- 1Burnett School of Biomedical Sciences, University of Central Florida, Orlando, FL, United States
- 2Department of Chemistry and Biochemistry, Brigham Young University, Provo, UT, United States
The A chains of ADP-ribosylating toxins exploit Hsp90 for translocation into the host cytosol. Here, we hypothesize that cis proline residues play a key role in toxin recognition by Hsp90. Our model is largely derived from studies on the unusual interplay between Hsp90 and the catalytic A1 subunit of cholera toxin (CTA1), including the recent identification of an RPPDEI-like binding motif for Hsp90 in CTA1 and several other bacterial toxins. Cis/trans proline isomerization is known to influence protein-protein interactions and protein structure/function, but it has not yet been proposed to affect Hsp90-toxin interactions. Our model thus provides a new framework to understand the molecular basis for Hsp90 chaperone function and Hsp90-driven toxin translocation.
Introduction
AB toxins contain an enzymatically active A subunit and a cell-binding B subunit (Zuverink and Barbieri, 2018). They are secreted into the external environment but attack targets within the cytosol of a host cell. To reach their cytosolic targets, AB toxins bind to the cell surface and are internalized by receptor-mediated endocytosis. Some toxins respond to the acidified lumen of the endosomes with a structural change in the B subunit that forms a channel in the endosome membrane. The A subunit then utilizes the toxin-generated pore to access the cytosol (Barth et al., 2004). Other AB toxins travel to the endoplasmic reticulum (ER) before toxin disassembly and A chain passage into the cytosol through a host “translocon” pore in the ER membrane (Teter, 2013).
Many AB toxins contain catalytic subunits that modify their cytosolic targets through ADP-ribosylation (Simon et al., 2014). The Barth lab has proposed that all of these ADP-ribosylating toxins (ADPRTs) require an interaction with the host chaperone Hsp90 for translocation to the cytosol (Barth, 2011; Ernst et al., 2017). There is considerable experimental evidence for this model, but the structural basis for Hsp90 interaction remains unknown. Here, we hypothesize that Hsp90 specifically recognizes the cis isomer of proline in the toxin A chain.
Proline Isomerization
Most Xaa-Xaa peptide bonds (with Xaa representing any amino acid) are in the trans state but can, under certain conditions, rotate 180° to the cis conformation. Proline is the most common amino acid for cis/trans isomerization because the cis and trans forms are almost isoenergetic, so neither form is strongly favored. Proline isomerization plays a critical role in protein folding and several cellular processes, as the cis and trans forms of a Xaa-Pro bond can each independently influence protein structure/function and protein-protein interactions (Andreotti, 2003; Lu et al., 2007; Joseph et al., 2012). For example, protein disulfide isomerase specifically recognizes the cis configuration of two Xaa-Pro bonds in RNase T1. Its interaction with RNase T1 is greatly enhanced by the trans-to-cis isomerization of these two bonds by cyclophilin A, a peptidyl prolyl cis-trans isomerase (PPI) (Schönbrunner and Schmid, 1992). We predict a similar interaction between Hsp90 and the cis conformation of proline in the A chains of ADPRTs.
Hsp90, A Molecular Chaperone
Hsp90 exhibits a greater level of substrate selectivity than other chaperones, yet it is not thought to recognize any specific sequence or structural motif. Instead, it usually partners with co-chaperones for substrate binding (Pearl and Prodromou, 2006; Li et al., 2012; Karagoz and Rudiger, 2015; Schopf et al., 2017). Processing often begins with the binding of another chaperone, Hsp40. This facilitates the sequential recruitment of Hsp70, Hsp70-Hsp90 organizing protein (Hop), and, finally, Hsp90. Other co-chaperones such as PPIs may further regulate the interaction of Hsp90 with its client protein. ATP hydrolysis by substrate-bound Hsp90 converts the chaperone to an open conformation, releases the processed client protein, and resets the chaperone cycle. This general process - including the involvement of co-chaperones - is frequently linked to the translocation and/or cytosolic refolding of toxin A chains.
Toxin Translocation From the Endosomes to the Cytosol
After internalization into the host cell, the A chains of endosome-translocating toxins respond to the acidified vesicle lumen by shifting to an unfolded or partially unfolded state. Some toxins then use a pH-driven ratchet mechanism for A chain delivery to the cytosol (Krantz et al., 2006). For other toxins, the A chain is extracted from the endosomes by chaperones and other proteins in the host cytosol. The subset of endosome-translocating ADPRTs exploits Hsp90 for this purpose (Haug et al., 2003; Ratts et al., 2003; Haug et al., 2004; Kaiser et al., 2011; Lang et al., 2014; Schuster et al., 2017). These toxins also require host PPI activity for A chain passage into the cytosol (Kaiser et al., 2009; Kaiser et al., 2011; Kaiser et al., 2012; Barth, 2013; Lang et al., 2014; Ernst et al., 2015; Schuster et al., 2017). Hsp90 and PPIs may interact with a common structural feature of ADPRTs, but a specific chaperone binding site has only been identified for Hsp90 recognition of ER-translocating ADPRTs (Kellner et al., 2019).
Toxin Translocation From the ER to the Cytosol
The A chains of ER-translocating toxins shift to a disordered state upon holotoxin disassembly (Teter, 2013). Unfolding results from an interaction with the anionic phospholipids of the ER membrane or the intrinsic instability of the free A chain (Day et al., 2002; Pande et al., 2006; Pande et al., 2007; Mayerhofer et al., 2009; Kim et al., 2011). In either case, A chain unfolding activates the ER-associated degradation (ERAD) mechanism for export to the cytosol. Refolding of the cytosolic toxin, along with a paucity of lysine residues required for ubiquitin conjugation, allows the A chain to evade the ubiquitin-dependent proteasomal degradation that usually accompanies ERAD-mediated export to the cytosol (Hazes and Read, 1997; Deeks et al., 2002; Rodighiero et al., 2002; Worthington and Carbonetti, 2007).
Cholera toxin (CT) and several other ER-translocating toxins require Hsp90 for productive intoxication. For CT, Hsp90 binds to an N-terminal RPPDEI sequence in the catalytic CTA1 subunit and then couples CTA1 refolding with CTA1 extraction from the ER: refolding of the toxin as it emerges at the cytosolic face of the ER membrane prevents the folded toxin from sliding back into the narrow diameter of the translocon pore, thus ensuring unidirectional movement out of the ER (Taylor et al., 2010; Burress et al., 2014; Kellner et al., 2019). Mutagenesis studies have demonstrated both RPPDEI prolines are required for CTA1 export to the cytosol (Kellner et al., 2019). Continued association of Hsp90 with the cytosolic pool of CTA1 maintains the toxin in a folded, active conformation for the ADP-ribosylation of its G protein target (Banerjee et al., 2014; Burress et al., 2014).
Other ER-translocating ADPRTs also contain an N- or C-terminal RPPDEI-like motif in the toxin A chain that is recognized by Hsp90 (Kellner et al., 2019). Several of these toxins require Hsp90 for productive intoxication, whereas the A chains from ER-translocating toxins that are not ADPRTs do not contain a RPPDEI motif and do not utilize Hsp90 for export to the cytosol (Table 1). Endosome-translocating toxins also lack the RPPDEI motif. These observations suggest ER-translocating ADPRTs exploit a common Hsp90-dependent mechanism that is distinct from the Hsp90 pathway followed by endosome-translocating ADPRTs. Additional support for this possibility is derived from the role of PPIs in toxin translocation: this chaperone family is essential for intoxication with endosome-translocating ADPRTs (Barth, 2013) but is not necessary for CT activity against cultured cells (Burress et al., 2019). Like CT, Pseudomonas aeruginosa exotoxin A (ETA) can affect cells treated with the PPI inhibitor cyclosporin A (CsA). In fact, CsA actually sensitizes cells to both ETA (Figure 1) and ETA-based immunotoxins (Andersson et al., 2009). It should be noted that another ER-translocating ADPRT - pertussis toxin (PT) - does require a PPI for intoxication. CsA thus blocks the ER-to-cytosol translocation of the catalytic PTS1 subunit (Figure 2) (Ernst et al., 2018) and resulting intoxication of cultured cells (Ernst et al., 2018). However, CsA also inhibits the ER-localized PPI cyclophilin B (Bernasconi et al., 2010) and may therefore disrupt host interactions with PTS1 before its extraction to the cytosol. Preliminary experiments have documented the binding of cyclophilin B to PTS1, and the affinity between cyclophilin B and PTS1 is apparently higher than the established association between cyclophilin A and PTS1 (Figure 3). The functional role of cyclophilin B binding to PTS1 remains to be established.
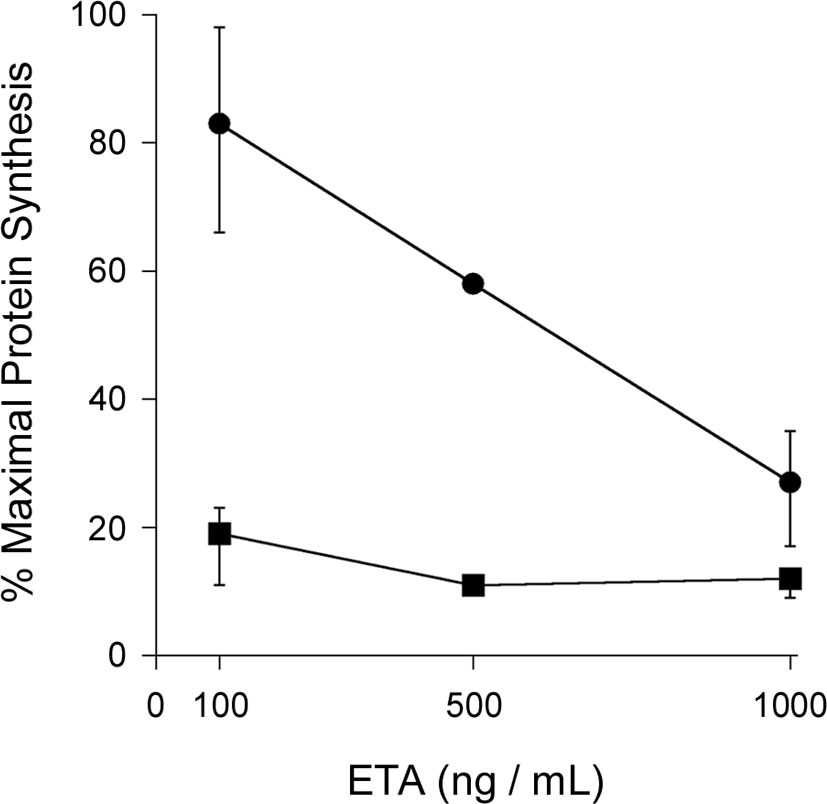
Figure 1 PPI inhibition does not protect cultured cells from ETA. Untreated CHO cells (circles) and CHO cells treated with 10 μM of the cyclophilin inhibitor CsA (squares) were incubated with various concentrations of ETA for 4 h before the extent of protein synthesis was recorded. Maximal protein synthesis was established from parallel samples of unintoxicated cells. Data are presented as the averages ± ranges of 2 (500 ng/mL) or 4 (100, 1000 ng/mL) independent experiments.
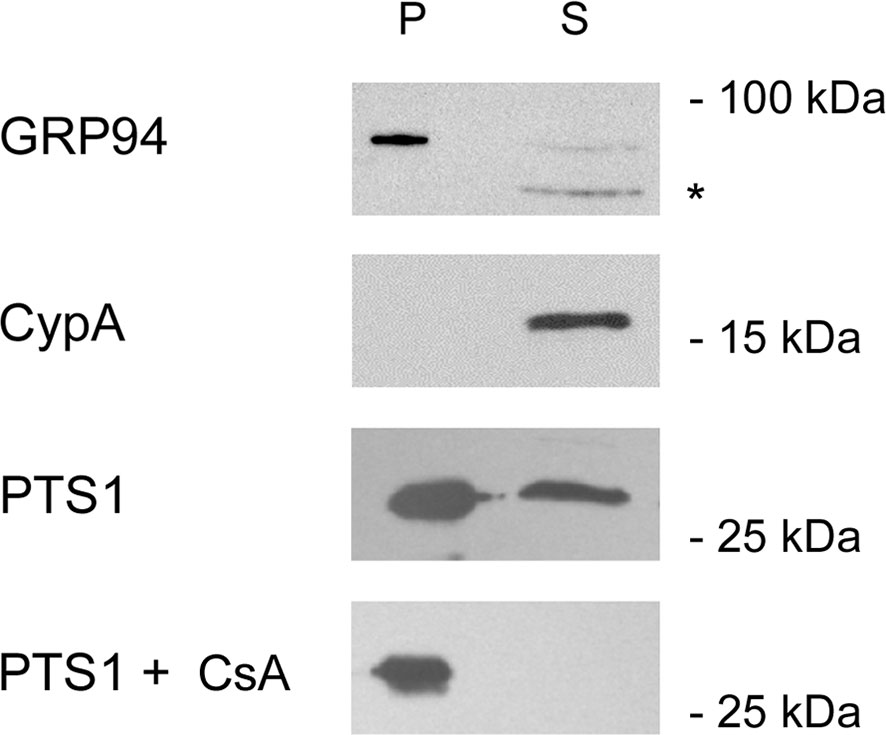
Figure 2 Cyclophilin inhibition blocks PTS1 translocation to the cytosol. CHO cells were mock transfected or transfected with an expression plasmid that directs PTS1 for co-translational insertion into the ER. The toxin is then dislocated from the ER into the cytosol. At 24 h post-transfection, permeabilization of the plasma membrane with digitonin was used in conjunction with differential centrifugation to separate cell extracts into two fractions: a pellet (P) containing intact organelles and a supernatant (S) containing the cytosol. Both fractions from mock-transfected cells were probed by Western blot with antibodies against the lumenal ER protein GRP94 or the cytosolic protein cyclophilin A (CypA). Fractions from PTS1-expressing cells incubated in the absence or presence of cyclophilin inhibitor CsA were probed by Western blot with an antibody against PTS1. The asterisk denotes a non-specific band, most likely due to the secondary antibody, also detected in the cropped region of the CypA and PTS1 blots. One of three representative results is shown.

Figure 3 Cyclophilin binding to PTS1. Two-fold serial dilutions of cyclophilin A (CypA) or cyclophilin B (CypB) were placed on nitrocellulose with a slot blot apparatus. Protein loading was confirmed by Ponceau stain (left panel). The membrane was then incubated overnight at 4°C in the presence of HRP-labeled PTS1. Chemiluminescence visualized the bound toxin (right panel). One of two representative experiments is shown. The previously established association between PTS1 and cyclophilin A (Ernst et al., 2018; Ernst et al., 2021) was barely detected in this assay, possibly because our assay used an HRP-conjugated PTS1 subunit whereas previous dot blot assays used a signal amplification strategy with primary and HRP-labeled secondary antibodies to detect the bound PTS1. Our results with HRP-conjugated PTS1 suggest the association of PTS1 with cyclophilin B is stronger than the binding of PTS1 to cyclophilin A.
Unique Chaperone Interactions Drive the Translocation and Cytosolic Activity of CTA1
The interplay between Hsp90 and CTA1 is highly unusual. Hsp90 recognizes a defined RPPDEI sequence in CTA1 and binds directly to this motif without the need for co-chaperones. However, it only binds to unfolded CTA1 (Taylor et al., 2010) despite the surface accessibility of the RPPDEI motif in folded CTA1 (Figure 4). The Hsp90-driven refolding of a client protein often results in the release of Hsp90 (Pearl and Prodromou, 2006; Schopf et al., 2017), yet Hsp90 remains tightly associated with refolded CTA1 (Burress et al., 2014). These interactions are essential for the intoxication process (Taylor et al., 2010). As detailed below, we propose proline isomerization in the RPPDEI motif is responsible for these atypical chaperone-substrate interactions.
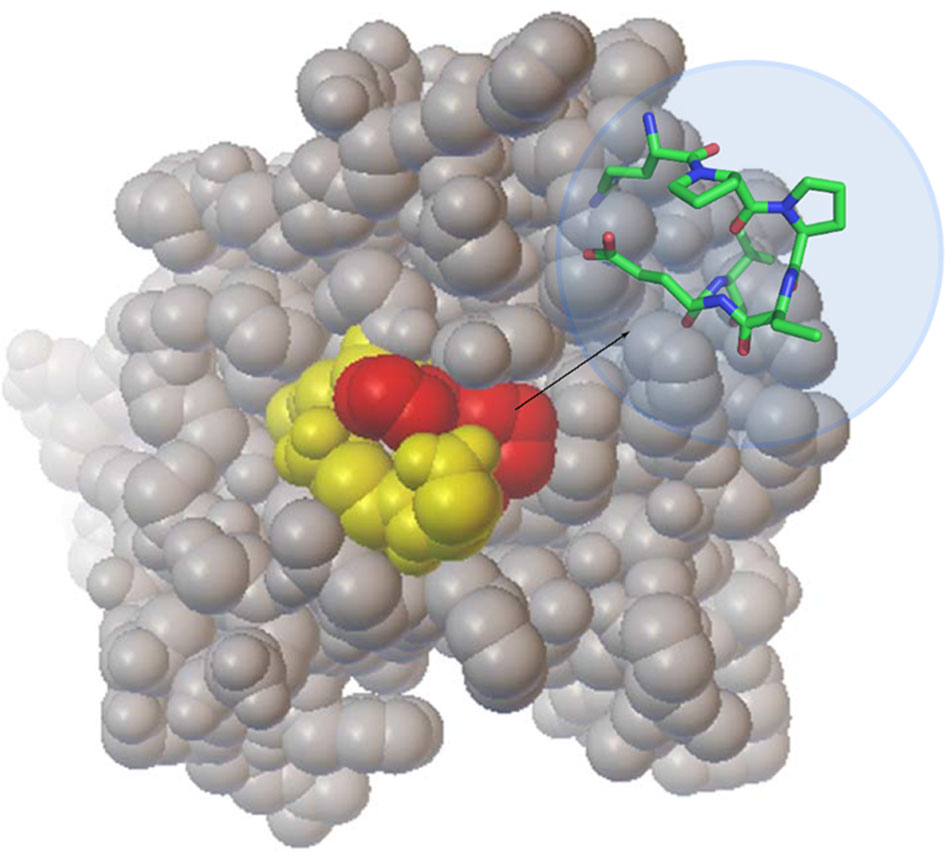
Figure 4 Location of the RPPDEI motif in CTA1. A space-filling model of folded, holotoxin-associated CTA1 (PDB entry 1S5E) highlights the PP residues in red and the RDEI residues in yellow. The inset stick structure provides a more detailed view of the trans conformations present at the PP residues of ordered CTA1.
Hypothesis
Proline Isomerization Plays a Key Role in Toxin Recognition by Hsp90
We predict the order-disorder-order transitions of CTA1 affect proline isomerization in its RPPDEI motif. We further predict that Hsp90 only recognizes cis prolines in the RPPDEI motif. When associated with the holotoxin (PDB entries 1S5E and 1XTC) (Zhang et al., 1995; O’Neal et al., 2004), CTA1 is in an ordered conformation with trans prolines in the RPPDEI motif (Figure 4, also see Materials and Methods). Thus, Hsp90 does not bind to the folded conformation of CTA1 that contains surface-exposed trans prolines in the RPPDEI sequence. Proline isomerization often accompanies protein unfolding (Wedemeyer et al., 2002), with Pro-Pro bonds exhibiting a greater tendency to the cis conformation than other Xaa-Pro bonds (Stewart et al., 1990; Pal and Chakrabarti, 1999). We accordingly predict that the ER-localized unfolding of dissociated CTA1 relaxes the structural constraints on its RPPDEI prolines and allows isomerization to the cis state. Hsp90 can then bind to the RPPDEI cis proline(s) as linearized CTA1 emerges from the ER translocon pore, thereby initiating the extraction process. If CTA1 retains its RPPDEI cis proline(s) after chaperone-induced refolding, this would result in the long-term association of Hsp90 with cytosolic CTA1 (Figure 5). Although our model is derived from studies with CTA1, it likely applies to other ER-translocating ADPRTs with an RPPDEI motif.
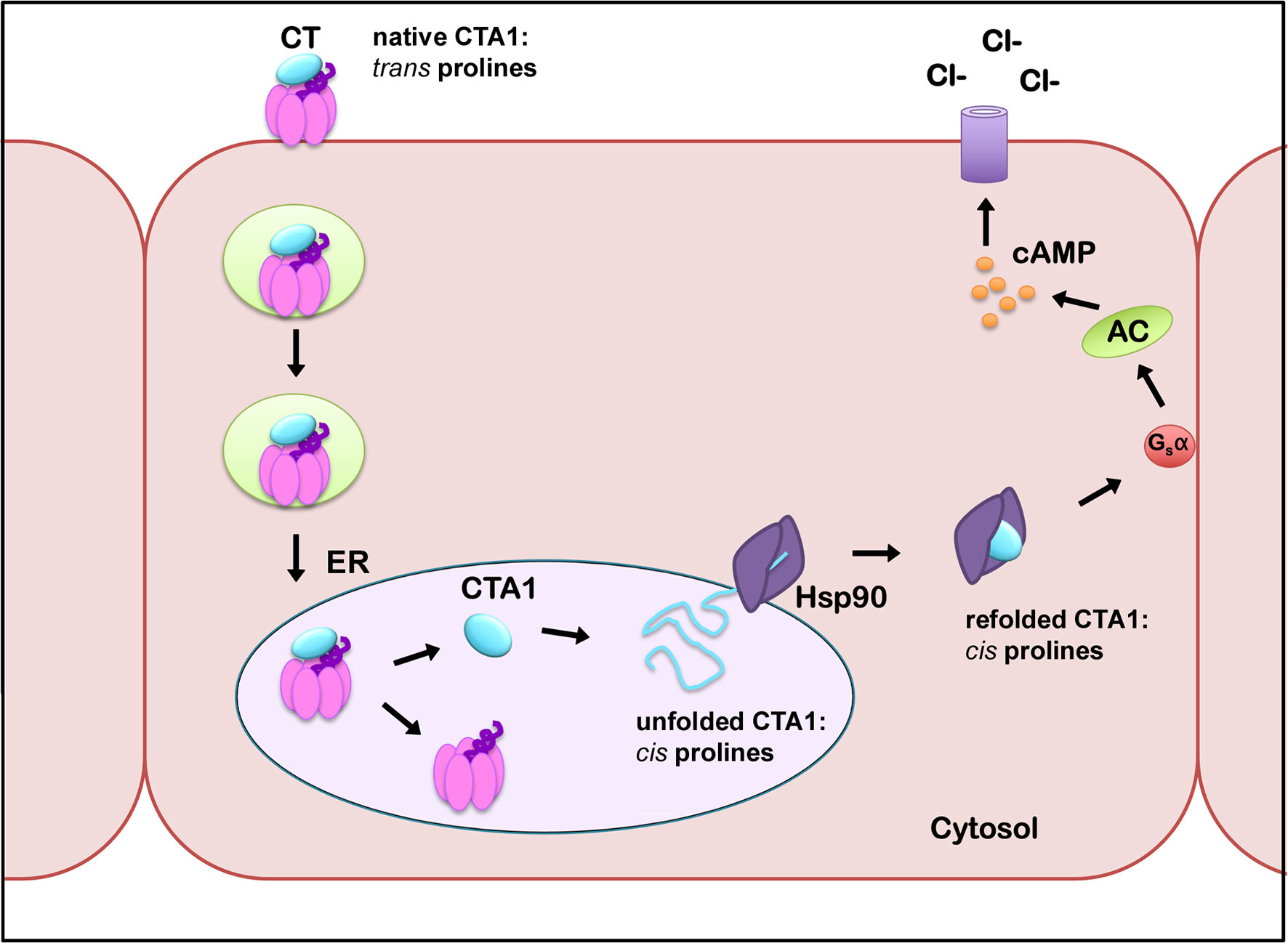
Figure 5 A model for the impact of proline cis/trans isomerization on CTA1-Hsp90 interactions. CTA1 enters the ER as a folded component of intact CT, with trans prolines in the RPPDEI motif. Separation of CTA1 from the rest of the toxin leads to its spontaneous unfolding and a hypothesized shift in its RPPDEI proline residues to the cis conformation. Those cis prolines are recognized by Hsp90 at the cytosolic face of the ER membrane after ERAD initiates the process of toxin translocation. Hsp90 then couples CTA1 refolding with CTA1 extraction from the ER translocon pore, but we predict refolding does not convert the RPPDEI prolines back to the trans state. Hsp90 thus remains in a stable complex with refolded CTA1 and its cis proline residues. Continued association of Hsp90 with CTA1 allows the otherwise unstable toxin to maintain its enzymatic activity in the host cytosol. AC, adenylate cyclase.
Proline isomerization provides a possible molecular basis for the atypical interactions between Hsp90 and CTA1. It also explains why, in contrast to endosome-translocating ADPRTs, CT does not require the action of PPIs (Burress et al., 2019): one or both of the prolines in the RPPDEI motif of disordered CTA1 is already in the proper cis conformation for Hsp90 recognition, whereas endosome-translocating ADPRTs rely upon PPIs to place a proline in the cis form required for Hsp90 binding. The presence of PPIs in the Hsp90 chaperone machinery (Tai et al., 1992; Zuehlke and Johnson, 2010; Cano et al., 2013; Li and Buchner, 2013) may likewise place the prolines of endogenous client proteins in the proper cis configuration for processing by Hsp90. PPI activity is currently thought to directly promote the folding of endogenous proteins and toxin A chains by shortening the rate-limiting step of proline isomerization during protein renaturation (Ernst et al., 2017), but our model suggests an additional role for PPIs in A chain translocation from the endosomes to the cytosol and, possibly, Hsp90-directed protein refolding in general. It should be noted that Hsp90 can bind directly to the A chains from several endosome-translocating ADPRTs, although this interaction has been demonstrated using an in vitro dot blot assay with toxins that may have contained a minor pool of protein with spontaneous conversion of prolines to the cis state (Dmochewitz et al., 2011; Kaiser et al., 2011; Schuster et al., 2017).
Discussion
Proline isomerization has an established role in regulating protein function but has not yet been shown to influence substrate recognition by Hsp90. In fact, Hsp90 is not thought to recognize defined amino acid sequences (Li et al., 2012; Karagoz and Rudiger, 2015; Schopf et al., 2017). Our work with the RPPDEI binding motif for Hsp90 has demonstrated, for the first time, that Hsp90 recognizes a defined amino acid sequence in addition to the general motif of surface-exposed hydrophobic amino acid residues (Kellner et al., 2019). We posit trans-to-cis isomerization in one or both of the RPPDEI prolines accompanies the ER-localized unfolding of the toxin A chain and serves as the signal for Hsp90 binding. Endosome-translocating ADPRTs that lack the RPPDEI motif do not undergo spontaneous trans-to-cis proline isomerization during the translocation process and must therefore utilize host PPIs to prepare the toxin A chain for Hsp90 binding. Structural analysis of folded and unfolded variants of the toxin A chain by NMR (Alderson et al., 2018) or other techniques could directly test this model. The propensity for cis proline isomerization in the toxin A chain thus provides a new context to understand the general roles of Hsp90 and PPIs in AB toxin translocation.
Materials and Methods
ETA Intoxication Assay
CHO-K1 cells (American Type Culture Collection, Manassas, VA) seeded to 24-well plates were grown overnight to ~80% confluency. The cells were then exposed to 10 μM CsA (Sigma-Aldrich, St. Louis, MO) for 30 min before challenge with various concentrations of ETA in the continued presence of CsA. A second set of cells were challenged with ETA in the absence of CsA. After a 4 h incubation with toxin, all cells were washed with phosphate-buffered saline (PBS) and incubated in methionine-free medium for 30 min before supplementation of the medium with 10 μCi/mL of [35S]methionine (PerkinElmer, Waltham, MA) for another 30 min. The cells were subsequently bathed in ice-cold PBS containing 10% trichloroacetic acid for sequential 30 and 10 min incubations. Cell lysates generated with 0.2 N NaOH were placed in vials filled with scintillation fluid, and the acid-precipitated radiolabel that had been incorporated into newly synthesized proteins was detected with a Beckman Coulter (Brea, CA) LS6500 scintillation counter. Results from toxin-treated cells were expressed as percentages of the value obtained from a corresponding set of cells incubated without toxin. All measurements were performed in triplicate.
PTS1 Translocation Assay
As previously described (Kellner et al., 2019), CHO cells grown to ~70% confluency in 6-well plates were transfected with 1 μg of empty pcDNA3.1 vector or the ssPTS1/pCMV/myc/ER expression vector (Castro et al., 2001). One set of cells was incubated overnight with 10 μM CsA. At 24 h post-transfection, all cells were lifted using PBS with 0.5 mM EDTA. Three wells of cells were pooled for each condition and centrifuged at 5,200 x g for 2 min at 4°C. After a 10 min incubation at 4°C, the cell pellet was resuspended in HCN buffer (50 mM Hepes pH 7.5, 2 mM CaCl2, 150 mM NaCl, 10 mM N-ethylmaleimide, protease inhibitor cocktail) containing 0.04% digitonin. After an additional 10 min at 4°C, separate membrane and cytosolic fractions were generated by centrifugation at 14,000 x g for 10 min at 4°C. The supernatant was lyophilized before resuspension in 2x sample buffer, while the pellet was directly resuspended in 2x sample buffer. Both fractions were resolved by SDS-PAGE with 15% polyacrylamide gels, using five-fold more supernatant sample than pellet. Antibody dilutions for Western blot analysis were 1:30,000 for the rabbit antibody against PT (Abcam, Cambridge, UK), 1:20,000 for rabbit antibodies against GRP94 (Stressgen, Victoria, British Columbia) or cyclophilin A (Abcam), and 1:20,000 for the secondary horseradish peroxidase-conjugated goat anti-rabbit IgG antibody (Jackson ImmunoResearch, West Grove, PA). Incubations with the primary antibodies were overnight at 4°C, while incubations with the secondary antibody were 1 h at room temperature.
Cyclophilin Binding Assay
Two-fold serial dilutions of cyclophilin A or cyclophilin B (both from Sigma-Aldrich) at starting concentrations of 5 µg/mL were absorbed in 200 µL volumes to nitrocellulose using the BioRad (Hercules, CA) slot blot apparatus. After Ponceau stain, the membrane was blocked for 30 min at room temperature in a 4% milk solution of Tris-buffered saline with 0.05% Tween-20 (TBST). The membrane was then incubated overnight at 4°C in 15 mL of TBST/4% milk containing 5 µg of PTS1 (Aviva Systems Biology, San Diego, CA) labeled with horseradish peroxidase via the Lightning Link conjugation kit (Abcam). Chemiluminescence was used to visualize membrane-bound PTS1.
Determination of Proline Isomerization State in Holotoxin-Associated CTA1
Proline conformation in the RPPDEI sequence of holotoxin-associated CTA1 can be assessed by extracting coordinates of only the atoms from the six-amino acid moiety. This subset of atoms is then saved as a text file having a .xyz extension and opened in a molecular viewer (e.g., Jmol). The measurement tool provides a direct method for measuring the relevant torsion angle. In this case, however, a visual assessment is usually sufficient as the cis and trans proline structures differ significantly.
Data Availability Statement
The raw data supporting the conclusions of this article will be made available by the authors, without undue reservation.
Author Contributions
AK, PC, and KT performed experiments. AK, JH, and KT conceptualized the hypothesis. AK, PC, JH, and KT analyzed the data. KT wrote the paper. AK and JH edited the paper. All authors contributed to the article and approved the submitted version.
Funding
Research in the Teter lab on Hsp90-toxin interactions was funded by the National Institute of Allergy and Infectious Diseases of the National Institutes of Health under Award Number R01AI099493. The content is solely the responsibility of the authors and does not necessarily represent the official views of the National Institutes of Health.
Conflict of Interest
The authors declare that the research was conducted in the absence of any commercial or financial relationships that could be construed as a potential conflict of interest.
Publisher’s Note
All claims expressed in this article are solely those of the authors and do not necessarily represent those of their affiliated organizations, or those of the publisher, the editors and the reviewers. Any product that may be evaluated in this article, or claim that may be made by its manufacturer, is not guaranteed or endorsed by the publisher.
References
Alderson, T. R., Lee, J. H., Charlier, C., Ying, J., Bax, A. (2018). Propensity for Cis-Proline Formation in Unfolded Proteins. Chembiochem 19, 37–42. doi: 10.1002/cbic.201700548
Andersson, Y., Engebraaten, O., Fodstad, Ø. (2009). Synergistic Anti-Cancer Effects of Immunotoxin and Cyclosporin In Vitro and In Vivo. Br. J. Cancer 101, 1307–1131. doi: 10.1038/sj.bjc.6605312
Andreotti, A. H. (2003). Native State Proline Isomerization: An Intrinsic Molecular Switch. Biochemistry 42, 9515–9524. doi: 10.1021/bi0350710
Banerjee, T., Taylor, M., Jobling, M. G., Burress, H., Yang, Z., Serrano, A., et al. (2014). ADP-Ribosylation Factor 6 Acts as an Allosteric Activator for the Folded But Not Disordered Cholera Toxin A1 Polypeptide. Mol. Microbiol. 94, 898–912. doi: 10.1111/mmi.12807
Barth, H. (2011). Exploring the Role of Host Cell Chaperones/PPIases During Cellular Up-Take of Bacterial ADP-Ribosylating Toxins as Basis for Novel Pharmacological Strategies to Protect Mammalian Cells Against These Virulence Factors. Naunyn Schmiedebergs Arch. Pharmacol. 383, 237–245. doi: 10.1007/s00210-010-0581-y
Barth, H. (2013). “Role of Peptidyl-Prolyl Cis/Trans Isomerases in Cellular Uptake of Bacterial Protein Toxins,” in Moonlighting Cell Stress Proteins in Microbial Infections. Ed. Henderson, B. (New York: Springer), 251–265.
Barth, H., Aktories, K., Popoff, M. R., Stiles, B. G. (2004). Binary Bacterial Toxins: Biochemistry, Biology, and Applications of Common Clostridium and Bacillus Proteins. Microbiol. Mol. Biol. Rev. 68, 373–402. doi: 10.1128/MMBR.68.3.373-402.2004
Bernasconi, R., Soldà, T., Galli, C., Pertel, T., Luban, J., Molinari, M. (2010). Cyclosporine A-Sensitive, Cyclophilin B-Dependent Endoplasmic Reticulum-Associated Degradation. PloS One 5, e13008. doi: 10.1371/journal.pone.0013008
Burress, H., Kellner, A., Guyette, J., Tatulian, S. A., Teter, K. (2019). HSC70 and HSP90 Chaperones Perform Complementary Roles in Translocation of the Cholera Toxin A1 Subunit From the Endoplasmic Reticulum to the Cytosol. J. Biol. Chem. 294, 12122–12131. doi: 10.1074/jbc.RA119.008568
Burress, H., Taylor, M., Banerjee, T., Tatulian, S. A., Teter, K. (2014). Co- and Post-Translocation Roles for Hsp90 in Cholera Intoxication. J. Biol. Chem. 289, 33644–33654. doi: 10.1074/jbc.M114.609800
Cano, L. Q., Lavery, D. N., Bevan, C. L. (2013). Foldosome Regulation of Androgen Receptor Action in Prostate Cancer. Mol. Cell Endocrinol. 369, 52–62. doi: 10.1016/j.mce.2013.01.023
Castro, M. G., McNamara, U., Carbonetti, N. H. (2001). Expression, Activity and Cytotoxicity of Pertussis Toxin S1 Subunit in Transfected Mammalian Cells. Cell Microbiol. 3, 45–54. doi: 10.1046/j.1462-5822.2001.00092.x
Day, P. J., Pinheiro, T. J., Roberts, L. M., Lord, J. M. (2002). Binding of Ricin A-Chain to Negatively Charged Phospholipid Vesicles Leads to Protein Structural Changes and Destabilizes the Lipid Bilayer. Biochemistry 41, 2836–2843. doi: 10.1021/bi012012i
Deeks, E. D., Cook, J. P., Day, P. J., Smith, D. C., Roberts, L. M., Lord, J. M. (2002). The Low Lysine Content of Ricin A Chain Reduces the Risk of Proteolytic Degradation After Translocation From the Endoplasmic Reticulum to the Cytosol. Biochemistry 41, 3405–3413. doi: 10.1021/bi011580v
Dmochewitz, L., Lillich, M., Kaiser, E., Jennings, L. D., Lang, A. E., Buchner, J., et al. (2011). Role of CypA and Hsp90 in Membrane Translocation Mediated by Anthrax Protective Antigen. Cell Microbiol. 13, 359–373. doi: 10.1111/j.1462-5822.2010.01539.x
Ernst, K., Eberhardt, N., Mittler, A. K., Sonnabend, M., Anastasia, A., Freisinger, S., et al. (2018). Pharmacological Cyclophilin Inhibitors Prevent Intoxication of Mammalian Cells With Bordetella Pertussis Toxin. Toxins 10, E181. doi: 10.3390/toxins10050181
Ernst, K., Langer, S., Kaiser, E., Osseforth, C., Michaelis, J., Popoff, M. R., et al. (2015). Cyclophilin-Facilitated Membrane Translocation as Pharmacological Target to Prevent Intoxication of Mammalian Cells by Binary Clostridial Actin ADP-Ribosylated Toxins. J. Mol. Biol. 427, 1224–1238. doi: 10.1016/j.jmb.2014.07.013
Ernst, K., Mittler, A. K., Winkelmann, V., Kling, C., Eberhardt, N., Anastasia, A., et al. (2021). Pharmacological Targeting of Host Chaperones Protects From Pertussis Toxin In Vitro and In Vivo. Sci. Rep. 11, 5429. doi: 10.1038/s41598-021-84817-2
Ernst, K., Schnell, L., Barth, H. (2017). Host Cell Chaperones Hsp70/Hsp90 and Peptidyl-Prolyl Cis/Trans Isomerases are Required for the Membrane Translocation of Bacterial ADP-Ribosylating Toxins. Curr. Top. Microbiol. Immunol. 406, 163–198. doi: 10.1007/82_2016_14
Fowler, C. C., Chang, S. J., Gao, X., Geiger, T., Stack, G., Galán, J. E. (2017). Emerging Insights Into the Biology of Typhoid Toxin. Curr. Opin. Microbiol. 35, 70–77. doi: 10.1016/j.mib.2017.01.012
Haug, G., Aktories, K., Barth, H. (2004). The Host Cell Chaperone Hsp90 Is Necessary for Cytotoxic Action of the Binary Iota-Like Toxins. Infect. Immun. 72, 3066–3068. doi: 10.1128/IAI.72.5.3066-3068.2004
Haug, G., Leemhuis, J., Tiemann, D., Meyer, D. K., Aktories, K., Barth, H. (2003). The Host Cell Chaperone Hsp90 is Essential for Translocation of the Binary Clostridium Botulinum C2 Toxin Into the Cytosol. J. Biol. Chem. 278, 32266–32274. doi: 10.1074/jbc.M303980200
Hazes, B., Read, R. J. (1997). Accumulating Evidence Suggests That Several AB-Toxins Subvert the Endoplasmic Reticulum-Associated Protein Degradation Pathway to Enter Target Cells. Biochemistry 36, 11051–11054. doi: 10.1021/bi971383p
Joseph, A. P., Srinivasan, N., de Brevern, A. G. (2012). Cis-Trans Peptide Variations in Structurally Similar Proteins. Amino Acids 43, 1369–1381. doi: 10.1007/s00726-011-1211-9
Kaiser, E., Böhm, N., Ernst, K., Langer, S., Schwan, C., Aktories, K., et al. (2012). FK506-Binding Protein 51 Interacts With Clostridium Botulinum C2 Toxin and FK506 Inhibits Membrane Translocation of the Toxin in Mammalian Cells. Cell Microbiol. 14, 1193–1205. doi: 10.1111/j.1462-5822.2012.01788.x
Kaiser, E., Kroll, C., Ernst, K., Schwan, C., Popoff, M., Fischer, G., et al. (2011). Membrane Translocation of Binary Actin-ADP-Ribosylating Toxins From Clostridium Difficile and Clostridium Perfringens is Facilitated by Cyclophilin A and Hsp90. Infect. Immun. 79, 3913–3921. doi: 10.1128/IAI.05372-11
Kaiser, E., Pust, S., Kroll, C., Barth, H. (2009). Cyclophilin A Facilitates Translocation of the Clostridium Botulinum C2 Toxin Across Membranes of Acidified Endosomes Into the Cytosol of Mammalian Cells. Cell Microbiol. 11, 780–795. doi: 10.1111/j.1462-5822.2009.01291.x
Karagoz, G. E., Rudiger, S. G. D. (2015). Hsp90 Interaction With Clients. Trends Biochem. Sci. 40, 117–125. doi: 10.1016/j.tibs.2014.12.002
Kellner, A., Taylor, M., Banerjee, T., Britt, C. B. T., Teter, K. (2019). A Binding Motif for Hsp90 in the A Chains of ADP-Ribosylating Toxins That Move From the Endoplasmic Reticulum to the Cytosol. Cell Microbiol. 21, e13074. doi: 10.1111/cmi.13074
Kim, S. H., Ryu, S. H., Lee, S. H., Lee, Y. H., Lee, S. R., Huh, J. W., et al. (2011). Instability of Toxin A Subunit of AB(5) Toxins in the Bacterial Periplasm Caused by Deficiency of Their Cognate B Subunits. Biochim. Biophys. Acta 1808, 2359–2365. doi: 10.1016/j.bbamem.2011.06.016
Krantz, B. A., Finkelstein, A., Collier, R. J. (2006). Protein Translocation Through the Anthrax Toxin Transmembrane Pore is Driven by a Proton Gradient. J. Mol. Biol. 55, 968–979. doi: 10.1016/j.jmb.2005.11.030
Lang, A. E., Ernst, K., Lee, H., Papatheodorou, P., Schwan, C., Barth, H., et al. (2014). The Chaperone Hsp90 and PPIases of the Cyclophilin and FKBP Families Facilitate Membrane Translocation of Photorhabdus Luminescens ADP-Ribosyltransferases. Cell Microbiol. 16, 490–503. doi: 10.1111/cmi.12228
Li, J., Buchner, J. (2013). Structure, Function, and Regulation of the Hsp90 Machinery. BioMed. J. 36, 106–117. doi: 10.4103/2319-4170.113230
Li, J., Soroka, J., Buchner, J. (2012). The Hsp90 Chaperone Machinery: Conformational Dynamics and Regulation by Co-Chaperones. Biochim. Biophys. Acta 1823, 624–635. doi: 10.1016/j.bbamcr.2011.09.003
Lu, K. P., Finn, G., Lee, T. H., Nicholson, L. K. (2007). Prolyl Cis-Trans Isomerization as a Molecular Timer. Nat. Chem. Biol. 3, 619–629. doi: 10.1038/nchembio.2007.35
Mayerhofer, P. U., Cook, J. P., Wahlman, J., Pinheiro, T. T., Moore, K. A., Lord, J. M., et al. (2009). Ricin A Chain Insertion Into Endoplasmic Reticulum Membranes is Triggered by a Temperature Increase to 37 {Degrees}C. J. Biol. Chem. 284, 10232–10242. doi: 10.1074/jbc.M808387200
O’Neal, C. J., Amaya, E. I., Jobling, M. G., Holmes, R. K., Hol, W. G. (2004). Crystal Structures of an Intrinsically Active Cholera Toxin Mutant Yield Insight Into the Toxin Activation Mechanism. Biochemistry 43, 3772–3782. doi: 10.1021/bi0360152
Pal, D., Chakrabarti, P. (1999). Cis Peptide Bonds in Proteins: Residues Involved, Their Conformations, Interactions and Locations. J. Mol. Biol. 294, 271–288. doi: 10.1006/jmbi.1999.3217
Pande, A. H., Moe, D., Jamnadas, M., Tatulian, S. A., Teter, K. (2006). The Pertussis Toxin S1 Subunit is a Thermally Unstable Protein Susceptible to Degradation by the 20S Proteasome. Biochemistry 45, 13734 –113740. doi: 10.1021/bi061175+
Pande, A. H., Scaglione, P., Taylor, M., Nemec, K. N., Tuthill, S., Moe, D., et al. (2007). Conformational Instability of the Cholera Toxin A1 Polypeptide. J. Mol. Biol. 374, 1114–1128. doi: 10.1016/j.jmb.2007.10.025
Pearl, L. H., Prodromou, C. (2006). Structure and Mechanism of the Hsp90 Molecular Chaperone Machinery. Annu. Rev. Biochem. 75, 271–294. doi: 10.1146/annurev.biochem.75.103004.142738
Ramasamy, K., Balasubramanian, S., Manickam, K., Pandranki, L., Taylor, A. B., Hart, P. J., et al. (2018). Mycoplasma Pneumoniae Community-Acquired Respiratory Distress Syndrome Toxin Uses a Novel KELED Sequence for Retrograde Transport and Subsequent Cytotoxicity. mBio 9, e01663–e01617. doi: 10.1128/mBio.01663-17
Ratts, R., Zeng, H., Berg, E. A., Blue, C., McComb, M. E., Costello, C. E., et al. (2003). The Cytosolic Entry of Diphtheria Toxin Catalytic Domain Requires a Host Cell Cytosolic Translocation Factor Complex. J. Cell Biol. 160, 1139–1150. doi: 10.1083/jcb.200210028
Rodighiero, C., Tsai, B., Rapoport, T. A., Lencer, W. I. (2002). Role of Ubiquitination in Retro-Translocation of Cholera Toxin and Escape of Cytosolic Degradation. EMBO Rep. 3, 1222–1227. doi: 10.1093/embo-reports/kvf239
Schönbrunner, E. R., Schmid, F. X. (1992). Peptidyl-Prolyl Cis-Trans Isomerase Improves the Efficiency of Protein Disulfide Isomerase as a Catalyst of Protein Folding. Proc. Natl. Acad. Sci. U.S.A. 89, 4510–4513. doi: 10.1073/pnas.89.10.4510
Schopf, F. H., Biebl, M. M., Buchner, J. (2017). The HSP90 Chaperone Machinery. Nat. Rev. Mol. Cell Biol. 18, 345–360. doi: 10.1038/nrm.2017.20
Schuster, M., Schnell, L., Feigl, P., Birkhofer, C., Mohr, K., Roeder, M., et al. (2017). The Hsp90 Machinery Facilitates the Transport of Diphtheria Toxin Into Human Cells. Sci. Rep. 7, 613. doi: 10.1038/s41598-017-00780-x
Simon, N. C., Aktories, K., Barbieri, J. T. (2014). Novel Bacterial ADP-Ribosylating Toxins: Structure and Function. Nat. Rev. Microbiol. 12, 599–611. doi: 10.1038/nrmicro3310
Spooner, R. A., Hart, P. J., Cook, J. P., Pietroni, P., Rogon, C., Hohfeld, J., et al. (2008). Cytosolic Chaperones Influence the Fate of a Toxin Dislocated From the Endoplasmic Reticulum. Proc. Natl. Acad. Sci. U.S.A. 105, 17408–17413. doi: 10.1073/pnas.0809013105
Stewart, D. E., Sarkar, A., Wampler, J. E. (1990). Occurrence and Role of Cis Peptide Bonds in Protein Structures. J. Mol. Biol. 214, 253–260. doi: 10.1016/0022-2836(90)90159-J
Tai, P. K., Albers, M. W., Chang, H., Faber, L. E., Schreiber, S. L. (1992). Association of a 59-Kilodalton Immunophilin With the Glucocorticoid Receptor Complex. Science 256, 1315–1318. doi: 10.1126/science.1376003
Taylor, M., Britt, C. B. T., Fundora, J., Teter, K. (2015). “Modulation of Cholera Toxin Structure/Function by Hsp90,” in Proceedings from the Physical Biology of Proteins and Peptides Conference, 67–80 (New York: Springer).
Taylor, M., Navarro-Garcia, F., Huerta, J., Burress, H., Massey, S., Ireton, K., et al. (2010). Hsp90 is Required for Transfer of the Cholera Toxin A1 Subunit From the Endoplasmic Reticulum to the Cytosol. J. Biol. Chem. 285, 31261–31267. doi: 10.1074/jbc.M110.148981
Teter, K. (2013). Toxin Instability and its Role in Toxin Translocation From the Endoplasmic Reticulum to the Cytosol. Biomolecules 3, 997–1029. doi: 10.3390/biom3040997
Wedemeyer, W. J., Welker, E., Scheraga, H. A. (2002). Proline Cis-Trans Isomerization and Protein Folding. Biochemistry 41, 14637–14644. doi: 10.1021/bi020574b
Worthington, Z. E., Carbonetti, N. H. (2007). Evading the Proteasome: Absence of Lysine Residues Contributes to Pertussis Toxin Activity by Evasion of Proteasome Degradation. Infect. Immun. 75, 2946–2953. doi: 10.1128/IAI.02011-06
Zhang, R. G., Scott, D. L., Westbrook, M. L., Nance, S., Spangler, B. D., Shipley, G. G., et al. (1995). The Three-Dimensional Crystal Structure of Cholera Toxin. J. Mol. Biol. 251, 563–573. doi: 10.1006/jmbi.1995.0456
Zuehlke, A., Johnson, J. L. (2010). Hsp90 and Co-Chaperones Twist the Functions of Diverse Client Proteins. Biopolymers 93, 211–217. doi: 10.1002/bip.21292
Keywords: AB toxins, chaperone, cholera toxin, Hsp90, peptidyl prolyl cis-trans isomerase, toxin translocation
Citation: Kellner A, Cherubin P, Harper JK and Teter K (2021) Proline Isomerization as a Key Determinant for Hsp90-Toxin Interactions. Front. Cell. Infect. Microbiol. 11:771653. doi: 10.3389/fcimb.2021.771653
Received: 06 September 2021; Accepted: 05 October 2021;
Published: 22 October 2021.
Edited by:
Vincenzo Scarlato, University of Bologna, ItalyReviewed by:
Holger Barth, University of Ulm, GermanyFederica Chiappori, National Research Council (CNR), Italy
Copyright © 2021 Kellner, Cherubin, Harper and Teter. This is an open-access article distributed under the terms of the Creative Commons Attribution License (CC BY). The use, distribution or reproduction in other forums is permitted, provided the original author(s) and the copyright owner(s) are credited and that the original publication in this journal is cited, in accordance with accepted academic practice. No use, distribution or reproduction is permitted which does not comply with these terms.
*Correspondence: Ken Teter, a3RldGVyQG1haWwudWNmLmVkdQ==
†Present address: Patrick Cherubin, Department of Microbiology, Perelman School of Medicine, University of Pennsylvania, Philadelphia, PA, United States