- 1State Key Laboratory of Oral Diseases, National Clinical Research Center for Oral Diseases, West China Hospital of Stomatology, Sichuan University, Chengdu, China
- 2Department of Pediatric Dentistry, West China Hospital of Stomatology, Sichuan University, Chengdu, China
- 3Department of Cariology and Endodontics, West China Hospital of Stomatology, Sichuan University, Chengdu, China
- 4Department of Periodontology, West China Hospital of Stomatology, Sichuan University, Chengdu, China
Periodontitis is a polymicrobial infectious disease characterized by alveolar bone loss. Systemic diseases or local infections, such as diabetes, postmenopausal osteoporosis, obesity, and inflammatory bowel disease, promote the development and progression of periodontitis. Accumulating evidences have revealed the pivotal effects of gut microbiota on bone health via gut-alveolar-bone axis. Gut pathogens or metabolites may translocate to distant alveolar bone via circulation and regulate bone homeostasis. In addition, gut pathogens can induce aberrant gut immune responses and subsequent homing of immunocytes to distant organs, contributing to pathological bone loss. Gut microbial translocation also enhances systemic inflammation and induces trained myelopoiesis in the bone marrow, which potentially aggravates periodontitis. Furthermore, gut microbiota possibly affects bone health via regulating the production of hormone or hormone-like substances. In this review, we discussed the links between gut microbiota and periodontitis, with a particular focus on the underlying mechanisms of gut-bone axis by which systemic diseases or local infections contribute to the pathogenesis of periodontitis.
Introduction
Periodontitis is an irreversible chronic inflammatory disease that typically manifests as the destruction of tooth-supporting tissues, including gingiva, periodontal ligament, and alveolar bone. In severe form, persistent pathologic alveolar bone loss in periodontitis may result in tooth loss and subsequent damage of masticatory function, negatively affecting general health and quality of life. National Health and Nutrition Examination Surveys (NHANES) from 2009 to 2012 reported a high prevalence (up to 46%) of chronic periodontitis in US population aged 30 years and older, among which 8.9% adults suffer from severe periodontitis (Eke et al., 2015). A heavier burden of periodontitis was found in US adults aged 65 years old and above, with 64.1% for mild/moderate periodontitis (Eke et al., 2016). Korea NHANES in 2014 revealed that 41.1% of survey population aged from 40 to 79 years old suffered from periodontitis (Lee and Lee, 2019). Additionally, cross-sectional studies in Norway presented that nearly 50% of population had periodontitis, with 9.1% in severe form or 20.1% in periodontitis stage III/IV (Holde et al., 2017; Bongo et al., 2020). Higher prevalence of severe periodontitis was also found in adult population of North Italy (Aimetti et al., 2015). At present, periodontitis is one of the major public health concerns with a rapidly growing prevalence in middle-aged and elderly population.
Periodontitis is a multifactorial pathological condition mainly induced by oral microbiota. The interactions between host immune responses and periodontal pathogens, such as Porphyromonas gingivalis, Treponema denticola, and Tannerella forsythia, contribute to the pathogenesis of periodontitis (Frederick et al., 2011; Silva et al., 2015). In addition, general health status affects periodontitis. Systemic diseases or local infections of other tissues are critical contributors to periodontal pathology (Genco and Borgnakke, 2013; Reynolds, 2014). As an established risk factor, diabetes mellitus increases the prevalence and severity of periodontitis (Lalla and Papapanou, 2011; Genco and Borgnakke, 2020). Systemic review and meta-analysis showed increased clinical attachment loss in women with postmenopausal osteoporosis (PMO) or osteopenia (ON) compared to healthy women (Penoni et al., 2017). Epidemiological studies showed an increased prevalence of periodontitis and aggravated periodontal bone loss in patients with inflammatory bowel diseases (IBDs), including ulcerative colitis and Crohn’s disease (Papageorgiou et al., 2017; She et al., 2020). Obesity and hyperlipidemia as well as metabolic syndrome are also suggested as risk factors for periodontitis, which increase the risk to gain periodontitis, exacerbate periodontal bone destruction, and are potentially linked with the poor response to non-surgical periodontal therapy (Cavagni et al., 2013; Li et al., 2015; Cavagni et al., 2016; Suvan et al., 2018; Virto et al., 2018). Systemic or intestinal diseases increase the complexity of periodontitis etiology and therapy; however, the underlying mechanisms remain unclear.
Gut Microbiota and Bone Health
Gut microbiota is the collection of microorganisms residing in the host luminal stream or adhering to the gut mucosa (Zaiss et al., 2019). Regarded as a whole, trillions of gut microorganisms interact with the host via releasing various signals, and affect host development, physiology, and general health. Gut microbiota or specific microbial metabolites not only locally influence host inflammatory responses, nutrition intake, or gut barrier function, but also link with host immune system, glucose homeostasis, lipid metabolism, energy balance, non-alcoholic fatty liver disease, adiposity and related comorbidity, and other metabolic diseases (Kamada et al., 2013; Rosenbaum et al., 2015; Morrison and Preston, 2016; Rooks and Garrett, 2016; Li et al., 2017; Fei et al., 2020; Fan and Pedersen, 2021).
The Role of Gut Microbiota in Bone Turnover
Gut-bone axis refers to the communications between gut microbiota and skeletal system whereby gut microbiota affects bone health. Accumulating evidences have revealed that gut microbiota is a critical factor in bone turnover. The analyses via Mendelian randomization approach or polygenetic risk scoring suggest significant association between gut microbiota and human bone mineral density (BMD) in different sites, such as heel or pelvis, by utilizing gut microbiota statistic from genome-wide association study (GWAS) and BMD values from UK biobank cohort (Cheng et al., 2020; Ni et al., 2021). Gnotobiotic animal models directly prove gut microbiota as a key regulator of bone turnover. Germ-free (GF) adult mice exhibit increased both cortical and trabecular bone mass in femur and improved trabecular morphology than conventionally raised controls (Sjögren et al., 2012; Ohlsson et al., 2017). Short-term gut microbial colonization promotes bone turnover and reduces femoral bone mass in GF mice, while adult mice with long-term gut microbial colonization gain enhanced longitudinal and radial growth of femur (Yan et al., 2016). Interestingly, gut microbial colonization induces varied bone phenotypes in GF mice according to the donors of different ages or nutritional status (Blanton et al., 2016), possibly resulting from the variance of gut microbial composition. Emerging evidences have indicated the impact of specific gut microbe segmented filamentous bacteria (SFB) on bone growth and maturation (Hathaway-Schrader et al., 2020; Tyagi et al., 2021). Compared to GF mice, SFB-monoassociation caused reduced bone loss and inferior trabecular morphology in the tibia of mice (Hathaway-Schrader et al., 2020). Similarly, a complex gut microbiota colonization within SFB also induced an osteopenic tibial phenotype in mice compared to gut microbiota colonization devoid of SFB (Hathaway-Schrader et al., 2020). The negative effects of SFB on skeletal maturation have been also proved via cohabitation, fecal material transplantation, and maternal/offspring transmission models, further indicating gut microbiota as a non-genomic hereditary factor in shaping offspring bone phenotype (Tyagi et al., 2021). In addition, the disruption of gut microbial homeostasis due to antibiotic intervention alters bone mass and biomechanical properties, further suggesting the regulation of gut microbiota on physiological bone remodeling (Yan et al., 2016; Rios-Arce et al., 2020).
The Role of Gut Microbiota in Bone diseases
Osteoporosis/Osteopenia
Osteoporosis (OP) is a systemic skeletal disease characterized by decreased bone mass and microarchitecture destruction, with elevated risk of fracture (Glaser and Kaplan, 1997). PMO induced by estrogen deficiency is the most common clinical form, and OP can be also secondary to medication or other systemic diseases, such as hypercortisolism and hyper-parathyroidism (Glaser and Kaplan, 1997). Osteopenia (ON) is an abnormal state with low bone density but not as severe as osteoporosis (Karaguzel and Holick, 2010). Estrogen deficiency due to gonadotropin-releasing hormone agonists induced no significant bone loss in GF mice, suggesting gut microbiota as a key determinant in PMO development (Li J.Y et al., 2016). PMO or ON patients showed distinct alterations in gut microbial taxa and metabolites compared to healthy individuals, including elevated levels of phylum Gemmatimonadetes and Chloroflexi, as well as genera Blautia, Parabacteroides, and Ruminococcaceae (Wang et al., 2017; Das et al., 2019; He et al., 2020). In addition, the genus Bacteroides and family Rikenellaceae were linked with high risk of fracture and low BMD in Japanese postmenopausal women (Ozaki et al., 2021). Glucocorticoid (GC) caused bone loss along with altered gut microbiota in mice, which can be prevented by antibiotic or probiotic treatment (Schepper et al., 2020). The mice that received fecal material from GC-treated subjects also showed pathological bone loss, supporting the involvement of gut microbiota in GC-induced osteoporosis (Schepper et al., 2020). In addition, the gut taxa SFB-dependent gut-bone crosstalk is also involved in the hyperparathyroidism-induced bone loss (Yu et al., 2020).
Osteoarthritis
Osteoarthritis (OA) is the most common degenerative joint disease. The results in both Rotterdam Study and LifeLines-DEEP cohort showed an association between higher abundance of gut Streptococcus spp. and increased OA-related knee pain and joint inflammation (Boer et al., 2019). Another pilot study also demonstrated a distinct alteration in gut microbiota composition in knee OA patients compared to healthy individuals (Ramasamy et al., 2021). Additionally, animal models demonstrated the involvement of gut microbiota in OA development as well as the association between OA severity and certain gut genera, including Fusobacterium, Faecalibacterium, and Ruminococcacea (Huang et al., 2020).
The Involvement of Gut Microbiota in Periodontitis
The studies by Uchida and Irie reported that commensal gut microbiota-colonized mice showed increased alveolar bone loss and compromised trabecular morphology compared with GF mice (Irie et al., 2018; Uchida et al., 2018). Compared to the rats with normal diet, obese-insulin-resistant rats fed with high-fat diet presented with enhanced osteoclast-mediated bone resorption, decreased jawbone mineral density, as well as impaired jawbone microarchitecture, and the jawbone morphology could be improved by the administration of probiotics, prebiotics, or symbiotics supplements (Eaimworawuthikul et al., 2019; Eaimworawuthikul et al., 2020) (Table 1).
Periodontitis is a complex inflammatory condition in periodontium with multiple contributory factors. Although periodontal microbiota drives the onset of periodontitis, systemic diseases or local infections of other tissues, such as PMO, obesity, diabetes mellitus, and IBDs, can promote the progression of periodontal destruction. Gut microbiota potentially provides critical links between periodontitis and general health. The mice treated by P. gingivalis gavage alone or in combination with local ligation exhibited increased alveolar bone loss than those with simply ligation-induced periodontitis, and gut commensal microbe Akkermansia muciniphila or its pili-like protein Amuc_1100 protected the mice gavaged with P. gingivalis from alveolar bone loss in periodontitis (Palioto et al., 2019; Mulhall et al., 2020). The protective role of A. muciniphila on alveolar bone was also observed in obese murine model (Huck et al., 2020). Obese mice showed elevated alveolar bone loss in P. gingivalis–induced periodontitis than lean mice, and gut commensal microbe A. muciniphila supplement by oral gavage significantly reduced obese-related alveolar bone loss in periodontitis (Huck et al., 2020). Our previous studies reported that estrogen deficiency due to ovariectomy (OVX) led to decreased abundance of gut butyrate-producing bacteria as well as enhanced periodontal bone loss in periodontitis rats, and that berberine or probiotics supplements enriched specific gut butyrate-producing genera and prevented OVX-induced alveolar bone resorption in periodontitis, further suggesting that PMO can affect periodontitis via regulating gut microbiota (Jia et al., 2019; Jia et al., 2021) (Table 1).
Underlying Mechanisms of Gut-Alveolar Bone Axis
Bidirectional regulations between gut and oral cavity account for the complex pathologies and interaction of gut and oral diseases. The translocation of oral pathogens to gut via alimentary canal or hematogenous way contributes to gut physiology or pathology (Kitamoto et al., 2020a) . The patients suffering from gut diseases, including irritable bowel syndrome, IBD, and colorectal cancer, showed an enrichment of oral pathobionts, such as the family Enterobacteriaceae, Peptostreptococcaceae, Pasteurellaceae, and Veillonellaceae, as well as the genus Klebsiella, Porphyromonas, Fusobacterium, and Streptococcus (Geng et al., 2014; Gevers et al., 2014; Pittayanon et al., 2019; Kitamoto et al., 2020a). The mice with periodontitis exhibited ectopic gut colonization of oral pathobionts belonging to Enterobacteriaceae, which could induce TH1 immune responses and directly aggravate the gut inflammation in colitis (Atarashi et al., 2017; Kitamoto et al., 2020b). Additionally, periodontitis indirectly exacerbates gut inflammation via the gut homing of oral pathobiont-reactive Th17 cells (Kitamoto et al., 2020b). The crosstalk between oral microbiota and gut diseases in “oral-gut” axis explicitly indicates the effect of oral inflammation on gut health. However, the potential mechanisms whereby gut microbiota regulates oral health in “gut-oral” axis remain uncertain. Mounting evidences provide multiple mechanisms potentially involved in “gut-alveolar bone” axis, supporting the role of gut microbiota in periodontitis development.
Microbial Pathway
Ectopic gut colonization of oral pathogens mainly accounts for the effects of oral inflammation on gut health. Similarly, gut pathobionts and metabolites potentially translocate to distant organs in hematogenous way and directly affect general health. The translocation of gut microbes and metabolites across gut epithelium includes transcellular and paracellular ways. Enterocyte phagocytosis is responsible for transcellular translocation. Paracellular pathway depends on the permeability of gut epithelium, the barrier physically resisting exogenous pathogens and selectively allowing the passage of nutrients. Tight junction (TJ) underlies the paracellular pathway of gut epithelial barrier, which consists of TJ protein complexes including claudin, occludin, and zonula occludens (ZO) proteins. Multiple pathologies can impair gut epithelial barrier function via modulating the production and distribution of TJ proteins, eliciting the “leakage” of gut pathobionts or metabolites into systemic circulation and microbial expansion to distant organs (Figure 1).
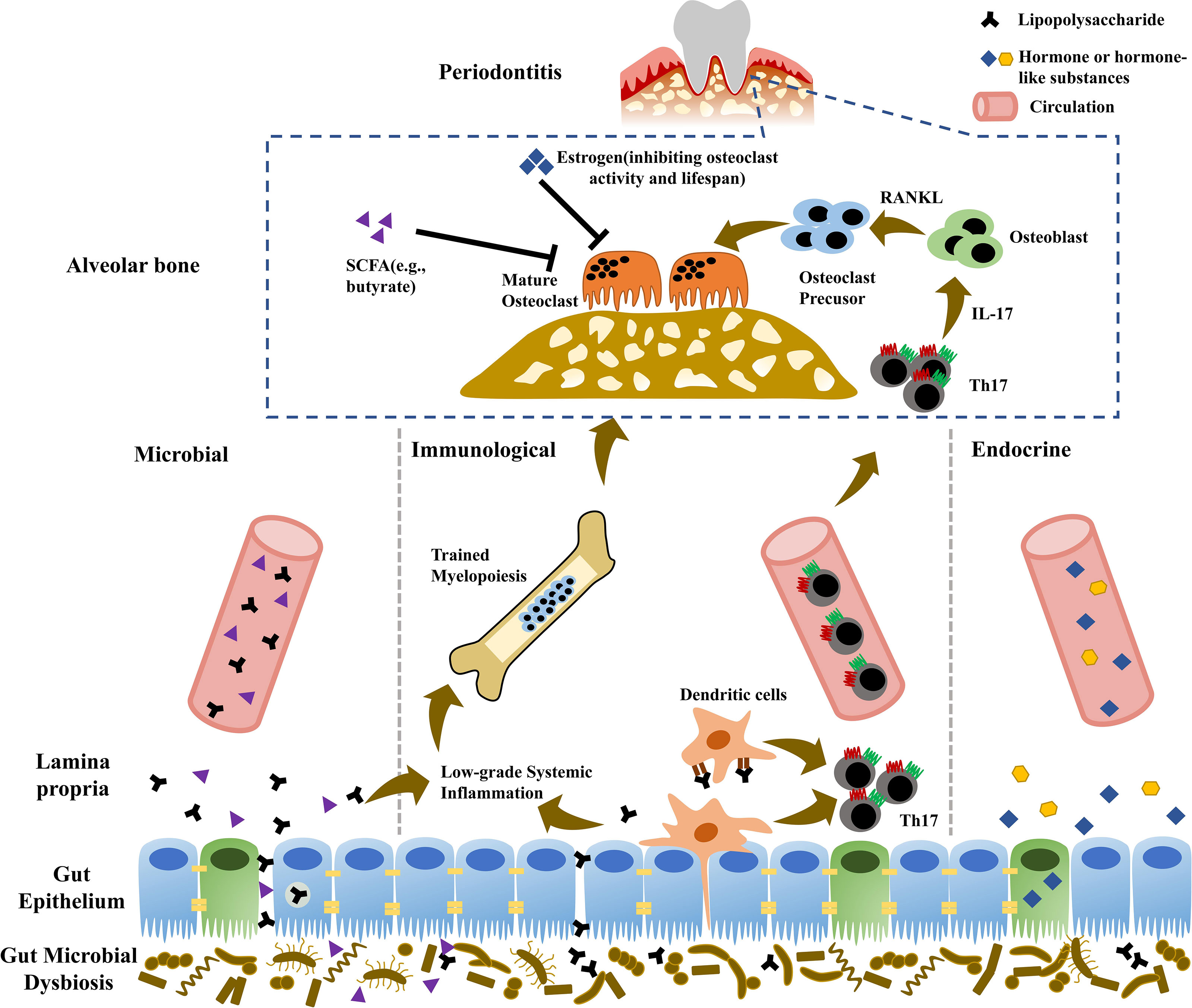
Figure 1 Potential mechanisms involved in “gut-alveolar bone” axis. Microbial pathway: due to impaired gut barrier, gut pathobionts or metabolites possibly translocate to distant alveolar bone via hematogenous way, provoking local inflammatory responses and aggravating periodontitis. Immunological pathway: gut pathogens can induce the expansion of gut pathogenic Th17 cells, which potentially migrate to alveolar bone and promote periodontitis development. Additionally, elevated systemic inflammation burden due to microbial translocation enhances trained myelopoiesis in the bone marrow with increased generation of neutrophils and monocyte lineage, which are recruited to periodontal tissue and exacerbate periodontitis. Endocrine pathway: gut microbiota can regulate the production of human hormone or hormone-like chemicals (e.g., growth hormone, insulin-like growth factors, and gonadal steroids), which further influence bone homeostasis and periodontitis.
Lipopolysaccharide
Lipopolysaccharides (LPS), the important component of lipoproteins derived from Gram-negative gut microbes, is a strong stimulatory endotoxin triggering inflammatory immune responses and affecting general health. As a result of enrichment of gut LPS-containing microbes and increased gut paracellular permeability, gut-derived LPS expansion in circulation, defined as endotoxemia, or LPS translocation in distant tissues can potentially induce low-grade inflammation and promote the development of systemic disorders, such as Parkinson’s disease, obesity, insulin resistance, diabetes, NAFLD, ST-elevation myocardial infarction, and atherosclerosis (Cani et al., 2007; Cani et al., 2008; Geng et al., 2016; Perez-Pardo et al., 2019; Carnevale et al., 2020; Cho et al., 2021). In addition, GF mice model further provided strong evidence for the causative role of LPS in NAFLD development, as indicated by that only simultaneous high-fat diet administration and endotoxin-producing pathobiont mono-association induce NAFLD (Fei et al., 2020). The administration of gut commensal microbes, such as A. muciniphila and Roseburia intestinalis, and the modulation of gut microbiota via certain natural substances induced improved gut barrier function and attenuated LPS-associated systemic inflammation, consequently improving related systemic diseases (Cani et al., 2009; Jiang et al., 2016; Li J. et al., 2016; Song et al., 2016; Kasahara et al., 2018; Bian et al., 2020). In addition to paracellular pathway, LPS can translocate gut barrier in transcellular way via the phagocytosis of enterocyte mediated by toll-like receptor 4 (TLR4) (Neal et al., 2006). The obese rats administrated by natural pectin exhibited both increased TJ protein expression and reduced TLR4 expression in the ileum, leading to attenuated systemic inflammation (Jiang et al., 2016).
In gut-bone axis, leaky gut-induced LPS translocation and systemic inflammation potentially link to bone pathologies. Transplantation of fecal materials from metabolic syndrome patients in GF mice enhanced gut permeability by downregulating TJ proteins ZO-1 and occludin, causing elevated plasma level of LPS and subsequently exacerbated OA severity (Huang et al., 2020). Antibiotic treatment altered gut microbiota and decreased serum LPS level, alleviating circulating inflammation and preventing OA progression (Guan et al., 2020). Estrogen-deficient mice exhibited pathological bone loss and impaired bone microarchitecture in femur, with downregulated gut epithelial TJ protein expression and increased serum endotoxin levels, and these pathologies could be prevented by probiotics administration (Li J. Y. et al., 2016). Consistently, our previous studies observed enhanced gut permeability, elevated serum LPS, and systemic inflammation in OVX mice, consequently resulting in Th17-related immune responses in alveolar bone and aggravated periodontitis (Jia et al., 2019; Jia et al., 2021). In addition, administration of probiotics or berberine increased gut butyrate-producing bacteria, enhanced gut barrier function with reduced circulating LPS, and thus ameliorated estrogen deficiency-induced alveolar bone loss (Jia et al., 2019; Jia et al., 2021). Estrogen is potentially an important modulator of gut barrier. 17β-estradiol (E2), the predominant form of estrogen, can directly enhance gut barrier function by upregulating the expression of gel-forming mucin 2 (MUC2) and TJ proteins (ZO-1, occludin, and claudin 4) in colonic mucosa upon estrogen β signaling (Song et al., 2018). Additionally, estrogen can indirectly affect gut barrier by modulating gut microbiota. Estrogen deficiency induces reduction in gut butyrate-producing bacteria (Jia et al., 2019; Jia et al., 2021). Butyrate is a beneficial bacterial metabolite that reinforces gut barrier (Geirnaert et al., 2017). As a result, increased gut permeability due to estrogen deficiency induces gut pathogens translocation into circulation, causing inflammatory responses and promoting alveolar bone loss. Thus, gut barrier can be a potential therapeutic target for periodontitis under estrogen deficiency. Probiotics, such as Lactobacilli and Bifidobacteria, are live bacteria that benefit the host if provided as dietary or medical supplements in adequate quantities. Berberine is an alkaloid present in some medicinal plants, such as Berberis vulgaris, and Hydrastis canadens. With selective enrichment of gut butyrate-producing bacteria, probiotics and berberine supplements enhance gut barrier function and exert protective roles on alveolar bone under estrogen deficiency, representing a promising adjuvant treatment against periodontitis in postmenopausal women.
Short-Chain Fatty Acids
Short-chain fatty acids (SCFAs) are end-products deriving from gut microbial fermentation of dietary indigestible fibers, which escape from host digestion and absorption, mainly including butyrate, propionate, and acetate (Koh et al., 2016; Morrison and Preston, 2016). The type and amount of SCFAs generated in the gut depend on gut microbial composition, substrate types, and intestinal transit time (Macfarlane and Macfarlane, 2003). SCFA concentration varies among different segments of human intestinal tract, with high level in the cecum and proximal colon (Cummings et al., 1987). SCFAs are absorbed in the cecum and colon via active transport mediated by Na+- and H+-coupled monocarboxylate transporters, or via protonation-dependent non-ionic diffusion, or possibly via special exchange with intracellular bicarbonate or other SCFAs (Titus and Ahearn, 1988; Canfora et al., 2015; Koh et al., 2016). In addition to being partially metabolized by colonocytes as energy source, other absorbed SCFAs translocate into circulation. Although distinctly lower than the concentration in colonic contents, three major SCFAs are detected in human portal, hepatic, and peripheral venous blood with a descending trend among the levels of acetate, propionate, and butyrate (Cummings et al., 1987). Consistently, propionic and butyric acid levels in cecal contents are also correlated with that in aortic serum of the rats fed with dietary fibers (Jakobsdottir et al., 2013).
Via hematogenous way, gut-derived SCFAs are potentially transmitted to distant organs and involved in physiological processes or diseases. Transported through portal circulation to liver, SCFAs are consumed by hepatocytes in energy metabolism as well as the biosynthesis of cholesterol, glucose, and fatty acids (Dalile et al., 2019). Following hepatic utilization, small amounts of SCFAs travel to other organs via systemic circulation. SCFAs translocate to brain across blood-brain barrier and affect brain physiology and pathology, such as central appetite regulation, and post-stroke improvement (Frost et al., 2014; Lee et al., 2020). SCFAs generated by maternal gut microbiota are reflected in embryo tissues or organs, including sympathetic nerves, gut epithelium, and pancreas, participating in the development of postnatal nerve and metabolic systems via GPR41 and GPR43 signaling (Kimura et al., 2020).
Gut-derived SCFAs, especially butyrate and propionate, are the regulators of bone homeostasis, traveling to bone marrow and directly affecting bone metabolism. Fructo-oligosaccharide administration significantly increases serum level of butyrate and promotes bone formation in rats, ameliorating the pathological bone loss due to estrogen deficiency (Porwal et al., 2020). Study by Lucas and colleagues directly demonstrated the translocation of SCFAs to bone tissue, as evidenced by augmented butyrate or propionate concentrations in bone marrow of the mice with exogenous supplement of corresponding SCFA in drinking water (Lucas et al., 2018). Previous studies proved the direct regulation of SCFAs on the differentiation of osteoclasts and osteoblasts (Iwami and Moriyama, 1993; Rahman et al., 2003; Chen et al., 2007; Morozumi, 2011; Chang et al., 2016; Lucas et al., 2018). Butyrate, the strong histone deacetylase inhibitor, have been reported as a strong inhibitor of osteoclastogenesis. Early study showed the inhibitory role of sodium butyrate on murine osteoclast differentiation in bone marrow, possibly depending on the cytotoxicity of sodium butyrate (Iwami and Moriyama, 1993). Subsequent researches further updated related specific mechanisms. The in vitro study on murine macrophage cell line RAW264 showed that sodium butyrate inhibited osteoclast formation via regulating nuclear factor-κB (NF-κB) and mitogen-activated protein kinase (MAPK) signaling pathways (Rahman et al., 2003). Furthermore, butyrate and propionate can inhibit osteoclast differentiation via enhancing glycolysis in osteoclast precursors and subsequently downregulating the expression of osteoclast marker genes TRAF6 and NFATc1 (Lucas et al., 2018). The role of SCFAs on osteoblast formation remains uncertain. Sodium butyrate can promote osteogenic differentiation of mesenchymal stem cells via activating ERK pathway (Chen et al., 2007). However, osteoblastic differentiation of ROS17/2.8 cells was halted in the presence of high-level butyrate (10-3M) (Morozumi, 2011). MG-63 osteoblasts treated by butyrate demonstrated suppressed cell proliferation with increased cell cycle arrest and reactive oxygen species (ROS) production (Chang et al., 2016). However, the in vivo study by Lucas showed no effects of SCFAs on osteoblasts and bone formation (Lucas et al., 2018).
Our previous studies indicated that estrogen deficiency led to reduction of gut butyrate-producing bacteria, such as Clostridium leptum, Clostridium coccoides, Fecalibacterium prausnitzii, and Roseburia, as well as decreased butyrate production, leading to more severe periodontitis (Jia et al., 2019; Jia et al., 2021). Rescue of gut butyrate-producing bacteria and butyrate production by berberine or probiotics prevented the alveolar bone loss induced by estrogen deficiency, suggesting the pivotal roles of gut-derived butyrate in periodontitis (Jia et al., 2019; Jia et al., 2021). The indirect regulation of gut-derived butyrate on periodontitis possibly depends on gut barrier. In addition, butyrate may potentially translocate to alveolar bone via circulation and directly affects periodontitis progression.
Of note, some pathogenic bacteria in periodontitis, such as Porphyromonas gingivalis and Fusobacterium nucleatum, can produce SCFAs, including butyrate and propionate. Interestingly, butyrate has cytotoxic effects in patients with periodontitis. The concentration of butyrate in gingival crevices positively correlates to the severity of periodontitis. Butyric acid could reach to millimolar concentration in the gingival crevicular fluid of periodontitis patients, whereas the maximum plasma concentration of butyrate of adult leukemia patients after intravenous infusion only reached to 0.05 millimolar (Miller et al., 1987; Niederman et al., 1997; Lu et al., 2014). Oral-derived butyrate can promote periodontitis development (Guan et al., 2021), likely due to its cytotoxic effects on gingival epithelial cells and fibroblasts. Butyrate at millimolar concentrations can induce apoptosis, autophagy, and pyroptosis of gingival epithelial cells and impair epithelial TJ, leading to the destruction of gingival epithelial barrier and periodontitis initiation (Tsuda et al., 2010; Evans et al., 2017; Liu et al., 2019; Magrin et al., 2020). Butyric acid can negatively affect cell growth and cell cycle progression of gingival fibroblasts by inducing reactive oxygen species production (Kurita-Ochiai et al., 2008; Chang et al., 2013), and lead to mitochondria- and caspase-dependent apoptosis in inflamed gingival fibroblasts in a dose-dependent manner (Kurita-Ochiai et al., 2008). Long-term exposure to butyrate can also lead to cytostasis and apoptosis of healthy gingival fibroblasts via intrinsic and extrinsic pathways, promoting periodontitis progression (Shirasugi et al., 2017; Shirasugi et al., 2018). In addition, butyrate can dose-dependently induce the production of proinflammatory cytokines in gingival fibroblasts, including IL-6 and IL-11, which rescue T-cell from apoptosis and contributed to the development of periodontitis (Kurita-Ochiai et al., 2002). Thus, unlike circulating butyrate derived from gut microbiota, local accumulation of concentrated butyrate due to periodontal pathogens has deleterious effects on alveolar bone, which should be taken into consideration in future investigation.
Immunological Pathway
The Immune Responses Involved in Periodontitis
The bone homeostasis is the dynamic balance between the coupled processes of osteoblast-mediated bone formation and osteoclast-mediated bone resorption. The immune system has long been recognized as an essential regulator of bone metabolism. The crosstalk between immune and skeletal systems, termed as “osteoimmunology,” underlies bone physiological and pathological processes (Rho et al., 2004). The components of immune systems, such as immune cells and cytokines, are involved in bone remodeling and metabolic or inflammatory bone diseases, including osteoporosis, arthritis, and periodontitis (Weitzmann and Pacifici, 2006; Hajishengallis, 2015; Kuhn and Morrison, 2020). Periodontitis is an inflammation induced by exogenous pathogens. Microbial pathogens are recognized and presented by innate immune system to activate adaptive immune responses, thereby inducing the production of effector cells and molecules and driving the initiation of periodontal destruction.
Neutrophil
Neutrophils are terminally differentiated white blood cells produced abundantly in the bone marrow and recruited to infected or damaged tissues via circulation (Borregaard, 2010). Neutrophils are functionally versatile effector cells, displaying antibacterial and cytotoxic properties, as well as de novo biosynthesis of chemokines and cytokines with multiple functions (e.g., proinflammatory, anti-inflammatory, and immunoregulatory) (Scapini and Cassatella, 2014). Apart from acute infections, it has been currently well established that neutrophils are also implicated in chronic inflammatory diseases such as periodontitis (Hajishengallis, 2020). Neutrophils are integral to maintain periodontal homeostasis via transmigrating into gingival crevice and constituting the first defense line against subgingival bacterial plaque (Hajishengallis and Hajishengallis, 2014). The neutrophils with hyperactivity or in excessive amount contribute to periodontal tissue destruction and periodontitis progression (Hajishengallis et al., 2015). In addition, neutrophils are capable of producing chemokines CCL2 and CCL20, selectively recruiting pathogenic T-helper 17 (Th17), the pivotal cells in periodontitis development (Pelletier et al., 2010).
T-Helper 1 Cell and T-Helper 2 Cell
T-helper 1 cell (Th1) and Th2 are the major effector cells responsible for cellular immunity and humoral immunity, respectively. Th1/Th2 paradigm mainly accounts for the mechanism of periodontitis progression. Th1 cells are predominant in gingivitis or stable periodontal lesion, while established or progressive periodontal lesion in chronic periodontitis is mediated by Th2 cells, which subsequently activate B cell/plasma cell response (Ohlrich et al., 2009). T and B lymphocytes highly express receptor activator for NF-κB ligand (RANKL) and promote RANKL-mediated osteoclast differentiation in periodontitis (Kawai et al., 2006).
Th17 and Regulatory T Cell
Accumulating evidences have indicated the pivotal roles of novel T subsets Th17 and regulatory T cell (Treg) in the pathogenesis of bone diseases, such as osteoporosis, autoimmune arthritis, and ankylosing spondylitis (Komatsu et al., 2014; Li J. Y. et al., 2016; Schmidt et al., 2019; Xu et al., 2019; Xie et al., 2020). Th17 and Treg cells share common precursor cells and cytokines for initial differentiation, but result in opposite functions. Th17 cells are the T-cell subset producing IL-17 and triggering immune responses against exogeneous pathogens, while Treg cells suppress immune responses for immune homeostasis. In consistent with Th1/Th2 cells balance, Th17/Treg cells are maintained in dynamic equilibrium, while Th17/Treg shifting in favor of Th17 elicits pathological conditions. Th17/Treg imbalance is involved in periodontitis development, as indicated by enhanced Th17 response with increased IL-17A production and suppressed anti-osteoclastogenic function of Treg, which is also associated with exacerbated periodontitis during pregnancy (Hays et al., 2019; Alvarez et al., 2020). Additionally, Aggregatibacter actinomycetemcomitans–induced periodontitis mice show strong Th17 immune responses in periodontal lesions (Monasterio et al., 2018). The orthodontic tooth movement under periodontitis depends on osteoclast metabolism modulated by Th17/Treg equilibrium (Ge et al., 2020). In addition, Th17 proportion in periodontal lesions from the patients with periodontitis is distinctly higher than that in healthy individuals and correlated with the severity of periodontitis, while Th17 cell defects reduce susceptibility to periodontitis (Dutzan et al., 2018). Oral pathogens drive pathogenic Th17 differentiation in periodontitis via interleukin-6 (IL-6) and IL-23 signaling (Dutzan et al., 2018; Bunte and Beikler, 2019). Pathogenic Th17 cells further induce mucosal immune response and periodontal bone destruction to eradicate pathogens at the expense of bone damage (Tsukasaki et al., 2018). Our previous studies explored Th17/Treg imbalance in the pathogenesis of periodontitis under estrogen deficiency (Jia et al., 2019; Jia et al., 2021). Estrogen-deficient rats demonstrated exacerbated bone loss in periodontitis with increased Th17 cells or an elevated Th17/Treg ratio in both alveolar bone and femoral bone marrow, while probiotics or berberine administration rescued Th17/Treg imbalance and prevented estrogen deficiency-induced alveolar bone destruction in periodontitis (Jia et al., 2019; Jia et al., 2021). In addition, IL-17 derived from Th17 can amplify the production of RANKL by osteoblasts, indirectly inducing osteoclast differentiation (Hajishengallis and Chavakis, 2021).
Potential Pathways in the “Gut-Immunity-Periodontitis” Axis
Gut-Derived Lymphocytes Migration
Multiple lymphocytes are evoked in intestinal lamina propria and migrate to effector sites depending on the mediation of chemokines, participating in the development, physiology, and pathogenesis of distant tissues (Gray et al., 2017; Huang et al., 2018). In addition to microbial translocation, gut-derived immunocytes migration to bone tissue is an alternative pathway in “gut-bone” axis. Th17 cells abundantly reside in intestinal lamina propria. Specific gut microbes induce the differentiation of Th17 cells with distinct functions, namely, homeostatic tissue-resident type and pathogenic inflammatory type (Omenetti et al., 2019). Homeostatic Th17 cells with non-pathogenic plasticity are elicited by gut commensal segmented filamentous bacteria and function as quiescent or memory T cells, maintaining gut barrier integrity and homeostasis (Stockinger and Omenetti, 2017; Ladinsky et al., 2019; Omenetti et al., 2019). During the infection of intestine, gut pathogens, such as Citrobacter rodentium, induce intestinal epithelial cells to generate ROS, which promotes pathogenic Th17 differentiation and impairs gut barrier, as followed by translocation of pathogens to the lamina propria and further enhancement of inflammatory Th17 response (Stockinger and Omenetti, 2017). Gut pathogenic Th17 cells migrate to extraintestinal tissues via circulation, contributing to the development of multiple diseases. The migration of Th17 can be directly proved using transgenic mice expressing photoconvertible fluorescence protein Kaede, an ideal system to track lymphocyte movement (Morton et al., 2014; Krebs et al., 2016; Calcinotto et al., 2018; Mathies et al., 2018). Kaede is fluorescent green and can be photoconverted to red by violet or UV light exposure (Tomura et al., 2008). Accordingly, the cells in the region of interest can be labeled by photoconverted Kaede protein in vivo (Tomura et al., 2008). Intestine infection with C. rodentium can provoke the expansion of gut pathogenic Th17 cells, which migrate into the kidney and aggravate the pathology in experimental crescentic glomerulonephritis mice (Krebs et al., 2016). During T cell-mediated colitis, pathogenic Th17 migration and absence of Treg are responsible for colitis-associated liver inflammation (Mathies et al., 2018). Gut-derived inflammatory Th17 cells migrate to spleen and interact with B cells, promoting autoantibody production and inducing autoinflammatory arthritis (Morton et al., 2014). Gut pathogenic Th17 cells elicited by Prevotella heparinolytica migrate to bone marrow and accelerate multiple myeloma progression in cooperation with eosinophils (Calcinotto et al., 2018). Additionally, gut Th17 migration into the lung also contributes to the development of pulmonary complications during arthritis (Bradley et al., 2017). Furthermore, parathyroid hormone (PTH)-induced bone loss depends on gut microbe SFB capable of inducing Th17 cells (Yu et al., 2020). With the enrichment of gut SFB, PTH promotes the expansion of gut TNF+ T cells and Th17 cells, which are recruited from gut to bone marrow and contribute to bone loss (Yu et al., 2020). Although there still lacks direct evidence, gut Th17 migration to alveolar bone is possible, thus potentially contributing to the development of periodontitis (Figure 1).
Gut-derived immunocytes migration to distant tissues contains the translocation from intestinal lamina propria to circulation and following migration from circulation to effector tissue. Sphingosine-1-phosphate (S1P) is the major regulator of lymphocyte migration out of lymph nodes. Depending on gradient concentration of S1P, effector T cells expressing S1P receptor-1 (S1PR1) emigrate from lymph node to circulation (Benechet et al., 2016; Yu et al., 2021). In addition, T cells further migrate to distant tissues via expressing various chemokine receptors, including CCR subfamily (e.g., CCR4, CCR5, CCR6, CCR7) and CXCR subfamily (e.g., CXCR3, CXCR5, CXCR6), which interacting with the corresponding chemokines (e.g., CCL22, CCL20, CCL21, CXCL9, CXCL10) (Sokol and Luster, 2015). CCR6 signaling regulates the egress of Treg and Th17 to kidney, involving in the development of glomerulonephritides (Turner et al., 2010). Intestinal pathogenic Th17 cells can emigrate out of intestinal lamina propria via S1P/S1PR1 signaling, and then travel to the kidney guided by CCL20/CCR6 axis, promoting the pathology of glomerulonephritides (Krebs et al., 2016). Additionally, the influx of gut TNF+ cells and Th17 cells to bone marrow induced by PTH or estrogen deficiency is respectively mediated by CXCR3 and CCL20 signaling (Yu et al., 2020; Yu et al., 2021).
Trained Myelopoiesis in the Bone Marrow
Estrogen-deficiency-associated low-grade systemic inflammation, possibly induced by impaired gut barrier and hematogenous dissemination of gut pathogens or metabolites, contributes to development of bone pathologies including periodontitis (Li J.Y. et al., 2016; Jia et al., 2019; Jia et al., 2021). However, the underlying mechanism whereby elevated systemic inflammation burden destroys bone homeostasis remains unclear. Bone marrow, the primary site of hematopoiesis, can quickly sense and respond to systemic inflammation, eliciting a cascade of reactions (Hajishengallis and Chavakis, 2021). Historically, innate and adaptive immunity are classic immune forms resistant to infection or injury. In addition to innate and adaptive immunity, trained immunity is a novel immune mode induced by microorganisms or inflammation. Trained immunity could “train” innate immune cells to produce highly reactive and non-specific immune memory via epigenetic modifications and metabolic reprogramming, eliminating pathogens and resisting secondary infection (Netea et al., 2016; Netea et al., 2020). Non-innate immune cells, such as hematopoietic stem and progenitor cells (HSPCs), could be also involved in training immunity. Trained immunity initiated in the bone marrow can enhance myelopoiesis with expansion and myeloid-biased differentiation of HSPCs, increasing the production of effector cells against infection, such as neutrophils, and monocytes (Mitroulis et al., 2018; Chavakis et al., 2019). Intravenous Bacillus Calmette-Guérin (BCG) in mice can induce the proliferation and differentiation of hematopoietic stem cells (HSCs) and multipotent progenitors in bone marrow into monocyte/macrophage lineage, resisting the infection of Mycobacterium tuberculosis (Kaufmann et al., 2018). In addition to resisting infection and promoting host survival, trained immunity potentially has a harmful impact on the host. Neutrophils or monocytes/macrophages generated in training immunity can be recruited to other infection or injury sites to promote chronic inflammation and the development of systemic diseases such as obesity and atherosclerosis (Bekkering et al., 2020; Schloss et al., 2020). Monocyte lineage, the osteoclast precursors, may migrate to the sites of active bone resorption (e.g., alveolar bone in periodontitis) and differentiate into osteoclasts via macrophage colony-stimulating factor (M-CSF) and RANKL signaling, further aggravating periodontal bone loss (Hajishengallis and Chavakis, 2021). Similarly, excessive amounts of neutrophils are possibly recruited to periodontal tissue and exacerbate periodontitis. Of note, periodontitis itself can also induce low-grade systemic inflammation due to hematogenous dissemination of periodontal pathogens or the expansion of periodontal inflammatory mediators (Hajishengallis and Chavakis, 2021). It suggests that periodontitis-associated systemic inflammation potentially in turn aggravated periodontal bone destruction (Figure 1).
Endocrine Pathway
Currently, hormone or hormone-like chemicals, such as growth hormone, PTH, insulin-like growth factors (IGFs), and gonadal steroids, have been considered as important regulators of bone metabolism. Gut microbiota, newly referred as an “endocrine organ”, regulates the production of human hormone and hormone-like substances, influencing host physiology and disorders (Figure 1).
Insulin-Like Growth Factor-1
IGF-1 belongs to the IGF family, which plays an important role in childhood growth and has anabolic effects in adults. IGF-1 in serum, existing as a complex containing IGF-1 molecule, IGF binding protein 3 (IGFBP-3), and acid labile subunit (ALS), is mainly generated by hepatocytes in response to growth hormone and travels to distant organs via circulation (Daughaday et al., 1972). In addition, IGF-1 is also locally produced via autocrine or paracrine fashion in non-hepatic organs/tissues, including bone. Accumulating evidences have suggested IGF-1 as an important regulator of skeletal development and bone remolding (Yakar et al., 2010; Tahimic et al., 2013; Wang et al., 2013). With the absence of ALS, reduction of circulating IGF-1 results in disrupted linear growth and decreased bone mineral density (Yakar et al., 2002). Circulating IGF-1 also affects skeletal integrity and mechanical properties via regulating lateral growth of long bone and deposition of mineralized cortical bone tissue (Yakar et al., 2009; Courtland et al., 2011). Subcutaneous administration of IGF-1 improves bone density and cortical thickness in osteopenia mice by upregulating osteogenic-related proteins and reducing protein expression in osteoclastic activity (Guerra-Menéndez et al., 2013). Of note, reduced circulating IGF-1 during early lifetime can be compensated by local IGF-1 signaling, as evidenced by increased bone mass with early-life loss of circulating IGF-1 in female mice (Ashpole et al., 2016). IGF-1 binds to IGF-1 receptor (a tyrosine kinase receptor) and elicits IGF-1 signaling, which regulates the growth and differentiation of osteoclasts and osteoblasts. IGF-1 can promote osteoclast differentiation through maintaining the normal interplay between osteoblasts and osteoclast precursors, depending on regulating the production of RANKL and M-CSF (Wang et al., 2006). Furthermore, IGF/IGF-IR signaling mediates the proliferation and differentiation of osteoprogenitor regulated by PTH, influencing periosteal bone formation (Wang et al., 2007).
Mounting evidences have suggested that gut microbiota affects host development and general health via regulating systemic IGF-1 levels (Yan and Charles, 2018). Commensal microbe Acetobacter pomorum regulates insulin/IGF signaling in Drosophila depending on the activity of pyrroloquinoline quinone-dependent alcohol dehydrogenase, contributing to host development and metabolic homeostasis (Shin et al., 2011). During intestinal or pneumonic infection, gut bacterium Escherichia coli O21:H+ maintains systemic IGF-1 level, which links to increased IGF-1 level and E.coli O21:H+ colonization in white adipose tissue, thus preventing muscle wasting and promoting disease tolerance (Schieber et al., 2015). The catabolic and anabolic effects of commensal gut microbiota on bone remolding are also partially mediated by regulation of systemic and local IGF-1 signaling (Yan et al., 2016; Novince et al., 2017). Gut microbiota colonization in GF mice promotes bone turnover with elevated IGF-1 levels in serum and bone marrow, while antibiotic treatment in SPF mice reduces serum IGF-1 level and increases bone mass, which can be rescued by SCFA supplement (Yan et al., 2016).
Sex Hormones
Sex hormones, such as follicle-stimulating hormone (FSH), estrogen, and androgen, have been considered as important regulators of bone metabolism (Leder, 2007; Khosla, 2020). Both estrogen and androgen prevent bone resorption and sustain bone formation (Syed and Khosla, 2005). A clinical randomized study demonstrated that hormone/estrogen replacement therapy could ameliorate postcranial bone density and alveolar bone mass in postmenopausal women (Civitelli et al., 2002). Furthermore, estrogen deficient rats had lower periodontal bone density than control rats, while estradiol treatment protects against alveolar bone loss induced by estrogen deficiency (Duarte et al., 2006). Additionally, estrogen deficiency has adverse effects on the bone healing after bone extraction (Bezerra et al., 2013). Estrogen doesn’t directly regulate osteoclast differentiation, instead it inhibits mature osteoclast function by downregulating genes relevant to bone resorption, and controls mature osteoclast lifespan by promoting apoptosis in binding with estrogen receptor α (ERα) (Imai et al., 2009). Consistently, estrogen-deficient rats presented increased osteoclasts in alveolar bone, and exogenous estrogen supplement reduces alveolar bone osteoclasts partially due to osteoclast apoptosis (Faloni et al., 2007; Bezerra et al., 2013). Of note, instead of femur and vertebrae, estrogen affects maxillary bone phenotype via IL-33/ST2 signaling in a site-specific manner (Macari et al., 2018). In addition, without direct action on bone cells, high FSH activity promotes bone formation depending on ovary pathway (Allan et al., 2010).
Consistently, gut microbiota has been suggested as an important regulator of hormone homeostasis (Baker et al., 2017; Rizzetto et al., 2018). As early as about 40 years ago, it was reported that gut microbiota intervention by antibiotic treatment resulted in increased fecal estrogen levels with reduced urinary estrogen excretion, especially estradiol and estriol, which potentially related to the suppressed gut reductive estrogen metabolism (Adlercreutz et al., 1984). More evidences have shown the association between gut microbial composition and urinary or systemic levels of estrogen and estrogen metabolites (Flores et al., 2012; Fuhrman et al., 2014). As for testosterone, early-life colonization of gut commensal microbes or gut microbial transfer alters serum level of testosterone, affecting the development of autoimmune diseases (Markle et al., 2013). However, the mechanism whereby gut microbiota affects hormone remains uncertain. Recently, it has been reported that gut microbiota affects hormone homeostasis depending on “endobolome,” a novel term that refers to the aggregate of gut microbial genes involved in the production and metabolism of sex steroid hormones including estrogen (Aguilera et al., 2020). Gut microbiota metabolizes hormones via distinct enzymes, such as β-glucuronidases, β-glucuronides, and hydroxysteroid dehydrogenase, affecting circulating and local hormone levels (Plottel and Blaser, 2011; García-Gómez et al., 2013). Enzyme β-glucuronidases derived from gut microbiota promote deconjugated estrogens production, which travel into circulation and carry out physiological functions (Rizzetto et al., 2018). Additionally, gut microbe Clostridium scindens could transfer glucocorticoids to androgens via side-chain cleavage (Ridlon et al., 2013). Some gut bacteria, mostly belonging to the family Coriobacteriaceae, are capable of metabolizing soy-derived isoflavone-glycosides and producing metabolites, including equol, a compound with strong estrogenic activity (Guadamuro et al., 2017; Chen and Chen, 2021). Although direct evidence is still lacking, interplays in “gut-hormone-bone” axis provide another pathway whereby gut microbiota regulates periodontal diseases.
Serotonin
Serotonin, namely, 5-hydroxytrypatamine (5-HT), is a neurotransmitter with hormone-like activity, which can affect bone homeostasis via circulation. Circulating serotonin derived from duodenum inhibits bone formation and regulates bone mass (Yadav et al., 2008). Serotonin transporter is a plasma membrane transporter expressing on bone cells, which is responsible for the uptake of extracellular serotonin and subsequent intracellular serotonin storage and degradation. Extracellular serotonin signaling enhancement via serotonin transporter inhibition exerts detrimental effects on skeletal growth and bone homeostasis, as indicated by reduced bone mass, altered microarchitecture, as well as impaired mechanical properties, relating to increased risk of osteoporotic fracture (Warden et al., 2005; Warden et al., 2008; Eom et al., 2012; Wu et al., 2012; Kerbage et al., 2014; Gorgas et al., 2021). Serotonin can promote osteoclast proliferation and differentiation via NF-κB signaling and influence osteoblast proliferation in a dose-dependent manner (Battaglino et al., 2004; Gustafsson et al., 2006). Serotonin directly prevents osteoblast differentiation via Htr1b and CREB signaling (Yadav et al., 2008).
Serotonin is mainly synthesized by enterochromaffin cells and exerts biological effects via the circulation. Accumulating evidences indicate that gut microbiota affects the biosynthesis and availability of serotonin. SCFAs derived from gut microbiota, such as acetate and butyrate, promote serotonin secretion via upregulating the expression of tryptophan hydroxylase 1 (Tph1), which is the rate-limiting enzyme responsible for serotonin biosynthesis in enterochromaffin cells (Reigstad et al., 2015; Vincent et al., 2018). Gut microbe Clostridium ramosum or its components are also reported to increase serotonin secretion and availability from enterochromaffin cells (Mandić et al., 2019). Hence, gut microbiota also potentially regulates alveolar bone homeostasis via serotonin.
Conclusion
Periodontitis is characterized by inflammatory periodontal bone loss resulting from impaired alveolar bone homeostasis, which is influenced by multiple factors including systemic diseases or local intestinal infections. The deleterious effects of systemic or intestinal diseases on alveolar bone induce complexity of periodontitis treatment and inferior prognosis. Recent findings have revealed the pivotal roles of gut microbiota in bone homeostasis and pathologies, providing a potential mechanism whereby systemic conditions affect periodontitis. Given the crosstalk between gut microbiota and host, alteration in gut microbiota due to systemic disorders may regulate alveolar bone homeostasis. Future investigations are needed to provide adequate evidences and advance the understanding of “gut-alveolar bone” axis, thus promoting the better management of periodontitis.
Author Contributions
XX contributed to the conception and manuscript revision, read, and approved the submitted version. XJ wrote the first draft of the manuscript and contributed to manuscript revision, read, and approved the submitted version. Other authors contributed to manuscript revision, read, and approved the submitted version.
Funding
This work was supported by National Natural Science Foundation of China (81870754, 81800989, 81991500, 81991501), and a research grant from the West China Hospital of Stomatology, Sichuan University (LCYJ2019-4).
Conflict of Interest
The authors declare that the research was conducted in the absence of any commercial or financial relationships that could be construed as a potential conflict of interest.
Publisher’s Note
All claims expressed in this article are solely those of the authors and do not necessarily represent those of their affiliated organizations, or those of the publisher, the editors and the reviewers. Any product that may be evaluated in this article, or claim that may be made by its manufacturer, is not guaranteed or endorsed by the publisher.
References
Adlercreutz, H., Pulkkinen, M. O., Hämäläinen, E. K., Korpela, J. T. (1984). Studies on the Role of Intestinal Bacteria in Metabolism of Synthetic and Natural Steroid Hormones. J. Steroid Biochem. 20 (1), 217–229. doi: 10.1016/0022-4731(84)90208-5
Aguilera, M., Gálvez-Ontiveros, Y., Rivas, A. (2020). Endobolome, a New Concept for Determining the Influence of Microbiota Disrupting Chemicals (MDC) in Relation to Specific Endocrine Pathogenesis. Front. Microbiol. 11, 578007. doi: 10.3389/fmicb.2020.578007
Aimetti, M., Perotto, S., Castiglione, A., Mariani, G. M., Ferrarotti, F., Romano, F. (2015). Prevalence of Periodontitis in an Adult Population From an Urban Area in North Italy: Findings From a Cross-Sectional Population-Based Epidemiological Survey. J. Clin. Periodontol. 42 (7), 622–631. doi: 10.1111/jcpe.12420
Allan, C. M., Kalak, R., Dunstan, C. R., McTavish, K. J., Zhou, H., Handelsman, D. J., et al. (2010). Follicle-Stimulating Hormone Increases Bone Mass in Female Mice. Proc. Natl. Acad. Sci. U. S. A. 107 (52), 22629–22634. doi: 10.1073/pnas.1012141108
Alvarez, C., Suliman, S., Almarhoumi, R., Vega, M. E., Rojas, C., Monasterio, G., et al. (2020). Regulatory T Cell Phenotype and Anti-Osteoclastogenic Function in Experimental Periodontitis. Sci. Rep. 10 (1), 19018. doi: 10.1038/s41598-020-76038-w
Ashpole, N. M., Herron, J. C., Mitschelen, M. C., Farley, J. A., Logan, S., Yan, H., et al. (2016). IGF-1 Regulates Vertebral Bone Aging Through Sex-Specific and Time-Dependent Mechanisms. J. Bone Miner. Res. 31 (2), 443–454. doi: 10.1002/jbmr.2689
Atarashi, K., Suda, W., Luo, C., Kawaguchi, T., Motoo, I., Narushima, S., et al. (2017). Ectopic Colonization of Oral Bacteria in the Intestine Drives T(H)1 Cell Induction and Inflammation. Science 358 (6361), 359–365. doi: 10.1126/science.aan4526
Baker, J. M., Al-Nakkash, L., Herbst-Kralovetz, M. M. (2017). Estrogen-Gut Microbiome Axis: Physiological and Clinical Implications. Maturitas 103, 45–53. doi: 10.1016/j.maturitas.2017.06.025
Battaglino, R., Fu, J., Späte, U., Ersoy, U., Joe, M., Sedaghat, L., et al. (2004). Serotonin Regulates Osteoclast Differentiation Through Its Transporter. J. Bone Miner. Res. 19 (9), 1420–1431. doi: 10.1359/JBMR.040606
Bekkering, S., Saner, C., Riksen, N. P., Netea, M. G., Sabin, M. A., Saffery, R., et al. (2020). Trained Immunity: Linking Obesity and Cardiovascular Disease Across the Life-Course? Trends Endocrinol. Metab. 31 (5), 378–389. doi: 10.1016/j.tem.2020.01.008
Benechet, A. P., Menon, M., Xu, D., Samji, T., Maher, L., Murooka, T. T., et al. (2016). T Cell-Intrinsic S1PR1 Regulates Endogenous Effector T-Cell Egress Dynamics From Lymph Nodes During Infection. Proc. Natl. Acad. Sci. U. S. A. 113 (8), 2182–2187. doi: 10.1073/pnas.1516485113
Bezerra, J. P., de Siqueira, A., Pires, A. G., Marques, M. R., Duarte, P. M., Bastos, M. F. (2013). Effects of Estrogen Deficiency and/or Caffeine Intake on Alveolar Bone Loss, Density, and Healing: A Study in Rats. J. Periodontol. 84 (6), 839–849. doi: 10.1902/jop.2012.120192
Bian, M., Wang, J., Wang, Y., Nie, A., Zhu, C., Sun, Z., et al. (2020). Chicory Ameliorates Hyperuricemia via Modulating Gut Microbiota and Alleviating LPS/TLR4 Axis in Quail. BioMed. Pharmacother. 131, 110719. doi: 10.1016/j.biopha.2020.110719
Blanton, L. V., Charbonneau, M. R., Salih, T., Barratt, M. J., Venkatesh, S., Ilkaveya, O., et al. (2016). Gut Bacteria That Prevent Growth Impairments Transmitted by Microbiota From Malnourished Children. Science 351 (6275), 10.1126/science.aad3311 aad3311. doi: 10.1126/science.aad3311
Boer, C. G., Radjabzadeh, D., Medina-Gomez, C., Garmaeva, S., Schiphof, D., Arp, P., et al. (2019). Intestinal Microbiome Composition and its Relation to Joint Pain and Inflammation. Nat. Commun. 10 (1), 4881. doi: 10.1038/s41467-019-12873-4
Bongo, A. S., Brustad, M., Oscarson, N., Jönsson, B. (2020). Periodontal Health in an Indigenous Sámi Population in Northern Norway: A Cross-Sectional Study. BMC Oral. Health 20 (1), 104. doi: 10.1186/s12903-020-01098-3
Borregaard, N. (2010). Neutrophils, From Marrow to Microbes. Immunity 33 (5), 657–670. doi: 10.1016/j.immuni.2010.11.011
Bradley, C. P., Teng, F., Felix, K. M., Sano, T., Naskar, D., Block, K. E., et al. (2017). Segmented Filamentous Bacteria Provoke Lung Autoimmunity by Inducing Gut-Lung Axis Th17 Cells Expressing Dual TCRs. Cell Host Microbe 22 (5), 697–704.e694. doi: 10.1016/j.chom.2017.10.007
Bunte, K., Beikler, T. (2019). Th17 Cells and the IL-23/IL-17 Axis in the Pathogenesis of Periodontitis and Immune-Mediated Inflammatory Diseases. Int. J. Mol. Sci. 20 (14), 3394. doi: 10.3390/ijms20143394
Calcinotto, A., Brevi, A., Chesi, M., Ferrarese, R., Garcia Perez, L., Grioni, M., et al. (2018). Microbiota-Driven Interleukin-17-Producing Cells and Eosinophils Synergize to Accelerate Multiple Myeloma Progression. Nat. Commun. 9 (1), 4832. doi: 10.1038/s41467-018-07305-8
Canfora, E. E., Jocken, J. W., Blaak, E. E. (2015). Short-Chain Fatty Acids in Control of Body Weight and Insulin Sensitivity. Nat. Rev. Endocrinol. 11 (10), 577–591. doi: 10.1038/nrendo.2015.128
Cani, P. D., Amar, J., Iglesias, M. A., Poggi, M., Knauf, C., Bastelica, D., et al. (2007). Metabolic Endotoxemia Initiates Obesity and Insulin Resistance. Diabetes 56 (7), 1761–1772. doi: 10.2337/db06-1491
Cani, P. D., Bibiloni, R., Knauf, C., Waget, A., Neyrinck, A. M., Delzenne, N. M., et al. (2008). Changes in Gut Microbiota Control Metabolic Endotoxemia-Induced Inflammation in High-Fat Diet-Induced Obesity and Diabetes in Mice. Diabetes 57 (6), 1470–1481. doi: 10.2337/db07-1403
Cani, P. D., Possemiers, S., Van de Wiele, T., Guiot, Y., Everard, A., Rottier, O., et al. (2009). Changes in Gut Microbiota Control Inflammation in Obese Mice Through a Mechanism Involving GLP-2-Driven Improvement of Gut Permeability. Gut 58 (8), 1091–1103. doi: 10.1136/gut.2008.165886
Carnevale, R., Sciarretta, S., Valenti, V., di Nonno, F., Calvieri, C., Nocella, C., et al. (2020). Low-Grade Endotoxaemia Enhances Artery Thrombus Growth via Toll-Like Receptor 4: Implication for Myocardial Infarction. Eur. Heart J. 41 (33), 3156–3165. doi: 10.1093/eurheartj/ehz893
Cavagni, J., de Macedo, I. C., Gaio, E. J., Souza, A., de Molon, R. S., Cirelli, J. A., et al. (2016). Obesity and Hyperlipidemia Modulate Alveolar Bone Loss in Wistar Rats. J. Periodontol. 87 (2), e9–17. doi: 10.1902/jop.2015.150330
Cavagni, J., Wagner, T. P., Gaio, E. J., Rêgo, R. O., Torres, I. L., Rösing, C. K. (2013). Obesity may Increase the Occurrence of Spontaneous Periodontal Disease in Wistar Rats. Arch. Oral. Biol. 58 (8), 1034–1039. doi: 10.1016/j.archoralbio.2013.03.006
Chang, M. C., Tsai, Y. L., Chen, Y. W., Chan, C. P., Huang, C. F., Lan, W. C., et al. (2013). Butyrate Induces Reactive Oxygen Species Production and Affects Cell Cycle Progression in Human Gingival Fibroblasts. J. Periodontal. Res. 48 (1), 66–73. doi: 10.1111/j.1600-0765.2012.01504.x
Chang, M. C., Tsai, Y. L., Liou, E. J., Tang, C. M., Wang, T. M., Liu, H. C., et al. (2016). Effect of Butyrate on Collagen Expression, Cell Viability, Cell Cycle Progression and Related Proteins Expression of MG-63 Osteoblastic Cells. PloS One 11 (11), e0165438. doi: 10.1371/journal.pone.0165438
Chavakis, T., Mitroulis, I., Hajishengallis, G. (2019). Hematopoietic Progenitor Cells as Integrative Hubs for Adaptation to and Fine-Tuning of Inflammation. Nat. Immunol. 20 (7), 802–811. doi: 10.1038/s41590-019-0402-5
Chen, L. R., Chen, K. H. (2021). Utilization of Isoflavones in Soybeans for Women With Menopausal Syndrome: An Overview. Int. J. Mol. Sci. 22 (6), 3212. doi: 10.3390/ijms22063212
Chen, T. H., Chen, W. M., Hsu, K. H., Kuo, C. D., Hung, S. C. (2007). Sodium Butyrate Activates ERK to Regulate Differentiation of Mesenchymal Stem Cells. Biochem. Biophys. Res. Commun. 355 (4), 913–918. doi: 10.1016/j.bbrc.2007.02.057
Cheng, S., Qi, X., Ma, M., Zhang, L., Cheng, B., Liang, C., et al. (2020). Assessing the Relationship Between Gut Microbiota and Bone Mineral Density. Front. Genet. 11, 6. doi: 10.3389/fgene.2020.00006
Cho, Y. E., Kim, D. K., Seo, W., Gao, B., Yoo, S. H., Song, B. J. (2021). Fructose Promotes Leaky Gut, Endotoxemia, and Liver Fibrosis Through Ethanol-Inducible Cytochrome P450-2e1-Mediated Oxidative and Nitrative Stress. Hepatology 73 (6), 2180–2195. doi: 10.1002/hep.30652
Civitelli, R., Pilgram, T. K., Dotson, M., Muckerman, J., Lewandowski, N., Armamento-Villareal, R., et al. (2002). Alveolar and Postcranial Bone Density in Postmenopausal Women Receiving Hormone/Estrogen Replacement Therapy: A Randomized, Double-Blind, Placebo-Controlled Trial. Arch. Intern. Med. 162 (12), 1409–1415. doi: 10.1001/archinte.162.12.1409
Courtland, H. W., Sun, H., Beth-On, M., Wu, Y., Elis, S., Rosen, C. J., et al. (2011). Growth Hormone Mediates Pubertal Skeletal Development Independent of Hepatic IGF-1 Production. J. Bone Miner. Res. 26 (4), 761–768. doi: 10.1002/jbmr.265
Cummings, J. H., Pomare, E. W., Branch, W. J., Naylor, C. P., Macfarlane, G. T. (1987). Short Chain Fatty Acids in Human Large Intestine, Portal, Hepatic and Venous Blood. Gut 28 (10), 1221–1227. doi: 10.1136/gut.28.10.1221
Dalile, B., Van Oudenhove, L., Vervliet, B., Verbeke, K. (2019). The Role of Short-Chain Fatty Acids in Microbiota-Gut-Brain Communication. Nat. Rev. Gastroenterol. Hepatol. 16 (8), 461–478. doi: 10.1038/s41575-019-0157-3
Das, M., Cronin, O., Keohane, D. M., Cormac, E. M., Nugent, H., Nugent, M., et al. (2019). Gut Microbiota Alterations Associated With Reduced Bone Mineral Density in Older Adults. Rheumatol. (Oxford). 58 (12), 2295–2304. doi: 10.1093/rheumatology/kez302
Daughaday, W. H., Hall, K., Raben, M. S., Salmon, W. D., Jr., van den Brande, J. L., van Wyk, J. J. (1972). Somatomedin: Proposed Designation for Sulphation Factor. Nature 235 (5333), 107. doi: 10.1038/235107a0
Duarte, P. M., Gonçalves, P., Casati, M. Z., de Toledo, S., Sallum, E. A., Nociti, F. H., Jr. (2006). Estrogen and Alendronate Therapies may Prevent the Influence of Estrogen Deficiency on the Tooth-Supporting Alveolar Bone: A Histometric Study in Rats. J. Periodontal. Res. 41 (6), 541–546. doi: 10.1111/j.1600-0765.2006.00903.x
Dutzan, N., Kajikawa, T., Abusleme, L., Greenwell-Wild, T., Zuazo, C. E., Ikeuchi, T., et al. (2018). A Dysbiotic Microbiome Triggers T(H)17 Cells to Mediate Oral Mucosal Immunopathology in Mice and Humans. Sci. Transl. Med. 10 (463), eaat0797. doi: 10.1126/scitranslmed.aat0797
Eaimworawuthikul, S., Tunapong, W., Chunchai, T., Suntornsaratoon, P., Charoenphandhu, N., Thiennimitr, P., et al. (2020). Altered Gut Microbiota Ameliorates Bone Pathology in the Mandible of Obese-Insulin-Resistant Rats. Eur. J. Nutr. 59 (4), 1453–1462. doi: 10.1007/s00394-019-02002-8
Eaimworawuthikul, S., Tunapong, W., Chunchai, T., Yasom, S., Wanchai, K., Suntornsaratoon, P., et al. (2019). Effects of Probiotics, Prebiotics or Synbiotics on Jawbone in Obese-Insulin Resistant Rats. Eur. J. Nutr. 58 (7), 2801–2810. doi: 10.1007/s00394-018-1829-4
Eke, P. I., Dye, B. A., Wei, L., Slade, G. D., Thornton-Evans, G. O., Borgnakke, W. S., et al. (2015). Update on Prevalence of Periodontitis in Adults in the United States: NHANES 2009 to 2012. J. Periodontol. 86 (5), 611–622. doi: 10.1902/jop.2015.140520
Eke, P. I., Wei, L., Borgnakke, W. S., Thornton-Evans, G., Zhang, X., Lu, H., et al. (2016). Periodontitis Prevalence in Adults ≥ 65 Years of Age, in the USA. Periodontol. 2000. 72 (1), 76–95. doi: 10.1111/prd.12145
Eom, C. S., Lee, H. K., Ye, S., Park, S. M., Cho, K. H. (2012). Use of Selective Serotonin Reuptake Inhibitors and Risk of Fracture: A Systematic Review and Meta-Analysis. J. Bone Miner. Res. 27 (5), 1186–1195. doi: 10.1002/jbmr.1554
Evans, M., Murofushi, T., Tsuda, H., Mikami, Y., Zhao, N., Ochiai, K., et al. (2017). Combined Effects of Starvation and Butyrate on Autophagy-Dependent Gingival Epithelial Cell Death. J. Periodontal. Res. 52 (3), 522–531. doi: 10.1111/jre.12418
Faloni, A. P., Sasso-Cerri, E., Katchburian, E., Cerri, P. S. (2007). Decrease in the Number and Apoptosis of Alveolar Bone Osteoclasts in Estrogen-Treated Rats. J. Periodontal. Res. 42 (3), 193–201. doi: 10.1111/j.1600-0765.2006.00932.x
Fan, Y., Pedersen, O. (2021). Gut Microbiota in Human Metabolic Health and Disease. Nat. Rev. Microbiol. 19 (1), 55–71. doi: 10.1038/s41579-020-0433-9
Fei, N., Bruneau, A., Zhang, X., Wang, R., Wang, J., Rabot, S., et al. (2020). Endotoxin Producers Overgrowing in Human Gut Microbiota as the Causative Agents for Nonalcoholic Fatty Liver Disease. mBio 11 (1), e03263–19. doi: 10.1128/mBio.03263-19
Flores, R., Shi, J., Fuhrman, B., Xu, X., Veenstra, T. D., Gail, M. H., et al. (2012). Fecal Microbial Determinants of Fecal and Systemic Estrogens and Estrogen Metabolites: A Cross-Sectional Study. J. Transl. Med. 10, 253. doi: 10.1186/1479-5876-10-253
Frederick, J. R., Sarkar, J., McDowell, J. V., Marconi, R. T. (2011). Molecular Signaling Mechanisms of the Periopathogen, Treponema Denticola. J. Dent. Res. 90 (10), 1155–1163. doi: 10.1177/0022034511402994
Frost, G., Sleeth, M. L., Sahuri-Arisoylu, M., Lizarbe, B., Cerdan, S., Brody, L., et al. (2014). The Short-Chain Fatty Acid Acetate Reduces Appetite via a Central Homeostatic Mechanism. Nat. Commun. 5, 3611. doi: 10.1038/ncomms4611
Fuhrman, B. J., Feigelson, H. S., Flores, R., Gail, M. H., Xu, X., Ravel, J., et al. (2014). Associations of the Fecal Microbiome With Urinary Estrogens and Estrogen Metabolites in Postmenopausal Women. J. Clin. Endocrinol. Metab. 99 (12), 4632–4640. doi: 10.1210/jc.2014-2222
García-Gómez, E., González-Pedrajo, B., Camacho-Arroyo, I. (2013). Role of Sex Steroid Hormones in Bacterial-Host Interactions. BioMed. Res. Int. 2013, 928290. doi: 10.1155/2013/928290
Geirnaert, A., Calatayud, M., Grootaert, C., Laukens, D., Devriese, S., Smagghe, G., et al. (2017). Butyrate-Producing Bacteria Supplemented In Vitro to Crohn’s Disease Patient Microbiota Increased Butyrate Production and Enhanced Intestinal Epithelial Barrier Integrity. Sci. Rep. 7 (1), 11450. doi: 10.1038/s41598-017-11734-8
Genco, R. J., Borgnakke, W. S. (2013). Risk Factors for Periodontal Disease. Periodontol. 2000. 62 (1), 59–94. doi: 10.1111/j.1600-0757.2012.00457.x
Genco, R. J., Borgnakke, W. S. (2020). Diabetes as a Potential Risk for Periodontitis: Association Studies. Periodontol. 2000. 83 (1), 40–45. doi: 10.1111/prd.12270
Geng, S., Chen, K., Yuan, R., Peng, L., Maitra, U., Diao, N., et al. (2016). The Persistence of Low-Grade Inflammatory Monocytes Contributes to Aggravated Atherosclerosis. Nat. Commun. 7, 13436. doi: 10.1038/ncomms13436
Geng, J., Song, Q., Tang, X., Liang, X., Fan, H., Peng, H., et al. (2014). Co-Occurrence of Driver and Passenger Bacteria in Human Colorectal Cancer. Gut. Pathog. 6, 26. doi: 10.1186/1757-4749-6-26
Ge, N., Peng, J., Yu, L., Huang, S., Xu, L., Su, Y., et al. (2020). Orthodontic Treatment Induces Th17/Treg Cells to Regulate Tooth Movement in Rats With Periodontitis. Iran J. Basic. Med. Sci. 23 (10), 1315–1322. doi: 10.22038/ijbms.2020.44437.10419
Gevers, D., Kugathasan, S., Denson, L. A., Vázquez-Baeza, Y., Van Treuren, W., Ren, B., et al. (2014). The Treatment-Naive Microbiome in New-Onset Crohn’s Disease. Cell Host Microbe 15 (3), 382–392. doi: 10.1016/j.chom.2014.02.005
Glaser, D. L., Kaplan, F. S. (1997). Osteoporosis. Definition and Clinical Presentation. Spine (Phila. Pa. 1976). 22 (24 Suppl), 12s–16s. doi: 10.1097/00007632-199712151-00003
Gorgas, M. Q., Torres, F., Vives, R., Lopez-Rico, I., Capella, D., Pontes, C. (2021). Effects of Selective Serotonin Reuptake Inhibitors and Other Antidepressant Drugs on the Risk of Hip Fracture: A Case-Control Study in an Elderly Mediterranean Population. Eur. J. Hosp. Pharm. 28 (1), 28–32. doi: 10.1136/ejhpharm-2019-001893
Gray, J., Oehrle, K., Worthen, G., Alenghat, T., Whitsett, J., Deshmukh, H. (2017). Intestinal Commensal Bacteria Mediate Lung Mucosal Immunity and Promote Resistance of Newborn Mice to Infection. Sci. Transl. Med. 9 (376), eaaf9412. doi: 10.1126/scitranslmed.aaf9412
Guadamuro, L., Dohrmann, A. B., Tebbe, C. C., Mayo, B., Delgado, S. (2017). Bacterial Communities and Metabolic Activity of Faecal Cultures From Equol Producer and non-Producer Menopausal Women Under Treatment With Soy Isoflavones. BMC Microbiol. 17 (1), 93. doi: 10.1186/s12866-017-1001-y
Guan, Z., Jia, J., Zhang, C., Sun, T., Zhang, W., Yuan, W., et al. (2020). Gut Microbiome Dysbiosis Alleviates the Progression of Osteoarthritis in Mice. Clin. Sci. (Lond). 134 (23), 3159–3174. doi: 10.1042/CS20201224
Guan, X., Li, W., Meng, H. (2021). A Double-Edged Sword: Role of Butyrate in the Oral Cavity and the Gut. Mol. Oral. Microbiol. 36 (2), 121–131. doi: 10.1111/omi.12322
Guerra-Menéndez, L., Sádaba, M. C., Puche, J. E., Lavandera, J. L., de Castro, L. F., de Gortázar, A. R., et al. (2013). IGF-I Increases Markers of Osteoblastic Activity and Reduces Bone Resorption via Osteoprotegerin and RANK-Ligand. J. Transl. Med. 11, 271. doi: 10.1186/1479-5876-11-271
Gustafsson, B. I., Thommesen, L., Stunes, A. K., Tommeras, K., Westbroek, I., Waldum, H. L., et al. (2006). Serotonin and Fluoxetine Modulate Bone Cell Function In Vitro. J. Cell Biochem. 98 (1), 139–151. doi: 10.1002/jcb.20734
Hajishengallis, G. (2015). Periodontitis: From Microbial Immune Subversion to Systemic Inflammation. Nat. Rev. Immunol. 15 (1), 30–44. doi: 10.1038/nri3785
Hajishengallis, G. (2020). New Developments in Neutrophil Biology and Periodontitis. Periodontol. 2000. 82 (1), 78–92. doi: 10.1111/prd.12313
Hajishengallis, G., Chavakis, T. (2021). Local and Systemic Mechanisms Linking Periodontal Disease and Inflammatory Comorbidities. Nat. Rev. Immunol. 21 (7), 426–440. doi: 10.1038/s41577-020-00488-6
Hajishengallis, G., Chavakis, T., Hajishengallis, E., Lambris, J. D. (2015). Neutrophil Homeostasis and Inflammation: Novel Paradigms From Studying Periodontitis. J. Leukoc. Biol. 98 (4), 539–548. doi: 10.1189/jlb.3VMR1014-468R
Hajishengallis, E., Hajishengallis, G. (2014). Neutrophil Homeostasis and Periodontal Health in Children and Adults. J. Dent. Res. 93 (3), 231–237. doi: 10.1177/0022034513507956
Hathaway-Schrader, J. D., Poulides, N. A., Carson, M. D., Kirkpatrick, J. E., Warner, A. J., Swanson, B. A., et al. (2020). Specific Commensal Bacterium Critically Regulates Gut Microbiota Osteoimmunomodulatory Actions During Normal Postpubertal Skeletal Growth and Maturation. JBMR. Plus. 4 (3), e10338. doi: 10.1002/jbm4.10338
Hays, A., Duan, X., Zhu, J., Zhou, W., Upadhyayula, S., Shivde, J., et al. (2019). Down-Regulated Treg Cells in Exacerbated Periodontal Disease During Pregnancy. Int. Immunopharmacol. 69, 299–306. doi: 10.1016/j.intimp.2019.01.031
He, J., Xu, S., Zhang, B., Xiao, C., Chen, Z., Si, F., et al. (2020). Gut Microbiota and Metabolite Alterations Associated With Reduced Bone Mineral Density or Bone Metabolic Indexes in Postmenopausal Osteoporosis. Aging (Albany. NY). 12 (9), 8583–8604. doi: 10.18632/aging.103168
Holde, G. E., Oscarson, N., Trovik, T. A., Tillberg, A., Jönsson, B. (2017). Periodontitis Prevalence and Severity in Adults: A Cross-Sectional Study in Norwegian Circumpolar Communities. J. Periodontol. 88 (10), 1012–1022. doi: 10.1902/jop.2017.170164
Huang, Z., Chen, J., Li, B., Zeng, B., Chou, C. H., Zheng, X., et al. (2020). Faecal Microbiota Transplantation From Metabolically Compromised Human Donors Accelerates Osteoarthritis in Mice. Ann. Rheum. Dis. 79 (5), 646–656. doi: 10.1136/annrheumdis-2019-216471
Huang, Y., Mao, K., Chen, X., Sun, M. A., Kawabe, T., Li, W., et al. (2018). S1P-Dependent Interorgan Trafficking of Group 2 Innate Lymphoid Cells Supports Host Defense. Science 359 (6371), 114–119. doi: 10.1126/science.aam5809
Huck, O., Mulhall, H., Rubin, G., Kizelnik, Z., Iyer, R., Perpich, J. D., et al. (2020). Akkermansia Muciniphila Reduces Porphyromonas Gingivalis-Induced Inflammation and Periodontal Bone Destruction. J. Clin. Periodontol. 47 (2), 202–212. doi: 10.1111/jcpe.13214
Imai, Y., Youn, M. Y., Kondoh, S., Nakamura, T., Kouzmenko, A., Matsumoto, T., et al. (2009). Estrogens Maintain Bone Mass by Regulating Expression of Genes Controlling Function and Life Span in Mature Osteoclasts. Ann. N. Y. Acad. Sci. 1173 (Suppl 1), E31–E39. doi: 10.1111/j.1749-6632.2009.04954.x
Irie, K., Tomofuji, T., Ekuni, D., Fukuhara, D., Uchida, Y., Kataoka, K., et al. (2018). Age-Related Changes of CD4(+) T Cell Migration and Cytokine Expression in Germ-Free and SPF Mice Periodontium. Arch. Oral. Biol. 87, 72–78. doi: 10.1016/j.archoralbio.2017.12.007
Iwami, K., Moriyama, T. (1993). Effects of Short Chain Fatty Acid, Sodium Butyrate, on Osteoblastic Cells and Osteoclastic Cells. Int. J. Biochem. 25 (11), 1631–1635. doi: 10.1016/0020-711X(93)90522-G
Jakobsdottir, G., Jädert, C., Holm, L., Nyman, M. E. (2013). Propionic and Butyric Acids, Formed in the Caecum of Rats Fed Highly Fermentable Dietary Fibre, are Reflected in Portal and Aortic Serum. Br. J. Nutr. 110 (9), 1565–1572. doi: 10.1017/S0007114513000809
Jia, X., Jia, L., Mo, L., Yuan, S., Zheng, X., He, J., et al. (2019). Berberine Ameliorates Periodontal Bone Loss by Regulating Gut Microbiota. J. Dent. Res. 98 (1), 107–116. doi: 10.1177/0022034518797275
Jiang, T., Gao, X., Wu, C., Tian, F., Lei, Q., Bi, J., et al. (2016). Apple-Derived Pectin Modulates Gut Microbiota, Improves Gut Barrier Function, and Attenuates Metabolic Endotoxemia in Rats With Diet-Induced Obesity. Nutrients 8 (3), 126. doi: 10.3390/nu8030126
Jia, L., Tu, Y., Jia, X., Du, Q., Zheng, X., Yuan, Q., et al. (2021). Probiotics Ameliorate Alveolar Bone Loss by Regulating Gut Microbiota. Cell Prolif. 54(7), e13075. doi: 10.1111/cpr.13075
Kamada, N., Seo, S. U., Chen, G. Y., Núñez, G. (2013). Role of the Gut Microbiota in Immunity and Inflammatory Disease. Nat. Rev. Immunol. 13 (5), 321–335. doi: 10.1038/nri3430
Karaguzel, G., Holick, M. F. (2010). Diagnosis and Treatment of Osteopenia. Rev. Endocr. Metab. Disord. 11 (4), 237–251. doi: 10.1007/s11154-010-9154-0
Kasahara, K., Krautkramer, K. A., Org, E., Romano, K. A., Kerby, R. L., Vivas, E. I., et al. (2018). Interactions Between Roseburia Intestinalis and Diet Modulate Atherogenesis in a Murine Model. Nat. Microbiol. 3 (12), 1461–1471. doi: 10.1038/s41564-018-0272-x
Kaufmann, E., Sanz, J., Dunn, J. L., Khan, N., Mendonça, L. E., Pacis, A., et al. (2018). BCG Educates Hematopoietic Stem Cells to Generate Protective Innate Immunity Against Tuberculosis. Cell 172 (1-2), 176–190.e119. doi: 10.1016/j.cell.2017.12.031
Kawai, T., Matsuyama, T., Hosokawa, Y., Makihira, S., Seki, M., Karimbux, N. Y., et al. (2006). B and T Lymphocytes are the Primary Sources of RANKL in the Bone Resorptive Lesion of Periodontal Disease. Am. J. Pathol. 169 (3), 987–998. doi: 10.2353/ajpath.2006.060180
Kerbage, H., Bahadori, S., Léger, J., Carel, J. C., Purper Ouakil, D. (2014). [Effect of SSRIs on Bone Metabolism]. Encephale 40 (1), 56–61. doi: 10.1016/j.encep.2013.04.007
Khosla, S. (2020). Estrogen Versus FSH Effects on Bone Metabolism: Evidence From Interventional Human Studies. Endocrinology 161 (8), bqaa111. doi: 10.1210/endocr/bqaa111
Kimura, I., Miyamoto, J., Ohue-Kitano, R., Watanabe, K., Yamada, T., Onuki, M., et al. (2020). Maternal Gut Microbiota in Pregnancy Influences Offspring Metabolic Phenotype in Mice. Science 367 (6481), eaaw8429. doi: 10.1126/science.aaw8429
Kitamoto, S., Nagao-Kitamoto, H., Hein, R., Schmidt, T. M., Kamada, N. (2020a). The Bacterial Connection Between the Oral Cavity and the Gut Diseases. J. Dent. Res. 99 (9), 1021–1029. doi: 10.1177/0022034520924633
Kitamoto, S., Nagao-Kitamoto, H., Jiao, Y., Gillilland, M. G., 3rd, Hayashi, A., Imai, J., et al. (2020b). The Intermucosal Connection Between the Mouth and Gut in Commensal Pathobiont-Driven Colitis. Cell 182 (2), 447–462.e414. doi: 10.1016/j.cell.2020.05.048
Koh, A., De Vadder, F., Kovatcheva-Datchary, P., Bäckhed, F. (2016). From Dietary Fiber to Host Physiology: Short-Chain Fatty Acids as Key Bacterial Metabolites. Cell 165 (6), 1332–1345. doi: 10.1016/j.cell.2016.05.041
Komatsu, N., Okamoto, K., Sawa, S., Nakashima, T., Oh-hora, M., Kodama, T., et al. (2014). Pathogenic Conversion of Foxp3+ T Cells Into TH17 Cells in Autoimmune Arthritis. Nat. Med. 20 (1), 62–68. doi: 10.1038/nm.3432
Krebs, C. F., Paust, H. J., Krohn, S., Koyro, T., Brix, S. R., Riedel, J. H., et al. (2016). Autoimmune Renal Disease Is Exacerbated by S1P-Receptor-1-Dependent Intestinal Th17 Cell Migration to the Kidney. Immunity 45 (5), 1078–1092. doi: 10.1016/j.immuni.2016.10.020
Kuhn, K. A., Morrison, T. E. (2020). Introduction: Evolution of Inflammatory Arthritis From Innate to Adaptive Immune Mechanisms. Immunol. Rev. 294 (1), 5–8. doi: 10.1111/imr.12843
Kurita-Ochiai, T., Ochiai, K., Suzuki, N., Otsuka, K., Fukushima, K. (2002). Human Gingival Fibroblasts Rescue Butyric Acid-Induced T-Cell Apoptosis. Infect. Immun. 70 (5), 2361–2367. doi: 10.1128/IAI.70.5.2361-2367.2002
Kurita-Ochiai, T., Seto, S., Suzuki, N., Yamamoto, M., Otsuka, K., Abe, K., et al. (2008). Butyric Acid Induces Apoptosis in Inflamed Fibroblasts. J. Dent. Res. 87 (1), 51–55. doi: 10.1177/154405910808700108
Ladinsky, M. S., Araujo, L. P., Zhang, X., Veltri, J., Galan-Diez, M., Soualhi, S., et al. (2019). Endocytosis of Commensal Antigens by Intestinal Epithelial Cells Regulates Mucosal T Cell Homeostasis. Science 363 (6431), eaat4042. doi: 10.1126/science.aat4042
Lalla, E., Papapanou, P. N. (2011). Diabetes Mellitus and Periodontitis: A Tale of Two Common Interrelated Diseases. Nat. Rev. Endocrinol. 7 (12), 738–748. doi: 10.1038/nrendo.2011.106
Leder, B. (2007). Gonadal Steroids and Bone Metabolism in Men. Curr. Opin. Endocrinol. Diabetes Obes. 14 (3), 241–246. doi: 10.1097/MED.0b013e32814db87b
Lee, J., d’Aigle, J., Atadja, L., Quaicoe, V., Honarpisheh, P., Ganesh, B. P., et al. (2020). Gut Microbiota-Derived Short-Chain Fatty Acids Promote Poststroke Recovery in Aged Mice. Circ. Res. 127 (4), 453–465. doi: 10.1161/CIRCRESAHA.119.316448
Lee, E., Lee, S. W. (2019). Prevalence of Periodontitis and its Association With Reduced Pulmonary Function: Results From the Korean National Health and Nutrition Examination Survey. Med. (Kaunas). 55 (9), 581. doi: 10.3390/medicina55090581
Li, J. Y., Chassaing, B., Tyagi, A. M., Vaccaro, C., Luo, T., Adams, J., et al. (2016). Sex Steroid Deficiency-Associated Bone Loss is Microbiota Dependent and Prevented by Probiotics. J. Clin. Invest. 126 (6), 2049–2063. doi: 10.1172/JCI86062
Li, J., Lin, S., Vanhoutte, P. M., Woo, C. W., Xu, A. (2016). Akkermansia Muciniphila Protects Against Atherosclerosis by Preventing Metabolic Endotoxemia-Induced Inflammation in Apoe-/- Mice. Circulation 133 (24), 2434–2446. doi: 10.1161/CIRCULATIONAHA.115.019645
Li, Y., Lu, Z., Zhang, X., Yu, H., Kirkwood, K. L., Lopes-Virella, M. F., et al. (2015). Metabolic Syndrome Exacerbates Inflammation and Bone Loss in Periodontitis. J. Dent. Res. 94 (2), 362–370. doi: 10.1177/0022034514561658
Liu, J., Wang, Y., Meng, H., Yu, J., Lu, H., Li, W., et al. (2019). Butyrate Rather Than LPS Subverts Gingival Epithelial Homeostasis by Downregulation of Intercellular Junctions and Triggering Pyroptosis. J. Clin. Periodontol. 46 (9), 894–907. doi: 10.1111/jcpe.13162
Li, G., Xie, C., Lu, S., Nichols, R. G., Tian, Y., Li, L., et al. (2017). Intermittent Fasting Promotes White Adipose Browning and Decreases Obesity by Shaping the Gut Microbiota. Cell Metab. 26 (4), 672–685.e674. doi: 10.1016/j.cmet.2017.08.019
Lucas, S., Omata, Y., Hofmann, J., Böttcher, M., Iljazovic, A., Sarter, K., et al. (2018). Short-Chain Fatty Acids Regulate Systemic Bone Mass and Protect From Pathological Bone Loss. Nat. Commun. 9 (1), 55. doi: 10.1038/s41467-017-02490-4
Lu, R., Meng, H., Gao, X., Xu, L., Feng, X. (2014). Effect of non-Surgical Periodontal Treatment on Short Chain Fatty Acid Levels in Gingival Crevicular Fluid of Patients With Generalized Aggressive Periodontitis. J. Periodontal. Res. 49 (5), 574–583. doi: 10.1111/jre.12137
Macari, S., Madeira, M. F. M., Lima, I. L. A., Pereira, T. S. F., Dias, G. J., Cirelli, J. A., et al. (2018). ST2 Regulates Bone Loss in a Site-Dependent and Estrogen-Dependent Manner. J. Cell Biochem. 119 (10), 8511–8521. doi: 10.1002/jcb.27080
Macfarlane, S., Macfarlane, G. T. (2003). Regulation of Short-Chain Fatty Acid Production. Proc. Nutr. Soc. 62 (1), 67–72. doi: 10.1079/PNS2002207
Magrin, G. L., Strauss, F. J., Benfatti, C. A. M., Maia, L. C., Gruber, R. (2020). Effects of Short-Chain Fatty Acids on Human Oral Epithelial Cells and the Potential Impact on Periodontal Disease: A Systematic Review of In Vitro Studies. Int. J. Mol. Sci. 21 (14), 4895. doi: 10.3390/ijms21144895
Mandić, A. D., Woting, A., Jaenicke, T., Sander, A., Sabrowski, W., Rolle-Kampcyk, U., et al. (2019). Clostridium Ramosum Regulates Enterochromaffin Cell Development and Serotonin Release. Sci. Rep. 9 (1), 1177. doi: 10.1038/s41598-018-38018-z
Markle, J. G., Frank, D. N., Mortin-Toth, S., Robertson, C. E., Feazel, L. M., Rolle-Kampczyk, U., et al. (2013). Sex Differences in the Gut Microbiome Drive Hormone-Dependent Regulation of Autoimmunity. Science 339 (6123), 1084–1088. doi: 10.1126/science.1233521
Mathies, F., Steffens, N., Kleinschmidt, D., Stuhlmann, F., Huber, F. J., Roy, U., et al. (2018). Colitis Promotes a Pathological Condition of the Liver in the Absence of Foxp3(+) Regulatory T Cells. J. Immunol. 201 (12), 3558–3568. doi: 10.4049/jimmunol.1800711
Miller, A. A., Kurschel, E., Osieka, R., Schmidt, C. G. (1987). Clinical Pharmacology of Sodium Butyrate in Patients With Acute Leukemia. Eur. J. Cancer Clin. Oncol. 23 (9), 1283–1287. doi: 10.1016/0277-5379(87)90109-X
Mitroulis, I., Ruppova, K., Wang, B., Chen, L. S., Grzybek, M., Grinenko, T., et al. (2018). Modulation of Myelopoiesis Progenitors Is an Integral Component of Trained Immunity. Cell 172 (1-2), 147–161.e112. doi: 10.1016/j.cell.2017.11.034
Monasterio, G., Castillo, F., Ibarra, J. P., Guevara, J., Rojas, L., Alvarez, C., et al. (2018). Alveolar Bone Resorption and Th1/Th17-Associated Immune Response Triggered During Aggregatibacter Actinomycetemcomitans-Induced Experimental Periodontitis are Serotype-Dependent. J. Periodontol. 89 (10), 1249–1261. doi: 10.1002/JPER.17-0563
Morozumi, A. (2011). High Concentration of Sodium Butyrate Suppresses Osteoblastic Differentiation and Mineralized Nodule Formation in ROS17/2.8 Cells. J. Oral. Sci. 53 (4), 509–516. doi: 10.2334/josnusd.53.509
Morrison, D. J., Preston, T. (2016). Formation of Short Chain Fatty Acids by the Gut Microbiota and Their Impact on Human Metabolism. Gut. Microbes 7 (3), 189–200. doi: 10.1080/19490976.2015.1134082
Morton, A. M., Sefik, E., Upadhyay, R., Weissleder, R., Benoist, C., Mathis, D. (2014). Endoscopic Photoconversion Reveals Unexpectedly Broad Leukocyte Trafficking to and From the Gut. Proc. Natl. Acad. Sci. U. S. A. 111 (18), 6696–6701. doi: 10.1073/pnas.1405634111
Mulhall, H., DiChiara, J. M., Deragon, M., Iyer, R., Huck, O., Amar, S. (2020). Akkermansia Muciniphila and Its Pili-Like Protein Amuc_1100 Modulate Macrophage Polarization in Experimental Periodontitis. Infect. Immun. 89 (1), e00500–20. doi: 10.1128/IAI.00500-20
Neal, M. D., Leaphart, C., Levy, R., Prince, J., Billiar, T. R., Watkins, S., et al. (2006). Enterocyte TLR4 Mediates Phagocytosis and Translocation of Bacteria Across the Intestinal Barrier. J. Immunol. 176 (5), 3070–3079. doi: 10.4049/jimmunol.176.5.3070
Netea, M. G., Domínguez-Andrés, J., Barreiro, L. B., Chavakis, T., Divangahi, M., Fuchs, E., et al. (2020). Defining Trained Immunity and its Role in Health and Disease. Nat. Rev. Immunol. 20 (6), 375–388. doi: 10.1038/s41577-020-0285-6
Netea, M. G., Joosten, L. A., Latz, E., Mills, K. H., Natoli, G., Stunnenberg, H. G., et al. (2016). Trained Immunity: A Program of Innate Immune Memory in Health and Disease. Science 352 (6284), aaf1098. doi: 10.1126/science.aaf1098
Niederman, R., Buyle-Bodin, Y., Lu, B. Y., Robinson, P., Naleway, C. (1997). Short-Chain Carboxylic Acid Concentration in Human Gingival Crevicular Fluid. J. Dent. Res. 76 (1), 575–579. doi: 10.1177/00220345970760010801
Ni, J. J., Yang, X. L., Zhang, H., Xu, Q., Wei, X. T., Feng, G. J., et al. (2021). Assessing Causal Relationship From Gut Microbiota to Heel Bone Mineral Density. Bone 143, 115652. doi: 10.1016/j.bone.2020.115652
Novince, C. M., Whittow, C. R., Aartun, J. D., Hathaway, J. D., Poulides, N., Chavez, M. B., et al. (2017). Commensal Gut Microbiota Immunomodulatory Actions in Bone Marrow and Liver Have Catabolic Effects on Skeletal Homeostasis in Health. Sci. Rep. 7 (1), 5747. doi: 10.1038/s41598-017-06126-x
Ohlrich, E. J., Cullinan, M. P., Seymour, G. J. (2009). The Immunopathogenesis of Periodontal Disease. Aust. Dent. J. 54 Suppl 1, S2–10. doi: 10.1111/j.1834-7819.2009.01139.x
Ohlsson, C., Nigro, G., Boneca, I. G., Bäckhed, F., Sansonetti, P., Sjögren, K. (2017). Regulation of Bone Mass by the Gut Microbiota is Dependent on NOD1 and NOD2 Signaling. Cell Immunol. 317, 55–58. doi: 10.1016/j.cellimm.2017.05.003
Omenetti, S., Bussi, C., Metidji, A., Iseppon, A., Lee, S., Tolaini, M., et al. (2019). The Intestine Harbors Functionally Distinct Homeostatic Tissue-Resident and Inflammatory Th17 Cells. Immunity 51 (1), 77–89.e76. doi: 10.1016/j.immuni.2019.05.004
Ozaki, D., Kubota, R., Maeno, T., Abdelhakim, M., Hitosugi, N. (2021). Association Between Gut Microbiota, Bone Metabolism, and Fracture Risk in Postmenopausal Japanese Women. Osteoporos. Int. 32 (1), 145–156. doi: 10.1007/s00198-020-05728-y
Palioto, D. B., Finoti, L. S., Kinane, D. F., Benakanakere, M. (2019). Epigenetic and Inflammatory Events in Experimental Periodontitis Following Systemic Microbial Challenge. J. Clin. Periodontol. 46 (8), 819–829. doi: 10.1111/jcpe.13151
Papageorgiou, S. N., Hagner, M., Nogueira, A. V., Franke, A., Jäger, A., Deschner, J. (2017). Inflammatory Bowel Disease and Oral Health: Systematic Review and a Meta-Analysis. J. Clin. Periodontol. 44 (4), 382–393. doi: 10.1111/jcpe.12698
Pelletier, M., Maggi, L., Micheletti, A., Lazzeri, E., Tamassia, N., Costantini, C., et al. (2010). Evidence for a Cross-Talk Between Human Neutrophils and Th17 Cells. Blood 115 (2), 335–343. doi: 10.1182/blood-2009-04-216085
Penoni, D. C., Fidalgo, T. K., Torres, S. R., Varela, V. M., Masterson, D., Leão, A. T., et al. (2017). Bone Density and Clinical Periodontal Attachment in Postmenopausal Women: A Systematic Review and Meta-Analysis. J. Dent. Res. 96 (3), 261–269. doi: 10.1177/0022034516682017
Perez-Pardo, P., Dodiya, H. B., Engen, P. A., Forsyth, C. B., Huschens, A. M., Shaikh, M., et al. (2019). Role of TLR4 in the Gut-Brain Axis in Parkinson’s Disease: A Translational Study From Men to Mice. Gut 68 (5), 829–843. doi: 10.1136/gutjnl-2018-316844
Pittayanon, R., Lau, J. T., Yuan, Y., Leontiadis, G. I., Tse, F., Surette, M., et al. (2019). Gut Microbiota in Patients With Irritable Bowel Syndrome-A Systematic Review. Gastroenterology 157 (1), 97–108. doi: 10.1053/j.gastro.2019.03.049
Plottel, C. S., Blaser, M. J. (2011). Microbiome and Malignancy. Cell Host Microbe 10 (4), 324–335. doi: 10.1016/j.chom.2011.10.003
Porwal, K., Pal, S., Kulkarni, C., Singh, P., Sharma, S., Singh, P., et al. (2020). A Prebiotic, Short-Chain Fructo-Oligosaccharides Promotes Peak Bone Mass and Maintains Bone Mass in Ovariectomized Rats by an Osteogenic Mechanism. BioMed. Pharmacother. 129, 110448. doi: 10.1016/j.biopha.2020.110448
Rahman, M. M., Kukita, A., Kukita, T., Shobuike, T., Nakamura, T., Kohashi, O. (2003). Two Histone Deacetylase Inhibitors, Trichostatin A and Sodium Butyrate, Suppress Differentiation Into Osteoclasts But Not Into Macrophages. Blood 101 (9), 3451–3459. doi: 10.1182/blood-2002-08-2622
Ramasamy, B., Magne, F., Tripathy, S. K., Venugopal, G., Mukherjee, D., Balamurugan, R. (2021). Association of Gut Microbiome and Vitamin D Deficiency in Knee Osteoarthritis Patients: A Pilot Study. Nutrients 13 (4), 1272. doi: 10.3390/nu13041272
Reigstad, C. S., Salmonson, C. E., Rainey, J. F., 3rd, Szurszewski, J. H., Linden, D. R., Sonnenburg, J. L., et al. (2015). Gut Microbes Promote Colonic Serotonin Production Through an Effect of Short-Chain Fatty Acids on Enterochromaffin Cells. FASEB J. 29 (4), 1395–1403. doi: 10.1096/fj.14-259598
Reynolds, M. A. (2014). Modifiable Risk Factors in Periodontitis: At the Intersection of Aging and Disease. Periodontol. 2000. 64 (1), 7–19. doi: 10.1111/prd.12047
Rho, J., Takami, M., Choi, Y. (2004). Osteoimmunology: Interactions of the Immune and Skeletal Systems. Mol. Cells 17 (1), 1–9. doi: 10.14348/.1970.0.0
Ridlon, J. M., Ikegawa, S., Alves, J. M., Zhou, B., Kobayashi, A., Iida, T., et al. (2013). Clostridium Scindens: A Human Gut Microbe With a High Potential to Convert Glucocorticoids Into Androgens. J. Lipid Res. 54 (9), 2437–2449. doi: 10.1194/jlr.M038869
Rios-Arce, N. D., Schepper, J. D., Dagenais, A., Schaefer, L., Daly-Seiler, C. S., Gardinier, J. D., et al. (2020). Post-Antibiotic Gut Dysbiosis-Induced Trabecular Bone Loss is Dependent on Lymphocytes. Bone 134, 115269. doi: 10.1016/j.bone.2020.115269
Rizzetto, L., Fava, F., Tuohy, K. M., Selmi, C. (2018). Connecting the Immune System, Systemic Chronic Inflammation and the Gut Microbiome: The Role of Sex. J. Autoimmun. 92, 12–34. doi: 10.1016/j.jaut.2018.05.008
Rooks, M. G., Garrett, W. S. (2016). Gut Microbiota, Metabolites and Host Immunity. Nat. Rev. Immunol. 16 (6), 341–352. doi: 10.1038/nri.2016.42
Rosenbaum, M., Knight, R., Leibel, R. L. (2015). The Gut Microbiota in Human Energy Homeostasis and Obesity. Trends Endocrinol. Metab. 26 (9), 493–501. doi: 10.1016/j.tem.2015.07.002
Scapini, P., Cassatella, M. A. (2014). Social Networking of Human Neutrophils Within the Immune System. Blood 124 (5), 710–719. doi: 10.1182/blood-2014-03-453217
Schepper, J. D., Collins, F., Rios-Arce, N. D., Kang, H. J., Schaefer, L., Gardinier, J. D., et al. (2020). Involvement of the Gut Microbiota and Barrier Function in Glucocorticoid-Induced Osteoporosis. J. Bone Miner. Res. 35 (4), 801–820. doi: 10.1002/jbmr.3947
Schieber, A. M., Lee, Y. M., Chang, M. W., Leblanc, M., Collins, B., Downes, M., et al. (2015). Disease Tolerance Mediated by Microbiome E. Coli Involves Inflammasome and IGF-1 Signaling. Science 350 (6260), 558–563. doi: 10.1126/science.aac6468
Schloss, M. J., Swirski, F. K., Nahrendorf, M. (2020). Modifiable Cardiovascular Risk, Hematopoiesis, and Innate Immunity. Circ. Res. 126 (9), 1242–1259. doi: 10.1161/CIRCRESAHA.120.315936
Schmidt, T., Schwinge, D., Rolvien, T., Jeschke, A., Schmidt, C., Neven, M., et al. (2019). Th17 Cell Frequency Is Associated With Low Bone Mass in Primary Sclerosing Cholangitis. J. Hepatol. 70 (5), 941–953. doi: 10.1016/j.jhep.2018.12.035
She, Y. Y., Kong, X. B., Ge, Y. P., Liu, Z. Y., Chen, J. Y., Jiang, J. W., et al. (2020). Periodontitis and Inflammatory Bowel Disease: A Meta-Analysis. BMC Oral. Health 20 (1), 67. doi: 10.1186/s12903-020-1053-5
Shin, S. C., Kim, S. H., You, H., Kim, B., Kim, A. C., Lee, K. A., et al. (2011). Drosophila Microbiome Modulates Host Developmental and Metabolic Homeostasis via Insulin Signaling. Science 334 (6056), 670–674. doi: 10.1126/science.1212782
Shirasugi, M., Nakagawa, M., Nishioka, K., Yamamoto, T., Nakaya, T., Kanamura, N. (2018). Relationship Between Periodontal Disease and Butyric Acid Produced by Periodontopathic Bacteria. Inflamm Regener. 38, 23. doi: 10.1186/s41232-018-0081-x
Shirasugi, M., Nishioka, K., Yamamoto, T., Nakaya, T., Kanamura, N. (2017). Normal Human Gingival Fibroblasts Undergo Cytostasis and Apoptosis After Long-Term Exposure to Butyric Acid. Biochem. Biophys. Res. Commun. 482 (4), 1122–1128. doi: 10.1016/j.bbrc.2016.11.168
Silva, N., Abusleme, L., Bravo, D., Dutzan, N., Garcia-Sesnich, J., Vernal, R., et al. (2015). Host Response Mechanisms in Periodontal Diseases. J. Appl. Oral. Sci. 23 (3), 329–355. doi: 10.1590/1678-775720140259
Sjögren, K., Engdahl, C., Henning, P., Lerner, U. H., Tremaroli, V., Lagerquist, M. K., et al. (2012). The Gut Microbiota Regulates Bone Mass in Mice. J. Bone Miner. Res. 27 (6), 1357–1367. doi: 10.1002/jbmr.1588
Sokol, C. L., Luster, A. D. (2015). The Chemokine System in Innate Immunity. Cold Spring Harb. Perspect. Biol. 7 (5), a016303. doi: 10.1101/cshperspect.a016303
Song, H., Chu, Q., Yan, F., Yang, Y., Han, W., Zheng, X. (2016). Red Pitaya Betacyanins Protects From Diet-Induced Obesity, Liver Steatosis and Insulin Resistance in Association With Modulation of Gut Microbiota in Mice. J. Gastroenterol. Hepatol. 31 (8), 1462–1469. doi: 10.1111/jgh.13278
Song, C. H., Kim, N., Sohn, S. H., Lee, S. M., Nam, R. H., Na, H. Y., et al. (2018). Effects of 17β-Estradiol on Colonic Permeability and Inflammation in an Azoxymethane/Dextran Sulfate Sodium-Induced Colitis Mouse Model. Gut. Liver. 12 (6), 682–693. doi: 10.5009/gnl18221
Stockinger, B., Omenetti, S. (2017). The Dichotomous Nature of T Helper 17 Cells. Nat. Rev. Immunol. 17 (9), 535–544. doi: 10.1038/nri.2017.50
Suvan, J. E., Finer, N., D’Aiuto, F. (2018). Periodontal Complications With Obesity. Periodontol. 2000. 78 (1), 98–128. doi: 10.1111/prd.12239
Syed, F., Khosla, S. (2005). Mechanisms of Sex Steroid Effects on Bone. Biochem. Biophys. Res. Commun. 328 (3), 688–696. doi: 10.1016/j.bbrc.2004.11.097
Tahimic, C. G., Wang, Y., Bikle, D. D. (2013). Anabolic Effects of IGF-1 Signaling on the Skeleton. Front. Endocrinol. (Lausanne). 4, 6. doi: 10.3389/fendo.2013.00006
Titus, E., Ahearn, G. A. (1988). Short-Chain Fatty Acid Transport in the Intestine of a Herbivorous Teleost. J. Exp. Biol. 135, 77–94. doi: 10.1242/jeb.135.1.77
Tomura, M., Yoshida, N., Tanaka, J., Karasawa, S., Miwa, Y., Miyawaki, A., et al. (2008). Monitoring Cellular Movement In Vivo With Photoconvertible Fluorescence Protein “Kaede” Transgenic Mice. Proc. Natl. Acad. Sci. U. S. A. 105 (31), 10871–10876. doi: 10.1073/pnas.0802278105
Tsuda, H., Ochiai, K., Suzuki, N., Otsuka, K. (2010). Butyrate, a Bacterial Metabolite, Induces Apoptosis and Autophagic Cell Death in Gingival Epithelial Cells. J. Periodontal. Res. 45 (5), 626–634. doi: 10.1111/j.1600-0765.2010.01277.x
Tsukasaki, M., Komatsu, N., Nagashima, K., Nitta, T., Pluemsakunthai, W., Shukunami, C., et al. (2018). Host Defense Against Oral Microbiota by Bone-Damaging T Cells. Nat. Commun. 9 (1), 701. doi: 10.1038/s41467-018-03147-6
Turner, J. E., Paust, H. J., Steinmetz, O. M., Peters, A., Riedel, J. H., Erhardt, A., et al. (2010). CCR6 Recruits Regulatory T Cells and Th17 Cells to the Kidney in Glomerulonephritis. J. Am. Soc. Nephrol. 21 (6), 974–985. doi: 10.1681/ASN.2009070741
Tyagi, A. M., Darby, T. M., Hsu, E., Yu, M., Pal, S., Dar, H., et al. (2021). The Gut Microbiota Is a Transmissible Determinant of Skeletal Maturation. Elife 10, e64237. doi: 10.7554/eLife.64237
Uchida, Y., Irie, K., Fukuhara, D., Kataoka, K., Hattori, T., Ono, M., et al. (2018). Commensal Microbiota Enhance Both Osteoclast and Osteoblast Activities. Molecules 23 (7), 1517. doi: 10.3390/molecules23071517
Vincent, A. D., Wang, X. Y., Parsons, S. P., Khan, W. I., Huizinga, J. D. (2018). Abnormal Absorptive Colonic Motor Activity in Germ-Free Mice is Rectified by Butyrate, an Effect Possibly Mediated by Mucosal Serotonin. Am. J. Physiol. Gastrointest. Liver. Physiol. 315 (5), G896–g907. doi: 10.1152/ajpgi.00237.2017
Virto, L., Cano, P., Jiménez-Ortega, V., Fernández-Mateos, P., González, J., Esquifino, A. I., et al. (2018). Obesity and Periodontitis: An Experimental Study to Evaluate Periodontal and Systemic Effects of Comorbidity. J. Periodontol. 89 (2), 176–185. doi: 10.1902/jop.2017.170355
Wang, Y., Bikle, D. D., Chang, W. (2013). Autocrine and Paracrine Actions of IGF-I Signaling in Skeletal Development. Bone Res. 1 (3), 249–259. doi: 10.4248/BR201303003
Wang, Y., Nishida, S., Boudignon, B. M., Burghardt, A., Elalieh, H. Z., Hamilton, M. M., et al. (2007). IGF-I Receptor is Required for the Anabolic Actions of Parathyroid Hormone on Bone. J. Bone Miner. Res. 22 (9), 1329–1337. doi: 10.1359/jbmr.070517
Wang, Y., Nishida, S., Elalieh, H. Z., Long, R. K., Halloran, B. P., Bikle, D. D. (2006). Role of IGF-I Signaling in Regulating Osteoclastogenesis. J. Bone Miner. Res. 21 (9), 1350–1358. doi: 10.1359/jbmr.060610
Wang, J., Wang, Y., Gao, W., Wang, B., Zhao, H., Zeng, Y., et al. (2017). Diversity Analysis of Gut Microbiota in Osteoporosis and Osteopenia Patients. PeerJ 5, e3450. doi: 10.7717/peerj.3450
Warden, S. J., Nelson, I. R., Fuchs, R. K., Bliziotes, M. M., Turner, C. H. (2008). Serotonin (5-Hydroxytryptamine) Transporter Inhibition Causes Bone Loss in Adult Mice Independently of Estrogen Deficiency. Menopause 15 (6), 1176–1183. doi: 10.1097/gme.0b013e318173566b
Warden, S. J., Robling, A. G., Sanders, M. S., Bliziotes, M. M., Turner, C. H. (2005). Inhibition of the Serotonin (5-Hydroxytryptamine) Transporter Reduces Bone Accrual During Growth. Endocrinology 146 (2), 685–693. doi: 10.1210/en.2004-1259
Weitzmann, M. N., Pacifici, R. (2006). Estrogen Deficiency and Bone Loss: An Inflammatory Tale. J. Clin. Invest. 116 (5), 1186–1194. doi: 10.1172/JCI28550
Wu, Q., Bencaz, A. F., Hentz, J. G., Crowell, M. D. (2012). Selective Serotonin Reuptake Inhibitor Treatment and Risk of Fractures: A Meta-Analysis of Cohort and Case-Control Studies. Osteoporos. Int. 23 (1), 365–375. doi: 10.1007/s00198-011-1778-8
Xie, J., Wang, Z., Wang, W. (2020). Semaphorin 4d Induces an Imbalance of Th17/Treg Cells by Activating the Aryl Hydrocarbon Receptor in Ankylosing Spondylitis. Front. Immunol. 11, 2151. doi: 10.3389/fimmu.2020.02151
Xu, F., Guanghao, C., Liang, Y., Jun, W., Wei, W., Baorong, H. (2019). Treg-Promoted New Bone Formation Through Suppressing TH17 by Secreting Interleukin-10 in Ankylosing Spondylitis. Spine (Phila. Pa. 1976). 44 (23), E1349–e1355. doi: 10.1097/BRS.0000000000003169
Yadav, V. K., Ryu, J. H., Suda, N., Tanaka, K. F., Gingrich, J. A., Schütz, G., et al. (2008). Lrp5 Controls Bone Formation by Inhibiting Serotonin Synthesis in the Duodenum. Cell 135 (5), 825–837. doi: 10.1016/j.cell.2008.09.059
Yakar, S., Canalis, E., Sun, H., Mejia, W., Kawashima, Y., Nasser, P., et al. (2009). Serum IGF-1 Determines Skeletal Strength by Regulating Subperiosteal Expansion and Trait Interactions. J. Bone Miner. Res. 24 (8), 1481–1492. doi: 10.1359/jbmr.090226
Yakar, S., Courtland, H. W., Clemmons, D. (2010). IGF-1 and Bone: New Discoveries From Mouse Models. J. Bone Miner. Res. 25 (12), 2543–2552. doi: 10.1002/jbmr.234
Yakar, S., Rosen, C. J., Beamer, W. G., Ackert-Bicknell, C. L., Wu, Y., Liu, J. L., et al. (2002). Circulating Levels of IGF-1 Directly Regulate Bone Growth and Density. J. Clin. Invest. 110 (6), 771–781. doi: 10.1172/JCI0215463
Yan, J., Charles, J. F. (2018). Gut Microbiota and IGF-1. Calcif. Tissue Int. 102 (4), 406–414. doi: 10.1007/s00223-018-0395-3
Yan, J., Herzog, J. W., Tsang, K., Brennan, C. A., Bower, M. A., Garrett, W. S., et al. (2016). Gut Microbiota Induce IGF-1 and Promote Bone Formation and Growth. Proc. Natl. Acad. Sci. U. S. A. 113 (47), E7554–e7563. doi: 10.1073/pnas.1607235113
Yu, M., Malik Tyagi, A., Li, J. Y., Adams, J., Denning, T. L., Weitzmann, M. N., et al. (2020). PTH Induces Bone Loss via Microbial-Dependent Expansion of Intestinal TNF(+) T Cells and Th17 Cells. Nat. Commun. 11 (1), 468. doi: 10.1038/s41467-019-14148-4
Yu, M., Pal, S., Paterson, C. W., Li, J. Y., Tyagi, A. M., Adams, J., et al. (2021). Ovariectomy Induces Bone Loss via Microbial-Dependent Trafficking of Intestinal TNF+ T Cells and Th17 Cells. J. Clin. Invest. 131 (4), e143137. doi: 10.1172/JCI143137
Keywords: gut microbiota, periodontitis, gut-bone axis, gut epithelial barrier, osteoimmunology, alveolar bone loss
Citation: Jia X, Yang R, Li J, Zhao L, Zhou X and Xu X (2021) Gut-Bone Axis: A Non-Negligible Contributor to Periodontitis. Front. Cell. Infect. Microbiol. 11:752708. doi: 10.3389/fcimb.2021.752708
Received: 03 August 2021; Accepted: 26 October 2021;
Published: 16 November 2021.
Edited by:
Julien Santi-Rocca, Science and Healthcare for Oral Welfare, FranceReviewed by:
Robert P. Hirt, Newcastle University, United KingdomCorrie M. Whisner, Arizona State University, United States
Copyright © 2021 Jia, Yang, Li, Zhao, Zhou and Xu. This is an open-access article distributed under the terms of the Creative Commons Attribution License (CC BY). The use, distribution or reproduction in other forums is permitted, provided the original author(s) and the copyright owner(s) are credited and that the original publication in this journal is cited, in accordance with accepted academic practice. No use, distribution or reproduction is permitted which does not comply with these terms.
*Correspondence: Xin Xu, xin.xu@scu.edu.cn