard, K. S., Jaumdally, S. Z., Gamieldien, H., Balle, C., et al. (2019). Defining Characteristics of Genital Health in South African Adolescent Girls and Young Women at High Risk for HIV Infection. PloS One 14 (4), 1–20. doi: 10.1371/journal.pone.0213975
Delgado-diaz, D. J., Tyssen, D., Hayward, J. A., Gugasyan, R., Hearps, A. C., Tachedjian, G. (2020). Distinct Immune Responses Elicited From Cervicovaginal Epithelial Cells by Lactic Acid and Short Chain Fatty Acids Associated With Optimal and Non-Optimal Vaginal Microbiota. Front. Cell. Infect. Microbiol. 9, 446. doi: 10.3389/fcimb.2019.00446
De Seta, F., Campisciano, G., Zanotta, N., Ricci, G., Comar, M. (2019). The Vaginal Community State Types Microbiome-Immune Network as Key Factor for Bacterial Vaginosis and Aerobic Vaginitis. Front. Microbiol. 10, 2451. doi: 10.3389/fmicb.2019.02451
Dizzell, S., Nazli, A., Reid, G., Kaushic, C. (2019). Protective Effect of Probiotic Bacteria and Estrogen in Preventing HIV-1-Mediated Impairment of Epithelial Barrier Integrity in Female Genital Tract. Cells 8 (10), 1120. doi: 10.3390/CELLS8101120
Dodd, J. M., Crowther, C. A. (2009). The Role of Progesterone in Prevention of Preterm Birth. Int. J. Women’s Health 1 (1), 73–84. doi: 10.2147/ijwh.s4730
Doerflinger, S. Y., Throop, A. L., Herbst-Kralovetz, M. M. (2014). Bacteria in the Vaginal Microbiome Alter the Innate Immune Response and Barrier Properties of the Human Vaginal Epithelia in a Species-Specific Manner. J. Infect. Dis. 209 (12), 1989–1999. doi: 10.1093/infdis/jiu004
Dusio, G. F., Cardani, D., Zanobbio, L., Mantovani, M., Luchini, P., Battini, L., et al. (2011). Stimulation of TLRs by LMW-HA Induces Self-Defense Mechanisms in Vaginal Epithelium. Immunol. Cell Biol. 89 (5), 630–639. doi: 10.1038/ICB.2010.140
Fahrbach, K. M., Malykhina, O., Stieh, D. J., Hope, T. J. (2013). Differential Binding of IgG and IgA to Mucus of the Female Reproductive Tract. PloS One 8 (10), e76176. doi: 10.1371/journal.pone.0076176
Fan, S. R., Liu, X. P., Liao, Q. P. (2008). Human Defensins and Cytokines in Vaginal Lavage Fluid of Women With Bacterial Vaginosis. Int. J. Gynaecol. Obstet.: Off. Organ Int. Fed. Gynaecol. Obstet. 103 (1), 50–54. doi: 10.1016/J.IJGO.2008.05.020
Ferreira, V. H., Nazli, A., Mossman, K. L., Kaushic, C. (2013). Proinflammatory Cytokines and Chemokines - But Not Interferon-β - Produced in Response to HSV-2 in Primary Human Genital Epithelial Cells are Associated With Viral Replication and the Presence of the Virion Host Shutoff Protein. Am. J. Reprod. Immunol. 70 (3), 199–212. doi: 10.1111/AJI.12133
Fettweis, J. M., Paul Brooks, J., Serrano, M. G., Sheth, N. U., Girerd, P. H., Edwards, D. J., et al. (2014). Differences in Vaginal Microbiome in African American Women Versus Women of European Ancestry. Microbiol. (United Kingdom) 160, 2272–2282. doi: 10.1099/mic.0.081034-0
Fichorova, R. N., Rheinwald, J. G., Anderson, D. J. (1997). Generation of Papillomavirus-Immortalized Cell Lines From Normal Human Ectocervical, Endocervical, and Vaginal Epithelium That Maintain Expression of Tissue-Specific Differentiation Proteins. Biol. Reprod. 57 (4), 847–855. doi: 10.1095/biolreprod57.4.847
Fox, J. M., Wiggins, R. C., Moore, J. W. J., Brewer, C., Andrew, A. C., Martin, F. (2017). Methodology for Reliable and Reproducible Cryopreservation of Human Cervical Tissue. Cryobiology 77, 14–18. doi: 10.1016/J.CRYOBIOL.2017.06.004
Frew, L., Makieva, S., McKinlay, A. T. M., McHugh, B. J., Doust, A., Norman, J. E., et al. (2014). Human Cathelicidin Production by the Cervix. PloS One 9 (8), e103434. doi: 10.1371/JOURNAL.PONE.0103434
Gajer, P., Brotman, R. M., Bai, G., Sakamoto, J., Schütte, U. M. E., Zhong, X., et al. (2012). Temporal Dynamics of the Human Vaginal Microbiota. Sci. Trans. Med. 4 (132), 132ra52. doi: 10.1126/scitranslmed.3003605
Gali, Y., Arien, K. K., Praet, M., Van den Bergh, R., Temmerman, M., Delezay, O., et al. (2010). Development of an In Vitro Dual-Chamber Model of the Female Genital Tract as a Screening Tool for Epithelial Toxicity. J. Virol. Methods 165 (2), 186–197. doi: 10.1016/j.jviromet.2010.01.018
Gautam, R., Borgdorff, H., Jespers, V., Francis, S. C., Verhelst, R., Mwaura, M., et al. (2015). Correlates of the Molecular Vaginal Microbiota Composition of African Women. BMC Infect. Dis. 15 (1). doi: 10.1186/s12879-015-0831-1
Gliniewicz, K., Schneider, G. M., Ridenhour, B. J., Williams, C. J., Song, Y., Farage, M. A., et al. (2019). Comparison of the Vaginal Microbiomes of Premenopausal and Postmenopausal Women. Front. Microbiol. 10, 193. doi: 10.3389/fmicb.2019.00193
Goldfien, G. A., Barragan, F., Chen, J., Takeda, M., Irwin, J. C., Perry, J., et al. (2015). Progestin-Containing Contraceptives Alter Expression of Host Defense-Related Genes of the Endometrium and Cervix. Rep. Sci. 22, (7), 814–828. doi: 10.1177/1933719114565035
Gregory, T.S., Sabrina, R.K., Hua, Y.C., Tin, T.T., Mieoak, B., Robert, B., et al. (2011). Multiplex Immunoassay of Lower Genital Tract Mucosal Fluid From Women Attending an Urban STD Clinic Shows Broadly Increased IL1ß and Lactoferrin. PloS One 6 (5), e19560. doi: 10.1371/JOURNAL.PONE.0019560
Grivel, J.-C., Margolis, L. (2009). Use of Human Tissue Explants to Study Human Infectious Agents. Nat. Protoc. 4 (2), 256–269. doi: 10.1038/nprot.2008.245
Gunn, B., Schneider, J., Shansab, M., Rosemary, A., Fahrbach, K., Iv, A. S., et al. (2016). ). Enhanced Binding of Antibodies Generated During Chronic HIV Infection to Mucus Component MUC16. Mucosal Immunol. 9 (6), 1549–1558. doi: 10.1038/mi.2016.8.Enhanced
Gupta, S., Kakkar, V., Bhushan, I. (2019). Crosstalk Between Vaginal Microbiome and Female Health: A Review. Microb. Pathogene. 136 (August):103696. doi: 10.1016/j.micpath.2019.103696
Hearps, A. C., Tyssen, D., Srbinovski, D., Bayigga, L., Diaz, D., Aldunate, M., et al. (2017). Vaginal Lactic Acid Elicits an Anti-Inflammatory Response From Human Cervicovaginal Epithelial Cells and Inhibits Production of Pro-Inflammatory Mediators Associated With HIV Acquisition. Mucosal Immunol. 10 (6), 1480–1490. doi: 10.1038/mi.2017.27
Hedges, S. R., Barrientes, F., Desmond, R. A., Schwebke, J. R. (2006). Local and Systemic Cytokine Levels in Relation to Changes in Vaginal Flora. J. Infect. Dis. 193 (4), 556–562. doi: 10.1086/499824
Hjelm, B. E., Berta, A. N., Nickerson, C. A., Arntzen, C. J., Herbst-Kralovetz, M. M. (2010). Development and Characterization of a Three-Dimensional Organotypic Human Vaginal Epithelial Cell Model. Biol. Reprod. 82 (3), 617–627. doi: 10.1095/biolreprod.109.080408
Ilhan, Z. E., Łaniewski, P., Tonachio, A., Herbst-Kralovetz, M. M. (2020). Members of Prevotella Genus Distinctively Modulate Innate Immune and Barrier Functions in a Human Three-Dimensional Endometrial Epithelial Cell Model. J. Infect. Dis. 222 (12), 2082–2092. doi: 10.1093/INFDIS/JIAA324
Irvin, S. C., Herold, B. C. (2015). Molecular Mechanisms Linking High Dose Medroxyprogesterone With HIV-1 Risk. PloS One 10 (3), 1–15. doi: 10.1371/journal.pone.0121135
Jennifer, M.M., Swathi, B., Sundeep, G.K., Julie, C.H. (2021). An Avant-Garde Model of Injury-Induced Regenerative Vaginal Wound Healing. Adv. Wound Care 10 (4), 165–173. doi: 10.1089/WOUND.2020.1198
Jensen, M. A., Forest, M. G., Lai, Y. W. S. K., Mckinley, S. A. (2019). Antibody-Mediated Immobilization of Virions in Mucus. Bull. Math. Biol. 81 (10), 4069–4099. doi: 10.1007/s11538-019-00653-6
Kallak, T. K., Uvnäs-Moberg, K. (2017). Oxytocin Stimulates Cell Proliferation in Vaginal Cell Line Vk2E6E7. Post Reprod. Health 23 (1), 6–12. doi: 10.1177/2053369117693148
Kaushic, C., Nazli, A., Ferreira, V. H., Kafka, J. K. (2011). Primary Human Epithelial Cell Culture System for Studying Interactions Between Female Upper Genital Tract and Sexually Transmitted Viruses, HSV-2 and HIV-1. Methods 55 (2), 114–121. doi: 10.1016/J.YMETH.2011.09.022
Kleppa, E., Holmen, S. D., Lillebø, K., Kjetland, E. F., Gundersen, S. G., Taylor, M., et al. (2015). Cervical Ectopy: Associations With Sexually Transmitted Infections and HIV. A Cross-Sectional Study of High School Students in Rural South Africa. Sexually Transmitted Infect. 91 (2), 124–129. doi: 10.1136/sextrans-2014-051674
Koumans, E. H., Sternberg, M., Bruce, C., McQuillan, G., Kendrick, J., Sutton, M., et al. (2007). The Prevalence of Bacterial Vaginosis in the United State-2004; Associations With Symptoms, Sexual Behaviors, and Reproductive Health. Sexually Transmitted Dis. 34 (11), 864–869. doi: 10.1097/OLQ.0b013e318074e565
Kyongo, J. K., Crucitti, T., Menten, J., Hardy, L., Cools, P., Michiels, J., et al. (2015). Cross-Sectional Analysis of Selected Genital Tract Immunological Markers and Molecular Vaginal Microbiota in Sub-Saharan African Women, With Relevance to HIV Risk and Prevention. Clin. Vaccine Immunol. 22 (5), 526–538. doi: 10.1128/CVI.00762-14
Lacroix, G., Gouyer, V., Gottrand, F., Desseyn, J. L. (2020). The Cervicovaginal Mucus Barrier. Int. J. Mol. Sci. 21 (21), 1–23. doi: 10.3390/ijms21218266
Laniewski, P., Gomez, A., Hire, G., So, M., Herbst-Kralovetz, M. M. (2017). Human Three-Dimensional Endometrial Epithelial Cell Model to Study Host Interactions With Vaginal Bacteria and Neisseria Gonorrhoeae. Infect. Immun. 85 (3), e01049–16. doi: 10.1128/IAI.01049-16
Łaniewski, P., Herbst-Kralovetz, M. M. (2019). Analysis of Host Responses to Neisseria Gonorrhoeae Using a Human Three-Dimensional Endometrial Epithelial Cell Model. Methods Mol. Biol. 1997, 347–361. doi: 10.1007/978-1-4939-9496-0_20
Łaniewski, P., Ilhan, Z. E., Herbst-Kralovetz, M. M. (2020). The Microbiome and Gynaecological Cancer Development, Prevention and Therapy. Nat. Rev. Urol. 17 (April), 19–22. doi: 10.1038/s41585-020-0286-z
Lee, Y., Dizzell, S. E., Leung, V., Nazli, A., Zahoor, M. A., Fichorova, R. N., et al. (2016). Effects of Female Sex Hormones on Susceptibility to HSV-2 in Vaginal Cells Grown in Air-Liquid Interface. Viruses 8:241. doi: 10.3390/v8090241
Lee, V., Tobin, J. M., Foley, E. (2006). Relationship of Cervical Ectopy to Chlamydia Infection in Young Women. J. Family Plann. Reprod. Health Care 32 (2), 104–105. doi: 10.1783/147118906776276440
Lewis, W. G., Robinson, L. S., Gilbert, N. M., Perry, J. C., Lewis, A. L. (2013). Degradation, Foraging, and Depletion of Mucus Sialoglycans by the Vagina-Adapted Actinobacterium Gardnerella Vaginalis. J. Biol. Chem. 288 (17), 12067–12079. doi: 10.1074/JBC.M113.453654
Liman, L., Ting, F., Weijie, Z., Yuan, L., Hong, L. (2021). miRNAs in Decidual NK Cells: Regulators Worthy of Attention During Pregnancy. Reprod. Biol. Endocrinol.: RB&E 19 (1), 150. doi: 10.1186/S12958-021-00812-2
Linhares, I. M., Summers, P. R., Larsen, B., Giraldo, P. C., Witkin, S. S. (2011). Contemporary Perspectives on Vaginal pH and Lactobacilli. YMOB 204 (2), 120.e1–120.e5. doi: 10.1016/j.ajog.2010.07.010
Linlin, W., Sujeethra, K., Catherine, C., Bernard J, M., Sharon L, H., Lara K, M. (2015). Studying the Effects of Reproductive Hormones and Bacterial Vaginosis on the Glycome of Lavage Samples From the Cervicovaginal Cavity. PloS One 10 (5), e0127021. doi: 10.1371/JOURNAL.PONE.0127021
Lõhmussaar, K., Oka, R., Espejo Valle-Inclan, J., Smits, M. H. H., Wardak, H., Korving, J., et al. (2021). Patient-Derived Organoids Model Cervical Tissue Dynamics and Viral Oncogenesis in Cervical Cancer. Cell Stem Cell 28 (8), 1380–1396.e6. doi: 10.1016/J.STEM.2021.03.012
Loudon, J. D. O., Grant, G., Vessey, M. P. (1978). Epidemiology and Clinical Significance of Cervical Erosion in Women Attending a Family Planning Clinic. Br. Med. J. 1 (6115), 748–750. doi: 10.1136/bmj.1.6115.748
Macklaim, J. M., Fernandes, A. D., Di Bella, J. M., Hammond, J.-A., Reid, G., Gloor, G. B. (2013) Comparative Meta-RNA-Seq of the Vaginal Microbiota and Differential Expression by Lactobacillus Iners in Health and Dysbiosis. Available at: http://www.microbiomejournal.com/content/1/1/12.
Ma, B., Forney, L. J., Ravel, J. (2012). Vaginal Microbiome: Rethinking Health and Disease. Annu. Rev. Microbiol. 66, 371–389. doi: 10.1146/annurev-micro-092611-150157
Ma, Z. S., Li, L. (2017). Quantifying the Human Vaginal Community State Types (CSTs) With the Species Specificity Index. PeerJ 2017 (6), e3366. doi: 10.7717/peerj.3366
Maud, D., Andrew, D. L. (2013). BLT Humanized Mice as Model to Study HIV Vaginal Transmission. J. Infect. Dis. 208 Suppl (Suppl 2), S131–S136. doi: 10.1093/INFDIS/JIT318
Mei, C., Yang, W., Wei, X., Wu, K., Huang, D. (2019). The Unique Microbiome and Innate Immunity During Pregnancy. Front. Immunol. 10:2886 (December). doi: 10.3389/fimmu.2019.02886
Mendes-Soares, H., Suzuki, H., Hickey, R. J., Forney, L. J. (2014). Comparative Functional Genomics of Lactobacillus spp. Reveals Possible Mechanisms for Specialization of Vaginal Lactobacilli to Their Environment. J. Bacteriol. 196 (7), 1458–1470. doi: 10.1128/JB.01439-13
Mich`, M., Delley, M., Bruttin, A., Richard, M., Affolter, M., Rezzonico, E., et al. (2015). In Vitro Activity of Commercial Probiotic Lactobacillus Strains Against Uropathogenic Escherichia Coli. FEMS Microbiol. Lett. 362, 96. doi: 10.1093/femsle/fnv096
Mirmonsef, P., Gilbert, D., Zariffard, M. R., Hamaker, B. R., Kaur, A., Landay, A. L., et al. (2011). The Effects of Commensal Bacteria on Innate Immune Responses in the Female Genital Tract. Am. J. Reprod. Immunol. 65 (3), 190–195. doi: 10.1111/j.1600-0897.2010.00943.x
Mitchell, C. M., McLemore, L., Westerberg, K., Astronomo, R., Smythe, K., Gardella, C., et al. (2014). Long-Term Effect of Depot Medroxyprogesterone Acetate on Vaginal Microbiota, Epithelial Thickness and HIV Target Cells. J. Infect. Dis. 210 (4), 651–655. doi: 10.1093/INFDIS/JIU176
Monin, L., Ushakov, D. S., Arnesen, H., Bah, N., Jandke, A., Muñoz-Ruiz, M., et al. (2020). γδ T Cells Compose a Developmentally Regulated Intrauterine Population and Protect Against Vaginal Candidiasis. Mucosal Immunol. 13 (6), 969–981. doi: 10.1038/s41385-020-0305-7
Murphy, K., Keller, M. J., Anastos, K., Sinclair, S., Cooper Devlin, J., Shi, Q., et al. (2019). Impact of Reproductive Aging on the Vaginal Microbiome and Soluble Immune Mediators in Women Living With and at-Risk for HIV Infection. PloS One 14 (4), 1–19. doi: 10.1371/journal.pone.0216049
Nirk, E. Q. C., Rodolfo, D. V. M., Melissa, E. G., Jesse, J.K., Janelle, M. G., Thomas, L. C. (2018). Exogenous Oestrogen Inhibits Genital Transmission of Cell-Associated HIV-1 in DMPA-Treated Humanized Mice. J. Int. AIDS Soc. 21 (1), e25063. doi: 10.1002/JIA2.25063
Nunn, K. L., Wang, Y.-Y., Harit, D., Humphrys, M. S., Ma, B., Cone, R., et al. (2015). Enhanced Trapping of HIV-1 by Human Cervicovaginal Mucus Is Associated With Lactobacillus Crispatus-Dominant Microbiota. mBio 6 (5), e01084–15. doi: 10.1128/mBio.01084-15
O’Callaghan, J. L., Turner, R., Dekker Nitert, M., Barrett, H. L., Clifton, V., Pelzer, E. S. (2020). Re-Assessing Microbiomes in the Low-Biomass Reproductive Niche. BJOG: Int. J. Obstet. Gynaecol. 127 (2), 147–158. doi: 10.1111/1471-0528.15974
Ochiel, D., Fahey, J., Ghosh, M., Haddad, S., Wira, C. (2008). Innate Immunity in the Female Reproductive Tract: Role of Sex Hormones in Regulating Uterine Epithelial Cell Protection Against Pathogens. Curr. Women’s Health Rev. 4 (2), 102–117. doi: 10.2174/157340408784246395
Oh, J. E., Iijima, N., Song, E., Lu, P., Klein, J., Jiang, R., et al. (2019). Migrant Memory B Cells Secrete Luminal Antibody in the Vagina. Nature 571 (7763), 122–126. doi: 10.1038/s41586-019-1285-1
Parolin, C., Croatti, V., Laghi, L., Giordani, B., Tondi, M. R., De Gregorio, P. R., et al. (2021). Vaginal Lactobacillus Biofilms Influence Anti-Candida Activity. Front. Microbiol. 0, 750368. doi: 10.3389/FMICB.2021.750368
Parolin, C., Foschi, C., Laghi, L., Zhu, C., Banzola, N., Gaspari, V., et al. (2018). Insights Into Vaginal Bacterial Communities and Metabolic Profiles of Chlamydia Trachomatis Infection: Positioning Between Eubiosis and Dysbiosis. Front. Microbiol. 9, 600. doi: 10.3389/FMICB.2018.00600
Passmore, J. A. S., Jaspan, H. B., Masson, L. (2016). Genital Inflammation, Immune Activation and Risk of Sexual HIV Acquisition. Curr. Opin. HIV AIDS 11 (2), 156–162. doi: 10.1097/COH.0000000000000232
Paul, W.D., Florence, O., Francisco, M.-T., Wei, Z., John F, K., Elisa, F., et al. (2011). One Percent Tenofovir Applied Topically to Humanized BLT Mice and Used According to the CAPRISA 004 Experimental Design Demonstrates Partial Protection From Vaginal HIV Infection, Validating the BLT Model for Evaluation of New Microbicide Candidates. J. Virol. 85 (15), 7582–7593. doi: 10.1128/JVI.00537-11
Petrova, M. I., Lievens, E., Malik, S., Imholz, N., Lebeer, S. (2015). Lactobacillus Species as Biomarkers and Agents That can Promote Various Aspects of Vaginal Health. Front. Physiol. 6:81. doi: 10.3389/FPHYS.2015.00081
Phalguni, G., Deena, R., Bruce K, P., Kathy, K., Lisa C, R., Michael A, P., et al. (2006). Use of Frozen-Thawed Cervical Tissues in the Organ Culture System to Measure Anti-HIV Activities of Candidate Microbicides. AIDS Res. Hum. Retroviruses 22 (5), 419–424. doi: 10.1089/AID.2006.22.419
Radtke, A. L., Quayle, A. J., Herbst-Kralovetz, M. M. (2012). Microbial Products Alter the Expression of Membrane-Associated Mucin and Antimicrobial Peptides in a Three-Dimensional Human Endocervical Epithelial Cell Model 1. Biol. Reprod. 87 (6), 132–133. doi: 10.1095/biolreprod.112.103366
Ratter, J. M., Rooijackers, H. M. M., Hooiveld, G. J., Hijmans, A. G. M., de Galan, B. E., Tack, C. J., et al. (2018). In Vitro and In Vivo Effects of Lactate on Metabolism and Cytokine Production of Human Primary PBMCs and Monocytes. Front. Immunol. 0:2564. doi: 10.3389/FIMMU.2018.02564
Ravel, J., Gajer, P., Abdo, Z., Schneider, G. M., Koenig, S. S. K., McCulle, S. L., et al. (2011). Vaginal Microbiome of Reproductive-Age Women. Proc. Natl. Acad. Sci. 108 (Supplement_1), 4680–4687. doi: 10.1073/pnas.1002611107
Schellenberg, J. J., Links, M. G., Hill, J. E., Dumonceaux, T. J., Kimani, J., Jaoko, W., et al. (2011). Molecular Definition of Vaginal Microbiota in East African Commercial Sex Workers. Appl. Environ. Microbiol. 77 (12), 4066–4074. doi: 10.1128/AEM.02943-10
Scott, S. A., Fu, J., Chang, P. V. (2020). Microbial Tryptophan Metabolites Regulate Gut Barrier Function via the Aryl Hydrocarbon Receptor. Proc. Natl. Acad. Sci. 117 (32), 19376–19387. doi: 10.1073/PNAS.2000047117
Shariq, M. ,. U., Thomas, T. ,. M., Maud, D., Wan Hon, K., Radwa, R. ,. S., Mauro, D. P., et al. (2019). HIV-1 Balances the Fitness Costs and Benefits of Disrupting the Host Cell Actin Cytoskeleton Early After Mucosal Transmission. Cell Host Microbe 25 (1), 73–86.e5. doi: 10.1016/J.CHOM.2018.12.008
Shattock, R. J., Moore, J. P. (2003). Inhibiting Sexual Transmission of HIV-1 Infection. Nat. Rev. Microbiol. 1 (1), 25–34. doi: 10.1038/nrmicro729
Soares, C. P., Midlej, V., Oliveira, M. E. W., Benchimol, M., Costa, M. L., Mermelstein, C. (2012). 2D and 3D-Organized Cardiac Cells Shows Differences in Cellular Morphology, Adhesion Junctions, Presence of Myofibrils and Protein Expression. PloS One 7 (5), e38147. doi: 10.1371/JOURNAL.PONE.0038147
Song, S. D., Acharya, K. D., Zhu, J. E., Deveney, C. M., Walther-Antonio, M. R. S., Tetel, M. J., et al. (2020). Daily Vaginal Microbiota Fluctuations Associated With Natural Hormonal Cycle, Contraceptives, Diet, and Exercise. MSphere 5 (4), e00593–20. doi: 10.1128/MSPHERE.00593-20
Srinivasan, S., Morgan, M. T., Fiedler, T. L., Djukovic, D., Hoffman, N. G., Raftery, D., et al. (2015). Metabolic Signatures of Bacterial Vaginosis. mBio 6 (2), e00204–e00215. doi: 10.1128/mBio.00204-15
Steven, S. W., Helena, M.-S., Iara, M. L., Aswathi, J., William, J. L., Larry, J. F. (2013). Influence of Vaginal Bacteria and D- and L-Lactic Acid Isomers on Vaginal Extracellular Matrix Metalloproteinase Inducer: Implications for Protection Against Upper Genital Tract Infections. MBio 4 (4), e00460–13. doi: 10.1128/MBIO.00460-13
Swain, E. H. A., Ewald, P. W. (2018). Focus: Ecologyand Evolution: Natural Selection, The Microbiome, and Public Health. Yale J. OF Biol. Med. 91 (4), 445–455.
Tantengco, O. A. G., Richardson, L. S., Medina, P. M. B., Han, A., Menon, R. (2021). Organ-On-Chip of the Cervical Epithelial Layer: A Platform to Study Normal and Pathological Cellular Remodeling of the Cervix. FASEB J. 35 (4), e21463. doi: 10.1096/FJ.202002590RRR
Tjernlund, A., Carias, A. M., Andersson, S., Gustafsson-Sanchez, S., Röhl, M., Petersson, P., et al. (2015). Progesterone-Based Intrauterine Device Use is Associated With a Thinner Apical Layer of the Human Ectocervical Epithelium and a Lower ZO-1 mRNA Expression. Biol. Reprod. 92 (3), 1–10. doi: 10.1095/biolreprod.114.122887
Torky, H. A., Taha, A., Marie, H., El-Desouky, E., Raslan, O., Moussa, A. A., et al. (2018). Role of Topical Oxytocin in Improving Vaginal Atrophy in Postmenopausal Women: A Randomized, Controlled Trial. Climacteric 21 (2), 174–178. doi: 10.1080/13697137.2017.1421924
Trifonova, R. T., Bollman, B., Barteneva, N. S., Lieberman, J. (2018). Myeloid Cells in Intact Human Cervical Explants Capture HIV and Can Transmit It to CD4 T Cells. Front. Immunol. 0, 2719. doi: 10.3389/FIMMU.2018.02719
Uberoi, A., Bartow-McKenney, C., Zheng, Q., Flowers, L., Campbell, A., Knight, S. A. B., et al. (2021). Commensal Microbiota Regulates Skin Barrier Function and Repair via Signaling Through the Aryl Hydrocarbon Receptor. Cell Host Microbe 29 (8), 1235–1248.e8. doi: 10.1016/J.CHOM.2021.05.011
Vagios, S., Mitchell, C. M. (2021). Mutual Preservation: A Review of Interactions Between Cervicovaginal Mucus and Microbiota. Front. Cell. Infect. Microbiol. 11. doi: 10.3389/fcimb.2021.676114
Vallor, A. C., Antonio, M. A., Hawes, S. E., Hillier, S. L. (2001). Factors Associated With Acquisition of, or Persistent Colonization by, Vaginal Lactobacilli: Role of Hydrogen Peroxide Production. J. Infect. Dis. 184 (11), 1431–1436. doi: 10.1086/324445
Verstraelen, H., Verhelst, R., Claeys, G., De Backer, E., Temmerman, M., Vaneechoutte, M. (2009). Longitudinal Analysis of the Vaginal Microflora in Pregnancy Suggests That L. crispatus Promotes the Stability of the Normal Vaginal Microflora and That L. gasseri and/or L. iners are More Conducive to the Occurrence of Abnormal Vaginal Microflora. BMC Microbiol. 9. doi: 10.1186/1471-2180-9-116
Vitali, D., Wessels, J. M., Kaushic, C. (2017). Role of Sex Hormones and the Vaginal Microbiome in Susceptibility and Mucosal Immunity to HIV-1 in the Female Genital Tract. AIDS Res. Ther. 14 (1), 1–5. doi: 10.1186/s12981-017-0169-4
Wessels, J. M., Lajoie, J., Hay Cooper, M. I. J., Omollo, K., Felker, A. M., Vitali, D., et al. (2019). …Medroxyprogesterone Acetate Alters the Vaginal Microbiota and Microenvironment in Women and Increases Susceptibility to HIV-1 in Humanized Mice. DMM Dis. Models Mech. 12 (10), dmm039669. doi: 10.1242/dmm.039669
Wira, C. R., Fahey, J. V., Sentman, C. L., Pioli, P. A., Shen, L. (2005). Innate and Adaptive Immunity in Female Genital Tract: Cellular Responses and Interactions. Immunol. Rev. 206, 306–335. doi: 10.1111/J.0105-2896.2005.00287.X
Woods, M. W., Zahoor, M. A., Lam, J., Bagri, P., Dupont, H., Verschoor, C. P., et al. (2021). Transcriptional Response of Vaginal Epithelial Cells to Medroxyprogesterone Acetate Treatment Results in Decreased Barrier Integrity. J. Reprod. Immunol. 143, 103253. doi: 10.1016/J.JRI.2020.103253
Wright, K. O., Mohammed, A. S., Salisu-Olatunji, O., Kuyinu, Y. A. (2014). Cervical Ectropion and Intra-Uterine Contraceptive Device (IUCD): A Five-Year Retrospective Study of Family Planning Clients of a Tertiary Health Institution in Lagos Nigeria. BMC Res. Notes 7 (1), 1–6. doi: 10.1186/1756-0500-7-946
Yarbrough, V. L., Winkle, S., Herbst-Kralovetz, M. M. (2015). Antimicrobial Peptides in the Female Reproductive Tract: A Critical Component of the Mucosal Immune Barrier With Physiological and Clinical Implications. Hum. Reprod. Update 21 (3), 353–377. doi: 10.1093/humupd/dmu065
Yasmine, B., Oliver, J. H. (2017). Homeostatic Immunity and the Microbiota. Immunity 46 (4), 562–576. doi: 10.1016/J.IMMUNI.2017.04.008
Yeaman, G. R., Collins, J. E., Fanger, M. W., Wira, C. R. (2001). CD8+ T Cells in Human Uterine Endometrial Lymphoid Aggregates: Evidence for Accumulation of Cells by Trafficking. Immunology 102 (4), 434. doi: 10.1046/J.1365-2567.2001.01199.X
Zalenskaya, I. A., Chandra, N., Yousefieh, N., Fang, X., Adedipe, O. E., Jackson, S. S., et al. (2018). Use of Contraceptive Depot Medroxyprogesterone Acetate is Associated With Impaired Cervicovaginal Mucosal Integrity. J. Clin. Invest. 128 (10), 4622. doi: 10.1172/JCI120583
Zheng, D., Liwinski, T., Elinav, E. (2020). Interaction Between Microbiota and Immunity in Health and Disease. Cell Res. 30 (6), 492–506. doi: 10.1038/S41422-020-0332-7
Keywords: microbial factors, host factors, microbiome, barrier, vagina, female genital tract (FGT), tissue explant, sexually transmitted infection (STI)
Citation: Plesniarski A, Siddik AB and Su RC (2021) The Microbiome as a Key Regulator of Female Genital Tract Barrier Function. Front. Cell. Infect. Microbiol. 11:790627. doi: 10.3389/fcimb.2021.790627
Received: 07 October 2021; Accepted: 30 November 2021;
Published: 17 December 2021.
Edited by:
Mariya Ivanova Petrova, KU Leuven, BelgiumReviewed by:
Daniel Alford Powell, University of Arizona, United StatesCopyright © 2021 Plesniarski, Siddik and Su. This is an open-access article distributed under the terms of the Creative Commons Attribution License (CC BY). The use, distribution or reproduction in other forums is permitted, provided the original author(s) and the copyright owner(s) are credited and that the original publication in this journal is cited, in accordance with accepted academic practice. No use, distribution or reproduction is permitted which does not comply with these terms.
*Correspondence: Ruey-Chyi Su, cnVleS5jLnN1QHBoYWMtYXNwYy5nYy5jYQ==
†These authors have contributed equally to this work and share first authorship
Disclaimer: All claims expressed in this article are solely those of the authors and do not necessarily represent those of their affiliated organizations, or those of the publisher, the editors and the reviewers. Any product that may be evaluated in this article or claim that may be made by its manufacturer is not guaranteed or endorsed by the publisher.
Research integrity at Frontiers
Learn more about the work of our research integrity team to safeguard the quality of each article we publish.